- 1The Swire Institute of Marine Science and School of Biological Sciences, The University of Hong Kong, Hong Kong, China
- 2Marine Science Center, Northeastern University, Nahant, MA, United States
The ability of an organism to alter its physiology in response to environmental conditions offers a short-term defense mechanism in the face of weather extremes resulting from climate change. These often manifest as multiple, interacting drivers, especially pH and temperature. In particular, decreased pH can impose constraints on the biological mechanisms which define thermal limits by throwing off energetic equilibrium and diminishing physiological functions (e.g., in many marine ectotherms). For many species, however, we do not have a detailed understanding of these interactive effects, especially on short-term acclimation responses. Here, we investigated the metabolic plasticity of a tropical subtidal gastropod (Trochus maculatus) to increased levels of CO2 (700 ppm) and heating (+3°C), measuring metabolic performance (Q10 coefficient) and thermal sensitivity [temperature of maximum metabolic rate (TMMR), and upper lethal temperature (ULT)]. Individuals demonstrated metabolic acclimation in response to the stressors, with TMMR increasing by +4.1°C under higher temperatures, +2.7°C under elevated CO2, and +4.4°C under the combined stressors. In contrast, the ULT only increased marginally in response to heating (+0.3°C), but decreased by −2.3°C under CO2, and −8.7°C under combined stressors. Therefore, although phenotypic plasticity is evident with metabolic acclimation, acute lethal temperature limits seem to be less flexible during short-term acclimation.
Introduction
Physiological responses to abiotic conditions, mediated by individual organisms, manifest as population- and ultimately community-level responses. Therefore, identifying the mechanisms by which the survival and fitness of individual organisms change in response to environmental extremes will help determine broader ecosystem effects to ongoing climate change (Pörtner, 2008). Changes in body temperature are often among the most commonly studied responses for ectothermic marine invertebrates as temperature plays a large part in controlling cellular to physiological reactions, which in turn affects metabolism, growth and reproductive rates (Grigaltchik et al., 2012; Sinclair et al., 2016). Even small changes in the environment can cause a broad range of physiological responses due to variation among and within species (Harley et al., 2017; Wang et al., 2018). In particular, there has been an increasing focus on the physiological impacts of heatwaves, which are increasing in magnitude and frequency, increasing the likelihood that organisms are being subjected to thermal conditions beyond their optimal and lethal limits (Lannig et al., 2010; Sinclair et al., 2016).
Large variation in responses, even within a species, highlights that genetic variation and variability in physiological plasticity can affect the likelihood that populations survive short-term extremes (Wang et al., 2018). Should enough individuals be able to acclimate to novel conditions during extreme events, populations and communities may be able to handle future conditions better than currently predicted (Seebacher et al., 2014). Phenotypic plasticity allows organisms to respond to stressful environmental conditions and is thought to be determined by environmental selection pressure and an organism’s sensitivity to change (Parmesan and Yohe, 2003; Hoffmann and Sgró, 2011). For example, intertidal species are naturally exposed to large variation in temperature, regularly being exposed to extremes, and as such are typically already living much nearer their thermal maxima. Consequently, intertidal species generally demonstrate at least phenotypic plasticity in their thermal optima but little capability of increasing upper lethal limits (Stillman and Somero, 2000; Nguyen et al., 2011). In contrast, subtidal organisms are generally considered to live in a relatively stable environment and therefore thought to exhibit lower plasticity but potentially a greater capacity for acclimation.
Importantly, however, we understand considerably less of how organisms acclimate to changes in multiple stressors, particularly temperature and altered pH due to increases in CO2 concentrations (Gunderson et al., 2016; Kroeker et al., 2017). We know especially little about the ability of subtidal species to modulate their physiology in response to multiple stressors, since until recently it has largely been assumed that subtidal environments are temporally and spatially stable (Bates et al., 2018). With the advent of increased ability to monitor environmental conditions such as temperature and pH at finer temporal and broader spatial scales, it is now apparent that these environments are anything but stable and that underwater “weather” is an important yet relatively understudied feature of the marine environment (Bates et al., 2018). For example, in coastal habitats pH can fluctuate by an entire unit within hours to days (Kelly and Hofmann, 2013; Hofmann et al., 2014). Changes in temperature can likewise occur over a broad range of temporal and spatial scales, from increases of several degrees due to solar heating (or decreases due to upwelling) to seasonal increases of the same magnitude that can last several months (Bates et al., 2018; Pansch et al., 2018). Therefore, understanding the ability of organisms to remodel their physiology is crucial to predict their capacity to respond to, and persist under, combinations of environmental stressors such as increasing CO2 concentrations and temperature (Hoffmann and Sgró, 2011; Seebacher et al., 2014).
Exposure to elevated CO2 and temperatures at levels expected in the coming century (or even much sooner) have been shown to have greater effects on marine ectotherms than the stressors in isolation (Byrne, 2011; Byrne and Przeslawski, 2013; Brothers et al., 2016; Carey et al., 2016). For example, tropical urchins (Echinometra sp.) suffer far greater reduction in growth and higher metabolic activity when exposed simultaneously to elevated CO2 (∼860–940 μatm) and temperature (ambient +2 to 3°C) than when either is considered alone (Uthicke et al., 2014). Intertidal limpets not only have higher sensitivity to hotter environments under elevated CO2 concentrations, but at a population level have greater variation in individual responses to temperature, signifying that some individuals have greater ability to alter their tolerance limits (Wang et al., 2018). Such variable responses ultimately determine the potential for acclimation and plasticity within populations, producing a selective advantage by extending the organism’s physiological (fundamental) niche. Critical thermal maximum and minimum (CTMAX and CTMIN) are commonly used to assess the temperature beyond which organisms can no longer supply adequate oxygen to cells to fuel metabolism (Pörtner, 2010). Elevated CO2 in conjunction with increased temperature can alter thermal acclimation of metabolic capacity by decreasing the CTMAX, potentially resulting in a greater susceptibility to global heating (Manríquez et al., 2019). Whether organisms can shift their maximum threshold temperatures will therefore provide an indication of the extent of the ability of populations and species to respond to rapidly changing environmental conditions.
Here we assessed the acclimation potential of a key grazing subtidal gastropod to elevated levels of CO2 and temperature, in particular the ability to alter metabolic performance (Q10 coefficient), shift their temperature of maximum metabolic rate (TMMR) or their upper lethal temperature (ULT). The gastropod Trochus maculatus is commonly found in shallow subtidal habitats in Hong Kong, feeding on a variety of different micro- and macroalgae, playing an important role in maintenance of benthic habitats (Cox and Murray, 2006; Maboloc and Mingoa-Licuanan, 2013). We experimentally tested the influence of elevated CO2 and temperature on the physiology of T. maculatus to determine their acclimation potential in the face of ongoing and future climate change. We tested the hypothesis that whilst elevated temperature would cause a shift in metabolic thresholds, the addition of elevated CO2 would inhibit this effect. Under ocean heating, an inability to increase upper lethal temperatures, either through phenotypic plasticity or longer-term acclimation, would likely lead to population declines and loss in ecological function.
Materials and Methods
Field Collection
Adult gastropods (Trochus maculatus) are widely distributed in south-east Asia and the central Western-Pacific Ocean found in shallow coral and rocky reef habitats, and commonly collocated with other benthic grazers such as sea urchins (Cox and Murray, 2006; Maboloc and Mingoa-Licuanan, 2013). In Hong Kong, the sub-tropical climate exposes these organisms to cold winters with lows of ∼16°C, and warm summers, with shallow marine water temperatures having reached a maximum of 31°C locally (Environmental Protection Department (EPD) Hong Kong, 2019). T. maculatus were collected from subtidal rocky substrate (∼1–4 m depth) in Clearwater Bay (Shek Mei Tau), Hong Kong (22°16′58.5′′N 114°17′40.6′′E) and taken to the Swire Institute of Marine Science (SWIMS) where they were transferred immediately into indoor plastic aquaria (12 L) (n = 15 per treatment with 1 individual per tank) with flow-through, sand-filtered seawater at ambient summer conditions (28°C, Hong Kong summer average for subtidal waters). Each aquarium was aerated with ambient air and maintained 12:12 day/night lighting conditions with LED lights fitted above the tanks. Food was provided ad libitum as turf-forming and filamentous algae growing on rocks taken from the field collection site during acclimation and experimental treatments.
CO2 and Temperature Manipulation
We tested the effects of two CO2 conditions [400 ppm/8.1 pH (control) and 700 ppm/7.8 pH (elevated)] and two temperatures [28°C (control) and 31°C] in fully crossed combinations (n = 15 per treatment) representing the present day and IPCC RCP 6.0 scenario for the year 2100, and the highest locally experienced temperatures. After a 2-week acclimation period to ambient conditions in the laboratory, CO2 and temperature were raised to treatment conditions gradually over a period of 2 days and maintained for 12 weeks. Aquaria were placed within water baths to help maintain thermal stability, with each aquarium containing a submersible glass heater to control temperature to the pre-designated treatment. Each aquarium was supplied with an independent, intermittent flow-through system which allowed daily water changes with sand-filtered seawater taken from the bay at SWIMS. CO2 treatment conditions were maintained by bubbling each individual aquarium with air that was pre-mixed to the treatment CO2 concentration using gas flow meters (Masterflex® Direct-Reading Variable-Area Flowmeters with Valve for Air and CO2, with Masterflex® Correlated and Direct-Reading Variable Area Flowmeter Multitube Frames Cole-Palmer, Ill, United States). The pH and temperature in each aquarium were monitored using a handheld multiprobe twice per day (Seven2go, Mettler Toledo, OH, United States). Salinity was measured once weekly and water samples were analyzed weekly for total alkalinity using an Alkalinity Titrator (T50, Mettler Toledo, OH, United States). Seawater carbonate parameters [CO2, bicarbonate (HCO3 –) and carbonate (CO3 2–) ion concentration] and calcite and aragonite saturation states (Ωcal and Ωara) were calculated using CO2SYS program for Excel (Lewis and Wallace, 1998) with constants from Mehrbach et al. (1973) as adjusted by Dickson and Millero (1987) from recorded temperature, pH and TA values (Table 1).
Thermal Ramps
After 12 weeks at treatment conditions, n = 10 individuals were subjected to gradually increasing temperatures over a thermal ramp in order to assess how their metabolic rates changes after exposure to treatment conditions, to determine the Q10 coefficient, the upper lethal temperature (ULT) and temperature of maximum metabolic rate (TMMR). Individual gastropods were placed into sealed respirometry chambers (0.5 L) with filtered, oxygen saturated seawater which had been CO2 treated according to the relevant treatment. Each chamber was placed into a water bath set at 17°C (average winter seawater temperature in Hong Kong) with a small water pump placed in the bath to maintain a homogenous temperature. After being left for 30 min for the gastropods to settle, the temperature was increased by 2°C per hour until all animals died (lack of reaction to physical stimulus). After every 1 h (2°C) increment, temperature was held constant for 30 min for the metabolic rate to be recorded. Oxygen concentration within the respirometry chambers was recorded before and after each 30 min incubation using an internal optical oxygen spot measuring system (Fibox4, PreSens). Metabolic rate MO2 (mg L–1 g FW–1 h–1) was calculated using the following equation:
where ΔO2 is the linear regression slope of oxygen concentration over time (mg L–1 h–1), V is the volume of seawater (L), and W is the fresh weight of the gastropod (g). Blank chambers with no organisms inside were used to account for any possible biological activity in the water and deducted accordingly.
Temperature Coefficient (Q10)
The Q10 coefficient was calculated using metabolic rate data from the thermal ramp, and calculated from the rates at 10°C below and up to the TMMR [TMMR based off the data from an Exponentially Modified Gaussian Function (EMG) model described below] using the following equation:
where R is the metabolic rate (R1 and R2 are the metabolic rate at T1 and T2, respectively), and T is the temperature (°C).
Morphological Biometrics
We also measured morphological parameters at the beginning and end of the experimental period to test for any physical changes caused by elevated CO2 (reduced pH), relating to total weight, shell size and shape (n = 15). The total weight of each intact individual (g), height and width were recorded (mm) before and after exposure to the experimental treatments. These dimensions were then used to calculate measures relating to shell shape, i.e., aspect ratio, which was determined as width: height. The values of these measures were calculated for each individual as percentage change between the values at the beginning and end of the experimental exposure period.
Statistical Analysis
Two-factor ANOVAs were used to test for the effects of CO2 (ambient control vs. elevated) and temperature (control vs. elevated) (fixed and orthogonal) on weight (total weight), shell morphology (height, width) and aspect ratio (width: height) using Euclidean distance in PERMANOVA for PRIMER 7. An Exponentially Modified Gaussian Function (EMG) model was fitted for metabolic rates over the temperature ramp to extract the TMMR, ULT, and to model the change in metabolic rate over the temperature ramp. Temperature coefficient (Q10) data were analyzed using a two-way ANOVA analysis with Tukey’s post-doc test, both of which were performed using RStudio Version 1.1.463 with 95% confidence intervals with all analysis.
Results
Thermal Ramps and Critical Limits
Metabolic rates of the gastropods across thermal ramps were well described by the Exponentially Modified Gaussian Function, demonstrating a positive relationship with temperature up until the temperature of maximum metabolic rate (TMMR) followed by a sharp decline to lethal limits which resulted in mortality (ULT). The TMMR increased from 31.6°C in the control treatment to 35.6°C in the high temperature treatment, 34.3°C in the high CO2 treatment and 36°C in the combination treatment (Figure 1 and Table 2). The ULT did not increase following exposure to elevated temperature (47.5°C vs. 47.8°C in control and elevated temperature, respectively). In contrast, high CO2 caused in a decline in the ULT to 45.2°C (−2.3°C decrease), and an even greater decline of 8.7°C in the ULT under the combination of high CO2 and temperature (38.8°C; Figure 1 and Table 2).
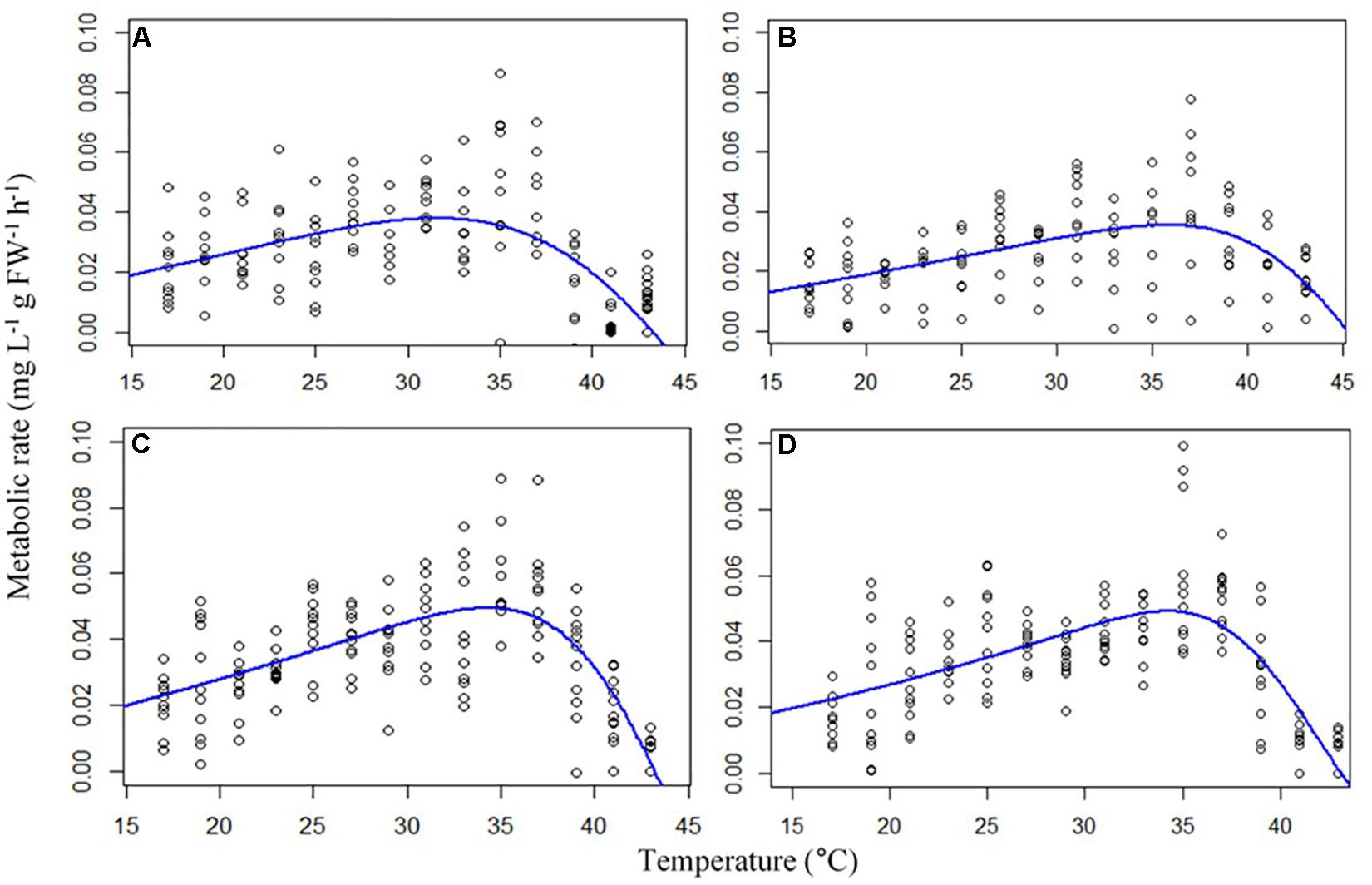
Figure 1. Thermal ramps showing metabolic rates (mg L –1 g FW –1 h –1) of the gastropod Trochus maculatus (n = 10) after 12-weeks exposure to (A) control conditions (28°C, 400 ppm) (B) high temperature (31°C, 400 ppm) (C) high CO2 (28°C, 700 ppm) and (D) a combination of both high temperature and CO2 (31°C, 700 ppm). Curves are modeled using an Exponentially Modified Gaussian Function.
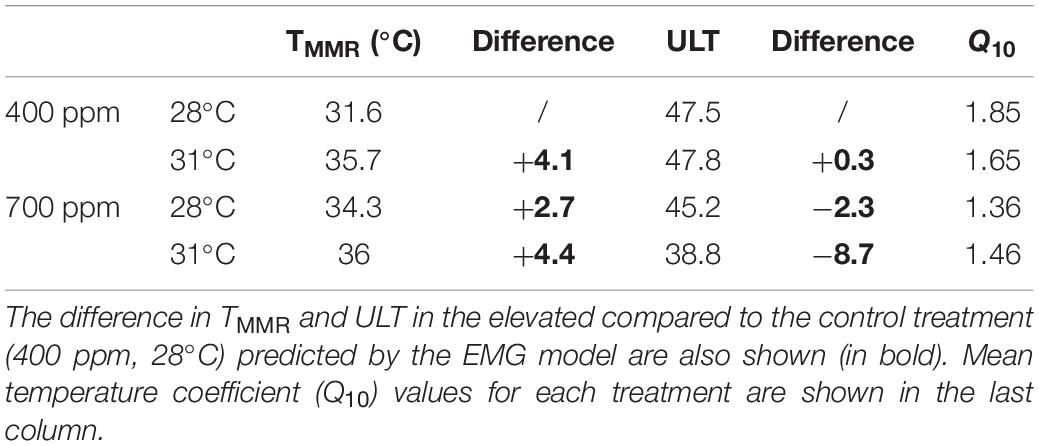
Table 2. Temperature at maximum metabolic rate (TMMR) and upper lethal temperature (ULT) as described by an Exponentially Modified Gaussian Function (EMG) model for Trochus maculatus after 12-weeks exposure to elevated temperature (31°C) and elevated CO2 (700 ppm).
Temperature Coefficient (Q10)
Elevated CO2 negatively affected the temperature coefficients (Q10 rates) of T. maculatus, with individuals exposed to elevated CO2 having Q10 values >25% lower than under ambient CO2 [two-way ANOVA, F(1, 1) = 4.3, P = 0.04, Figure 2 and Table 2]. In contrast, there was no significant effect of elevated temperature [two-way ANOVA, F(1, 1) = 0.06, P > 0.05] or for the interaction between temperature and CO2 [two-way ANOVA, F(1, 1) = 0.79, P > 0.05] on Q10 rates.
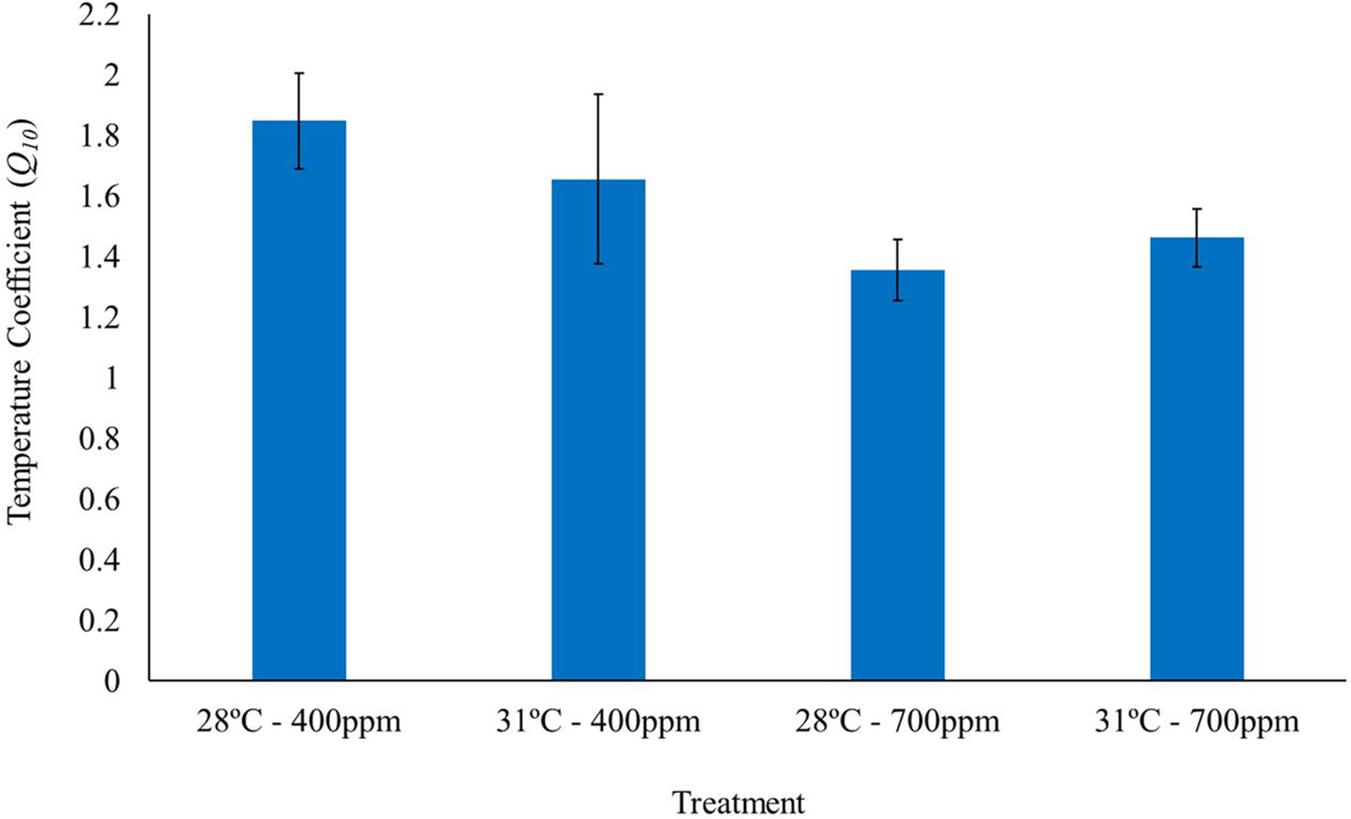
Figure 2. Temperature coefficients (Q10) of gastropods acclimated to different temperatures (28 and 31°C) and CO2 concentrations (400 and 700 ppm) (n = 15). Temperature coefficient values were calculated for each individual gastropod, as the respiration rate at 10°C below and up to the corresponding TMMR for that treatment. Bars represent mean Q10 of all individuals in the given treatment ± standard error.
Morphological Biometrics
As could be expected from exposure to elevated CO2 for relatively short periods (relative to lifespan), none of the experimental treatments caused any significant change to gastropod weight or shell morphometrics over the length of the experiment (Figure 3 and Supplementary Tables S1, S2).
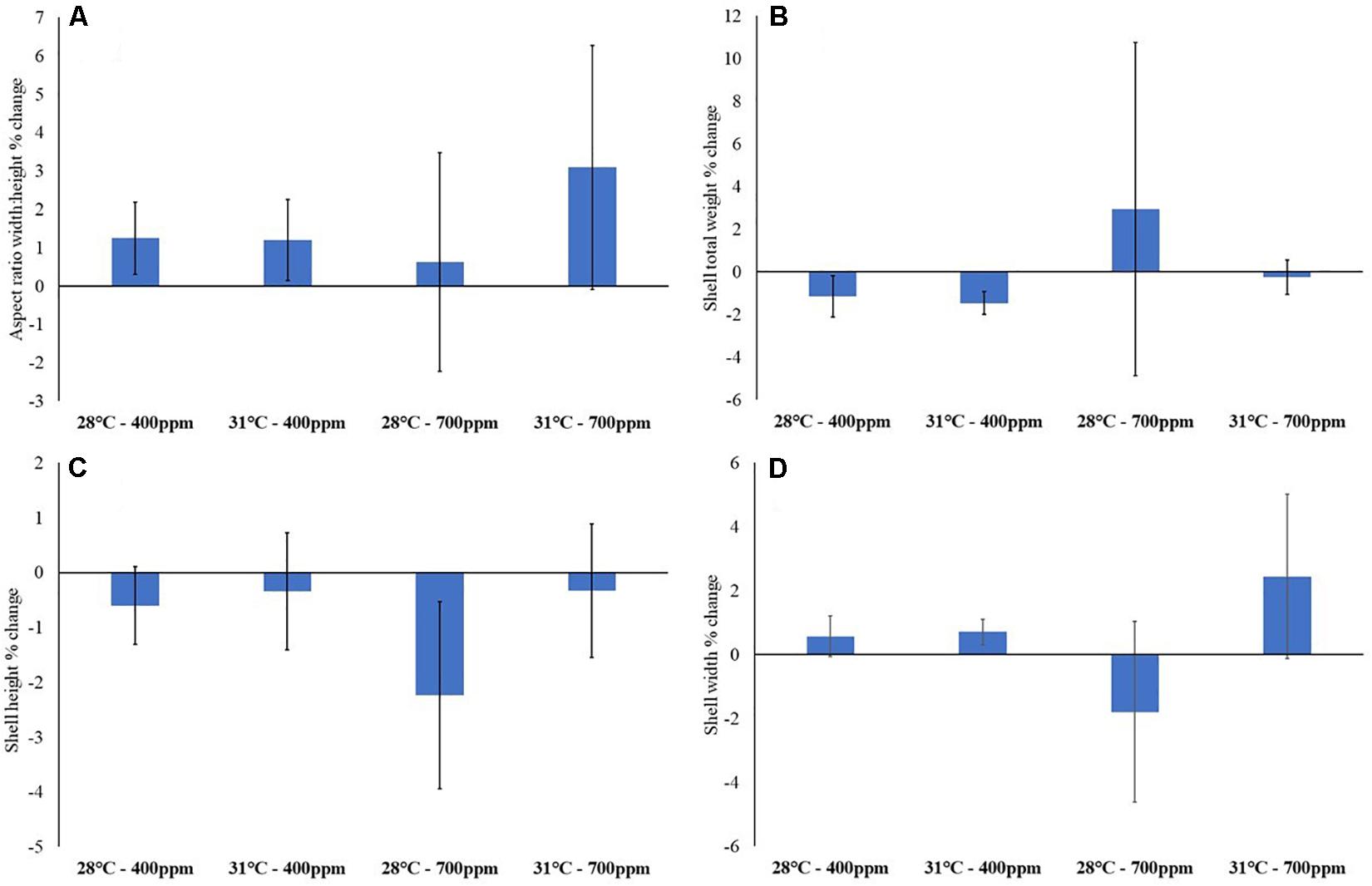
Figure 3. Gastropod biometrics after 12-weeks exposure to control conditions (28°C, 400 ppm), high temperature (31°C, 400 ppm), high CO2 (28°C, 700 ppm) and a combination of both high temperature and CO2 (31°C, 700 ppm). (A) Aspect ratio (width: height); (B) total weight (g); (C) shell height; and (D) shell width (mm) (n = 15). Values are percentage change from prior to exposure to experimental conditions ± standard error.
Experimental Conditions
The control treatment at 28°C–400 ppm had a measured pH of 8.08 ± 0.02 and calculated CO2 (μatm) of 329.9 ± 15.7, with similar pCO2 conditions found in the higher temperature treatment at 31°C–400 ppm, with a pH of 8.08 ± 0.02 and calculated CO2 (μatm) of 330.0 ± 19.4. In the higher CO2 treatment at 28°C–700 ppm, there was a lower measured pH of 7.81 ± 0.01 and higher calculated CO2 (μatm) of 721.8 ± 8.90 compared with the controls. Lastly, with the higher temperature and higher CO2 treatment at 31°C–700 ppm, there was a lower measured pH of 7.82 ± 0.01 and higher calculated CO2 (μatm) of 683.9 ± 18.4 compared with the controls (Table 1).
Discussion
Whether organisms can withstand altered environmental conditions brought about by global climate change depends on the capacity for their physiological mechanisms to adjust and acclimate to continually changing local conditions. Here, we demonstrate that a key subtidal gastropod, T. maculatus, showed the potential for acclimation to higher temperatures, but this effect was diminished by coincident elevated CO2. When exposed to hotter conditions alone, the temperature at which metabolic rates are the highest (TMMR) increased, but the upper lethal temperature (ULT) was static, which would suggest that when exposed to elevated temperatures alone T. maculatus can display metabolic plasticity but that the upper lethal limits are relatively fixed and cannot increase. Deviation from energetic homeostasis as a result of sub-optimal conditions is generally thought to be due to energetic demands exceeding energy gain, which can be compensated for with increased consumption (Leung et al., 2017) or elevated metabolic flux to sustain the ATP demand (Lannig et al., 2010). These strategies are time-limited, meaning that a lack of compensatory feeding to fulfill new higher energy demands and capacity for cellular energy generation results in metabolic depression, an adaptive strategy used across all animal phyla (Guppy and Withers, 1999; Marshall et al., 2011), suggesting lack of energy acquisition may have played an important part in this species’ response. This energy mismatch is exacerbated further when the stressors are combined, as it is likely that the energetic stress of the CO2 exposure drove mortality at lower temperatures. The increased TMMR under future conditions (combined elevated CO2 and temperature) is therefore likely to be due to the influence of temperature- induced acclimation, meaning that energetic demands at a given temperature would be lower than prior to acclimation (Seebacher et al., 2014; Leung et al., 2021). In contrast, the reduction in ULT under these conditions would not likely pose a major offset to this acclimation in the near future for subtidal organisms as environmental conditions should not reach the reduced ULT (∼39°C when current summer extremes are ∼32°C). For intertidal organisms, however, this benefit would be eliminated by the greater reduction in upper threshold temperatures whenever elevated CO2 exposure occurs because they are regularly exposed to extreme temperatures (up to 55°C intertidal rock temperature recorded in Hong Kong; Ng et al., 2017).
The effects of CO2 and temperature on biological functions have been shown to be synergistic across numerous taxa, with the extent of the effects dependent on life-stages and species-specific sensitivities (Dupont et al., 2010; Byrne and Przeslawski, 2013; Hardy et al., 2014). For example, hypercapnia reduces oxygen partial pressure in the haemolymph of crabs (Cancer pagurus) during progressive warming (when exposed to 1% CO2) as well as a 5°C decline in the upper thermal limits of aerobic scope, substantially narrowing the thermal window of this species (Metzger et al., 2007). A similar effect was demonstrated with green abalone (Haliotis fulgens) under a combination of hypoxia and hypercapnia, which elicited large changes to metabolic rates, as well as strong accumulation of amino acids, osmolytes and anaerobic end products during moderate temperatures (Tripp-Valdez et al., 2017), indicating the species had reached critical limits and entered a state severe stress (Pörtner, 2010; Tripp-Valdez et al., 2017). In contrast, higher molluscs can regulate acid-base balance by upregulating HCO3– to compensate for hypercapnia induced respiratory acidosis, thus avoiding loss of metabolic equilibria or disrupting aerobic capacity (e.g., the cephalopod Sepia officinalis; Gutowska et al., 2010). Therefore, the capacity for acid-base regulation is highly variable, particularly among invertebrates, and plays an important role in defense against CO2-induced physiological stress. Based on the reduction in metabolic function in our species under hypercapnia, we suggest that it is likely that our study species may not have the capacity to regulate acid-base balance to a large degree.
Increased concentrations of CO2 can directly affect shell-forming invertebrates by erosion of calcium-carbonate containing skeletons or decreasing calcification rates (Feely et al., 2004; Kroeker et al., 2010; Harvey et al., 2013). Some gastropods, including Trochidae species, have a nacreous layer in their inner shell structure which is formed by hexagonal platelets of aragonite along with other microstructures of aragonite and calcite which are unique to some gastropods and cephalopods (Chunhabundit et al., 2001; Nudelman et al., 2006). A meta-analysis by Kroeker et al. (2010) of the biological responses to ocean acidification including survival, calcification, growth and reproduction highlighted that organisms which use less soluble, more stable forms of calcium carbonate (CaCO3) (e.g., some sea urchins), such as the trochid gastropod in this study, are more resilient to ocean acidification that those which utilize less stable and less soluble forms. While we did not see any significant shell erosion and this could be interpreted as a more resilient shell chemistry, the experiment here was explicitly designed to test for the physiological effects of the stressors which manifest over shorter exposures. Therefore, whilst the immediate impact of these environmental stressors on key physiological processes will determine the ability of an organism to maintain energetic homeostasis, as well as plasticity and critical limits of thermotolerance, the impact of these stressors on shell formation and degradation should be considered over the longer term.
Although the temperature at which metabolic rate is at its peak appears to be plastic, critical limits are far less flexible in terms of acclimation, but rather more susceptible to reduction under stress. Indeed, with the added effect of CO2, upper lethal temperatures decreased substantially. This phenomenon has been demonstrated within a unique wild population of European perch fish (Perca fluviatilis) which had been warmed 5–10°C over three decades by the thermal discharge of a nearby nuclear power plant. Whilst the chronically heated population displayed thermally compensated resting cardio-respiratory functions, maximum temperature threshold limits exhibited little to no thermal compensation across generations, suggesting that whilst metabolic functions can be flexible, critical limits show much less plasticity (Sandblom et al., 2016). Lack of compensation in lethal limits as oceans gradually warm therefore reduces overall aerobic scope of organisms by narrowing the thermal window between habitat temperature and upper limits, resulting in species operating closer to the bounds of their functional capacity and subsequently leaving them vulnerable to more acute changes in temperature during events such as heatwaves (Pörtner and Farrell, 2008; Hemraj et al., 2020).
The relationship between plasticity and lethal temperatures is largely negative, with strong negative correlations between thermal plasticity and CTMAX (Armstrong et al., 2019). Whilst the shift in TMMR found here may demonstrate plasticity and acclimation to higher temperatures, the inability of this gastropod to substantially shift its lethal limits may not favor this species under continual climate change and especially exposure to heatwaves (Pansch et al., 2018; Leung et al., 2021). Given limited resources and the high maintenance cost of thermal response strategies, a greater acclimation potential but elevated vulnerability to acute exposure due to inability to shift threshold temperatures, highlights the importance of addressing multiple proxies for physiological responses to warming rather than chronic acclimation potential alone (Magozzi and Calosi, 2015; Armstrong et al., 2019)… Nevertheless, even with the impact of CO2 causing a decrease of ∼9°C the upper lethal temperature to 38.8°C, this still lies well above any temperature experienced in the region (highest summer seawater temperature experienced in Hong Kong in the past 10-years ∼31°C, Environmental Protection Department (EPD) Hong Kong, 2019). Of greater concern are longer-term metabolic effects and potential for energetic deficits which make mortality more likely at temperatures below the lethal limits (Mertens et al., 2015; Leung et al., 2018). Therefore, consideration of the additional effect of other environmental stressors that moderate gastropod biological processes (Marchant et al., 2010; Falkenberg et al., 2014) which may consequently exacerbate sensitivity to temperature should be considered when aiming to determine the long-term survival of populations of marine species.
Data Availability Statement
The original contributions presented in the study are included in the article/Supplementary Material, further inquiries can be directed to the corresponding author/s. The full data set for this paper is publically available at: https://datahub.hku.hk/ and DOI: 10.25442/hku.14176961.
Author Contributions
CC and BR conceived the ideas and designed methodology. CC collected the data. JM and CC analyzed the data. JM and BR led the writing of the manuscript. All authors contributed critically to the drafts and gave final approval for publication.
Funding
This project was funded by a Hong Kong Research Grants Council General Research Fund grant (GRF17122916) to BR.
Conflict of Interest
The authors declare that the research was conducted in the absence of any commercial or financial relationships that could be construed as a potential conflict of interest.
Acknowledgments
We thank the staff of the Swire Institute of Marine Science for helping to maintain the experimental systems.
Supplementary Material
The Supplementary Material for this article can be found online at: https://www.frontiersin.org/articles/10.3389/fmars.2021.643377/full#supplementary-material
References
Armstrong, E. J., Tanner, R. L., and Stillman, J. H. (2019). High heat tolerance is negatively correlated with heat tolerance plasticity in nudibranch mollusks. Physiol. Biochem. Zool. 92, 430–444. doi: 10.1086/704519
Bates, A. E., Helmuth, B., Burrows, M. T., Duncan, M. I., Garrabou, J., Guy-Haim, T., et al. (2018). Biologists ignore ocean weather at their peril. Nature 560:299. doi: 10.1038/d41586-018-05869-5
Brothers, C. J., Harianto, J., McClintock, J. B., and Byrne, M. (2016). Sea urchins in a high-CO 2 world: the influence of acclimation on the immune response to ocean warming and acidification. Proc. R. Soc. B Biol. Sci. 283, 1–7. doi: 10.1098/rspb.2016.1501
Byrne, M. (2011). “Impact of ocean warming and ocean acidification on marine invertebrate life history stages: vulnerabilities and potential for persistence in a changing ocean,” in Oceanography and Marine Biology: An Annual Review, eds R. N. Gibson, R. J. A. Atkinson and J. D. M. Gordon (Boca Raton, FL: CRC Press), 1–42.
Byrne, M., and Przeslawski, R. (2013). Multistressor impacts of warming and acidification of the ocean on marine invertebrates’ life histories. Integr. Comp. Biol. 53, 582–596. doi: 10.1093/icb/ict049
Carey, N., Harianto, J., and Byrne, M. (2016). Sea urchins in a high-CO2 world: partitioned effects of body size, ocean warming and acidification on metabolic rate. J. Exp. Biol. 219, 1178–1186. doi: 10.1242/jeb.136101
Chunhabundit, S., Chunhabundit, P., Aranyakananda, P., and Moree, N. (2001). Dietary effects on shell microstructures of cultured, maculate top shell (Trochidae: Trochus maculatus, Linnaeus, 1758). SPC Trochus Inf. Bull. 8, 15–22.
Cox, T. E., and Murray, S. N. (2006). Feeding preferences and the relationships between food choice and assimilation efficiency in the herbivorous marine snail Lithopoma undosum (Turbinidae). Mar. Biol. 148, 1295–1306. doi: 10.1007/s00227-005-0166-3
Dickson, A. G., and Millero, F. J. (1987). A comparison of the equilibrium constants for the dissociation of carbonic acid in seawater media. Deep Sea Res. Part A Oceanogr. Res. Pap. 34, 1733–1743. doi: 10.1016/0198-0149(87)90021-5
Dupont, S., Ortega-Martínez, O., and Thorndyke, M. (2010). Impact of near-future ocean acidification on echinoderms. Ecotoxicology 19, 449–462. doi: 10.1007/s10646-010-0463-6
Environmental Protection Department (EPD) Hong Kong (2019). Marine Water Quality Data [Data File]. Available online at: https://cd.epic.epd.gov.hk/EPICRIVER/marine/
Falkenberg, L. J., Connell, S. D., and Russell, B. D. (2014). Herbivory mediates the expansion of an algal habitat under nutrient and CO2 enrichment. Mar. Ecol. Prog. Ser. 497, 87–92. doi: 10.3354/meps10557
Feely, R. A., Sabine, C. L., Lee, K., Berelson, W., Kleypas, J., Fabry, V. J., et al. (2004). Impact of anthropogenic CO2 on the CaCO3 system in the oceans. Science 305, 362–366. doi: 10.1126/science.1097329
Grigaltchik, V. S., Ward, A. J. W., and Seebacher, F. (2012). Thermal acclimation of interactions: differential responses to temperature change alter predator-prey relationship. Proc. R. Soc. B Biol. Sci. 279, 4058–4064. doi: 10.1098/rspb.2012.1277
Gunderson, A. R., Armstrong, E. J., and Stillman, J. H. (2016). Multiple stressors in a changing world: the need for an improved perspective on physiological responses to the dynamic marine environment. Ann. Rev. Mar. Sci. 8, 357–378. doi: 10.1146/annurev-marine-122414-033953
Guppy, M., and Withers, P. (1999). Metabolic depression in animals: physiological perspectives and biochemical generalizations. Biol. Rev. Camb. Philos. Soc. 74, 1–40. doi: 10.1017/S0006323198005258
Gutowska, M. A., Melzner, F., Langenbuch, M., Bock, C., Claireaux, G., and Pörtner, H. O. (2010). Acid-base regulatory ability of the cephalopod (Sepia officinalis) in response to environmental hypercapnia. J. Comp. Physiol. B. 180, 323–325. doi: 10.1007/s00360-009-0412-y
Hardy, N. A., Lamare, M., Uthicke, S., Wolfe, K., Doo, S., Dworjanyn, S., et al. (2014). Thermal tolerance of early development in tropical and temperate sea urchins: inferences for the tropicalization of eastern Australia. Mar. Biol. 161, 395–409. doi: 10.1007/s00227-013-2344-z
Harley, C. D. G., Connell, S. D., Doubleday, Z. A., Kelaher, B., Russell, B. D., Sarà, G., et al. (2017). Conceptualizing ecosystem tipping points within a physiological framework. Ecol. Evol. 7, 6035–6045. doi: 10.1002/ece3.3164
Harvey, B. P., Gwynn-Jones, D., and Moore, P. J. (2013). Meta-analysis reveals complex marine biological responses to the interactive effects of ocean acidification and warming. Ecol. Evol. 3, 1016–1030. doi: 10.1002/ece3.516
Hemraj, D. A., Posnett, N. C., Minuti, J. J., Firth, L. B., and Russell, B. D. (2020). Survived but not safe: marine heatwave hinders metabolism in two gastropod survivors. Mar. Environ. Res. 162, 105–117. doi: 10.1016/j.marenvres.2020.105117
Hoffmann, A. A., and Sgró, C. M. (2011). Climate change and evolutionary adaptation. Nature 470, 479–485. doi: 10.1038/nature09670
Hofmann, G. E., Evans, T. G., Kelly, M. W., Padilla-Gamiño, J. L., Blanchette, C. A., Washburn, L., et al. (2014). Exploring local adaptation and the ocean acidification seascape – studies in the California current large marine ecosystem. Biogeosciences 11, 1053–1064. doi: 10.5194/bg-11-1053-2014
Kelly, M. W., and Hofmann, G. E. (2013). Adaptation and the physiology of ocean acidification. Funct. Ecol. 27, 980–990. doi: 10.1111/j.1365-2435.2012.02061.x
Kroeker, K. J., Kordas, R. L., Crim, R. N., and Singh, G. G. (2010). Meta-analysis reveals negative yet variable effects of ocean acidification on marine organisms. Ecol. Lett. 13, 1419–1434. doi: 10.1111/j.1461-0248.2010.01518.x
Kroeker, K. J., Kordas, R. L., and Harley, C. D. G. (2017). Embracing interactions in ocean acidification research: confronting multiple stressor scenarios and context dependence. Biol. Lett. 13:20160802. doi: 10.1098/rsbl.2016.0802
Lannig, G., Eilers, S., Pörtner, H. O., Sokolova, I. M., and Bock, C. (2010). Impact of ocean acidification on energy metabolism of oyster, Crassostrea gigas–changes in metabolic pathways and thermal response. Mar. Drugs 8, 2318–2339. doi: 10.3390/md8082318
Leung, J. Y. S., Connell, S. D., and Russell, B. D. (2017). Heatwaves diminish the survival of a subtidal gastropod through reduction in energy budget and depletion of energy reserves. Sci. Rep. 7:17688. doi: 10.1038/s41598-017-16341-1
Leung, J. Y. S., Nagelkerken, I., Russell, B. D., Ferreira, C. M., and Connell, S. D. (2018). Boosted nutritional quality of food by CO2 enrichment fails to offset energy demand of herbivores under ocean warming, causing energy depletion and mortality. Sci. Total Environ. 639, 360–366. doi: 10.1016/j.scitotenv.2018.05.161
Leung, J. Y. S., Russell, B. D., Coleman, M. A., Kelaher, B. P., and Connell, S. D. (2021). Long-term thermal acclimation drives adaptive physiological adjustments of a marine gastropod to reduce sensitivity to climate change. Sci. Total Environ. 771, 145–208. doi: 10.1016/j.scitotenv.2021.145208
Lewis, E., and Wallace, D. (1998). Program Developed for CO2 System Calculations. ORNL/CDIAC-105. Oak Ridge, TN: Oak Ridge National Laboratory.
Maboloc, E. A., and Mingoa-Licuanan, S. S. (2013). Spawning in Trochus maculatus: field observations from Bolinao, Pangasinan (Philippines). Coral Reefs 32, 1141. doi: 10.1007/s00338-013-1060-y
Magozzi, S., and Calosi, P. (2015). Integrating metabolic performance, thermal tolerance, and plasticity enables for more accurate predictions on species vulnerability to acute and chronic effects of global warming. Glob. Chang. Biol. 21, 181–194. doi: 10.1111/gcb.12695
Manríquez, P. H., González, C. P., Brokordt, K., Pereira, L., Torres, R., Lattuca, M. E., et al. (2019). Ocean warming and acidification pose synergistic limits to the thermal niche of an economically important echinoderm. Sci. Total Environ. 693, 133469. doi: 10.1016/j.scitotenv.2019.07.275
Marchant, H. K., Calosi, P., and Spicer, J. I. (2010). Short-term exposure to hypercapnia does not compromise feeding, acid-base balance or respiration of Patella vulgata but surprisingly is accompanied by radula damage. J. Mar. Biol. Assoc. U.K. 90, 1379–1384. doi: 10.1017/S0025315410000457
Marshall, D. J., Dong, Y., McQuaid, C. D., and Williams, G. A. (2011). Thermal adaptation in the intertidal snail Echinolittorina malaccana contradicts current theory by revealing the crucial roles of resting metabolism. J. Exp. Biol. 214, 3649–3657. doi: 10.1242/jeb.059899
Mehrbach, C., Culberson, C. H., Hawley, J. E., and Pytkowicx, R. M. (1973). Measurement of the apparent dissociation constants of carbonic acid in seawater at atmospheric pressure. Limnol. Oceanogr. 18, 879–907. doi: 10.4319/lo.1973.18.6.0897
Mertens, N. L., Russell, B. D., and Connell, S. D. (2015). Escaping herbivory: ocean warming as a refuge for primary producers where consumer metabolism and consumption cannot pursue. Oecologia 179, 1223–1229. doi: 10.1007/s00442-015-3438-8
Metzger, R., Sartoris, F. J., Langenbuch, M., and Pörtner, H. O. (2007). Influence of elevated CO2 concentrations on thermal tolerance of the edible crab Cancer pagurus. J. Therm. Biol. 32, 144–151. doi: 10.1016/j.jtherbio.2007.01.010
Ng, T. P. T., Lau, S. L. Y., Seuront, L., Davies, M. S., Stafford, R., Marshall, D. J., et al. (2017). Linking behaviour and climate change in intertidal ectotherms: insights from littorinid snails. J. Exp. Mar. Biol. Ecol. 492, 121–131. doi: 10.1016/j.jembe.2017.01.023
Nguyen, K. D. T., Morley, S. A., Lai, C.-H., Clark, M. S., Tan, K. S., Bates, A. E., et al. (2011). Upper temperature limits of tropical marine ectotherms: global warming implications. PLoS One 6:e29340. doi: 10.1371/journal.pone.0029340
Nudelman, F., Gotliv, B. A., Addadi, L., and Weiner, S. (2006). Mollusk shell formation: mapping the distribution of organic matrix components underlying a single aragonitic tablet in nacre. J. Struct. Biol. 153, 176–187. doi: 10.1016/j.jsb.2005.09.009
Pansch, C., Scotti, M., Barboza, F. R., Al-Janabi, B., Brakel, J., Briski, E., et al. (2018). Heat waves and their significance for a temperate benthic community: a near-natural experimental approach. Glob. Chang. Biol. 24, 4357–4367. doi: 10.1111/gcb.14282
Parmesan, C., and Yohe, G. (2003). A globally coherent fingerprint of climate change impacts across natural systems. Nature 421, 37–42. doi: 10.1038/nature01286
Pörtner, H. O. (2008). Ecosystem effects of ocean acidification in times of ocean warming: a physiologist’s view. Mar. Ecol. Prog. Ser. 373, 203–217. doi: 10.3354/meps07768
Pörtner, H. O. (2010). Oxygen- And capacity-limitation of thermal tolerance: a matrix for integrating climate-related stressor effects in marine ecosystems. J. Exp. Biol. 213, 881–893. doi: 10.1242/jeb.037523
Pörtner, H. O., and Farrell, A. P. (2008). Ecology: physiology and climate change. Science 322, 690–692. doi: 10.1126/science.1163156
Sandblom, E., Clark, T. D., Gräns, A., Ekström, A., Brijs, J., Sundström, L. F., et al. (2016). Physiological constraints to climate warming in fish follow principles of plastic floors and concrete ceilings. Nat. Commun. 7:11447. doi: 10.1038/ncomms11447
Seebacher, F., White, C. R., and Franklin, C. E. (2014). Physiological plasticity increases resilience of ectothermic animals to climate change. Nat. Clim. Chang. 5, 61–66. doi: 10.1038/nclimate2457
Sinclair, B. J., Marshall, K. E., Sewell, M. A., Levesque, D. L., Willett, C. S., Slotsbo, S., et al. (2016). Can we predict ectotherm responses to climate change using thermal performance curves and body temperatures? Ecol. Lett. 19, 1372–1385. doi: 10.1111/ele.12686
Stillman, J. H., and Somero, G. N. (2000). A comparative analysis of the upper thermal tolerance limits of eastern pacific porcelain crabs, genus Petrolisthes: influences of latitude, vertical zonation, acclimation, and phylogeny. Physiol. Biochem. Zool. 73, 200–208. doi: 10.1086/316738
Tripp-Valdez, M. A., Bock, C., Lucassen, M., Lluch-Cota, S. E., Sicard, M. T., Lannig, G., et al. (2017). Metabolic response and thermal tolerance of green abalone juveniles (Haliotis fulgens: Gastropoda) under acute hypoxia and hypercapnia. J. Exp. Mar. Bio. Ecol. 497, 11–18. doi: 10.1016/j.jembe.2017.09.002
Uthicke, S., Liddy, M., Nguyen, H. D., and Byrne, M. (2014). Interactive effects of near-future temperature increase and ocean acidification on physiology and gonad development in adult Pacific sea urchin, Echinometra sp. A. Coral Reefs 33, 831–845. doi: 10.1007/s00338-014-1165-y
Keywords: thermal physiology, ocean warming, ocean acidification, metabolic function, physiological plasticity, acclimation, marine gastropod
Citation: Minuti JJ, Corra CA, Helmuth BS and Russell BD (2021) Increased Thermal Sensitivity of a Tropical Marine Gastropod Under Combined CO2 and Temperature Stress. Front. Mar. Sci. 8:643377. doi: 10.3389/fmars.2021.643377
Received: 18 December 2020; Accepted: 26 February 2021;
Published: 19 March 2021.
Edited by:
Peng Jin, University of Guangzhou, ChinaReviewed by:
Xiutang Yuan, Chinese Academy of Sciences (CAS), ChinaWei Li, Huangshan University, China
Copyright © 2021 Minuti, Corra, Helmuth and Russell. This is an open-access article distributed under the terms of the Creative Commons Attribution License (CC BY). The use, distribution or reproduction in other forums is permitted, provided the original author(s) and the copyright owner(s) are credited and that the original publication in this journal is cited, in accordance with accepted academic practice. No use, distribution or reproduction is permitted which does not comply with these terms.
*Correspondence: Bayden D. Russell, YnJ1c3NlbGxAaGt1Lmhr