- University of Texas Marine Science Institute, Port Aransas, TX, United States
We examined the patterns of propagule recruitment to assess the timescale and trajectory of succession and the possible roles of physical factors in controlling benthic community structure in a shallow High Arctic kelp bed in the Beaufort Sea, Alaska. Spatial differences in established epilithic assemblages were evaluated against static habitat attributes (depth, distance from river inputs) and environmental factors (temperature, salinity, current speed, underwater light) collected continuously over 2–6 years. Our measurements revealed that bottom waters remained below freezing (mean winter temperatures ∼−1.8°C) and saline (33–36) with negligible light levels for 8–9 months. In contrast, the summer open water period was characterized by variable salinities (22–36), higher temperatures (up to 8–9°C) and measurable irradiance (1–8 mol photons m–2 day–1). An inshore, near-river site experienced strong, acute, springtime drops in salinity to nearly 0 in some years. The epilithic community was dominated by foliose red algae (47–79%), prostrate kelps (2–19%), and crustose coralline algae (0–19%). Strong spatial distinctions among sites included a positive correlation between cover by crustose coralline algae and distance to river inputs, but we found no significant relationships between multi-year means of physical factors and functional groups. Low rates of colonization and the very slow growth rates of recruits are the main factors that contribute to prolonged community development, which augments the influence of low-frequency physical events over local community structure. Mortality during early succession largely determines crustose coralline algal and invertebrate prevalence in the established community, while kelp seem to be recruitment-limited. On scales > 1 m, community structure varies with bathymetry and exposure to freshwater intrusion, which regulate frequency of primary and physiological disturbance. Colonization rates (means of 3.3–69.9 ind. 100 cm–1 year–1 site–1) were much lower than studies in other Arctic kelp habitats, and likely reflect the nature of a truly High Arctic environment. Our results suggest that community development in the nearshore Beaufort Sea occurs over decades, and is affected by combinations of recruitment limitation, primary disturbance, and abiotic stressors. While seasonality exerts strong influence on Arctic systems, static habitat characteristics largely determine benthic ecosystem structure by integrating seasonal and interannual variability over timescales longer than most ecological studies.
Introduction
Kelp bed communities in the Arctic are expected to change dramatically as climate warming reduces ice cover and increases temperatures. Boreal species are expected to shift distributions poleward into the Arctic realm and contribute to predicted increases in primary productivity and biomass in rocky nearshore habitats (Müller et al., 2009; Krause-Jensen et al., 2012; Krause-Jensen and Duarte, 2014; Filbee-Dexter et al., 2019). However, the future of kelp beds adjacent to erosional coasts and rivers may be more uncertain than those along stable, rocky coasts (Filbee-Dexter et al., 2019). In these inner shelf areas, salinity stress from augmented freshwater inputs can reduce kelp photosynthetic capacity and recruitment (Karsten, 2007; Fredersdorf et al., 2009). Increased sediments (Gordeev, 2006; Lantuit et al., 2012; Fritz et al., 2017) that attenuate light (Van Duin et al., 2001; Aumack et al., 2007) and hinder recruitment (Devinny and Volse, 1978; Zacher et al., 2016; Lind and Konar, 2017; Traiger and Konar, 2017) may further obstruct persistence of local kelps. As these conditions characterize a considerable portion of Arctic kelp beds, especially in Alaska and Russia (Lantuit et al., 2012; Filbee-Dexter et al., 2019), baseline data on the physical environment and biological patterns of shallow, river-influenced areas are vital to assess and evaluate the redistribution of Arctic biota and ecosystems.
The Arctic coastal ocean is distinctly seasonal. Event-driven processes that begin with ocean freeze-up in the fall, development of 1.8 m thick shore fast sea ice, followed by a large pulse of terrestrial freshwater transform the physiochemical marine environment from ice-covered to open water in just weeks. Seasonal ice scour is a common ecological disturbance in some areas (Conlan et al., 1998). Sudden, seasonal abiotic shifts may also act as an ecosystem disturbance. The range of conditions experienced over the year, including disturbance rates, could have strong effects on ecosystem characteristics and processes. Priorities for Arctic studies include establishing linkages between abiotic variability and biological ecosystem characteristics. Increased surface air temperatures rapidly shift seasonal patterns in the Arctic (Tedesco et al., 2019) that expand the summer open-water season and reduce ice scour disturbance (Wadhams, 2012). These changes have already altered community structure and ecosystem function in some Arctic marine habitats (Wassmann, 2011; Kortsch et al., 2012; Molis et al., 2019). Shifts in sea ice extent and sea surface temperature—which can be measured remotely—have demonstrable effects on benthic community composition (Beuchel et al., 2006; Grebmeier et al., 2006; Kortsch et al., 2012). Connections between biological characteristics, such as community structure and recruitment, and in situ environmental conditions allow for stronger predictions of Arctic ecosystem responses to climatic warming and increased industrial activity.
The drivers and spatiotemporal patterns of benthic recruitment and succession in the Arctic are currently understood via a handful of studies in Svalbard and Eastern Greenland (Barnes and Kuklinski, 2005; Schmiing, 2005; Beuchel and Gulliksen, 2008; Fricke et al., 2008; Kuklinski et al., 2013; Balazy and Kuklinski, 2017; Meyer et al., 2017), and one in the Beaufort Sea (Konar, 2013). In some habitats, recruitment can occur relatively quickly, such as in shallow, Atlantic-influenced Svalbardian fjords (Meyer et al., 2017), while in other areas, recruitment and community development can proceed very slowly, taking over a decade for cleared experimental plots to resemble the benthic community (Beuchel and Gulliksen, 2008; Konar, 2013; Molis et al., 2019). Kortsch et al. (2012) hypothesized that climate-driven shifts in temperature and ice cover had reduced the resilience of benthic communities in Svalbard by altering patterns of recruitment and succession. These processes are a key component in the future persistence of Arctic kelp beds and may even be more influential in regions where apparent recruitment is low, such as the Beaufort Sea (Konar, 2013).
Successional trajectory is shaped by the interplay between colonization, environmental factors, and species interactions (Connell and Slatyer, 1977). Under the traditional successional framework, “early succession” colonizers are replaced by “late-succession” taxa in community dominance. Through experimentation, this progression of community structure can reveal mechanisms that relate abiotic differences to dissimilarity in benthic communities (e.g., Kroeker et al., 2012, 2013). Abiotic conditions can determine outcomes of species interactions during community development, but can also directly constrain community composition at the outset if species interactions have little to no effect (Connell and Slatyer, 1977). Seasonal abiotic variation can also have large effects on species interactions and preclude deterministic succession (Benincà et al., 2015). Previous work indicates that primary succession can take a decade or more in our study area (Konar, 2013), which could amplify the influence of abiotic variability on community structure.
Differences in community development could result from variation in recruitment, changes in hydrography, or strong abiotic controls on post-recruitment growth and survival. The goal of the present study was to determine the relative influence of these forces in a shallow (<9 m), river-influenced High Arctic kelp bed through an examination of benthic community structure and community development at several sites over a large spatial scale. We paired long-term environmental data at each site with benthic surveys to assess how community composition may reflect spatial variability in both static (depth, distance from river inputs) and dynamic (temperature, salinity, current speed, underwater light) habitat characteristics. Previous studies and our own observations indicate that benthic community structure varies spatially (Martin and Gallaway, 1994), leading us to hypothesize that changing patterns in species assemblages are related to one or more environmental factors. To assess the relative influence of both recruitment and abiotic factors, we followed the trajectory of community development on artificial surfaces and compared our results to the composition of the surrounding established community. We expected that recruited communities would not initially reflect the surrounding benthic community due to differences in taxa-specific life history, but that they would become more similar to established communities over time as succession progressed.
Materials and Methods
Study Area
The Boulder Patch (∼63 km2 of rock cover >10%, Figure 1) is the largest of the discrete kelp beds scattered along the Alaskan Beaufort Sea coast, located in the shallow (∼4–7 m) nearshore waters of Stefansson Sound, east of Prudhoe Bay, and adjacent to the Sagavanirktok River Delta. Stefansson Sound is estuarine in character due to inflow from the Sagavanirktok River and other large rivers (including the Mackenzie River) along the Beaufort Sea coast. Boulder and cobble substrates that characterize the Boulder Patch support a rich epibenthic assemblage with greater diversity than the surrounding soft-sediment habitat (Dunton et al., 1982; Dunton and Schonberg, 2000; Wilce and Dunton, 2014). The diversity and structure of this community also varies within the Boulder Patch (Martin and Gallaway, 1994). The dominant kelp is the Arctic endemic species, Laminaria solidungula; however, Saccharina latissima and Alaria esculanta are also present, though the latter is rare. Foliose red algae, including Coccotylus trucatus, Dilsea socialis, and Phycodrus finbriata form a dense understory throughout much of the Boulder Patch. Nestled amongst the red algae are filter feeders: sponges (such as Halichondria panicea and Semisubrites cribrosa), bryozoans (such as Eucratea loricata), and hyrdoids (Sertularia spp.). The soft coral Gersemia rubiformis is a charismatic community member, and common in certain areas. At some sites, crustose coralline algae (Leptophytum laeve and L. foecundum) coat much of the rock surface unoccupied by the preceding groups, though it is completely absent in other areas. The only significant algal grazers are the chitons Amicula vestita and Stenosemus albus (Dunton et al., 1982; Dunton and Schell, 1987).
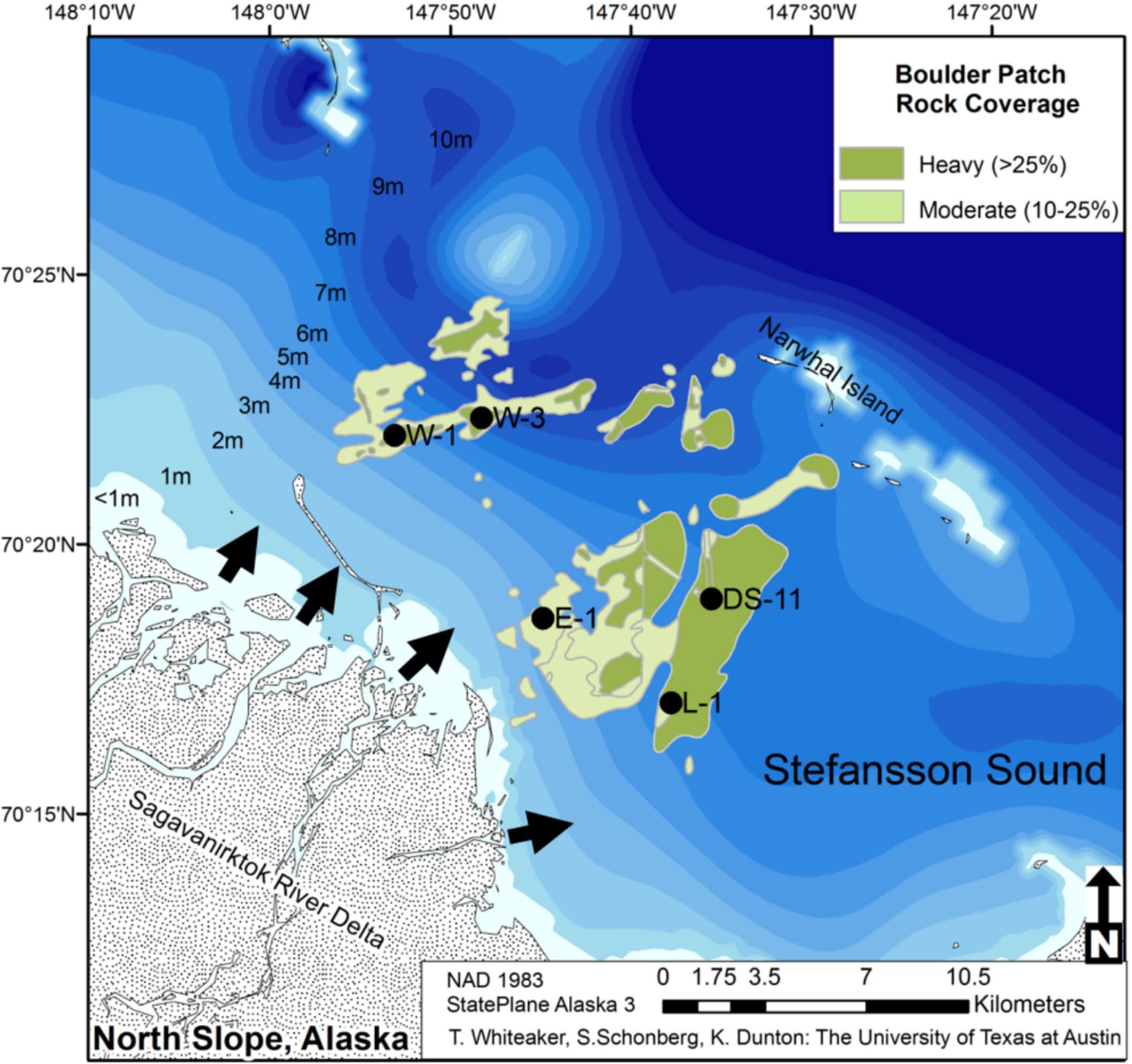
Figure 1. Location of study sites (W1, W3, E1, L1, and DS11) in the Boulder Patch with rock cover indicated in green. Adapted from Bonsell and Dunton (2018). Arrows indicate channel inputs from the Sagavanirktok River.
Study sites (n = 5) were selected from a set of long-term dive sites (e.g., Dunton, 1990; Martin and Gallaway, 1994; Bonsell and Dunton, 2018) in an effort to capture the range of abiotic variability in the Boulder Patch with varying depths, distances to shore, and proximity to the mouth of the Sagavanirktok River (Figure 1). Three sites (DS11, E1, L1) lie between a set of barrier islands (including Narwhal Island) and the mainland, while the other two sites (W1 and W3) are more exposed to open-ocean conditions, located near a deeper-water channel that connects Stefansson Sound to the Beaufort Sea (Figure 1). Sites range from depths of 4.4 m (E1) to 6.6 m (W3), and from 3.5 km (E1) to 9.0 km (DS11) from the mouth of the Sagavanirktok River.
Abiotic Factors
Beneath ice cover, the abiotic environment homogenizes across Stefansson Sound Boulder Patch (Figure 2). We compared spatial patterns during the period of complete ice-cover (December through April) with that of transitional and open periods when abiotic conditions diverged (May through November). Mean values of temperature, salinity, underwater light levels (photosynthetically active radiation, PAR), and current velocity at each site were derived from in situ data from these two periods. Briefly, benthic loggers were deployed at each site for multiple years, bounded by the period of August 2011–August 2017. Photosynthetically active radiation (PAR) was recorded ∼50 cm above the seabed using a LI-COR LI-193SA spherical quantum sensor (instantaneous measurements every minute, averaged every 3 h; accuracy: ±5%) connected to LI-1000, LI-1400, or LI-1500 dataloggers. Sensors were cleaned once a year, as biofouling in this environment is negligible. Some of these data have been incorporated in previous studies to examine multidecadal underwater light patterns in the Boulder Patch (Bonsell and Dunton, 2018). Continuous in situ measurements of conductivity and temperature ∼30 cm above the seabed were collected with HOBO U24 Conductivity/Temperature Loggers (hourly sample interval) and Star Oddi DST loggers (30 min sample interval; accuracy for both loggers: ±0.1°C, ±1.0 salinity). Instantaneous measurements of temperature and conductivity were measured upon deployment and retrieval of loggers using a YSI Data Sonde to calibrate records at each site. Temperature and conductivity were used to calculate salinity (PSS-78). Measurements of temperature, current velocity and current bearing within ∼1.2 m of the bottom was collected using SeaHorse Tilt Current meter fitted with a MAT-1 accelerometer or with a Lowell MAT-1 Tilt Current Meter (30 min sample intervals). Distance from each site to nearest input of a river tributary (Figure 1) was estimated in ArcGIS by the straight linear distance between each site and the nearest land boundary associated with a river channel mouth. Site depths were derived from Aumack et al. (2007) and Bonsell and Dunton (2018).
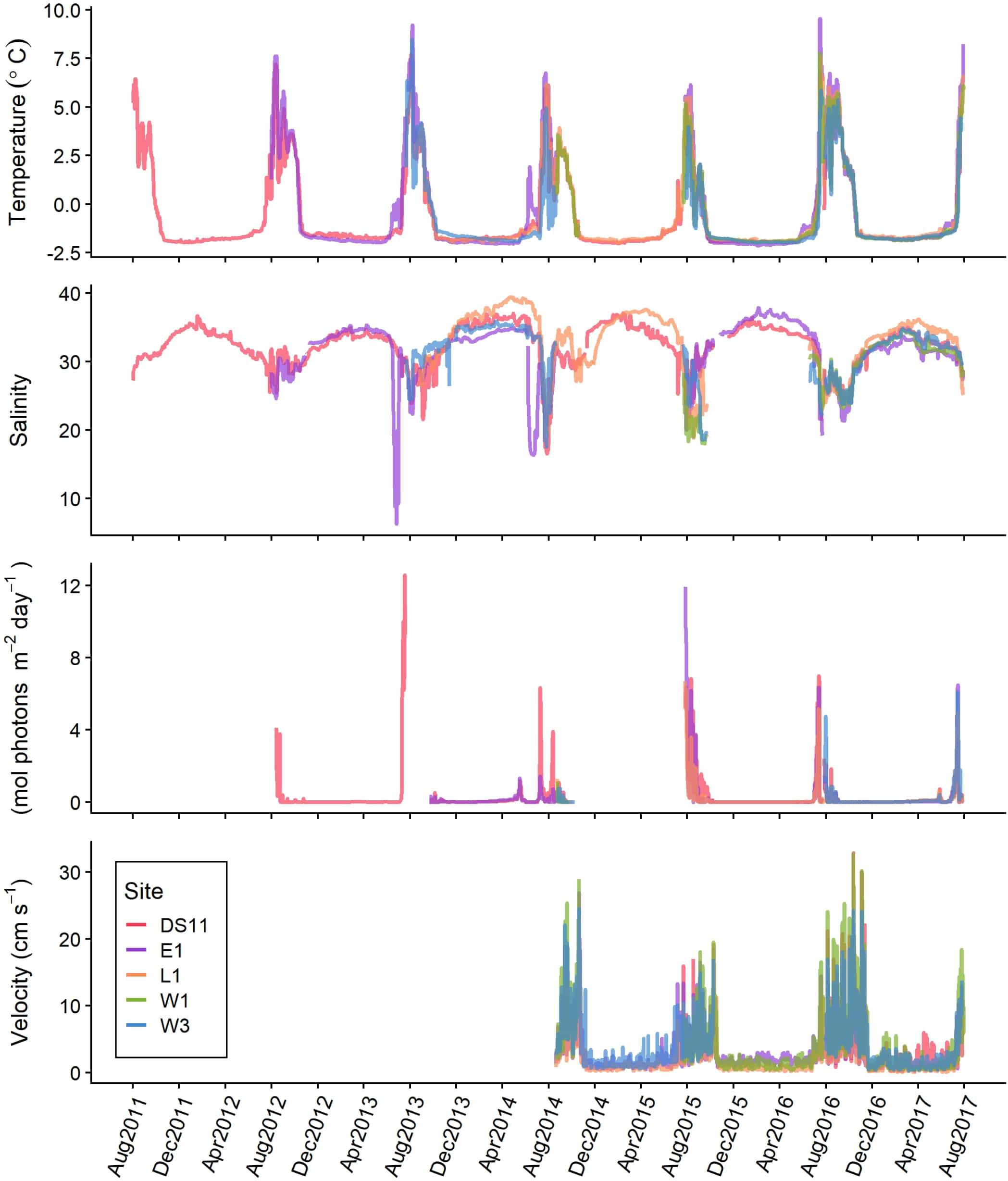
Figure 2. Timeseries of benthic temperature (°C), salinity, photosynthetically active radiation (PAR, mol photons m–2 day–1), and horizontal current velocity (cm s–1) at each site within Stefansson Sound (Figure 1), August 2011–August 2017. Values represent daily mean conditions.
Structure of Established Benthic Community, Recruited Community, and Successional Community
To characterize established benthic community structure at each site, 0.05 m2 photoquadrats were acquired by a diver using a Nikon 1 AW1 waterproof digital camera in July 2016 (sites DS11, E1, W1, and W3) and July 2017 (sites E1, L1, and W1). Divers acquired photos approximately every 2 m while working in a spiral pattern by rising above the benthos and “landing” with the photoquadrat. Photos were analyzed for percent cover to the lowest possible benthic taxonomic group using point intercept of 56 random points stratified in a grid using the online program CoralNet (coralnet.ucsd.edu). Photos with >25% soft sediment or >20% unclear points (photo blur or suspended sediment precluding identification) were removed from the final analysis, resulting in 24–43 photos for each site (n: E1 = 36, DS11 = 31, L1 = 24, W1 = 43, W3 = 35). All data were normalized to percent rock cover in each photoquadrat.
To assess recruitment and succession patterns at each site, settlement tiles (10 × 10 cm fibercement) were deployed in July 2015. Briefly, tiles were attached with cable ties to PVC frames at a ∼30° angle to horizontal and elevated ∼10 cm above the substrate with weights. Each subsequent year, a subset of eight tiles was removed and a new set was deployed by divers (Supplementary Table 1). This provided data from tiles that had been deployed for durations of 1 (2015–2016, 2016–2017, and 2017–2018), 2 (2015–2017 and 2016–2018), and 3 years (2015–2018), with each tile representing an independent unit. Ice scour and adverse diving conditions prevented recovery of some plates (Supplementary Table 1). After retrieval by divers, tiles were placed in individually sealed containers with seawater and transported live to the laboratory on shore where they were examined under a dissecting microscope. Organisms were identified to the lowest taxonomic level possible (often only to phylum or class as dictated by small size) and counted.
Five functional groups were used to evaluate differences in community structure among samples for both the established benthic community and the community from settlement tiles: kelp, crustose coralline algae (hereafter abbreviated as CCA), fleshy (foliose) red algae, filter feeders, and suspension feeders (Table 1). There are various definitions for “filter” and “suspension” feeding, and the two are sometimes used synonymously. Here, these terms are used to separate bryozoans, corals, and hydroids from sponges and ascidians. In this study, suspension feeders were considered species in the former group that extend appendages (cilia or tentacles) into the water column to acquire food particles, while the filter feeders are the latter group which actively pump water through their bodies to feed. These groups also differ in their gross morphology: the filter feeders are generally robust while the suspension feeders are more filamentous. Differences in feeding behavior and morphology may lead to divergent responses to variation in flow and suspended sediments, and use of multiple traits to group biota leads to higher sensitivity when assessing variation in community composition (Bremner et al., 2003).
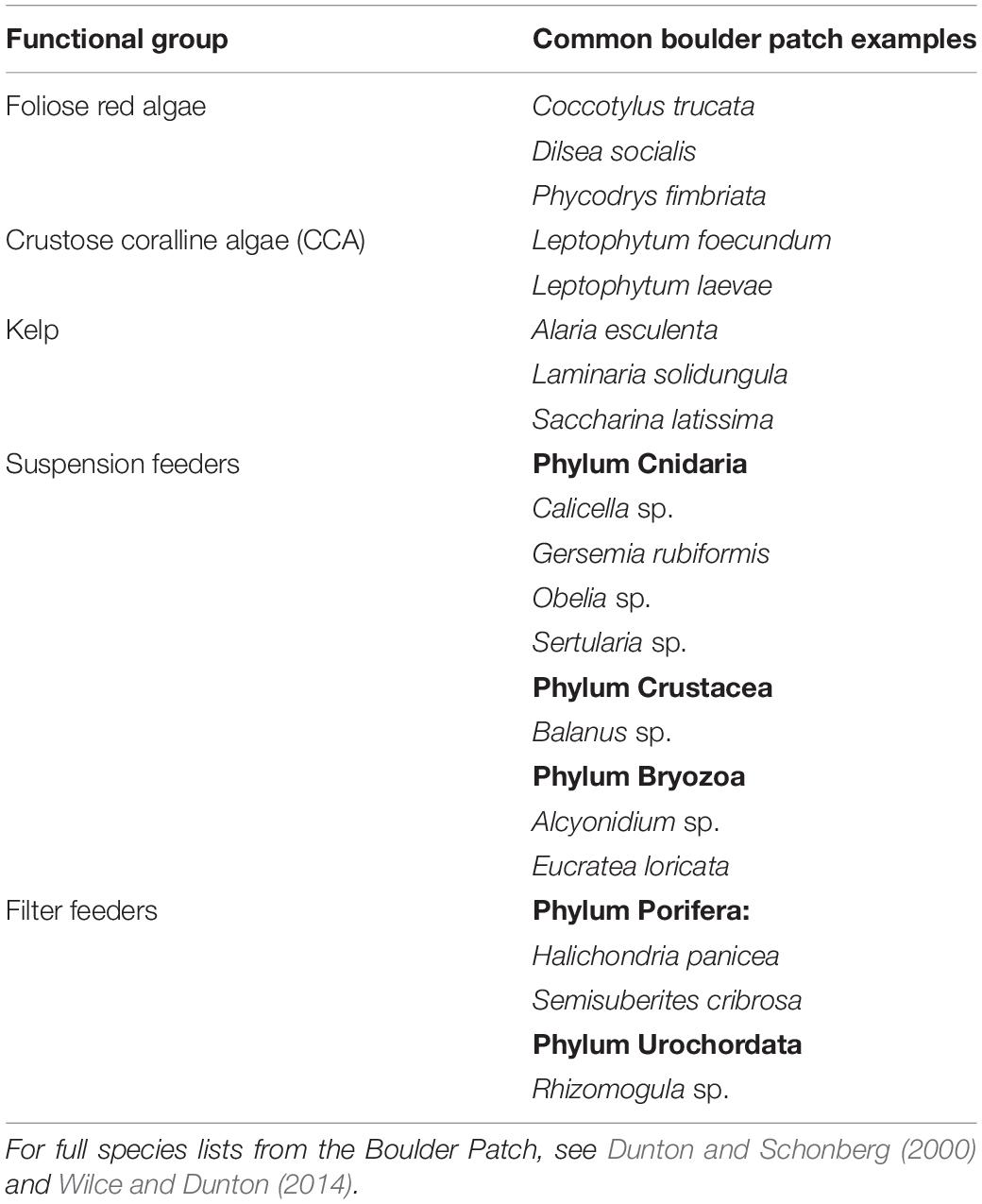
Table 1. Common species and genera from the Boulder Patch associated with each functional group category used in community analysis.
Data Analysis
To determine differences in established benthic communities among sites, a Bray-Curtis matrix was calculated from square-root transformed percent cover data, then analyzed statistically using PERMANOVA as well as visually using a non-metric multidimensional scaling plot. Square-root transformation decreased the number of pairwise differences in homogeneity, but the transformation did not change PERMANOVA results. Differences in total abundance of biota on settlement tiles within sites and within deployment durations (1, 2, and 3 years) were assessed with Welsh one-way ANOVA, or Welsh two-sample t-test, depending on the number of groups. To compare the benthic community to settlement tile communities, percent cover (photoquadrats) and abundance (tiles) data were transformed into proportional abundance of each functional group, then square-root transformed, and a Bray-Curtis matrix was calculated for each. The trajectory of community development on the tiles (i.e., whether it became more similar to the benthic community over time) was evaluated by plotting the pairwise Bray-Curtis dissimilarity of the benthic community and each tile deployment duration group at each site.
The relationship between May through November abiotic characteristics and percent cover by each functional group was assessed with Spearman’s rank-correlations due to non-normality of the variables. The use of correlations to connect the physical environment to biological characteristics is meant to springboard future field studies and experiments. While low sample number (five sites) prevented more rigorous statistical tests, we aim to characterize broad patterns that link abiotic factors to community structure to present a baseline for poorly studied Arctic kelp communities. All data analysis was carried out in R (R Core Team, 2019), and code is available at: https://github.com/cbonsell/bonsell-pubs.
Results
Hydrographic Conditions
Signatures of the annual sea ice cycle and the spring freshet are apparent in the hydrographic data. Generally, sites experienced synchronous variations in all parameters except salinity, though the magnitude of change could vary considerably between sites (Figure 2). Within-site seasonal variation was much larger overall than between-site variation (Figure 2 and Table 2). Bottom waters approached the freezing point (about −1.8°C with salinities ∼33–36) during the period of ice cover, from approximately October through May (Figure 2 and Table 2). At E1, the site most shallow and close to Sagavanirktok River input, distinct and conspicuous increases in temperature and drops in salinity occurred in May 2013 and 2014 (Figure 2). Generally, the summer open water period had variable salinities (22–36) and higher temperatures (up to 8–9°C; Figure 2). Mean daily temperature during May–November was highest at E1 (mean ± SD: 0.6 ± 2.9°C; Table 2). Lowest salinities were recored at E1, the most inshore site (May-November minimums: 6 and 16 in 2013 and 2014, respectively; Figure 2). November through June was prevalently dark, except for small (<2 mol photons m–2 day–1) amounts of light in 2014 and 2017 from March to June, after which PAR returned to 0 before increasing again in July (Figure 2). Maximum daily PAR occurred during July and August but varied by site and year (Figure 2). The highest daily PAR recorded was 12.6 mol photons m–2 day–1 at DS11 (Table 2). Currents were highly variable but reached speeds an order of magnitude larger in the summer (maximum daily means of ∼20–30 cm s–1) than in the winter (maximum daily means <6 cm s–1). Mean current velocity was highest at the deepest sites (W3 and DS11), and the sites closest to a deep channel into Stefansson Sound (W1 and W3; W1: 6 ± 6 cm s–1, W3 and DS11: 5 ± 5 cm s–1; Figures 1, 2 and Table 2).
Established Benthic Community Structure
Benthic community composition (as recorded by photoquadrats) varied among sites (Figures 3–5 and Table 3). The species assemblage at the deepest (6.1 m) and most offshore site, DS11, prominently featured kelp (mostly Laminaria solidungula, though Saccharina latissimia and Alaria esculenta were common) and CCA (Figures 4, 5 and Table 3). The foliose red alga Dilsea socialis was more common at this site than at the shallower sites. Dilsea socialis was also relatively common at the other deep site, W3 (6.6 m), along with Coccotylus truncata and CCA. Gersemia rubiformis was also present at W3, though other invertebrate groups were rare (Figures 4, 5 and Table 3). This contrasts with an adjacent but shallower site (W1; 6.0 m), where G. rubiformis, sponges, hydroids, and bryozoans were relatively common, though the community was dominated by foliose red algae (especially Phycodrys fimbriata) (Figures 4, 5 and Table 3). The shallowest site, E1 (4.4 m), exhibited the highest cover by foliose red algae, dominated by P. fimbriata, though C. truncata was also abundant (Figures 4, 5 and Table 3). Barnacles were recorded in photoquadrats at E1 but neither CCA nor G. rubiformis were noted. Gersemia rubiformis was also absent from L1 and DS11 photoquadrats, though it is commonly observed by divers at DS11. Site L1 was dominated by foliose red algae (as E1) and had the second-highest kelp cover (Figures 4, 5 and Table 3). CCA was present at L1, but rare. All sites had > 10% cover by bare rock, and sites W3 and W1 had over 30% bare rock cover (Table 3).
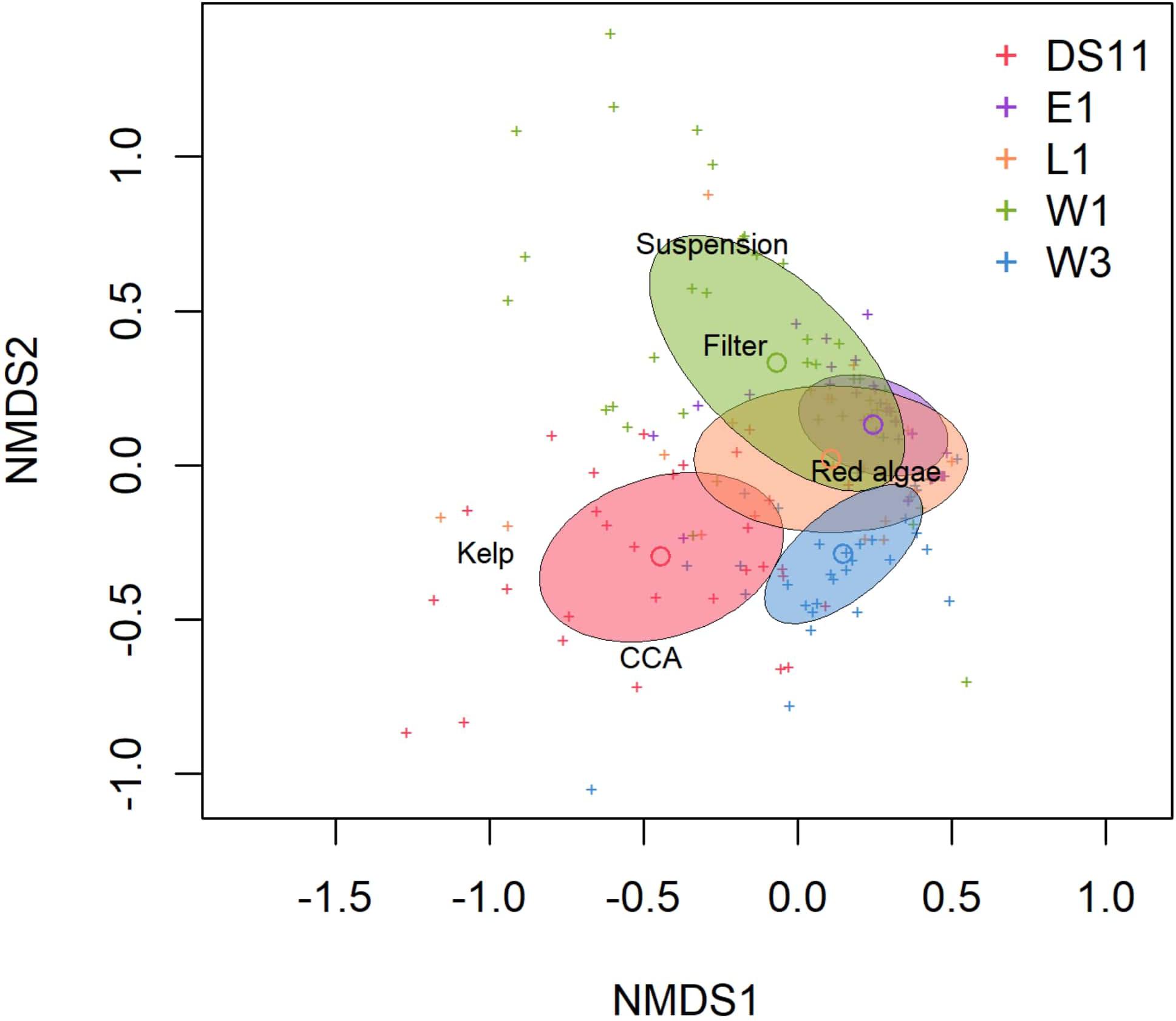
Figure 4. Non-metric multidimensional scaling plot (Bray-Curtis matrix, square-root transform) of benthic community structure by functional group at each site. Center circle in ellipses indicate median community structure; ellipse area indicates standard deviation. Crosses indicate individual photoquadrats. Functional groups names represent their vector of percent cover, such that crosses furthest in that direction have the highest percent cover of that group. 2D stress = 0.15.
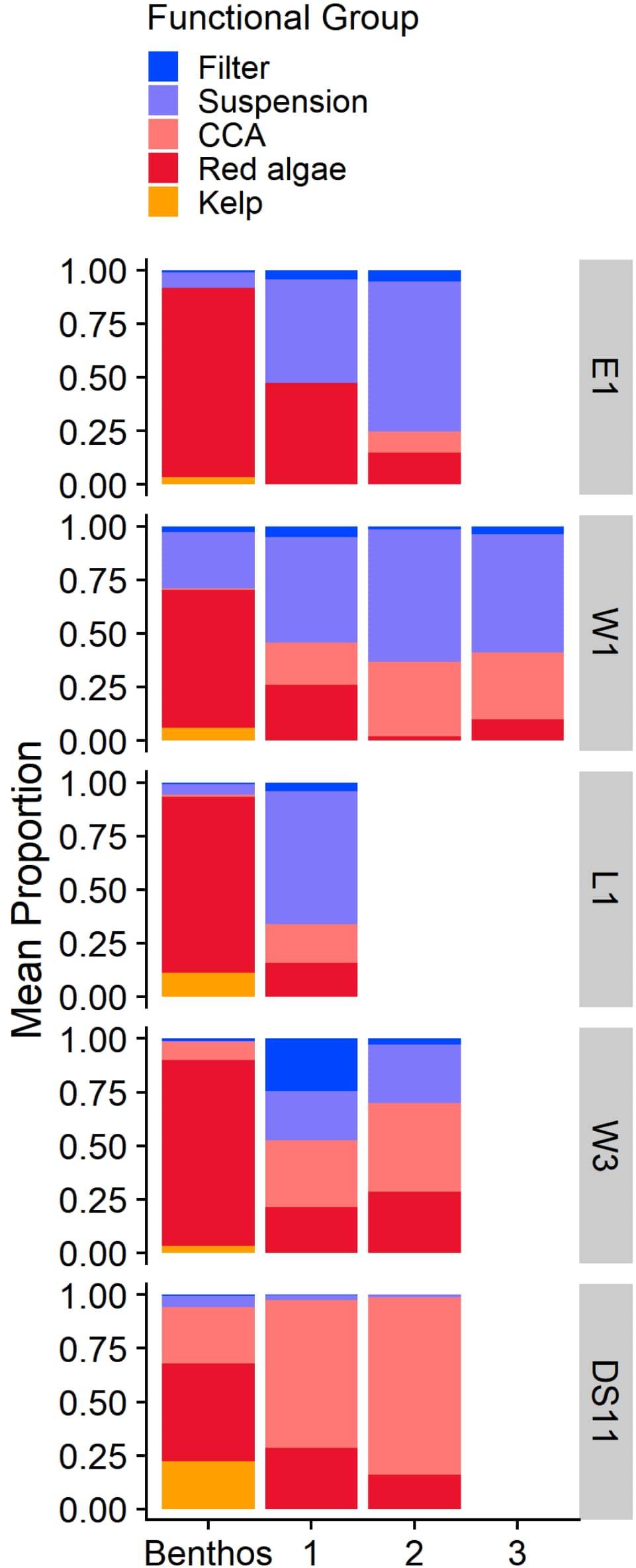
Figure 5. Mean proportion of each functional group at each site based on in situ benthic photoquadrats (Benthos) and settlement tiles by deployment time (1, 2, 3 years). Values represent the proportion of total biotic percent cover for the benthic community (Benthos) and proportional abundance for tile communities. Note that no kelp recruited to settlement tiles. Sites are ordered by distance to river inputs (Table 2).

Table 3. Mean percent cover (±SD) of each functional group in the established benthic community at each site.
Based on photoquadrat data collected over 2 consecutive years at E1 and W1, community structure did not vary between years at each of the two sites (PERMANOVA, p > 0.05), which reflects findings from a 6 years monitoring study performed at DS11 and L1 (Konar, 2013). Consequently, photoquadrat data were combined across both years for E1 and W1 to allow analysis of community differences among all sites by site only. Benthic community structure based on functional groups differed among sites [PERMANOVA, F(4, 164) = 23.45, R2 = 0.36, p < 0.01; Figure 3 and Supplementary Table 2]. Pairwise comparisons demonstrated that all sites were unique from each other, except E1 and L1 (Supplementary Table 3).
Established Community vs. Static and Dynamic Habitat Characteristics
Comparisons between functional group percent cover and environmental variables at each site revealed a positive correlation between CCA cover and distance from Sagavanirktok River channels (ρ = 1.0; Figures 6A,B). Distance from river channels had stronger correlations with functional group percent cover than depth (Figure 6A). There were negative, but non-significant, relationships between mean salinity and cover by both invertebrate groups, and generally positive relationships between invertebrate groups with current velocity and temperature (Figure 6A).
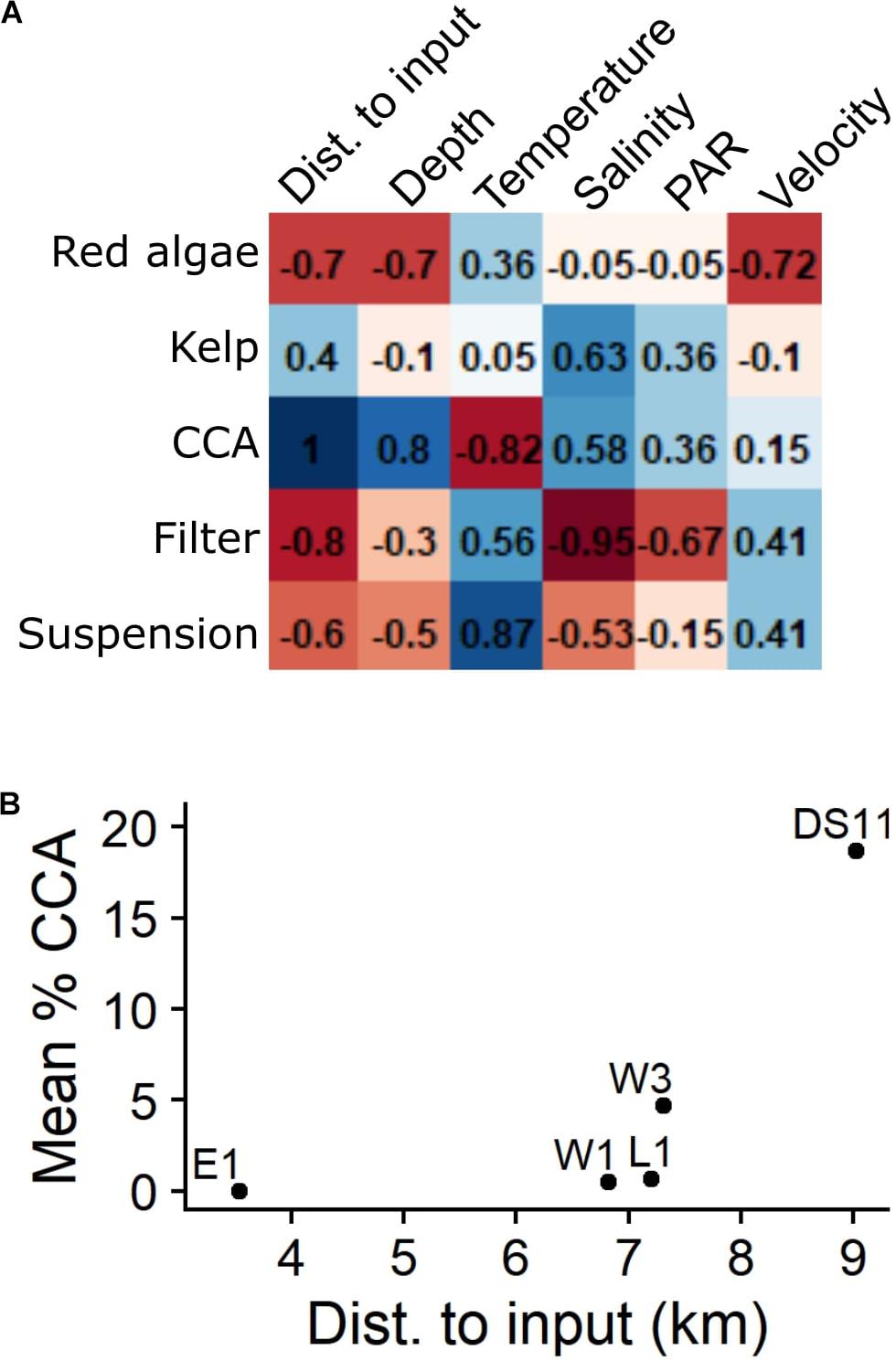
Figure 6. (A) Correlation matrix between percent cover of functional groups and mean environmental variables at each site. Depth and distance to the Sagavanirktok River delta are geographic characteristics, other variables reflect mean May–November conditions (Table 2). Number denotes Spearman rank correlation (ρ); ρ = 1 is statistically significant (p < 0.05). Blue and red color shades depict degree of positive and negative correlation, respectively. (B) Mean percent cover of crustose coralline algae (CCA) in the benthic community at each site compared to distance to nearest river input (see Figure 1).
Settlement Tiles, Recruitment, and Comparisons to Established Community
Abundance of individual biota was extremely variable among settlement tiles. We found that mean overall abundance generally increased over time and was greatest for tiles deployed at DS11, but exhibited high variability (Table 4). At W1, abundance did not increase on tiles deployed continuously over the first 2 years, while at other sites mean abundance approximately doubled or more during the same time frame (Table 4). Although size data was not rigorously recorded, most individuals on all tiles were small (<3 mm).
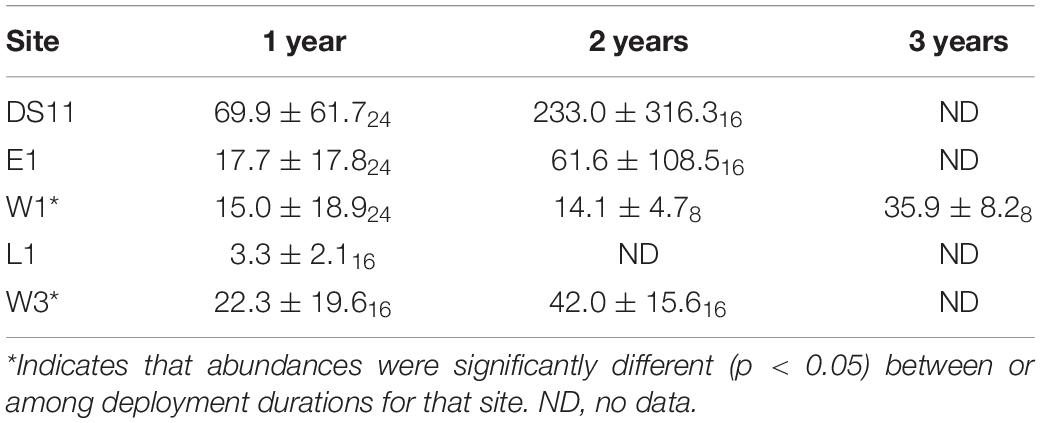
Table 4. Mean total abundance (±SD) of individual biota (number 100 cm–2 settlement tile) and number of tiles for each deployment duration (subscript).
Settlement tile communities varied by site and deployment time (PERMANOVA, p < 0.05). Within each site, tile communities differed among specific years of deployment (e.g., at DS11, the community on 1 year tiles from 2015 to 2016 was different from 1 year tiles from 2016 to 2017) as well as deployment time (pairwise PERMANOVAs, p < 0.05). Within durations, communities were also different among sites (PERMANOVA, p < 0.05), except for 1 year tiles from L1 and W1. Microscopic red algal recruits and juveniles were common on many of the tiles, though they were too small for species identification (Figure 5). Small (usually <1 mm) CCA were also fairly common on tiles at most sites and were also present at E1 (on tiles deployed in 2017–2018 only) despite the absence of this group from the established benthic community at this site (Figure 5). Notably, no juvenile kelp recruited to the tiles.
At DS11, tiles were numerically dominated by CCA, while other sites were dominated by both CCA and/or red algae and suspension feeders (Figure 5). Many filter feeders also recruited to W3 tiles in year 1 (Figure 5). Comparison of tile communities (proportional abundance) to the benthic community (proportional percent cover) demonstrated that tile communities were generally dissimilar to the benthic community, and only became slightly more like the benthic community in year 2 at site W3, and in year 3 at W1 (Figure 7). Tile communities at DS11 were most like the established benthic community. However, tile communities at all sites remained > 40% dissimilar from the surrounding benthic community for the entire study period (mean Bray-Curtis dissimilarity, Figure 7).
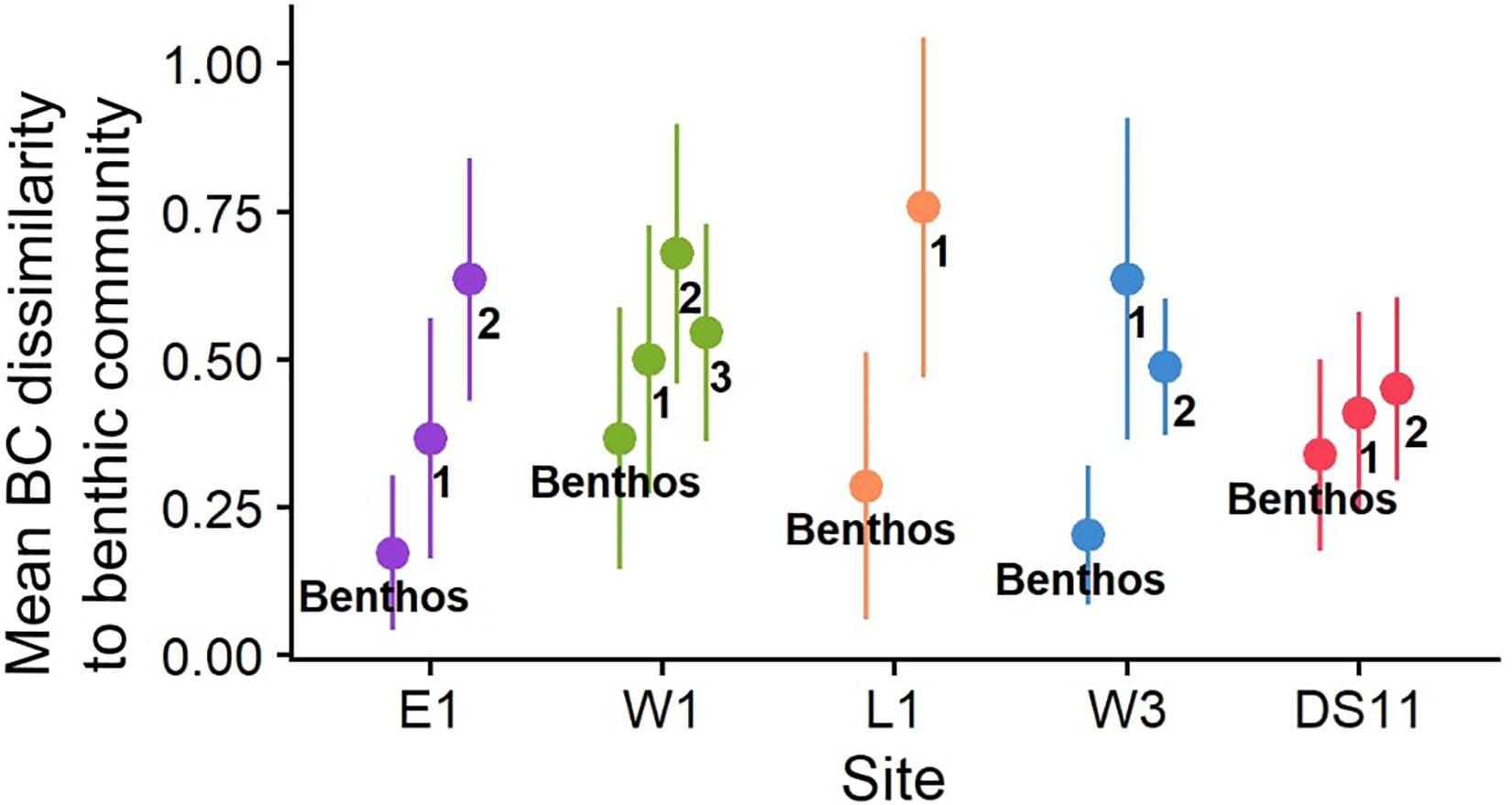
Figure 7. Mean Bray-Curtis (BC) dissimilarity within the established benthic community (“Benthos”) and between the established benthic community and settlement tile communities (points numbered to represent deployment duration in years) as a function of site. For “Benthos” points, higher dissimilarity values reflect higher among-photoquadrat variability within the site. Higher dissimilarity values for numbered points represents higher mean pairwise group dissimilarity between the tile communities and the benthic community. Line ranges indicate SD. Sites are ordered by distance to river inputs (Table 2).
Discussion
Our results show that propagule delivery stemming from interactions between local production and hydrodynamics leads to an initial recruited community, but with recruits remaining small and the resulting assemblage remaining dissimilar from the established community for several years. Kelp is recruitment limited, but other taxa colonized across all sites. Lack of similarity between recruited and established assemblages demonstrated that successional processes shape the epilithic species community. The slow colonization and rates of growth in recruits provide opportunities for both seasonal and spatial differences in the environment to produce distinct species assemblages across the landscape.
Recruitment Patterns
Recruitment and succession can reveal the extent to which community structure is determined by propagule input vs. abiotic control and/or biotic interaction (Connell and Slatyer, 1977). Dissimilarity between benthos and 1 year tiles suggests that the benthic community recruits from a propagule pool mainly derived from resident biota at interior sites (E1, W1, and DS11; Figure 7), but supplemental inputs of propagules from further locations is important at sites on the margins of the Boulder Patch (e.g., L1 and W3; Figure 7). These spatial differences suggest an “edge effect,” where marginal sites receive proportionally greater propagule input from adjacent soft-bottom habitats. Relatively abundant recruits at DS11 (Table 4) may result from advantageous environmental conditions and elevated local propagule production, or simply enhanced priority effects (numerical dominance of local propagules) from high epilithic cover.
Invertebrate taxa and CCA were in higher proportions on settlement tiles than in the benthic community, while sporophyte kelp recruits were entirely absent (Figure 5). Konar (2013) also found no macroscopic kelp recruitment on cleared boulders over a period of 7 years. In another analogous study from the Boulder Patch, the first kelp to recruit to bare boulders only occurred after 7 years (Martin and Gallaway, 1994). Juvenile kelp are also quite rare (Konar, 2013, pers. obs.). In comparison, S. latissima (and some Alaria esculenta) was the dominant colonizer (hundreds of individuals per 225 cm2) after 1 year in an Arctic fjord when free from grazing (Meyer et al., 2017). Even in past experiments in the Boulder Patch where grazer access was minimized experimentally, kelp did not colonize (Konar, 2007). Although kelp is prominent in the benthic community (Figure 5), was reproductive during the time of the study, and readily recruited to tiles in large numbers in the lab (Muth, 2020), it clearly experiences recruitment limitation in situ. This may reflect the level of environmental stress in the Boulder Patch and the species-specific life history strategy for L. solidungula, which develops extremely slowly relative to other kelps (Roleda et al., 2015; Muth, 2020). The low colonization rate of L. solidungula could pose a serious hurdle to the persistence of this species in the face of rapid environmental changes by climatic warming and increased competition by poleward-moving species.
We recorded lower recruitment rates than all other standardized studies of annual colonization rates published for Arctic euphotic zones by order of magnitude or more (Table 5). Comparable studies took place in the Svalbard Archipelago and most at greater depths. Fjord environments in Svalbard differ considerably from those of Arctic inner shelves, with less variable salinity, higher mean temperatures, and shorter periods of ice cover which results in higher annual photon flux (Table 5; Svendsen et al., 2002; Carmack and Wassmann, 2006; Cottier et al., 2010; Bischof et al., 2019). The biogeographical history of these fjords is also different. Kongsfjorden, for example, is considered a sub-Arctic bioregion (Adey and Steneck, 2001; Fredriksen et al., 2014). Additionally, the Boulder Patch’s geographic isolation may result in a “diluted” local propagule pool that constrains recruitment. Clearly, the limited number of recruits sets up a comparatively protracted trajectory to community recovery.
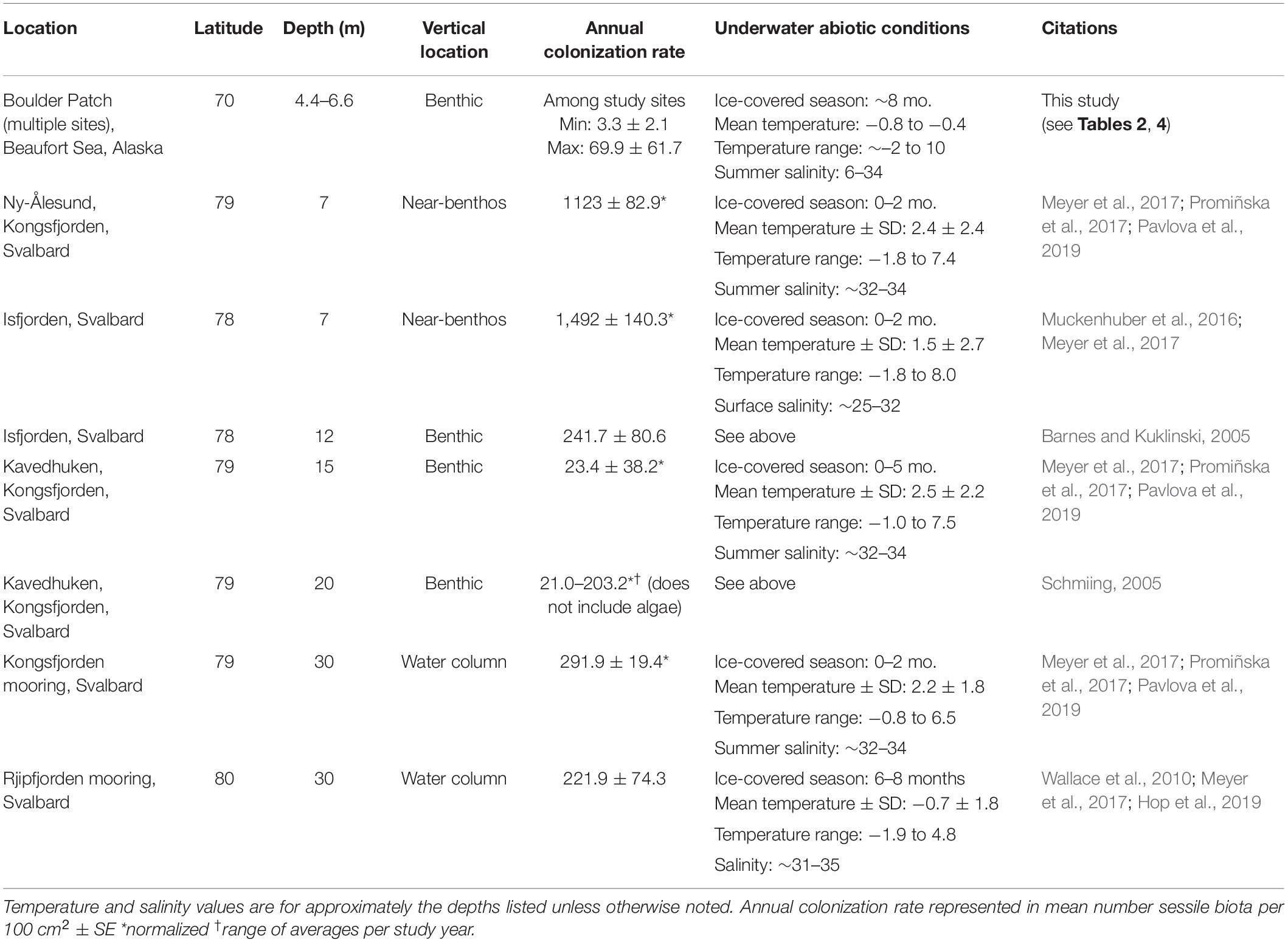
Table 5. Comparison of annual colonization rates from similar studies in Arctic subtidal habitats, ordered by depth.
Community Development
Dominance patterns during community development in the Arctic subtidal also differ among locations and studies. We found that invertebrates dominate developing communities at shallower sites (E1, L1, W1), while algae dominate at deeper sites (W3, DS11; Figure 5). Both Barnes and Kuklinski (2005) and Schmiing (2005) found that invertebrates, especially bryozoans, dominated successional communities at most sites in Kongsfjorden. Interestingly, five of six shallow subtidal sites in Meyer et al. (2017) were dominated by various algae after 1 year, including three locations within Kongsfjorden. A major contrast to our study is the lack of foliose red algae colonization in Svalbard, despite its common presence in the local benthic community (Fredriksen and Kile, 2012; Fredriksen et al., 2014; Bartsch et al., 2016).
In agreement with Meyer et al. (2017), we found that CCA are not a “classically late succession” species in the Arctic as they are in temperate rocky reefs (Foster, 1975). In fact, their tendency for overgrowth (Konar and Iken, 2005; Kuklinski, 2009), early recruitment, capacity for regrowth (Konar, 2013), but limited environmental tolerance (King and Schramm, 1982; Schoenrock et al., 2018) indicate that Arctic CCA act as “early successional crusts” (Steneck, 1986). This life history strategy is particularly advantageous in areas that undergo frequent disturbance. Erratic disturbances (e.g., ice scouring events), inherent to most coastal Arctic environments, likely contribute to dominance by CCA on shallow Arctic rocky reefs (Kuklinski, 2009).
Overall, community development in the Boulder Patch occurs extremely slowly (Tables 4, 5). Tile communities only became more progressively similar to the benthic assemblage over the study period at two sites, W1 and W3, and all tile assemblages were still >40% dissimilar on average to the local benthic community at the study’s end (Figure 7). Even after 2–3 years in situ, recruit abundances were low. The large majority of individuals on tiles remained <1 mm, and overgrowth was negligible, unlike similar studies in Arctic fjords (Table 4; Beuchel and Gulliksen, 2008; Meyer et al., 2017). These slow growth rates prevented assessment of interspecific competition for space during succession. Prolonged community development challenges our ability to describe successional patterns over timescales less than a decade (Martin and Gallaway, 1994; Konar, 2000; Beuchel and Gulliksen, 2008; Meyer et al., 2017).
Spatial Heterogeneity in Abiotic Environment and Resultant Benthic Community Structure
While the Boulder Patch abiotic environment undergoes predictable seasonal changes (Figure 2), interannual variability can result from differing sea ice and meteorological conditions. Static factors such as depth, distance from freshwater inflows, and surrounding bathymetry determine the long-term character and patterns in abiotic conditions at a given location. Consequently, slow forming benthic assemblages undergo multiple years of environmental variation while individuals are still small and competition for space is limited. Benthic community structure therefore reflects an integration of long-term hydrography, which is mediated by static location.
During the spring freshet, Stefansson Sound can become extremely stratified due to river inflow and sea ice melt (Weingartner et al., 2017), with shallow, inshore sites above the halocline (Figure 2 and Table 2). In general, there was a higher proportion of invertebrates at sites closer to the Sagavanirktok River Delta that were characterized by lower mean salinities, while kelp and CCA showed an opposite pattern (Figure 6A). This relationship was strongest with CCA cover, which increased with distance from river inputs (Figure 6B). CCA was a prevalent colonizer at all sites in this study and recruited to E1, but was completely absent from the established community (Figure 5), which demonstrates the importance of post-recruitment processes. While CCAs are sensitive to low pH (e.g., Kuffner et al., 2008), Sagavanirktok River water is considerably basic (Craig and McCart, 1975; Beaufort Lagoon Ecosystems LTER Core Program, 2020; Muth, 2020). Instead, the absence of macroscopic CCA can be linked to low salinity (i.e., low ionic concentration), which inhibits production and calcification (King and Schramm, 1982; Schoenrock et al., 2018). Muth et al. (2020) hypothesized that physiological stress and mortality caused by acute freshwater exposure in certain years prevents these slow growing, calcifying algae from becoming part of the established community. Laminaria solidungula also exhibits reduced photo-physiological performance at low salinities, particularly when combined with warmer temperatures (Karsten, 2007; Diehl et al., 2020), which may contribute to low kelp density at E1 (Figure 5). Salinity gradients structure kelp forests globally (Sundene, 1953; Buschmann et al., 2004; Spurkland and Iken, 2011) and high freshwater and sediment loads are negatively related to kelp recruitment in other high latitude areas (Traiger and Konar, 2017). In shallow reaches of Stefansson Sound, warmer, lower saline waters during the spring and summer favor foliose red algae by impeding the growth of CCA and kelp which are not as physiologically tolerant (King and Schramm, 1982; Harley et al., 2012; Schoenrock et al., 2018).
Bathymetry also affects benthic community structure in the Boulder Patch by influencing local suspended sediment loads, current regimes, and rate of primary disturbance. During the ice-free season, suspended sediment load decreases with depth and distance offshore (Aumack et al., 2007; Bonsell and Dunton, 2018). Although the Boulder Patch is non-depositional over decadal timescales, suspended sediments may smother and abrade epilithic fauna, particularly in shallower areas (Dunton et al., 1982). Water column transparency and PAR is greater offshore, which contributes to higher algal density (Aumack et al., 2007; Bonsell and Dunton, 2018; Figure 5). Current velocities were highest at the deeper sites (W3 and DS11) and the sites nearest to channels into Stefansson Sound (W1 and W3; Figure 1 and Table 2). Current regime determines delivery of propagules, food, and nutrients, and high currents can cause mechanical stress or removal of entire organisms. High current velocities may favor filter and suspension feeders at W1 by providing higher delivery rates of particulate food (Pequegnat, 1964; Sebens, 1984; Witman and Dayton, 2001; Figure 5 and Table 3). Although not quantified directly in this study, prominent bare rock cover at W1 and W3 (Table 3) implies that primary disturbance occurs frequently relative to the scale of recovery (decades). Proximity to the channel exposes these sites to high water velocities and relative lack of protection from islands may increase incidence of ice scouring, a common disturbance in benthic polar habitats (Barnes et al., 1984; Conlan et al., 1998; Beuchel and Gulliksen, 2008; Konar, 2013), and which we directly observed at W3 in 2016 (pers. obs.). High rates of primary disturbance would preclude dominance by slow-growing, but competitively dominant Arctic CCA (Konar and Iken, 2005; Kuklinski, 2009; Schoenrock et al., 2018) and slow-to-establish kelp (Konar, 2013, this study).
Conclusion: Controls on Benthic Community Structure in an Arctic Kelp Bed
Our results agree with the paradigm that recruitment and succession in Arctic subtidal communities is under strong abiotic control (Campana et al., 2009). Community development is limited by the near-freezing, low light environment, which slows recruitment and succession relative to lower latitudes, and then shaped by low-frequency environmental stress and physical disturbance. In the Arctic, communities remain at earlier successional states by the relative frequency of disturbance compared to the long timescale of community development (Molis et al., 2019). The established epilithic community in the Boulder Patch is clearly a “mosaic of patches” of different successional stages across the benthic landscape (sensu Watt, 1947), where rates of primary and physiological disturbance, when superimposed on colonization and community development, produce spatially heterogenous patterns in benthic assemblages (Figure 8). Greater exposure to high currents and open-ocean conditions elevates the rate of primary disturbance, such as at Boulder Patch sites W1 and W3. Low salinity, high turbidity conditions occur more often at inshore sites like E1, L1, and W1, and limits the establishment and persistence of CCA, and likely kelp as well (Muth, 2020). CCAs are early successional (but competitively dominant) taxa while macroscopic kelp appear to be late successional taxa in this environment. While our conclusions are based on ecological observation and limited environmental manipulation, further experimental work can clarify the role of salinity shifts, sedimentation, and rate of disturbance in Arctic kelp beds.
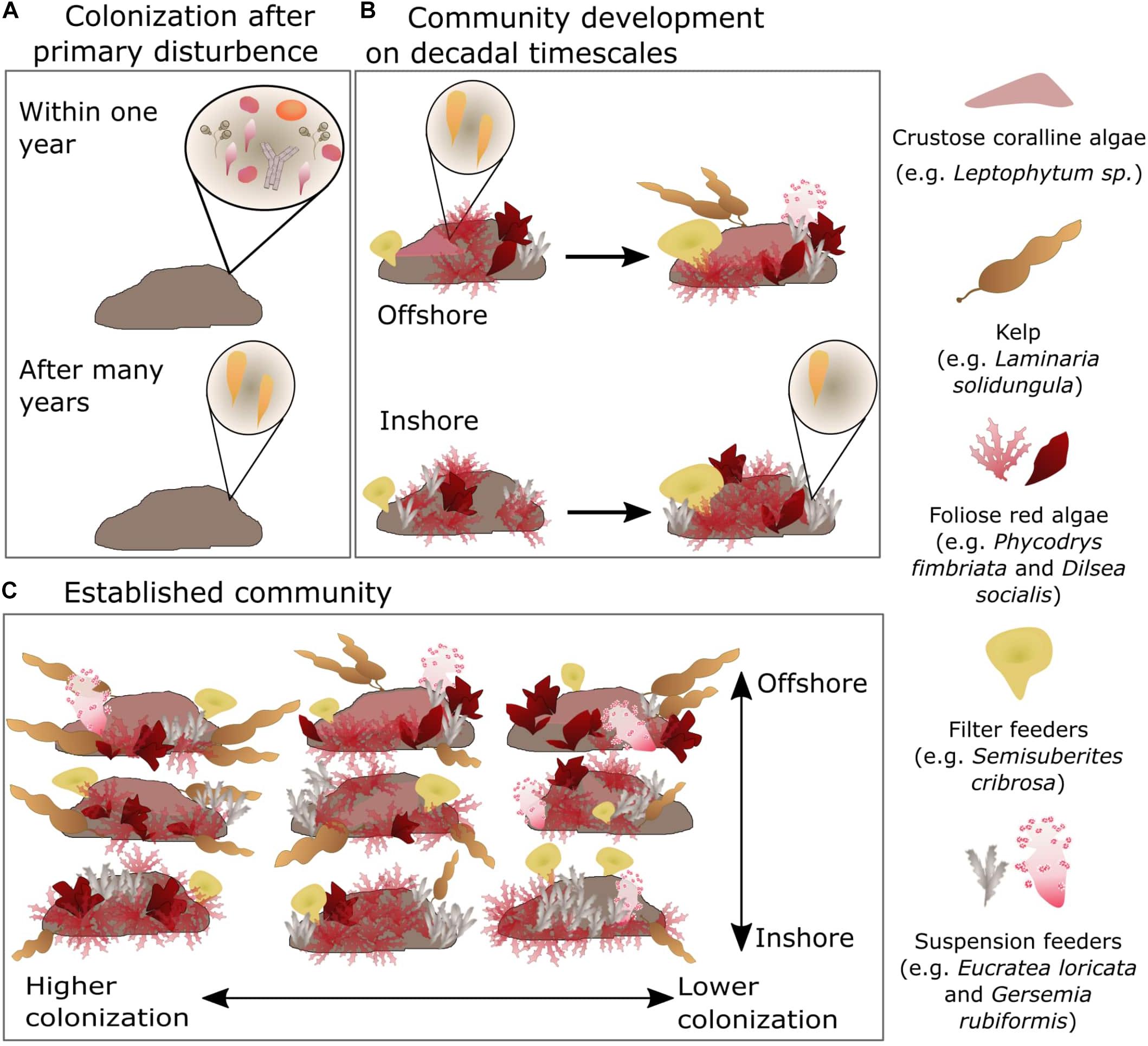
Figure 8. Theorized process by which location and relative colonization rate determine community structure across scales in the Boulder Patch. (A) All functional groups except for kelp colonize primary substrate within 1 year. (B) Slow growth rates reflect pulse events, like freshwater intrusion at inshore sites, can prevent establishment of sensitive taxa (e.g., CCA). (C) Community structure at landscape scales depends on inshore/offshore location and relative rate of colonization compared to rate of primary disturbance.
Arctic Ocean ecosystems are transforming rapidly as climate warming shifts the spatial patterns of species and habitats. Development of the coastline for industry and infrastructure also directly impacts coastal ecosystems. Experimental data from Arctic rocky subtidal habitats pale in comparison to those from temperate latitudes yet are an important foundation for determining mechanisms of ecological patterns. Kelp beds that inhabit the “Arctic” realm cover a range of substrate types, environmental regimes, and biogeographic provinces (Adey et al., 2008; Filbee-Dexter et al., 2019). Slow community development in the Boulder Patch may well represent the true nature of interior Arctic Ocean kelp beds, which are adapted to extremely low annual temperatures and light levels as well as seasonal disruption by ice, freshwater, and sediments. The present study enforces the importance of local factors when considering impacts of climatic changes on kelp habitats (Starko et al., 2019) and suggests that those of the interior Arctic Ocean may be particularly vulnerable. Persistence of ecosystem structure and function in the face of disturbance hinges on whether there is sufficient time for recovery (Margalef, 1969). For High Arctic kelp beds, rapid environmental changes compounded with innately strong seasonality and prolonged community development could easily tip the scales and markedly restructure benthic assemblages.
Data Availability Statement
The datasets presented in this study can be found in online repositories. The names of the repository/repositories and accession number(s) can be found below: Submitted to Arctic Data Center: doi: 10.18739/A23J3922B, doi: 10.18739/A2ZS2KD7B, and doi: 10.18739/A2Q814T07.
Author Contributions
CB and KD designed the study and carried out field work. CB analyzed the data and wrote the manuscript. KD revised and edited the text. Both authors contributed to the article and approved the submitted version.
Funding
This study was funded by the U.S. Department of Interior, Bureau of Ocean Energy and Management (BOEM), Alaska Outer Continental Shelf Region, Anchorage, Alaska under BOEM Cooperative Agreement No. M12AC00007 and from the National Science Foundation (award OPP-1656026).
Conflict of Interest
The authors declare that the research was conducted in the absence of any commercial or financial relationships that could be construed as a potential conflict of interest.
Acknowledgments
We thank C. Coon and D. Prentki for their long and sustained support for the long-term studies in the Boulder Patch. A. P. Bucolo contributed important ideas and support in the nascent days of this project. S. Schonberg provided expertise in invertebrate taxonomy and natural history. A. Muth, J. Dunton, and T. Dunton aided in field work and field levity. Early drafts of this manuscript were improved by thoughtful reviews from K. Iken, A. Muth, and N. McTigue.
Supplementary Material
The Supplementary Material for this article can be found online at: https://www.frontiersin.org/articles/10.3389/fmars.2021.592295/full#supplementary-material
References
Adey, W. H., and Steneck, R. S. (2001). Thermogeography over time creates biogeographic regions: a temperature/space/time-integrated model and an abundance-weighted test for benthic marine algae. J. Phycol. 37, 677–698. doi: 10.1046/j.1529-8817.2001.00176.x
Adey, W. H., Lindstrom, S. C., Max, H. H., and Müller, K. M. (2008). The Biogeographic origin of arctic endemic seaweeds: a thermogeographic view 1. J. Phycol. 44, 1384–1394. doi: 10.1111/j.1529-8817.2008.00605.x
Aumack, C. F., Dunton, K. H., Burd, A. B., Funk, D. W., and Maffione, R. A. (2007). Linking light attenuation and suspended sediment loading to benthic productivity within an arctic kelp-bed community 1. J. Phycol. 43, 853–863. doi: 10.1111/j.1529-8817.2007.00383.x
Balazy, P., and Kuklinski, P. (2017). Journal of experimental marine biology and ecology arctic field experiment shows differences in epifaunal assemblages between natural and artificial substrates of different heterogeneity and origin. J. Exp. Mar. Biolo. Ecol. 486, 178–187. doi: 10.1016/j.jembe.2016.10.012
Barnes, D. K. A., and Kuklinski, P. (2005). Low colonisation on artificial substrata in arctic spitsbergen. Polar Biol. 29, 65–69. doi: 10.1007/s00300-005-0044-y
Barnes, P. W., Rearic, D. M., and Reimnitz, E. (1984). “Ice gouging characteristics and processes,” in The Alaskan Beaufort Sea, eds P. W. Barnes, D. M. Schell, and E. Reimnitz (Amsterdam: Elsevier), 185–212.
Bartsch, I., Paar, M., Fredriksen, S., Schwanitz, M., Daniel, C., Hop, H., et al. (2016). Changes in kelp forest biomass and depth distribution in kongsfjorden, svalbard, between 1996–1998 and 2012–2014 reflect arctic warming. Polar Biol. 39, 2021–2036. doi: 10.1007/s00300-015-1870-1
Beaufort Lagoon Ecosystems LTER, and Core Program, (2020). Physiochemical water column parameters and hydrographic time series from river, lagoon, and open ocean sites along the Alaska Beaufort Sea coast, 2018-ongoing ver 1. Environ. Data Init. doi: 10.6073/pasta/e0e71c2d59bf7b08928061f546be6a9a
Benincà, E., Ballantine, B., Ellner, S. P., and Huisman, J. (2015). Species fluctuations sustained by a cyclic succession at the edge of chaos. Proc. Natl. Acad. Sci. U.S.A. 112, 6389–6394. doi: 10.1073/pnas.1421968112
Beuchel, F., and Gulliksen, B. (2008). Temporal patterns of benthic community development in an arctic fjord (Kongsfjorden, Svalbard): results of a 24-Year Manipulation Study. Polar Biol. 31, 913–924. doi: 10.1007/s00300-008-0429-9
Beuchel, F., Gulliksen, B., and Carroll, M. L. (2006). Long-term patterns of rocky bottom macrobenthic community structure in an arctic fjord (Kongsfjorden, Svalbard) in relation to climate variability (1980–2003). J. Mar. Syst. 63, 35–48. doi: 10.1016/j.jmarsys.2006.05.002
Bischof, K., Buschbaum, C., Fredriksen, S., Gordillo, F. J. L., Heinrich, S., Jiménez, C., et al. (2019). “Kelps and environmental changes in kongsfjorden: stress perception and responses,” in The Ecosystem of Kongsfjorden, Svalbard: Advances in Polar Ecology, Vol. 2, eds H. Hop and C. Wiencke (Cham: Springer International Publishing), 373–422. doi: 10.1007/978-3-319-46425-1_10
Bonsell, C., and Dunton, K. H. (2018). Long-term patterns of benthic irradiance and kelp production in the central beaufort sea reveal implications of warming for arctic inner shelves. Prog. n Oceanogr. 162, 160–170. doi: 10.1016/j.pocean.2018.02.016
Bremner, J., Rogers, S., and Frid, C. (2003). Assessing functional diversity in marine benthic ecosystems: a comparison of approaches. Mar. Ecol. Prog. Ser. 254, 11–25. doi: 10.3354/meps254011
Buschmann, A. H., Vasquez, J. A., Osorio, P., Reyes, E., Filun, L., Hernandez-Gonzalez, M. C., et al. (2004). The effect of water movement, temperature and salinity on abundance and reproductive patterns of Macrocystis Spp. (Phaeophyta) at different latitudes in Chile. Mar. Biol. 145, 849–862. doi: 10.1007/s00227-004-1393-8
Campana, G. L., Zacher, K., Fricke, A., Molis, M., Wulff, A., Quartino, M. L., et al. (2009). Drivers of colonization and succession in polar benthic macro- and microalgal communities. Bot. Mar. 52, 655–667. doi: 10.1515/BOT.2009.076
Carmack, E., and Wassmann, P. (2006). Food webs and physical-biological coupling on pan-arctic shelves: unifying concepts and comprehensive perspectives. Prog. Oceanogr. 71, 446–477. doi: 10.1016/j.pocean.2006.10.004
Conlan, K. E., Lenihan, H. S., Kvitek, R. G., and Oliver, J. S. (1998). Ice scour disturbance to benthic communities in the canadian high arctic. Mar. Ecol. Prog. Ser. 166, 1–16. doi: 10.3354/meps166001
Connell, J. H., and Slatyer, R. O. (1977). Mechanisms of succession in natural communities and their role in community stability and organization. Am. Nat. 111, 1119–1144.
Cottier, F. R., Nilsen, F., Skogseth, R., Tverberg, V., Skarðhamar, J., and Svendsen, H. (2010). Arctic Fjords: a review of the oceanographic environment and dominant physical processes. Geol. Soc. Lond. Spec. Publ. 344, 35–50. doi: 10.1144/SP344.4
Craig, P. C., and McCart, P. J. (1975). Classification of Stream types in beaufort sea drainages between prudhoe bay, Alaska, and the Mackenzie Delta, N. W. T., Canada. Arct. Alp. Res. 7, 183–198. doi: 10.2307/1550320
Devinny, J. S., and Volse, L. A. (1978). Effects of sediments on the development of Macrocystis pyrifera gametophytes. Mar. Biol. 348, 343–348.
Diehl, N., Karsten, U., and Bischof, K. (2020). Impacts of combined temperature and salinity stress on the endemic arctic brown seaweed laminaria solidungula j. agardh. Polar Biol. 43, 647–656. doi: 10.1007/s00300-020-02668-5
Dunton, K. H. (1990). Growth and production in Laminaria solidungula: relation to continuous underwater light Levels in the Alaskan High Arctic. Mar. Biol. 106, 297–304. doi: 10.1007/BF01314813
Dunton, K. H., Reimnitz, E., and Schonberg, S. V. (1982). An arctic kelp community in the alaskan beaufort sea. Arctic 35, 465–484. doi: 10.14430/arctic2355
Dunton, K. H., and Schell, D. M. (1987). Dependence of consumers on macroalgal (Laminaria solidungula) carbon in an arctic kelp community: D 13 C evidence. Mar. Biol. 93, 615–625. doi: 10.1007/BF00392799
Dunton, K. H., and Schonberg, S. V. (2000). “The benthic faunal assemblage of the boulder patch kelp community,” in The Natural History of an Arctic Oil Field, eds J. C. Truett and S. R. Johnson (Amsterdam: Elsevier), 371–397. doi: 10.1016/B978-012701235-3/50020-9
Filbee-Dexter, K., Wernberg, T., Fredriksen, S., Norderhaug, K. M., and Pedersen, M. F. (2019). Arctic kelp forests: diversity, resilience and future. Glob. Planet. Change 172, 1–14. doi: 10.1016/j.gloplacha.2018.09.005
Foster, M. S. (1975). Algal succession in a macrocystis pyrifera forest. Mar. Biol. 32, 313–329. doi: 10.1007/BF00388989
Fredersdorf, J., Müller, R., Becker, S., Wiencke, C., and Bischof, K. (2009). Interactive effects of radiation, temperature and salinity on different life history stages of the arctic kelp alaria esculenta (Phaeophyceae). Oecologia 160, 483–492. doi: 10.1007/s00442-009-1326-9
Fredriksen, S., Bartsch, I., and Wiencke, C. (2014). New additions to the benthic marine flora of kongsfjorden, western svalbard, and comparison between 1996/1998 and 2012/2013. Bot. Mar. 57, 203–216. doi: 10.1515/bot-2013-0119
Fredriksen, S., and Kile, M. R. (2012). The algal vegetation in the outer part of isfjorden, spitsbergen: revisiting per svendsen’s sites 50 years later. Polar Res. 31, 1–9. doi: 10.3402/polar.v31i0.17538
Fricke, A., Molis, M., Wiencke, C., Valdivia, N., and Chapman, A. S. (2008). Natural succession of macroalgal-dominated epibenthic assemblages at different water depths and after transplantation from deep to shallow water on spitsbergen. Polar Biol. 31, 1191–1203. doi: 10.1007/s00300-008-0458-4
Fritz, M., Vonk, J. E., and Lantuit, H. (2017). Collapsing arctic coastlines. Nat. Clim. Change 7, 6–7. doi: 10.1038/nclimate3188
Gordeev, V. V. (2006). Fluvial sediment flux to the arctic ocean. Geomorphology 80, 94–104. doi: 10.1016/j.geomorph.2005.09.008
Grebmeier, J. M., Cooper, L. W., Feder, H. M., and Sirenko, B. I. (2006). Ecosystem dynamics of the pacific-influenced northern bering and chukchi seas in the amerasian arctic. Prog. Oceanogr. 71, 331–361. doi: 10.1016/j.pocean.2006.10.001
Harley, C. D. G., Anderson, K. M., Demes, K. W., Jorve, J. P., Kordas, R. L., Coyle, T. A., et al. (2012). Effects of climate change on global seaweed communities. J. Phycol. 48, 1064–1078. doi: 10.1111/j.1529-8817.2012.01224.x
Hop, H., Assmy, P., Wold, A., Sundfjord, A., Daase, M., Duarte, P., et al. (2019). Pelagic ecosystem characteristics across the atlantic water boundary current from rijpfjorden, svalbard, to the arctic ocean during summer (2010–2014). Front. Mar. Sci. 6:181. doi: 10.3389/fmars.2019.00181
Karsten, U. (2007). Research note: salinity tolerance of arctic kelps from spitsbergen. Phycol. Res. 55, 257–262. doi: 10.1111/j.1440-1835.2007.00468.x
King, R. J., and Schramm, W. (1982). Calcification in the maerl coralline alga phymatolithon-calcareum - effects of salinity and temperature. Mar. Biol. 70, 197–204. doi: 10.1007/BF00397685
Konar, B. (2000). Seasonal inhibitory effects of marine plants on sea urchins: structuring communities the algal way. Oecologia 125, 208–217.
Konar, B. (2007). Recolonization of a high latitude hard-bottom nearshore community. Polar Biol. 30, 663–667. doi: 10.1007/s00300-007-0261-7
Konar, B. (2013). Lack of recovery from disturbance in high-arctic boulder communities. Polar Biol. 36, 1205–1214. doi: 10.1007/s00300-013-1340-6
Konar, B., and Iken, K. (2005). Competitive dominance among sessile marine organisms in a high arctic boulder community. Polar Biol. 29, 61–64. doi: 10.1007/s00300-005-0055-8
Kortsch, S., Primicerio, R., Beuchel, F., Renaud, P. E., Rodrigues, J., Lønne, O. J., et al. (2012). Climate-driven regime shifts in arctic marine benthos. Proc. Natl. Acad. Sci. U.S.A. 109, 14052–14057. doi: 10.1073/pnas.1207509109
Krause-Jensen, D., and Duarte, C. M. (2014). Expansion of vegetated coastal ecosystems in the future arctic. Front. Mar. Sci. 1:77. doi: 10.3389/fmars.2014.00077
Krause-Jensen, D., Marbà, N., Olesen, B., Sejr, M. K., Christensen, P. B., Rodrigues, J., et al. (2012). Seasonal sea Ice cover as principal driver of spatial and temporal variation in depth extension and annual production of kelp in greenland. Glob. Change Biol. 18, 2981–2994. doi: 10.1111/j.1365-2486.2012.02765.x
Kroeker, K. J., Gambi, M. C., and Micheli, F. (2013). Community dynamics and ecosystem simplification in a High-CO2 Ocean. Proc. Natl. Acad. Sci. U.S.A. 110, 12721–12726. doi: 10.1073/pnas.1216464110
Kroeker, K. J., Micheli, F., and Gambi, M. C. (2012). Ocean acidification causes ecosystem shifts via altered competitive interactions. Nat. Clim. Change 2, 1–4. doi: 10.1038/nclimate1680
Kuffner, I. B., Andersson, A. J., Jokiel, P. L., Rodgers, K. S., and Mackenzie, F. T. (2008). Decreased abundance of crustose coralline algae due to ocean acidification. Nat. Geosci. 1, 114–117. doi: 10.1038/ngeo100
Kuklinski, P. (2009). Ecology of stone-encrusting organisms in the greenland sea-a review. Polar Res. 28, 222–237. doi: 10.1111/j.1751-8369.2009.00105.x
Kuklinski, P., Berge, J., McFadden, L., Dmoch, K., Zajaczkowski, M., Nygård, H., et al. (2013). Seasonality of occurrence and recruitment of arctic marine benthic invertebrate larvae in relation to environmental variables. Polar Biol. 36, 549–560. doi: 10.1007/s00300-012-1283-3
Lantuit, H., Overduin, P. P., Couture, N., Wetterich, S., Aré, F., Atkinson, D., et al. (2012). The arctic coastal dynamics database: a new classification scheme and statistics on arctic permafrost coastlines. Estuar. Coast. 35, 383–400. doi: 10.1007/s12237-010-9362-6
Lind, A. C., and Konar, B. (2017). Effects of abiotic stressors on kelp early life-history stages. Algae 32, 223–233. doi: 10.4490/algae.2017.32.8.7
Margalef, R. (1969). Diversity and stability: a practical proposal and a model of interdependence. Brookhav. Symp. Biol. 22, 25–37.
Martin, L. R., and Gallaway, B. J. (1994). The effects of the endicott development project on the boulder patch, an arctic kelp community in stefansson sound. Alaska. Arctic 47, 54–64.
Meyer, K. S., Sweetman, A. K., Kuklinski, P., Leopold, P., Vogedes, D., Berge, J., et al. (2017). Recruitment of benthic invertebrates in high arctic Fjords: relation to temperature, depth, and season. Limnol. Oceanogr. 62, 2732–2744. doi: 10.1002/lno.10602
Molis, M., Beuchel, F., Laudien, J., łodarska-Kowalczuk, M. W., and Buschbaum, C. (2019). “Ecological drivers of and responses by arctic benthic communities, with an emphasis on Kongsfjorden, Svalbard,” in The Ecosystem of Kongsfjorden, Svalbard, Vol. 2, eds H. Hop, and C. Wiencke, (Berlin: Springer International Publishing), 423–481. doi: 10.1007/978-3-319-46425-1_11
Muckenhuber, S., Nilsen, F., Korosov, A., and Sandven, S. (2016). Sea Ice Cover in Isfjorden and Hornsund, Svalbard (2000–2014) from remote sensing data. Cryosph. 10, 149–158. doi: 10.5194/tc-10-149-2016
Müller, R., Laepple, T., Bartsch, I., and Wiencke, C. (2009). Impact of oceanic warming on the distribution of seaweeds in polar and cold-temperate waters. Bot. Mar. 52, 617–638. doi: 10.1515/BOT.2009.080
Muth, A. (2020). Salinity and pH Variations in the Nearshore Arctic Ocean: Implications for Benthic Species Physiology and Biodiversity. Dissertation, University of Texas at Austin, Austin, TX.
Muth, A. F., Esbaugh, A. J., and Dunton, K. H. (2020). Physiological responses of an arctic crustose coralline alga (Leptophytum foecundum) to variations in salinity. Front. Plant Sci. 11:1272. doi: 10.3389/fpls.2020.01272
Pavlova, O., Gerland, S., and Hop, H. (2019). “Changes in sea-ice extent and thickness in Kongsfjorden, Svalbard (2003–2016),” in The Ecosystem of Kongsfjorden, Svalbard, eds H. Hop and C. Wiencke, (Cham: Springer International Publishing), 105–136. doi: 10.1007/978-3-319-46425-1_4
Promińska, A., Cisek, M., and Walczowski, W. (2017). Kongsfjorden and hornsund hydrography – comparative study based on a multiyear survey in Fjords of West Spitsbergen. Oceanologia 59, 397–412. doi: 10.1016/j.oceano.2017.07.003
R Core Team (2019). R: A Language and Environment for Statistical Computing. Vienna: R Foundation for Statistical Computing.
Roleda, M. Y., Cornwall, C. E., Feng, Y., McGraw, C. M., Smith, A. M., Hurd, C. L. et al. (2015). Effect of ocean acidification and PH fluctuations on the growth and development of coralline algal recruits, and an associated benthic algal assemblage. PLoS One 10:140394. doi: 10.1371/journal.pone.0140394
Schmiing, M. (2005). Sukzession in benthischen makrofuana-gemeinschaften Der Arktis - Ein Jahresvergleich. Alfred Wegner Inst. 110, 1–110. doi: 10.1594/PANGAEA.351151
Schoenrock, K. M., Bacquet, M., Pearce, D., Rea, B. R., Schofield, J. E., Lea, J., et al. (2018). Influences of salinity on the physiology and distribution of the Arctic Coralline Algae, Lithothamnion glaciale (Corallinales, Rhodophyta). J. Phycol. 702, 690–702. doi: 10.1111/jpy.12774
Sebens, K. P. (1984). Water flow and coral colony size: interhabitat comparisons of the octocoral Alcyonium siderium. Proc. Natl. Acad. Sci. U.S.A. 81, 5473–5477. doi: 10.1073/pnas.81.17.5473
Spurkland, T., and Iken, K. (2011). Kelp bed dynamics in estuarine environments in Subarctic Alaska. J. Coast. Res. 275, 133–143. doi: 10.2112/JCOASTRES-D-10-00194.1
Starko, S., Bailey, L. A., Creviston, E., James, K. A., Warren, A., Brophy, M. K., et al. (2019). Environmental heterogeneity mediates scale-dependent declines in kelp diversity on intertidal rocky shores. PLoS One 14:e0213191. doi: 10.1371/journal.pone.0213191
Steneck, R. (1986). The ecology of coralline algal crusts: convergent patterns and adaptative strategies. Annu. Rev. Ecol. Syst. 17, 273–303. doi: 10.1146/annurev.ecolsys.17.1.273
Svendsen, H., Beszczynska-Møller, A., Hagen, J. O., Lefauconnier, B., Tverberg, V., Gerland, S., et al. (2002). The physical environment of Kongsfjorden–Krossfjorden, an arctic fjord system in svalbard. Polar Res. 34, 133–166.
Tedesco, L., Vichi, M., and Scoccimarro, E. (2019). Sea-Ice algal phenology in a warmer arctic. Sci. Adv. 5:eaav4830. doi: 10.1126/sciadv.aav4830
Traiger, S. B., and Konar, B. (2017). Supply and survival: glacial melt imposes limitations at the kelp microscopic life stage. Bot. Mar. 60, 603–617. doi: 10.1515/bot-2017-0039
Van Duin, E. H. S., Blom, G., Los, F. J. S., Maffione, R., Zimmerman, R., Cerco, C. F., et al. (2001). Modeling underwater light climate in relation to sedimentation, resuspension, water quality and autotrophic growth. Hydrobiologia 444, 25–42. doi: 10.1023/A:1017512614680
Wadhams, P. (2012). New predictions of extreme keel depths and scour frequencies for the beaufort Sea Using Ice thickness statistics. Cold Reg. Scie. Technol. 76–77, 77–82. doi: 10.1016/j.coldregions.2011.12.002
Wallace, M. I., Cottier, F. R., Berge, J., Tarling, G. A., Griffiths, C., and Brierley, A. S. (2010). Comparison of zooplankton vertical migration in an ice-free and a seasonally ice-covered arctic Fjord: an insight into the influence of sea Ice cover on zooplankton behavior. Limnol. Oceanogr. 55, 831–845. doi: 10.4319/lo.2010.55.2.0831
Wassmann, P. (2011). Arctic marine ecosystems in an era of rapid climate change. Prog. Oceanogr. 90, 1–17. doi: 10.1016/j.pocean.2011.02.002
Weingartner, T. J., Danielson, S. L., Potter, R. A., Trefry, J. H., Mahoney, A., Savoie, M., et al. (2017). Circulation and water properties in the landfast Ice Zone of the Alaskan Beaufort Sea. Contin. Shelf Res. 148, 185–198. doi: 10.1016/j.csr.2017.09.001
Wilce, R. T., and Dunton, K. H. (2014). The boulder patch (North Alaska, Beaufort Sea) and Its Benthic Algal Flora. Arctic 67, 43–56.
Witman, J. D., and Dayton, P. K. (2001). “Rocky subtidal communities,” in Marine Community Ecology, eds M. D. Bertness, S. D. Gaines, and M. E. Hay (Sunderland, MA: Sinauer Associates, Inc.), 339–366.
Keywords: benthic ecology, community structure, arctic, kelp, coastal oceanography, recruitment
Citation: Bonsell C and Dunton KH (2021) Slow Community Development Enhances Abiotic Limitation of Benthic Community Structure in a High Arctic Kelp Bed. Front. Mar. Sci. 8:592295. doi: 10.3389/fmars.2021.592295
Received: 06 August 2020; Accepted: 07 January 2021;
Published: 18 February 2021.
Edited by:
Heliana Teixeira, University of Aveiro, PortugalReviewed by:
João N. Franco, Center for Marine and Environmental Sciences (MARE-IPLeiria), PortugalNelson Valdivia, Universidad Austral de Chile, Chile
Copyright © 2021 Bonsell and Dunton. This is an open-access article distributed under the terms of the Creative Commons Attribution License (CC BY). The use, distribution or reproduction in other forums is permitted, provided the original author(s) and the copyright owner(s) are credited and that the original publication in this journal is cited, in accordance with accepted academic practice. No use, distribution or reproduction is permitted which does not comply with these terms.
*Correspondence: Christina Bonsell, Y2JvbnNlbGxAdXRleGFzLmVkdQ==