- School of Biological Sciences, Monash University, Clayton, VIC, Australia
For animals discarded after fishing capture (unwanted bycatch), the effects of fishing-capture stress can extend beyond immediate or delayed death, causing long-term, sub-lethal effects such as injuries and physiological, behavioral, immune, energetic, and reproductive consequences. Given the importance of successful reproduction for population recruitment, investigating reproductive impairment is essential, even more so for animals with low reproductive rates such as chondrichthyans. However, data for these species are lacking, even though the poor conservation status of many chondrichthyan populations and the threats from overexploitation and discard require a better understanding of fishing-capture stress consequences. In this study, we investigated the reproductive consequences of trawling and air exposure stress when experienced by southern fiddler rays (Trygonorrhina dumerilii) during late pregnancy, with a focus on neonatal traits. Compared with neonates from unstressed mothers, neonates born from mothers subjected to capture simulation had significantly lower body mass and yolk sac volume at birth, showed a granulocyte-to-lymphocyte ratio indicative of a stressed condition, reduced growth, altered burying behavior, reduced boldness, and swam for shorter distances after a simulated predator attack. Smaller size and lower growth rate might expose neonates to a higher rate of predation, and similarly, the altered burying and swimming behaviors may reduce their ability to escape from predators. Decreased boldness could impact neonate survival by reducing their ability to compete and obtain food and resources. Further investigations are needed, but these initial results suggest that capture stress suffered by pregnant rays may alter traits and survival of their offspring with consequences for recruitment and population abundance.
Introduction
Chondrichthyans (sharks, rays, and chimeras) are among the most threatened groups of vertebrates (Dulvy et al., 2008, 2014; IUCN, 2020). The high vulnerability of these species originates from their peculiar life-history traits of late sexual maturity, long reproductive cycles, long gestation or embryonic development, and low fecundity (Camhi et al., 1998), resulting in low reproductive rates and low ability to recover from population decreases. Among various threats, the most severe and frequent are overfishing and discard, i.e., the release of unwanted captured animals due to management measures or low economic value (Dulvy et al., 2008, 2014; Ferretti et al., 2010). For chondrichthyans, discard is a serious problem (Molina and Cooke, 2012) because fishing procedures can cause injuries and extreme homeostatic alterations resulting in immediate or delayed death (Frick et al., 2010; Braccini et al., 2012). Even when fishes survive, the elicited stress response, which is necessary to maintain or restore homeostasis and increase survival (Skomal and Mandelman, 2012), can also be detrimental if fishing stressors are severe or prolonged. Indeed, chronic alterations of homeostasis, increased stress hormone levels, and altered energetic allocation may result in long-term consequences such as altered growth, immune competence, behavior, and reproduction, which ultimately affect survival and fitness (Wendelaar-Bonga, 1997; Skomal and Mandelman, 2012; Watson et al., 2020). Given the importance of successful reproduction to the maintenance of sustainable populations, reproductive impairments that affect the individuals’ ability to contribute to increasing population abundance through recruitment or affect the survival of their offspring need to be understood (Schreck et al., 2001; Sopinka et al., 2016a). This is especially important for chondrichthyans, not only because of the poor conservation status of many populations but also because of the generally large energy investment in a single reproductive event (Hamlett et al., 2005; Trinnie et al., 2012) and long reproductive cycles (up to 3 years in school shark Galeorhinus galeus; Hamlett et al., 2005; Walker, 2005b). Therefore, reproductive impairments could jeopardize the large investment already allocated to reproduction over the several prior years, further hindering population recovery.
In teleost fishes, stressors can affect a large variety of reproductive traits, both in parents, including reproductive hormone concentrations, reproductive behavior, vitellogenesis, gamete quality, fecundity, and ovulation timing, and in offspring born from stressed parents, such as behavior, hatching success, size, growth, swimming performance, feeding ability, and survival (Schreck et al., 2001; Milla et al., 2009; Leatherland et al., 2010; Schreck, 2010; Li and Leatherland, 2012; Pankhurst, 2016; Sopinka et al., 2016a). Few data exist for chondrichthyans and almost entirely concern observations of capture-induced premature parturition and abortion (Adams et al., 2018; Wosnick et al., 2019; de Sousa Rangel et al., 2020). Given the low probability of neonates surviving after premature parturition (Charvet-Almeida et al., 2005; Campbell et al., 2018), this is the most severe outcome representing the likely complete failure of the reproductive event. However, even when not causing premature parturition, in southern fiddler rays (Trygonorrhina dumerilii), trawling-capture stress experienced by mothers during pregnancy affects neonate size at birth and causes a state of chronic stress in neonates, potentially reducing survival in the long-term (Guida et al., 2017). In teleosts, various other offspring traits associated with survival are affected by parental stress, and given the similarity of many of the stress consequences reported in teleosts and chondrichthyans (Wendelaar-Bonga, 1997; Skomal and Mandelman, 2012), further research aimed at verifying whether these alterations occur also in chondrichthyan offspring is needed. These data will help better assess the extent of the fishing-capture consequences and the potential need for management measures to improve the conservation status of these threatened populations. Moreover, studying stress effects in wild animals is intrinsically difficult due to various confounding factors that cannot be controlled, and especially for poorly researched areas, reliable conclusions can be drawn only from investigating different stress indices and their consequences (Johnstone et al., 2012, 2017), calling for further research on this topic in chondrichthyans.
In this experiment, the consequences of maternal exposure to trawling capture simulation and air (Frick et al., 2010) during late pregnancy on neonatal traits in T. dumerilii (Castelnau, 1873) were investigated. T. dumerilii is a lecithotrophic viviparous species, meaning that, during development, embryos rely solely on yolk sac reserves. Females give birth to two to five neonates yearly in April–May (Marshall et al., 2007). The species is commonly captured by commercial and recreational fisheries in southern Australian waters; and due to low market values, most animals, including pregnant females (Marshall et al., 2007), are released after capture (Walker and Gason, 2007; Last and Stevens, 2009). Despite not classed in a high extinction risk category (IUCN, 2020) and despite being quite resilient to fishing-capture stress, even when pregnant (Guida et al., 2017; Martins, 2017), the reproductive impairments previously observed by Guida et al. (2017) in pregnant mothers and neonates of this species indicate the need for further research. Investigations were focused on alteration in traits that persist in neonate life, including size, growth rate, residual yolk sac volume and its consumption rate, swimming performance, and burying and boldness behavior.
Materials and Methods
Animal Collection and Husbandry
In March and April 2018, 14 pregnant T. dumerilii females were hand-collected in Swan Bay, Victoria, Australia. Pregnancy was determined in-water by the presence of embryos in the uteri using ultrasound scanning (L6.2 linear transducer probe at 8–5 MHz, Ibex Pro Portable Ultrasound, E. I. Medical Imaging, Loveland, CO., United States). Upon collection, pregnant animals were placed in a 200-L holding tank, with a continuous flow of ambient seawater and transported to the housing facility (Victorian Fisheries Authority, Queenscliff, Victoria, Australia) in <2 h with up to three animals (20 kg combined mass) held together in the holding tank. At the facility, females’ body mass (BMF) to the nearest 0.1 kg and total length (TLF) to the nearest 0.5 cm were measured. Females were tagged applying different color combinations of cable ties to their tail (loose enough to avoid skin injuries) to allow identification of each individual. Animals were randomly assigned to two experimental groups (see below) and transferred to two separate 9,000-L circular housing tanks (seven animals in each tank, for a maximum biomass of 45 kg). The tanks were located in the open air, under a covered structure and supplied with continuously flowing ambient seawater and aeration. Animals were exposed to natural photoperiod and water temperature (mean ± SD: 17.2 ± 1.1°C) and fed sardines and prawns twice a week (∼10% of their total BM per week). Females were maintained in captivity until a maximum of 4 days after parturition and, after veterinary health check, were released back in Swan Bay. All experiments were performed under Monash University Animal Ethics Protocol BSCI/2016/37, Parks Victoria research permit no. 10008588, VFA general permit no. RP1286, and complied with the current Australian law.
Experimental Design
The 14 near-term, pregnant females were randomly assigned to one of two maternal treatment groups. Females in the trawl group (n = 7) were subjected to trawl simulation with air exposure, while those in the control group (n = 7) were treated as controls. The consequences for neonates were investigated by assessing stress at birth and monitoring morphometric and behavioral changes during the first 30 days after birth. The effects of maternal treatment on number of stillborn neonates and neonate size, volume of yolk reserves, and stress, indicated by blood parameters, at birth were investigated. Growth, indicated by length and body-mass increments, and consumption of the yolk, indicated by the percentage reduction in yolk sac volume, were measured over 30 days. The effects of maternal treatment on neonate behavior expressed as burying success, time to bury, the time before initial movement, total time spent moving, and speed and distance moved in response to simulated predation attack were also examined.
Maternal Treatments of Trawling Simulation With Air Exposure and Control
Trawling capture was simulated at least 14 days after collection to allow recovery from the initial capture stress and acclimation to the housing conditions. Females were also used in a metabolic study (Finotto et al., unpublished), and due to limitations on equipment availability for that study, each female in the trawl group was towed one at each time. Females experienced the trawling simulation on different days, and it took 7 days to expose all females to the treatment conditions. Females were dip-netted from the housing tank and individually placed inside a cod-end (a 110-cm-long bag constructed of monofilament webbing, 102 mm between opposite knots of diamond-shaped mesh if stretched) mounted on a circular support hanging from a metal bar suspended over a 19,000-L experimental tank. In the center of the tank, a rotating wood panel (2.0 × 1.0 m; W × H) generated a water current of ∼0.6 m s–1 in front of the cod-end, simulating the condition of towing a net through the water (Frick et al., 2010). Each female was towed for 7 h, then retrieved from the cod-end, and placed for the 30-min air exposure in an empty tub (2.0 × 1.0 × 0.7 m; L × W × H) outdoors in a shaded area. At the end of the air exposure period, females in the trawl group had their metabolic rate measured and then were transferred back to the housing tank where they remained until 4 days after parturition. Each day, concomitantly with each female of the trawl group, a female in the control group experienced the same dip-netting, handling, and transfer (to a 5,000-L tank) procedures but was neither towed nor measured for metabolic rate. The fact that Trawl females experienced the confinement in a respirometry chamber during metabolic measurement, while Control females did not, might have influenced their stress load. However, blacktip reef sharks (Carcharhinus melanopterus) spending 3 h in a respirometry chamber did not experience the physiological alterations associated with a stress response (Bouyoucos et al., 2018). Therefore, it seems unlikely that the small stress potentially associated with metabolic measurement significantly impacted Trawl females and subsequent measurements, especially when compared with the highly stressful conditions of the trawling simulation. The experimental tank was supplied with constant seawater flow such that the whole mass of water was replaced between successive trawl simulations in separate days, to eliminate the possibility of stress-related chemical clues influencing the responses of animals treated subsequently.
Neonates’ Morphological Measurements
Parturition was indicated by the presence of neonates in the two separate tanks holding the Trawl mothers and Control mothers observed during daily inspections, and the numbers of live and stillborn neonates were recorded. T. dumerilii litter size ranges from two to five pups (Marshall et al., 2007), so the presence of four or more pups indicated that potentially two females gave birth the same night. The identification of the female(s) that gave birth was initially based on visual inspection of females’ body appearance because near-term pregnancy is evident from dorsal bulges on the two sides, which disappear immediately after parturition (de Sousa Rangel et al., 2020). To confirm parturition, females suspected to have given birth were dip-netted, drawn to the side of the housing tank, and, while lightly restrained, checked with the ultrasound. This protocol allowed assigning a female to each parturition event. On one occasion, two females gave birth the same night. From ultrasound scanning performed at initial capture, an estimate of the number of embryos carried by each female was available. Moreover, data collected during a preliminary experiment highlighted that neonate total length (TLN) at birth was positively correlated with maternal size [linear mixed effects (LME) model: TLN ∼ TLM + (1| MotherID); TLN ∼ 21.95 + 0.04 × TLM; F1,20 = 4.35, p = 0.037] and that siblings showed similar coloration patterns (Figure 1). Based on the similarity of the coloration patterns among siblings, on maternal and neonate sizes and on data on the number of the embryos determined by ultrasound scanning the mothers when initially captured, it was possible to assign all neonates to the respective mother.
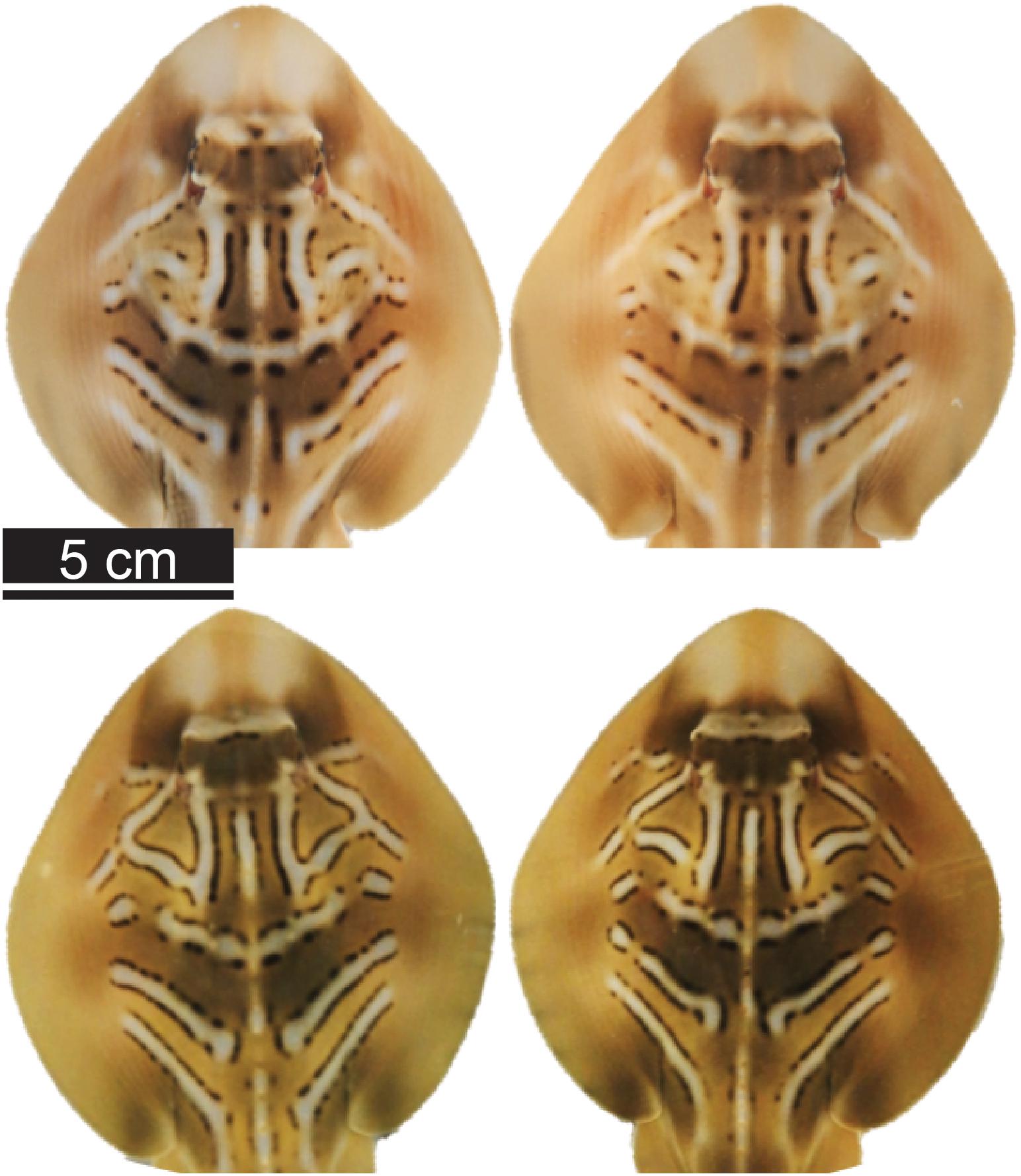
Figure 1. Dorsal photos of southern fiddler ray (Trygonorrhina dumerilii) neonates. Photos show the similarity in coloration and pattern between pairs of siblings (pair A, top two photos; and pair B, bottom two photos).
At birth (day 0), living neonates were individually removed from mothers’ housing tanks and transferred to a bucket filled with a known mass of water; and BM (BMN) to the nearest 0.1 g and total length (TLN) to the nearest 0.1 cm were measured. A blood sample (500 μl) was collected in <1 min through caudal venipuncture using a 27-gage needle and a 3-ml heparinized syringe. The dorsal side of each neonate was photographed, allowing later identification by checking its coloration pattern (Figure 1). Neonates underwent longitudinal and transversal ultrasound scanning (Figure 2), and from still images, using the measuring tool provided by the ultrasound machine software, the length, height, and width of neonate internal yolk sac (LY, HY, and WY; mm) were estimated and used to calculate yolk sac volume (see section “Data Analysis”). Afterward, neonates from both maternal treatment groups were transferred to a single 5,000-L housing tank (maximum of 20 neonates for a biomass of 2,273.4 g), fed chopped prawns every other day (∼10% of their BM weekly), maintained in captivity for 30 days, and then, after a veterinary health check, released in Swan Bay. All the neonates born live survived the period in captivity.
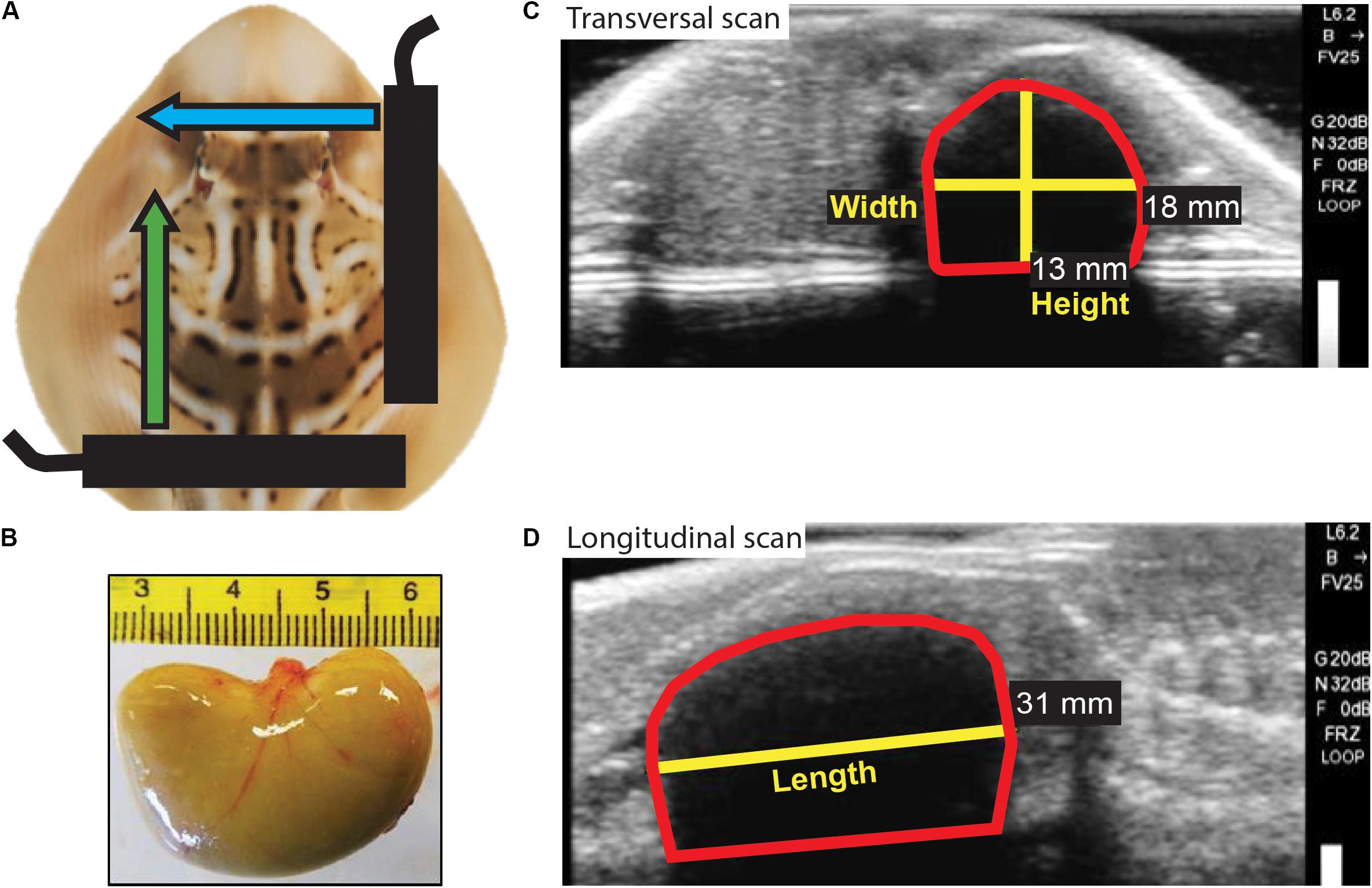
Figure 2. Neonate yolk sac measurements. (A) Schematic representation of ultrasound scans performed on neonates to obtain a longitudinal view and a transversal view of the yolk sac. Black bars represent the ultrasound probe; blue and green arrows represent the movement of the probe during the longitudinal and transversal scans, respectively. (B) Photo of the bean-shaped yolk sac excised from a stillborn neonate. (C) Still image obtained from the transversal scan and used to measure yolk sac height and width. (D) Still image obtained from the longitudinal scan and used to measure yolk sac length. The red shape in (C) and (D) delineates the yolk sac.
Neonate Growth and Yolk Sac Consumption Rates
For a month after the birth of each neonate, every 10 days (days 10, 20, and 30), measurements of BM and total length and ultrasound, to monitor the volume of the remaining internal yolk sac, were performed as described above. To reduce the number of times neonates had to be dip-netted from the tank and the minor stress associated with the procedure, neonate swimming performance and behaviors (see below) were tested concomitantly with these measurements.
Swimming Test
Swimming was tested on day 10. Neonates were individually placed at the entrance of a 2-m-long corridor set inside a 5,000-L tank. The corridor was built shaping two portions of plastic-coated metallic wire mesh weighted down on the bottom of the tank. An enclosed funnel-shaped space, separated from the main section of the corridor by a removable panel, served as an acclimation area. The neonate was allowed to settle on the bottom of the tank in the acclimation area (<2 min), and after removal of the panel, it was gently touched on the tail to simulate a predator attack and stimulate its movement (Gyssels and Stoks, 2005). Three consecutive trials were performed and video-recorded. Most neonates did not swim from the entrance to the end of the corridor in a single movement but stopped at various distances from the entrance. On these occasions, neonates were again touched on the tail to stimulate swimming. If movement did not resume within 5 s, the test was concluded. At the end of the test, neonates were transferred back to their housing tank.
For each neonate and trial, the length of the segment covered during the first burst of swimming (Swimming distance, m) was measured from a still image of the video analyzed in ImageJ (Rasband, 2018). The time taken for the neonate to swim to the end of the corridor, measured starting from after the first stimulus and including any time the neonate stopped, was recorded to calculate completion speed (see section “Data Analysis”).
Burying and Boldness Tests
Each neonate underwent two different tests, one on day 20 and one on day 30. Neonates were individually transferred to a separate tank (100 cm × 210 cm × 35 cm, 735 L) with a 5-cm-deep layer of sand. After a 2-min acclimation, neonates were either left undisturbed for 20 min in the “Undisturbed test” or chased with a dip net for 30 s, to simulate a predatory attack eliciting an escape response, and then left undisturbed for 20 min in the “Chased test.” Tests were video-recorded, and at the conclusion, neonates were transferred back to their housing tank.
From the video for each neonate, two dichotomous categorical variables (Burying success and Moving success) and three continuous variables (Burying time, Latency time, and Total moving time) were quantified for each of the Undisturbed test and Chased test. Following the acclimation period in the Undisturbed test and after the chasing procedure in the Chased test, Burying success, and Moving success related to neonate success in submerging in the sand and moving from its initial position, respectively. The neonate’s Burying time was the elapsed time before the neonates started the burying process, the Latency time was the elapsed time before the first movement, and Total moving time was the time spent moving during the first 5 min following acclimation or the chasing procedure. When neonates did not bury or move before the end of the test, the maximum time (20 min) was recorded as Burying time or Latency time. In preliminary experiments, these variables did not differ between the first and second times the neonates were tested. Nevertheless, to minimize potential systematic error and habituation, whether a neonate was tested first in the Chased or Undisturbed test was randomly allocated.
Granulocyte-to-Lymphocyte Ratio
Smears were prepared of neonate blood samples collected on day 0, air-dried in a closed container, and then fixed by immersion in 100% methanol for 10 min. Blood smears were stained with May–Grünwald (15 min; solution diluted 1:1 with water; Australian Biostain, Traralgon, Victoria, Australia) and Giemsa (15 min; solution diluted 1:9 with water; Australian Biostain) stains, rinsed three times in distilled water, and then immersed in fresh distilled water for 5 min. Slides were examined, with hidden identity, using a compound microscope at ×400 magnification. Granulocytes (neutrophils, heterophils, eosinophils, and basophils) and lymphocytes were identified according to available descriptions (Van Rijn and Reina, 2010; Haines and Arnold, 2015; Guida et al., 2017). Leukocytes were counted in at least four fields of view moving diagonally along the slide and avoiding the edges of the blood smear. Counting ceased when a minimum of 350 leukocytes were counted and all the cells in the last field of view were identified (Van Rijn and Reina, 2010; Johnstone et al., 2012). A random subsample of eight slides was counted twice to test for the repeatability of the leukocyte counting profile (see section “Data Analysis”).
Data Analysis
Yolk sac measurements obtained from the ultrasound scanning were used to calculate the volume of the bean-shaped yolk sac (Figure 2) through the following equation:
where VOL is the estimated yolk sac volume in ml, WY is the width of the yolk sac measured at its widest part, LY is the length, and HY is the height, all expressed in mm; and 10–3 is the factor for converting volume expressed in mm3 to ml. In four stillborn neonates, yolk sac measurements obtained indirectly from the ultrasound video (Figures 2C,D) did not significantly differ from direct measurements of excised yolk sacs (data not shown; Figure 2B), supporting the validity of indirectly measuring yolk sac volume from ultrasound scanning.
The normality of the datasets was assessed with the Shapiro–Wilk test and the homoscedasticity with Levene’s test. t-tests were used to investigate differences in adult female total length and BM (TLF and BMF) at capture between pregnant females of the Trawl and Control treatment groups (Treatment).
Differences in the occurrence of stillborn neonates between treatments were analyzed in terms of both number of stillborn neonates and number of parturitions with stillborn neonates, using Fisher’s test. LMEs were used to examine differences in neonate total length (TLN), BM (BMN), and G:L ratio at birth, including Treatment as a fixed effect and Mother ID as a random effect. To test for G:L counting repeatability, the two readings obtained from the eight re-counted slides were compared, after log transformation, using a paired t-test. To test for the presence of a learning process in the ability to identify different cells, a generalized LME (GLME) model was used to compare the G:L readings of the first eight versus the last eight slides examined including Mother ID as a random effect. With the use of only data from Control neonates, the relationship between yolk sac volume (VOL) at birth and BMN was investigated using an LME model including Mother ID as a random effect. To analyze difference in VOL at birth between Control and Trawl neonates, an LME model was used, including Treatment and the interaction between Treatment and BMN as fixed effects and Mother ID as a random effect [VOL ∼ Treatment + Treatment:BMN + (1| MotherID)]. Data and generated residuals were visually assessed for normality and homoscedasticity. Significance of the fixed terms was evaluated using a Wald test.
To investigate TLN and BMN increments over time (as continuous variables; days 0, 10, 20, and 30), GLME models were used, including the interaction between Treatment and Time as a fixed effect and Neonate ID as a random effect. Yolk consumption rate was investigated with a similar GLME model, but to account for potentially faster consumption rates in larger neonates, the proportion of yolk volume remaining relative to the initial value (VOLL) was used in the analysis. VOLL was calculated using the following equation:
where VOLL is the proportional volume left at time t (days 0, 10, 20, or 30), VOLt is the volume at time t in ml, and VOLi is the initial volume at d0 in ml. Before running the model, VOLL data were transformed as the arcsin of the square root, but for clarity, results are reported as percentages.
Completion speed (m s–1) was calculated dividing the length of the corridor (2 m) by the total elapsed time taken to swim this distance, including the duration of any potential stop (s). For each neonate, Swimming distance (m) and Completion speed were averaged between the three different trials. The relationship between TLN and Swimming distance and Completion speed was investigated using only data from Control neonates and two LME models including Mother ID as a random effect. To investigate the effect of Treatment on these two swimming variables, GLME models were used, including Treatment as a fixed effect and Mother ID as a random effect.
Differences in Burying success and Movement success were determined using Fisher’s tests. To investigate differences in Burying time, Latency time, and Total moving time, GLME models were used. Differences in these variables were investigated both between Treatment, within each test type (Undisturbed or Chased test), including Treatment as a fixed effect and Mother ID as a random effect, and between test type, separately for Control and Trawl Treatments, including test type as a fixed effect and Neonate ID as a random effect.
Data presented are derived from the most parsimonious models, obtained from the full models through stepwise backward elimination of non-significant terms. Results are reported as mean ± standard error of the mean (SE). All tests used a significance level of α ≤ 0.05. Data were processed using R statistical software (R Core Team, 2020). The datasets analyzed for this study can be found at DOI: 10.26180/5f81a746928ad (Finotto et al., 2020).
Results
There was no significant difference between treatments in maternal TLF (Control: 96.9 ± 1.9 cm; Trawl: 93.4 ± 3.1 cm; t1,11 = 0.83, p = 0.43) and BMF (Control: 6.3 ± 0.3 kg; Trawl: 5.8 ± 0.4 kg; t1,11 = 0.85, p = 0.41). Neonates were born an average of 21 days after trawling capture simulation for Trawl females (1–34 days). Females in the control and trawl groups gave birth to an average of 2.8 and 2.0 pups, respectively. One Control female never gave birth, and ultrasound indicated that the embryos were likely non-viable because no mouth movements associated with gill oxygenation was observed. One Trawl female gave birth to two neonates the night after trawling simulation but was not considered a premature, stress-induced parturition because pregnancy was in an advanced state and occurred within the normal parturition period (Marshall et al., 2007). Excluding this female, parturition occurred an average of 24 days after trawling simulation (6–34 days).
The number of stillborn neonates (Control: 1 of 17 neonates; Trawl: 3 of 14 neonates; Fisher’s test p = 0.30) or of parturitions with stillborn neonates (Control: 1 of 6 parturition events; Trawl: 2 of 7 parturition events; Fisher’s test p = 1) did not differ between treatments.
Morphological Traits
Neonate TLN at birth did not significantly differ between treatments (Control: 27.3 ± 0.2 cm; Trawl: 26.4 ± 0.5 cm; F1,25 = 0.004, p = 0.95), but Control neonates were significantly heavier (BMN; Control: 118.9 ± 3.3 g; Trawl: 102.8 ± 5.0 g; F1,25 = 3.99, p = 0.04). In Control neonates, yolk sac volume at birth was significantly and positively correlated with neonate BMN (VOL = −8.72 + 0.12 × BMN; F1,14 = 18.89, p < 0.0001). Both Treatment (F1,25 = 5.48, p = 0.02) and the interaction between Treatment and BMN (F2,24 = 32.14, p < 0.0001) significantly influenced yolk sac volume at birth, with Trawl neonates having a smaller yolk sac (Control: 5.41 ± 0.46 ml; Trawl: 4.39 ± 0.43 ml) and manifesting a lower increase in yolk sac volume with BMN (Table 1 and Figure 3).
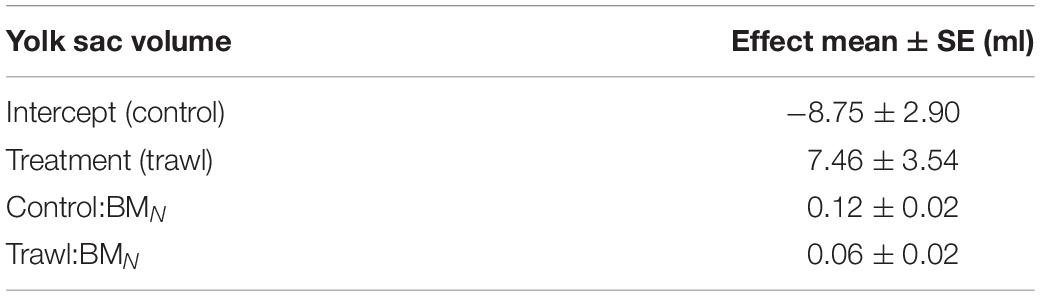
Table 1. Effects obtained from the most parsimonious linear mixed effects model illustrating the influence of neonate body mass (BMN) and maternal treatment group [Control (n = 16) or Trawl (n = 11)] on yolk sac volume at birth.
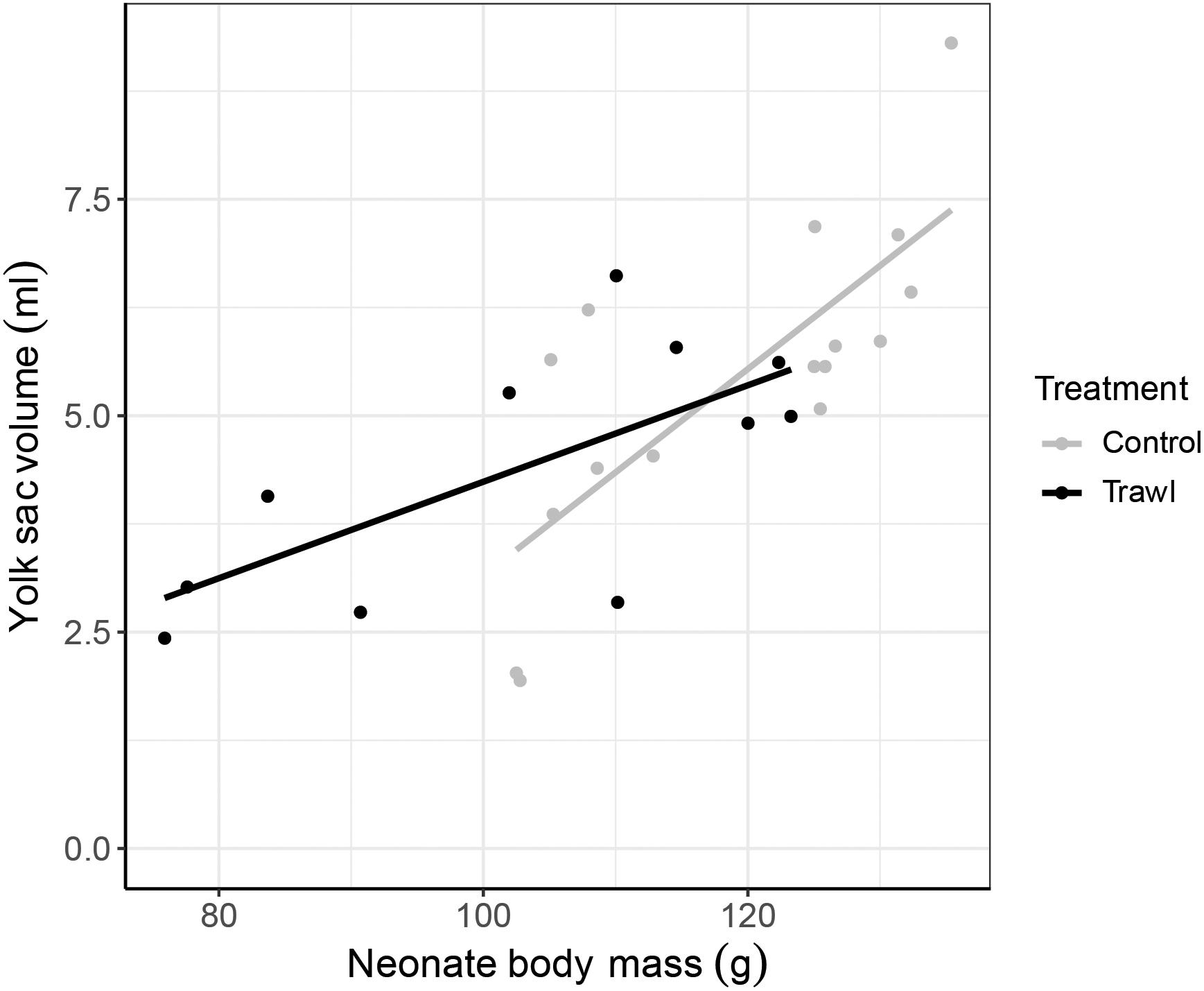
Figure 3. Relationship between neonate yolk sac volume (ml) and neonate body mass (g) at birth (day 0). Data are plotted separately for each maternal Treatment: Control (gray; n = 16) and Trawl (black; n = 11). Points represent original data, and lines represent the linear regressions fitted to the data.
Granulocyte-to-Lymphocyte Ratio
Trawl neonates showed significantly higher G:L ratio values (Control: 0.05 ± 0.01; Trawl: 0.22 ± 0.09; F1,25 = 2.57, p = 0.02). No difference between repeated counts (First: 0.10 ± 0.03; Second: 0.14 ± 0.07; Z1,14 = 0.77, p = 0.74) and no learning process (Early: 0.16 ± 0.05; Late: 0.14 ± 0.07; F1,14 = 1.22, p = 0.27) were observed.
Neonate Growth and Yolk Sac Consumption Rates
Time (F1,102 = 8.24, p < 0.0001) and the interaction between treatment and time (F1,102 = 2.33, p = 0.02) significantly influenced neonate TLN. TLN increased with time, and length increments with time were smaller in neonates from Trawl mothers, indicative of slower growth (TL at day 30: Control: 28.0 ± 0.2 cm; Trawl: 26.9 ± 0.5 cm; Table 2 and Figure 4A). Neonate BMN was not influenced by Time (F1,102 = 1.32, p = 0.19), Treatment (F1,102 = 2.02, p = 0.06), or their interaction (F1,102 = 0.83, p = 0.41; BM at day 30: Control: 119.1 ± 3.2 g; Trawl: 107.8 ± 6.1 g; Table 2 and Figure 4B). Only Time significantly influenced the proportion of yolk sac volume remaining (F1,102 = 16.36, p < 0.0001), which decreased over time at a similar rate for both Trawl and Control neonates (F1,102 = 1.03, p = 0.31; yolk sac volume remaining at day 30: Control: 2.47 ± 0.22 ml; Trawl: 2.36 ± 0.35 ml, corresponding to the 47 ± 3 and 55 ± 5% of the initial volume, respectively; Table 2 and Figure 5).
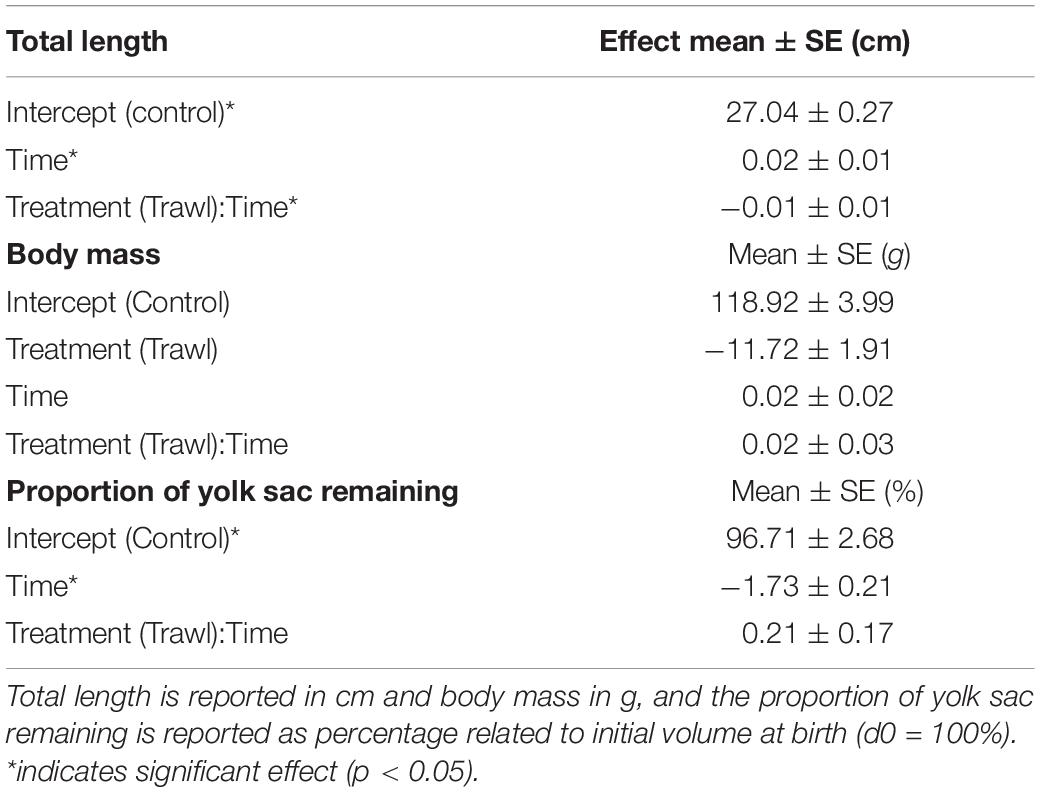
Table 2. Effects obtained from the most parsimonious linear mixed effects model illustrating the influence of time and maternal treatment group [Control (n = 16) or Trawl (n = 11)] on neonate total length, body mass, and proportion of yolk sac volume remaining.
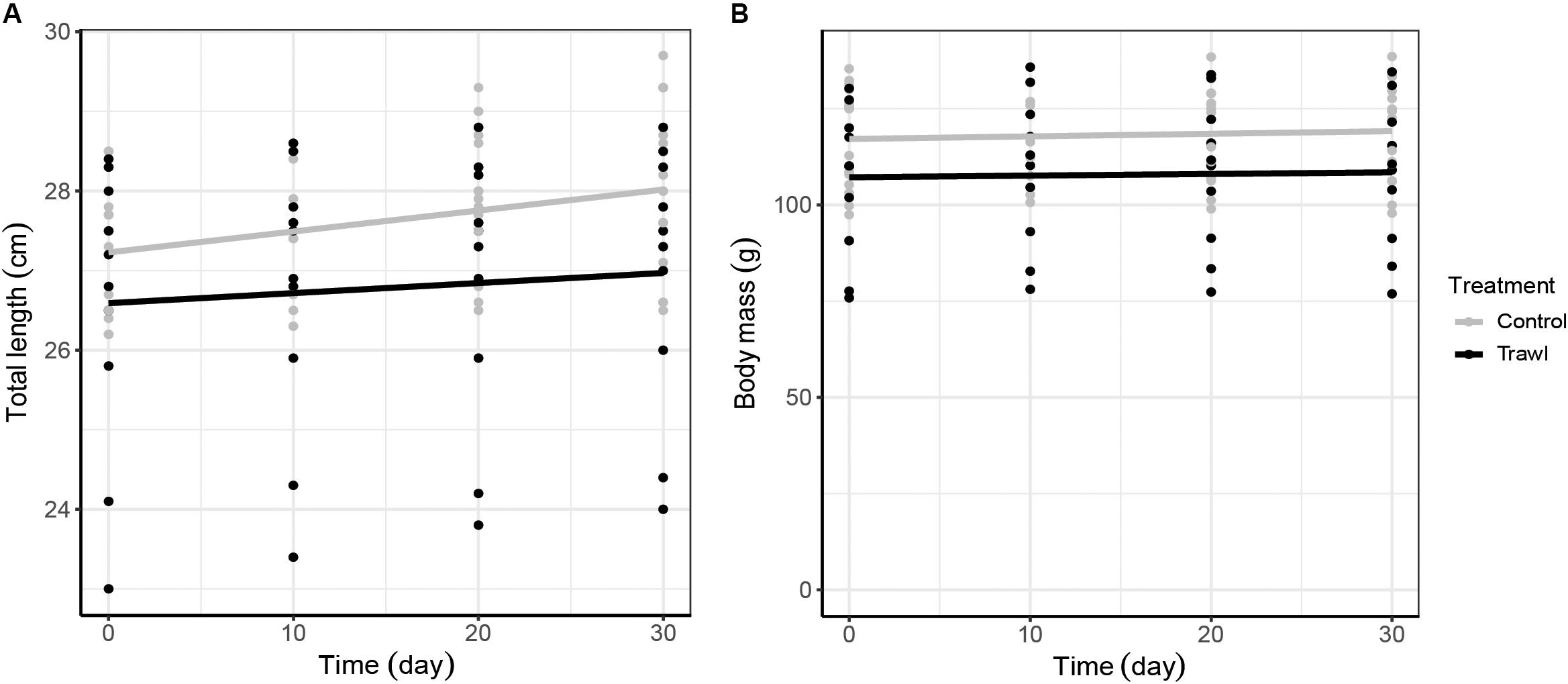
Figure 4. Neonate total length (cm; A) and body mass (g; B) growth increment with time. Total length and body mass data are plotted against time separately for each maternal Treatment: Control (gray; n = 16) and Trawl (black; n = 11). Points represent original data, and the lines were fitted by linear regression. Note that in (A), the y axis starts from 23 cm.
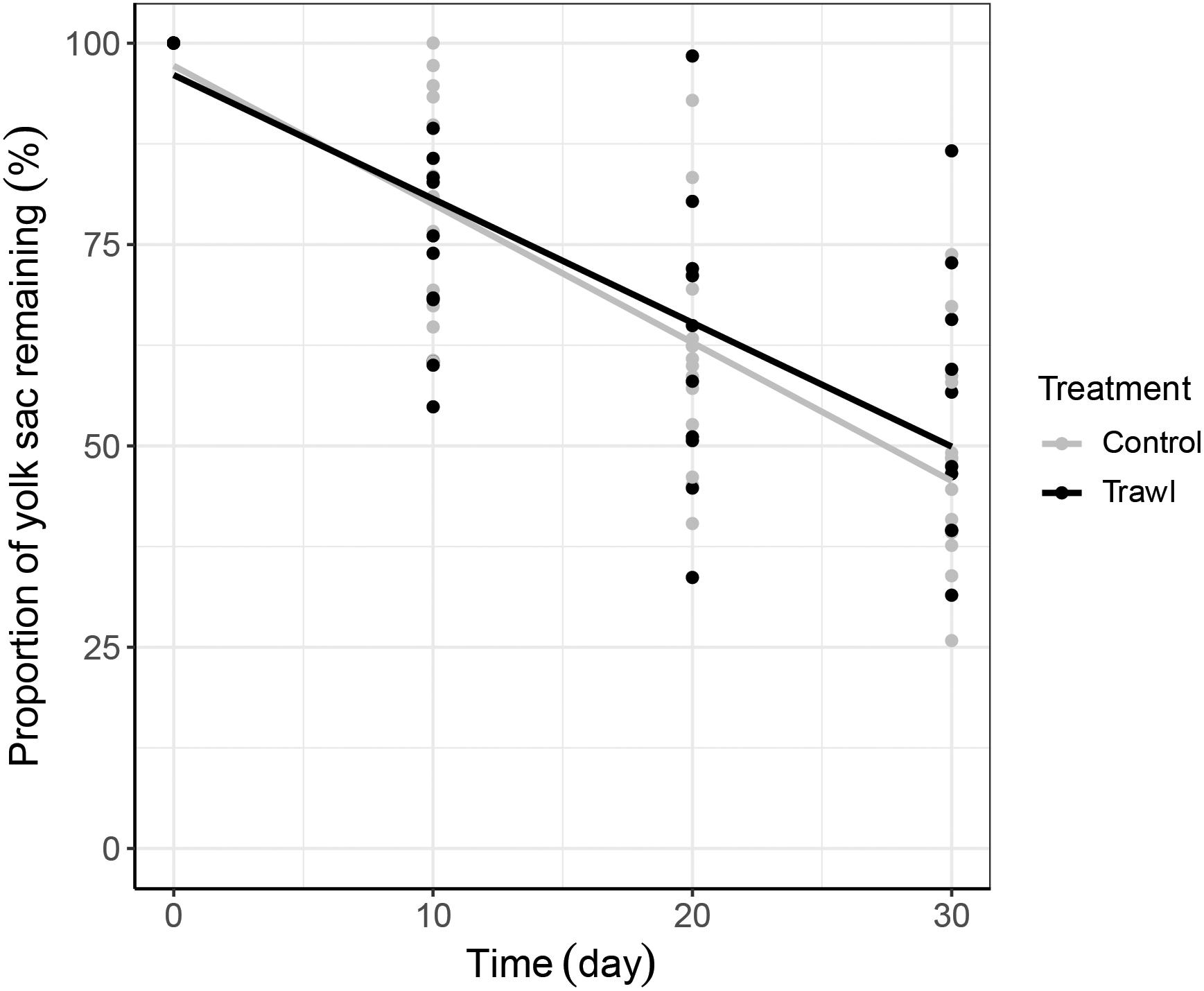
Figure 5. Yolk sac consumption rate. The proportion of neonate yolk sac volume remaining as a percentage (related to initial volume at day 0 = 100%) is plotted against time separately for each maternal Treatment: Control (gray; n = 16) and Trawl (black; n = 11). Points represent original data, and the lines were fitted by linear regression.
Swimming Performance
In Control neonates, Completion speed (Speed = −0.58 + 0.03 × TLN; F1,14 = 5.65, p = 0.02) but not Swimming distance (F1,14 = 0.04, p = 0.84) was significantly and positively correlated with neonate TLN. Treatment significantly influenced Swimming distance (F1,25 = 3.96, p = 0.04), with Control neonates swimming for longer distances (Control: 0.97 ± 0.06 m; Trawl: 0.71 ± 0.09 m), while it did not affect Completion speed (Control: 0.14 ± 0.01 m s–1; Trawl: 0.13 ± 0.01 m s–1; F1,25 = 0.32; p = 0.57).
Burying and Boldness Behavior
Results are reported in Table 3 and Figure 6. No difference emerged in Burying success or Burying time between treatments in the Undisturbed test (Burying success: Fisher’s test p = 0.26; Burying time: F1,24 = 0.02, p = 0.88) or the Chased test (Burying success: Fisher’s test p = 0.69; Burying time: F1,24 = 0.88, p = 0.35).
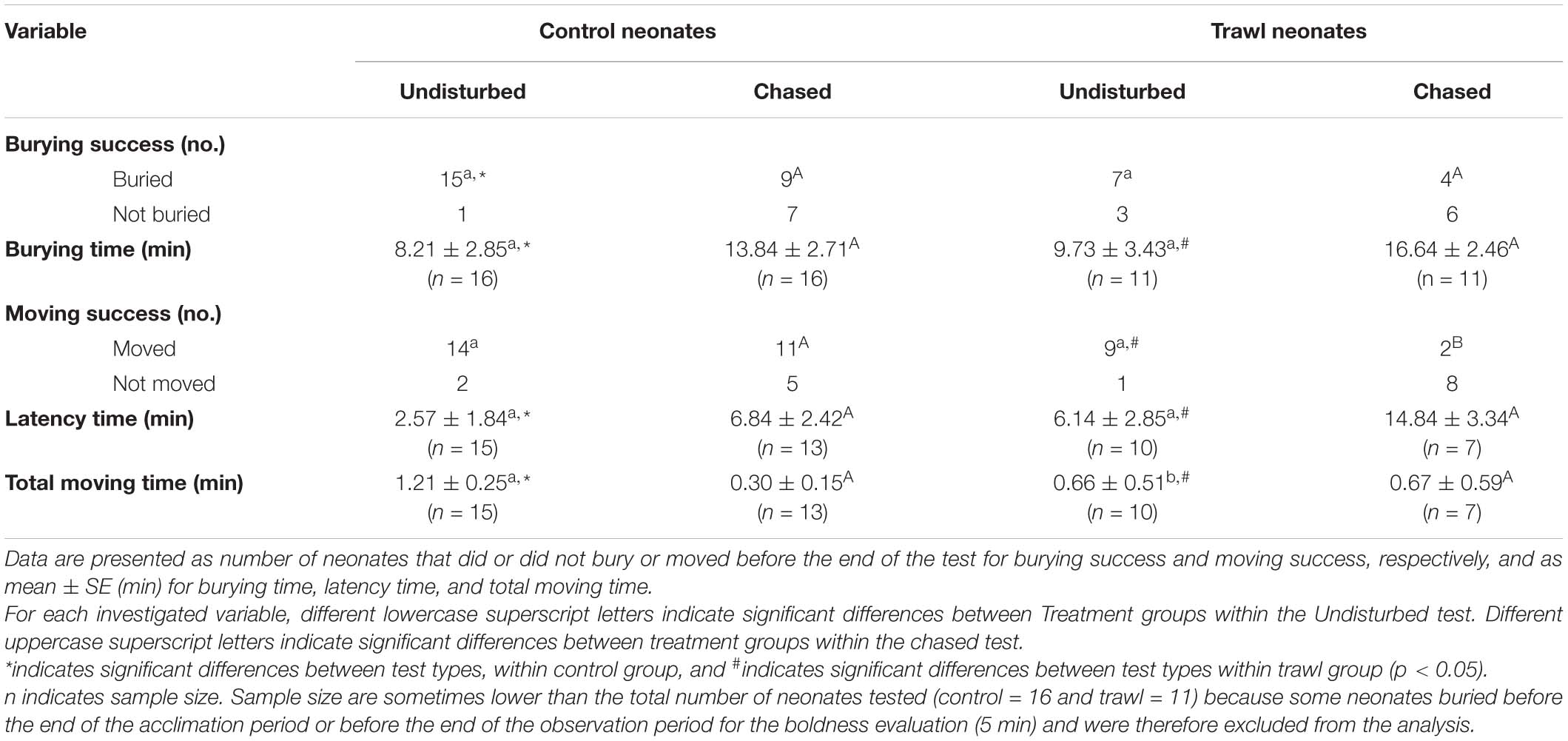
Table 3. Influence of test type (undisturbed or chased) and maternal treatment group (control or trawl) on neonate burying and boldness variables.
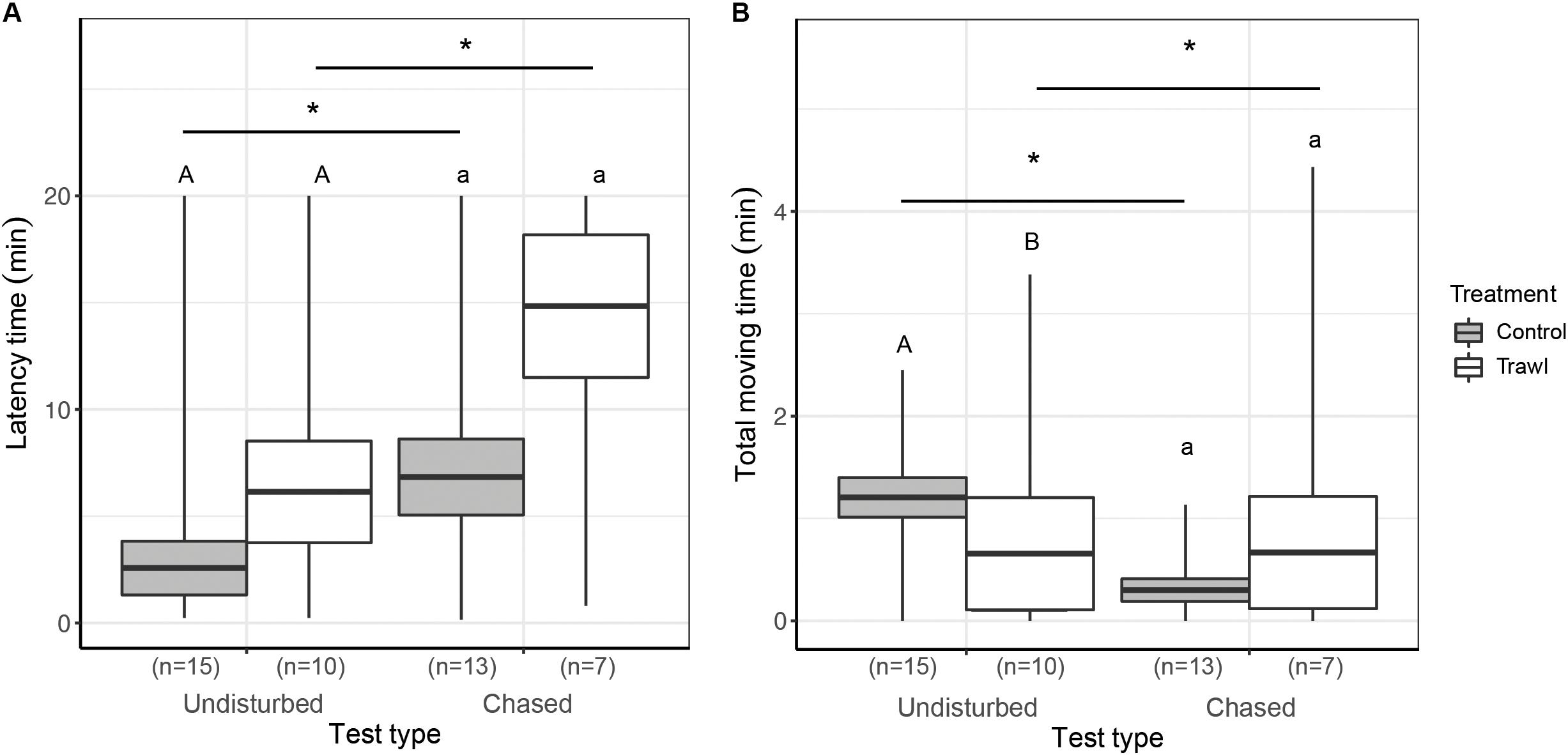
Figure 6. Mean ± SE time elapsed before the first movement (Latency time, min; A) and total time spent moving around the experimental tank (Total moving time, min) during the first 5 min of the test (B). Data are reported separately for each maternal Treatment: Control (gray boxes) and Trawl (white boxes) during the Undisturbed and Chased tests (test type). Different uppercase and lowercase letters indicate significant differences between Treatments, in the Undisturbed and Chased tests, respectively. * indicates significant differences between different test types within Stress or Control neonates. Sample sizes are reported in parentheses. Sample size is sometimes smaller than the total number of neonates tested (Control = 16 and Trawl = 11) because some neonates buried before the end of the observation period for the boldness evaluation (5 min) and were therefore excluded from the analysis.
Comparing Burying success between the two types of test (Chased test or Undisturbed test), no significant difference emerged within Trawl neonates (Fisher’s test p = 0.37), but Control neonates had a significantly higher Burying success in the Undisturbed test (Fisher’s test p = 0.04). Test type was significant for Burying time, which was higher for the Chased test than the Undisturbed test, both in Control (F1,24 = 22.49, p < 0.0001) and Trawl neonates (F1,24 = 17.68, p < 0.0001).
No difference was detected in Movement success between treatments in the Undisturbed test (Fisher’s test p = 1), while Control neonates had a significantly higher Movement success in the Chased test (Fisher’s test p = 0.04). No difference in Latency time was found between treatments in the Undisturbed test (F1,23 = 0.98, p = 0.32) or the Chased test (F1,18 = 1.19, p = 0.27). In the Undisturbed test, Control neonates had a significantly higher Total moving time than Trawl neonates (F1,16 = 4.09, p = 0.04), while no difference emerged in the Chased test (F1,19 = 0.69, p = 0.41).
Comparing Movement success between the two types of test, no difference was found within Control neonates (Fisher’s test p = 0.39), but within Trawl neonates, success was significantly lower in the Chased test (Fisher’s test p = 0.01). Within both Control (F1,26 = 22.77, p < 0.0001) and Trawl neonates (F1,15 = 16.44, p < 0.0001), neonates showed a lower Latency time in the Undisturbed test. Test type significantly affected also Total moving time in both Control (F1,23 = 293.65, p < 0.0001) and Trawl neonates (F1,12 = 11.95, p < 0.001). Control neonates moved for a longer time when tested in the Undisturbed test, while the opposite was observed for Trawl neonates. This contrasting result seems to be driven by a single Trawl neonate that in the Chased test largely increased its Total moving time, while four neonates reduced it.
Given the statistically significant results for Burying time and Latency time might have been partially driven by the high number of animals that did not bury or move and the inclusion of these maximal times (20 min), we repeated the analyses excluding these animals. The results did not change except for the comparison of Latency time between the Undisturbed and Chased Trawl neonates, which became non-significant. Because the sample size was as small as two neonates, there would be advantages in repeating the experiments with larger sample sizes.
Discussion
Given that successful reproduction is essential in maintaining sustainable populations, studying the reproductive consequences of stress is important, especially for chondrichthyans given their poor conservation status (Dulvy et al., 2014) and low reproductive rates (Camhi et al., 1998). In this study, to contribute to addressing the need for improved understanding of the intergenerational effects of capture stress in chondrichthyans, the consequences of maternal exposure to fishing-capture stress persisting in neonates up to 1 month after birth were evaluated.
At birth, neonates born from trawled mothers (stressed neonates) had lower BM than control neonates. This was previously reported by Guida et al. (2017). The same study, in contrast to the results of this study, also observed a smaller total length in stressed neonates, the difference likely resulting from differences in stress timing. While in Guida et al. (2017) trawling was simulated 40–86 days before parturition, in this study, it occurred as little as 1 day before. Stressors applied so close to parturition, once embryonic development had almost concluded, would be unlikely to affect total length but could have impacted BM, a more plastic trait.
In this study and that of Guida et al. (2017), the neonates born from stressed mothers had higher G:L ratios than control neonates, suggesting a state of stress (Van Rijn and Reina, 2010) persisting in the offspring after trawling simulation and lasting at least till birth, potentially due to neonate exposure to maternal stress hormones (Skomal and Mandelman, 2012). The increase in G:L ratio is an important response to stress that minimizes the chances of infection by mobilizing different leukocyte populations toward areas of the body with a higher probability for pathogen entrance (Van Rijn and Reina, 2010; Heard et al., 2014). However, a continuous alteration of the immune strategy might induce an immunosuppressed state impairing disease resistance and causing a generally lowered health status (Wendelaar-Bonga, 1997). Additionally, immune responses are energetically expensive (French et al., 2007), and a prolonged reaction might impact the energy reserves, reducing the amount the neonates can allocate to other biological activities.
At birth, compared with control neonates, stressed neonates had smaller yolk sacs, and the difference became more pronounced as BM increased. Alterations in energy allocation to oocytes during vitellogenesis are suggested as the cause of the reduction in offspring energetic reserves (yolk sac and oil globules) observed following parental exposure to stress in Atlantic salmon (Salmo salar; Eriksen et al., 2006) and damselfish (Pomacentrus amboinensis; Gagliano and McCormick, 2009). In this study, stress could not have impacted vitellogenesis because capture was simulated after its conclusion. The increased energy allocation toward the immunological response (French et al., 2007) hypothesized for stressed neonates is a more likely explanation for the reduction in yolk sac volume. The smaller residual yolk sac reserves might impact stressed neonate survival given that these reserves sustain offspring in the first period after birth and, in teleost fishes, a decreased quantity of yolk slows growth rate (Rothschild, 1986) and reduces the length of the starvation period that offspring can endure (Miller et al., 1988; Leggett and Deblois, 1994).
Compared with control neonates, stressed neonates manifested a smaller increase in total length over time. In contrast, in teleost fishes, stressed neonates manifest faster growth (Li et al., 2010; Eriksen et al., 2013; Nesan and Vijayan, 2016), but the opposite response observed in this study, given that this is the only report for chondrichthyans, cannot yet be ascribed to taxonomic differences. Stressed and control neonates of T. dumerilii showed a similar rate of yolk sac consumption. Despite stressed neonates using the same amount of resources, this is fueling a smaller increase in length, suggesting that energy is allocated to different activities, such as prolonged stress or immunological responses (French et al., 2007). Such alteration in energy allocation, occurring to the detriment of growth, might present severe drawbacks because faster growth promotes a more rapid passage through critical life stages when small body size is associated with slower swimming performance, thus impairing prey capture and predator escape abilities (Bergenius et al., 2002; Wilson and Meekan, 2002). BM did not change with time in control or stressed neonates, possibly because neonates did not consume much food (LF personal observation) and growth was mainly supported by the energy acquired from the yolk sac, further confirming the importance of these reserves for neonate sustenance.
Given that two stressed neonates were born only 1 day after maternal exposure to fishing-capture stress, we recognize that there might not have been enough time for the stress consequences to impact some neonate morphological traits, masking potential differences. In contrast, more plastic traits, such as behavioral, physiological, and immunological responses (Mandelman and Skomal, 2009; Frick et al., 2010; Harris et al., 2010; Van Rijn and Reina, 2010), would have likely been impacted despite the short time elapsed from the stressful event. If we exclude these neonates from the analysis, yolk reserve consumption rate was slower in stressed neonates as also observed in offspring of stressed S. salar, likely a response originating from the need to conserve the maternally derived reserves (Eriksen et al., 2006). Nevertheless, we decided to include these neonates in all analyses and to accept the results as such to avoid drawing untested assumptions and to maintain a more balanced sample size.
In teleosts, maternal stress alters offspring swimming variables (Sopinka et al., 2014, 2016b). Similarly, in this study, stressed T. dumerilii neonates swam for shorter distances after a simulated predator attack. This species relies on camouflage and burying to escape predators (LF personal observation), and animals’ inability to distance themselves enough from predators, thus remaining in their field of view, might increase predation risk. Completion speed is a measure of the combination of swimming speed and neonate responsiveness to predator simulation (i.e., willingness/reluctance to move), which relates to predator escape abilities (Gyssels and Stoks, 2005). Neonate completion speed was not affected by the trawling stress experienced by the mothers, but it was positively correlated with neonate total length. Stressed neonates have a slower growth rate, and a difference in completion speed might appear later in life when differences in total length emerge and should be further investigated. Indeed, impaired swimming performance, and specifically reduced completion speed with the associated delayed response to predator and reduced swimming speed, might impact prey capture and predator escape abilities (Sopinka et al., 2016b).
No difference emerged between control and stressed neonates in the success with which they buried themselves or in the time taken before burying, indicating that burying ability is not impacted by maternal stress or is not a reliable indicator of a stressed state. After a simulated predatory attack (Chased test), both groups of neonates delayed burying and control neonates manifested also a lower burying success. Burying is an essential anti-predatory tactic (Hobson, 1965; Stein and Magnuson, 1976; Couffer and Benseman, 2015), but if a predator observes the burying event, the benefit of hiding is lost. It seems that neonates perceived the chasing procedure as a predatory attack and postponed or avoided burying, perhaps waiting for the predator to leave the area. Indeed, it is known that fish can modify their behavior in response to the presence of predators so as to reduce the chances of being preyed upon (Harris et al., 2010), and stressed T. dumerilii neonates, not reducing their burying success, might experience a higher predation risk.
Spending a higher amount of time moving around the tank (Total moving time in the Undisturbed test) and with a higher proportion of animals that started movements (Movement success in the Chased test), control neonates manifested behavior consistent with higher boldness. In contrast, teleost offspring born from stressed parents manifested an increased boldness (Sopinka et al., 2015; Best et al., 2017). Higher boldness allows exploring larger areas and increasing chances of finding food and resources, suggesting a competitive advantage for control T. dumerilii neonates. Nevertheless, the higher activity associated with higher boldness might also increase the chances of being located by predators (Martel and Dill, 1993; Fuiman and Magurran, 1994; Archard and Braithwaite, 2003), and, for control neonates, the advantages of easier access to resources might be offset by higher predation risk. However, both stressed and control neonates, when chased, potentially recognizing the presence of a predator, adopted a more cautious behavior, reducing boldness by increasing Latency time and reducing Movement success and Total moving time. Moreover, the behavioral difference observed between undisturbed neonate groups disappeared in the Chased test, eliminating any potential higher vulnerability of control neonates to predation risk. Despite control neonates not decreasing movement success when chased, they delayed the first movement, likely waiting to ascertain whether the predator left the area. Similarly, in perch (Perca fluviatilis), animals experiencing higher predation occurrence show decreased boldness indicated by an increased time spent in a refuge, an increased latency to start feeding, and a reduced duration of the feeding event (Magnhagen and Borcherding, 2008).
Methodologies similar to the one used in this experiment have been previously adopted in other studies to simulate trawling (Frick et al., 2010; Guida et al., 2017; Martins et al., 2018). The fishing-capture treatment towing the animals individually for 7 h is slightly longer than typical commercial durations of fisheries in the general area of our study, which range from 4 to 6 h (Frick et al., 2010). However, the treatment excludes a variety of other highly variable stressors such as compression, injury, and associated hypoxia from the presence of other animals and debris in the cod-end, and environmental changes in temperature and pressure, which relate to towing speed and environmental and weather conditions dependent on locality, depth, and time of year. Similarly, the conditions encountered after the end of the capture simulation are mild, with an absence of predators and occurring in an optimal environment with abundant food. All this considered, and despite longer tow durations, our capture simulation was unlikely to have overstressed the animals; rather, we think it represented a best-case scenario compared with commercial procedures. Moreover, despite being a mild-to-moderate trawl treatment, this capture simulation measurably affected neonate traits, indicating the importance of these first data and the need to investigate the effects on offspring fitness from some of the more extreme conditions that arise during normal trawl operations.
Trawling-capture stress experienced by near-term pregnant T. dumerilii appeared not to induce premature parturition or abortion, as found by a previous study (Guida et al., 2017), or increase the occurrence of stillborn neonates, even though larger sample sizes are required to confirm this. Nevertheless, despite the capture simulation occurring shortly before parturition, when embryonic development was almost concluded, capture stress still impacted neonate traits, potentially affecting their competitiveness and survival. T. dumerilii is a lecithotrophic viviparous species (Marshall et al., 2007); therefore, in matrotrophic species, where mothers supply additional nutrients as histotroph to the embryos (Hamlett et al., 2005), reproductive consequences will likely be more severe due to stress-caused energy constraints. Indeed, pregnant T. dumerilii exposed to capture seem to reduce the energy allocation to pregnancy, and together with potentially affected oxygen delivery to the embryos and impacted waste removal (Finotto et al., unpublished), the embryos of stressed matrotrophic species experience the additional burden of reduced supplies of nutrient. Moreover, being a resilient species (Martins, 2017), consequences in T. dumerilii are probably milder than those that might be observed in species with higher sensitivity to fishing-capture stress (Dapp et al., 2015).
Although more investigation is needed to confirm whether the alterations observed in this study affect neonate survival in the natural environment, the potentially severe outcomes hypothesized a call for a precautionary approach to fishing effort management. In teleosts, a reduction in the severity of the fishing-capture stress can lessen some of the observed reproductive consequences (Pettit, 1977; Booth et al., 1995; Lowerre-Barbieri et al., 2003; Hall et al., 2009, 2017). Therefore, reductions in fishing time, air exposure periods and handling, and the adoption of best handling and releasing practices (AFMA, 2014) are measures that could be easily implemented to reduce stress load and the likely severity of its reproductive consequences. Actions to reduce the likelihood of encountering and/or capturing pregnant females at certain times and using gear or handling practices to minimize stress in areas where they are in high abundance would also be beneficial. Ultimately, to develop effective management measures, additional to quantitative measures of the direct effects of fishing-capture stress on the survival of released animals, the effects of stress on the reproductive capacity of populations also need to be accounted in population dynamics and risk assessment models used for assessing fishery sustainability (Punt and Walker, 1998; Pribac et al., 2005; Walker, 2005a, b). Given the relatively good conservation status of T. dumerilii (IUCN, 2020), the observed neonatal impairments might seem of minor concern from a management perspective. However, taxonomically close species, such as the shortnose guitarfish (Zapteryx brevirostris) and the spotted shovelnose ray (Aptychotrema timorensis), have poor conservation status (IUCN, 2020); and the observations recorded in this study might be helpful for their management, especially given the paucity of general and species-specific data.
Unlike fishing-induced parturition, the neonatal consequences observed in this study will probably go unnoticed in the absence of specific research, highlighting the need to investigate reproductive consequences of fishing-capture stress in more species, characterized by varying sensitivity to stress and different reproductive modes. Alterations in offspring foraging skills, stress response, metabolic rate, cardiac performance, and reproductive output after fishing-capture stress experienced by the parents should also be researched. Despite the merit of having highlighted impairments to neonate yolk reserves, growth, swimming, and behavior, to understand the full extent of the consequences of fishing-capture stress, the impact that alterations observed in captive conditions has on survival, recruitment, and fitness of neonates in the natural environment must be evaluated.
Data Availability Statement
The datasets presented in this study can be found in online repositories. The names of the repository/repositories and accession number(s) can be found below: https://doi.org/10.26180/5f81a746928ad.
Ethics Statement
The animal study was reviewed and approved by Monash University, School of Biological Sciences Animal Ethics Committee (AEC), Chairperson: Assoc. Prof. Anne Peters. AEC number: BSCI/2016/37.
Author Contributions
LF: conceptualization, methodology, data collection, data analysis, investigation, and writing—original draft. TW and RR: writing—review & editing and supervision. LF, TW, and RR funding acquisition. All authors contributed to the article and approved the submitted version.
Funding
This work was funded by The Holsworth Wildlife Research Endowment and The Ecological Society of Australia, an Australian Government Research Training Program (RTP) Scholarship to LF, and Australian Research Council Linkage grant LP 110200572 to RR and TW.
Conflict of Interest
The authors declare that the research was conducted in the absence of any commercial or financial relationships that could be construed as a potential conflict of interest.
Acknowledgments
We thank the Victorian Fisheries Authority, Prof. John Donald, and Deakin University for the use of research infrastructure. We thank the three reviewers for their constructive comments that helped improve the quality of the manuscript.
References
Adams, K. R., Fetterplace, L. C., Davis, A. R., Taylor, M. D., and Knott, N. A. (2018). Sharks, rays and abortion: the prevalence of capture-induced parturition in elasmobranchs. Biol. Conserv. 217, 11–27. doi: 10.1016/j.biocon.2017.10.010
AFMA, (2014). Shark and Ray Handling Practices - A Guide for Commercial Fishers in Southern Australia. Canberra: Australian Fisheries Management Authority.
Archard, G. A., and Braithwaite, V. A. (2003). Variation in aggressive behaviour in the poeciliid fish Brachyrhaphis episcopi: population and sex differences. Behav. Process. 86, 52–57. doi: 10.1016/j.beproc.2010.09.002
Bergenius, M. A. J., Meekan, M. G., Robertson, D. R., and McCormick, M. I. (2002). Larval growth predicts the recruitment success of a coral reef fish. Oecologia 131, 521–525. doi: 10.1007/s00442-002-0918-4
Best, C., Kurrasch, D. M., and Vijayan, M. M. (2017). Maternal cortisol stimulates neurogenesis and affects larval behaviour in zebrafish. Sci. Rep. 7:40905. doi: 10.1038/srep40905
Booth, R. K., Kieffer, J. D., Davidson, K., Bielak, A. T., and Tufts, B. L. (1995). Effects of late-season catch and release angling on anaerobic metabolism, acid-base status, survival, and gamete viability in wild Atlantic salmon (Salmo salar). Can. J. Fish. Aquat. Sci. 52, 283–290. doi: 10.1139/f95-029
Bouyoucos, I. A., Weideli, O. C., Planes, S., Simpfendorfer, C. A., and Rummer, J. L. (2018). Dead tired: evaluating the physiological status and survival of neonatal reef sharks under stress. Conserv. Physiol. 6:coy053. doi: 10.1093/conphys/coy053
Braccini, J. M., Van Rijn, J., and Frick, L. (2012). High post-capture survival for sharks, rays and chimaeras discarded in the main shark fishery of Australia? PLoS One 7:e32547. doi: 10.1371/journal.pone.0032547
Camhi, M. D., Fowler, S. L., Musick, J. A., Bräutigam, A., and Fordham, S. V. (1998). Sharks and their relatives - ecology and conservation. Paper Presented at the Occasional Paper of the IUCN Species Survival Commission, (Gland: IUCN/SSC Shark Specialist Group).
Campbell, M. J., Mclennan, M. F., Courtney, A. J., and Simpfendorfer, C. A. (2018). Post-release survival of two elasmobranchs, the eastern shovelnose ray (Aptychotrema rostrata) and the common stingaree (Trygonoptera testacea), discarded from a prawn trawl fishery in southern Queensland, Australia. Mar. Freshw. Res. 69, 551–561. doi: 10.1071/MF17161
Charvet-Almeida, P., Góes De Araújo, M. L., and De Almeida, M. P. (2005). Reproductive aspects of freshwater stingrays (Chondrichthyes: Patamotrygonidae) in the Brazilian Amazon basin. J. Northwest Atl. Fish. Sci. 35, 165–171. doi: 10.2960/J.v35.m502
Couffer, M. C., and Benseman, S. A. (2015). A Young-of-the-year giant sea bass, stereolepis gigas buries itself in sandy bottom: a possible predator avoidance mechanism. Bull. South. Calif. Acad. Sci. 114, 54–57. doi: 10.3160/0038-3872-114.1.54
Dapp, D. R., Walker, T. I., Huveneers, C., and Reina, R. D. (2015). Respiratory mode and gear type are important determinants of Elasmobranch immediate and post-release mortality. Fish Fish. 17, 507–524. doi: 10.1111/faf.12124
de Sousa Rangel, B., de Castro Ribeiro, D., Chagas, J. M. A., Spada, L., Moreira, R. G., and da Silva Ribeiro, C. (2020). Effects of biological traits on capture-induced parturition in a freshwater stingray and perspectives for species management. J. Fish Biol. 97, 546–551. doi: 10.1111/jfb.14412
Dulvy, N. K., Baum, J. K., Clarke, S., Compagno, L. J. V., Cortés, E., Domingo, A., et al. (2008). You can swim but you can’t hide: the global status and conservation of oceanic pelagic sharks and rays. Aquat. Conserv. Mar. Freshwat. Ecosyst. 18, 459–482. doi: 10.1002/aqc.975
Dulvy, N. K., Fowler, S. L., Musick, J. A., Cavanagh, R. D., Kyne, P. M., Harrison, L. R., et al. (2014). Extinction risk and conservation of the world’s sharks and rays. eLife 3:e00590. doi: 10.7554/eLife.00590
Eriksen, M. S., Bakken, M., Espmark, ÅM., Braastad, B. O., and Salte, R. (2006). Prespawning stress in farmed Atlantic salmon Salmo salar: maternal cortisol exposure and hyperthermia during embryonic development affect offspring survival, growth and incidence of malformations. J. Fish Biol. 69, 114–129. doi: 10.1111/j.1095-8649.2006.01071.x
Eriksen, M. S., Poppe, T. T., McCormick, M., Damsgård, B., Salte, R., Braastad, B. O., et al. (2013). Simulated maternal pre-spawning stress affects offspring’s attributes in farmed Atlantic salmon Salmo salar (Linnaeus, 1758). Aquacult. Res. 46, 1480–1489. doi: 10.1111/are.12301
Ferretti, F., Worm, B., Britten, G. L., Heithaus, M. R., and Lotze, H. K. (2010). Patterns and ecosystem consequences of shark declines in the ocean. Ecol. Lett. 13, 1055–1071. doi: 10.1111/j.1461-0248.2010.01489.x
Finotto, L., Walker, T. I., and Reina, R. D. (2020). Dataset from: Prolonged Alteration of Neonate Traits Following Maternal Exposure to Fishing-Capture Stress during Late Pregnancy in a Chondrichthyan. London: Monash University Research Repository.
French, S. S., Denardo, D. F., and Moore, M. C. (2007). Trade-offs between the reproductive and immune systems: facultative responses to resources or obligate responses to reproduction? Am. Nat. 170, 79–89.
Frick, L. H., Walker, T. I., and Reina, R. D. (2010). Trawl capture of Port Jackson sharks, Heterodontus portusjacksoni, and gummy sharks, Mustelus antarcticus, in a controlled setting: effects of tow duration, air exposure and crowding. Fish. Res. 106, 344–350. doi: 10.1016/j.fishres.2010.08.016
Fuiman, L. A., and Magurran, A. E. (1994). Development of predator defences in fishes. Rev. Fish Biol. Fish. 4, 145–183.
Gagliano, M., and McCormick, M. I. (2009). Hormonally mediated maternal effects shape offspring survival potential in stressful environments. Oecologia 160, 657–665. doi: 10.1007/s00442-009-1335-8)
Guida, L., Awruch, C., Walker, T. I., and Reina, R. D. (2017). Prenatal stress from trawl capture affects mothers and neonates: a case study using the southern fiddler ray (Trygonorrhina dumerilii). Sci. Rep. 7:46300. doi: 10.1038/srep46300
Gyssels, F. G. M., and Stoks, R. (2005). Threat-sensitive responses to predator attacks in a damselfly. Ethology 111, 411–423. doi: 10.1111/j.1439-0310.2005.01076.x
Haines, A. N., and Arnold, J. E. (2015). “Elasmobranch blood cells,” in Immunobiology of the Shark, eds S. L. Smith, R. B. Sim, and M. F. Flajnik, (Boca Raton, FL: CRC Press), 89–104.
Hall, K. C., Broadhurst, M. K., Butcher, P. A., Cameron, L., Rowland, S. J., and Millar, R. B. (2017). Sublethal effects of angling and release on golden perch Macquaria ambigua: implications for reproduction and fish health. J. Fish Biol. 90, 1980–1998. doi: 10.1111/jfb.13282
Hall, K. C., Broadhurst, M. K., Butcher, P. A., and Rowland, S. J. (2009). Effects of angling on post-release mortality, gonadal development and somatic condition of Australian bass Macquaria novemaculeata. J. Fish Biol. 75, 2737–2755. doi: 10.1111/j.1095-8649.2009.02474.x
Hamlett, W. C., Kormanik, G., Storrie, M., Stevens, B., and Walker, T. I. (2005). “Chondrichthyan parity, lecithotrophy and matrotrophy,” in Reproductive Biology and Phylogeny of Chondrichthyes, ed. W. C. Hamlett, (Enfield, NH: Science Publishers, Inc), 395–434.
Harris, S., Ramnarine, I. W., Smith, H. G., and Pettersson, L. B. (2010). Picking personalities apart: estimating the influence of predation, sex and body size on boldness in the guppy Poecilia reticulata. Oikos 119, 1711–1718. doi: 10.1111/j.1600-0706.2010.18028.x
Heard, M., Van Rijn, J. A., Reina, R. D., and Huveneers, C. (2014). Impacts of crowding, trawl duration and air exposure on the physiology of stingarees (family: Urolophidae). Conserv. Physiol. 2:cou040. doi: 10.1093/conphys/cou040
Hobson, E. S. (1965). Diurnal-nocturnal activity of some inshore fishes in the Gulf of California. Copeia 3, 291–302. doi: 10.2307/1440790
IUCN, (2020). Available at: https://www.iucnredlist.org/ (accessed October, 2020).
Johnstone, C. P., Lill, A., and Reina, R. D. (2017). Use of erythrocyte indicators of health and condition in vertebrate ecophysiology: a review and appraisal. Biol. Rev. 92, 150–168. doi: 10.1111/brv.12219
Johnstone, C. P., Reina, R. D., and Lill, A. (2012). Interpreting indices of physiological stress in free-living vertebrates. J. Comp. Physiol. B 182, 861–879. doi: 10.1007/s00360-012-0656-9
Leatherland, J. F., Li, M., and Barkataki, S. (2010). Stressors, glucocorticoids and ovarian function in teleosts. J. Fish Biol. 76, 86–111. doi: 10.1111/j.1095-8649.2009.02514.x
Leggett, W. C., and Deblois, E. (1994). Recruitment in marine fishes: is it regulated by starvation and predation in the egg and larval stages? Neth. J. Sea Res. 32, 119–134.
Li, M., Bureau, D. P., King, W. A., and Leatherland, J. F. (2010). The actions of in ovo cortisol on egg fertility, embryo development and the expression of growth-related genes in rainbow trout embryos, and the growth performance of juveniles. Mol. Rep. Dev. 77, 922–931. doi: 10.1002/mrd.21239
Li, M., and Leatherland, J. F. (2012). The interaction between maternal stress and the ontogeny of the innate immune system during teleost embryogenesis: implications for aquaculture practice. J. Fish Biol. 81, 1793–1814. doi: 10.1111/j.1095-8649.2012.03447.x
Lowerre-Barbieri, S. K., Vose, F. E., and Whittington, J. A. (2003). Catch-and-release fishing on a spawning aggregation of common snook: does it affect reproductive output? Trans. Am. Fish. Soc. 132, 940–952. doi: 10.1577/T02-001
Magnhagen, C., and Borcherding, J. (2008). Risk-taking behaviour in foraging perch: does predation pressure influence age-specific boldness? Anim. Behav. 75, 509–517. doi: 10.1016/j.anbehav.2007.06.007
Mandelman, J. W., and Skomal, G. B. (2009). Differential sensitivity to capture stress assessed by blood acid-base status in five carcharhinid sharks. J. Comp. Physiol. B 179, 267–277. doi: 10.1007/s00360-008-0306-4
Marshall, L. J., White, W. T., and Potter, I. C. (2007). Reproductive biology and diet of the southern fiddler ray, Trygonorrhina fasciata (Batoidea: Rhinobatidae), an important trawl bycatch species. Mar. Freshw. Res. 58, 104–115. doi: 10.1071/MF05165
Martel, G., and Dill, L. M. (1993). Feeding and aggressive behaviours in juvenile coho salmon (Oncorhynehus kisutch) under chemically-mediated risk of predation. Behav. Ecol. Sociobiol. 32, 365–370. doi: 10.1007/BF00168819
Martins, C. L. (2017). Stress, Survival and Movement Following Fishing Gear Capture in Chondrichthyan Species. Ph. D thesis, Monash University, Melbourne, VIC.
Martins, C. L., Walker, T. I., and Reina, R. D. (2018). Stress-related physiological changes and post-release survival of elephant fish (Callorhinchus milii) after longlining, gillnetting, angling and handling in a controlled setting. Fish. Res. 204, 116–124. doi: 10.1016/j.fishres.2018.01.016
Milla, S., Wang, N., Mandiki, S. N. M., and Kestemont, P. (2009). Corticosteroids: friends or foes of teleost fish reproduction? Comp. Bioch. Physiol. A 153, 242–251. doi: 10.1016/j.cbpa.2009.02.027
Miller, T. J., Crowder, L. B., Rice, J. A., and Marschall, E. A. (1988). Larval size and recruitment mechanisms in fishes: toward a conceptual framework. Can. J. Fish. Aquat. Sci. 45, 1657–1670.
Molina, J. M., and Cooke, S. J. (2012). Trends in shark bycatch research: current status and research needs. Rev. Fish Biol. Fish. 22, 719–737. doi: 10.1007/s11160-012-9269-3
Nesan, D., and Vijayan, M. M. (2016). Maternal cortisol mediates hypothalamus-pituitary-interrenal axis development in zebrafish. Sci. Rep. 4:22582. doi: 10.1038/srep22582
Pankhurst, N. W. (2016). “Reproduction and development,” in Biology of Stress in Fish: Fish Physiology, eds C. B. Schreck, L. Tort, A. P. Farrell, and C. J. Brauner, (Amsterdam: Elsevier Inc), 295–331.
Pettit, S. W. (1977). Comparative reproductive success of caught-and-released and unplayed hatchery female steelhead trout (Salmo gairdneri) from the Clearwater river, Idaho. Trans. Am. Fish. Soc. 106, 431–435. doi: 10.1577/1548-86591977106<431:CRSOCA<2.0.CO;2
Pribac, F., Punt, A. E., Walker, T. I., and Taylor, B. L. (2005). Using length, age and tagging data in a stock assessment of a length selective fishery for gummy shark (Mustelus antarcticus). J. Northwest Atl. Fish. Sci. 35, 267–290.
Punt, A. E., and Walker, T. I. (1998). Stock assessment and risk analysis for the school shark (Galeorhinus galeus) off southern Australia. Mar. Freshw. Res. 49, 719–731. doi: 10.1071/MF96101
Rasband, W. S. (2018). ImageJ, U. S. National Institutes of Health, Maryland, MD: Bethesda. Available online at: https://imagej.nih.gov/ij/
R Core Team (2020). R: A Language and Environment for Statistical Computing. Vienna: R Foundation for Statistical Computing. Available online at: https://www.R-project.org/
Rothschild, B. J. (1986). Dynamics of Marine Fish Populations. Cambridge, MA: Harvard University Press.
Schreck, C. B. (2010). Stress and fish reproduction: the roles of allostasis and hormesis. Gen. Comp. Endocrinol. 165, 549–556. doi: 10.1016/j.ygcen.2009.07.004
Schreck, C. B., Contreras-Sanchez, W., and Fitzpatrick, M. S. (2001). Effects of stress on fish reproduction, gamete quality, and progeny. Aquaculture 197, 3–24. doi: 10.1016/S0044-8486(01)00580-4
Skomal, G. B., and Mandelman, J. W. (2012). The physiological response to anthropogenic stressors in marine elasmobranch fishes: a review with a focus on the secondary response. Comp. Biochem. Physiol. A 162, 146–155. doi: 10.1016/j.cbpa.2011.10.002
Sopinka, N. M., Capelle, P. M., Semeniuk, C. A. D., and Love, O. P. (2016a). Glucocorticoids in fish eggs: variation, interactions with the environment, and the potential to shape offspring fitness. Physiol. Biochem. Zool. 90, 15–33. doi: 10.1086/689994
Sopinka, N. M., Hinch, S. G., Healy, S. J., Raby, G. D., and Patterson, D. A. (2016b). Effects of experimentally elevated egg cortisol on offspring traits in two species of wild Pacific salmon. Environ. Biol. Fish. 99, 717–728. doi: 10.1007/s10641-016-0513-x
Sopinka, N. M., Hinch, S. G., Healy, S. J., Harrison, P. M., and Patterson, D. A. (2015). Egg cortisol treatment affects the behavioural response of Coho salmon to a Conspecific intruder and threat of predation. Anim. Behav. 104, 115–122. doi: 10.1016/j.anbehav.2015.03.011
Sopinka, N. M., Hinch, S. G., Middleton, C. T., Hills, J. A., and Patterson, D. A. (2014). Mother knows best, even when stressed? Effects of maternal exposure to a stressor on offspring performance at different life stages in a wild semelparous fish. Oecologia 175, 493–500. doi: 10.1007/s00442-014-2915-9
Stein, R. A., and Magnuson, J. J. (1976). Behavioral response of crayfish to a fish predator. Ecol. Soc. Am. 57, 751–761. doi: 10.2307/1936188
Trinnie, F. I., Walker, T. I., Jones, P. L., and Laurenson, L. J. (2012). Biennial reproductive cycle in an extensive matrotrophic viviparous batoid: the sandyback stingaree Urolophus bucculentus from South-eastern Australia. J. Fish Biol. 80, 1267–1291. doi: 10.1111/j.1095-8649.2012.03259.x
Van Rijn, J. A., and Reina, R. D. (2010). Distribution of leukocytes as indicators of stress in the Australian swellshark, Cephaloscyllium laticeps. Fish Shellf. Immunol. 29, 534–538. doi: 10.1016/j.fsi.2010.04.016
Walker, T. I. (2005a). Management Measures. Management Techniques for Elasmobranch Fisheries. FAO Fisheries Technical Paper, Vol. 474. Rome: FAO, 216–242.
Walker, T. I. (2005b). “Reproduction in fisheries science,” in Reproductive Biology and Phylogeny of Chondrichthyes. Sharks, Batoids and Chimaeras, ed. W. C. Hamlett, (Enfield, NH: Science Publishers, Inc), 81–128.
Walker, T. I., and Gason, A. S. (2007). Shark and other chondrichthyan byproduct and bycatch estimation in the Southern and Eastern Scalefish and Shark Fishery. Final Report to Fisheries and Development Corporation. Queenscliff, Vic: Primary Industries Research Victoria.
Watson, J. W., Hyder, K., Boyd, R., Thorpe, R., Weltersbach, M., Ferter, K., et al. (2020). Assessing the sublethal impacts of anthropogenic stressors on fish: an energy-budget approach. Fish Fish. 21, 1034–1045. doi: 10.1111/faf.12487
Wilson, D. T., and Meekan, M. G. (2002). Growth-related advantages for survival to the point of replenishment in the coral reef fish Stegastes partitus (Pomacentridae). Mar. Ecol. Prog. Ser. 231, 247–260. doi: 10.3354/meps231247
Keywords: bycatch, intergenerational consequences, neonate performance, offspring, reproductive consequences, trawling stress, prenatal stress, southern fiddler ray
Citation: Finotto L, Walker TI and Reina RD (2021) Prolonged Alteration of Neonate Traits Following Maternal Exposure to Fishing-Capture Stress During Late Pregnancy in a Chondrichthyan Species. Front. Mar. Sci. 8:631798. doi: 10.3389/fmars.2021.631798
Received: 20 November 2020; Accepted: 28 January 2021;
Published: 25 February 2021.
Edited by:
Antonio Di Franco, Stazione Zoologica Anton Dohrn, ItalyReviewed by:
Manfredi Di Lorenzo, National Research Council (CNR), ItalyNathan Knott, New South Wales Department of Primary Industries, Australia
Katie McGhee, Sewanee: The University of the South, United States
Copyright © 2021 Finotto, Walker and Reina. This is an open-access article distributed under the terms of the Creative Commons Attribution License (CC BY). The use, distribution or reproduction in other forums is permitted, provided the original author(s) and the copyright owner(s) are credited and that the original publication in this journal is cited, in accordance with accepted academic practice. No use, distribution or reproduction is permitted which does not comply with these terms.
*Correspondence: Licia Finotto, bGljaWEuZmlub3R0b0BnbWFpbC5jb20=