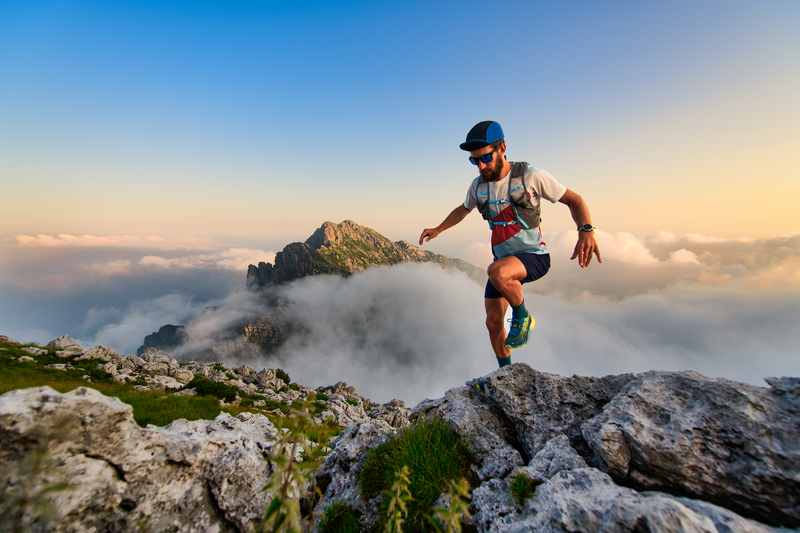
94% of researchers rate our articles as excellent or good
Learn more about the work of our research integrity team to safeguard the quality of each article we publish.
Find out more
ORIGINAL RESEARCH article
Front. Mar. Sci. , 24 March 2021
Sec. Marine Ecosystem Ecology
Volume 8 - 2021 | https://doi.org/10.3389/fmars.2021.594447
A species’ ability to spread is in part governed by the extent to which recipient habitats either resist, tolerate or promote the species’ recruitment. In disturbed marine environments, there is a general trend for the loss of algal canopies, thought to resist invasion, toward algal turf or rock barrens habitat. This study tested whether the spread of the range-expanding native macroalga Caulerpa filiformis was resisted by algal canopies but facilitated by algal turf or barrens habitats. Large-scale field surveys generally supported the predicted recruitment patterns, with C. filiformis recruits being most abundant on turf (but not barrens) and absent under kelp canopies. However, a discrepancy existed between different structural forms of turf, with the positive association holding true only for geniculate corallines, not filamentous turf. Secondly, a laboratory experiment tested whether the physical structure and/or the sediment-trapping properties of coralline turf influenced the recruitment success of C. filiformis. Whilst the structural complexity of turf aided overall recruitment performance (i.e., increased rhizoid production, attachment speed, success, and strength), a positive influence of turf-derived sediment on recruits’ growth was less obvious, at least over 10 days. The high morphological plasticity of C. filiformis propagules resulted in possible benefits of faster or stronger attachment of more developed propagules being only temporary, and that recruitment may be regulated in accordance with habitat preference. Finally, a field experiment confirmed the observed positive role of turf and the negative influence of algal canopies in the short-term, however, adverse environmental conditions in the longer-term resulted in the loss of most fragments. In conclusion, this study demonstrated the importance of both positive and negative species interactions for the recruitment success of a native alga, suggesting that a shift from kelp to turf algae can initiate further community change.
Foundation species (those that form for example coral reefs, mangrove, and kelp forests) shape the functioning of entire ecosystems (Stachowicz, 2001). They often support unique communities of high biodiversity and productivity and perform important ecosystem functions including the provision of biotic resistance to invasion (e.g., Moberg and Folke, 1999; Dugan et al., 2011; Lee et al., 2014; Bennett et al., 2016; South and Thomsen, 2016; Gribben et al., 2018; Uyà et al., 2018). However, human disturbances acting at a range of scales are eroding the resistance and resilience of foundation species, often leading to phase shifts (or alternative stable states) in which foundation species are lost or replaced by more stress-tolerant species that may differ in their functioning (Hughes et al., 2007; Norström et al., 2009; Montefalcone et al., 2015; O’Brien and Scheibling, 2018). These resulting alternative communities may then facilitate further community change (Didham et al., 2007; Tylianakis et al., 2008). While the replacement of foundation species by altered communities has received considerable attention, the follow-on consequences of altered communities driving further community change are less well understood.
Globally, marine ecosystems have experienced large losses of large canopy-forming macroalgae such as kelp (Thibaut et al., 2005; Coleman et al., 2008; Voerman et al., 2013; Thomsen et al., 2019; Wernberg et al., 2019). Overgrazing by sea urchins can result in a shift from macroalgae to structurally simpler crustose coralline or bare rock ‘barren’ habitats (Andrew and Underwood, 1993; Benedetti-Cecchi et al., 1998; Ling, 2008), that can persist for decades (Filbee-Dexter and Scheibling, 2014; Glasby and Gibson, 2020). Alternatively, the loss of macroalgae due to abiotic (e.g., increased temperature, nutrients, sedimentation) or biotic (e.g., overgrazing) disturbances frees up limiting resources such as light and space that can allow for a transition to more stress-tolerant and previously sub-dominant species. For example, loss of kelp can lead to invasive species becoming dominant on rocky reefs (Valentine and Johnson, 2003; Britton−Simmons and Abbott, 2008; Thompson and Schiel, 2012), or lead to a transition to smaller turf-forming algae (hereafter turf) (Airoldi and Beck, 2007; Connell et al., 2008; Moy and Christie, 2012; Filbee-Dexter and Wernberg, 2018; O’Brien and Scheibling, 2018; Straub et al., 2019).
Whilst turf is often regarded as an alternate stable state, it can also promote the recruitment and spread of invaders. For example, turf can promote the recruitment and spread of several invasive macroalgae including Caulerpa spp. and Codium fragile ssp. fragile (Ceccherelli et al., 2002; Watanabe et al., 2009; Gennaro and Piazzi, 2014), although this positive association does not exist for all invasive macroalgae (Arenas et al., 2006; Britton−Simmons and Abbott, 2008). Similarly, recruitment of Undaria pinnatifida can be facilitated by removal of native canopies, especially if the understory is dominated by coralline turf (Thompson and Schiel, 2012). This suggests that turf may have important positive interactions by facilitating the proliferation of invasive species. Many native algae are also spreading (e.g., Díez et al., 2012; Yesson et al., 2015), but whether they are directly (provision of resources) or indirectly (e.g., via turf) facilitated by the loss of foundation species has not been explored.
Turf habitat can enhance the recruitment of other species via several mechanisms. The physical structure of turf can enhance trapping of vegetative propagules (fragments) of invasive C. fragile (Watanabe et al., 2009) or C. cylindracea (Bulleri and Benedetti-Cecchi, 2008). The biogeochemical environment created by turf via trapping of nutrient-rich sediment may also benefit rhizoidal algae that are able to utilize increased nutrient concentrations in the sediment (Williams, 1984). We hypothesize that the physical structure will promote propagule attachment, whereas the presence of sediments will contribute to subsequent growth of recruited fragments. To date, however, no studies have parsed the relative importance of sediment properties versus turf physical attributes in facilitating spreading species.
Many of the world’s most invasive macrophytes spread vegetatively via asexual fragmentation (Wright, 2005; Williams and Smith, 2007). The recruitment success of macroalgal fragments (i.e., propagules) can be influenced by their quality (van Kleunen et al., 2015; Estrada et al., 2016), the recipient environment (Gribben et al., 2017; Gribben et al., 2018), and the interaction between the two (Bulleri et al., 2018, 2019). For example, larger, more intact fragments such as those with intact rhizoid structures generally show higher recruitment potential (Khou et al., 2007; Wu et al., 2007; Uyà et al., 2018). In addition, in disturbed seagrass habitats, nominally good (with fronds and rhizoids) and poor quality (with fronds but without rhizoids) fragments of Caulerpa taxifolia established, whereas only good quality fragments established in healthy seagrass beds (Bulleri et al., 2019). Similarly, we predict that fragments with rhizome + rhizoids structures will have higher recruitment rates and growth because of quicker attachment rates and increased ability to utilize nutrients in sediments trapped by turfs.
On shallow rocky shores in New South Wales (NSW), Australia, the native green alga C. filiformis has become locally more abundant and has spread outside its known historic distribution. Previously described as having a restricted distribution (approximately 200 km of coastline), C. filiformis has spread 500 km north of its known traditional range and is now found across > 700 km of coastline (see Glasby et al., 2015 for an overview). C. filiformis is the dominant habitat-forming species on many shallow rocky reefs along the coast, where it can form extensive monospecific stands of >1000 m2 (Glasby et al., 2015; Voerman et al., 2017). The spread of the alga can have large impacts on associated flora and invertebrate fauna (Voerman et al., 2014; Lanham et al., 2015; Voerman, 2017), with weaker effects on herbivorous fish assemblages (Bradley et al., 2018). The mechanisms behind the localized increases in abundance of the alga are unknown (Glasby et al., 2015). However, disturbance to canopies of competing algal species can facilitate the spread of established patches of C. filiformis as it is a superior competitor for space (Zhang et al., 2014), and historical losses of a large fucoid around the Sydney metropolitan may have contributed to C. filiformis’ increased abundance (Glasby et al., 2015). Moreover, sediment disturbance appears to contribute to the success of the species when occurring on rocky reefs (Voerman et al., 2019), however, the alga is rarely found on sandy substrate itself potentially due to the reduced recruitment on unstable substrate (Voerman et al., 2017). C. filiformis reproduces vegetatively via fragmentation (Zhang et al., 2014), which has likely contributed to its current range expansion (Glasby et al., 2015). Fragment morphologies are highly variable, with both fronds with and without rhizome + rhizoid structures present (Khou et al., 2007). Thousands of suspended fragments can be found at a site (Khou et al., 2007), yet how recruitment success may be influenced by propagule quality, or how this may interact with features of the recipient habitat, remains largely unknown.
This study investigated the roles of canopy-forming habitats (kelp and Sargassum) and less structured habitats (turf and barrens, which represent degraded habitats in some situations), in the recruitment success of C. filiformis. In addition, the mechanisms behind the expected positive role of turf in facilitating C. filiformis recruitment were explored, as was the role of propagule quality (i.e., rhizome presence). First, habitat associations of C. filiformis’ recruits in the field were examined to test the prediction that recruitment is enhanced by turf and reduced by macroalgal canopies. Secondly, a laboratory experiment tested hypotheses about the positive roles of substrate structure on C. filiformis attachment success (measured as fragment attachment success, speed and strength) and turf-derived sediment on C. filiformis growth. Those positive associations were predicted to be enhanced by the presence of rhizome + rhizoids on C. filiformis fragments. Finally, this study further explored the observed habitat associations by experimentally testing the retention success of C. filiformis fragments in macroalgal canopies (kelp, Sargassum) vs. turf and barren habitats under field conditions.
Caulerpa filiformis (Suhr) Hering is a green macroalga, with long, thin fronds up to ∼ 70 cm in length (Voerman et al., 2019). Once established, populations consist of a dense network of creeping rhizomes with rhizoid clusters that attach the alga to the substrate (Khou et al., 2007). It has a disjunct distribution along ∼ 700 km of the warm temperate coast of eastern Australia where it occurs primarily subtidal down to ∼ 7 m and also occurs in low intertidal areas (Zhang et al., 2014; Glasby et al., 2015). The alga is principally found on hard (rock) substrate but can also occur on soft (coarse sand) substrate (Voerman et al., 2017). Disturbed fragments (hereafter propagules) predominantly consist of partial or full fronds, with or without a rhizome present, and are predominantly 5–15 cm in frond length (Voerman, unpublished data). C. filiformis has a siphonous body structure, which is highly plastic, allowing for rapid growth and creation of new fronds and/or rhizoid structures (Khou et al., 2007), wound healing and fast fragment propagation (Walters and Smith, 1994; Smith and Walters, 1999).
The six sites surveyed (Figure 1) encompassed the full distribution of C. filiformis in NSW (Glasby et al., 2015) and all contained large beds of C. filiformis (Voerman, 2017). All sites were positioned along the exposed coastline, shallow sloping and contained a mixture of the habitats investigated (Table 1).
Figure 1. Location of six sites surveyed for Caulerpa filiformis recruits and their habitat associations in New South Wales (dark gray), Australia.
To investigate the density of C. filiformis’ recruits across sites and to test the prediction that recruitment is enhanced by turf habitat and reduced by macroalgal canopies, the six sites were sampled during August–December 2013. Recruits were defined as small individual fragments (<5 cm diameter substrate cover with a maximum of five fronds) that had attached to the substrate (Figure 2). Whilst size is not a measure of recruitment, individual fragments of this size have not yet expanded horizontally, and likely represent fragments that have recently attached to the substrate.
Recruits were enumerated in transects (1 m wide, n = 4) orientated perpendicular to the shoreline from the low-tide mark to >6 m beyond the edge of the reef (i.e., onto sand). Transects covered a similar depth range (between 0.5 and 6.5 m below low tide level) but varied in length from 30 to 60 m depending on the width of the reef. At two sites (Seal Rocks and Wollongong), transects stopped at 30 m without reaching the end of the reef due to unfavorable sampling conditions. For each recruit encountered, we noted the habitat to which it was attached (see Table 1 for habitat classifications). New recruits in dense adult C. filiformis beds could not be distinguished, so this algal habitat was not sampled.
Information on the relative area of habitats surveyed was used to explain variation in recruit densities. The area of recipient habitat surveyed was estimated as a function of the known percentage cover of each habitat per site quantified from quadrats positioned along the same transects (one 50 × 50 cm quadrat every 3 m, n = 43 – 95 per site, see Voerman et al., 2017), multiplied by the area covered by the entire transects surveyed in this study. Recruit density at a site was calculated as a function of the entire rocky reef sampled (i.e., excluding the area covered by adult C. filiformis and sand outside the rocky reef). Recruit density per habitat was calculated as a function of the total area of the individual habitat surveyed at each site (# recruits observed/habitat area surveyed).
ANOVA was used to test for differences in the association of C. filiformis recruits among habitats. Only sites with recruits encountered were included in the analysis. Model assumptions were tested with the “GVLMA” package (Pena and Slate, 2014), and adjustments were made to comply with model assumptions when required. Significant treatment effects were further investigated with Post Hoc Ls means comparisons (“Lsmeans” package, Lenth, 2016). All statistics were conducted in R version 3.5.1 (R Core Team., 2018).
Results from the habitat sampling suggested an important role of turf (geniculate corallines) in promoting fragment recruitment. To explore this further, we investigated whether C. filiformis attachment success (attachment speed and strength) and fragment growth (biomass increase and changes in morphology) were related to the physical properties of the turf (structure) and/or the properties of the sediment of the turf-sediment complex. We also tested how these properties interacted with quality (presence or absence of rhizome) of C. filiformis propagules.
Seven substrate treatments were created to tease apart the potential effects of substrate structure from the effects of sediment on C. filiformis attachment and growth (Table 2). Treatments with physical structure (either natural or artificial) were predicted to enhance C. filiformis attachment and a treatment with turf-derived sediment was predicted to enhance subsequent growth of C. filiformis. To investigate the effect of propagule quality on recruitment performance, two types of C. filiformis fragments were used; high-quality propagules consisting of fragments with rhizome and rhizoids present or low-quality propagules where rhizomes were removed. The two types of propagules were added separately to each of the treatments listed in Table 2. The general prediction for high quality propagules (with rhizome and rhizoids) vs. low quality fragments was that attachment would be enhanced relative to low quality propagules for all treatments, while growth would be enhanced only in treatments with turf-derived sediment.
Substrate treatments were created by collecting small (5–10 cm widest diameter) rocks, either with (n = 30) or without (n = 10) turf cover. Rocks were collected from the shallow subtidal region at Seal Rocks (–32.431° S, 152.525° E) and transported in seawater to the research facility at DPI Port Stephens. Rocks were kept in large, aerated tanks with flow through estuarine water (∼34 ppt) and kept under 12:12 h light:dark cycle at ambient temperature (∼25–30°C daily max) for 4 days until the start of the experiment. Turf consisted of numerous species < 5 cm tall but was dominated (>50% cover) by geniculate corallines. In the laboratory, all sediment was removed from the turf-sediment matrix from rocks with turf to create the R + T – S treatment, or mixed, and re-introduced in naturally occurring amounts to rocks with turf to form the R + T + S treatment. To form the R – T treatment, rocks with turf were carefully cleared of vegetation with a heavy-duty tile brush. Naturally bare rocks were not treated and formed the R treatment. Artificial rock consisted of a kitchen tile with the ceramic side facing upwards. This side consisted of an even surface with small-scale pores and a fish-scale pattern with ±1 mm surface relief. A 4 mm thick heavy-duty scouring pad (70 nylon and 30% polyester© MrClean) was glued onto artificial rock to create the artificial R + T treatment. Coarse sand was sourced adjacent to the rocks, and a 2 cm thick layer was created to form the S treatment. Habitat treatments were placed in shallow plastic trays (150 × 95 × 60 mm) with openings added on the sides to allow for water flow. Each treatment was added to a large tank (n = 10 large tanks, seven habitat treatments/tank) to create a randomized block design.
Intact C. filiformis was collected from Newcastle (–32.926° S, 151.793° E) from large boulders around the low tide mark and transported to the laboratory in seawater, where propagule quality types were created by pruning the rhizome and rhizoids. Fronds were 8–15 cm in length. High quality propagules consisted of a frond with 2 cm rhizome attached to it each with 2–4 rhizoid clusters. Low quality propagules consisted of only a frond. After pruning, all propagules were kept suspended in aerated seawater with constant flow for 48 h to allow for wounds to heal which takes < 48 h (Goddard and Dawes, 1983). Number of rhizoid clusters and wet weight was determined prior to the start of the experiment for later growth analysis for all fragments individually. Rhizomes constitute a very low proportion of propagule biomass, and initial fragment weight did not differ between substrate or propagule quality treatments at the start of the experiment (F6,55 = 0.85, p > 0.4 and F1,55 = 1.46, p > 0.1 respectively, mean fragment weight 122 ± 6 g). A single fragment was then placed in the center of its allocated substrate treatment (n = 5 replicate trays per Substrate × Propagule quality combination), with random distribution of propagule quality across tanks. Tanks were kept under the same conditions as described above for 12 days.
Attachment speed (days to attach) was assessed by carefully shaking the treatment tray every second day and checking for movement vs. attachment of the fragments. On the final day of the experiment (day 10), attachment strength was measured by attaching a clasp with two wooden stirring sticks to all the rhizoids to evenly distribute the force over the fragment attached to a pull balance. Once fragments were removed, change biomass (wet weight) and production of new attachment points (# rhizoid clusters) from the start of the experiment were determined. From the attachment strength measure and the known number of rhizoid clusters, we determined the force per attachment point. The effects of Substrate and Propagule quality on attachment speed, strength (entire fragment and individual rhizoids estimates), and growth (change in total wet weight and rhizoid cluster production) were tested with general linear models with tank as blocking factor and type II ANOVA tests (“Car” package, Fox et al., 2016) using methods as described above. Fragments that had not attached were excluded from tests for attachment speed and strength but not growth analysis.
A field experiment was designed to determine whether recruit density patterns observed in the field sampling were explained by differences in fragment retention among habitats, i.e., retention on turf > Sargassum spp. > kelp and barren. At Malabar, Sydney (–33.966° S, 151.255° E), we placed fragments in either canopy-forming habitats (Sargassum and kelp) or in less structured habitats (turf and barren), which in some situations may represent degraded conditions. The experiment was conducted twice, once in January 2015 and again in January 2016. In each habitat, plots were created (n = 8 plots for turf and Sargassum in 2015, and n = 4 plots for kelp and barren in 2015 and all habitats in 2016). Plots consisted of four stainless steel bolts on each corner of a 25 × 25 cm plastic mesh (25 × 25 mm mesh size; ©Gardenmaster). Individual plots were placed in interspersed patches of habitat, each > 4 m2, but kelp plots were all positioned inside the same large kelp patch (> 40 m2), with plots > 2m apart. Plots were positioned on horizontal surfaces at depths of 1.5 – 3 m below low tide and >50 cm from any edge of the habitat patch. The turf treatment consisted of a natural assemblage of a dense cover dominated by red geniculate coralline algae (>75% cover) and with some filamentous turf (species unidentified), all < 5 cm tall. Kelp, Sargassum and barren habitats were as described in Table 1.
Caulerpa filiformis was collected from a patch at the same site and transported to the lab where we created fragments consisting of single, unbranched, blades, each 10 cm in length, similar to the “poor quality” fragment type in the section above. Fragments were kept in aerated ocean water with constant flow at a 12:12 h light:dark cycle at 21°C for 3 days to allow for wound healing, after which intact fragments were transported back to the site where they were added to the plots. Fragments were placed in the plots (five fragments/plot in 2015 and seven fragments/plot in 2016) and loosely attached to the mesh with two thin (<0.5 mm wide) strips of duct tape. Although this method excluded potential differences in fragment entrapment, it could test for differences in fragment retention and recruitment potential among habitats.
In 2015, plots were revisited every 3 – 6 days during the first 2 weeks, and then every 2 weeks until the experiment stopped after 8 weeks due to the loss of most fragments. In 2016, plots were revisited every 2 days, ocean conditions permitting, and ran for 3 weeks. During every visit, the number of fragments still present was counted. Differences in fragment retention among habitats were tested with ANOVA tests for each sampling date with using methods as described above. As fragment retention was very low, other response variables such as growth rate could not be investigated.
A measure of ocean conditions (max. wave height) was used to test for associations with C. filiformis fragment retention over time. Wave data recorded by the Sydney offshore Waverider buoy located at 33.772° S; 151.409° E (∼26 km NE of Malabar) were collected and provided by the Manly Hydraulics Laboratory (see Supplementary Material II).
Canopies of kelp and Sargassum were abundant at all sites except Kingsley Beach (Figure 3). Habitats of turf (coralline and filamentous forms) and barrens were abundant at all sites except Sharkies Cove (Figure 3). Adult C. filiformis covered large parts of the reef at Kingsley Beach, Bronte, and Coogee (47, 36, and 33% of rocky reef area surveyed respectively, Figure 3), but was less abundant at Sharkies Cove, Seal Rocks and Wollongong (14, 6, and 9% of rocky reef area surveyed respectively, Figure 3).
Figure 3. Estimated habitat area surveyed at each site. Note that C. filiformis habitat refers to adult cover. See Table 1 for habitat descriptions.
Caulerpa filiformis recruits were found at four of the six reefs sampled and absent from Sharkies Cove and Kingsley Beach. The average density of recruits on the rocky reef at the four sites with recruits present was 9 (±2) per 100 m2, with the highest density of 13 individuals per 100 m2 at Seal Rocks and Wollongong.
Recruits were found across a broad range of habitats, but in most habitats the density was low (<0.2 m–2) (Figure 4). Recruit density in the structurally complex geniculate coralline habitat was significantly greater than in all other habitats (F7,20 = 7.154, p < 0.001; Figure 4). There was no indication that recruit density was enhanced in filamentous forms of turf (Figure 4). Recruits were generally rare in barrens and Sargassum habitat (Figure 4). Any recruits in barrens were always in association with small-scale substrate relief, such as small crevices, cracks, and folds. No recruits were found on sand, rock with a sediment veneer, or in kelp habitat (Figure 4).
Figure 4. Caulerpa filiformis recruit density (±SE) per habitat. Only sites with recruits present and habitats available at those sites (n = 3, 4, 2, 3, 4, 4, 3, 4 for respective habitats) were included. Letters indicate differences according to post hoc comparison (p < 0.05). See Table 1 for habitat descriptions.
Attachment success of fragments across all treatments at the end of the 10-day experiment was high for substrates containing three-dimensional structure (artificial turf, R + T – S and R + T + S) and on Sand (90 – 100%), but less so on hard substrates lacking three-dimensional structure (Artificial R, R, R – T; 20–70%). Attachment speed varied significantly among substrates (Figure 5A), with the same patterns among substrates for both the low- and high-quality propagules (Table 3). Overall, fragments on R + T (with or without sediment) attached the quickest (4 days), followed by Artificial Rock and Artificial Turf (Figure 5A). Fragments on Sand took the longest to attach (10 days). Fragment attachment strength and rhizoid cluster attachment strength differed among substrates (Figures 5B,C), but no differences between propagules of low of high quality were found (Table 3). Attachment strength of C. filiformis propagules was significantly less on substrates lacking three-dimensional complexity (artificial R, R, R – T) or structural integrity (Sand) (Figure 5B). Attachment strength of rhizoid clusters followed similar patterns among substrates but only some post hoc comparisons were significant, due to the large variation in rhizoid attachment (Figure 5C).
Figure 5. Attachment speed (days to attach) (A), attachment strength (N) of entire fragment (B) and average attachment strength (N) per rhizoid cluster (C), averaged over levels of Propagule quality. Only fragments that had attached within 10 days of experimentation were included in the analysis. N = 10, 2, 7, 4, 10, 10, 10 for habitats Sand, Artificial Rock, R, R – T, Artificial Turf, R + T – S and R + T + S respectively. Letters indicate differences according to post hoc comparison (p < 0.05). No post hoc comparison is available for the Artificial Rock treatment, as only two fragments had attached at the end of the 10 days experimental period. R, rock; T, turf; S, sediment. Error bars represent SE.
Table 3. ANOVA (type II) test results on attachment performance (attachment speed, fragment- and estimated rhizoid attachment strength) for factors Substrate type and Propagule quality and their interaction measured at the end of the 10 days experimental period, with Aquarium added as blocking factor.
Overall growth rates were not related to Substrate type (Table 4) but were higher for high quality propagules compared to without rhizoids respectively (Table 4 and Figure 6).
Table 4. ANOVA (type II) test results on growth performance (change in total wet weight, production of rhizoid clusters, and final rhizoid number) for factors Substrate type and Propagule quality and their interaction over the 10 days experimental period, with Aquarium added as blocking factor.
Figure 6. Propagule growth (A) and production of rhizoid clusters (B) over 10-day experimental period across Propagule qualities, averaged over levels of Substrate type (n = 35). Symbol indicates significant propagule quality effect (Table 4). Error bars represent SE. *p < 0.05 and ***p < 0.001.
Fragments with rhizome removed (low quality) had increased production of rhizoid clusters compared to the rhizome intact (high quality) treatments (Table 4 and Figure 6). By the end of the experiment, the final numbers of rhizoid clusters did not differ with propagule quality (Table 4), but were greater on natural turf substrata, particularly on turf with sediment present (Figure 7).
Figure 7. Production of rhizoid clusters (± SE) over 10-day experimental period across substrata, averaged over levels of Propagule quality (n = 10). Different letters indicate significant differences between levels of Substrate (p < 0.05). R, rock; T, turf; S, sediment.
Recruitment success differed among habitats (see Supplementary Material I for ANOVA test results), but patterns were not consistent over time and did not always follow those predicted.
For the 2015 experiment, nearly all fragments (90%) were retained in the turf habitat during the first 12 days. Retention was reduced in kelp and Sargassum habitat (50 and 54%) but was lowest on barrens, with only ∼20% of fragments remaining after 12 days (Figure 8A). However, this pattern reversed after 14 days, with the loss of all propagules from turf, and minimal further loss from the other habitats (Figure 8A). This resulted in Sargassum and kelp habitats having higher overall retention success than turf and barrens. This event coincided with a change from a period of low to moderate swell (up to 1 m) to high swell events (>1.5 m) (Supplementary Material II). During this subsequent period of frequent, intense swell, overall propagule retention was very low, with nearly all fragments being dislodged after 2 months of experimentation.
Figure 8. Fragment retention success (±SE) per habitat at days since the initial addition of five fragments per mat on 16/02/2015 (A) or seven fragments per mat on 11/01/2016 (B). 8 Replicate plots for Sargassum and Turf habitat for the 2015 experiment, four replicate plots for all others. Different letters indicate differences among substrates (p < 0.05) per sampling round. Note the different scales of the y-axes.
Similarly, the 2016 experiment was characterized by frequent high swell periods (Supplementary Material II). Again, overall fragment retention was low, and most fragments were lost after less than 20 days (Figure 8B). As observed in the previous year, even though overall success was low, Sargassum habitat showed higher fragment retention rates than any of the other habitats investigated at most sampling times during this high-swell period.
Here, we investigated if turf and barrens habitats (which in some situations are considered degraded habitats) facilitate the spread of a previously sub-dominant community member. Surveys and experiments showed that recruitment of the rapidly spreading native macroalga C. filiformis was consistently higher in algal turf compared to other native habitats due to the provision of a superior surface for attachment. There was no evidence that C. filiformis was colonizing barrens habitats.
A species’ potential spread is governed in part by the availability of suitable habitat for recruitment. The absence of suitable habitat may therefore explain the absence of C. filiformis recruits at two of the six sites investigated here. At Kingsley Beach, large parts of the reef were covered by dense adult stands of C. filiformis possibly limiting the quantity of space available for recruitment, while other reef areas were low-quality habitat for propagule attachment (predominantly barrens) as defined in the laboratory experiment. Similarly, at Sharkies Cove, recruits may have not been observed due to the absence of high-quality habitat for recruitment (turf) as defined in the laboratory and field experiments. However, the relatively low abundance of adult C. filiformis may have simultaneously reduced propagule supply at this site.
Habitat suitability varies with the recipient biotic community, that can either resist, tolerate or promote macroalgal recruitment (Scheibling and Melady, 2008; Hughes, 2010; Burek et al., 2018; Layton et al., 2019). As predicted, relatively few C. filiformis recruits were found under algal canopies, which supports the notion that intact habitat-formers resist invasion, although response to different canopies varied. No recruits were found under kelp canopies, and similarly recruits were not retained under kelp when experimentally added in the field. Kelps are especially efficient in taking up available light limiting the potential for many other macroalgae to persist (Kennelly, 1987b; Valentine and Johnson, 2003; Clark et al., 2004) and/or the continual physical sweeping of fronds at wave-swept shores and the abrasion caused can remove benthic organisms (Kennelly, 1987b; Irving and Connell, 2006; Hughes, 2010) and may simultaneously inhibit the supply and recruitment of C. filiformis propagules to the substrate. Unlike for kelp, there was not a consistent negative association between the smaller canopy-forming Sargassum habitat and C. filiformis recruits. Although the arrival of propagules may be restricted by Sargassum canopies (Arenas et al., 2006), the presence of scattered understory turf amongst Sargassum habitat likely facilitated retention and recruitment success of C. filiformis propagules.
Field observations showed a clear distinction in C. filiformis recruitment between different types of turf, with higher recruitment in communities dominated by geniculate corallines, but not filamentous turf. Although the two types of turf can co-occur, our results support the importance of distinguishing the morphologies of the assemblage often collectively termed “turf” (e.g., Connell et al., 2014; O’Brien and Scheibling, 2018). The discrepancy in their respective roles in shaping C. filiformis’ recruitment patterns likely results from variation in the provision of suitable substrate for attachment by either form of turf. While geniculate coralline algae provide complex three-dimensional rigid surface created by their calcium carbonate deposits, filamentous turf does not. How altered relative abundance of geniculate coralline vs. filamentous forms affect invaders recruitment success – or more broadly species interactions in general – is an interesting area of further study, especially if the relative abundances of the two are expected to change due to further environmental change (Hepburn et al., 2011; Short et al., 2014).
The density of C. filiformis recruits was greater on geniculate coralline turf compared to any other habitat present, and this was remarkably consistent across sites. Habitat suitability of coralline turf was also demonstrated by our field experiment, albeit only under benign weather conditions, and in the laboratory experiment which demonstrated how turf facilitated C. filiformis’ recruitment. Several studies have proposed the positive role of turf in trapping propagules of vegetatively spreading species including those from the genera Caulerpa, Dictyota, and Codium (Ceccherelli et al., 2002; Herren et al., 2006; Bulleri and Benedetti-Cecchi, 2008; Watanabe et al., 2009). Our laboratory experiment results also indicate a subsequent facilitation of Caulerpa recruitment, created by a combination of more successful, faster and stronger attachment of propagules. Increased attachment performance resulted from the structure turf provides, as indicated by the similar attachment on artificial turf substrata that mimicked the structural complexity of algal turf. The increased attachment strength on turf substrata was not solely a result of increased number of attachment points following increased rhizoid production, but also increased attachment strength per rhizoid cluster. The latter may have resulted from increased time to attach resulting from faster attachment speed on turf, as increased attachment time allows for stronger attachment (Khou et al., 2007), or because of the mechanical properties of the turf allowing for stronger attachment per attachment point per se.
Although rhizoid production was highest in the presence of turf and sediment, there was no evidence for the role of turf-sediment in promoting C. filiformis’ recruitment success (attachment success, speed or strength) or the hypothesized subsequent growth. Similarly, Bulleri and Benedetti-Cecchi (2008) found no effects of turf-derived sediment on the recruitment success or size of C. cylindracea after 45 days in the field. Equally, no changes in growth rates of already established individuals of C. filiformis were observed under the influence of turf-derived sediment over an 8 week period in the laboratory (Voerman, 2017).
The presence of rhizome and rhizoid structures can aid recruitment success of vegetatively spreading algae (Smith and Walters, 1999; Khou et al., 2007; Uyà et al., 2018), although their importance may vary across habitats (Uyà et al., 2018; Bulleri et al., 2019). The overall lack of difference in recruitment success (attachment success, speed or strength) between low and high quality C. filiformis propagules observed in this study is likely a result of the high plasticity of the alga aided by its siphonous body structure that allows for a high capacity to rapidly reallocate resources (Vroom and Smith, 2001). Low quality propagules (without rhizomes and rhizoids) quickly grew rhizoid clusters, such that at the end of the 10 days experiment the two types of propagules had similar numbers of rhizoids. However, fragments with rhizoids present did show higher growth rates, irrespective of habitat. Increased growth may be due to high-quality propagules being able to direct energy toward photosynthesis and growth without first having to invest in rhizoid production for attachment. High quality propagule driven increased growth rates may in turn increase competitive strength for limited space in the field over the short-term. Results also revealed a clear differentiation in rates of production and/or maintenance of rhizoid clusters among substrata, with highest investment on natural turf substrata, especially R + T + S, while other substrata showed low rhizoid production or even rhizoid loss. Rhizoid production is inhibited when fragments are kept in solution and only form when in contact with a substratum (Khou et al., 2007). We propose substrate types can similarly either stimulate energy investment toward the creation or maintenance of rhizoid clusters or alternatively away from this allowing for facultative recruitment depending on habitat type. The mechanisms behind the observed differentiation in rhizoid investment are unclear. Allelopathy has been suggested to explain the role of coralline turf in the settlement of kelp sporelings (Denboh et al., 1997), and chemical cues from crustose coralline algae drastically enhance settlement coral larvae in tropical systems (Dixson et al., 2014). Perhaps similar mechanisms can explain the increased rhizoid investment and subsequent attachment of C. filiformis propagules on coralline turf.
Patterns of C. filiformis fragment retention from our field experiment suggested that differences among habitats may be related to oceanic swell. Specifically, under relatively benign swell conditions, retention in turf habitat was greater than that in other habitats and least in kelp and barren habitats, which agrees with our observations of the distribution of recruits in the field. The predominantly negative association of fragment retention with canopy formers reversed under challenging environmental conditions (i.e., increased swell). Larger species of macroalgae can buffer near-ground wave action (Masteller et al., 2015), with ameliorating effects becoming more beneficial when conditions become more severe (Hughes, 2010; O’Brien and Scheibling, 2018). However, high wave action events are simultaneously responsible for the creation (Smith et al., 2004; Watanabe et al., 2009) and potentially the dispersal of propagules. Whilst rough conditions generate fragments, we found that benign conditions promote recruitment. Thus, although retention was enhanced under canopies when abiotic conditions became challenging, ultimately it is a poor-quality recruitment habitat. Wave action and low attachment strength on sand (as per our laboratory experiment) also likely explains the absence of adult C. filiformis stands on large sandy stretches along the exposed shoreline of NSW but not in sheltered bays (Glasby et al., 2015; Voerman et al., 2017).
Turf is considered a normal component of the mosaic of shallow rocky reef habitats in NSW (Underwood et al., 1991; Andrew and Underwood, 1993), however, turf may also represent degraded conditions where has resulted from the loss of canopy-forming algae (Airoldi and Beck, 2007; Filbee-Dexter and Wernberg, 2018). There are increasing concerns about losses of the kelp Ecklonia radiata due to ocean warming (Wernberg et al., 2018), while the expanding distribution of C. filiformis is close to the northern distribution limit of E. radiata in NSW where temperatures are most likely to exceed the tolerance of kelp and where there are increasing negative effects of tropical herbivorous fish (Vergés et al., 2014; Glasby et al., 2015; Wernberg et al., 2018). The loss of the large fucoid Phyllospora comosa (not present in our sites at time of study) from the Sydney region (Coleman et al., 2008) has similarly been proposed as a potential mechanism behind the spread of C. filiformis (Glasby et al., 2015). Any loss of canopy formers will likely benefit the spread of C. filiformis if they are replaced by turf, but not if replaced by barrens. This may be exacerbated as turf inhibits the recruitment of many sexually reproducing macroalgae including the kelp E. radiata (Kennelly, 1987a; Burek et al., 2018; Layton et al., 2019) and several fucoids (e.g., O’Brien and Scheibling, 2018). Species that, indeed, are important in resisting C. filiformis recruitment (this study) and its subsequent horizontal spread (Zhang et al., 2014; Voerman et al., 2017). Our results suggest that turfing habitat is an important facilitator of C. filiformis, and where turfs represent an alternate state previously dominated by canopy forming habitat (e.g., Feehan et al., 2019; Layton et al., 2019), they may act as a transitional habitat for further community change by promoting the recruitment of C. filiformis. While the replacement of canopy forming algae with turf can have large implications for the functioning and productivity of coastal systems (Filbee-Dexter and Wernberg, 2018), this may be exacerbated by coralline turf facilitating the colonization of C. filiformis. For example, dominance by C. filiformis, which is structurally and functionally very different from other native habitats in terms of forming dense meadows without a large canopy, potentially enhancing sediment trapping and having chemical defense, has been linked to dramatic changes associated flora and fauna relatively to other native habitats (Voerman et al., 2014; Lanham et al., 2015; Voerman, 2017; Voerman et al., 2017).
In conclusion, positive and negative species interactions define the recruitment success of the native alga C. filiformis. Whilst turf is often considered an alternative state, geniculate coralline turfing habitats also promote the recruitment of invasive algae, in our case, C. filiformis, which has been termed a ‘native-invader.’ The extensive spread of C. filiformis represents a clear challenge to ecological communities on rocky reefs on which it is becoming locally more abundant. However, our results indicate that recruitment success, and therefore its impacts, is promoted by the loss of algal canopies. Thus, environmental managers may be able to identify reefs at particular risk from the spread to C. filiformis.
The raw data supporting the conclusions of this article will be made available by the authors, without undue reservation.
SV, PG, and TG designed the study and wrote the manuscript. SV executed the work and analyzed the data. All the authors contributed to the article and approved the submitted version.
This research was funded by an Australian Research Council Future Fellowship (FT140100322) to PG, a UTS President Scholarship and a UTS Australian Wildlife Society award to SV, and NSW Government funding.
The authors declare that the research was conducted in the absence of any commercial or financial relationships that could be construed as a potential conflict of interest.
We would like to thank Daniel Bradley, Vicky von Bernard, Samuel vande Walle, and Michael Simpson for assistance with field sampling and Graham Housefield for assistance with laboratory experiments.
The Supplementary Material for this article can be found online at: https://www.frontiersin.org/articles/10.3389/fmars.2021.594447/full#supplementary-material
Supplementary Material I | ANOVA (Type II) test results in retention success among recipient habitats. Eight replicate plots for Sargassum and turf during the 2015 experiment, and four replicate plots for all other treatments.
Supplementary Material II | Max wave height recorded by the Office of Environment and Heritage’s (OEH) Sydney offshore Waverider buoy during the fragment-retention experiments in 2015 and 2016. Wave data was collected and provided by the Manly Hydraulics Laboratory.
Airoldi, L., and Beck, M. W. (2007). Loss, status and trends for coastal marine habitats of Europe. Oceanogr. Mar. Biol. Ann. Rev. 45, 345–405. doi: 10.1201/9781420050943.ch7
Andrew, N. L., and Underwood, A. J. (1993). Density dependent foraging in the sea urchin Centrostephanus rogersii on shallow subtidal reefs in New South Wales, Australia. Mar. Ecol. Prog. Ser. 99, 89–98. doi: 10.3354/meps099089
Arenas, F., Sánchez, I., Hawkins, S. J., and Jenkins, S. R. (2006). The invasibility of marine algal assemblages: role of functional diversity and identity. Ecology 87, 2851–2861. doi: 10.1890/0012-9658(2006)87[2851:tiomaa]2.0.co;2
Benedetti-Cecchi, L., Bulleri, F., and Cinelli, F. (1998). Density dependent foraging of sea urchins in shallow subtidal reefs on the west coast of Italy (western Mediterranean). Mar. Ecol. Prog. Ser. 163, 203–211. doi: 10.3354/meps163203
Bennett, S., Wernberg, T., Connell, S. D., Hobday, A. J., Johnson, C. R., and Poloczanska, E. S. (2016). The‘Great Southern Reef’: social, ecological and economic value of Australia’s neglected kelp forests. Mar. Freshw. Res. 67, 47–56. doi: 10.1071/mf15232
Bradley, D., Gladstone, W., and Gribben, P. (2018). Relationships between the spread of Caulerpa filiformis and fish communities on temperate rocky reefs. J. Fish Biol. 93, 12–20. doi: 10.1111/jfb.13664
Britton−Simmons, K. H., and Abbott, K. C. (2008). Short−and long−term effects of disturbance and propagule pressure on a biological invasion. J. Ecol. 96, 68–77. doi: 10.1111/j.1365-2745.2007.01319.x
Bulleri, F., and Benedetti-Cecchi, L. (2008). Facilitation of the introduced green alga Caulerpa racemosa by resident algal turfs: experimental evaluation of underlying mechanisms. Mar. Ecol. Prog. Ser. 364, 77–86. doi: 10.3354/meps07484
Bulleri, F., Marzinelli, E. M., Voerman, S. E., and Gribben, P. E. (2019). Propagule composition regulates the success of an invasive seaweed across a heterogeneous seascape. J. Ecol. 108, 1061–1073. doi: 10.1111/1365-2745.13313
Bulleri, F., Tamburello, L., Pusceddu, A., Bonechi, L., Cau, A., Moccia, D., et al. (2018). Fragment quality and sediment organic loading regulate the survival of an invasive, clonal seaweed. Biol. Invasions 20, 1953–1959. doi: 10.1007/s10530-018-1685-4
Burek, K. E., Brien, J. M., and Scheibling, R. E. (2018). Wasted effort: recruitment and persistence of kelp on algal turf. Mar. Ecol. Prog. Ser. 600, 3–19. doi: 10.3354/meps12677
Ceccherelli, G., Piazzi, L., and Balata, D. (2002). Spread of introduced Caulerpa species in macroalgal habitats. J. Exp. Mar. Biol. Ecol. 280, 1–11. doi: 10.1016/s0022-0981(02)00336-2
Clark, R., Edwards, M., and Foster, M. (2004). Effects of shade from multiple kelp canopies on an understory algal assemblage. Mar. Ecol. Prog. Ser. 267, 107–119. doi: 10.3354/meps267107
Coleman, M. A., Kelaher, B. P., Steinberg, P. D., and Millar, A. J. (2008). Absence of a large brown macroalga on urbanized rocky reefs around Sydney, Australia, and evidence for historical decline 1. J. Phycol. 44, 897–901. doi: 10.1111/j.1529-8817.2008.00541.x
Connell, S., Foster, M., and Airoldi, L. (2014). What are algal turfs? Towards a better description of turfs. Mar. Ecol. Prog. Ser. 495, 299–307. doi: 10.3354/meps10513
Connell, S. D., Russell, B. D., Turner, D. J., Shepherd, S. A., Kildea, T., Miller, D., et al. (2008). Recovering a lost baseline: missing kelp forests from a metropolitan coast. Mar. Ecol. Prog. Ser. 360, 63–72. doi: 10.3354/meps07526
Denboh, T., Suzuki, M., Mizuno, Y., and Ichimura, T. (1997). Suppression of Laminaria sporelings by allelochemicals from coralline red algae. Bot. Mar. 40, 249–256.
Didham, R. K., Tylianakis, J. M., Gemmell, N. J., Rand, T. A., and Ewers, R. M. (2007). Interactive effects of habitat modification and species invasion on native species decline. Trends Ecol. Evol. 22, 489–496. doi: 10.1016/j.tree.2007.07.001
Díez, I., Muguerza, N., Santolaria, A., Ganzedo, U., and Gorostiaga, J. (2012). Seaweed assemblage changes in the eastern Cantabrian Sea and their potential relationship to climate change. Estuar. Coast. Shelf Sci. 99, 108–120. doi: 10.1016/j.ecss.2011.12.027
Dixson, D. L., Abrego, D., and Hay, M. E. (2014). Chemically mediated behavior of recruiting corals and fishes: a tipping point that may limit reef recovery. Science 345, 892–897. doi: 10.1126/science.1255057
Dugan, J. E., Hubbard, D. M., Page, H. M., and Schimel, J. P. (2011). Marine macrophyte wrack inputs and dissolved nutrients in beach sands. Estuar. Coasts 34, 839–850. doi: 10.1007/s12237-011-9375-9
Estrada, J. A., Wilson, C. H., NeSmith, J. E., and Flory, S. L. (2016). Propagule quality mediates invasive plant establishment. Biol. Invasions 18, 2325–2332. doi: 10.1007/s10530-016-1163-9
Feehan, C. J., Grace, S. P., and Narvaez, C. A. (2019). Ecological feedbacks stabilize a turf-dominated ecosystem at the southern extent of kelp forests in the Northwest Atlantic. Sci. Rep. 9:7078.
Filbee-Dexter, K., and Scheibling, R. E. (2014). Sea urchin barrens as alternative stable states of collapsed kelp ecosystems. Mar. Ecol. Prog. Ser. 495, 1–25. doi: 10.3354/meps10573
Filbee-Dexter, K., and Wernberg, T. (2018). Rise of turfs: a new battlefront for globally declining kelp forests. BioScience 68, 64–76. doi: 10.1093/biosci/bix147
Fox, J., Weisberg, S., Adler, D., Bates, D., Baud-Bovy, G., Ellison, S., et al. (2016). car: An R Companion to Applied Regression. R Package Version 3.2-0.
Gennaro, P., and Piazzi, L. (2014). The indirect role of nutrients in enhancing the invasion of Caulerpa racemosa var cylindracea. Biol. Invasions 16, 1709–1717. doi: 10.1007/s10530-013-0620-y
Glasby, T. M., and Gibson, P. T. (2020). Decadal dynamics of subtidal barrens habitat. Mar. Environ. Res. 154:104869. doi: 10.1016/j.marenvres.2019.104869
Glasby, T. M., Gibson, P. T., West, G., Davies, P., and Voerman, S. (2015). Range and habitat associations of the native macroalga Caulerpa filiformis in New South Wales, Australia. Mar. Freshw. Res. 66, 1018–1026. doi: 10.1071/mf14282
Goddard, R., and Dawes, C. (1983). An ultrastructural and histochemical study of the wound response in the coenocytic green algaCaulerpa ashmeadii (Caulerpales). Protoplasma 114, 163–172. doi: 10.1007/bf01283698
Gribben, P. E., Nielsen, S., Seymour, J. R., Bradley, D. J., West, M. N., and Thomas, T. (2017). Microbial communities in marine sediments modify success of an invasive macrophyte. Sci. Rep. 7:9845.
Gribben, P. E., Thomas, T., Pusceddu, A., Bonechi, L., Bianchelli, S., Buschi, E., et al. (2018). Below−ground processes control the success of an invasive seaweed. J. Ecol. 106, 2082–2095. doi: 10.1111/1365-2745.12966
Hepburn, C., Pritchard, D., Cornwall, C., McLeod, R., Beardall, J., Raven, J., et al. (2011). Diversity of carbon use strategies in a kelp forest community: implications for a high CO2 ocean. Glob. Change Biol. 17, 2488–2497. doi: 10.1111/j.1365-2486.2011.02411.x
Herren, L. W., Walters, L. J., and Beach, K. S. (2006). Fragment generation, survival, and attachment of Dictyota spp. at Conch Reef in the Florida Keys, USA. Coral Reefs 25, 287–295. doi: 10.1007/s00338-006-0096-7
Hughes, B. B. (2010). Variable effects of a kelp foundation species on rocky intertidal diversity and species interactions in central California. J. Exp. Mar. Biol. Ecol. 393, 90–99. doi: 10.1016/j.jembe.2010.07.003
Hughes, T. P., Rodrigues, M. J., Bellwood, D. R., Ceccarelli, D., Hoegh-Guldberg, O., McCook, L., et al. (2007). Phase shifts, herbivory, and the resilience of coral reefs to climate change. Curr. Biol. 17, 360–365. doi: 10.1016/j.cub.2006.12.049
Irving, A. D., and Connell, S. D. (2006). Physical disturbance by kelp abrades erect algae from the understorey. Mar. Ecol. Prog. Ser. 324, 127–137. doi: 10.3354/meps324127
Kennelly, S. J. (1987a). Inhibition of kelp recruitment by turfing algae and consequences for an Australian kelp community. J. Exp. Mar. Biol. Ecol. 112, 49–60. doi: 10.1016/s0022-0981(87)80014-x
Kennelly, S. J. (1987b). Physical disturbances in an Australian kelp community. II. Effects on understorey species due to differences in kelp cover. Mar. Ecol. Prog. Ser. 40, 155–165. doi: 10.3354/meps040155
Khou, M., Paul, N. A., Wright, J., and Steinberg, P. (2007). Intrinsic factors influence the attachment of fragments of the green alga Caulerpa filiformis. J. Exp. Mar. Biol. Ecol. 352, 331–342. doi: 10.1016/j.jembe.2007.08.010
Lanham, B. S., Gribben, P. E., and Poore, A. G. (2015). Beyond the border: effects of an expanding algal habitat on the fauna of neighbouring habitats. Mar. Environ. Res. 106, 10–18. doi: 10.1016/j.marenvres.2015.02.006
Layton, C., Cameron, M. J., Shelamoff, V., Fernández, P. A., Britton, D., Hurd, C. L., et al. (2019). Chemical microenvironments within macroalgal assemblages: implications for the inhibition of kelp recruitment by turf algae. Limnol. Oceanogr. 64, 1600–1613. doi: 10.1002/lno.11138
Lee, S. Y., Primavera, J. H., Dahdouh−Guebas, F., McKee, K., Bosire, J. O., Cannicci, S., et al. (2014). Ecological role and services of tropical mangrove ecosystems: a reassessment. Glob. Ecol. Biogeogr. 23, 726–743. doi: 10.1111/geb.12155
Lenth, R. (2016). Lsmeans: least-squares means. version 2.23-5. Comprehensive R Archive Network (CRAN). J. Stat. Softw. 69, 1–33
Ling, S. (2008). Range expansion of a habitat-modifying species leads to loss of taxonomic diversity: a new and impoverished reef state. Oecologia 156, 883–894. doi: 10.1007/s00442-008-1043-9
Masteller, C. C., Finnegan, N. J., Warrick, J. A., and Miller, I. M. (2015). Kelp, cobbles, and currents: biologic reduction of coarse grain entrainment stress. Geology 43, 543–546. doi: 10.1130/g36616.1
Moberg, F., and Folke, C. (1999). Ecological goods and services of coral reef ecosystems. Ecol. Econ. 29, 215–233. doi: 10.1016/s0921-8009(99)00009-9
Montefalcone, M., Vassallo, P., Gatti, G., Parravicini, V., Paoli, C., Morri, C., et al. (2015). The exergy of a phase shift: ecosystem functioning loss in seagrass meadows of the Mediterranean Sea. Estuar. Coast. Shelf Sci. 156, 186–194. doi: 10.1016/j.ecss.2014.12.001
Moy, F. E., and Christie, H. (2012). Large-scale shift from sugar kelp (Saccharina latissima) to ephemeral algae along the south and west coast of Norway. Mar. Biol. Res. 8, 309–321. doi: 10.1080/17451000.2011.637561
Norström, A. V., Nyström, M., Lokrantz, J., and Folke, C. (2009). Alternative states on coral reefs: beyond coral–macroalgal phase shifts. Mar. Ecol. Prog. Ser. 376, 295–306. doi: 10.3354/meps07815
O’Brien, J. M., and Scheibling, R. E. (2018). Turf wars: competition between foundation and turf-forming species on temperate and tropical reefs and its role in regime shifts. Mar. Ecol. Prog. Ser. 590, 1–17. doi: 10.3354/meps12530
Pena, E. A., and Slate, E. H. (2014). gvlma: Global Validation of Linear Models Assumptions. R Package Version 1(0.2).
R Core Team. (2018). R: A Language and Environment for Statistical Computing. Vienna: R Foundation for Statistical Computing.
Scheibling, R. E., and Melady, R. A. (2008). Effect of water movement and substratum type on vegetative recruitment of the invasive green alga Codium fragile ssp. tomentosoides. Bot. Mar. 51, 341–349.
Short, J., Kendrick, G., Falter, J., and McCulloch, M. (2014). Interactions between filamentous turf algae and coralline algae are modified under ocean acidification. J. Exp. Mar. Biol. Ecol. 456, 70–77. doi: 10.1016/j.jembe.2014.03.014
Smith, C. M., and Walters, L. J. (1999). Fragmentation as a strategy for Caulerpa species: fates of fragments and implications for management of an invasive weed. Mar. Ecol. 20, 307–319. doi: 10.1046/j.1439-0485.1999.2034079.x
Smith, J. E., Hunter, C. L., Conklin, E. J., Most, R., Sauvage, T., Squair, C., et al. (2004). Ecology of the invasive red alga Gracilaria salicornia (Rhodophyta) on O’ahu, Hawai’i. Pac. Sci. 58, 325–343. doi: 10.1353/psc.2004.0023
South, P. M., and Thomsen, M. S. (2016). The ecological role of invading Undaria pinnatifida: an experimental test of the driver–passenger models. Mar. Biol. 163:175.
Stachowicz, J. J. (2001). Mutualism, facilitation, and the structure of ecological communities: positive interactions play a critical, but underappreciated, role in ecological communities by reducing physical or biotic stresses in existing habitats and by creating new habitats on which many species depend. Bioscience 51, 235–246. doi: 10.1641/0006-3568(2001)051[0235:mfatso]2.0.co;2
Straub, S. C., Wernberg, T., Thomsen, M. S., Moore, P. J., Burrows, M. T., Harvey, B. P., et al. (2019). Resistance, extinction, and everything in between – the diverse responses of seaweeds to marine heatwaves. Front. Mar. Sci. 6:763. doi: 10.3389/fmars.2019.00763
Thibaut, T., Pinedo, S., Torras, X., and Ballesteros, E. (2005). Long-term decline of the populations of Fucales (Cystoseira spp. and Sargassum spp.) in the Alberes coast (France, North-western Mediterranean). Mar. Pollut. Bull. 50, 1472–1489. doi: 10.1016/j.marpolbul.2005.06.014
Thompson, G. A., and Schiel, D. R. (2012). Resistance and facilitation by native algal communities in the invasion success of Undaria pinnatifida. Mar. Ecol. Prog. Ser. 468, 95–105. doi: 10.3354/meps09995
Thomsen, M. S., Mondardini, L., Alestra, T., Gerrity, S., Tait, L., South, P., et al. (2019). Local extinction of bull kelp (Durvillaea spp.) due to a marine heatwave. Front. Mar. Sci. 6:84. doi: 10.3389/fmars.2019.00084
Tylianakis, J. M., Didham, R. K., Bascompte, J., and Wardle, D. A. (2008). Global change and species interactions in terrestrial ecosystems. Ecol. Lett. 11, 1351–1363. doi: 10.1111/j.1461-0248.2008.01250.x
Underwood, A., Kingsford, M., and Andrew, N. (1991). Patterns in shallow subtidal marine assemblages along the coast of New South Wales. Austr. J. Ecol. 16, 231–249.
Uyà, M., Bulleri, F., and Gribben, P. E. (2018). Propagules are not all equal: traits of vegetative fragments and disturbance regulate invasion success. Ecology 99, 957–965.
Valentine, J. P., and Johnson, C. R. (2003). Establishment of the introduced kelp Undaria pinnatifida in Tasmania depends on disturbance to native algal assemblages. J. Exp. Mar. Biol. Ecol. 295, 63–90.
van Kleunen, M., Dawson, W., and Maurel, N. (2015). Characteristics of successful alien plants. Mol. Ecol. 24, 1954–1968.
Vergés, A., Steinberg, P. D., Hay, M. E., Poore, A. G., Campbell, A. H., Ballesteros, E., et al. (2014). The tropicalization of temperate marine ecosystems: climate-mediated changes in herbivory and community phase shifts. Proc. R. Soc. Lond. B Biol. Sci. 281:20140846.
Voerman, S. E. (2017). The spread of the native macroalga Caulerpa filiformis. PhD. Broadway, NSW: University of Technology Sydney.
Voerman, S. E., Glasby, T. M., Gladstone, W., and Gribben, P. E. (2017). Habitat associations of an expanding native alga. Mar. Environ. Res. 131, 205–214.
Voerman, S. E., Glasby, T. M., Gladstone, W., and Gribben, P. E. (2019). Morphological variation of a rapidly spreading native macroalga across a range of spatial scales and its tolerance to sedimentation. Mar. Environ. Res. 147, 149–158.
Voerman, S. E., Llera, E., and Rico, J. M. (2013). Climate driven changes in subtidal kelp forest communities in NW Spain. Mar. Environ. Res. 90, 119–127.
Voerman, S. E., Zhang, D., Lloyd, H. B., Glasby, T. M., and Gribben, P. (2014). “Biodiversity responses associated with the potential spread of a native macroalgae in Eastern Australia,” in Proceedings of the International Temperate Reefs Conference, Perth.
Vroom, P. S., and Smith, C. M. (2001). The challenge of siphonous green algae. Am. Sci. 89, 525–531.
Walters, L. J., and Smith, C. M. (1994). Rapid rhizoid production in Halimeda discoidea Decaisne (Chlorophyta, Caulerpales) fragments: a mechanism for survival after separation from adult thalli. J. Exp. Mar. Biol. Ecol. 175, 105–120.
Watanabe, S., Metaxas, A., and Scheibling, R. E. (2009). Dispersal potential of the invasive green alga Codium fragile ssp. fragile. J. Exp. Mar. Biol. Ecol. 381, 114–125.
Wernberg, T., Coleman, M., Babcock, R., Bell, S., Bolton, J., Connell, S., et al. (2018). Biology and ecology of the globally significant kelp Ecklonia radiata. Oceanogr. Mar. Biol. Annu. Rev. 57, 265–324.
Wernberg, T., Krumhansl, K., Filbee-Dexter, K., and Pedersen, M. F. (2019). “Status and trends for the world’s kelp forests,” in World Seas: An Environmental Evaluation, C. Sheppard ed (Amsterdam: Elsevier), 57–78.
Williams, S. L. (1984). Uptake of sediment ammonium and translocation in a marine green macroalga Caulerpa cupressoides 1. Limnol. Oceanogr. 29, 374–379.
Williams, S. L., and Smith, J. E. (2007). A global review of the distribution, taxonomy, and impacts of introduced seaweeds. Annu. Rev. Ecol. Evol. Syst. 38, 327–359.
Wright, J. (2005). Differences between native and invasive Caulerpa taxifolia: a link between asexual fragmentation and abundance in invasive populations. Mar. Biol. 147, 559–569.
Wu, Z., Zuo, J., Ma, J., Wu, J., Cheng, S., and Liang, W. (2007). Establishing submersed macrophytes via sinking and colonization of shoot fragments clipped off manually. Wuhan Univ. J. Nat. Sci. 12, 553–557.
Yesson, C., Bush, L. E., Davies, A. J., Maggs, C. A., and Brodie, J. (2015). Large brown seaweeds of the British Isles: evidence of changes in abundance over four decades. Estuar. Coast. Shelf Sci. 155, 167–175.
Keywords: native invader, kelp, barrens, caulerpa, turf, community shifts
Citation: Voerman SE, Gribben PE and Glasby TM (2021) Positive and Negative Species Interactions Shape Recruitment Patterns of a Range Expanding Native Alga. Front. Mar. Sci. 8:594447. doi: 10.3389/fmars.2021.594447
Received: 13 August 2020; Accepted: 22 February 2021;
Published: 24 March 2021.
Edited by:
Christos Dimitrios Arvanitidis, Hellenic Centre for Marine Research (HCMR), GreeceReviewed by:
Megan Jane Huggett, University of Newcastle, AustraliaCopyright © 2021 Voerman, Gribben and Glasby. This is an open-access article distributed under the terms of the Creative Commons Attribution License (CC BY). The use, distribution or reproduction in other forums is permitted, provided the original author(s) and the copyright owner(s) are credited and that the original publication in this journal is cited, in accordance with accepted academic practice. No use, distribution or reproduction is permitted which does not comply with these terms.
*Correspondence: Sofie E. Voerman, cy52b2VybWFuQGh3LmFjLnVr
†Present address: Sofie E. Voerman, Lyell Centre for Earth and Marine Science and Technology, Heriot-Watt University, Edinburgh, United Kingdom
Disclaimer: All claims expressed in this article are solely those of the authors and do not necessarily represent those of their affiliated organizations, or those of the publisher, the editors and the reviewers. Any product that may be evaluated in this article or claim that may be made by its manufacturer is not guaranteed or endorsed by the publisher.
Research integrity at Frontiers
Learn more about the work of our research integrity team to safeguard the quality of each article we publish.