- 1Instituto de Ciencias Marinas y Pesquerías, Universidad Veracruzana, Boca del Río, Mexico
- 2LGL Ecological Research Associates Inc., Bryan, TX, United States
Coral reefs are the most biodiverse ecosystems on earth and are presently experiencing severe declines globally. Shallow coral reef ecosystems (<30 m) have been studied extensively while mesophotic coral ecosystems (MCE) are poorly studied. As a result, MCE are rarely included in marine reserve design and management, despite their ecological importance and connectivity to shallow reefs. In this study, we assessed the fine-scale topographic complexity, a proxy for structural complexity, for a group of coastal coral reefs in a marine park in the southwestern Gulf of Mexico, in depths between 2 and 49 m. We conducted hydrographic surveys using a semi-portable multibeam echosounder system to produce 3D bathymetry digital terrain models (DTM) with a 2.5 m spatial resolution for three submerged bank reefs and two emerging reefs. From these models, descriptive terrain parameters were calculated for each reef, including slope, aspect, curvature, rugosity and ruggedness. Results show that all reefs are predominantly northeast-southwest oriented, with well-defined leeward and windward sides. For the three submerged bank reefs, structural complexity increased with depth. Estimated mean ruggedness and rugosity were highest at 20–40 m depth range on windward side slopes. Emerging reefs showed high structural complexity, particularly at the 25–40 m depth range. We identified a spur and groove zone with maximum ruggedness (0.26) and rugosity (3.17) values, and four channels with steep slopes (68°) and dispersed mounds. We found that at mesophotic depths (>30 m), southern reefs basements from two distinct reefs merge to form a continuous complex. This has important management implications since presently, only 28.7% of this reef complex (mostly shallow areas) are within the existing limits of the marine park’s core zone. Considering the newly recognized importance of MCE, we propose expanding and reshaping the core zone to include the entire reef complex which mostly encompasses MCE with high structural complexity. Our study illustrates the value of semi-portable MBES for marine planning in developing countries and remote poorly studied areas.
Introduction
Many studies have shown the global loss in live coral cover and critical degradation of coral reef ecosystems due to natural and anthropogenic disturbances (Gardner et al., 2003; Bellwood et al., 2004; Hoegh-Guldberg et al., 2007; Sweatman et al., 2011; De’ath et al., 2012; Gilmour et al., 2019). Evidence that the loss of live coral led to a drastic decline in the reef structural complexity has been shown for a region-wide scale in the Caribbean (Alvarez-Filip et al., 2009; Prachett et al., 2014; Bozec et al., 2015; Medina-Valmaseda et al., 2020). Such degradation trends generate uncertainty about the possible recovery of shallow coral reefs (Gardner et al., 2003) in which biodiversity, productivity and ecosystem services have been compromised (Moberg and Folke, 1999; Prachett et al., 2014). Current global-scale degradation of shallow coral reefs has encouraged the scientific community to study mesophotic coral ecosystems (MCE) (Menza et al., 2008) that typically occur between 30 and 150 m (Hinderstein et al., 2010) to determine if MCE are facing similar threats to those faced by shallow coral reefs (Bak et al., 2005; Reed et al., 2007; Lesser and Slattery, 2011; Bongaerts et al., 2013; Appeldoorn et al., 2015).
MCE are defined as light-dependent communities distributed between the middle and lower depths of the euphotic zone in tropical and subtropical regions (Hinderstein et al., 2010). Mesophotic reef depth limits vary within and between regions (Kahng et al., 2010) depending on water optical properties (Kirk, 2011). MCE occur at depths where downwelling irradiance reaches the 10 and 1% of subsurface irradiance (Jerlov, 1968). The euphotic zone may extend beyond 200 m deep in clear oceanic waters, while reduced to depths below 30 m near the coast because of increased water turbidity (Wright and Colling, 1995). MCE are considered an extension of shallow coral reefs since the distribution range of some species span from the shallow to the mesophotic zone (Kahng et al., 2010). Connectivity between shallow and mesophotic fish (Bejarano et al., 2014; Tenggardjaja et al., 2014; Papastamatiou et al., 2015) and coral species (Van Oppen et al., 2011; Holstein D.M. et al., 2016) suggest that MCE could be important contributors to reef ecosystem recovery.
Shallow coral reef ecosystems have been studied extensively while MCE remain poorly understood. Menza et al. (2008) showed an exponential decline in scientific literature on coral reefs as depth increases, showing the lack of information on MCE. Turner et al. (2017) showed that most of the studies on MCE are concentrated in a few geographic areas including Hawaii and Australia in the Pacific, Israel in the Middle East, the Caribbean and the northern Gulf of Mexico. Only 5.2% of those studies include reef geomorphology (Turner et al., 2017). The information gap on MCE is due in part to the financial and logistical constraints of conducting research in waters deeper than 30 m which requires specialized equipment and training (Locker et al., 2010).
Scleractinian corals are the dominant framework builders that provide the three-dimensional structure and complexity of coral reefs (Jones et al., 1994; Bruno and Bertness, 2001). Higher structural complexity is positively correlated with higher species biodiversity (Risk, 1972), reef fish biomass, and the abundance and size spectra of fish (Bell and Galzin, 1984; Rogers et al., 2014). Corals provide refuge for reef-dwelling species by reducing predation and competition (Almany, 2004) and space for larval settlement (Idjadi and Edmunds, 2006). Also, structural complexity has a positive effect on the provision of ecosystem services (Graham and Nash, 2013) including the important physical role of protecting coastal areas from waves (Harris et al., 2018).
Geomorphology in marine environments is an inherent physical attribute of the seabed. Geomorphic classification, based on maps of the seabed is a fundamental descriptor that provides a synthesis of attributes and information relevant for characterizing habitats (Harris, 2011) in scales from centimeters to kilometers (Greene et al., 1999). Specific geomorphic features are associated with particular suites of benthic habitats (Harris and Baker, 2011) and can be used as a proxy to identify critical life habitats of marine species (Heyman and Wright, 2011).
Benthic habitats are physically distinct areas of seabed associated with species, communities, or assemblages that consistently occur together (Harris and Baker, 2011). The physical structures of habitat, including its surfaces and substrates, are significant components of habitat complexity playing a key role in the function and structure of the marine communities (Bruno and Bertness, 2001). The habitat complexity of the aquatic systems is characterized by at least five different physical traits: (1) spatial scales, (2) diversity of complexity-generating physical elements, (3) elements spatial arrangement, (4) elements size and (5) elements abundance and density (Tokeshi and Arakani, 2012). Topographic variability is a primary component of habitat complexity. Topographic variability is a basic terrain parameter for seafloor characterization and can be used for delimiting regions or boundaries between habitats that in turn could be associated with distinct faunal assemblages (Wilson et al., 2007).
Coral reef structural complexity has been studied at different spatial scales and resolutions, using a variety of methods. The most commonly used field method is the belt chain, i.e., laying a chain directly over the substrate and estimating a rugosity index as a ratio of the actual surface distance relative to linear distance (Luckhurst and Luckhurst, 1978). Optical remote sensing has also been used to study landscape ecology, bathymetry, rugosity and even to assess the structural complexity of coral reefs (Brock et al., 2004; Zawada and Brock, 2009; Ferrari et al., 2016). Nonetheless, the utility of optical remote sensing techniques is restricted to clear shallow waters (Kenny et al., 2003; Wölfl et al., 2019). These techniques detect and measure energy patterns from different portions of the electromagnetic spectrum (Yang, 2009). Only wavelengths of the visible region of the spectrum (0.4–0.7 μm) penetrate the water column and this penetration decreases for longer wavelengths, becoming opaque for the infrared region (Green et al., 2000). The precise degree of penetration in a spectral region is influenced by the optical properties of the water, including the concentration of dissolved organic matter and suspended sediments (Mumby et al., 2004).
Acoustic hydrographic methods based on SoNAR (Sound Navigation and Ranging) or echosounders are suitable tools for seafloor mapping, which in turn supports geomorphic features identification and classification. These methods have been used in coral reefs, including the MCE (Locker et al., 2010; Sherman et al., 2010). Single beam echosounders (SBES) measure water depth by timing the period from when the echo is emitted by the SBES to its return from the seabed (Ainslie, 2010). Acoustic ground discrimination systems (AGDS) based upon an SBES, use the strength and character of the echo to provide an indication of the bottom type (Kenny et al., 2003; Walker et al., 2008; Bejarano et al., 2011). Multibeam echosounder systems (MBES) acquire multiple depth measurements simultaneously and are used to map large areas of the seafloor at high resolution up to 0.1 m (Kenny et al., 2003). Multibeam data combined with data gathered with remotely operated vehicles (ROVs) or autonomous underwater vehicles (AUVs) allows a detailed description of geomorphology (Armstrong et al., 2008), terrain variability (Sherman et al., 2010), topographic variability and its relationship with MCE benthic community structure (Bridge et al., 2011). Descriptors such as slope, texture and landforms have been used for benthic characterizations and benthic habitat delimitating (e.g., Wilson et al., 2007; Costa and Battista, 2013).
Seafloor mapping and habitat modeling are essential for marine spatial planning, designing marine protected areas (MPAs), planning and conducting scientific research on benthic ecosystems and marine geology, and assessing economic resources (Harris and Baker, 2011). The main objectives of this study are to: (1) generate fine-scale bathymetric maps for a group of poorly studied coastal coral reefs in a marine park in the southwestern Gulf of Mexico, including both shallow and mesophotic depths; (2) describe the seafloor geomorphology; (3) assess the topographic variability as a proxy for structural complexity to identify potential areas of interest for conservation and management.
Materials and Methods
Study Area
The “Sistema Arrecifal Veracruzano” National Park (SAVNP) in the southwestern Gulf of Mexico, includes 45 fringing and platform coral reef structures (Liaño-Carrera et al., 2019). The SAVNP is located in a terrigenous environment influenced by freshwater discharge from three rivers: the Antigua in the North, the Papaloapan to the South and the Jamapa in the middle (Krutak, 1997). Three weather seasons have been described for the region: dry, rainy and northerly (Gutiérrez de Velasco and Winant, 1996). Water turbidity varies seasonally according to weather conditions. Five coral reefs were selected for this study: three from the northern group and two from the southern group (Figure 1). The northern reefs, known as the “Holandesas” are three submerged banks located in front of the port of Veracruz which we refer to herein as Holandesa 1 (H1), Holandesa 2 (H2), and Holandesa 3 (H3). The southern reefs, commonly known as Santiaguillo and Anegadilla are emergent platform reefs located offshore of Anton Lizardo village (Liddell and Tunnell, 2011).
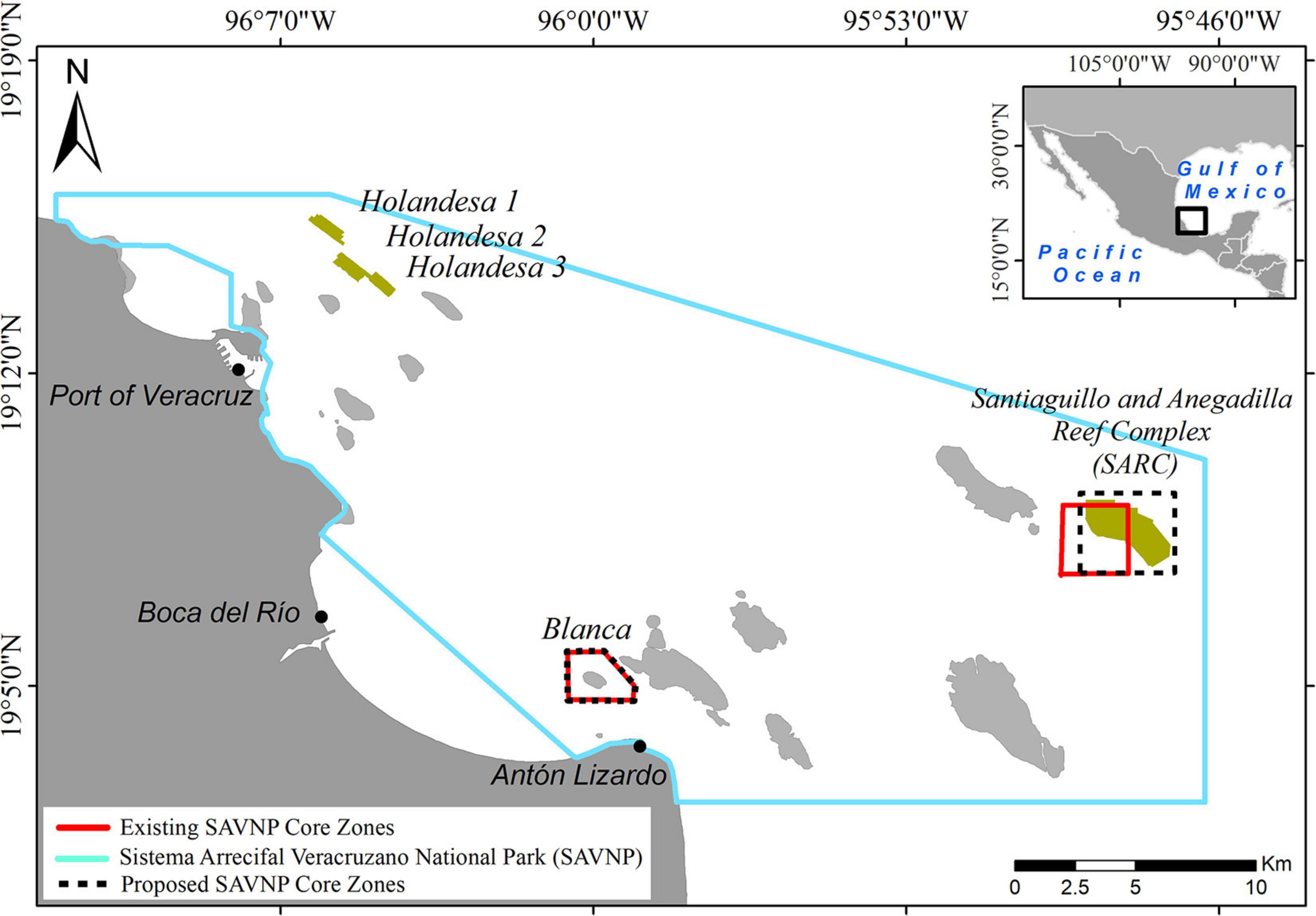
Figure 1. Sistema Arrecifal Veracruzano National Park (SAVNP) located in the southwestern Gulf of Mexico; the two groups of reefs studied are highlighted. Polygons in red indicate core zones; polygons in dashed line indicate the proposed core zone.
Hydrographic Surveys
Hydrographic surveys were carried out during 2015–2017 using a 30 foot Mexican style skiff equipped with an outboard engine and covered a total area of 6.42 km2. The boat was equipped with a semi-portable MBES consisting of a 400 kHz SONIC 2020 multibeam echosounder (R2Sonic, Texas, United States) pole-mounted port-side, amidship 0.60 m below the surface, a sound velocity Micro X probe (AML Oceanographic, British Columbia, Canada) mounted at the rear of the transducer, a differential GPS Receiver Vector VS131 (Hemisphere GNSS, Scottsdale, Arizona, United States) with two antennas mounted 1 m apart on top of the pole, an Inertial Navigation System Ekinox-E (SBG systems, Carrières-sur-Seine, France) aligned to the center line of the vessel and used as our central reference point, and the 1PPS box (Hypack/Xylem, Middle-town, Connecticut, United States) installed between the GPS and computer. The box was used to improve time-tagging by reducing the clock sync error to less than 1 ms.
The multibeam echosounder was operated at 300 kHz with an emitted fanned arc of 256 equidistant beams per ping through a 130° swath sector, sampled. Samples were recorded every 15 μs and received using a 60 kHz bandwidth. Surveys were conducted following 180 parallel-line transects at ∼2 km/h average speed. Transects were planned to obtain 100% sector coverage. Sound velocity casts were obtained using an SV-Xchange probe (AML Oceanographic, British Columbia, Canada). Calibration transects were conducted for each survey to determine pitch, roll, and yaw offsets.
Bathymetry Data Processing
Bathymetry data were processed using Hypack hydrographic software (version 2015). Sound velocity, tide, pitch, roll and yaw offsets corrections were applied according to the manual (Hypack, 2015). The noise was manually removed from every transect. The highest estimated resolution was 0.71 m; however, all data were scaled down to generate a standard 2.5 m gridded matrix (x, y, z) and exported to ArcMap software (ESRI, United States). A 3D bathymetry digital terrain model (DTM) was created in raster format with 2.5 m spatial resolution and projected to the UTM zone 14N Transverse Mercator Datum.
Topographic Variability Analysis
Topographic variability analysis was performed using the Benthic Terrain Modeler (BTM) tools for ArcMap, calculated using a 3 × 3 moving window (Walbridge et al., 2018). To describe the geomorphology and structural complexity of the reefs, several key terrain parameters and their variation were measured, as described in Table 1. A Pearson correlation coefficient was computed to assess the relationship between the depth and the slope, depth and ruggedness, as well as depth and rugosity for the H1, H2, and H3 coral reefs.
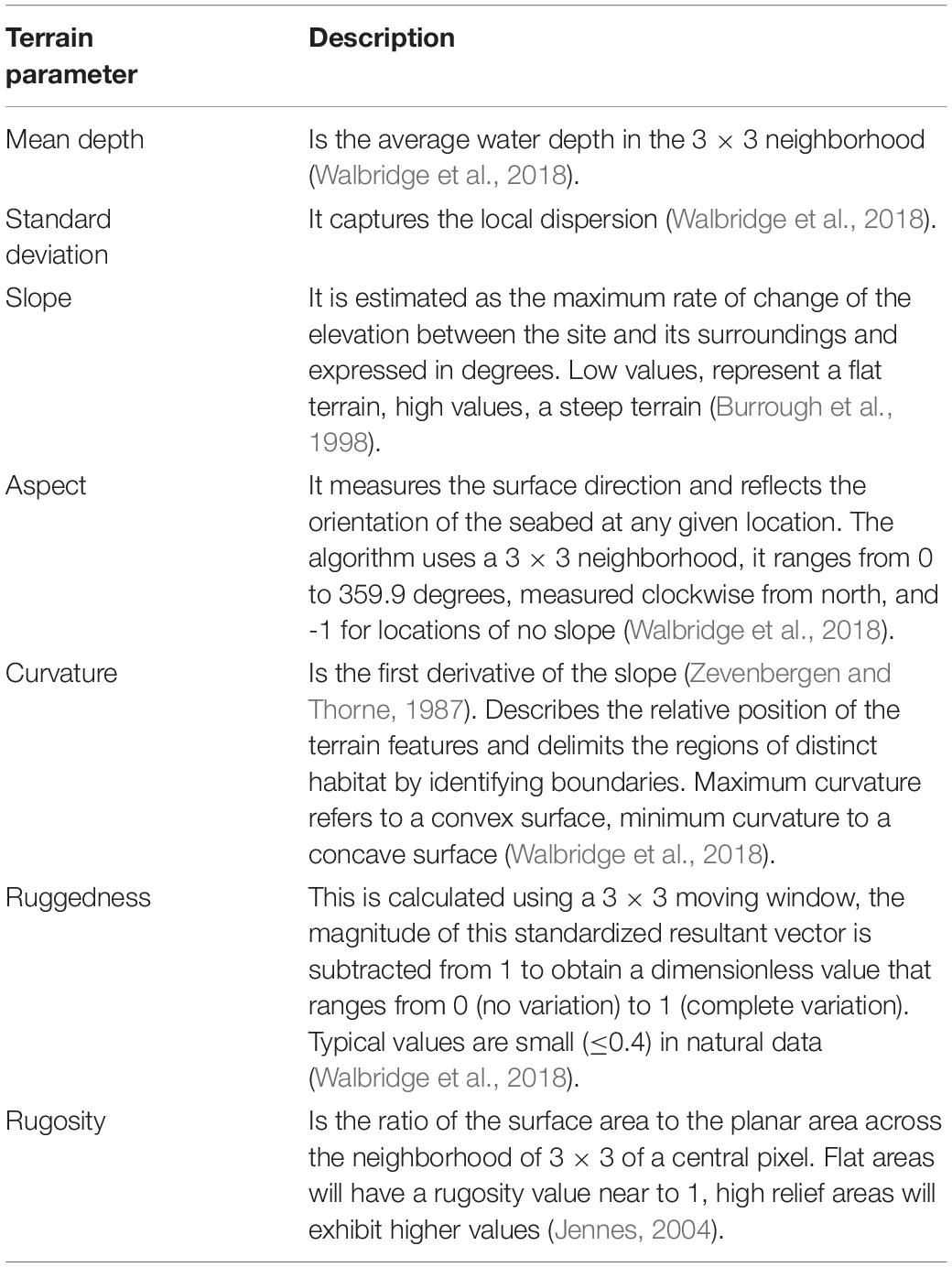
Table 1. Description of terrain parameters obtained from BTM (Walbridge et al., 2018).
Results
Coral Reef Geomorphology
For the Holandesas reef group, the depth ranged from 15 to 44 m (see Supplementary Material). Three-dimensional models (Figure 2) of those reefs showed three well-defined geomorphologic zones; (1) the leeward side, (2) the reef flat, and (3) the windward side. H1 and H3 present an oval-shape, while H2 is kidney-shaped with a concave bay at the leeward side. H1 is the largest of the northern reef group and has the deepest basement (Table 2).
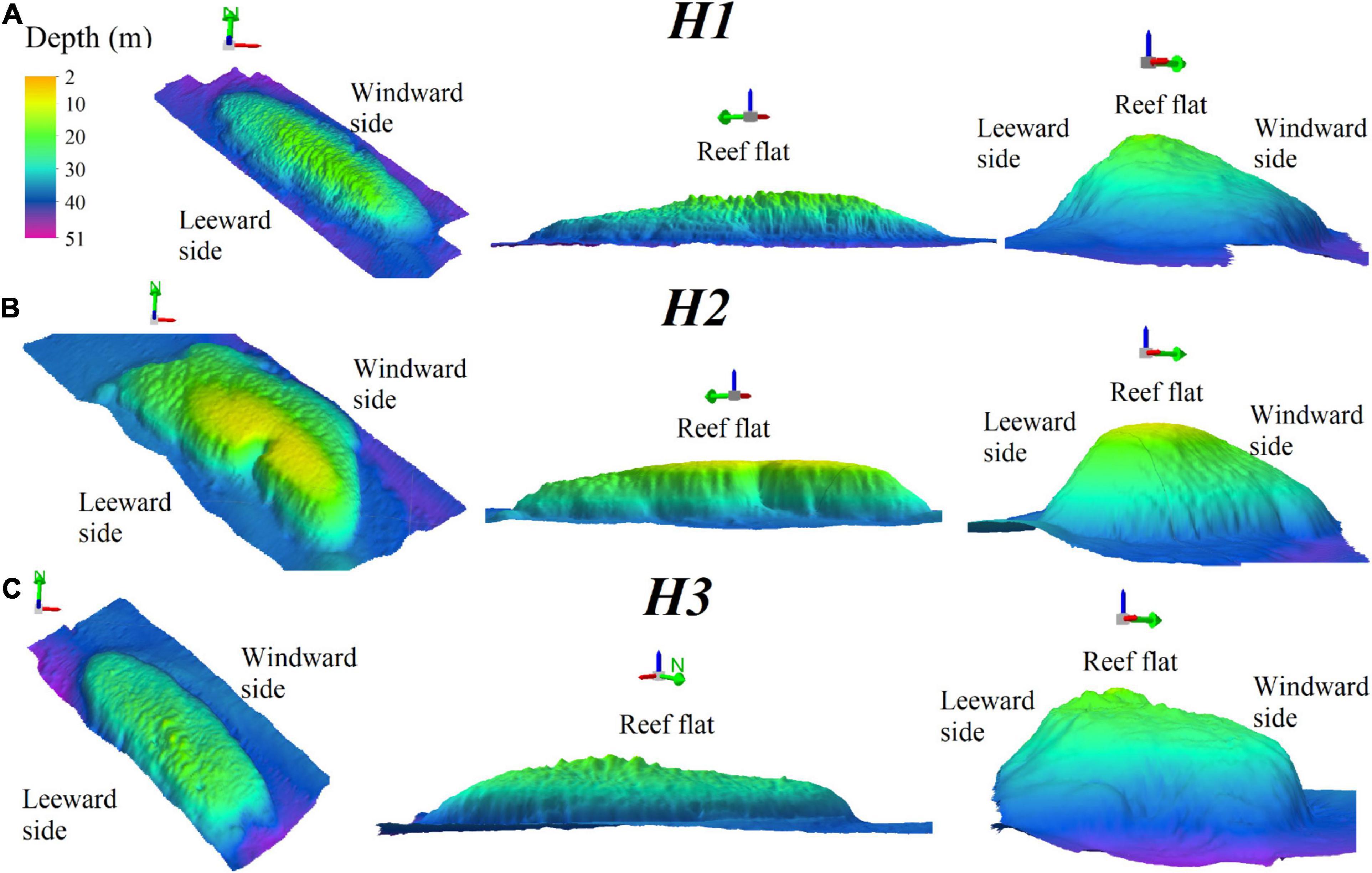
Figure 2. Three-dimensional bathymetric models of H1 (A), H2 (B), and H3 (C) showing a northwest-southeast orientation view from above, the lateral view for maximum length and the lateral view for minimum length; the green arrow points North. Vertical exaggeration 8x.
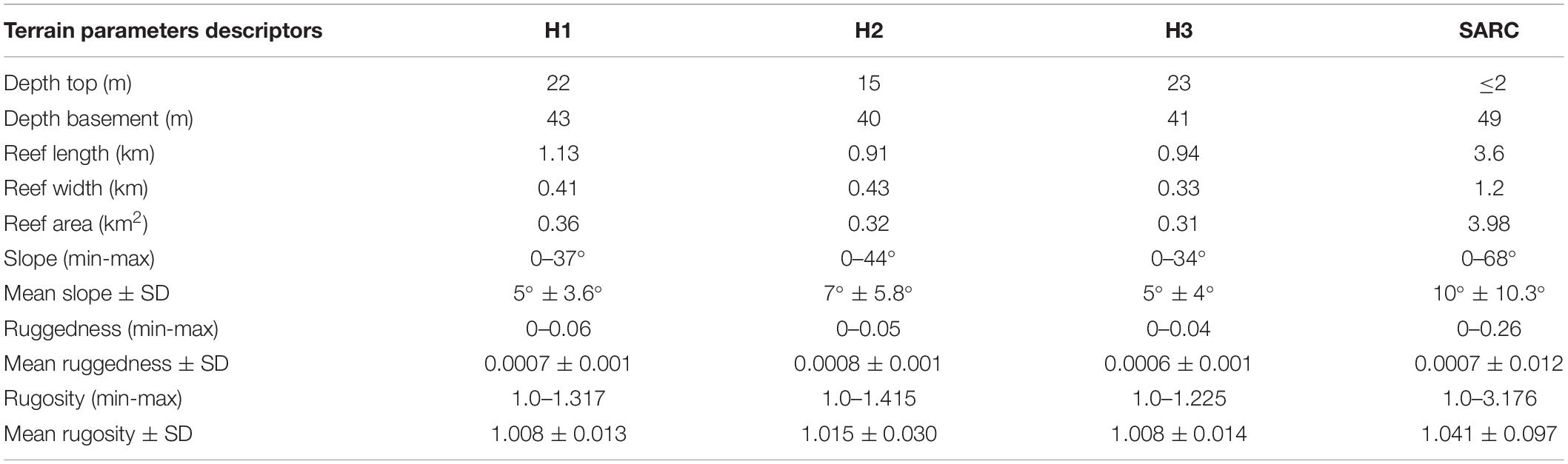
Table 2. Terrain parameters descriptors for each individual reef in the Holandesas group, and the SARC: depth basement (m), depth top (m), reef length (km), reef area (km2), slope (minimum-maximum), mean slope and standard deviation (SD), ruggedness (minimum-maximum), mean ruggedness (SD) rugosity (minimum-maximum), and mean rugosity (SD).
For Santiaguillo and Anegadilla reef group, the depth ranged from 2 to 51 m (see Supplementary Material). The reef complex is partially divided by a 0.9 km long channel (Channel-1) oriented northwest to southeast with a width ranging from 0.02 to 0.22 km and depths ranging from 32 to 38 m. Other channels include: Channel-2 which is 0.4 × 0.19 km having depths between 32 to 36 m, Channel-3 is 0.3 × 0.08 km with depths ranging from 33 to 36 m and Channel-4 is 0.7 × 0.07 km with depths ranging from 34 to 43 m. Multiple and well-defined mounds about 0.002–0.008 km2 area were identified, away from the main reef within sand beds surrounding the leeward side of Anegadilla (Figure 3A). Spur and groove were the dominant geomorphological features at depths > 30 m, where the reefs show high structural complexity and are continuous between the two reefs (Figure 3B). Our data suggest that Santiaguillo and Anegadilla may constitute a single reef complex, instead of two separate reefs. As such, for the rest of the analyses, these two coral reefs are hereafter referred as the “Santiaguillo and Anegadilla reef complex” (SARC).
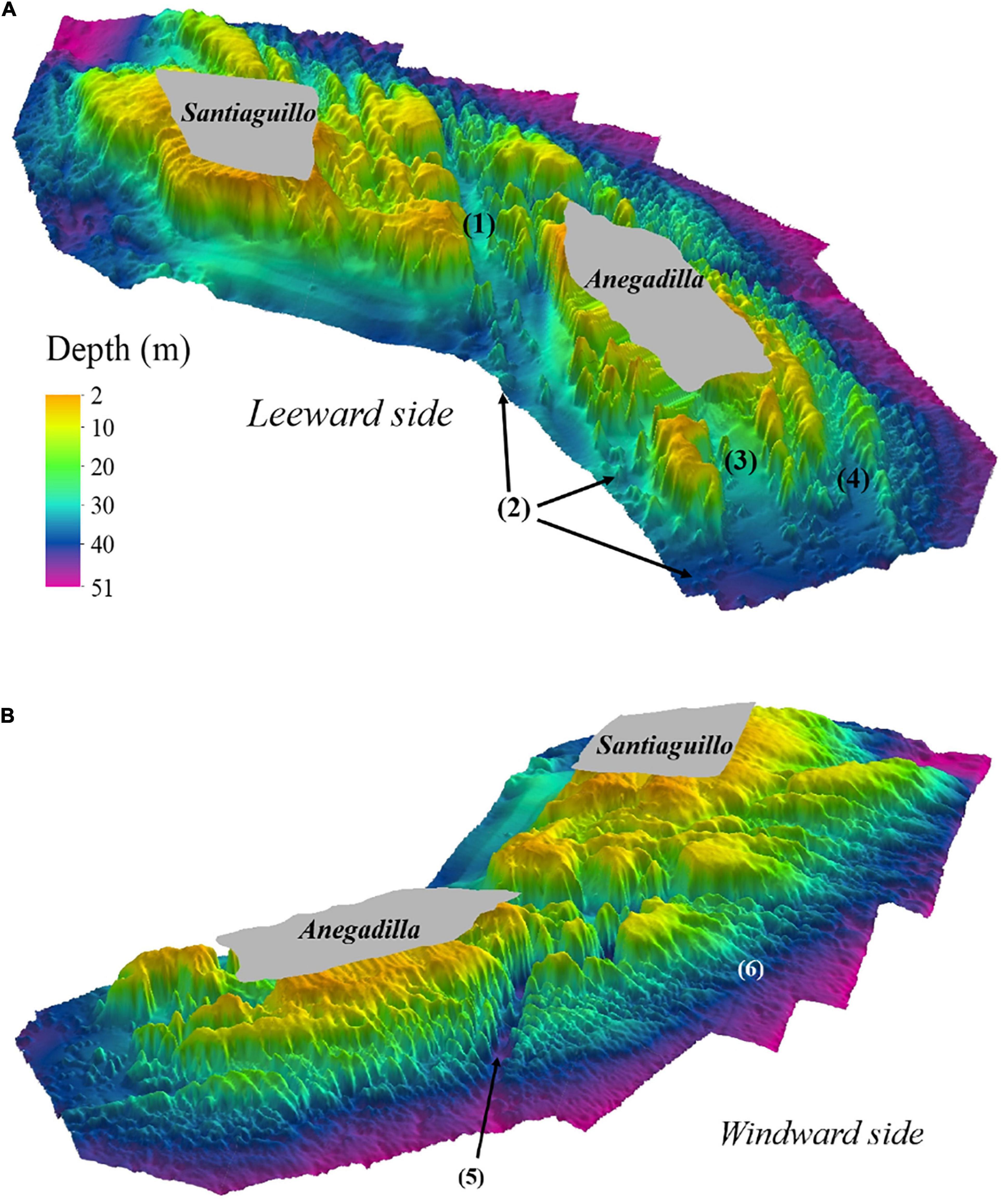
Figure 3. SARC’s three-dimensional bathymetric model, showing a leeward side view (A). Remarkable geomorphic features are shown: (1) Channel-1, (2) dispersed mounds, (3) Channel-2 and (4) Channel-3. SARCs’ three-dimensional bathymetric model, showing a windward side view (B). Geomorphic features shown are: (5) Channel-4 and (6) spur and grooves. Shallow areas (≤2 m deep) not surveyed are shown in gray. Vertical exaggeration 8x.
Structural Complexity of Holandesas Reef Group
The overall results of the estimated terrain parameters for the Holandesas reef group are summarized in Table 2 and the DTM of the topographic variability showing the results of the analysis are presented in Figure 4 in the following order: mean depth, depth standard deviation, slope, aspect, curvature, ruggedness and rugosity. The estimated mean depth ranged from 15 to 42 m and its standard deviation for depth ranged from 0 to 7.2 m. Steepest slopes (Table 2) are found in the 25–43 m depth range in specific areas: northeast windward side for H1, southwest leeward side for H2 and southeast windward side for H3. At the reef’s flat (within 20–25 m depth), H3 (4.8° ± 2.9°) and H1 (4.5° ± 3.1°) showed moderate mean slope. For the H2 reef flat (15–20 m depth), the mean slope was closer to level (3.2° ± 2.8°). The estimated mean slope increased significantly with depth [H1 r(56,149) = 0.17, p < 0.001, H2 r(53,039) = 0.49, p < 0.001, and H3 r(41,894) = 0.40, p < 0.001; Figure 6A].
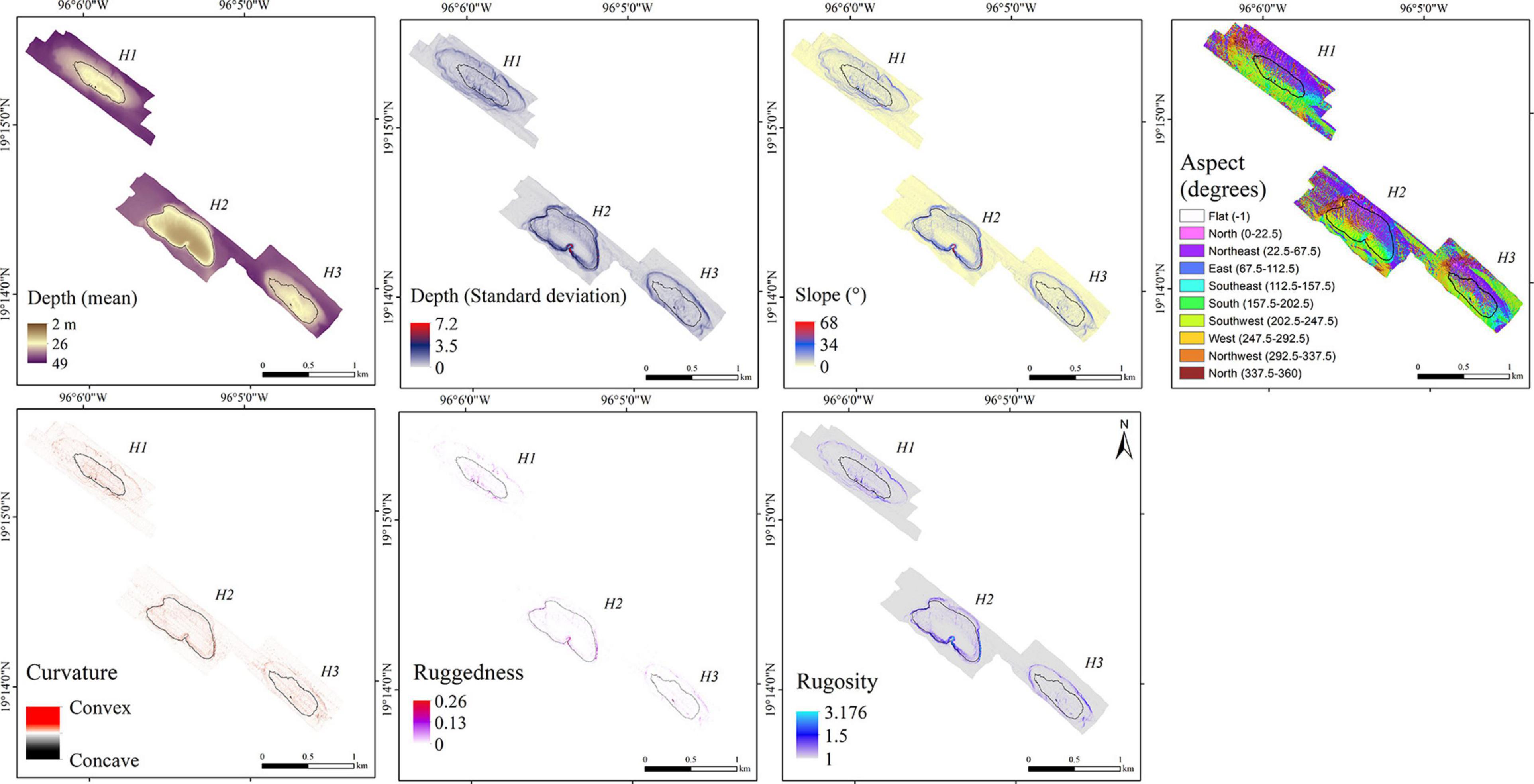
Figure 4. Digital terrain models showing the result of the topographic variability analysis: mean depth, depth standard deviation, slope, aspect, curvature, ruggedness and rugosity for the H1, H2 and H3; 30 m isobath shown in black.
The bank walls were mainly southwest and northeast oriented for the well-defined leeward and windward sides, respectively. For H1, the northeast surface area was 0.17 km2 (46.8% of the reef) and the southwest surface area was 0.16 km2 (46.3% of the reef). For H2, the northeast surface area was 0.18 km2 (56.2% of the reef); southwest surface area was 0.09 km2 (29%). For H3, the northeast surface area was 0.12 km2 (46.2% of the reef); southwest surface area was 0.09 km2 (34.6%).
Convex surfaces (curvature range from 100 to 356) delineate the edge of each reef from the Holandesas group. Concave surfaces (curvature range from −100 to −350) revealed notches on the edge of the reefs and depressed areas.
Ruggedness and rugosity analysis revealed highly structurally complex patchy areas that were found at the windward slopes. The H1 showed the maximum ruggedness and H2 showed the highest estimated mean ruggedness among the Holandesas, while H3 presented the lowest ruggedness. Maximum rugosity and estimated mean rugosity were higher for H2, followed for H1 and H3 (Table 2). Estimated mean ruggedness [H1 r(56,140) = 0.080, p < 0.001, H2 r(53,039) = 0.22, p < 0.001 and H3 r(41,894) = 0.14, p < 0.001] and rugosity increased significantly with increasing depth [H1 r(59,322) = 0.11, p < 0.001; H2 r(53,039) = 0.32, p < 0.001 and H3 r(41,894) = 0.33, p < 0.001], showing that deeper areas (up to 43 m) are structurally more complex (Figures 6B,C).
Structural Complexity of Santiaguillo and Anegadilla Reef Complex
The overall results of the estimated terrain parameters for the SARC are summarized in Table 2 and the DTM of the topographic variability showing the results of the analysis are presented in Figure 5 in the following order: mean depth, depth standard deviation, slope, aspect, curvature, ruggedness and rugosity. The estimated mean depth for SARC ranged from 2 to 49 m and its standard deviation ranged from 0 to 7.2 m. The overall estimated mean slope was 10° ± 10.3°. The mean slope was 6.9° ± 5.6° for shallow areas (5–10 m deep), increased up to 18° ± 12.9° between 20–25 m depth and decreased down to 4.4° ± 3.3° between 45 and 49 m depth (Figure 6A). The steepest areas were at the channel walls (68°) and the spur and groove areas ranging from 59° to 35°, within 35–49 m depth, respectively.
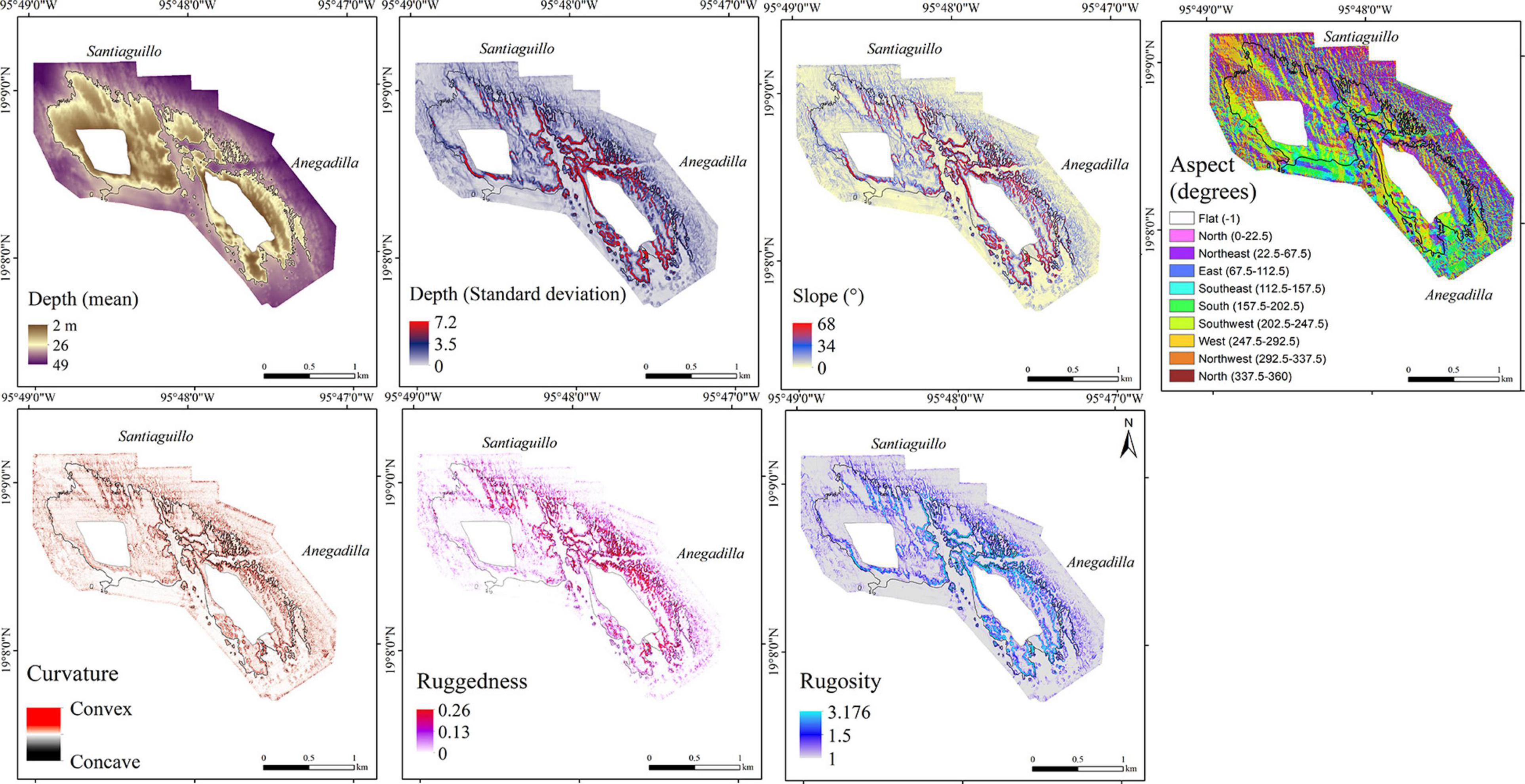
Figure 5. Digital terrain models showing the result of the topographic variability analysis: mean depth, depth standard deviation, slope, aspect, curvature, ruggedness and rugosity for the SARC; 30 m isobath shown in black.
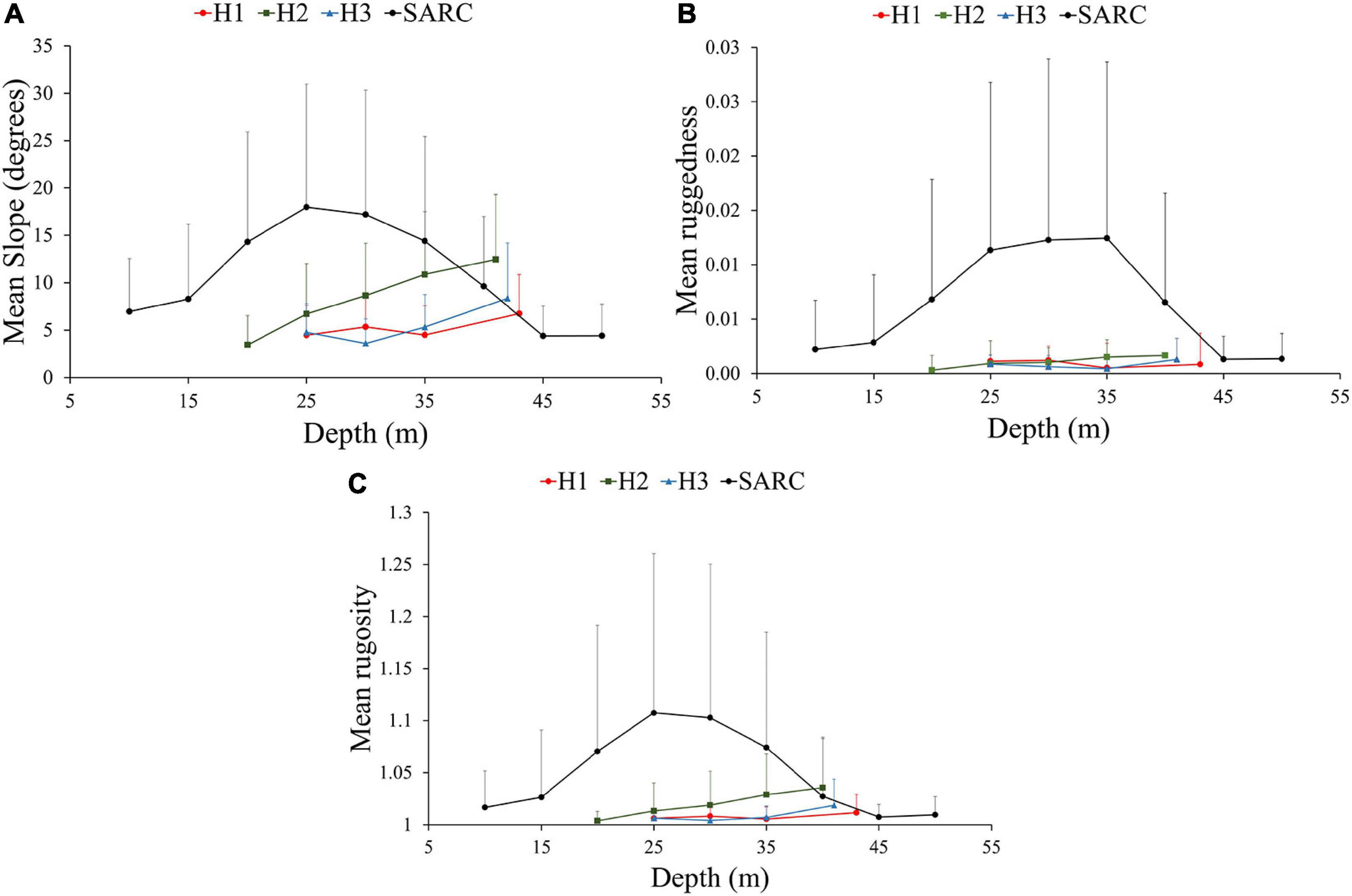
Figure 6. The estimated (A) mean slope, (B) mean ruggedness and (C) mean rugosity, for 5 m depth intervals with standard deviation bars (SD ± 1) for Holandesa group and the SARC.
Aspect analysis showed that leeward and the windward surfaces are both oriented in a southwest-northeast direction, with geomorphologically well-defined slopes. The northeast surface area was ≈1.88 km2, accounting for 47.2% of the total reef complex. The southwest surface area was approximately 0.97 km2, accounting for 24.4% of the total reef complex.
The convex surfaces (curvature range 100–356) delineated the channel walls, several mounds dispersed on the sand bed and spur and groove features. Concave surfaces (curvature range from -100 to -350) delineate the edge of the geomorphological features and depressed areas. In shallow areas (2–20 m deep) and the sand bed, the curvature surface ranged from 50 to −50.
The SARC showed the maximum values for slope, ruggedness and rugosity of all reefs (Table 2). Channel slopes and spur and groove zones within the 25–40 m depth range of the SARC had the highest structural complexity, considering both, ruggedness and rugosity. The shallow areas (5–10 m) showed the lowest structural complexity with 0.002 ± 0.004 mean ruggedness and 1.016 ± 0.03 mean rugosity. The highest mean ruggedness (0.012 ± 0.016) was observed for the 30–35 m depth range, while the highest mean rugosity (1.107 ± 0.15) was observed for the 20–25 m depth range. Structural complexity was lower for the 45–49 m depth range, with mean ruggedness of 0.002 ± 0.003 and rugosity of 1.017 ± 0.020 (Figures 6B,C). For the SARC, the middle depths showed the highest structural complexity, while the shallower and deeper areas showed lower structural complexity.
Discussion
Geomorphological Features
The platform reefs from the SAVPN are characterized by three zones: (1) leeward slope, (2) reef lagoon or reef flat, which shows a crest delimiting the fore reef and (3) windward slope, a surface orientated to the dominant swell (Chávez et al., 2007). For Santiaguillo and Anegadilla, previous descriptions indicated that they are geomorphologically well-formed platform reefs, characterized by relatively steep slopes (Lara et al., 1992), while Santiaguillo is topographically rough with cliffs (Chávez et al., 2007). Gross-scale geomorphological descriptions of Santiaguillo and Anegadilla from Lara et al. (1992) and Chávez et al. (2007) are consistent with our results. Nonetheless, our fine-scale geomorphological description of SARC provided more details than previous studies, demonstrating previously undescribed geomorphological features, including four channels at the windward side with steep slopes (68°) and high structural complexity based on ruggedness (0.26) and rugosity (3.176) and a spur and groove zones that merge at depths > 30 m and sandy flats with patchy mounds at the leeward side (Figure 3). Spur and groove features, shore-normal coral ridges separated by shore-normal patches of sediment (grooves) are typically the most prominent features of fore reefs (Storlazzi et al., 2003), which is consistent with results for the SARC. Fine-scale spur and groove geomorphological characterization is fundamental for the study and understanding of coral reef hydrodynamics processes. Rogers et al. (2013) showed that spur and groove formations drive circulation patterns and are linked to debris transportation processes outside the coral reef.
Definition of the windward and leeward side reef slopes was based on the aspect analysis, which reflects the predominant orientation to the northeast of the reef surface. According to Wilson et al. (2007), such an orientation may be the result of the local hydrodynamics. For the Holandesas reefs, which are bank submerged reefs, the aspect analysis revealed a well-defined slope at both leeward and windward sides deeper than 15 m. Aspect analysis includes the estimation of the slope and curvature in one measure (Walbridge et al., 2018), therefore, as a result of this study, we highlight the relevance of the aspect analysis to be used for defining zones for different types of reefs in an objective way, including emergent or submerged platform, as well as submerged bank coral reefs.
Surface curvature (concave or convex) is an important geomorphological feature that is liked to ecological processes. Concave surfaces typically accumulate current-carried sediment and foster colonization of sessile organisms (Tokeshi and Arakani, 2012), while convex surfaces favor fish grazing (Bellwood and Choat, 1990). Geomorphological features in coral reefs are related to complex interactions between local oceanographic conditions and ecological functions. Highly complex reef geomorphology is positively correlated to higher biodiversity (Risk, 1972). Analysis of spatial array and complexity of those features can help scientists and decision-makers to identify areas of interest for research, management and conservation, thus, reducing monitoring costs. This is particularly important for developing countries with low budgets, where, having an accurate and detailed bathymetric base map describing the most conspicuous geomorphologic features can be very cost-effective.
Structural Complexity of MCE in the Southwestern Gulf of Mexico
Our study is one of the first that describes and characterizes the MCE in the Southern Gulf of Mexico in a systematic way and provides the basis for assessing the ecological and economic implications of including MCE explicitly in management strategies for the region. Results presented herein differ from previous studies regarding reef extension and topographic variability. Our detailed results showed that the platform sizes of the Santiaguillo and Anegadilla reefs have been underestimated in previous studies that were based on aerial photographs and Mexican Naval Charts (Lara et al., 1992), single beam (Ortiz-Lozano et al., 2018), and multibeam (Liaño-Carrera et al., 2019). The differences in reef extension and topography previously reported can be attributed to methodological aspects and the spatial resolution used for data acquisition and interpolation (Kenny et al., 2003; Walbridge et al., 2018).
MBES data offers higher resolution and more accurate maps than the techniques used in previous studies and can thus be valuable for both ecological studies and management including MPA design. High spatial resolution bathymetric maps, such as those produced in our study, are useful for studying ecological, geological and oceanographic processes. According to Kenny et al. (2003), MBES provide larger seafloor coverage with greater precision (≥0.1 m) and it is a useful technique for studying the MCE spatial distribution (Locker et al., 2010). The 3D bathymetric maps and associated physical characterizations can serve as the basis for carefully designed benthic habitat studies in which replicate sampling sites can be selected from within each of the distinctive geomorphological zones. This can be used to validate the predictions of MCE associations with structural complexity in appropriate depth zones. The technological improvement of acoustic methods has allowed the development and use of semi-portable and relatively affordable equipment for geomorphological sea bottom exploration (Kenny et al., 2003). These improvements are critical for both developing countries and remote and poorly studied areas such as those in the southwestern Gulf of Mexico.
Geomorphology as a Proxy for Benthic Habitat
Our analyses revealed that the highest structural complexity was shown at mesophotic depths (>30 m), for the Holandesas and SARC, which is a remarkably interesting result that needs further study. Structural complexity has been shown to correlate positively with coral cover (Gardner et al., 2003). We are currently assessing the benthic community structure of MCE in the SAVNP and our preliminary results on the relative benthic cover showed that the general pattern of structure community of deeper areas host MCE communities mainly composed of fleshy macroalgae, turf algae, crustose coralline algae and in less proportion sponges, hermatypic coral and other sessile invertebrates (in preparation).
There is a well-documented decline in shallow coral cover (CC) and structural complexity in the Caribbean region (Gardner et al., 2003; Alvarez-Filip et al., 2009; Medina-Valmaseda et al., 2020). For the SAVNP, there is also a decline in CC that has been documented since 1970s for shallow areas (<20 m), where the coral cover dropped from 50 to 18% (Chávez et al., 2007; Horta-Puga, 2007; Horta-Puga et al., 2015; Pérez-España et al., 2015). Current CC is similar among the northern and southern coral reefs in the SAVNP (Horta-Puga et al., 2015). In this study, mesophotic areas (>30 m depth) showed high structural complexity for both reef groups, considering slope, ruggedness and rugosity (Figure 6). Preliminary results on relative benthic cover for the SARC, show that there is a considerable average of the relative cover of hermatypic coral for mesophotic depths, particularly in spur and groove zones (in preparation). Having the fine-scale geomorphological characterization is important for benthic habitat mapping, according to Sherman et al. (2010); the distribution of well-developed MCE can be in the form of patches (Sherman et al., 2010). According to Bridge et al. (2011), community structure distribution depends on small-scale geomorphology. They demonstrated that distinctive functional ecological groups were consistently associated with specific fine-scale habitat types. For example, autotrophs dominated the submerged reef flat tops while heterotrophs dominated steep slopes.
Management Implications
This study presents evidence of previously undescribed structural complexity and geomorphological continuity in deep SARC reefs (Figure 3). Our new data may have implications for the management of the SAVNP. In 1992, SAVNP was declared a National Park with a protected area of 532.38 km2 (Diario Oficial de la Federación [DOF], 1992). The general boundary of the SAVNP was modified in 2012 and is currently covering 655.16 km2. Core zone boundaries for both, Santiaguillo and Blanca reefs, cover 11.14 km2 (Diario Oficial de la Federación [DOF], 2012; Figure 1). Activities permitted in the core zone include only monitoring, scientific research and maritime equipment installation for navigation. According to the management plan (Programa de Manejo Parque Nacional Sistema Arrecifal veracruzano, 2017) the Santiaguillo core zone includes 7.12 km2 of reef area. However, when overlaid onto our bathymetric model using the published coordinates, the polygon covered only 1.84 km2, equal to only 25.8% of the SARC and includes flat sandy areas with low structural complexity. Based on our results we propose expanding the core zone to 12.2 km2 to include all of the areas with high structural complexity and associated MCE (Figure 1). Marine reserves can enhance the recovery of corals both within and outside the boundaries (Mumby and Harborne, 2010), promote resilience and connectivity by expanding the range of an MPA to include deep reefs (Abelson et al., 2016) since submerged reefs or deep habitats may contribute significantly to larval production (Thomas et al., 2015).
High structural complexity has been related to the provision of ecosystem services, supporting, local culture, tourism and fisheries. MCE provides essential habitat for fish and other species to spawn, shelter, feed and grow to maturity, particularly to important commercial fish (Bejarano et al., 2014; Holstein D. et al., 2016). The more structurally complex are MCE, the greater abundances of fish they have, as they can shelter under steep overhangs and in caves and crevices (Bejarano et al., 2014). In the SAVNP important ecosystem services have been reported: (1) regulation: protection to the coast, protection from erosion processes, (2) support: habitat and biodiversity maintenance, shelter; (3) cultural: recreation, tourism-recreational activities, and academic and research activities; and (4) Provision: fisheries (Arceo et al., 2010; Reyna-González et al., 2014). The structural complexity is an important element that divers take into consideration when selecting diving sites for recreational purposes (Williams and Polunin, 2000). Hence, the highest structural complexity areas are potential sites for recreational diving. However, appropriate regulation is needed for the management of these areas.
We support improved understanding of the MCE and their inclusion in studies, monitoring programs and management strategies (Rooney et al., 2010). The Flower Garden Banks National Marine Sanctuary (FGBNMS) in northwestern Gulf of Mexico, and the Papahānaumokuākea Marine National Monument (PMNM) in northwestern Hawaiian Islands (Papahānaumokuākea Marine National Monument, 2011), are some examples where MCE were included in specific management plans for conservation. FGBNMS includes three separate undersea features, the East and West Flower Garden Banks were designated a National Marine Sanctuary in 1992 and Stetson Bank was added in 1996. Fourteen additional reefs and banks were recently added (Office of National Marine Sanctuaries, 2020), in large part to provide additional protection for sensitive underwater features and marine habitats associated with continental shelf-edge reefs and banks in the northwestern Gulf of Mexico including MCE habitat, as Habitat Areas of Particular Concern (HAPC). HAPCs are high priority areas for conservation, management, or research because they are rare, sensitive, stressed by development, or important to ecosystem function, and they help prioritize and focus conservation effort (U.S. Department of Commerce National Oceanic and Atmospheric Administration, 2012).
Conclusion
The present study highlighted the importance of the use of new technologies such as the MBES, which provided accurate fine-scale bathymetric data for the 3D mapping and the assessment of the structural complexity from the shallow reefs to the mesophotic zone. This exploratory study of the geomorphology of a group of poorly studied coral reefs in the Southwestern Gulf of Mexico with a semi-portable MBES offers several novel findings. A striking result was discovering the physical continuity between Santiaguillo and Anegadilla reefs at depths >30 m on the windward side. Previously considered as two separate reefs our study demonstrates that Santiaguillo and Anegadilla are part of a single reef complex (SARC). This finding supports our proposal to expand the SAVNP core zone to include the entire SARC and thus enhance its contribution to coral reef recovery and resilience. In addition, our fine-scale geomorphology analysis revealed a large array of previously undescribed geomorphological features in the deeper areas of both reef groups, the Holandesas and SARC. Features include various channels, spur and grooves and sand flats with intermittent mounds.
Data Availability Statement
The raw data cannot be provided due to size (> 100 GB). A downscaled version of data can be provided by contacting first or corresponding authors.
Author Contributions
MM-M: idea developer, survey designer, data collection, processing and analysis. JB-P: idea developer, project responsible, funding administrator, data collection, processing and analysis. HP-V: data collection, processing and analysis. HP-E: data analysis. WDH: data analysis. All authors contributed to the article writing and approved the submitted version.
Funding
MM-M Ph.D. scholarship was funded by the Consejo Nacional de Ciencia y Tecnología (CONACyT). This project was funded by CONACyT PN-2015-01-606. Article processing charges were funded by Universidad Veracruzana.
Conflict of Interest
WDH is employed by the company LGL Ecological Research Associates Inc. which does no business in the study area.
The remaining authors declare that the research was conducted in the absence of any commercial or financial relationships that could be construed as a potential conflict of interest.
Supplementary Material
The Supplementary Material for this article can be found online at: https://www.frontiersin.org/articles/10.3389/fmars.2021.639359/full#supplementary-material
Abbreviations
AGDS, Acoustic ground discrimination systems; CC, Coral Cover; DTM, Digital Terrain Model; H1, Holandesa 1; H2, Holandesa 2; H3, Holandesa 3; MBES, Multibeam Echosounder System; MCE, Mesophotic Coral Ecosystems; MPA, Marine Protected Area; ROV, Remotely Operated Vehicle; SARC, Santiaguillo Anegadilla Reef Complex; SAVNP, Sistema Arrecifal Veracruzano National Park; SBES, Single beam echosounders; SoNAR, Sound Navigation and Ranging.
References
Abelson, A., Nelson, P. A., Edgar, G. J., Shashar, N., Reed, D. C., Belmaker, J., et al. (2016). Expanding marine protected areas to include degraded coral reefs. Conserv. Biol. 30, 1182–1191. doi: 10.1111/cobi.12722
Ainslie, M. A. (2010). Principles Of Sonar Performance Modeling. Berlin Heildelberg: Springer-Verlag. 2010.
Almany, G. R. (2004). Does increased habitat complexity reduce predation and competition in coral reef fish assemblages? Oikos 106, 275–284. doi: 10.1111/j.0030-1299.2004.13193.x
Alvarez-Filip, L., Dulvy, N. K., Gill, J. A., Cote, I. M., and Watkinson, A. R. (2009). Flattening of Caribbean coral reefs: region-wide declines in architectural complexity. Proc. R. Soc. B Biol. Sci. 276, 3019–3025. doi: 10.1098/rspb.2009.0339
Appeldoorn, R., Ballantine, D., Bejarano, I., Carlo, M., Nemeth, M., Otero, E., et al. (2015). Mesophotic coral ecosystems under anthropogenic stress: a case study at Ponce, Puerto Rico. Coral Reefs 35, 63–75. doi: 10.1007/s00338-0151360-5
Arceo, P., Pérez-España, H., Bello-Pineda, J., Granados-Barba, A., Salas-Monreal, D., and Ortiz-Lozano, L. (2010). “Economic evaluation of fisheries and tourist services of the Veracruz Reef System National Park, Mexico: A spatial approach,” in Proceedings of the 15th Biennial Conference of the International Institute of Fisheries Economics & Trade (iifet). Economics of fish Resources and Aquatic Ecosystems: Balancing uses, Balancing Costs, (Montpellier: International Institute of Fisheries Economics and Trade), 1–10.
Armstrong, R. A., Singh, H., Rivero, S., and Gilbes, F. (2008). Monitoring coral reefs in optically-deep waters. Proc. 11th Int. Coral Reef Symp. 1, 593–597.
Bak, R. P. M., Nieuwland, G., and Meesters, E. H. (2005). Coral reef crisis in deep and shallow reefs: 30 years of constancy and change in reefs of Curacao and Bonaire. Coral Reefs 24, 475–479.
Bejarano, I., Appeldoorn, R. S., and Nemeth, M. (2014). Fishes associated with mesophotic coral ecosystems in La Parguera, Puerto Rico. Coral Reefs 33, 313–328. doi: 10.1007/s00338-014-1125-6
Bejarano, S., Mumby, P. J., and Sotheran, I. (2011). Predicting structural complexity of reefs and fish abundance using acoustic remote sensing (RoxAnn). Mar. Bio. 158, 489–504. doi: 10.1007/s00227-010-1575-5
Bell, J. D., and Galzin, R. (1984). Influence of live coral cover on coral reef fish communities. Mar. Ecol. Prog. Ser. 15, 265–274. doi: 10.3354/meps015265
Bellwood, D. R., and Choat, J. H. (1990). A functional analysis of grazing in parrotfishes (family Scaridae): the ecological implications. Environ. Biol. Fish. 28:189–214. doi: 10.1007/bf00751035
Bellwood, D. R., Hughes, T. P., Folke, C., and Nyström, M. (2004). Confronting the coral reef crisis. Nature 429, 824–833.
Bongaerts, P., Muir, P., Englebert, N., Bridge, T. L. C., and Hoegh-Guldberg, O. (2013). Cyclone damage at mesophotic depths on Myrmidon Reef (GBR). Coral Reefs 32:935. doi: 10.1007/s00338-013-1052-y
Bozec, Y. M., Álvarez-Filip, L., and Mumby, P. J. (2015). The dynamics of architectural complexity on coral reefs under climate change. Glob. Chang. Biol. 21, 223–235. doi: 10.1111/gcb.12698
Bridge, T. C. L., Done, T. J., Beaman, R. J., Friedman, A., Williams, S. B., Pizarro, O., et al. (2011). Topography, substratum and benthic macrofaunal relationships on a tropical mesophotic shelf margin, central Great Barrier Reef. Coral Reefs 30, 143–153. doi: 10.1007/s00338-010-0677-3
Brock, J. C., Wright, C. W., Clayton, T. D., and Nayeghandhi, A. (2004). LIDAR optical rugosity of coral reefs in Biscayne National Park, Florida. Coral Reefs 23, 48–59. doi: 10.1007/s00338-003-0365-7
Bruno, J. F., and Bertness, M. D. (2001). “Habitat modification and facilitation in benthic marine communities,” in Marine Community Ecology, eds M. D. Bertness, S. D. Gaines, and M. E. Hay (Sunderland, Massachusetts: Sinauer Associates, Inc), 201–218.
Burrough, P. A., McDonell, R. A., and Lloyd, C. D. (1998). Principles of Geographical Information Systems. New York: Oxford University Press.
Chávez, E. A., Tunnell, J. W., and Whiters, K. (2007). “Coral reef zonation and ecology: Veracruz shelf and Campeche Bank,” in Coral Reefs of the Southern Gulf of Mexico, eds J. W. Tunnell, E. A. Chávez, K. Withers, and S. Earle (College Station, Texas: Texas A&M University Press). muse.jhu.edu/book/2721.
Costa, B. M., and Battista, T. A. (2013). The semi-automated classification of acoustic imagery for characterizing coral reef ecosystems. Int. J. Remote Sens. 34, 6389–6422. doi: 10.1080/01431161.2013.800661
Diario Oficial de la Federación [DOF], (1992). Decreto Por El Que Se Declara Área Natural Protegida Con Carácter De Parque Nacional La Zona Conocida Como Sistema Arrecifal Veracruzano. Mexico: Secretariat of the Interior. Lunes 24 de agosto de 1992.
Diario Oficial de la Federación [DOF], (2012). Decreto Que Modifica Al Diverso Por El Que Se Declara Área Natural Protegida, Con El Carácter De Parque Marino Nacional, La Zona Conocida Como Sistema Arrecifal Veracruzano. Mexico: Secretariat of the Interior. Jueves 29 de noviembre de 2012.
De’ath, G., Fabricius, K. E., Sweatman, H., and Puotinen, M. (2012). The 27-year decline of coral cover on the Great Barrier Reef and its causes. PNAS 109, 17995–17999. doi: 10.1073/pnas.1208909109
Ferrari, R., McKinnon, D., He, H., Smith, R. N., Corke, P., González-Rivero, M., et al. (2016). Quantifying Multiscale Habitat Structural Complexity: a Cost-Effective Framework for Underwater 3D Modelling. Remote Sens. 8:113. doi: 10.3390/rs8020113
Gardner, T. A., Côte, I. M., Gill, J. A., Grant, A., and Watkinson, A. R. (2003). Long-term region-wide declines in Caribbean corals. Science 301, 958–960. doi: 10.1126/science.1086050
Gilmour, J. P., Cook, K. L., Nicole, M. R., Poutinen, M. L., Green, R. H., Shedrawi, G., et al. (2019). The state of Western Australia’s coral reefs. Coral Reefs 38, 651–667. doi: 10.1007/s00338-019-01795-8
Graham, N. A. J., and Nash, K. L. (2013). The importance of structural complexity in coral reef ecosystems. Coral Reefs 32, 315–326. doi: 10.1007/s00338-012-0984-y
Green, E. P., Mumby, P. J., Edwards, A. J., and Clark, C. D. (2000). Remote sensing handbook for tropical coastal management. Coastal management sourcebooks 3. Paris: UNESCO.
Greene, H. G., Yoklavich, M. M., Starr, R. M., O’Connell, V. M., Wakefield, W. W., Sullivan, D. E., et al. (1999). A classification scheme for deep seafloor habitats. Oceanol. Acta 22, 663–678. doi: 10.1016/s0399-1784(00)88957-4
Gutiérrez de Velasco, G., and Winant, C. D. (1996). Seasonal patterns of wind stress and wind stress curl over the Gulf. J. Geophys. Res. 101, 18127–18140. doi: 10.1029/96jc01442
Harris, D. L., Rovere, A., Casella, E., Power, H., Canavesio, R., Collin, A., et al. (2018). Coral reef structural complexity provides important coastal protection from waves under rising sea levels. Sci. Adv. 4:eaao4350. doi: 10.1126/sciadv.aao4350
Harris, P. T. (2011). “Seafloor geomorphology – Coast, shelf, and abyss,” in Seafloor Geomorphology as Benthic Habitat, eds P. T. Harris and E. K. Baker (Netherlands: ELSEVIER), doi: 10.1016/B978-0-12-385140-6.00001-3
Harris, P. T., and Baker, E. K. (2011). “Why Map Benthic Habitats?,” in Seafloor Geomorphology as Benthic Habitat, eds P. T. Harris and E. K. Baker (Netherlands: ELSEVIER), 3–22. doi: 10.1016/B978-0-12-385140-6.00001-3
Heyman, W., and Wright, D. J. (2011). Marine geomorphology in the design of marine reserve networks. Prof. Geog. 63, 429–442. doi: 10.1080/00330124.2011.585074
Hinderstein, L. M., Marr, J. C. A., Martinez, F. A., Dowgiallo, M. J., Puglise, K. A., Pyle, R. L., et al. (2010). Theme section on Mesophotic Coral Ecosystems: characterization, Ecology, and Management. Coral Reefs 29, 247–251. doi: 10.1007/s00338-010-0614-5
Hoegh-Guldberg, O., Mumby, P. J., Hooten, A. J., Steneck, R. S., Greenfield, P., Gomez, E., et al. (2007). Coral reefs under rapid climate change and ocean acidification. Science 318, 1737–1742.
Holstein, D. M., Paris, C. B., Vaz, A. C., and Smith, T. B. (2016). Modeling vertical coral connectivity and mesophotic refugia. Coral Reefs 35, 23–37. doi: 10.1007/s00338-015-1339-2
Holstein, D., Smith, T. B., and Appeldoorn, R. S. (2016). “Ecosystem services provided by mesophotic coral ecosystems,” in Mesophotic Coral Ecosystems – A Lifeboat For Coral Reefs?, eds E. K. Baker, K. A. Puglise, and P. T. Harris (Nairobi and Arendal: The United Nations Environment Programme and GRID-Arendal), 98.
Horta-Puga, G. (2007). “Environmental impacts, in Coral reefs of the southern Gulf of Mexico,” in Coral Reefs of the Southern Gulf of Mexico, eds J. W. Tunnell, E. A. Chávez, K. Withers, and S. Earle (College Station, Texas: Texas A&M University Press). muse.jhu.edu/book/2721.
Horta-Puga, G., Tello-Musi, J. L., Beltrán-Torres, A., Carricart-Ganivet, J. P., Carriquiry, J. D., and Villaescusa-Celaya, J. (2015). “Veracruz Reef System: a hermatypic coral community thriving in a sedimentary terrigenous environment,” in Aportes al conocimiento del Sistema Arrecifal Veracruzano: hacia el Corredor Arrecifal del Suroeste del Golfo de México, eds A. Granados-Barba, L. Ortiz-Lozano, D. Salas-Monreal, and C. González-Gándara (Campeche: Universidad Autónoma de Campeche), 181–208.
Idjadi, J. A., and Edmunds, P. J. (2006). Scleractinian corals as facilitators for other invertebrates on a Caribbean reef. Mar. Ecol. Prog. Ser. 319, 117–127. doi: 10.3354/meps319117
Jennes, J. S. (2004). Calculating landscape surface area from digital elevation models. Wildl. Soc. Bull. 32, 829–839. doi: 10.2193/0091-7648(2004)032[0829:clsafd]2.0.co;2
Jones, C. G., Lawton, J. H., and Shachak, M. (1994). Organism as ecosystem engineers. Oikos 69, 373–386.
Kahng, S. E., Garcia-Sais, J. R., Spalding, H. L., Brokovich, E., Wagner, D., Weil, E., et al. (2010). Community ecology of mesophotic coral reef ecosystems. Coral Reefs 29, 255–275.
Kenny, A. J., Cato, I., Desprez, M., Fader, G., Schüttenhelm, R. T. E., and Side, J. (2003). An overview of seabed-mapping technologies in the context of marine habitat classification. ICES J. Mar. Sci. 60, 411–418. doi: 10.1016/s1054-3139(03)00006-7
Kirk, J. T. O. (2011). Light And Photosynthesis In Aquatic Ecosystems. Cambridge: Cambridge University Press, 665.
Krutak, P. R. (1997). Petrography and provenance of siliciclastic sediments, Veracruz-Anton Lizardo Reefs, Mexico: a hybrid carbonate-siliciclastic system. Rev. Soc. Mex. Hist. Nat. 47, 167–177.
Lara, M., Padilla, C., García, C., and Espejel, J. J. (1992). “Coral Reef of Veracruz Mexico I. Zonation and Community,” in Proceedings of the Seventh International Coral Reef Symposium, (Mangilao, GU: University of Guam Press), 535–544.
Lesser, P., and Slattery, M. (2011). Phase shift to algal dominated communities at mesophotic depths associated with lionfish (Pterois volitans) invasion on a Bahamian coral reef. Biol. Invasions. 13, 1855–1868. doi: 10.1007/s10530-011-0005-z
Liaño-Carrera, F., Camarena-Luhrs, T., Gómez-Barrero, A., Martos-Fernández, F. J., Ramírez-Macias, J. I., and Salas-Monreal, D. (2019). New coral reef structures in a tropical coral reef system. LAJAR 47, 270–281.
Liddell, W. D., and Tunnell, jr. J. W (2011). “Mexican coral reefs,” in Gulf of Mexico, origin, waters, and biota: Volume 3, Geology, eds N. A. Buster and C. W. Holmes (College Station, Texas: Texas A&M University Press), 354.
Locker, S. D., Armstrong, R. A., Battista, T. A., Rooney, J. J., Sherman, C., and Zawada, D. G. (2010). Geomorphology of mesophotic coral ecosystems: current perspectives on morphology, distribution, and mapping strategies. Coral Reefs 29, 329–345. doi: 10.1007/s00338-010-0613-6
Luckhurst, B. E., and Luckhurst, K. (1978). Analysis of the influence of substrate variables on coral reef fish communities. Mar. Biol. 49, 317–323. doi: 10.1007/bf00455026
Medina-Valmaseda, A. E., Rodríguez-Martínez, R. E., Álvarez-Filip, L., Jordan-Dahlgren, E., and Blanchon, P. (2020). The role of geomorphic zonation in long-term changes in coral-community structure on a Caribbean fringing reef. PeerJ 8:e10103. doi: 10.7717/peerj.10103
Menza, C., Kendall, M., and Hile, S. (2008). The deeper we go the less we know. Rev. Biol. Trop. 56, 11–24.
Moberg, F., and Folke, C. (1999). Ecological goods and services of coral reef ecosystems. Ecol. Econ. 29, 215–233. doi: 10.1016/s0921-8009(99)00009-9
Mumby, P. J., and Harborne, A. R. (2010). Marine reserves enhance the recovery of corals on Caribbean reefs. PLoS One 5:e8657. doi: 10.1371/journal.pone.0008657
Mumby, P. J., Skirving, W., Strong, J., Hardy, E., LeDrew, E., Hochberg, E., et al. (2004). Remote sensing of coral reefs and their physical environment. Mar. Pollut. Bull. 48, 219–228.
Office of National Marine Sanctuaries. (2020). Flower Garden Banks National Marine Sanctuary Expansion Final Environmental Impact Statement. U.S. Department of Commerce, National Oceanic and Atmospheric Administration, Office of National Marine Sanctuaries. Silver Spring, Maryland: Office of National Marine Sanctuaries.
Ortiz-Lozano, L., Colmenares-Campos, C., and Gutiérrez-Velázquez, A. (2018). Submerged Coral Reefs in the Veracruz Reef System, Mexico, and its implications for marine protected area management. Ocean. Coast. Manag 158, 11–23. doi: 10.1016/j.ocecoaman.2018.03.012
Papahānaumokuākea Marine National Monument. (2011). Maritime Heritage Research, Education, and Management Plan. Honolulu, Hawai’i: Papahānaumokuākea Marine National Monument, 97.
Papastamatiou, Y. P., Meyer, C. G., Kosaki, R. K., Wallsgrove, N. J., and Popp, B. N. (2015). Movements and foraging of predators associated with mesophotic coral reefs and their potential for linking ecological habitats. Mar. Ecol. Prog. Ser. 521, 155–170. doi: 10.3354/meps11110
Pérez-España, H., Ávila-Gutiérrez, P. S., Melo-Merino, S. M., Berumen-Solórzano, P., and Flores-Arévalo, R. R. (2015). “Patrones interanuales e interarrecifales de las comunidades de peces, corales y equinodermos en el Sistema Arrecifal Veracruzano,” in Aportes al conocimiento del Sistema Arrecifal Veracruzano: hacia el Corredor Arrecifal del Suroeste del Golfo de México, eds A. Granados-Barba, L. D. Ortiz-Lozano, D. Salas-Monreal, and C. González-Gándara (Campeche: Universidad Autónoma de Campeche), 159–178.
Prachett, M., Hoey, A. S., and Wilson, S. K. (2014). Reef degradation and the loss of critical ecosystem goods and services provided by coral reef fishes. J. Environ. Sustain. 7, 37–43. doi: 10.1016/j.cosust.2013.11.022
Programa de Manejo Parque Nacional Sistema Arrecifal veracruzano. (2017). Secretaría de Medio Ambiente y Recursos Naturales. Mexico: Comisión Nacional de Áreas Naturales Protegidas.
Reed, J. K., Koening, C. C., and Shepard, A. N. (2007). Impacts of bottom trawling on a deep-water Oculina coral ecosystem off Florida. Bull. Mar. Sci. 81, 481–496.
Reyna-González, P. C., Bello-Pineda, J., Ortiz-Lozano, L., Pérez-España, H., Arceo, P., and Brenner, J. (2014). Incorporating expert knowledge for development spatial modeling in assessing ecosystem services provided by coral Reefs: a tool for decision-making. Rev. Biol. Mar. Oceanogr. 49, 279–292. doi: 10.4067/s0718-19572014000200008
Risk, M. J. (1972). Fish diversity on a coral reef in the Virgin Islands. Atoll Res. Bull. 153, 1–7. doi: 10.5479/si.00775630.153.1
Rogers, A., Blanchard, J. L., and Mumby, P. J. (2014). Vulnerability of coral reef fisheries to a loss of structural complexity. Curr. Biol. 24, 1000–1005. doi: 10.1016/j.cub.2014.03.026
Rogers, J. S., Monismith, S. G., Feddersen, F., and Stolarzzi, C. D. (2013). Hydrodynamics of spur and groove formations on a coral reef. J. Geophys. Res. Oceans 118, 1–15. doi: 10.1002/jgrc.20225
Rooney, J., Donham, E., Montgomery, A., Spalding, H., Parrish, F., and Boland, R., et al. (2010). Mesophotic coral ecosystems in the Hawaiian Archipelago. Coral Reefs. 29, 361–367.
Sherman, C., Nemeth, M., Ruíz, H., Bejarano, I., Appeldoorn, R., Pagán, F., et al. (2010). Geomorphology and benthic cover of mesophotic coral ecosystems of the upper insolar slope of southwest Puerto Rico. Coral Reefs 29, 347–360. doi: 10.1007/s00338-010-0607-4
Storlazzi, C. D., Logan, J. B., and Field, M. E. (2003). Quantitative morphology of a fringing reef tract from high-resolution laser bathymetry: southern Molokai, Hawaii. Geol. Soc. Am. Bull. 115, 1344–1355. doi: 10.1130/b25200.1
Sweatman, H., Delean, S., and Syms, C. (2011). Assessing loss of coral cover on Australia’s Great Barrier Reef over two decades, with implications for longer-term trends. Coral Reefs 30, 521–531. doi: 10.1007/s00338-010-0715-1
Tenggardjaja, K. A., Bowen, B. W., and Bernardi, G. (2014). Vertical and horizontal genetic connectivity in Chromis verater, an endemic damselfish found on shallow and mesophotic reefs in the Hawaiian Archipelago and adjacent Johnston Atoll. PLoS One 9:e115493. doi: 10.1371/journal.pone.0115493
Thomas, C. J., Bridge, T. C. L., Figueiredo, J., Deleersnijder, E., and Hanert, E. (2015). Connectivity between submerged and near-sea-surface coral reefs: can submerged reef populations act as refuges? Divers. Distrib. 21, 1254–1266. doi: 10.1111/ddi.12360
Tokeshi, M., and Arakani, S. (2012). Habitat complexity in aquatic systems: fractals and beyond. Hydrobiologia 685, 27–47. doi: 10.1007/s10750-011-0832-z
Turner, J. A., Babcock, R. C., Hovey, R., and Kendrick, G. A. (2017). Deep thinking: a systematic review of mesophotic coral ecosystems. ICES J. Mar. Sci 74, 2309–2320..
U.S. Department of Commerce National Oceanic and Atmospheric Administration, (2012). Flower Garden Banks National Marine Sanctuary Final Management Plan. Silver Spring. MD: Createspace Independent.
Van Oppen, M. J. H., Bongaerts, P., Underwood, J. N., Peplow, L. M., and Cooper, T. F. (2011). The role of deep reefs in shallow reef recovery: an assessment of vertical connectivity in a brooding coral from west and east Australia. Mol. Ecol. 20, 1647–1660. doi: 10.1111/j.1365-294x.2011.05050.x
Walbridge, S., Slocum, N., Pobuda, M., and Wright, D. J. (2018). Geomorphological Analysis Workflows with Benthic Terrain Modeler. Geosciences 8:94. doi: 10.3390/geosciences8030094
Walker, B. K., Riegl, B., and Dodge, R. E. (2008). Mapping coral reef habitats in Southeast Florida using combined technique approach. J. Coast. Res. 24, 1138–1150. doi: 10.2112/06-0809.1
Williams, I. D., and Polunin, N. V. C. (2000). Differences between protected and unprotected reefs of the western Caribbean in attributes preferred by dive tourists. Environ. Conserv. 27, 382–391. doi: 10.1017/s0376892900000436
Wilson, M. F. J., O’Connell, B., Brown, C., Guinan, J. C., and Grehan, A. J. (2007). Multiscale Terrain Analysis of multibeam bathymetry data for habitat mapping on the continental slope. Mar. Geod. 30, 3–35. doi: 10.1080/01490410701295962
Wölfl, A. C., Snaith, H., Amirebrahimi, S., Devey, C. W., Dorschel, B., Ferrini, V., et al. (2019). Seafloor mapping – The challenge of a truly global ocean bathymetry. Front. Mar. Sci. 6:283. doi: 10.3389/fmars.2019.00283
Wright, J., and Colling, A. (1995). “Light and sound on seawater,” in Seawater: Its Composition Properties And Behaviour, ed. G. Bearman (Amsterdam: Elsevier), 61–84. doi: 10.1016/b978-075063715-2/50006-x
Yang, X. (2009). “Remote sensing, geospatial technologies and coastal ecosystems,” in Remote Sensing And Geospatial Technologies For Coastal Ecosystem, ed. X. Yang (Berlin Heidelberg: Springer-Verlag), 561.
Zawada, D. G., and Brock, J. C. (2009). A multiscale analysis of coral reef topographic complexity using Lidar-derived bathymetry. J. Coast. Res. 53, 6–15.
Keywords: bathymetry, geomorphology, Gulf of Mexico, mapping, mesophotic coral ecosystem, multibeam echosounder, structural complexity
Citation: Mayorga-Martínez M, Bello-Pineda J, Perales-Valdivia H, Pérez-España H and Heyman WD (2021) Characterizing Geomorphology of Mesophotic Coral Reef Ecosystems in the Southwestern Gulf of Mexico: Implications for Conservation and Management. Front. Mar. Sci. 8:639359. doi: 10.3389/fmars.2021.639359
Received: 08 December 2020; Accepted: 05 May 2021;
Published: 02 June 2021.
Edited by:
Stuart A. Sandin, University of California, San Diego, United StatesReviewed by:
Xinming Lei, South China Sea Institute of Oceanology (CAS), ChinaCaroline Eve Dubé, Laval University, Canada
Copyright © 2021 Mayorga-Martínez, Bello-Pineda, Perales-Valdivia, Pérez-España and Heyman. This is an open-access article distributed under the terms of the Creative Commons Attribution License (CC BY). The use, distribution or reproduction in other forums is permitted, provided the original author(s) and the copyright owner(s) are credited and that the original publication in this journal is cited, in accordance with accepted academic practice. No use, distribution or reproduction is permitted which does not comply with these terms.
*Correspondence: Javier Bello-Pineda, amFiZWxsb0B1di5teA==