- 1College of Marine and Environmental Sciences, Tianjin University of Science and Technology, Tianjin, China
- 2State Key Laboratory of Satellite Ocean Environment Dynamics, Second Institute of Oceanography, Ministry of Natural Resources, Hangzhou, China
- 3School of Marine Sciences, University of Maine, Orono, ME, United States
- 4Department of Biological Sciences, University of Southern California, Los Angeles, CA, United States
In addition to ocean acidification, a significant recent warming trend in Chinese coastal waters has received much attention. However, studies of the combined effects of warming and acidification on natural coastal phytoplankton assemblages here are scarce. We conducted a continuous incubation experiment with a natural spring phytoplankton assemblage collected from the Bohai Sea near Tianjin. Experimental treatments used a full factorial combination of temperature (7 and 11°C) and pCO2 (400 and 800 ppm) treatments. Results suggest that changes in pCO2 and temperature had both individual and interactive effects on phytoplankton species composition and elemental stoichiometry. Warming mainly favored the accumulation of picoplankton and dinoflagellate biomass. Increased pCO2 significantly increased particulate organic carbon to particulate organic phosphorus (C:P) and particulate organic carbon to biogenic silica (C:BSi) ratios, and decreased total diatom abundance; in the meanwhile, higher pCO2 significantly increased the ratio of centric to pennate diatom abundance. Warming and increased pCO2 both greatly decreased the proportion of diatoms to dinoflagellates. The highest chlorophyll a biomass was observed in the high pCO2, high temperature phytoplankton assemblage, which also had the slowest sinking rate of all treatments. Overall, there were significant interactive effects of increased pCO2 and warming on dinoflagellate abundance, pennate diatom abundance, diatom vs. dinoflagellates ratio and the centric vs. pennate ratio. These findings suggest that future ocean acidification and warming trends may individually and cumulatively affect coastal biogeochemistry and carbon fluxes through shifts in phytoplankton species composition and sinking rates.
Introduction
Anthropogenic activities have increased atmospheric pCO2 at a rate of ∼1.8 ppm per year over the last century (IPCC, 2014), with surface seawaters absorbing ∼25% of these CO2 emissions (Le Quéré et al., 2016). This results in increased CO2 and HCO3– concentrations and decreased pH and CO32– concentrations in surface seawaters, collectively referred to as “ocean acidification (OA)” (Caldeira and Wickett, 2003). The average pH of ocean surface waters is predicted to fall from ∼8.1 to ∼7.8 by the end of this century (Feely et al., 2009). In addition, coastal seawater carbonate chemistry can be affected by organic- and acidic sulfate-rich freshwater runoff (Fitzer et al., 2018), or the respiration of organic matter produced as a consequence of eutrophication (Cai et al., 2011), and thus coastal ecosystems are expected to experience more severe acidification (Zhai et al., 2012).
In addition to OA, increasing atmospheric CO2 also has elevated sea surface temperatures (SST) as a consequence of the greenhouse effect, with predicted 1 to 4°C increases in SST over the next 100 years (Bopp et al., 2013). Observed warming trends are not homogeneous between coastal and oceanic regions (Varela et al., 2018). For example, in Chinese coastal waters, a significant warming trend of ∼0.8–2°C per century has been observed in recent years, almost double that of global SST (Carton and Giese, 2008; Feng and Lin, 2009; Wu et al., 2017).
Both CO2 and temperature are important environmental drivers controlling marine phytoplankton physiology. The low CO2 concentrations in seawater can limit the photosynthetic activities of some groups of marine phytoplankton due to the low affinity of the enzyme Ribulose-1,5-bisphosphate carboxylase/oxygenase (RubisCO) for dissolved CO2 (Riebesell et al., 1993; Falkowski and Raven, 2007). As a consequence, phytoplankton have evolved carbon concentrating mechanisms (CCMs) to enhance carbon availability, but the efficiencies of CCMs differ among groups and taxa. Consequently, the increases in CO2 concentrations with ocean acidification may have disproportionate effects among different phytoplankton groups/species. For instance, CO2 enrichment promoted the photosynthetic efficiency of the marine coccolithophore Emiliania huxleyi more than the diatom Skeletonema costatum or the flagellate Phaeocystis globosa (Rost et al., 2003). Rising pCO2 could thus lead to shifts in phytoplankton taxonomic composition and species succession in the natural environment (Schulz et al., 2017; Bach and Taucher, 2019). It has been shown that CO2 enrichment can cause a shift in dominance from small pennate to larger centric diatom species in a Southern Ocean phytoplankton community (Tortell et al., 2008; Feng et al., 2010), whereas some other studies found little effect of changing CO2 concentration on the composition of a coastal phytoplankton assemblage (Kim et al., 2006). In addition, changes in pCO2 may also play an important role in regulating the biomineralization of calcifying coccolithophores (Raven and Crawfurd, 2012) and siliceous diatoms (Milligan et al., 2004), as well as other physiological processes that alter the overall elemental stoichiometry of phytoplankton (Burkhardt et al., 1999; Hutchins et al., 2009; Finkel et al., 2010). During the past decades, numerous studies have especially focused on the sensitivity of calcification process to ocean acidification, with a general trend of weakened calcification (Riebesell et al., 2000; Beaufort et al., 2011; Krumhardt et al., 2017). While for the silicification process of diatoms, although theoretically the formation of biogenic silica should be facilitated at lower pH (Martin-Jézéquel et al., 2000), controversial results has been reported (Milligan et al., 2004). All of these changes will in turn profoundly influence marine biogeochemical cycles.
Temperature is one of the most fundamental environmental drivers determining the growth, metabolic activities, elemental composition, and distribution of marine phytoplankton (Eppley, 1972). Warming has induced changes in geographic distribution, harmful algal bloom intensity, and biodiversity in marine ecosystems (reviewed by Sommer et al., 2012; Hutchins and Fu, 2017). Previous studies have suggested that CO2 enrichment and warming may have combined or interactive effects on phytoplankton physiology (Hutchins et al., 2007; Feng et al., 2008) and species succession and biogeochemistry of natural phytoplankton communities (Hare et al., 2007b; Feng et al., 2009; Sommer et al., 2015; Sett et al., 2018).
However, studies examining the influence of multiple environmental driver interactions on marine life are scarce, and more understanding is needed, especially at the community level (Joint et al., 2011; Boyd et al., 2018). Here we present a continuous incubation experiment that investigated the interactive effects of increased pCO2 and warming on a natural marine phytoplankton assemblage collected from coastal Bohai Sea waters near Tianjin, China, in a region impacted by intense anthropogenic activity. The objective of this study was to investigate the effects of future environmental changes of increased CO2 concentration and temperature both individually and interactively on phytoplankton assemblage succession, elemental composition, and community sinking rates. The findings help us to understand the consequences of future increases in environmental stressors for biogeochemical processes such as carbon export and elemental cycling by coastal phytoplankton assemblages.
Materials and Methods
Experimental Setup and Sampling
Seawater just below the surface (<5 m depth) containing the intact coastal phytoplankton assemblage was collected using a Niskin sampler deployed from a pier near the Tianjin Coastal Recreational Area, Bohai Bay (39.10°N, 117.84°E; temperature: 7°C; salinity: 31; pH: 8.02; nitrate: 8.1 μM; phosphate: 0.4 μM; silicate: 4.2 μM) on March 15, 2017. The whole water was filtered through 100 μm Nitex mesh to eliminate large zooplankton, and then dispensed into 12 4-L clear polycarbonate bottles for incubation. The measured Chl-a biomass was low on the 100 μm Nitex mesh, having little effects on the remaining phytoplankton assemblages. Seawater (∼500 L) collected at the same sampling site was filtered through 0.2 μm in-line filters and stored in 25 L clean carboys, to be used for making dilution medium during the continuous culture incubation experiment.
A continuous culture incubation system (Hutchins et al., 2003; Hare et al., 2005, 2007a,b; Feng et al., 2009, 2010) was used to carry out an outdoor experiment under model projected pCO2 and temperature conditions for the end of this century under some scenarios (IPCC, 2014). Four experimental treatments from full factorial combination of temperature (7 and 11°C) and pCO2 (400 and 800 ppm) were examined in triplicate bottles: (1) control: 7°C and 400 ppm pCO2; (2) high pCO2: 7°C and 800 ppm pCO2; (3) high temperature: 11°C and 400 ppm pCO2; and (4) combined: 11°C and 800 ppm pCO2. Nutrient stock solutions were added to both the initial incubation bottles and the filtered seawater medium to final concentrations of 10 μM nitrate, 1 μM phosphate, and 10 μM silicate. Light levels for the incubations were adjusted using neutral density screens to provide an irradiance of 50% of the incident sea surface level (I0). The incubation temperatures were maintained by submersing the bottles in a circulating water bath connected to temperature control systems (HC1000BC, Hailea, China) via water pumps. Two different CO2 levels were adjusted in both the incubation bottles and carboys containing the filtered seawater for dilution, by gentle bubbling (∼4 mL min–1) of ambient air (∼400 ppm) and an air/CO2 mixture with pCO2 level of 800 ppm controlled by a gas mixer (CE100, Ruihua, China). The bubbling of the incubation bottles and seawater medium was continuous throughout the whole incubation period in order to maintain the seawater carbonate chemistry.
The phytoplankton community was grown in batch mode during the first 3 days of incubation. The continuous incubation mode with a dilution rate of 0.4 d–1 was started on the fourth day (T3) by turning on the peristaltic pumps (Shenchen, China) connected to the bottles through inflow lines going into the bottles from the caps. The dilution rate was chosen based on the average growth rate of the early spring phytoplankton assemblages under nutrient replete conditions in the Bohai Sea (Zou et al., 2001). The outflow outlets were connected at the shoulders of the bottles, in order to maintain a constant volume in the bottles. The bottles were gently shaken and mixed 5–7 times a day to ensure the phytoplankton cells remained suspended.
Samples for pH and total chlorophyll a (Chl-a) measurements were taken daily directly from the incubation bottles. The daily sampling volume was limited to less than 10% of the incubation volume to avoid significant perturbations of the biomass equilibrium (Feng et al., 2009). Size-fractionated Chl-a was sampled only on T0, T3, and T21. The continuous incubation was ended on T21, and samples for measurements of dissolved inorganic carbon (DIC), pH, total alkalinity, Chl-a, phytoplankton community structure, biogenic silica (BSi), particulate organic carbon (POC), particulate organic nitrogen (PON), particulate organic phosphorus (POP) and sinking rates were taken on T0 and the final sampling day. T0 samples were taken from the 100 μm Nitex mesh filtered source seawater in triplicates.
Sample Analysis
Seawater Carbonate Chemistry Measurements
Two hundred and fifty mL samples for total alkalinity analysis were preserved with 200 μL of 5% HgCl2 solution in Shott glass bottles (Shott AG, Germany) with screw caps. Alkalinity was then measured using potentiometric titration following the method of Dickson et al. (2007). The accuracy of the method as determined by analysis of Certified Reference Material provided by Andrew Dickson from Scripps Institution of Oceanography was estimated to be ±2 μmol kg–1. Samples for DIC measurements were fixed in 20 mL borosilicate vials with addition of 0.2 mL of 5% HgCl2 solution. The DIC concentration was measured using a total inorganic carbon analyzer (CM140, UIC, United States) by acidification and coulometric detection, and corrected to the Dickson seawater standards (Dickson et al., 2007). The estimated accuracy of the analysis was ± 5 μmol kg–1. pH values were checked daily using a pH meter (S210-B, Mettler Toledo) calibrated with commercial NBS buffers. The seawater carbonate chemistry (data shown in Supplementary Table 1, output at the incubation temperatures) was calculated using the program CO2sys (E. Lewis and D. W. R. Wallace) with measured DIC and alkalinity values, using the constants in Mehrbach et al. (1973), re-fitted by Dickson and Millero (1987).
Phytoplankton Chl-a Biomass and Assemblage Structure Analyses
20–30 mL samples for Chl-a analysis were filtered and extracted in 90% acetone at –20°C in the dark for 24 h, and then measured using a Turner Trilogy fluorometer. Samples for total Chl-a biomass analysis were filtered through 0.2 μm polycarbonate filters (Millipore, United States); while size-fractionated Chl-a was filtered onto 0.2, 2, and 20 μm polycarbonate filters (Millipore, United States) in series. Samples for phytoplankton taxonomic analysis were preserved with a final concentration of 1% glutaraldehyde and stored at 4°C until analysis. The samples were then concentrated using a 25 mL Utermöhl cell count chamber and counted at 400× magnification under an inverted microscope (AE2000, Motic, China) (Utermöhl, 1958; Thomas, 1997).
Particulate Matter
POC and PON samples (30 mL) were filtered onto pre-combusted (450°C, 2 h) GF/F glass fiber filters (Whatman, United States) and then dried at 55°C. C and N elemental composition was then measured with an elemental analyzer (270, Costech Analytical Technologies, Italy). Thirty mL samples for BSi analysis were filtered onto 0.6 μm polycarbonate filters (Millipore, United States), dried at 60°C, and analyzed using the spectrophotometric method following Brzezinski and Nelson (1995). POP samples (30 mL) were filtered onto pre-combusted GF/F glass fiber filters (Whatman, United States), rinsed with 2 mL of 0.17 mol L–1 Na2SO4, stored in pre-combusted borosilicate vials with addition of 2 mL of 0.017 mol L–1 MgSO4. The samples were then dried and analyzed following the molybdate colorimetric method (Solórzano and Sharp, 1980).
Phytoplankton Sinking Rate
The sinking rate of the phytoplankton assemblage based on Chl-a measurements was determined in triplicate samples using the SETCOL method as described in Bienfang (1981). In brief, a plexiglass column (4 cm in diameter, 1 m in length) was filled up to the top with a homogeneous seawater sample containing the whole phytoplankton assemblage and covered tightly with a glass lid. The column was placed in the dark at the corresponding incubation temperature for a settling time of 3 h, yielding a maximum measurable sinking rate of 8 m d–1. The settling was terminated by carefully draining the upper, middle, and bottom compartments of the column through valved tubing connected through the wall of the column at each depth. The total Chl-a concentration of each compartment was then measured, and the sinking rate was calculated using the following equation:
Here, S is the sinking rate; Cu, Cm, and Cb are the Chl-a concentrations in the upper, middle and bottom compartments of the sinking column, respectively; Vu, Vm, and Vb are the volumes in the upper, middle, and bottom compartments, respectively; L is the length of the column; and t is the total sinking time (Bienfang, 1981).
Data Analysis
Statistics
A two-way ANOVA test was used to detect two-way interactions among temperature and CO2 with the assumptions of homogeneity of variance, independence of observations and independent distribution of variables. Pair-wise tests were conducted using Tukey’s multiple comparison post hoc analysis. The statistical analysis was performed using GraphPadPrism 7.0 software (GraphPad Software, Inc., San Diego, CA, United States).
Types of Driver Interactions
Two-way interactions among temperature and CO2 were determined by quantitative comparisons between the observed effects and the model predicted effects of two drivers. The observed effect was calculated as the percentage of change between the combined treatment and control treatment. A model was used for the predictions of two-way effects (Folt et al., 1999; Liao et al., 2019; Feng et al., 2020):
Here, E1 and E2 denote the individual observed effect of increased pCO2 and warming on the measured parameter, respectively, calculated as the percentage of changes relative to control treatment.
The interactions among increased pCO2 and warming were defined as follows:
(1) Synergistic interactive effect: the observed effect > the calculated multiplicative effect (Folt et al., 1999; Boyd and Hutchins, 2012; Boyd et al., 2018);
(2) Antagonistic interactive effect: the observed effect < the calculated multiplicative effect (Folt et al., 1999; Boyd and Hutchins, 2012; Boyd et al., 2018).
Both synergistic and antagonistic interactive effects can be positive (increase) or negative (decrease).
Results
Total and Size-Fractionated Chl-a Biomass
The total phytoplankton Chl-a biomass kept increasing quickly in the four experimental treatments in the first 5 days of the incubation period (Figure 1). The total Chl-a concentration on T0 at the sampling site was 14.4 ± 1.4 μg L–1, and it increased throughout the 3-day batch incubation mode. The average value of the total Chl-a concentration in the control treatment was lower than those in the other three treatments during the entire course of the incubation (Figure 1). By T5, the Chl-a concentration in all four treatments were more than double the T0 value, with the highest value observed in the combined treatment. During the first 5 days of incubation, the total Chl-a concentration was overall higher the two higher temperature treatments (high temperature and combined) compared to that under control and high pCO2 (Figure 1). The total Chl-a biomass declined in all the experimental treatments from T11 to T15 and the differences between treatments became less than those in the earlier stage of the incubation. The values also became relatively stable as growth and dilution rates came into balance starting from T15 until the final sampling day (T21, Figure 1).
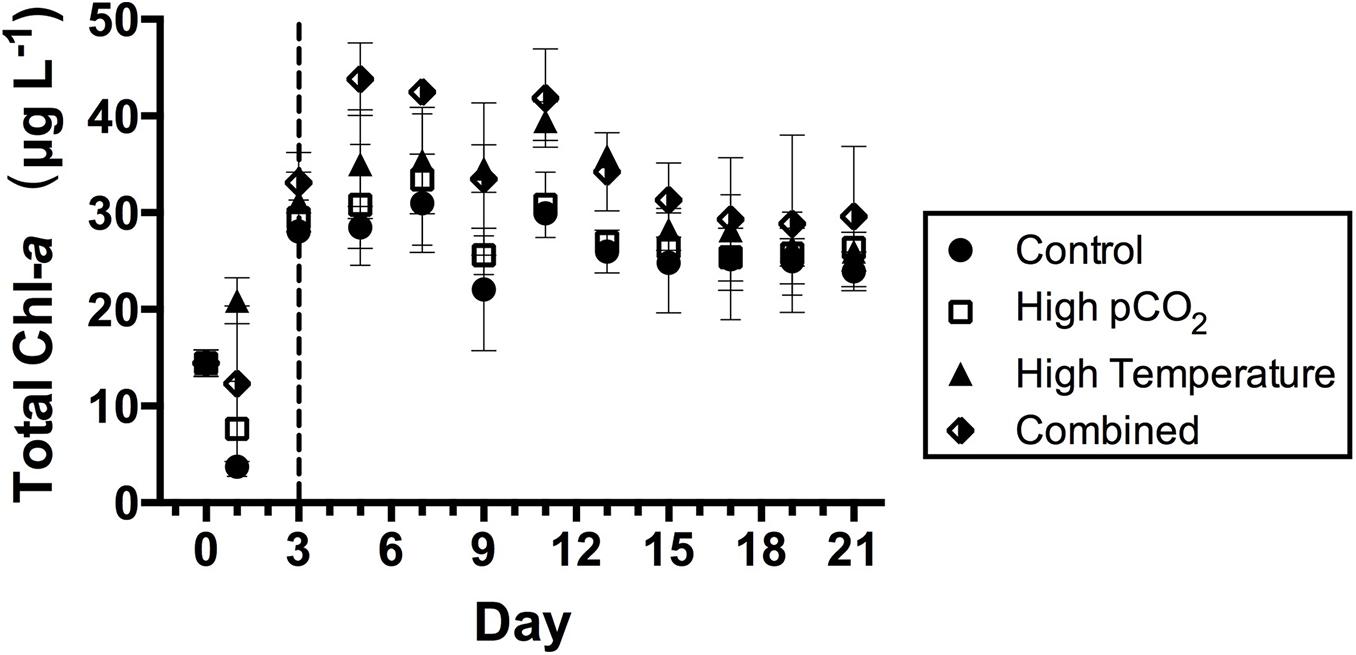
Figure 1. Total Chl-a concentrations during the time course of the incubation in the four experimental treatments. Values are the means and error bars are the standard deviations of triplicates. The dashed line separates the two incubation stages: T0–T3 batch growth mode, and T3–T21 continuous growth code with dilution rate of 0.4 d– 1.
Phytoplankton Chl-a biomass size-fractions responded differently to changes in temperature and pCO2 conditions (Figure 2). The initial (T0) phytoplankton assemblage collected for the incubation was dominated by nano-phytoplankton (2–20 μm), contributing 74.5% of the total Chl-a biomass. The micro-phytoplankton (>20 μm) and pico-phytoplankton accounted for 18.0 and 7.50% of the total Chl-a biomass, respectively (Figure 2). The nano-phytoplankton continued to dominate the Chl-a biomass (>60%) during the incubation in all the experimental treatments. On the final sampling day, there was a general trend of relatively lower percentages of micro-phytoplankton Chl-a biomass but higher percentage of nano-phytoplankton and pico-phytoplankton Chl-a biomass under higher temperature. The percentage of Chl-a biomass in the micro-phytoplankton group was in general lower in the elevated temperature (high temperature and combined) treatments, with the lowest value (14%) observed in the high temperature treatment. Comparatively, the percentage of total Chl-a in micro-phytoplankton was higher (p < 0.05, t-test) under rising pCO2 when temperature was the same, with the highest value of 33.2% in the high CO2 treatment. In contrast, the percentage of nano-phytoplankton increased under elevated temperature but decreased with rising pCO2, with the highest value (81.4%) in the high temperature treatment and lowest percentage (64.0%) under high CO2. The percentage of Chl-a biomass as pico-phytoplankton was small but showed a similar trend with that of the nano-phytoplankton, with the highest and lowest percentages of pico-phytoplankton being 4.6 and 2.8% under high temperature and high CO2, respectively (p < 0.05, t-test, between the two treatments).
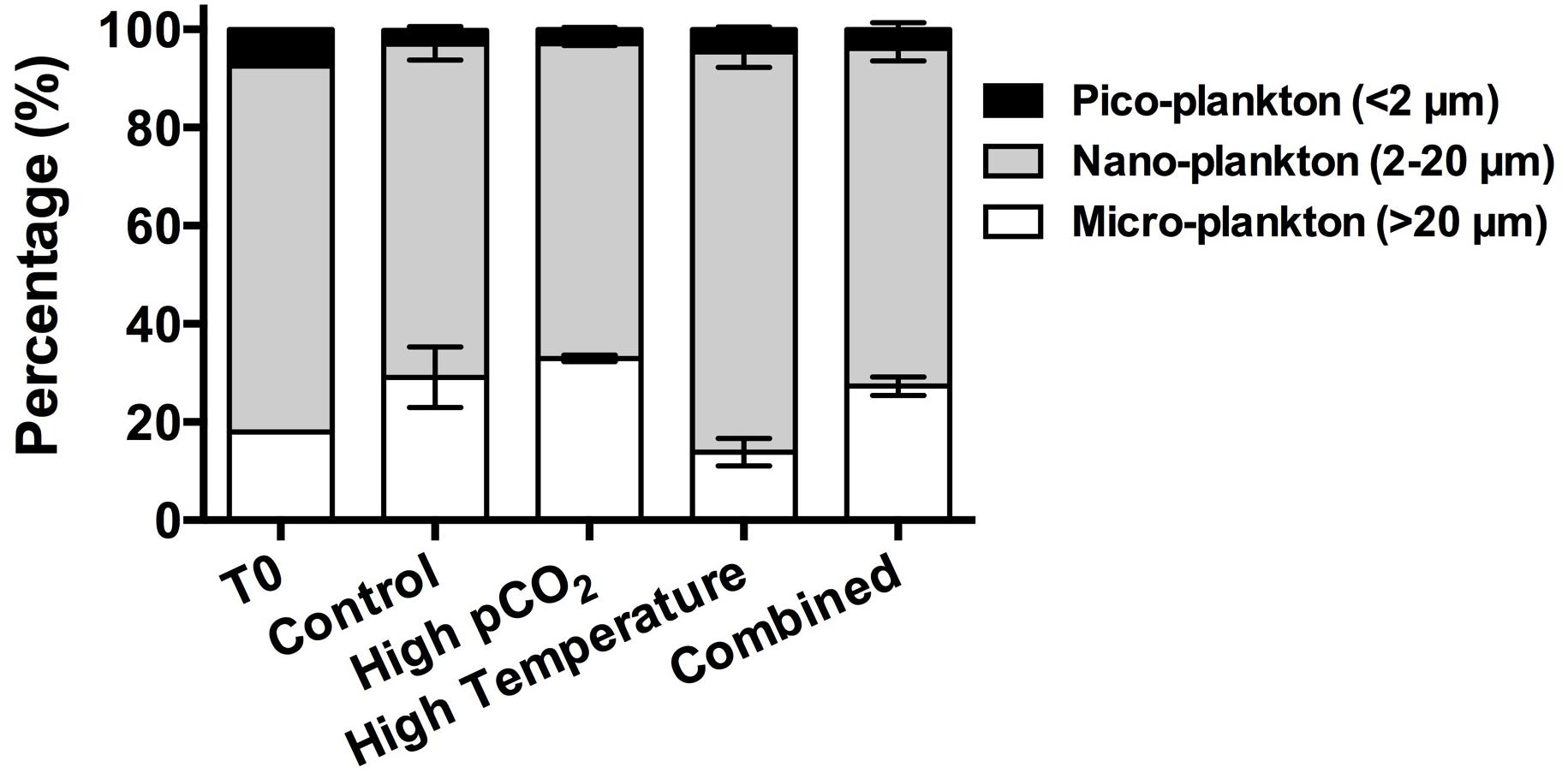
Figure 2. Chl-a biomass percentages of micro-phytoplankton (>20 μm), nanoplankton (2–20 μm), and picoplankton (0.2–2 μm) in the beginning of the incubation (T0) and in the four experimental treatments on the final sampling day. Values are the means and error bars are the standard deviations of triplicates.
Species Composition of the Phytoplankton Assemblage
Microscopic cell counts indicated that the dominant phytoplankton group was diatoms throughout the time course of the incubation, with initial diatom and dinoflagellate abundances of 1090 cells L–1 and 300 cells L–1, respectively. On the final sampling day, there were significant differences in the abundances of diatoms (Figure 3A) and dinoflagellates (Figure 3B) under different temperature and pCO2 treatments. Diatom abundance was significantly lower (∼0.5 fold, p < 0.05) in the high pCO2 and combined treatments compared to the control (4.518 ± 0.468 × 104 cells L–1) and high temperature treatments (4.221 ± 0.293 × 104 cells L–1). However, at the same pCO2 level, there was no significant difference between different temperature treatments (p > 0.05). The dinoflagellate abundance was highest in the high temperature treatment (1016 ± 155 cells L–1, 5.6 fold of the control value). Compared to the control treatment (180 ± 122 cells L–1, the abundance of dinoflagellates was also significantly higher in the high pCO2 treatment (653 ± 151 cells L–1). However, there was no significant difference between the control and combined treatments. The ratio of diatom to dinoflagellates abundances was highest at control (Figure 3C). The ratio significantly decreased by 89, 87, and 74% at high pCO2, high temperature and combined treatments, respectively.
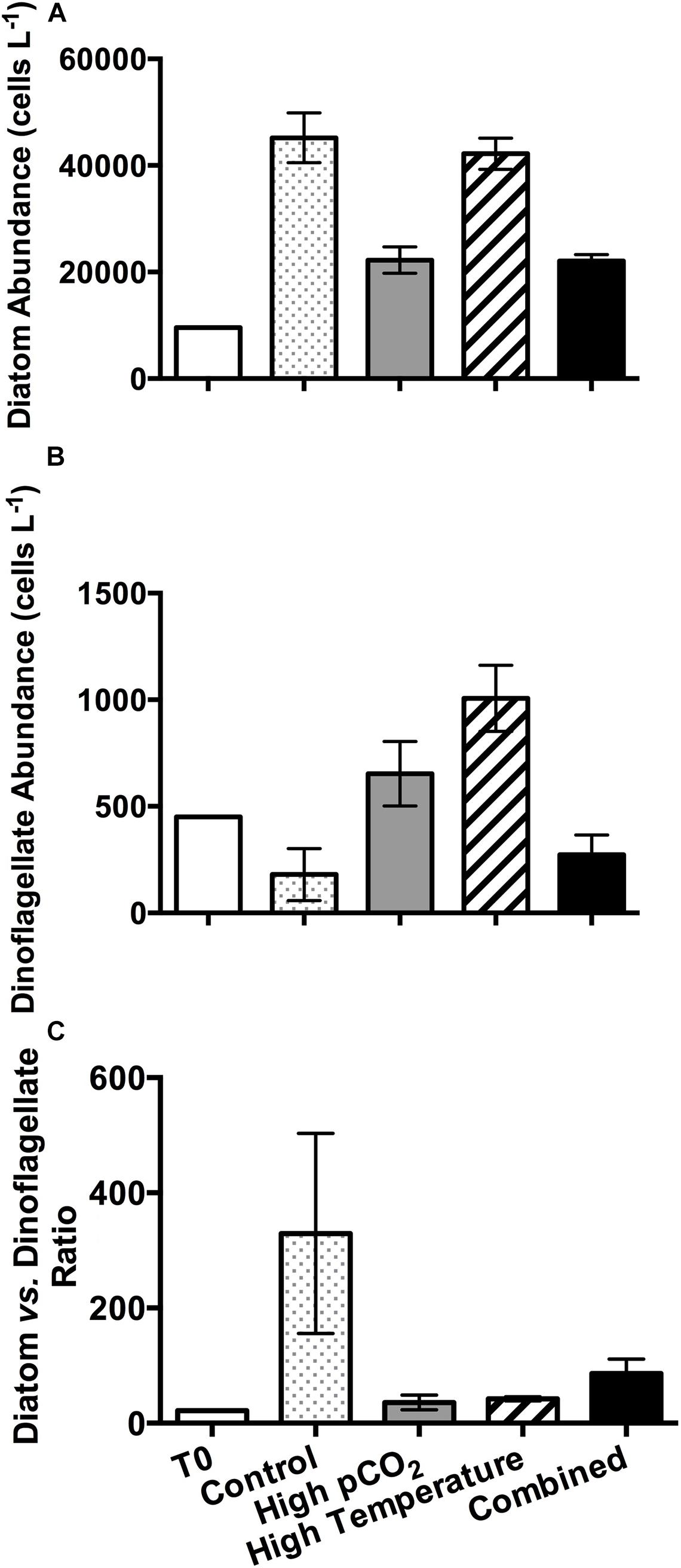
Figure 3. Cell abundances of (A) diatoms and (B) dinoflagellates and (C) ratio of diatom to dinoflagellate abundances in the beginning of the incubation (T0) and in the four experimental treatments on the final sampling day. Values are the means and error bars are the standard deviations of triplicates.
The species composition of the diatom community was also affected by the CO2 and temperature treatments. The full list of diatom and dinoflagellate species and their abundances at the beginning and the four experimental treatments on the final sampling day of the incubation are listed in Table 1. The two most dominant diatom species at the beginning of the incubation were the centric species Thalassiosira pacifica (accounting for 55.91% of the total cell abundance on T0) and the pennate diatom Navicula sp. (18.12%). They continued to be the dominant centric and pennate diatom species respectively in the end of the incubation (Table 1). On the final sampling day, both the centric and pennate diatom abundances were significantly lower in the high pCO2 and combined treatments, compared to the control and high temperature treatments (Figure 4A). The lowest abundance of centric diatoms was observed in the high pCO2 treatment (0.57 fold of the control value), while the abundance of pennate diatoms was lowest in the combined treatment (10% of the control value). In contrast, the ratio of centric to pennate diatom abundance was significantly higher under the higher pCO2 level, with the highest ratio of 9.8 ± 3.0 (ninefold of the control value) in the combined treatment (Figure 4B). Similarly, in the combined treatment, the abundance of the dominant centric diatom species Thalassiosira pacifica was highest, while that of the dominant pennate diatom species Navicula spp. was lowest among all the four experimental treatments (Figure 4C).
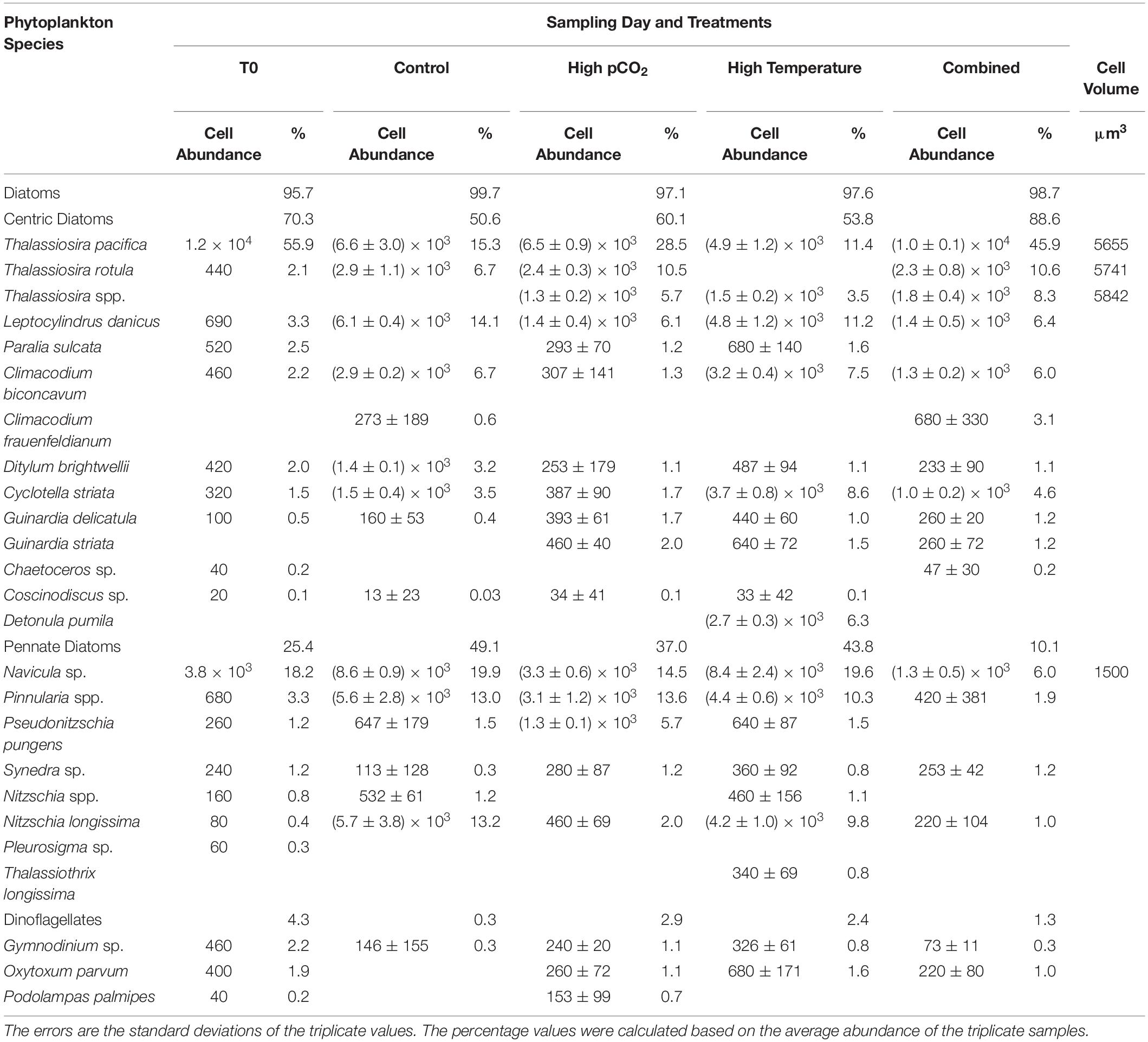
Table 1. List of all diatom and dinoflagellate species and the cell abundances (cells L–1) identified with microscopy on T0 and the four experimental treatments on the final sampling day and cell volume of the dominant species.
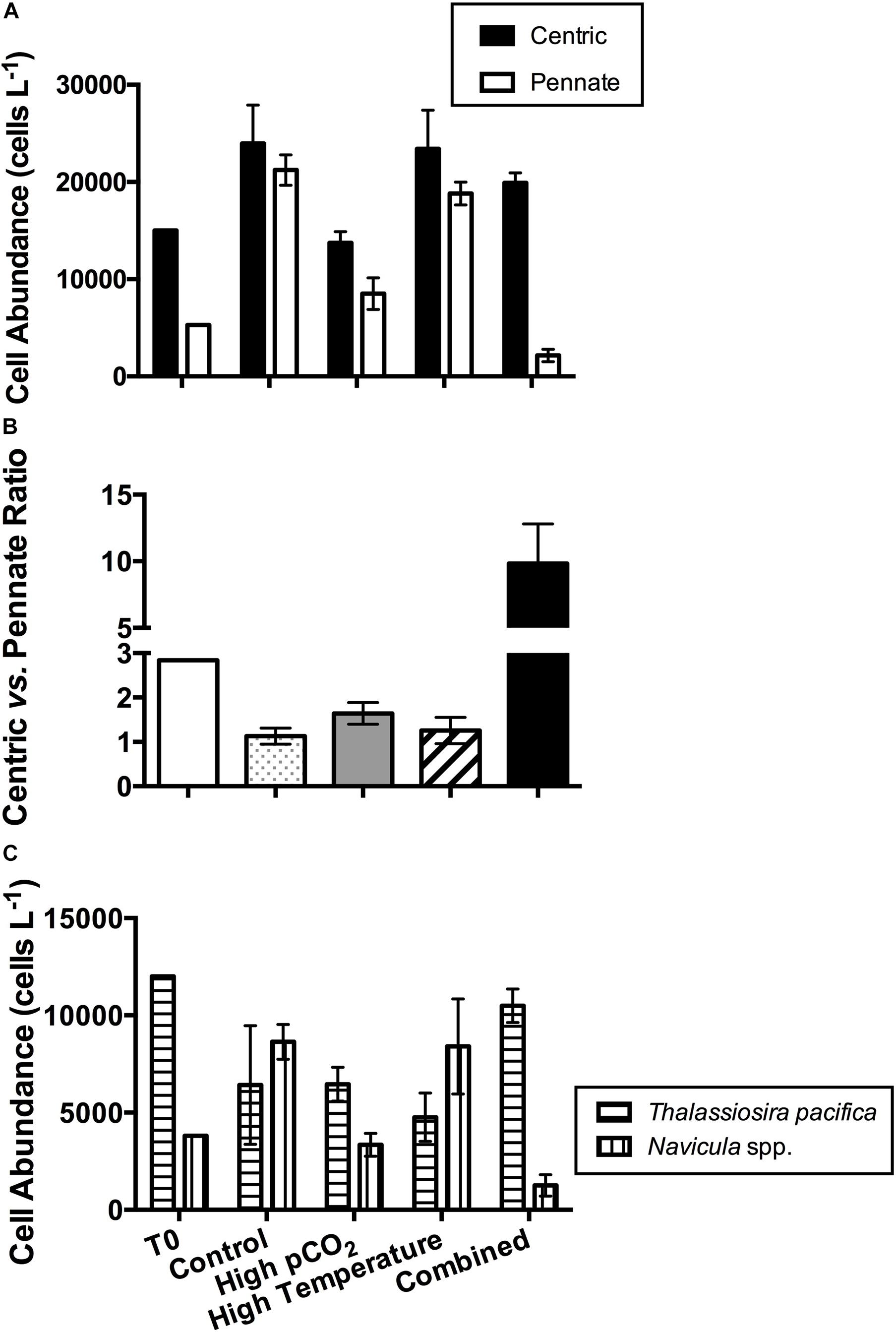
Figure 4. Diatom assemblage composition in the four experimental treatments in the beginning of the incubation (T0) and on the final sampling day: (A) cell abundances of centric and pennate diatoms; (B) ratio of centric diatom to pennate diatom cell abundances; (C) cell abundances of dominant diatom species. Values are the means and error bars are the standard deviations of triplicates.
Elemental Composition and Community Sinking Rate
The average POC concentration was higher under higher pCO2, especially with a significantly higher value in the combined treatment relative to the high temperature treatment (Figure 5A, p < 0.05). Similar to diatom concentration, the BSi concentrations were significantly lower in at higher pCO2 when temperature was the same (Figure 5B, p < 0.05). Changing temperature alone had no significant effect on POC; while elevated temperature significantly increased BSi concentration at both pCO2 levels (p < 0.05). The POP concentration was lowest (Supplementary Data Sheet) in the high pCO2 treatment, with no significant difference among the other three treatments. However, there were no significant differences in PON concentrations in the four experimental treatments (Supplementary Data Sheet).
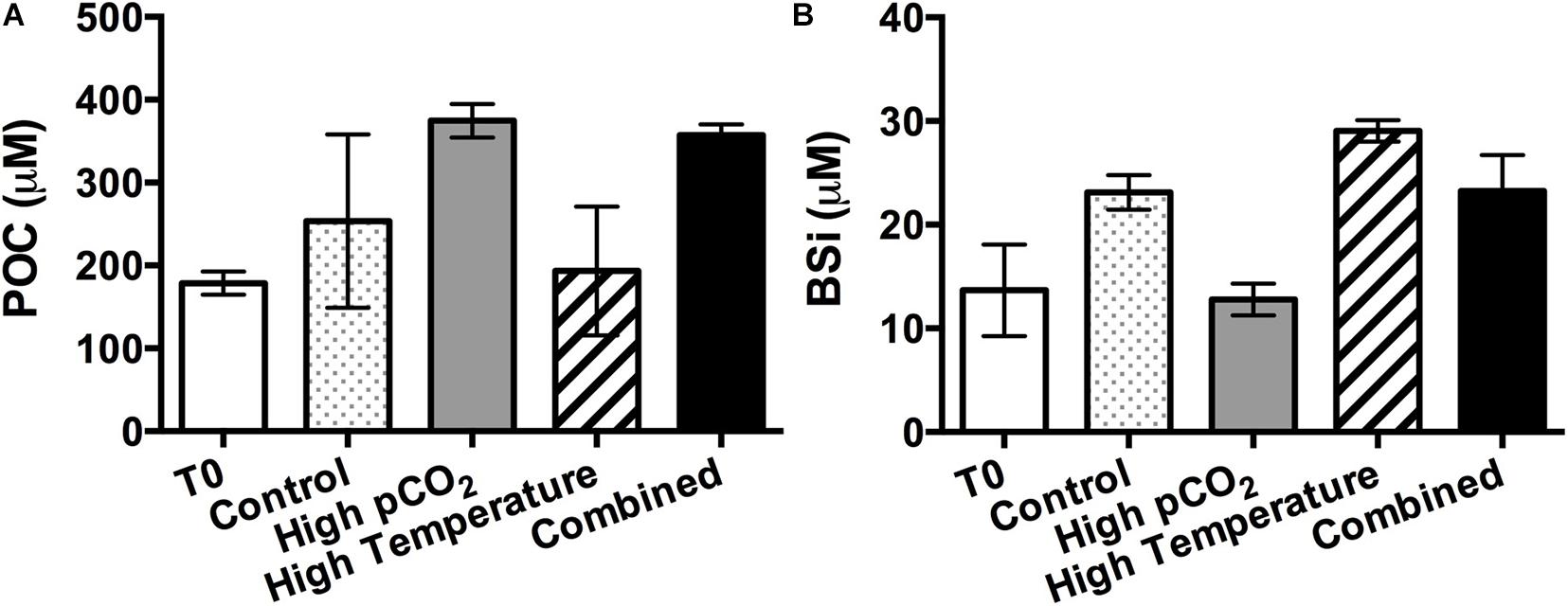
Figure 5. Particulate organic carbon (POC, A) and biogenic silica (BSi, B) concentrations in the beginning of the incubation (T0) and in the four experimental treatments on the final sampling day. Values are the means and error bars are the standard deviations of triplicates.
Both the POC to PON (C:N) and POC to POP (C:P) ratios were highest in the high pCO2 treatment (Figures 6A,B); whereas there were no significant differences among the other three treatments (Figures 6A,B). Similarly, the POC to BSi (C:BSi) ratios were also significantly higher in the high pCO2 and combined treatments compared to the control and high temperature treatments, with the highest ratio observed under high pCO2 (25 ± 4, more than twofold of the control value, Figure 6D).
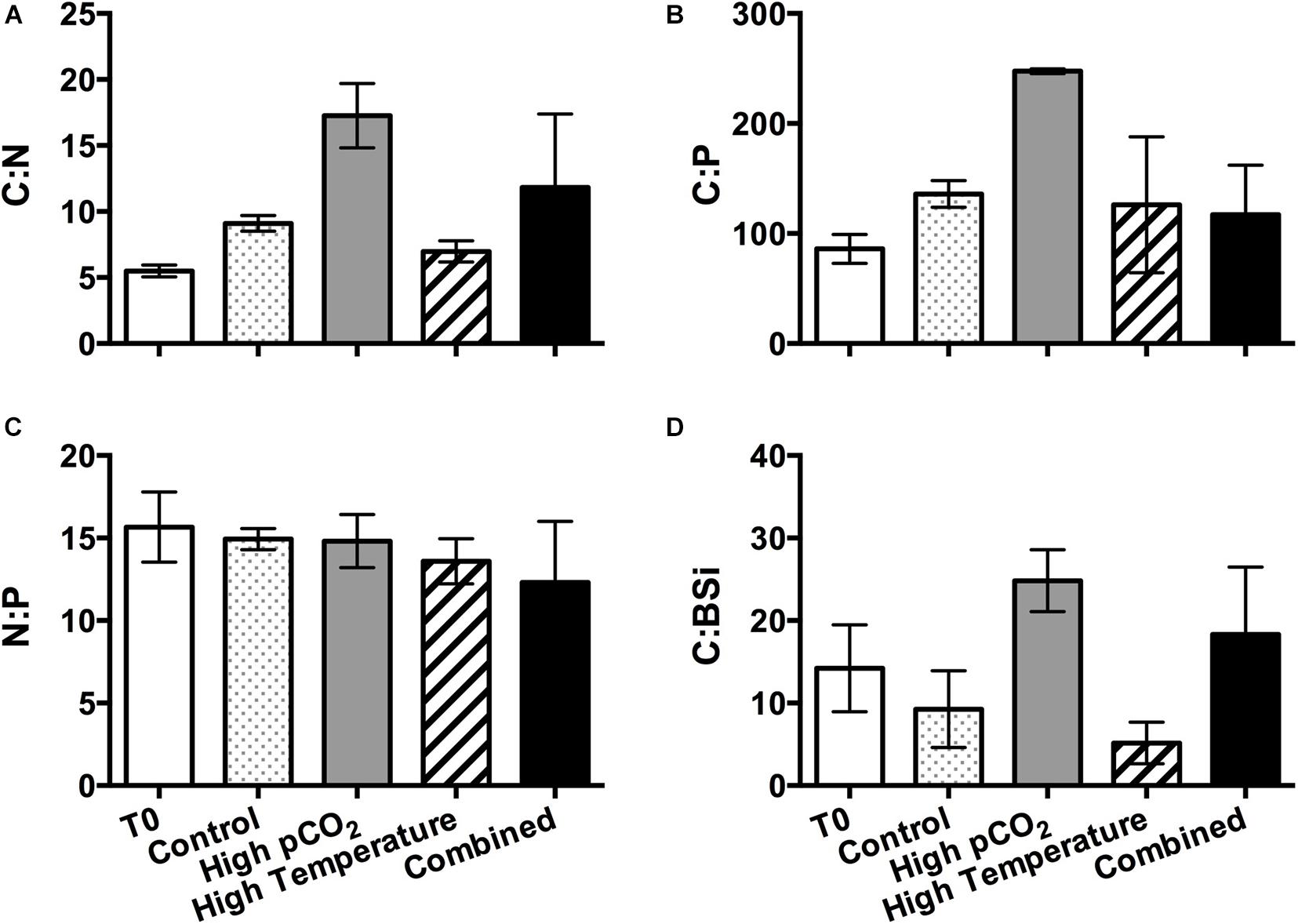
Figure 6. Elemental molar ratios in the beginning of the incubation (T0) and in the four experimental treatments on the final sampling day: (A) particulate organic carbon to particulate organic nitrogen ratio (C:N); (B) particulate organic carbon to particulate organic phosphorus ratio (C:P); (C) particulate organic nitrogen to particulate organic phosphorus ratio (N:P); and (D) particulate organic carbon to biogenic silica ratio (C:BSi). Values are the means and error bars are the standard deviations of triplicates.
The sinking rate of the whole phytoplankton community was lowest in the combined treatment (0.12 ± 0.01 m d–1, half of the control value), which coincided with the markedly higher centric:pennate diatom ratio in this treatment. However, there was no statistically significant difference among the other three treatments (Figure 7).
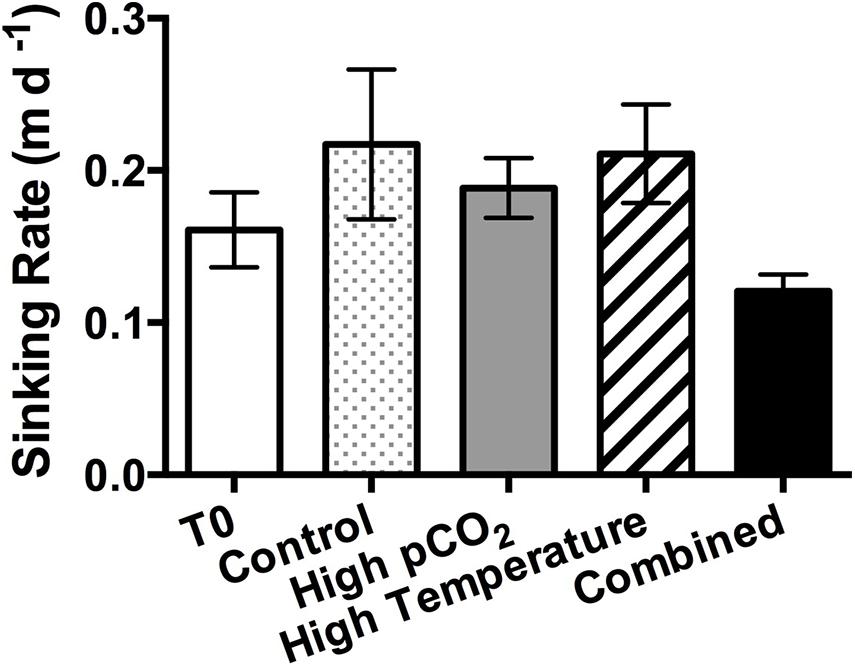
Figure 7. Sinking rates of the phytoplankton community in the beginning of the incubation (T0) and in the four experimental treatments on the final sampling day. Values are the means and error bars are the standard deviations of triplicates.
Individual and Interactive Effects of CO2 and Temperature
The results of two-way ANOVA (Table 2) indicate that CO2 had significant effects on most of the parameters sampled on the final sampling day, including the total diatom, centric diatom and pennate diatom abundances, ratios of diatom vs. dinoflagellate and centric vs. pennate abundances, POC and BSi concentrations, C:N, C:P, C:BSi and sinking rate of the community. Temperature had significant effects on dinoflagellate and pennate diatom abundances, ratios of diatom vs. dinoflagellate and centric vs. pennate abundances, BSi concentration and C:BSi.
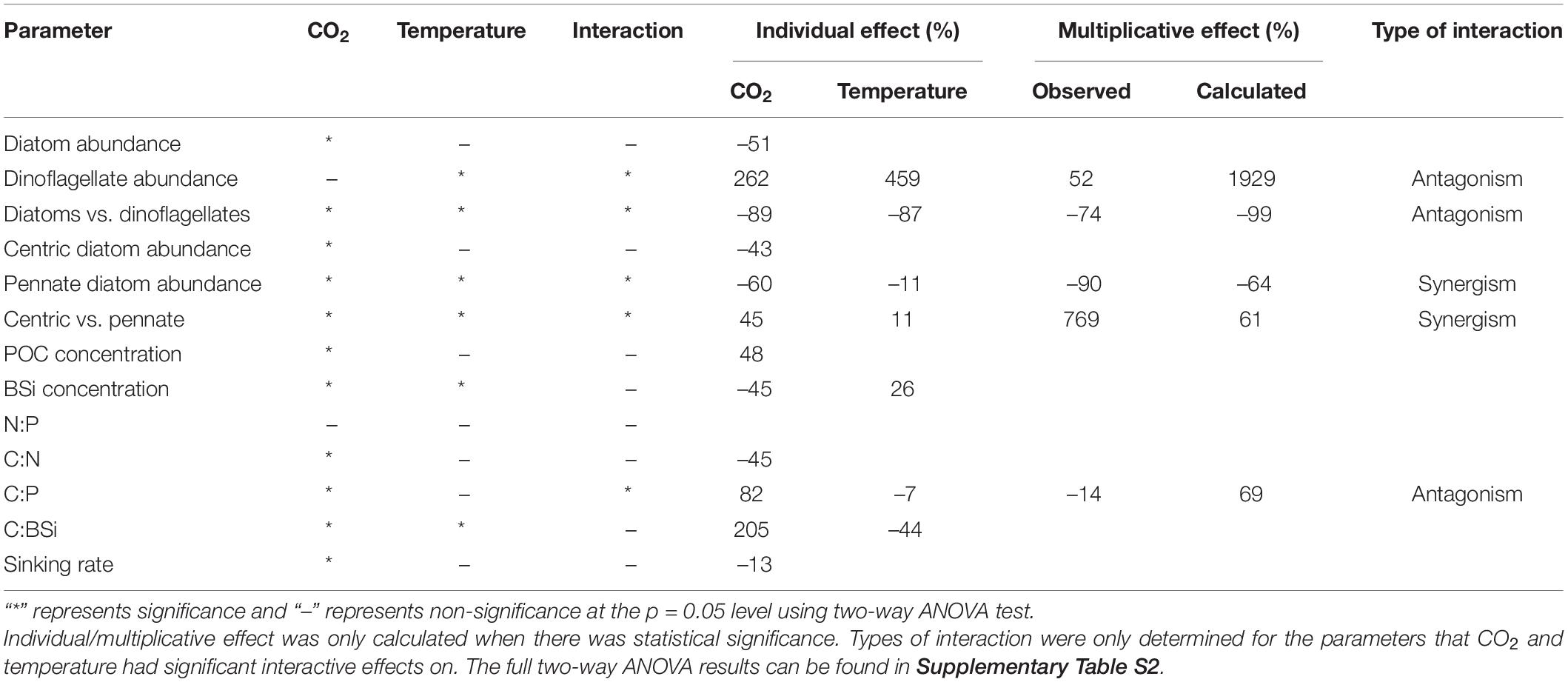
Table 2. The individual and multiplicative effects of temperature and CO2 and types of driver interactions on the parameters of the final sampling day of the experiment.
For the two-way factorial interaction, CO2 and temperature had significant interactive effects on the dinoflagellate and pennate diatom abundances, centric vs. pennate ratio and C:P (Table 2). Among these two-way interactive effects, CO2 and temperature interaction had antagonistic effects on the dinoflagellate abundance, diatom vs. dinoflagellate ratio and C:P, but synergistic effects on the pennate diatom abundance and centric vs. pennate ratio.
Discussion
This study reveals that phytoplankton community size and species composition, elemental ratios, and the sinking rate of the phytoplankton community collected from the Tianjin coastal area all responded to increased pCO2 and/or warming. Apart from the individual effects, the interaction of CO2 and temperature had significant interactive effects on some of the examined parameters, thus having implications for marine biogeochemistry in the future environment. It is noteworthy that our continuous incubation experiment is different from the commonly used short-term batch/grow-out culture technique. This continuous incubation method allows the acclimation of the natural phytoplankton assemblage to the experimental conditions for a much longer period of time (21 days in our study). Community changes in a continuous culture system reflect differences in the growth rates of individual phytoplankton species under the experimental conditions, as faster-growing species come to dominate the assemblage while slower-growing species are ‘washed out’ by dilution. In addition, nutrient enrichment, the “bottle effects” and excluding the large grazers may favor certain phytoplankton groups such as diatoms (Buma et al., 1991; Litchman et al., 2007), as suggested by the differences in the sampled parameters between T0 and Tfinal in our study. Nevertheless, it allows our final sampling to reflect the responses of the natural phytoplankton community to the environmental changes under a relatively steady growth phase (Hutchins et al., 2003; Feng et al., 2009).
Phytoplankton Community Size Structure
The total phytoplankton Chl-a biomass was significantly promoted by warming, especially during the batch growth mode in the first few days with higher values in the high temperature and combined treatments (Figure 1). This warming effect is similar to some previous studies on spring phytoplankton assemblages in both coastal regions (Lee et al., 2019) and the open ocean (Feng et al., 2009), mainly due to the thermal stimulation of phytoplankton metabolism within a favorable temperature range (Eppley, 1972). However, a mesocosm study using a phytoplankton assemblage in the coastal Kiel Fjord showed an opposite trend of decreased Chl-a biomass under warming, mainly due to the fact that temperature increases of 6°C promoted zooplankton grazing more than phytoplankton growth (Sommer et al., 2015). In our study, however, we deliberately removed or reduced larger zooplankton numbers at the outset of the study using a coarse mesh screen, yielding much weakened grazing pressure on the phytoplankton biomass, with only few microzooplankton cells detected in the T0 samples. The removal of large grazers, together with the nutrient enrichment, also resulted in the rapid increase in the total Chl-a concentration in all the incubation bottles during the batch growth mode.
Size-fractionated Chl-a biomass changes suggested that the size of the phytoplankton assemblage may be affected by both temperature and pCO2. Warming greatly reduced the relative biomass percentage of larger micro-plankton cells, while increasing the nano-plankton and pico-plankton percentages (Figure 2). This trend is consistent with some previously published results, showing that elevated temperature may favor smaller species in competition at the community level (Hare et al., 2007a; Daufresne et al., 2009), or simply decrease the cell-size of some species when they grow faster (Atkinson et al., 2003; Sheridan and Bickford, 2011). On the other hand, the larger cell (>20 μm) contribution to total Chl-a biomass was increased by CO2 enrichment under both temperature conditions in our study, and yielded the highest relative abundance of micro-plankton in the high pCO2 treatment. There is no compelling evidence of CO2 effects on cell size within individual species (reviewed by Finkel et al., 2010). Therefore, the changes in community size structure were mainly caused by shifts in phytoplankton species, in agreement with a previous study conducted off the coast of Norway (Engel et al., 2008). Compared to small phytoplankton cells, the growth of larger phytoplankton species tends to be more favored by CO2 enrichment, mainly due to differences in CCM and/or diffusion boundary layer thicknesses (Raven et al., 2008; Finkel et al., 2010).
Phytoplankton Dominance Shifts
Along with the effects of CO2 and warming on the size structure of the phytoplankton assemblage, changes in CO2/temperature also caused shifts in the taxonomic composition of the phytoplankton assemblage in our study. Due to the constraints of the microscopic method that small phytoplankton cells (<5 μm) are harder to be distinguished, the species determined here mostly belonged to the micro-plankton and nano-plankton groups. Here we observed that the abundance of dinoflagellates was especially promoted in the high temperature treatment (Figure 3B). Although diatoms generally grow much faster than dinoflagellates at the same temperature (Chen and Laws, 2017), the distribution of many dinoflagellate species tends to be more favored in warm waters, compared to diatoms (Finkel et al., 2010; Xiao et al., 2018). Nevertheless, dinoflagellate abundance decreased again in the combined treatment, likely due to the outcome of competition with other nano-phytoplankton and pico-plankton groups. In coastal regions, diatoms and dinoflagellates are the major dominating phytoplankton functional groups (Smayda and Reynolds, 2003). The ratio of diatoms to dinoflagellates is considered to be an environmental indicator, with higher diatoms: dinoflagellates values indicating better seawater quality (Wasmund et al., 2017) and alleviating the eutrophication problem of the water column (Spilling et al., 2018). Changes in phytoplankton species composition may affect the food quality provided to the upper food web (Rose et al., 2009) and reproduction of the zooplankton, with higher copepod reproduction related to relative higher dinoflagellates abundance (Vehmaa et al., 2011). In addition, during costal spring blooms with low grazing pressure, sinking of ungrazed phytoplankton cells contributes to the bottom hypoxia. Diatom cells tend to sink out of the surface layer faster than dinoflagellates and these generally larger cells consume more oxygen during degradation in the subsurface waters; hence, decrease in diatom abundance likely results in reduced oxygen demand of the bottom water in the coastal regions (Spilling and Lindström, 2008). Our results suggest that the proportion of diatoms to dinoflagellates significantly decreased when either pCO2 or temperature was increased individually or simultaneously, indicating a potential negative feedback of future climate change on the oxygen demand in bottom waters in the Bohai Bay area, as demonstrated for the Baltic Sea (Wasmund et al., 2017).
Both diatom abundance and species composition was significantly affected by changes in pCO2 (Figure 3A). The diatom RubisCO enzyme tends to have a high affinity for CO2, supplied by efficient CCMs (Gao and Campbell, 2014), and thus diatom photosynthesis may be already saturated under present day CO2 conditions (Burkhardt et al., 2001; Rost et al., 2003). As a result, although diatoms were still dominant in the phytoplankton assemblage, total diatom abundance as well as centric and pennate diatom abundances were not promoted, but rather all decreased at higher pCO2 in our study (Figure 4). This is most likely due to the outcome of the competition with other phytoplankton groups that were favored more under higher pCO2. Similarly, a study on the South China Sea phytoplankton community also found diatom abundance declined with elevated CO2 (Gao et al., 2012). However, the abundance of the dominant centric diatom species T. pacifica and the centric vs. pennate diatom ratio showed an opposite trend in our study. The higher centric vs. pennate diatom ratio observed in our study is consistent with previous findings of CO2 enrichment experiments on the Southern Ocean phytoplankton community, which also reported larger centric diatoms were favored more under rising pCO2 (Tortell et al., 2008; Feng et al., 2010), further indicating differential responses to CO2 among different diatom taxonomic groups (Wu et al., 2014). By synthesizing the results of more than a decade research on natural diatom communities responses to OA, Bach and Taucher (2019) also found that more than 65% of the experiments showed changes in diatom species composition with a trend of shifting toward larger species. The taxonomic changes toward larger cells may in turn accelerate the energy transfer efficiency into higher trophic levels in marine food web (Bach and Taucher, 2019). In our study, the centric vs. pennate diatom ratio was further increased in the combined treatment, suggesting potential further influence on the energy transfer in the food web of Bohai Bay area in the future climate change scenario.
Elemental Stoichiometry and Sinking Rate
As a consequence of the shifts in phytoplankton species composition and the direct intra-specific physiological responses to the experimental conditions under different temperature and CO2 levels, our results also showed significant changes in the elemental composition (POC and BSi concentrations, C:N, C:P, and C:BSi, Figure 6) of the phytoplankton community. The significantly higher C:N and C:P ratios in the high pCO2 treatment were consistent with the results of previous laboratory studies on phytoplankton, including isolates of cyanobacteria (Fu et al., 2007), coccolithophores (Feng et al., 2008), and diatoms (Burkhardt and Riebesell, 1997). This finding likely indicates that CO2 enrichment promotes carbon fixation more than N and P uptake, and may further have implications on predicting the elemental stoichiometry of the organic matter export by marine phytoplankton into the deeper layers. Nevertheless, Taucher et al. (2020) synthesized the data of in situ manipulation experiments on natural plankton community and observed OA-induced 20% increase to 17% decrease in C:N export ratio in the sinking particles. Their results also suggest the C:N export ratio can be regulated greatly by plankton feeding and microbial degradation, as well as other environmental drivers such as temperature and nutrient availability (Taucher et al., 2020 and references therein).
Another significant trend was the increased C:BSi ratios under CO2 enrichment mainly caused by enhanced POC accumulation and reduced BSi concentration under higher pCO2. The latter may be attributed not only to reduced total diatom abundance, but also to decreased BSi production by diatoms. Milligan et al. (2004) reported enhanced efflux of silicate from the diatom cells at high pCO2 while silicate influx was not changed; as a result, the cellular silica quota was reduced with elevated pCO2 (Milligan et al., 2004). A study on a Southern Ocean diatom assemblage also found decreased community silica production under seawater acidification, but with strain-specific responses (Petrou et al., 2019). In general, mean silica production has a linear relationship with the surface area of diatom cells (McNair et al., 2018), but is not necessarily correlated with the growth rate (Petrou et al., 2019). However, the complete mechanisms of CO2 regulation of BSi production are still not clearly understood. The silica cell walls of diatoms are considered in the context of functions that include defense from grazing (Hamm et al., 2003) and ballast for sinking and carbon export (Buesseler, 1998). As such, further understanding the regulatory mechanisms for BSi production in the changing marine environment will help to unravel the impacts of environmental changes on carbon export in diatom-dominated waters.
In our study, the sinking rate of the phytoplankton assemblage was lowest in the combined treatment, mainly affected by pCO2 based on the two-way ANOVA results, despite the lowest BSi concentration being observed in the high pCO2 treatment. Although the ballasting effect of BSi is an important factor driving the sinking rate of diatoms, the reduced sinking rate in the combined treatment might be attributed to the changes in phytoplankton species composition instead. Compared to the other three experimental treatments, the abundance of the dominant centric diatom species T. pacifica was remarkably higher in the combined treatment (Table 1 and Figure 4C). It is noteworthy that T. pacifica is a chain-forming species, and the formation of chains can influence buoyancy and slow the sinking rates of the cells (Smayda and Boleyn, 1966). On top of that, the chain length of the chain-forming diatoms tends to increase with rising pCO2 as in the case of Asterionellopsis glacialis (Barcelos e Ramos et al., 2014), while the size of the phytoplankton community may be decreased under elevated temperature, together further reducing the sinking rate in the combined treatment (Denny, 1993). The sinking rate of a phytoplankton community can also be affected the production of transparent exopolymer particles (TEP), which have lower density but facilitate the cells to form large aggregates (Alldredge and Jackson, 1995). As the carbon production in the surface layer is the major contributor of carbon export to depth (Tréguer et al., 2018), if our finding represents a general future trend, OA and warming together may weaken carbon export from the surface layer in diatom-dominated coastal environments. However, we also should consider that, in the real oceanic environment, the actual carbon export to depth will generally be further influenced by grazing and remineralization processes (Armstrong et al., 2002).
Driver Interactions and Implications for the Coastal Biogeochemistry Under Global Change
The findings of our study suggest that both the individual and interactive effects of OA and warming are important to consider for coastal phytoplankton assemblages. There is clear evidence that many environmental drivers are already changing simultaneously in coastal and oceanic regions, including ocean acidification, warming, deoxygenation, changes in nutrient supply, and surface irradiance regimes (IPCC, 2014; Hutchins and Fu, 2017). Therefore, it is urgent to understand the cumulative effects of multiple drivers on the marine biota, and disentangle the interactive patterns of these drivers (Boyd et al., 2018). Our results suggest that CO2 and temperature synergistically affected pennate diatom abundance and the centric vs. pennate diatom ratio, and had antagonistic effects on dinoflagellate abundance and the ratio of diatom:dinoflagellate abundances. The cumulative effects of OA and warming together may either “amplify” or “shrink” the effects of the individual-driver effects, further emphasizing the importance of considering multiple driver interactions for predicting the biogeochemical responses to future environmental changes. Similarly, a study on the South China Sea phytoplankton community observed that although OA and warming alone promoted the phytoplankton productivity, the combination of the two drivers had an antagonistic effect (Zhang et al., 2018). Sett et al. (2018) also reported strongly shifted diatom species composition by the combined effect of OA and warming. During the past decade, many studies have focused on examining the combined effects of OA and other environmental drivers on natural phytoplankton community (Bach and Taucher, 2019 and references therein). Future work also needs to compile the published results and synthesize the general patterns of the interactive effects of OA and warming on natural phytoplankton assemblages in different oceanic regions.
Overall, our study on a spring bloom phytoplankton assemblage reveals that under future environmental conditions when both OA and warming effects are considered, the centric to pennate diatom ratio will increase, while the diatom:dinoflagellate ratio and phytoplankton-induced sinking rate will significantly decrease in the coastal Bohai Bay area. These changes may further impact carbon export and elemental cycles in the coastal ecosystem, and may impact food and nutritional availability to higher trophic levels, thus affecting coastal fisheries. However, there are limitations to how far we can extrapolate our findings. Our study examined CO2 and warming effects using a coastal phytoplankton assemblage acclimated to constant experimental conditions. In contrast, CO2/temperature variability is generally high in the coastal environment (Dai et al., 2009; Li et al., 2016; Kling et al., 2020), especially in regions highly influenced by anthropogenic activities like Bohai Bay. Consequently, coastal phytoplankton assemblages may possess relatively high tolerance limits to thermal and CO2 shifts. Future studies are needed that examine the effects of ocean acidification and warming in the context of natural fluctuations in pCO2 and temperature in coastal ecosystems.
Data Availability Statement
The original contributions presented in the study are included in the article/Supplementary Material, further inquiries can be directed to the corresponding author/s.
Author Contributions
YF designed the study. YL, PL, and TZ conducted the experiments. YL, PL, TZ, TC, and FF analyzed the samples. YF, FC, MW, FF, and DH thoroughly discussed the results and interpreted the data. YF, FC, MW, and DH wrote the manuscript with contributions from all co-authors. All the authors contributed to the article and approved the submitted version.
Funding
This work was supported by the National Natural Science Foundation of China grants (nos. 41676160 and 41306118), the Tianjin Natural Science Foundation (19JCYBJC22900), Tianjin Municipal Education Commission Project (2017KJ014), and the open fund of State Key Laboratory of Satellite Ocean Environment Dynamics, Second Institute of Oceanography (QNHX1803) to YF, and US National Science Foundation grants (OCE 1638804 and OCE 1851222) to DH.
Conflict of Interest
The authors declare that the research was conducted in the absence of any commercial or financial relationships that could be construed as a potential conflict of interest.
Acknowledgments
We would like to thank undergraduate students Dandan Hou, Meiqi Li, and Wenzhang Feng at Tianjin University of Science and Technology for helping with seawater collection and experimental setup. We also thank Dr. Guisheng Song’s laboratory at Tianjin University for analyzing the dissolved inorganic carbon concentration.
Supplementary Material
The Supplementary Material for this article can be found online at: https://www.frontiersin.org/articles/10.3389/fmars.2021.622319/full#supplementary-material
References
Alldredge, A. L., and Jackson, G. A. (1995). Aggregation in marine systems — preface. Deep Sea Res. II 42, 1–7. doi: 10.1016/s0924-7963(02)00049-0
Armstrong, R. A., Lee, C., Hedges, J. I., Honjo, S., and Wakeham, S. G. (2002). A new mechanistic model for organic carbon fluxes in the ocean based on the quantitative association of POC with ballast minerals. Deep Sea Res. II 49, 219–236. doi: 10.1016/s0967-0645(01)00101-1
Atkinson, D., Ciotti, B. J., and Montagnes, D. J. S. (2003). Protists decrease in size linearly with temperature: CA. 2.5% degrees C-1. Proc. R. Soc. B Biol. Sci. 270, 2605–2611. doi: 10.1098/rspb.2003.2538
Bach, L. T., and Taucher, J. (2019). CO2 effects on diatoms: a synthesis of more than a decade of ocean acidification experiments with natural communities. Ocean Sci. 15, 1159–1175. doi: 10.5194/os-15-1159-2019
Barcelos e Ramos, J., Schulz, K. G., Brownlee, C., Sett, S., and Azevedo, E. B. (2014). Effects of increasing seawater carbon dioxide concentrations on chain formation of the diatom Asterionellopsis glacialis. PLoS One 9:e90749. doi: 10.1371/journal.pone.0090749
Beaufort, L., Probert, I., de Garidel-Thoron, T., Bendif, E. M., Ruiz-Pino, D., Metzl, N., et al. (2011). Sensitivity of coccolithophores to carbonate chemistry and ocean acidification. Nature 476, 80–83. doi: 10.1038/nature10295
Bienfang, P. K. (1981). SETCOL—a technologically simple and reliable method for measuring phytoplankton sinking rates. Can. J. Fishe. Aquat. Sci. 38, 1289–1294. doi: 10.1139/f81-173
Bopp, L., Resplandy, L., Orr, J. C., Doney, S. C., Dunne, J. P., Gehlen, M., et al. (2013). Multiple stressors of ocean ecosystems in the 21st century: projections with CMIP5 models. Biogeosciences 10, 6225–6245. doi: 10.5194/bg-10-6225-2013
Boyd, P. W., Collins, S., Dupont, S., Fabricius, K., Gattuso, J. P., Havenhand, J., et al. (2018). Experimental strategies to assess the biological ramifications of multiple drivers of global ocean change—a review. Glob. Chang. Biol. 24, 2239–2261. doi: 10.1111/gcb.14102
Boyd, P. W., and Hutchins, D. A. (2012). Understanding the responses of ocean biota to a complex matrix of cumulative anthropogenic change. Mar. Ecol. Prog. Ser. 470, 125–135. doi: 10.3354/meps10121
Brzezinski, M. A., and Nelson, D. M. (1995). The annual silica cycle in the Sargasso Sea near Bermuda. Deep Sea Res. Part I Oceanogr. Res. Pap. 42, 1215–1237. doi: 10.1016/0967-0637(95)93592-3
Buesseler, K. O. (1998). The decoupling of production and particulate export in the surface ocean. Glob. Biogeochemi. Cycles 12, 297–310. doi: 10.1029/97gb03366
Buma, A. G., De Baar, H. J., Nolting, R. F., and Van Bennekom, A. J. (1991). Metal enrichment experiments in the Weddell−Scotia Seas: effects of iron and manganese on various plankton communities. Limnol. Oceanogr. 36, 1865–1878. doi: 10.4319/lo.1991.36.8.1865
Burkhardt, S., Amoroso, G., Riebesell, U., and Sultemeyer, D. (2001). CO2 and HCO3– uptake in marine diatoms acclimated to different CO2 concentrations. Limnol. Oceanogr. 46, 1378–1391. doi: 10.4319/lo.2001.46.6.1378
Burkhardt, S., and Riebesell, U. (1997). CO2 availability affects elemental composition (C: N: P) of the marine diatom Skeletonema costatum. Mar. Ecol. Prog. Ser. 155, 67–76. doi: 10.3354/meps155067
Burkhardt, S., Zondervan, I., and Riebesell, U. (1999). Effect of CO2 concentration on C: N: P ratio in marine phytoplankton: a species comparison. Limnol. Oceanogr. 44, 683–690. doi: 10.4319/lo.1999.44.3.0683
Cai, W.-J., Hu, X., Huang, W.-J., Murrell, M. C., Lehrter, J. C., Lohrenz, S. E., et al. (2011). Acidification of subsurface coastal waters enhanced by eutrophication. Nat. Geosci. 4, 766–770. doi: 10.1038/ngeo1297
Caldeira, K., and Wickett, M. E. (2003). Anthropogenic carbon and ocean pH. Nature 425, 365–365. doi: 10.1038/425365a
Carton, J. A., and Giese, B. S. (2008). A reanalysis of ocean climate using simple ocean data assimilation (SODA). Mon. Weather Rev. 136, 2999–3017. doi: 10.1175/2007mwr1978.1
Chen, B., and Laws, E. A. (2017). Is there a difference of temperature sensitivity between marine phytoplankton and heterotrophs? Limnol. Oceanogr. 62, 806–817. doi: 10.1002/lno.10462
Dai, M., Lu, Z., Zhai, W., Chen, B., Cao, Z., Zhou, K., et al. (2009). Diurnal variations of surface seawater pCO2 in contrasting coastal environments. Limnol. Oceanogr. 54, 735–745. doi: 10.4319/lo.2009.54.3.0735
Daufresne, M., Lengfellner, K., and Sommer, U. (2009). Global warming benefits the small in aquatic ecosystems. Proc. Natl. Acad. Sci. U.S.A. 106, 12788–12793. doi: 10.1073/pnas.0902080106
Denny, M. (1993). Air and Water: The Biology and Physics of Life’s Media. Princeton, NJ: Princeton University Press.
Dickson, A. G., and Millero, F. J. (1987). A comparison of the equilibrium-constants for the dissociation of carbonic-acid in seawater media. Deep Sea Res. Part A Oceanogr. Res. Pap. 34, 1733–1743. doi: 10.1016/0198-0149(87)90021-5
Dickson, A. G., Sabine, C. L., and Christian, J. R. (2007). Guide to Best Practices for Ocean CO2 Measurements. Sidney, BC: North Pacific Marine Science Organization.
Engel, A., Schulz, K. G., Riebesell, U., Bellerby, R., Delille, B., and Schartau, M. (2008). Effects of CO2 on particle size distribution and phytoplankton abundance during a mesocosm bloom experiment (PeECE II). Biogeosciences 5, 509–521. doi: 10.5194/bg-5-509-2008
Falkowski, P. G., and Raven, J. A. (2007). Aquatic Photosynthesis. Princeton, NJ: Princeton University Press.
Feely, R. A., Doney, S. C., and Cooley, S. R. (2009). Ocean acidification: present conditions and future changes in a high-CO2 world. Oceanography 22, 36–47. doi: 10.5670/oceanog.2009.95
Feng, L., and Lin, X.-P. (2009). Long-term trend of the east china sea surface temperature during 1945~ 2006. Period. Ocean Univ. China 39, 13–18. (In Chinese with English abstract),Google Scholar
Feng, Y., Hare, C. E., Leblanc, K., Rose, J. M., Zhang, Y. H., Ditullio, G. R., et al. (2009). Effects of increased pCO2 and temperature on the North Atlantic spring bloom. I. The phytoplankton community and biogeochemical response. Mar. Ecol. Prog. Ser. 388, 13–25. doi: 10.3354/meps08133
Feng, Y., Hare, C. E., Rose, J. M., Handy, S. M., Ditullio, G. R., Lee, P. A., et al. (2010). Interactive effects of iron, irradiance and CO2 on Ross Sea phytoplankton. Deep Sea Res. Part I Oceanogr. Res. Pap. 57, 368–383. doi: 10.1016/j.dsr.2009.10.013
Feng, Y., Roleda, M. Y., Armstrong, E., Summerfield, T. A., Law, C. S., Hurd, C. L., et al. (2020). Effects of multiple drivers of ocean global change on the physiology and functional gene expression of the coccolithophore Emiliania huxleyi. Glob. Chang. Biol. 26, 5630–5645. doi: 10.1111/gcb.15259
Feng, Y., Warner, M. E., Zhang, Y., Sun, J., Fu, F. X., Rose, J. M., et al. (2008). Interactive effects of increased pCO2, temperature and irradiance on the marine coccolithophore Emiliania huxleyi (Prymnesiophyceae). Eur. J. Phycol. 43, 87–98. doi: 10.1080/09670260701664674
Finkel, Z. V., Beardall, J., Flynn, K. J., Quigg, A., Rees, T. A. V., and Raven, J. A. (2010). Phytoplankton in a changing world: cell size and elemental stoichiometry. J. Plankton Res. 32, 119–137. doi: 10.1093/plankt/fbp098
Fitzer, S. C., Torres Gabarda, S., Daly, L., Hughes, B., Dove, M., O’connor, W., et al. (2018). Coastal acidification impacts on shell mineral structure of bivalve mollusks. Ecol. Evol. 8, 8973–8984. doi: 10.1002/ece3.4416
Folt, C. L., Chen, C. Y., Moore, M. V., and Burnaford, J. (1999). Synergism and antagonism among multiple stressors. Limnol. Oceanogr. 44, 864–877. doi: 10.4319/lo.1999.44.3_part_2.0864
Fu, F. X., Warner, M. E., Zhang, Y. H., Feng, Y. Y., and Hutchins, D. A. (2007). Effects of increased temperature and CO2 on photosynthesis, growth, and elemental ratios in marine Synechococcus and Prochlorococcus (Cyanobacteria). J. Phycol. 43, 485–496. doi: 10.1111/j.1529-8817.2007.00355.x
Gao, K., and Campbell, D. A. (2014). Photophysiological responses of marine diatoms to elevated CO2 and decreased pH: a review. Funct. Plant Biol. 41, 449–459. doi: 10.1071/fp13247
Gao, K., Xu, J., Gao, G., Li, Y., Hutchins, D. A., Huang, B., et al. (2012). Rising CO2 and increased light exposure synergistically reduce marine primary productivity. Nat. Clim. Chang. 2, 519–523. doi: 10.1038/nclimate1507
Hamm, C. E., Merkel, R., Springer, O., Jurkojc, P., Maier, C., Prechtel, K., et al. (2003). Architecture and material properties of diatom shells provide effective mechanical protection. Nature 421, 841–843. doi: 10.1038/nature01416
Hare, C. E., DiTullio, G. R., Riseman, S. F., Crossley, A. C., Popels, L. C., Sedwick, P. N., et al. (2007a). Effects of changing continuous iron input rates on a Southern Ocean algal assemblage. Deep Sea Res. I 54, 732–746. doi: 10.1016/j.dsr.2007.02.001
Hare, C. E., DiTullio, G. R., Trick, C. G., Wilhelm, S. W., Bruland, K. W., Rue, E. L., et al. (2005). Phytoplankton community structure changes following simulated upwelled iron inputs in the Peru Upwelling region. Aquat. Microb. Ecol. 38, 269–282. doi: 10.3354/ame038269
Hare, C. E., Leblanc, K., Ditullio, G. R., Kudela, R. M., Zhang, Y., Lee, P. A., et al. (2007b). Consequences of increased temperature and CO2 for phytoplankton community structure in the Bering Sea. Mar. Ecol. Prog. Ser. 352, 9–16. doi: 10.3354/meps07182
Hutchins, D. A., and Fu, F. X. (2017). Microorganisms and ocean global change. Nat. Microbiol. 2:17508. doi: 10.1038/nmicrobiol.2017.58
Hutchins, D. A., Fu, F. X., Warner, M. E., Feng, Y., Portune, K., Bernhardt, P. W., et al. (2007). CO2 control of Trichodesmium N2 fixation, photosynthesis, growth rates, and elemental ratios: implications for past, present, and future ocean biogeochemistry. Limnol. Oceanogr. 52, 1293–1340. doi: 10.4319/lo.2007.52.4.1293
Hutchins, D. A., Mulholland, M. R., and Fu, F. X. (2009). Nutrient cycles and marine microbes in a CO2-enriched ocean. Oceanography 22, 128–145. doi: 10.5670/oceanog.2009.103
Hutchins, D. A., Pustizzi, F., Hare, C. E., and Ditullio, G. R. (2003). A shipboard natural community continuous culture system for ecologically relevant low-level nutrient enrichment experiments. Limnol. Oceanogr. Methods 1, 82–91. doi: 10.4319/lom.2011.1.82
IPCC (2014). Climate Change 2014: Synthesis Report. Contribution of Working Groups I, II and II to the FIfth Assessment Report of the Intergovernmental Panel on Climate Change. Geneva: IPCC.
Joint, I., Doney, S. C., and Karl, D. M. (2011). Will ocean acidification affect marine microbes? ISME J. 5, 1–7. doi: 10.1038/ismej.2010.79
Kim, J.-M., Lee, K., Shin, K., Kang, J.-H., Lee, H.-W., Kim, M., et al. (2006). The effect of seawater CO2 concentration on growth of a natural phytoplankton assemblage in a controlled mesocosm experiment. Limnol. Oceanogr. 51, 1629–1636. doi: 10.4319/lo.2006.51.4.1629
Kling, J. D., Lee, M. D., Fu, F.-X., Phan, M. D., Wang, X., Qu, P. P., et al. (2020). Transient exposure to novel high temperatures reshapes coastal phytoplankton communities. ISME J. 14, 413–424. doi: 10.1038/s41396-019-0525-6
Krumhardt, K. M. L., Lovenduskib, N. S., Iglesias-Rodriguezc, M. D., and Kleypasd, J. A. (2017). Coccolithophore growth and calcification in a changing ocean. Prog. Oceanogr. 159, 276–295. doi: 10.1016/j.pocean.2017.10.007
Le Quéré, C., Andrew, R., Canadell, J. G., Sitch, S., Korsbakken, J. I., Peters, G. P., et al. (2016). Global carbon budget 2016. Earth Syst. Sci. Data 8, 605–649.
Lee, K. H., Jeong, H. J., Lee, K., Franks, P. J., Seong, K. A., Lee, S. Y., et al. (2019). Effects of warming and eutrophication on coastal phytoplankton production. Harm. Algae 81, 106–118. doi: 10.1016/j.hal.2018.11.017
Li, F., Wu, Y., Hutchins, D. A., Fu, F., and Gao, K. (2016). Physiological responses of coastal and oceanic diatoms to diurnal fluctuations in seawater carbonate chemistry under two CO2 concentrations. Biogeosciences 13, 6247–6259. doi: 10.5194/bg-13-6247-2016
Liao, Y., Feng, Y., Liu, Y., Li, W., Li, J., Ni, H., et al. (2019). The interative effects of nitrogen limitation and ocean acidification on the physiology of the coccolithophore Emiliania huxleyi NIWA 1108. J. Tianjin Univ. Sci. Technol. 34, 56–62. (In Chinese with English abstract),Google Scholar
Litchman, E., Klausmeier, C. A., Schofield, O. M., and Falkowski, P. G. (2007). The role of functional traits and trade-offs in structuring phytoplankton communities: scaling from cellular to ecosystem level. Ecol. Lett. 10, 1170–1181. doi: 10.1111/j.1461-0248.2007.01117.x
Martin-Jézéquel, V., Hildebrand, M., and Brzezinski, M. A. (2000). Review silicon metabolism in diatoms: implications for growth. J. Phycol. 36, 821–840. doi: 10.1046/j.1529-8817.2000.00019.x
McNair, H. M., Brzezinski, M. A., Till, C. P., and Krause, J. W. (2018). Taxon−specific contributions to silica production in natural diatom assemblages. Limnol. Oceanogr. 63, 1056–1075. doi: 10.1002/lno.10754
Mehrbach, C., Culberson, C. H., Hawley, J. E., and Pytkowic, R. M. (1973). Measurement of apparent dissociation cosntants of carbonic acid in seawater at atmospheric pressure. Limnol. Oceanogr. 18, 897–907. doi: 10.4319/lo.1973.18.6.0897
Milligan, A. J., Varela, D. E., Brzezinski, M. A., and Morel, F. M. (2004). Dynamics of silicon metabolism and silicon isotopic discrimination in a marine diatomas a function of pCO2. Limnol. Oceanogr. 49, 322–329. doi: 10.4319/lo.2004.49.2.0322
Petrou, K., Baker, K. G., Nielsen, D. A., Hancock, A. M., Schulz, K. G., and Davidson, A. T. (2019). Acidification diminishes diatom silica production in the Southern Ocean. Nat. Clim. Chang. 9, 781–786. doi: 10.1038/s41558-019-0557-y
Raven, J. A., Cockell, C. S., and De La Rocha, C. L. (2008). The evolution of inorganic carbon concentrating mechanisms in photosynthesis. Philos. Trans. R. Soc. Lond. B Biol. Sci. 363, 2641–2650. doi: 10.1098/rstb.2008.0020
Raven, J. A., and Crawfurd, K. (2012). Environmental controls on coccolithophore calcification. Mar. Ecol. Prog. Ser. 470, 137–166. doi: 10.3354/meps09993
Riebesell, U., Wolfgladrow, D. A., and Smetacek, V. (1993). Carbon dioxide limitation of marine phytoplankton growth rates. Nature 361, 249–251. doi: 10.1038/361249a0
Riebesell, U., Zondervan, I., Rost, B., Tortell, P. D., Zeebe, R. E., and Morel, F. M. (2000). Reduced calcification of marine plankton in response to increased atmospheric CO2. Nature 407, 364–367. doi: 10.1038/35030078
Rose, J. M., Feng, Y., Ditullio, G. R., Dunbar, R. B., Hare, C. E., Lee, P. A., et al. (2009). Synergistic effects of iron and temperature on Antarctic phytoplankton assemblages. Biogeosciences 6, 3131–3147. doi: 10.5194/bg-6-3131-2009
Rost, B., Riebesell, U., Burkhardt, S., and Sultemeyer, D. (2003). Carbon acquisition of bloom-forming marine phytoplankton. Limnol. Oceanogr. 48, 55–67. doi: 10.4319/lo.2003.48.1.0055
Schulz, K. G., Bach, L. T., Bellerby, R. G. J., Bermúdez, R., Büdenbender, J., Boxhammer, T., et al. (2017). Phytoplankton blooms at increasing levels of atmospheric carbon dioxide: experimental evidence for negative effects on prymnesiophytes and positive on small picoeukaryotes. Front. Mar. Sci. 4:64. doi: 10.3389/fmars.2017.00064
Sett, S., Schulz, K. G., Bach, L. T., and Riebesell, U. (2018). Shift towards larger diatoms in a natural phytoplankton assemblage under combined high-CO2 and warming conditions. J. Plankton Res. 40, 391–406. doi: 10.1093/plankt/fby018
Sheridan, J. A., and Bickford, D. (2011). Shrinking body size as an ecological response to climate change. Nat. Clim. Change 1, 401–406. doi: 10.1038/nclimate1259
Smayda, T. J., and Boleyn, B. J. (1966). Experimental observations on the floatation of marine diatoms. II. Skeletonema costatum and Rhizosolenia setigera. Limnol. Oceanogr. 11, 18–34. doi: 10.4319/lo.1966.11.1.0018
Smayda, T. J., and Reynolds, C. S. (2003). Strategies of marine dinoflagellate survival and some rules of assembly. J. Sea Res. 49, 95–106. doi: 10.1016/s1385-1101(02)00219-8
Solórzano, L., and Sharp, J. H. (1980). Determination of total dissolved phosphorus and particulate phosphorus in natural waters. Limnol. Oceanogr. 25, 756–760.
Sommer, U., Adrian, R., Bauer, B., and Winder, M. (2012). The response of temperate aquatic ecosystems to global warming: novel insights from a multidisciplinary project. Mar. Biol. 159, 2367–2377. doi: 10.1007/s00227-012-2085-4
Sommer, U., Paul, C., and Moustaka-Gouni, M. (2015). Warming and ocean acidification effects on phytoplankton—from species shifts to size shifts within species in a mesocosm experiment. PLoS One 10:e0125239. doi: 10.1371/journal.pone.0125239
Spilling, K., and Lindström, M. (2008). Phytoplankton life cycle transformations lead to species-speci?c effects on sediment processes in the Baltic Sea. Cont. Shelf Res. 28, 2488–2495. doi: 10.1016/j.csr.2008.07.004
Spilling, K., Olli, K., Lehtoranta, J., Kremp, A., Tedesco, L., Tamelander, T., et al. (2018). Shifting diatom—dinoflagellate dominance during spring bloom in the Baltic Sea and its potential effects on biogeochemical cycling. Front. Mar. Sci. 5:327. doi: 10.3389/fmars.2018.00327
Taucher, J., Boxhammer, T., Bach, L. T., Paul, A. J., Schartau, M., Stange, P., et al. (2020). Changing carbon-to-nitrogen ratios of organic-matter export under ocean acidification. Nat. Clim. Change 11, 52–57. doi: 10.1038/s41558-020-00915-5
Tortell, P. D., Payne, C. D., Li, Y. Y., Trimborn, S., Rost, B., Smith, W. O., et al. (2008). CO2 sensitivity of Southern ocean phytoplankton. Geophys. Res. Lett. 35, 1–5.
Tréguer, P., Bowler, C., Moriceau, B., Dutkiewicz, S., Gehlen, M., Aumont, O., et al. (2018). Influence of diatom diversity on the ocean biological carbon pump. Nat. Geosci. 11, 27–37. doi: 10.1038/s41561-017-0028-x
Utermöhl, H. (1958). Zur Vervollkommnung der quantitativen Phytoplankton-Methodik. Mitt. Int. Ver. Theor. Angew. Limnol. 9, 1–38. doi: 10.1080/05384680.1958.11904091
Varela, R., Lima, F. P., Seabra, R., Meneghesso, C., and Gómez-Gesteira, M. (2018). Coastal warming and wind-driven upwelling: a global analysis. Sci. Total Environ. 639, 1501–1511. doi: 10.1016/j.scitotenv.2018.05.273
Vehmaa, A., Kremp, A., Tamminen, T., Hogfors, H., Spilling, K., and Engström-Öst, J. (2011). Copepod reproductive success in spring-bloom communities with modified diatom and dinoflagellate dominance. ICES J. Mar. Sci. 69, 351–357. doi: 10.1093/icesjms/fsr138
Wasmund, N., Kownacka, J., Göbel, J., Jaanus, A., Johansen, M., Jurgensone, I., et al. (2017). The diatom/dinoflagellate index as an indicator of ecosystem changes in the Baltic Sea. 1. Principle and handling instruction. Front. Mar. Sci. 4:22. doi: 10.3389/fmars.2017.00022
Wu, R., Li, C., and Lin, J. (2017). Enhanced winter warming in the Eastern China Coastal Waters and its relationship with ENSO. Atmos. Sci. Lett. 18, 11–18. doi: 10.1002/asl.718
Wu, Y., Campbell, D. A., Irwin, A. J., Suggett, D. J., and Finkel, Z. V. (2014). Ocean acidification enhances the growth rate of larger diatoms. Limnol. Oceanogr. 59, 1027–1034. doi: 10.4319/lo.2014.59.3.1027
Xiao, W., Liu, X., Irwin, A. J., Laws, E. A., Wang, L., Chen, B., et al. (2018). Warming and eutrophication combine to restructure diatoms and dinoflagellates. Water Res. 128, 206–216. doi: 10.1016/j.watres.2017.10.051
Zhai, W., Zhao, H., Zheng, N., and Xu, Y. (2012). Coastal acidification in summer bottom oxygen-depleted waters in northwestern-northern Bohai Sea from june to august in 2011. Chin. Sci. Bull. 57, 1062–1068. doi: 10.1007/s11434-011-4949-2
Zhang, Y., Wang, T., Li, H., Bao, N., Hall-Spencer, J. M., and Gao, K. (2018). Rising levels of temperature and CO2 antagonistically affect phytoplankton primary productivity in the South China Sea. Mar. Environ. Res. 141, 159–166. doi: 10.1016/j.marenvres.2018.08.011
Keywords: ocean acidification, warming, phytoplankton, diatoms, biogenic silica, sinking rate, coastal environment
Citation: Feng Y, Chai F, Wells ML, Liao Y, Li P, Cai T, Zhao T, Fu F and Hutchins DA (2021) The Combined Effects of Increased pCO2 and Warming on a Coastal Phytoplankton Assemblage: From Species Composition to Sinking Rate. Front. Mar. Sci. 8:622319. doi: 10.3389/fmars.2021.622319
Received: 28 October 2020; Accepted: 03 March 2021;
Published: 24 March 2021.
Edited by:
Xianghui Guo, Xiamen University, ChinaReviewed by:
Allanah J. Paul, GEOMAR Helmholtz Centre for Ocean Research Kiel, GermanyGang Li, South China Sea Institute of Oceanology (CAS), China
Kalle Olli, Estonian University of Life Sciences, Estonia
Copyright © 2021 Feng, Chai, Wells, Liao, Li, Cai, Zhao, Fu and Hutchins. This is an open-access article distributed under the terms of the Creative Commons Attribution License (CC BY). The use, distribution or reproduction in other forums is permitted, provided the original author(s) and the copyright owner(s) are credited and that the original publication in this journal is cited, in accordance with accepted academic practice. No use, distribution or reproduction is permitted which does not comply with these terms.
*Correspondence: Yuanyuan Feng, yfeng@tust.edu.cn; yyfengcocco@126.com