- Mote Marine Laboratory’s International Center for Coral Reef Research and Restoration, Summerland Key, FL, United States
Global decline of coral reefs has led to a widespread adoption of asexual propagation techniques for coral restoration, whereby coral colonies are fragmented and allowed to re-grow before being returned to the reef. While this approach has become increasingly popular and successful, many questions remain regarding best practices to maximize restoration speed, efficiency, and survival. Two variables that may influence growth and survival of asexually fragmented colonies include coral genet and growth substrate. Here, we evaluate the effects of genet and substrate (commercially available ceramic vs. in-house made cement) on the survival and growth of 221 microfragments of elkhorn coral Acropora palmata over 193 days. All corals survived the experimental period, and doubled their initial size in 45 days, with an average growth of 545% over the study duration. Growth was generally linear, though the growth of some corals more closely matched logistic, logarithmic, or exponential curves. Both genet and substrate had significant effects on coral growth, though the two factors did not interact. Genet had a stronger influence on coral growth than substrate, with the fastest genet growing at 216% the rate of the slowest genet. Corals on cement substrate grew at 111.9% the rate of those grown on ceramic. This represents both a significant cost savings and elimination of logistical challenges to restoration practitioners, as the cement substrate ingredients are cheap and globally available. Our work shows that both genet and substrate should be considered when undertaking asexual restoration of Acropora palmata to maximize restoration speed and efficiency.
Introduction
Coral reefs, often characterized as underwater rainforests, are among the most biodiverse ecosystems on Earth (Reaka-Kudla, 1997; Jaap, 2000) and perform critical ecological and economic functions for millions worldwide (e.g., Moberg and Folke, 1999; Ferrario et al., 2014). However, dramatic declines in coral cover globally over the past several decades (Gardner et al., 2003; Côté et al., 2005; De’ath et al., 2012) have eroded the functionality of these ecosystems and gravely threaten them and the services they provide (Jones et al., 2004; Alvarez-Filip et al., 2011). These declines have been driven by both natural and anthropogenic stressors such as overfishing, disease, storms, nutrient pollution, coastal development, and climate change (e.g., Gladfelter, 1982; Hughes, 1994; Bruno et al., 2007; Burke et al., 2011; Walton et al., 2018). Reefs will continue to decline as stressors such as bleaching, ocean acidification, and overall impacts of climate change become more prevalent (Hoegh-Guldberg et al., 2007; Albright et al., 2010; Hughes et al., 2017).
The Florida Reef Tract (FRT), located at the southern terminus of the Florida Peninsula spanning from the Florida Keys up the eastern part of the state to Martin County, is the third largest barrier coral reef on Earth and the only barrier coral reef in the continental United States. As of 2015, the Florida Keys National Marine Sanctuary located within the FRT produced an estimated $4.2 billion in economic output to the state of Florida and attracts over three million visitors each year (Tbd Economics, LLC, 2019). However, like most Caribbean reef systems, the FRT has suffered dramatic declines in coral cover from an estimated 25% in the 1980s to an average stony coral cover of 6% today (Ruzicka et al., 2013). Among the several dozen coral species that have dominated shallow water reefs in the western Atlantic for hundreds of thousands of years, elkhorn coral, Acropora palmata, was historically among the most abundant (Jackson, 1992; Precht and Miller, 2007). However, like other coral species, A. palmata has declined dramatically in Florida over recent decades. This decline, driven largely by outbreaks of white-band disease in the 1970s (Gladfelter, 1982; Aronson and Precht, 2001) and more recently by additional stressors including ocean warming, human activities, and storm damage (Baums et al., 2003; Burke et al., 2011; Williams and Miller, 2012; Williams et al., 2017), led to A. palmata being listed as critically threatened by the IUCN in 2008 (Aronson et al., 2008). No significant signs of recovery have been recorded (Miller et al., 2002; Johnson et al., 2011), and recent data suggest that A. palmata populations are now too sparsely distributed across the reef tract to successfully reproduce without direct intervention (Knowlton, 2001; Williams et al., 2008; Miller et al., 2018; National Academies of Sciences Engineering Medicine, 2019). More broadly, because no other Caribbean corals closely resemble A. palmata morphologically or ecologically, the only way in which Caribbean coral reefs could regain their architectural composition and structural and biological function would be to restore A. palmata specifically (Chamberland et al., 2015). Because species such as A. palmata have experienced relatively rapid declines over the last several decades (Bruckner, 2002; Miller et al., 2002; Sutherland and Ritchie, 2004), it is important for practitioners to grow corals quickly and efficiently to reach goals of population enhancement.
The dire state of coral reefs worldwide has led to a surge in active coral restoration efforts. These efforts, which have become increasingly effective (Young et al., 2012; Carne et al., 2016), provide a critical capability to help coral reefs survive the Anthropocene until the drivers of coral loss can be directly addressed. Generally, coral restoration efforts are classified either as sexual restoration, where individual genets are fertilized, grown, and released (or “outplanted”) into the wild, or asexual restoration, in which larger colonies are broken into clonal sub-fragments. Though hobbyists have been asexually fragmenting aquarium corals for many decades, the asexual restoration approach known as “coral gardening” has only been in use for a quarter century (Rinkevich, 1995). This approach, which involves raising corals in nurseries before ‘outplanting’ them back onto reefs, is practiced worldwide (e.g., Montoya-Maya et al., 2016), and includes over 150 programs in more than 20 countries in just the Caribbean and Western Atlantic alone (Lirman and Schopmeyer, 2016). Coral gardening has reached ecologically meaningful scales with 10,000s of coral grown in nurseries and outplanted each year (Lirman and Schopmeyer, 2016). To date, most restoration practitioners focus on asexual coral production through fragmentation (fragment size of several to many cm2) or microfragmentation (fragment size of ∼1 cm2) in part because it is relatively straight-forward in methodology, inexpensive, and can produce large amounts of biomass within short periods of time thus increasing the capability of restoring reefs at large scales. Another advantage of asexual restoration is the capability to propagate “winning” genets at large scales, maximizing the probability of restoration success and reef persistence.
Despite the large number of coral restoration practitioners now operating worldwide, and the environmental and social benefits of sharing best practices, and specific coral propagation methods are often poorly documented. Indeed, such methods often remain unpublished, or are relegated to hobbyist forums or gray literature (Barton et al., 2017), hampering effective, evidence based coral restoration (Boström-Einarsson et al., 2020). Accordingly, the publishing of best practices, failures, benchmarks, and even raw restoration data has been identified as a critical need to help develop the rapidly evolving field of coral restoration (Lirman and Schopmeyer, 2016; Boström-Einarsson et al., 2020).
There are many questions that remain regarding the best practices of coral restoration; among those are identifying how genet and substrate type alter the growth and survival of microfragments. Identifying superior growth substrates is critical for scaling up production at restoration facilities while keeping costs low. Similarly, understanding the various strengths and weaknesses of genets (including growth potential, resilience to disturbance, and reproductive potential) is particularly critical for asexual propagation programs, which often propagate fragments from a small number of genets in their nurseries. As new genets are obtained, practitioners may need to evaluate the characteristics of these genets (e.g., growth rate, stress tolerance) against those in the collection to prioritize propagation and outplanting. While genetic difference in growth across other species has been documented (Osinga et al., 2011; Lirman et al., 2014; Drury et al., 2017), the effects of genet or substrate on the growth rate of A. palmata in restoration facilities are unknown. To help fill this knowledge gap, we performed an experiment to determine the effect of substrate, genet, and their interaction on the growth and survival of A. palmata microfragments during the first six months of life following fragmentation. We hypothesized that coral will grow faster on commercially available ceramic disks than cheaper in-house made cement disks, and that growth rate would significantly differ among genets.
Materials and Methods
Coral Housing
We housed the experimental corals in an outdoor 681 L flow through system (raceway) under a constant flow of 2.5 L/min drawn from a 24 m well. Prior to entering the raceway, water passed through a forced draft degasifier (R&B Aquatic Distribution, Inc., TX, United States), was aerated in a holding tank and mechanically filtered through sand. Water was further aerated within raceways using an air wand (Pentair plc, Minneapolis, MS, United States). We tested water quality twice daily with a YSI Professional Plus handheld multiparameter meter (Yellow Springs, OH, United States). Water remained within the restoration facility’s optimal zones (∼26–28°C, ∼7.7–8.0 pH, ∼38 psu). We used permanent overhead shade cloth (∼67% light reduction), with additional plastic UV lids covered with ∼67% shadecloth (added daily from 12:30 pm to 7:30 am) to maintain raceway temperature and reduce afternoon sunlight. Photosynthetically Active Radiation under the shade cloth was ambient; corals were exposed to a monthly average maximum of 186 μMol/m2/s (January) to 520 μMol/m2/s (July). To reduce fouling and overgrowth by filamentous algae, we added Lithopoma tectum snails as grazers.
Coral Fragmentation and Mounting
In June 2019, we microfragmented 22 ramets from four genets of A. palmata to create the 221 replicate colonies for the experiment (Supplementary Table 1). All ramets were captive, having been settled and raised at Mote Marine Laboratory’s Elizabeth Moore International Center for Coral Reef Research and Restoration in Summerland Key, FL, United States. Corals had been raised within the common garden land-based nursery since fertilization in 2013 and 2014.
We cut each original ramet, measuring ∼7 cm2 in size, using a wet C40 diamond band saw (Gryphon Corporation, CA, United States) into microfragments averaging 0.57 ± 0.12 cm2 SD (range 0.32–0.91 cm2) using previously described methods (see Page et al., 2018). We then used cyanoacrylate glue (Bulk Reef Supply, MN, United States) to adhere the microfragments to one of two substrates: a commercially available ∼7 cm2 ceramic plug (Boston Aqua Farms, MA, United States), or a similarly sized concrete composite plug made in-house from a 3:12:7 ratio mix of commercially available playground sand, portland cement, and fresh water set in a rubber mold (see Supplementary Material for plug construction specifics and Supplementary Figure 1). Finally, we labeled the bases where microfragments were glued (hereafter “plugs”) with unique identifiers and placed them on plastic racks in haphazard orientation. We held racks in a shaded recovery raceway for 3–4 days to stabilize post-fragmentation, then moved the corals to experimental raceways.
Data Collection and Analysis
We assessed coral growth by analyzing photographs taken at c.a. 2-week intervals in the photo analysis program ImageJ (Schindelin et al., 2012). Each photo included a scale bar, which was used in conjunction with the trace tool to calculate total coral area (Figure 1). Photos were analyzed by two trained observers; in the rare case the area calculations were not within 5% agreement, a third observer was used and the two most similar measurements averaged. Because coral plugs differed in their height relative to the scale bar, we applied plug-specific correction factors to reduce growth estimate biases related to plug height. This provided an accurate and precise estimate of coral growth.
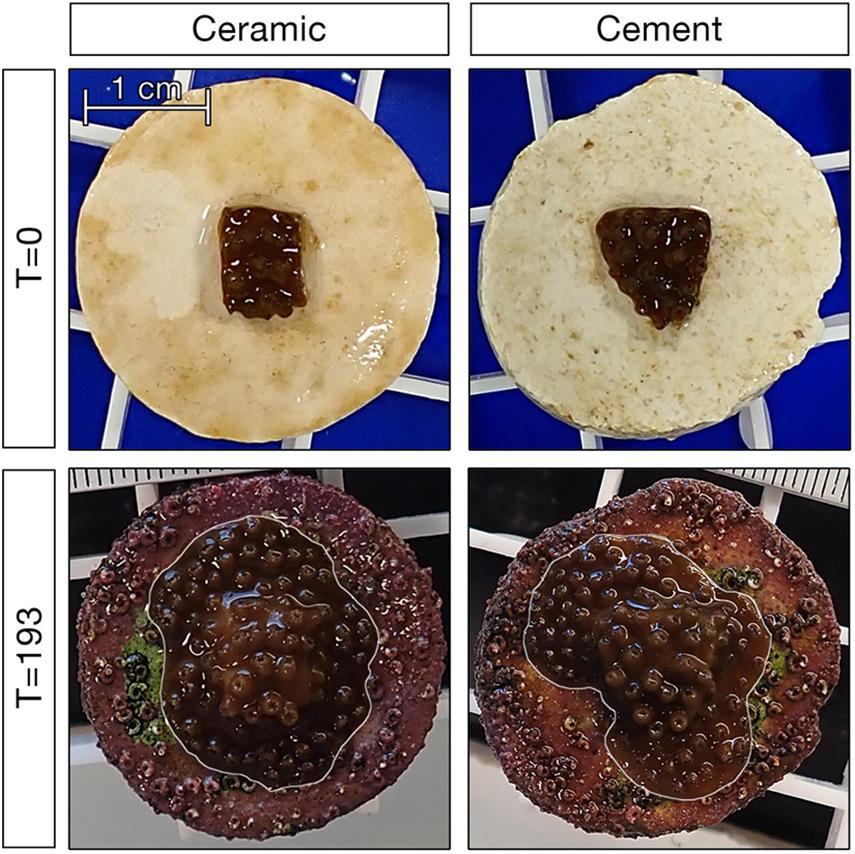
Figure 1. Example of corals on ceramic and cement plugs at the start and end of the 193 day experiment. Corals in the bottom panels are outlined in white to differentiate them from fouling organisms on the plug.
We assessed the effects of genet and substrate type and their interaction on coral growth using a linear mixed effects model, model selection, and model weighting using R 3.6.1 (R Core Team, 2019) and RStudio 1.2.1578 (RStudio Team, 2015). Linear models were used as the vast majority of corals exhibited roughly linear growth rates over time (Supplementary Figure 2). Models were run using the nlme and MuMIn packages (Bartoń, 2020; Pinheiro et al., 2020) following Anderson (2007) and Zuur et al. (2009). The data were right skewed, so we applied a log10 transformation to approximate normality of residuals. General observation during the experiment suggested that ramet (i.e., parent colony) may affect growth. Because ramet was a potentially important source of variability that might otherwise obscure real effects of genet, substrate, or their interaction, we evaluated whether adding ramet as a random effect would improve the model. Four models were compared via AIC until we arrived at the optimal random effects structure: no random effects, ramet only, an interaction of ramet with substrate, and an interaction of ramet with genet (Supplementary Table 2). The optimal model included ramet alone as the random intercept, so it was added to all models that evaluated the fixed effects. To assess the significance of fixed effects, we compared the full model which included substrate, genet, and their interaction to three smaller nested models- one including genet only, one including substrate only, and one including substrate and genet but not their interaction. To complement the binary acceptance/rejection of model parameters and to minimize the problematic use of artificial thresholds (i.e., p = 0.05) to assess significance (Halsey, 2019; Hurlbert et al., 2019), we applied the dredge function from the MuMIn package (Bartoń, 2020) to determine the relative importance of genet, substrate, and their interaction on coral growth rates. Finally, we calculated summary statistics including daily rate of growth and area doubling time for each group retained in the final models.
Results
All corals in this experiment survived and experienced positive growth over the course of the experiment. On average, corals grew from a starting size of 0.57–3.08 cm2, an increase in size of 545% (Table 1).
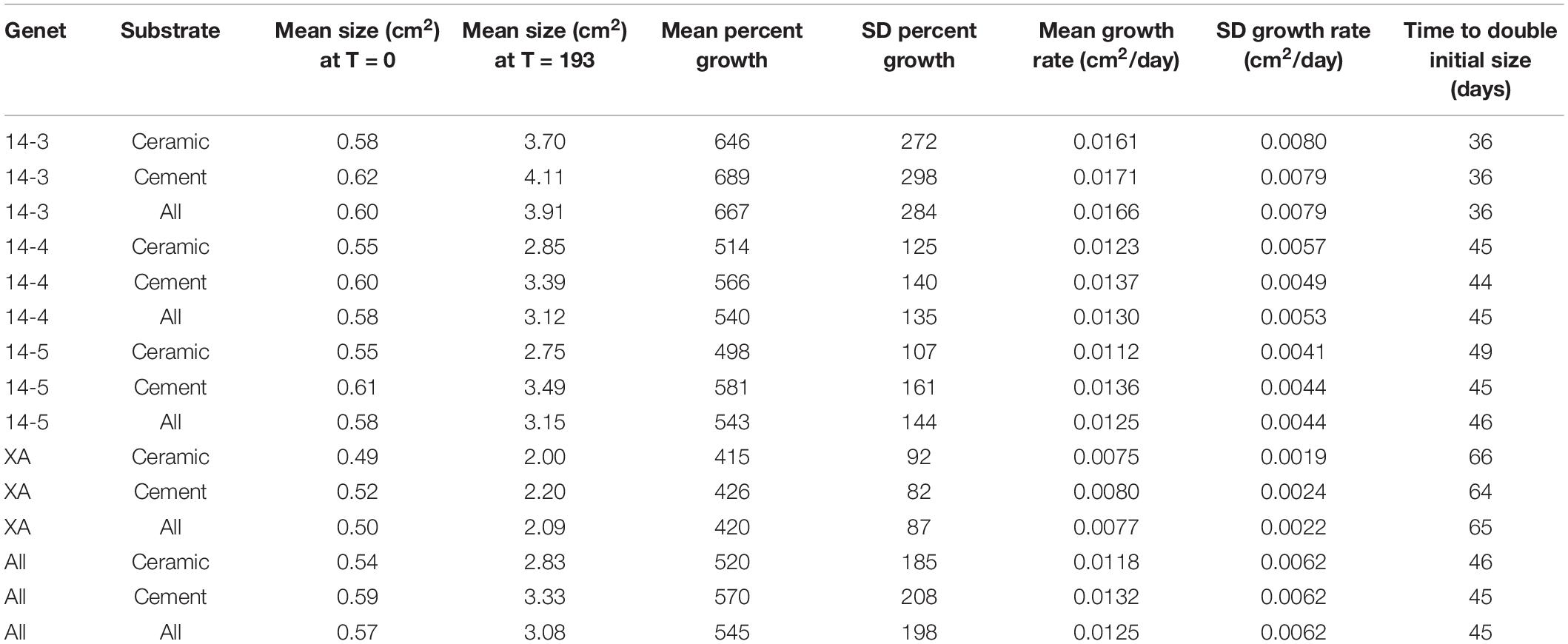
Table 1. Summaries of growth rates, initial fragment sizes, and estimated doubling times (assuming linear growth from time = 0) of coral groups.
As a whole, colony growth was right skewed with a mean of 0.0125 ± σ 0.0062 cm2/day (0.08774 ± σ 0.0434 cm2/week) and a median of 0.0109 cm2/day (0.0760 cm2/week) (Supplementary Figure 3). There was significant variation among microfragments, with the fastest growing microfragments exhibiting an order of magnitude faster growth than the slowest microfragment (daily growth of 0.0031 vs 0.0348 cm2/day or 0.0217 vs 0.2437 cm2/week). Growth was also more variable for faster growing genets (Table 1 and Figure 2).
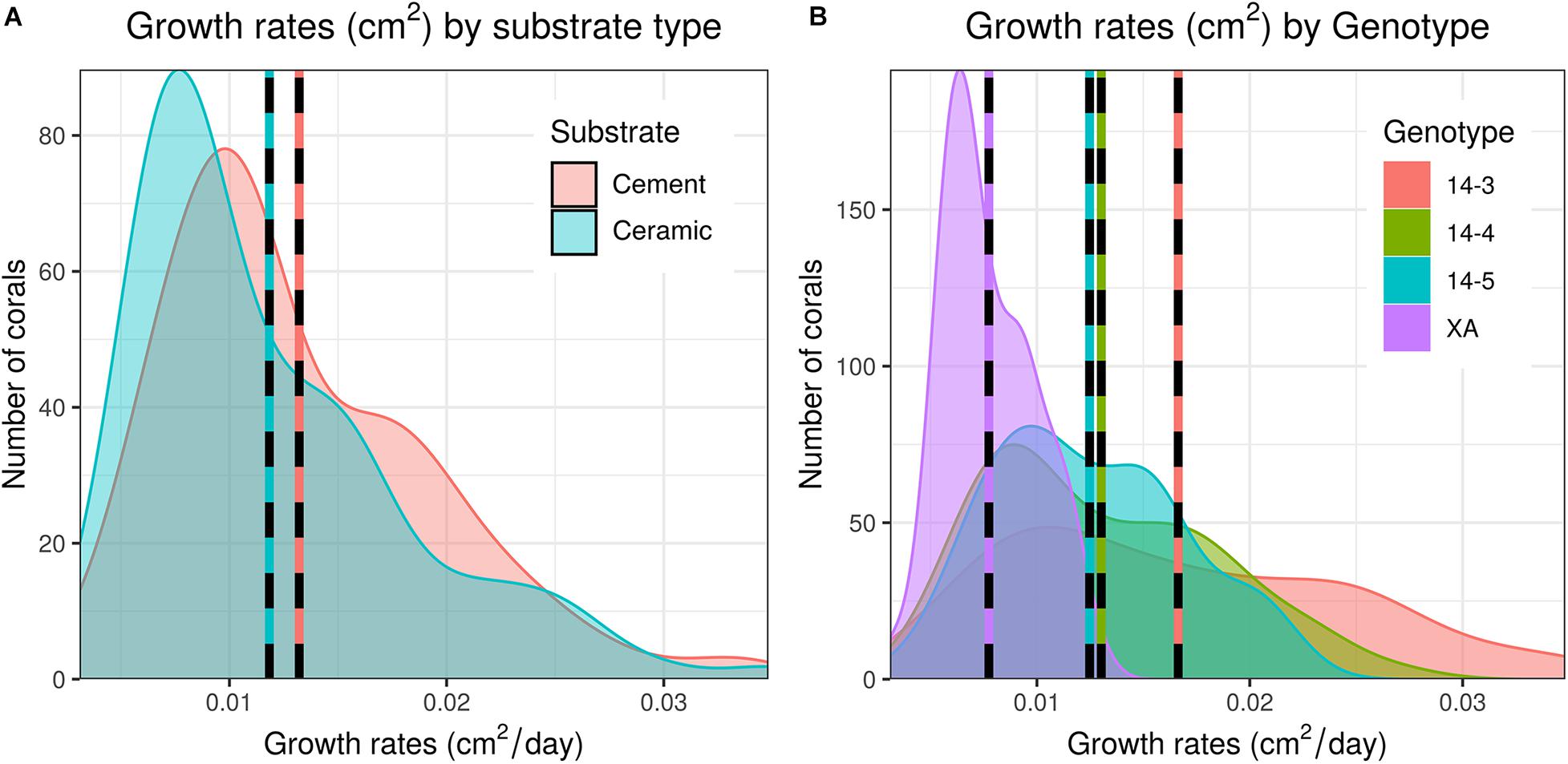
Figure 2. Distribution of daily growth rates of corals between both substrate types (A) and genets (B). Dashed lines indicate mean growth rates within each group. Untransformed data are shown here; data were Log10 transformed for statistical analysis.
The addition of parent colony (i.e., ramet) as a random intercept significantly improved model fit over a model with no random effects, indicating parent colony had a significant influence on coral growth (Likelyhood Ratio Test, L = 66.46, df = 1, p < 0.0001). This model was also more parsimonious than models which included a ramet effect that varied by genet or substrate (Supplementary Table 1). Similarly, model selection indicated that the optimal model included both genet and substrate as fixed effects. This model outperformed both the genet only model (LRT, L = 6.48, df = 1, p = 0.0055) and the substrate only model (LRT, L = 12.72, df = 3, p = 0.0026). However, adding an interaction between substrate and genet did not significantly improve model fit (LRT, L = 3.25, df = 3, p = 0.1773). Multi-model averaging indicated that out of all possible model configurations, 96.6% of model weights included genet as a factor and 91.2% included substrate as a factor, but only 14.4% of model weights included their interaction (Table 2).
Corals placed on in-house made cement plugs grew at 111.9% the rate of corals placed on mass-produced ceramic plugs (0.0132 vs 0.0118 cm2/day, Table 1 and Figure 2). There were also significant differences in coral growth rates between genets (Table 1 and Figure 2), with corals from genet 14-3 growing at 216% the rate of corals belonging to genet XA.
Discussion
Numerous factors can impact the outcomes of coral restoration including coral growth, success/survivorship, and cost-efficiency of coral propagation. Many of these factors are controllable by restoration practitioners, and thus represent valuable opportunities to optimize the restoration process. Here, we isolated two such factors, plug substrate and genet, to determine their effects on growth rates of Acropora palmata microfragments. To our surprise, we found that there was a slight, but significant increase in coral growth on cement plugs compared with commercially available ceramic plugs. Additionally, our cost calculations have determined that the ceramic plugs cost more money than purchasing the materials to produce cement plugs in house. Because of this difference, as well as the availability of the cement supplies at various retailers, the use of cement plugs produces an increase not only in coral growth, but also in cost-efficiency and accessibility. This becomes even more significant when considering that nascent coral restoration operations, including those in remote areas or with limited funding or resources, can use widely available materials to produce cost-efficient coral substrates.
Despite the growth and cost advantages of cement plugs, ceramic plugs still hold several distinct advantages. First, ceramic plugs are smoother and more regular in construction. This not only makes it easier to maintain labels on corals, but also makes it easier to hold, clean, and outplant them, as their stems are uniform in length, width, and angle. Ceramic plugs also provide a more uniform growth surface which may reduce variability during experiments. Furthermore, after cement plugs are constructed, they must be soaked for approximately six weeks before they are chemically inert and ready for use, a step that is not necessary when using ceramic plugs. Indeed, corals mounted on cement plugs initially grew slower than those mounted on ceramic, only overtaking ceramic plugs around 90 days into the experiment. While we did not explicitly test the reason for this, it is possible that leaching of cement plugs may have not been complete. This highlights the significant preparation time required when using cement plugs and is of particular importance for seasonal restoration efforts. Nevertheless, our results show that cement plugs made on-site can be a viable alternative to more expensive, ceramic plugs.
Coral growth significantly differed between substrates, but our results also show that substrate effects are dwarfed by the effect of genet. Faster growing genets can be fragmented more frequently, producing greater numbers of potential outplants (Baums et al., 2019). Identifying these fast-growing genets may help speed up the restoration process and success of restoration programs, maximizing output while minimizing cost. Additionally, because colony size is one of the main criteria for sexual maturity in corals, higher growth may allow corals to more quickly reach sexual maturity and increase fecundity at the colony level due to increased numbers of oocyte producing polyps (Álvarez-Noriega et al., 2016). Finally, rapid growth can allow outplants to better avoid size-specific mortality factors, such as corallivory or algae overgrowth (Drury et al., 2017).
Though rapid growth is a desirable coral trait, growth rate alone is not the only important consideration when choosing coral genets. Indeed, genet impacts variables other than growth (Williams et al., 2017; Pausch et al., 2018), and high growth rates may correlate with tradeoffs in other areas such as recovery from thermal stress (Ladd et al., 2017). Since the ultimate goal of most coral restoration is to create resilient, self-reproducing reefs, genets should also be screened for disturbance resilience as well as fecundity when possible. If individual genetic “stress testing” is not possible, coral gardening must be supplemented with restoration activities using coral larvae to increase genetic diversity (Lirman and Schopmeyer, 2016). With high genetic diversity, mass die offs might not be as common as some corals will be more thermally tolerant or disease resistant (Muller et al., 2018).
While the results of this study contribute to knowledge surrounding the efficient growth of A. palmata microfragments ex situ, they are not necessarily indicative of how these microfragments perform as outplants in the wild. Indeed, since genet performance can interact with environmental variables, future work should consider the success and growth rates of microfragments once they have been reintroduced to their natural habitat, and individual practitioners should conduct growth experiments similar to the one described here not only to identify differences in growth rates within their own genet supply, but also to ensure the substrate effect we describe also holds under local environmental parameters. Importantly, if using growth experiments for restoration optimization purposes, it is critical to track growth rates over the same duration that corals are going to be raised ex situ, since growth rates can change over time and may interact with treatment group or season. Finally, there is a need to expand such work to other coral species used for restoration. As reefs continue to face increasing human stressors, coral propagation will continue to be a critical tool in maintaining reef survival and, eventually, restoration. Our study shows that decisions such as which substrate or genets to choose for restoration can have measurable and significant impacts on restoration speed, efficiency, and cost. As coral propagation expands, sharing such best practices will become increasingly more important for coral restoration to become more efficient and effective in the future.
Data Availability Statement
The raw data supporting the conclusions of this article will be made available by the authors, without undue reservation.
Author Contributions
SH and RN conceptualized the study. EP and BW performed the experiments. RN analyzed the data. All authors wrote the manuscript.
Funding
This work was funded in part by a Mote Marine Laboratory REU award funded by the Monda family awarded to EP, and a postdoctoral research fellowship awarded to RN.
Conflict of Interest
The authors declare that the research was conducted in the absence of any commercial or financial relationships that could be construed as a potential conflict of interest.
Acknowledgments
We would like to acknowledge the assistance of multiple interns and staff at the Mote Marine Laboratory’s Elizabeth Moore International Center for Coral Reef Research and Restoration for expertise and logistical help including Dr. Erinn Muller, Joshua Pi, Zack Craig, Samantha Simpson, Marina Villoch, Chelsea Petrik, Kyle Knoblock, and Brian Richard.
Supplementary Material
The Supplementary Material for this article can be found online at: https://www.frontiersin.org/articles/10.3389/fmars.2021.623963/full#supplementary-material
References
Albright, R., Mason, B., Miller, M., and Langdon, C. (2010). Ocean acidification compromises recruitment success of the threatened Caribbean coral Acropora palmata. Proc. Natl. Acad. Sci. U.S.A. 107, 20400–20404. doi: 10.1073/pnas.1007273107
Alvarez-Filip, L., Côté, I. M., Gill, J. A., Watkinson, A. R., and Dulvy, N. K. (2011). Region-wide temporal and spatial variation in Caribbean reef architecture: is coral cover the whole story? Global. Chang. Biol. 17, 2470–2477. doi: 10.1111/j.1365-2486.2010.02385.x
Álvarez-Noriega, M., Baird, A. H., Dornelas, M., Madin, J. S., Cumbo, V. R., and Connolly, S. R. (2016). Fecundity and the demographic strategies of coral morphologies. Ecology 97, 3485–3493. doi: 10.1002/ecy.1588
Anderson, D. R. (2007). Model Based Inference in the Life Sciences: a Primer on Evidence. New York: Springer Science & Business Media.
Aronson, R. B., and Precht, W. F. (2001). White-band disease and the changing face of Caribbean coral reefs. Hydrobiologia 460, 25–38. doi: 10.1007/978-94-017-3284-0_2
Aronson, R., Bruckner, A., Moore, J., Precht, B., and Weil, E. (2008). Acropora palmata. The IUCN Red List of Threatened Species. Available online at: https://dx.doi.org/10.2305/IUCN.UK.2008.RLTS.T133006A3536699.en. (accessed 19 May 2020).
Barton, J. A., Willis, B. L., and Hutson, K. S. (2017). Coral propagation: a review of techniques for ornamental trade and reef restoration. Rev. Aquac. 9, 238–256. doi: 10.1111/raq.12135
Baums, I. B., Baker, A. C., Davies, S. W., Grottoli, A. G., Kenkel, C. D., Kitchen, S. A., et al. (2019). Considerations for maximizing the adaptive potential of restored coral populations in the western Atlantic. Ecol. Appl. 29, e01978.
Baums, I. B., Miller, M. W., and Szmant, A. M. (2003). Ecology of a corallivorous gastropod, Coralliophila abbreviata, on two scleractinian hosts. i: population structure of snails and corals. Mar. Biol. 142, 1083–1091. doi: 10.1007/s00227-003-1024-9
Boström-Einarsson, L., Babcock, R. C., Bayraktarov, E., Ceccarelli, D., Cook, N., Ferse, S. C. A., et al. (2020). Coral restoration - a systematic review of current methods, successes, failures and future directions. PLoS One 15:e0226631. doi: 10.1371/journal.pone.0226631
Bruckner, A. W. (2002). Proceedings of the Caribbean Acropora Workshop: Potential Application of the U.S. Endangered Species Act as a Conservation Strategy. Silver Spring, MD: NOAA Technical Memorandum NMFS-OPR-24.
Bruno, J. F., Selig, E. R., Casey, K. S., Page, C. A., Willis, B. L., Harvell, C. D., et al. (2007). Thermal stress and coral cover as drivers of coral disease outbreaks. PLoS. Biol. 5:e124. doi: 10.1371/journal.pbio.0050124
Burke, L., Reytar, K., Spalding, M., and Perry, A. (2011). Reefs at Risk Revisited. Washington DC: World Resources Institute.
Carne, L., Kaufman, L., and Scavo, K. (2016). “Measuring success for Caribbean acroporid restoration: key results from ten years of work in southern Belize,” in Proceedings 13th International Coral Reef Symposium (Honolulu).
Chamberland, V. F., Vermeij, M. J. A., Brittsan, M., Carl, M., Schick, M., Snowden, S., et al. (2015). Restoration of critically endangered elkhorn coral (Acropora palmata) populations using larvae reared from wild-caught gametes. Glob. Ecol. Conserv. 4, 526–537. doi: 10.1016/j.gecco.2015.10.005
Côté, I. M., Gill, J. A., Gardner, T. A., and Watkinson, A. R. (2005). Measuring coral reef decline through meta-analyses. Philos. Trans. R. Soc. B 360, 385–395. doi: 10.1098/rstb.2004.1591
De’ath, G., Fabricius, K., Sweatman, H., and Puotinen, M. (2012). The 27–year decline of coral cover on the great barrier reef and its causes. Proc. Natl. Acad. Sci. U. S. A. 109, 17995–17999. doi: 10.1073/pnas.1208909109
Drury, C., Manzello, D., and Lirman, D. (2017). Genotype and local environment dynamically influence growth, disturbance response and survivorship in the threatened coral, Acropora cervicornis. PLoS. One 12:e0174000. doi: 10.1371/journal.pone.0174000
Ferrario, F., Beck, M. W., Storlazzi, C. D., Micheli, F., Shepard, C. C., and Airoldi, L. (2014). The effectiveness of coral reefs for coastal hazard risk reduction and adaptation. Nat. Commun. 5:3794.
Gardner, T. A., Côté, I. M., Gill, J. A., Grant, A., and Watkinson, A. R. (2003). Long-term region-wide declines in Caribbean corals. Science 301, 958–960. doi: 10.1126/science.1086050
Gladfelter, W. B. (1982). White-band disease in Acropora palmata: implications for the structure and growth of shallow reefs. Bull. Mar. Sci. 32, 639–643.
Halsey, L. G. (2019). The reign of the p-value is over: what alternative analyses could we employ to fill the power vacuum? Biol. Lett. 15:20190174. doi: 10.1098/rsbl.2019.0174
Hoegh-Guldberg, O., Mumby, P. J., Hooten, A. J., Steneck, R. S., Greenfield, P., Gomez, E., et al. (2007). Coral reefs under rapid climate change and ocean acidification. Science 318, 1737–1742.
Hughes, T. P. (1994). Catastrophes, phase shifts, and large-scale degradation of a Caribbean coral reef. Science 265, 1547–1551. doi: 10.1126/science.265.5178.1547
Hughes, T. P., Kerry, J. T., Álvarez-Noriega, M., Álvarez-Romero, J. G., Anderson, K. D., Baird, A. H., et al. (2017). Global warming and recurrent mass bleaching of corals. Nature 543, 373–377.
Hurlbert, S. H., Levine, R. A., and Utts, J. (2019). Coup de grâce for a tough old bull: “statistically significant” expires. Am. Stat. 73, 352–357. doi: 10.1080/00031305.2018.1543616
Jackson, J. B. C. (1992). Pleistocene perspectives on coral reef community structure. Am. Zool. 32, 719–731. doi: 10.1093/icb/32.6.719
Johnson, M. E., Lustic, C., Bartels, E., Baums, I. B., Gilliam, D. S., Larson, E. A., et al. (2011). Caribbean Acropora Restoration Guide: Best Practices for Propagation and Population Enhancement. Arlington, VA: The Nature Conservancy.
Jones, G. P., McCormick, M. I., Srinivasan, M., and Eagle, J. V. (2004). Coral decline threatens fish biodiversity in marine reserves. Proc. Natl. Acad. Sci. U.S.A. 101, 8251–8253. doi: 10.1073/pnas.0401277101
Ladd, M. C., Shantz, A. A., Bartels, E., and Burkepile, D. E. (2017). Thermal stress reveals a genotype-specific tradeoff between growth and tissue loss in restored Acropora cervicornis. Mar. Ecol. Prog. Ser. 572, 129–139. doi: 10.3354/meps12169
Lirman, D., and Schopmeyer, S. (2016). Ecological solutions to reef degradation: optimizing coral reef restoration in the Caribbean and western Atlantic. PeerJ 4:e2597. doi: 10.7717/peerj.2597
Lirman, D., Schopmeyer, S., Galvan, V., Drury, C., Baker, A. C., and Baums, I. B. (2014). Growth dynamics of the threatened caribbean staghorn coral Acropora cervicornis: influence of host genotype, symbiont identity, colony size, and environmental setting. PLoS One 9:e107253. doi: 10.1371/journal.pone.0107253
Miller, M. W., Baums, I. B., Pausch, R. E., Bright, A. J., Cameron, C. M., Williams, D. E., et al. (2018). Clonal structure and variable fertilization success in Florida Keys broadcast-spawning corals. Coral Reefs 37, 239–249. doi: 10.1007/s00338-017-1651-0
Miller, M., Bourque, A., and Bohnsack, J. (2002). An analysis of the loss of acroporid corals at Looe Key, Florida, USA: 1983–2000. Coral Reefs 21, 179–182. doi: 10.1007/s00338-002-0228-7
Moberg, F., and Folke, C. (1999). Ecological goods and services of coral reef ecosystems. Ecol. Econ. 29, 215–233. doi: 10.1016/s0921-8009(99)00009-9
Montoya-Maya, P. H., Smit, K. P., Burt, A. J., and Frias-Torres, S. (2016). Large-scale coral reef restoration could assist natural recovery in Seychelles, Indian Ocean. Nat. Conserv. 16, 1–17. doi: 10.3897/natureconservation.16.8604
Muller, E. M., Bartels, E., and Baums, I. B. (2018). Bleaching causes loss of disease resistance within the threatened coral species Acropora cervicornis. Elife 7:e35066.
National Academies of Sciences, Engineering Medicine. (2019). A Decision Framework for Interventions to Increase the Persistence and Resilience of Coral Reefs. Washington, DC: The National Academies Press.
Osinga, R., Schutter, M., Griffioen, B., Wijffels, R. H., Verreth, J. A. J., Shafir, S., et al. (2011). The biology and economics of coral growth. Mar. Biotechnol. 13, 658–671.
Page, C. A., Muller, E. M., and Vaughan, D. E. (2018). Microfragmenting for the successful restoration of slow growing massive corals. Ecol.Eng. 123, 86–94. doi: 10.1016/j.ecoleng.2018.08.017
Pausch, R. E., Williams, D. E., and Miller, M. W. (2018). Impacts of fragment genotype, habitat, and size on outplanted elkhorn coral success under thermal stress. Mar. Ecol. Prog. Ser. 592, 109–117. doi: 10.3354/meps12488
Pinheiro, J., Bates, D., DebRoy, S., Sarkar, D., and R Core Team (2020). nlme: Linear and Nonlinear Mixed Effects Models. R package version 3.1-149.
Precht, W. F., and Miller, S. L. (2007). “Ecological shifts along the Florida Reef Tract: the past as a key to the future,” in Geological Approaches to Coral Reef Ecology, ed. R. B. Aronson (New York, NY: Springer), 237–312. doi: 10.1007/978-0-387-33537-7_9
R Core Team (2019). R: a Language and Environment for Statistical Computing. Vienna: R Foundation for Statistical Computing.
Reaka-Kudla, M. L. (1997). “The global biodiversity of coral reefs: a comparison with rainforests,” in Biodiversity II: Understanding and Protecting Our Natural Resources, eds M. L. Reaka-Kudla, D. E. Wilson, and E. O. Wilson (Washington, D.C: National Academy Press), 83–108.
Rinkevich, B. (1995). Restoration strategies for coral reefs damaged by recreational activities: the use of sexual and asexual recruits. Restor. Ecol. 3, 241–251. doi: 10.1111/j.1526-100x.1995.tb00091.x
Ruzicka, R. R., Colella, M. A., Porter, J. W., Morrison, J. M., Kidney, J. A., Brinkhuis, V., et al. (2013). Temporal changes in benthic assemblages on Florida Keys reefs 11 years after the 1997/1998 El Niño. Mar. Ecol. Prog. Ser. 489, 125–141. doi: 10.3354/meps10427
Schindelin, J., Arganda-Carreras, I., Frise, E., Kaynig, V., Longair, M., Pietzsch, T., et al. (2012). Fiji: an open-source platform for biological-image analysis. Nat. Methods 9, 676–682. doi: 10.1038/nmeth.2019
Sutherland, K. P., and Ritchie, K. B. (2004). “White pox disease of the caribbean elkhorn coral, Acropora palmata,” in Coral Health and Disease, eds E. Rosenberg and Y. Loya (Berlin: Springer), 289–300. doi: 10.1007/978-3-662-06414-6_16
Tbd Economics, LLC (2019). The Economic Contribution of Spending in the Florida Keys National Marine Sanctuary to the Florida Economy. Gaithersburg, MD: TBD Economics, LLC.
Walton, C. J., Hayes, N. K., and Gilliam, D. S. (2018). Impacts of a regional, multi-year, multi-species coral disease outbreak in southeast Florida. Front. Mar. Sci. 5:323.
Williams, D. E., and Miller, M. W. (2012). Attributing mortality among drivers of population decline in Acropora palmata in the Florida keys (USA). Coral Reefs 31, 369–382. doi: 10.1007/s00338-011-0847-y
Williams, D. E., Miller, M. W., and Kramer, K. L. (2008). Recruitment failure in Florida Keys Acropora palmata, a threatened Caribbean coral. Coral Reefs 27, 697–705. doi: 10.1007/s00338-008-0386-3
Williams, D. E., Miller, M. W., Bright, A. J., Pausch, R. E., and Valdivia, A. (2017). Thermal stress exposure, bleaching response, and mortality in the threatened coral Acropora palmata. Mar. Pollut. Bull. 124, 189–197. doi: 10.1016/j.marpolbul.2017.07.001
Young, C. N., Schopmeyer, S. A., and Lirman, D. (2012). A review of reef restoration and coral propagation using the threatened genus Acropora in the Caribbean and western Atlantic. Bull. Mar. Sci. 88, 1075–1098. doi: 10.5343/bms.2011.1143
Keywords: Acropora, substrate, genotype, coral restoration, microfragmentation, asexual propagation
Citation: Papke E, Wallace B, Hamlyn S and Nowicki R (2021) Differential Effects of Substrate Type and Genet on Growth of Microfragments of Acropora palmata. Front. Mar. Sci. 8:623963. doi: 10.3389/fmars.2021.623963
Received: 30 October 2020; Accepted: 24 March 2021;
Published: 13 April 2021.
Edited by:
Iliana B. Baums, Pennsylvania State University (PSU), United StatesReviewed by:
Andrea Oliveira Ribeiro Junqueira, Federal University of Rio de Janeiro, BrazilNathan Formel, University of Miami, United States
Copyright © 2021 Papke, Wallace, Hamlyn and Nowicki. This is an open-access article distributed under the terms of the Creative Commons Attribution License (CC BY). The use, distribution or reproduction in other forums is permitted, provided the original author(s) and the copyright owner(s) are credited and that the original publication in this journal is cited, in accordance with accepted academic practice. No use, distribution or reproduction is permitted which does not comply with these terms.
*Correspondence: Erin Papke, ZXJpbmdwYXBrZUBnbWFpbC5jb20=