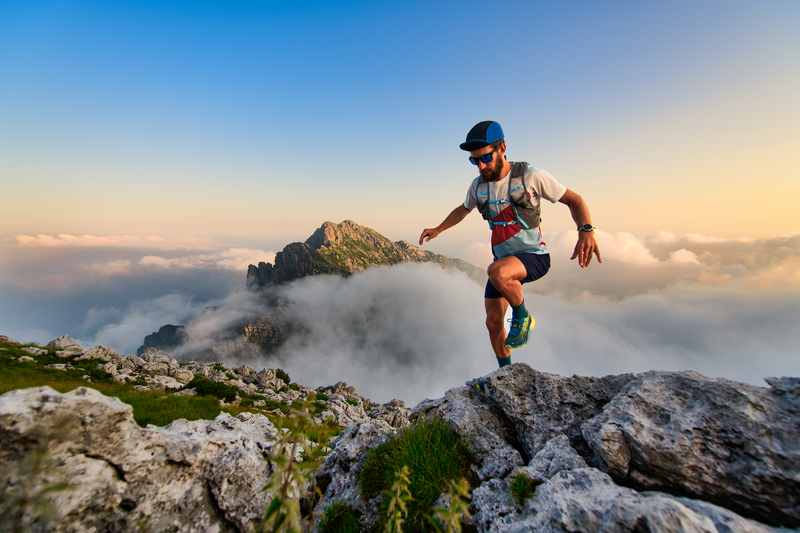
95% of researchers rate our articles as excellent or good
Learn more about the work of our research integrity team to safeguard the quality of each article we publish.
Find out more
REVIEW article
Front. Mar. Sci. , 26 February 2021
Sec. Marine Fisheries, Aquaculture and Living Resources
Volume 8 - 2021 | https://doi.org/10.3389/fmars.2021.609248
This article is part of the Research Topic Zoonosis Associated with Parasites and Infectious Diseases in Aquatic Animals View all 5 articles
Although research into the ecology and impacts of invasive species is prevalent, there are knowledge gaps relating to the role of invasive species in parasite transmission. This work synthesises invasive host–parasite interactions and impacts, using marine bivalves as a model group, to consider how global movement of shellfish consignments for aquaculture purposes facilitates the unintentional transfer of invasives. We discuss how invasive species can act as both hosts or parasitic organisms themselves, and introductions may lead to diseases within the bivalve aquaculture sector. This review highlights the importance of interdisciplinary research, with particular regard to the fields of parasitology and invasion ecology. We suggest that further integrating these fields will enhance critical knowledge of marine diseases, parasite-invasive-bivalve interplay dynamics, and potential mitigation strategies, including temperature-based disease surveillance models. We also address how climate change might impact invasive species, again with a focus on marine bivalves, and the potential outcomes for parasite transmission, including changes in host/parasite distribution, life-history and virulence. We acknowledge the importance of horizon scanning for future invasive host–parasite introductions and note that increased screening of invasive species, both in their native and invaded ranges, will provide clarity on invasion dynamics and potential impacts.
The purpose of this review is to highlight the intrinsic links between marine bivalves and invasive host–parasite interactions, and the subsequent impacts on the aquaculture sector. There is a particular focus on the cyclical nature of these links, as commercial bivalve culture often provides a pathway for invasive host–parasite complexes and yet these same bivalves are then impacted by disease outbreaks caused by the parasites. Throughout the existing literature a number of synonyms exist for species that have extended their geographic distribution outside of their native range, primarily ‘alien’, ‘exotic’, ‘non-indigenous’ and ‘non-native’. However, these terms must be carefully distinguished from ‘invasive species’, defined here as having spread widely beyond the point of initial establishment (Lodge et al., 2006) and likely to have deleterious effects on biodiversity and associated ecosystem services in their new range (EU regulation 1146,2014). Here, we distinguish between non-native species, which although introduced do not necessarily have negative impacts, and invasive species (as defined above) and use these terms and definitions throughout.
The movement of invasive species in the marine environment has been exacerbated in recent decades due to increased globalisation resulting from trade and travel (Hulme, 2009; Katsanevakis et al., 2016). Introductions can be mediated by deliberate human intervention but vectors of introduction are primarily accidental, with transport of the invasive species secondary to primary purposes such as trade and tourism (Lodge et al., 2006; Hulme, 2015). The relative importance of vectors for accidental introductions differs both spatially and temporally. However, aquaculture, ballast water and hull fouling are consistently cited as prominent vectors (Minchin et al., 2009; Williams et al., 2013; Hulme, 2015; Laeseke et al., 2020). Anthropogenic debris is another vector, and may be influenced by aquaculture activities due to the contribution of aquaculture gear to what is termed ‘abandoned, lost or otherwise discarded fishing gear’ (ALDFG) (Rech et al., 2018). Accidental introductions may also arise through escapes from captivity (Hulme, 2009), from recreational water use (Hulme, 2015) and from static marine structures (Laeseke et al., 2020).
Global production of marine bivalves, particularly oysters, mussels, clams and scallops, measures over 15 million tonnes per year, with 89% of this production coming from aquaculture rather than wild fisheries (Wijsman et al., 2019). Indeed, culture of shelled molluscs accounts for 58.8% of the combined production of marine and coastal aquaculture (FAO, 2018). Over 85% of bivalve production originates in Asia, particularly China (Wijsman et al., 2019), and the sector is projected to increase globally to provide food security as a source of affordable protein (Steeves et al., 2018). It is therefore important to fully elucidate the potential risks and challenges to growth in an effort to develop sustainable practices. Intentional translocation of bivalves for aquaculture is common practice, for example Atlantic European countries regularly engage in local, national and regional transfer throughout the European economic zone, along with occasional international transfers (Muehlbauer et al., 2014). These transfers can lead to one of the primary risks to sustainable growth; the movement of invasive species and their associated parasites.
Aquaculture is one of the primary anthropogenic activities that results in the unintentional movement of invasive species, as they may be inadvertently shipped with consignments of cultured species (Naylor et al., 2001). Potentially invasive organisms can be transported in a number of ways, for example as epifauna on the shells, internally within the shell cavity, in water, sediment or equipment, or in association with other fouling epifauna. The three major groups that may be introduced are phytoplankton, macrofauna, and macroalgae (McKindsey et al., 2007). It follows therefore that parasitic introductions will increase, as parasites travel with invasive hosts, or are unintentionally introduced with purposefully translocated cultured hosts (Peeler et al., 2011). The term parasite is often used in its broadest sense to include macroparasites, defined as those in which direct multiplication in the definitive host is low or entirely absent, and microparasites, specifically protozoa, bacteria and viruses that display high rates of direct reproduction within the host (Anderson and May, 1979). Both macro- and microparasites affect naturally occurring aquatic species and also the commercial bivalve species utilised both in cultured systems and in local, often traditional, shellfisheries.
Climate change will influence the geographic range, abundance and impacts of invasive species (Walther et al., 2009; Beaury et al., 2020). Warming temperatures will likely allow invasive species to expand into regions where previously they could not survive and reproduce. Altered climatic factors might also facilitate extended spawning times, increases in reproductive output and growth rates and altered dispersal due to modified hydrodynamic conditions (Mellin et al., 2016). However, the impact of climate change on invasive-parasite interactions, and the subsequent potential for disease transmission in the marine environment is still a developing field of research. Climate change may enhance the virulence of introduced pathogens, or cause disease outbreaks in new ranges if pathogens are introduced (Conn, 2014; Rowley et al., 2014). Furthermore, susceptible hosts may already be under increased thermal or osmotic stress (Harvell et al., 2002; Burge et al., 2016a), rendering them vulnerable to infection and potential mortality events, which can in turn negatively impact the aquaculture sector.
Awareness of the links between parasitology and invasion ecology is still not fully reflected in research output. Indeed Poulin (2017) noted circa 4000 studies per year on invasive species, but circa 50 per year only on parasitism in the context of biological invasions. However, the potential role of parasites in marine invasions is increasingly recognised as an important factor in invasion biology (Lagrue, 2017). The importance of marine bivalves in invasive parasite/host interactions and their influence on native community dynamics are synthesised in the following sections.
Bivalves are an interesting model group when considering invasion ecology as they can enter new regions via accidental introductions or as a result of deliberate translocations for aquaculture (McKindsey et al., 2007) where they can be stocked at high densities (Wijsman et al., 2019), spread quickly from initial introduction points, are susceptible to co-infections (Zannella et al., 2017) and can transfer parasites to native bivalves. They may also introduce other cohabiting and potentially invasive species (McKindsey et al., 2007). Ruesink et al. (2005) noted, in a study of nine regions, that the culturing of non-native oysters introduced 78 species of invasive marine algae, invertebrates and protozoa. The role of bivalve culture in species introductions holds true across other studies, as an estimated 40% of non-native species in the North Sea arrived via oyster culture (Gallardi, 2014). Furthermore, an analysis of current and historical introductions of both marine and estuarine species in California found that 126 introductions arose from aquaculture practices, and of these 106 species became established. A number of the species that established are also bivalve predators, specifically the Atlantic oyster drill Urosalpinx cinerea and Japanese oyster drill Ocenebra inornata, and are now considered severe oyster pests (Grosholz et al., 2015).
In the marine environment the introduction of bivalves for aquaculture, similar in function to the expansion of invasive species to new ranges, may be considered a vector for unintentional parasite introduction and can be used as a proxy to investigate how the movement of invasive species may transport parasites. A number of these heavily cultured species, specifically the Pacific cupped oyster Crassostrea gigas (Goedknegt et al., 2017), the Mediterranean mussel Mytilus galloprovincialis (Lynch et al., 2020) and the Manila clam Ruditapes philippinarum (Cordero et al., 2017) are considered to display invasive tendencies despite also being commercially important species. The American razor clam Ensis directus (Gollasch et al., 2015) underwent accidental introductions to Europe and also displays invasive tendencies, despite being the subject of commercial fisheries (Table 1).
Table 1. A sample of introduced marine bivalves and/or parasites demonstrating the diversity of bivalve-parasite interactions and the impacts on commercial species, both wild and cultured.
Parasites and diseases in the marine environment are currently a challenge to bivalve aquaculture practices (Solomieu et al., 2015). For example, microvariants of ostreid herpesvirus OsHV-1 threaten global production of C. gigas by inducing mass mortalities in early life-stages (Carnegie et al., 2016; Bookelaar et al., 2018). Macroparasites, often in the form of trematode species, can compromise the immunology and physiology of the hosts, thereby impacting the ecology of the species (Morley, 2010). Microparasites may include bacterial diseases, often belonging to the genus Vibrio, protistans and viruses. A number of protistan species, particularly within the genera Bonamia, Haplosporidium, Marteilia, and Perkinsus, are recognised as threats to bivalve populations (Fernández-Robledo et al., 2014), as are viruses (Coen and Bishop, 2015) and bacterial species such as Vibrio aestuarianus and V. splendidus (Solomieu et al., 2015).
Parasites associated with freshwater biological invasions are well-documented. For example, upon introduction to the Great Lakes, the invasive zebra mussel Dreissena polymorpha introduced the parasitic platyhelminth Bucephalus polymorphus to cyprinid fish (Crowl et al., 2008). Furthermore, the zebra mussel has been the subject of numerous baseline parasitological studies early in its invasion, partly in an effort to detect viable biological controls, confirming the presence of helminths, protistans, and bacteria (Toews et al., 1993). However, although the interactions between parasites and marine invasive species are increasingly recognised (Goedknegt et al., 2016), information relating to marine ecosystems is less available (Vignon and Sasal, 2010). Accordingly, questions about the co-introduction of parasites with invasive species, for example their origin or impacts, or the potential role of invasive species in the transmission of native parasites still arise (Lagrue, 2017; Poulin, 2017; Lucy et al., 2020). Please see Goedknegt et al. (2016) for an in-depth review of invasive species-parasite interactions across multiple taxa in the marine environment.
Marine diseases are a field important to both aquaculture research and management, particularly given the well-documented diversity of parasites and pathogens as seen in Table 1 (Lafferty and Hofmann, 2016). Aquaculture practices have long been associated with repeated introductions of infectious diseases (Lafferty and Kuris, 1999), particularly as high stocking densities under aquaculture conditions can encourage the spread of parasitic infections (Cheng and Combes, 1990). An increased understanding of how invasive species also influence disease dynamics in bivalve aquaculture is necessary, as rapid detection of pathogens is important in preventing wide scale disease outbreaks (Telfer and Bowen, 2012). Interactions between parasites, invasive species and native communities are complex as invaders may benefit from parasite loss, transmit novel parasites to native hosts (spillover) or acquire new generalist parasites native to the invaded range, which may then spillback to native hosts (Figure 1) (Dunn et al., 2012).
Figure 1. Major interactions between invasive hosts, parasites and native hosts featuring: (clockwise) (A) Enemy release hypothesis whereby an invasive host escapes some or all of its natural parasites as it moves through a series of biotic and abiotic filters (the invasion pathway) to a new range. (B) The introduction of free-living parasite stages. (C) Climate change and the potential for changes in disease prevalence/virulence. (D) Spillback whereby an invasive species proves a suitable host for native parasites thus amplifying the load. (E) Spillover whereby an invasive host spills its parasites to native hosts.
Parasite spillover from an invasive species can occur when a parasite that co-invades with the invasive host infects a new susceptible native host species. For example, the parasitic copepod Myicola ostreae travelled from Asia (native to Korea and Japan) with C. gigas consignments to France where it succeeded in becoming established and infecting the European flat oyster Ostrea edulis. The copepod can reduce the growth rate of the host, impacting the market quality and also potentially leading to mortality (CABI, 2020b datasheet 110220). Mechanisms to address the questions relating to parasite spillover in the marine environment include screening invasive species in their native and invaded ranges to compare the parasitic burden in each locality, and then comparing the parasitic assemblages of invasive species in the invaded range with those of native species (Krakau et al., 2006).
Parasite spillback is when a parasite in a native host infects an invasive host, with the presence of an additional host increasing parasite abundance and/or dispersal thereby providing more opportunities to encounter and infect native species (Kelly et al., 2009; Chalkowski et al., 2018). Previous research conducted laboratory transmission trials between oysters and other invertebrate species to investigate whether they can act as carriers or vectors of parasites and confirmed that the native brittle star Ophiothrix fragilis was capable of transmitting Bonamia ostreae to naive O. edulis (Lynch et al., 2007). This principle could also be utilised to investigate parasite spillback, as transmission trials could be conducted between native and invasive species to determine if parasites from the native species can infect the invasive species and then in turn be transmitted back to native hosts.
Conceptually, parasite spillover and spillback influence community dynamics through the process of ‘host-switching’ and may be considered augmentative interactions. However, it is important to note that there is also the possibility for parasite dilution, when the addition of novel hosts decreases infection intensity in the native hosts (Blakeslee et al., 2020). Invasive hosts may reduce infection intensity in bivalves by acting as pathogen sinks, if hosts become infected but do not transmit the pathogen to other hosts (Burge et al., 2016a) or if they prey on free-living infectious stages (Thieltges et al., 2009). Alternatively, invasive hosts can be pathogen sources if they act as carriers or reservoirs of disease.
Non-bivalve taxa can serve as carriers or reservoirs of bivalve disease, maintaining bivalve pathogens even when bivalve hosts are absent. For example, Costello et al. (2020) confirmed that invasive tunicates can harbour oyster pathogens, both protistan and bacterial. One such species (the club tunicate Styela clava) maintained the protistan B. ostreae with no oyster hosts present, indicating it can potentially act as a reservoir of the parasite. Additionally, protistan M. mercenariae-like sp. sporulation in S. clava suggested the tunicate can facilitate replication of this parasite. Non filter-feeders have also been found to transmit bivalve diseases; the European shore crab Carcinus maenas can effect transmission of ostreid herpesvirus-1 microvariant (OsHV-1 μVar) from wild-caught C. maenas (from oyster trestles where the presence of the virus has been confirmed) to uninfected C. gigas, demonstrating the role of the crab as a carrier and reservoir of the virus (Bookelaar et al., 2018). The presence of pathogens in these diverse taxa demonstrates the necessity of screening bivalve-associated organisms when investigating pathogen maintenance and transmission.
In addition to maintaining their own suite of parasites, bivalves may also serve as habitat for a multitude of fouling species that have their own associated parasites. Thus, bivalves can carry numerous species that may serve as intermediate or definitive hosts for parasites/pathogens. The relationship between oysters, sponges and parasites is note-worthy, as sponges have networks of canals permeating the interior and are home to a diverse array of invertebrates in relationships that vary from symbiosis to parasitism (Ďuriš et al., 2011). Invertebrates associated with sponges can number up to thousands of individuals and encompass amphipods, crustaceans, isopods, ophiuroids, ostracods, and polychaetes. Even microbial communities within host sponges may differ from the habitat-specific bacteria present in the surrounding environment (Pierce and Ward, 2018). Essentially, the movement of bivalves also facilitates the movement of their associated fouling organisms and potentially the flora and fauna harboured within these fouling pests. Fouling organisms may then potentially interact with parasites resulting in further instances of spillover or spillback.
The strong association between fouling organisms and bivalves means that fouling organisms acting as pests or parasites may also influence the degree of parasitism within the bivalve host. For example, boring sponges in the genus Cliona are considered macroparasites that reduce oyster growth, condition and recruitment. The sponges compromise the integrity of the oyster shells, thus decreasing fitness and potentially increasing susceptibility to predators and parasites (Hanley et al., 2019). Indeed, a study of two different sample sites on the east coast of the United States found that Crassostrea virginica fouled with these sponges were more likely to have a second pest, the pea crab Zaops (Pinnotheres) ostreum. Furthermore, when both sponge and pea crab were present their effects were additive, demonstrating that interactions between bivalve host and fouling pest/parasite can increase susceptibility to other parasites (Watts et al., 2018). While these species are native to the study region, interactions such as these may also be of relevance to invasive bivalve/parasite complexes, if associated fouling organisms influence the degree of parasitism.
With processes such as spillover, spillback and introductions of fouling organisms the invasive host directly interacts with the parasites or fouling species, with subsequent effects on native organisms. However, invasive bivalves can also indirectly affect native community host–parasite dynamics. For example, habitat modifications by the reef-building C. gigas influence parasitism in blue mussels Mytilus edulis, as mussels at the top of the reefs have a higher prevalence of parasitic copepods (Mytilicola spp.) compared to mussels seeking refuge at the bottom of the reef. Conversely the trematode Renicola roscovita, known to reduce blue mussel condition, displays higher prevalence in mussels at the bottom of the reef rather than mussels on the top. The study suggests indirect effects may be more common than currently understood and warrant further research (Goedknegt et al., 2020). There are also examples of the indirect effects of invasive parasites on native hosts, as in separate studies of M. edulis, individuals infected with the parasitic copepod Mytilicola intestinalis were more susceptible to secondary infections with a virulent bacterial Vibrio orientalis/tubiashii strain, thus highlighting the role of indirect effects arising from what was initially parasite spillover of copepod to mussel (Demann and Wegner, 2019).
Invasive bivalves can also interact with other extrinsic factors to influence parasitism in native bivalve communities. For example, a study of invasive C. gigas investigated its role in determining parasitic infection levels in native blue mussel M. edulis in relation to other biotic factors such as salinity, tidal exposure and host densities (both oyster and mussel) (Goedknegt et al., 2019). The results suggested that the presence of an invasive species could affect parasite interactions with native hosts in different ways. For example, when oyster density was high, the prevalence and abundance of the parasitic copepod M. intestinalis decreased in its native mussel host, while prevalence of the trematode R. roscovita increased. The study did note that interactions may be further mediated by environmental and biotic factors, as invasive species are not the sole drivers of infection levels (Goedknegt et al., 2019).
Parasites are particularly relevant to invasion ecology, as escape from parasites may facilitate host invasion via a mechanism known as the enemy release hypothesis (ERH) (Figure 1) (Torchin et al., 2001). Invasive species may lose their parasites as they colonise a new range, perhaps due to unsuitable environmental conditions, or the absence of a required secondary host. It may also be because the source population of invasive hosts has low parasite prevalence and only a subset of these hosts, some of which may be uninfected, extend their range, meaning that upon entry to the new range parasite abundance is low. Infected hosts may also be more likely to die during transfer due to the additional stress of parasitism. Investigations of the ERH are broadly split into biogeographical studies, which examine native and introduced populations of a specific host, and community studies which compare parasitism between cohabiting native and introduced species (Colautti et al., 2004). One such study identified low levels of digenean trematode infection in introduced Manila clams R. philippinarum compared to native sympatric bivalves. However, digenean infection is also low in native clam populations, potentially due to characteristics intrinsic to the Ruditapes genus (specifically tough epithelial tissue that is difficult for cercariae to penetrate) rather than a classical enemy escape, further highlighting the complexities underpinning bivalve host–parasite interactions (Dang et al., 2009).
If invasive hosts do escape their parasites when colonising a new area, it is unlikely that they will remain free of parasites, especially if native generalist parasites are present. For example, Miller et al. (2008) used laboratory choice-chambers to demonstrate that generalist native pea crabs, Pinnotheres novaezelandiae, could adopt the introduced Asian date mussel Musculista senhousia (now listed on the Global Invasive Species Database), although their preference was for the native New Zealand green lipped mussel Perna canaliculus and the little black mussel Xenostrobus pulex. The impact of native parasites on introduced hosts is host–parasite specific. For example, if the parasite has negative effects it could potentially act as a control and slow the expansion of the host. Furthermore, if the introduced species is not a competent host it may reduce the disease risk for native species by acting as a sink for parasites, but if it is a competent host this amplifies the potential for spillback (Poulin et al., 2011).
The previous examples have focused on invasive species as hosts, however, it is important to note that invasive species can also act as bivalve parasites themselves rather than hosts. For example, invasive shell-boring polychaete worms are parasitic to commercial molluscs and impact host condition by damaging the protective shell and thus reducing the commercial viability (David et al., 2016). The spionid polychaete Polydora hoplura is one such species, an invasive pest that burrows into the shells of commercial bivalves and univalves including Cape rock oysters Striostrea margaritacea and South African abalone Haliotis midae, creating tubes and mud blisters, and reducing the growth rate of the mollusc (Coen and Bishop, 2015).
There is also the potential for parasites to be introduced to an area without a host (e.g., in free-living life stages) and these novel parasites could severely impact native hosts with whom they have no evolutionary history who therefore lack immune defenses (Poulin, 2017). Ostreid herpesvirus OsHV-1 (Evans et al., 2014) and the protistan B. ostreae (Arzul et al., 2009) are known to persist in the water column and, in the case of B. ostreae, within larvae brooded in the adult pallial cavity even though parents were negative for infection (Flannery et al., 2016). The fact that free living life stages of bacteria, viruses and protistans are potentially present in coastal waters highlights the intrinsic link between disease and filter-feeding bivalves, as consumption is a major pathway for parasite transmission in the bivalve hosts (Ben-Horin et al., 2015).
Introductions of free-living life-stages of parasites without an obvious associated host may give rise to cryptogenic invasions, meaning the origin and biogeographic status of the invasive species is unknown. For example, the cryptogenic protistan parasite Haplosporidium pinnae is the causative agent of mass-mortality events of the protected fan mussel Pinna nobilis in the western Mediterranean (Katsanevakis et al., 2019). These mortality events first occurred in 2016 and are continuing to spread, and the study noted that it is probable that this parasite is alien to the Mediterranean Sea. The fan mussel is Critically Endangered (IUCN Red List) due to historical exploitation and ongoing poaching, and the parasite poses a further threat to the conservation of the species (Katsanevakis et al., 2019). Of additional concern is the fact that H. pinnae is not the only parasite present in fan mussels undergoing mortality events. A gram-positive bacteria belonging to the genus Mycobacterium (considered invasive within the study) has also been detected, resulting in co-infections within fan mussels in Greek waters and causing high mortalities (Lattos et al., 2020).
Parasite–host interactions can be increasingly complex. For example, the digenean trematode Parvatrema duboisi uses the Manila clam R. philippinarum as an intermediate host (Yanagida et al., 2009). However, the trematode is in turn hyperparasitised by a protistan Urosporidium sp. and the Urosporidium burden on its trematode host causes mortality, thus decreasing the trematode load on the clam host (Le et al., 2015). While hyperparasitism in this instance is not causing deleterious effects to the clam itself, it can be the case that hyperparasitism can lead to decreased market value of commercial species (Le et al., 2015) and as such parasitological studies may be enhanced by elucidating all forms of parasitism within a host.
Disentangling host–parasite relationships may also lead to novel forms of monitoring and control, particularly when the functional role of bivalves as filter-feeders is taken into consideration. Ford et al. (2009) postulated that as bivalves are filter-feeders, both hosts and non-hosts take in parasites from the water column, but while susceptible hosts then become infectious, non-susceptible hosts discard the parasites in faeces. This principle was applied to investigate the environmental distribution of the protistan Haplosporidium nelsoni using faecal samples from both a non-host bivalve, the ribbed mussel Geukensia demissa, and a host with developed resistance, the Eastern oyster C. virginica. Data from faecal samples was then used in conjunction with tissue samples to describe pathogen distribution and temporal patterns that were not evident using traditional histology. Bivalves have also been posited as having the ability to remove or reduce pathogen concentrations. However, while filtration can remove pathogens from the water column thereby reducing transmission it can also have the converse effect of increasing disease risk if the filter-feeder acts as a reservoir. The outcome is contingent on factors such as the selectivity of the filter-feeder, mechanisms of pathogen transmission, susceptibility of that pathogen to degradation and the degree of infectivity (Burge et al., 2016a).
The control of parasitic diseases can benefit from knowledge of the entire lifecycle of the focal parasite. Blasco-Costa and Poulin (2017) used helminths as a model group to demonstrate that many species are known only from their adult stage and neither juvenile stages or intermediate hosts are confirmed, and that this issue may be applied more broadly to other taxa. This is important for integrated ecology, as predicting and mitigating against invasive introductions and their effects on parasites requires knowledge of potential hosts, not only to confirm potential reservoirs but to know what life history stage to target if attempting eradication. Ultimately, understanding the mechanisms by which invasives transmit parasites and extending studies to different phyla, particularly those with links to aquaculture, may inform management strategies and minimise the risk of disease cycling.
The occurrence of disease requires synergy between three factors; the host, the pathogen and the environment. The interaction between these three factors is termed the epidemiological triangle (Zannella et al., 2017), and the impacts of climate change have the potential to radically change the characteristics of this triangle, particularly the environment (Figure 2). Environmental change increases physiological stress in bivalves in a number of ways, leaving them susceptible to pathogens. Warming water can enhance microbial growth, thereby increasing the content of organic matter in the water, with the subsequent microbial decomposition reducing dissolved oxygen levels (Soon and Zheng, 2019). Hypoxic conditions can impact bivalve immune systems and interact with diseases, for example rendering bivalve hosts less efficient at eliminating bacterial cells (Macey et al., 2008). Higher water temperatures have also led to concerns about the spread of harmful algal blooms (HABs), for example the dinoflagellate Prorocentrum minimum has been implicated in mass mortalities of oysters, scallops and hard clams in North America. For oysters in particular, blooms of this dinoflagellate were found to alter immune system competence in juveniles, thereby comprising their disease resistance. In addition to low dissolved oxygen levels and HABs, toxins and pollutants have been shown to promote infection by the bacterium Vibrio splendidus, the pathogen responsible for juvenile summer mortality in C. gigas spat, also known as bacillary necrosis disease (Soon and Zheng, 2019).
The ecological traits that facilitate the movement of invasive species, for example plastic life-histories coupled with the tendency to occupy generalist and opportunistic niches, are disproportionately favoured under climate change (Mellin et al., 2016). As a result, non-native organisms in the aquatic environment may often be more resilient than native organisms when faced with environmental change, and appear to be at a performance advantage relative to co-occurring native species when subjected to warming or acidification (Sorte et al., 2013). Increased temperatures can cause persistent stress and mortality of native species, thus facilitating the establishment and spread of invasive species that are more tolerant to the higher temperatures or can occupy environmental niches previously held by native species (Diez et al., 2012). Furthermore, although reduction in body size has been suggested as a response to warming in aquatic systems, the inverse effect has been observed in bivalves introduced to the Mediterranean Sea, leading to their competitive dominance over native species. Not only are the introduced bivalves larger than cohabiting native species, there is evidence to suggest that some invasives, for example the mussel Brachidontes pharaonis, are larger in their invaded range than their native range (Nawrot et al., 2017).
Climatic vectors have implications for the movement of invasive species, with hurricanes and storm surges enhancing the dispersal of invasive propagules in the water column (Hellmann et al., 2008). An association between marine disease outbreaks and storm activity is also recognised (Burge et al., 2014). Climate change has increased extreme sea level events such as flooding and heat waves, and is predicted to increase the magnitude of storm surges in coastal areas (IPCC, 2019), where aquaculture occurs. Coastal marine environments are therefore extremely vulnerable to change (Holt et al., 2010) and an altered marine environment can in turn impact the distribution, life-history and physiological status of pathogens, hosts and vectors (Gallana et al., 2013). Furthermore, poleward advances of subtropical species into Europe, referred to as ‘African Creep’ are occurring in the eastern Atlantic (Canning-Clode and Carlton, 2017) and this phenomenon is mirrored by ‘Caribbean Creep’ in the western Atlantic, including the extension of species such as the invasive Asian green mussel Perna viridis (Canning-Clode et al., 2011).
The impacts of climate change on parasite transmission in the marine environment is in the early stages of our understanding (Burge et al., 2014). However, marine invertebrates demonstrate strong links between disease and climate (Marcogliese, 2008) and there is increasing evidence that climate change will affect the ecology of infectious diseases and the physiology of bivalve species and their resistance to infection (Soon and Zheng, 2019). Climate change could affect parasites by influencing the distribution and life-history of hosts (Callaway et al., 2012) and potentially increase host susceptibility to infection due to thermal stress (Harvell et al., 2002). Alterations in water temperature may also lead to osmotic stress, again rendering hosts more susceptible to disease (Burge et al., 2016a).
Warmer temperatures may allow disease-causing organisms to complete their lifecycle more rapidly, thus attaining higher population densities (Marcogliese, 2001; Lõhmus and Björklund, 2015; Schade et al., 2016). Furthermore, climate change may increase the virulence of pathogens, for example Vibrio spp. may demonstrate increased growth and an upregulation of virulence genes, or cause epizootic outbreaks in areas that were previously free from pathogens if disease-carrying organisms from warmer climates colonise waters at colder latitudes (Conn, 2014; Rowley et al., 2014). Historically pathogens have been known to maintain their pathogenicity when expanding. For example, the protistan oyster pathogen Perkinsus marinus first expanded northwards in the United States in the early 1990s and was well established in the new range by 1996–97, suggesting that range expansion had not limited its prevalence, infection capacity or proliferation (Ford and Smolowitz, 2001). Although rising temperatures are posited to be favourable to parasites, it is important to note that parasites with complex life-cycles may be adversely affected, as if secondary or definitive hosts breach their environmental thresholds for survival and undergo mortality events this will in turn negatively affect parasite abundance (Byers, 2020).
In the marine environment, the synergistic effects of global warming and ocean acidification are forecast as two of the primary threats to bivalve health (Soon and Zheng, 2019). Mackenzie et al. (2014) investigated the effects of these coinciding stressors, acidification and warming, on the blue mussel M. edulis in a laboratory setting and confirmed that exposure to these conditions led to altered host pathological conditions, in addition to changes in parasite diversity and prevalence. Species-specific environmental pH tolerances may alter previously stable parasite–host relationships, as either host or parasite may prove more susceptible to the stressors associated with acidification. For example, trematode cercarial longevity and metacercarial survival may be reduced in acidified water (MacLeod and Poulin, 2015). It is therefore important for future studies to measure the response of both host and parasite to acidification, particularly if the host is molluscan, as that acidification affects the ability of molluscs to lay down calcium carbonate, potentially imposing a further stress on the host (MacLeod and Poulin, 2015).
From an aquaculture perspective, warming temperatures may facilitate the spread of bacterial and viral diseases associated with bivalve mortality events. Vibrio species are ubiquitous in the aquatic environment and may become more prevalent in the context of a warming climate, as higher temperature enhances Vibrio growth. This may impact bivalve culture, as species including V. aestuarianus and V. splendidus are linked to mortalities of C. gigas in western Europe (Vezzulli et al., 2013). Mortality events have also been linked to outbreaks of ostreid herpesvirus OsHV-1, variants of which have spread through Australia, New Zealand and Asia, in addition to Europe (Pernet et al., 2016). Mortensen et al. (2016) recorded high and sudden mortalities of C. gigas associated with OsHV-1 μVar in both a hatchery in Sweden and wild populations in its expanded Scandinavian range. This study represented the first time this variant was detected in Scandinavia and demonstrates how range extension of bivalve species, whether deliberately for aquaculture purposes or via the establishment of wild populations, can facilitate the spread of disease. The ostreid herpesvirus OsHV-1 is lacking in host-specificity, meaning that it can also be found in scallops and clams including the invasive R. philippinarum (Ben-Horin et al., 2015), as is the microvariant which can be found in Mytilus spp. (O’Reilly et al., 2018) and C. maenas (Bookelaar et al., 2018), and this could further extend dispersal capability.
Temperature-based surveillance models have been used to model disease outbreaks and, given the sensitivity of many parasite–host systems to temperature change, may be useful for predicting the spread of bivalve diseases. Maynard et al. (2016) tested temperature modelling as a disease surveillance technique by modelling the maximum monthly mean ocean floor temperature (using 12°C as the threshold under which outbreaks are minimised) and using it to predict epizootic shell disease (ESD) in American lobster Homarus americanus in southern New England. The results suggested outbreaks may continue for years especially in sheltered warm water bays. They also postulated that the model may be suitable to predict the spread of bivalves and pathogens, including the spread of C. gigas/V. splendidus in western Europe. Monitoring of bivalve aquaculture farms may also present an opportunity to create a network to map disease outbreaks, similar in function to the NOAA Mussel Watch Program, an ecosystem-based approach to monitoring that measures the concentration of chemical contaminants in sediment and bivalve tissues (NOAA, 2017).
In the context of a changing environment, the impacts of invasive species and diseases may pose greater risks to bivalve aquaculture in the future. When diagnosing marine disease outbreaks, it is important to combine classic techniques such as histopathology with more modern techniques such as metagenomics (Burge et al., 2016b). However, it is equally important to trace the source and pathways of the diseases, thus creating a comprehensive understanding of parasite–host dynamics, particularly when facilitated by invasive species.
Population genetic analyses may be useful to elucidate the source and pathways of invasive parasites. For example, a recent study combined genetic sequencing of the two parasitic copepods, Mytilicola intestinalis and Mytilicola orientalis, with an in-depth literature review to identify native and invaded ranges. In the case of M. orientalis there was strong overall population differentiation between the native Japanese range and the invaded North American and European ranges, with the invasion history of this copepod species reflecting the movement of its principle host, C. gigas (Feis et al., 2019). However, although population sequencing proved effective for M. orientalis, the native range of M. intestinalis remained unclear due to a lack of population genetic structure, suggesting that for some species extrapolation of the invasion history using genetic analyses is not feasible.
Combining the fields of invasion ecology and parasitology will provide clarity on their impacts in the context of climate change. Rowley et al. (2014) noted that even with comprehensive models relating to climate change in marine systems, a lack of understanding as to how invertebrates transfer infectious diseases, for example uncertainties about the host range of pathogens (Carnegie et al., 2016), creates difficulties in understanding disease dynamics. These difficulties are exacerbated by the fact that parasite–host interactions are fluid, with a number of accepted hypotheses as to whether invasive hosts travel with parasites to a new range or whether the host escapes its native parasites. Pernet et al. (2016) highlighted the need for an integrated approach using ostreid herpesvirus OsHV-1 as a case study, and noted that a primary knowledge gap is the lack of information as to how other animals are involved in the transfer, as often host and parasite are considered in a vacuum but there is a need to consider both sources of dilution and reservoirs when developing management strategies. The need for monitoring of both farmed and wild hosts has also been highlighted, in an effort to quantify the exchange between cultured stocks and the surrounding wildlife, as this may provide valuable information on the extent of disease transmission (Bouwmeester et al., 2020).
When coupled with the environmental stressors of warming and acidification, the impacts of invasive species and parasites may make bivalve species more susceptible to infection and disease. This has implications for the aquaculture sector and as such merits further discussion, particularly if commercial bivalves are purposely moved to new locations thus creating a vector for cohabiting invasive species to also move (McKindsey et al., 2007). Ensuring that relevant legislation is accessible and consistent is a vital management aspect when considering the impacts of invasive species (Shannon et al., 2020). Furthermore, if bivalve movements are increased to meet growing consumer demand, this necessitates augmented monitoring by management bodies to enhance biosecurity measures to ensure only the commercial target species is shipped and prevent the movement of associated invasive species. Strict implementation of the ICES Code of Practice on the Introduction and Transfers of Marine Organisms will minimise transfer of invasive species (Figure 2). This protocol requires that before an introduction occurs the species undergo quarantine in the recipient region, and organisms that are to be released require documented examinations, including microscopic inspection, to confirm that there are no associated invasives (ICES, 2005). Additionally, David and Loveday (2018) highlighted global trade routes and the continuous movement of species as a factor in cryptic species dispersal and suggested that tracking the movement of shellfish consignments and investigating their associated cohabiting species is a feasible mechanism to monitor the movement of potentially invasive species.
One issue relating to invasive species and parasites in the marine environment is that their presence often goes undetected for long periods of time, and as such it is difficult to determine the mode and timing of arrival. This can in turn lead to uncertainty as to whether disease outbreaks arise from new introductions or just changes in the environmental conditions (Pagenkopp Lohan et al., 2020). Epizootics of previously undocumented parasites should not instantly be considered exotic parasites, because it is possible they were present at a low prevalence but may be able to infect established invasive hosts and reach the required prevalence to be pathogenic (Torchin et al., 2002).
A useful mechanism to determine the current health status of populations is the collection of baseline data in potentially sensitive regions, for example potential aquaculture sites or coastal areas vulnerable to climate change. This will ensure managers and policy-makers fully understand the current breadth of host and parasite species and alleviate any future uncertainty about the timing of emerging diseases. One such baseline study, conducted in Patagonia, sampled both wild and cultured populations of the edible mussels Mytilus platensis and Mytilus chilensis. The study detected a number of parasites including prokaryote inclusions, protozoa and metazoa but concluded that none currently pose a problem to the industry. However, the benefits of conducting such a survey were also evident as there was a low incidence of the cancerous disease disseminated neoplasia present, and the authors stressed this required further monitoring (Vázquez et al., 2020).
Horizon scanning of future potential invasive species and pathogens, along with identification of new methods of detection, management and control acts as an early warning system, which can inform management strategies to prevent or mitigate future introductions (Dunn and Hatcher, 2015; Lucy et al., 2020). In the context of bivalve aquaculture, regional management could entail maintaining watch lists of species with known deleterious impacts, monitoring potential pathways of introduction and networks of connectivity, eliminating vectors of introduction for hitchhiking species, for example biological packaging material (Haska et al., 2012), and engaging in citizen science to augment observer numbers as early intervention is key to preventing species establishments. Documenting the environmental tolerance range of invasive species and their parasites may also provide clarity on which environments outside their native range are most at risk, i.e., are most suitable, with both abiotic and biotic drivers present to facilitate successful establishment. Furthermore, an increased effort and focus on the screening of invasive species, both in their native and invaded range, will also provide clarity on co-invasions of hosts and their parasites. This may then be extended to investigate transmission between invasive and native hosts, and further describe aspects of disease-cycling in the marine environment.
All authors conceived the study, provided feedback on early drafts, and approved the manuscript for final submission.
This work was supported by the Bluefish Project (Grant Agreement No. 80991), part-funded by the European Regional Development Fund (ERDF) through the Ireland Wales Co-operation Programme. A cross-border programme investing in the overall economic, environmental and social well-being of Ireland and Wales.
The authors declare that the research was conducted in the absence of any commercial or financial relationships that could be construed as a potential conflict of interest.
Anderson, R. M., and May, R. M. (1979). Population biology of infectious diseases: part I. Nature 280, 361–367. doi: 10.1038/280361a0
Arzul, I., Gagnaire, B., Bond, C., Chollet, B., Morga, B., Ferrand, S., et al. (2009). Effects of temperature and salinity on the survival of Bonamia ostreae, a parasite infecting flat oysters Ostrea edulis. Dis. Aquat. Organ. 85, 67–75. doi: 10.3354/dao02047
Beaury, E. M., Fusco, E. J., Jackson, M. R., Laginhas, B. B., Morelli, T. L., Allen, J. M., et al. (2020). Incorporating climate change into invasive species management: insights from managers. Biol. Invasions 22, 233–252. doi: 10.1007/s10530-019-02087-6
Ben-Horin, T., Bidegain, G., Huey, L., Narvaez, D. A., and Bushek, D. (2015). Parasite transmission through suspension feeding. J. Invertebr. Pathol. 131, 155–176. doi: 10.1016/j.jip.2015.07.006
Bishop, M. J., Carnegie, R. B., Stokes, N. A., Peterson, C. H., and Burreson, E. M. (2006). Complications of a non-native oyster introduction: facilitation of a local parasite. Mar. Ecol. Prog. Ser. 325, 145–152. doi: 10.3354/meps325145
Blakeslee, A. M. H., Barnard, R. B., Matheson, K., and McKenzie, C. H. (2020). Host-switching among crabs: species introduction results in a new target host for native parasites. Mar. Ecol. Prog. Ser. 636, 91–106. doi: 10.3354/meps13214
Blakeslee, A. M. H., Fowler, A. E., and Keogh, C. L. (2013). Marine invasions and parasite escape: updates and new perspectives. Adv. Mar. Biol. 66, 87–169. doi: 10.1016/B978-0-12-408096-6.00002-X
Blasco-Costa, I., and Poulin, R. (2017). Parasite life-cycle studies: a plea to resurrect an old parasitological tradition. J. Helminthol. 91, 647–656. doi: 10.1017/s0022149x16000924
Bookelaar, B., O’Reilly, A. J., Lynch, S. A., and Culloty, S. C. (2018). Role of the intertidal predatory shore crab Carcinus maenas in transmission dynamics of ostreid herpesvirus-1 microvariant. Dis. Aquat. Organ. 130, 221–233. doi: 10.3354/dao03264
Bouwmeester, M. M., Goedknegt, M. A., Poulin, R., and Thieltges, D. (2020). Collateral diseases: aquaculture impacts on wildlife infections. J. Appl. Ecol. 1–12. doi: 10.1111/1365-2664.13775
Burge, C. A., Closek, C. J., Friedman, C. S., Groner, M. L., Jenkins, C. M., Shore-Maggio, A., et al. (2016a). The use of filter-feeders to manage disease in a changing world. Integr. Comp. Biol. 56, 537–587. doi: 10.1093/icb/icw048
Burge, C. A., Friedman, C. S., Getchell, R., House, M., Lafferty, K. D., Mydlarz, L. D., et al. (2016b). Complementary approaches to diagnosing marine diseases: a union of the modern and the classic. Philos. T. R. Soc. B 371:20150207. doi: 10.1098/rstb.2015.0207
Burge, C. A., Eakin, C. M., Friedman, C. S., Froelich, B., Hershberger, P. K., Hofmann, E. E., et al. (2014). Climate change influences on marine infectious diseases: implications for management and society. Annu. Rev. Mar. Sci. 6, 249–277. doi: 10.1146/annurev-marine-010213-135029
Burreson, C. A., Stokes, N. A., and Carnegie, R. (2004). Bonamia sp. (Haplosporidia) found in nonnative oysters Crassostrea ariakensis in Bogue Sound. North Carolina. J. Aquat. Anim. Health 16, 1–9. doi: 10.1577/H03-008.1
Burreson, E. M., and Stokes, N. A. (2000). Increased Virulence in an Introduced Pathogen: Haplosporidium nelsoni (MSX) in the Eastern Oyster Crassostrea virginica. J. Aquat. Anim. Health 12, 1–8. doi: 10.1577/1548-8667(2000)012<0001:IVIAIP>2.0.CO;2
Byers, J. E. (2020). Marine Parasites and Disease in the Era of Global Climate Change. Ann, Rev. Mar. Sci. 13, 397–420. doi: 10.1146/annurev-marine-031920-100429
CABI (2020a). Mytilicola Intestinalis. Avaliable at: https://www.cabi.org/isc/datasheet/73758. (accessed 12/08/2020).
CABI (2020b). Myicola Ostreae. Avaliable at: https://www.cabi.org/isc/datasheet/110220. (accessed 12/08/2020).
Callaway, R., Shinn, A. P., Grenfell, S. E., Bron, J. E., Burnell, G., Cook, E. L., et al. (2012). Review of climate change impacts on marine aquaculture in the UK and Ireland. Aquat. Conserv. 22, 389–421. doi: 10.1002/Aqc.2247
Canning-Clode, J., and Carlton, J. T. (2017). Refining and expanding global climate change scenarios in the sea: poleward creep complexities, range termini, and setbacks and surges. Divers. Distrib. 23, 463–473. doi: 10.1111/ddi.12551
Canning-Clode, J., Fowler, A. E., Byers, J. E., Carlton, J. T., and Ruiz, G. M. (2011). ‘Caribbean Creep’ chills out: climate change and marine invasive species. PLoS One 6:e29657. doi: 10.1371/journal.pone.0029657
Carnegie, R. B., Arzul, I., and Bushek, D. (2016). Managing marine mollusc diseases in the context of regional and international commerce: policy issues and emerging concerns. Philos. T. R. Soc. B 371:20150215. doi: 10.1098/rstb.2015.0215
Carnegie, R. B., Stokes, N. A., Audemard, C., Bishop, M. J., Wilbur, A. E., Alphin, T. D., et al. (2008). Strong seasonality of Bonamia sp. infection and induced Crassostrea ariakensis mortality in bogue and masonboro sounds. North Carolina, USA. J. Invertebr. Pathol. 98, 335–343. doi: 10.1016/j.jip.2008.03.009
Carrasco, N., Green, T., and Itoh, N. (2015). Marteilia spp. parasites in bivalves: a revision of recent studies. J. Invertebr. Pathol. 131, 43–57. doi: 10.1016/j.jip.2015.07.016
Chalkowski, K., Lepczyk, C. A., and Zohdy, S. (2018). Parasite ecology of invasive species: conceptual framework and new hypotheses. Trends Parasitol. 34, 655–663. doi: 10.1016/j.pt.2018.05.008
Cheng, T. C., and Combes, C. (1990). “Influence of environmental factors on the invasion of molluscs by parasites: with special reference to Europe,” in Biological Invasions in Europe and the Mediterranean Basin, eds F. di Castri, A. J. Hansen, and M. Debussche (Dordrecht: Kluwer Academic Publishers), 307–332.
Coen, L. D., and Bishop, M. (2015). The ecology, evolution, impacts and management of host–parasite interactions of marine molluscs. J. Invertebr. Pathol. 131, 177–211. doi: 10.1016/j.jip.2015.08.005
Colautti, R. I., Ricciardi, A., Grigorovich, I. A., and MacIsaac, H. J. (2004). Is invasion success explained by the enemy release hypothesis? Ecol. Lett. 7, 721–733. doi: 10.1111/j.1461-0248.2004.00616.x
Conn, D. B. (2014). Aquatic invasive species and emerging infectious disease threats: a one health perspective. Aquat. Invasions 9, 383–390. doi: 10.3391/ai.2014.9.3.12
Cordero, D., Delgado, M., Liu, B., Ruesink, J., and Saavedra, C. (2017). Population genetics of the Manila clam (Ruditapes philippinarum) introduced in North America and Europe. Sci. Rep. 7:39745. doi: 10.1038/srep39745
Costello, K. E., Lynch, S. A., McAllen, R., O’Riordan, R. M., and Culloty, S. C. (2020). The role of invasive tunicates as reservoirs of molluscan pathogens. Biol. Invasions 23, 641–655. doi: 10.1007/s10530-020-02392-5
Crowl, T. A., Crist, T. O., Parmenter, R. R., Belovsky, G., and Lugo, A. E. (2008). The spread of invasive species and infectious disease as drivers of ecosystem change. Front. Ecol. Environ. 6:238–246. doi: 10.1890/070151
Dang, C., de Montaudouin, X., Bald, J., Jude, F., Raymond, N., Lanceleur, L., et al. (2009). Testing the enemy release hypothesis: trematode parasites in the non-indigenous Manila clam Ruditapes philippinarum. Hydrobiologia 630, 139–148. doi: 10.1007/s10750-009-9786-9
David, A. A., and Loveday, B. R. (2018). The role of cryptic dispersal in shaping connectivity patterns of marine populations in a changing world. J. Mar. Biol. Assoc. U. K. 98, 647–655. doi: 10.1017/S0025315417000236
David, A. A., Matthee, C. A., Loveday, B. R., and Simon, C. A. (2016). Predicting the dispersal potential of an invasive polychaete pest along a complex coastal biome. Integr. Comp. Biol. 56, 600–610. doi: 10.1093/icb/icw011
Demann, F., and Wegner, K. M. (2019). Infection by invasive parasites increases susceptibility of native hosts to secondary infection via modulation of cellular immunity. J. Anim. Ecol. 88, 427–438. doi: 10.1111/1365-2656.12939
Diez, J. M., D’Antonio, C. M., Dukes, J. S., Grosholz, E. D., Olden, J. D., Sorte, C. J. B., et al. (2012). Will extreme climatic events facilitate biological invasions? Front. Ecol. Environ. 10:249–257. doi: 10.1890/110137
Dunn, A. M., and Hatcher, M. J. (2015). Parasites and biological invasions: parallels, interactions, and control. Trends. Parasitol. 31, 189–199. doi: 10.1016/j.pt.2014.12.003
Dunn, A. M., Torchin, M. E., Hatcher, M. J., Kotanen, P. M., Blumenthal, D. M., Byers, J. E., et al. (2012). Indirect effects of parasites in invasions. Funct. Ecol. 26, 1262–1274. doi: 10.1111/j.1365-2435.2012.02041.x
Ďuriš, Z., Horká, I., Juračka, P. J., Petrusek, A., and Sanford, F. (2011). These squatters are not innocent: the evidence of parasitism in sponge-inhabiting shrimps. PLoS One 6:e21987. doi: 10.1371/journal.pone.0021987
Elsner, N. O., Jacobsen, S., Thieltges, D. W., and Reise, K. (2011). Alien parasitic copepods in mussels and oysters of the Wadden Sea. Helgol. Mar. Res. 65, 299–307. doi: 10.1007/s10152-010-0223-2
EU regulation 1146 (2014). On Invasive Alien Species. Avaliable at: https://eur-lex.europa.eu/legal-content/EN/TXT/PDF/?uri=CELEX:32014R1143&rid=5. (accessed 29/01/2021).
Evans, O., Paul-Pont, I., Hick, P., and Whittington, R. J. (2014). A simple centrifugation method for improving the detection of Ostreid herpesvirus-1 (OsHV-1) in natural seawater samples with an assessment of the potential for particulate attachment. J. Virol. Methods 210, 59–66. doi: 10.1016/j.jviromet.2014.09.023
FAO (2018). The State of World Fisheries and Aquaculture 2018 - Meeting the Sustainable Development Goals. Rome: FAO. Licence: CC BY-NC-SA 3.0 IGO.
Feis, M. E., Goedknegt, M. A., Arzul, I., Chenuil, A., den Boon, O., Gottschalck, L., et al. (2019). Global invasion genetics of two parasitic copepods infecting marine bivalves. Sci. Rep. 9:12730. doi: 10.1038/s41598-019-48928-1
Fernández-Robledo, J. A., Vasta, G. R., and Record, N. R. (2014). Protozoan parasites of bivalve molluscs: literature follows culture. PLoS One 9:e100872. doi: 10.1371/journal.pone.0100872
Flannery, G., Lynch, S. A., and Culloty, S. C. (2016). Investigating the significance of the role of Ostrea edulis larvae in the transmission and transfer of Bonamia ostreae. J. Invertebr. Pathol. 136, 7–9. doi: 10.1016/j.jip.2016.02.001
Ford, S. E., Allam, B., and Xu, Z. (2009). Using bivalves as particle collectors with PCR detection to investigate the environmental distribution of Haplosporidium nelsoni. Dis. Aquat. Organ. 83, 159–168. doi: 10.3354/dao02018
Ford, S. E., and Smolowitz, R. (2001). Infection dynamics of an oyster parasite in its newly expanded range. Mar. Biol. 151, 119–133. doi: 10.1007/s00227-006-0454-6
Gallana, M., Ryser-Degiorgis, M.-P., Wahli, T., and Segner, H. (2013). Climate change and infectious diseases of wildlife: altered interactions between pathogens, vectors and hosts. Curr. Zool. 59, 427–437. doi: 10.1093/czoolo/59.3.427
Gallardi, D. (2014). Effects of bivalve aquaculture on the environment and their possible mitigation: a review. Fish. Aqu. J.. 5, 1–8.
Goedknegt, M. A., Buschbaum, C., van der Meer, J., Wegner, K. M., and Thieltges, D. W. (2020). Introduced marine ecosystem engineer indirectly affects parasitism in native mussel hosts. Biol. Invasions 22, 3223–3237. doi: 10.1007/s10530-020-02318-1
Goedknegt, M. A., Feis, M. E., Wegner, K. M., Luttikhuizen, P. C., Buschbaum, C., Camphuysen, C. J., et al. (2016). Parasites and marine invasions: ecological and evolutionary perspectives. J. Sea Res. 113, 11–27. doi: 10.1016/j.seares.2015.12.003
Goedknegt, M. A., Nauta, R., Markovic, M., Buschbaum, C., Folmer, E. O., Luttikhuizen, P. C., et al. (2019). How invasive oysters can affect parasite infection patterns in native mussels on a large spatial scale. Oecologia 190, 99–113. doi: 10.1007/s00442-019-04408-x
Goedknegt, M. A., Schuster, A. K., Buschbaum, C., Gergs, R., Jung, A. S., Luttikhuizen, P. C., et al. (2017). Spillover but no spillback of two invasive parasitic copepods from invasive Pacific oysters (Crassostrea gigas) to native bivalve hosts. Biol. Invasions 19, 365–379. doi: 10.1007/s10530-016-1285-0
Gollasch, S., Kerckhof, F., Craeymeersch, J., Goulletquer, Jensen, K., Jelmert, A., et al. (2015). Alien Species Alert: Ensis directus. Current Status of Invasions by the Marine Bivalve Ensis Directus. 1–32. ICES Cooperative Research Report. No. 323.
Grosholz, E. D., Crafton, R. E., Fontana, R. E., Pasari, J. R., Williams, S. L., and Chabin, C. J. (2015). Aquaculture as a vector for marine invasions in California. Biol. Invasions 17, 1471–1484. doi: 10.1007/s10530-014-0808-9
Hanley, T. C., White, J. W., Stallings, C. D., and Kimbro, D. L. (2019). Environmental gradients shape the combined effects of multiple parasites on oyster hosts in the northern Gulf of Mexico. Mar. Ecol. Prog. Ser. 612, 111–125. doi: 10.3354/meps12849
Harvell, C. D., Mitchell, C. E., Ward, J. R., Altizer, S., Dobson, A. P., Ostfeld, R. S., et al. (2002). Climate warming and disease risks for terrestrial and marine biota. Science 296, 2158–2162. doi: 10.1126/science.1063699
Haska, C. L., Yarish, C., Kraemer, G., Blaschik, N., Whitlatch, R., Zhang, H., et al. (2012). Bait worm packaging as a potential vector of invasive species. Biol. Invasions 14, 481–493. doi: 10.1007/s10530-011-0091-y
Hellmann, J. J., Byers, J. E., Bierwagen, B. G., and Dukes, J. S. (2008). Five potential consequences of climate change for invasive species. Conserv. Biol. 22, 534–543. doi: 10.1111/j.1523-1739.2008.00951.x
Holt, J., Wakelin, S., Lowe, J., and Tinker, J. (2010). The potential impacts of climate change on the hydrography of the northwest European continental shelf. Prog. Oceanogr. 86, 361–379. doi: 10.1016/j.pocean.2010.05.003
Hulme, P. E. (2009). Trade, transport and trouble: managing invasive species pathways in an era of globalization. J. Appl. Ecol. 46, 10–18. doi: 10.1111/j.1365-2664.2008.01600.x
Hulme, P. E. (2015). Invasion pathways at a crossroad: policy and research challenges for managing alien species introductions. J. Appl. Ecol. 52, 1418–1424. doi: 10.1111/1365-2664.12470
ICES (2005). ICES Code of Practice on the Introductions and Transfers of Marine Organisms 2005. Burnaby: ICES, 1–30.
IPCC (2019). “IPCC 2019: summary for policymakers,” in IPCC Special Report on the Ocean and Cryosphere in a Changing Climate, eds H.-O. Pörtner, D. C. Roberts, V. Masson-Delmotte, P. Zhai, M. Tignor, E. Poloczanska, et al. (Geneva: IPCC), 1–36.
Katsanevakis, S., Tempera, F., and Teixeira, H. (2016). Mapping the impact of alien species on marine ecosystems: the Mediterranean Sea case study. Divers. Distrib. 22, 694–707. doi: 10.1111/ddi.12429
Katsanevakis, S., Tsirintanis, K., Tsaparis, D., Doukas, D., Sini, M., Athanassopoulou, F., et al. (2019). The cryptogenic parasite Haplosporidium pinnae invades the Aegean Sea and causes the collapse of Pinna nobilis populations. Aquat. Invasions 14, 150–164. doi: 10.3391/ai.2019.14.2.01
Kelly, D. W., Paterson, R. A., Townsend, C. R., Poulin, R., and Tompkins, D. M. (2009). Parasite spillback: a neglected concept in invasion ecology? Ecology 90, 2047–2056. doi: 10.1890/08-1085.1
Kerr, R., Ward, G. M., Stentiford, G. D., Alfjorden, A., Mortensen, S., Bignell, J. P., et al. (2018). Marteilia refringens and Marteilia pararefringens sp. nov. are distinct parasites of bivalves and have different European distributions. Parasitology 145, 1483–1492. doi: 10.1017/S003118201800063X
Krakau, M., Thieltges, D. W., and Reise, K. (2006). Native parasites adopt introduced bivalves of the North Sea. Biol. Invasions 8, 919–925. doi: 10.1007/s10530-005-4734-8
Laeseke, P., Schiller, J., Letschert, J., and Doolittle Llanos, S. (2020). “Theories, vectors, and computer models: marine invasion science in the anthropocene,” in YOUMARES 9 - The Oceans: Our Research, Our Future, eds S. Jungblut, V. Liebich, and M. Bode-Dalby (Cham: Springer), 195–209.
Lafferty, K. D., and Hofmann, E. E. (2016). Marine disease impacts, diagnosis, forecasting, management and policy. Philos. T. R. Soc. B 371:20150200. doi: 10.1098/rstb.2015.0200
Lafferty, K. D., and Kuris, A. M. (1999). How environmental stress affects the impacts of parasites. Limnol. Oceanog. 44, 925–931. doi: 10.4319/lo.1999.44.3_part_2.0925
Lagrue, C. (2017). Impacts of crustacean invasions on parasite dynamics in aquatic ecosystems: a plea for parasite-focused studies. Int. J. Parasitol. Parasites Wildl. 6, 364–374. doi: 10.1016/j.ijppaw.2017.03.008
Lattos, A., Giantsis, I. A., Karagiannis, D., and Michaelidis, B. (2020). First detection of the invasive haplosporidian and mycobacteria parasites hosting the endangered bivalve Pinna nobilis in Thermaikos Gulf. North Greece. Mar. Environ. Res. 155:104889. doi: 10.1016/j.marenvres.2020.104889
Le, T. C., Kang, H.-S., Hong, H.-K., Park, K.-J., and Choi, K.-S. (2015). First report of Urosporidium sp., a haplosporidian hyperparasite infecting digenean trematode Parvatrema duboisi in Manila clam, Ruditapes philippinarum on the west coast of Korea. J. Invertebr. Pathol. 130, 141–146. doi: 10.1016/j.jip.2015.08.004
Lodge, D. M., Williams, S., MacIsaac, H. J., Hayes, K. R., Leung, B., Reichard, S., et al. (2006). Biological invasions: recommendations for U.S. policy and management. Ecol. Appl. 16, 2035–2054.
Lõhmus, M., and Björklund, M. (2015). Climate change: what will it do to fish – parasite interactions? Biol. J. Linn. Soc. 116, 397–411. doi: 10.1111/bij.12584
Lucy, F. E., Davis, E., Anderson, R., Booy, O., Bradley, K., Britton, J. R., et al. (2020). Horizon scan of invasive alien species for the island of Ireland. Manag. Biol. Invasion 11, 155–177. doi: 10.3391/mbi.2020.11.2.01
Lynch, S. A., Armitage, D. V., Coughlan, J., Mulcahy, M. F., and Culloty, S. C. (2007). Investigating the possible role of benthic macroinvertebrates and zooplankton in the life cycle of the haplosporidian Bonamia ostreae. Exp. Parasitol. 115, 359–368. doi: 10.1016/j.exppara.2006.09.021
Lynch, S. A., Coghlan, A., O’Leary, B., Morgan, E., and Culloty, S. (2020). Northward establishment of the mediterranean mussel Mytilus galloprovincialis limited by changing climate. Biol. Invasions 22, 2725–2736. doi: 10.1007/s10530-020-02294-6
Macey, B. M., Achilihu, I. O., Burnett, K. G., and Burnett, L. E. (2008). Effects of hypercapnic hypoxia on inactivation and elimination of Vibrio campbellii in the eastern oyster, Crassostrea virginica. Appl. Environ. Microbiol. 74, 6077–6084. doi: 10.1128/AEM.00317-08
Mackenzie, C. L., Lynch, S. A., Culloty, S. C., and Malham, S. K. (2014). Future oceanic warming and acidification alter immune response and disease status in a commercial shellfish species, Mytilus edulis L. PLoS One 9:e99712. doi: 10.1371/journal.pone.0099712
MacLeod, C. D., and Poulin, R. (2015). Differential tolerances to ocean acidification by parasites that share the same host. Int. J. Parasitol. 45, 485–493. doi: 10.1016/j.ijpara.2015.02.007
Marcogliese, D. J. (2001). Implications of climate change for parasitism of animals in the aquatic environment. Can. J. Zool. 79, 1331–1352. doi: 10.1139/cjz-79-8-1331
Marcogliese, D. J. (2008). The impact of climate change on the parasites and infectious diseases of aquatic animals. Rev. Sci. Tech. 27, 467–484.
Maynard, J., van Hooidonk, R., Harvell, C. D., Eakin, C. M., Liu, G., Willis, B. L., et al. (2016). Improving marine disease surveillance through sea temperature monitoring, outlooks and projections. Philos. T. R. Soc. B 379:20150208. doi: 10.1098/rstb.2015.0208
McKindsey, C. W., Landry, T., O’Beirn, F. X., and Davies, I. M. (2007). Bivalve aquaculture and exotic species: a review of ecological considerations and management issues. J. Shellfish Res. 26, 281–294.
Mellin, C., Lurgi, M., Matthews, S., MacNeil, M. A., Caley, M. J., Bax, N., et al. (2016). Forecasting marine invasions under climate change: Biotic interactions and demographic processes matter. Biol. Conserv. 204, 459–467. doi: 10.1016/j.biocon.2016.11.008
Miller, A., Inglis, G. J., and Poulin, R. (2008). Use of the introduced bivalve, Musculista senhousia, by generalist parasites of native New Zealand bivalves. New Zeal. J. Mar. Fresh. Res. 42, 143–151. doi: 10.1080/00288330809509944
Minchin, D., Gollasch, S., Cohen, A. N., Hewitt, C. L., and Olenin, S. (2009). “Characterizing vectors of marine invasion,” in Biological Invasions in Marine Ecosystems. Ecological Studies (Analysis and Synthesis), Vol. 204, eds G. Rilov and J. A. Crooks (Berlin: Springer), 109–116.
Morley, N. J. (2010). Interactive effects of infectious diseases and pollution in aquatic molluscs. Aquat. Toxicol. 96, 27–36. doi: 10.1016/j.aquatox.2009.09.017
Mortensen, S., Strand, Å, Bodvin, T., Alfjorden, A., Skår, C. K., Jelmert, A., et al. (2016). Summer mortalities and detection of ostreid herpesvirus microvariant in Pacific oyster Crassostrea gigas in Sweden and Norway. Dis. Aquat. Organ. 117, 171–176. doi: 10.3354/dao02944
Muehlbauer, F., Fraser, D., Brenner, M., Van Nieuwenhove, K., Buck, B. H., Strand, O., et al. (2014). Bivalve aquaculture transfers in Atlantic Europe. Part a: transfer activities and legal framework. Ocean Coast. Manag. 89, 127–138. doi: 10.1016/j.ocecoaman.2013.12.003
Nawrot, R., Albano, P. G., Chattopadhyay, D., and Zuschin, M. (2017). Climate change and body size shift in Mediterranean bivalve assemblages: unexpected role of biological invasions. Proc. Royal Soc. B 284:20170357. doi: 10.1098/rspb.2017.0357
Naylor, R. L., Williams, S. L., and Strong, D. R. (2001). Aquaculture – A gateway for exotic species. Science 294, 1655–1656. doi: 10.1126/science.1064875
NOAA (2017). NOAA. Avaliable at: https://coastalscience.noaa.gov/project/mussel-watch-program-assessment-chesapeake-bay-charleston-harbor/. (accessed January 10, 2021).
O’Reilly, A. J., Laide, C., Maloy, A., Hutton, S., Bookelaar, B., O’Sullivan, K., et al. (2018). The role of the mussel Mytilus spp. in the transmission of ostreid herpesvirus-1 microVar. Parasitology 145, 1095–1104. doi: 10.1017/S0031182017002244
Pagenkopp Lohan, K. M., Ruiz, G. M., and Torchin, M. E. (2020). “Invasions can drive marine disease dynamics,” in Marine Disease Ecology, eds D. C. Behringer, B. R. Silliman, and K. D. Lafferty (Oxford: Oxford University Press), 115–138.
Paillard, C. (2004). A short-review of brown ring disease, a vibriosis affecting clams, Ruditapes philippinarum and Ruditapes decussatus. Aquat. Living Resour. 17, 467–475. doi: 10.1051/alr:2004053
Park, K.-I., Paillard, C., le Chevalier, P., and Choi, K.-S. (2006). Report on the occurrence of brown ring disease (BRD) in Manila clam, Ruditapes philippinarum, on the west coast of Korea. Aquaculture 255, 610–613. doi: 10.1016/j.aquaculture.2005.12.011
Peeler, E. J., Oidtmann, B. C., Midtlyng, P. J., Miossec, L., and Gozlan, R. E. (2011). Non-native aquatic animals introductions have driven disease emergence in Europe. Biol. Invasions 13, 1291–1303. doi: 10.1007/s10530-010-9890-9
Pernet, F., Lupo, C., Bacher, C., and Whittington, R. J. (2016). Infectious diseases in oyster aquaculture require a new integrated approach. Philos. T. R. Soc. B 371:20150213. doi: 10.1098/rstb.2015.0213
Pierce, M. L., and Ward, J. E. (2018). Microbial ecology of the bivalvia, with an emphasis on the family ostreidae. J. Shellfish Res. 37, 793–806. doi: 10.2983/035.037.0410
Poulin, R. (2017). Invasion ecology meets parasitology: advances and challenges. Int. J. Parasitol. Parasites Wildl. 6, 361–363. doi: 10.1016/j.ijppaw.2017.03.006
Poulin, R., Paterson, R. A., Townsend, C. R., Tompkins, D. M., and Kelly, D. W. (2011). Biological invasions and the dynamics of endemic diseases in freshwater ecosystems. Freshw. Biol. 56, 676–688. doi: 10.1111/j.1365-2427.2010.02425.x
Rech, S., Salmina, S., Borrell Pichs, Y. J., and García-Vazquez, E. (2018). Dispersal of alien invasive species on anthropogenic litter from European mariculture areas. Mar. Pollut. Bull. 131, 10–16. doi: 10.1016/j.marpolbul.2018.03.038
Rowley, A. F., Cross, M. E., Culloty, S. C., Lynch, S. A., Mackenzie, C. L., Morgan, E., et al. (2014). The potential impact of climate change on the infectious diseases of commercially important shellfish populations in the Irish Sea - A review. ICES J. Mar. Sci. 71, 741–759. doi: 10.1093/icesjms/fst234
Ruesink, J. L., Lenihan, H. S., Trimble, A. C., Heiman, K. W., Micheli, F., Byers, J. E., et al. (2005). Introduction of non-native oysters: ecosystem effects and restoration implications. Annu. Rev. Ecol. Evol. Syst. 36, 643–689. doi: 10.1146/annurev.ecolsys.36.102003.152638
Schade, F. M., Raupach, M. J., and Wegner, K. M. (2016). Seasonal variation in parasite infection patterns of marine fish species from the Northern Wadden Sea in relation to interannual temperature fluctuations. J. Sea Res. 113, 73–84. doi: 10.1016/j.seares.2015.09.002
Shannon, C., Quinn, C. H., Dunn, A. M., and Stebbing, P. D. (2020). Coherence of marine alien species biosecurity legislation: a study of England and Wales. Mar. Pollut. Bull. 161:111796. doi: 10.1016/j.marpolbul.2020.111796
Smolowitz, R. (2013). A review of current state of knowledge concerning Perkinsus marinus effects on Crassostrea virginica (Gmelin) (the Eastern Oyster). Vet. Pathol. 50, 404–411. doi: 10.1177/0300985813480806
Solomieu, V. B., Renault, T., and Travers, M.-A. (2015). Mass mortality in bivalves and the intricate case of the Pacific oyster, Crassostrea gigas. J. Invertebr. Pathol. 131, 2–10. doi: 10.1016/j.jip.2015.07.011
Soon, T. K., and Zheng, H. (2019). Climate change and bivalve mass mortality in temperate regions. Rev. Environ. Contam. Toxicol. 251, 109–129. doi: 10.1007/398_2019_31
Sorte, C. J. B., Ibáñez, I., Blumenthal, D. M., Molinari, N. A., Miller, L. P., Grosholz, E. D., et al. (2013). Poised to prosper? A cross-system comparison of climate change effects on native and non-native species performance. Ecol. Lett. 16, 261–270. doi: 10.1111/ele.12017
Steeves, L. E., Filgueira, R., Guyondet, T., Chassé, J., and Comeau, L. (2018). Past, present, and future: performance of two bivalve species under changing environmental conditions. Front. Mar. Sci. 5:184. doi: 10.3389/fmars.2018.00184
Telfer, S., and Bowen, K. (2012). The effects of invasion on parasite dynamics and communities. Funct. Ecol. 26, 1288–1299. doi: 10.1111/j.1365-2435.2012.02049.x
Thieltges, D. W., Engelsma, M. Y., Wendling, C. C., and Wegner, K. M. (2013). Parasites in the Wadden Sea food web. J. Sea Res. 82, 122–133. doi: 10.1016/j.seares.2012.06.002
Thieltges, D. W., Reise, K., Prinze, K., and Jensen, K. T. (2009). Invaders interfere with native parasite–host interactions. Biol. Invasions 11, 1421–1429. doi: 10.1007/s10530-008-9350-y
Toews, S., Beverley-Burton, M., and Lawrimore, T. (1993). Helminth and protist parasites of zebra mussels, Dreissena polymorpha (Pallas, 1771), in the Great Lakes region of southwestern Ontario, with comments on associated bacteria. Can. J. Zool. 71, 1763–1766. doi: 10.1139/z93-250
Torchin, M. E., Lafferty, K. D., and Kuris, A. M. (2001). Release from parasites as natural enemies: increased performance of a globally introduced marine crab. Biol. Invasions 3, 333–345. doi: 10.1023/A:1015855019360
Torchin, M. E., Lafferty, K. D., and Kuris, A. M. (2002). Parasites and marine invasions. Parasitology 124(Suppl.), S137–S151. doi: 10.1017/S0031182002001506
Vázquez, N., Frizzera, A., and Cremonte, F. (2020). Diseases and parasites of wild and cultivated mussels along the patagonian coast of argentina, southwest atlantic ocean. Dis. Aquat. Org. 139, 139–152. doi: 10.3354/dao03467
Vezzulli, L., Colwell, R. R., and Pruzzo, C. (2013). Ocean warming and spread of pathogenic vibrios in the aquatic environment. Microb. Ecol. 65, 817–825. doi: 10.1007/s00248-012-0163-2
Vignon, M., and Sasal, P. (2010). Fish introduction and parasites in marine ecosystems: a need for information. Environ. Biol. Fishes 87, 1–8. doi: 10.1007/s10641-009-9553-9
Walther, G. R., Roques, A., Hulme, P. E., Sykes, M. T., Pyšek, P., Kühn, I., et al. (2009). Alien species in a warmer world: risks and opportunities. Trends. Ecol. Evol. 24, 686–693. doi: 10.1016/j.tree.2009.06.008
Watts, J. C., Carroll, J. M., Munroe, D. M., and Finelli, C. M. (2018). Examination of the potential relationship between boring sponges and pea crabs and their effects on eastern oyster condition. Dis. Aquat. Organ. 130, 25–36. doi: 10.3354/dao03257
Wijsman, J. W. M., Troost, K., Fang, J., and Roncarati, A. (2019). “Global production of marine bivalves. trends and challenges,” in Goods and Services of Marine Bivalves, eds A. C. Smaal, J. G. Ferreira, J. Grant, J. K. Petersen, and Ø Strand (Berlin: Springer), 7–26.
Williams, S., Davidson, I. C., Pasari, J. R., Ashton, G. V., Carlton, J. V., Crafton, R. E., et al. (2013). Managing multiple vectors for marine invasions in an increasingly connected world. BioScience 63, 952–966. doi: 10.1525/bio.2013.63.12.8
Yanagida, T., Shirakashi, S., Iwaki, T., Ikushima, N., and Ogawa, K. (2009). Gymnophallid digenean Parvatrema duboisi uses Manila clam as the first and second intermediate host. Parasitol. Int. 58, 308–310. doi: 10.1016/j.parint.2009.05.006
Keywords: invasion ecology, parasites, transmission trials, climate change, marine bivalves, management
Citation: Costello KE, Lynch SA, O’Riordan RM, McAllen R and Culloty SC (2021) The Importance of Marine Bivalves in Invasive Host–Parasite Introductions. Front. Mar. Sci. 8:609248. doi: 10.3389/fmars.2021.609248
Received: 22 September 2020; Accepted: 04 February 2021;
Published: 26 February 2021.
Edited by:
Simone Libralato, National Institute of Oceanography and Applied Geophysics, ItalyReviewed by:
Colette Wabnitz, Stanford University, United StatesCopyright © 2021 Costello, Lynch, O’Riordan, McAllen and Culloty. This is an open-access article distributed under the terms of the Creative Commons Attribution License (CC BY). The use, distribution or reproduction in other forums is permitted, provided the original author(s) and the copyright owner(s) are credited and that the original publication in this journal is cited, in accordance with accepted academic practice. No use, distribution or reproduction is permitted which does not comply with these terms.
*Correspondence: Katie E. Costello, a2F0aWVjb3N0ZWxsb0B1Y2MuaWU=
Disclaimer: All claims expressed in this article are solely those of the authors and do not necessarily represent those of their affiliated organizations, or those of the publisher, the editors and the reviewers. Any product that may be evaluated in this article or claim that may be made by its manufacturer is not guaranteed or endorsed by the publisher.
Research integrity at Frontiers
Learn more about the work of our research integrity team to safeguard the quality of each article we publish.