- 1State Key Laboratory of Estuarine and Coastal Research, East China Normal University, Shanghai, China
- 2Laboratory of Synthetic Biology, Key Laboratory of Advanced Drug Preparation Technologies, Ministry of Education, School of Pharmaceutical Sciences, Zhengzhou University, Zhengzhou, China
- 3Biogeochemistry Research Group, School of Natural Sciences, Trinity College Dublin, Dublin, Ireland
- 4Instituto de Investigacións Mariñas, Consejo Superior de Investigaciones Científicas (IIM-CSIC), Vigo, Spain
- 5School of Oceanography, Shanghai Jiao Tong University, Shanghai, China
Supply of bio-labile dissolved organic matter (DOM) has been assumed to be a key factor for the intensity of nitrate (NO3–) removal in permeable coastal sediments. In the present study, a series of flow through reactor experiments were conducted using glucose as a N-free bio-labile DOM source to permeable sediments from a sandy beach seepage face to identify its effect on benthic NO3– removal. The results revealed a shift from the dominance of NO3– production to removal processes when NO3– input concentration increased from 10 to 80 μM under oxic conditions. Sediment microbiota information suggests that nitrification (e.g., Nitrosomonas and Nitrososphaera) and denitrification (e.g., Marinobacter and Bacillus) were dominant pathways for benthic NO3– production and removal in the studied sediment. Compared with the active response of sediment microbiota to NO3– additions, the supply of glucose (approximately 300 μM final concentration added) did not significantly change the NO3– removal efficiency under aerobic conditions (dissolved oxygen saturation approximately 100%). Similarly, an insignificant increase of NO3– removal rate after glucose amendment of the circulating water was obtained when dissolved oxygen (DO) saturation decreased to approximately 70% in the input solution. When DO at the input solution was decreased to 30% saturation (sub-oxic conditions), the removal rate of NO3– in the group amended with glucose increased, suggesting that glucose stimulated denitrifiers. These results revealed that NO3– removal relied mainly on the anaerobic environment at particle surfaces, with a dependence on the sedimentary organic matter as an electron supplier under bulk aerobic conditions, while the bio-labile DOM was consumed mainly by aerobic respiration instead of stimulating NO3– reduction. However, the respiration triggered by the over-supply of bio-labile DOM reduced the DO in the porewater, likely depressing the activity of aerobic reactions in the permeable sediment. At this point, the benthic microbiota, especially potential denitrifiers, shifted to anaerobic reactions as the key to support nitrogen metabolism. The glucose amendment benefited NO3– reduction at this point, under sub-oxic conditions.
Introduction
Coastal permeable sediments, mainly composed by coarse silicate or carbonate particles with a permeability >10–12 m2, cover more than 70% of global coastal areas (Huettel et al., 2014). In this permeable environment, advection is the dominant pathway for the transport and dispersion of solutes in porewater (Jiang et al., 2021a), and feeds a wide range of biogeochemical reactions (Huettel et al., 2014; Mouret et al., 2020). Bioavailable nitrogen (N) has been intensively introduced into terrestrial ecosystems via different anthropogenic activities. The indiscriminate use of fertilizers, manure and waste sludge in land together with industrial spillages has led to the widespread accumulation of nitrate (NO3–) in coastal groundwater on a global scale (Rivett et al., 2008). Coupled with the transport of groundwater from aquifers to coastal oceans driven by hydraulic gradients, a significant portion of land-derived NO3– reaches permeable coastal sediments in subterranean estuaries (STEs; defined as the underground mixing zone between continental groundwater and infiltrating seawater; Moore, 1999) prior to discharge to the coast (Jung et al., 2020; Ibánhez et al., 2021a). High groundwater-borne NO3– loadings frequently trigger a series of negative feedbacks on coastal ecosystems such as blooms of toxic phytoplankton, accumulation of organic matter, and oxygen depletion (Troccoli-Ghinaglia et al., 2010; Amato et al., 2016; Rocha et al., 2016).
As active reactors in coastal belts, permeable sediments have a strong capability to modulate NO3– mass transport via a wide range of biogeochemical reactions, such as autotrophic nitrification, microbial assimilation, and heterotrophic denitrification (Marchant et al., 2014; Kuypers et al., 2018; Ahmerkamp et al., 2020; Jiang et al., 2021c). The main pathway for NO3– removal in permeable coastal sediments is frequently identified as denitrification (Ibánhez et al., 2013; Marchant et al., 2014; Ibánhez and Rocha, 2017). Denitrification rates in permeable sediments are deemed to be deeply influenced by environmental factors, including the supply of organic matter, dissolved oxygen (DO) concentration, and advection rate, etc. (Santos et al., 2012; Ibánhez and Rocha, 2017; Ahmerkamp et al., 2020). As a heterotrophic microbial pathway, organic matter availability has widely been acknowledged to be a key factor for benthic denitrification (Crawshaw et al., 2019). In intertidal permeable sediments, the organic matter inventory includes both dissolved organic matter (DOM) and sedimentary organic matter (SOM). There, DOM shows high mobility due to seawater intrusion caused by tidal pumping, wave setup and temperature-driven porewater movement (Suryaputra et al., 2015; Meredith et al., 2020), while the SOM concentration is frequently related to adsorption and retention of particulate organic matter near the infiltration zones (Jiang et al., 2020). Both DOM and SOM can feed carbon-dependent reactions (Weston and Joye, 2005). In terms of denitrification, previous research outcomes frequently assumed that bio-labile DOM would control sediment/soil denitrification capability according to the requirement of electron transfer from carbon compounds (Cornwell et al., 1999; Bernhardt and Likens, 2002; Surey et al., 2020). However, permeable sediments are frequently enriched in DO by porewater exchange with DO saturated seawater and DO diffusion from the atmosphere (Berg et al., 2013; Ibánhez and Rocha, 2016). The bio-labile DOM might be consumed in aerobic mineralization prior to be available for denitrification (Jiang et al., 2018), suggesting a possible spatial isolation between bio-labile DOM enrichment and denitrifiers (e.g., spatial mismatch in coastal aquifers; Siemens et al., 2003). In addition, DO availability might directly hamper the denitrifying bacterial activity, leading to depression of denitrification process rates under oxic conditions (Evard et al., 2012). Alternatively, the consumption of bio-labile DOM in aerobic reactions triggers the decline of DO in porewater (Heiss et al., 2020), creating sub-oxic or anaerobic environments that are suitable for benthic denitrifiers along the porewater transport path (Kessler et al., 2012; Heiss et al., 2020). Nevertheless, our understanding of the interrelations between bio-labile DOM and DO supply and denitrification, i.e., the dominant pathway of NO3– removal, in coastal sands is still limited and hinders our understanding of NO3– cycling in coastal permeable environments and the resulting NO3– fluxes across the land-ocean boundary.
Targeting this knowledge gap, in the present study, a series of laboratory simulations were conducted using flow through reactor (FTR) cells to mimic the dominant advective transport pathways of coastal sands. Glucose was added to the pumped solution as a source of bio-labile DOM to sediment microbes. In addition, the DO concentration in the input solution was adjusted by injecting nitrogen gas. Rates of NO3– removal and production were evaluated under different levels of glucose and DO availability. The main hypothesis of the present study was that glucose amendment could significantly stimulate NO3– removal rates. The research aim was to test the hypothesis and evaluate the possible mechanisms underlying observed reaction patterns.
Materials and Methods
Sample Collection
Permeable coastal sediments were collected from a sandy beach located at the inner part of Sanggou Bay (China). The porosity of the beach sediment was approximately 0.31 and the mean hydraulic conductivity of the sampled sandy sediments was 7.5 × 10–5 m s–1. There, the outflow of low salinity porewater into the bay was verified during ebb tide (Jiang et al., 2020). This brackish water discharge is associated to continental groundwater inputs that also trigger the porewater enrichment in radium isotopes and nutrients (Wang et al., 2014). Around Sanggou Bay, agriculture activities, mainly focused on apples, corn and wheat are intensively developed. These activities lead to a significant accumulation of NO3– in the terrestrial groundwaters surrounding the bay, with concentrations >350 μM in coastal wells (Wang et al., 2014). This continental groundwater enriched with NO3– reaches the sampled seepage face, leading to a variation of NO3– concentration from 20 to 60 μM in porewater. DOC concentrations locally vary between 180 and 390 μM, while the P concentration in the porewater was much lower (below 2 μM) due to adsorption on sandy particles (Wang et al., 2014). Apart from agriculture, the local economy is also supported by intensive marine culture activities conducted in the bay (Fang et al., 2016). High-biomass primary producers, such as diatoms and kelp, introduce a significant amount of pelagic DOM into the bay water via basic metabolism (e.g., secretion), diffusion across the cell membrane and decomposition (Thornton, 2014; Mahmood et al., 2017). This pelagic DOM is injected into the seepage face with seawater infiltration, creating a local mixing zone between bio-labile DOM and terrestrial NO3–. Sediment cores (20 cm depth, 10 cm diameter) were collected in the seepage face during ebb tide in April (spring) and October (autumn) 2018. Sediment at the sampling site presented a large accumulation of bivalve debris at the surface originated from the intense bivalve aquaculture in the area. This surface layer of bivalve debris (typically 0–1 cm depth) was removed before the collection of sediment cores.
Laboratory FTR Simulation
Flow through reactor cells were used to mimic in situ advective conditions and thus explore benthic reactions in the seepage face (Figure 1). FTR cells of 10 cm length and approximately 8 cm of diameter were used (total volume for sediments: 500 cm3). These include two collimators and fine meshes (pore size: 30 μm) to ensure an even transversal distribution of the pumped solutions through the incubated sediments. After collection, the sediments were gently repacked into the reactor cells after removing large pieces of debris and the inner walls of the cells were scrubbed to increase the contact between sediment particles and the containers and hence to eliminate the build-up of preferential flow paths along the walls. Aluminum foil was wrapped around each cell to eliminate the influence of light exposure on benthic microbial activity or primary producers. After setting up, all FTR cells were placed into a thermo-constant incubator at 20°C, which was comparable to the porewater temperature during both sampled seasons (Jiang et al., 2020). FTR cells were then flushed with seawater overnight (approximately 10 h) at a constant rate of 3 mL min–1 (discharge rate as 11.5 cm h–1), equivalent to the advection rate quantified in the seepage face during active seepage (Jiang et al., 2020). With these conditions, porewater retention time was calculated as the quotient of system flow rate and total porewater volume (product of total sediment volume and porosity) in a cell (approximately 52 min). Afterward, the flushed sediment from three reactors was kept for further physico-chemical analyses.
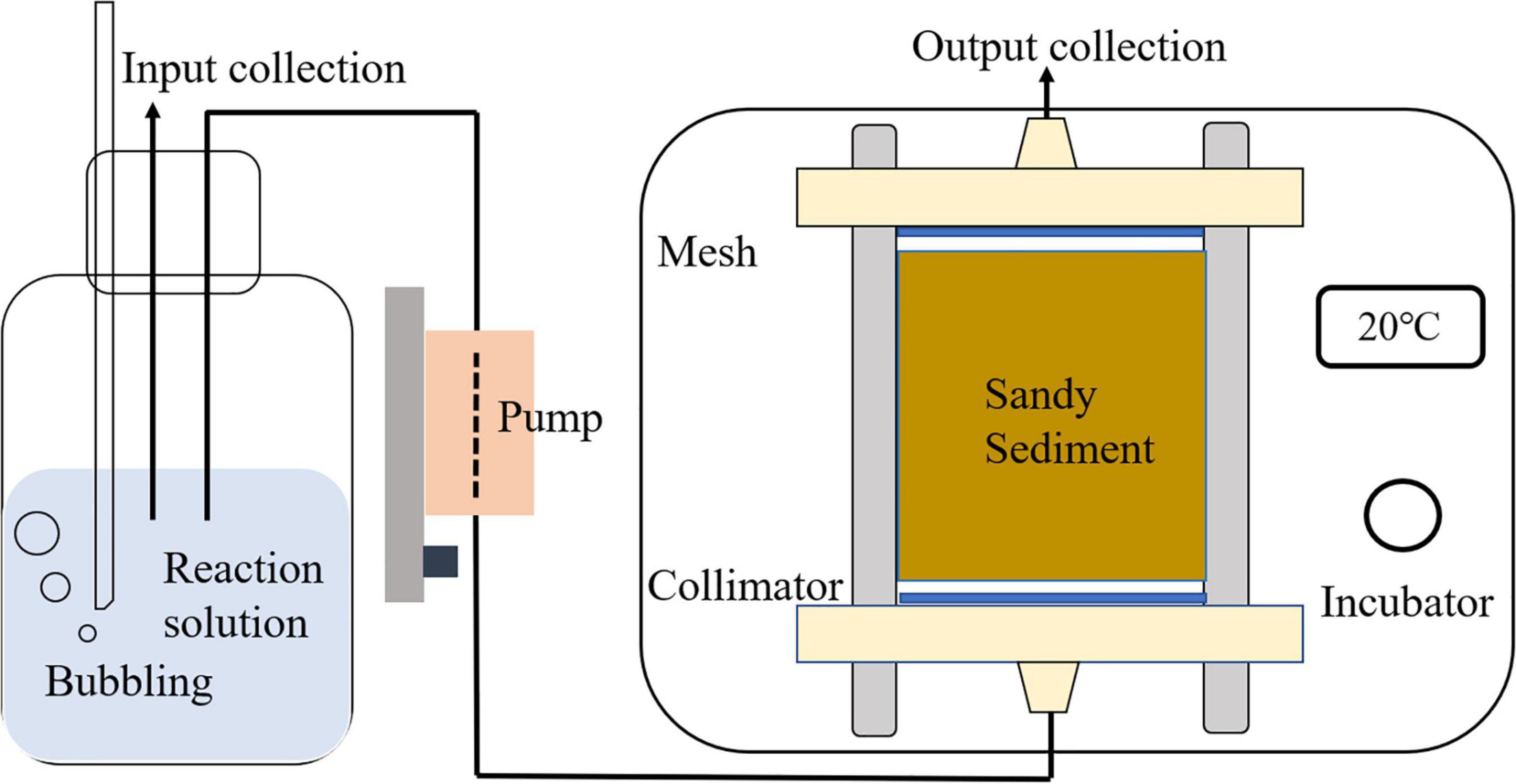
Figure 1. Sketch of flow through reactor (FTR) experiments established in the laboratory. This set highlights the injection of nitrogen gas into the reaction liquid to regulate the oxygen content level. This sketch is modified from Jiang et al. (2018).
After conditioning of the FTR cells, the working solutions were pumped through the test reactor cells using a high-accuracy peristaltic pump (BT-100, Longer®, China) at the same pumping rate as that used during the flushing period. The series of sediment incubations included seven groups (one control and six treatments) with different levels of NO3–, dissolved organic carbon (DOC) and DO in the input solution, as outlined in Table 1. The carrier solution used was aged seawater (storage > 100 days) with a salinity of 33 to maintain strict control on the labile DOM content of the circulating porewater. In the six treatments, NO3– was kept constant (∼80 μM), while DOC concentrations ranged from ca. 140 (i.e., without amendment) to 480 μM via the addition of glucose. Additionally, three different DO levels in the input solution were employed (Table 1). In the DO saturation group (approximately 240 μM), the working solution was bubbled with air before the incubation. For the DO unsaturation groups (70% saturation: nearly 170 μM), nitrogen gas (99.999% purity) was bubbled into the working solution from the bottom of the container at a constant rate for the partial removal of DO. For groups with 30% DO saturation (nearly 75 μM), the input solution was treated in an ultrasonic bath for 3 min prior to the start of the bubbling process. For each treatment, triplicate reactor cells were used. During the experiments, the complete replacement of the porewater inside the FTR cells was determined by dye tracer release-balance (input concentration was identical to output concentration), and it was complete after approximately 3 h at the settled pumping rate (3 mL min–1) based on the input-output balancing experiments using Rhodamine tracer (the input and output balance displayed in Supplementary Figure 1). Accordingly, sampling of the input and outflow solutions from each reactor took place after pumping the working solution for 6 h to guarantee solute dispersion in sediments (Figure 1). In each reactor, four samplings were conducted at 1 h intervals. The collected samples were filtered with Millipore syringe filters (0.22 μm pore size) and stored in acid prewashed plastic bottles for dissolved inorganic nitrogen (DIN) species and pre-combusted glass vials for DOC and chromophoric dissolved organic matter (CDOM) determinations and kept at −20°C prior to laboratory analyses to avoid potential microbial degradation of the samples (Jiang et al., 2017). Besides the collection of water samples, DO concentrations at both the input solution and the outflow stream were directly measured with a portable probe (WTW Multi 3630). Reaction rates for DIN species, DOC and DO were calculated from the difference in concentration between the input and the output solutions, porewater residence time (52 min) and the sediment volume contained in the FTR cell (500 cm–3) according to Ibánhez and Rocha (2014). Negative reaction rates indicate consumption of the target solute within the sediment, while positive values represent benthic production.
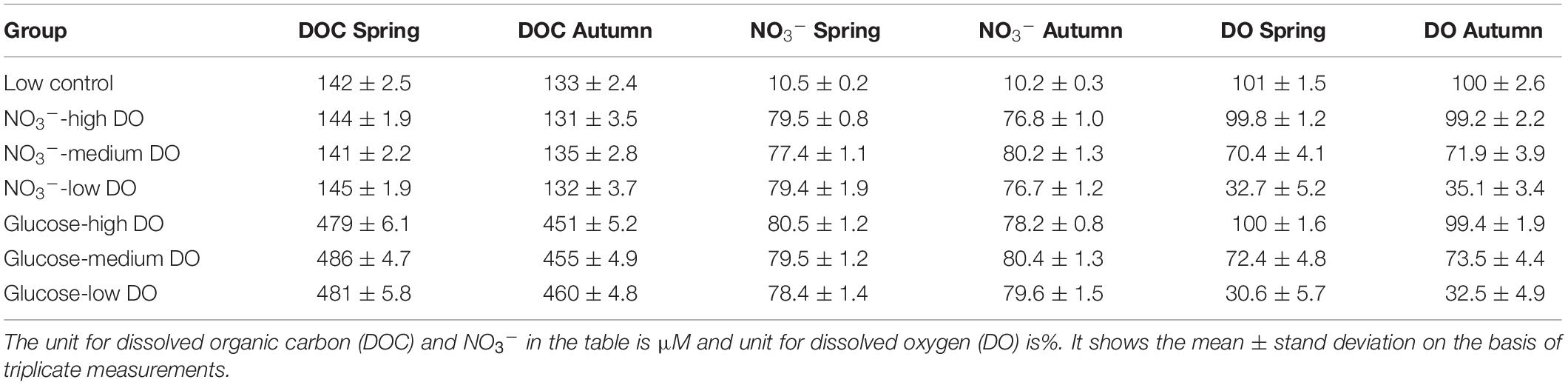
Table 1. Initial concentrations of DOC, NO3–, and DO in each batch of the flow through reactor (FTR) incubation experiment.
Sediment Physico-Chemical Analyses
Total organic carbon (TOC) content in the sediment samples was determined via a CHNOS Elementar cube (Vario EL III) after removing inorganic fractions using 2 M hydrochloric acid (Wu et al., 2019). Sediment total nitrogen (TN) concentration was measured with the same equipment without the acid pretreatment. The equipment accuracy for TOC and TN determinations in sandy sediments was approximately 5%. Exchangeable DIN, DOC, and CDOM from sediment particles (approximately 4 g dry weight) was extracted with a 0.5 M potassium chloride (KCl, approximately 40 mL) solution for 12 h under constant agitation, with a rotation rate of 200 rpm (20°C). Subsequently, the supernatant was filtered with syringe filters and kept at −20°C until analysis. Sediment grain size was measured using a Coulter LS 100Q particle analyzer (Coulter Company, United States). The particle size <2 μm was defined as clay, 2–63 μm as silt, and >63 μm as sandy grains (Wu et al., 2019).
Sediment samples from both sampled seasons were also used in a series of adsorption experiments to quantitively determine the influence of adsorption on glucose and NO3– concentrations under aerobic conditions. In particular, the sediment used in the adsorption was autoclaved for 30 min at 121°C to eliminate the influence of sediment prokaryotes (Jiang et al., 2018). After gently washing with filtered seawater, the autoclaved sediments (10 g) were mixed with aged seawater (40 mL) spiked with NO3– (final concentration: 80 μM) and glucose (final DOC concentration: 480 μM), respectively. The adsorption experiments covered incubation periods ranging from 0.5 to 12 h. The spiked solutions before and after adsorption experiments were collected and stored for laboratory analyses. The adsorbed quantity of glucose and NO3– was calculated from the solute concentration difference in these two solutions divided by the sediment dry weight (Ibánhez and Rocha, 2017).
Sediment Microbial Analyses
Total DNA from approximately 0.30 g of sediment was extracted using the DNeasy PowerSoil Kit (Qiagen, Germany). Primers for the V3–V4 regions for 16S rDNA were designated to identify microbial diversity (Wei et al., 2021). The 16S rDNA gene amplicon library from PCR cycling was constructed with the KAPA Hyper Prep Kit (Roche). The built library was paired-end sequenced on an Illumina Miseq system (Illumina). The raw reads have been deposited in the NCBI database. The diversity indices from the spring and autumn samples, including the Shannon index and Equitability, were analyzed with USEARCH alpha_div (Edgar, 2010).
Water Analyses
Dissolved inorganic nitrogen species (NH4+, NO2–, and NO3–) in the samples collected from the FTR experiments, the solutions for extraction of the sediment exchangeable compounds and adsorption experiments (only NO3–) were quantified using a flow injection system (SAN++ plus, SKALAR Analytical B.V., Netherlands). DOC concentrations in these water samples were analyzed by high-temperature catalytic combustion using a Shimadzu TOC-LCPH TOC analyzer (TOC-LCPH, Shimadzu, Japan) with an ASI-L autosampler (Jiang et al., 2020). CDOM of the collected water samples was analyzed on a dual channel spectrophotometer (PERSEE®, China, TU-1901) with ultrapure water as blank (water resistivity: 18.2 MΩ cm). After equipment stabilization, CDOM samples were continuously scanned from 400 to 250 nm on the spectrophotometer with 1 nm intervals. CDOM content in the tested water samples was estimated by the absorption coefficient at 355 nm (a355, Zhang et al., 2007). In addition, the spectral slope from 275 to 295 nm was determined as a proxy of the molecular weight of CDOM (S275–295; terrestrial materials frequently show small slopes, while pelagic materials tend to show larger slopes; Zhang et al., 2007). The isotopic composition of NO3– (δ15N/18O–NO3–) was determined in the collected samples to understand reaction pathways and rates in place (Jiang et al., 2019, 2021b). The isotopic ratios were measured by the bacterial reduction method (Sigman et al., 2001) using a Thermo-Fisher Delta V advantage analyzer coupled with a PreCon system (more details in Jin et al., 2020). The method reproductivity was approximately 0.2‰ for δ15N–NO3– and 0.5‰ for δ18O–NO3–.
Statistical Analyses
To explore the effect of glucose supply on NO3– removal rate under different DO saturations, a series of one-way ANOVAs and Tukey Honestly Significant Difference (HSD) test were performed with the Minitab software (version 17.0) with the statistical significance as p < 0.05. Linear correlation between variables was also explored with Minitab.
Results
Sediment Physico-Chemical Factors and Variability
During the two sampled periods, the mean grain size of the collected sediment, mostly composed by sand, was highly similar (204 μm mean grain size in spring to 199 μm mean grain size during autumn, Table 2). The grain skewness was 0.23 in spring and 0.31 in autumn and the kurtosis was ∼2.4 during both seasons. Sediment TOC concentration, determined before the use of the working solutions in the FTR experiments, was 92.5 μmol g–1 during spring, and decreased to 72.4 μmol g–1 in autumn (Supplementary Figure 2). TN content was 7.2 and 5.9 μmol g–1 during spring and autumn, respectively. No significant variations of TOC and TN were observed among treatments after the FTR incubations (Figures 2A,B). Compared with TN content, concentrations of exchangeable DIN species on sediment particles were much lower (Table 2). In addition, the FTR incubations slightly decreased the exchangeable NH4+ concentration in the control group as well as DO saturation and 70% saturation treatments during autumn, while the exchangeable NH4+ content increased in all the FTR groups containing NO3– amended circulating water during spring (groups B–D, Figure 2C). Compared with extracted NH4+, the exchangeable NO3– (E–NO3–) content was 3–4 times lower (Supplementary Figure 2). During spring, the offset in E–NO3– became smaller as DO increased, while a reverse trend was found in autumn (Figure 2D). As a minor contributor to the DIN pool, extracted NO2– concentrations were <1 nmol g–1 (Supplementary Figure 2).
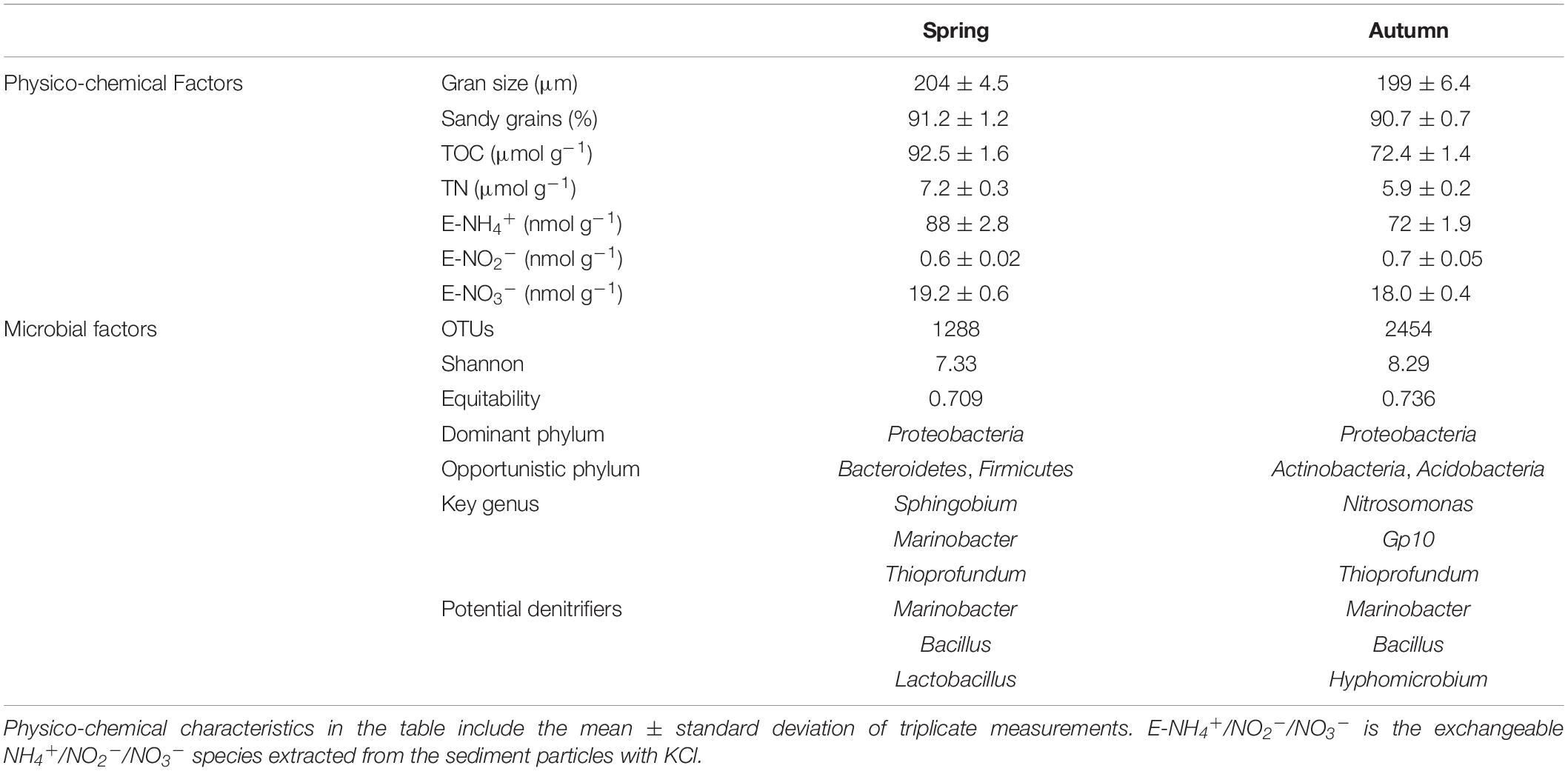
Table 2. Sediment physico-chemical characteristics and microbial indices determined during spring and autumn.
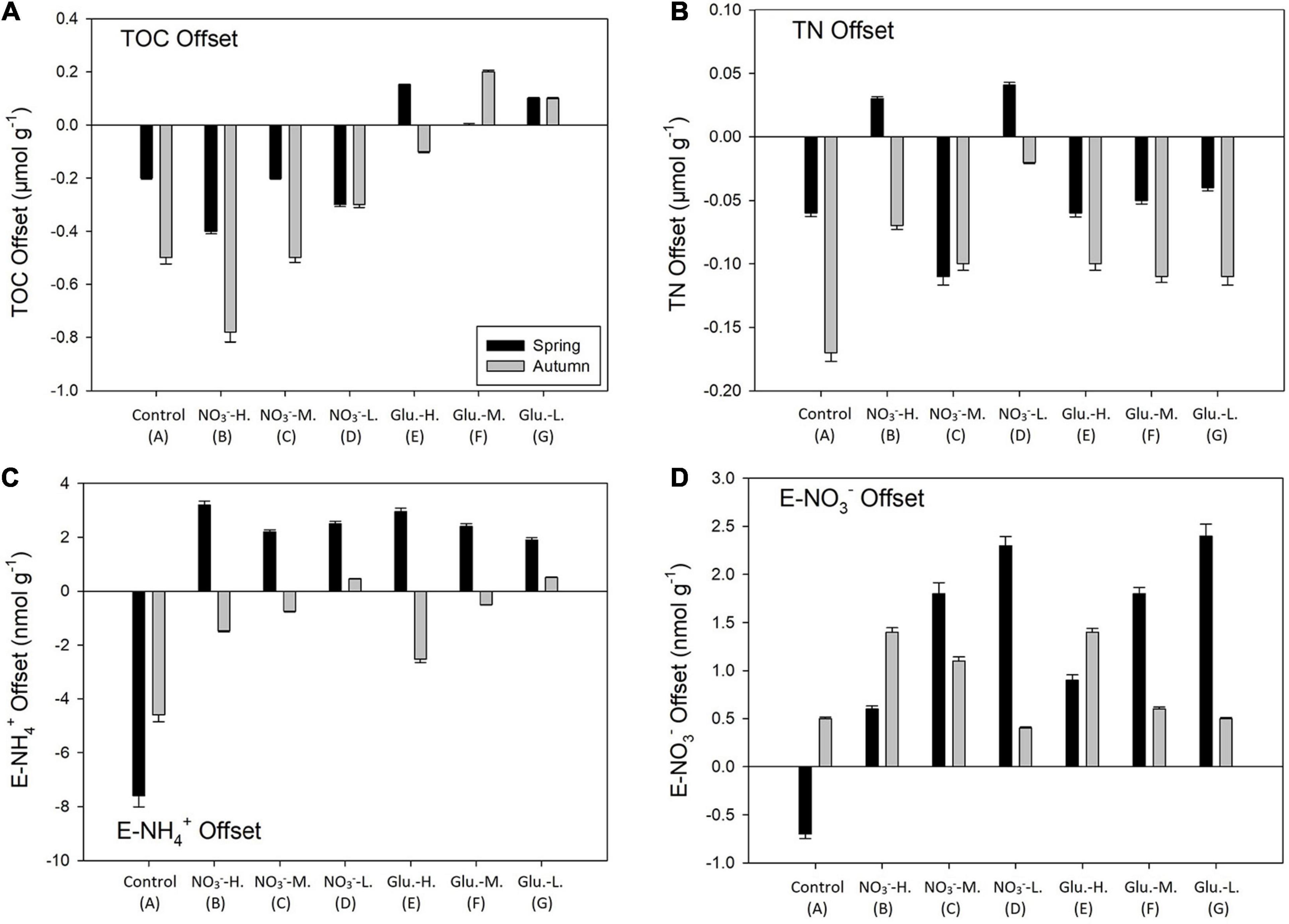
Figure 2. The offset of sediment total organic carbon (TOC) (A), TN (B), exchangeable NH4+ (E-NH4+, C) and exchangeable NO3– (E-NO3–, D) before and after a series of incubations during spring and autumn. Positive values indicate solute concentration increases after incubation and negative is concentration decreases.
Solute Adsorption and Heterogeneous Equilibrium in the Studied Sediments
During spring, the adsorbed glucose in the tested sediment was ∼0.03 μmol glucose g–1 after 0.5 h incubation regardless of the adsorption of other DOC solutes (Supplementary Figure 2). The adsorbed amount logarithmically increased when the incubation time prolonged, reaching 0.14 μmol glucose g–1 after 12 h. During autumn, the sediment still retained glucose from the reaction solution via adsorption, similarly to the pattern observed during spring. The NO3– content remained relatively constant after 4 h of incubation in spite of the weak NO3– adsorption capacity shown by the sediments (Supplementary Figure 2), indicating that adsorption was fast.
Dissolved Oxygen and DOM Variation
The DO content in the working solutions was set at three different levels. In the DO saturated group, the DO concentration at the input solution was approximately 230 μM (Supplementary Figure 3). After flowing through the sediments, DO concentrations decreased (Supplementary Figure 3). In the control group, the outflow stream DO content decreased approximately 30 μM DO, implying benthic respiration rates of 12.9 and 11.6 nmol g–1 h–1 during spring and autumn, respectively. Introducing NO3– did not significantly change the DO consumption rate (group B), while the DO consumption was negatively influenced by the decreasing DO concentration in the input solution (groups C and D). The amendment of the circulating solution with glucose significantly increased DO consumption rates (group E; p < 0.01) while a decay in benthic respiration rates at suboxic conditions (groups F and G) was observed for both seasons. Different from DO, the sediment acted as a net source of DOC in the control group, especially during spring (Figure 3B). The addition of NO3– enhanced the DOC production rate (group B), while decreasing DO in the input solution slightly reduced DOC production rates. In contrast, glucose addition to the circulating solution completely changed the sediment mediation of DOC fluxes into a strong DOC sink due to the rapid consumption of glucose. For CDOM (a355), sediments in all groups showed a production trend (Figure 3C), especially at the group B during both seasons (DO saturation, spiked with NO3–). After flowing through the sediment, S275–295, the index of DOM structure, increased in all groups, revealing increases of small-molecular DOM in the porewater via benthic reactions. The most active addition still occurred at group B during both seasons, while the slowest addition was found at the groups run under suboxic conditions (Figure 3D).
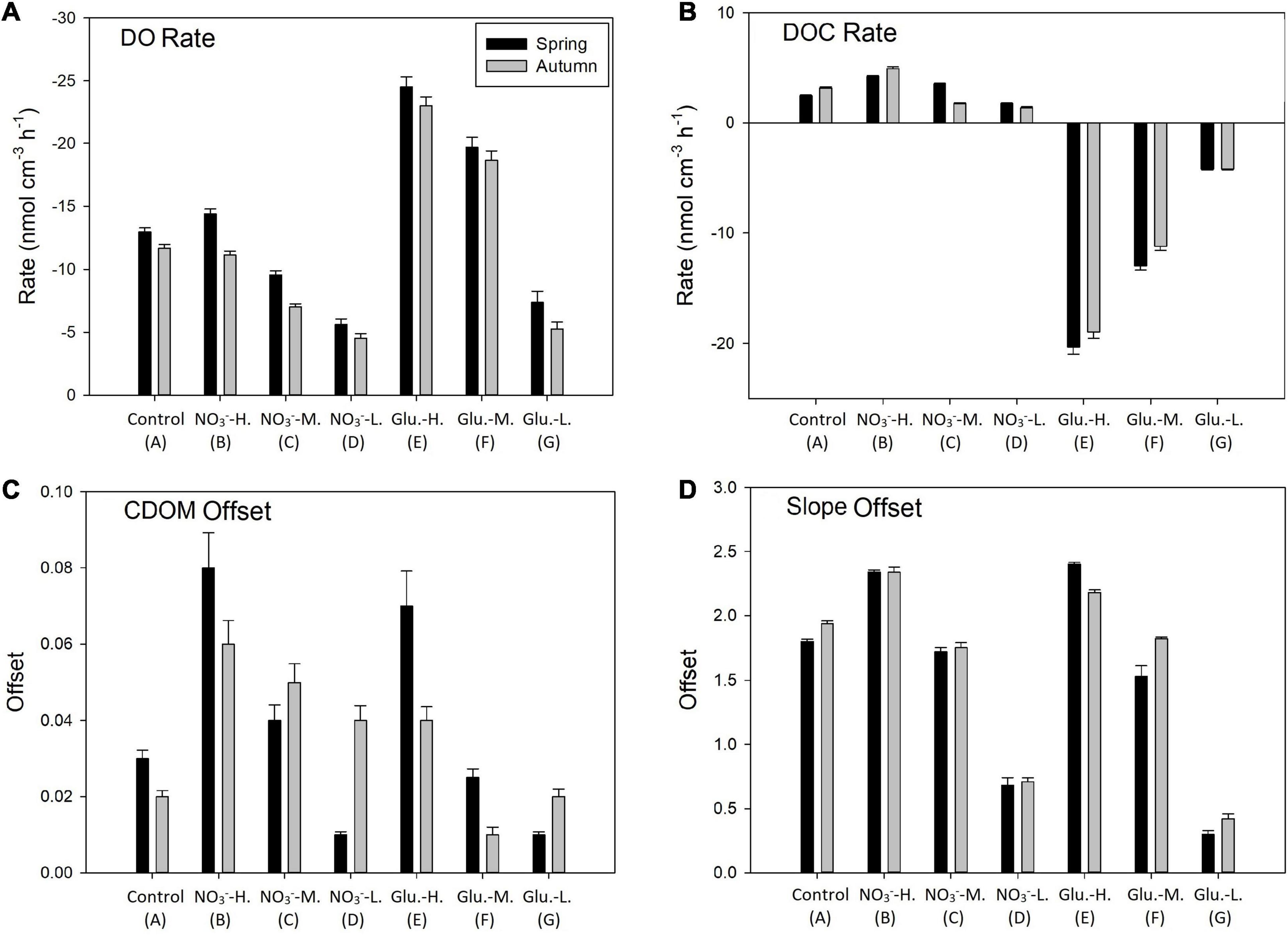
Figure 3. Reaction rates for dissolved oxygen (DO) (A) and dissolved organic carbon (DOC) (B) during spring and autumn, as well as offsets for chromophoric dissolved organic matter (CDOM) characters (C for a355; D for S275–295) before and after FTR incubations during both seasons. Positive values indicate production/offset enhancement after incubation and negative is removal/offset decrease.
Dissolved Inorganic Nitrogen Species and NO3– Isotopes
Similarly, the sediment acted as a net source of NH4+ in the control group during both seasons (Figure 4A). The amendment of NO3– decreased the NH4+ production rate during spring, although the sediment still acted as a net NH4+ source (group B). The NH4+ production was stimulated by NO3– addition during autumn. In contrast, the decrease of DO concentration in the input solution significantly decreased the NH4+ production rate during both seasons (p = 0.01), especially after the concomitant amendment with glucose (group G). Compared with NH4+, NO2– concentrations in both input and output solutions were much lower, ranging from 0.31 to 0.47 μM (Supplementary Figure 4). Such low concentrations led to minor variations among groups (Figure 4B).
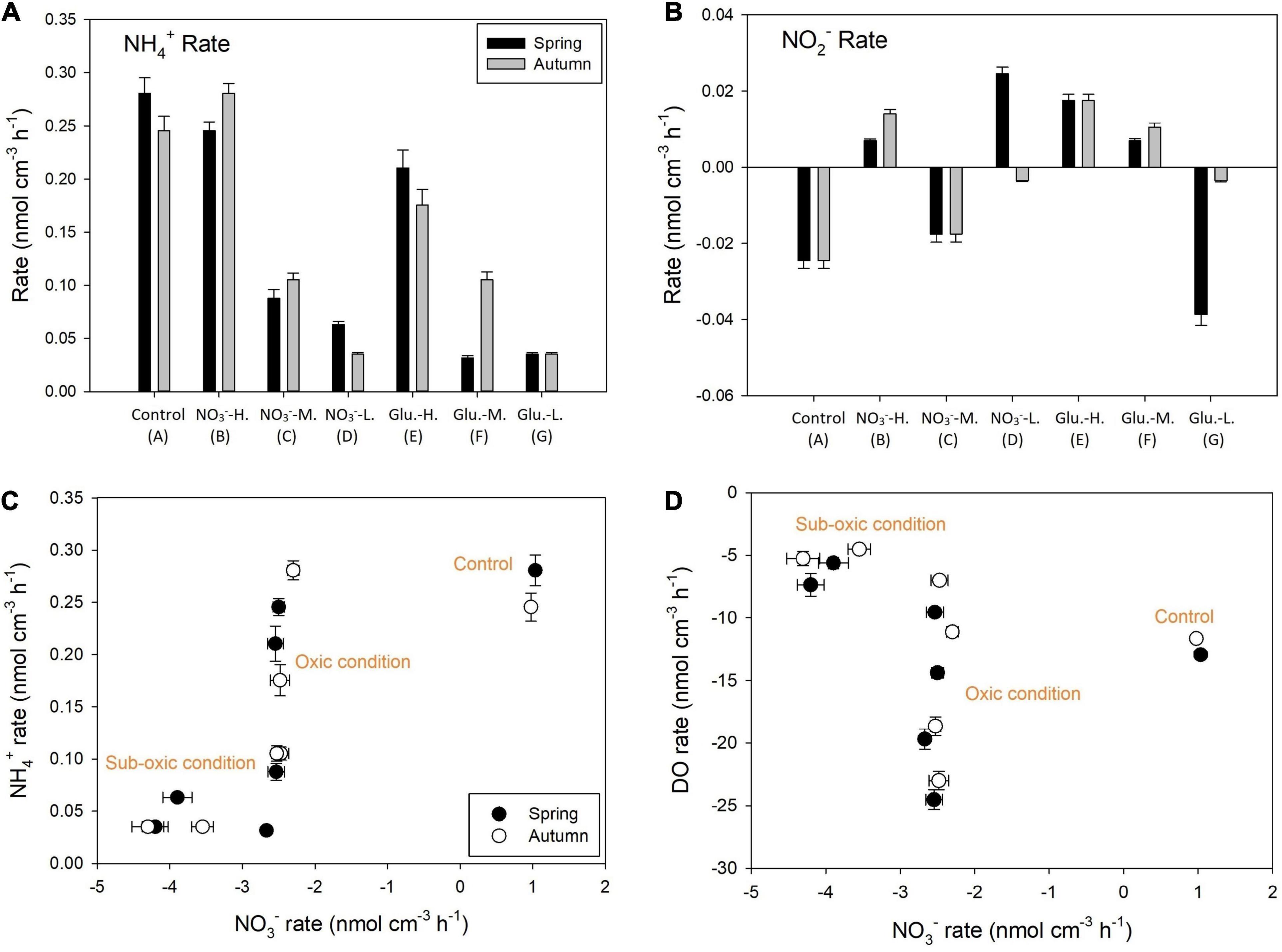
Figure 4. Reaction rates of NH4+ (A) and NO2– (B) during spring and autumn. Positive rates indicate production after incubation and negative is removal. It also shows the correlation between NH4+ and NO3– reaction rates (C) and DO and NO3– reaction rates (D).
Different to the mediation of NO2– fluxes, the sediment acted as a net source of NO3– in the control group during both seasons (Figure 5A), leading to a decrease in both δ15N–NO3– (Figure 5B) and δ18O–NO3– (Supplementary Figure 5). The sediment became a net NO3– sink in response to amendment with NO3–, while the NH4+ production and DO consumption rates remained similar under oxic conditions (Figures 4C,D). Amongst the different treatments, the glucose addition did not significantly decrease the NO3– concentration in the output solutions under aerobic conditions (group B vs. E). Accordingly, the isotope offset of both δ15N–NO3– and δ18O–NO3– (difference between the input and output fractions) was statistically similar between these two groups. Even when the DO concentration in the input solution dropped to 70% saturation, the glucose stimulation of NO3– removal rates was still insignificant (group C vs. F) and the isotopic response was similar. In the sub-oxic groups (D and G), NO3– removal rates significantly increased compared to the oxic group (p = 0.01), leading to a linear relationship between NO3– reduction rates and δ15N–NO3– increase (Figure 5C). In terms of the offset between δ15N–NO3– and δ18O–NO3– (Figure 5D), a linear correlation was observed, suggesting that the dominant NO3– consumption pathway remained the same from B to G groups.
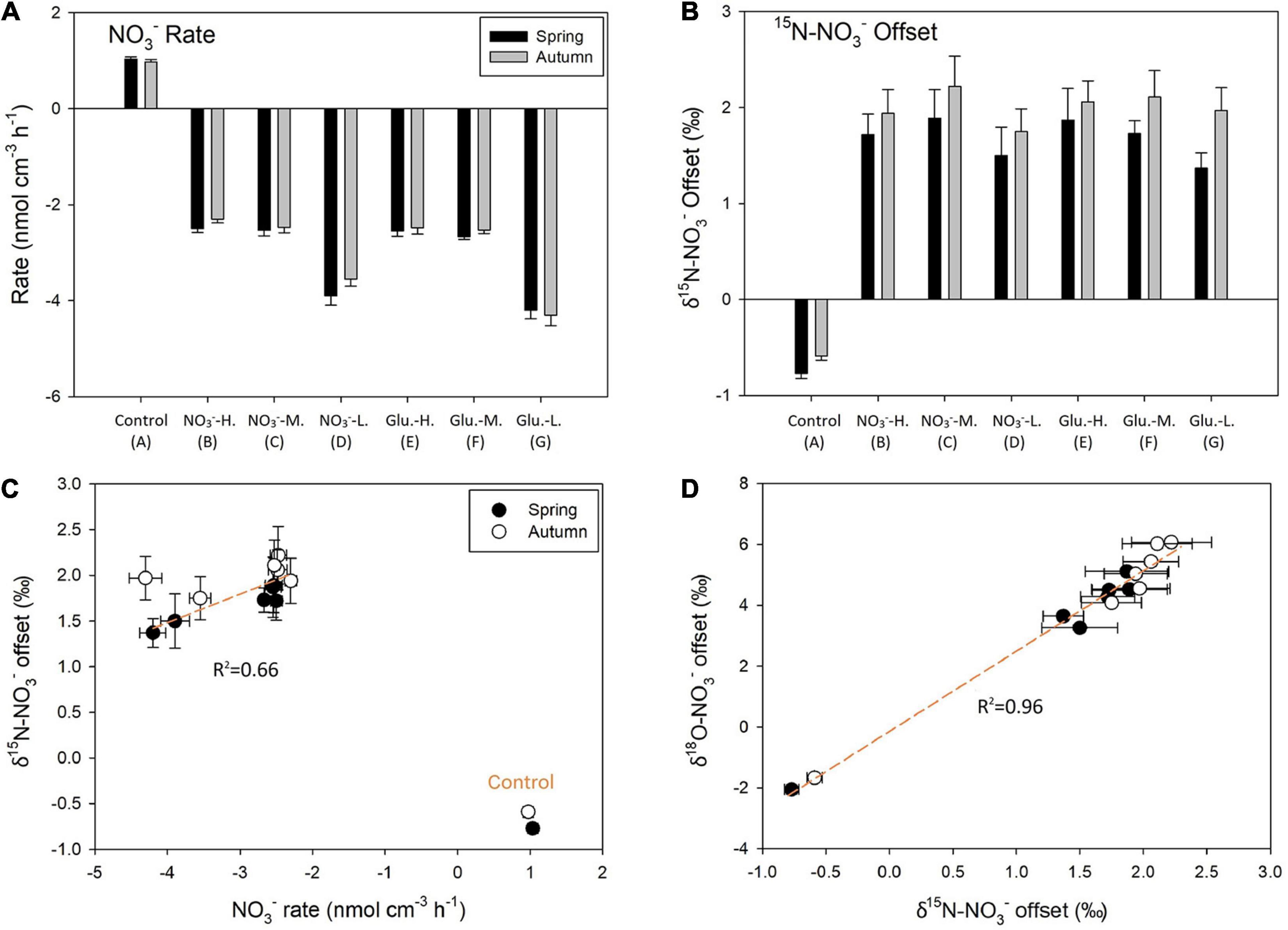
Figure 5. Reaction rates NO3– during both seasons (A) and offsets for δ15N–NO3– from a series of incubations (B). Positive values indicate production/offset enhancement after incubation and negative is removal/offset decrease. It also shows the correlation between NO3– reaction rates and δ15N–NO3– (C) as well as δ15N–NO3– and δ18O–NO3– offsets (D).
Benthic Prokaryotes
During spring, the operational taxonomic units (OTUs) in the sediment were 1,288, increasing to 2,454 during autumn (Table 2). Concurrently, the Shannon index and Equitability also increased during autumn. In both periods, the dominant phylum was Proteobacteria while important opportunistic groups varied between seasons. The key genera were Sphingobium, Marinobacter, and Thioprofundum during spring, while the relative abundance of Nitrosopumilus and Gp10 increased during autumn. For the potential metabolic functionality (Supplementary Figure 6), a wide range of functions were present with benthic carbon, nitrogen and sulfur metabolism, suggesting a significant requirement of electron donors. The nitrogen metabolism was also taken as a key function with a minor seasonal variation. For the microbiota involved in nitrogen transformations, nitrifiers, e.g., Nitrosopumilus, were observed in the seepage face. Denitrifying bacteria, characterized at the genus level, such as Marinobacter, Bacillus, Hyphomicrobium, or Lactobabillus, were also observed in the sediment (Table 2). The microorganisms involved in Anammox and DNRA were not identified at the genus level in the studied seepage face, indicating that NO3– removal was mainly conducted by denitrification.
Discussion
Methodological Considerations of the Use of FTR
Flow through reactor simulations under pre-defined environmental conditions, have been identified as an effective tool to quantitively determine nitrogen transformations in permeable coastal sediments, and have been widely applied in many research sites, e.g., Ria Formosa Lagoon, Portugal (Ibánhez and Rocha, 2014, 2016); South Atlantic Bight (Rao et al., 2007) and the North Sea (Marchant et al., 2016). FTR can effectively mimic 1-D advective flow in permeable sediments, adequately simulating the essentially vertical nature of porewater transport in the seepage face during ebbing tide (Ibánhez et al., 2011). The use of FTR with permeable sediments requires a “checklist” prior to laboratory incubations. Apart from flow rate, light exposure and reasonable temperature, the preferential flow paths that might form inside the cells are one of the pivotal factors in FTR simulations, and may lead to uneven transport and dispersion of the target solutes within the reactor cells, questioning the reliability of the reaction rates obtained. In the present study, these environmental variables were constrained at laboratory conditions with limited fluctuations during incubation experiments to reduce the potential errors. To further identify the reliability of our simulation results, a comparison of the obtained reaction rates with in situ rates was undertaken. During in situ field surveys at the sampling site, the DOC production ranged from 12.5 to 68.9 nmol cm–3 h–1 during spring and 2.8–62.7 nmol cm–3 h–1 during autumn, calculated from solute distribution at the seepage face together with measured seepage rates and applying mass balance approaches (data from Jiang et al., 2020). The reaction rates obtained in the FTR experiments were similar to the lower boundary of these in situ data. Moreover, on a global scale, the DOC production rates obtained in the present study are in line with the results found in a subterranean estuary seepage face in the Ria Formosa Lagoon, Portugal (FTR experiments; Ibánhez and Rocha, 2014). The DO consumption rate in the control group was 12.9 nmol cm–3 h–1 during spring, which was in line with those determined by Rao et al. (2007) in the South Atlantic Bight using FTRs (3.8–16.6 nmol cm–3 h–1). The rapid DO consumption in permeable sediments after glucose amendment was also comparable to the results obtained by Rusch et al. (2006) with sediments from the Atlantic Bight (35–57% enhancement). NO3– removal rates obtained in the present study are similar to those calculated from field survey data in the same STE (13.6 nmol cm–3 h–1, unpublished data) and those measured with FTR in the Ria Formosa Lagoon (0.7–6.8 nmol cm–3 h–1; Jiang et al., 2018).
Bio-labile DOM Supply and Benthic NO3– Transformations
The effects of bio-labile DOM availability on benthic aerobic respiration and mineralization have been widely documented as a key factor for a wide range of heterotrophic transformations in many coastal permeable environments, such as in Skagerrak, North Sea (Hulthe et al., 1998), St. George Island, Gulf of Mexico (Chipman et al., 2010) or the Ria Formosa Lagoon, Portugal (Ibánhez and Rocha, 2016). In our FTR experiments, the addition of glucose enhanced aerobic respiration rates as expected. Nevertheless, despite DO being more energetically favorable as an electron acceptor than NO3– for sediment microbes, net NO3– reduction is observed after the addition of NO3– to the circulating porewater even at DO saturated conditions, revealing the temporal synchronism of both reactions.
In the present study, nitrogen transformations were identified as a crucial function of sediment microbial metabolism (Supplementary Figure 6). Substantial microbial groups were identified as potential nitrifiers and denitrifiers in our sampled site based on the 16S rDNA information. For potential nitrifiers, the dominant genus was Nitrosomonas (Table 2). During laboratory incubations, Nitrosomonas genus showed a significant reliance on the supply of organic carbon during their growth stages in culture solutions despite the autotrophic character of the nitrification process (Clark and Schmidt, 1967). Similarly, the potential key denitrifiers found in the studied seepage face, included Bacillus, Hyphomicrobium, Lactobacillus and several of these were identified as facultative denitrifiers with different metabolism functions at variable DO levels (Ji et al., 2015). They have previously shown strong dependence on organic carbon supply (Harder and Attwood, 1978; Condón et al., 1996; Surono et al., 2008), likely due to their aerobic reaction pathway catalyzed by cytochrome o oxidase (Ji et al., 2015). Often, nitrification and facultative denitrification are closely coupled in coastal sediments (Ouyang et al., 2021) while their reaction rates vary under different environmental settings (Calvo-Martin et al., 2021). Net NO3– production in our experiments (group A) reveals the dominance of nitrification, probably fed by organic matter ammonification with contributions from the adsorbed pool, while NO3– removal stimulated by addition likely results from both denitrification and NO3– assimilation under such aerobic environment (Jiang and Jiao, 2016), since Anammox-capable microorganisms were not identified here. Given that the biological assimilation of NH4+ is more energetically favorable than NO3– assimilation, the net NH4+ production in all the treatments employed in this study (Figures 4A,B) suggests a relatively weak NO3– assimilation rate and supports the contention that denitrification may be the dominant NO3– removal pathway in place.
However, in contrast to the reliance of denitrifiers on bio-labile DOM reported elsewhere, the addition of glucose under oxic conditions did not lead to a significantly positive response of NO3– reduction rates during both seasons (Figure 5A). Moreover, the potential denitrifier genera and their relative abundances varied among the two studied seasons, while the response of benthic NO3– reduction rates to exogenous DOM remained unchanged under fully oxic conditions. In addition, the isotopic fractionation of NO3– (15N–NO3–) during net NO3– reduction in both glucose-free and glucose-amended groups was similar in the two studied seasons, suggesting that the addition of glucose was in fact not altering NO3– reduction rates and pathways (Figure 5B). Considering the biological significance of bio-labile organic matter to heterotrophic microbes, the insignificant variation in NO3– reduction rates after glucose addition under DO saturated conditions suggests that the added glucose is not used by the denitrifying bacteria as the primary carbon source in these FTR experiments. Despite a significant consumption of glucose by aerobic reactions plus adsorption losses, the outflow porewater still contained substantial quantities of glucose, as evidenced by the measured high DOC concentrations (Figure 4B), indicating that the concentration of glucose was not a limiting factor for heterotrophic metabolism throughout the entire sediment column used in the FTR experiments.
In the test sediment, several strains of benthic microbes could be facultative denitrifiers. They may host the function of NO3– removal under aerobic conditions, while the processing of NO3– removal and glucose consumption might be clearly separated in their metabolism, suggesting that electrons from glucose may flow into the DO respiration in these denitrifiers (cytochrome o; Ji et al., 2015). Alternatively, the presence of active NO3– reduction under fully oxic conditions in permeable sediments suggests that porewater transport mechanisms and niches of active denitrifiers on sediment particles would be the key to understand this apparent contradiction. Particularly, in contrast with cohesive sediments, surface permeable coastal sediments are frequently enriched in DO due to the regular porewater flushing with surface seawater together with the periodic undersaturation of intertidal sediments, which permits invasion of the sediment by atmospheric oxygen (Ibánhez and Rocha, 2016). Several strains of Arthrobacter, Pseudomonas, or Rhodococcus, for instance, could actively perform denitrification in aerobic environments (Qiao et al., 2020), a process that has been observed in coastal sites (e.g., the Wadden Sea, Germany, Marchant et al., 2017) and wastewater treatment (Wang et al., 2020). The majority of facultative denitrifiers operate well under a wide range of DO concentrations, while an anaerobic environment favors the expression of Nir and Nor genes (Ji et al., 2015), suggesting that a fraction of denitrifiers might be closely attached onto the sediment particle surfaces, thus relying on the aerobic microbiota for DO consumption and the creation of anaerobic niches (outlined in Figure 6). Though a fraction of denitrifiers might be located outside of these anaerobic niches (niche circularity > 0.8, area < 3 mm2, Widerlund and Davison, 2007), due to the exposure to DO and the facultative metabolism of denitrifiers (Hayatsu et al., 2008; Wu et al., 2014), denitrification may not be conducted by these DO-exposed denitrifiers, similarly to the results found in oxygen minimum zones in the open ocean (Dalsgaard et al., 2014). The facultative denitrifiers involved in the NO3– isotope determinations, e.g., Pseudomonas aureofaciens, are usually characterized by variable metabolic functions at different DO concentrations (Sigman et al., 2001). Due to the “isolation” afforded by the “aerobic cover,” and sustained by the benthic oxidative metabolism (Figure 6), the glucose delivered from the porewater flowing through the sediment would have been consumed by aerobes, such as Sphingobium (Table 2, active carbon decomposer, Bashir et al., 2013), before reaching active NO3– reducers attached to the particle surface. Furthermore, given the difference in molecular weight, the diffusion rate of glucose is much slower than that of DO (regardless of difference in solute concentration gradient), indicating that only a fraction of the microbiota would be fueled by this extra bio-labile DOM source. Accordingly, denitrifying bacteria in permeable sediment micro-niches has to rely on the SOM as a carbon source for NO3– reduction (Hong et al., 2010), especially the bio-labile fractions loosely attached on the particle surface (e.g., extractable by KCl solutions, Jiang et al., 2020). This reliance on the SOM provides stability for benthic microbial function and therefore reduces the reaction shock following switching between carbon sources (Wang et al., 2011). In addition, the utilization of SOM during denitrification likely adds DOM content to the porewater. Amendments with NO3– increased DOC production rates and added small-molecular DOM into the porewater during both seasons at saturated DO conditions (Figures 3B–D). A similar DOC production pattern during net NO3– reduction under aerobic conditions was found by Jiang et al. (2018) in intertidal permeable sediments of the Ria Formosa Lagoon, Portugal. On the other hand, Ibánhez et al. (2021b) found that porewater nitrate enrichment accelerates SOM mineralization by overcoming N limitation of the local microbial metabolism, thus shortening SOM turnover times. This priming effect of nitrate over oxic SOM mineralization results in the production of both humic and labile DOM and could also explain the production of labile DOM in our experiments run under oxic conditions. Given the relatively high concentration of SOM compared to DOC (minor SOM concentration variation during the incubations, Figure 2), the effects of glucose at the micro-niche scale might require a longer incubation period (e.g., 10 days) to allow for the exhaustion of the labile fractions of SOM, as well as increasing abundance of functional genes (e.g., nirS and nosZ; Henderson et al., 2010), and thus are not observed under our experimental strategy (10 h of duration).
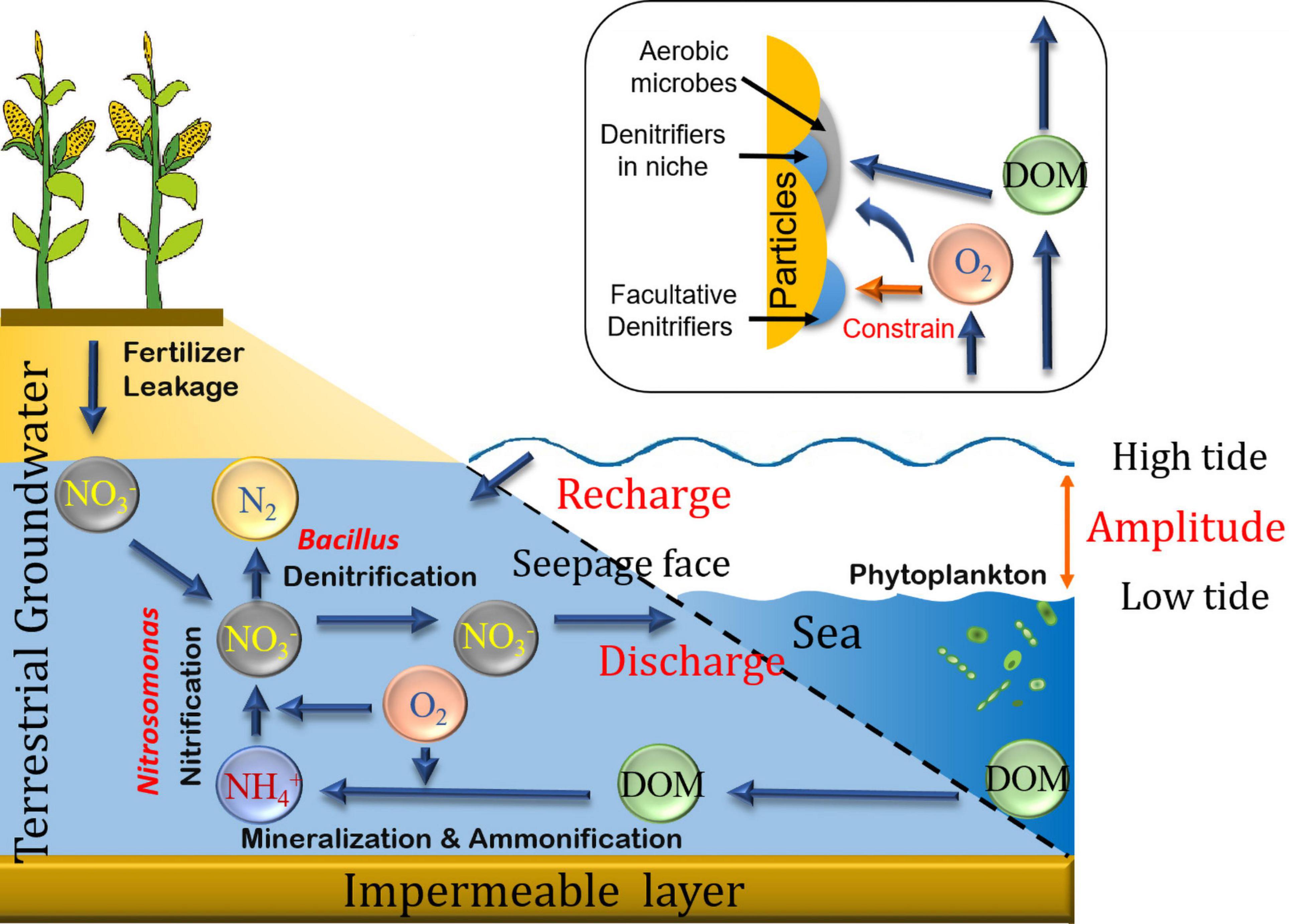
Figure 6. Sketch of the NO3– processing in the aerobic seepage face, it highlights the interaction between benthic carbon content [both sedimentary organic matter (SOM) and dissolved organic matter (DOM)] and NO3– processing with key microbes. The porewater exchange in the seepage face is mainly driven by the tidal pumping. The top right panel is a zoom-in of anaerobic niches in sandy sediments. The majority of denitrifiers in the sediment are facultative denitrifiers, respiring oxygen in the aerobic environment; Nevertheless, the denitrifiers in these niches might be capable for conducting denitrification process due to the anaerobic environment.
When the DO content at the inflow decreased to approximately 70% saturation, the DO concentration in the outflow concurrently dropped to ca. 60% saturation (Figure 3A). However, the NO3– removal rate remained statistically similar to that under DO saturation conditions (Figure 5A). Intriguingly, the consumption of glucose dropped significantly under 70% of DO saturation (DOC consumption rates; p < 0.01), indicating that the aerobic mineralization pathways were affected by the decrease in DO (Figure 3B). Under these circumstances, the potential amount of bio-labile DOM that could reach denitrifying bacteria via porewater advection should increase. Still, at 70% of DO saturation in the inflowing water, denitrification rates didn’t change compared to DO saturation conditions, adding support to the proposed niche structure and the reliance of NO3– reducers on SOM rather than bio-labile DOM circulating through the sediment. Delayed reaction of microbial communities to changing metabolite availability was previously discussed by Evard et al. (2012), and can be induced by the changing redox conditions commonly found in permeable sediments.
The significant effect of added bio-labile DOM over benthic NO3– consumption rates occurred when the DO concentration at the input solution fell to nearly 30% saturation (p = 0.01), while the dominant NO3– removal pathway was still deemed to be denitrification based on the linearity in the isotopic fractionation for the remaining NO3– (Figure 5D). The addition of glucose increased NO3– consumption rates compared to the unamended treatment (group D vs. G; approximately 0.32–0.71 nmol NO3– cm–3 h–1 enhancement during both seasons). In the present study, microbial composition was only determined at the initial stage. The more rapid biomass increase at the anaerobic environment during the incubation may lead to the active removal observed at group G. Presuming an insignificant increase for denitrifier biomass during the incubation, the enhancement of NO3– consumption rates at lowering DO levels suggest that under these conditions, glucose was reaching the denitrifying community due to the low adsorption potential of NO3– onto sediment particles. This delivery further benefited the NO3– removal due to the increase of the anaerobic sites on particle surface suitable for NO3– consumption at low DO levels. The key genera present at the studied seepage face, including Sphingobium, Gp10 (Table 2), are generally adapted to aerobic environments using organic matter as energy supply (Berlendis et al., 2010; Roy et al., 2013). When the DO concentration decreased to nearly 30% saturation, these facultative denitrifiers tend to select NO3– as electron acceptor due to the enrichment in porewater (Group D and G). Furthermore, once the DO constraint is removed (30% saturation here), reduced cytochrome from all facultative denitrifiers may resume their function and lead to the increase in NO3– reduction (Ji et al., 2015). Consequently, the glucose amendment is linked to the increases in NO3– removal rates in this treatment. Interestingly, in the treatments without glucose addition, the 30% DO saturation at the input solution also triggered a significant enhancement of the denitrification rates. Compared with the aerobic group (100% DO saturation), the increase in NO3– removal rates reached approximately 1.3 nmol cm–3 h–1 (group A vs. D in spring). In addition, the significant linear correlation between δ15N–NO3– and δ18O–NO3– suggests that the dominant process of NO3– removal among different groups remained the same as in the other groups (Figure 5D). Such marked increase observed in these treatments suggests that DO levels in the porewater are a strong modulator of denitrification rates because of the shift of terminal electron acceptor in benthic reactions from O2 (oxic) to NO3– (suboxic) during organic matter degradation by microbes (Chen et al., 2017). Generally, denitrification in the open ocean requires DO levels < 2 mg L–1, approximately 28% saturation in the seawater (Codispoti and Christensen, 1985). Correspondingly, the DO input concentration in the sub-oxic treatment could fit this standard and all sediment denitrifying bacteria might be active under these conditions, including the microbiota located outside of the niches (Figure 6).
Benthic N and C Interactions and Coastal Management
Coastal permeable sediments in seepage faces are assumed to be active reactors capable of removing terrestrial NO3– before it is discharged into coastal waters (Calvo-Martin et al., 2021). Accordingly, denitrification, i.e., the main pathway for a permanent NO3– removal (Burgin and Hamilton, 2007), in the exit sites of continental NO3– into the sea plays a key role in the coastal NO3– inventory. Coastal zones are also an important node for carbon cycling, especially in the production of pelagic organic matter via a wide range of primary producers (Jiang et al., 2020). In the present study, those aerobic metabolic functions (Figure 6), likely including reactions of aerobic mineralization and subsequent ammonification and nitrification, were benefited from the addition of bio-labile DOM. In contrast, the significant addition of glucose (nearly 300 μM) did not boost denitrification rates even if the DO concentration in the inputs decreased to approximately 70% saturation. This interaction between the NO3– production and DOC supply reveals that the retention of NO3– in coastal systems might be higher than previously thought, since the SOM dependent NO3– removal capability tends to be stable during the high loading of bio-labile DOM. Within many coastal ecosystems, a large amount of terrestrial NO3–, derived from overuse of chemical fertilizer or leakage from sewage pipes, reaches the coastal seawater via the benthic system (Tamborski et al., 2020). Compared with seawater concentration, submarine groundwater discharge-derived NO3– concentration was several orders of magnitude higher at our site (e.g., more than 10 times higher in Sanggou Bay, China, Wang et al., 2014). The influx of land-derived NO3– frequently triggers the algae blooms at the coast, e.g., Ria Formosa Lagoon, Portugal (Rocha et al., 2016), Jeju Island, Korea (Kang et al., 2019) as well as at our study site (Sanggou Bay, China, Yu et al., 2018). Coupled with cell decay and remineralization of debris, a significant fraction of bio-labile DOM is released into the coastal water and accumulates in subterranean estuaries (Mahmood et al., 2017). This way, STEs in coastal belts may act as catalysts for NO3– removal and eliminate the ecological stress caused by excessive NO3–, as can be seen from the simulation in group B among two seasons (Figure 5A). However, the accumulation of bio-labile DOM could not rapidly lead to the enhancement of denitrification rates in the seepage face in oxic conditions, at least in Sanggou Bay during spring and autumn. DO concentrations in STEs could drop to the saturation level from 20 to 30%, e.g., Ker Chalon beach, France (Mouret et al., 2020), the DO concentration in STE outflow locations, or coastal permeable beaches frequently remains at high-level (Boufadel et al., 2010), e.g., 57–93% in the STE outflow site in Ria Formosa lagoon (Ibánhez and Rocha, 2016) and 87–107% saturation in the Aquitanian coast, France (Charbonnier et al., 2013). Such aerobic environments indicate that denitrification would still not be stimulated on the short term unless conditions reach a specific DO concentration threshold. More importantly, the benthic reduction of NO3– may further produce bio-labile DOM via SOM decomposition (non-glucose amendment groups in Figure 3B) and drain into coastal seawater (Ibánhez et al., 2021b), potentially increasing the coastal DOM inventory. Consequently, this freshly produced bio-labile DOM could yet yield increases in NO3– availability via aerobic nitrification and be made available in the coastal water as a “second-hand” NO3–, and stimulating dinoflagellate blooms in the absence of equivalent silicate inflows (Rocha et al., 2002). In Sangou Bay, diatoms and dinoflagellates are often the dominant phytoplankton species (Yuan et al., 2014). Compared to diatoms, dinoflagellates are unfavored food source for consumers, especially for filter-feeders. Notably, frequency of dinoflagellate blooms has increased (Yuan et al., 2014) while aquaculture yields in Sanggou Bay and adjacent areas declined in recent years (local government data1), indicating the necessity for considering the feedback loop between NO3– and bio-labile DOM in future nutrient management.
Conclusion
The present study focused on the interactions between bio-labile DOM supply, DO concentration and NO3– removal in STE outflow areas using FTR experiments. Permeable sediments clearly revealed high NO3– reduction potential, mainly via denitrification, when the input NO3– concentration increased from approximately 10–80 μM. The decrease of DO concentration from 100% saturation to 70% saturation did not trigger a significant increase of the denitrification rate regardless of season, revealing that facultative denitrifiers in the sediment might rely on anaerobic microniches maintained by aerobic reactions. Denitrification rates significantly increased when the DO concentrations decreased to ∼30% saturation, suggesting that NO3– reducing potential supported by all facultative denitrifiers present in the sediment came into play then. In such a sub-oxic environment, the addition of glucose also triggered a significant increase in denitrification rate, revealing that a fraction of bio-labile DOM was involved in the denitrification process. However, STE outflow areas are usually characterized by high DO concentration in porewaters. Accordingly, the bio-labile DOM in STEs may not enhance NO3– removal, but stimulate aerobic reactions, such as aerobic mineralization and decomposition instead, leading to an increase in NO3– availability via nitrification. Accordingly, the land-derived NO3– may take part on a self-sustaining vicious cycle supporting the reaction chain “NO3––algae-bio-labile DOM −NO3–” at the water-sediment interface along coastlines hosting STEs. This overlooked biogeochemical chain in coastal systems requires further attention from coastal managers and stakeholders.
Data Availability Statement
The datasets presented in this study can be found in online repositories. The names of the repository/repositories and accession number(s) can be found below: https://www.ncbi.nlm.nih.gov/, SRX7825212; https://www.ncbi.nlm.nih.gov/, SRX7825225.
Author Contributions
SJ: conceptualization, investigation, methodology, writing–original draft, project administration, and funding acquisition. JJ and YZ: investigation. YW: methodology, resources, and writing–original draft. YWe: formal analysis and writing–original draft. CR and JI: methodology and writing–original draft. JZ: conceptualization, resources, writing–original draft, and funding acquisition. All authors contributed to the article and approved the submitted version.
Funding
This work was supported by the National Natural Science Foundation of China (Nos. 41706081 and 42111530026) and Scientific Research Foundation of SKLEC (2017RCDW04).
Conflict of Interest
The authors declare that the research was conducted in the absence of any commercial or financial relationships that could be construed as a potential conflict of interest.
Acknowledgments
We thank DeepBiome Co., Ltd. for bioinformatic assistance. The comments of three reviewers on an early version of the manuscript are greatly appreciated.
Supplementary Material
The Supplementary Material for this article can be found online at: https://www.frontiersin.org/articles/10.3389/fmars.2021.642143/full#supplementary-material
Footnotes
References
Ahmerkamp, S., Marchant, H. K., Peng, C., Probandt, D., Littmann, S., Kuypers, M. M. M., et al. (2020). The effect of sediment grain properties and porewater flow on microbial abundance and respiration in permeable sediments. Sci. Rep. 10:3573.
Amato, D. W., Bishop, J. M., Glenn, C. R., Dulai, H., and Smith, C. M. (2016). Impact of submarine groundwater discharge on marine water quality and reef biota of Maui. PLoS One 11:e0165825. doi: 10.1371/journal.pone.0165825
Bashir, S., Fischer, A., Nijenhuis, I., and Richnow, H.-H. (2013). Enantioselective carbon stable isotope fractionation of hexachlorocyclohexane during aerobic biodegradation by Sphingobium spp. Environ. Sci. Technol. 47, 11432–11439. doi: 10.1021/es402197s
Berg, P., Long, M. H., Huettel, M., Rheuban, J. E., McGlathery, K. J., Howarth, R. W., et al. (2013). Eddy correlation measurements of oxygen fluxes in permeable sediments exposed to varying current flow and light. Limnol. Oceanogr. 58, 1329–1343. doi: 10.4319/lo.2013.58.4.1329
Berlendis, S., Cayol, J.-L., Verhé, F., Laveau, S., Tholozan, J.-L., Ollivier, B., et al. (2010). First evidence of aerobic biodegradation of BTEX compounds by pure cultures of Marinobacter. Appl. Biochem. Biotechnol. 160, 1992–1999. doi: 10.1007/s12010-009-8746-1
Bernhardt, E. S., and Likens, G. E. (2002). Dissloved organic carbon enrichment alters nitrogen dynamics in a forest stream. Ecology 83, 1689–1700. doi: 10.1890/0012-9658(2002)083[1689:docean]2.0.co;2
Boufadel, M. C., Sharifi, Y., Van Aken, B., Wrenn, B. A., and Lee, K. (2010). Nutrient and oxygen concentrations within the sediments of an Alaskan beach polluted with the Exxon Valdez oil spill. Environ. Sci. Technol. 44, 7418–7424. doi: 10.1021/es102046n
Burgin, A. J., and Hamilton, S. K. (2007). Have we overemphasized the role of denitrification in aquatic ecosystems? A review of nitrate removal pathways. Front. Ecol. Environ. 5, 89–96. doi: 10.1890/1540-9295(2007)5[89:hwotro]2.0.co;2
Calvo-Martin, E., Álvarez-Salgado, X. A., Rocha, C., and Ibánhez, J. S. P. (2021). Reactive solute transport through two contrasting subterranean estuary exit sites in the Ría de Vigo (NW Iberian Peninsula). Front. Mar. Sci. 8:94. doi: 10.3389/fmars.2021.626813
Charbonnier, C., Anschutz, P., Poirier, D., Bujan, S., and Lecroart, P. (2013). Aerobic respiration in a high-energy sandy beach. Mar. Chem. 155, 10–21. doi: 10.1016/j.marchem.2013.05.003
Chen, M., Kim, J., Choi, J., Lee, Y. K., and Hur, J. (2017). Biological early diagenesis and insolation-paced paleoproductivity signified in deep core sediment organic matter. Sci. Rep. 7:1581.
Chipman, L., Podgorski, D., Green, S., Kostka, J., Cooper, W., and Huettel, M. (2010). Decomposition of plankton-derived dissolved organic matter in permeable coastal sediments. Limnol. Oceanogr. 55, 857–871. doi: 10.4319/lo.2010.55.2.0857
Clark, C., and Schmidt, E. L. (1967). Growth response of Nitrosomonas europaea to amino acids. J. Bacteriol. 93, 1302–1308. doi: 10.1128/jb.93.4.1302-1308.1967
Codispoti, L. A., and Christensen, J. P. (1985). Nitrification, denitrification and nitrous oxide cycling in the eastern tropical South Pacific ocean. Mar. Chem. 16, 277–300. doi: 10.1016/0304-4203(85)90051-9
Condón, S., Palop, A., Raso, J., and Sala, F. J. (1996). Influence of the incubation temperature after heat treatment upon the estimated heat resistance values of spores of Bacillus subtilis. Lett. Appl. Microbiol. 22, 149–152. doi: 10.1111/j.1472-765x.1996.tb01130.x
Cornwell, J. C., Kemp, W. M., and Kana, T. M. (1999). Denitrification in coastal ecosystems: methods, environmental controls, and ecosystem level controls, a review. Aquat. Ecol. 33, 41–54.
Crawshaw, J., O’Meara, T., Savage, C., Thomson, B., Baltar, F., and Thrush, S. F. (2019). Source of organic detritus and bivalve biomass influences nitrogen cycling and extracellular enzyme activity in estuary sediments. Biogeochemistry 145, 315–335. doi: 10.1007/s10533-019-00608-y
Dalsgaard, T., Stewart, F. J., Thamdrup, B., De Brabandere, L., Revsbech, N. P., Ulloa, O., et al. (2014). Oxygen at nanomolar levels reversibly suppresses process rates and gene expression in anammox and denitrification in the oxygen minimum zone off Northern Chile. mBio 5:e01966-14.
Edgar, R. C. (2010). Search and clustering orders of magnitude faster than BLAST. Bioinformatics 26, 2460–2461. doi: 10.1093/bioinformatics/btq461
Evard, V., Glud, R. N., and Cook, P. L. M. (2012). The kinetics of denitrification in permeable sediments. Biogeochemistry 113, 563–572. doi: 10.1007/s10533-012-9789-x
Fang, J., Zhang, J., Xiao, T., Huang, D., and Liu, S. (2016). Integrated multi-trophic aquaculture (IMTA) in Sanggou Bay, China. Aquac. Environ. Interact. 8, 201–205. doi: 10.3354/aei00179
Harder, W., and Attwood, M. M. (1978). “Biology, physiology and biochemistry of Hyphomicrobia,” in Advances in Microbial Physiology, eds A. H. Rose and J. G. Morris (Cambridge, MA: Academic Press), 303–359. doi: 10.1016/s0065-2911(08)60060-0
Hayatsu, M., Tago, K., and Saito, M. (2008). Various players in the nitrogen cycle: diversity and functions of the microorganisms involved in nitrification and denitrification. Soil Sci. Plant Nutr. 54, 33–45. doi: 10.1111/j.1747-0765.2007.00195.x
Heiss, J. W., Michael, H. A., and Koneshloo, M. (2020). Denitrification hotspots in intertidal mixing zones linked to geologic heterogeneity. Environ. Res. Lett. 15:084015. doi: 10.1088/1748-9326/ab90a6
Henderson, S. L., Dandie, C. E., Patten, C. L., Zebarth, B. J., Burton, D. L., Trevors, J. T., et al. (2010). Changes in denitrifier abundance, denitrification gene mRNA levels, nitrous oxide emissions, and denitrification in anoxic soil microcosms amended with glucose and plant residues. Appl. Environ. Microboiol. 76, 2155–2164. doi: 10.1128/aem.02993-09
Hong, S. W., Kim, H. S., and Chung, T. H. (2010). Alteration of sediment organic matter in sediment microbial fuel cells. Environ. Pollut. 158, 185–191. doi: 10.1016/j.envpol.2009.07.022
Huettel, M., Berg, P., and Kostka, J. E. (2014). Benthic exchange and biogeochemical cycling in permeable sediments. Annu. Rev. Mar. Sci. 6, 23–51. doi: 10.1146/annurev-marine-051413-012706
Hulthe, G., Hulth, S., and Hall, P. O. J. (1998). Effect of oxygen on degradation rate of refractory and bio-labile organic matter in continental margin sediments. Geochim. Cosmochim. Acta 62, 1319–1328. doi: 10.1016/s0016-7037(98)00044-1
Ibánhez, J. S. P., Álvarez-Salgado, X. A., Nieto-Cid, M., and Rocha, C. (2021a). Fresh and saline submarine groundwater discharge in a large coastal inlet affected by seasonal upwelling. Limnol. Oceanogr. doi: 10.1002/lno.11733
Ibánhez, J. S. P., Álvarez-Salgado, X. A., and Rocha, C. (2021b). Does nitrate enrichment accelerate organic matter turnover in subterranean estuaries? Front. Mar. Sci. 8:661201. doi: 10.3389/fmars.2021.661201
Ibánhez, J. S. P., Leote, C., and Rocha, C. (2011). Porewater nitrate profiles in sandy sediments hosting submarine groundwater discharge described by an advection–dispersion-reaction model. Biogeochemistry 103, 159–180. doi: 10.1007/s10533-010-9454-1
Ibánhez, J. S. P., Leote, C., and Rocha, C. (2013). Seasonal enhancement of submarine groundwater discharge (SGD)-derived nitrate loading into the Ria Formosa coastal lagoon assessed by 1-D modeling of benthic NO3- profiles. Estuar. Coast. Shelf Sci. 132, 56–64. doi: 10.1016/j.ecss.2012.04.015
Ibánhez, J. S. P., and Rocha, C. (2014). Effects of recirculation of seawater enriched in inorganic nitrogen on dissolved organic carbon processing in sandy seepage face sediments. Mar. Chem. 166, 48–58. doi: 10.1016/j.marchem.2014.09.012
Ibánhez, J. S. P., and Rocha, C. (2016). Oxygen transport and reactivity within a sandy seepage face in a mesotidal lagoon (Ria Formosa, Southwestern Iberia). Limnol. Oceanogr. 61, 61–77. doi: 10.1002/lno.10199
Ibánhez, J. S. P., and Rocha, C. (2017). Kinetics of inorganic nitrogen turnover in a sandy seepage face on a subterranean estuary. Appl. Geochem. 87, 108–121. doi: 10.1016/j.apgeochem.2017.10.015
Ji, B., Yang, K., Zhu, L., Jiang, Y., Wang, H., Zhou, J., et al. (2015). Aerobic denitrification: a review of important advances of the last 30 years. Biotechnol. Bioprocess Eng. 20, 643–651. doi: 10.1007/s12257-015-0009-0
Jiang, S., Ibánhez, J. S. P., and Rocha, C. (2018). Influence of labile dissolved organic matter on nitrate reduction in a seepage face. Environ. Sci. Pollut. Res. 25, 10654–10667. doi: 10.1007/s11356-018-1302-1
Jiang, S., Ibánhez, J. S. P., Wu, Y., and Zhang, J. (2021a). Geochemical tracers in submarine groundwater discharge research: practice and challenges from a view of climate changes. Environ. Rev. doi: 10.1139/er-2020-0093
Jiang, S., Jin, J., Jiang, S., Wu, Y., Wang, J., Chen, J., et al. (2021b). Nitrogen in atmospheric wet depositions over the East Indian Ocean and West Pacific Ocean: spatial variability, source identification, and potential influences. Front. Mar. Sci. 7:600843. doi: 10.3389/fmars.2020.600843
Jiang, S., Kavanagh, M., Ibánhez, J. S. P., and Rocha, C. (2021c). Denitrification-nitrification process in permeable coastal sediments: an investigation on the effect of salinity and nitrate availability using flow-through reactors. Acta Oceanol. Sin. doi: 10.1007/s13131-021-1811-5
Jiang, S., Kavanagh, M., and Rocha, C. (2017). Evaluation of the suitability of vacutainers for storage of nutrient and dissolved organic carbon analytes in water samples. Biol. Environ. Proc. R. Ir. Acad. 177B, 33–46. doi: 10.3318/bioe.2017.01
Jiang, S., Müller, M., Jin, J., Wu, Y., Zhu, K., Zhang, G., et al. (2019). Dissolved inorganic nitrogen in a tropical estuary in Malaysia: transport and transformation. Biogeosciences 16, 2821–2836. doi: 10.5194/bg-16-2821-2019
Jiang, S., Zhang, Y., Jin, J., Wu, Y., Wei, Y., Wang, X., et al. (2020). Organic carbon in a seepage face of a subterranean estuary: turnover and microbial interrelations. Sci. Total Environ. 725:138220. doi: 10.1016/j.scitotenv.2020.138220
Jiang, X., and Jiao, N. (2016). Nitrate assimilation by marine heterotrophic bacteria. Sci. China Earth Sci. 59, 477–483. doi: 10.1007/s11430-015-5212-5
Jin, J., Jiang, S., and Zhang, J. (2020). Nitrogen isotopic analysis of nitrate in aquatic environment by cadmium-hydroxylamine hydrochloride reduction. Rapid Commun. Mass Spectrom. 34:8804.
Jung, H., Koh, D.-C., Kim, Y. S., Jeen, S.-W., and Lee, J. (2020). Stable Isotopes of Water and Nitrate for the Identification of Groundwater Flowpaths: a Review. Water 12:138. doi: 10.3390/w12010138
Kang, J. H., Jang, J. E., Kim, J. H., Byeon, S. Y., Kim, S., Choi, S. K., et al. (2019). Species composition, diversity, and distribution of the genus Ulva along the coast of Jeju Island, Korea based on molecular phylogenetic analysis. PLoS One 14:e0219958. doi: 10.1371/journal.pone.0219958
Kessler, A. J., Glud, R. N., Gardenas, M. B., Larsen, M., Bourke, M. F., and Cook, P. L. M. (2012). Quantifying denitrification in rippled permeable sands through combined flume experiments and modeling. Limnol. Oceanogr. 57, 1217–1232. doi: 10.4319/lo.2012.57.4.1217
Kuypers, M. M., Marchant, H. K., and Kartal, B. (2018). The microbial nitrogen-cycling network. Nat. Rev. Microbiol. 16, 263–276.
Mahmood, T., Fang, J., Jiang, Z., Ying, W., and Zhang, J. (2017). Seasonal distribution, sources and sink of dissolved organic carbon in integrated aquaculture system in coastal waters. Aquac. Int. 25, 71–85. doi: 10.1007/s10499-016-0014-0
Marchant, H. K., Ahmerkamp, S., Lavik, G., Tegetmeyer, H. E., Graf, J., Klatt, J. M., et al. (2017). Denitrifying community in coastal sediments performs aerobic and anaerobic respiration simultaneously. ISME J. 11, 1799–1812. doi: 10.1038/ismej.2017.51
Marchant, H. K., Holtappels, M., Lavik, G., Ahmerkamp, S., Winter, C., and Kuypers, M. M. M. (2016). Coupled nitrification denitrification leads to extensive N loss in subtidal permeable sediments. Limnol. Oceanogr. 61, 1033–1048. doi: 10.1002/lno.10271
Marchant, H. K., Lavik, G., Holtappels, M., and Kuypers, M. M. (2014). The fate of nitrate in intertidal permeable sediments. PLoS One 9:e104517. doi: 10.1371/journal.pone.0104517
Meredith, K. T., Baker, A., Andersen, M. S., O’Carroll, D. M., Rutlidge, H., McDonough, L. K., et al. (2020). Isotopic and chromatographic fingerprinting of the sources of dissolved organic carbon in a shallow coastal aquifer. Hydrol. Earth Syst. Sci. 24, 2167–2178. doi: 10.5194/hess-24-2167-2020
Moore, S. (1999). The subterranean estuary: a reaction zone of ground water and sea water. Mar. Chem. 65, 111–125. doi: 10.1016/s0304-4203(99)00014-6
Mouret, A., Charbonnier, C., Lecroart, P., Metzger, E., Howa, H., Deflandre, B., et al. (2020). Biogeochemistry in an intertidal pocket beach. Estuar. Coast. Shelf Sci. 243:106920. doi: 10.1016/j.ecss.2020.106920
Ouyang, L., Thamdrup, B., and Trimmer, M. (2021). Coupled nitrification and N2 gas production as a cryptic process in oxic riverbeds. Nat. Commun. 12:1217.
Qiao, Z., Sun, R., Wu, Y., Hu, S., Liu, X., Chan, J., et al. (2020). Characteristics and metabolic pathway of the bacteria for heterotrophic nitrification and aerobic denitrification in aquatic ecosystems. Environ. Res. 191:110069. doi: 10.1016/j.envres.2020.110069
Rao, A. M. F., McCarthy, M. J., Gardner, W. S., and Jahnke, R. A. (2007). Respiration and denitrification in permeable continental shelf deposits on the South Atlantic Bight: rates of carbon and nitrogen cycling from sediment column experiments. Cont. Shelf Res. 27, 1801–1819. doi: 10.1016/j.csr.2007.03.001
Rivett, M. O., Buss, S. R., Morgan, P., Smith, J. W. N., and Bemment, C. D. (2008). Nitrate attenuation in groundwater: a review of biogeochemical controlling processes. Water Res. 42, 4215–4232. doi: 10.1016/j.watres.2008.07.020
Rocha, C., Galvão, H. M., and Barbosa, A. B. (2002). Role of transient silicon limitation in the development of cyanobacteria blooms in the Guadiana estuary, south-western Iberia. Mar. Ecol. Prog. Ser. 228, 35–45. doi: 10.3354/meps228035
Rocha, C., Veiga-Pires, C., Scholten, J., Knoeller, K., Gröcke, D. R., Carvalho, L., et al. (2016). Assessing land-ocean connectivity via submarine groundwater discharge (SGD) in the Ria Formosa Lagoon (Portugal): combining radon measurements and stable isotope hydrology. Hydrol. Earth Syst. Sci. 20, 3077–3098. doi: 10.5194/hess-20-3077-2016
Roy, M., Khara, P., Basu, S., and Dutta, T. (2013). Catabolic versatility of Sphingobium sp. strain PNB capable of degrading structurally diverse aromatic compounds. J. Bioremediat. Biodegrad. 4:173.
Rusch, A., Huettel, M., Wild, C., and Reimers, C. E. (2006). Benthic oxygen consumption and organic matter turnover in organic-poor, permeable shelf sands. Aquat. Geochem. 12, 1–19. doi: 10.1007/s10498-005-0784-x
Santos, I. R., Eyre, B. D., and Glud, R. N. (2012). Influence of porewater advection on denitrification in carbonate sands: evidence from repacked sediment column experiments. Geochim. Cosmochim. Acta 96, 247–258. doi: 10.1016/j.gca.2012.08.018
Siemens, J., Haas, M., and Kaupenjohann, M. (2003). Dissolved organic matter induced denitrification in subsoils and aquifers? Geoderma 113, 253–271. doi: 10.1016/s0016-7061(02)00364-6
Sigman, D. M., Casciotti, K. L., Andreani, M., Barford, C., Galanter, M., and Böhlke, J. (2001). A bacterial method for the nitrogen isotopic analysis of nitrate in seawater and freshwater. Anal. Chem. 73, 4145–4153. doi: 10.1021/ac010088e
Surey, R., Schimpf, C. M., Sauheitl, L., Mueller, C. W., Rummel, P. S., Dittert, K., et al. (2020). Potential denitrification stimulated by water-soluble organic carbon from plant residues during initial decomposition. Soil Biol. Biochem. 147:107841. doi: 10.1016/j.soilbio.2020.107841
Surono, I. S., Collado, M. C., Salminen, S., and Meriluoto, J. (2008). Effect of glucose and incubation temperature on metabolically active Lactobacillus plantarum from dadih in removing microcystin-LR. Food Chem. Toxicol. 46, 502–507. doi: 10.1016/j.fct.2007.08.017
Suryaputra, I. G. N. A., Santos, I. R., Huettel, M., Burnett, W. C., and Dittmar, T. (2015). Non-conservative behavior of fluorescent dissolved organic matter (FDOM) within a subterranean estuary. Cont. Shelf Res. 110, 183–190. doi: 10.1016/j.csr.2015.10.011
Tamborski, J., Brown, C., Bokuniewicz, H., Cochran, J. K., and Rasbury, E. T. (2020). Investigating boron isotopes for identifying nitrogen sources supplied by submarine groundwater discharge to coastal waters. Front. Mar. Sci. 8:126. doi: 10.3389/fenvs.2020.00126
Thornton, D. C. O. (2014). Dissolved organic matter (DOM) release by phytoplankton in the contemporary and future ocean. Eur. J. Phycol. 49, 20–46. doi: 10.1080/09670262.2013.875596
Troccoli-Ghinaglia, L., Herrera-Silveira, J. A., Comín, F. A., and Díaz-Ramos, J. R. (2010). Phytoplankton community variations in tropical coastal area affected where submarine groundwater occurs. Cont. Shelf Res. 30, 2082–2091. doi: 10.1016/j.csr.2010.10.009
Wang, M., Cao, G., Feng, N., and Pan, Y. (2020). Bioaugmentation of two-stage aerobic sequencing batch reactor with mixed strains for high nitrate nitrogen wastewater treatment. Chin. J. Chem. Eng. 28, 3103–3109. doi: 10.1016/j.cjche.2020.08.014
Wang, X., Du, J., Ji, T., Wen, T., Liu, S., and Zhang, J. (2014). An estimation of nutrient fluxes via submarine groundwater discharge into the Sanggou Bay-A typical multi-species culture ecosystem in China. Mar. Chem. 167, 113–122. doi: 10.1016/j.marchem.2014.07.002
Wang, Y., Geng, J., Guo, G., Wang, C., and Liu, S. (2011). N2O production in anaerobic/anoxic denitrifying phosphorus removal process: the effects of carbon sources shock. Chem. Eng. J. 172, 999–1007. doi: 10.1016/j.cej.2011.07.014
Wei, Y., Jiang, S., Tian, L., Wei, L., Jin, J., Ibánhez, J. S. P., et al. (2021). Benthic microbial biogeography along the continental shelf shaped by substrates from the Changjiang (Yangtze) river plume. Acta Oceanol. Sin. doi: 10.1007/s13131-021-1861-8
Weston, N. B., and Joye, S. B. (2005). Temperature-driven decoupling of key phases of organic matter degradation in marine sediments. PNAS 102, 17036–17040. doi: 10.1073/pnas.0508798102
Widerlund, A., and Davison, W. (2007). Size and density distribution of sulfide-producing microniches in lake sediments. Environ. Sci. Technol. 41, 8044–8049. doi: 10.1021/es071510x
Wu, B., Lan, T., Lu, D., and Liu, Z. (2014). Ecological and enzymatic responses to petroleum contamination. Environ. Sci. Process. Impacts 16, 1501–1509. doi: 10.1039/c3em00731f
Wu, Y., Zhu, K., Zhang, J., Müller, M., Jiang, S., Mujahid, A., et al. (2019). Distribution and degradation of terrestrial organic matter in the sediments of peat-draining rivers, Sarawak, Malaysian Borneo. Biogeosciences 16, 4517–4533. doi: 10.5194/bg-16-4517-2019
Yu, R.-C., Lü, S.-H., and Liang, Y.-B. (2018). “Harmful Algal Blooms in the Coastal Waters of China,” in Global Ecology and Oceanography of Harmful Algal Blooms, eds P. M. Glibert, E. Berdalet, M. A. Burford, G. C. Pitcher, and M. Zhou (Cham: Springer), 309–316. doi: 10.1007/978-3-319-70069-4_15
Yuan, M., Zhang, C., Jiang, Z., Guo, S., and Sun, J. (2014). Seasonal variations in phytoplankton community structure in the Sanggou, Ailian, and Lidao Bays. J. Ocean Univ. China 13, 1012–1024. doi: 10.1007/s11802-014-2305-2
Keywords: nitrate, dissolved organic matter, denitrification, subterranean estuary, flow through reactors
Citation: Jiang S, Jin J, Wu Y, Zhang Y, Wei Y, Rocha C, Ibánhez JSP and Zhang J (2021) Response of Nitrate Processing to Bio-labile Dissolved Organic Matter Supply Under Variable Oxygen Conditions in a Sandy Beach Seepage Face. Front. Mar. Sci. 8:642143. doi: 10.3389/fmars.2021.642143
Received: 15 December 2020; Accepted: 01 June 2021;
Published: 23 June 2021.
Edited by:
Christian Lønborg, Aarhus University, DenmarkReviewed by:
Sara Benelli, University of Parma, ItalySoeren Ahmerkamp, Max Planck Institute for Marine Microbiology (MPG), Germany
Kaijun Lu, University of Texas at Austin, United States
Copyright © 2021 Jiang, Jin, Wu, Zhang, Wei, Rocha, Ibánhez and Zhang. This is an open-access article distributed under the terms of the Creative Commons Attribution License (CC BY). The use, distribution or reproduction in other forums is permitted, provided the original author(s) and the copyright owner(s) are credited and that the original publication in this journal is cited, in accordance with accepted academic practice. No use, distribution or reproduction is permitted which does not comply with these terms.
*Correspondence: Shan Jiang, c2ppYW5nQHNrbGVjLmVjbnUuZWR1LmNu