- Centro de Investigación en Ecosistemas de la Patagonia (CIEP), COPAS Sur Austral, Universidad de Concepción, Coyhaique, Chile
At the southern tip of South America, evidence of shellfish toxicity has been recorded in the accounts of early explorers and shipwreck survivors since the late 16th Century. Blooms of the toxic dinoflagellate Alexandrium catenella were described in the western Magellan Strait in the early 1970s and have since shown a northward progression through Chilean Patagonia, culminating in a catastrophic toxic event around Chiloé Island in 2016. This shift has taken place through coastal areas of extremely sparse human population density, and anthropogenically driven eutrophication is therefore unlikely to be significantly involved, at least in the south. However, human activities – such as salmon cultivation – may play a role in the intensification of blooms in the more densely populated areas of northern Patagonia. In the fjords and channels of Chilean Patagonia, phytoplankton assemblages are shaped by complex interactions between freshwater (FW) run-off and intrusions of subantarctic surface water (SASW). In the context of blooms of A. catenella, we review the properties of SASW – transformed in coastal waters into modified subantarctic water (MSAW). FW input is characterized by very low concentrations of dissolved inorganic nitrogen (DIN) and phosphorus (DIP), but relatively high concentrations of silicic acid (DSi); DIN and DIP are instead supplied predominantly by SASW which is severely deficient in DSi. These waters therefore show strong vertical gradients in DIN, DIP and DSi, but also potentially in dissolved trace metals and CO2. Large scale shifts in the relative inputs of SASW or FW can modify these vertical gradients, potentially forcing competitive changes in phytoplankton assemblages with latitude, with implications for growth and toxicity of A. catenella and other harmful species. The northward shift of blooms of A. catenella could be associated with anomalies in the Southern Annular Mode (SAM) that modify the influence of MSAW through variations in FW input to coastal waters. The historical presence of blooms in southern Patagonia and Tierra del Fuego, combined with the strongly contrasting conditions with latitude and depth, mean that southern Chile represents an ideal natural laboratory to study climatic and oceanographic influences on dynamics of A. catenella populations.
Historical Background
In their search for a passage to the ocean that they would later name the “Pacific,” Ferdinand Magellan’s ships arrived on the 21st October, 1520 at a cape alongside a promising looking entrance. Here, according to Antonio Pigafetta, who accompanied Magellan, they named this the “Cape of the Eleven Thousand Virgins” (now Cape of the Virgins, or Cabo Vírgenes, Figure 1) before entering the strait (Pigafetta, 1874). After passing through the first and second narrows, the ships entered a wider body of water and rounded a cape before heading north west and naming Cabo Deseado (close to present day Cape Pilar, Figure 1) as they entered the Pacific Ocean on 28th November, 1520 (Pigafetta, 1874).
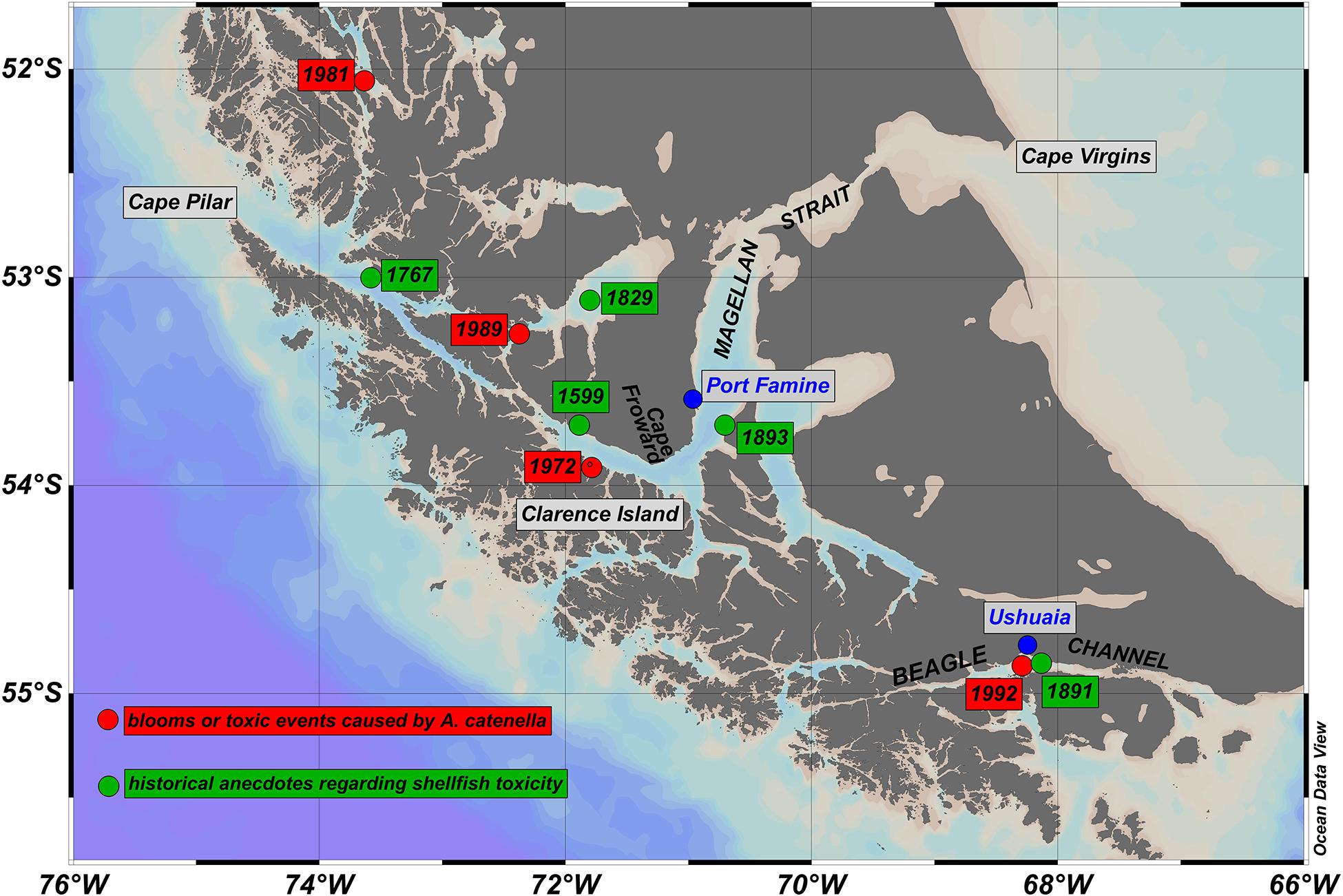
Figure 1. Map of Magallanes and Tierra del Fuego regions of southern Chile and Argentina. The Magellan Strait and Beagle Channel directly connect the southern Pacific and Atlantic Oceans. Green dots show the approximate position of anecdotal reports of shellfish toxicity from pre-1900 historical sources. Accounts are reported (see text for details) for Cordes Bay, 1599 (Kerr, 1824), Cape Providence, 1767 (Hawkesworth, 1773), Englefield Island, 1829 (Fitzroy, 1839), Beagle Channel, 1891 (Segers, 1891), and Fuegian shore opposite Port Famine (O’Sullivan, 1893). Also shown in red are the approximate positions of the focal points of blooms of A. catenella and/or associated events of shellfish poisoning between the early 1970s and early 1990s. Events were centered on Bahia Bell, Clarence Island, 1972 (Guzmán et al., 1975a, b; Guzmán and Lembeye, 1975; Lembeye et al., 1975), Seno Union, 1981 (Lembeye, 1981a, b), Estero Núñez, 1989 (Uribe, 1988; Cited reference is given as 1988, even though the event was given as 1989), Beagle Channel, 1992 (Lembeye, 1992; Carreto and Benavides, 1993; Benavides et al., 1995). In all cases, blooms or shellfish toxicity were spread across a much wider area than indicated by the red dots (see also Figure 2).
At the cape approximately halfway through the strait, Magellan was still some 160 miles from Cabo Deseado, and unaware that Pacific water lay less than 100 feet beneath his ship, overlain by a layer of water derived principally from the surrounding mountains, streams and glaciers. Over 60 years later, this most southerly cape on the Patagonian mainland was named “Cape Froward” (Figure 1) by the English explorer Thomas Cavendish during his 1586–1588 circumnavigation of the globe. In 1587, just north of Cape Froward, Cavendish encountered many corpses in the remains of Rey Don Felipe, a settlement established by Pedro Sarmiento de Gamboa in 1584…
“It seemed unto us, that their whole living for a great space was altogether on muscles [mussels] and limpets; for there was not anything else to be had” (Henry, 1875).
With the population of Rey Don Felipe apparently decimated by starvation, Cavendish renamed the town as Port Famine (Puerto del Hambre, Figure 1). However, the settlement had survived more than two years, and it has also been proposed that the residents may have been poisoned through eating shellfish contaminated by a harmful algal bloom (HAB) (Espinoza and Espinoza, 2010). Although speculative, this suggestion is supported by an account only twelve years later during the voyage of the Dutch explorer Sebald de Weert. In May 1599 in Great Bay1 (Figure 1) to the west of Cape Froward, the following reaction to eating mussels was noted…
‘‘…and afterwards satisfied their hunger with raw muscles and green herbs, which occasioned them to fall into dropsies2 and other lingering sickness, of which several died…” (Kerr, 1824).
In April 1767, during the voyage around the world by Samuel Wallis aboard the Dolphin, similar observations were noted in the vicinity of Cape Providence (Figure 1), further west in the Magellan Strait…
‘‘We continued daily to gather muscles till the 5th, when several of the people being seized with fluxes3, the surgeon desired that no more muscles might be brought into the ship” (Hawkesworth, 1773).
During the first voyage of the Beagle in May 1829 near Englefield Island in Seno Otway (Figure 1), to the west of Cape Froward, Captain Fitzroy’s journal noted…
“One of my boat’s crew was ill this day; the first man that had been seriously so, although several had been slightly affected by the muscles and limpets; and one had fits” (Fitzroy, 1839).
By the late 1800s, the potential toxicity of shellfish in this region was clearly evident in an account of a survivor from a vessel foundered in the middle (Figure 1) of the Strait of Magellan4 …
“The mussels, which covered the rocks in great profusion, were useless for purposes of food: all of our party who partook of them became violently ill with symptoms of irritant poisoning, and quickly developed a crimson, papular rash from head to foot, accompanied by a dreadful thirst and a maddening itching. These very mussels constitute, strange to say, the staple diet of the natives, who consume them in enormous quantities and, apparently, without suffering any ill effects…” (O’Sullivan, 1893, see also, Anonymous, 1893).
Regarding the diet of indigenous people, there seems little available evidence for toxicity in shellfish (Guzmán et al., 2002), but it is noteworthy that in a Yaghan Dictionary – cited in Bruce Chatwin’s famous travelog In Patagonia – a list of synonyms includes…
“Mussels out of season – Shriveled skin – Old age” (Chatwin, 1977).
Indigenous peoples such as the Yaghan might have recognized seasonality in shellfish toxicity and could perhaps mitigate the effects, as has been noted for the Tinglit people of the Pacific coast of North America (Moss, 1993). However, there is evidence in 1891 that indigenous peoples could at times be susceptible to shellfish poisoning further south (Figure 1) in the Beagle Channel (Segers, 1891; Lagos, 1998).
In November 1972, a toxic phytoplankton bloom caused by the dinoflagellate Alexandrium catenella5 (Guzmán et al., 1975a; Guzmán and Lembeye, 1975; Lembeye et al., 1975) occurred in the Magellan Strait, slightly to the west of Cape Froward. Cell abundance and paralytic shellfish poison (PSP) were concentrated around Bahía Bell, Isla Clarence on the north shore of the Tierra del Fuego archipelago (Figure 1), although toxins in shellfish were noted over a much wider area (Guzmán et al., 1975b).
In February 1981, a second toxic event caused by A. catenella was recorded around Seno Unión (Canal Union, Figure 1), north of the Magellan Strait (Lembeye, 1981a, b). Here, abundance was relatively low, but ecdisic cysts of A. catenella were found in the digestive system of shellfish (Lembeye, 1981b) resulting in massive intoxication of the mussel Aulacomya ater over a wide area (Lembeye, 1981a). A further toxic event was recorded in the vicinity of Estero Núñez in April 1989 (Figure 1); again, cysts of A. catenella were detected in the digestive tract of A. ater and PSP toxins were found at lower concentrations across a much wider area (Uribe, 1988). Toxins were also detected in the Magellan Strait and Beagle Channel starting in October and November 1991 (Lembeye, 1992), with a bloom of A. catenella subsequently recorded in January–February 1992 (Figure 1) centered around Ushuaia in the Beagle Channel (Carreto and Benavides, 1993; Benavides et al., 1995). Although toxins initially appeared around the western entrance to the Magellan Strait, the event spread to all southern channels from approximately 49° 20′ S to 54° 55′ S (Lembeye, 1992; Benavides et al., 1995).
Northward Progression of Blooms
Fewer blooms have been reported in Magellan and Fuegian regions since the 1990s, although A. catenella is still present at lower abundances (Guzmán et al., 2013; Pizarro et al., 2011). However, blooms and/or toxic episodes have apparently expanded northward (Table 1 and Figure 2), with A. catenella initially detected in the early 1990s in the Aysén Region (Muñoz et al., 1992; Guzmán et al., 2002), and now routinely detected at lower abundances even in winter (Pizarro et al., 2018). Monitoring programs were initiated in 1995, and blooms expanded northwards from 45°47′ S in 1996 to the Los Lagos region around 42°S at Chiloé in 2002 (Clément et al., 2002; Molinet et al., 2003). Mardones et al. (2010) reported an outbreak in 2009 in the Aysén region that later expanded into the Inland Sea of Chiloé and, for the first time, the oceanic waters off Chiloé. This expansion reached the northern end of the Inland Sea of Chiloé following an exceptional bloom of the Dictyochophyte6 Pseudochattonella verruculosa that killed many farmed salmon through gill tissue damage in February 2016 (Buschmann et al., 2016; Clément et al., 2016; León-Muñoz et al., 2018; Mardones et al., 2021). This event was closely followed in April 2016 by extensive PSP poisoning of marine organisms caused by an exceptional bloom of A. catenella (Buschmann et al., 2016; Hernández et al., 2016). These were the worst mass mortality events caused by phytoplankton in the country’s history, with major impacts on the fishing industry and the economy of the region. Media reports immediately implicated a variety of potential causative factors for the A. catenella bloom such as (1) the dumping 130 km offshore of rotting salmon killed by the P. verruculosa bloom (2) the sharp recent increase in aquaculture activities in the region (3) the coinciding of the bloom with the 2016 El Niño. Although the role of disposed salmon was later dismissed (Buschmann et al., 2016), it has been suggested that this dumping may have been related to a secondary peak in A. catenella (Armijo et al., 2020). The complexity of factors involved mean that we remain unable to rationalize the specific factors behind either this specific catastrophic event, or the general trends of bloom formation and toxicity of A. catenella throughout southern Chile.
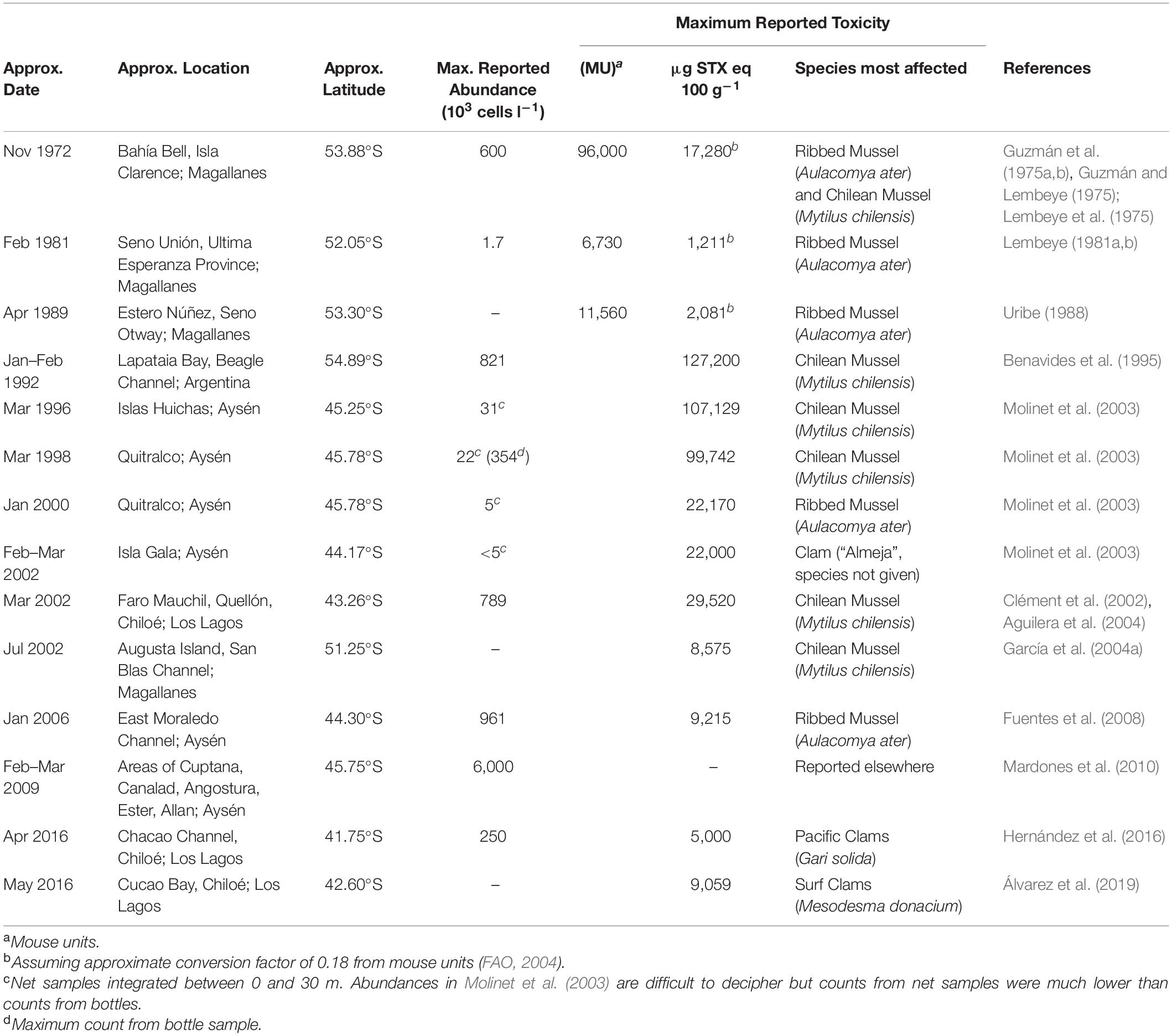
Table 1. Approximate location and timing of the peak of major blooms or toxic events caused by A. catenella in Patagonian and Fuegian coastal waters. Approximate date and position of the focal point of each major event is given, although these blooms and toxic events often extended over much greater areas and periods than indicated below (see individual studies for details).
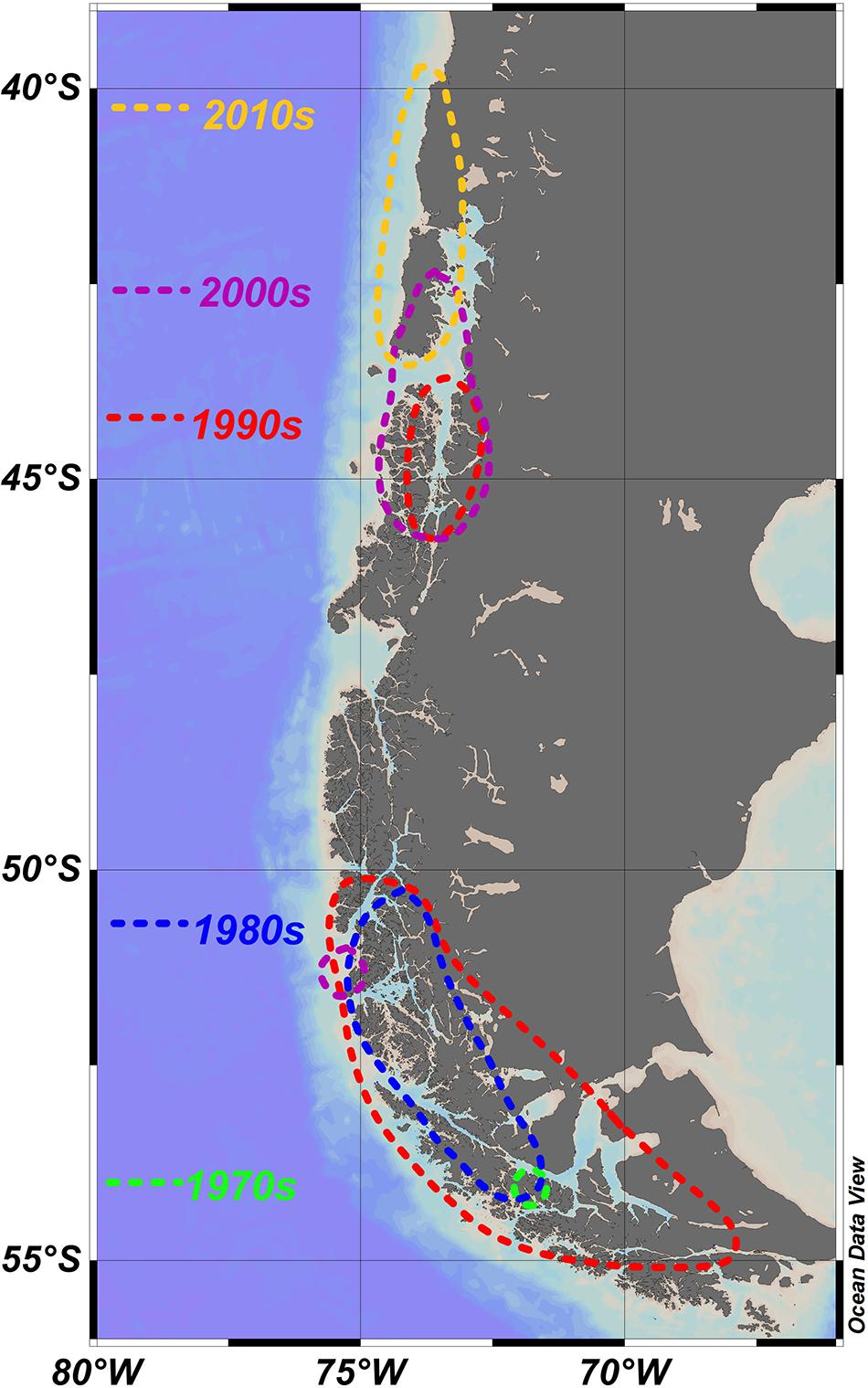
Figure 2. Apparent northward shift of blooms and/or toxic episodes caused by A. catenella; dotted lines show approximate spread of reports for each decade. Focal points of each of the southerly blooms is also shown in Figure 1. Associated details are shown in Table 1. 1970s (Guzmán et al., 1975a, b; Guzmán and Lembeye, 1975; Lembeye et al., 1975). 1980s (Lembeye, 1981a, b; Uribe, 1988). 1990s (Lembeye, 1992; Muñoz et al., 1992; Only mentioned the presence of A. catenella at the mouth of Aysén Fjord: first recording in Aysén region; Carreto and Benavides, 1993; Benavides et al., 1995; Molinet et al., 2003). 2000s (Clément et al., 2002; Molinet et al., 2003; García et al., 2004a; Mardones et al., 2010). 2010s (Hernández et al., 2016).
Blooms of Alexandrium spp. and other HABs appear to be increasing in intensity and proliferating globally, with most research focusing on the role of eutrophication (e.g., Nixon, 1995). However, evidence for the role of increased anthropogenic nutrients in promoting HABs can be equivocal, and site-specific (Davidson et al., 2014). In the fjords and channels of Patagonia, surface freshwater (FW) input is almost devoid of dissolved inorganic nitrogen (DIN) and phosphorus (DIP) but enriched in dissolved Si (DSi) (Silva, 2008), suggesting that anthropogenically driven eutrophication may not be a significant factor, at least in the sparsely populated south. With few large ports, the role of discharge of ships ballast waters (e.g., Hallegraeff, 1998), would also appear to be minimal. However, increased aquaculture activities, particularly in the Los Lagos region, may have had a regional influence on HABs in northern Patagonia. The genetic diversity of populations of A. catenella in southern Chile indicates that populations were not recently established as a result of human introduction (Varela et al., 2012). Recent genetic analyses have confirmed that A. catenella was the species responsible for the 2016 bloom, but that the genetic patterns consistent with range expansion were not evident (Paredes et al., 2019).
Toxic events have focused considerable research effort on A. catenella in southern Chile. This dinoflagellate has a complex life cycle with a biannual occurrence (Molinet et al., 2003), and alternation between vegetative planktonic cells and benthic resting cysts. In Patagonian waters, the role of cyst reservoirs and encystment/excystment cycles can seed recurrence of blooms within a given area (Uribe et al., 2010; Díaz et al., 2014). However, low abundance and fast depletion of cysts within sediments (<3 months, Díaz et al., 2014) has indicated a relatively minor role for cysts in bloom development (Guzmán et al., 2002; Díaz et al., 2014), although high cyst abundance was in fact observed in certain fjords following the 2009 bloom (Mardones et al., 2016a). Major blooms and their geographical expansion in Chilean waters do not appear to be the result of massive in situ gemination (Hernández et al., 2016), but cysts probably do play a more important role than previously thought (Mardones et al., 2016a). Indeed, studies of A. catenella on the eastern coast of North America have suggested a greater conversion of vegetative cells to resting cysts than previously documented and have emphasized the potential role of cyst precursors (planozygotes) in bloom dynamics (Brosnahan et al., 2017).
Oceanic and Climatic Influence?
Temperature is known to play a significant role in blooms of A. catenella (e.g., Fu et al., 2012), with a critical temperature of 13°C apparently required for blooms to develop off northwest North America (Moore et al., 2008). In Chilean Patagonia, the situation appears to be more complex, with no significant relationship noted between temperature and abundance of A. catenella (Molinet et al., 2003), but with elevated temperatures sometimes cited as an important factor in bloom development (Guzmán et al., 2002). However, frequency of A. catenella blooms in the northwestern Chilean Inland Sea was weakly associated with lower temperatures but not with salinity (Díaz et al., 2014). Cultures of A. catenella isolated from Patagonian waters and maintained under controlled conditions have also shown complex relationships between temperature/salinity, growth rate and cell yields (Uribe et al., 2010; Aguilera-Belmonte et al., 2013; Avila et al., 2015). The optimal growth of Chilean strains of A. catenella (i.e., highest growth rate and highest maximal cell density) was reached within a narrow thermal range (12–15°C), while optimal growth salinity (20–30) showed a broader range (Paredes-Mella et al., 2020). Lower temperatures of ∼10°C have been shown to increase the excystment and shorten the dormancy of resting cysts (Mardones et al., 2016a) and to increase the toxin content of A. catenella (Aguilera-Belmonte et al., 2013; Navarro et al., 2006). The response of A. catenella to temperature and salinity changes shows a considerable plasticity that appears to be partially dependent on individual strains (Paredes-Mella et al., 2020).
Despite research on life cycle and physiology, the temporal and spatial variations in abundance and toxicity of A. catenella in southern Chile is not well understood. Given the geographically extensive nature of toxic episodes (Figure 2), and the circumstantial evidence of their long term presence, at least in Magellan and Fuegian regions (Figure 1), it is likely that larger scale influences are involved, as speculated by many authors (Table 2). It is also clear that in the Magellan Strait and Beagle Channel that directly connect Pacific and Atlantic oceans, elevated abundances are more prevalent on the western side, more influenced by Pacific water (Figure 2, see also Lembeye, 1992; Benavides et al., 1995).
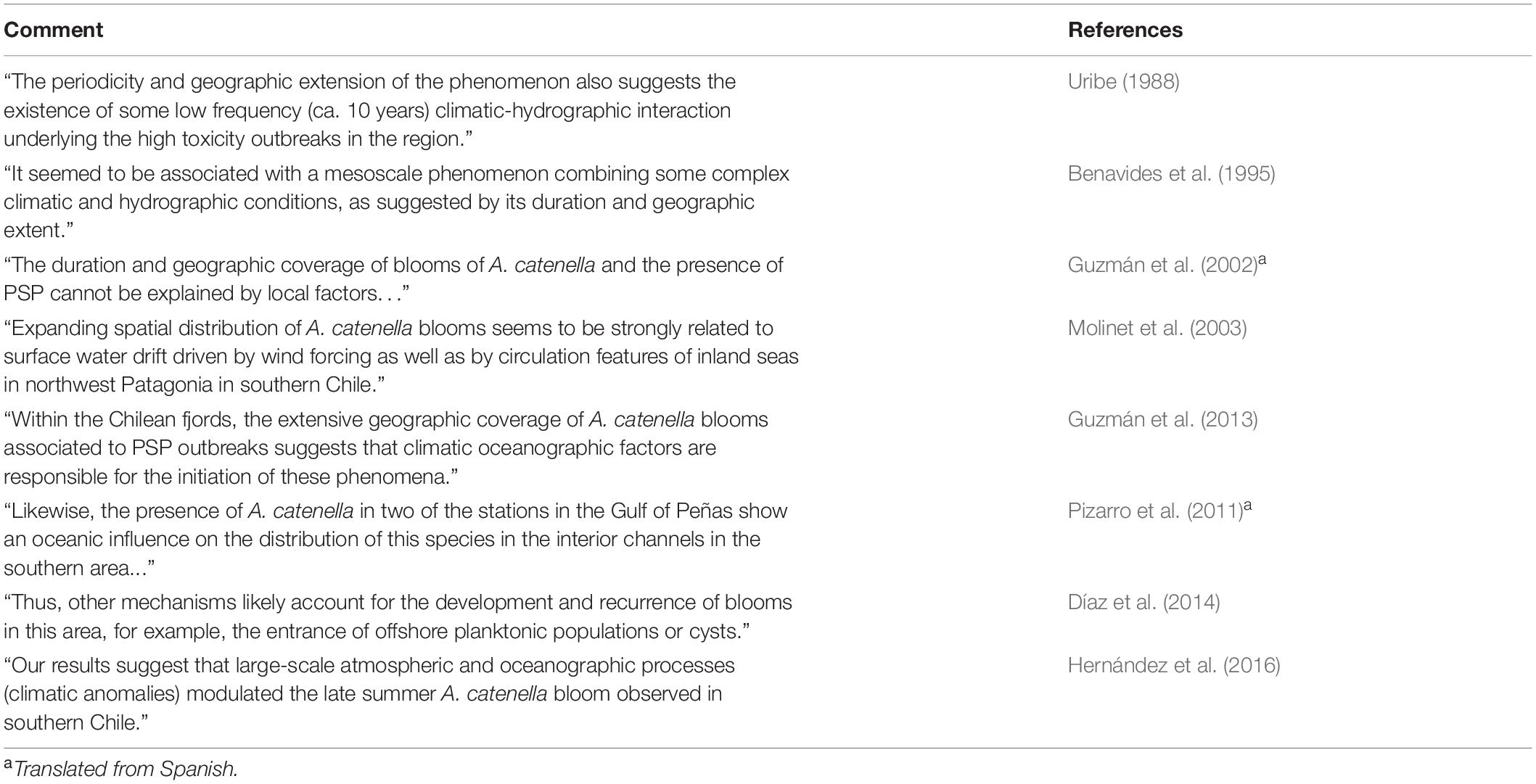
Table 2. Summary of comments regarding potential larger-scale oceanic/climatic influences on blooms of A. catenella in Patagonian and Fuegian regions of southern Chile.
The water column of the fjords and channels of Chilean Patagonia is shaped by complex interactions between FW run-off and intrusions of oceanic water (Figure 3), but few detailed in situ hydrographic data has been published for blooms of A. catenella in these waters, particularly in the vertical dimension. Because of the widely differing properties of continentally derived FW and oceanic waters, caution is required in the interpretation of simple growth rate versus temperature (T) and salinity (S) experiments conducted under controlled conditions. Using raw data from the first two blooms in 1972 and 1981 (Guzmán et al., 1975a; Guzmán and Lembeye, 1975; Lembeye et al., 1975; Lembeye, 1981b), a TS plot suggests that in contrast to the diatom Skeletonema costatum, higher abundances of A. catenella were more closely associated with the low temperature and higher salinity (and density) that characterize subsurface oceanic waters (Figure 4). Moreover, the higher abundances of A. catenella were more associated with depths of 5 m and greater, in contrast to the elevated abundances of S. costatum associated with estuarine water (EW) at depths of 5 m and shallower (Figure 5). Using a larger data set, a similar response has been shown for field populations of A. catenella in the Magellan region (Paredes-Mella et al., 2020). An association with higher temperatures was evident in the Aysén and Los Lagos regions (Paredes-Mella et al., 2020), but it is notable that the elevated abundances of A. catenella were still associated with the temperature and salinity characteristic of oceanic waters at these latitudes (Sievers and Silva, 2008).
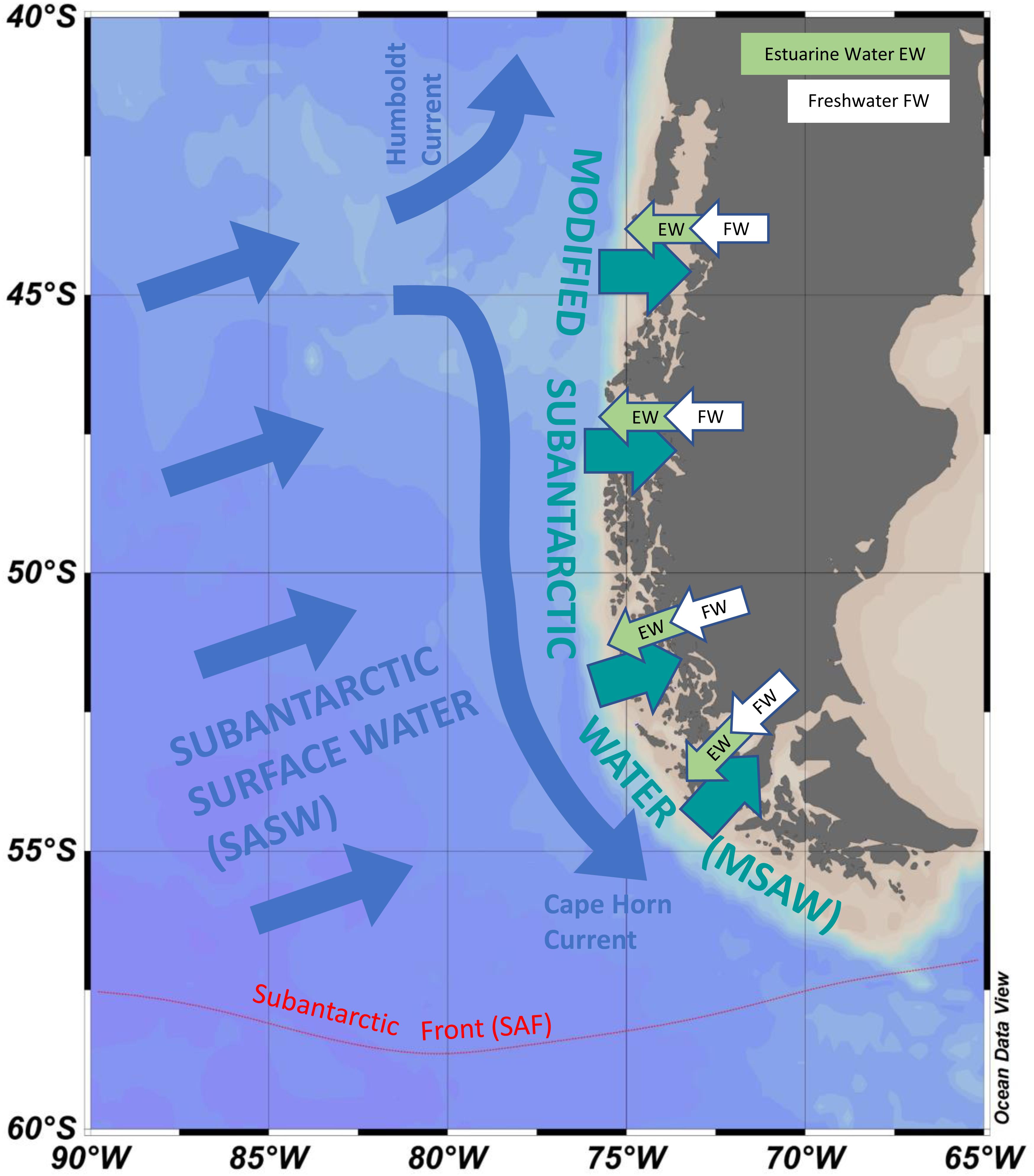
Figure 3. Approximate schematic representation of how the southern Pacific Ocean influences fjords and channels of Patagonia and Tierra del Fuego. Subantarctic surface water (SASW) approaches the coast and interacts with continental run-off to form modified subantarctic water (MSAW) along the coast. MSAW enters the fjords and channels under a surface layer of estuarine water (EW) formed from freshwater flow (FW) derived from rainfall, rivers and glacial melt. Red dotted line gives approximate position of the Subantarctic Front (SAF). Position of currents and water masses are not drawn to scale.
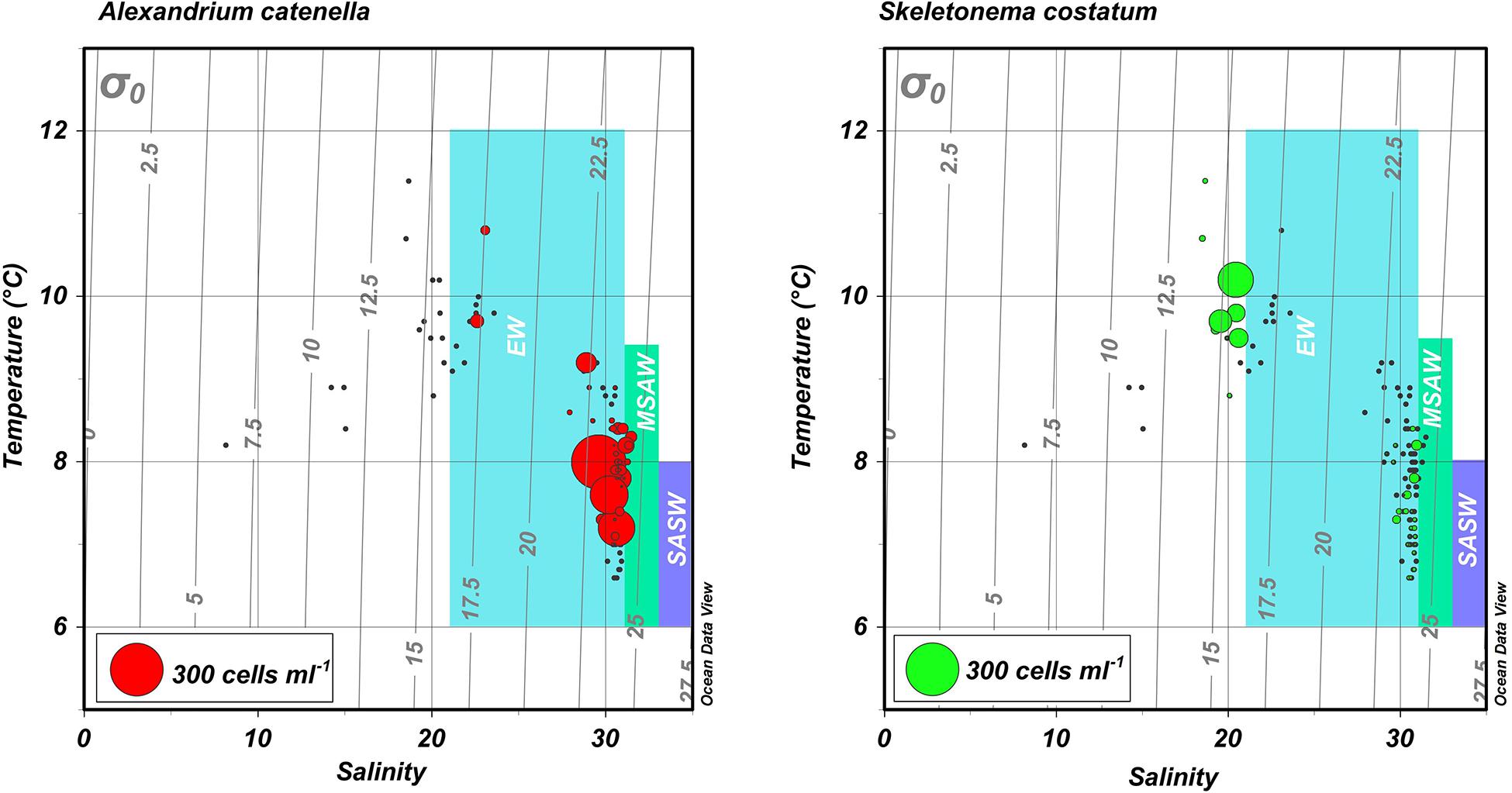
Figure 4. Temperature-salinity (TS) diagrams for blooms of A. catenella in Magallanes and Tierra del Fuego regions in 1972 and 1981. Hydrographic and cell abundance data were taken from the area (Figures 1, 2) centering on Bahia Bell, Clarence Island in 1972 (Guzmán et al., 1975a; Guzmán and Lembeye, 1975; Lembeye et al., 1975) and Seno Union, 1981 (Lembeye, 1981a, b). Circles represent abundance of the dinoflagellate A. catenella (left) and the diatom S. costatum (right). Gray lines represent contours of constant density (σ0). Shaded areas are a schematic representation of the approximate salinity ranges of subantartic surface water (SASW; ∼33–34.6), modified subantarctic water (MSAW; ∼31–33), and estuarine water (EW; ∼21–31) according to Sievers and Silva (2008).
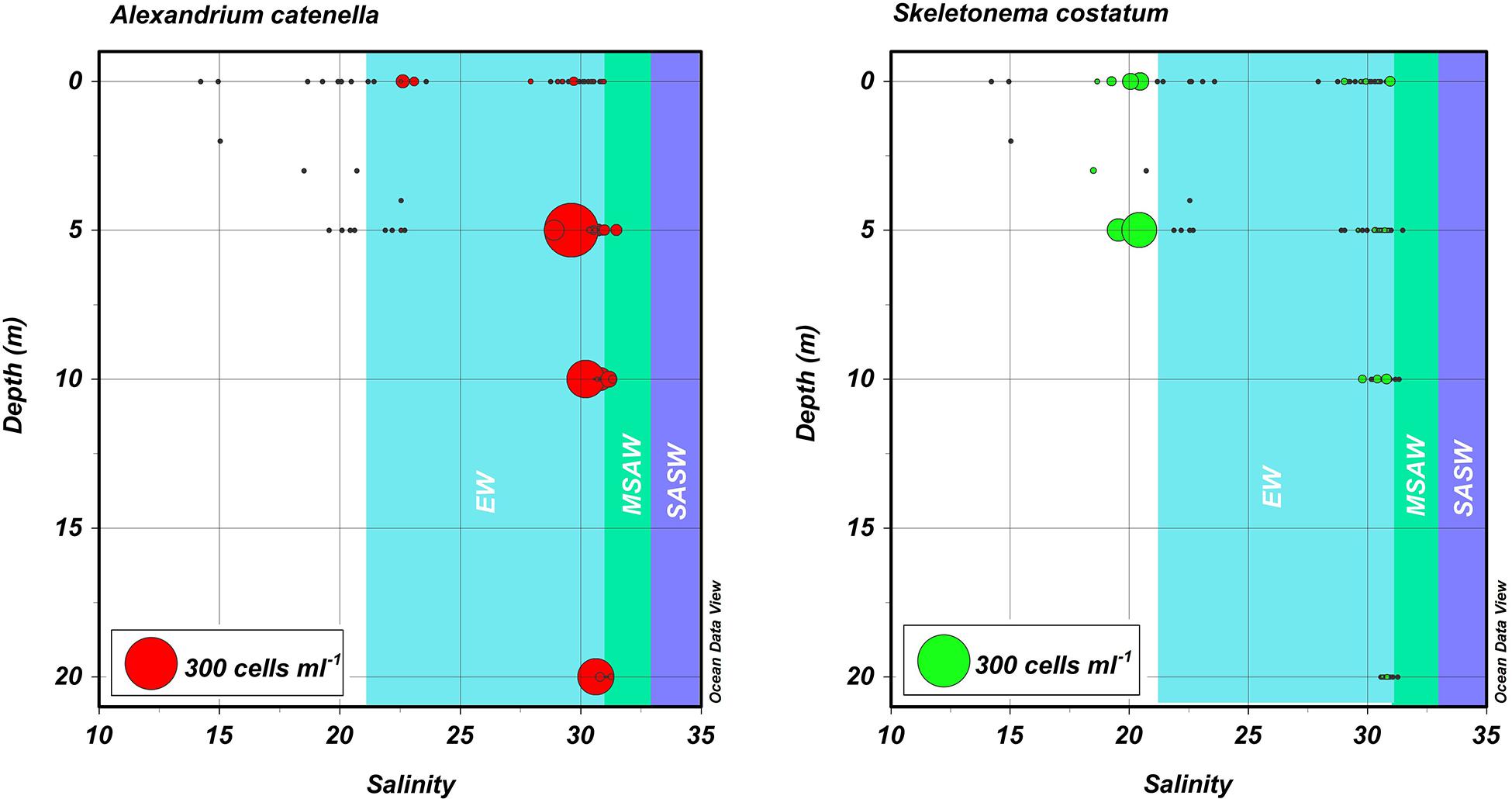
Figure 5. Depth, salinity, abundance plots for blooms of A. catenella in Magallanes and Tierra del Fuego regions in 1972 and 1981. Hydrographic and cell abundance data were taken from the area (Figures 1, 2) centering on Bahia Bell, Clarence Island in 1972 (Guzmán et al., 1975a; Guzmán and Lembeye, 1975; Lembeye et al., 1975) and from Seno Union, 1981 (Lembeye, 1981a, b). Circles represent abundance of the dinoflagellate A. catenella (left) and the diatom S. costatum (right). Shaded areas are a schematic representation of the approximate salinity ranges of subantartic surface water (SASW; ∼33–34.6), modified subantarctic water (MSAW; ∼31–33), and estuarine water (EW; ∼21–31) according to Sievers and Silva (2008).
In the context of blooms of A. catenella, this region presents a paradox: despite extremely poor weather conditions, and almost pristine coastal environments with low human population density, the southern tip of South America suffers from some of the highest levels of natural bioaccumulation of PSP toxins by shellfish in the world (HAEDAT, webpage; Carreto and Benavides, 1993; Benavides et al., 1995; Lagos, 1998). Here we now examine features of Patagonian coastal waters that might be pertinent to blooms of A. catenella, with particular focus on oceanic waters.
Properties of Subantarctic Surface Water
South America is the only land mass to interrupt the subtropical front (STF) (Orsi et al., 1995) that defines the northern extent of subantarctic surface water (SASW). The coast of Chilean Patagonia is therefore in a unique oceanographic position, being strongly influenced by the presence of SASW (Figure 3), which shares properties with subantarctic mode water (SAMW) formed north of the polar frontal zone (Sarmiento et al., 2004; Schneider and Bravo, 2006). SAMW forms at the base of deep winter mixed layers in a circumpolar belt in the Southern Ocean, and is defined by specific oceanographic properties, with density (σt) in the range 26.5–27.1 kg m–3 (Sarmiento et al., 2004). In the south-eastern Pacific off the south-western coast of Patagonia (70–92°W), SAMW forms south of 56°S, varying spatially and seasonally between temperature of ca. 6–8°C, salinity of ca. 34.1–34.4, and σt of 26.5–27.1 (Schneider and Bravo, 2006). Immediately to the north of 56°S, surface waters have a temperature of 7–8°C and salinity of 33.9–34.1 (Schneider and Bravo, 2006), and further north off central Chile, SASW warms to 11.5–12°C and slightly freshens to 33.8–33.9 (Silva et al., 2009) due to continental run-off from northern Patagonia and the associated tongue of lower salinity water extending offshore (Orsi et al., 1995).
Another important characteristic of SAMW is a severe deficiency in silicic acid (Si(OH)4 or DSi) (Sarmiento et al., 2004), a property also notable in SASW. Whereas concentrations of nitrate (NO3–) in SASW vary from ∼3 to ∼20 μM, depending upon latitude and season, concentrations of Si(OH)4 vary from undetectable to only 2–3 μM (Odate and Fukuchi, 1995; Timmermans et al., 1998; Sedwick et al., 1999; Hiscock et al., 2003; Boyd et al., 2008), and are of the order (or less than) of measured half-saturation constants for growth of Antarctic diatoms on Si (e.g., Nelson and Tréguer, 1992). These observed nutrient concentrations lead to a negative Si∗ (Si−NO3) of −10 to −15 μmol kg–1 in SAMW, which are the lowest Si∗ values observed anywhere in the surface of the ocean (Sarmiento et al., 2004). Negative Si∗ results from iron (Fe) limitation in the Southern Ocean, which increases the ratio of biogenic silica to particulate organic nitrogen (Si:N) of diatoms and exported biogenic particles, thus decreasing the dissolved Si(OH)4 to NO3– ratio in the upper water column. Negative Si∗ is an important feature of SAMW, which, as the primary conduit of nutrients for the thermocline throughout the southern hemisphere and North Atlantic (Sarmiento et al., 2004), has a significant impact upon global productivity of diatoms (Sarmiento et al., 2004). Similarly, the strongly negative Si∗ of SASW must in some circumstances constrain the growth of diatoms in the coastal waters of Chilean Patagonia, making this region potentially sensitive to biogeochemical processes occurring in the Southern Ocean. However, modeling suggests little evidence for short term changes in properties of SASW related to recent climate change (e.g., Boyd et al., 2008).
Trace metals have received little attention in terms of their potential role in the dynamics of HABs in this region. As well as having a strongly negative Si∗, SASW is strongly deficient in Fe, with total concentrations of dissolved Fe typically of the order ∼0.03–0.1 nM (Sedwick et al., 1999; Hutchins et al., 2001; Leblanc et al., 2005; Boyd et al., 2008). SASW approaching the coast of Chile is therefore likely to have total Fe concentrations low enough to limit the growth of diatoms and possibly other phytoplankton. A strong linear relationship between DSi and zinc (Zn) has been noted for both the South Atlantic (Wyatt et al., 2014) and the Southern Ocean (Ellwood, 2008; Croot et al., 2011), suggesting that SASW with negative Si∗ could also be strongly deficient in dissolved Zn. Concentrations of total dissolved Zn of 0.2 to 0.3 nM have been measured in SASW both in the Australian region (Ellwood, 2008) and the northern Drake Passage (Croot et al., 2011). In New Zealand subantarctic waters, levels of total dissolved Zn as low as 6 pM have been reported, concentrations which are the lowest documented so far for any open ocean region (Leblanc et al., 2005). Given that Zn availability can significantly alter silicification in diatoms (De La Rocha et al., 2000), the combination of Si, Fe, and Zn deficiency in SASW could have a significant impact on growth of diatoms, and potentially other phytoplankton. Although the evidence for growth limitation of phytoplankton by Zn can be equivocal in some high-nitrate low-chlorophyll (HNLC) ocean areas, Zn could be a potentially limiting micronutrient where SASW meets the more productive coastal waters of Patagonia. Other trace elements could be significant, with the lowest global concentrations of manganese (Mn) also reported in the Drake Passage (Browning et al., 2014).
Within and north of the subantarctic front, SASW is generally relatively rich in dinoflagellates (Kopczynska et al., 2001; Wolf et al., 2014), albeit at much lower abundances than in coastal waters. In contrast, the relative abundance of diatoms increases from the polar frontal zone southwards (Wolf et al., 2014) presumably because of Si limitation north of the polar front. In enrichment studies in subantarctic waters, where Fe was added without Si, growth of dinoflagellates and other flagellated cells were stimulated, whereas growth of diatoms was limited by availability of Si(OH)4 (Coale et al., 2004). In the Drake Passage, A. tamarense has been shown to be present at concentrations of 5–20 cells L–1 north of the polar front, but rarely encountered to the south of the front (Ho et al., 2003); this Antarctic strain of A. tamarense has been isolated and shown to be toxic (Lee et al., 2012). Routine phytoplankton counts may miss dinoflagellates present at these low abundances, which represent only 0.5–2 cells in a 100 ml sedimented sample under an inverted microscope. We have no specific information on the presence of A. catenella itself in SASW, but the concept of offshore low density “seed” populations advected into the coastal region (Hernández et al., 2016) cannot be ruled out.
Continental Freshwater and Modified Subantarctic Water
Continental Patagonia is subject to high levels of precipitation and glacier melt, resulting in an enormous estuarine zone with one of the greatest influences of FW on the planet (Calvete and Sobarzo, 2011). The intricate network of fjords and channels are typically characterized by a two-layer system, where continental run-off delivers DSi into near surface low salinity waters that overly oceanic water richer in DIN and DIP (Sievers and Silva, 2008). Further from shore, physical mixing and high levels of production, sinking and remineralization of biogenic particles produce what is known as modified subantarctic water (MSAW) from SASW. The properties of MSAW are therefore driven by differential inputs of both SASW and FW, with Si∗ more positive with proximity to FW source, and varying with latitude, from positive in the north to negative in southern Patagonia and Fuegian coastal waters (Torres et al., 2014). Average Si(OH)4 concentrations in the surface waters in spring vary from ∼19 μM in northern Patagonian waters down to only ∼2 μM in southern waters (Torres et al., 2014), values similar to those of ∼3 μM at two stations north of the subantarctic front, off the tip of south-western South America (Timmermans et al., 1998). This Si deficit has been proposed as a factor in shaping phytoplankton assemblages in southern waters, where dinoflagellates dominate chlorophyll in NO3– rich (5–10 μM), Si(OH)4 poor (<2 μM) waters of an inner-fjord in the Strait of Magellan (Torres et al., 2011a) and where concentrations of Si are lower than, or similar to, half saturation constants for growth of Antarctic diatoms (Nelson and Tréguer, 1992). In contrast, northern Patagonian coastal waters, having higher concentrations of Si(OH)4, have been characterized by high rates of diatom productivity in austral spring (e.g., Montero et al., 2017). Although Si∗ in MSAW tends to be positive in the north, diatoms acclimated to these high levels of Si could have relatively higher half saturation constants for growth on Si, making them particularly susceptible to limitation during conditions of reduced Si. There is clear potential for the negative Si∗ in southern coastal waters to spread northwards because of spatial and temporal variations in continental run-off (Torres et al., 2014) or from large scale circulation changes that affect the relative input of SASW into MSAW (see later section on Latitudinal Shifts in Water Column Properties). However, to date there has been little specific attention given to the role of such variations of Si∗ in driving competition between toxic dinoflagellates and diatoms in this area. The ratio of concentrations of DIN and DIP have also been shown to be important in driving variations in toxicity of Alexandrium spp. (e.g., Lee et al., 2012), although Si∗ itself should have no direct bearing upon toxicity because N:P ratios are likely to be relatively constant within SASW. However, vertical gradients of DIN and DIP resulting from variations in relative inputs of SASW and freshwater could have some impact on toxicity. Optimum growth of A. catenella in cultures has been shown to occur at unbalanced (elevated) N:P ratios (Paredes-Mella et al., 2020), however, these experiments were conducted by varying P concentrations at very high saturating concentrations of N.
Oceanographic studies clearly focus on NO3– as the principal source of DIN, but any impact of increased relative presence of SASW upon the competition between diatoms and dinoflagellates could be compounded by additional sources of dissolved nitrogen. Preference for ammonium (NH4+) is an important functional trait potentially driving competition within phytoplankton assemblages (Glibert, 2016). NH4+ has been shown to be utilized by another bloom forming dinoflagellate in Patagonian coastal waters (Iriarte et al., 2005), and NH4+, urea and dissolved organic nitrogen (DON) are known to be utilized by some species of Alexandrium (Anderson et al., 2012; Dagenais-Bellefeuille and Morse, 2013). The presence of these alternative forms of dissolved N could drive the “effective” Si∗ to be even more negative, further favoring non-siliceous phytoplankton, of clear significance in areas such as the Inner Sea of Chiloé, where anthropogenic influences and aquaculture activities are higher, and where resulting variations in dissolved N:P ratio could also impact on toxicity of dinoflagellates. In controlled cultures, slightly higher growth rates on reduced forms of N have been shown for Chilean strains of A. catenella (Paredes-Mella et al., 2020). However, these experiments were conducted under highly saturating (>100 μM) concentrations of NO3–, NH4+ and urea that are not representative of Patagonian fjords (e.g., Silva, 2008); the implications of these recent data are therefore yet be resolved.
The exceptionally low concentrations of trace elements in SASW suggest that their availability to phytoplankton will be largely driven by supply from continental run-off into surface coastal waters, and so complex vertical interactions are likely between surface FW and EW, recycling within the water column, and intrusions of SASW and MSAW. Potentially limiting concentrations of Si, Fe, Zn, and other elements could not only force competitive shifts in phytoplankton but could also influence toxicity of dinoflagellates, although few data are presently available. Stratification of trace metal availability in MSAW could favor those taxa with vertical migration behaviors and the ability to feed on other cells. Indeed, mixotrophy is increasingly thought to be an important factor in nutrition of dinoflagellates and potentially in HAB formation (e.g., Jeong et al., 2015; Glibert, 2016). Alexandrium catenella is not only phototrophic but can also feed on Skeletonema (Yoo et al., 2009), a common diatom in Patagonian coastal waters (Montero et al., 2017).
Ocean “acidification” from increasing atmospheric CO2 has been responsible for relatively small changes in pH of surface oceanic waters to date. However, Patagonian coastal waters are not only subject to this acidification, but also to much greater dynamic vertical changes in the carbonate system caused by variability in the interaction between SASW, MSAW and continentally derived FW. The dissolved concentration of free CO2 (or its partial pressure, pCO2) and pH are driven by complex physico-chemical and biological influences on total CO2 (CT) and total alkalinity (AT), with CT in SASW likely to be ∼2.1 mM (e.g., Boyd et al., 2008), but only <0.2 mM in melting glacial freshwater (Vargas et al., 2018). A strong linear relationship is generally observed between AT and salinity in Patagonian waters (Torres et al., 2011b), although this relationship can also be influenced by non-carbonate sources of AT, or variability in the FW end-members (Vargas et al., 2018). The strong vertical gradients in CT, AT, and pCO2 (Torres et al., 2011b) have potential implications for HAB dynamics, with members of the A. tamarense species complex showing increased growth and toxicity under conditions of high pCO2 (Fu et al., 2012; Hattenrath-Lehmann et al., 2015), a response apparently exacerbated by N limitation (Eberlein et al., 2016). Mardones et al. (2016b) have also described morphological variations and non-linear growth responses of A. catenella to pH/pCO2 carbonate changes. Latitudinal gradients in the interaction between FW, SASW and MSAW could have an impact on CO2 induced growth and toxicity of dinoflagellates such as Alexandrium, and further study is clearly required.
Stratification and Vertical Distribution
Despite variable wind forcing, the fjords and channels of Chilean Patagonia are generally subject to weakened vertical mixing resulting both from topographical protection, and, from the interaction between MSAW, EW, and FW. The resulting gradients in temperature, salinity and density have consequences for availability of nutrients and the light field experienced by phytoplankton, although Smayda (2002) has questioned whether the stratification that accompanies flagellate blooms is a significant trigger factor. Nonetheless, stratification of the water column regulates competition between different functional forms of phytoplankton, with vertically migrating red-tide dinoflagellates thriving under conditions of low turbulence (e.g., Margalef, 1978; Glibert, 2016). Turbulence directly inhibits the growth of some dinoflagellates (Berdalet and Estrada, 1993) and is known to reduce growth rate and chain length in cultures of A. catenella (Sullivan et al., 2003). In the field, subsurface populations of A. catenella have been observed to concentrate within a narrow depth interval (∼2 m) where both current shear and turbulence intensity were at a minimum (Sullivan et al., 2003).
In the two-layered system characteristic of Magellan and Fuegian coastal waters, the inflow of MSAW beneath a seaward flow of EW allows motile dinoflagellates such as A. catenella to maintain an optimum position in the water column. Some dense blooms of A. catenella have been noted to occur during periods of increased water column stability (e.g., Guzmán and Lembeye, 1975; Benavides et al., 1995), although it is not clear whether reduced turbulence, improved irradiance, or access to nutrients in a stratified water column were the most significant factors. Few data are available on vertical distribution of A. catenella in Chilean waters, but aggregation of A. catenella cells in the upper water column has been noted during blooms in Aysén (Molinet et al., 2003), and in the Magellan Strait (Figure 5). Strong stratification of PSP toxins (Figure 6) has also been noted in mussels held at fixed depths near Cailín Island, off Chiloé Island (García et al., 2004b), with maxima of toxins occurring at ∼5–7 m depth. This suggests an average position of A. catenella cells approximately at the depth of a weak pycnocline (σt ∼25.20) described in Quellón Bay (Cáceres et al., 2008). However, during a dense bloom in an inlet in the eastern Moraledo Channel, A. catenella aggregated at shallower depths of 1–3 m, in lower salinity water (Fuentes et al., 2008).
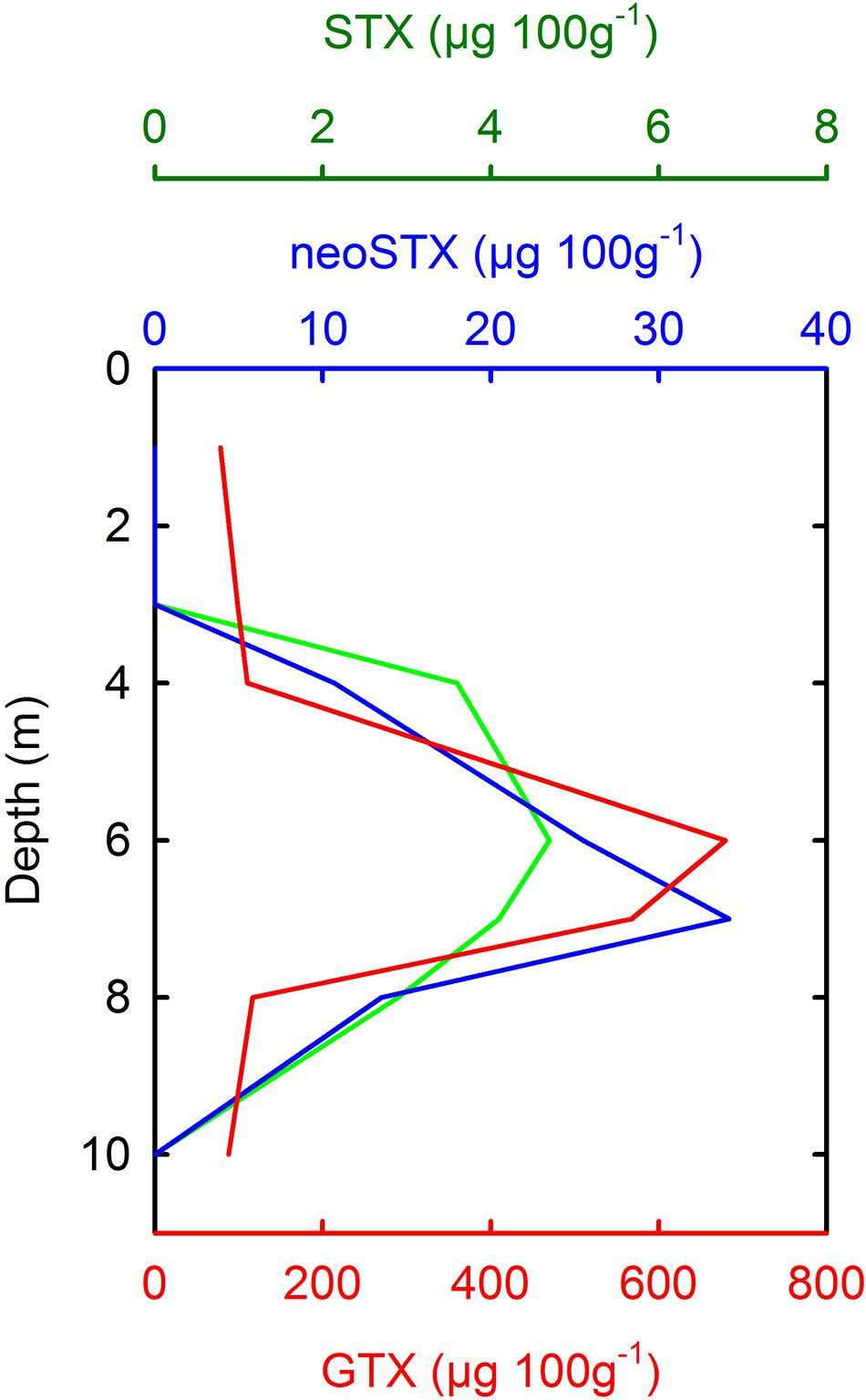
Figure 6. Vertical stratification of PSP toxins in samples of mussel (Mytilus chilensis) tissue from Cailín Island, off Chiloé Island on April 24, 2002 (Data taken from García et al., 2004b). PSP toxins were associated with the presence of A. catenella (García et al., 2004b). M. chilensis were suspended from an aquaculture shellfish lantern at various depths, and the toxin contents therefore reflect the average position and toxicity of A. catenella cells in the water column. GTXs, Gonyautoxins; neoSTX, neoSaxitoxin; STX = Saxitoxin.
These contrasting vertical distributions could be consistent with observations on the east coast of North America that emphasized the combined role of vertical migration patterns and life cycle events in bloom dynamics of A. catenella (Brosnahan et al., 2017). A diel pattern of vertical migration of vegetative cells was observed, with migration to a fixed daytime light intensity (30–40% of maximum surface irradiance) during bloom development (Brosnahan et al., 2017). However, planozygotes (cyst precursors) seemed to abandon this light threshold and aggregate closer to the surface, thus potentially increasing dispersal (Brosnahan et al., 2017). Should this pattern be replicated in Patagonian waters, vegetative cells could be retained by the two-layer flow within fjords and channels, with planozygotes being dispersed by the near surface flow of low salinity EW.
Latitudinal Shifts in Water Column Properties
Clearly some features of Patagonian and Fuegian coastal waters provide favorable conditions for growth or accumulation of A. catenella. Figure 7 provides a generalized schematic overview of the principal properties of the upper water column in these waters, as outlined in previous sections. The figure also indicates how vertical distribution and patterns of vertical migration might influence growth and retention of A. catenella.
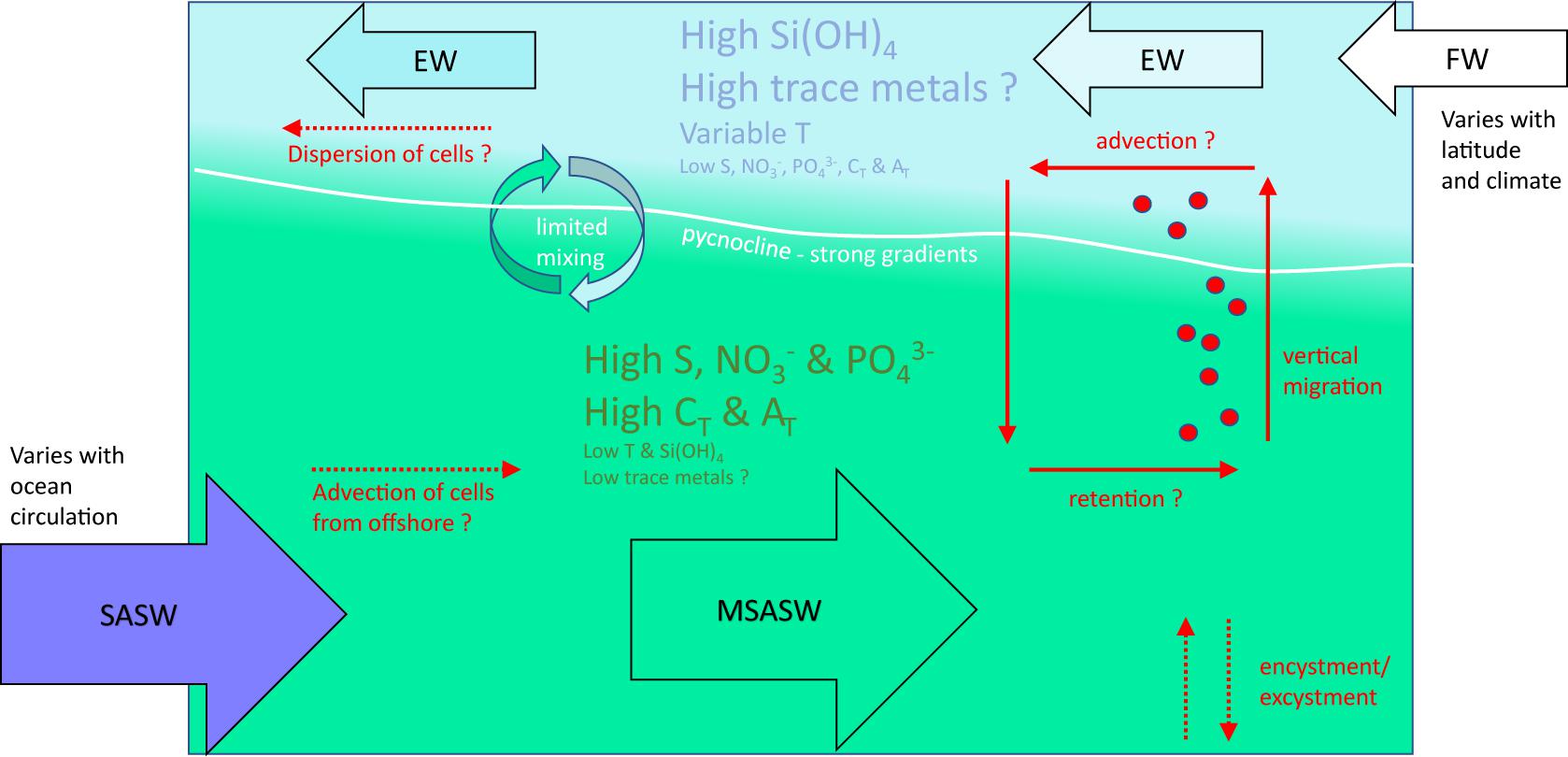
Figure 7. Schematic diagram of the generalized structure of the water column in the fjords and channels of Chilean Patagonia and Tierra del Fuego, indicating the principal processes that potentially influence populations of the dinoflagellate A. catenella. Subantarctic surface water (SASW) flows into coastal waters and forms modified subantarctic water (MSAW) through the interaction with estuarine water (EW). MSAW is overlain by a near-surface layer of EW fed by input of continental freshwater (FW) derived from precipitation, streamflow and glacier melt; the resulting pycnocline reduces mixing between the two layers. Temperature (T) is variable in FW and EW, which are high in silicic acid (Si(OH)4) and potentially in dissolved trace metals (few data available), but low in salinity (S), nitrate and phosphate (NO3– and PO43–), total CO2 (CT) and total alkalinity (AT). SASW and MSAW are high in S, NO3–, PO43–, CT and AT, but low in T, Si(OH)4, and dissolved trace metals. Vertical distribution is not well described for these waters, but A. catenella is thought to aggregate close to the pycnocline where it can optimize vertical position within strong gradients of T, S, irradiance, macro- and micro-nutrients, dissolved CO2, and potentially turbulence. Gradients of pH and pCO2 (not indicated) within the pycnocline – and in the surface layer – will be driven by the strong gradients in CT and AT, and by air-sea exchange of gaseous CO2. Physical retention of A. catenella within certain fjords and channels could be promoted by vertical migration patterns within the two-layered flow. Encystment and excystment processes ensure recurrence of A. catenella under favorable conditions. Advection of A. catenella into coastal waters and dispersal of cells within the upper EW layer are major unknowns in Chilean waters. The above diagram represents a broad schematic description that depends on relative inputs of SASW and FW and associated properties; local patterns vary with ocean circulation, latitude, and climate changes.
The first recorded bloom in 1972 (Guzmán et al., 1975a) – and several of the major blooms that followed – occurred in El Niño years, and it has been suggested that development of A. catenella blooms may be influenced by ENSO variability (Guzmán et al., 2002). The exceptional 2016 bloom also coincided with positive El-Nino and Southern Annular Mode (SAM) anomalies (Hernández et al., 2016; Trainer et al., 2020), although the timing of major events over the past 50 years (from Table 1) has not consistently corresponded with fluctuations in the Niño 3.4 index (Figure 8A; see also Trainer et al., 2020). The raw monthly index for the SAM also suggests no consistent long-term relationship with bloom events (Trainer et al., 2020). However, the major bloom peaks in southern Patagonia and Tierra del Fuego between the 1970s and early 1990s seem to be associated with negative SAM anomalies when using a 12-month trailing mean (Figure 8B). The northward shift of blooms was then gradually associated with positive SAM anomalies (Figure 8B) resulting in a highly significant relationship between SAM index (12-month trailing mean) and approximate latitude (Table 1) of major bloom events (Figure 8C). The relationship was significant using SAM trailing means of 6–12 months (see legend Figure 8C), but not significant using either raw SAM index or a 3-month trailing mean (see legend Figure 8C). With flushing times in some fjords of the order of several months (Mardones et al., 2021), and long timescales involved in development and persistence of A. catenella blooms (Guzmán et al., 2002), a long lag period between climatic effects and bloom events would appear to be realistic.
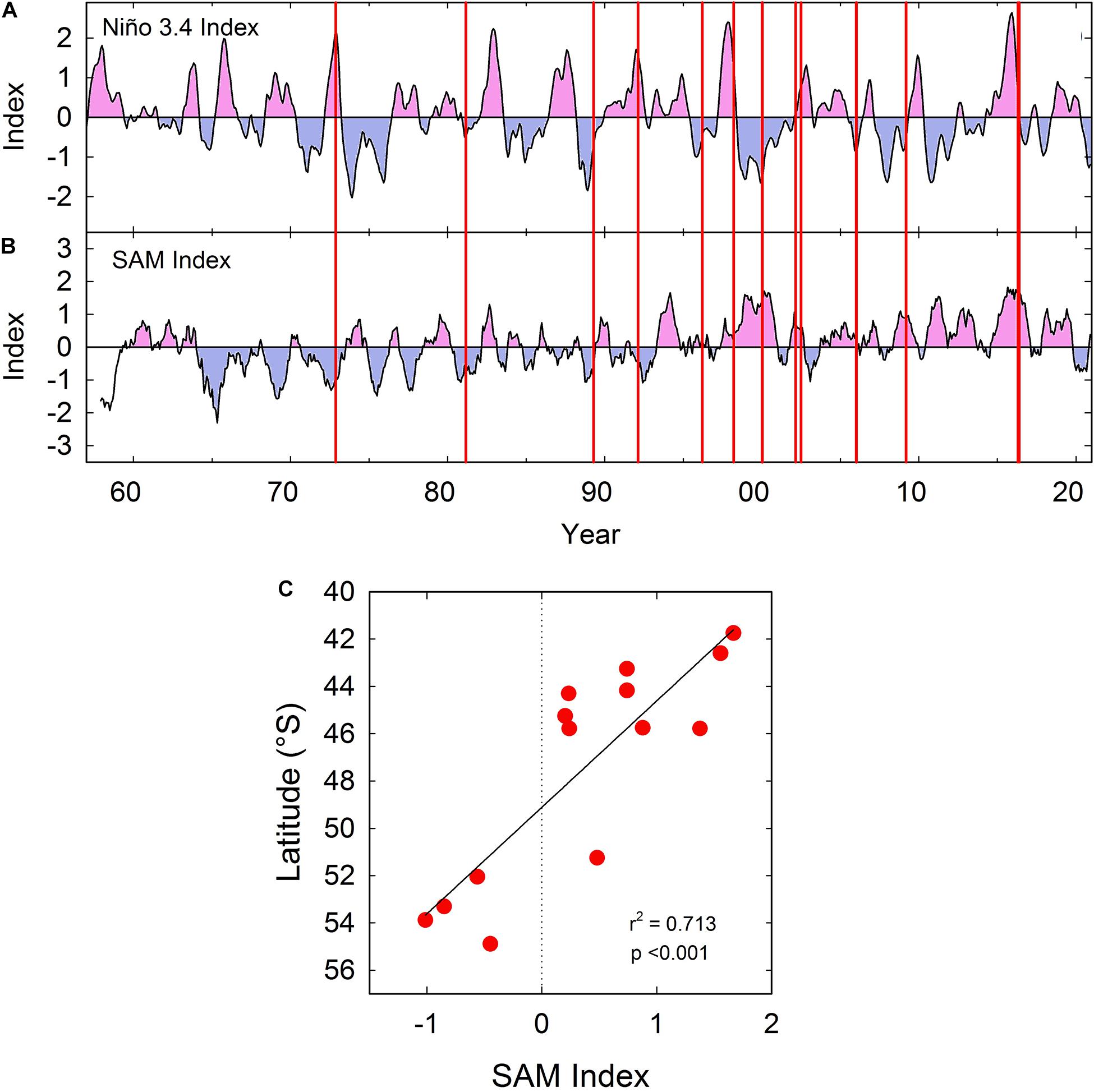
Figure 8. (A) Variation in monthly Niño 3.4 index between 1957 and 2020 (3-month running mean). Data provided by Climate Prediction Center, NOAA (http://www.cpc.ncep.noaa.gov/data/indices/oni.ascii.txt). Positive anomalies shaded in pink, negative shaded in blue. Vertical red lines indicate the approximate time of peak events caused by A. catenella in Patagonian and Fuegian coastal waters (taken from Table 1). (B) Variation in monthly Southern Annular Mode (SAM) index (12-month trailing mean) between 1957 and 2020. Data provided by (http://www.nerc-bas.ac.uk/icd/gjma/sam.html). Vertical red lines as described in (A). (C) Significant relationship between monthly SAM index shown in (B) and latitude of peak events caused by A. catenella (taken from Table 1). Linear regression is also significant with a 9 month (r2 = 0.601, p < 0.01) or a 6 month (r2 = 0.428, p < 0.05) trailing mean for SAM index, but is not significant with a 3-month trailing mean, or with the raw monthly index.
The Southern Annular Mode is an indicator of the relative position of the belt of westerly winds prevailing over southern South America. A positive phase of SAM indicates a southerly shift in westerlies, with negative SAM anomalies associated with a northward shift of the wind belt. The timing and latitude of exceptional A. catenella events suggests a complicated relationship with these SAM anomalies (Figures 8B,C), and the mechanisms involved are as yet unclear. Water column properties (Figure 7) vary with latitude, and with composition of MSAW, which is potentially driven by intrusions of SASW, input of FW, variations in wind mixing and vertical stratification, or combinations of these processes.
Regarding the relative input of SASW, the ocean offshore of northern Patagonia is sensitive to the latitudinal position of the bifurcation of the west wind drift (WWD) which can be a macro scale indicator of oceanographic and meteorological variability (Gatica et al., 2009). There is also evidence for episodic decreases in sea surface temperature in the Inner Sea of Chiloé associated with a shift of sea surface currents to a zonal direction and the entry of large “parcels” of subantarctic water (Giesecke et al., 2014) which have been responsible for mass occurrence of salps, decreases in chlorophyll-a, and changes in phytoplankton community structure (Giesecke et al., 2014). A weakening in the annual cycle of chlorophyll-a concentration and abnormally cold sea surface temperature in the Inner Sea of Chiloé during 2009–2010 has also been linked to transient large-scale climate forcing (Lara et al., 2016). Intrusions of SASW into coastal waters clearly have potential biological implications, but more information is required on any direct role of SASW on dynamics of A. catenella.
Regarding variations in FW input into EW and MSAW, latitudinal gradients in continental precipitation can impact the Si∗ ratio in Patagonian coastal waters (Torres et al., 2014) with consequences for competition between siliceous and non-siliceous phytoplankton (Torres et al., 2011a, 2014). The exceptional A. catenella blooms of 2009 and 2016 were the first to be observed in coastal oceanic waters off Chiloé Island (Mardones et al., 2010; Buschmann et al., 2016) with the latter associated with one the driest Patagonian summers on record (Clément et al., 2016; Hernández et al., 2016; León-Muñoz et al., 2018). The reduced input of FW into coastal waters apparently increased the relative supply of subsurface SASW into near surface waters (Buschmann et al., 2016; León-Muñoz et al., 2018).
The Southern Annular Mode is a weak but significant predictor of Andean streamflow south of 43°S (Masiokas et al., 2019). Over the past few decades, as positive SAM anomalies become more frequent, precipitation (Garreaud et al., 2013) and streamflow (Masiokas et al., 2019) have been significantly lower in north and central Patagonia. In contrast, precipitation and streamflow in recent years have been significantly higher south of 50°S (Garreaud et al., 2013; Masiokas et al., 2019), with dryer conditions more frequent during negative SAM phases in the far south of Patagonia (Weidemann et al., 2018).
The slightly counter-intuitive differential influences of SAM anomalies in the north and south of Patagonia could explain the northward shift in distribution of blooms. The events caused by A. catenella in the 1970s–1990s in southern Patagonia and Tierra del Fuego occurred during significantly drier conditions than the long-term average (Masiokas et al., 2019), whereas the region has experienced significantly wetter conditions in recent years (Masiokas et al., 2019). These observations could help to explain the lower frequency of exceptional blooms in the far south in recent years. North and central Patagonia has seen a significant reduction in streamflow from conditions that were previously wetter (Masiokas et al., 2019), potentially explaining the increased frequency of blooms in recent years.
The changing position of the westerly wind belt seems to play some long-term role in distribution and intensity of blooms, and the influence of these climatic shifts on FW flow into coastal waters appears to be significant. Variations in streamflow with latitude and consequential changes in availability of DSi, DIN and DIP could have been involved in observed shifts in distribution of A. catenella blooms, but other properties of a stratified water column could also be involved, such as trace metals and CO2 (Figure 7). A shallower surface EW layer driven by reductions in FW would also have implications for the vertical distribution of A. catenella regarding optimization of irradiance and availability of DIN and DIP. SAM anomalies not only influence FW input into coastal waters but will also be associated with latitudinal variations in wind forcing and cloud cover; vertical mixing and incident irradiance are both likely drivers of the vertical position of A. catenella in the water column. Further research is clearly required on the detailed vertical dynamics of A. catenella populations and how these are influenced by oceanic and climatic forcing.
Other HAB Species
Because of the records extending over several decades, the present review has focused upon blooms of A. catenella. However, variations in DSi, DIN and other key properties driven by relative inputs of SASW and FW could also impact other HAB species that have been recorded in Chilean coastal waters (e.g., Lagos, 1998; Lembeye, 2008; Díaz et al., 2019).
Several dinoflagellates produce lipophilic toxins, such as Dinophysis spp., responsible for DSP toxins, and Protoceratium reticulatum, which produces yessotoxins (YTX) (Díaz et al., 2019). Blooms of P. reticulatum occur off the northern Chilean coast (Díaz et al., 2019) but also occur in the fjords and channels of southern Chile (Alves-de-Souza et al., 2014, 2019). Thin layers of P. reticulatum and Dinophysis acuminata are present in Reloncaví Fjord (Alves-de-Souza et al., 2014), with blooms apparently linked to shallowing of the salinity driven pycnocline and intrusion of MSAW (Alves-de-Souza et al., 2019) presumably rich in DIN and DIP. The preference of P. reticulatum for low streamflow and positive SAM (Alves-de-Souza et al., 2019) may indicate a similar niche to A. catenella, although blooms of P. reticulatum are not thought to be highly toxic to humans (Díaz et al., 2019).
The catastrophic mass mortality event in northern Patagonia in early 2016 was the result of a convergence of a southerly expansion of P. verruculosa with the northerly expansion of A. catenella (Trainer et al., 2020) that coincided with positive anomalies of both El-Niño and SAM (Trainer et al., 2020). High cell densities of P. verruculosa were noted at relatively high temperatures (>15°C) during previous bloom episodes (Trainer et al., 2020), but in culture, growth rates and maximum cell densities do not vary strongly between temperatures of 12–18°C (Mardones et al., 2021). Cell growth of Chilean P. verruculosa is enhanced under moderate-high salinity conditions (Mardones et al., 2019), and cells aggregate into thin layers within the halocline in Reloncaví Sound (Mardones et al., 2021) with a vertical migration behavior that might allow access to DIN and DIP in MSAW (León-Muñoz et al., 2018) and promote retention in a fjord with low flushing rates (Mardones et al., 2021). The apparent convergence of blooms of P. verruculosa and A. catenella in 2016 from opposite latitudes suggests a possible overlap of differential preferences along the MSAW-EW spectrum.
Dinoflagellates of the genus Karenia are known to produce ichthyotoxins in Chilean waters (Mardones, 2020; Mardones et al., 2020). Extensive blooms of various species of Karenia have been observed in Magellanic fjords (Uribe and Ruiz, 2001), around Chiloé Island (Clément et al., 2001; Mardones, 2020), off the Aysén coast (Villanueva et al., 2017), and off the Chilean coast further north ∼37–44°S (Mardones et al., 2020). In March–April 1999, an extensive bloom of Karenia sp. originated off the coast of Patagonia between 42° and 54°S, with toxicity extending into inshore waters around Chiloé Island (Clément et al., 2001). In the Gulf of Peñas in 2017 (Villanueva et al., 2017), high abundance of several species of Karenia were associated with a frontal zone between MSAW and upwelling of SASW (Villanueva et al., 2017). This association of blooms of Karenia spp. with offshore waters (Villanueva et al., 2017; Mardones, 2020) is supported by culture studies showing optimal growth of K. selliformis at temperatures and salinities between those of MSAW and SASW (Mardones et al., 2020). Extensive blooms of Karenia spp. in inshore waters seem to be associated with periods of low precipitation (Clément et al., 2001), indicating that offshore fronts between MSAW and SASW could shift during climate anomalies thus promoting ichthyotoxin problems closer to shore (Mardones, 2020).
Regarding siliceous species, diatoms of the genus Pseudo-nitzschia have been associated with amnesic shellfish poisoning (ASP) on the northern and southern coasts of Chile (Díaz et al., 2019). Other diatoms such as Leptocylindrus spp. and Chaetoceros spp. cause respiratory dysfunction in fish through mechanical gill damage (Díaz et al., 2019; Mardones, 2020). An interesting corollary to the apparent increases in non-siliceous HAB blooms would be whether blooms of HAB diatoms decrease in response to reduced availability of DSi under conditions of low FW inputs into MSAW.
The sporadic nature of blooms means that we have insufficient data on the role of large-scale oceanographic processes on growth of HAB species in Patagonian and Fuegian waters, particularly with reference to eco-physiological niches, and how competitive shifts may be driven by changing water column properties.
Implications and Conclusions
The Magellan and Fuegian regions are extremely sparsely populated, and many events of water discoloration and toxicity of shellfish will have gone either unnoticed, or unrecorded. Our historical understanding of the spatial and temporal distribution of these events is therefore limited by the availability of relevant records of indigenous peoples and early explorers. Toxic episodes have been reported in the south over several centuries, but over more recent years, a northward expansion of exceptional A. catenella blooms has occurred. It is not known whether this represents a novel spread or is part of a longer-term cycle in distribution.
We have briefly reviewed evidence for an oceanic influence on these blooms, but there is a sparsity of available associated data on physical and chemical properties of the water column on which to speculate on detailed mechanisms. However, we have highlighted notable properties of SASW that have received little research attention. When interacting with coastal waters, properties of SASW and MSAW can potentially impact on the growth, retention, toxicity and encystment/excystment cycles of dinoflagellates such as A. catenella and other HAB species, and on competitive interactions between species. Variation of input of continental FW into coastal waters seems to be a major factor shaping the influence of MSAW on HAB bloom dynamics. In an enhanced dinoflagellate setting potentially driven by low DSi in SASW and MSAW, other factors could also be significant in bloom dynamics, such as dissolved trace metals and CO2, and allelopathic interactions between species (e.g., Granéli et al., 2008).
Larger scale studies are required that extend from the fjords into the ocean, employing high resolution vertical and horizontal sampling of water column properties and life cycle stages of A. catenella. Elucidation of the factors involved with triggering blooms may depend on our ability to conduct studies at temporal and spatial scales appropriate for examining the interactions between FW and oceanic waters in these complex coastal areas. Chilean Patagonia represents an expansive natural mesocosm where blooms of A. catenella occur over an extensive latitudinal range encompassing contrasting anthropogenic influences. Even without a significant role for eutrophication, these blooms could nevertheless be influenced by large scale anthropogenically driven ocean/atmosphere interactions.
Author Contributions
DC wrote the text and prepared figures. PM and GD provided reviews of available information from the literature in Chile. All authors contributed to the article and approved the submitted version.
Funding
This work was funded in part by Programa Regional CONICYT R17A10002, and COPAS Sur-Austral ANID PIA APOYO CCTE AFB 170006.
Conflict of Interest
The authors declare that the research was conducted in the absence of any commercial or financial relationships that could be construed as a potential conflict of interest.
Acknowledgments
We greatly appreciate comments from referees on an earlier draft of the manuscript. We thank Nancy Hamilton for helpful discussions on historical sources. Data and map plotting were conducted using Ocean Data View (ODV) software.
Footnotes
- ^ Or Green Bay, which the Dutch named Bay of de Cordes (Kerr, 1824), or Cordes Bay.
- ^ Dropsy is also now known as oedema, or edema. Victims of PSP poisoning also exhibited symptoms of edema off Augusta Island, western Patagonia (García et al., 2004a) and in the Magallanes region (Montebruno, 1993).
- ^ Not clear whether “seized” refers to PSP poisoning, but “fluxes” could indicate diarrhetic shellfish poisoning (DSP). It is however worth noting here that PSP and DSP toxins can co-occur in Patagonian coastal waters (García et al., 2004b).
- ^ The survivor’s encampment was 12 miles directly across the strait from Port Famine (O’Sullivan, 1893).
- ^ For simplicity we have retained the Alexandrium catenella name commonly used in reports in this region, but some earlier reports used the name Gonyaulax catenella. We acknowledge that there has been considerable recent taxonomic debate regarding A. catenella in particular (John et al., 2014a; Fraga et al., 2015) and the Alexandrium tamarense species complex generally (John et al., 2014b).
- ^ Pseudochattonella verruculosa was formerly classified in raphidophyceae but has now been ascribed to the Dictyochophycean class (Eckford-Soper and Daugbjerg, 2016). Identification of Chilean P. verruculosa was confirmed using the large subunit (LSU) of the nuclear ribosomal RNA gene (Mardones et al., 2019).
References
Aguilera, A., Arriagada, G., Caniggia, M., Clément, A., Contreras, V., Córdova, M., et al. (2004). “Distribution of toxic algae blooms of Alexandrium catenella and their impact upon the Chiloé Archipelago,” in GeoHab, Global Ecology and Oceanography of Harmful Algal Blooms. Open Science Meeting, Viña del Mar, Chile, April 2004, Chile, 24.
Aguilera-Belmonte, A., Inostroza, I., Sáez Carrillo, K., Franco, J. M., Riobó, P., and Gómez, P. I. (2013). The combined effect of salinity and temperature on the growth and toxin content of four Chilean strains of Alexandrium catenella (Whedon and Kofoid) Balech 1985 (Dinophyceae) isolated from an outbreak occurring in southern Chile in 2009. Harmful Algae 23, 55–59. doi: 10.1016/j.hal.2012.12.006
Álvarez, A., Díaz, P. A., Godoy, M., Araya, M., Ganuza, I., Pino, R., et al. (2019). Paralytic shellfish toxins in Surf Clams Mesodesma donacium during a large bloom of Alexandrium catenella dinoflagellates associated to an intense shellfish mass mortality. Toxins 11:188. doi: 10.3390/toxins11040188
Alves-de-Souza, C., Varela, D., Contreras, C., de La Iglesia, P., Fernández, P., Hipp, B., et al. (2014). Seasonal variability of Dinophysis spp. and Protoceratium reticulatum associated to lipophilic shellfish toxins in a strongly stratified Chilean fjord. Deep Sea Res. II 101, 152–162. doi: 10.1016/j.dsr2.2013.01.014
Alves-de-Souza, C., Iriarte, J. L., and Mardones, J. I. (2019). Interannual variability of Dinophysis acuminata and Protoceratium reticulatum in a Chilean Fjord: insights from the realized niche analysis. Toxins 11:19. doi: 10.3390/toxins11010019
Anderson, D. M., Alpermann, T. J., Cembella, A. D., Collos, Y., Masseret, E., and Montresor, M. (2012). The globally distributed genus Alexandrium: multifaceted roles in marine ecosystems and impacts on human health. Harmful Algae 14, 10–35. doi: 10.1016/j.hal.2011.10.012
Anonymous (1893). Pater’s Chats With the Boys. Tierra del Fuego. Magellan Strait: Otago Witness (NZ).
Armijo, J., Oerder, V., Auger, P.-A., Bravo, A., and Molina, E. (2020). The 2016 red tide crisis in southern Chile: possible influence of the mass oceanic dumping of dead salmons. Mar. Pollut. Bull. 150:110603. doi: 10.1016/j.marpolbul.2019.110603
Avila, M., De Zarate, C., Clément, A., Carbonell, P., and Pérez, F. (2015). Efecto de factores abióticos en el crecimiento vegetative de Alexandrium catenella proveniente de quistes en laboratorio. Revista de Biología Marina y Oceanografía 50(S1), 177–185. doi: 10.4067/S0718-19572015000200004
Benavides, H., Prado, L., Díaz, S., and Carreto, J. I. (1995). “An exceptional bloom of Alexandrium catenella in the Beagle Channel, Argentina,” in Harmful Marine Algal Blooms. Proceedings of the Sixth Intemational Conference on Toxic Marine Phytoplankton, October 1993, Nantes, France, eds P. Lassus, G. Arzul, E. Erard, P. Gentien, and C. Marcaillou (Nantes: Lavoisier Publishing and Intercept Ltd), 113–119. doi: 10.13140/2.1.4195.1683
Berdalet, E., and Estrada, M. (1993). “Effects of turbulence on several dinoflagellate species,” in Toxic Phytoplankton in the Sea, eds T. J. Smayda and Y. Shimizu (Amsterdam: Elsevier), 737–741.
Brosnahan, M. L., Ralston, D. K., Fischer, A. D., Solow, A. R., and Anderson, D. M. (2017). Bloom termination of the toxic dinoflagellate Alexandrium catenella: vertical migration behavior, sediment infiltration, and benthic cyst yield. Limnol. Oceanogr. 62, 2829–2849. doi: 10.1002/lno.10664
Browning, T. J., Bouman, H. A., Henderson, G. M., Mather, T. A., Pyle, D. M., Schlosser, C., et al. (2014). Strong responses of Southern Ocean phytoplankton communities to volcanic ash. Geophys. Res. Lett. 41, 2851–2857. doi: 10.1002/2014GL059364
Boyd, P. W., Doney, S. C., Strzepek, R., Dusenberry, J., Lindsay, K., and Fung, I. (2008). Climate-mediated changes to mixed-layer properties in the Southern Ocean: assessing the phytoplankton response. Biogeosciences 5, 847–864. doi: 10.5194/bg-5-847-2008
Buschmann, A., Farías, F., Tapia, F., Varela, D., and Vásquez, M. (2016). Comisión Marea Roja: Informe Final. Osorno: Universidad de Los Lagos, 2016.
Cáceres, M., Valle-Levinson, A., and Bello, M. (2008). Residual flow over a bump in Quellón Bay. Revista de Biología Marina y Oceanografía 43, 629–639. doi: 10.4067/S0718-19572008000300022
Calvete, C., and Sobarzo, M. (2011). Quantification of the surface brackish water layer and frontal zones in southern Chilean fjords between Boca del Guafo (43° 30’S) and Estero Elefantes (46° 30’S). Continental Shelf Res. 31, 162–171. doi: 10.1016/j.csr.2010.09.013
Carreto, J. I., and Benavides, H. R. (1993). World record of PSP in Southern Argentina. Harmful Algae News 5:2.
Clément, A., Seguel, M., Arzul, G., Guzmán, L., and Alarcón, C. (2001). “Widespread outbreak of hemolytic, ichthyotoxic Gymnodinium sp. in southern Chile,” in Harmful Algal Blooms 2000, eds G. M. Hallegraeff and S. I. Blackburn, C. J. Bolch, and R. J. Lewis (Paris: Intergovernmental Oceanographic Commission of UNESCO), 66–69.
Clément, A., Aguilera, A., and Fuentes, C. (2002). “Análisis de marea roja en archipiélago de Chiloé, contingencia verano 2002,” in XXII Congreso de Ciencias del Mar. Resumenes, (Valdivia), 124.
Clément, A., Lincoqueo, L., Saldivia, M., Brito, C. G., Muñoz, F., Fernández, C., et al. (2016). Exceptional summer conditions and HABs of Pseudochattonella in southern Chile create record impacts on salmon farms. Harmful Algae News 53, 1–3.
Coale, K. H., Johnson, K. S., Chavez, F. P., Buesseler, K. O., Barber, R. T., Brzezinski, M. A., et al. (2004). Southern Ocean iron enrichment experiment: carbon cycling in high- and low-Si waters. Science 304, 408–414. doi: 10.1126/science.1089778
Croot, P. L., Baars, O., and Streu, P. (2011). The distribution of dissolved zinc in the Atlantic sector of the Southern Ocean. Deep Sea Res. II 58, 2707–2719. doi: 10.1016/j.dsr2.2010.10.041
Dagenais-Bellefeuille, S., and Morse, D. (2013). Putting the N in dinoflagellates. Front. Microbiol. 4:369. doi: 10.3389/fmicb.2013.00369
Davidson, K., Gowen, R. J., Harrison, P. J., Fleming, L. E., Hoagland, P., and Moschonas, G. (2014). Anthropogenic nutrients and harmful algae in coastal waters. J. Environ. Manage. 146, 206–216. doi: 10.1016/j.jenvman.2014.07.002
De La Rocha, C. L., Hutchins, D. A., Brzezinski, M. A., and Zhang, Y. (2000). Effects of iron and zinc deficiency on elemental composition and silica production by diatoms. Mar. Ecol. Prog. Ser. 195, 71–79.
Díaz, P. A., Molinet, C., Seguel, M., Díaz, M., Labra, G., and Figueroa, R. I. (2014). Coupling planktonic and benthic shifts during a bloom of Alexandrium catenella in southern Chile: implications for bloom dynamics and recurrence. Harmful Algae 40, 9–22. doi: 10.1016/j.hal.2014.10.001
Díaz, P. A., Álvarez, G., Varela, D., Pérez-Santos, I., Díaz, M., Molinet, C., et al. (2019). Impacts of harmful algal blooms on the aquaculture industry: Chile as a case study. Perspect. Phycol. 6, 39–50. doi: 10.1127/pip/2019/0081
Eberlein, T., Van de Waal, D. B., Brandenburg, K. M., John, U., Voss, M., Achterberg, E. P., et al. (2016). Interactive effects of ocean acidification and nitrogen limitation on two bloom-forming dinoflagellate species. Mar. Ecol. Prog. Ser. 543, 127–140. doi: 10.3354/meps11568
Eckford-Soper, L., and Daugbjerg, N. (2016). The ichthyotoxic genus Pseudochattonella (Dictyochophyceae): distribution, toxicity, enumeration, ecological impact, succession and life history – a review. Harmful Algae 58, 51–58. doi: 10.1016/j.hal.2016.08.002
Ellwood, M. J. (2008). Wintertime trace metal (Zn, Cu, Ni, Cd, Pb and Co) and nutrient distributions in the Subantarctic Zone between 40–52°S; 155–160°E. Mar. Chem. 112, 107–117. doi: 10.1016/j.marchem.2008.07.008
Espinoza, J. B., and Espinoza, R. (2010). La increíble empresa de Sarmiento de Gamboa y su triste fin: Posibles causas de la tragedia en el Estrecho de Magallanes en el siglo XVI. The possible causes of the tragedy of “Port Famine” in the Strait of Magellan. Revista Médica Chile 138, 1456–1460.
FAO (2004). Marine Biotoxins, FAO Food and Nutrition Paper 80. Rome: Food and Agriculture Organization of the United Nations, 278.
Fitzroy, R. (1839). “Narrative of the surveying voyages of His Majesty’s ships Adventure and Beagle between the years 1826 and 1836,” in Proceedings of the First Expedition, 1826–1830, Vol. 1, (London: Henry Colburn).
Fraga, S., Sampedro, N., Larsen, J., and Moestrup, Ø, and Calado, A. J. (2015). Arguments against the proposal 2302 by John & al. to reject the name Gonyaulax catenella (Alexandrium catenella). Taxon 64, 634–635. doi: 10.12705/643.15
Fu, F. X., Tatters, A. O., and Hutchins, D. A. (2012). Global change and the future of harmful algal blooms in the ocean. Mar. Ecol. Prog. Ser. 470, 207–233. doi: 10.3354/meps10047
Fuentes, C., Clément, A., and Aguilera, A. (2008). “Summer Alexandrium catenella bloom and the impact on fish farming, in the XI Aysén region, Chile,” in Proceedings of the 12th International Conference on Harmful Algae, Copenhagen, Denmark, 4–8 September, 2006, ed. Ø Moestrup (Paris: Intergovernmental Oceanographic Commission of UNESCO), 183–186.
García, C., del Carmen Bravo, M., Lagos, M., and Lagos, N. (2004a). Paralytic shellfish poisoning: post-mortem analysis of tissue and body fluid samples from human victims in the Patagonia fjords. Toxicon 43, 149–158. doi: 10.1016/j.toxicon.2003.11.018
García, C., Mardones, P., Sfeir, A., and Lagos, N. (2004b). Simultaneous presence of paralytic and diarrheic shellfish poisoning toxins in Mytilus chilensis samples collected in the Chiloe Island, Austral Chilean Fjords. Biol. Res. 37, 721–731.
Garreaud, R., Lopez, P., Minvielle, M., and Rojas, M. (2013). Large-scale control on the Patagonian climate. J. Clim. 26, 215–230. doi: 10.1175/JCLI-D-12-00001.1
Gatica, C., Quiñones, R. A., Figueroa, D., Wiff, R., Navarro, E., and Donoso, M. (2009). Asociación entre la Corriente de Deriva de los Vientos del Oeste y la abundancia relativa del pez espada (Xiphias gladius) frente a la costa de Chile. Lat. Am. J. Aquat. Res. 37, 97–105. doi: 10.3856/vol37-issue1-fulltext-8
Giesecke, R., Clément, A., Garcés-Vargas, J., Mardones, J. I., González, H. E., Caputo, L., et al. (2014). Massive salp outbreaks in the inner sea of Chiloé Island (Southern Chile): possible causes and ecological consequences. Lat. Am. J. Aquat. Res. 42, 604–621. doi: 103856/vol42-issue3-fulltext-18
Glibert, P. M. (2016). Margalef revisited: a new phytoplankton mandala incorporating twelve dimensions, including nutritional physiology. Harmful Algae 55, 25–30. doi: 10.1016/j.hal.2016.01.008
Granéli, E., Weberg, M., and Salomon, P. S. (2008). Harmful algal blooms of allelopathic microalgal species: the role of eutrophication. Harmful Algae 8, 94–102. doi: 10.1016/j.hal.2008.08.011
Guzmán, L., Campodonico, I., and Hermosilla, J. (1975a). Estudios sobre un florecimiento tóxico causado por Gonyaulax catenella en Magallanes. I.— Distribución espacial y temporal de G. catenella. Ans. Inst. Pat. VI, 173–183.
Guzmán, L., Campodonico, I., and Antunovic, M. (1975b). Estudios sobre un florecimiento tóxico causado por Gonyaulax catenella en Magallanes. IV.— Distribución y niveles de toxicidad del Veneno Paralítico de los Mariscos (Noviembre de 1972 - Noviembre de 1973). Ans. Inst. Pat. VI, 209–223.
Guzmán, L., and Lembeye, G. (1975). Estudios sobre un florecimiento tóxico causado por Gonyaulax catenella en Magallanes. II. – Algunas condiciones hidrográficas asociadas. Ans. Inst. Pat. VI, 185–195.
Guzmán, L., Pacheco, H., Pizarro, G., and Alarcón, C. (2002). “Alexandrium catenella y veneno paralizante de los mariscos en Chile,” in Floraciones Algales Nocivas En el Cono Sur Americano, eds E. A. Sar, M. E. Ferrario, and B. Reguera (Madrid: Instituto Español de Oceanografía), 237–255.
Guzmán, L., Vivanco, X., Vidal, G., Pizarro, G., Hernández, C., Tocornal, M. A., et al. (2013). “Spatial and temporal variability of Alexandrium catenella and PSP in southern Chile (43°−55° S) (May 2006 – July 2010),” in Proceedings 14th International Conference on Harmful Algae, Hersonissos-Crete (Greece), 69–71.
HAEDAT Harmful Algal Event Database. Available online at: http://haedat.iode.org/
Hallegraeff, G. M. (1998). Transport of toxic dinoflagellates via ships’ ballast water: bioeconomic risk assessment and efficacy of possible ballast water management strategies. Mar. Ecol. Prog. Ser. 168, 297–309.
Hattenrath-Lehmann, T. K., Smith, J. L., Wallace, R. B., Merlo, L. R., Koch, F., Mittelsdorf, H., et al. (2015). The effects of elevated CO2 on the growth and toxicity of field populations and cultures of the saxitoxin-producing dinoflagellate, Alexandrium fundyense. Limnol. Oceanogr. 60, 198–214. doi: 10.1002/lno.10012
Hawkesworth, J. (1773). An Account of the Voyages Undertaken by the Order of his Present Majesty for Making Discoveries in the Southern Hemisphere, and Successively Performed by Commodore Byron, Captain Carteret, Captain Wallis, and Captain Cook, in the Dolphin, the Swallow, and the Endeavour. London: W. Strahan & T. Cadell.
Henry, D. (ed.) (1875). An Historical Account of all the Voyages Round the World, Performed by English Navigators; Including those Lately Undertaken by Order of his Present Majesty. The whole Faithfully Extracted from the Journals of the Voyagers. In Four Volumes. London: Printed for F. Newbery.
Hernández, C., Díaz, P. A., Molinet, C., and Seguel, M. (2016). Exceptional climate anomalies and northwards expansion of Paralytic Shellfish Poisoning outbreaks in Southern Chile. Harmful Algae News 54, 1–2.
Hiscock, M. R., Marra, J., Smith, W. O., Goericke, R., Measures, C., Vink, S., et al. (2003). Primary productivity and its regulation in the Pacific Sector of the Southern Ocean. Deep Sea Res. II 50, 533–558. doi: 10.1016/S0967-0645(02)00583-0
Ho, K.-C., Kang, S.-H., Lam, I. H. Y., and Hodgkiss, I. J. (2003). Distribution of Alexandrium tamarense in Drake Passage and the threat of harmful algal blooms in the Antarctic Ocean. Ocean Polar Res. 25, 625–631.
Hutchins, D. A., Sedwick, P. N., DiTullio, G. R., Boyd, P. W., Quéguiner, B., Griffiths, F. B., et al. (2001). Control of phytoplankton growth by iron and silicic acid availability in the subantarctic Southern Ocean: experimental results from the SAZ Project. J. Geophys. Res. 106, 31559–31572. doi: 10.1029/2000JC000333
Iriarte, J. L., Quiñones, R. A., and Gonzáles, R. R. (2005). Relationship between biomass and enzymatic activity of a bloom-forming dinoflagellate (Dinophyceae) in southern Chile (41°S): a field approach. J. Plankton Res. 27, 159–166. doi: 10.1093/plankt/fbh167
Jeong, H. J., Lim, A. S., Franks, P. J. S., Lee, K. H., Kim, J. H., Kang, N. S., et al. (2015). A hierarchy of conceptual models of red-tide generation: Nutrition, behavior, and biological interactions. Harmful Algae 47, 97–115. doi: 10.1016/j.hal.2015.06.004
John, U., Litaker, W., Montresor, M., Murray, S., Brosnahan, M. L., and Anderson, D. M. (2014a). (2302) Proposal to reject the name Gonyaulax catenella (Alexandrium catenella) (Dinophyceae). Taxon 63, 932–933. doi: 10.12705/634.21
John, U., Litaker, R. W., Montresor, M., Murray, S., Brosnahan, M. L., and Anderson, D. M. (2014b). Formal revision of the Alexandrium tamarense species complex (Dinophyceae) taxonomy: the introduction of five species with emphasis on molecular-based (rDNA) classification. Protist 165, 779–804. doi: 10.1016/j.protis.2014.10.001
Kerr, R. (1824). A General History and Collection of Voyages and Travels, Arranged in Systematic Order: Forming a Complete History of the Origin and Progress of Navigation, Discovery, and Commerce, by Sea and Land, from the Earliest Ages to the Present Time, Vol. 10. Edinburgh & London: William Blackwood & T. Cadell.
Kopczynska, E. E., Dehairs, F., Elskens, M., and Wright, S. (2001). Phytoplankton and microzooplankton variability between the Subtropical and Polar Fronts south of Australia: thriving under regenerative and new production in late summer. J. Geophys. Res. 106, 31597–31609.
Lagos, N. (1998). Microalgal blooms: a global issue with negative impact in Chile. Biol. Res. 31, 375–386.
Lara, C., Saldías, G. S., Tapia, F. J., Iriarte, J. L., and Broitman, B. R. (2016). Interannual variability in temporal patterns of Chlorophyll-a and their potential influence on the supply of mussel larvae to inner waters in northern Patagonia (41–44°S). J. Mar. Syst. 155, 11–18. doi: 10.1016/j.jmarsys.2015.10.010
Leblanc, K., Hare, C. E., Boyd, P. W., Bruland, K. W., Sohst, B., Pickmere, S., et al. (2005). Fe and Zn effects on the Si cycle and diatom community structure in two contrasting high and low-silicate HNLC areas. Deep Sea Res. I 52, 1842–1864. doi: 10.1016/j.dsr.2005.06.005
Lee, T. C.-H., Kwok, O.-T., Ho, K.-C., and Lee, F. W.-F. (2012). Effects of different nitrate and phosphate concentrations on the growth and toxin production of an Alexandrium tamarense strain collected from Drake Passage. Mar. Environ. Res. 81, 62–69. doi: 10.1016/j.marenvres.2012.08.009
Lembeye, G. (1981a). Segunda aparición del veneno paralitico de los mariscos (VPM) asociado a Gonyaulax catenella, en Magallanes (Chile), 1981. Ans. Inst. Pat. 12, 273–276.
Lembeye, G. (1981b). Estructura del fitoplancton asociado a la presencia del veneno paralitico de los mariscos en Seno Union y áreas adyacentes (Magallanes, Chile), 1981. Ans. Inst. Pat. 12, 277–289.
Lembeye, G. (2008). “Harmful algal blooms in the austral Chilean channels and fjords,” in Progress in the Oceanographic Knowledge of Chilean Interior Waters, from Puerto Montt to Cape Horn, eds N. Silva and S. Palma (Valparaíso: Comité Oceanográfico Nacional - Pontificia Universidad Católica de Valparaíso), 99–103.
Lembeye, G., Guzmán, L., and Campodónico, I. (1975). Estudios sobre un florecimiento tóxico causado por Gonyaulax catenella en Magallanes. III. – Fitoplancton asociado. Ans. Inst. Pat. VI, 197–208.
León-Muñoz, J., Urbina, M. A., Garreaud, R., and Iriarte, J. L. (2018). Hydroclimatic conditions trigger record harmful algal bloom in western Patagonia (summer 2016). Sci. Rep. 8:1330. doi: 10.1038/s41598-018-19461-4
Mardones, J., Clément, A., Rojas, X., and Aparicio, C. (2010). Alexandrium catenella during 2009 in Chilean waters, and recent expansion to coastal ocean. Harmful Algae News 41, 8–9.
Mardones, J. I., Bolch, C., Guzmán, L., Paredes, J., Varela, D., and Hallegraeff, G. M. (2016a). Role of resting cysts in Chilean Alexandrium catenella dinoflagellate blooms revisited. Harmful Algae 55, 238–249. doi: 10.1016/j.hal.2016.03.020
Mardones, J. I., Müller, M. N., and Hallegraeff, G. M. (2016b). Toxic dinoflagellate blooms of Alexandrium catenella in Chilean fjords: a resilient winner from climate change. ICES J. Mar. Sci. 74, 988–995. doi: 10.1093/icesjms/fsw164
Mardones, J. I., Fuenzalida, G., Zenteno, K., Alves-de-Souza, C., Astuya, A., and Dorantes-Aranda, J. J. (2019). Salinity-growth response and ichthyotoxic potency of the Chilean Pseudochattonella verruculosa. Front. Mar. Sci. 6:24. doi: 10.3389/fmars.2019.00024
Mardones, J. I. (2020). Screening of Chilean fish-killing microalgae using a gill cell-based assay. Latin Am. J. Aquatic Res. 48, 329–335. doi: 10.3856/vol48-issue2-fulltext-2400
Mardones, J. I., Norambuena, L., Paredes, J., Fuenzalida, G., Dorantes-Aranda, J. J., Lee Chang, K. J., et al. (2020). Unraveling the Karenia selliformis complex with the description of a non-gymnodimine producing Patagonian phylotype. Harmful Algae 98:101892. doi: 10.1016/j.hal.2020.101892
Mardones, J. I., Paredes, J., Godoy, M., Suarez, R., Norambuena, L., Vargas, V., et al. (2021). Disentangling the environmental processes responsible for the world’s largest farmed fish-killing harmful algal bloom: Chile, 2016. Sci. Total Environ. 766:144383. doi: 10.1016/j.scitotenv.2020.144383
Margalef, R. (1978). Life forms of phytoplankton as survival alternatives in an unstable environment. Oceanol. Acta 1, 493–509.
Masiokas, M. H., Cara, L., Villalba, R., Pitte, P., Luckman, B. H., Toum, E., et al. (2019). Streamflow variations across the Andes (18°–55°S) during the instrumental era. Sci. Rep. 9:17879. doi: 10.1038/s41598-019-53981-x
Molinet, C., Lafon, A., Lembeye, G., and Moreno, C. A. (2003). Patrones de distribución espacial y temporal de floraciones de Alexandrium catenella (Whedon & Kofoid) Balech 1985, en aguas interiores de la Patagonia noroccidental de Chile. Revista Chilena de Historia Natural 76, 681–698.
Montero, P., Daneri, G., Tapia, F., Iriarte, J. L., and Crawford, D. (2017). Diatom blooms and primary production in a channel ecosystem of central Patagonia. Latin Am. J. Aquatic Res. 45, 999–1016. doi: 10.3856/vol45-issue5-fulltext-16
Moore, S. K., Trainer, V. L., Mantua, N. J., Parker, M. S., Laws, E. A., Backer, L. C., et al. (2008). Impacts of climate variability and future climate change on harmful algal blooms and human health. Environ. Health 7 (Suppl. 2):S4. doi: 10.1186/1476-069X-7-S2-S4
Moss, M. (1993). Shellfish, gender, and status on the Northwest Coast: reconciling archeological, ethnographic, and ethnohistorical records of the Tlingit. Am. Anthropol. 95, 631–652.
Muñoz, P., Avaria, S., Sievers, H., and Prado, R. (1992). Presencia de dinoflagelados toxicos del genero en el Seno Aysen, Chile. Revista de Biología Marina Valparaíso 27, 187–212.
Navarro, J. M., Muñoz, M. G., and Contreras, A. M. (2006). Temperature as a factor regulating growth and toxin content in the dinoflagellate Alexandrium catenella. Harmful Algae 5, 762–769. doi: 10.1016/j.hal.2006.04.001
Nelson, D. M., and Tréguer, P. (1992). Role of silicon as a limiting nutrient to Antarctic diatoms: evidence from kinetic studies in the Ross Sea ice-edge zone. Mar. Ecol. Prog. Ser. 80, 255–264.
Nixon, S. W. (1995). Coastal marine eutrophication: a definition, social causes, and future concerns. Ophelia 41, 199–219.
Odate, T., and Fukuchi, T. (1995). Physical and chemical properties of surface water in the Southern Ocean in summer 1991/92. Proc. NIPR Symp. Polar Biol. 8, 77–85.
Orsi, A. H., Whitworth, T., and Nowlin, W. D. (1995). On the meridional extent and fronts of the Antarctic Circumpolar Current. Deep Sea Res. I 42, 641–673.
O’Sullivan, D. R. (1893). Tierra del Fuego. The Fortnightly Review. Old Series LIX (New Series LIII). London: Chapman & Hall, 36–53.
Pigafetta, A. (1874). The First Voyage Around the World by Magellan, Translated from the Accounts of Pigafetta and Other Contemporary Writers. Accompanied by Original Documents, with Notes and an Introduction by Lord Stanley of Alderley. London: Hakluyt Society.
Paredes, J., Varela, D., Martínez, C., Zúñiga, A., Correa, K., Villarroel, A., et al. (2019). Population genetic structure at the northern edge of the distribution of Alexandrium catenella in the Patagonian fjords and its expansion along the open Pacific Ocean coast. Front. Mar. Sci. 5:532. doi: 10.3389/fmars.2018.00532
Paredes-Mella, J., Varela, D., Fernández, P., and Espinoza-González, O. (2020). Growth performance of Alexandrium catenella from the Chilean fjords under environmental drivers: plasticity as a response to a highly variable environment. J. Plankton Res. 42, 119–134. doi: 10.1093/plankt/fbaa011
Pizarro, G., Garrido, C., Cárdenas, C., Frangópulos, M., Alarcón, C., Guzmán, L., et al. (2011). Distribución espacial de Alexandrium catenella y de toxinas paralizantes en el plancton y mariscos entre el Golfo de Penas y Canal Trinidad (Primavera 2008). Ciencia y Tecnología del Mar 34, 19–30.
Pizarro, G., Paz, P., Alarcón, C., Toro, C., Frangópulos, M., Salgado, P., et al. (2018). Winter distribution of toxic, potentially toxic phytoplankton, and shellfish toxins in fjords and channels of the Aysén region, Chile. Lat. Am. J. Aquat. Res. 46, 120–139. doi: 10.3856/vol46-issue1-fulltext-13
Sarmiento, J. L., Gruber, N., Brzezinski, M. A., and Dunne, J. P. (2004). High-latitude controls of thermocline nutrients and low latitude biological productivity. Nature 427, 56–60.
Schneider, W., and Bravo, L. (2006). Argo profiling floats document Subantarctic Mode Water formation west of Drake Passage. Geophys. Res. Lett. 33:L16609. doi: 10.1029/2006GL026463
Sedwick, P. N., DiTullio, G. R., Hutchins, D. A., Boyd, P. W., Griffiths, F. B., Crossley, A. C., et al. (1999). Limitation of algal growth by iron deficiency in the Australian Subantarctic region. Geophys. Res. Lett. 26, 2865–2868. doi: 10.1029/1998GL002284
Sievers, H. A., and Silva, N. (2008). “Water masses and circulation in austral Chilean channels and fjords,” in Progress in the Oceanographic Knowledge of Chilean Interior Waters, from Puerto Montt to Cape Horn, eds N. Silva and S. Palma (Valparaíso: Comité Oceanográfico Nacional - Pontificia Universidad Católica de Valparaíso), 53–58.
Silva, N. (2008). “Dissolved oxygen, pH, and nutrients in the austral Chilean channels and fjords,” in Progress in the Oceanographic Knowledge of Chilean Interior Waters, from Puerto Montt to Cape Horn, eds N. Silva and S. Palma (Valparaíso: Comité Oceanográfico Nacional - Pontificia Universidad Católica de Valparaíso), 37–43.
Silva, N., Rojas, N., and Fedel, A. (2009). Water masses in the Humboldt Current System: properties, distribution, and the nitrate deficit as a chemical water mass tracer for Equatorial Subsurface Water off Chile. Deep Sea Res. II 56, 1004–1020. doi: 10.1016/j.dsr2.2008.12.013
Smayda, T. J. (2002). Turbulence, water mass stratification and harmful algal blooms: an alternative view and frontal zones as “pelagic seed banks”. Harmful Algae 1, 95–112.
Sullivan, J. M., Swift, E., Donaghay, P. L., and Rines, J. E. B. (2003). Small-scale turbulence affects the division rate and morphology of two red-tide dinoflagellates. Harmful Algae 2, 183–199. doi: 10.1016/S1568-9883(03)00039-8
Timmermans, K. R., van Leeuwe, M. A., de Jong, J. T. M., McKay, R. M. L., Nolting, R. F., Witte, H. J., et al. (1998). Iron stress in the Pacific region of the Southern Ocean: evidence from enrichment bioassays. Mar. Ecol. Prog. Ser. 166, 27–41.
Torres, R., Frangópulos, M., Hamamé, M., Montecino, V., Maureira, C., Pizarro, G., et al. (2011a). Nitrate to silicate ratio variability and the composition of micro-phytoplankton blooms in the inner-fjord of Seno Ballena (Strait of Magellan, 54°S). Continental Shelf Res. 31, 244–253. doi: 10.1016/j.csr.2010.07.014
Torres, R., Pantoja, S., Harada, N., González, H. E., Daneri, G., Frangópulos, M., et al. (2011b). Air-sea CO2 fluxes along the coast of Chile: From CO2 outgassing in central northern upwelling waters to CO2 uptake in southern Patagonian fjords. J. Geophys. Res. 116:C09006. doi: 10.1029/2010JC006344
Torres, R., Silva, N., Reid, B., and Frangópulos, M. (2014). Silicic acid enrichment of subantarctic surface water from continental inputs along the Patagonian archipelago interior sea (41–56°S). Prog. Oceanogr. 129, 50–61. doi: 10.1016/j.pocean.2014.09.008
Trainer, V. L., Moore, S. K., Hallegraeff, G., Kudela, R. M., Clement, A., Mardones, J. I., et al. (2020). Pelagic harmful algal blooms and climate change: lessons from nature’s experiments with extremes. Harmful Algae 91:101591. doi: 10.1016/j.hal.2019.03.009
Uribe, J. C. (1988). Antecedentes sobre un tercer brote de veneno paralizante de moluscos (VPM), en la región de Magallanes. Ans. Inst. Pat. Ser. Cs. Nts., Punta Arenas (Chile) 18, 97–101.
Uribe, J. C., and Ruiz, M. (2001). Gymnodinium brown tide in the Magellanic fjords, Southern Chile. Revista de Biología Marina y Oceanografía 36, 155–164.
Uribe, J. C., Oyarzún, S., and Latorre, V. (2010). Alexandrium catenella (Whedon & Kofoid) Balech, 1985, in Magellan waters, Chile. Anales Instituto Patagonia (Chile) 38, 103–110.
Varela, D., Paredes, J., Alves-de-Souza, C., Seguel, M., Sfeir, A., and Frangópulos, M. (2012). Intraregional variation among Alexandrium catenella (Dinophyceae) strains from southern Chile: Morphological, toxicological and genetic diversity. Harmful Algae 15, 8–18. doi: 10.1016/j.hal.2011.10.029
Vargas, C. A., Cuevas, L. A., Silva, N., González, H. E., De Pol-Holz, R., and Narváez, D. A. (2018). Influence of glacier melting and river discharges on the nutrient distribution and DIC recycling in the Southern Chilean Patagonia. J. Geophys. Res. Biogeosci. 123, 256–270. doi: 10.1002/2017JG003907
Villanueva, F., Cortez, H., Uribe, C., Peña, P., and Cassis, D. (2017). Mortality of Chilean farmed salmon in wellboats in transit through a Karenia bloom. Harmful Algae News 57, 4–5.
Weidemann, S. S., Sauter, T., Kilian, R., Steger, D., Butorovic, N., and Schneider, C. (2018). A 17-year record of meteorological observations across the Gran Campo Nevado Ice Cap in Southern Patagonia, Chile, related to synoptic weather types and climate modes. Front. Earth Sci. 6:53. doi: 10.3389/feart.2018.00053
Wolf, C., Frickenhaus, S., Kilias, E. S., Peeken, I., and Metfies, K. (2014). Protist community composition in the Pacific sector of the Southern Ocean during austral summer 2010. Polar Biol. 37, 375–389. doi: 10.1007/s00300-013-1438-x
Wyatt, N. J., Milne, A., Woodward, E. M. S., Rees, A. P., Browning, T. J., Bouman, H. A., et al. (2014). Biogeochemical cycling of dissolved zinc along the GEOTRACES South Atlantic transect GA10 at 40°S. Global Biogeochem. Cycles 28, 44–56. doi: 10.1002/2013GB004637
Keywords: Chile, Pacific Ocean, phytoplankton “bloom”, Alexandrium catenella, HAB (harmful algal blooms), fjords and channels, Patagonia, subantarctic water (SAW)
Citation: Crawford DW, Montero P and Daneri G (2021) Blooms of Alexandrium catenella in Coastal Waters of Chilean Patagonia: Is Subantarctic Surface Water Involved? Front. Mar. Sci. 8:612628. doi: 10.3389/fmars.2021.612628
Received: 30 September 2020; Accepted: 15 March 2021;
Published: 20 April 2021.
Edited by:
Stelios Katsanevakis, University of the Aegean, GreeceReviewed by:
Suzanne Jane Painting, Centre for Environment, Fisheries and Aquaculture Science (CEFAS), United KingdomJorge I. Mardones, Instituto de Fomento Pesquero (IFOP), Chile
Copyright © 2021 Crawford, Montero and Daneri. This is an open-access article distributed under the terms of the Creative Commons Attribution License (CC BY). The use, distribution or reproduction in other forums is permitted, provided the original author(s) and the copyright owner(s) are credited and that the original publication in this journal is cited, in accordance with accepted academic practice. No use, distribution or reproduction is permitted which does not comply with these terms.
*Correspondence: Giovanni Daneri, Z2RhbmVyaUBjaWVwLmNs