Corrigendum: Imprint of Climate Change on Pan-Arctic Marine Vegetation
- 1Arctic Research Centre, Aarhus University, Århus, Denmark
- 2Department of Bioscience, Aarhus University, Silkeborg, Denmark
- 3ArcticNet, Québec-Océan, Université Laval, Québec, QC, Canada
- 4Centre of Marine Sciences, University of Algarve, Faro, Portugal
- 5Alfred-Wegener Institute, Helmholtz Centre for Polar and Marine Research, Bremerhaven, Germany
- 6Department of Marine Botany, Faculty of Biology/Chemistry and MARUM, University of Bremen, Bremen, Germany
- 7Institute of Marine Research, His, Norway
- 8Marine Science Institute, University of Texas at Austin, Port Aransas, TX, United States
- 9Shirshov Institute of Oceanology, Moscow, Russia
- 10Icelandic Institute of Natural History, Akureyri, Iceland
- 11Department of Bioscience, Aarhus University, Roskilde, Denmark
- 12Greenland Climate Research Centre, Greenland Institute of Natural Resources, Nuuk, Greenland
- 13Red Sea Research Center, King Abdullah University of Science and Technology, Thuwal, Saudi Arabia
The Arctic climate is changing rapidly. The warming and resultant longer open water periods suggest a potential for expansion of marine vegetation along the vast Arctic coastline. We compiled and reviewed the scattered time series on Arctic marine vegetation and explored trends for macroalgae and eelgrass (Zostera marina). We identified a total of 38 sites, distributed between Arctic coastal regions in Alaska, Canada, Greenland, Iceland, Norway/Svalbard, and Russia, having time series extending into the 21st Century. The majority of these exhibited increase in abundance, productivity or species richness, and/or expansion of geographical distribution limits, several time series showed no significant trend. Only four time series displayed a negative trend, largely due to urchin grazing or increased turbidity. Overall, the observations support with medium confidence (i.e., 5–8 in 10 chance of being correct, adopting the IPCC confidence scale) the prediction that macrophytes are expanding in the Arctic. Species distribution modeling was challenged by limited observations and lack of information on substrate, but suggested a current (2000–2017) potential pan-Arctic brown macroalgal distribution area of 655,111 km2 (140,433 km2 intertidal, 514,679 km2 subtidal), representing an increase of about 45% for subtidal- and 8% for intertidal macroalgae since 1940–1950, and associated polar migration rates averaging 18–23 km decade–1. Adjusting the potential macroalgal distribution area by the fraction of shores represented by cliffs halves the estimate (340,658 km2). Warming and reduced sea ice cover along the Arctic coastlines are expected to stimulate further expansion of marine vegetation from boreal latitudes. The changes likely affect the functioning of coastal Arctic ecosystems because of the vegetation’s roles as habitat, and for carbon and nutrient cycling and storage. We encourage a pan-Arctic science- and management agenda to incorporate marine vegetation into a coherent understanding of Arctic changes by quantifying distribution and status beyond the scattered studies now available to develop sustainable management strategies for these important ecosystems.
Introduction
Rapid warming of the Arctic with associated melting of ice sheets, glaciers, and reduced extent and thickness of sea ice is causing major changes in high latitude coastal ecosystems (Pörtner et al., 2019). While sea-ice associated communities and endemic Arctic species are experiencing losses, benthic marine vegetation may respond positively to warming and loss of sea ice, which potentially allows more light to reach the seafloor (Krause-Jensen and Duarte, 2014; Filbee-Dexter et al., 2019). The Arctic marine vegetation does include some endemic species that depend on the low temperatures currently experienced in the Arctic (Müller et al., 2009; Wulff et al., 2009; Wilce, 2016; Küpper et al., 2016; Bringloe et al., 2020). However, a large component of macroalgae growing in the Arctic have a boreal origin, shaped through cycles of glaciation to unique assemblages in polar waters (Bringloe et al., 2020). Many of these macroalgae as well as eelgrass (Zostera marina), the sole seagrass species occurring in sub-Arctic areas, are characterized by optimum temperatures for growth which are considerably higher than those currently experienced in the Arctic (Müller et al., 2009; Wulff et al., 2009; Beca-Carretero et al., 2018). Higher temperatures are therefore likely to stimulate the growth of these species (Olesen et al., 2015; Marbà et al., 2017; Wilson and Lotze, 2019; Franke and Bartsch, unpublished data). However, while the combination of reduced sea-ice cover and warming is expected to stimulate growth of Arctic marine vegetation (Krause-Jensen and Duarte, 2014), sediments delivered with glacier runoff may locally increase water column light attenuation and, thereby, counteract the effect of reduced extent of sea ice on light availability (Bartsch et al., 2016; Bonsell and Dunton, 2018; Pavlov et al., 2019).
Benthic vegetation (including microalgae) has been estimated to contribute approximately 20% of the total marine primary production in the Arctic (Attard et al., 2016). Marine vegetation supports key ecosystem functions such as providing habitat that promote biodiversity and climate change mitigation and adaptation (Duarte et al., 2013; Smale et al., 2013; Filbee-Dexter et al., 2019), and changes in the distribution of these vegetated habitats will affect the functioning of Arctic marine ecosystems (Paar et al., 2016, 2019a; Marbà et al., 2018). Given the vast extension of Arctic permafrost coastline (34% of the global coastline), with 20% of the Arctic shelf areas being shallower than 20 m (Lantuit et al., 2012), changes in marine vegetation in this region should also be significant in a global context.
While there are clear global imprints of climate change on marine vegetation, with several reports on warming as a stressor at the equatorial edge of distribution (Raybaud et al., 2013; Filbee-Dexter et al., 2016; Wernberg et al., 2016), documentation is sparse for polar regions. A decade ago, a review of trends identified multiple responses of Arctic marine biota to climate change (Wassmann et al., 2011), but no reports for Arctic marine vegetation were available. Likewise, a review in 2013 of global imprint of climate change on marine life included no studies reporting realized responses of Arctic marine vegetation (Poloczanska et al., 2013), which are also lacking from the recent special IPCC Oceans and the Cryosphere report (Pörtner et al., 2019). In the interim, the focus on Arctic marine vegetation has intensified both in terms of research, monitoring effort, and assessment status of Arctic kelp forests (Filbee-Dexter et al., 2019). Several Arctic research programs address marine vegetation and while the Circumpolar Biodiversity Monitoring Program (CMBP) does not yet include a marine vegetation component, efforts to assess changes in marine vegetation are ongoing in Alaska, Arctic Canada (i.e., ArcticNet-ArcticKelp project, Hudson Bay coastal habitat research project (seagrass), Greenland (i.e., Greenland Ecosystem Monitoring1), Iceland, Svalbard Islands (Bartsch et al., 2016), and Arctic Russia (assessment of macrophytobenthos within the Arctic Centre program of preparation of ecological atlases of Russian Arctic seas, i.e., Maximova, 2016, 2017b). Thus, there is a need to compile available information on trends in marine vegetation across the Arctic.
The status of marine vegetation can be characterized through a variety of metrics that include species composition and diversity, distribution area, depth extent, abundance, productivity, nutrient content (e.g., Marbà et al., 2013), and phenology (e.g., Clausen et al., 2014; Blok et al., 2018). Trends can be assessed as site-specific changes over time in such parameters, and if information is available along a latitudinal gradient, changes in species occurrence can also be used to derive poleward migration rates (Poloczanska et al., 2013).
Here, we review, compile, and synthesize available time series on Arctic marine vegetation in relation to climate change with the aim of providing an overview of trends in distribution, abundance, and performance of intertidal and subtidal macroalgae and eelgrass in the Arctic. The focus is Pan-Arctic, encompassing all major coastal Arctic regions (Alaska, Canada, Greenland, Iceland, Norway/Svalbard, Russia). We supplement by modeling past (1940–1950) and current (2000–2017) potential distribution areas and associated distributional shifts of macroalgae in the region. We hypothesize that Arctic marine vegetation is exhibiting rapid change in response to warming and melting of the cryosphere in terms of polar expansion of distribution limits and changed local distribution, community composition, process rates, and associated ecosystem functions.
Materials and Methods
Data Compilation and Analysis
We searched the literature to generate an overview of available trends on Arctic marine vegetation. We looked for studies reporting trends in macroalgae or seagrasses (eelgrass, Zostera marina) from the Arctic region as defined by the Arctic Council (Huntington, 2001), including Arctic Canada, Alaska, Greenland, Iceland, Norway/Svalbard, and Russia (Figure 1). Our major objective focused on locating time-series observations at a given site/area encompassing at least a 10-year span, which could be represented at a minimum by initial and final observations, although three or more data points over time provide more robust assessments. If a time series contained various sub-periods and trends, a trend was reported for each sub-period that fulfilled the above criteria. We also searched for the records (location, time) of species reported further north than the previous northernmost observation and potentially allowed estimating the migration rate (km yr–1) of the leading, poleward, biogeographical range (Poloczanska et al., 2013).
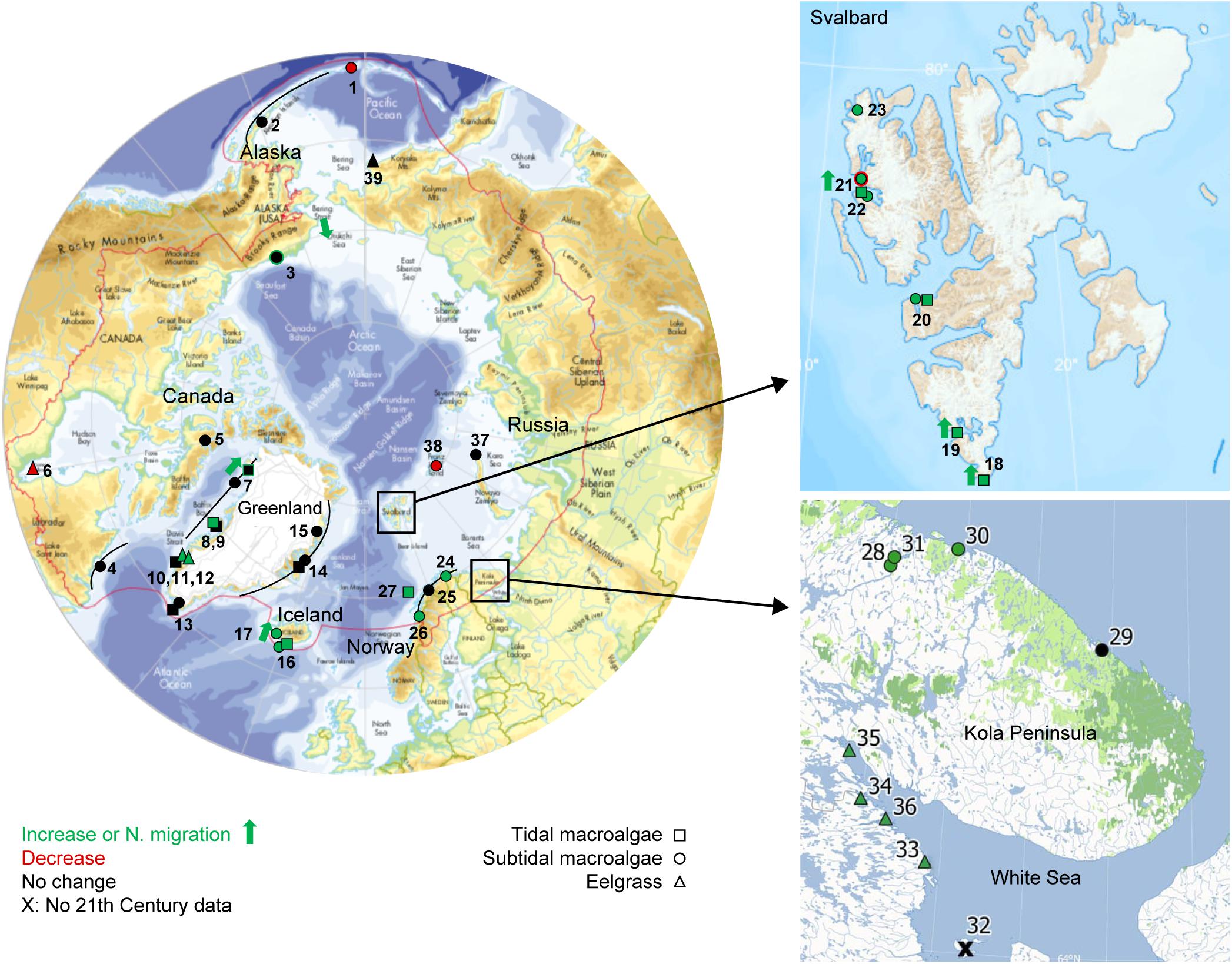
Figure 1. Overview of compiled studies on pan-Arctic marine vegetation trends spanning at least 10 years and entering into the 21th Century. Trends are marked as increasing (green), declining (red) or no change (black) for subtidal macroalgae (circles), intertidal macroalgae (squares), and eelgrass (triangles). Northward migration is indicated by green arrow. If the site represents trends for more than one period, only the most recent is shown, and if this did not include the 21th Century, the site is marked by X. A line through a circle/square indicates that there are several sites along a gradient. The red line defines the Arctic region based on the Arctic Council definition (Huntington, 2001). Numbers refer to sites specified in Table 1. Close-up of study sites in Svalbard and Russian Barents Sea/White Sea. Main map from Huntington, 2001, Svalbard map from Norwegian Polar Institute.
We searched the web of Science (accessed 30 June 2019) using the search string: “[(“marine vegetation” or macroalga∗ or seaweed or kelp or seagrass or Zostera) and (Arctic or subarctic or polar) and (change or trend) and (Russia or Canada or Alaska or Greenland or Svalbard or Norway or Iceland)].” This rendered 84 hits, of which only 13 publications contained relevant information on time series of Arctic marine vegetation, while the remainder represented laboratory studies, referred to other organism groups or contained information on marine vegetation other than data on trends. Additional studies from Arctic regions, whether published or unpublished, were also compiled to yield a total of 39 Arctic sites (some of these including sub sites) with information on time series in one or more vegetation parameters (Figure 1 and Table 1).
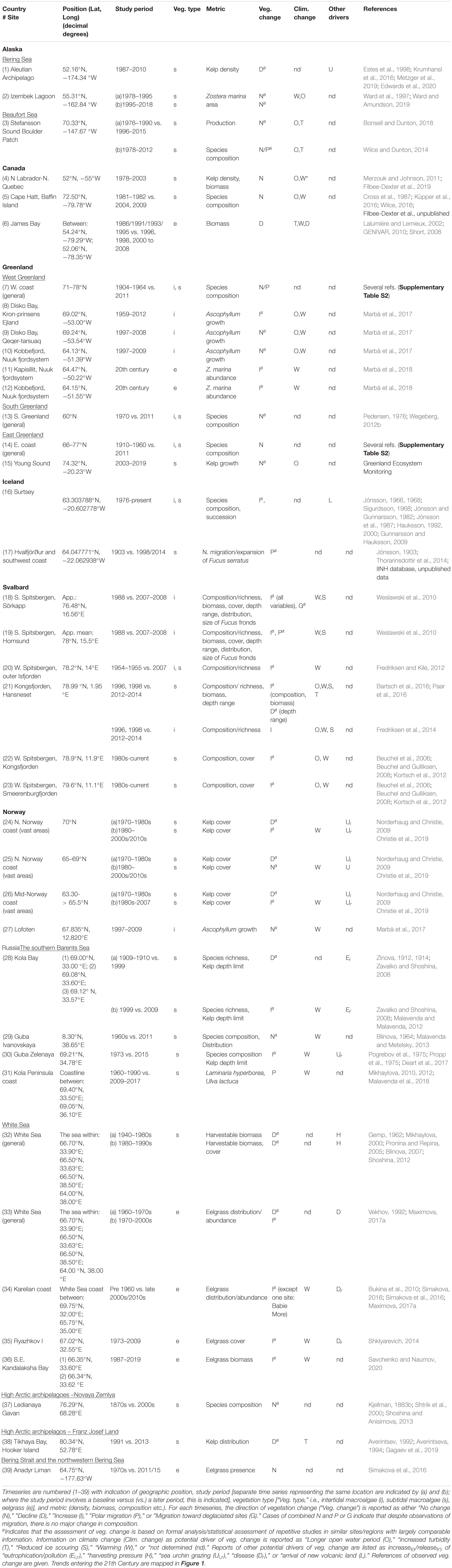
Table 1. Overview of compiled timeseries for pan-Arctic marine vegetation spanning at least 10 years.
For each time series, we noted location, tidal zone (intertidal or subtidal), vegetation type (macroalgae or eelgrass; species if relevant), metric/parameter (e.g., number of species, depth limit, biomass), direction of change [increase (I), decline (D), no change (N), polar migration (P), migration toward deglaciated sites (G)], whether climate drivers were identified [longer open water period (O), increased turbidity (T), reduced ice scouring (S), warming (W), not determined (nd)], and whether other potential drivers were specified [increase(i)/release(r) of eutrophication/pollution (Ei,r), harvesting pressure (H), sea urchin grazing (Ui,r), disease (Dr), and new volcanic habitats (V)]. An overview of trends was compiled for each region. Additional information and synthesis is provided in the Supplementary Information (SI) for Greenland (Supplementary Information I), Iceland (Supplementary Information II), Svalbard (SI-III), and Russia (Supplementary Information IV).
Modeling of Past and Current Potential Pan-Arctic Distribution Area of Macroalgae
Species distribution models (SDM), also known as habitat suitability models, bioclimatic envelope models, or ecological niche models (for review and definition see, e.g., Peterson et al., 2011) were used to estimate recent distributional shifts of Arctic marine macroalgae. These methods identify and describe correlation patterns between species occurrences and environmental data, and provide useful ecological understanding of large-scale biogeographic patterns by predicting regions of habitat suitability where it is likely for species to occur (Dormann et al., 2012). Among the numerous algorithms available (Peterson et al., 2011) we chose Boosted Regression Trees (BRT) and Adaptive Boosting (AdaBoost), two machine learning approaches that systematically retrieve high predictive performances (Elith et al., 2008; Assis et al., 2017) by fitting complex interactions between predictors and non-linear relationships, while avoiding overfitting through monotonic responses and optimal parametrization (Elith et al., 2008; Hofner et al., 2011).
To estimate recent distributional shifts of Arctic marine macroalgae, SDM were developed with the machine learning algorithms Boosted Regression Tress (BRT) and Adaptive Boosting (AdaBoost) fitting environmental predictors against distribution records (see Supplementary Information V). These algorithms were chosen because they fit complex interactions between predictors and non-linear relationships, while avoiding overfitting through monotonic responses and optimal parametrization (Elith et al., 2008; Hofner et al., 2011).
Biologically meaningful environmental predictors for macroalgae were extracted from Bio-ORACLE V2.1 (Assis et al., 2017); surface predictors for intertidal species and benthic predictors (i.e., along bottom) for subtidal species. Predictors were selected to reflect factors affecting the physiology of species (ocean temperature and salinity), disturbance (sea ice cover), and essential resources (nutrients as nitrate). These data were derived from the Global Ocean Biogeochemistry Non-assimilative Hindcast and the Global Ocean Physics Reanalysis of the European Centre for Medium-Range Weather Forecasts. To model subtidal species, predictors were clipped down to 30 m depth (the typical depth distribution of macroalgae), while for intertidal species, predictors were clipped with a continuous gridded mask delimiting global coastlines (e.g., Assis et al., 2017).
Records of brown macroalgae presence were compiled from the fine-tuned dataset of marine forests (Assis et al., 2020) for the Arctic marine realm (Spalding et al., 2007), as well as the temperate Northern Atlantic and Pacific realms, from which species might potentially shift poleward. For modeling purposes, the same amount of pseudo-absences as presences were randomly generated in cells where no presences were recorded (Barbet-Massin et al., 2012). To reduce the potential effect of spatial autocorrelation in the models, the spatial variability of predictors was tested as a function of distance. In this approach, a correlogram was built for intertidal and subtidal species to pinpoint the minimum significant correlated distances of predictors. These were used to prune the records of both datasets, by randomly choosing one record of occurrence from a pool of records found within such distances (e.g., Boavida et al., 2016).
A cross-validation framework using sixfold independent latitudinal bands (e.g., Assis et al., 2017) was implemented to tune the models by testing distinct parameter combinations of tree complexity (1–6), number of trees (50–1000, step 50), and learning rate (0.01, 0.005, and 0.001) for BRT, shrinkage (0.25–1, step 0.25), degrees of freedom (1–12), and number of interactions (50–250, step 50) for AdaBoost. Cross-validation also allowed assessing the performance and transferability of models with the area under the curve (AUC) and sensitivity (true positive rate; Allouche et al., 2006). Models were forced to produce positive monotonic responses while fitting nitrate, salinity, minimum temperature, cloud cover, and negative responses while fitting maximum temperature and ice thickness (Hofner et al., 2011; Assis et al., 2017; Gouvêa et al., 2020).
Distribution maps were developed by ensembling (mean function; Araújo and New, 2007) the outputs of both BRT and AdaBoost models using the optimal parameters. Maps were then reclassified to binomial responses - reflecting presence and absences – using a threshold maximizing the sum of specificity (true negative rate) and sensitivity (Jiménez-Valverde and Lobo, 2007; Assis et al., 2017). Range shifts were estimated, based on the change in potential distribution area of the intertidal and subtidal vegetation by comparing the past (period 1940–1950) and present (period 2000–2017) potential distributions. Because we lack data on benthic substrate composition (i.e., discrimination between rocky and sedimentary coastlines), the distribution maps reflect suitable habitats based on climate- and seawater conditions alone, and may therefore overestimate potential distribution areas.
Results
Changes in Benthic Vegetation in Alaska (United States)
Arctic macroalgal habitats in Alaska have been studied for decades and three time-series exist between the cold temperate Aleutian Islands in the southern Bering Sea and the High Arctic Beaufort Sea (Figure 1). All time-series data relate to shallow subtidal eelgrass and macroalgal stands at depths less than 15 m (Table 1). Intertidal macroalgae are very abundant in the Aleutians, but the Beaufort Sea coast is devoid of intertidal algae because of ice scour. Overall, patterns in the abundance of subtidal marine vegetation are not correlated with regional climatic change with respect to temperature or ice extent, although there is some indication of northward migration of subarctic or boreal species.
A recent review of trends in kelp abundance include a long-term (>10 years) record of kelp abundance in the Aleutian Archipelago (Table 1, Site 1) that was included in Krumhansl et al.’s (2016) global change analysis of kelp abundance. The Aleutian Islands in Alaska have quantitative records of kelp abundance from the 1980s to 2016 and anecdotal records of high kelp abundance starting in the 1970s (Estes et al., 1998; Metzger et al., 2019). In this chain of islands, Adak Island has the most extensive and the longest time series of data (from 1987 to 2010) and shows a loss of kelp in the 1990s (Krumhansl et al., 2016; see Figures 1, 2). This was attributed to declining sea otter populations during this period, which triggered a dramatic increase in sea urchins that destructively grazed kelp forests creating barrens along the archipelago between the islands of Amchitka (179°E) and Adak (176°W) (Estes et al., 2004). Although sea otter abundances throughout the Aleutian Archipelago remain low and many kelp forests have not recovered, small patches of isolated kelp forests still persist over 800 km (from 173°E to 171°W) in shallow waters and on pinnacles around some of the islands (Konar et al., 2014). This suggests that top down control of sea urchins has been more important in driving kelp abundance compared to climate change.
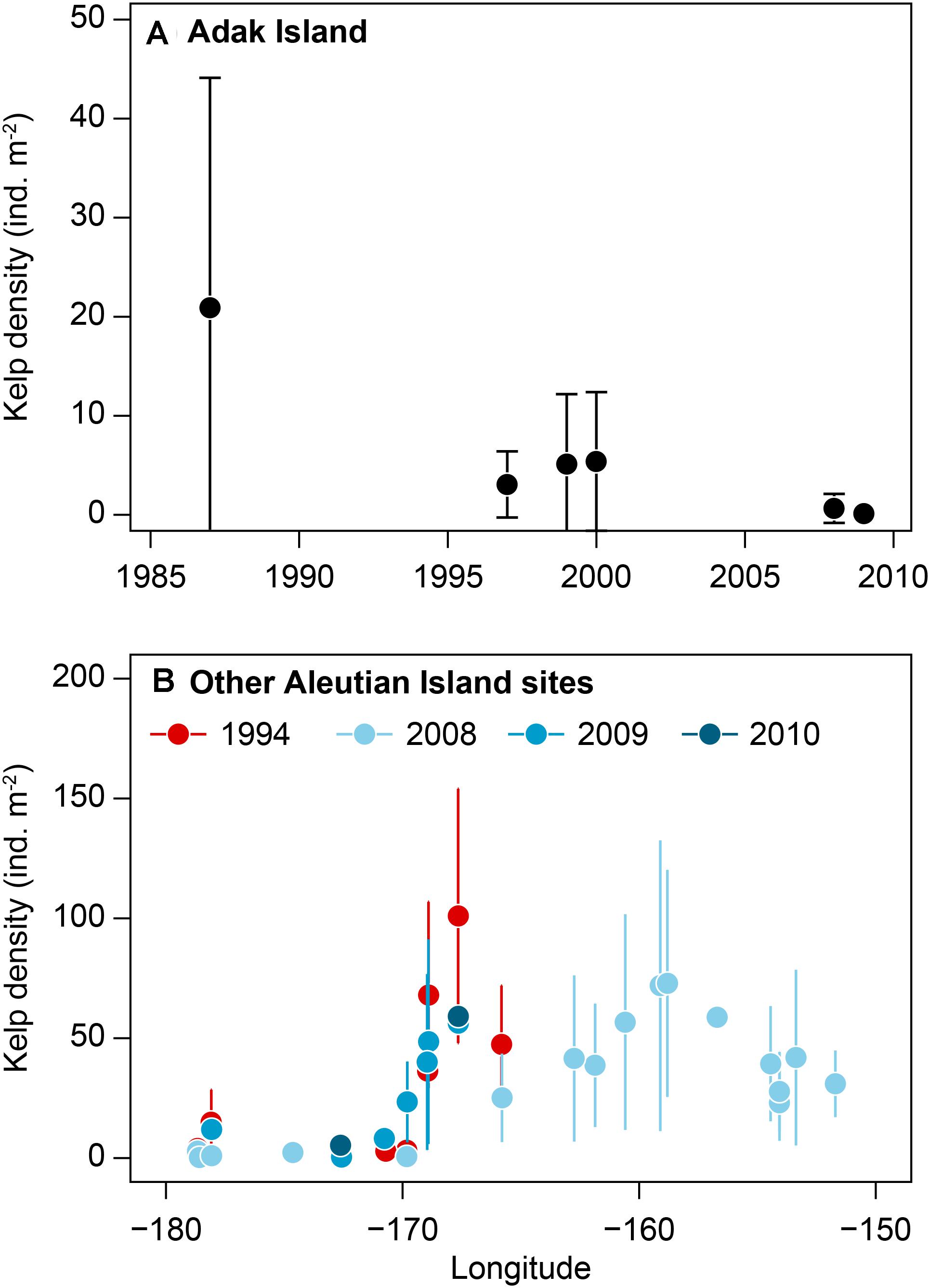
Figure 2. Time series of kelp density (Mean ± SD) in Aleutian Islands, Alaska: (A) Adak Island 1987–2009 (the most data-rich site, –176.6°W), (B) various sites in Aleutian Islands in 1994 and late 2000s. Data sourced from NCEAS dataset (Krumhansl et al., 2016).
Izembek Lagoon (Site 2) in the northeast Aleutian Islands, possess the largest eelgrass meadow along the Pacific Coast of North America, which covers about half the lagoon (15,000–16,000 ha) that has had stable area distribution over a 17-year study period from 1978 to 1995 (Ward et al., 1997). Trends from 2007 to 2018 are also reported as stable (Ward and Amundson, 2019) (Table 1 and Figure 1, Site 2). Overall, the lower Alaska Peninsula has 31,000 ha eelgrass (Hogrefe et al., 2014). Coastal Alaska represents both the northern- and western-most limits of Z. marina in the Northeast Pacific region and is also of phytogeographic interest because the meadows occur along the southern margin of what was once the Bering Land Bridge (Talbot et al., 2016). Increasing water temperature and decreased ice cover since 1943 (Petrich et al., 2014) suggest the potential for future expansion of the meadows.
Over 800 km north of the Bering Strait at the confluence of the northern Chukchi and western Beaufort Sea near Barrow (now Utqiaġvik, Alaska), qualitative beach collecting efforts between 1992 and 1997 documented live Saccharina latissima and Laminaria solidungula attached to mussels and cobbles after storm events (Feder et al., 2003). This occurred in an area where brown algae are not common although a kelp forest is well documented 60 km south of the area, in Peard Bay (Mohr et al., 1957). Feder et al. (2003) were not able to associate this change with warming and/or Arctic range extension, although genetic assessments of the mussels suggest they moved from the Bering Strait into the Chukchi Sea.
In the Beaufort Sea’s ‘Boulder Patch’ (Site 3), long-term records of kelp forest productivity exist for Laminaria solidungula at 10 sites during two periods: from 1976 to 1990 and from 1996 to 2015 (Bonsell and Dunton, 2018) (Figure 1 and Table 1, Site 3a). The ice-free season in this area increased by 17 days since 1979, leading the researchers to hypothesize that less sea ice would translate to more light reaching the seafloor and increased benthic macroalgal productivity. Yet, the time series data set shows there was no significant change in annual productivity over the entire 40-year time period. Bonsell and Dunton (2018) found no evidence that earlier ice break-up or a longer summer ice-free period resulted in an increase in kelp production. This lack of change was attributed to increased turbidity from winds and coastal run-off due to the extended period of open water and increased fetch from sea ice loss. Lower water clarity buffered any positive effects of reduced sea ice, greatly attenuating the amount of light reaching the seabed (Bonsell and Dunton, 2018). This data set is particularly comprehensive and is unique for the entire Arctic.
Wilce and Dunton (2014) described the benthic algal species composition of the Boulder Patch based on collections made between 1978 and 2012 (Figure 1 and Table 1, Site 3b). They state that, despite evident climate warming and sea ice loss, “by [2012], the impact of these changing physical environments on the composition of the Boulder Patch algal community had not yet become apparent.” Certainly, the relative isolation of the Boulder Patch from sources of immigrants from both the North Pacific (via the Chukchi Sea) and the North Atlantic (via the Canadian Archipelago) provides a long-term refugia for the established fauna and flora. Wilce and Dunton (2014) only reported four subarctic or boreal species that invaded the Boulder Patch community, two red algae and two green algae, but that no substantial change in the algal community had taken place.
Kelp abundance data from the Stefansson Boulder Patch (Krumhansl et al., 2016) includes a 5-year time series (2003–2007) of kelp biomass at multiple dive sites. Although this dataset does not meet the 10-year required time span, the documented average biomass level of 66.3 (±117 SD) g dw m–2 also showed no significant change over the 5-year period.
Changes in Benthic Vegetation in Arctic Canada
Macroalgae form dominant habitats along Canada’s extensive Arctic coastline and are found in areas that have experienced sea ice retreat of 2–15 km yr–1 and rapidly rising sea temperature (0.35°C ± 0.20 per decade) over the period 1986–2016 (Filbee-Dexter et al., 2019). Benthic marine algae of the Canadian Arctic have been studied intermittently since the early 19th century, yet early reports consisted mainly of little more than species lists (Lee, 1980) or single observation studies. Time series data allowing analysis of trends in distribution, community composition, and abundance are rare. One exception is a compilation of kelp data for northern Labrador and northern Quebec between 1978 and 2003, which show a distinct division between Arctic kelp communities and more temperate assemblages (Merzouk and Johnson, 2011). While there was no significant change over the period, the analysis was limited by relatively few data points distributed across multiple species, sites, depths, and regions (Merzouk and Johnson, 2011; Table 1 and Figure 1, Site 4). In general, marine Canadian Arctic ecologists are likely confronting the shifting baselines problem (Knowlton and Jackson, 2008), when the lack of baseline data sets prior to the onset of major environmental change renders change-detection difficult.
Further north in the Canadian Archipelago, algae diversity records from diving research at Cape Hatt, Baffin Island span 25 years (Cross et al., 1987; Küpper et al., 2016; Wilce, 2016). From 1981, 1982, 2004, and 2009, a total of 73 benthic algal species have been recorded. Of this total, 13 species were recorded in 1981/1982 that were not observed in 2004 and 2009, and 5 more species, including some of temperate origin, observed in 2009 were not found in 1982 (Table 1 and Figure 1, Site 5). Misidentifications and new techniques could potentially explain the different species lists between these periods, but the reduced diversity comes despite more advanced genomic techniques and assessment capability in 2009. In the same region in 2019, similar zonation patterns and communities of benthic algae were found compared to 1981 and 1982, although lack of historic biomass data for species prevents detection of changes in biomass and relative composition (K. Filbee-Dexter, personal observations and unpublished data). Interestingly there were no sea urchins recorded in 1982 at Cape Hatt and sea urchins were abundant in 2009 (Cross et al., 1987; Küpper et al., 2016), and 2019 (Filbee-Dexter et al., unpublished data), which could suggest increased abundances of these species.
Recent surveys from across 2500 km of the eastern Canadian Arctic show that the current macroalgal biomass and species composition are strongly related to sea ice cover, with larger macroalgal biomass and taller forests of Laminariales occurring in areas with longer open water periods (K. Filbee-Dexter et al., unpublished). This suggests loss of sea ice in the future could lead to increased benthic vegetation.
Eelgrass beds (Zostera marina) are not well documented in the Canadian Arctic, however, eelgrass is known to occur sporadically around the coast of Hudson Bay (Curtis, 1975). In James Bay, dense and extensive eelgrass beds have been documented since the 1970s (Curtis, 1975). During a 5-year survey of eelgrass (1986–1991) along the coast of James Bay in the Grande river area, Lalumière et al. (1994) observed large variations, both in density and biomass of eelgrass, with depth, season, and from year to year. Natural variability in climatic conditions appear to be responsible for these variations. However, from 1975 and 2013 there was a 75% loss of eelgrass along the east James Bay coast (Cree Traditional Knowledge, Consortium Genivar-Waska, 2017; Figure 1, Site 6). This decline was first reported by the Cree Nation from their observations while hunting and fishing. Since 1996, there has been less eelgrass habitat in James Bay, and the eelgrass health has declined (Lalumière and Lemieux, 2002). In 1998, a sudden, large-scale decline of eelgrass occurred along the entire east coast of James Bay and Hudson Bay. The decline was thought to be caused by a microorganism bloom stimulated by abnormally high spring temperatures, changes on the coast due to isostatic rebound, and other changes related to global warming (Lalumière and Lemieux, 2002). Furthermore, an altered growth and survival rate due to a decrease in salinity of James Bay waters was observed as a result of more frequent and larger freshwater discharges from the La Grande River (Short, 2008). Eelgrass in some places are sparse, but in other areas it has disappeared altogether. Some eelgrass remains healthy, but much of the eelgrass habitat in James Bay is impacted to some degree by low salinity waters, overgrowth of seaweeds, epiphytes, and by reduced water clarity (Short, 2019).
Changes in Benthic Vegetation in Greenland
Investigations of the diversity of intertidal and nearshore macroalgal communities in Greenland are sparse and sporadic since observations began in the late 19th Century (Rosenvinge, 1893, 1898). Nevertheless, the studies document that macroalgae occur from the southern tip at 60°N to 82 °N (Supplementary Table S1), and that kelps tend to grow deeper and faster toward the south where the open water period is longest (Krause-Jensen et al., 2012).
Information on macroalgal biodiversity from the original surveys (Supplementary Table S1) are compiled in a series of reports (Wegeberg, 2011, 2012a,b, 2013) and in a book that also reports new records (Pedersen, 2011). On basis of the surveys, we assessed potential changes in distribution of species in W. and E. Greenland from the first half of the 20th century (incl. Lund, 1959a, b; Wilce, 1964) to recent, a time gap of >50 years. We also assessed changes at Cape Farewell, a potential port for introduction of new species at the southern tip of Greenland over a 40-year period based on Pedersen’s (1976) survey in 1970 and a comparable (involving the same principal taxonomist Dr. Poul Møller Pedersen), but less intense survey in 2011 (Supplementary Table S1). As very few focused and systematic floristic surveys have been performed along the coasts of Greenland, only very prominent changes in common and characteristic macroalgal species can be detected. In addition to the floristic surveys, we included available time series for other metrics, such as growth parameters included in the Greenland Ecosystem Monitoring program (GEM, see footnote 1), which covers three regions (Nuuk in SW, Disko Bay in mid-W, Young Sound in NE).
Greenland’s West Coast
For Greenland’s west coast, the floristic investigation in North Star Bay (78°N) in 2011 (designed to assess potential impacts from the Thule Airbase; Wegeberg, 2011) reported a suite of species (23) that had not been reported at such high latitudes in the past (Andersen et al., 2005; Table 1 and Figure 1, Site 7). This may not necessarily reflect change, as the area had not been thoroughly investigated before the 2011-survey (Andersen et al., 2005). This first High Arctic survey documented Fucus vesiculosus among the 23 species which also included mostly filamentous species of Rhodophyta and Phaeophyceae. Since a conspicuous species as Fucus vesiculosus is expected to have been detected in less thorough earlier surveys, but was not, we propose that the finding of F. vesiculosus at North Star Bay in 2011 likely represents an expansion of the northern distribution from 73°N to 76°N since 1970, within 40 years (Pedersen, 1976; Wegeberg, 2011). This represents a northern migration rate of about 83 km per decade. Wilce (1964) did also not observe F. vesiculosus in the macroalgal communities at Qaanaaq (78°N), but reported a northern distribution limit for this species at 71°N. Fucus vesiculosus grows in the upper intertidal zone in Greenland (Høgslund et al., 2014; Thyrring et al., 2020) and, hence, is likely sensitive to changes in ambient environment, including ice scouring (Thyrring et al., 2020).
The intertidal habitat-forming macroalga Ascophyllum nodosum develops a biomass up to >30 kg m–2 near Nuuk (Ørberg et al., 2018), and has a reported northern distribution limit in the Disko Bay at about 69°N (Pedersen, 2011). The growth rate of this species has been quantified at several sites along Greenland’s west coast from Nuuk to the Disko Bay, and has been monitored annually at Nuuk and Disko Bay as part of the GEM program. Ascophyllum nodosum growth shows a positive trend in growth rate at Kronprinsens Ejland, Disko Bay, over the period 1957–2012 (Table 1 and Figure 1, Site 8, Figure 3), whereas time series from a site nearby (Qeqertarsuaq, Figure 1, Site 9), and a site further south along the west coast (Kobbefjord, Nuuk; Figure 1, Site 10) do not exhibit significant trends. Although growth rates oscillate, they tend to be higher in warmer years and warmer regions (Marbà et al., 2017).
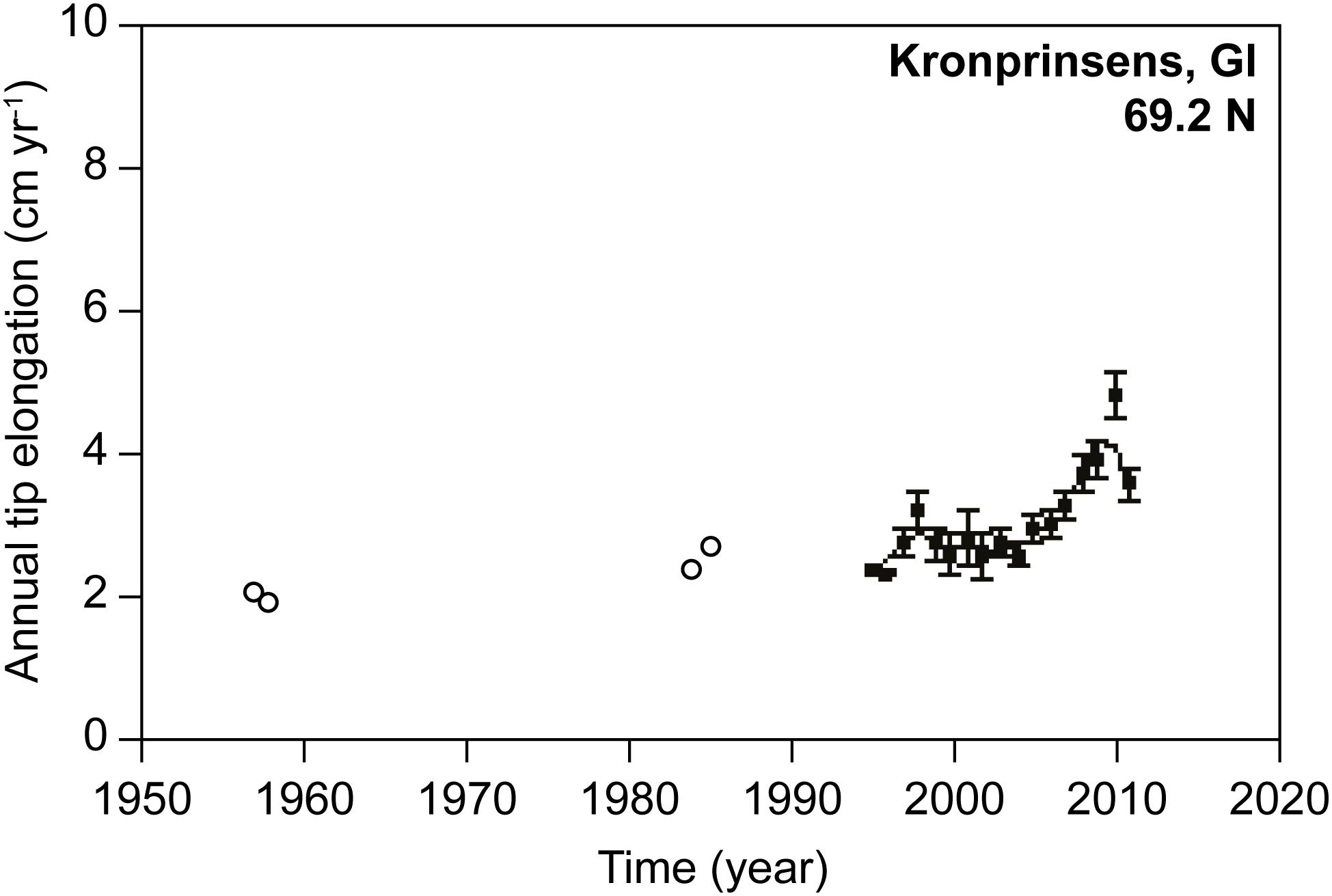
Figure 3. Time series of Ascophyllum nodosum tip growth at Kronprinsens Ejland, Disko Bay, Greenland (69°N). Circles represent growth observations and solid lines 3-year running means. Data from 1957–1958 and 1984–1985 are shown with open circles while more recent data are shown with filled circles. Adapted from Marbà et al. (2017) (http://creativecommons.org/licenses/by/4.0/).
Eelgrass, Zostera marina, also occurs in Greenland and has been studied on the west coast near Nuuk where it occurs in inner protected branches of the Nuup Kangerlua fjord system (64°N) with the first reported occurrence in 1830 (Olesen et al., 2015). Dating of sediments in Z. marina meadows along with analyses of the origin of organic matter in these sediments suggest that eelgrass has been expanding in these locations over the past century (Marbà et al., 2018; Table 1 and Figure 1, Sites 11, 12). Further expansion of eelgrass in the region is expected with increasing temperature as leaf formation rates are fastest in the warmest fjord branches (Olesen et al., 2015) and sexual reproduction also appears to be most successful in the warmer years, since the sites where eelgrass occurs are located near the thermal limit for eelgrass reproduction (Olesen et al., 2015; Blok et al., 2018).
South Greenland
In the Cape Farewell area in South Greenland, Pedersen (1976) registered 104 species in 1970 (entities currently accepted taxonomically in algaebase, Guiry and Guiry, 2020, assessed 7 February 2020), while 70 species (about two thirds) were observed in 2011 (Wegeberg, 2012a) (Supplementary Table S2). However, all of the conspicuous, habitat-forming species, such as fucoid and kelp species, that were recorded in 1970 were re-registered in 2011. Likewise, although ten species from the 2011-survey were not recorded in 1970, these were all relatively inconspicuous types (Supplementary Table S2). The species sampled in both 1970 and 2011 were identified by Dr. Poul Møller Pedersen with high taxonomic precision, so differences in identification are unlikely to have caused the differences in species number. It is more likely that the intensity and scope of sampling explains the differences in species number. For example, the relatively low number of species re-registered within Chlorophyta (Supplementary Table S2) is mainly due to lack of registration in 2011 of endophytes within this group, which were included in the 1970 survey. On this basis, we cannot verify changes in species composition within the 40-year gap between the two collections (Table 1 and Figure 1, Site 13).
East Greenland
Along Greenland’s East coast, no prominent changes in macroalgal species distribution were identified. Species observed by underwater video camera in 2016–2017 (S. Wegeberg and O. Geertz-Hansen, unpublished data), such as the kelp species Agarum clathratum, Alaria esculenta, Laminaria solidungula, and Saccharina spp., as well as the red algal species Coccotylus truncatus and Turnerella pennyi, reach the same northward distribution as described in the past, based on numerous but local and sporadic samples (Rosenvinge, 1898, 1910, 1933; Jónsson, 1904; Lund, 1959b; Table 1 and Figure 1, Site 14). While the generally northward currents on the west coast of Greenland (Buch, 2000) likely support fast northward migration rates, the overall southward currents in NE Greenland may, on the contrary, limit northward migration. In the recent floristic baseline study at Mestersvig, E. Greenland (72°N), Platysiphon verticillatus was registered (Birklund et al., 2006; Wegeberg, 2012b). This species, likely a component of High-Arctic biodiversity, has not been observed in Greenland since it was erected by Wilce (1962) on material from Qaanaaq (78°N) on the west coast. It is possible that (Lund (1959a, b), working on material from the same east coast area, Ella Ø, may have identified this species, found epiphytically on Fucus, as Punctaria plantaginea. Platysiphon verticillatus and P. plantaginea differ only in that the former has a long attenuated tip, therefore making it impossible to discriminate these two if the tip had been lost (both are found epiphytic on Fucus; Lund, 1959a, b; Birklund et al., 2006). Hence, we cannot conclude, on the basis of this evidence that P. verticillatus has expanded its distribution.
In Young Sound, NE Greenland, 74°N, the annual growth of Saccharina latissima has been estimated since 2003 as part of the GEM program. About 20 specimens are collected in early August every year at 10 m depth and the length of the new blade is measured as a proxy for annual growth (Borum et al., 2002; Krause-Jensen et al., 2012). Sea ice conditions in the fjord have been monitored for more than 70 years (Figure 4A), and show a significant increase in the duration of the ice-free season at a rate of 0.38 ± 0.082 (SE) days per year (p < 0.05, R2 = 0.26). This suggests an overall increase in light availability for kelp growth but with considerable interannual oscillations. The time series of annual kelp growth shows marked interannual variation over the period 2003–2019, which may mask the increase expected from increased light availability (Table 1 and Figure 1, Site 15; Figure 4B). However, considering that kelp is able to store and transfer energy between years so that annual blade growth is determined by the light climate (as estimated by ice-free duration) of the year of collection and the previous year, we find a significant relationship between the total ice-free days in the year of collection and the previous year and annual leaf growth (p < 0.05, R2 = 0.38, Figure 4C), confirming the relationship established earlier based on data from 2003 to 2011 (Krause-Jensen et al., 2012).
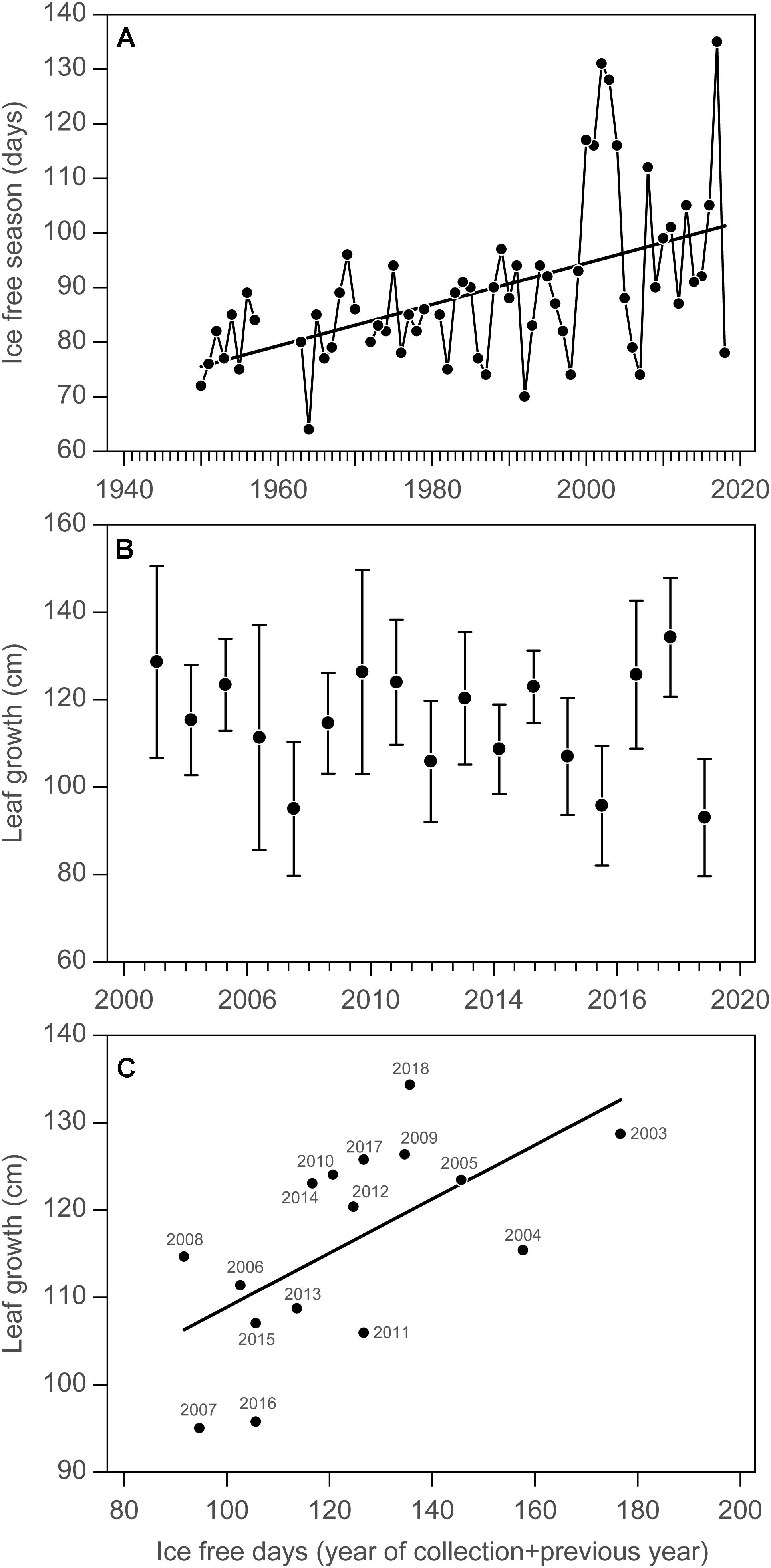
Figure 4. Time series of kelp growth in relation to climate change in Young Sound, NE Greenland 74°N. (A) Changes in the duration of the ice free season in outer Young Sound over the period 1950–2019. (B) Average leaf length growth (±95% CI) of Saccharina latissima at 10 m depth in Young Sound over the period 2003–2019. (C) Relationship between leaf growth and ice-free season of the year of growth and the previous year. Data from the Greenland Ecosystem Monitoring Program “MarinBasis Zackenberg.”
Changes in Benthic Vegetation in Iceland
The intertidal areas of Iceland have an estimated cover of 1.008 km2, including skerries, island and beach ridges (Ottósson et al., 2016). Shores in Iceland range from very sheltered to heavily exposed. Apart from the southern coastline, the majority of shores are in bays and fjords and have little to moderate exposure. Sheltered innermost parts of fjords and lagoons frequently freeze over during the winter months but coastal drift-ice has become rare during the last decades in Iceland (Ogilvie and Jónsson, 2001; Simmonds, 2015), though Arctic sea ice occasionally drifts up to the northern and eastern shores.
Rocky shores with dominant macroalgae cover 28% (280 km2) of the coast (Ottósson et al., 2016). Macrovegetation on littoral sediments include eelgrass beds, which cover roughly 1% (11 km2) of the coast and are dominated by a narrow-leaved form of Zostera marina (Ottósson et al., 2016). Other, less dominant habitat types also exist on some coastal stretches. The algal vegetation on Icelandic shores has been relatively well known from the beginning of the 20th century (Jónsson, 1912), although trends in biomass or species composition changes for macroalgae have not been assessed for Iceland except for Surtsey (Table 1 and Figure 1, Site 16). There, macroalgae succession has been documented since the island, of volcanic origin, rose from the sea in late 1963. Existing data is relatively scattered in both space and time and much of it comes from surveys done in relation with human disturbances and environmental assessments at specific sites not involving trend surveys. These studies as well as other broad scale studies nevertheless establish a good baseline information (Supplementary Table S3) serving as a foundation for future studies on trends and patterns of macroalgae in Iceland.
It is very likely that Fucus serratus was introduced to Icelandic waters by man. The first record of an occurrence of F. serratus dates back to 1903 when the species was described in two locations in Iceland; Vestmanneyjar (63.4065, −20.2735) and Hafnarfjörður (64.0522, −22.0105) (Jónsson, 1903). In 1998 the species was found in Hvalfjörður (64.3775, −21.7331) (Thorarinsdottir et al., 2014), and confirmed in 2014 (IINH database, unpublished data) (Table 1 and Figure 1, Site 17), implying a relatively slow migration rate of just over 4 km per decade. Fucus serratus has extended its range in recent decades and can now be found on most of the western and northern shores of Reykjanes Peninsula as well as being prominent in Hvalfjörður (Ottósson et al., 2016). Whereas the range expansion of F. serratus may reflect its non-native origin, ocean warming may have facilitated its northward expansion.
Changes in Benthic Vegetation in Svalbard Fjords
The Svalbard archipelago is located at the interface of High-Arctic and boreal climate regimes (Svendsen et al., 2002; Hop and Wiencke, 2019) (Figure 1) (Supplementary Information III). Particularly, the west coast of Spitsbergen (the largest island of the Svalbard archipelago) receives considerable amounts of relatively warm Atlantic water via the West Spitsbergen Current while the eastern and southern part are under the influence of the cold Spitsbergen Polar current (Tverberg et al., 2019). West Spitsbergen is strongly impacted by environmental change. Since the year 2000, the average annual air temperature at Ny-Ålesund, Kongsfjorden, West Spitsbergen, has increased by 0.16°C per year, with most pronounced increases (0.32°C per year) during winter, and more moderate increases (0.06°C per year) during summer (Maturilli et al., 2019). All western fjords of Spitsbergen experienced a synoptic temperature increase in 1983–2009 (Tislenko and Ivanov, 2015). Based on these warmer temperatures, neither Kongsfjorden nor Isfjorden are representative High Arctic fjord systems, but rather harbingers of change for the future of Arctic fjord systems (Bischof et al., 2019).
Although the phytobenthic macroalgal community of Svalbard has been thoroughly studied since the 1870s (Kjellman, 1883a), and comprehensive species lists are available (e.g., Vinogradova, 1995a, b; Hansen and Jenneborg, 1996; Fredriksen et al., 2019), a strong spatial bias exists toward specific fjord systems. Up to now, a total of 197 species of macroalgae with 51 Chlorophyta, 76 Phaeophyceae and 70 Rhodophyta have been recorded for Svalbard. However, there is demand for taxonomic revisions and in depth-analysis of cryptic diversity in a series of macroalgal genera that may substantially change our perception of Arctic biodiversity and functionality, not only for Svalbard (Fredriksen et al., 2014, 2019). For Spitsbergen and other Arctic sites, the kelp Hedophyllum nigripes, described as Laminaria nigripes by Agardh (1868), has been confused with L. digitata for quite some time due to similar external morphology but their temperature demands are substantially different (Dankworth et al., 2020; K. Franke and I. Bartsch, unpublished data).
Data facilitating insights into emerging change have only recently become available for several western Spitsbergen fjord systems. Currently, there are only four studies that re-investigated sites with comparable methods that had been sampled 10 or more years before. The studies represent Hornsund and South Kapp area in southern Spitsbergen (Weslawski et al., 2010; Table 1 and Figure 1, Site 18, 19) as well as outer Isfjorden (Fredriksen and Kile, 2012; Table 1 and Figure 1, Site 20) and Kongsfjorden (Fredriksen et al., 2014; Bartsch et al., 2016; Table 1 and Figure 1, Site 21, 22) in West Spitsbergen. In addition, there is one photographic time series initiated in the 1980s that nearly annually documents the succession of hard-bottom communities including macroalgae in several fjords (Beuchel et al., 2006; Beuchel and Gulliksen, 2008; Kortsch et al., 2012; Table 1 and Figure 1, Site 23).
In Hornsund and the adjacent Sorkappland coast (76–77° N) intertidal macrozoobenthos and phytobenthos were quantitatively and destructively sampled in 1988 and the same stations were revisited with the same method 20 years later in 2007/2008 (Weslawski et al., 2010). The number of all intertidal species doubled, but the increase was even more pronounced for macroalgae. While there were only two species present in 1988 (Fucus and Pilayella), 12 more species were present in the later period, mostly comprising annual forms. This change was also reflected in a threefold increase in macroalgal biomass and a considerable increase in macroalgal percentage cover in 11 out of 12 stations. In addition, the length of Fucus fronds increased (though not significantly), and macrophytes advanced into the colder inner fjord parts and there was an upward shift of macroalgal species. Overall, the intertidal biocoenosis changed considerably between both time periods and although in Hornsund and Sörkappland colder waters still prevail, the changes were connected to sea-ice retreat and increased turbidity from melting glaciers (Weslawski et al., 2010; Table 1 and Figure 1, Site 18, 19).
At the same time, in 2007, Fredriksen and Kile re-investigated two different sites in the outer part of Isfjorden (Kap Linné and Ymerbukta) (Fredriksen and Kile, 2012) that had formerly been visited by Svendsen in 1954 and 1955 (Svendsen, 1957). While there were 39 intertidal macroalgal taxa recorded in 2007, there were 25 in the 1950s. Similarly, in the sublittoral, the new investigation recorded 81 taxa, while Svendsen had only found 50 taxa. In total, 24 more species were recorded in 2007 relative to the 1950s, but care has to be taken in interpreting these data as the increase may reflect a difference in precision, identification skills, sampling effort or focus between studies (Fredriksen and Kile, 2012; Table 1 and Figure 1, Site 20). Nevertheless, the study adds to an emerging picture of increased intertidal species richness accompanying the observed warming trend in western Spitsbergen.
Based on non-destructive photographic surveying at permanent monitoring stations at 15 m water depth, Kortsch et al. (2012) analyzed time-series of zoobenthos and macroalgal cover in Kongsfjorden and the more northern Smeerenburgfjorden. There were abrupt community shifts in Kongsfjorden in 1995 when the previously sparse filamentous brown algal cover suddenly increased to 80% and then fluctuated around 40%. A similar but less pronounced increase in macroalgal cover occurred in Smeerenburgfjorden 5 years later, in 2000 (Kortsch et al., 2012 summarized in Fredriksen et al., 2019). Sites that were dominated by calcareous red-algae showed substantial increase in cover by erect red and brown macroalgae (Phycodrys rubens, Desmarestia sp., Saccorhiza dermatodea) (Table 1 and Figure 1, Site 23). Kortsch et al. (2012) attributed the observed change to increasing seawater temperatures, and increased light availability resulting from reduced sea-ice cover.
An in-depth analysis of biomass and species composition changes at high spatial and taxonomic resolution can only be achieved by the labor-intensive analysis of dive surveys including destructive sampling. Such data are extremely scarce in general and on Svalbard only one site in Kongsfjorden (Hansneset, 78° 58.101′N, 11°57.793′E; Figure 1 Site 21) has been re-sampled 2012–2014 (Bartsch et al., 2016; Figure 5) to allow comparison with data from 1996/1998 (Hop et al., 2012). Although only two time-points are available for this specific site, these two studies represent the only detailed comparison of sublittoral depth-related macroalgal species composition and biomass over timescales of more than a decade. Contrasting to the benthic community composition in the early study, the more recent survey revealed a substantial increase in seaweed biomass, which was mostly driven by the kelp Laminaria digitata (Bartsch et al., 2016; Table 1 and Figure 1, Site 21). This increase in biomass was most prominent in shallow waters, where kelp biomass was 8.2-fold higher in the later study. Interestingly, for most of the big brown algae studied (Laminariales, Desmarestiales, Tilopteridales) the lower distribution limit has shifted upward, indicative for a degradation of light climate related to increased terrestrial run-off, resulting in high water turbidity in fjord systems with limited water exchange (Pavlov et al., 2019).
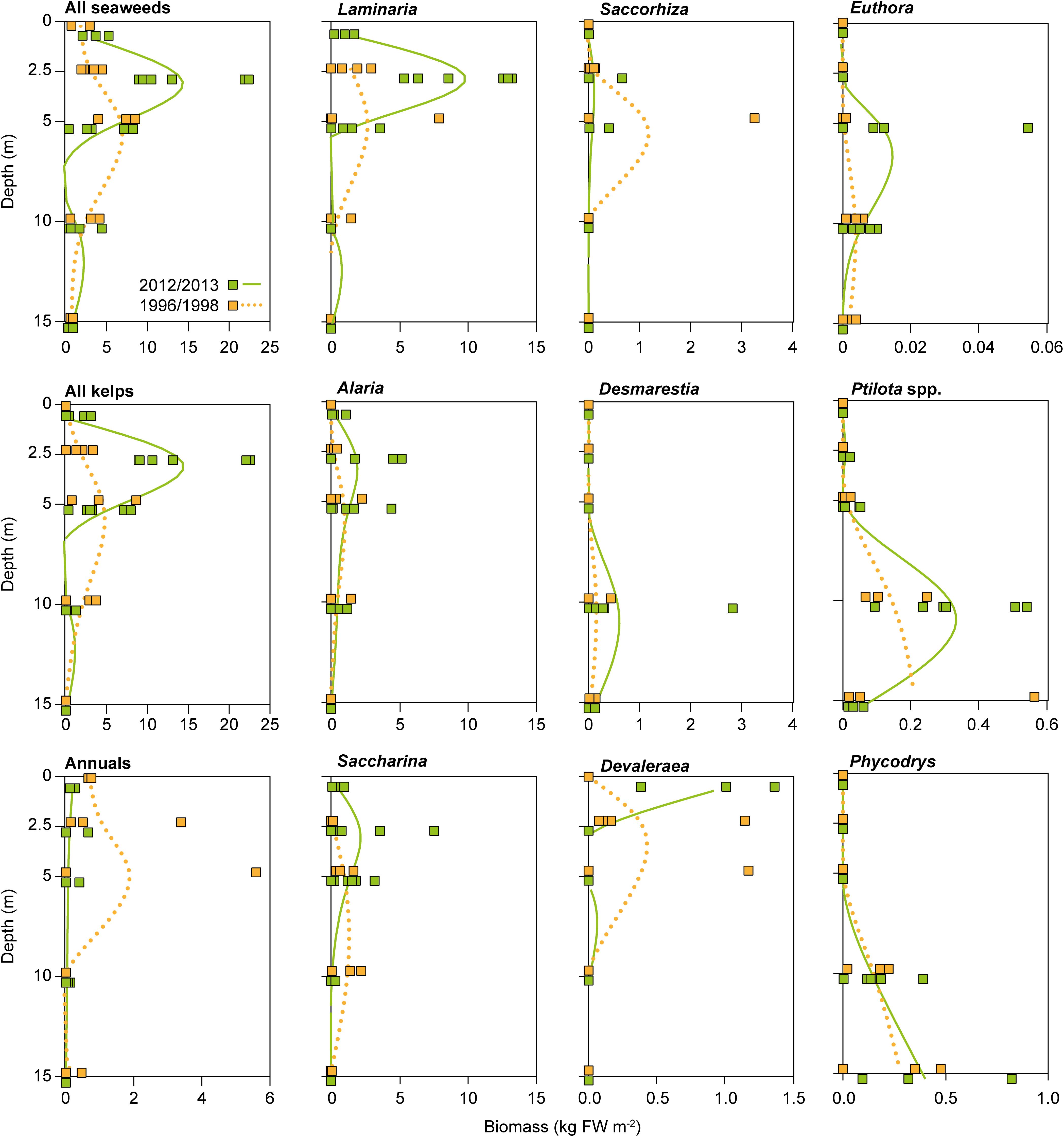
Figure 5. Comparison of fresh weight (FW) m–2 of biomass-dominant seaweed species or groups along the depth gradient at Hansneset, Kongsfjorden (Svalbard), between 1996/1998 and 2012/2013. Green symbols and lines: 2012/2013 data. Orange symbols and dotted lines: 1996/1998 data. Regression curves are cubic-fit splines visualizing the general trend in biomass distribution. 2012/2013: 0 and 15 m (n = 3), 2.5, 5, and 10 m (n = 6); 1996/1998: 0 m (n = 2), 2.5 m (n = 4; original 1.5 and 2.5 m data combined), 5, 10, and 15 m (n = 3). Only genus names are provided. Desmarestia refers to D. aculeata. Euthora is synonymous to Callophyllis in Hop et al. (2012). Adapted from Bartsch et al. (2016) with permission.
Increased import of terrestrial sediments as a consequence of runoff under pronounced snow melting and precipitation is also reflected by a change in the invertebrate community composition associated with the kelp forest at the same site and study period. There was a significant increase in the abundance and diversification of filter feeders, deposition feeders, and an increase in omnivorous species (Paar et al., 2016, 2019a,b). Ecological network analysis of this kelp ecosystem at 2.5 and 5 m water depths from both time periods investigated parallel pathways, and the number of direct and indirect interactions suggest that the kelp belt ecosystem became more mature in 2012–2014 compared to 1996/1998 (Paar et al., 2019b). These authors also suggest that herbivory might become more pronounced in a warming Arctic. As abundance, depth distribution, and biodiversity of kelp forest systems are highly variable in space and time (Hurd et al., 2014), two-point comparisons have to be judged with care. In addition, in fjord systems with their pronounced abiotic gradient, benthic communities and dominance pattern change along the fjord axis, which is also the case for the macrophytobenthos of Kongsfjorden (Hop et al., 2016; Kruss et al., 2017).
Parallel to the increase in biomass reported by Bartsch et al. (2016), an increase in intertidal and shallow subtidal macroalgal species richness was reported for Hansneset (Fredriksen et al., 2014) when comparing data from 2012/2013 with those of Hop et al. (2012) from 1996/1998 (Table 1 and Figure 1, Site 21). In total, 42 macroalgal species were common for both periods. Fourteen of 58 species from 2012/2013 had not been recorded in 1996/1998 and 17 species from 1996/1998 were not recorded in 2012/2013. The most striking differences between both periods were the number of species from the intertidal and shallow subtidal zone that more than doubled in the later period (45 compared to 20 species). Although authors are aware of the difficulties inherent in qualitative species comparisons, they suggest that the increase might be due to the decrease in sea-ice formation and ice-scouring since 2006 at the site (Pavlova et al., 2019) and corroborate the intermediate disturbance hypothesis of Fox (1979).
In addition to the increased species richness in the intertidal and shallow subtidal, the overall functionality at Hansneset changed as evidenced by the significant decrease of the biomass of annual macroalgal species, the shifts in community structure, and food web functionality (Bartsch et al., 2016; Paar et al., 2016, 2019a,b). As the phytoplankton spring bloom is utilizing all available nutrients leading to near zero nitrate values (Piquet et al., 2014; Hegseth et al., 2019), later onset of spring blooms may result in elongated availability of macronutrients for macroalgal spring growth, potentially enhancing benthic primary productivity, although this has not been tested.
Changes in Benthic Vegetation Along Norway’s Main Coast and Associated Archipelagos
In the 1970–1980s, 1300 km of coastline in mid and northern Norway were overgrazed by sea urchins, which removed most vegetation (∼2000 km2 of kelp forest loss, Norderhaug and Christie, 2009). Recent studies covering more than 1,500−km coastline in northern Norway (65–70°N) document that large areas of sea urchin barrens have shifted back to kelp forests, in parallel with increases in sea temperature and predator abundances (Norderhaug and Christie, 2009; Christie et al., 2019; Table 1 and Figure 1, Site 24). In the northernmost and coldest region (around 70°N), kelp forests are recovering from open bedrock due to increased predation on sea urchins from the invasive “red king crab” (Paralithodes camtschaticus), which is shifting its range from Russia into Norway (Christie et al., 2019). These crabs move up from deep regions and remove sea urchins from exposed barrens. In areas beyond the range of king crabs (65–69°N) kelp forest recovery is generally slow (Table 1 and Figure 1, Site 25).
Further south, in mid Norway, the border between kelp forest-dominated areas and sea urchin barrens has moved 300 km north in the last four decades, from 63°20 N in 1980 to 65°30 N in 2007 (Norderhaug and Christie, 2009; Table 1 and Figure 1, Site 26). Warming temperature in this area is considered a key factor driving kelp recovery by limiting sea urchin settlement, recruitment (Fagerli et al., 2013), and driving increases in another predator, the “edible crab” (Cancer pagurus) (Norderhaug and Christie, 2009; Christie et al., 2019). Kelp forests (Laminaria hyperborea) in this entire region show clear differences in age, size, and growth along this environmental gradient, with tall forests of faster growing individuals in warmer mid-Norway transitioning to less tall forests of older, slower growing individuals toward the north (Rinde and Sjøtun, 2005; Pessarrodona et al., 2018). This suggests kelp forests in northern Norway, just above the Arctic circle, could become more extensive and larger with climate change.
Time series on Ascophyllum nodosum growth from Lofoten, Norway (69°N) show increasing trends over the period 1997–2010, while a shorter time series from Tromsø (that does not meet the 10-year timespan requirement) does not show any change (Marbà et al., 2017; Table 1 and Figure 1, Site 27).
Changes in Benthic Vegetation in Arctic Russia
The Russian Arctic coast can be subdivided into several regions significantly differing in environmental conditions and macrophyte floras and communities (for complete review, see Supplementary Information IV). These include (1) the southern Barents Sea; (2) the White Sea; (3) the Siberian continental coast (Kara and Laptev Seas eastward to West Chukotka); (4) high-latitude Arctic archipelagoes (Franz Josef Land, Novaya Zemlya, archipelagoes of small islands of the south-eastern Kara Sea, Severnaya Zemlya and New Siberian Islands); (5) the continental coast of Chukotka in the East Siberian and the Chukchi Seas (from Chaun Bay to Dezhnev Cape, including the Wrangel Island); (6) the Chukotka coast of the Bering Strait and the northwestern Bering Sea. Repeated observations on kelp and/or eelgrass communities are available for some of these areas as listed below.
The Southern Barents Sea
The southern Barents Sea can be further subdivided into the western and the eastern parts. The western part includes the shore eastward of the Russian – Norwegian border in the Varanger-fjord, Kola Peninsula. The eastern part includes the Chioshskaya Bay, and the continental coast and islands of the Pechora Sea. Only for the western part are multi-year observations on marine vegetation available, as summarized below. Climatic changes along the ice-free inshore waters of the generally rocky Murmansk coast mainly include increased water temperature in the last decades (Supplementary Information IV). The macroalgal flora and communities are generally similar to the northeast Atlantic ones, with some decrease of species richness. Kelp communities are dominated by Laminaria digitata, Saccharina latissima, and Alaria esculenta (Supplementary Information IV).
The Kola Bay (Table 1 and Figure 1, Site 28), the longest fjord-like bay of the Kola Peninsula, is housing the city of Murmansk (about 290,000 inhabitants in 2020) with a big harbor and several other harbors and terminals. It is considered as one of the most anthropogenically impacted areas north of the Polar Circle (Matishov, 2009). For this area, generally comparable data on the composition and spatial structure of macroalgal communities are available for 1909–1910 (Zinova, 1912, 1914), 1999 (Zavalko and Shoshina, 2008), and 2009 (Malavenda and Malavenda, 2012). In 2009, species richness of all groups of macroalgae in the southern and the middle part of the Kola Bay was lower than in 1909 but higher than in 1999. This decrease in comparison to 1909 affected all major biogeographic groups of species. The percentage of species with broad (including temperate and tropical areas) distribution was always low and did not show any trend of changes. Significant differences were reported for the spatial structure of macroalgal communities, i.e., the lower boundary of the belt of abundant vegetation had shifted to shallow waters. In particular, kelp communities extended to 10–12 m in 1909 while in 2009 the deepest kelps occurred at 4–5 m depth. These changes have been attributed to anthropogenic impact rather than to climate change (pollution, eutrophication and increasing turbidity of water), with some improvement in 2009 compared to 1999 (Malavenda and Malavenda, 2012).
Outside the Kola Bay there are few case studies of kelp communities in different periods. A particular inlet with observations in the early 1960s and the early 2010s is Guba Ivanovskaya (also known as Ivanovka) (Table 1 and Figure 1, Site 29), which is a fjordic lagoon on the north-eastern coast of Kola Peninsula (Blinova, 1964; Malavenda and Metelsky, 2013). It consists of three basins separated by shallow sills and narrow straits, and supports diverse macroalgal communities with a significant share of boreal species and four major algal associations in the mouth of the inlet and one in the inner lagoon. The survey in 2011 indicated no significant changes in species composition and distribution of algal associations (Malavenda and Metelsky, 2013).
The other repeatedly studied site is the small semi-enclosed fjord Guba Zelenaya (middle part of the Kola Peninsula Coast; Table 1 and Figure 1, Site 30). There, environmental conditions and benthic communities have been investigated in 1973 (Propp et al., 1975; Pogrebov et al., 1975) and again in 2015 (Deart et al., 2017). The seasonal water column stratification pattern remained unchanged but in 2015 summer temperatures were 0.2–0.5°C higher in the middle layers and 1–2°C higher in the upper layer (Deart et al., 2017). In 1973, the characteristic “urchin barren” with the kelp community developed only to about 3 m depth, while deeper communities were partly degraded and replaced by calcareous algae and an abundant sea urchin (Strongylocentrotus droebachiensis) population. In contrast, the kelp community in 2015 was more typical to other inlets of the coast. The zone of sea urchin abundance became restricted to a narrow range of the upper depths in the Alaria belt. The observed situation was interpreted as kelp community restoration from the barren state, facilitated by predation pressure of the introduced red king crab (Paralithodes camtschaticus) on sea urchins (Deart et al., 2017), similar to that observed in Northern Norway (Christie et al., 2019; see above).
Eelgrass (Zostera marina) meadows are very fragmentarily distributed along the coast of Varangerfjord and the Barents coast of Kola Peninsula. Limited observations indicate the persistence of one eelgrass patch in the inner lagoon of Guba Ivanovskaya from the 1960s to 2000s (Blinova, 1964; Simakova et al., 2016; see Supplementary Information IV).
The coasts of the Varangerfjord and the Kola Peninsula are likely directly affected by warming and some boreal species extended their range, such as the boreal kelp Laminaria hyperborea and the green alga Ulva lactuca. The first species, which was not recorded at the Kola Peninsula coast to the east of Varanger-fjord in the 1960s, became a common and association-forming species on the Kola coast in the 1990-2010s (Schoschina, 1997; Mikhaylova, 2010, 2012). The second species, common in Norway, sporadically occurred on the Kola Peninsula coast during the warm period in the 1930s, was not recorded in 1985 – early 1990s, but regularly observed between 2009 and 2017 (Malavenda et al., 2018; Table 1 and Figure 1, Site 31). However, the impact of climatic change on macroalgal communities is difficult to reveal due to limited long-term observations and several other important factors that can overshadow this influence, including anthropogenic changes in the harbor areas and the impact of sea urchins and their predators (such as the red king crab).
White Sea
The macroalgal vegetation of the semi-landlocked and seasonally ice covered White Sea are in many respects similar to the southern Barents Sea. Due to the isolation from the direct input of the Atlantic water, the regime of the White Sea is largely determined by regional scale processes. The effects of changing climate in the last decades is mostly attributed to variation in timing of winter sea ice cover and somewhat earlier spring warming of the inshore waters (Supplementary Information IV).
Most studies of kelp communities in the White Sea in the past were associated with their commercial exploitation since the early 20th century (Pronina, 2011; Table 1 and Figure 1, Site 32). Estimates of harvestable biomass are available for the entire White Sea or its subdivisions and show some decline from the 1940s to 1990s (Gemp, 1962; Blinova, 2007; Shoshina, 2012). In the last decades of the 20th century, the percentage of kelp communities with highest projective cover also decreased (Pronina and Repina, 2005; Pronina, 2011). This decline can be partly attributed to unsustainable methods of harvesting, that employed mechanical dredges until the 1980s (Pronina, 2011; Shoshina, 2012). However, the methodology of harvestable biomass assessment changed through time. By the 2000s, seaweed harvesting also decreased due to economic reasons while several regulation measures were introduced to achieve sustainability (Pronina, 2011).
Besides rough biomass estimates for applied purposes, there are few quantitative data on the inter-annual changes in the kelp communities of the White Sea. Mikhaylova (2000) presented a short-term (1994–1998, unfortunately interrupted since that time) monitoring series for the kelp community in Solovki Archipelago (Laminaria digitata and Saccharina latissima with red algae subdominants) that included detailed recording of a number of community characteristics. Most of them showed little variation that indicated this community to be in a close-to-climax state by the turn of the century.
Eelgrass (Zostera marina) is fairly common in the White Sea and performs a number of ecosystem functions. It underwent a drastic decline over the entire region (Table 1 and Figure 1, Site 33) in 1961 (Vekhov, 1992; Bukina et al., 2010; Maximova, 2017a) with some clear ecosystem consequences, such as drastic decline of fish populations [e.g., herring (Clupea harengus), three-spined stickleback (Gasterosteus aculeatus)] that use eelgrass meadows as a spawning habitat (Berger, 2001; Yershov and Sukhotin, 2015). It is likely that these eelgrass meadows suffered from the so-called “wasting disease” caused by the protist Labyrinthula macrocystis; this organism is present nowadays in healthy eelgrass populations (Maximova, 2017a). Although a site-by-site comparison with the pre-decline level is largely missing, several inlets and bays of the Karelian Coast known for an extensive eelgrass coverage have been observed to house significant meadows in the early 2010s (Bukina et al., 2010; Simakova et al., 2016; Maximova, 2017a; Table 1 and Figure 1, Site 34). However, in the fjordic lagoon Babie More, known for particularly extensive eelgrass meadows until 1961 (Vekhov, 1992), the meadows have practically not recovered, and in the early 2010s declined further compared with the 1998–1999 surveys (Simakova, 2016). As a semi-isolated waterbody, Babie More may be particularly susceptible to incrasing air temperatures although no relevant series of surface water temperature and salinity exists.
The time series of intertidal coverage of eelgrass at Ryazhkov I. (northern Kandalkasha Bay, Kandalaksha State Nature reserve) extends from 1973 up to present although published data are limited by the year 2009 (Shklyarevich, 2014; Table 1 and Figure 1, Site 35). A general positive trend of increasing eelgrass coverage reached its maximum by the early 2000s and the coverage has appeared to fluctuate thereafter.
More regular seasonal monitoring series of intertidal communities with a significant contribution of eelgrass exists for two small inlets near the Cape Kartesh Marine Biological Station (south-eastern Kandalaksha Bay) since 1987 (Naumov, 2013; Savchenko and Naumov, 2020; Table 1 and Figure 1, Site 36). The eelgrass shows a trend toward increasing biomass in the lower intertidal zone from the late 1980s to the 2000s. This trend is superimposed by 4–5 years cycles, occasionally disturbed by irregular episodes of intensive ice gouging during the break of fast ice in spring. The pattern of cycles does not follow the multi-year dynamics of temperature and salinity and most probably reflects auto-oscillations although the general trend partly corresponds to the increase of average temperature (Savchenko and Naumov, 2020). However, the spring – early summer appears to be the most critical season for eelgrass populations in the White Sea and the risk of stimulation of “wasting disease” by some combination of climatic factors in that season remains (Maximova, 2017a).
High Arctic Archipelagoes
Novaya Zemlya
Novaya Zemlya Archipelago separates the Barents Sea and the Kara Sea. The environmental conditions and the macroalgal flora differ on both the Barents and the Kara sides and in the north and the south of the archipelago. The major climate change related impact on the marine vegetation may be related to the significant retreat of glaciers and changing timing of seasonal ice cover (Supplementary Information IV). Unfortunately, only historical descriptions of macroalgal associations of the west coast of the archipelago (Flerov, 1932; Sorokin and Peltikhina, 1991) and fragmentary recent observations from both coasts are available (see Supplementary Information IV).
The only site for which some material for studying the macroalgal species composition dynamic exists is Ledianaya Gavan Bay (the place of Willem Barents’s expedition wintering in 1596/1597) (Table 1 and Figure 1, Site 37). It was investigated three times: in the 1870s (Kjellman, 1883b), 1990s (Shtrik et al., 2000), and the 2000s (Shoshina and Anisimova, 2013). The united list includes 41 species. The dominant species of bottom vegetation did not change during the 125 years: kelp (Laminaria digitata, Saccharina latissima), Fucus distichus, and 9-11 species of Rhodophyta (Maximova, 2016; Supplementary Information IV).
Franz Josef Land
Franz Josef Land (FJL) with its 192 large and small islands (over 80% of land covered by glaciers) is the northernmost archipelago in the Eurasian sector of the Arctic. Manifestations of changing climate are expressed particularly as the shrinkage of sea ice cover in the coastal waters, the increase of glacial discharge, and decrease of the area of marine terminating glaciers (Supplementary Information IV).
Franz Josef Land hosts the most high-latitude known kelp communities, first recorded by Nansen (1897) in the northern part of the archipelago in the 1890s, which was a significantly colder period than today (Supplementary Information IV). Kelp communities occur across the archipelago; although they had not been recorded in the areas where permanent fast ice was present in 1970 (Golikov and Averintsev, 1977). No repetitive observations of these sites have been done in recent years when these inshore areas may become ice-free in summer. The only site with published repetitive observations is Tikhaya Bay at Hooker Island (Table 1 and Figure 1, Site 38) where macroalgal communities were first studied in 1991, showing extensive kelp communities (Averintsev, 1992; Averintseva, 1994). In 2013, no kelp community was found, possibly as a result of increased siltation due to intensification of glacier melting (Gagaev et al., 2019).
Bering Strait and the Northwestern Bering Sea
The northwestern Bering Sea as part of the Russian Arctic is considered here in the restricted sense to include the Bering Strait and the Anadyr Gulf. This region is characterized by a variety of shore types, coastal processes, and productive waters of the Anadyr Current. Seasonal ice cover has declined with a particularly strong negative trend in the early winter and spring (see Supplementary Information IV). In the Anadyr Liman (the external part of the Anadyr River estuary), the northernmost site on the Asian Arctic coast, intertidal eelgrass (Zostera marina) meadows were first discovered in 1971 and repeatedly recorded in 2011–2015 (Simakova et al., 2016; Table 1 and Figure 1, Site 39).
In summary, a variety of conditions along the Eurasian Arctic coastline suggest variable response of kelp and eelgrass communities to climate change (Table 1). These communities themselves are well understudied and there is an urgent need to revisit the historically surveyed sites in the Russian Arctic (Supplementary Information IV) to document the current situation, comparing it to the baseline information on habitats, species composition, vertical structure, and biomass of macroalgal assemblages.
Modeled Potential Past and Present Pan-Arctic Macroalgal Distribution Area
The marine forests dataset (Assis et al., 2020) retrieved 275,154 occurrence records for 31 intertidal species and 552,542 records for 233 subtidal species throughout the Arctic and temperate Northern Atlantic and Pacific realms. The spatial correlograms showed predictors positively autocorrelated at distances between 11 and 14 km, depending on the datasets (Supplementary Figure S4). These distances pruned records to a final database of 2085 records of intertidal species and 3731 records of subtidal species. The models using the optimal parameters identified in cross-validation showed good potential for temporal transferability (CV Sensitivities > 0.85; CV AUC > 0.8; Supplementary Table S4), and their combination in a unique ensemble largely matched the known distribution of Arctic intertidal and subtidal macroalgae (Assis et al., 2020) (Sensitivities > 0.85, AUC > 0.85; Supplementary Table S4 and Supplementary Figure S5).
The models included multiple environmental predictors. For both intertidal and subtidal species, maximum ocean temperature and sea ice cover had a prominent role in explaining distributions (relative contributions > 5%). The distribution of subtidal species was further largely explained by nutrients and salinity (salinity only identified as important by BRT algorithm). Substrate conditions were, however, not considered.
Within the geographic boundaries defined by the Arctic Council, models developed for present conditions (2000–2017) predicted 140,433 km2 and 514,679 km2 of suitable habitats for intertidal and subtidal species, respectively, i.e., a total potential distribution area of 655,111 km2 (Figure 6 and Tables 2, 3). This potential area in north appears to be underestimated. For example, we report time series of Saccharina latissima growth in Young Sound, NE Greenland at 74° N, which is approximately ∼4 degrees of latitude north of the modeled range limit. Extensive surveys in East Greenland also documented widespread occurrence of marine vegetation in fjord systems between 72 and 74°N in the 1931–1932 (Thorson, 1933), which is approximately 10° of latitude beyond the estimated 1940–1950 range in East Greenland. The model also does not predict the kelps in the Russian high Arctic Franz Josef Land, New Siberian Islands, and Wrangel I, documented in the 1970s before on onset rapid warming and ice loss. This underestimation likely reflects the limited macroalgal presence data from the High-Arctic for model input as well as failure to capture polynya areas. The models inferred a gain in suitable habitats between 1950 and present times of 10,468 km2 (8.1%) and 158,747 km2 (44.6%) for intertidal and subtidal species, respectively (Figure 6 and Tables 2, 3). Across Arctic sectors, Canada represents the largest potential macroalgal distribution area followed by Russia, Alaska, and Greenland, however, Svalbard shows the largest relative gain in potential distribution area and N. Norway and Iceland the smallest (Figure 6 and Tables 2, 3). Modeled polar migration rates average 23.1 km decade–1 for intertidal algae and slightly less, 18.3 km for subtidal algae; with the largest sector-specific rates modeled for intertidal algae in Russia and subtidal algae in Canada (Figure 6 and Tables 2, 3).
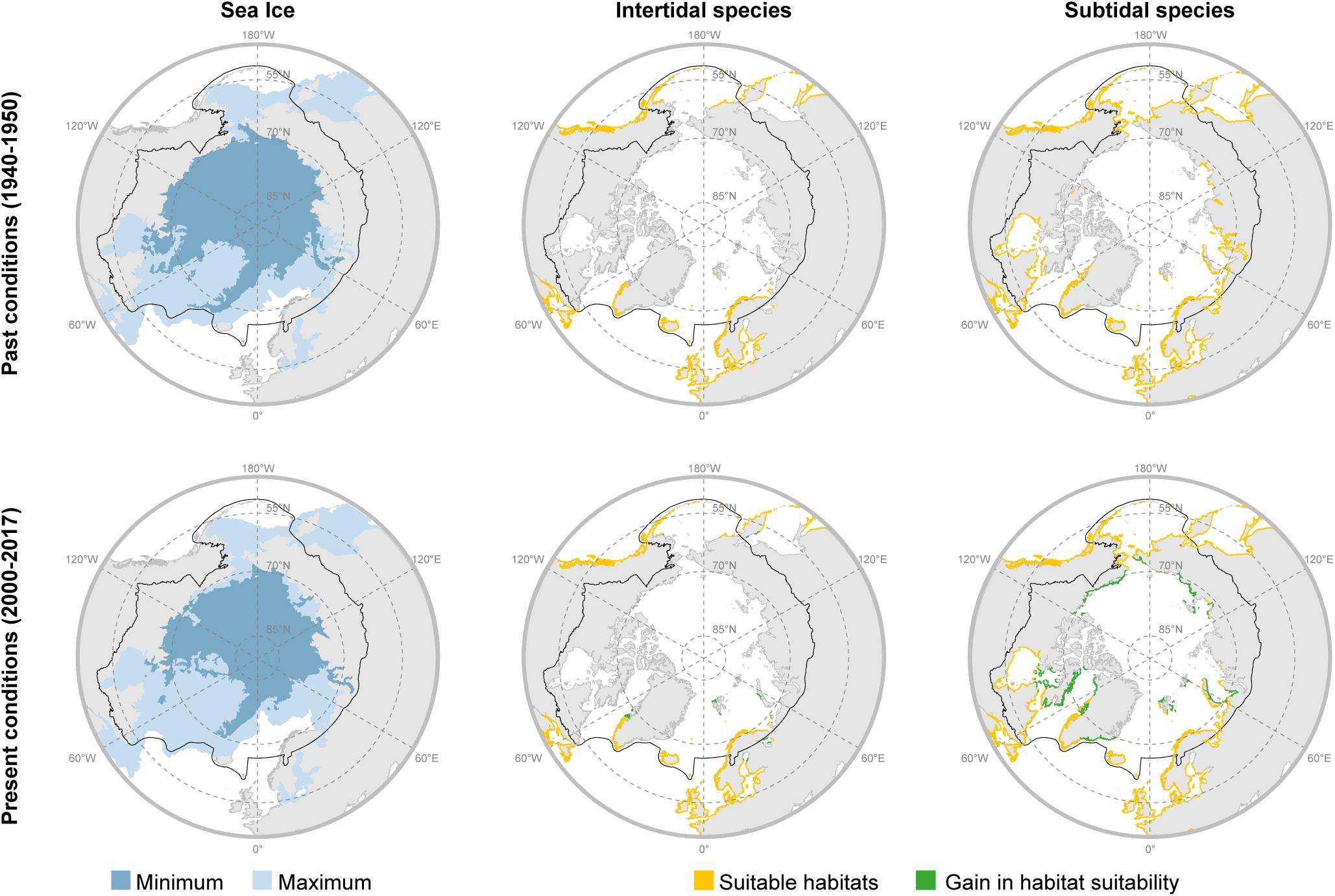
Figure 6. Sea ice conditions and suitable habitat areas for intertidal and subtidal macroalgae predicted for the past (period 1940–1950) and the present (period 2000–2017) in terms of temperature, ice conditions nutrients and salinity, not substrate conditions. Black line depicts the geographic boundaries defined by the Arctic Council.
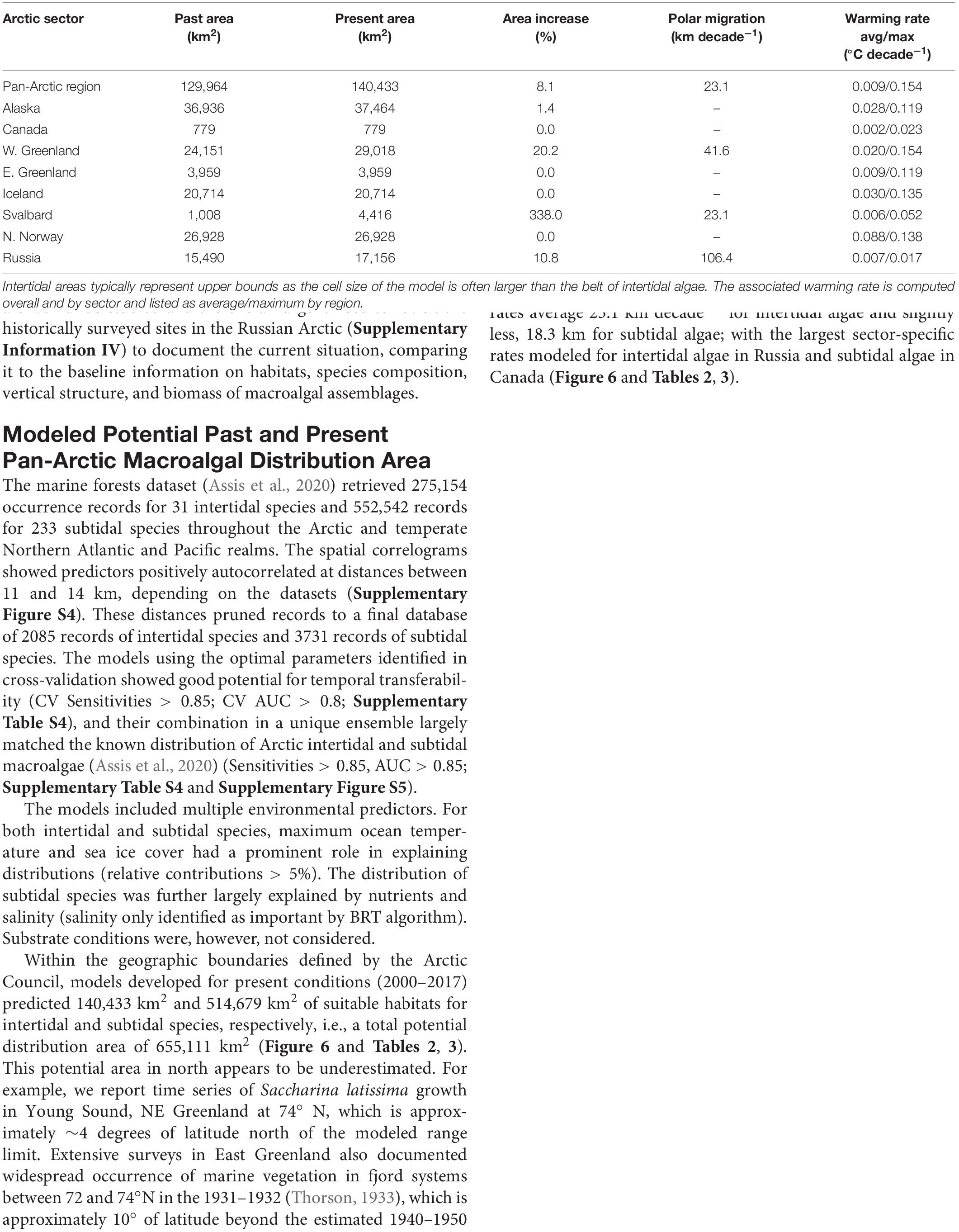
Table 2. Past (period 1940–1950) and present (period 2000–2017) potential pan-Arctic intertidal brown macroalgal distribution areas (km2), and associated area increase and polar migration rate of key habitat-forming macrovegetation, assessed based on niche modeling for the pan-Arctic region and by Arctic sector (based on the Arctic Council definition of the Arctic, Huntington, 2001).
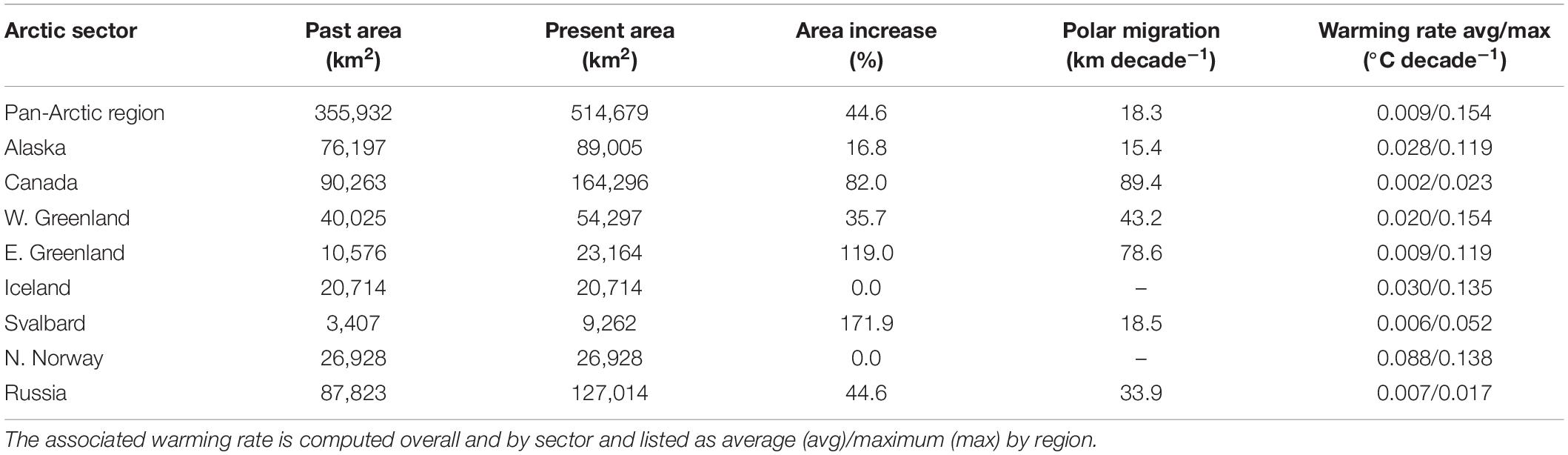
Table 3. Past (period 1940–1950) and present (period 2000–2017) potential pan-Arctic subtidal brown macroalgal distribution areas (km2), and associated area increase and polar migration rate of key habitat-forming macrovegetation, assessed based on niche modeling for the pan-Arctic region and by Arctic sector (based on the Arctic Council definition of the Arctic, Huntington, 2001).
While the model does not address substrate conditions, information on the spatial occurrence of hard substrate allowed a coarse adjustment of the modeled macroalgal distribution to reflect areas that support epilithic communities. Lantuit et al. (2012) reported that 35% of Arctic coastlines facing the Arctic Ocean are lithified, but did not address the wider Arctic coastline. Young and Carilli (2019) provided a more complete global estimate of coastal cliffs (52% of coastlines), including estimates by country, which we therefore used, although cliffs are not equivalent to rocky shore. For most of our study area, national cliff estimates were available, and where lacking (Alaska, Iceland) we used the global average. On this basis, the substrate-adjusted modeled potential pan-Arctic distribution area of macroalgae represents about half of the overall modeled area (340,658–351,915 km2, Table 4).
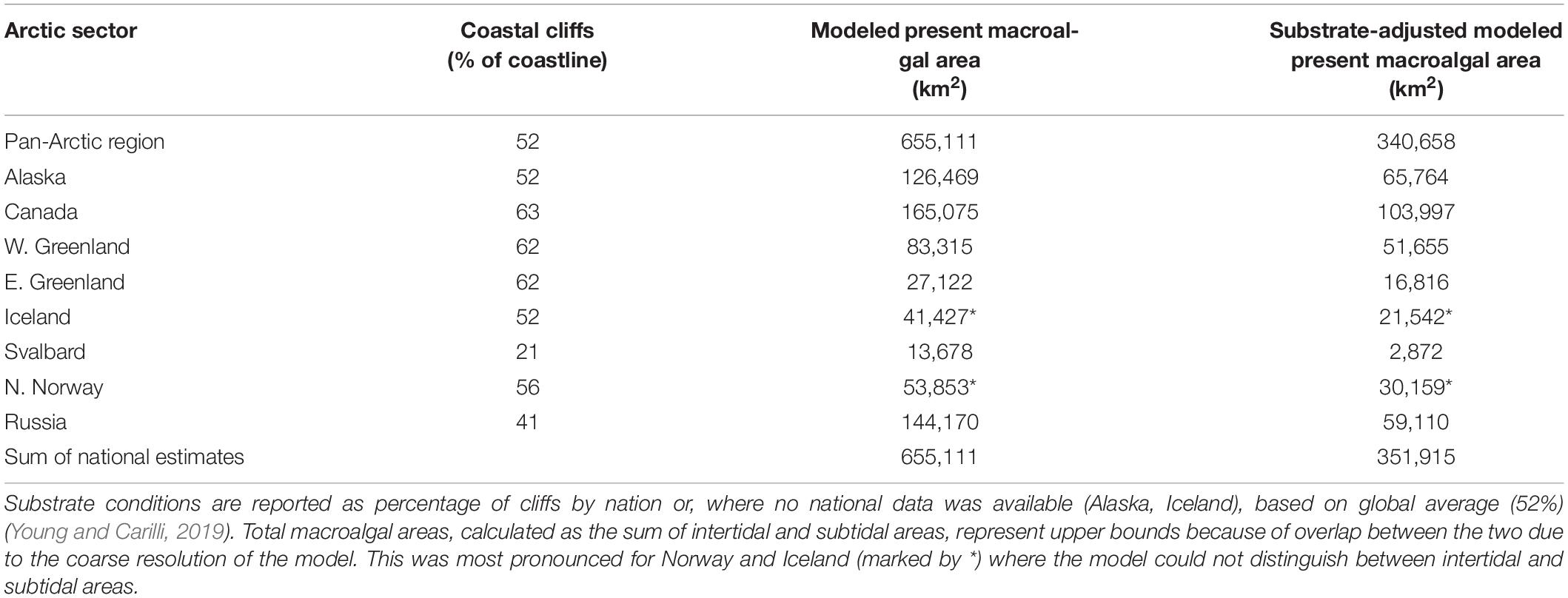
Table 4. Information on substrate conditions for Arctic coastlines and potential distribution area adjusted by substrate conditions.
Discussion: Pan-Arctic Panorama
The Arctic permafrost coastline accounts for an estimated 34% of the global shoreline with 20% of the shelf area having shallow (<20 m) depth (Lantuit et al., 2012). This region represents an enormous potential habitat for marine macrophytes, although we recognize that substrate conditions, which are still not well described, affect habitat suitability. Our distribution model quantified the potential current suitable habitat at 655,111 km2 within the Arctic Council definition of the Arctic, based on sea ice, temperature, nutrients, and salinity but not substrate conditions (Figure 6 and Tables 2, 3). Demarcation of the modeled area that solely incorporates shorelines with coastal cliffs reduces the potential distribution area to about half (340,658 km2). However, even along sedimentary coastlines, macroalgae occur on scattered stones (e.g., in Young Sound, Greenland), and sedimentary coasts also provide habitat for eelgrass (Z. marina) as reported for protected coastal stretches in inner regions of the Nuuk fjord system, as well as for several sites in Russia, Alaska, and Canada. Although the model is conservative in that we could not reproduce observed distribution in all regions (such as the northernmost sites), it provides the first Arctic overview of potential marine vegetation habitat, and an important framework for integrating further observations.
While only a handful of macrophytes occurring in the Arctic may be considered endemic Arctic species, most are boreal and temperate species (Hop et al., 2012; Wilce, 2016), persisting through cycles of glaciation, leading to unique assemblages in polar waters (Bringloe et al., 2020). The prediction that warming alone and declining ice cover has led to a northward expansion of marine macrophytes along Arctic shores (Krause-Jensen and Duarte, 2014) has mainly been informed by space for time substitutions. These patterns of change along latitudinal gradients show increased algal growth and size with decreasing latitude along the coast of Greenland (Krause-Jensen et al., 2012) and Norway (Christie et al., 2019). Our macroalgal habitat model assessed, based on modeled changes in key habitat conditions, that the potential suitable area for Arctic macroalgae has expanded by about 8.1% for intertidal algae and 44.6% for subtidal algae over the past 60–70 years, with the largest relative increase in Svalbard and the smallest in N. Norway and Iceland (Figure 6 and Table 2).
We collated observations of Arctic marine vegetation spanning at least 10 years from 39 locations across the pan-Arctic region (Table 1 and Figure 1). The majority of the series (i.e., 38) extend into the 21st century, when Arctic warming has greatly accelerated and lead to a new historical minimum of sea ice extent in 2012 (Pörtner et al., 2019), with even lower ice cover through the spring of 20202. The compiled observations of trends extending into the 21st Century were distributed across the Arctic coastlines, with three locations in Alaska, three in Canada, nine in Greenland, two in Iceland, six in Svalbard, four in Norway and eleven in Russia, thereby providing a pan-Arctic assessment (Figure 1 and Table 1). These reports of change were derived from multiple metrics, including changes in community composition and species’ northern biogeographic boundaries, growth, density, biomass, and production. However, despite these disparities, the data allowed evaluation of the consistency of reported changes with those expected with Arctic warming.
Of the 38 time series extending into the 21st Century, 22 (58%) showed an increasing trend and/or migration of the leading biogeographical edge toward north/deglaciated areas, 15 (39%) showed no obvious trend and only 4 (11%) showed a negative trend. We therefore conclude that the prediction that macrophytes are expanding in the Arctic is supported, adopting the IPCC+ confidence scale (Shapiro et al., 2010), with medium confidence (i.e., 5–8 in 10 chance) of being correct. Where several consecutive time series are available for the same site, only the most recent is included in this statement, and because three sites showed combined responses, the percentages do not sum to 100%.
The observed changes encompassed shifting ranges (6 out of the 38 time series) including poleward migration of temperate species in response to higher temperature/reduced sea ice in Alaska, Greenland, Iceland, and Russia, as well as expansion of macroalgae to new areas available for colonization with glacier retreat (e.g., Svalbard Fjords, Table 1). Colonization of new areas upon glacier retreat have also been reported from Antarctica (e.g., Quartino et al., 2013; Clark et al., 2015; Deregibus et al., 2016). However, more subtle changes within the habitats, such as changes in macroalgal dominance patterns or productivity were common responses to climate change (18 out of the 38 time series). Hence, the time series documented examples of faster vegetation growth or larger fronds/biomass/cover with warming and/or longer open water periods in both Greenland, Canada, Norway, Svalbard, and Russia (Table 1). Likewise, there are records of changed species composition over time across all Arctic regions. Overall, the multiple compiled time series confirmed the predictions of increased growth, biomass, and northward expansion of species. There are also observations of local reduction in macrophyte cover due to increased turbidity in glacier discharge areas in the Beaufort Sea (Bonsell and Dunton, 2018), Svalbard fjords (Bartsch et al., 2016; Paar et al., 2016), and Franz Josef Land, Russia (Gagaev et al., 2019) (Table 1).
Of the observations that reported contraction or no change in algal composition, distribution and/or abundance, some were associated with the existence of local pressures, such as severe eutrophication/pollution (e.g., Kola Bay), harvesting (e.g., White Sea), eelgrass wasting disease (e.g., White Sea), or sea urchin grazing (e.g., Aleutian Islands, N. Norway coast, Svalbard, Russia Barents Sea coast) (Table 1). Temperate kelp forests experience regular declines due to bursts of sea urchin grazers, often related to predator losses (Ling et al., 2015). Sea urchin populations have been reported to experience such blooms along the Aleutian Islands (Estes et al., 2004; Konar et al., 2014), along the Northern Norwegian coast extending into the Arctic (Christie et al., 2019; Norderhaug et al., 2020), and along the Russian Barents Sea coast (Deart et al., 2017), leading to kelp declines. Blicher et al. (2007) reported sea urchin populations to increase with decreasing sea ice cover along Greenland’s coast, suggesting that their abundance, along with those of macroalgae, may increase with reduced sea ice in a warming Arctic. Paar et al. (2019b) also reported increased abundance and grazing by sea urchins in Svalbard in recent warmer years (2012–2014) compared to earlier periods (1996–1998) and Küpper et al. (2016) reported recent appearance of sea urchins in the Canadian Archipelago, where they were absent in the past, but formed extensive barrens further south near the subarctic boundary in Labrador (Adey and Hayek, 2011). However, sea urchin decline has been reported recently along the Norwegian (70°N) and the Russian Barents Sea coast with the introduction of the king crab (Deart et al., 2017; Christie et al., 2019) and warming-driven increases in the edible crab in mid Norway (63–65.5°N), both predators to sea urchins (Christie et al., 2019). Sea urchin proliferations and decline may deviate changes in Arctic kelps from the predicted effects of climate change. The reported sea urchin changes may be themselves related to warming, either by directly affecting sea urchin life cycles or those of their predators. Although these interactions with climate change are being studied (e.g., Christie et al., 2019), the complexity is still poorly understood and needs attention as a possible important driver of changes in Arctic macroalgae in the future.
Poloczanska et al. (2013) document an average global poleward expansion of the leading edge of macroalgae of 52 km (0.47° latitude) decade–1, with maximum rates up to 165 km (1.49° latitude) decade–1, but did not include any observations from the Arctic region. Here we reported polar expansion of Fucus vesiculosus along Greenland’s west coast of 83 km (0.75° latitude) decade–1, despite dispersal potential for intertidal key species being relatively limited (Serrão et al., 1997; Dudgeon et al., 2001; Capdevila et al., 2018). Our modeled results infer potential polar migration rates for Arctic macroalgae (1940/1950 – 2000/2017), based primarily on increases in ocean temperature and loss of sea ice, of up to 106 km decade–1, with an average of 23.1 km decade–1 for intertidal algae and slightly less, 18.3 km for subtidal ones (Tables 2, 3).
The poleward spread of the biogeographical limits of boreal macrophyte species requires suitable, northward flowing currents, dispersal vectors, and availability of suitable substrates, which are not considered in our niche-based models. For instance, suitable current systems to support poleward transport of macrophyte propagules are present along the coasts of Svalbard, Barents Sea, the Bering Strait (Pickart et al., 2005), and Western Greenland (Rysgaard et al., 2020). Dispersal vectors include floating substrata, such as buoyant algae, wood or especially plastic (Thiel and Gutow, 2004; Wȩsławski and Kotwicki, 2018), since the Arctic Ocean is a dead end of floating plastics in the North Atlantic branch of the thermohaline circulation (Cózar et al., 2017). Migratory seabirds, such as brent geese feeding on intertidal eelgrass, or herbivorous fish feeding on marine vegetation, may also potentially serve as dispersal vectors, independent of current direction (Clausen et al., 2002; Ruz et al., 2018). Shipping is also a well-known dispersal agent. The contrast between observed expansion of macrophytes along Western Greenland and lack of such reports for Eastern Greenland is consistent with the different current systems along these coasts, while the distribution model, which ignores current patterns, reports larger expansion of potential macroalgal areas for Eastern –rather than for Western Greenland. The currents systems are characterized by a poleward transport along Western Greenland and the equatorward East Greenland Current that transports large volumes of ice and Arctic meltwater (Buch, 2000). The East Greenland Current maintains cold water temperature and also prevents the poleward dispersal of macrophytes. The important biogeographic significance of the contrast between Western and Eastern Greenland current systems is reflected in large-scale population genetic patterns for the kelp Saccharina latissima, which show that Eastern and Western populations are disconnected (Neiva et al., 2018).
Changes in macrophyte habitats in the Arctic, involving a poleward expansion and increased productivity with climate change, are of significance as these habitats supply a range of ecosystem functions (Smale et al., 2013). Seagrass, but also macroalgae, support carbon sequestration (Duarte et al., 2013; Krause-Jensen and Duarte, 2016; Pedersen et al., 2020) and, therefore, increased distribution of these habitats contribute to buffer climate change. Arctic eelgrass meadows may not sequester and store as much carbon as seagrass meadows elsewhere (Marbà et al., 2018), but large carbon stocks may accumulate over time, which will require conservation measures to maintain organic carbon sequestration. Macrophyte beds are also important sources of production for coastal food webs, as well as habitat for numerous species. For example, exported kelp carbon plays an important role in supporting Arctic benthic food webs (Petrowski et al., 2016; Vilas et al., 2020). High macrophyte productivity over the long Arctic summer days also raise locally the pH, thereby potentially providing refugia from ocean acidification to vulnerable calcifiers (Krause-Jensen et al., 2016). Moreover, expansive eelgrass meadows, such as those in Alaska with biomasses of up to 1.5 kg dw m–2 (McRoy, 1970) are important feeding areas for migratory water birds. Arctic eelgrass meadows and macroalgal forests also provide important habitat for fish (Dean et al., 2000), such as the role of eelgrass meadows and kelp forests as nurseries for juvenile cod (Gotceitas et al., 1995; Dean et al., 2000) and other fish species (Brand and Fisher, 2016), and as habitat used by schools of adult saith and pollack (Norderhaug et al., 2020).
The compilation of trends in Arctic marine vegetation reported here provides a first baseline for evaluating future changes, and contributes directly to the CMBP by gathering comparable circumpolar long-term dataset to determine pan-Arctic baseline biodiversity conditions and evaluate habitat changes with changing climate. The compiled data are still limited and biased in their spatial resolution due to differences in research infrastructure and accessibility across the Arctic. Data sets typically represent single community surveys scattered in space and time, but nevertheless provide valuable baseline information for upcoming and urgently needed further studies. We encourage the Arctic research community and monitoring programs to increase the frequency, as well as the spatial coverage, of assessments to generate time series that are robust to assess trends, rather than comparing dispersed observations in time, which may confound oscillations with trends. For instance, the Greenland Ecosystem Monitoring Program includes annual assessments of kelp growth in Young Sound (NE Greenland) and intertidal macroalgal growth in Kobbefjord (SW Greenland) since 2003 and 2012, respectively (see footnote 1), which allow identification of potential trends.
Advances in remote sensing and under-ice observation technologies may also relax constraints for direct observations under challenging Arctic conditions. For instance, fixed, continuously recording cameras and unmanned aerial vehicles, drones, are now being used to map intertidal vegetation in the Greenland Ecosystem Monitoring Program. These technologies can be used to improve data availability moving forward, but cannot compensate for paucity of records in the past. Where such past records are absent, sediment records may provide evidence of change, including coupled sediment chronologies and stable isotope analyses, which provided evidence for an expansion of eelgrass meadows in SW Greenland (Marbà et al., 2018). Advances in sediment eDNA analyses, supported with increasing libraries for eDNA assessments of Arctic macrophytes (Ortega et al., 2020), coupled with sediment chronologies, can also provide insights into changes in Arctic macrophytes over the past century, thereby compensating for the paucity of records.
The synthesis of changes in Arctic macrophytes presented here underlines a scope for a pan-Arctic science agenda to quantify actual and potential large-scale distribution of marine vegetation beyond the scattered study sites now available. Our assessment provides medium confidence for the predicted expansion of Arctic macrophytes with climate change, which is likely to accelerate in the future with rapid Arctic warming and ice melting. These changes will have important ecosystems consequences, given the major ecological roles that macrophyte habitats play. Some species of brown macroalgae are also common food items used by Inuit communities (e.g., Fucus sp., Alaria esculenta, Saccharina longicruris) (Ainana and Zagrebin, 2014; Rapinski et al., 2018). Understanding changes in macrophyte habitats is, therefore, of importance for Arctic communities. While abrupt Arctic changes are a matter of concern (Duarte et al., 2012), most attention at the ecosystem level have focused on responses of pelagic systems and charismatic and commercial fauna, and macrophyte habitats have received very limited attention. Our assessment should help incorporate macrophytes into a coherent understanding of Arctic changes, and into sustainable management strategies to avoid biodiversity losses.
Data Availability Statement
Publicly available datasets were analyzed in this study. This data can be found here: Updated data from the Greenland ecosystem monitoring program were analyzed (Figure 4, https://data.g-e-m.dk). Other individual case study time series were assessed from the literature as referenced in the paper. Species distribution modeling of past and current macroalgal distribution was based on existing datasets as referenced in the paper [Records of brown macroalgae presence were compiled from the fine-tuned dataset of marine forests (Assis et al., 2020); Biologically meaningful environmental predictors for macroalgae were extracted from Bio-ORACLE V2.1 (Assis et al., 2017)].
Author Contributions
DK-J conceived the initial idea. JA conducted the species distribution modeling. DK-J and CD drafted the non-regional sections of the manuscript. All the authors contributed information from their respective Arctic research region, and commented, improved, and approved the manuscript. KD contributed to proof edited language. MW edited the references.
Funding
This study is based on ideas discussed at an international workshop on pan-Arctic marine systems in Motovun, Croatia, organized by P. Wassmann and supported by funding from Arctic SIZE (http://site.uit.no/arcticsize/). The study was further supported by the Independent Research Fund Denmark (8021-00222 B, “CARMA” to DK-J), the Government of Greenland and the Danish Environmental Protection Agency (DANCEA, J.nr. 2019 – 8703 to DK-J, MS, and SW), the EU Horizon 2020 (FACE-IT, contract # 869154 to DK-J, IB, and KB, and INTAROS, contract # 727890 to MS), the Foundation for Science and Technology (FCT) UID/Multi/04326/2019, PTDC/BIA-CBI/6515/2020 and the transitional norm -DL57/2016/CP1361/CT0035 and MARFOR (BIODIVERSA/004/2015) to JA, the Russian Foundation for Basic Research (grant 18-05-70114 to VS, OM, and US). ArcticNet (the ArcticKelp project to PA and KF-D), and the Australian Research Council (DE190100692 to KF-D). The study is a contribution to the Greenland Ecosystem Monitoring Program (http://g-e-m.dk/) through the programs NuukBasis, ZackenbergBasis and DiskoBasis.
Conflict of Interest
The authors declare that the research was conducted in the absence of any commercial or financial relationships that could be construed as a potential conflict of interest.
Acknowledgments
We thank the team involved in sampling at Cape Farewell, Greenland, September 2011: Jens Deding, Signe Høgslund, Michael Bo Rasmussen, and Jozef Wiktor, Jr.; special thanks goes to late Dr. Poul Møller Pedersen, who passed away in June 2012. Gabrielle Martineau is thanked for assistance with data collation. Alexander Kovalevsky is thanked for preparing a map of Russian sites and Tinna Christensen for further graphical assistance.
Supplementary Material
The Supplementary Material for this article can be found online at: https://www.frontiersin.org/articles/10.3389/fmars.2020.617324/full#supplementary-material
Footnotes
References
Adey, W. H., and Hayek, L. A. C. (2011). Elucidating marine biogeography with macrophytes: quantitative analysis of the North Atlantic supports the thermogeographic model and demonstrates a distinct subarctic region in the Northwestern Atlantic. Northeastern Nat. 18, 1–128. doi: 10.1656/045.018.m801
Agardh, J. G. (1868). Bidrag till kännedomen af Spesbergen alger. Tillägg till föregaende afhandling. Handlingar 7, 28–49.
Ainana, L., and Zagrebin, I. (2014). Edible Plants of Inuits. Ethnobotanocal Guide for Local Schools of the Chukchi National District. Anchorage: Programme of US National Park Service.
Allouche, O., Tsoar, A., and Kadmon, R. (2006). Assessing the accuracy of species distribution models: prevalence, Kappa and the True Skill Statistic (TSS). J. Appl. Ecol. 43, 1223–1232. doi: 10.1111/j.1365-2664.2006.01214.x
Andersen, J. B., Böcher, J., Guttmann, B., Johnsen, I., Kampp, K., Skov, H., et al. (2005). Baseline Ecological Survey – Thule Air Base. Zoological and Botanical Inventory of the Thule Air Base and north Star Bay. Hørsholm: DHI Water & Environment.
Araújo, M. B., and New, M. (2007). Ensemble forecasting of species distributions. Trends Ecol. Evol. 22, 42–47. doi: 10.1016/j.tree.2006.09.010
Assis, J., Araújo, M. B., and Serrão, E. A. (2017). Projected climate changes threaten ancient refugia of kelp forests in the North Atlantic. Glob. Chang. Biol. 24, e55–e66. doi: 10.1111/gcb.13818
Assis, J., Fragkopoulou, E., Frade, D., Neiva, J., Oliveira, A., Abecasis, D., et al. (2020). A fine-tuned global distribution dataset of marine forests. Sci. Data 7:119. doi: 10.1038/s41597-020-0459-x
Attard, K. M., Hancke, K., Sejr, M. K., and Glud, R. N. (2016). Benthic primary production and mineralization in a High Arctic fjord: in situ assessments by aquatic eddy covariance. Mar. Ecol. Prog. Ser. 554, 35–50. doi: 10.3354/meps11780
Averintsev, V. G. (1992). “Coastal ecosystems of shelf of the Tikhaia Bay, Hooker Island and Hayes Island, Franz Josef Land,” in Environmental studies from Franz Josef Land, with emphasis on Tikhaia Bay, Hooker Island, Vol. 120, ed. I. Giertz (Oslo: Meddelelser Norsk Polarinstitutt), 43–50.
Averintseva, S. G. (1994). “Phytocenoses of Hooker Island,” in Okruzhayuschaya sreda I ekosistemy Zemli Frantsa-Iosifa (arhipelag i shelf), (Apatity: Kola Science Centre of Russian Academy of Sciences), 106–108.
Barbet-Massin, M., Jiguet, F., Albert, C. H., and Thuiller, W. (2012). Selecting pseudo-absences for species distribution models: how, where and how many? Methods Ecol. Evol. 3, 327–338. doi: 10.1111/j.2041-210X.2011.00172.x
Bartsch, I., Paar, M., Fredriksen, S., Schwanitz, M., Daniel, C., Hop, H., et al. (2016). Changes in kelp forest biomass and depth distribution in Kongsfjorden, Svalbard, between 1996–1998 and 2012–2014 reflect Arctic warming. Polar Biol. 39, 2021–2036. doi: 10.1007/s00300-015-1870-1
Beca-Carretero, P., Olesen, B., Marbà, N., and Krause-Jensen, D. (2018). Response to experimental warming in northern eelgrass populations: comparison across a range of temperature adaptations. Mar. Ecol. Prog. Ser. 589, 59–72. doi: 10.3354/meps12439
Berger, V. Y. (2001). “Fishes,” in White Sea. Ecology and Environment, eds V. Berger and S. Dahle (St. Petersburg: Derzhavets Publisher), 55–76. doi: 10.1023/A:1005655025798
Beuchel, F., and Gulliksen, B. (2008). Temporal patterns of benthic community development in an Arctic fjord (Kongsfjorden, Svalbard): results of a 24-year manipulation study. Polar Biol. 31, 913–924. doi: 10.1007/s00300-008-0429-9
Beuchel, F., Gulliksen, B., and Carroll, M. L. (2006). Long-term patterns of rocky bottom macrobenthic community structure in an Arctic fjord (Kongsfjorden, Svalbard) in relation to climate variability (1980–2003). J. Mar. Syst. 63, 35–48. doi: 10.1016/j.jmarsys.2006.05.002
Birklund, J., Wegeberg, S., and Mortensen, S. (2006). Macroalgae and Macrobenthos at Mestersvig. Marine Biological Baseline Survey in 2005 – Malmbjerg Project. Hørsholm: DHI Water & Environment.
Bischof, K., Convey, P., Duarte, P., Gattuso, J. P., Granberg, M., Hop, H., et al. (2019). “Kongsfjorden as harbinger of the future arctic: knowns, unknowns and research priorities,” in The Ecosystem of Kongsfjorden, Svalbard, eds H. Hop and C. Wiencke (Berlin: Springer International Publishing), 537–562. doi: 10.1007/978-3-319-46425-1_14
Blicher, M. E., Rysgaard, S., and Sejr, M. K. (2007). Growth and production of sea urchin Strongylocentrotus droebachiensis in a high-Arctic fjord, and growth along a climatic gradient (64 to 77°N). Mar. Ecol. Prog. Ser. 341, 89–102. doi: 10.3354/meps341089
Blinova, E. I. (1964). “Eco-floristical review of Guba Ivanovka, a relic waterbody of the Barents Sea,” in Resources of Marine Plants and their Use, (Moscow: Nauka).
Blinova, E. I. (2007). Macrophyte Seaweed and Seagrasses of the Seas of European Russia. Moscow: VNIRO.
Blok, S. E., Olesen, B., and Krause-Jensen, D. (2018). Life history events of eelgrass Zostera marina L. populations across gradients of latitude and temperature. Mar. Ecol. Prog. Ser. 590, 79–93. doi: 10.3354/meps12479
Boavida, J., Assis, J., Silva, I., and Serrão, E. A. (2016). Overlooked habitat of a vulnerable gorgonian revealed in the Mediterranean and Eastern Atlantic by ecological niche modelling. Sci. Rep. 6:36460. doi: 10.1038/srep36460
Bonsell, C., and Dunton, K. H. (2018). Long-term patterns of benthic irradiance and kelp production in the central Beaufort sea reveal implications of warming for Arctic inner shelves. Prog. Oceanogr. 162, 160–170. doi: 10.1016/j.pocean.2018.02.016
Borum, J., Pedersen, M. F., Krause-Jensen, D., Christensen, P. B., and Nielsen, K. (2002). Biomass, photosynthesis and growth of Laminaria saccharina in a high-arctic fjord, NE Greenland. Mar. Biol. 141, 11–19. doi: 10.1007/s00227-002-0806-9
Brand, M., and Fisher, P. (2016). Species composition and abundance of the shallow water fish community of Kongsfjorden, Svalbard. Polar Biol. 39, 2155–2167. doi: 10.1007/s00300-016-2022-y
Bringloe, T. T., Verbruggen, H., and Saunders, G. W. (2020). Unique biodiversity in Arctic marine forests is shaped by diverse recolonization pathways and far northern glacial refugia. Proc. Natl. Acad. Sci. U.S.A. 117, 22590–22596. doi: 10.1073/pnas.2002753117
Buch, E. (2000). A Monograph on the Physical Oceanography of the Greenland Waters. Copenhagen: Danish Meteorological Institute (DMI).
Bukina, M. B., Ivanov, M. V., and Shatskih, E. N. (2010). “Recovering of seagrass Zostera marina Linnaeus in the White Sea: the modern stag,” in Ninth All-Russian conference “Problems in Research, Exploitation and Conservation of Natural Resources of the White Sea, (St. Petersburg: Derzhavets Publisher), 27–29.
Capdevila, P., Linares, C., Aspillaga, E., Riera, J. L., and Hereu, B. (2018). Effective dispersal and density-dependence in mesophotic macroalgal forests: insights from the Mediterranean species Cystoseira zosteroides. PLoS One 13:e0191346. doi: 10.1371/journal.pone.0191346
Christie, H., Gundersen, H., Rinde, E., Filbee−Dexter, K., Norderhaug, K. M., Pedersen, T., et al. (2019). Can multitrophic interactions and ocean warming influence large-scale kelp recovery? Ecol. Evol. 9, 2847–2862. doi: 10.1002/ece3.4963
Clark, G. F., Marzinelli, E. M., Fogwill, C. J., Turney, C. S., and Johnston, E. L. (2015). Effects of sea-ice cover on marine benthic communities: a natural experiment in Commonwealth Bay, East Antarctica. Polar Biol. 38, 1213–1222. doi: 10.1007/s00300-015-1688-x
Clausen, K. K., Krause-Jensen, D., Olesen, B., and Marbà, N. (2014). Seasonality of eelgrass biomass across gradients in temperature and latitude. Mar. Ecol. Prog. Ser. 506, 71–85. doi: 10.3354/meps10800
Clausen, P., Nolet, B. A., Fox, A. D., and Klaassen, M. (2002). Long-distance endo-zoochorous dispersal of submerged macrophyte seeds by migratory waterbirds in northern Europe—a critical review of possibilities and limitations. Acta Oecol. 23, 191–203. doi: 10.1016/S1146-609X(02)01150-5
Consortium Genivar-Waska (2017). Eastmain-1-A and Sarcelle Powerhouses and Rupert Diversion: Follow-up of Eelgrass on Northeast Coast of Baie James (James Bay). Study Report, 2014. Report prepared by Consortium Genivar-Waska for HydroQuebec Production. Quebec, QC: Consortium Genivar-Waska, 83.
Cózar, A., Martí, E., Duarte, C. M., García-de-Lomas, J., Van Sebille, E., Ballatore, T. J., et al. (2017). The Arctic Ocean as a dead end for floating plastics in the North Atlantic branch of the thermohaline circulation. Sci. Adv. 3:e1600582. doi: 10.1126/sciadv.1600582
Cross, W. E., Wilce, R. T., and Fabijan, M. F. (1987). Effects of experimental releases of oil and dispersed oil on Arctic nearshore macrobenthos. III. Macroalgae. Arctic 40, 211–219. doi: 10.14430/arctic1813
Curtis, S. G. (1975). Répartition de la Zostère marine: côte est, baie James. Carte à l’échelle 1 :125 000 produite par le Service Canadien de la Faune. Ottawa: Canadian Wildlife Service.
Dankworth, M., Heinrich, S., Fredriksen, S., and Bartsch, I. (2020). DNA barcoding and mucilage ducts in the stipe reveal the presence of Hedophyllum nigripes (Laminariales, Phaeophyceae) in Kongsfjorden (Spitsbergen). J. Phycol. doi: 10.1111/jpy.13012-18-196 [Epub ahaed of print].
Dean, T. A., Haldorson, L., Laur, D. R., Jewett, S. C., and Blanchard, A. (2000). The distribution of nearshore fishes in kelp and eelgrass communities in Prince William Sound, Alaska: associations with vegetation and physical habitat characteristics. Environ. Biol. Fishes 57, 271–287. doi: 10.1023/A:1007652730085
Deart, Y. V., Antokhina, T. I, Spiridonov, V. A., and Rzhavsky, A. V. (2017). “Dynamics of hydrological regime and distribution of macrobenthos in Guba Zelanaya (East Murmansk Coast) of the Barents Sea,” in Proceedings of VI International Conference “Marine Research and Education, Moscow, 30 November – 02 December 2017, (Tver: Polipress), 447–451.
Deregibus, D., Quartino, M. L., Campana, G. L., Momo, F. R., Wiencke, C., and Zacher, K. (2016). Photosynthetic light requirements and vertical distribution of macroalgae in newly ice-free areas in Potter Cove, South Shetland Islands, Antarctica. Polar Biol. 39, 153–166. doi: 10.1007/s00300-015-1679-y
Dormann, C. F., Schymanski, S. J., Cabral, J., Chuine, I., Graham, C., Hartig, F., et al. (2012). Correlation and process in species distribution models: bridging a dichotomy. J. Biogeogr. 39, 2119–2131. doi: 10.1111/j.1365-2699.2011.02659.x
Duarte, C. M., Lenton, T. M., Wadhams, P., and Wassmann, P. (2012). Abrupt climate change in the Arctic. Nat. Clim. Change 2, 60–62. doi: 10.1038/nclimate1386
Duarte, C. M., Losada, I. J., Hendriks, I. E., Mazarrasa, I., and Marbà, N. (2013). The role of coastal plant communities for climate change mitigation and adaptation. Nat. Clim. Change 3, 961–968. doi: 10.1038/nclimate1970
Dudgeon, S., Kübler, J. E., Wright, W. A., Vadas, R. L. Sr., and Petraitis, P. S. (2001). Natural variability in zygote dispersal of Ascophyllum nodosum at small spatial scales. Funct. Ecol. 15, 595–604. doi: 10.1046/j.0269-8463.2001.00559.x
Edwards, M., Konar, B., Kim, J. H., Gabara, S., Sullaway, G., McHugh, T., et al. (2020). Marine deforestation leads to widespread loss of ecosystem function. PLoS One 15:e0226173. doi: 10.1371/journal.pone.0226173
Elith, J., Leathwick, J. R., and Hastie, T. (2008). A working guide to boosted regression trees. J. Animal Ecol. 77, 802–813. doi: 10.1111/j.1365-2656.2008.01390.x
Estes, J. A., Danner, E. M., Doak, D. F., Konar, B., Springer, A. M., Steinberg, P. D., et al. (2004). Complex trophic interactions in kelp forest ecosystems. Bull. Mar. Sci. 74, 621–638.
Estes, J. A., Tinker, M. T., Williams, T. M., and Doak, D. F. (1998). Killer whale predation on sea otters linking oceanic and nearshore ecosystems. Science 282, 473–476. doi: 10.1126/science.282.5388.473
Fagerli, C. W., Norderhaug, K. M., and Christie, H. C. (2013). Lack of sea urchin settlement may explain kelp forest recovery in overgrazed areas in Norway. Mar. Ecol. Prog. Ser. 488, 119–132. doi: 10.3354/meps10413
Feder, H. M., Norton, D. W., and Geller, J. B. (2003). A review of apparent 20th century changes in the presence of mussels (Mytilus trossulus) and macroalgae in Arctic Alaska, and of historical and paleontological evidence used to relate mollusc distributions to climate change. Arctic 56, 391–407. doi: 10.14430/arctic636
Filbee-Dexter, K., Feehan, C., and Scheibling, R. E. (2016). Large-scale degradation of a kelp ecosystem in an ocean warming hotspot. Mar. Ecol. Prog. Ser. 543, 141–152. doi: 10.3354/meps11554
Filbee-Dexter, K., Wernberg, T., Fredriksen, S., Norderhaug, K. M., and Pedersen, M. F. (2019). Arctic kelp forests: diversity, resilience and future. Glob. Planet. Change 172, 1–14. doi: 10.1016/j.gloplacha.2018.09.005
Flerov, B. K. (1932). Algae of the Novaya Zemlya coast. Distribution of algae at the shores of Novaya Zemlya. Trudy GOIN 2, 7–45.
Fox, J. F. (1979). Intermediate disturbance hypothesis. Science 204, 1344–1345. doi: 10.1126/science.204.4399.1344
Fredriksen, S., Bartsch, I., and Wiencke, C. (2014). New additions to the benthic marine flora of Kongsfjorden, western Svalbard, and comparison between 1996/1998 and 2012/2013. Bot. Mar. 57, 203–216. doi: 10.1515/bot-2013-0119
Fredriksen, S., Karsten, U., Bartsch, I., Woelfel, J., Koblowsky, M., Schumann, R., et al. (2019). “Biodiversity of benthic macro- and microalgae from svalbard with special focus on kongsfjorde,” in The Ecosystem of Kongsfjorden, Svalbard, eds H. Hop and C. Wiencke (Berlin: Springer International Publishing), 331–371. doi: 10.1007/978-3-319-46425-1_9
Fredriksen, S., and Kile, M. R. (2012). The algal vegetation in the outer part of Isfjorden, Spitsbergen: revisiting Per Svendsen’s sites 50 years later. Polar Res. 31:1. doi: 10.3402/polar.v31i0.17538
Gagaev, S. Y., Grebelny, S. D., Sirenko, B. I., Potin, V. V., and Savinkin, O. V. (2019). Benthic habitats in the Tikhaya Bight (the Hooker Island, Franz Josef Land). Proc. Zool. Inst. RAS 323, 3–15. doi: 10.31610/trudyzin/2019.323.1.3
Gemp, K. P. (1962). “Resources of seaweed and seagrasses and perspectives of their further use in the White Sea,” in Trudy Vsesoyuznogio Soveschaniya Rabotnikov Vodoroslevooi Promyshlennosti SSSR, (Arkhangel: SevNIIP), 15–31.
GENIVAR (2010). Centrales de “Eastmain-1-A et de la Sarcelle et dérivation Rupert. Suivi de la Zostère Marine de la côte nord-est de la baie James. État de référence 2009. Rapport de GENIVAR Société en commandite pour Hydro-Québec et la Société “énergie de la Baie James. Montreal, QC: GENIVAR.
Golikov, A. N., and Averintsev, V. G. (1977). “Biocenoses of the upper part of the shelf of the Franz Josef Land Archipelago,” in Biocoenoses of the Franz Josef Land Shelf and Adjacent Aquatories, (Leningrad: Nauka), 5–54.
Gotceitas, V., Fraser, S., and Brown, J. A. (1995). Habitat use by juvenile Atlantic cod (Gadus morhua) in the presence of an actively foraging and non-foraging predator. Mar. Biol. 123, 421–430. doi: 10.1007/BF00349220
Gouvêa, L. P., Assis, J., Gurgel, C. F. D., Serrão, E. A., Silveira, T. C. L., Santos, R., et al. (2020). Golden carbon of Sargassum forests revealed as an opportunity for climate change mitigation. Sci. Total Environ. 729, 138745. doi: 10.1016/j.scitotenv.2020.138745
Gunnarsson, K., and Hauksson, E. (2009). Succession and benthic community development in the sublittoral zone at the recent volcanic island, Surtsey, southern Iceland. Surtsey Res. 12, 161–166.
Hansen, J. R., and Jenneborg, J. H. (1996). “Bentic marine algae and cyanobacteria,” in A catalogue of Svalbard Plants, Fungi, Algae and Cyanobacteria, Vol. 198, eds A. Elvebakk and P. Presterud (Oslo: Norsk Polarinst Akr), 361–374.
Hauksson, E. (1992). Studies of the subtidal fauna of Surtsey, Iceland in 1980 to 1987 and changes in subtidal fauna from 1964 to 1987. Surtsey Res. Progr. Rep. 10, 33–42.
Hauksson, E. (2000). A survey of the benthic coastal fauna of Surtsey, Iceland in 1997. Surtsey Res. 11, 85–88.
Hegseth, E. N., Assmy, P., Wiktor, J. M., Wiktor, J. Jr., Kristiansen, S., Leu, E., et al. (2019). “Phytoplankton seasonal dynamics in Kongsfjorden, Svalbard and the adjacent shelf,” in The Ecosystem of Kongsfjorden, Svalbard, eds H. Hop and C. Wiencke (Berlin: Springer International Publishing), 173–228. doi: 10.1007/978-3-319-46425-1
Hofner, B., Müller, J., and Hothorn, T. (2011). Monotonicity-constrained species distribution models. Ecology 92, 1895–1901. doi: 10.1890/10-2276.1
Hogrefe, K. R., Ward, D. H., Donnelly, T. F., and Dau, N. (2014). Establishing a baseline for regional scale monitoring of eelgrass (Zostera marina) habitat on the lower Alaska Peninsula. Rem. Sens. 6, 12447–12477. doi: 10.3390/rs61212447
Høgslund, S., Sejr, M. K., Wiktor, J., Blicher, M. E., and Wegeberg, S. (2014). Intertidal community composition along rocky shores in Southwest Greenland: a quantitative approach. Polar Biol. 37, 1549–1561. doi: 10.1007/s00300-014-1541-7
Hop, H., Kovaltchouk, N. A., and Wiencke, C. (2016). Distribution of macroalgae in Kongsfjord, Svalbard. Polar Biol. 39, 2037–2051. doi: 10.1007/s00300-016-2048-1
Hop, H., and Wiencke, C. (2019). The ecosystem of Kongsfjord, Svalbard: Advances in Polar Ecology. Berlin: Springer International Publishing, doi: 10.1007/978-3-319-46425-1
Hop, H., Wiencke, C., Vögele, B., and Kovaltchouk, N. A. (2012). Species composition, zonation, and biomass of marine benthic macroalgae in Kongsfjorden. Svalbard. Bot. Mar. 55, 399–414. doi: 10.1515/bot-2012-0097
Huntington, H. P. (2001). Arctic Flora and Fauna: Status and Conservation. Akureyri: Conservation of Arctic Flora and Fauna.
Hurd, C. L., Harrison, P. J., Bischof, K., and Lobban, C. S. (2014). Seaweed Ecology and Physiology, 2nd Edn. Cambridge: Cambridge University Press. doi: 10.1017/CBO9781139192637
Jiménez-Valverde, A., and Lobo, J. M. (2007). Threshold criteria for conversion of probability of species presence to either–or presence–absence. Acta Oecol. 31, 361–369. doi: 10.1016/j.actao.2007.02.001
Jónsson, H. (1912). “The marine algal vegetation,” in The Botany of Iceland, eds L. K. Rosenvinge and E. Warming (Copenhagen: Frimodt), 1–186.
Jónsson, S. (1966). Le commencement du peuplement benthique des côtes rocheuses du Surtsey, la nouvelle île volcanique dans l’Atlantique nord. C. R. Acad. Sci. 262, 915–918.
Jónsson, S. (1968). Survey on the intertidal and subtidal algae on Surtsey in 1967. Surtsey Res. Progr. Rep. 4, 67–73. doi: 10.1016/s0022-0981(02)00442-2
Jónsson, S., and Gunnarsson, K. (1982). Marine algal colonization at Surtsey. Surtsey Res. Progr. Rep. 9, 33–45.
Jónsson, S., Gunnarsson, K., and Briane, J.-P. (1987). Évolution de la nouvelle flore marine de l’île volcanique de Surtsey, islande. J. Mar. Res. Inst. Reykjavík 10, 1–30.
Kjellman, F. R. (1883a). Norra Ishafvets algflora. Vega-Exped Vetensk Iakttag 3, 1–431. doi: 10.5962/bhl.title.60248
Kjellman, F. R. (1883b). The Algae of the Arctic Sea. A Survey of the Species, Together with an Exposition of the General Characters and the Development of the Flora. Kongl. Svenska Vetenskaps-akademiens Handlingar, Vol. 20. Stockholm: P.A. Norstedt & Söner, 351.
Knowlton, N., and Jackson, J. B. C. (2008). Shifting baselines, local impacts, and global change on coral reefs. PLoS Biol. 6:e54. doi: 10.1371/journal.pbio.0060054
Konar, B., Edwards, M. S., and Estes, J. A. (2014). Biological interactions maintain the boundaries between kelp forests and urchin barrens in the Aleutian Archipelago. Hydrobiologia 724, 91–107. doi: 10.1007/s10750-013-1727-y
Kortsch, S., Primiceiro, R., Beuchel, F., Renaud, P. E., Rodrigues, J., and Lønne, O. J. (2012). Climate-driven regime shifts in Arctic marine benthos. Proc. Natl. Acad. Sci. U.S.A. 109, 14052–14057. doi: 10.1073/pnas.1207509109
Krause-Jensen, D., and Duarte, C. M. (2014). Expansion of vegetated coastal ecosystems in the future Arctic. Front. Mar. Sci. 1:77. doi: 10.3389/fmars.2014.00077
Krause-Jensen, D., and Duarte, C. M. (2016). Substantial role of macroalgae in marine carbon sequestration. Nat. Geosci. 9, 737–742. doi: 10.1038/ngeo2790
Krause-Jensen, D., Marbà, N., Olesen, B., Sejr, M. K., Christensen, P. B., Rodrigues, J., et al. (2012). Seasonal sea ice cover as principal driver of spatial and temporal variation in the depth extension and annual production of kelp in Greenland. Glob. Change Biol. 18, 2981–2994. doi: 10.1111/j.1365-2486.2012.02765.x
Krause-Jensen, D., Marbà, N., Sanz-Martin, M., Hendriks, I. E., Thyrring, J., Carstensen, J., et al. (2016). Long photoperiods sustain high pH in Arctic kelp forests. Sci. Adv. 2:e1501938. doi: 10.1126/sciadv.1501938
Krumhansl, K. A., Okamoto, D. K., Rassweiler, A., Novak, M., Bolton, J. J., and Cavanaugh, K. C. (2016). Global patterns of kelp forest change over the past half-century. Proc. Natl. Acad. Sci. U.S.A. 113, 13785–13790. doi: 10.1073/pnas.1606102113
Kruss, A., Tegowski, J., Tatarek, A., Wiktor, J., and Blondel, P. (2017). Spatial distribution of macroalgae along the shores of Kongsfjorden (West Spitsbergen) unsing acoustic imaging. Pol. Polar Res. 38, 205–229. doi: 10.1515/popore-2017-0009
Küpper, F. C., Peters, A. F., Shewring, D. M., Sayer, M. D. J., Mystikou, A., Brown, H., et al. (2016). Arctic marine phytobenthos of northern Baffin Island. J. Phycol. 52, 532–549. doi: 10.1111/jpy.12417
Lalumière, R., and Lemieux, C. (2002). Suivi Environnemental des Projets La Grande-2-A et La Grande-1. La Zostère Marine de la Côte Nord-Est de la baie James. Rapport Synthèse pour la Période 1988-2000. Montreal, QC: Groupe conseil GENIVAR inc.
Lalumière, R., Messier, D., Fournier, J.-J., and McRoy, C. P. (1994). Eelgrass meadows in a low arctic environment, the northeast coast of James Bay, Québec. Aquat. Bot. 47, 303–315. doi: 10.1016/0304-3770(94)90060-4
Lantuit, H., Overduin, P. P., Couture, N., Wetterich, S., Aré, F., Atkinson, D., et al. (2012). The Arctic coastal dynamics database: a new classification scheme and statistics on Arctic permafrost coastlines. Estuaries Coasts 35, 383–400. doi: 10.1007/s12237-010-9362-6
Lee, R. K. S. (1980). A Catalogue of the Marine Algae of the Canadian Arctic. Ottawa, ON: National Museum of Natural Sciences.
Ling, S. D., Scheibling, R. E., Rassweiler, A., Johnson, C. R., Shears, N., Connell, S. D., et al. (2015). Global regime shift dynamics of catastrophic sea urchin overgrazing. Philos. Trans. R. Soc. Lond. B Biol. Sci. 370:20130269. doi: 10.1098/rstb.2013.0269
Lund, S. (1959a). The marine algae of East Greenland. I. taxonomical part. Meddelelser om Grønland 156, 1–247.
Lund, S. (1959b). The marine algae of East Greenland. II. Geographic distribution. Meddelelser om Grønland 156, 1–72.
Malavenda, S., Makarov, M., Ryzhik, I., Mityae, M., and Malavenda, S. (2018). Occurrence of Ulva lactuca L. 1753 (Ulvaceae, Chlorophyta) at the Murman coast of the Barents Sea. Pol. Res. 37:1503912. doi: 10.1080/17518369.2018.1503912
Malavenda, S. S., and Malavenda, S. A. (2012). Indications of degradation in the phytocoenoses of the southern and middle parts of the Kola Bay. Vestnik Murmanskogo Technicheskogo Univ. 15, 794–802.
Malavenda, S. V., and Metelsky, A. A. (2013). Associations of kelp algae of the inlets Ivanovskaya and Drozdovka of the Eastern Murmanks coast. Vestnik Murmanskogo Technicheskogo Univ. 16, 493–500.
Marbà, N., Krause-Jensen, D., Alcoverro, T., Birk, S., Pedersen, A., Neto, J. M., et al. (2013). Diversity of European seagrass indicators: patterns within and across regions. Hydrobiologia 704, 265–278.
Marbà, N., Krause-Jensen, D., Masqué, P., and Duarte, C. M. (2018). Expanding Greenland seagrass meadows contribute new sediment carbon sinks. Sci. Rep. 8:14024. doi: 10.1038/s41598-018-32249-w
Marbà, N., Krause-Jensen, D., Olesen, B., Christensen, P. B., Merzouk, A., Rodrigues, J., et al. (2017). Climate change stimulates the growth of the intertidal macroalgae Ascophyllum nodosum near the northern distribution limit. Ambio 46, 119–131. doi: 10.1007/s13280-016-0873-7
Maturilli, M., Hanssen-Bauer, I., Neuber, R., Rex, M., and Edvardsen, K. (2019). “The atmosphere above Ny-Ålesund – climate and global warming, ozone and surface UV radiation,” in The Ecosystem of Kongsfjorden, Svalbard, eds H. Hop and C. Wiencke (Berlin: Springer International Publishing), 23–46. doi: 10.1007/978-3-319-46425-1_2
Maximova, O. V. (2016). “Macrophytobenthos of the Kara Sea,” in Kara Sea. Ecological Atlas, ed. A. I. Isachenko (Moscow: Arctic Centre), 89–95.
Maximova, O. V. (2017a). “Macrophytobenthos of the White Sea,” in The System of the White Sea. The Processes of Sedimentation, Geology and History, ed. A. P. Lisitsyn (Moscow: Nauchnyi Mir), 386–430.
Maximova, O. V. (2017b). “Macrophytobenthos,” in Laptev Sea. Ecological Atlas, ed. A. I. Isachenko (Moscow: Arctic Centre), 109–118.
McRoy, C. P. (1970). Standing stocks and other features of eelgrass (Zostera marina) populations on the coast of Alaska. Can. J. Fish. Aquat. Sc. 27, 1811–1821. doi: 10.1139/f70-199
Merzouk, A., and Johnson, L. E. (2011). Kelp distribution in the northwest Atlantic Ocean under a changing climate. J. Exp. Mar. Biol. Ecol. 400, 90–98. doi: 10.1016/j.jembe.2011.02.020
Metzger, J. R., Konar, B., and Edwards, M. S. (2019). Assessing a macroalgal foundation species: community variation with shifting algal assemblages. Mar. Biol. 166:156. doi: 10.1007/s00227-019-3606-1
Mikhaylova, T. A. (2000). Structure and interannual dynamics of Laminaria phytocoenosis in the White Sea. Botanicheskiy Zhurnal 80, 78–88.
Mikhaylova, T. A. (2010). Laminaria hyperborea (Laminariacea) on the Murman Coast of the Barents Sea. Botanicheskiy Zhurnal 95, 326–338.
Mikhaylova, T. A. (2012). Laminaria hyperborea (Laminariacea) association on the Murman Coast of the Barents Sea. Botanicheskiy Zhurnal 97, 712–729.
Mohr, J. L., Wilimovsky, N. J., and Dawson, E. Y. (1957). An Arctic Alaskan kelp bed. Arctic 10, 45–52. doi: 10.14430/arctic3754
Müller, R., Laepple, T., Bartsch, I., and Wiencke, C. (2009). Impact of oceanic warming on the distribution of seaweeds in polar and cold-temperate waters. Bot. Mar. 52, 617–638. doi: 10.1515/BOT.2009.080
Naumov, A. D. (2013). Long-term fluctuations of soft-bottom intertidal community structure affected by ice cover at two small sea bights in the Chupa Inlet (Kandalaksha Bay) of the White Sea. Hydrobiologia 706, 159–173. doi: 10.1007/s10750-012-1339-y
Neiva, J., Paulino, C., Nielsen, M. M., Krause-Jensen, D., Saunders, G. W., Assis, J., et al. (2018). Glacial vicariance drives phylogeographic diversification in the amphi-boreal kelp Saccharina latissima. Sci. Rep. 8:1112. doi: 10.1038/s41598-018-19620-7
Norderhaug, K. M., and Christie, H. C. (2009). Sea urchin grazing and kelp re-vegetation in the NE Atlantic. Mar. Biol. Res. 5, 515–528. doi: 10.1080/17451000902932985
Norderhaug, K. M., Filbee-Dexter, K., Freitas, C., Birkely, S. R., Christensen, L., Mellerud, I., et al. (2020). Ecosystem-Level Effects of Large-Scale Disturbance in Kelp Forests. Marine Ecology Progress Series. London: Inter-Research Science Publisher, doi: /10.3354/meps13426
Ogilvie, A. E., and Jónsson, T. (2001). “Little ice age” research: a perspective from Iceland. Clim. Change 48, 9–52. doi: 10.1023/A:1005625729889
Olesen, B., Krause-Jensen, D., Marbà, N., and Christensen, P. B. (2015). Eelgrass (Zostera marina L.) in subarctic Greenland: dense meadows with slow biomass turnover in cold waters. Mar. Ecol. Prog. Ser. 518, 107–121. doi: 10.3354/meps11087
Ørberg, S. B., Krause-Jensen, D., Mouritsen, K. N., Olesen, B., Marbà, N., Larsen, M. H., et al. (2018). Canopy-forming macroalgae facilitate recolonization of sub-Arctic intertidal fauna and reduce temperature extremes. Front. Mar. Sci. 5:332. doi: 10.3389/fmars.2018.00332
Ortega, A., Geraldi, N. R., Díaz−Rúa, R., Ørberg, S. B., Wesselmann, M., Krause−Jensen, D., et al. (2020). A DNA mini−barcode for marine macrophytes. Mol. Ecol. Resour. 20, 920–935. doi: 10.1111/1755-0998.13164
Ottósson, J. G., Sveinsdóttir, A., and Harðardóttir, M. (2016). Vistgerðir á Íslandi. Fjölrit Náttúrufræðistofnunar no. 54. Náttúrufræðistofnun Islands.
Paar, M., de la Vega, C., Horn, S., Asmus, R., and Asmus, H. (2019b). Kelp belt ecosystem response to a changing environment in Kongsfjorden (Spitsbergen). Ocean Coastal Manag. 167, 60–77. doi: 10.1016/j.ocecoaman.2018.09.003
Paar, M., Lebreton, B., Graeve, M., Greenacre, M., Asmus, R., and Asmus, H. (2019a). Food sources of macrozoobenthos in an Arctic kelp belt: trophic relationships revealed by stable isotope and fatty acid analyses. Mar. Ecol. Prog. Ser. 615, 31–49. doi: 10.3354/meps12923
Paar, M., Voronkov, A., Hop, H., Brey, T., Bartsch, I., Schwanitz, M., et al. (2016). Temporal shift in biomass and production of macrozoobenthos in the macroalgal belt at Hansneset, Kongsfjorden, after 15 years. Polar Biol. 39, 2065–2076. doi: 10.1007/s00300-015-1760-6
Pavlov, A. K., Leu, E., Hanelt, D., Bartsch, I., Karsten, U., Hudson, S. R., et al. (2019). “The underwater light climate in Kongsfjorden and its ecological implications,” in The Ecosystem of Kongsfjorden, Svalbard, eds H. Hop and C. Wiencke (Berlin: Springer International Publishing), 137–172. doi: 10.1007/978-3-319-46425-1_5
Pavlova, O., Gerland, S., and Hop, H. (2019). “Changes in sea-ice extent and thickness in Kongsfjorden, Svalbard (2003-2016),” in The Ecosystem of Kongsfjorden, Svalbard, eds H. Hop and C. Wiencke (Berlin: Springer International Publishing), 105–136. doi: 10.1007/978-3-319-46425-1_4
Pedersen, M. F., Filbee-Dexter, K., Norderhaug, K. M., Fredriksen, S., Frisk, N. L., and Wernberg, T. (2020). Detrital carbon production and export in high latitude kelp forests. Oecologia 192, 227–239. doi: 10.1007/s00442-019-04573-z
Pedersen, P. M. (1976). Marine, benthic algae from southernmost Greenland. Meddelelser om Grønland 199, 1–80. doi: 10.1515/botm.1972.15.1.1
Pessarrodona, A., Moore, P. J., Sayer, M. D., and Smale, D. A. (2018). Carbon assimilation and transfer through kelp forests in the NE Atlantic is diminished under a warmer ocean climate. Glob. Change Biol. 24, 4386–4398. doi: 10.1111/gcb.14303
Peterson, A., Soberón, J., Pearson, R., Anderson, R., Martínez-Meyer, E., Nakamura, M., et al. (2011). Ecological Niches and Geographic Distributions (MPB-49). Oxford: Princeton University Press, doi: 10.23943/princeton/9780691136868.001.0001
Petrich, C., Tivy, A. C., and Ward, D. H. (2014). Reconstruction of historic sea ice conditions in a sub-Arctic lagoon. Cold Reg. Sci. Technol. 98, 55–62. doi: 10.1016/j.coldregions.2013.10.011
Petrowski, S., Molis, M., Bender, A., and Buschbaum, C. (2016). Disturbance effects of kelp thalli on structure and diversity of a coastal Arctic marine soft-bottom assemblage. Polar Biol. 39, 2131–2140. doi: 10.1007/s00300-015-1714-z
Pickart, R. T., Weingartner, T. J., Pratt, L. J., Zimmermann, S., and Torres, D. J. (2005). Flow of winter-transformed Pacific water into the Western Arctic. Deep Sea Res. Part II Top. Stud. Oceanogr. 52, 3175–3198. doi: 10.1016/j.dsr2.2005.10.009
Piquet, A. M.-T., van de Poll, W. H., Visser, R. J. W., Wiencke, C., Bolhuis, H., and Buma, A. G. J. (2014). Springtime phytoplankton dynamics in Arctic Korssfjorden and Kongsfjorden (Spitsbergen) as a function of glacier proximity. Biogeosciences 11, 2263–2279. doi: 10.5194/bg-11-2263-2014
Pogrebov, V. B., Propp, M. V., and Tarasov, V. G. (1975). Ecosystem of the Barents Sea fiord. II. Bottom communities. Russ. J. Mar. Biol. 4, 51–60.
Poloczanska, E. S., Brown, C. J., Sydeman, W. J., Kiessling, W., Schoeman, D. S., Moore, P. J., et al. (2013). Global imprint of climate change on marine life. Nat. Clim. Change. 3, 919–925. doi: 10.1038/nclimate1958
Pörtner, H. O., Roberts, D., Masson-Delmotte, V., Zhai, P., Tignor, M., Poloczanska, E., et al. (2019). IPCC Special Report on the Ocean and Cryosphere in a Changing Climate. Geneva: Intergovernmental Panel on Climate Change.
Pronina, O. A. (2011). “Harvesting of seaweed,” in Pomor Fisheries, ed. V. A. Stasenkov (Arkhangel: Northern Branch of PINRO), 232–262.
Pronina, O. A., and Repina, O. I (2005). “White sea commercial seaweed: resources. Harvesting and processing technology,” in IX Interational Conference “Problems of Study, Rational Use and Protection of Natural Resources of the White Sea, (Petrozavodsk: University of Zagreb), 269–273.
Propp, M. V., Denisov, V. A., Pogrebov, V. B., and Ryabushko, V. I. (1975). Ecosystem of the Barents Sea fiord. I. Hydrological and hydrochemical characteristics. Biol. Morja 3, 44–56.
Quartino, M. L., Deregibus, D., Campana, G. L., Latorre, G. E. J., and Momo, F. R. (2013). Evidence of macroalgal colonization on newly ice-free areas following glacial retreat in Potter Cove (South Shetland Islands), Antarctica. PLoS One 8:e58223. doi: 10.1371/journal.pone.0058223
Rapinski, M., Cuerrier, A., Harris, C., Elders of Ivujivik, Elders of Kangiqsujuaq, and Lemire, M. (2018). Inuit perception of marine organisms: from folk classification to food harvest. J. Ethnobiol. 38, 333–355. doi: 10.2993/0278-0771-38.3.333
Raybaud, V., Beaugrand, G., Goberville, E., Delebecq, G., Destombe, C., Valero, M., et al. (2013). Decline in kelp in west Europe and climate. PLoS One 8:e66044. doi: 10.1371/journal.pone.0066044
Rinde, E., and Sjøtun, K. (2005). Demographic variation in the kelp Laminaria hyperborea along a latitudinal gradient. Mar. Biol. 146, 1051–1062. doi: 10.1007/s00227-004-1513-5
Rosenvinge, L. K. R. (1898). Deuxiéme mémoire sur les algues du Groenland. Meddelelser om Grønland 20, 125.
Rosenvinge, L. K. R. (1910). On the marine algæ from North-East Greenland collected by the” Danmark Expedition”. Meddelelser om Grønland 43, 91–133.
Ruz, C. S., Muth, A. F., Tala, F., and Pérez-Matus, A. (2018). The herbivorous fish, Aplodactylus punctatus, as a potential facilitator of dispersal of kelp, Lessonia trabeculata, in Chile. J. Exp. Mar. Biol. Ecol. 500, 112–119. doi: 10.1016/j.jembe.2017.12.007
Rysgaard, S., Boone, W., Carlson, D., Sejr, M. K., Bendtsen, J., Juul-Pedersen, T., et al. (2020). An updated view on water masses on the pan−west Greenland continental shelf and their link to proglacial fjords. J. Geophys. Res. Oceans 125:e2019JC015564. doi: 10.1029/2019JC015564
Savchenko, O. N., and Naumov, A. D. (2020). The 30-year biomass dynamics of several species of the intertidal communities in two small bights of the Kandalaksha Bay (The White Sea). Zoologicheskiy Zhurnal 99, 728–744.
Schoschina, E. V. (1997). On Laminaria hyperborea on the Murman Coast of the Barents Sea. Sarsia 82, 371–383. doi: 10.1080/00364827.1997.10413663
Serrão, E. A., Kautsky, L., Lifvergren, T., and Brawley, S. H. (1997). Gamete dispersal and pre-recruitment mortality in Baltic Fucus vesiculosus. Phycologia 36, 101–102.
Shapiro, H. T., Diab, R., de Brito Cruz, C. H., Cropper, M., Fang, J., Fresco, L., et al. (2010). Climate Change Assessments: Review of the Processes and Procedures of the IPCC. Technical report. Amsterdam: InterAcademy Council.
Shklyarevich, G. A. (2014). Recovery of Zostera marina L. tickets in Kandalaksha Bay of White Sea. Uchenye Zapiski Petrozavodskogo Gosudarstvennogo Universiteta 4, 13–18.
Short, F. T. (2008). Report to the Cree Nation of Chisasibi on the Status of Eelgrass in James Bay: An Assessment of Hydro-Quebec data Regarding Eelgrass in James Bay, Experimental Studies on the Effects of Reduced Salinity on Eelgrass, and Establishment of James Bay Environmental Monitoring by the Cree Nation. Durham: Jackson Estuarine Laboratory.
Short, F. T. (2019). “Eelgrass in Hudson Bay,” in From Science to Policy in the Greater Hudson Bay Marine Region: An Integrated Regional Impact Study (IRIS) of Climate Change and Modernization, eds Z. A. Kuzyk and L. M. Candlish (Québec City, QC: ArcticNet), 236–238.
Shoshina, E. V. (2012). “Macrophytes,” in Biological resources of the White Sea: exploration and exploitation. Explorations of the Fauna of the Sea, ed. V. Y. Berger (St. Petersburg: Zoological Institute of Russian Academy of Sciences), 132–148.
Shoshina, E. V., and Anisimova, N. A. (2013). Macroalgae from the Ledianaya Gavan Bay area (Novaya Zemlya, North Island, Kara Sea). Vestnik Murmanskogo Technichesko Univeristeta 16, 530–535.
Shtrik, V. A., Vozzhinskaya, V. B., and Vekhov, N. V. (2000). “Marine algae of the Novaya Zemlya coast and Yugorsky Shar Strait,” in Marine Hydrobiological Research, (Moscow: Russian Federal Institute of Fishery and Oceanography), 88–98.
Sigurdsson, A. (1968). The coastal invertebrate fauna of Surtsey and Vestmannaeyjar. Surtsey Res. Progr. Rep. 4, 95–107.
Simakova, U. V. (2016). “Current status and long-term changes of Zostera marina L. in case of the Babye More Lagoon (White Sea),” in Studies of Babie More, A Semi-Enclosed Lagoon: Geology, Hydrology, Biota and Changes Under Coastal Transgression, Vol. 12, eds V. O. Mokievsky, P. Yu, A. I. I. Dgebuadze, and A. B. Tzetlin (Moscow: Moscow State University), 163–176.
Simakova, U. V., Neretina, T. V., Kolyuchkina, G. A., Spiridonov, V. A., and Loktionov, Y. A. (2016). “Distribution and genetic diversity of eelgrass Zostera marina L. in the northern seas of Russia,” in Proceedings of V International Conference “Marine research and education, Moscow, 19-22 October 2016, (Moscow: Pheoria), 423–426.
Simmonds, I. (2015). Comparing and contrasting the behaviour of Arctic and Antarctic sea ice over the 35 year period 1979-2013. Ann. Glaciol. 56, 18–28. doi: 10.3189/2015AoG69A909
Smale, D. A., Burrows, M. T., Moore, P., O’Connor, N., and Hawkins, S. J. (2013). Threats and knowledge gaps for ecosystem services provided by kelp forests: a northeast Atlantic perspective. Ecol. Evol. 3, 4016–4038. doi: 10.1002/ece3.774
Sorokin, A. A., and Peltikhina, T. S. (1991). Laminariacean Seaweed of the Barents Sea. Murmansk: PINRO.
Spalding, M. D., Fox, H. E., Allan, G. R., Davidson, N., Ferdaña, Z. A., Finlayson, M., et al. (2007). Marine ecoregions of the world: a bioregionalization of coastal and shelf areas. BioScience 57, 573–583. doi: 10.1641/B570707
Svendsen, H., Beszczynska-Møller, A., Hagen, J. O., Lefauconnier, B., Tverberg, V., Gerland, S., et al. (2002). The physical environment of Kongsfjorden–Krossfjorden, an Arctic fjord system in Svalbard. Polar Res. 21, 33–166. doi: 10.3402/polar.v21i1.6479
Svendsen, P. (1957). Om Algevetasjonen på Spitsbergen. En Undersøkelse av den Marine algefloraen i den ytre del av Isfjorden. Ph. D. Thesis, University of Oslo, Oslo.
Talbot, S. L., Sage, G. K., Rearick, J. R., Fowler, M. C., Muniz-Salazar, R., Baibak, B., et al. (2016). The structure of genetic diversity in eelgrass (Zostera marina L.) along the north Pacific and Bering Sea coasts of Alaska. PLoS One 11:e0152701. doi: 10.1371/journal.pone.0152701
Thiel, M., and Gutow, L. (2004). The ecology of rafting in the marine environment. I. The floating substrata. Oceanogr. Mar. Biol. Annu. Rev. 42, 181–264. doi: 10.1201/9780203507810.ch6
Thorarinsdottir, G. G., Gunnarsson, K., and Gíslason, ÓS. (2014). “Invasive species: case studies from Iceland,” in Marine Invasive Species in the Arctic, eds L. Fernandez, B. A. Kaiser, and N. Vestergaard (Copenhagen: Nordic Council), 83–103.
Thorson, G. (1933). Investigations on shallow water animal communities in the Franz Joseph Fjord (East Greenland) and adjacent waters. Meddelser om Grønland 100, 1–68.
Thyrring, J., Wegeberg, S., Blicher, M. E., Krause-Jensen, D., Høgslund, S., Olesen, B., et al. (2020). “Latitudinal patterns in intertidal ecosystem structure in west greenland suggest resilience to climate change,” in Proceedings of the Arctic Change 2020.
Tislenko, D. I., and Ivanov, B. V. (2015). Long-term variability of Atlantic water temperature in the Svalbard fjords in conditions of past and recent global warming. Czech Polar Rep. 5, 134–142. doi: 10.5817/CPR2015-2-12
Tverberg, V., Skogseth, R., Cottier, F., Sundfjord, A., Walczowski, W., Inall, M. E., et al. (2019). “The Kongsfjorden transect: seasonal and inter-annual variability,” in The Ecosystem of Kongsfjorden, Svalbard, eds H. Hop and C. Wiencke (Berlin: Springer International Publishing), 49–104. doi: 10.1007/978-3-319-46425-1_3
Vilas, D., Coll, M., Pedersen, T., Corrales, X., Filbee-Dexter, K., Pedersen, M. F., et al. (2020). Kelp-carbon uptake by Arctic deep-sea food webs plays a noticeable role in maintaining ecosystem structural and functional traits. J. Mar. Syst. 203:103268. doi: 10.1016/j.jmarsys.2019.103268
Vinogradova, K. L. (1995a). “Seaweeds,” in Atlas of Marine Flora of Southern Spitsbergen, eds R. Z. Klekowski and J. M. Weslaski (Gdansk: Polish Academy of Sciences).
Vinogradova, K. L. (1995b). The checklist of the marine algae from Spitsbergen. Bot. Z SSR 80, 50–61.
Ward, D. H., and Amundson, C. L. (2019). Monitoring Annual Trends in Abundance of Eelgrass (Zostera marina) at Izembek National Wildlife Refuge, Alaska, 2018 (No. 2019-1042). U.S. Geological Survey Open-File Report 2019-1042. Reston, VA: United States Geological Survey, doi: 10.3133/ofr20191042
Ward, D. H., Markon, C. J., and Douglas, D. C. (1997). Distribution and stability of eelgrass beds at Izembek Lagoon, Alaska. Aquat. Bot. 58, 229–240. doi: 10.1016/S0304-3770(97)00037-5
Wassmann, P., Duarte, C. M., Agusti, S., and Sejr, M. K. (2011). Footprints of climate change in the Arctic marine ecosystem. Glob. Change Biol. 17, 1235–1249. doi: 10.1111/j.1365-2486.2010.02311.x
Wegeberg, S. (2011). “Benthic flora,” in Eastern Baffin Bay – A Strategic Environmental Impact Assessment of Hydrocarbon Activities, eds D. M. Boertmann and A. Mosbech (Aarhus: Aarhus University), 55–62.
Wegeberg, S. (2012a). “Macrophytes,” in The Davis Strait. A Preliminary Strategic Environmental Impact Assessment of Hydrocarbon Activities in the Eastern Davis Strait, eds F. Merkel, D. Boertmann, A. Mosbech, and F. Ugarte (Aarhus: Aarhus University), 74–81.
Wegeberg, S. (2012b). “Benthic flora,” in The Western Greenland Sea, A Strategic Environmental Impact Assessment of Hydrocarbon Activities, eds D. Boertmann and A. Mosbech (Aarhus: Aarhus University), 48–55.
Wegeberg, S. (2013). “Benthic flora,” in Disko West. A Strategic Environmental Impact Assessment of Hydrocarbon Activities, eds D. Boertmann, A. Mosbech, D. Schiedek, and M. Dünweber (Aarhus: Aarhus University), 48–55.
Wernberg, T., Bennett, S., Babcock, R. C., de Bettignies, T., Cure, K., Depczynski, M., et al. (2016). Climate-driven regime shift of a temperate marine ecosystem. Science 353, 169–172. doi: 10.1126/science.aad8745
Wȩsławski, J. M., and Kotwicki, L. (2018). Macro-plastic litter, a new vector for boreal species dispersal on Svalbard. Pol. Polar Res. 39, 165–174. doi: 10.24425/118743
Weslawski, J. M., Wiktor, J. Jr., and Kotwicki, L. (2010). Increase in biodiversity in the arctic rocky littoral, Sorkappland, Svalbard, after 20 years of climate warming. Mar. Biodiv. 40, 123–130. doi: 10.1007/s12526-010-0038-z
Wilce, R. T. (1962). A new member of the Punctariaceae: Platysiphon verticillatus gen. nov., sp. nov. Botanisk Tidsskrift 58, 35–42.
Wilce, R. T. (1964). “Studies on benthic marine algae in north-west Greenland,” in Proceedings of the International Seaweed Symposium, eds A. D. de Virville and J. Feldmann (Amsterdam: Elsevier), 280–286.
Wilce, R. T. (2016). The “Arctic Stamp”, its imprint on an endangered marine flora. Perspect. Phycol. 3, 155–180. doi: 10.1127/pip/2016/0046
Wilce, R. T., and Dunton, K. H. (2014). The Boulder Patch (North Alaska, Beaufort Sea) and its benthic algal flora. Arctic 67, 43–56. doi: 10.14430/arctic4360
Wilson, K. L., and Lotze, H. K. (2019). Climate change projections reveal range shifts of eelgrass Zostera marina in the Northwest Atlantic. Mar. Ecol. Prog. Ser. 620, 47–62. doi: 10.3354/meps12973
Wulff, A., Iken, K., Quartino, M. L., Al-Handal, A., Wiencke, C., and Clayton, M. N. (2009). Biodiversity, biogeography and zonation of marine benthic micro- and macroalgae in the Arctic and Antactic. Bot. Mar. 52, 491–507. doi: 10.1515/BOT.2009.072
Yershov, P., and Sukhotin, A. (2015). Age and growth of marine three-spined stickleback in the White Sea 50 years after a population collapse. Polar Biol. 38, 1813–1823. doi: 10.1007/s00300-015-1743-7
Young, A. P., and Carilli, J. E. (2019). Global distribution of coastal cliffs. Earth Surf. Process Landf. 44, 1309–1316. doi: 10.1002/esp.4574
Zavalko, S. E., and Shoshina, E. V. (2008). Multilevel morphophysiological assessment of the fucoid algae conditions under the antropogenic pollution. Vestnik Murmanskogo Technichesko Univ. 11, 423–431.
Zinova, E. S. (1912). Algae of murman. Green and red algae. Trudy sankt-peterburgskogo obschestva estestvoispytatelei. Proc. St. Petersburg Soc. Naturalists 23, 170–343.
Keywords: distribution, abundance, trends, Arctic, sea-ice, warming, eelgrass (Zostera marina), macroalgae
Citation: Krause-Jensen D, Archambault P, Assis J, Bartsch I, Bischof K, Filbee-Dexter K, Dunton KH, Maximova O, Ragnarsdóttir SB, Sejr MK, Simakova U, Spiridonov V, Wegeberg S, Winding MHS and Duarte CM (2020) Imprint of Climate Change on Pan-Arctic Marine Vegetation. Front. Mar. Sci. 7:617324. doi: 10.3389/fmars.2020.617324
Received: 14 October 2020; Accepted: 30 November 2020;
Published: 23 December 2020.
Edited by:
Paul F. J. Wassmann, Arctic University of Norway, NorwayReviewed by:
Haakon Hop, Norwegian Polar Institute, NorwayUlf Karsten, University of Rostock, Germany
Copyright © 2020 Krause-Jensen, Archambault, Assis, Bartsch, Bischof, Filbee-Dexter, Dunton, Maximova, Ragnarsdóttir, Sejr, Simakova, Spiridonov, Wegeberg, Winding and Duarte. This is an open-access article distributed under the terms of the Creative Commons Attribution License (CC BY). The use, distribution or reproduction in other forums is permitted, provided the original author(s) and the copyright owner(s) are credited and that the original publication in this journal is cited, in accordance with accepted academic practice. No use, distribution or reproduction is permitted which does not comply with these terms.
*Correspondence: Dorte Krause-Jensen, ZGtqQGJpb3MuYXUuZGs=