- 1Department of BioMolecular Sciences, University of Mississippi, Oxford, MS, United States
- 2Department of Molecular, Cellular and Biomedical Sciences, School of Marine Science and Ocean Engineering, University of New Hampshire, Durham, NH, United States
Foundation species (FS) regulate ecological processes within communities often facilitating biodiversity and habitat complexity. Typically FS are dominant structure-forming taxa; but less dominant taxa having disproportionate ecological impacts to the community can also be FS. Mesophotic coral ecosystems (MCEs) are deep coral reef (∼30–150 m) communities, often dominated by emergent sponges in the Caribbean Basin. Despite the potential competitive advantage of sponges on MCEs, gorgonians are also common constituents of these reefs. Data from the Bahamas demonstrate increased biodiversity and densities of sponges on mesophotic reefs with gorgonians relative to reefs without these species. Drawing upon fifteen years of field surveys at five sites in the Caribbean Basin we assessed in situ interactions between gorgonians and sponges to quantify outcomes consistent with competition (i.e., tissue necrosis and overgrowth). Gorgonians were effective competitors against a variety of sponges, and two allelochemicals produced by Ellisella elongata were mechanistically important in interactions with Agelas clathrodes. We also examined invertebrate recruitment patterns near gorgonians to assess their role in facilitating MCE biodiversity. Our results indicate that live gorgonians, Antillogorgia bipinnata and E. elongata, facilitate biodiverse recruitment into MCEs, indicating that this process is governed by more than passive hydrodynamics. Collectively, these data indicate that these gorgonians exhibit both positive and negative ecological interactions (i.e., facilitation and competition, respectively) with sponges, and other taxa. Thus, these gorgonians are FS of MCE communities within the Caribbean Basin that display several traits contributing to the ecological structure of these understudied communities.
Introduction
Foundation species (FS) are critical constituents of a community that promote the success of other species by regulating ecological processes (Dayton, 1972); they are typically dominant structure-forming taxa that occupy low trophic positions within an ecosystem (e.g., red mangroves: Ellison et al., 1996; giant kelp: Lamy et al., 2020). However, less dominant and undersized species can also be FS (ark cockle: Gribben et al., 2009). These FS can be further parsed into primary FS responsible for the initial habitat modification that facilitates community structure and function (Dayton, 1972), and secondary FS (i.e., obligate FS: require primary habitat modification) that add important biocomplexity and/or biodiversity to the habitat (Thomsen et al., 2018). Multiple FS can coexist within the same habitat, or they can live in contiguous zones (“nested” and “adjacent” assemblages, respectively: Angelini et al., 2011). In marine communities, FS provide important ecosystem services that augment biodiversity through substrate stabilization (Wulff, 1984), water filtration (Altieri and Witman, 2006), and/or nutrient transfer (Fiore et al., 2013), as well as defense of other species (Hay, 1986). However, corals are often treated as a single functional FS regardless of the number of species, and their roles, within the community (Knowlton and Jackson, 2001). Recently, gorgonian corals have been identified as FS that enhance community diversity and ecological functioning (McLean and Yoshioka, 2007; Verdura et al., 2019). They can facilitate larval settlement (Ponti et al., 2014; Privitera-Johnson et al., 2015), and enhance sponge growth (Mercado-Molina et al., 2018), possibly by altering their hydrodynamic environment (Gili and Coma, 1998). Moreover, gorgonians provide significant structure and function to shallow (1–30 m) and deeper (30–100 m) coral reefs throughout the Caribbean Basin (Tsounis et al., 2018; Sánchez et al., 2019; Lasker et al., 2020).
The worldwide decline of corals, due to natural and anthropogenic stressors (Hughes et al., 2017), has resulted in regime shifts from hard coral dominance to algal, sponge, or gorgonian dominance (Norstrom et al., 2009). The roles of increasing algal cover on coral reefs has been well documented (McCook et al., 2001; Hay and Rasher, 2010), but all three structural groups are active competitors with corals, and with each other (Chadwick and Morrow, 2011). Although ecological processes such as overgrowth and/or shading are common amongst these taxa, contact-mediated or water-borne delivery of allelopathic compounds is another competitive option for some species of algae, sponges, and gorgonians (Granéli and Pavia, 2006; Slattery and Gochfeld, 2012). For example, allelochemicals from the Brazilian gorgonian, Phyllogorgia dilatata, reduced growth rates in the zoanthid, Palythoa caribaeorum (Ribeiro et al., 2017). Likewise, 29 gorgonian species exhibited competitive interactions that effected spatial distributions of this taxa in St. John USVI (Gambrel and Lasker, 2016). In addition, taxonomic relatives (i.e., Indo-Pacific soft corals) also demonstrate significant allelopathic activities against hard and soft corals (Maida et al., 2001; Slattery et al., 2008).
Mesophotic coral ecosystems (MCEs) are deep reef communities (∼30–150 m depth) structured by strong gradients of solar irradiance and food availability (Lesser et al., 2009, 2018). They are typically less affected by land-based anthropogenic stressors and are often below the depth limits of natural stressors affecting shallow reefs (but see: Lesser and Slattery, 2011; Smith et al., 2016; Slattery et al., 2018). This has led to speculation that MCEs, as a whole, are relatively stable habitats that might serve as important refuges or nursery grounds for depth-generalists that could reseed damaged shallow reefs through larval connectivity (Bongaerts et al., 2010; Slattery et al., 2011) although recent data indicate this “deep reef refuge hypothesis” is clearly context-dependent (e.g., Bongaerts et al., 2017). Throughout the Caribbean Basin MCEs are often dominated by habitat-forming sponges. Sponge biomass and density increase dramatically within these MCEs (Slattery and Lesser, 2012; Lesser and Slattery, 2018), and there is a clear demarcation between the transitional communities of the upper mesophotic (∼30–60 m) and the endemic communities of the lower mesophotic (∼61–150 m) (Lesser et al., 2019). While sponges dominate many MCE communities (Pomponi et al., 2019), other taxa, including gorgonian corals, also co-occur at high densities at these depths (Sánchez et al., 2019). Here we examine the ecological roles of the dominant species of Caribbean MCEs (i.e., sponges and gorgonians), and assess the relationships between these species through strong positive and negative interactions (i.e., facilitation and competition, respectively). Specifically, we test the hypotheses that: (1) gorgonians are the primary FS of MCEs, and (2) the gorgonian Ellisella elongata structures MCEs with allelopathic compounds.
Materials and Methods
Study Sites
This study was conducted on MCEs in the Bahamas (Exumas Archipelago, Bock Wall: 23°46.5′ N, 76°05.5′ W; BA Line: 23°46′ N, 76° 06′ W), the Cayman Islands (Grand Cayman, Sand Chute: 19°21.43′ N, 81°24.44′ W; Little Cayman, Rock Bottom Wall: 19°42.03′ N, 80°03.25′ W), Curaçao (Buoy 1: 12°7.33′ N, 69°03.8′ W), and Honduras (Utila, CJ’s Drop-off: 16°4.59′ N, 86°55.1′ W) over the period from 2003 to 2018 (Figure 1). Research in the Bahamas included field surveys, as well as manipulative laboratory and field experiments, over multiple years, while research at the other locations represent a few weeks of opportunistic field surveys. Data were collected via open-circuit and closed-circuit (i.e., rebreather) trimix technical dives at depths of 30–91 m. Given the extensive decompression schedules associated with the deeper dives, fewer replicate quadrats and transects were conducted at those depths; this resulted in unequal sample sizes relative to gorgonian and sponge density assessments.
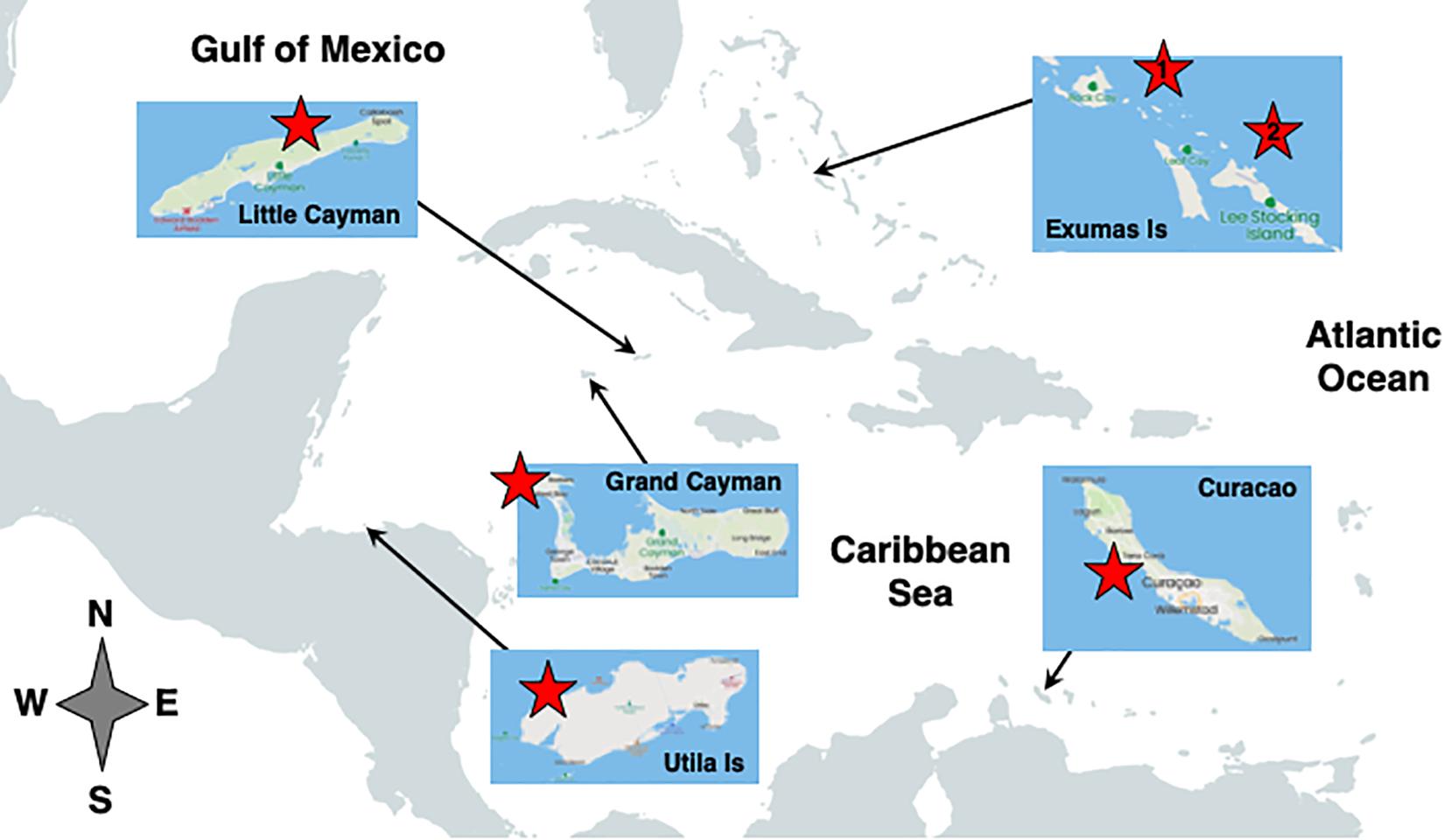
Figure 1. Location of MCE survey sites within the Caribbean Basin. Red stars indicate the approximate location of the survey sites at each location (see text for specific GPS coordinates); Bahamas site 1 = Bock Wall, and site 2 = BA Line, both in the Exumas Island chain. Figure was constructed from maps provided for free distribution by OpenStreetMap contributors.
Gorgonian and Sponge Densities at Mesophotic Depths
Gorgonian and sponge diversity and abundance were surveyed in replicate 1 m2 quadrats (n = 5–10) at random points along replicate 30 m transects (n = 3–9) at depths of 30, 46, 61, 76, and 91 m at all of the aforementioned sites, except Curaçao where surveys were conducted to a maximum depth of 61 m (Slattery and Lesser, 2019; and see Supplementary Table 1 for total replicates at each depth). Our sponge density data have been reported previously (Lesser and Slattery, 2018) for all sites except the BA Line, Bahamas, which we compared to Bock Wall, since the former site lacks gorgonians, and the latter site has a dense gorgonian population. These data were assessed using a two-way analysis of variance (ANOVA) with site and depth as the fixed factors. Significance in any of the main effects were further parsed using Tukey’s HSD post hoc test. Prior to all analyses, the data were tested for normality and homogeneity of variance and transformed as needed. All data within are presented as the mean ± SE.
Gorgonian abundance throughout the Caribbean Basin was analyzed using a one-way ANOVA, with site as the fixed factor. Due to the patchy distribution of gorgonians on these MCEs, all gorgonians were combined into “upper mesophotic” (30 and 46 m) and “lower mesophotic” (61, 76, and 91 m) groupings, consistent with the ecological zones described in Lesser et al. (2019), and the depth differences were tested using an unpaired Student’s t-test. The abundance of the dominant gorgonian species in the upper and lower mesophotic zones, Antillogorgia bipinnata and Ellisella elongata (formerly Pseudopterogorgia bipinnata and E. barbadensis: Castro et al., 2010; Williams and Chen, 2012), were also compared using an unpaired Student’s t-test.
Facilitation of Invertebrate Recruitment by Gorgonians
At each of our five sites within the Caribbean Basin we quantified invertebrate recruitment near the bases of A. bipinnata and E. elongata colonies, as well as to bare substrate further away, in the upper and lower mesophotic zones. The larvae of sponges, hard corals, and gorgonians typically recruit to rocky substrata and/or components of that substrate such as crustose coralline red algae (Maldonado, 2006; Ritson-Williams et al., 2009) that are common on many MCE reefs throughout the Caribbean (Slattery and Lesser, pers. obs.). Replicate 5 × 5 gridded m2 quadrats (n = 4) were positioned around conspecific patches of gorgonians (n = 1–4 colonies) at 45 ± 3 m and 75 ± 3 m, except in Curaçao where the lower mesophotic recruitment surveys occurred at 61 ± 3 m. We planned to assess recruitment near several dominant sponges (e.g., Agelas conifera, Plakortis spp., and Xestospongia muta) but there was either no recruitment or limited conspecific recruitment surrounding these taxa (Slattery and Lesser, pers. obs.). Replicate quadrats (n = 4) were positioned on “hardpan” substrate at each depth as a control for larval settlement cues (i.e., chemical and physical cues: Pawlik, 1992), and at least 5 m from any other conspecific or heterospecific gorgonians. However, a few of these plots (n = 11 of 80) included some turf and/or foliose algae, and/or small sponges (<5% cover of these structural groups). Within each quadrat, all invertebrate recruits were identified to the lowest possible taxon, counted, and their position relative to the central gorgonian(s) was noted. The recruit diversity (H’) and richness (S) were compared using a three-way ANOVA with site, depth, and treatment (i.e., quadrats: A. bipinnata, E. elongata, and hardpan) as fixed factors.
To address the influence of inherent quadrat-specific variables within our recruitment survey data, we conducted a field experiment into cleared 1 m2 plots at depths of 45 m and 75 m in the Bahamas, near the Caribbean Marine Research Center (CMRC). Quadrats (n = 12 at each depth) were affixed to the reef using stainless steel screws, and the substrate within was scoured to hardpan, using wire brushes and paint scrapers (Slattery and Lesser, 2014). Ellisella elongata colonies (∼30–60 cm tall) were collected from populations at 45 ± 3 m and 75 ± 3 m and transplanted into a “common garden” at 35 m, where they were affixed with Z-Spar epoxy compound into numbered PVC caps (2.5 cm diameter; Kim and Lasker, 1997), and allowed to acclimatize for 14 d. Dead gorgonian skeletons were collected from the beaches near CMRC, and the branchlets were trimmed from the main stalk to mimic the whip-like structure of E. elongata; these were also affixed into numbered PVC caps and transported to the common garden site. A 3 × 3 rack of nine “gorgonian caps” spaced 15 cm apart was positioned in the center of each quadrat and cable-tied to stainless steel “eye” bolts anchored in the substrate. These experimental racks contained either living E. elongata (n = 6) or dead gorgonian skeletons (n = 6) at each depth. This density exceeds averages of E. elongata recorded in our field surveys (see below), but it is representative of the patchy abundances of gorgonians on our transects (Slattery and Lesser, pers. obs.). Quadrats were examined 12 mo later, and within each quadrat all recruits were identified to the lowest possible taxon and enumerated. These data were analyzed using a two-way ANOVA with depth and treatment (i.e., live vs dead gorgonians) as fixed factors.
Gorgonian–Sponge Interactions
All contact interactions between gorgonians and sponges in the quadrats were quantified, as well as responses for each species pair (categorized as tissue necrosis and/or overgrowth). Although mortality was a potential outcome, we did not include this endpoint since we could not definitively attribute cause of death during single time-point observations. Given the limited number of contact interactions, we consolidated observations from all locations and collapsed the five depths into upper and lower mesophotic. These data were analyzed using a one sample Wilcoxon signed rank test with two potential outcomes (i.e., “win” or “lose”) compared to the null hypothesis (i.e., “draw”; Ladd et al., 2019).
Competition Between Gorgonians, Sponges, and Ellisella elongata
The success of E. elongata within the sponge-dominated lower mesophotic community was addressed in field and laboratory experiments. Live and dead gorgonian caps were cable-tied in contact with one of three sponge species (Agelas clathrodes, Plakortis angulospiculatus, or Xestospongia muta) at the common garden site. This provided contact treatments (live gorgonians; n = 12 replicates per sponge species) and contact controls (dead gorgonians; n = 12 replicates per sponge species). In addition, replicate non-contact sponges (n = 12 replicates per sponge species) were identified by attaching numbered cow-tags adjacent to each individual. The condition of these contact pairs and non-contact individuals were examined at three time points post-acclimatization (1, 3, and 12 mo) for evidence of overgrowth, tissue necrosis, or mortality. This allowed documentation of species responses through time, as well as competitive reversals (Chadwick and Morrow, 2011). Data were analyzed using the non-parametric equivalent of a repeated measures ANOVA (= Friedman’s Test) to address the number of interactions with the outcomes: win, lose, or draw. In addition to gorgonian-sponge competition, we also assessed competition between A. bipinnata and E. elongata. At each of two depths (45 and 75 m), we cabled-tied acclimatized gorgonian caps containing either A. bipinnata or E. elongata into contact with replicate conspecifics or heterospecifics (n = 15 of each combination). The responses of each gorgonian in these contact pairs were recorded after 3 mo and analyzed using a 3 × 2 contingency table that compared expected (= no allelopathic effect; healthy tissue) to observed rates of necrotic and/or overgrown tissue.
The allelopathic potential of E. elongata was assessed in samples from the Bahamas. Approximately 1,500 g wet mass of this gorgonian was collected from each of two depths (45 ± 3 m and 75 ± 3 m). In addition, 60 cc syringe arrays were used to collect replicate water samples at fixed distances of 0.5, 1.0, 1.5, and 2.0 cm from the surface of E. elongata (n = 4 each; Slattery et al., 1997) at each depth. Samples were returned to CMRC on ice and stored at -40°C for up to 2 mo prior to transport to the University of Mississippi. Tissue samples were freeze dried, weighed, extracted in 2:1 dichloromethane/methanol, and evaporated to dryness in pre-weighed scintillation vials. These crude extracts were separated on a Waters Alliance High Performance Liquid Chromatograph (HPLC) configured with a Photo Diode Array detector and a fraction collector. HPLC peaks, often representing pure compounds, were collected into pre-weighed vials to determine mass relative to the dry weight of the gorgonian. The three most abundant compounds were further characterized using Nuclear Magnetic Resonance (1H and 13C NMR) and High-Resolution Mass Spectrometry (HRMS) and compared to data from known compounds archived in MarinLit (Jones et al., 2020). These compounds were tested in a sponge cell-based allelopathy assay described in Slattery and Lesser (2014). Briefly, the sponge A. clathrodes (n = 4 replicates) was disassociated into cell suspensions through sterile gauze, filtered, layered with Percoll, and added to replicate Sedgwick Rafter counting chambers in the presence of the gorgonian crude extracts or pure compounds, with 3-([3-cholamidopropyl]-dimethylammonio) propanesulfonate (CHAPS) as a positive lysis control, and calcium-magnesium-free filtered seawater (CMFSW) as a negative control. The counting chambers were maintained at 25°C under a 12:12 L:D cycle for 48 h, after which the first 100 cells within the microscopic field of view were scored as intact or lysed. Cellular lysis mimics responses of allelopathic interactions in the field (Slattery and Lesser, 2014). Data were analyzed using a two-way ANOVA with depth and treatment (i.e., extract, compounds, or controls) as fixed factors. Water sampled near E. elongata was also assessed for allelochemicals using procedures described in Slattery et al. (1997). Briefly, the samples were partitioned across dichloromethane in 1 L separatory funnels, dried under vacuum, weighed, and quantified/identified using the analytical HPLC, NMR, and HRMS techniques described above. The concentrations of the water-borne compounds at four distances from the surface of the gorgonians were plotted, and differences in the slopes of each dilution curve were analyzed using linear regression.
Results
Gorgonian Densities at Mesophotic Depths
A total of 3,185 gorgonians were observed within 1,000 censused quadrats (Supplementary Table 1). Gorgonian abundance was not significantly different across the Caribbean Basin (ANOVA: F = 1.756, P = 0.1819; Bahamas: 1.3 ± 0.3 m–2; Grand Cayman: 1.9 ± 0.6 m–2; Little Cayman: 2.8 ± 0.5 m–2; Curaçao: 2.5 ± 0.5 m–2; Honduras: 4.0 ± 1.4 m–2) and averaged 2.5 ± 0.4 gorgonians m–2. However, there were significant differences in gorgonian abundances between the upper (3.8 ± 0.6 m–2) and lower (1.5 ± 0.2 m–2) mesophotic depths (t-test: t = 3.713, P = 0.0013). There were also site-specific significant differences between the dominant gorgonians on these reefs (A. bipinnata and E. elongata) at all depths in the upper mesophotic (Supplementary Table 1, t-tests: P < 0.05), but not in the lower mesophotic (Supplementary Table 1, t-tests: P > 0.05). When these gorgonians were merged into a Caribbean Basin location, there were significant differences between A. bipinnata and E. elongata in the upper and lower mesophotic zones (Supplementary Table 1, t-tests: P = 0.0211 and P < 0.0001, respectively). There were also greater sponge densities, and an increase in sponge species, in the presence of gorgonians relative to their absence (i.e., Bock Wall vs BA Line, respectively; Figure 2), and the interaction between site and depth was significant (Figure 2; 2-way ANOVAs: F = 5.593, P = 0.0223 and F = 21.339, P < 0.0001, respectively).
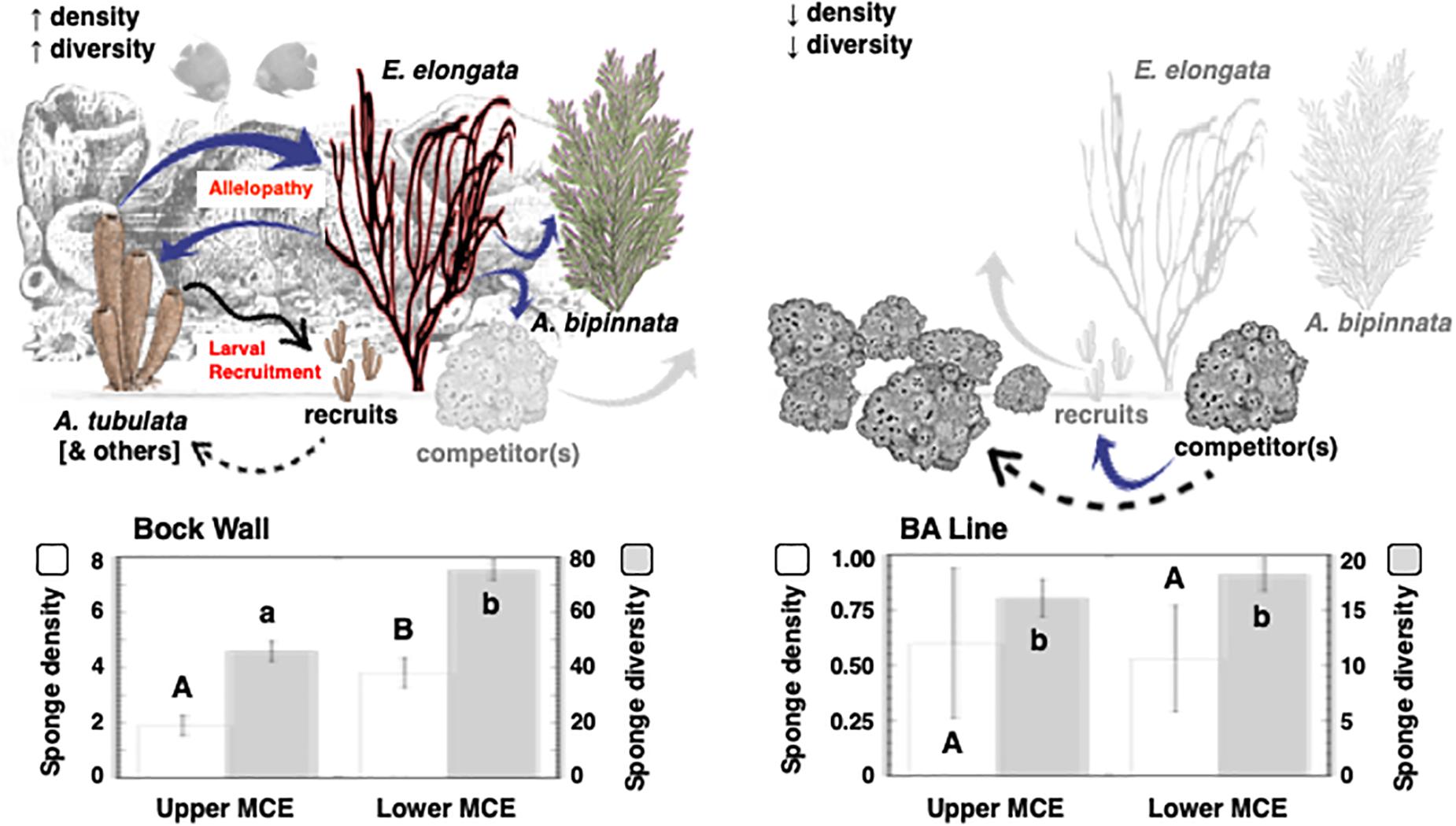
Figure 2. Sponge density and diversity in the presence and absence of gorgonians. Histograms represent replicate (n = 5–10) quadrats at random points along replicate 30 m transects (n = 3–9) at depths of 46 and 76 m at two sites in the Bahamas (Bock Wall and BA Line). Conceptual model includes blue arrows indicating likely paths of allelopathic activity, and black arrows indicating recruitment. An unidentified sponge competitor is possibly excluded from communities with the gorgonians A. bipinnata and E. elongata (gray arrows) but excludes most species in the absence of these gorgonians.
Gorgonian Facilitation of Invertebrate Recruitment
The number of recruits observed near the bases of live A. bipinnata and E. elongata during our field surveys were significantly higher than recruits onto bare substrate further away (Supplementary Table 2), and these results were particularly pronounced when data were scaled to the surface area available for recruitment (Figure 3A). At each of our locations within the Caribbean, the Depth × Distance × Treatment interaction was significant (three-way ANOVAs: P < 0.0001; Supplementary Tables 3–7). In general, diversity and richness were higher around A. bipinnata in the upper mesophotic, while diversity and richness were higher around E. elongata in the lower mesophotic (Figure 3B and Supplementary Tables 8, 9).
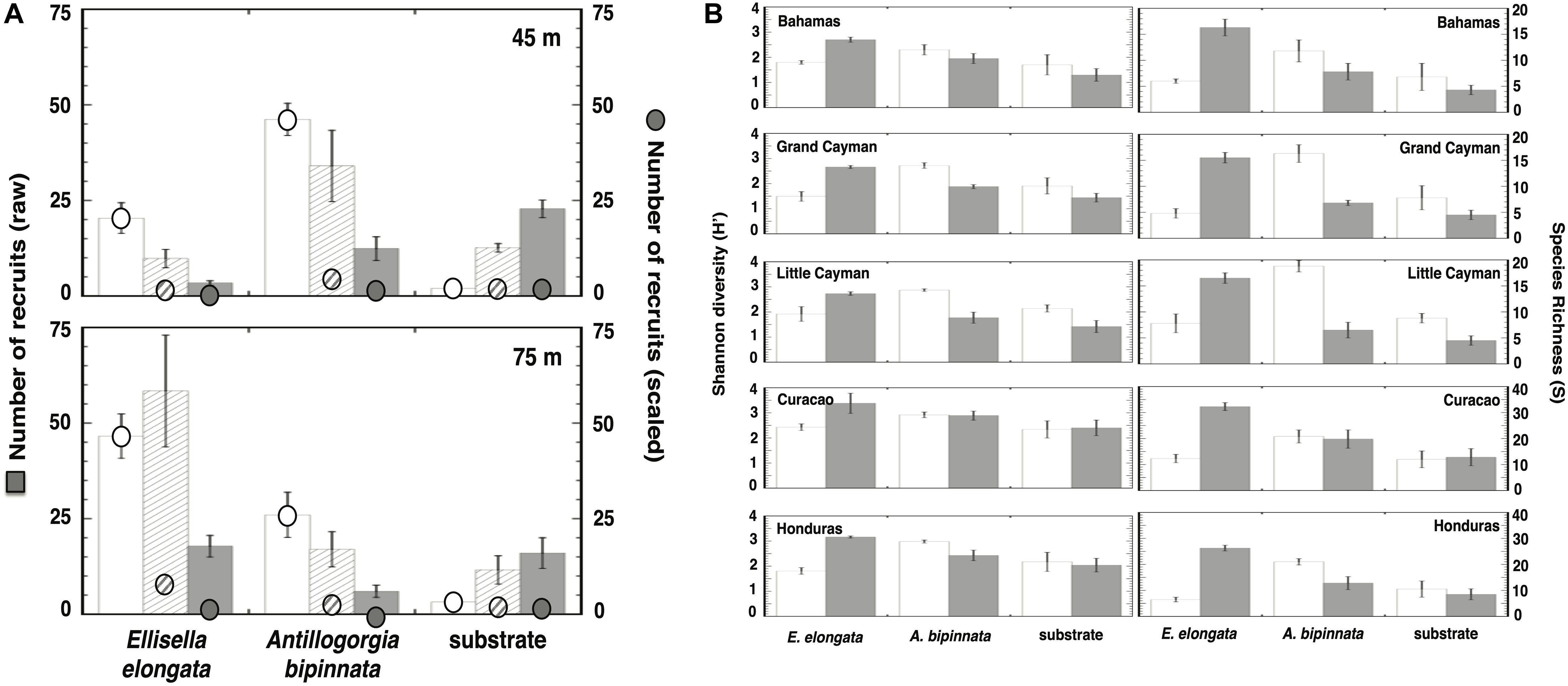
Figure 3. Recruitment in the presence or absence of gorgonians from field surveys throughout the Caribbean. Histograms represent the mean ± SE. (A) Recruit numbers per m2 (raw) and relative to area surrounding gorgonian (scaled: 400 cm2 [white bars], 3,200 cm2 [hatched bars], and 6,400 cm2 [gray bars]; see Figure 4B) at depths of 45 and 75 m. (B) Recruit diversity (H’) and richness (S) in the presence or absence of gorgonians from field surveys throughout the Caribbean (white bars = 45 m, gray bars = 75 m).
Invertebrate recruitment into cleared quadrats increased in the presence of live gorgonians (Figure 4A). Specifically, there were more recruits and greater diversity near living E. elongata than near dead gorgonian skeletons on upper and lower mesophotic reefs. In addition, these metrics declined with increasing distance from live gorgonians (Figure 4B), and the results were particularly pronounced when the data were scaled to the surface area available for recruitment (Figure 4A). There was a significant Depth × Distance × Treatment interaction (3-way ANOVA: P < 0.0001; Supplementary Table 10) that likely reflected differences in recruitment to the furthest plots relative to live vs dead skeleton treatments.
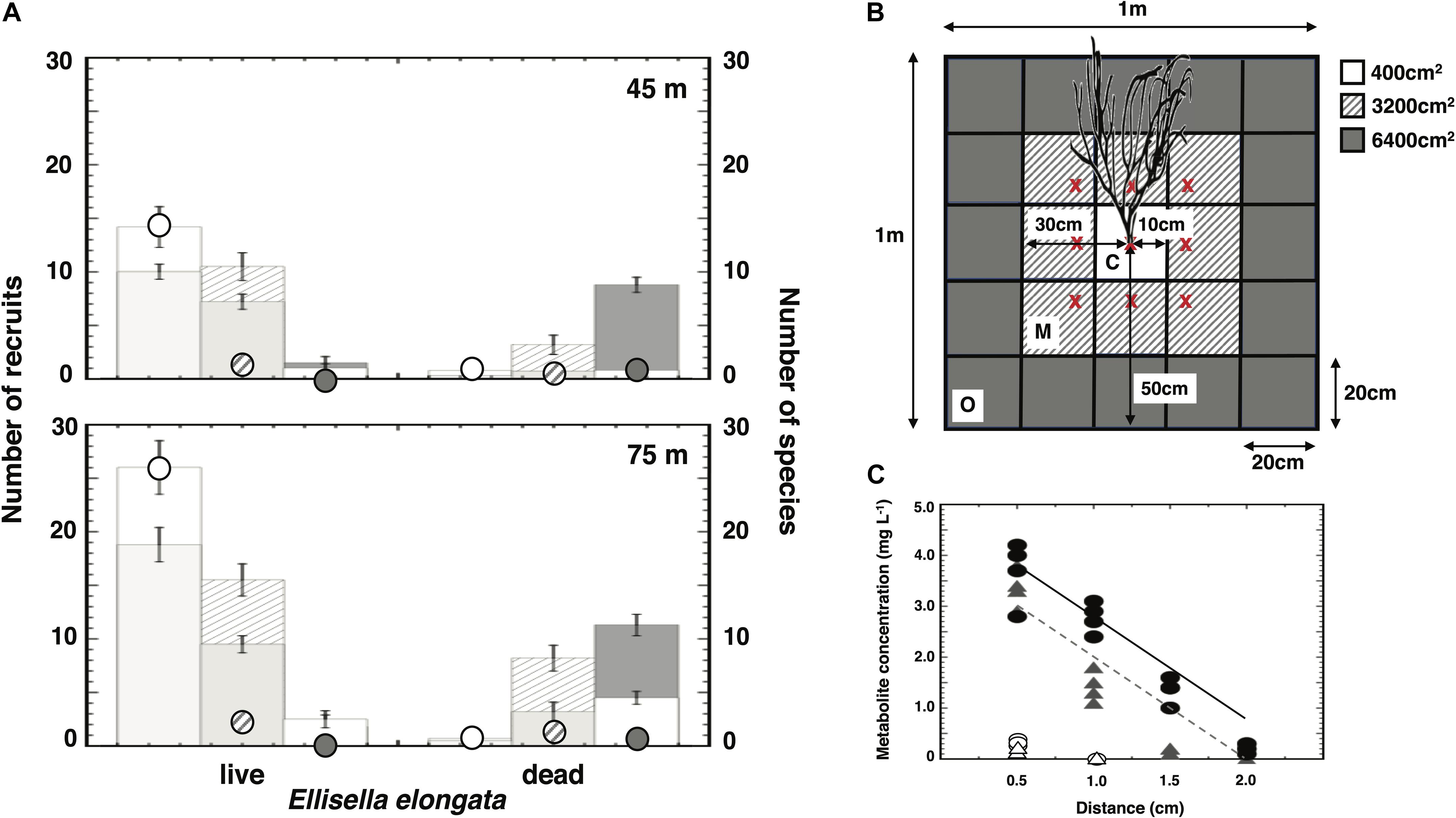
Figure 4. Recruitment in the presence of live or dead gorgonians. Histograms represent the mean ± SE. (A) Recruit numbers per m2 (raw: bars) and relative to area surrounding gorgonian (scaled: circles) at depths of 45 and 75 m. The number of species are represented by the shorter bars within each histogram (i.e., fewer species than number of recruits). (B) Schematic representing all 20 × 20 cm plots within 1 m2 relative to the central gorgonian (live or dead) (c = center: all recruits within 10 cm of gorgonian base; m = middle: all recruits within 10–30 cm of gorgonian base; o = outer: all recruits within 30–50 cm of gorgonian base). Note: x’s represent approximate locations of each gorgonian in the 3 × 3 PVC array. (C) Metabolite (7:3 mixture of junceelloside C: compound 2) concentration with increasing distance from surface of Ellisella elongata. Symbols represent the mean ± SE mg of mixture per liter of seawater extracted (gray triangles: 45 m, black circles: 75 m). Approximately six additional “compounds” were apparent on HPLC chromatograms at baseline concentrations (white triangles and circles) that could not be identified.
Gorgonian–Sponge Interactions
Contact interactions between gorgonians and sponges were relatively common across the mesophotic depth gradient and interactions between Antillogorgia bipinnata, E. elongata, M. laxa, or N. schmitti, and eight sponges were further examined (749 of 3,185 gorgonians observed: Table 1). Sponges were typically the competitive dominant in gorgonian-sponge interactions, with 20 wins vs 6 wins for gorgonians, in all interactions that resulted in an obvious outcome of tissue necrosis (85% of interactions) or overgrowth (30% of interactions). All gorgonian wins resulted in sponge tissue necrosis, and many interactions (n = 20) resulted in a draw. Three of the four gorgonians examined (i.e., all but M. laxa) exhibited some degree of competitive dominance over at least one sponge species (Table 1), and only the sponge Verongula rigida was a competitive dominant to all four gorgonians. In 15 of the 64 gorgonian-sponge interactions, there was at least one example of a competitive reversal (i.e., a winning individual within the population of a losing taxa). Finally, 14 of 32 gorgonian-sponge interactions included paired upper and lower mesophotic data; in 10 of those cases competition was significantly greater within the lower mesophotic samples (unpaired t-tests: P < 0.05).
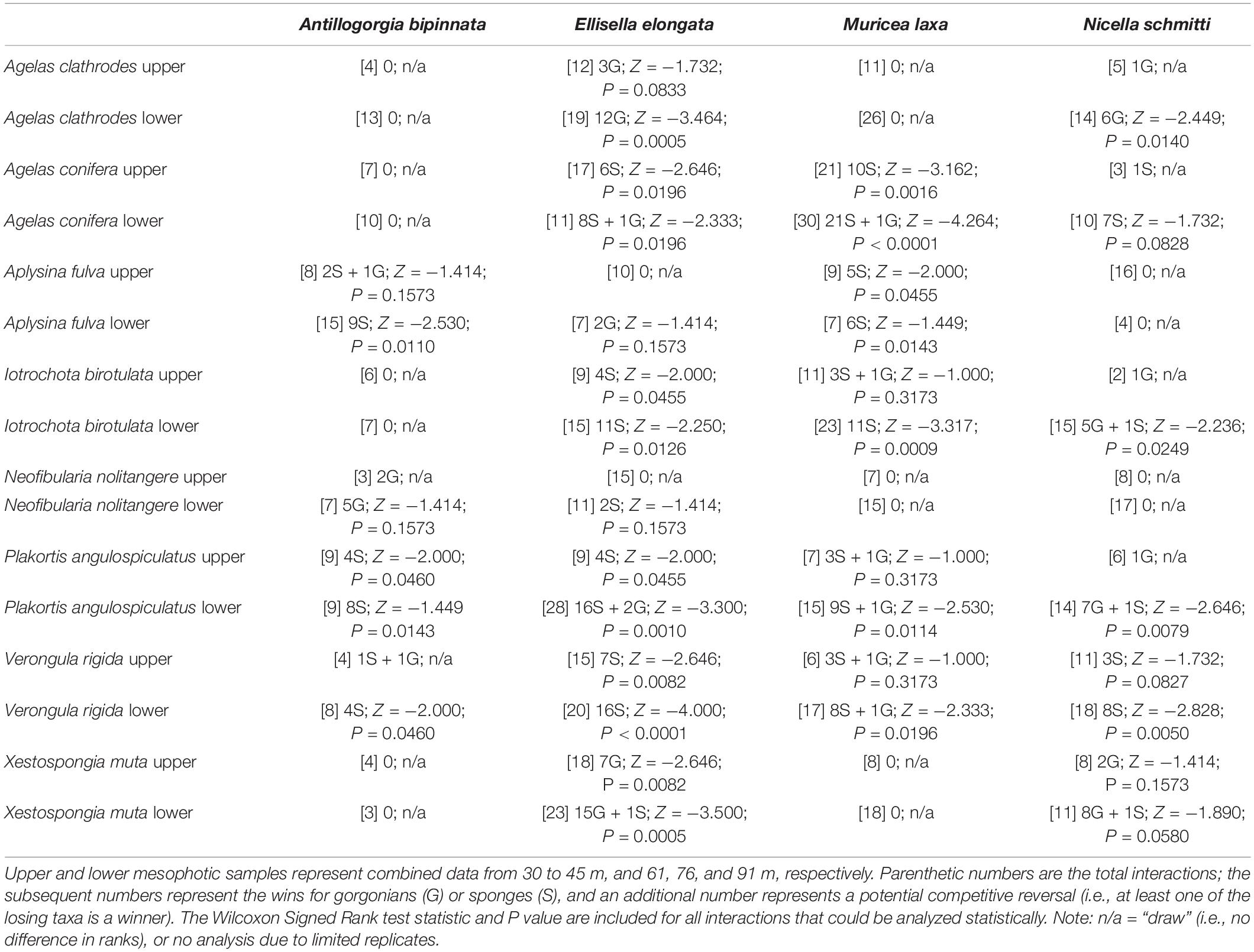
Table 1. Contact interactions between gorgonians (top row) and sponges (left column) from five sites in the Caribbean Basin.
Ellisella elongata Competition With Sponges and Other Gorgonians
The contact interactions between transplanted E. elongata and the sponges, A. clathrodes, P. angulospiculatus, and X. muta, exhibited species-specific outcomes. Specifically, E. elongata was the winner when in contact with A. clathrodes, and this outcome was enhanced with increasing time and depth (Figure 5A-A; Friedman’s Test: tie-corrected Chi Square = 24.111, P < 0.0001). In contrast, P. angulospiculatus was the winner when in contact with E. elongata, and this outcome was also enhanced with increasing time and depth (Figure 5A-B; Friedman’s Test: tie-corrected Chi Square = 10.330, P = 0.0057). However, the interaction between E. elongata and X. muta was a draw (Figure 5A-C; Friedman’s Test: tie-corrected Chi Square = 1.059, P = 0.5890). Although competitive interactions increased in the lower mesophotic for both E. elongata (Figure 5A-B) and P. angulospiculatus (Figure 5A-C), the negative effects on the sponge declined over 12 mo, while effects on the gorgonian did not. Nonetheless, the gorgonian was unable to exert a competitive reversal in this time period. Gorgonians placed in contact with conspecifics for 3 mo exhibited minimal tissue necrosis (= 1 out of 15 pairings between A. bipinnata, and 0 out of 15 pairings between E. elongata, Chi Square: P > 0.05), while heterospecific contact pairings exhibited significant tissue necrosis in A. bipinnata (= 12 out of 15 pairings, Chi Square = 28.774, P < 0.0001) but no impact to the E. elongata colonies.
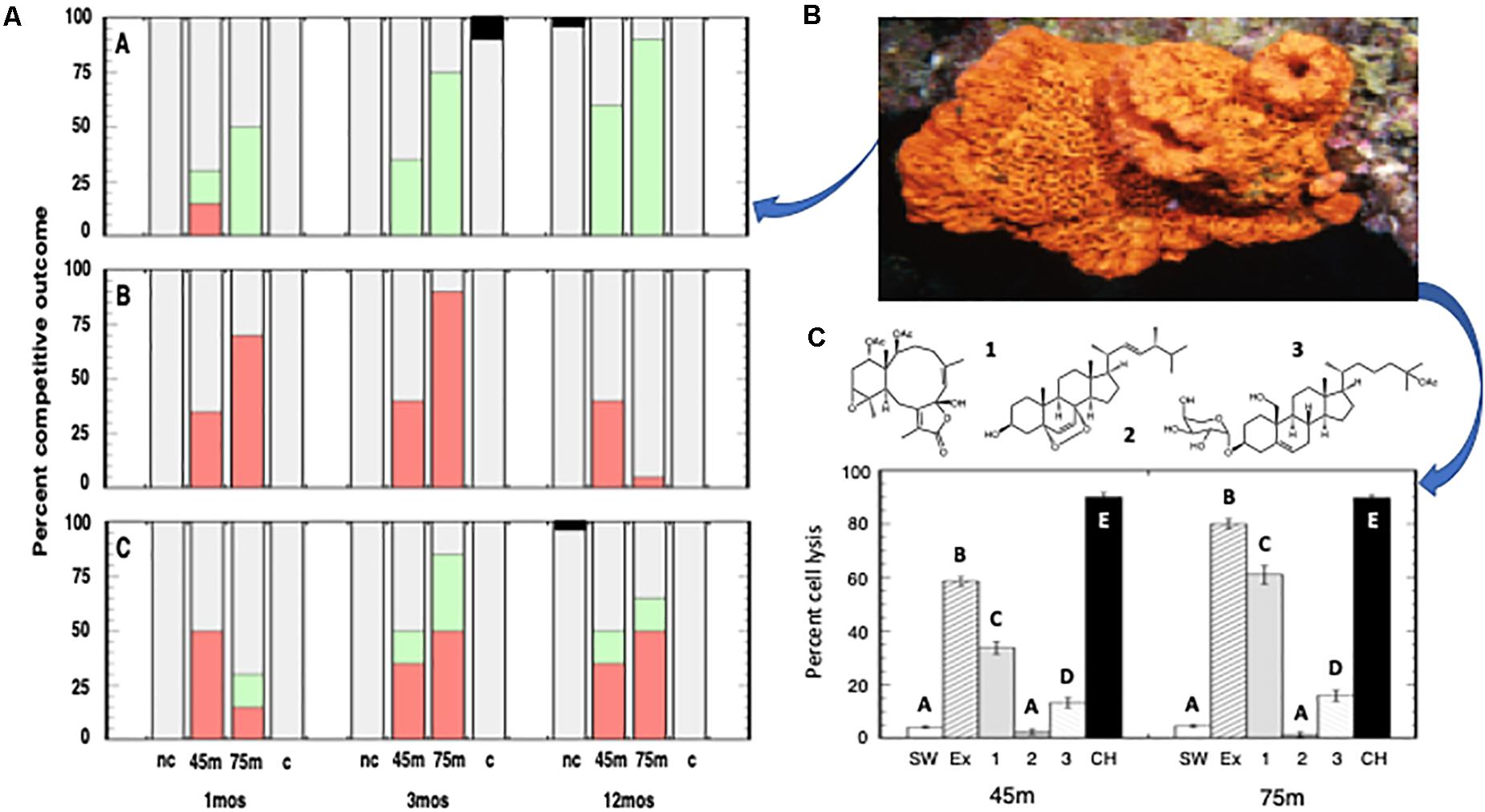
Figure 5. (A) Field contact interactions between the gorgonian Ellisella elongata, and three sponge species: (A) Agelas cathrodes, (B) Plakortis angulospiculatus, and (C) Xestospongia muta. Competitive outcomes, assessed at 1 mo, 3 mo, and 12 mo, included overgrowth, tissue necrosis, and/or mortality of either the gorgonian or the sponge (red: sponge wins; green: gorgonian wins; grey: draw; black: undetermined cause of mortality in a control). Histograms represent replicate (n = 12) interactions and include gorgonians from 45 m in contact with sponges, gorgonians from 75 m in contact with sponges, contact controls (“c”: dead gorgonian in contact with sponges), and non-contact controls (“nc”: sponges alone). (B) Agelas clathrodes, a common sponge of MCEs and a model for our field and laboratory assays. (C) Allelopathic responses of the sponge Agelas clathrodes to the gorgonian Ellisella elongata from 45 m and 75 m. Histograms represent the mean ± SE percent sponge cells lysed (n = 4 replicates) in response to either controls (SW: calcium-/magnesium-free filtered seawater; CH: CHAPS) or Ellisella elongata extract (EX) and three E. elongata compounds at ecologically relevant concentrations. Similar superscripts represent treatment groups not significantly different from each other (Tukey’s HSD: P > 0.05).
Extracts and pure compounds from E. elongata exhibited allelopathic activity against cells of A. clathrodes (Figure 5B) that were highly significant (ANOVA: F = 832.871, P < 0.0001). The crude extracts from 45 to 75 m resulted in 58.5 ± 1.9 and 80.0 ± 2.0 percent cell lysis, respectively, and included three pure compounds that occurred at 2.9–7.6% of the extract mass: 1) funicolide E (Guerriero et al., 1995), 2) (22E,24S)-5a,8a-epidioxy-24-methyl-cholesta-6,22-dien-3b-ol (Ioannou et al., 2009), and 3) junceelloside C (Jiang et al., 2013) (Figure 5C). Post hoc multiple comparison testing revealed that funicolide E and junceelloside C caused significant sponge cell lysis compared to the seawater controls (Tukey’s HSD: P < 0.05), while compound 2 had no effect on sponge cells (Tukey’s HSD: P > 0.05, Figure 5C). Moreover, these two compounds represented most of the allelopathic effect of the gorgonian crude extract. While the metabolomic profiles of the gorgonians collected at 45 and 75 m were qualitatively identical, there was a significant, and independent, increase in the concentrations of funicolide E (3.6 to 6.4%) with increasing depth that resulted in a significant increase in allelopathic activity (ANOVA: F = 66.502, P < 0.0001). Sponge cell lysis almost doubled (33.8 ± 2.3 vs. 61.0 ± 3.5%) in samples from 75 m compared to samples from 45 m (Figure 5C). The positive lysis control, CHAPS, caused about 90% cell lysis in A. clathrodes consistent with its measured bioactivity (Slattery and Lesser, 2014). There were no interactions between treatment and depth for the allelopathic assays (ANOVA: P > 0.05).
A 7:3 mixture of junceelloside C and compound 2 was isolated from seawater collected at distances of 0.5 to 2.0 cm from the surface of E. elongata (Figure 4C and Supplementary Table 11). The concentration of this mixture in seawater exhibited a significant decline with distance from the gorgonian (2-way ANCOVA: P < 0.0001), as well as a significant increase with increasing depth (i.e., 45–75 m; 2-way ANCOVA: P < 0.0001). At least six additional HPLC peaks, likely representing specific gorgonian compounds, were noted on the HPLC chromatograms, but the baseline concentrations (Figure 4C) could not be further purified for MS characterization.
Discussion
Gorgonians Are Primary Foundation Species of MCEs
Gorgonian abundance and diversity on Caribbean MCEs are lower than nearby shallow coral reefs, in contrast to the Red Sea where these metrics are higher at mesophotic depths (Shoham and Benayahu, 2017). Sponge abundance is similarly high at MCE depths in the Red Sea and eastern Mediterranean (Idan et al., 2018; Eyal et al., 2019), although their morphology is encrusting like most sponges on Indo-Pacific MCEs (Slattery and Lesser, 2012). The lower gorgonian abundance in the Caribbean may be due to competitive interactions with the space-dominant sponge fauna (Lesser and Slattery, 2018; Ladd et al., 2019), potentially mediated by sponge allelochemicals (Thacker et al., 1998; Slattery and Gochfeld, 2012). Nonetheless, gorgonians span the upper to lower mesophotic zones at densities that are second only to those of sponges (Pomponi et al., 2019; Sánchez et al., 2019). Given the abundance and biomass of sponges on Caribbean mesophotic reefs (Slattery and Lesser, 2012; Lesser and Slattery, 2018), it is not surprising that contact interactions with gorgonians are common. McLean and Yoshioka (2007) noted numerous contact interactions between gorgonians and sponges on the shallow reefs of Puerto Rico, with most interactions resulting in gorgonian mortality. The majority of the interactions documented here on Caribbean MCEs (= 70%) resulted in sub-lethal effects to gorgonians (i.e., limited tissue necrosis and/or overgrowth). Sponges are competitive dominants on coral reefs worldwide (Slattery and Gochfeld, 2012; Wulff, 2012) where they often out-compete corals (Porter and Targett, 1988; Aerts, 1998) and other sponges (Engel and Pawlik, 2000; Wulff, 2005), but not algae (Easson et al., 2014; Slattery and Lesser, 2014). However, competitive reversals by gorgonians were observed in many pairings during this study where sponges were the initial winner. Therefore, gorgonians can coexist with sponges in the MCE sponge belt, as they do in some shallow reef communities (Gili and Coma, 1998; Cúrdia et al., 2013).
While gorgonians do not exhibit the density and biomass of sponges on lower MCEs (Slattery and Lesser, 2012), the most common species on Caribbean MCEs (i.e., A. bipinnata and E. elongata) play a critical role in the facilitation of benthic invertebrate recruitment. An established MCE community, that lacked these gorgonians (= BA Line) exhibited 3–5 times lower sponge densities and diversity relative to a nearby site with these gorgonians (= Bock Wall). It is possible that site-specific abiotic factors (e.g., sedimentation on BA Line: Liddell et al., 1997) are partially responsible for differences in recruitment. However, our survey data of recruits near these gorgonians, at five locations throughout the Caribbean Basin, validated this observation of gorgonians as facilitators of recruitment which was higher near gorgonians than on bare substrate. Gorgonian forests in the Mediterranean and the USVI have also been shown to enhance recruitment of algae and invertebrates (Ponti et al., 2014, 2018; Privitera-Johnson et al., 2015), probably due to hydrodynamic processes and/or entrainment of larvae within the arborescent landscape (Gili and Coma, 1998). However, we observed higher recruitment into plots containing live E. elongata, compared to plots containing dead gorgonian skeletons, indicating that hydrodynamics was not solely responsible for the recruitment patterns we documented. Chemical settlement cues and/or substrate characteristics might also select for the success of recruits (Smith and Witman, 1999; Whalan et al., 2012). Alternatively, the presence of algae in a few of the plots might have inhibited recruitment to control plots via allelopathic mechanisms (Slattery and Lesser, 2014). In contrast to our data from the Caribbean, the presence of gorgonians within the Mediterranean had little effect on the percent cover of recruits, but they did select against certain taxa, and thereby lowered biodiversity, possibly due to size-selective feeding on larvae (Ponti et al., 2014).
Our survey data also demonstrated depth-specific differences in recruitment, with more recruits near A. bipinnata at 45 m than at 75 m, and more recruits near E. elongata at 75 m than at 45 m. This may be due to the higher population densities of A. bipinnata and E. elongata at 45 and 75 m, respectively. Yoshioka (1996) reported density-dependent recruitment of 14 gorgonian species into conspecific adult forests in Puerto Rico, implying species-specific “aggregative” settlement cues might be important in recruitment patterns (Pawlik, 1992). While similar trends were observed in 10 genera of gorgonians in St. John USVI, Antillogorgia spp. did not exhibit a positive relationship between recruits and adults (Privitera-Johnson et al., 2015) suggesting A. bipinnata may not respond to conspecific settlement cues. Instead, larval settlement patterns might be supported by characteristics of the gorgonian mucus and/or their microbial biofilms (Slattery, 1992; Webster et al., 2004; Whalan and Webster, 2014). Alternatively, depth-specific differences may be a function of species richness and diversity (i.e., S and H’, respectively) across the broader Caribbean Basin (sensu Witman et al., 2004). However, it is important to note that abiotic factors such as reef slope angle between the upper and lower mesophotic zones, with its influence on irradiances, water flow, and nutrients (Lesser et al., 2018), also varies at our sites.
Taken together, A. bipinnata and E. elongata facilitate the success of multiple species within MCEs by regulating recruitment processes consistent with the definition of a FS (Dayton, 1972). Moreover, these gorgonian species exhibit inter-taxa competitive interactions resulting in physical segregation and varying dominance hierarchies, throughout the upper and lower mesophotic zones, that are consistent with adjacent FS assemblages (Angelini et al., 2011). Therefore, it is likely that these gorgonians, as primary FS, structure their respective MCE zones to allow additional obligate FS (e.g., sponges) to be successful (Altieri et al., 2007; Sousa et al., 2007).
Ellisella elongata Structures MCEs With Allelopathic Compounds
Given that gorgonians and sponges are both suspension feeders one might assume that would result in a network of resource competitors, but these species often co-occur without evidence of competitive feeding interactions (Gili and Coma, 1998). In fact, gorgonians are passive suspension feeders that consume relatively large plankton (∼4–700 μm in size: e.g., Ribes et al., 1998, 1999), whereas sponges are active suspension feeders that primarily consume the abundantly available bacterioplankton (<1 μm in size: e.g., Lesser, 2006; Hadas et al., 2009; Lesser and Slattery, 2013), resulting in a significant increase in sponge growth, biomass, and density at mesophotic depths (Slattery and Lesser, 2012; Lesser and Slattery, 2018). Thus, food limitation and/or niche overlap seems unlikely to provide any pressure for competition between gorgonians and sponges on MCEs within the Caribbean Basin. Instead, it is possible that the space itself, and the access it provides to flow-mediated trophic subsidies (Vogel, 1977; Sebens, 1984), might be the cause of the observed competitive outcomes (i.e., tissue necrosis and/or overgrowth). In contrast, habitat-partitioning between A. bipinnata and E. elongata (upper and lower mesophotic zones, respectively) may be due, in part, to differences in their light requirements (e.g., Dattolo et al., 2017). Specifically, the former species hosts photosymbionts, while the latter species is photosymbiont free (Sánchez et al., 2019). However, it is also clear that E. elongata is competitively dominant over A. bipinnata which might provide the ecological pressure that constrains the latter species to the upper mesophotic zone.
Tissue necrosis often involves the production of allelochemicals that are active through surficial contact and/or water-borne routes of transmission (Granéli and Pavia, 2006), and both gorgonians and sponges produce a diverse array of compounds with known or presumed allelopathic roles (Slattery and Gochfeld, 2012). Tissue necrosis was the most common result of contact interactions for both gorgonians and sponges, suggesting that allelopathy is an important competitive mechanism at mesophotic depths (e.g., Slattery and Lesser, 2014). Although water-borne allelopathic affects are less common, due to dilution of compounds within the surrounding seawater (Slattery et al., 1997), we did note a few isolated sponges with evidence of tissue necrosis. This necrosis may have been due to prior contact during current-mediated abrasion (Box and Mumby, 2007), “retreat” from contact with a dominant species (Hoeksema and De Voogd, 2012), and/or to a true water-borne allelochemical (de Nys et al., 1991). In contrast, Ladd et al. (2019) noted that the gorgonian Erythropodium caribaeorum was the most aggressive species on the shallow reefs of Florida, but its competitive success was due to overgrowth rather than allelopathic interactions. That said, most gorgonians have a very small “footprint” relative to the substrate, and horizontal overgrowth is a limited competitive strategy for these species.
We observed competitive dominance by the gorgonian, E. elongata, over the sponge, A. clathrodes, in field surveys and contact transplants, as well as in a laboratory experiment. Our results indicate that the additive effects of two compounds, funicolide E and junceelloside C, are responsible for 80–96% of the activity of the gorgonian extract. Funicolide E is briarene diterpenoid, a class of compound that is broadly distributed geographically and taxonomically (Guerriero et al., 1995), and Junceelloside C is a steroid commonly associated with gorgonians from the family Ellisellidae throughout their geographic range (Sun et al., 2010). This study is the first report of ecological activity for either compound, although certain briarenes exhibit antifouling activity against barnacles (Qi et al., 2006) and apparently have some feeding deterrent activity (Harvell et al., 1993), and steroids can deter predators (Slattery et al., 1997). The presence of two allelopathic compounds in E. elongata is not surprising given the potential importance of competition on MCEs, and this may represent a form of “bet-hedging” against different species and/or species-specific acclimatization to individual compounds (Rasher et al., 2011). It is interesting that lower MCE gorgonians exhibited higher concentrations of funicolide E than their shallow MCE counterparts which resulted in greater allelopathic activity of the lower MCE gorgonians at depths where sponge abundance and biomass were significantly greater (Slattery and Lesser, 2012, 2019; Lesser and Slattery, 2018). Depth-dependent variation may indicate that funicolide E represents an inducible defense (Karban and Baldwin, 1997) to increasing competition with sponges at lower MCE depths (sensu Anstett et al., 2016).
Despite the presence of a potential inducible chemical defense, the gorgonian E. elongata actively facilitated the recruitment of a diverse benthic community to nearby substrata, including many species of sponges, scleractinian corals, gorgonians, and a tunicate. In the Mediterranean garrigue scrub community, a monoterpene produced by the herbaceous shrub Thymus vulgaris repressed the abundance of a space-dominant competitive grass, allowing other rarer species to persist (Ehlers et al., 2014). It is possible that the gorgonian allelochemicals we isolated were selected for their allelopathic effects on a particular space-dominant sponge species (e.g., A. clathrodes), and a result of that competitive success was the recruitment of less competitive species into the benthos. These results are consistent with associational defense systems (e.g., algae: Hay, 1986; soft corals: Kerr and Paul, 1995; Ribeiro et al., 2017; sponges: Farren and Donovan, 2007) often attributable to FS (Stachowicz, 2001). Alternatively, these gorgonians may make the substrate more suitable for recruits (sponges, hard and soft corals, and/or tunicates), akin to the role of the Mesquite in semi-arid savannas of the southwest United States (Archer et al., 1988). For example, gorgonians regularly slough mucus and mucus-associated bacteria (Rublee et al., 1980) that might induce larval settlement of multiple invertebrate taxa (e.g., Pawlik, 1992; Slattery, 1992). It is also possible that greater stress occurs on upper MCE reefs from disease or storm activity (e.g., Woodley et al., 1981; Smith et al., 2010, 2016; Frade et al., 2018), compared to the reduced stress on lower MCE reefs (Slattery et al., 2011), and this provides the selective environment for facilitation to override the ecological costs of competition (i.e., the Stress Gradient Hypothesis: Bertness and Callaway, 1994).
In conclusion, common gorgonians of the Caribbean Basin structure the upper and lower mesophotic zones through counterbalanced positive (i.e., facilitation) and negative (i.e., competition) interactions. Although gorgonians are less common than the space dominant MCE sponges on Caribbean reefs, A. bipinnata and E. elongata are FS that play critical roles in the recruitment and allelochemical-mediated survival (= associational defense) of diverse benthic communities in the adjacent upper and lower MCE zones (Lesser et al., 2019).
Data Availability Statement
The original contributions presented in the study are included in the article/Supplementary Material, further inquiries can be directed to the corresponding author/s.
Author Contributions
MS and ML designed the study, conducted the field studies, conducted the statistical analyses, and contributed to revisions. MS conducted the chemical analyses and wrote the first draft. Both authors contributed to the article and approved the submitted version.
Funding
This project was funded by grants from NOAA’s National Institute for Undersea Science and Technology, NOAA’s Ocean Exploration and Research, and the National Science Foundation Biological Oceanography program (OCE–1632333/1632348) to MS and ML, respectively.
Conflict of Interest
The authors declare that the research was conducted in the absence of any commercial or financial relationships that could be construed as a potential conflict of interest.
Acknowledgments
We thank S. Ankisetty, C. M. Diaz, C. G. Easson, C. Fiore, D. J. Gochfeld, H. Kamel, E. Kintzing, L. R. Krentz, K. J. Macartney, and M. S. Pankey for field and laboratory assistance. D. G. Nagle provided assistance with compound characterization, and D. J. Gochfeld assisted with the statistical analysis. The staffs of CMRC, LCRC, CARMABI, InDepth Watersports, and Utila Lodge provided logistical support. All experiments conducted for this study complied with laws and permits of the Bahamas (Permit Number: MAF/FIS/1,12,46,79), the Cayman Islands (Permit by the National Conservation Council), Curacao (Permit Number: 2012/48584), Honduras (Permit Number: ICF-124-12), and the United States.
Supplementary Material
The Supplementary Material for this article can be found online at: https://www.frontiersin.org/articles/10.3389/fmars.2021.654268/full#supplementary-material
References
Aerts, L. A. M. (1998). Sponge/coral interactions in Caribbean reefs: analysis of overgrowth patterns in relation to species identity and cover. Mar. Ecol. Prog. Ser. 175, 241–249. doi: 10.3354/meps175241
Altieri, A. H., and Witman, J. D. (2006). Local extinction of a foundation species in a hypoxic estuary: integrating individuals to ecosystem. Ecology 87, 717–730. doi: 10.1890/05-0226
Altieri, A. H., Silliman, B. R., and Bertness, M. D. (2007). Hierarchical organization via a facilitation cascade in intertidal cordgrass bed communities. Am. Nat. 169, 195–206. doi: 10.2307/4125317
Angelini, C., Altieri, A. H., Silliman, B. R., and Bertness, M. D. (2011). Interactions among foundation species and their consequences for community organization, biodiversity, and conservation. BioScience 61, 782–789. doi: 10.1525/bio.2011.61.10.8
Anstett, D. N., Chen, W., and Johnson, M. T. J. (2016). Latitudinal gradients in induced and constitutive resistance against herbivores. J. Chem. Ecol. 42, 772–281. doi: 10.1007/s10886-016-0735-6
Archer, S., Scifres, C., Bassham, C. R., and Maggio, R. (1988). Autogenic succession in a subtropical savanna: conversion of grassland to thorn woodland. Ecol. Monogr. 58, 111–127. doi: 10.2307/1942463
Bertness, M. D., and Callaway, R. (1994). Positive interactions in communities. Trends Ecol. Evol. 9, 191–193.
Bongaerts, P., Ridgway, T., Sampayo, E. M., and Hoegh-Guldberg, O. (2010). Assessing the “deep reef refugia” hypothesis: focus on Caribbean reefs. Coral Reefs 29, 309–327. doi: 10.1007/s00338-009-0581-x
Bongaerts, P., Riginos, C., Brunner, R., Englebert, N., Smith, S. R., and Hoegh-Guldberg, O. (2017). Deep reefs are not universal refuges: reseeding potential varies among coral species. Sci. Adv. 3:e1602373. doi: 10.1126/sciadv.1602373
Box, S. J., and Mumby, P. J. (2007). Effect of macroalgal competition on growth and survival of juvenile Caribbean corals. Mar. Ecol. Prog. Ser. 342, 139–149. doi: 10.3354/meps342139
Castro, C. B., Medeiros, M. S., and Loiola, L. L. (2010). Octocorallia (Cnidaria: Anthozoa) from Brazilian reefs. J. Nat. Hist. 44, 763–827. doi: 10.1080/00222930903441160
Chadwick, N. E., and Morrow, K. M. (2011). “Competition among sessile organisms on coral reefs,” in Coral Reefs: An Ecosystem in Transition, eds Z. Dubinsky and N. Stambler (New York, NY: Springer).
Cúrdia, J., Monteiro, P., Afonso, C. M., Santos, M. N., Cunha, M. R., and Gonçalves, J. M. (2013). Spatial and depth-associated distribution patterns of shallow gorgonians in the Algarve coast (Portugal, NE Atlantic). Helgoland Mar. Res. 67, 521–534. doi: 10.1007/s10152-012-0340-1
Dattolo, E., Marín−Guirao, L., Ruiz, J. M., and Procaccini, G. (2017). Long−term acclimation to reciprocal light conditions suggests depth−related selection in the marine foundation species Posidonia oceanica. Ecol. Evol. 7, 1148–1164. doi: 10.1002/ece3.2731
Dayton, P. K. (1972). “Toward an understanding of community resilience and the potential effects of enrichments to the benthos at McMurdo Sound, Antarctica,” in Proceedings of the Colloquium on Conservation Problems in Antarctica, ed. B. C. Parker (Lawrence, KS: Allen Press).
de Nys, R., Coll, J. C., and Price, I. R. (1991). Chemically mediated interactions between the red alga Plocamium hamatum (Rhodophyta) and the octocoral Sinularia cruciata (Alcyonacea). Mar. Biol. 108, 315–320. doi: 10.1007/bf01344346
Easson, C. G., Slattery, M., Baker, D. M., and Gochfeld, D. J. (2014). Complex ecological associations: competition and facilitation in a sponge–algal interaction. Mar. Ecol. Prog. Ser. 507, 153–167. doi: 10.3354/meps10852
Ehlers, B. K., Charpentier, A., and Grøndahl, E. (2014). An allelopathic plant facilitates species richness in the Mediterranean garrigue. J. Ecol. 102, 176–185. doi: 10.1111/1365-2745.12171
Ellison, A. M., Farnsworth, E. J., and Twilley, R. R. (1996). Facultative mutualism between red mangroves and root−fouling sponges in Belizean mangal. Ecology 77, 2431–2444. doi: 10.2307/2265744
Engel, S., and Pawlik, J. R. (2000). Allelopathic activities of sponge extracts. Mar. Ecol. Prog. Ser. 207, 273–281. doi: 10.3354/meps207273
Eyal, G., Tamir, R., Kramer, N., Eyal-Shaham, L., and Loya, Y. (2019). “The Red Sea: Israel,” in Mesophotic Coral Ecosystems, eds Y. Loya, K. A. Puglise, and T. C. L. Bridge (Cham: Springer). doi: 10.1560/hyc7-tuth-ev77-beuq
Farren, H. M., and Donovan, D. A. (2007). Effects of sponge and barnacle encrustation on survival of the scallop Chlamys hastata. Hydrobiologia 592, 225–234. doi: 10.1007/s10750-007-0743-1
Fiore, C. L., Baker, D. M., and Lesser, M. P. (2013). Nitrogen biogeochemistry in the Caribbean sponge, Xestospongia muta: a source or sink of dissolved inorganic nitrogen? PLoS One 8:e72961. doi: 10.1371/journal.pone.0072961
Frade, P. R., Bongaerts, P., Englebert, N., Rogers, A., Gonzalez-Rivero, M., and Hoegh-Guldberg, O. (2018). Deep reefs of the Great Barrier Reef offer limited thermal refuge during mass coral bleaching. Nat. Comm. 9:3447.
Gambrel, B., and Lasker, H. R. (2016). Interactions in the canopy among Caribbean reef octocorals. Mar. Ecol. Prog. Ser. 546, 85–95. doi: 10.3354/meps11670
Gili, J. M., and Coma, R. (1998). Benthic suspension feeders: their paramount role in littoral marine food webs. Trends Ecol. Evol. 13, 316–321. doi: 10.1016/s0169-5347(98)01365-2
Granéli, E., and Pavia, H. (2006). “Allelopathy in marine ecosystems,” in Allelopathy: A Physiological Process with Ecological Implications, eds M. J. Reigosa, N. Pedrol, and L. Gonzalez (Dordrecht: Springer).
Gribben, P. E., Byers, J. E., Clements, M., McKenzie, L. A., Steinberg, P. D., and Wright, J. T. (2009). Behavioural interactions between ecosystem engineers control community species richness. Ecol. Letts. 12, 1127–1136. doi: 10.1111/j.1461-0248.2009.01366.x
Guerriero, A., D’Ambrosio, M., and Pietra, F. (1995). Bis−allylic reactivity of the funicolides, 5, 8 (17)−diunsaturated briarane diterpenes of the sea pen Funiculina quadrangularis from the Tuscan archipelago, leading to 16−nortaxane derivatives. Helv. Chim. Acta 78, 1465–1478. doi: 10.1002/hlca.19950780606
Hadas, E., Shpigel, M., and Ilan, M. (2009). Particulate organic matter as a food source for a coral reef sponge. J. Exp. Biol. 212, 3643–3650. doi: 10.1242/jeb.027953
Harvell, C. D., Fenical, W., Roussis, V., Ruesink, J. L., Griggs, C. C., and Greene, C. H. (1993). Local and geographic variation in the defensive chemistry of a West Indian gorgonian coral (Briareum asbestinum). Mar. Ecol. Prog. Ser. 93, 165–173. doi: 10.3354/meps093165
Hay, M. E. (1986). Associational plant defenses and the maintenance of species diversity: turning competitors into accomplices. Am. Nat. 128, 617–641. doi: 10.1086/284593
Hay, M. E., and Rasher, D. B. (2010). Coral reefs in crisis: reversing the biotic death spiral. F1000 Biol. Rep. 2:71.
Hoeksema, B. W., and De Voogd, N. J. (2012). On the run: free-living mushroom corals avoiding interaction with sponges. Coral Reefs 31, 455–459. doi: 10.1007/s00338-011-0856-x
Hughes, T. P., Barnes, M. L., Bellwood, D. R., Cinner, J. E., Cumming, G. S., Jackson, J. B., et al. (2017). Coral reefs in the Anthropocene. Nature 546, 82–90.
Idan, T., Shefer, S., Feldstein, T., Yahel, R., Huchon, D., and Ilan, M. (2018). Shedding light on an East-Mediterranean mesophotic sponge ground community and the regional sponge fauna. Medit. Mar. Sci. 19, 84–106. doi: 10.12681/mms.13853
Ioannou, E., Abdel-Razik, A. F., Zervou, M., Christofidis, D., Alexi, X., Vagias, C., et al. (2009). 5α, 8α-Epidioxysterols from the gorgonian Eunicella cavolini and the ascidian Trididemnum inarmatum: Isolation and evaluation of their antiproliferative activity. Steroids 74, 73–80. doi: 10.1016/j.steroids.2008.09.007
Jiang, M., Sun, P., Tang, H., Lui, B. S., Li, T. J., Li, C., et al. (2013). Steroids glycosylated with both D-and L-arabinoses from the South China sea gorgonian Dichotella gemmacea. J. Nat. Prod. 76, 764–768. doi: 10.1021/np300906b
Jones, G., Potter, H., and Robinson, A. (2020). MarinLit, a database of marine natural products literature. London: Royal Society of Chemistry.
Karban, R., and Baldwin, I. T. (1997). Induced Responses to Herbivory. Chicago, IL: University of Chicago Press.
Kerr, J. N. Q., and Paul, V. J. (1995). Animal-plant defense association: the soft coral Sinularia sp. (Cnidaria, Alcyonacea) protects Halimeda spp. from herbivory. J. Exp. Mar. Biol. Ecol. 186, 183–205. doi: 10.1016/0022-0981(94)00153-5
Kim, K., and Lasker, H. R. (1997). Flow-mediated resource competition in the suspension feeding gorgonian Plexaura homomalla (Esper). J. Exp. Mar. Biol. Ecol. 215, 49–64. doi: 10.1016/s0022-0981(97)00015-4
Knowlton, N., and Jackson, J. B. C. (2001). “The ecology of coral reefs,” in Marine Community Ecology, eds M. D. Bertness, S. Gaines, and M. E. Hay (Sunderland, MA: Sinauer Associates).
Ladd, M. C., Shantz, A. A., and Burkepile, D. E. (2019). Newly dominant benthic invertebrates reshape competitive networks on contemporary Caribbean reefs. Coral Reefs 38, 1317–1328. doi: 10.1007/s00338-019-01832-6
Lamy, T., Koenigs, C., Holbrook, S. J., Miller, R. J., Stier, A. C., and Reed, D. C. (2020). Foundation species promote community stability by increasing diversity in a giant kelp forest. Ecology 101L:e02987.
Lasker, H. R., Martínez-Quintana, Á, Bramanti, L., and Edmunds, P. J. (2020). Resilience of octocoral forests to catastrophic storms. Sci. Reps. 10:4286.
Lesser, M. P. (2006). Benthic–pelagic coupling on coral reefs: feeding and growth of Caribbean sponges. J. Exp. Mar. Biol. Ecol. 328, 277–288. doi: 10.1016/j.jembe.2005.07.010
Lesser, M. P., and Slattery, M. (2011). Phase shift to algal dominated communities at mesophotic depths associated with lionfish (Pterois volitans) invasion on a Bahamian coral reef. Biol. Invas. 13, 1855–1868. doi: 10.1007/s10530-011-0005-z
Lesser, M. P., and Slattery, M. (2013). Ecology of Caribbean sponges: are top-down or bottom-up processes more important? PLoS One 8:e79799. doi: 10.1371/journal.pone.0079799
Lesser, M. P., and Slattery, M. (2018). Sponge density increases with depth throughout the Caribbean. Ecosphere 9:e02525. doi: 10.1002/ecs2.2525
Lesser, M. P., Slattery, M., and Leichter, J. J. (2009). Ecology of mesophotic coral reefs. J. Exp. Mar. Biol. Ecol. 375, 1–8.
Lesser, M. P., Slattery, M., and Mobley, C. D. (2018). Biodiversity and functional ecology of mesophotic coral reefs. Ann. Rev. Ecol. Evol. Syst. 49, 49–71. doi: 10.1146/annurev-ecolsys-110617-062423
Lesser, M. P., Slattery, M., Laverick, J. H., Macartney, K. J., and Bridge, T. C. (2019). Global community breaks at 60 m on mesophotic coral reefs. Glob. Ecol. Biogeogr. 28, 1403–1416. doi: 10.1111/geb.12940
Liddell, W. D., Avery, W. E., and Ohlhorst, S. L. (1997). “Patterns of benthic community structure, 10-250 m, the Bahamas,” in Proceedings of the 8th International Coral Reef Symposium, (Panama: Smithsonian Tropical Research Institute), 437–442.
Maida, M., Sammarco, P. W., and Coll, J. C. (2001). Effects of soft corals on scleractinian coral recruitment 2: allelopathy, spat survivorship, and reef community structure. Mar. Ecol. 22, 397–414. doi: 10.1046/j.1439-0485.2001.01709.x
Maldonado, M. (2006). The ecology of the sponge larva. Can. J. Zool. 84, 175–194. doi: 10.1139/z05-177
McCook, L., Jompa, J., and Diaz-Pulido, G. (2001). Competition between corals and algae on coral reefs: a review of evidence and mechanisms. Coral Reefs 19, 400–417. doi: 10.1007/s003380000129
McLean, E. L., and Yoshioka, P. M. (2007). “Associations and interactions between gorgonians and sponges,” in Porifera Research, Biodiversity, Innovation and Sustainability, eds M. R. Custodio, G. Lobo-Hadju, E. Hadju, and G. Muricy (Brazil: Museu Nacional de Rio de Janeiro).
Mercado-Molina, A. E., Rivera-Irizarry, F., Fonseca-Miranda, J., and Bruno-Laureano, Y. (2018). Growth facilitation by the octocoral Gorgonia ventalina explains spatial difference in the population size structure of the common demosponge Ircinia felix. Mar. Biol. Res. 14, 41–50. doi: 10.1080/17451000.2017.1367098
Norstrom, A. V., Nystrom, M., Lokrantz, J., and Folke, C. (2009). Alternati ve states on coral reefs: beyond coral-macroalgal phase shifts. Mar. Ecol. Prog. Ser. 376, 295–306. doi: 10.3354/meps07815
Pawlik, J. R. (1992). Chemical ecology of the settlement of benthic marine invertebrates. Oceanogr. Mar. Biol. Ann. Rev. 30, 273–335.
Pomponi, S. A., Diaz, M. C., Van Soest, R. W. M., Bell, L. J., Busutil, L., et al. (2019). “Sponges,” in Mesophotic Coral Ecosystems, eds Y. Loya, K. A. Puglise, and T. C. L. Bridge (Cham: Springer).
Ponti, M., Perlini, R. A., Ventra, V., Grech, D., Abbiati, M., and Cerrano, C. (2014). Ecological shifts in Mediterranean coralligenous assemblages related to gorgonian forest loss. PLoS One 9:e102782. doi: 10.1371/journal.pone.0102782
Ponti, M., Turicchia, E., Ferro, F., Cerrano, C., and Abbiati, M. (2018). The understorey of gorgonian forests in mesophotic temperate reefs. Aq. Cons. Mar. Fresh. Ecosyst. 28, 1153–1166. doi: 10.1002/aqc.2928
Porter, J. W., and Targett, N. M. (1988). Allelochemical interactions between sponges and corals. Biol. Bull. 175, 230–239. doi: 10.2307/1541563
Privitera-Johnson, K., Lenz, E. A., and Edmunds, P. J. (2015). Density-associated recruitment in octocoral communities in St. John, US Virgin Islands. J. Exp. Mar. Biol. Ecol. 473, 103–109. doi: 10.1016/j.jembe.2015.08.006
Qi, S. H., Zhang, S., Qian, P. Y., Xiao, Z. H., and Li, M. Y. (2006). Ten new antifouling briarane diterpenoids from the South China Sea gorgonian Junceella juncea. Tetrahedron 62, 9123–9130. doi: 10.1016/j.tet.2006.07.049
Rasher, D. B., Stout, E. P., Engel, S., Kubanek, J., and Hay, M. E. (2011). Macroalgal terpenes function as allelopathic agents against reef corals. Proc. Natl. Acad. Sci. 108, 17726–17731. doi: 10.1073/pnas.1108628108
Ribeiro, F. V., da Gama, B. A., and Pereira, R. C. (2017). Structuring effects of chemicals from the sea fan Phyllogorgia dilatata on benthic communities. PeerJ 5:e3186. doi: 10.7717/peerj.3186
Ribes, M., Coma, R., and Gili, J. M. (1998). Heterotrophic feeding by gorgonian corals with symbiotic zooxanthella. Limnol. Oceanogr. 43, 1170–1179. doi: 10.4319/lo.1998.43.6.1170
Ribes, M., Coma, R., and Gili, J. M. (1999). Heterogeneous feeding in benthic suspension feeders: the natural diet and grazing rate of the temperate gorgonian Paramuricea clavulata (Cnidaria: Octocorallia) over a year cycle. Mar. Ecol. Prog. Ser. 183, 125–137. doi: 10.3354/meps183125
Ritson-Williams, R., Arnold, S., Fogarty, N., Steneck, R. S., Vermeij, M. J. A., and Paul, V. J. (2009). New perspectives on ecological mechanisms affecting coral recruitment on reefs. Smithson. Contrib. Mar. Sci. 38, 437–457. doi: 10.5479/si.01960768.38.437
Rublee, P. A., Lasker, H. R., Gottfried, M., and Roman, M. R. (1980). Production and bacterial colonization of mucus from the soft coral Briarium asbestinum. Bull. Mar. Sci. 30, 888–893.
Sánchez, J. A., Dueñas, L. F., Rowley, S. J., Gonzalez-Zapata, F. L., Vergara, D. C., Montaño-Salazar, S. M., et al. (2019). “Gorgonian corals,” in Mesophotic Coral Ecosystems, eds Y. Loya, K. A. Puglise, and T. C. L. Bridge (Cham: Springer).
Sebens, K. P. (1984). Water flow and coral colony size: interhabitat comparisons of the octocoral Alcyonium siderium. Proc. Natl. Acad. Sci. 81, 5473–5477. doi: 10.1073/pnas.81.17.5473
Shoham, E., and Benayahu, Y. (2017). Higher species richness of octocorals in the upper mesophotic zone in Eilat (Gulf of Aqaba) compared to shallower reef zones. Coral Reefs 36, 71–81. doi: 10.1007/s00338-016-1528-7
Slattery, M. (1992). Larval settlement and juvenile survival in the red abalone (Haliotis rufescens), an examination of inductive cues and substrate selection. Aquaculture 102, 143–153. doi: 10.1016/0044-8486(92)90296-w
Slattery, M., and Gochfeld, D. J. (2012). “Chemically mediated competition and host–pathogen interactions among marine organisms,” in Handbook of Marine Natural Products, eds E. Fattorusso, W. H. Gerwick, and O. Taglialatela-Scafati (Berlin: Springer).
Slattery, M., and Lesser, M. P. (2012). “Mesophotic coral reefs: a global model of community structure and function,” in Proceedings of the 12th International Coral Reef Symposium, (Cairns: ICRS).
Slattery, M., and Lesser, M. P. (2014). Allelopathy in the tropical alga Lobophora variegata (Phaeophyceae): mechanistic basis for a phase shift on mesophotic coral reefs? J. Phycol. 50, 493–505. doi: 10.1111/jpy.12160
Slattery, M., and Lesser, M. P. (2019). “The Bahamas and Cayman Islands,” in Mesophotic Coral Ecosystems, eds Y. Loya, K. A. Puglise, and T. C. L. Bridge (Cham: Springer).
Slattery, M., Hamann, M. T., McClintock, J. B., Perry, T. L., Puglisi, M. P., and Yoshida, W. Y. (1997). Ecological roles for water-borne metabolites from Antarctic soft corals. Mar. Ecol. Prog. Ser. 161, 133–144. doi: 10.3354/meps161133
Slattery, M., Kamel, H. N., Ankisetty, S., Gochfeld, D. J., Hoover, C. A., and Thacker, R. W. (2008). Hybrid vigor in a tropical Pacific soft−coral community. Ecol. Monogr. 78, 423–443. doi: 10.1890/07-1339.1
Slattery, M., Lesser, M. P., Brazeau, D., Stokes, M. D., and Leichter, J. J. (2011). Connectivity and stability of mesophotic coral reefs. J. Exp. Mar. Biol. Ecol. 408, 32–41. doi: 10.1016/j.jembe.2011.07.024
Slattery, M., Moore, S., Boye, L., Whitney, S., Woolsey, A., and Woolsey, M. (2018). The Pulley Ridge deep reef is not a stable refugia through time. Coral Reefs 37, 391–396. doi: 10.1007/s00338-018-1664-3
Smith, F., and Witman, J. D. (1999). Species diversity in subtidal landscapes: maintenance by physical processes and larval recruitment. Ecology 80, 51–69. doi: 10.1890/0012-9658(1999)080[0051:sdislm]2.0.co;2
Smith, T. B., Blondeau, J., Nemeth, R. S., Pittman, S. J., Calnan, J. M., Kadison, E., et al. (2010). Benthic structure and cryptic mortality in a Caribbean mesophotic coral reef bank system, the Hind Bank Marine Conservation District, US Virgin Islands. Coral Reefs 29, 289–308. doi: 10.1007/s00338-009-0575-8
Smith, T. B., Brandtneris, V. W., Canals, M., Brandt, M. E., Martens, J., Brewer, R. S., et al. (2016). Potential structuring forces on a shelf edge upper mesophotic coral ecosystem in the US Virgin Islands. Frontiers Mar. Sci. 3:115. doi: 10.3389/fmars.2016.00115
Sousa, W. P., Kennedy, P. G., Mitchell, B. J., and Ordóñez, L. B. M. (2007). Supply-side ecology in mangroves: do propagule dispersal and seedling establishment explain forest structure? Ecol. Monogr. 77, 53–76. doi: 10.1890/05-1935
Stachowicz, J. J. (2001). Mutualism, facilitation, and the structure of ecological communities. Bioscience 51, 235–246. doi: 10.1641/0006-3568(2001)051[0235:mfatso]2.0.co;2
Sun, L. L., Shao, C. L., Mei, W. L., Huang, H., Wang, C. Y., and Dai, H. F. (2010). Chemical constituents of gorgonian Verrucella umbraculum from the South China Sea. Biochem. Syst. Ecol. 38, 1085–1087. doi: 10.1016/j.bse.2010.11.001
Thacker, R. W., Becerro, M. A., Lumbang, W. A., and Paul, V. J. (1998). Allelopathic interactions between sponges on a tropical reef. Ecology 79, 1740–1750. doi: 10.1890/0012-9658(1998)079[1740:aibsoa]2.0.co;2
Thomsen, M. S., Altieri, A. H., Angelini, C., Bishop, M. J., Gribben, P. E., Lear, G., et al. (2018). Secondary foundation species enhance biodiversity. Nature Ecol. Evol. 2, 634–639. doi: 10.1038/s41559-018-0487-5
Tsounis, G., Edmunds, P. J., Bramanti, L., Gambrel, B., and Lasker, H. R. (2018). Variability of size structure and species composition in Caribbean octocoral communities under contrasting environmental conditions. Mar. Biol. 165, 1–14.
Verdura, J., Linares, C., Ballesteros, E., Coma, R., Uriz, M. J., Bensoussan, N., et al. (2019). Biodiversity loss in a Mediterranean ecosystem due to an extreme warming event unveils the role of an engineering gorgonian species. Sci. Reps. 9, 1–11.
Vogel, S. (1977). Current-induced flow through living sponges in nature. Proc. Natl. Acad. Sci. 74, 2069–2071. doi: 10.1073/pnas.74.5.2069
Webster, N. S., Smith, L. D., Heyward, A. J., Watts, J. E., Webb, R. I., Blackall, L. L., et al. (2004). Metamorphosis of a scleractinian coral in response to microbial biofilms. Appl. Environ. Microbiol. 70, 1213–1221. doi: 10.1128/aem.70.2.1213-1221.2004
Whalan, S., and Webster, N. S. (2014). Sponge larval settlement cues: the role of microbial biofilms in a warming ocean. Sci. Reps. 4:4072. doi: 10.1038/srep04072
Whalan, S., Webster, N. S., and Negri, A. P. (2012). Crustose coralline algae and a cnidarian neuropeptide trigger larval settlement in two coral reef sponges. PLoS One 7:e30386. doi: 10.1371/journal.pone.0030386
Williams, G. C., and Chen, J. Y. (2012). Resurrection of the octocorallian genus Antillogorgia for Caribbean species previously assigned to Pseudopterogorgia, and a taxonomic assessment of the relationship of these genera with Leptogorgia (Cnidaria, Anthozoa, Gorgoniidae). Zootaxa 3505, 39–52. doi: 10.11646/zootaxa.3505.1.3
Witman, J. D., Etter, R. J., and Smith, F. (2004). The relationship between regional and local species diversity in marine benthic communities: a global perspective. Proc. Natl. Acad. Sci. 101, 15664–15669. doi: 10.1073/pnas.0404300101
Woodley, J. D., Chornesky, E. A., Clifford, P. A., Jackson, J. B. C., Kaufman, L. S., Knowlton, N., et al. (1981). Hurricane Allen’s impact on Jamaican coral reefs. Science 214, 749–759.
Wulff, J. L. (1984). Sponge-mediated coral reef growth and rejuvenation. Coral Reefs 3, 157–163. doi: 10.1007/bf00301960
Wulff, J. L. (2005). Trade−offs in resistance to competitors and predators, and their effects on the diversity of tropical marine sponges. J. Anim. Ecol. 74, 313–321. doi: 10.1111/j.1365-2656.2005.00925.x
Wulff, J. L. (2012). Ecological interactions and the distribution, abundance, and diversity of sponges. Adv. Mar. Biol. 61, 273–344. doi: 10.1016/b978-0-12-387787-1.00003-9
Keywords: allelopathy, biodiversity, competition, facilitation, foundation species, gorgonians, mesophotic coral ecosystems, recruitment
Citation: Slattery M and Lesser MP (2021) Gorgonians Are Foundation Species on Sponge-Dominated Mesophotic Coral Reefs in the Caribbean. Front. Mar. Sci. 8:654268. doi: 10.3389/fmars.2021.654268
Received: 15 January 2021; Accepted: 08 March 2021;
Published: 06 April 2021.
Edited by:
Xavier Pochon, The University of Auckland, New ZealandReviewed by:
Erika Gress, ARC Centre of Excellence for Coral Reef Studies, AustraliaSonia J. Rowley, University of Hawai‘i at Mānoa, United States
Frederic Sinniger, University of the Ryukyus, Japan
Copyright © 2021 Slattery and Lesser. This is an open-access article distributed under the terms of the Creative Commons Attribution License (CC BY). The use, distribution or reproduction in other forums is permitted, provided the original author(s) and the copyright owner(s) are credited and that the original publication in this journal is cited, in accordance with accepted academic practice. No use, distribution or reproduction is permitted which does not comply with these terms.
*Correspondence: Marc Slattery, c2xhdHRlcnlAb2xlbWlzcy5lZHU=