- 1Cancer Research Division, Center for Global Health, School of Public Health, Nanjing Medical University, Nanjing, China
- 2Department of Clinical Nutrition, The Second Affiliated Hospital of Nanjing Medical University, Nanjing, China
- 3Department of Urology, Taikang Xianlin Drum Tower Hospital, Nanjing, China
- 4Nanjing Center for Disease Control and Prevention, Nanjing, China
- 5Medical Center for Digestive Diseases, The Second Affiliated Hospital of Nanjing Medical University, Nanjing, China
- 6Department of Geriatric Cardiology, Jiangsu Province Hospital, The First Affiliated Hospital of Nanjing Medical University, Nanjing, China
Phthalic acid esters (PAEs) are environmental organic pollutants that are ubiquitous in the ocean, and di-(2-ethylhexyl) phthalate (DEHP) is the most widely used PAE. The environmental concentration of DEHP was reported to be up to 42.52 μg/L in seawater in the estuaries located in Jiaozhou Bay along the Yellow Sea. DEHP has been investigated with respect to its toxicity in marine organisms. However, evidence on the dose-dependent effects of DEHP remains contradictory and limited. We used marine mussel Mytilus galloprovincialis as the experimental animal to study the dose-dependent effects of various levels of exposure to DEHP (concentrations of 4, 12, 36, 108, and 324 μg/L). These effects and the underlying mechanisms were elucidated by the levels of antioxidant enzyme activity, gene expression, and metabolite. The results indicated that, at environmentally relevant concentrations (12 and 36 μg/L), DEHP induced significant hormetic effects. This was indicated by the U-shaped or inverted U-shaped responses of the gene expression levels related to stress response (CAT, GST, and MgGLYZ) and antioxidant enzyme activities (SOD and CAT). The metabolic profiles revealed that DEHP generally caused monophasic response in osmotic regulation (homarine) and biphasic response (hormesis) in energy metabolism (glucose, glycogen, and amino acids), respectively. These findings can aid in ecological risk assessment with respect to DEHP and the determination of hormetic dose responses.
Introduction
Phthalate acid esters (PAEs) are diesters of 1,2-benzenedicarboxylic acid (or phthalic acid) and are usually used as additives to enhance the plasticity of industrial polymers (Gao et al., 2018). They can be used in numerous products, such as toys, medical devices, food packaging, capsule drugs (to extend or enhance the drug’s release characteristics), and personal care products (Hu H. et al., 2020). Because PAEs are not chemically bonded to polymers through a covalent linkage, they are readily released to the environment (Fromme et al., 2002). PAEs are ubiquitous environmental organic pollutants, and di-(2-ethylhexyl) phthalate (DEHP) is one of the most found PAEs in the marine environment (Biemann et al., 2012). A previous study found that the DEHP concentration reached 42.52 μg/L in the seawater of the estuaries located in Jiaozhou Bay along the Yellow Sea, posing severe risks to marine organisms (Gao et al., 2015).
Recent studies have verified that DEHP can induce multiple adverse effects in aquatic organisms (such as zebrafish Danio rerio, Chinese rare minnow Gobiocypris rarus, and yellow catfish Pelteobagrus fulvidraco), including growth inhibition, reproductive dysfunction, interference, and reduction of endocrine and immune function and teratogenicity (Sung et al., 2003; Uren-Webster et al., 2010; Lu et al., 2013; Guo et al., 2015; Yuan et al., 2017). In addition, DEHP is listed by the European Union as a substance linked with potential endocrine-disrupting activity and is therefore classed as an environmental endocrine disruptor (EED) (Lu et al., 2020). The environmental concentration of DEHP in seawater is usually at the μg/L scale (Gao et al., 2015). With regard to ecotoxicology, however, not only a monophasic but also biphasic dose–response relationship may be present between the doses and tested endpoints at extremely low pollutant concentrations (e.g., at the μg/L scale) (Chapman, 2002). The acute toxicological effects of many environmental organic pollutants on organisms are usually caused by high doses, while the low concentrations are often beneficial. This effect is called hormesis (Calabrese et al., 2016; Agathokleous et al., 2018), and its mechanism is related to the organism’s adaptive biological stress response. The use of toxicant hormesis in measurement has, in recent years, constituted a new method for evaluating the toxicity of compounds (Stebbing, 1998, 2000). Furthermore, recent studies have discovered the hormetic effects of diverse environmental pollutants, including minerals, heavy metals, organic compounds, and nanoparticles (Zhang et al., 2009; Liu et al., 2019; Agathokleous and Calabrese, 2020; Zhan et al., 2021a,b). Studies have verified the hormetic effects of cadmium at environmentally relevant concentrations in the polychaete Perinereis aibuhitensis by testing multiple endpoints, such as metabolite levels, antioxidant enzyme activities, and mRNA expression levels (Liu et al., 2019). Tetrabromobisphenol A (TBBPA) is a weak EED that can induce apparent hormetic effects in the marine clam Ruditapes philippinarum at an identified mRNA expression level (Hu F. et al., 2020). As a typical marine environmental pollutant, DEHP may induce hormetic responses that remain unclear in the field of marine ecotoxicology.
Mussel Mytilus galloprovincialis exhibits strong bioaccumulation of environmental pollutants (Ji et al., 2016; Wu et al., 2018; Meng et al., 2020). Because of its high capacity, pollutant tolerance, easy collection, and wide distribution, M. galloprovincialis serves as an excellent specimen for monitoring the marine environment (Ji et al., 2014; Yu et al., 2016; Meng et al., 2019). Therefore, this marine mussel has been frequently used as bioindicator for biomarker selection in marine ecotoxicology (Cappello et al., 2015; Maisano et al., 2017; Zhong et al., 2020). For example, Cappello et al. (2013) used M. galloprovincialis to investigate the impact of environmental pollution, suggesting that marine pollution (mainly mercury and polycyclic aromatic hydrocarbons) could induce osmotic stress and neurotoxicity in M. galloprovincialis indicated by the biomarkers, such as amino acids and acetylcholine. In our study, the mussel M. galloprovincialis was selected as the experimental animal to investigate dose-dependent responses to DEHP. A few endpoints, including antioxidant enzyme activities, gene expression levels related to stress responses, and metabolic profiles, were tested to illustrate the dose-dependent effects of DEHP in M. galloprovincialis.
Materials and Methods
Exposure Experiment
We collected 120 adult mussels M. galloprovincialis with a length of 5.5 ± 0.3 cm from a pristine environment along Yangma Island in Yantai, China, and transported them to our laboratory immediately. These mussels were acclimated in aerated seawater (20°C ± 2.0°C, 31.1 psu) for 7 days. Subsequently, we randomly sorted the mussels into six groups: five DEHP (99.5%, Dr. Ehrenstorfer, Augsburg, Germany) treatment groups and one blank control. Each group had two replicate glass tanks. Each glass tank contained 10 mussels in 10 L of aerated seawater. Five different concentrations (4, 12, 36, 108, and 324 μg/L) of DEHP were used to study the effect of dose-dependent exposure on the mussels. Of these five experimental concentrations, the three experimental concentrations of 4, 12, and 36 μg/L were environmentally relevant (Gao et al., 2015). We used dimethyl sulfoxide (DMSO) as a solvent to dissolve DEHP. Studies on the mussel M. galloprovincialis have reported no significant biological difference between the solvent control group and the seawater control group (Ji et al., 2013). Therefore, we only had one solvent control group, and the concentrations of DMSO (0.00025%, v/v) in the solvent control and DEHP-treated groups were identical. In this work, Chlorella vulgaris was used to feed the mussels (approximately 2% of the tissue per dry weight daily) during the acclimation and exposure periods. After 14 days of exposure, eight mussels were randomly selected from each treatment, and the entire soft tissue was dissected. Subsequently, we quickly froze each sample in liquid nitrogen. Finally, each sample was divided into three parts before conducting enzyme activity determination, RNA extraction, and metabolite extraction.
Activities of Antioxidant Enzymes
The activities of antioxidant enzymes in mussel tissues (n = 6) were assayed by using commercially available enzyme kits (Jiancheng, Nanjing, China). Furthermore, we measured the levels of three necessary antioxidant enzymes: catalase (CAT; EC 1.11.1.6), glutathione S-transferase (GST; EC 2.5.1.18), and superoxide dismutase (SOD; EC 1.15.1.1). A bicinchoninic acid assay was employed to determine the protein content in mussel tissues.
RNA Extraction and Quantitative Real-Time Polymerase Chain Reaction
Total RNA was extracted from the whole soft tissue samples (n = 6) according to the manufacturer’s instructions. First-strand cDNA was synthesized based on information in the M-MLV reverse transcription kit (Promega, Madison, WI, United States). For the analysis of gene expression levels, six stress responsive genes, including SOD, CAT, GST, nuclear factor-k-gene binding (NF-κB), goose-type lysozymes (MgGLYZ), and heat shock protein 70 (HSP70), were selected, and the beta-actin gene (β-actin) was used as the reference gene. All the primers of these genes are listed in Table 1.
The sample cDNA was mixed, and fourfold serial dilution was performed. The diluted cDNA was used as a template for the fluorescent real-time quantitative polymerase chain reaction (qRT-PCR), with three replicates for each template. The fluorescent qRT-PCR reaction system was operated according to the manufacturer’s instructions (Liu et al., 2019). The value during the amplification process was continuously measured in real time to establish a standard curve. The obtained data were analyzed using the ABI 7500 SDS software (Applied Biosystems). The 2–ΔΔCT method was used to analyze the gene expression levels (Livak and Schmittgen, 2001). The qRT-PCR process is detailed in the Supplementary Material.
Metabolite Extraction, 1HNMR, and Data Analysis
Metabolites were extracted using the modified extraction protocol described by Ji et al. (2020). Briefly, the metabolites in each M. galloprovincialis sample (100 mg wet weight) were extracted by 485 μL of methanol/water (4/0.85, v/v), 200 μL of chloroform, and 225 μL of water. The mixture was first vortexed thoroughly and left to stand on ice for 10 min, and it was then centrifuged at 3,000 r/min for 10 min. Finally, the upper polar phase was absorbed and vacuum-dried for testing. We prepared 600 μL of phosphate buffer solution (100 mmol/L, pH = 7.0) in heavy water before adding the solution to a numbered EP sample tube. The tube was vortexed thoroughly for its contents to dissolve before being centrifuged at 3,000 r/min for 10 min, and 550 μL of supernatant was pipetted into a nuclear magnetic resonance (NMR) tube (Φ = 5 mm) for further NMR analysis.
1H NMR spectral analysis was conducted using a Bruker AV 500 NMR spectrometer with a 5-mm reverse probe (Wu et al., 2013a,b). The corresponding proton resonance frequency was 500.18 MHz. The other parameters were as follows: spectrum width = 6009.6 Hz, delay time = 0.3 s, mixing time = 0.1 s, line broadening factor = 1, and number of scans = 128. Moreover, the water peak was suppressed by presaturation irradiation during the relaxation delay and mixing period when the 1H NMR spectrum was obtained (Wu et al., 2013a).
TopSpin 2.1 was used to perform phase correction and baseline adjustment on the spectrum, and trimethylsilyl propanoic acid was used as the internal standard of the chemical shift reference peak (0.0 ppm) (Viant et al., 2009). The processing and analysis of NMR data are detailed in the Supplementary Material. In addition, Chenomx (Evaluation Version, Chenomx, Edmonton, Alberta, Canada) was used to identify major metabolites, specifically by analyzing the chemical shifts of metabolites (Viant et al., 2009; Wu et al., 2013c; Li et al., 2015).
Statistical Analysis
Data analysis was performed using Minitab (Version 15, Minitab, United States). The Shapiro–Wilk test was used to verify the normality of the distribution for all variables. These variables were antioxidant enzyme activities and gene expression levels in the mussel samples, which were subject to Ryan–Joiner’s test and Bartlett’s test, respectively (Liu et al., 2019). The values are presented in terms of the mean ± standard deviation for continuous variables. One-way analysis of variance (ANOVA) with Tukey’s test was performed to determine the differences between the control and DEHP treatments. A P-value less than 0.05 was regarded as statistically significant.
Results and Discussion
Dose-Dependent Effects of DEHP on Antioxidant Enzyme Activities in Mussels
The dose-dependent effects of environmental pollutants depend on the testing endpoints (Agathokleous et al., 2018). First, the activities of three typical antioxidant enzymes, namely CAT, SOD, and GST, were measured. As summarized in Figure 1, both SOD and CAT activities in mussel samples demonstrated typical inverted U-shaped curves with increasing DEHP concentration, which indicated the presence of DEHP-induced hormetic effects in mussels. Specifically, the environmentally realistic concentration of 12 μg/L induced significant (P < 0.05) increases in CAT and SOD activities, whereas the higher concentrations of 108 and 324 μg/L significantly (P < 0.05) inhibited the activities of these two antioxidant enzymes.
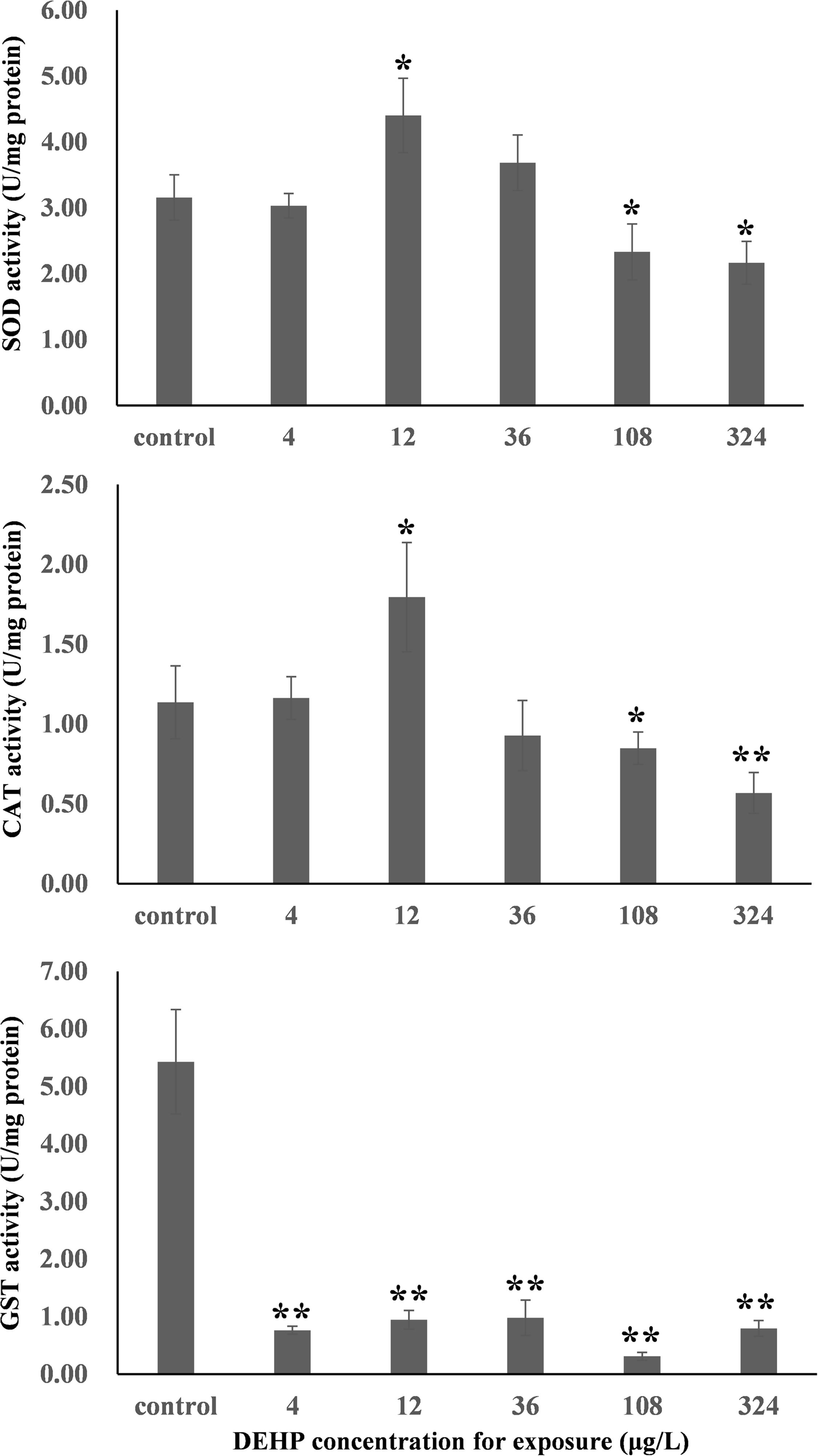
Figure 1. In the study of SOD, CAT, GST, NF-κB, MgGLYZ, and HSP70 in the samples from Mytilus galloprovincialis, the expression levels exhibited a significant difference relative to the negative controls. *P < 0.05. **P < 0.01. CAT, catalase; GST, glutathione S-transferase; SOD, superoxide dismutase; NF-κB, nuclear factor-k-gene binding; MgGLYZ, goose-type lysozymes in M. galloprovincialis; HSP70, heat shock protein 70.
As an antioxidant enzyme, SOD can catalyze the dismutation of superoxide radicals into oxygen (O2) or hydrogen peroxide (H2O2) in coordination with CAT, consequently preventing reactive oxygen radicals from damaging organisms. Therefore, these two enzymes play critical roles in protecting cells from the oxidative stress induced by reactive oxygen species. In this study, the increased activities of SOD and CAT revealed that the mussels enhanced the capacity of these two enzymes against the potential oxidative stress induced by DEHP. However, the inhibited activities of SOD and CAT indicated interference to antioxidant enzymes induced by high concentrations (108 and 324 μg/L) of DEHP, which was similarly observed in the DEHP-treated yellow catfish P. fulvidraco (Yuan et al., 2017). Notably, the hormetic effects of cadmium were also detected in both earthworm Eisenia fetida and polychaete P. aibuhitensis when CAT and SOD activities were observed (Zhang et al., 2009; Liu et al., 2019). As a known phase II metabolic isozyme, GST is the key antioxidant enzyme that catalyzes the conjugation of glutathione to several electrophilic substrates in organisms. Basically, it functions in the coupling of the electrophilic group of harmful substances with the sulfhydryl group of the reduced form of glutathione for the detoxification of toxicants (Chen et al., 2018). We noted no significant DEHP-induced hormetic or monophasic response in GST activity in the mussels. However, GST activity was significantly (P < 0.01) inhibited in all DEHP-treated groups, suggesting that DEHP might produce damage to proteins and subsequent enzymatic inactivation of GST and reduced the detoxification capacity of mussels.
Dose-Dependent Effects of DEHP at Gene Expression Levels in Mussels
Figure 1 shows the dose-dependent effects of DEHP at the mRNA level when we evaluated the levels of six stress-responsive genes (CAT, SOD, GST, NF-kB, MgGLYZ, and HSP70). In general, these genes were responsive to oxidative stress (SOD, CAT, and GST) and immune stress (NF-kB, MgGLYZ, and HSP70) (Cellura et al., 2006; Wang et al., 2012; Sang et al., 2020). After exposure to 36 μg/L of DEHP treatment for 14 days, the expression level of SOD was significantly (P < 0.05) downregulated. For both CAT and GST, their expression profiles exhibited U-shaped curves (Figure 1), which were intriguingly the inverse of the response profiles (inverted U-shaped curves) of CAT and GST activities (Figure 2). This constituted a major finding in understanding how mRNA expression is encoded protein’s tendency, which does not always happen due to post-translational or post-transcriptional modifications. Additionally, the enzyme activities contained the total activities of the entire enzyme family. Therefore, the disparity of the gene expressions of CAT and GST with the corresponding CAT and GST activities was not unexpected (Wu et al., 2013b).
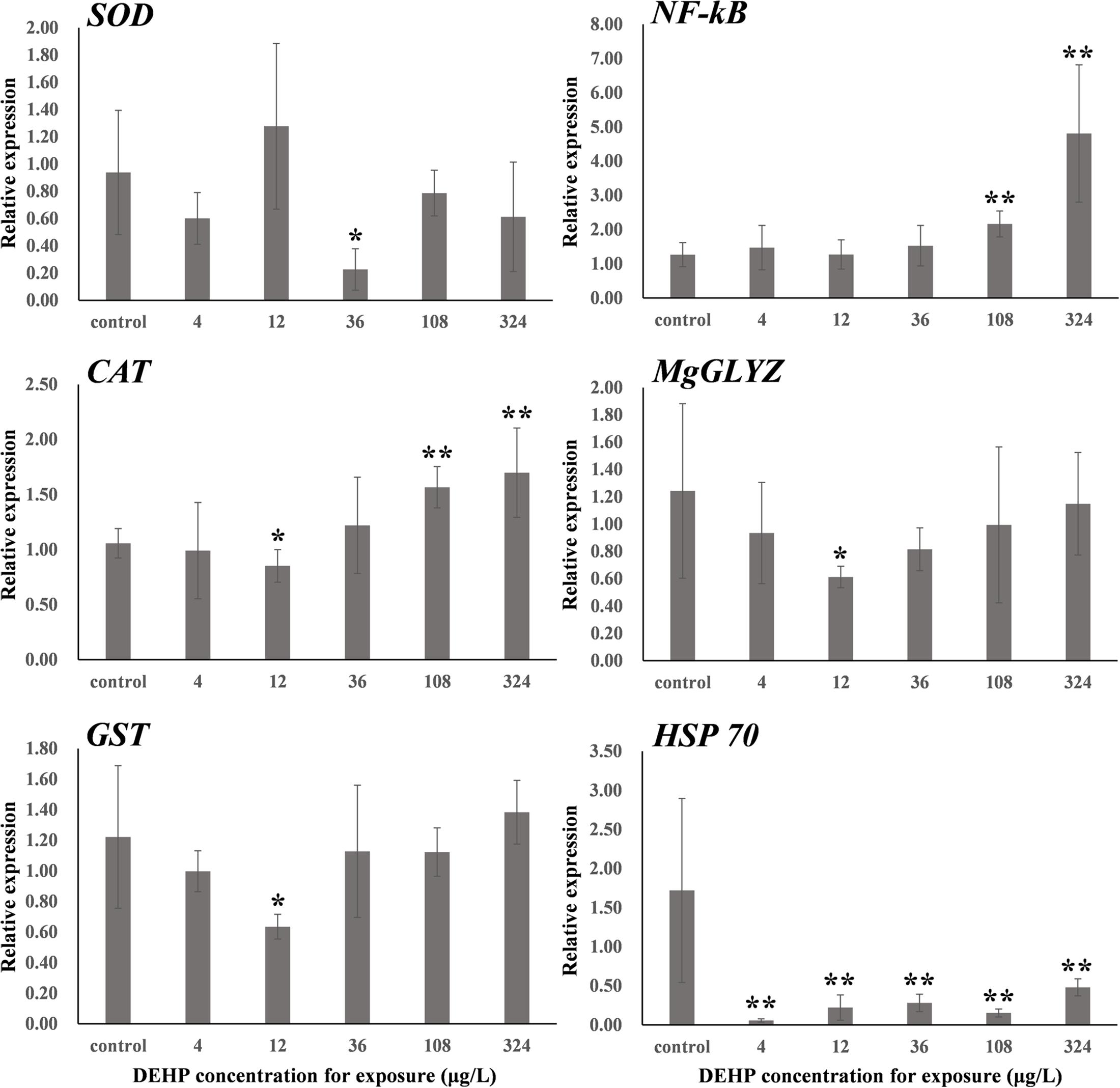
Figure 2. One-way ANOVA was used to analyze the differences of SOD, CAT, and GST activities (U/mg protein) between the DEHP exposed group and the control group. ∗P < 0.05. ∗∗P < 0.01. CAT, catalase; GST, glutathione S-transferase; SOD, superoxide dismutase.
NF-κB is an evolutionarily conserved family of regulatory molecule that is widely present in various tissues and plays a vital role in regulating the expression of diverse immune-related genes that respond to pathogens and the immune system (Hayden and Ghosh, 2008). Lysozymes are a non-specific immune factor that can hydrolyze the peptidoglycan of the bacterial cell wall, resulting in cell wall rupture and content escape. Furthermore, lysozyme scan inactivates pathogens by binding to the negatively charged viral protein, and thus, they play critical roles in the defense and elimination of pathogens (Wang et al., 2012). HSP70 is a molecular chaperone belonging to the HSP family and with standard features in the aggregation of unfolded or misfolded proteins and in the degradation of denatured protein (Wang et al., 2012). In this study, NF-κB expression levels were substantially and significantly (P < 0.01) upregulated in the groups treated with 108 and 324 μg/L of DEHP, suggesting that these two high doses of DEHP induced immune stress in mussels. Furthermore, the expression profile of MgGLYZ exhibited an approximately U-shaped curve with increasing dose, and we noted a significantly decreased expression level of MgGLYZ in the group treated with 12 μg/L of DEHP. This finding is consistent with our previous finding on CAT and GST expression profiles and on the presence of DEHP-induced hormesis in mussels at the mRNA level. In all DEHP treatments, HSP70 expression levels were significantly (P < 0.01) downregulated without linear or hormetic dose responses. Future studies should conduct transcriptomic analysis to globally profile the dose-dependent responses induced by DEHP at the mRNA level.
Dose-Dependent Effects of DEHP at Metabolite Level in Mussels
Metabolomic analysis can characterize the alteration of biological samples, allowing the researcher to identify perturbed metabolic pathways and obtain vital information pertaining to biochemical networks (Viant et al., 2009; Wu et al., 2012). Therefore, metabolomics has been extensively applied in marine ecotoxicology due to its capability to globally characterize the metabolic responses in organisms stressed by environmental pollutants, which can elucidate the toxicological effects and select biomarkers (Cappello et al., 2013, 2015; Ji et al., 2015; Yu et al., 2016; Liu et al., 2019). In our study, metabolomic analysis based on the NMR technique was employed to characterize the dose-dependent effects of DEHP in mussels at the metabolite level. As shown in Figure 3, we identified 34 metabolites in mussel tissue extracts, including energy storage compounds (glycogen, ATP, ADP, and glucose), amino acids (arginine, leucine, alanine, isoleucine, valine, glutamate, glycine, and tyrosine), organic osmolytes (betaine, hypotaurine, taurine, dimethylglycine, and homarine), and intermediates in the Krebs cycle (fumarate and succinate). Principal component analysis was conducted on the NMR data from the control group and all DEHP treatment groups. In general, the average scores along PC1 (46.38% variation) decreased linearly (Figure 4A), presenting a monophasic dose–response relationship along the PC1 axis. Although the lowest concentration (4 μg/L) of DEHP treatment did not significantly differ from the control, the three groups exposed to higher DEHP concentrations (36, 108, and 324 μg/L) significantly (P < 0.05) differed from the control group along the PC1 axis. However, the average scores along the PC4 (4.48% variation) axis had an inverted U-shaped curve with two moderate concentrations (12 and 36 μg/L) of DEHP treatments located at the top (Figure 4B), which was consistent with the dose–response curves for SOD and CAT activities. These findings confirmed that DEHP mainly induced a linear dose-dependent response at the metabolite level, accounting for 46.38% of the total variation induced by DEHP treatments. However, we also found significant hormetic responses, as indicated by the inverted U-shaped curve, verifying that at environmentally relevant concentrations, DEHP induced hormesis in mussels.
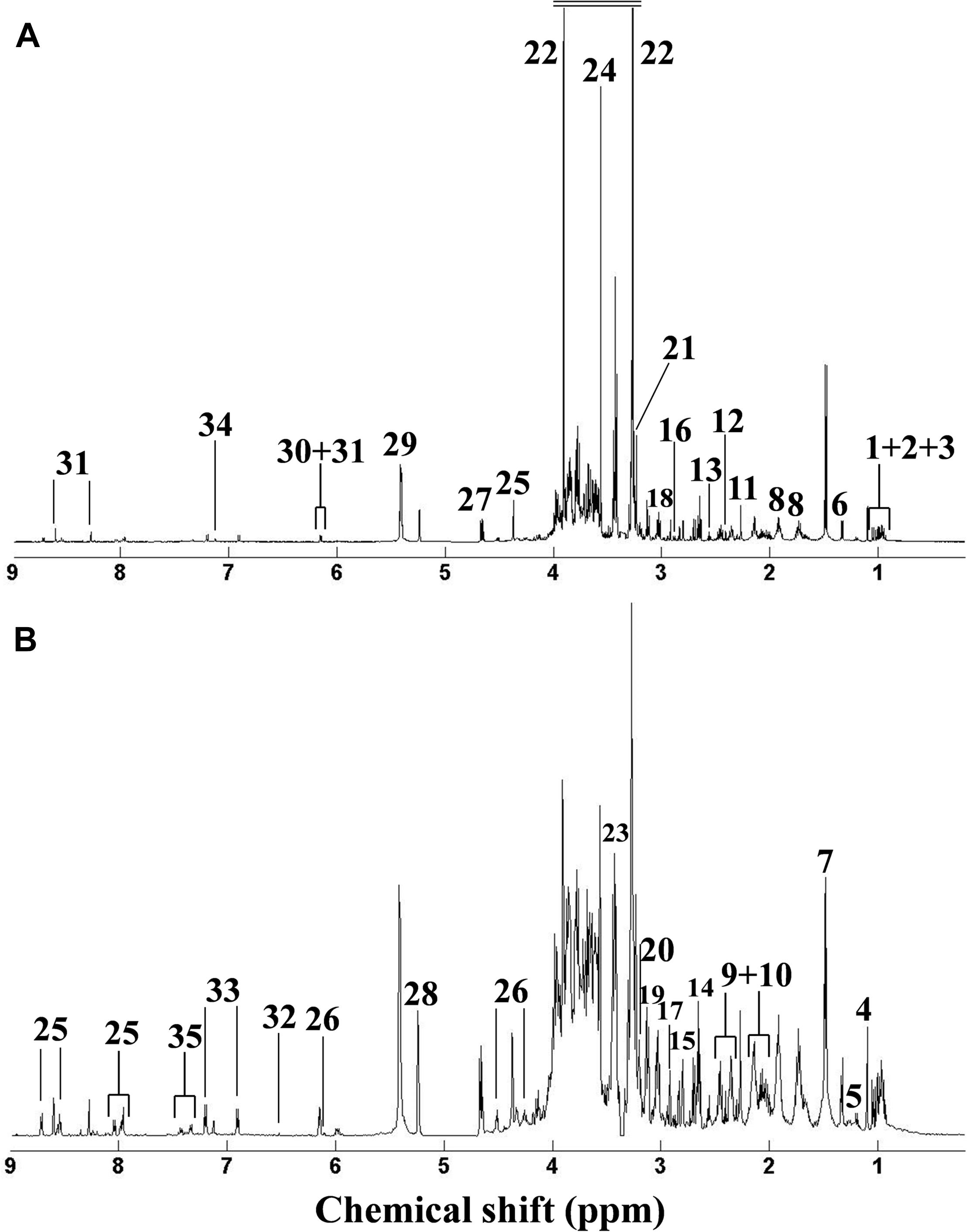
Figure 3. Typical 1H NMR spectra of serum extracts from the control group in the original. Typical 1HNMR spectrum of metabolite extracts of Mytilus galloprovincialis from the control group. Original (A) and generalized log-transformed (B) forms. (1) isoleucine, (2) leucine, (3) valine, (4) unknown 1 (1.10 ppm), (5) 3-aminoisobutyrate, (6) threonine, (7) alanine, (8) arginine, (9) glutamate, (10) glutamine, (11) acetoacetate, (12) succinate, (13) β-alanine, (14) hypotaurine, (15) aspartate, (16) asparagine, (17) dimethylglycine, (18) lysine, (19) malonate, (20) choline, (21) phosphocholine, (22) betaine, (23) taurine, (24) glycine, (25) homarine, (26) inosine, (27) β-glucose, (28) α-glucose, (29) glycogen, (30) ADP, (31) ATP, (32) fumarate, (33) tyrosine, (34) histidine, and (35) phenylalanine.
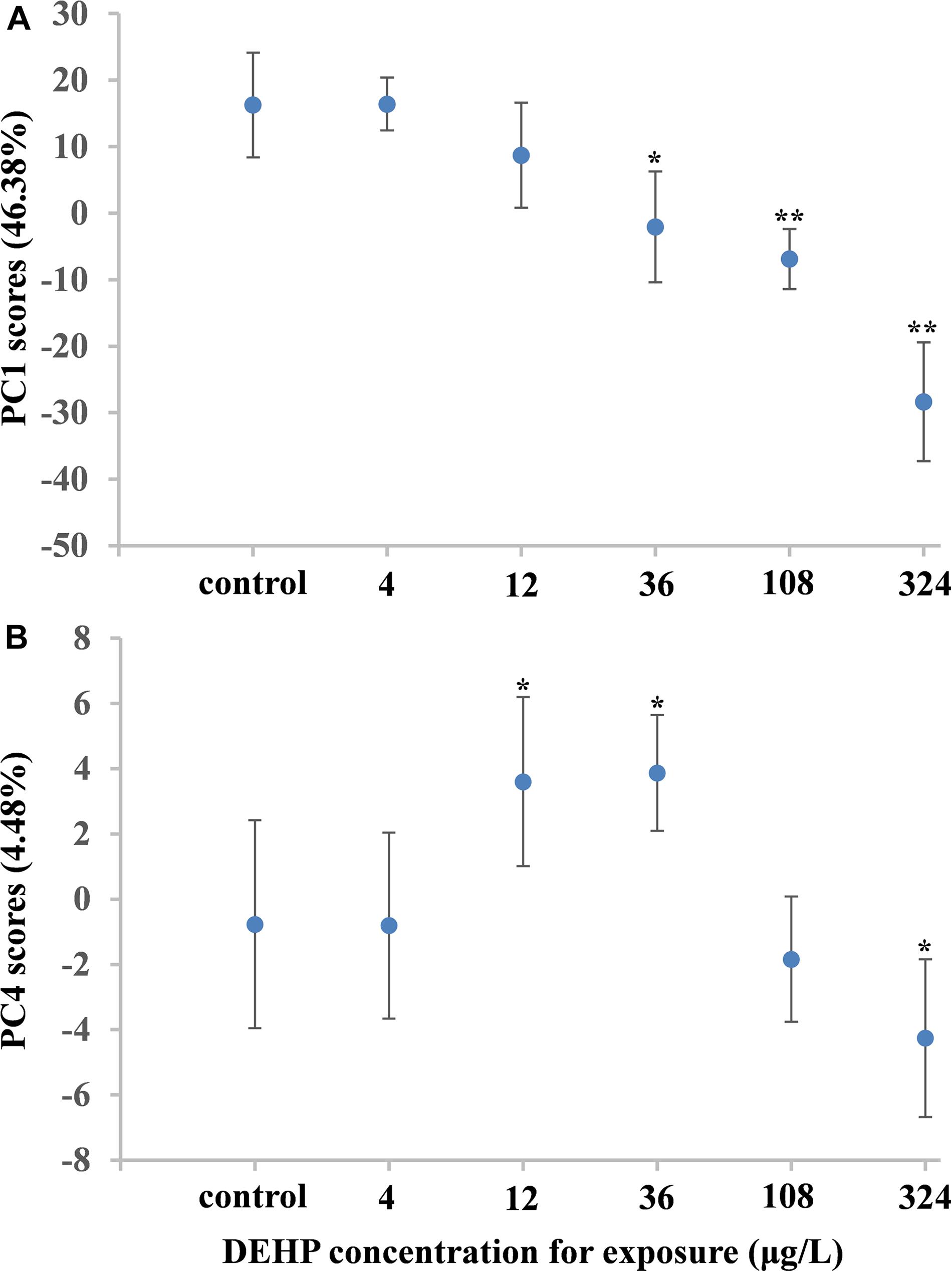
Figure 4. Characteristics of DEHP-treated groups and control using principal component analysis. Graphs show mean scores (with standard error bars). The average scores along PC1 (46.38% variation) decreased linearly, presenting a monophasic dose-response relationship along the PC1 axis (A). The average scores along the PC4 (4.48% variation) axis had an inverted U-shaped curve with two moderate concentrations (12 and 36 μg/L) of DEHP treatments located at the top (B). The one-way ANOVA indicated a significant difference (∗P < 0.05, or ∗∗P < 0.01) between DEHP-exposed groups and control groups.
As detailed in Figure 5, orthogonal projection to latent structures discriminant analysis (O-PLS-DA) was conducted. At the concentrations of 12 and 324 μg/L, DEHP exhibited a typical biphasic dose–response relationship in mussels. In mussel samples treated with 12 μg/L of DEHP, 12 metabolites, including 10 amino acids (such as threonine, asparagine, and lysine) and two energy storage metabolites (glycogen and glucose), were considerably (P < 0.05) altered. Of note, in the group treated with 12 μg/L of DEHP, these amino acids exhibited obviously reduced levels, whereas glucose and glycogen had markedly increased levels. In DEHP treatment at the highest concentration (324 μg/L), both arginine and lysine levels decreased, which was similar to what happened under the 12 μg/L DEHP treatment. However, glucose, glycogen, and tyrosine were, conversely, altered in the highest concentration of the DEHP-treated group. In addition, levels of glutamate, aspartate, homarine, and inosine were significantly elevated, whereas taurine was depleted in the group receiving the highest concentration (324 μg/L) of DEHP. Among these metabolites, the average concentration of homarine gradually increased with the increasing concentration of DEHP (from 4 to 324 μg/L), exhibiting a monophasic response. Other metabolites (especially amino acids, including isoleucine, threonine, alanine, and asparagine) recovered to normal levels in the control group, exhibiting DEHP-induced hormetic responses as well.
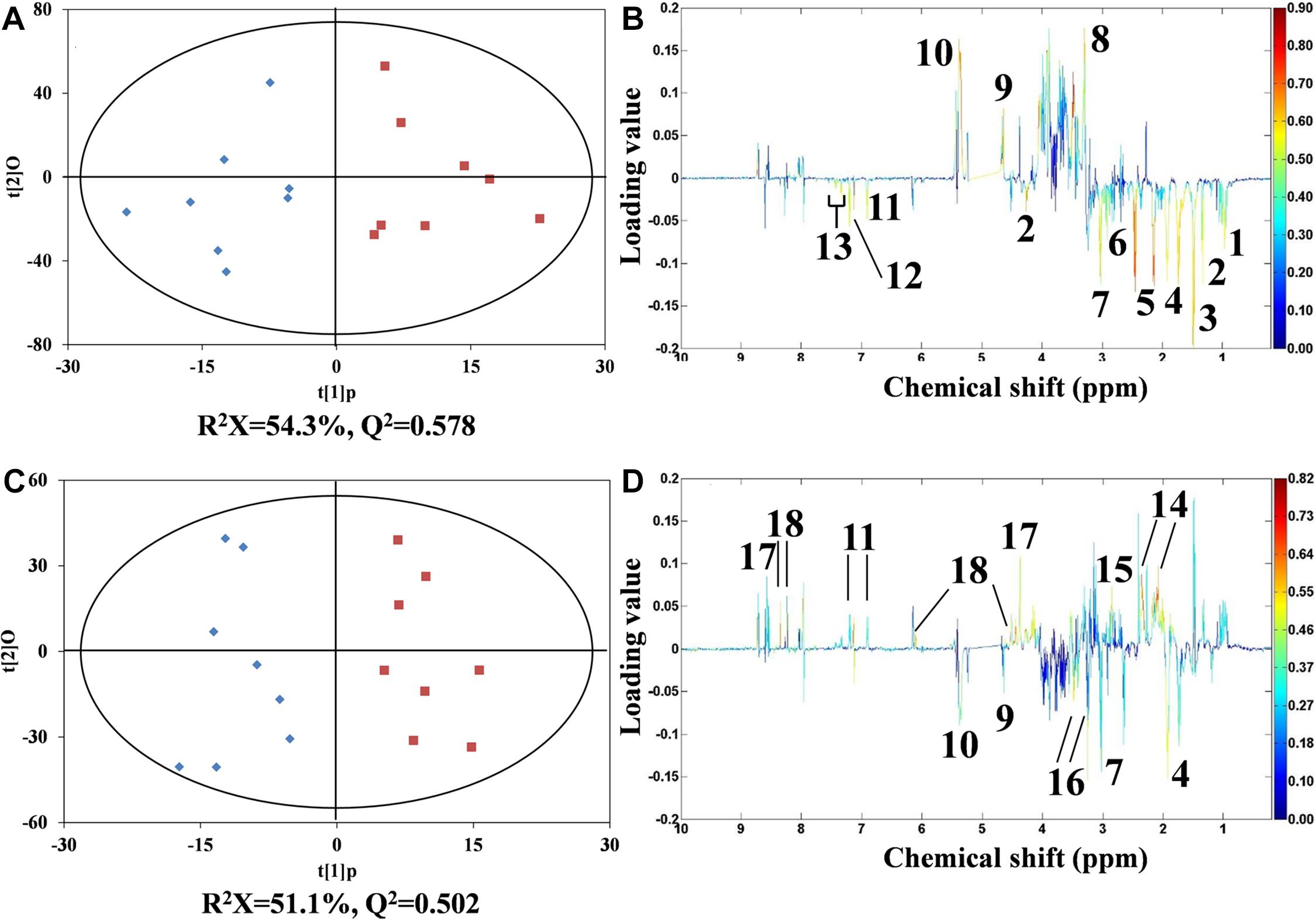
Figure 5. O-PLS-DA scores plots from the analysis of 1H NMR spectra using Mytilus galloprovincialis samples from the control (♠) and 12- and 324-μg/L DEHP-treated groups. Presenting hormesis (◼), control versus the 12-μg/L DEHP group (A), and control vs. the 324-μg/L DEHP group (B), with corresponding coefficient plots (C,D). The metabolite variations between the two classes (DEHP treatment vs. control) are indicated by the color map. Peaks in the positive direction indicate that metabolites are higher in the DEHP-treated groups. Accordingly, the negative peaks are labeled for the more marked metabolites in the control group. (1) isoleucine, (2) threonine, (3) alanine, (4) arginine, (5) glutamine, (6) asparagine, (7) lysine, (8) unknown 1 (3.30 ppm), (9) glucose, (10) glycogen, (11) tyrosine, (12) histidine, (13) phenylalanine, (14) glutamate, (15) aspartate, (16) taurine, (17) homarine, and (18) inosine.
In marine invertebrates, amino acids play essential roles in energy metabolism and osmotic regulation (Viant et al., 2003; Bonnefille et al., 2018; Dumas et al., 2020). In the 12 μg/L DEHP treatment, the levels of amino acids were approximately negatively correlated with the contents of glucose and glycogen. These results illustrated that DEHP exposure (12 μg/L) enhanced the consumption of amino acids and reduced the consumption of glucose for energy supply. This suggested that mussels elevated their energy storage to cope with the DEHP-induced stress, indicating an adaptive mechanism to DEHP exposure. However, in the group exposed to the highest concentration (324 μg/L) of DEHP, depleted glucose and glycogen, as well as the recovered amino acids, meant that the mussels consumed more energy to survive under this high concentration of DEHP. This hormetic response could be explained by the overcompensation of energy metabolism to enhance energy storage, ultimately leading to excessive energy consumption to maintain homeostasis (Calabrese, 2001). This overcompensation response has been considered to be the main mechanism accounting for the low-dose stimulation of hormesis (Kim et al., 2018; Agathokleous et al., 2019; Costantini and Borremans, 2019). Homarine and taurine are known as organic osmolytes in mussels (Ji et al., 2014). The increased homarine in DEHP treatments from 4 to 324 μg/L verified that DEHP induced monophasic osmotic stress in mussels, which means that DEHP could affect cell integrity in mussels. The decreased taurine level in the group exposed to the highest concentration (324 μg/L) of DEHP might be due to taurine being used to compensate for the increased homarine. Furthermore, the increased inosine level implied that at 324 μg/L, DEHP disturbed muscle movement in mussels.
Conclusion
This study demonstrated that DEHP can induce both monophasic and biphasic dose levels in mussel samples exposed to a serial concentration (4, 12, 36, 108, and 324 μg/L) of DEHP. Notably, the environmentally relevant concentrations (12 and 36 μg/L) of DEHP induced significant hormetic effects, as indicated by the endpoints, including antioxidant enzyme activities (SOD and CAT) and gene expression levels related to stress response (CAT, GST, and MgGLYZ), resulting in inverted U-shaped or U-shaped curves, respectively. At the metabolite level, DEHP caused a monophasic reaction in osmotic regulation (homarine) and biphasic response (hormesis) in energy metabolism (glucose, glycogen, and amino acids), respectively. These findings verified that hormetic dose response should be considered in marine ecotoxicology and in ecological risk assessments of DEHP.
Data Availability Statement
The raw data supporting the conclusions of this article will be made available by the authors, without undue reservation.
Author Contributions
HX: methodology, formal analysis, and writing first draft. WC: methodology, formal analysis, and writing review and editing. HS and PL: writing review and editing. SZ: project administration. SJ: supervision and writing—review and editing. CZ: supervision, writing review and editing, and project administration. All authors contributed to the article and approved the submitted version.
Funding
This work was supported by Key Project supported by Medical Science and Technology Development Foundation, Nanjing Department of Health (ZKX19049).
Conflict of Interest
The authors declare that the research was conducted in the absence of any commercial or financial relationships that could be construed as a potential conflict of interest.
Acknowledgments
We are grateful to all the participants of this study. We thank our colleagues for their work. We also thank Prof. Mark Viant for the use of ProMetab software.
Supplementary Material
The Supplementary Material for this article can be found online at: https://www.frontiersin.org/articles/10.3389/fmars.2021.658361/full#supplementary-material
References
Agathokleous, E., and Calabrese, E. J. (2020). A global environmental health perspective and optimisation of stress. Sci. Total Environ. 704:135263. doi: 10.1016/j.scitotenv.2019.135263
Agathokleous, E., Feng, Z., Iavicoli, I., and Calabrese, E. J. (2019). The two faces of nanomaterials: a quantification of hormesis in algae and plants. Environ. Int. 131:105044. doi: 10.1016/j.envint.2019.105044
Agathokleous, E., Kitao, M., and Calabrese, E. J. (2018). Environmental hormesis and its fundamental biological basis: rewriting the history of toxicology. Environ. Res. 165, 274–278. doi: 10.1016/j.envres.2018.04.034
Biemann, R., Navarrete Santos, A., Navarrete Santos, A., Riemann, D., Knelangen, J., Blüher, M., et al. (2012). Endocrine disrupting chemicals affect the adipogenic differentiation of mesenchymal stem cells in distinct ontogenetic windows. Biochem. Biophys. Res. Commun. 417, 747–752. doi: 10.1016/j.bbrc.2011.12.028
Bonnefille, B., Gomez, E., Alali, M., Rosain, D., Fenet, H., and Courant, F. (2018). Metabolomics assessment of the effects of diclofenac exposure on Mytilus galloprovincialis: potential effects on osmoregulation and reproduction. Sci. Total Environ. 61, 611–618. doi: 10.1016/j.scitotenv.2017.09.146
Calabrese, E. J. (2001). Overcompensation stimulation: a mechanism for hormetic effects. Crit. Rev. Toxicol. 31, 425–470. doi: 10.1080/20014091111749
Calabrese, E. J., Dhawan, G., Kapoor, R., Iavicoli, I., and Calabrese, V. (2016). HORMESIS: a fundamental concept with widespread biological and biomedical applications. Gerontology 62, 530–535. doi: 10.1159/000441520
Cappello, T., Maisano, M., Giannetto, A., Parrino, V., Mauceri, A., and Fasulo, S. (2015). Neurotoxicological effects on marine mussel Mytilus galloprovincialis caged at petrochemical contaminated areas (eastern Sicily, Italy): 1H NMR and immunohistochemical assays. Comp. Biochem. Physiol. Part C 169, 7–15. doi: 10.1016/j.cbpc.2014.12.006
Cappello, T., Mauceri, A., Corsaro, C., Maisano, M., Parrino, V., Lo Paro, G., et al. (2013). Impact of environmental pollution on caged mussels Mytilus galloprovincialis using NMR-based metabolomics. Mar. Pollut. Bull. 77, 132–139. doi: 10.1016/j.marpolbul.2013.10.019
Cellura, C., Toubiana, M., Parrinello, N., and Roch, P. (2006). HSP70 gene expression in Mytilus galloprovincialis hemocytes is triggered by moderate heat shock and Vibrio anguillarum, but not by V. splendidus or Micrococcus lysodeikticus. Dev. Comp. Immunol. 30, 984–997. doi: 10.1016/j.dci.2005.12.009
Chapman, P. M. (2002). Ecological risk assessment (ERA) and hormesis. Sci. Total Environ. 288, 131–140. doi: 10.1016/s0048-9697(01)01120-2
Chen, L., Wu, H., Zhao, J., Zhang, W., Zhang, L., Sun, S., et al. (2018). The role of GST omega in metabolism and detoxification of arsenic in clam Ruditapes philippinarum. Aquat. Toxicol. 204, 9–18. doi: 10.1016/j.aquatox.2018.08.016
Costantini, D., and Borremans, B. (2019). The linear no-threshold model is less realistic than threshold or hormesis-based models: an evolutionary perspective. Chem. Biol. Interact. 301, 26–33. doi: 10.1016/j.cbi.2018.10.007
Dumas, T., Bonnefille, B., Gomez, E., Boccard, J., Castro, N. A., Fenet, H., et al. (2020). Metabolomics approach reveals disruption of metabolic pathways in the marine bivalve Mytilus galloprovincialis exposed to a WWTP effluent extract. Sci. Total Environ. 712:136551. doi: 10.1016/j.scitotenv.2020.136551
Fromme, H., Küchler, T., Otto, T., Pilz, K., Müller, J., and Wenzel, A. (2002). Occurrence of phthalates and bisphenol A and F in the environment. Water Res. 36, 1429–1438. doi: 10.1016/s0043-1354(01)00367-0
Gao, C. C., Li, F. M., Lu, L., and Sun, Y. (2015). Application of stir bar sorptive extraction and gas chromatograph mass spectrometer to the phthalic acid esters analysis in seawater. Huan Jing Ke Xue 36, 3906–3912.
Gao, D., Li, Z., Wang, H., and Liang, H. (2018). An overview of phthalate acid ester pollution in China over the last decade: environmental occurrence and human exposure. Sci. Total Environ. 645, 1400–1409. doi: 10.1016/j.scitotenv.2018.07.093
Guo, Y., Yang, Y., Gao, Y., Wang, X., and Zhou, B. (2015). The impact of long term exposure to phthalic acid esters on reproduction in Chinese rare minnow (Gobiocypris rarus). Environ. Pollut. 203, 130–136. doi: 10.1016/j.envpol.2015.04.005
Hayden, M. S., and Ghosh, S. (2008). Shared principles in NF-kappaB signaling. Cell 132, 344–362. doi: 10.1016/j.cell.2008.01.020
Hu, F., Yuan, Y., Yang, R., Zhang, W., and Chen, X. (2020). Effect of air pre-exposure on Tetrabromobisphenol A resistance in the clam Ruditapes philippinarum. Environ. Toxicol. Pharmacol. 76:103357. doi: 10.1016/j.etap.2020.103357
Hu, H., Mao, L., Fang, S., Xie, J., Zhao, M., and Jin, H. (2020). Occurrence of phthalic acid esters in marine organisms from Hangzhou Bay, China: implications for human exposure. Sci. Total Environ. 721:137605. doi: 10.1016/j.scitotenv.2020.137605
Ji, C., Li, F., Wang, Q., Zhao, J., Sun, Z., and Wu, H. (2016). An integrated proteomic and metabolomic study on the gender-specific responses of mussels Mytilus galloprovincialis to tetrabromobisphenol A (TBBPA). Chemosphere 144, 527–539. doi: 10.1016/j.chemosphere.2015.08.052
Ji, C., Lu, Z., Xu, L., Li, F., Cong, M., Shan, X., et al. (2020). Global responses to tris(1-chloro-2-propyl)phosphate (TCPP) in rockfish Sebastes schlegeli using integrated proteomic and metabolomic approach. Sci. Total Environ. 724:138307. doi: 10.1016/j.scitotenv.2020.138307
Ji, C., Wang, Q., Wu, H., Tan, Q., and Wang, W. X. (2015). A metabolomic investigation of the effects of metal pollution in oysters Crassostrea hongkongensis. Mar. Pollut. Bull. 90, 317–322. doi: 10.1016/j.marpolbul.2014.11.006
Ji, C., Wu, H., Wei, L., and Zhao, J. (2014). iTRAQ-based quantitative proteomic analyses on the gender-specific responses in mussel Mytilus galloprovincialis to tetrabromobisphenol A. Aquat. Toxicol. 157, 30–40. doi: 10.1016/j.aquatox.2014.09.008
Ji, C., Wu, H., Wei, L., Zhao, J., and Yu, J. (2013). Proteomic and metabolomic analysis reveal gender-specific responses of mussel Mytilus galloprovincialis to 2,2’,4,4’-tetrabromodiphenyl ether (BDE 47). Aquat. Toxicol. 14, 449–457. doi: 10.1016/j.aquatox.2013.07.009
Kim, S. A., Lee, Y. M., Choi, J. Y., Jacobs, D. R. Jr., and Lee, D. H. (2018). Evolutionarily adapted hormesis-inducing stressors can be a practical solution to mitigate harmful effects of chronic exposure to low dose chemical mixtures. Environ. Pollut. 233, 725–734. doi: 10.1016/j.envpol.2017.10.124
Li, L., Wu, H., Ji, C., van Gestel, C. A., Allen, H. E., and Peijnenburg, W. J. (2015). A metabolomic study on the responses of daphnia magna exposed to silver nitrate and coated silver nanoparticles. Ecotoxicol. Environ. Saf. 119, 66–73. doi: 10.1016/j.ecoenv.2015.05.005
Liu, F., Lu, Z., Wu, H., and Ji, C. (2019). Dose-dependent effects induced by cadmium in polychaete Perinereis aibuhitensis. Ecotoxicol. Environ. Saf. 169, 714–721. doi: 10.1016/j.ecoenv.2018.11.098
Livak, K. J., and Schmittgen, T. D. (2001). Analysis of relative gene expression data using real-time quantitative PCR and the 2(-Delta Delta C(T)) method. Methods 25, 402–408. doi: 10.1006/meth.2001.1262
Lu, Y., Zhang, P., Li, C., Su, X., Jin, C., Li, Y., et al. (2013). Characterisation of immune-related gene expression in clam (Venerupis philippinarum) under exposure to di(2-ethylhexyl) phthalate. Fish Shellfish Immunol. 34, 142–146. doi: 10.1016/j.fsi.2012.10.015
Lu, Y. S., Yao, G. X., Wang, X. L., Liu, J. X., Yu, J., Qiu, J., et al. (2020). A comprehensive analysis of metabolomics and transcriptomics reveals new biomarkers and mechanistic insights on DEHP exposures in MCF-7 cells. Chemosphere 255:126865. doi: 10.1016/j.chemosphere.2020.126865
Maisano, M., Cappello, T., Natalotto, A., Vitale, V., Parrino, V., Giannetto, A., et al. (2017). Effects of petrochemical contamination on caged marine mussels using a multi-biomarker approach: histological changes, neurotoxicity and hypoxic stress. Mar. Environ. Res. 128, 114–123. doi: 10.1016/j.marenvres.2016.03.008
Meng, X., Li, F., Wang, X., Liu, J., Ji, C., and Wu, H. (2019). Combinatorial immune and stress response, cytoskeleton and signal transduction effects of graphene and triphenyl phosphate (TPP) in mussel Mytilus galloprovincialis. J. Hazard. Mater. 378:120778. doi: 10.1016/j.jhazmat.2019.120778
Meng, X., Li, F., Wang, X., Liu, J., Ji, C., and Wu, H. (2020). Toxicological effects of graphene on mussel Mytilus galloprovincialis hemocytes after individual and combined exposure with triphenyl phosphate. Mar. Pollut. Bull. 151:110838. doi: 10.1016/j.marpolbul.2019.110838
Sang, X., Dong, J., Chen, F., Wei, L., and Wang, X. (2020). Molecular cloning and immune function study of an oyster IκB gene in the NF-κB signaling pathway. Aquaculture 525:735322.
Stebbing, A. R. (1998). A theory for growth hormesis. Mutat. Res. 403, 249–258. doi: 10.1016/s0027-5107(98)00014-1
Stebbing, A. R. D. (2000). Maia hypothesis — growth control and toxicology. Hum. Ecol. Risk Assess. 6, 301–311.
Sung, H. H., Kao, W. Y., and Su, Y. J. (2003). Effects and toxicity of phthalate esters to hemocytes of giant freshwater prawn, Macrobrachium rosenbergii. Aquat. Toxicol. 64, 25–37. doi: 10.1016/s0166-445x(03)00011-0
Uren-Webster, T. M., Lewis, C., Filby, A. L., Paull, G. C., and Santos, E. M. (2010). Mechanisms of toxicity of di(2-ethylhexyl) phthalate on the reproductive health of male zebrafish. Aquat. Toxicol. 99, 360–369. doi: 10.1016/j.aquatox.2010.05.015
Viant, M. R., Bearden, D. W., Bundy, J. G., Burton, I. W., Collette, T. W., Ekman, D. R., et al. (2009). International NMR-based environmental metabolomics intercomparison exercise. Environ. Sci. Technol. 43, 219–225. doi: 10.1021/es802198z
Viant, M. R., Rosenblum, E. S., and Tieerdema, R. S. (2003). NMR-based metabolomics: a powerful approach for characterizing the effects of environmental stressors on organism health. Environ. Sci. Technol. 37, 4982–4989. doi: 10.1021/es034281x
Wang, Q., Zhang, L., Zhao, J., You, L., and Wu, H. (2012). Two goose-type lysozymes in Mytilus galloprovincialis: possible function diversification and adaptive evolution. PLoS One 7:e45148. doi: 10.1371/journal.pone.0045148
Wu, H., Ji, C., Wei, L., Zhao, J., and Lu, H. (2013a). Proteomic and metabolomic responses in hepatopancreas of Mytilus galloprovincialis challenged by Micrococcus luteus and Vibrio anguillarum. J. Proteomics 94, 54–67. doi: 10.1016/j.jprot.2013.09.001
Wu, H., Liu, X., Zhang, X., Ji, C., Zhao, J., and Yu, J. (2013b). Proteomic and metabolomic responses of clam Ruditapes philippinarum to arsenic exposure under different salinities. Aquat. Toxicol. 13, 91–100. doi: 10.1016/j.aquatox.2013.03.020
Wu, H., Liu, X., Zhao, J., and Yu, J. (2012). Toxicological responses in halophyte Suaeda salsa to mercury under environmentally relevant salinity. Ecotoxicol. Environ. Saf. 85, 64–71. doi: 10.1016/j.ecoenv.2012.03.016
Wu, H., Zhang, X., Wang, Q., Li, L., Ji, C., Liu, X., et al. (2013c). A metabolomic investigation on arsenic-induced toxicological effects in the clam Ruditapes philippinarum under different salinities. Ecotoxicol. Environ. Saf. 90, 1–6. doi: 10.1016/j.ecoenv.2012.02.022
Wu, H., Zhong, M., Lu, Z., Shan, X., Li, F., Ji, C., et al. (2018). Biological effects of tris (1-chloro-2-propyl) phosphate (TCPP) on immunity in mussel Mytilus galloprovincialis. Environ. Toxicol. Pharmacol. 61, 102–106. doi: 10.1016/j.etap.2018.05.022
Yu, D., Ji, C., Zhao, J., and Wu, H. (2016). Proteomic and metabolomic analysis on the toxicological effects of As (III) and As (V) in juvenile mussel Mytilus galloprovincialis. Chemosphere 150, 194–201. doi: 10.1016/j.chemosphere.2016.01.113
Yuan, L., Li, M., Meng, F., Gong, Y., Qian, Y., Shi, G., et al. (2017). Growth, blood health, antioxidant status, immune response and resistance to Aeromonas hydrophila of juvenile yellow catfish exposed to di-2-ethylhexyl phthalate (DEHP). Comp. Biochem. Physiol. C Toxicol. Pharmacol. 202, 79–84. doi: 10.1016/j.cbpc.2017.08.004
Zhan, J., Wang, S., Li, F., Ji, C., and Wu, H. (2021a). Dose-dependent responses of metabolism and tissue injuries in clam Ruditapes philippinarum after subchronic exposure to cadmium. Sci. Total Environ. 779:146479. doi: 10.1016/j.scitotenv.2021.146479
Zhan, J., Wang, S., Li, F., Ji, C., and Wu, H. (2021b). Global characterization of dose-dependent effects of cadmium in clam Ruditapes philippinarum. Environ. Pollut. 273:116443. doi: 10.1016/j.envpol.2021.116443
Zhang, Y., Shen, G., Yu, Y., and Zhu, H. (2009). The hormetic effect of cadmium on the activity of antioxidant enzymes in the earthworm Eisenia fetida. Environ. Pollut. 157, 3064–3068. doi: 10.1016/j.envpol.2009.05.039
Keywords: phthalate acid esters, di-(2-ethylhexyl) phthalate, dose-dependent response, hormesis, environmental endocrine disruptor
Citation: Xu H, Cao W, Sun H, Zhang S, Li P, Jiang S and Zhong C (2021) Dose-Dependent Effects of Di-(2-Ethylhexyl) Phthalate (DEHP) in Mussel Mytilus galloprovincialis. Front. Mar. Sci. 8:658361. doi: 10.3389/fmars.2021.658361
Received: 25 January 2021; Accepted: 06 April 2021;
Published: 20 May 2021.
Edited by:
Zhi-Hua Li, Shandong University, ChinaReviewed by:
Laura Canesi, University of Genoa, ItalyCarlos Gravato, University of Lisbon, Portugal
Copyright © 2021 Xu, Cao, Sun, Zhang, Li, Jiang and Zhong. This is an open-access article distributed under the terms of the Creative Commons Attribution License (CC BY). The use, distribution or reproduction in other forums is permitted, provided the original author(s) and the copyright owner(s) are credited and that the original publication in this journal is cited, in accordance with accepted academic practice. No use, distribution or reproduction is permitted which does not comply with these terms.
*Correspondence: Caiyun Zhong, Y3l6aG9uZ0Buam11LmVkdS5jbg==
†These authors have contributed equally to this work