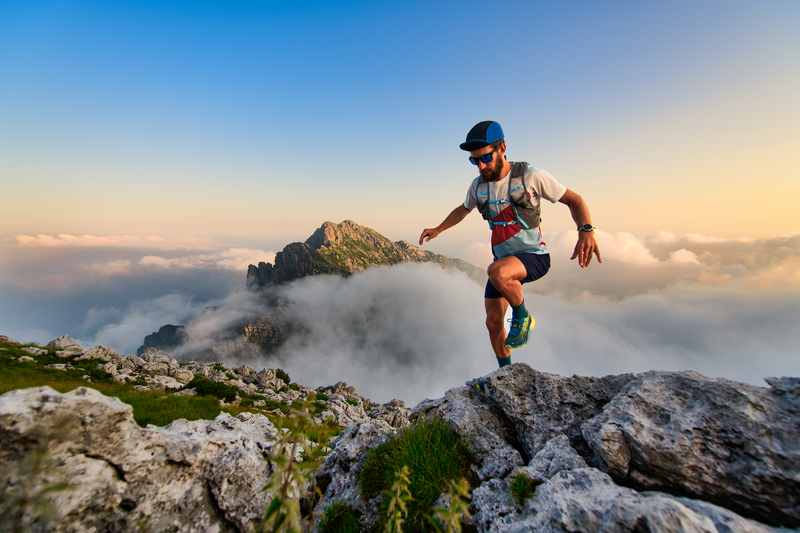
95% of researchers rate our articles as excellent or good
Learn more about the work of our research integrity team to safeguard the quality of each article we publish.
Find out more
ORIGINAL RESEARCH article
Front. Mar. Sci. , 09 July 2021
Sec. Aquatic Physiology
Volume 8 - 2021 | https://doi.org/10.3389/fmars.2021.657762
This article is part of the Research Topic Molecular Physiology in Molluscs, Volume II View all 26 articles
According to the RGB law display, the polymorphism of the giant clam mantle color pattern is through four iridocytes. The boring giant clam (Tridacna crocea) exhibits diverse mantle colors, including blue, green, purple, gold, and orange. In order to evaluate the genetic laws driving these mantle color patterns, a complete diallel cross between two color strains [blue strain (only blue iridocyte) and the yellow-green strain (yellow and green iridocytes)] was performed. Using a single-to-single mating system, two intra-strain crosses (BB and YY) and two reciprocal inter-strain crosses (BY and YB) were produced in triplicates. Higher fertilization rate and hatching rate were observed in all experimental groups, suggesting that there was no sperm–egg recognition barrier between the two strains. In the grow-out stage, the size of the reciprocal hybrids was larger than that of the two pure strains with a degree of heterosis. In addition, compared with the two pure strains, the hybrids have higher larval metamorphosis rate and higher survival rate. At 1 year of age, the mantle color pattern of pure strains showed 100% stable inheritance, while the reciprocal hybrids exhibited colorful patterns (a combination of blue, yellow, and green), suggesting that there was a genetic recombination of the mantle colors during the stable expression period. These results provide a theoretical basis for the formation of the mantle color of giant clam and its genetic segregation law, as well as provide guidance for genetic breeding of giant clams.
Throughout nature, biophotonic structures have evolved sophisticated arrangements of pigments and structural reflectors that can manipulate light in animal skin, cuticle, feathers, and fur (Mäthger et al., 2013). These colors are found in a variety of animal taxonomy, from diminutive marine copepods to terrestrial insects and birds, and have attracted great research interest in recent years. Recent studies on biophotonic structures focuses on the characterization of nanostructures responsible for iridescence and the behavioral function of iridescent colors (Kinoshita and Yoshioka, 2005; Cuthill et al., 2017).
Recently, scientists have discovered that the mantle color of giant clams is the result of structural color, which is aroused by a display of different iridocytes according to the RGB law display (Ozog, 2009; Todd et al., 2009; Holt et al., 2014; Rossbach et al., 2020; Long et al., 2021). During the photosymbiotic process of giant clams, the forward scattering of iridocytes illuminates the internal zooxanthellae deeper inside the mantle, and the backward reflection produces the unique color of the giant clam mantle (Ghoshal et al., 2016). Although the giant clam iridocytes distributed in the mantle have been well studied, little is known about the genetic basis or pattern of mantle color inheritance (Kim et al., 2017; Mies, 2019). Scientists have observed that giant clam strains with blue and yellow-green color patterns can be stably inherited, and there are phenotypic differences between the two color strains (Zhou et al., 2020). However, it is unclear whether the mantle color pattern is segregated or genetically recombined with the pigmentation of the shells during natural replenishment process.
The iridophores in the mantle of the giant clams are composed of iridocytes, which contain stacks of regularly arranged platelets of uniform thickness (Griffiths et al., 1992). These platelets form a crystal lattice, which produces maximum light interference at wavelengths of about 400 nm or slightly higher than 400 nm. Interference may extend the reflected light into the blue and green parts of the visible spectrum (Griffiths et al., 1992; Neo et al., 2015; Rossbach et al., 2020). Iridocytes can be divided into four types (red, yellow, green, and blue) and can be expressed as one, two, or more types in a single individual (Ghoshal et al., 2016). Blue, yellow, and green iridocytes are common, while red iridocytes are rare (Zhou et al., 2020). In our study, we examined the blue strain (blue iridocytes) and kelly strain (green and yellow iridocytes) of the boring giant clams.
In order to determine the genetic law of the reciprocal hybrids between two mantle color patterns of boring giant clams, complete diallel crosses were carried out in triplicate using a single-to-single mating system. The fertilization, survival, metamorphosis, and growth of progenies of each group during the larval, nursery, and grow-out phases were compared. In this study, the use of inter-strain hybridization technique clearly revealed the occurrence and genetic laws of the mantle coloration of reciprocal hybrids. The findings of this study can provide guidance for the genetic breeding of giant clams.
Sexually matured boring giant clams used as broodstock in this study were collected from Huangyan Island (East of Zhongsha Islands) (N 15.160812, E 117.760336) in the South China Sea. The clams were held in an insulated container filled with seawater and transported by boat to the Hainan Tropical Marine Life Experimental Station at the Chinese Academy of Sciences. Thirty parent clams from each of two mantle color strains (blue and yellow-green) were selected from the Huangyan Island population. The average shell length (SL) and total weight of the blue strain were 80.30 ± 7.96 mm and 165.32 ± 45.69 g, respectively, whereas the average SL and total weight of yellow-green clams were 89.53 ± 7.48 mm and 230.44 ± 72.56 g, respectively. The broodstock of these two strains was held separately in 2,000-L raceways and provided with gentle aeration and continuous flow of sand-filtered seawater from Sanya Bay, Hainan Island.
When these broodstocks from the two strains mature, they were induced to spawn by exposure to air for 10 min, followed by a temperature shock from 27.0 to 30.0°C. Once spawning was initiated, the individuals were placed separately in 5-L plastic beakers filled with seawater (30.0°C) and closely observed to collect uncontaminated eggs or sperm. Since Tridacninae is a simultaneous hermaphrodite animal (Braley et al., 2018), it is necessary to carefully collect sperm and eggs separately from each clam separately. If clams simultaneously release eggs and sperm, the eggs and sperm will be removed from the experiment. The collected sperm was filtered through a 25-μm mesh to ensure that there are no eggs. The collected eggs were set aside for at least 30 min before being inspected using a microscope. The eggs were discarded if fertilized. In total, the eggs or sperm of 18 individuals from each population were used in this study.
Four progenies, i.e., BB (B♀ × B♂), BY (B♀ × Y♂), YB (Y♀ × B♂), and YY (Y♀ × Y♂), were produced by factorial hybridization between the blue (B) and yellow-green strain (Y) clams. The fertilized eggs of each progeny were divided equally and placed into two 5-L beakers. A small number of fertilized eggs were sampled to evaluate the success rate of fertilization and the survival of D-stage larvae. The remaining fertilized eggs were suspended in a 100-L tank with a density of 30–40 eggs/ml for incubation. The water temperature and salinity were maintained at 28.6–29.7°C and 32 ppt, respectively. The entire experiment was repeated three times using three groups of broodstock, with each group consisting of one male and female blue and yellow-green stains (Table 1).
Table 1. Experimental design for the crossbreeding of the boring giant clam between the blue and yellow-green strains.
Thirty hours after fertilization, D-stage larvae of each cohort (BB, BY, YB, and YY) were collected using a 60-μm sieve and maintained separately in 1,000-L tanks. The initial larval density was adjusted to 2 larvae/ml and maintained at this level by controlling the water volume. In the first 5 days, the larvae were fed with Isochrysis galbana at a density of 3,000 cells/ml/day. From the sixth to eight day, they were immersed in seawater containing symbiotic algae (zooxanthellae) at a density of 30 cells/ml for 2 h per day. The outdoor larval rearing tanks was equipped with gentle aeration, transparent polycarbonate roof sheeting, and a 50% light transmittance shade-cloth, of which reduced the daily-maximum photosynthetically active radiation (PAR) from 0 to 572.6 μmol/s/m2 [Dataflow Systems Pty Ltd. (Christchurch, New Zealand) light logger] (Braley et al., 2018; Militz et al., 2017, 2019). The water temperature and salinity were maintained at 28.5–29.7°C and 32 ppt, respectively.
Most larvae (≥90%) attached and developed secondary shells, feet, gills, and symbiotic system. They reached the juvenile stage on the 20th day. No substrate was used during the spat nursing stage. Larvae settle within 7 days, and then the newly settled spats were transferred to 1,000-L tanks filled with sand-filtered seawater, with maintained gentle aeration, water flow, and 50% natural lighting for 10 weeks. When the SL reached 3–5 mm, the spats were transferred to coral stone substrate and kept in 1,000-L tanks for another 4 weeks. When the SL of spat reached 8–10 mm, they were transferred to an artificial raceway system with continuous circulating water until they reach 1 year old. The water temperature and salinity were maintained at 25.7 to 30.2°C and 32 ppt, respectively.
The hatching index (cleaved rate and D-stage larva rate), survival rate (at days 7, 15, 90, and 360), and growth (egg diameter, D-stage larvae size, and spat size on the same day) of each group were determined following the method of Zhou et al. (2020). The survival rate on day 7 and day 15 was defined as the ratio of the number of individuals at different developmental stages to the number of D-stage larvae. The survival rate on day 90 and day 360 was defined as the ratio of the number of individuals at different stages to the number of spat on day 30.
On day 90, spats (SL approx. 3–5 mm) of each of the three replicates in each group (BB, YY, BY, and YB) were divided equally into 40 substrates (coral stone 16–18 cm in diameter) with a density of 30 individuals/substrate. In other words, there were 3,600 spats on 120 substrates for each group, and 14,400 spats on 480 substrates in the entire experiment. The substrates were cleaned monthly, dead clams were removed, and the density of each group was readjusted to maintain similar levels among the groups. As the spat grew, the density was reduced from 30 individuals/substrate to 15 individuals/substrate.
Mantle iridocyte samples among each group were imaged and determined by microspectrophotometry under a Zeiss AxioObserver D1M inverted microscope (Carl Zeiss AG, Oberkochen, Germany) equipped with a broadband halogen lamp (providing ample light in the measured range of 400–700 nm) that provided illumination. For spectroscopy, a small region of the sample (∼1-μm diameter) was imaged on the entrance slit (0.2 mm width) of the imaging spectrometer (Horiba JobinYvon iHR320; Horiba Group, Kyoto, Japan), with light entering the spectrometer dispersed horizontally by a grating (150 lines/mm, blazed for 500 nm). The resulting image was captured with a thermoelectrically cooled silicon charge-coupled device (Horiba JobinYvon Synapse detector) using an integration time of 0.05 s. The spectrum was obtained from an area less than a single iridocyte and normalized to a calibrated specular reflectivity standard (Ocean Optics STAN-SSH) for analyses. The 50 × objective was a Zeiss EC EpiPlan-NEOFLUAR lens with a numerical aperture of 0.8 and 3.8-μm depth of field. Other details of microspectrophotometer and analytical methods are as previously described in Ghoshal et al. (2016).
Two-way analysis of variance (Fisher’s) was used for multiple comparisons to analyze the differences of the average hatching index, survival rate, and growth parameters among groups. In order to improve the normality and homoscedasticity, prior to analysis, the hatching rate and survival rate were arcsine-transformed, and the growth parameters were logarithmically transformed (base 10). All statistical analyses were performed using SPSS 23.0. p < 0.05 was considered statistically significant for all tests, unless noted otherwise.
Mid-parental heterosis (H) was analyzed to evaluate the potential application of hybrids in aquaculture in the first year using the following formula (Zhang et al., 2007, 2017; Wang et al., 2011; Wang and Côté, 2012):
where XF1 represents the mean phenotypic value (SL, survival rate, etc.) of the reciprocal hybrids, while XBB and XYY represent the mean phenotypic value (SL, survival rate, etc.) of the blue and yellow-green strain progenies on the same day, respectively.
In order to determine the effects of egg origin (B vs. Y) and mating strategies (homozygous vs. heterozygous crosses) on the survival and growth of clams, a two-factor analysis of variance was used (Cruz and Ibarra, 1997; Zhang et al., 2007, 2017), as follows:
Here, Yijk is the mean SL, wet weight, or survival rate of k replicate from the i egg origin, and the j is the mating strategy. EOi is the effect of egg origin on SL (survival rate) (i = 1, 2). MSj is the effect of mating strategy on SL (survival rate) (j = 1, 2). (EO × MS)ij is the interaction effect between the egg origin and mating strategy, while eijk is the random observation error (k = 1, 2, 3).
Single-parent heterosis is the improved performance over the purebred offspring of the maternal strain, calculated using the following formula (You et al., 2015; Zhang et al., 2017, 2020b):
where IBY and IYB indicate single-parent heterosis for the BY and YB offspring, respectively. XBB, XYY, XBY, and XYB indicate the mean phenotypic value (SL, survival rate, etc.) of BB, YY, BY, and YB, respectively.
There was no significant difference in the mean egg size between the blue strain (94.84 ± 2.53 μm) and yellow-green strain (95.30 ± 1.09 μm) (p > 0.05) (Table 2). Highly cleaved rates (>90%) were observed among all four groups, suggesting that there were no sperm–egg recognition barriers between the two strains. The D-stage larva rate of the YY group (81.26 ± 15.77%) was significantly lower (p < 0.05) than that of the other three groups, which was mainly affected by both mating strategies and egg origin.
Table 2. Hatching index, survival ability and metamorphism of two strain’s progeny (BB and YY) and their reciprocal hybrids (BY and YB), as well as heterosis (H and I).
On day 7, the mean survival rate of larvae in all four groups exceeded 90%, but the survival rate of the BY group was lower than that of other three groups. The heterosis of larval survival rate was −2.21%, and the single heterosis of the BY and YB progenies were −2.25 and −2.18%, respectively. The survival rate of larvae was affected by egg origin (Tables 2, 3). On day 15, the metamorphosis rates of BB (31.87 ± 10.57%) and BY (37.28 ± 16.84%) were significantly higher (p < 0.05) than those of YY (8.69 ± 5.81%) and YB (16.83 ± 10.48%). Mid-parental heterosis was 33.41%, and the single heterosis of the BY and YB groups were 16.98 and 93.67%, respectively. The rate of metamorphosis was primarily affected by egg origin (Tables 2, 3).
Table 3. Analysis of variance (ANOVA) showing the egg origin (EO) and mating strategy (MS) effects for survival and growth of each experimental group at different time points.
On day 90, the survival rates of progeny of all four groups were over 85%. The survival rates of the BY (94.61 ± 9.17%) and YB (90.45 ± 5.80%) progenies were significantly higher (p < 0.05) than those of the BB (85.10 ± 9.47%) and YY (87.72 ± 7.40%) progenies. Mid-parental heterosis was 3.86%, and the single heterosis of the BY and YB groups were 3.08 and 4.60%, respectively. The survival rate of spat was mainly affected by the interaction of egg origin and mating strategies (Tables 2, 3). On day 360, the survival rates of all four groups exceeded 80%, with the survival rates of reciprocal hybrids being slightly higher (p > 0.05) than those of the two pure strains. The mid-parental heterosis for survival was 3.37%, and the single heterosis of the BY and YB groups were 1.15 and 5.64%, respectively. The survival rate of giant clam spats was also affected by the interaction of egg origin and mating strategies (Tables 2, 3).
Thirty hours after fertilization, the size of D-stage larvae of the BY group was smaller than that of the other three groups without a significant difference, showing obvious effects of mating strategies (Tables 3, 4). At the end of the planktonic stage, the SL of the BB group was the largest among the four groups with a significant difference, which was affected by the maternal effect (Tables 3, 4). On day 15, the SL of BY (257.33 ± 55.48 μm) and YB (230.38 ± 53.18 μm) was significantly larger (p < 0.05) than that of BB (215.03 ± 40.02 μm) and YY (203.70 ± 17.66 μm). The mid-parental heterosis was 16.47%, and the single heterosis of the BY and YB groups were 19.67 and 13.10%, respectively (Table 4).
Table 4. Growth index of of two strain’s progeny (BB and YY) and their reciprocal hybrids (BY and YB), at different days post-fertilization, as well as heterosis (H and I).
On day 90, the SL of reciprocal hybrid progeny was significantly larger (p < 0.05) than that of pure strains, showing the interaction effect of egg origin and mating strategies (Tables 3, 4). The mid-parental heterosis was 10.18%, and the single heterosis of the BY and YB groups were 9.86% and 10.50%, respectively (Table 4). On day 360, SL of BB (37.86 mm) was significantly smaller (p < 0.05) than that of other three groups (40.83–45.46 mm), which was affected by the interaction between egg origin and mating strategies (Tables 3, 4). The mid-parental heterosis was 12.35%, and the single heterosis of the BY and YB groups were 13.44 and 11.34%, respectively (Table 4).
At 1 year of age, the mantle color of the BB progeny was 100% blue, and the blue iridocytes have a light absorption peak at 475 nm. The mantle color of the YY progeny was 100% yellow-green, and the yellow-green iridocytes have a light absorption peak range from 550 to 650 nm (Table 5 and Figures 1, 2). In contrast, the BY and YB progenies both showed mixed colors, including blue, yellow, and green colors. These iridocytes of all hybrids have two peaks at 475 and 550–650 nm (Table 5 and Figures 1, 2). In other words, the mantle color patterns of the reciprocal hybrids displayed genetic recombination, which can be defined as a phenomenon of the common dominant expression derived from two strains’ parents.
Table 5. Mantle coloration pattern of two strain’s progeny (BB and YY), and their reciprocal hybrids (BY and YB).
Figure 1. Photos of progeny for boring giant clam Tridacna crocea. (A) BB progeny. (B) YY progeny. (C) BY Progeny. (D) YB Progeny. The bar indicates 30 mm, and it applies to all samples in Figure 1.
Figure 2. Spectra from individual iridocytes from Tridacna crocea, corresponding to Figure 1. (A) BB progeny. (B) YY progeny. (C) BY Progeny. (D) YB Progeny. Each line indicates a single iridocyte’s color with one main peak in the outer mantle.
Fertilization rate is a key parameter used to evaluate the commercial production potential of crossbreeding progeny (You et al., 2015). Our study demonstrates that using one of the mantle color strain as a sperm donor (two-way fertilization) can achieve a higher fertilization rate and hatching rate, which indicates that there is no reproductive isolation between blue and yellow-green mantle color strains (Zhou et al., 2020; Zhang et al., 2021).
Zhou et al. (2021) previously investigated the growth difference between blue and yellow-green mantle color strains of boring giant clams and concluded that in wild populations, the yellow-green broodstock is always larger than the blue broodstock. Generally, the heterosis of a crossbreed between two particular strains depends on the square of the difference in gene frequency between strains. If the crossed strains have no difference in gene frequency, there will be no heterosis, while when one allele is fixed in one strain and the other allele is fixed in another strain, the heterosis will be the greatest (Zhang et al., 2007; Wang et al., 2011; Wang and Côté, 2012; Ma et al., 2021). In our study, the size of the reciprocal hybrids was larger than the progenies of the two pure strains during the grow-out stage, and these hybrids obviously have heterosis with a simple epistatic effect. Since differently colored iridocytes in the mantle have different solar utilization efficiencies, they caused growth differences between the two pure strains and the reciprocal hybrids (Holt et al., 2014).
As an aquaculture animal, the viability of the boring giant clam is a very important performance trait, and it is known to be affected by the surrounding environment (Rawson and Feindel, 2012). In our study, the survival rate of reciprocal hybrids was higher than that of pure strains, which is manifested as a significant increase in the level of metamorphosis. The reciprocal hybrids have three iridocytes, while the blue and yellow-green strains have one or two iridocytes, which means that the hybrids have a higher degree of heterozygosity than other strains (Brake et al., 2004). The higher heterozygosity of the hybrid means higher physiological and immune levels, thus a higher survival rate than the progenies of two pure stains.
The mantle coloration of giant clams can be divided into three stages: non-coloring period (days 0–60), coloring period (days 60–180), and stable expression period (>day 180) (Zhou et al., 2021). During the non-coloring period, no iridocytes occur, so the mantle color looks brown due to the accumulation of zooxanthellae. During the coloring period, iridocytes in the mantle begin to appear and increase in number, until they become visible to the naked eye about 6 months later. Interestingly, green iridocytes were found in early stage of both pure strains and reciprocal hybrids, suggesting that the green iridocyte may be ancestors of the others (Zhou et al., 2021).
After the early green iridocytes changed to blue (60–120 days), the mantle colors of the reciprocal hybrids were both blue during the coloring stage (120–180 days), which indicates that the expression of blue iridocytes is dominant in the mantle. The dominant expressions of phenotypic traits for progeny usually occur in the process of hybridization, which is likely to be an essential transitional phase in the overall life history of giant clams and may reflect the evolutionary strategy of nature. In some animals, particular color morphs may enhance camouflage, improve communication within species, and/or confer them a reproductive advantage (Korzan et al., 2008). Therefore, this staged dominant coloration is likely to be a camouflage, which may improve the survival rate of giant clam populations in wild coral reef areas (Fatherree, 2006; Neo et al., 2015).
During the stable expression period, the mantle color of two pure strains was 100% inherited, while all reciprocal hybrids have colorful colors in the mantle, which indicates that the genetic recombination of the mantle color has occurred. Genetic recombination usually occurs during meiosis, which involves the pairing of homologous chromosomes and is a key developmental program for gametogenesis (Brick et al., 2012). Novel phenotypic features are often formed in hybrid, which may accelerate the processes of species diversity via adaptive evolution (Abbott et al., 2013). In aquatic animals, the research on genetic recombination of body surface coloration is mainly focused on the ornamental carp (David et al., 2004). The mantle coloration of marine bivalves was only found on oysters and giant clams using hybridization or crossbreeding methods (Wang et al., 2015; Zhou et al., 2021). Furthermore, our results reveal that the colorful mantle of the giant clams may be caused by multiple random crossings between two individuals with different mantle colors during the reproductive stage.
Giant clams are often covered with unique patterns and various colors. For a long time, why they look as fancy has been a mystery (Fatherree, 2006; Neo et al., 2015; Rossbach et al., 2021). In the aquarium market, the price of giant clam is partially determined according to the different mantle colors. For example, blue and purple giant clams are known as “amethyst,” and their price is four to five times that of others varieties. The blue Tridacna squamosa can be sold for $180/ind, because the blue T. squamosa is an interspecific hybrid and is rare in nature. It is derived from female T. squamosa and male Tridacna crocea. Recently, these hybrids with heterosis have been mass produced, and they have the mantle color of T. crocea and shell of T. squamosa (Zhou et al., 2020).
With the use of mantle color as an indicator, it is important to carry out genetic breeding of giant clams, because the specific mantle color of individual clam can significantly increase economic value (Frias-Torres, 2017). According to our study, the mantle color lines can be produced by selective breeding, crossbreeding, and interspecific hybridization, which can then be used to breed new varieties of giant clams (Zhang et al., 2020a,b; Zhou et al., 2021). Therefore, this study provides a basic understanding and analysis of the mantle coloring inheritance of the boring giant clam and provides practice for the breeding of new giant clam varieties.
First, we found that the mantle color of blue and yellow-green giant clams can be stably inherited. Reciprocal hybrids express genetic recombination in the mantle color pattern and showed common dominant expression. This discovery demonstrates the formation and evolution of the mantle color polymorphism of T. crocea and provides a new direction for understanding the environmental adaptability and genetic breeding of the boring giant clams in the coral reef area.
The original contributions presented in the study are included in the article/supplementary materials, further inquiries can be directed to the corresponding author/s.
ZY and YHZ conceived and designed the experiments. ZZ, JL, HM, YQ, JW, XL, QL, and YL performed the experiments. YYZ, ZZ, GS, and YHZ analyzed the data. JW, YHZ, ZZ, JL, and ZY wrote the manuscript. All authors contributed to the article and approved the submitted version.
This work was supported by the Chinese Ministry of Science and Technology through the National Key Research and Development Program of China (2018YFD0901400 and 2020YFD0901100); the National Science Foundation of China (31872566, 31702340, and 32002387); Key Special Project for Introduced Talents Team of Southern Marine Science and Engineering Guangdong Laboratory (Guangzhou) (GML2019ZD0404); the China-ASEAN Maritime Cooperation Fund (CAMC-2018F); the Network Service Local Plan STS of the Chinese Academy of Sciences (KFJ-STS-QYZD-158); the Strategic Priority Research Program of the Chinese Academy of Sciences (XDA13020202); the Innovation Academy of South China Sea Ecology and Environmental Engineering, Chinese Academy of Sciences (ISEE2018PY01 and ISEE2018ZD02); the Open Foundation of the State Key Laboratory of Loess and Quaternary Geology (SKLLQG1813 and SKLLQG1918); the China Agricultural Shellfish Industry Technology System Project (CARS-49); and the Science and Technology Planning Project of Guangdong Province, China (2017B030314052).
The authors declare that the research was conducted in the absence of any commercial or financial relationships that could be construed as a potential conflict of interest.
The authors would like to thank the editor and several reviewers for their assistance in the manuscript revision process.
Abbott, R., Albach, D., Ansell, S., Arntzen, J. W., Baird, S. J., Bierne, N., et al. (2013). Hybridization and speciation. J. Evol. Biol. 26, 229–246. doi: 10.1111/j.1420-9101.2012.02599.x
Brake, J., Evans, F., and Langdon, C. (2004). Evidence for genetic control of pigmentation of shell and mantle edge in selected families of Pacific oysters, Crassostrea gigas. Aquaculture 229, 89–98. doi: 10.1016/S0044-8486(03)00325-9
Braley, R. D., Militz, T. A., and Southgate, P. C. (2018). Comparison of three hatchery culture methods for the giant clam Tridacna noae. Aquaculture 495, 881–887. doi: 10.1016/j.aquaculture.2018.05.044
Brick, K., Smagulova, F., Khil, P., Camerini-Otero, R. D., and Petukhova, G. V. (2012). Genetic recombination is directed away from functional genomic elements in mice. Nature 485, 642–645.
Cruz, P., and Ibarra, A. M. (1997). Larval growth and survival of two Catarina scallop (Argopecten circularis, Sowerby, 1835) populations and their reciprocal crosses. J. Exp. Mar. Biol. Ecol. 212, 95–110. doi: 10.1016/S0022-0981(96)02742-6
Cuthill, I. C., Allen, W. L., Arbuckle, K., Caspers, B., Chaplin, G., Hauber, M. E., et al. (2017). The biology of color. Science 357:eaan0221. doi: 10.1126/science.aan0221
David, L., Rothbard, S., Rubinstein, I., Katzman, H., Hulata, G., Hillel, J., et al. (2004). Aspects of red and black color inheritance in the Japanese ornamental (Koi) carp (Cyprinus carpio L.). Aquaculture 233, 129–147. doi: 10.1016/j.aquaculture.2003.10.033
Fatherree, J. (2006). Giant Clams in the Sea and the Aquarium: The Biology, Identification, and Aquarium Husbandry of Tridacnid Clams. Florida: Liquid Medium.
Frias-Torres, S. (2017). Captive bred, adult giant clams survive restoration in the wild in Seychelles, Indian Ocean. Front. Mar. Sci. 4:97. doi: 10.3389/fmars.2017.00097
Ghoshal, A., Eck, E., Gordon, M., and Morse, D. E. (2016). Wavelength-specific forward scattering of light by Bragg-reflective iridocytes in giant clams. J. R. Soc. Interface 13:20160285. doi: 10.1098/rsif.2016.0285
Griffiths, D. J., Winsor, H., and Luongvan, T. (1992). Iridophores in the mantle of giant clams. Aust. J. Zool. 40, 319–326. doi: 10.1071/ZO9920319
Holt, A. L., Vahidinia, S., Gagnon, Y. L., Morse, D. E., and Sweeney, A. M. (2014). Photosymbiotic giant clams are transformers of solar flux. J. R. Soc. Interface 11:20140678. doi: 10.1098/rsif.2014.0678
Kim, H. N., Vahidinia, S., Holt, A. L., Sweeney, A. M., and Yang, S. (2017). Geometric design of scalable forward scatterers for optimally efficient solar transformers. Adv. Mater. 29:1702922. doi: 10.1002/adma.201702922
Korzan, W. J., Robison, R. R., Zhao, S., and Fernald, R. D. (2008). Color change as a potential behavioral strategy. Horm. Behav. 54, 463–470. doi: 10.1016/j.yhbeh.2008.05.006
Long, C., Zhang, Y., Li, Y., Li, J., Zhou, Z., Qin, Y., et al. (2021). Effects of Symbiodinaaceae phylotypes in clades A-E on progeny performance of two giant clams (Tridacna squamosa and T. crocea) during early history life stages in the South China Sea. Front. Mar. Sci. 8:633761. doi: 10.3389/fmars.2021.633761
Ma, H., Gao, H., Zhang, Y., Qin, Y., Xiang, Z., Li, J., et al. (2021). Multiplex species-specific PCR identification of native giant clams in the South China Sea: a useful tool for application in giant clam stock management and forensic identification. Aquaculture 531:735991. doi: 10.1016/j.aquaculture.2020.735991
Mäthger, L. M., Senft, S. L., Gao, M., Karaveli, S., Bell, G. R., Zia, R., et al. (2013). Bright white scattering from protein spheres in color changing, flexible cuttlefish skin. Adv. Funct. Mater. 23, 3980–3989. doi: 10.1002/adfm.201203705
Mies, M. (2019). Evolution, diversity, distribution and the endangered future of the giant clam-Symbiodiniaceae association. Coral Reef 38, 1067–1084. doi: 10.1007/s00338-019-01857-x
Militz, T. A., Braley, R. D., and Southgate, P. C. (2017). Captive hybridization of the giant clams Tridacna maxima (Röding, 1798) and Tridacna noae (Röding, 1798). J. Shellfish Res. 36, 585–591. doi: 10.2983/035.036.0306
Militz, T. A., Braley, R. D., Schoeman, D. S., and Southgate, P. C. (2019). Larval and early juvenile culture of two giant clam (Tridacninae) hybrids. Aquaculture 500, 500–505. doi: 10.1016/j.aquaculture.2018.10.050
Neo, M. L., Eckman, W., Vicentuan, K., Teo, S. L.-M., and Todd, P. A. (2015). The ecological significance of giant clams in coral reef ecosystems. Biol. Conserv. 181, 111–123. doi: 10.1016/j.biocon.2014.11.004
Ozog, S. T. (2009). Balancing Anti-Predation and Energetic Needs: Color Polymorphism in the Giant Clam Tridacna Maxima. Berkeley, CA: University of California.
Rawson, P., and Feindel, S. (2012). Growth and survival for genetically improved lines of Eastern oysters (Crassostrea virginica) and interline hybrids in Maine, USA. Aquaculture 326, 61–67. doi: 10.1016/j.aquaculture.2011.11.030
Rossbach, S., Anton, A., and Duarte, C. M. (2021). Drivers of the abundance of Tridacna spp. giant clams in the Red Sea. Front. Mar. Sci. 7:592852. doi: 10.3389/fmars.2020.592852
Rossbach, S., Subedi, R. C., Ng, T. K., Ooi, B. S., and Duarte, C. M. (2020). Iridocytes mediate photonic cooperation between giant clams (Tridacninae) and their photosynthetic symbionts. Front. Mar. Sci. 7:465. doi: 10.3389/fmars.2020.00465
Wang, C., and Côté, J. (2012). Heterosis and combining abilities in growth and survival in sea scallops along the Atlantic coast of Canada. J. Shellfish Res. 31, 1145–1149. doi: 10.2983/035.031.0425
Wang, C., Liu, B., Li, J., Liu, S., Li, J., Hu, L., et al. (2011). Introduction of the Peruvian scallop and its hybridization with the bay scallop in China. Aquaculture 310, 380–387. doi: 10.1016/j.aquaculture.2010.11.014
Wang, Q., Li, Q., Cong, R., Kong, N., and Kong, L. (2015). Inheritance of mantle pigmentation in selected families of the Pacific oyster Crassostrea gigas. Mar. Sci. 39, 86–90.
You, W., Guo, Q., Fan, F., Ren, P., Luo, X., and Ke, C. (2015). Experimental hybridization and genetic identification of Pacific abalone Haliotis discus haHHai and green abalone H. fulgens. Aquaculture 448, 243–249. doi: 10.1016/j.aquaculture.2015.05.043
Zhang, H., Liu, X., Zhang, G., and Wang, C. (2007). Growth and survival of reciprocal crosses between two bay scallops, Argopecten irradians concentricus Say and A. irradians irradians Lamarck. Aquaculture 272, S88–S93. doi: 10.1016/j.aquaculture.2007.08.008
Zhang, Y., Li, J., Zhang, Y., Ma, H., Xiao, S., Xiang, Z., et al. (2017). Performance evaluation of reciprocal hybrids derived from the two brackish oysters, Crassostrea hongkongensis and Crassostrea sikamea in southern China. Aquaculture 473, 310–316. doi: 10.1016/j.aquaculture.2017.02.031
Zhang, Y., Ma, H., Li, X., Zhou, Z., Li, J., Wei, J., et al. (2020a). Analysis of inbreeding depression on performance traits of three giant clams (Tridacna derasa, T. squamosa, and T. crocea) in the South China Sea. Aquaculture 521:735023. doi: 10.1016/j.aquaculture.2020.735023
Zhang, Y., Zhou, Z., Qin, Y., Li, X., Ma, H., Wei, J., et al. (2020b). Phenotypic traits of two boring giant clam (Tridacna crocea) populations and their reciprocal hybrids in the South China Sea. Aquaculture 519:734890. doi: 10.1016/j.aquaculture.2019.734890
Zhou, Z., Li, J., Ma, H., Li, Y., Qin, Y., Wei, J., et al. (2021). The evaluation of culture performance and mantle coloration of two boring giant clam (Tridacna crocea) strains. Aquaculture Rep. 20:100646. doi: 10.1016/j.aqrep.2021.100646
Keywords: Tridacna crocea, the boring giant clam, crossbreeding, mantle color’s pattern, genetic recombination
Citation: Wang J, Zhou Z, Ma H, Li J, Qin Y, Wei J, Li X, Liao Q, Li Y, Shi G, Zhou Y, Zhang Y and Yu Z (2021) Genetic Recombination of the Mantle Color Pattern of Two Boring Giant Clam (Tridacna crocea) Strains. Front. Mar. Sci. 8:657762. doi: 10.3389/fmars.2021.657762
Received: 23 January 2021; Accepted: 03 June 2021;
Published: 09 July 2021.
Edited by:
Xiaotong Wang, Ludong University, ChinaCopyright © 2021 Wang, Zhou, Ma, Li, Qin, Wei, Li, Liao, Li, Shi, Zhou, Zhang and Yu. This is an open-access article distributed under the terms of the Creative Commons Attribution License (CC BY). The use, distribution or reproduction in other forums is permitted, provided the original author(s) and the copyright owner(s) are credited and that the original publication in this journal is cited, in accordance with accepted academic practice. No use, distribution or reproduction is permitted which does not comply with these terms.
*Correspondence: Yuehuan Zhang, eWh6aGFuZ0BzY3Npby5hYy5jbg==; Ziniu Yu, Y2FybHp5dUBzY3Npby5hYy5jbg==
†These authors have contributed equally to this work
Disclaimer: All claims expressed in this article are solely those of the authors and do not necessarily represent those of their affiliated organizations, or those of the publisher, the editors and the reviewers. Any product that may be evaluated in this article or claim that may be made by its manufacturer is not guaranteed or endorsed by the publisher.
Research integrity at Frontiers
Learn more about the work of our research integrity team to safeguard the quality of each article we publish.