- Pharmacognosy, Department of Pharmaceutical Biosciences, Uppsala University, Uppsala, Sweden
Siliceous spicules in demosponges exist in a variety of shapes, some of which look like minute spheres of glass. They are called “sterrasters” when they belong to the Geodiidae family (Tetractinellida order) and “selenasters” when they belong to the Placospongiidae family (Clionaida order). Today, the Geodiidae represent a highly diverse sponge family with more than 340 species, occurring in shallow to deep waters worldwide, except for the Antarctic. The molecular phylogeny of Geodiidae is currently difficult to interpret because we are lacking morphological characters to support most of its clades. To fill this knowledge gap, the surface microornamentations of sterrasters were compared in different genera. Observations with scanning electron microscopy revealed four types of surfaces, which remarkably matched some of the Geodiidae genera: type I characteristic of Geodia, type II characteristic of Pachymatisma, Caminus, and some Erylus; type III characteristic of other Erylus; type IV characteristic of Caminella. Two subtypes were identified in Geodia species: warty vs. smooth rosettes. These different microornamentations were mapped on new Geodiidae COI (Folmer fragment) and 28S (C1–D2) phylogenetic trees. The monophyly of the Geodiidae was once again challenged, thereby suggesting that sterrasters have evolved independently at least three times: in the Geodiinae, in the Erylinae and in Caminella. Surface microornamentations were used to review the fossil record of sterrasters and selenasters through the paleontology literature and examination of fossils. It was concluded that “rhaxes” in the literature may represent mixes of sterrasters and selenasters: while Rhaxella spicules may belong to the Placospongiidae, Rhaxelloides spicules belong to the Geodiidae. The putative Geodiidae fossil genera, Geoditesia, and Geodiopsis, are reallocated to Tetractinellida incertae sedis. Isolated Miocene-Pliocene fossil sterrasters Hataina (Huang, 1967), Silicosphaera (Hughes, 1985) and Conciliaspongia (Robinson and Haslett, 1995) become junior synonyms of Geodia (Lamarck, 1815). Overall, the fossil record suggested that Geodiidae was present at least since the Middle Jurassic (163–166 Mya), while Geodia sterrasters were present since the Santonian/Campanian boundary, Late Cretaceous (83.6 Mya).
ZooBank Article Registration: urn:lsid:zoobank.org:pub:91B1B3AC-8862-4751-B272-8A3BDF4DEE77.
Introduction
Siliceous spicules in demosponges exist in a variety of shapes, some of which look like minute spheres of glass. These were first described and illustrated in the literature by Donati (1753), who found them in the species Geodia cydonium from the Adriatic Sea: he called these spicules “little balls.” They are later called “globular crystalloids,” “globate spicules,” or “globostellates” by sponge taxonomists, until Sollas (1888, p. lxiv) finally coins the term “sterraster” (from the Greek “sterros” meaning “solid” and “firm”). Meanwhile, similar ball-shaped spicules are observed in another genus, Placospongia, and these are at first considered as “sterrasters” (Sollas, 1888) before Hanitsch (1895) coins the term “selenaster” for these different spicules (coming from the Greek word “selene” for “moon,” referring to the “half-moon” shape). Finally, an additional term “aspidaster” is created by von Lendenfeld (1910, p. 267), convinced that the flattened sterrasters in the genus Erylus are significantly different from those in Geodia.
Sterrasters/aspidaster spicules are currently the main synapomorphy of the family Geodiidae (suborder Astrophorina, order Tetractinellida). This family currently includes five genera with sterrasters (Geodia, Erylus, Pachymatisma, Caminella, and Caminus) and several others that have secondarily lost their sterrasters (Ecionemia, Stelletinopsis, Rhabdastrella, Melophlus, Penares), some Stelletta, maybe even the Calthropellidae (Cárdenas et al., 2010, 2011). The Geodiidae therefore includes more than 340 species, without the Calthropellidae (Van Soest et al., 2020). They occur in shallow to deep waters worldwide (except for the Antarctic) (Table 1). Geodia represents the largest group with 163 species (Van Soest et al., 2020), and dozens of undescribed ones (P. Cárdenas, unpublished data). They can be massive animals, more than a meter large (Santucci, 1922; Corriero, 1987 (1989); Cárdenas et al., 2013), and six species of Geodia are particularly abundant and therefore important for the North Atlantic deep-sea ecosystems as key boreo-arctic sponge ground forming species (Klitgaard and Tendal, 2004; Cárdenas et al., 2013).
Selenasters are the main synapomorphy of Placospongia (family Placospongiidae, order Clionaida), a well-supported monophyletic genus (Becking, 2013) from shallow temperate/tropical waters worldwide. It is not a very diverse genus with only 10 species currently described (WPD) and a handful of undescribed species (Hajdu et al., 2011; Moraes, 2011; Becking, 2013). Placospongia species are usually small, encrusting, and never occur in high densities.
Sterrasters/selenasters are big enough to examine in some detail their surfaces with an optical microscope. However, the use of the scanning electron microscope (SEM) enabled a significantly better understanding of the surface microornamentations. A few descriptive terms have also appeared to describe and compare in greater detail the microornamentations of these ball-shaped spicules. Polyaxial spicules such as the sterrasters and aspidasters, are the result of fused “actines” (= branches of asters), later covered with “rosettes” made of different “rays.” The “hilum” is a small area without rosettes or any kind of surface pattern. There are no particular terms to describe the surface of selenasters, except for the “hilum,” also present. A few authors in the 1990s started to show good quality SEM close-ups of sterraster/selenaster surface and hilum (González-Farías, 1989; Lehnert, 1993; Boury-Esnault et al., 1994) but it is Mothes et al. (1999) and Adams and Hooper (2001) who systematized the SEM observation of the rosettes while revising Erylus species. da Silva (2002) continued and surveyed the sterrasters of Western Atlantic and Eastern Pacific Geodia species: in particular, she looked at the number of rays in the rosettes and the shape of the hilum in adult-stage sterrasters. This was followed by Cárdenas et al. (2009, 2013) who added measurements of the rosettes and hilum. Although there appears to be no significant variation in the size of the rosettes and hilum between species, Cárdenas et al. (2009, 2013) noticed that rosettes could be smooth or warty and hypothesized that this character could be of phylogenetic value if studied more broadly. Furthermore, the rosette morphology also seemed to be variable between Geodia, Pachymatisma, and Caminella (Cárdenas and Rapp, 2013; Cárdenas et al., 2018) which suggests that a more detailed study of the sterraster/aspidaster surface would potentially bring new characters for Geodiidae genera identification.
The present study proposes to test these two hypotheses: (i) surface microornamentations are different in the Geodiidae genera and (ii) smooth or warty rosette are restricted to certain Geodia clades. To this end, new SEM observations were made and mapped on updated molecular COI and 28S phylogenies of the Geodiidae. Results will then be discussed in the light of the spicule microfossil record, especially with respect to the acknowledged and yet recurrent confusion of selenasters with sterrasters, as well as the nature of “rhax” fossils. Indeed, there is a need in sponge paleontology and micropaleontology for more robust loose-spicule markers. Finally, the timing of the emergence of sterrasters and selenasters in the fossil record will be estimated. This data will be essential for future time-calibrated sponge phylogenies, always in great need of robust calibration points.
Materials and Methods
Observation of Recent Sterrasters
SEM illustrations of sterrasters from the literature were compiled, especially for species for which molecular data exists, in order to later map these characters on a molecular phylogeny. Additionally, sterraster spicules from several species belonging to all Geodiidae genera (Geodia, Erylus, Pachymatisma, Caminella, and Caminus) were examined using SEM. Sterrasters were obtained from specimens by digesting a small piece of the cortex (0.5 × 0.2 cm) in chlorine. After several washes with water, 50% ethanol and 100% ethanol, spicules were placed on top of a cover slip glass taped to a stub and coated with a gold/palladium mix, before being observed with a SEM.
To examine the position of sterrasters in the cortex of Geodia barretti, two preparations were made. First, a fresh piece including cortex was preserved in 2.5% glutaraldehyde in 0.4 M PBS (with 0.34 M NaCl). The sample was further rinsed using a solution of 0.6 M NaCl and PBS and postfixed in 1% osmium tetroxide-potassium ferrocyanide for 1h at 4°C. Then it was rinsed in PBS and distilled water several times, and dehydrated through an ethanol series. Once in 100% ethanol, the sample was immersed in liquid nitrogen for cryofracture, and hit with a metal hammer to break it in pieces with smooth surfaces. Finally, the sample was critically point-dried, carbon-coated (20 nm), and imaged in a ZEISS Ultra Plus Field Emission SEM. Secondly, a piece was frozen on the field, preserved at −80°C, before being freeze-dried. A thick section cut with a scalpel blade was glued to an SEM stub before being gold/palladium coated and observed in a ZEISS Supra 35VP SEM.
Some Astrophorina subsamples from the U.S. National Cancer Institute’s (NCI) collection of sponges and sequenced by the PorToL project (Porifera Tree of Life)1 were re-examined for this study and their identifications revised. NTM Z005203 (off Darwin, northern Australia, 6 m) was originally identified as Geodia sp., “NCI135” in Redmond et al. (2013) but re-examination of the specimen for this study revealed a typical oscule-like cloaca and the presence of characteristic short-shafted triaenes and spherules (albeit irregular) in the ectocortex so it was re-identified as Caminus sp. (probably a new species). CASIZ 301086 (South Africa, 27 m) originally identified as Penares cf. alata, “NCI181” (Redmond et al., 2013; Thacker et al., 2013) was not Penares alata and so it was renamed Penares sp. CASIZ 300347 (Misima Island, Papua New Guinea, 23 m) originally identified as Penares nux, “NCI450” (Redmond et al., 2013; Thacker et al., 2013) was re-identified as Neamphius cf. huxleyi. HBOI 14-VIII-09-2-004 (Florida, Canaveral bioherms, 492 m) originally identified as Pachastrella sp., “JR15” in Thacker et al. (2013) was re-identified as Characella sp.
Observation of Fossil Material
Slides and specimens from the fossil collection of the NHM were examined, including slides of Rhaxella perforata Hinde, 1890 (NHMUK PI S1682, 1683a, 1684) from the type locality, Late Jurassic, Oxfordian (Lower Calcareous Grit Formation, Scarborough, North Yorkshire, England); R. perforata spicules were measured using an optical microscope. A thick section from a Rhaxella chert (S7502, Oxford, Suffolk, Red Crag Formation, Pliocene-Pleistocene) was also examined. Slides of potential Geodiidae fossils, genera Geodiopsis (Schrammen, 1910) and Geoditesia Zhuravleva, in Rezvoi et al. (1962) (previously Geodites; Carter, 1871), were examined: Geodiopsis cretacea (Schrammen, 1899), prepared from holotype (NHMUK PI P6702, P6703, P6704) from the Late Cretaceous, Campanian (Oberg, N. Germany); Pachymatisma virga (Hinde, 1885) (NHMUK PI S1070, S1041, S1069, syntypes), Geodites haldonensis (Hinde, 1885), Geodites carteri (Hinde, 1885), Geodites gracilis (Hinde, 1885), Geodites divergens (Hinde, 1885) (NHMUK PI S1054, S1073, syntypes), all from the Late Cretaceous, Albian-Cenomanian (Upper Greensand Formation, southern England).
Two specimens (S10–13, S10–18) of Geoditesia jordanensis (Ungureanu et al., 2018) (courtesy of F. Ahmad) were examined, they come from the Middle Jurassic, Callovian (Mughanniyya Formation, northwestern Jordan). Specimen S10–13 was sectioned with an ISOMET diamond saw (speed 5) to obtain a 1 mm thick section. After a mild dissolution with 1% acetic acid (7 min), the section was oven-dried (2 h, 60°C) and coated before SEM observation. The surface of half of the specimen S10–18 was directly coated before SEM observation.
Finally, the paleontology literature was surveyed for all records of ball-shaped spicules: sterrasters, selenasters, rhaxes and spherical/subspherical spicules. The information from 60 articles mentioning ball-shaped fossil spicules was compiled in Supplementary Table S1, where the following data was recorded: age, locality/lithostratigraphic unit, morphology of the spicule, abundance, size, siliceous or calcite, paleoenvironment/depth, and taxonomic ID.
Molecular Phylogenetic Study
In order to understand the evolution of sterraster morphology, surface microornamentations were mapped on a molecular phylogeny of the Geodiidae. However, because previous molecular phylogenetic studies challenge the monophyly of the Geodiidae (Cárdenas et al., 2011; Schuster et al., 2015; Kelly et al., 2019) all Astrophorina sequences were included in our analyses; one Thoosina (Alectona millari) and the deep-diverging Theneidae were used as outgroups. The Astrophorina cytochrome c oxidase I (COI) (Folmer fragment) and 28S (C1–D2) alignments from Kelly et al. (2019) were retrieved and COI was sequenced for a few more species for which sterraster SEM detailed observations were available in the literature or examined in this study: Geodia pocillum (Van Soest, 2017), holotype RMNH POR 10547; Geodia garoupa (Carvalho et al., 2016), holotype MNRJ 7349; Geodia cf. curacaoensis (Van Soest et al., 2014), HBOI 14-XI-02-1-003 (Bahamas, 439 m), id by PC; Geodia nodastrella (Carter, 1876), field# BANGAL 0710-041DR06110810, id by PC (Galicia Bank, 920 m); Geodia japonica (Sollas, 1888), field#AB15-0035 (Eastern Gulf of Alaska, United States, 89 m), id by Lehnert and Stone (2016); Pachymatisma nodosa (Sim-Smith and Kelly, 2015), holotype NIWA 53817; Caminella prima (Sim-Smith and Kelly, 2015), holotype NIWA 51723; Geodia margarita (Sim-Smith and Kelly, 2015), holotype NIWA 71189; Penares euastrum (Schmidt, 1868), previously called Erylus euastrum, PC631 (Capo Rizutto, Italy, 150 m); Caminus carmabi (Van Soest et al., 2014), HBOI 11-V-00-1-007 (Curacao, 282 m), id by PC; Caminus sp., NTM Z005203, PorToL project, id by PC (Darwin, North Australia, 6 m).
DNA was extracted using the DNeasy Blood and Tissue kit (Qiagen, Hilden, Germany). COI (658 bp) amplification was done using universal Folmer primers HCO and LCO with the PCR program: [5 min/94°C; 35 cycles (15 s/94°C, 15 s/46°C, 15 s/72°C); 7 min/72°C]. Folmer primers commonly fail to amplify COI for most Erylus/Penares species so Penares euastrum COI was sequenced in two parts: part (1) is the minibarcode (130 bp) obtained with primers LCO/Tetract-minibarR1 (Cárdenas and Moore, 2019) and part (2) obtained with primer jgHCO (Geller et al., 2013) and primer ErylusCOI-F2 (5’-CTCCYGGATCAATGTTGGG-3’) (Cárdenas et al., 2018); PCR program was the same for with Folmer primers. COI sequences from Erylus trisphaerus (de Laubenfels, 1953) (HBOI 12-VIII-95-2-2, Florida, United States, 55 m) could not be amplified so a part of 28S (the C1–D1–C2 domains, 369 bp) was sequenced instead using primer pair C1’/Ep3 (Chombard et al., 1998) with the same PCR program except for a 50°C annealing temperature. After many failed attempts to amplify 28S (C1–D2) of Geodia atlantica (Stephens, 1915) and Geodia phlegraei (Sollas, 1880b) by PCR with primers C1’/D2 (Cárdenas et al., 2011), the shorter C1–C2 fragment was obtained using primers C1’/Ep3. These sequences were used to fish the longer C1–D2 fragment in transcriptomes of these two species (Genbank BioProject ID: PRJNA603347), obtained from specimens from Northeast Atlantic specimens (courtesy of A. Riesgo and V. Koutsouveli); this was done through local Blasts using CLC Genomics Workbench 5.5. All new sequences were submitted to Genbank: 11 COI sequences (MT815820–MT815830) and three 28S sequences (MT835460–MT835462).
The COI data matrix included 112 sequences and was 658 bp long; the 28S data matrix included 110 sequences and was 857 pb long. The 28S alignment was automatically aligned using MAFFT v.7 (Katoh and Standley, 2013), L-INS-i option, implemented in AliView 1.26 (Larsson, 2014). COI and 28S final alignments can be found in the Supplementary Material. Phylogenetic analyses were conducted on the CIPRES science gateway v. 3.32 (Miller et al., 2010): RAxML 8.2.12 (Stamatakis, 2014) for maximum likelihood (ML). For RAxML, 1,000 bootstrap iterations were run.
Results and Discussion
Rosettes of Sterrasters, a Structure for Matrix Attachment
Sterrasters tethered with fibers in the cortex were observed in historical observations with optical microscopes (Donati, 1753; Sollas, 1880c; von Lendenfeld, 1894; Figures 1A,B) and much later confirmed with a transmission electron microscope (Müller et al., 2007; Figures 4G,H). In this study, these structures are observed for the first time with the SEM. Cryofractured and freeze-dried cortex preparations of G. barretti showed that sterrasters were inserted in a dense collagen-type matrix (Figure 1C) and linked to each other with fibrils (Figures 1C,D). Sollas (1882, p. 142) mentions that a few fusiform cells are intermingled with the collagen fibers but this could not be confirmed. In the cryofractured preparation empty cavities were left after the dissolution of the sterrasters. In the sterraster molds, every rosette had its own mold, giving it a honeycomb-like structure (Figures 1D,E); this showed that the rosettes are deeply inserted in the matrix. Moreover, additional holes and thread-like structures were present within each rosette mold (Figure 1E), representing the molds of the rosette rays. These observations highlight the role of these rosettes as hook structures on which the collagen matrix is interlocked. On the freeze-dried preparations, where spicules were not dissolved, fibers were clearly seen attached to the sterraster rosettes (Figure 1F), enveloping the rosettes rays, thus confirming previous historical observations (Sollas, 1888, p. lxiv). It is reasonable to suggest that the complex microornamentations of rosettes offer a stronger grip for the collagen fibers than if the sterrasters were smooth. This is of importance to fulfill the main function of the sterraster Geodiidae cortex: a solid physical protection of the sponge against predators. Indeed, sterrasters per se do not seem to be deterrent to fish spongivores (Chanas and Pawlik, 1995) but when strongly interconnected to each other they form a protective barrier against these predators: when Geodia species have a damaged cortex, it is common to see fish feed on them (Hill and Hill, 2002; personal observation). The beak of the hawksbill turtle (Meylan, 1988) or the radula of chitons (Todt et al., 2009) are specialized tools that can respectively rupture or dig into the sterraster cortex. And yet, the cortex must be malleable enough to also allow for growth and movement of cells that cross it to reach the surface, e.g., spherulous cells transporting specialized metabolites (Figure 1E). The cortex toughness and flexibility is the result of this network of sterrasters, interconnected by fibers hooked to the rosettes. These silica-collagen composite structures could have biomimetic potential and be a source of inspiration to produce strong and yet flexible structures such as coatings or fasteners.
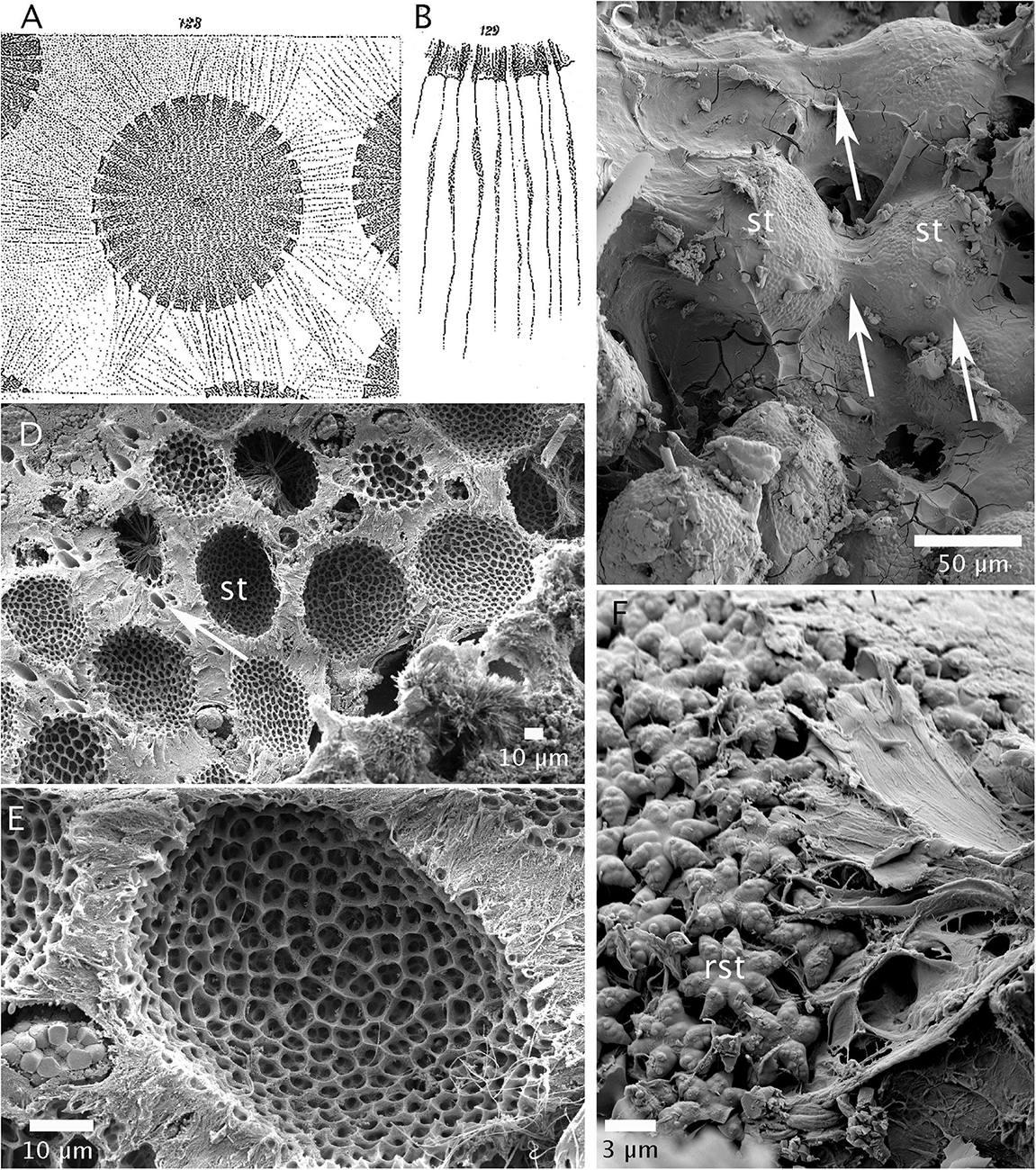
Figure 1. Position of sterrasters in the cortex. (A) Sterrasters in the endocortex of Geodia cydonium, connected via fibers, drawing is a copy from von Lendenfeld, (1894, plate VIII, 128). A sterraster in this species is 70 μm in diameter. (B) Possible fibrous cells attached to sterraster rosettes, drawing is a copy from von Lendenfeld, (1894, plate VIII, 129). A rosette is 5 μm in diameter. (C) Freeze-dried cortex of Geodia barretti showing fiber bundles (arrows) connecting the sterrasters (st). Scale: 50 μm. (D) Cryofracture in the cortex of G. barretti with spicules dissolved beforehand. Pockets in which sterrasters (st) are positioned in the endocortex, surrounded by a dense collagen-type matrix. Other holes (white arrow) indicate the position of the microxeas crossing the cortex. Scale: 10 μm. Picture: V. Koutsouveli and A. Riesgo. (E) Sterraster mold showing that every rosette is tightly inserted in a pocket, in the matrix. A spherulous cell is visible on the left (just above the scale). Same specimen as in D. Scale: 10 μm. Picture: V. Koutsouveli and A. Riesgo. (F) Close-up of a sterraster showing fibrils attached to the warty rosettes (rst), Same specimen as in C. Scale: 3 μm.
Differences and Similarities of Sterrasters and Selenasters
Although sterrasters and selenasters are both subspherical spicules, this is the result of convergent evolution as shown by the independent evolutionary history of Geodiidae and Placospongiidae (e.g., Redmond et al., 2013), and by the different ontogeny of these spicules: sterrasters originate from a polyaxial microsclere (euaster) while selenasters originate from a straight to slightly twisted monoaxial microsclere (spiraster-derived), which explains the common bean shape of selenasters (Vosmaer and Vernhout, 1902). Table 2 summarizes the resulting differences and similarities of both spicules, which will be detailed in this part, based on new SEM observations. These characters will be of importance for the micropaleontology discussions and interpretations that follow.
Both sterrasters and selenasters have a small shallow depression called “hilum” (Figures 2–8). In sterrasters, the hilum has been shown to mark the position of the microsclerocyte nucleus during spiculogenesis, taking place in the choanosome (Sollas, 1880c, p. 256). The hilum is usually a smooth depression, with occasional spines in sterrasters (Figures 2–6) and occasional ridges in selenasters (Figure 8). Therefore, bean-shaped selenasters have a notch marking the bend as well as a hilum (Figure 7); selenasters do not always have a notch, but they always have a hilum. In sterrasters, there is always a hilum (Figures 2–6), unless the sterraster is too small and it does not compete for space with the nucleus [e.g., no hilum in Geodia cordata, the sterrasters are only 25–30 μm (Figure 2G) vs. >50 μm in most other Geodia].
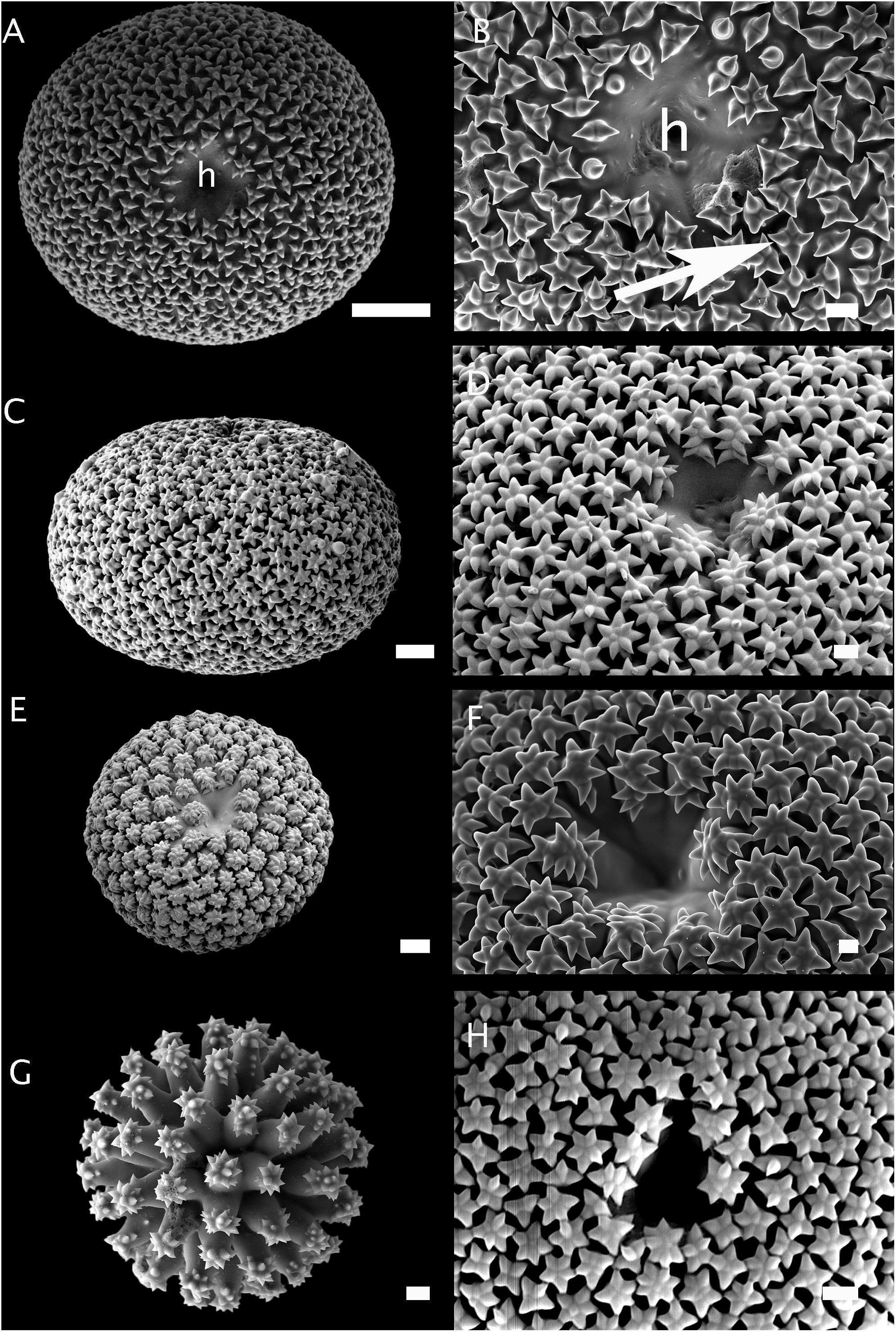
Figure 2. Morphology of sterrasters with smooth rosettes. (A,B) Sterraster of Geodia atlantica (holotype, S.R.151-27/364-1914); (h) is the hilum; the arrow points to a smooth rosette made up of rays. Scale A: 20 μm. Scale B. 2 μm. (C,D) Sterraster of Geodia phlegraei (holotype, NHM 1910.1.1.840). Scale C: 10 μm. Scale D. 2 μm. (E) Sterraster of Geodia parva (holotype, ZMBN 100). Scale: 10 μm. (F) Sterraster of Geodia gibberosa (holotype, MNHN-DT 608). Scale: 2 μm. (G) Sterraster of Geodia cordata, previously called Rhabdastrella cordata (South Australia, SAMA S1026). Scale: 2 μm. (H) Sterraster of Geodia vaubani (New Caledonia, MNHN-IP-2015-1667). Scale: 5 μm.
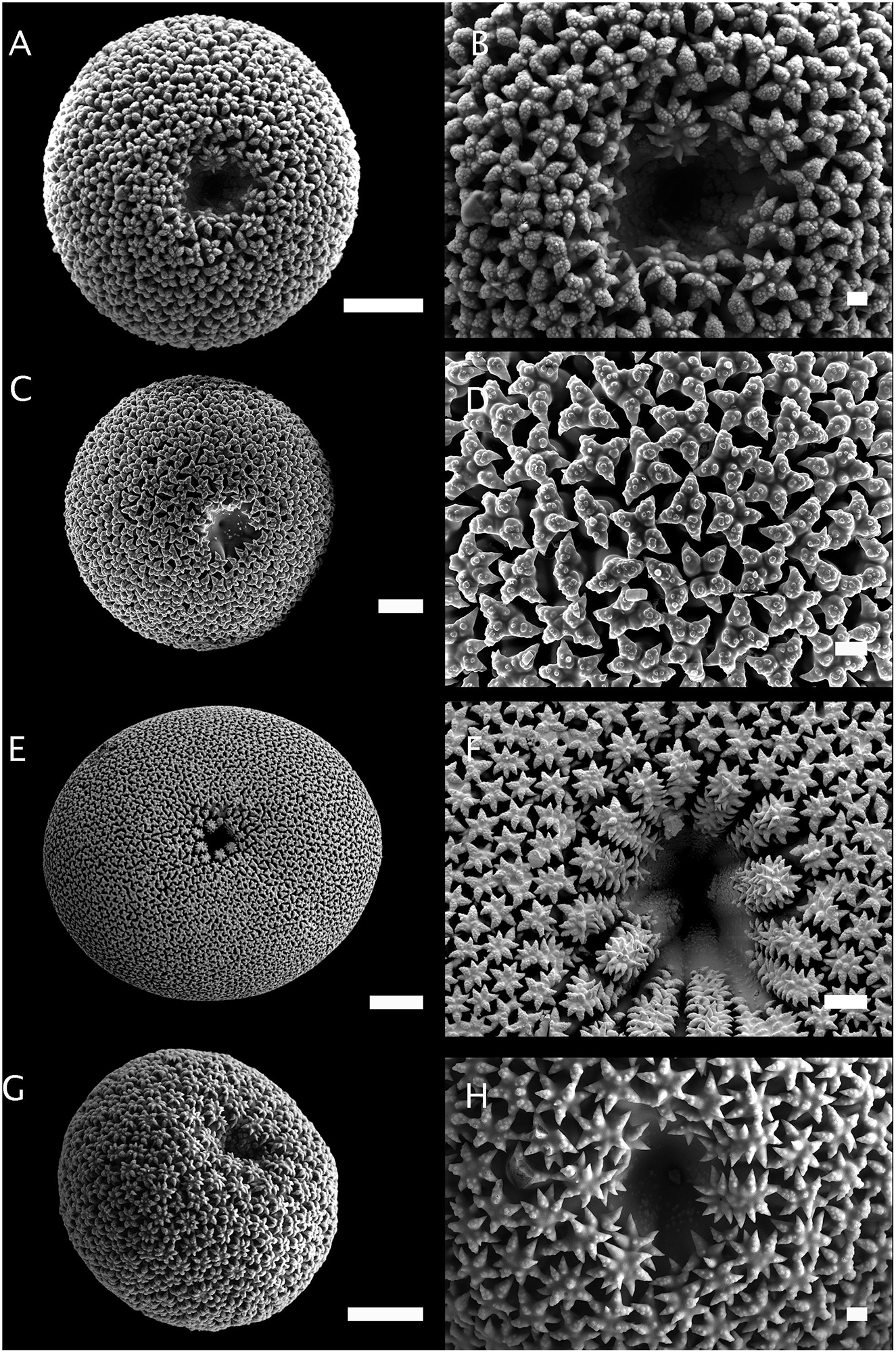
Figure 3. Morphology of sterrasters with warty rosettes. (A,B) Sterraster of Geodia barretti (Norway, ZMBN 77922). Scale A: 20 μm. Scale B. 2 μm. (C,D) Sterraster of Geodia papyracea (holotype, YPM 5045). Scale C: 10 μm. Scale D. 2 μm. (E,F) Sterraster of Geodia macandrewii (Norway, ZMBN 89717). Scale E: 50 μm. Scale F: 2 μm. (G,H) Sterraster of Geodia cydonium (Croatia, ZMBN 77923). Scale G: 20 μm. Scale H: 2 μm.
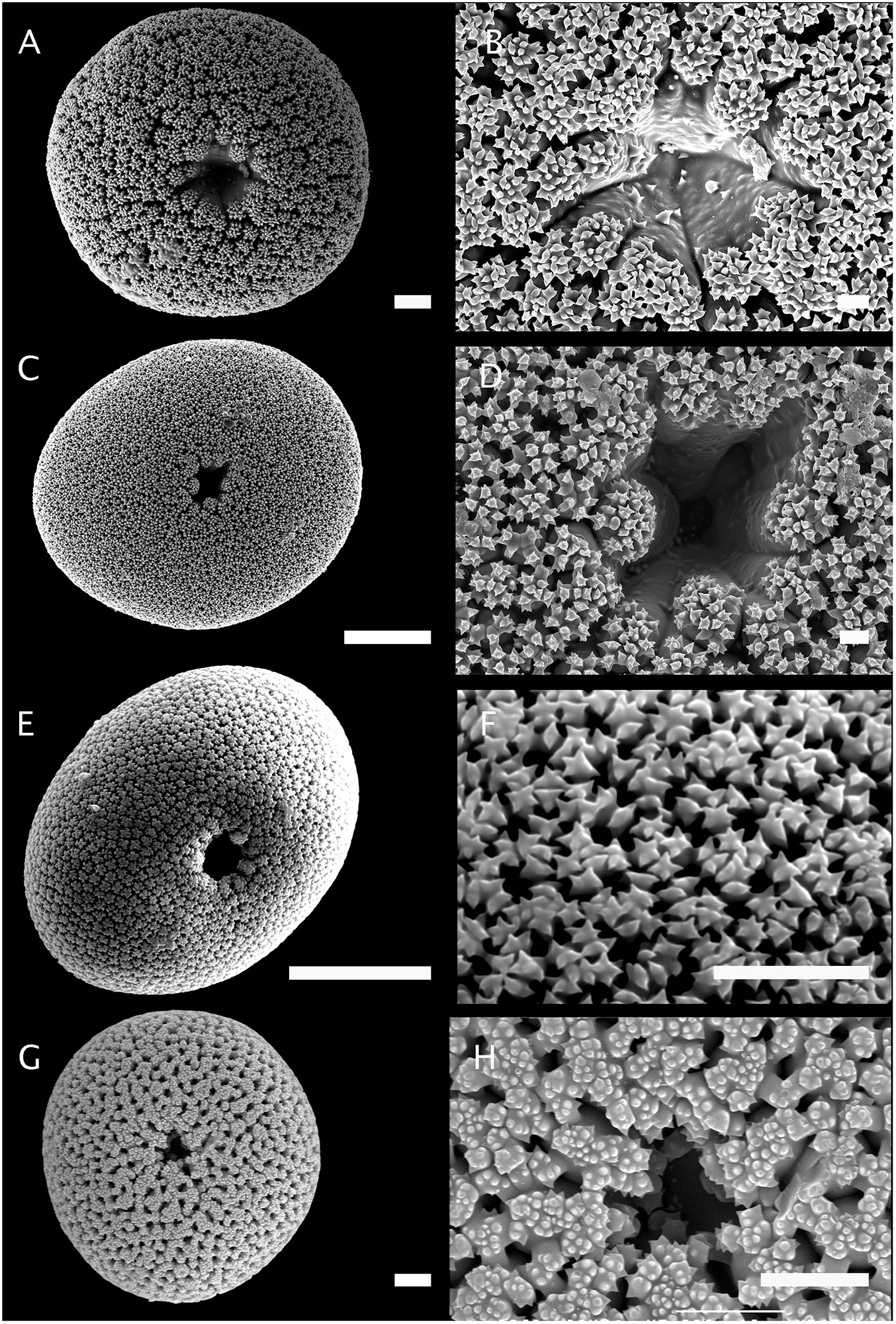
Figure 4. Morphology of sterrasters from Pachymatisma, Caminus, and Caminella. (A,B) Sterraster of Pachymatisma johnstonia (Roscoff, France, MNHN-DCL 4015). Scale A: 10 μm. Scale B. 3 μm. (C,D) Sterraster of Pachymatisma normani (Norway, ZMBN 77858). Scale C: 40 μm. Scale D. 2 μm. (E) Sterraster of Caminus chinensis (holotype, UPSZTY 2102). Scale E: 50 μm. (F) Sterraster of Caminus sp. (Îles Glorieuses, MNHN-IP-2015-1144). Scale F: 10 μm. (G,H) Sterraster of Caminella pustula (holotype, MNHN-IP-2008-4). Scale G: 10 μm. Scale H: 10 μm.
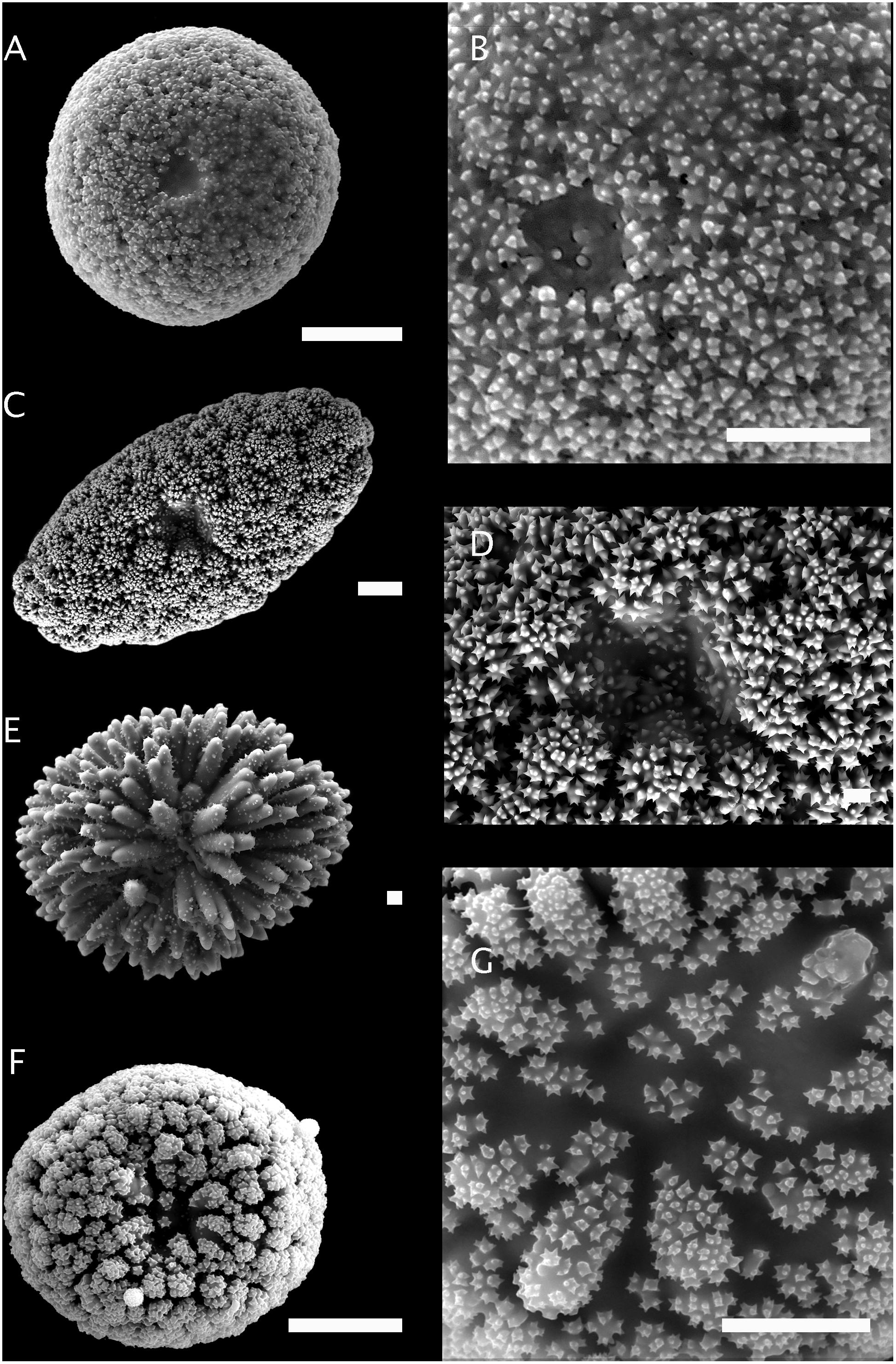
Figure 5. Morphology of sterrasters/aspidasters from Erylus and Caminus. (A,B) Sterraster of Erylus topsenti (South of the Azores, ZMAPOR 21657). Scale A: 38 μm. Scale B. 20 μm. (C,D) Aspidaster of Erylus mamillaris (Azores, ZMAPOR 20421). Scale C: 10 μm. Scale D. 2 μm. (E) Aspidaster of Erylus discophorus (Portugal, PC81). Scale: 2 μm. (F,G) Sterraster of Caminella vulcani (Canary Islands, ZMAPOR 20422). Scale F: 30 μm. Scale G: 15 μm.
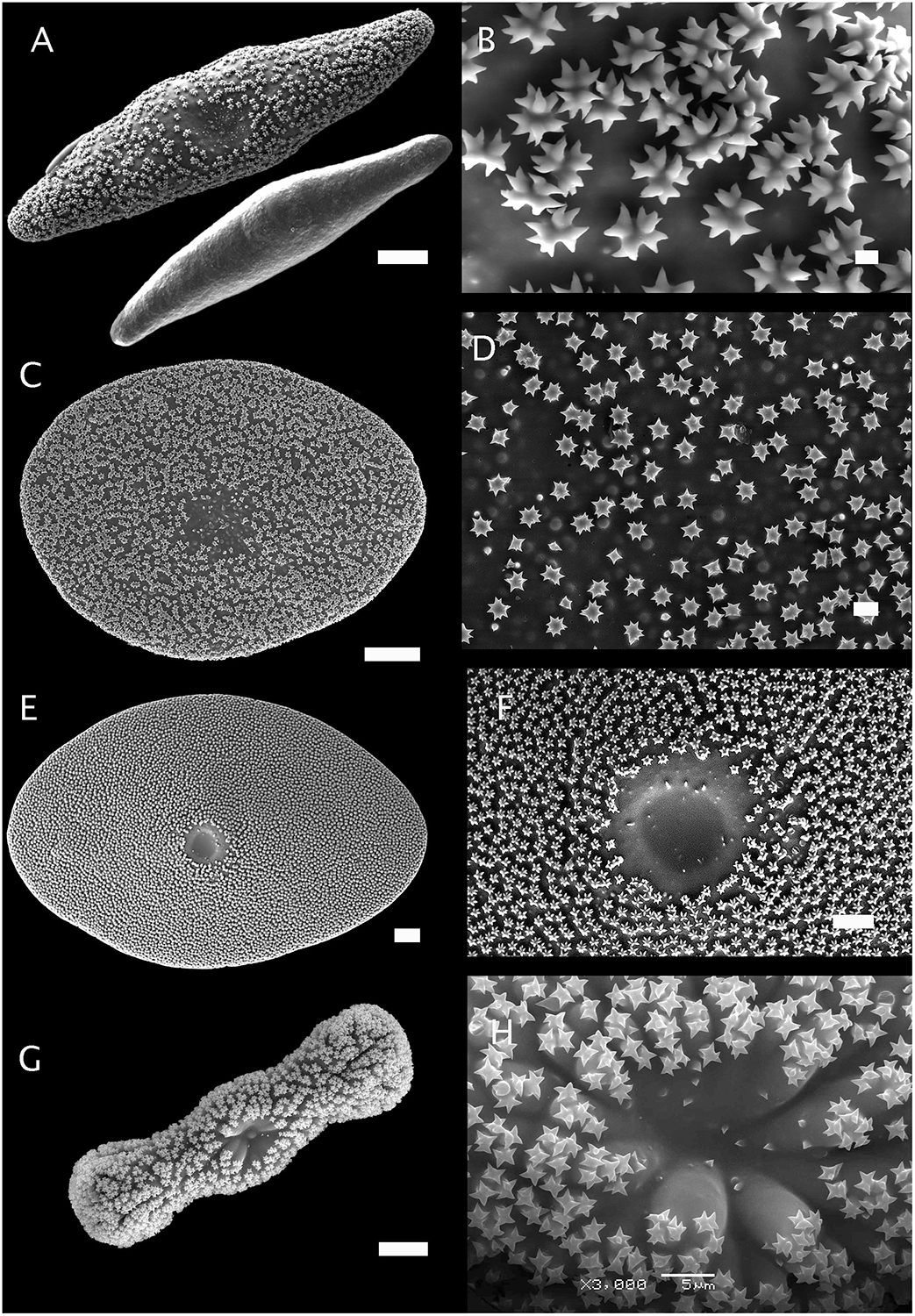
Figure 6. Morphology of aspidasters from Erylus and Penares. (A,B) Aspidaster of Erylus formosus (Bocas del Toro, Panama, ZMBN 81644) and its young smooth stage before the growth of rosettes. Scale A: 20 μm. Scale B. 1 μm. (C,D) Aspidaster of Penares euastrum, previously called Erylus euastrum (holotype, MNHN Schmidt collection#76). Scale C: 20 μm. Scale D. 2 μm. (E,F) Aspidaster of Erylus expletus (Rockall Bank, ZMAPOR 18142). Scale E: 20 μm. Scale F: 10 μm. (G,H) Aspidaster of Erylus trisphaerus (Campeche Bank, Mexico, CNPGG). Scale G: 20 μm. Scale H: 5 μm. Pictures: P. Gómez and D. Ugalde.
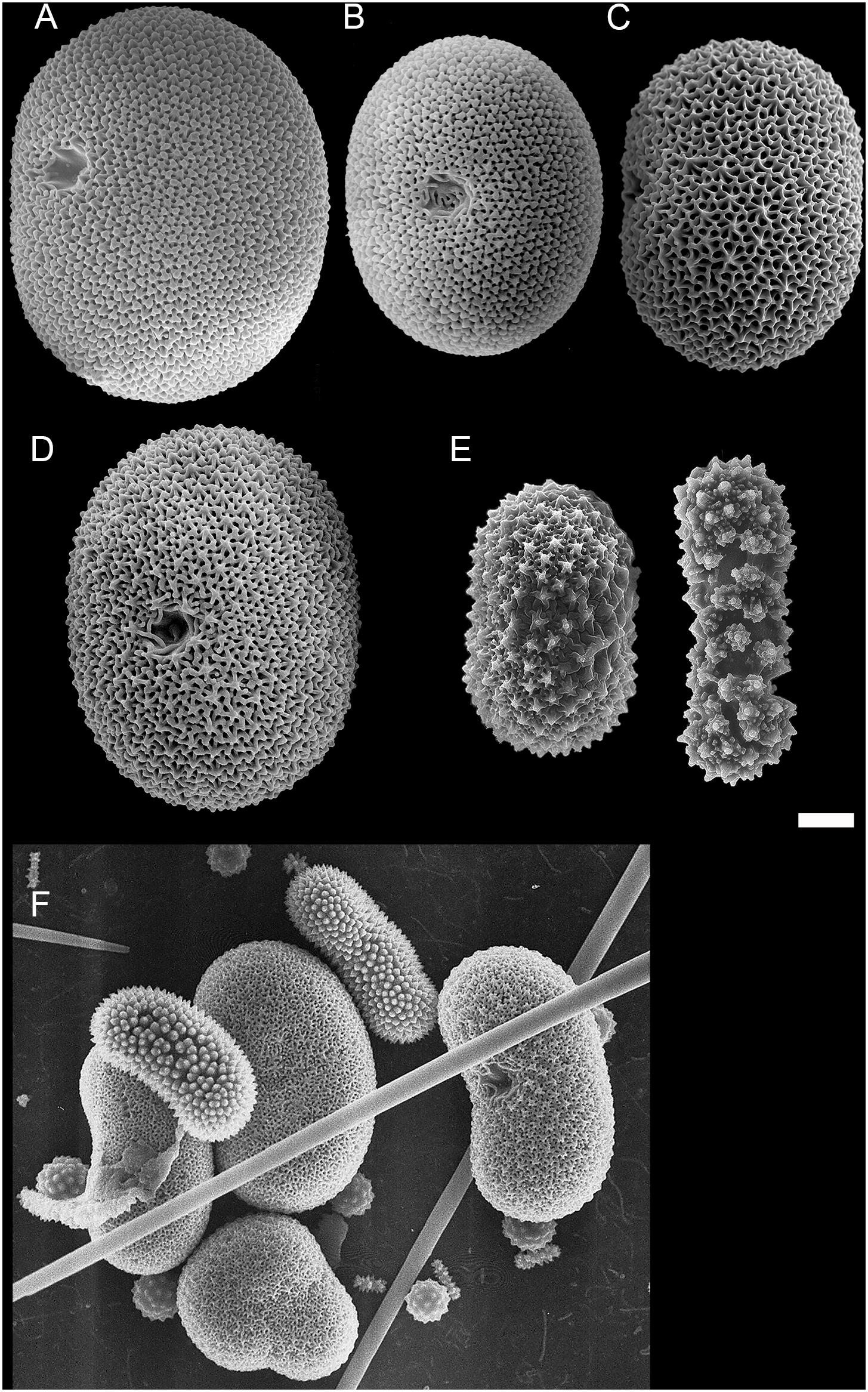
Figure 7. Morphology of selenasters from Placospongia. (A) Selenaster of Placospongia ruetzleri (holotype, RMNH 9872). Picture: R.W.M. van Soest. (B,C) Selenasters of Placospongia caribica (holotype, USNM 32873). Pictures: K. Rützler. (D). Selenaster of Placospongia melobesioides (Florida, USNM 39643). Picture: K. Rützler. (E) Selenasters of Placospongia sp. (Fernando de Noronha, Brasil, MNRJ 7878). Picture. F. Moraes. (F) Selenasters of Placospongia decorticans (Ras El Chakaa, cave, Lebanon, Endoume collection). This is a mix of late-stage and early-stage selenasters (smaller). Picture: S. Carteron. Scale A–D, F: 10 μm. Scale E: 50 μm.
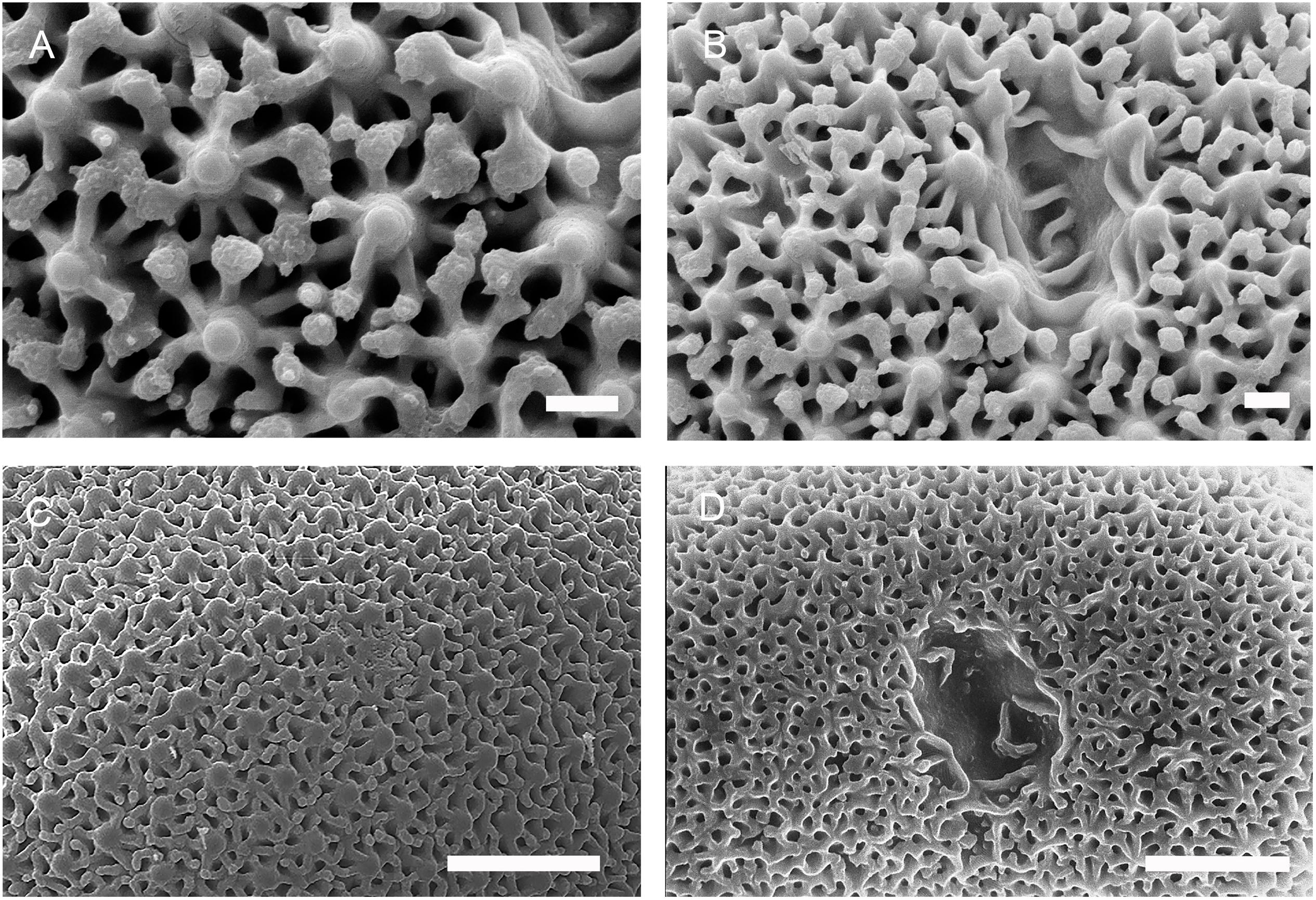
Figure 8. Morphology of selenaster surfaces from Placospongia. (A,B) Surface and hilum of selenaster, Placospongia melobesioides (Florida, USNM 39643). Note the typical polygonal pattern around a central knob. Scale: 2 μm. Picture: K. Rützler. (C) Surface of selenaster, Placospongia ruetzleri (holotype, RMNH 9872). Scale: 10 μm. Picture: R.W.M. van Soest. (D). Surface and hilum of selenaster, Placospongia decorticans (Ras El Chakaa, cave, Lebanon, Endoume collection). Scale: 10 μm. Picture: S. Carteron.
Hila size is 10–30 μm in diameter in the Geodiidae (Cárdenas and Rapp, 2013; Cárdenas et al., 2013; Figures 2– 6) and is 12–15 μm deep (this study) in sterrasters to very shallow in flat aspidasters. Selenaster hilum measurements are absent from the literature except for Placospongia ruetzleri, for which a diameter of 10–13 μm is reported (Van Soest, 2017). Selenaster hila measured from a specimen of Placospongia decorticans (Pointe Fauconnière, cave near Marseille, France, J. Vacelet collection, Endoume marine station) gave 5–10 μm in diameter and 5 μm deep. Moreover, selenaster hila measured on SEM plates from the literature (Becking, 2013; Rützler et al., 2014) gave dimensions of 4–10 μm. The hilum is therefore smaller and shallower in selenasters than in sterrasters. This incidentally suggests that nuclei in selenaster microsclerocytes are smaller than in sterraster microsclerocytes.
In terms of spicule size, selenasters are 30–100 μm in length (Becking, 2013). Geodia sterrasters are usually larger than 50 μm except for shallow water species which can have smaller sterraster sizes (25–50 μm) (da Silva, 2002). Furthermore, many Geodia have sterrasters that reach 100 μm in size, and beyond (da Silva, 2002; Cárdenas et al., 2013; Sim-Smith and Kelly, 2015): sterrasters of Geodia macandrewii are 124–360 μm in diameter (Cárdenas et al., 2013), Geodia australis sterrasters are 266–352 μm (da Silva and Mothes, 2000) while those of Geodia rex can reach 565 μm in length (Sim-Smith and Kelly, 2015). Sterrasters in the genus Erylus (50–300 μm in length) (Adams and Hooper, 2001; Sim-Smith and Kelly, 2015), Caminus (40–210 μm in diameter) (Shim and Sim, 2012; Van Soest et al., 2014), and Caminella (35–110 μm in diameter) (Cárdenas et al., 2018) have similar sizes than in Geodia. Despite the size overlap, it is noteworthy that sterrasters can be significantly larger than selenasters in some species.
With respect to shape, sterrasters and selenasters can have very similar subspherical shape but can also show differences, depending on the species. Sterrasters are spherical to subspherical (Figures 2–5), more rarely lozenge-shape (when viewed from the hilum side); aspidasters are oval to discoid, lemon- or lozenge-shape, to almost cylindrical (Figures 5, 6). Selenasters are never spherical but are commonly subspherical (e.g., in P. ruetzleri and Placospongia caribica, Figures 7A–C). Selenasters of P. decorticans are bent and elongate (sausage-like) (Figure 7F) while selenasters of Placospongia mixta, Placospongia melobesioides, and Placospongia carinata can have the typical reniform or bean shape (Becking, 2013) although they can also be subspherical without any obvious notch (Figure 7D). Finally, one noticeable difference is that selenasters can be colored, and may actually be responsible for the color of the species (Vosmaer and Vernhout, 1902); sterrasters are never colored.
A major difference between sterrasters and selenasters is their surface microornamentations. Selenasters have a polygonal surface, first mentioned by Hanitsch (1895, p. 215) and well illustrated by Lindgren (1898, p. 18). SEM observations of selenasters provided additional details on the surface microornamentations (Figure 8). The main knobs are 1 μm in diameter, and 2.5–5 μm away from each other (Figure 8A), depending on the species. Each knob was connected to 5–7 radial ridges, which form minor swellings when they meet from opposite sides. The main knobs and its radial ridges formed pentagons, hexagons or heptagons, 3–6 μm wide. Sterrasters from Geodia have characteristic surface rosettes, already mentioned by Sollas (1877). However, a comprehensive morphological comparison of these rosettes using the SEM revealed differences between the Geodiidae genera.
Surface Microornamentations, a New Character for Geodiidae Genera Assignation
In this study, only adult-stage sterrasters were considered since early-stage sterrasters are all fairly similar and look like polyaxial asters with no microornamentations. Figures 2–6 show the diversity of sterrasters within the Geodiidae, and especially the clear differences in surface microornamentations between genera. Four main types of surface microornamentations were singled out and listed in Table 3. (1) Geodia species have sterrasters with entirely fused actines at the tip of which radial sets of spines form the typical rosettes (Figures 2, 3). (2) Pachymatisma, Caminus and some Erylus (E. mamillaris, E. discophorus, E. granularis) have partly fused actines, the sides and tips of which are covered with numerous spines, often overlaying each other, and with no symmetry (i.e., difficult to distinguish clear separated rosettes) (Figures 4, 5). (3) Other Erylus/Penares (E. formosus, E. expletus, E. aleuticus, E. trisphaerus, E. topsenti, Penares euastrum) have partly or totally fused actines (with often an intermediate stage of entirely smooth aspidaster/sterraster) on which radial sets of spines make up clear single rosettes (Figure 6). (4) Finally, Caminella actines produce at their tips, small perpendicular bridges that reach toward the other actines, creating a complex fused network (Figure 4). These rosette morphology categories might be refined in the future to subdivide these groups even further but more SEM observations and sequenced species are necessary for this, especially amongst the Erylus species.
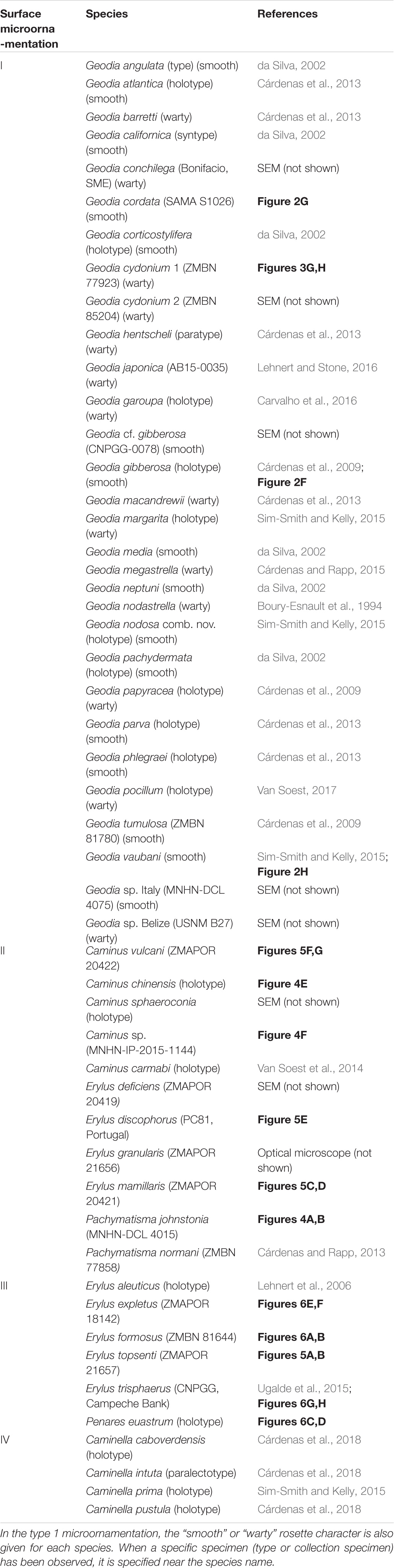
Table 3. Species list for the four types of surface microornamentations (within each type, species are in alphabetical order).
The phylogenetic distributions of these different categories of surface microornamentations match quite well the different Geodiidae clades, except in the Erylinae (Figure 9 and Supplementary Figure S1). Erylus is subdivided in at least two groups, with one group (Erylus discophorus complex and E. granularis) with surface microornamentations similar to those in Caminus and Pachymatisma than to the other Erylus. These Erylus species also share with Pachymatisma spiny microrhabds (vs. smooth microrhabds in other Erylus species). The polyphyly of Erylus has already been suggested based on molecular phylogenies and oscule morphology (Cárdenas et al., 2010). As in Cárdenas et al. (2011), the 28S (C1–D2) marker suggested a sister group relationship of Pachymatisma with the Erylus discophorus/granularis clade (Supplementary Figure S1), albeit unsupported (bootstrap of 48); the COI tree does not suggest that relationship (Figure 9). Overall, these results suggest that the flattening of sterrasters (aspidasters) happened independently at least twice in the Erylinae.
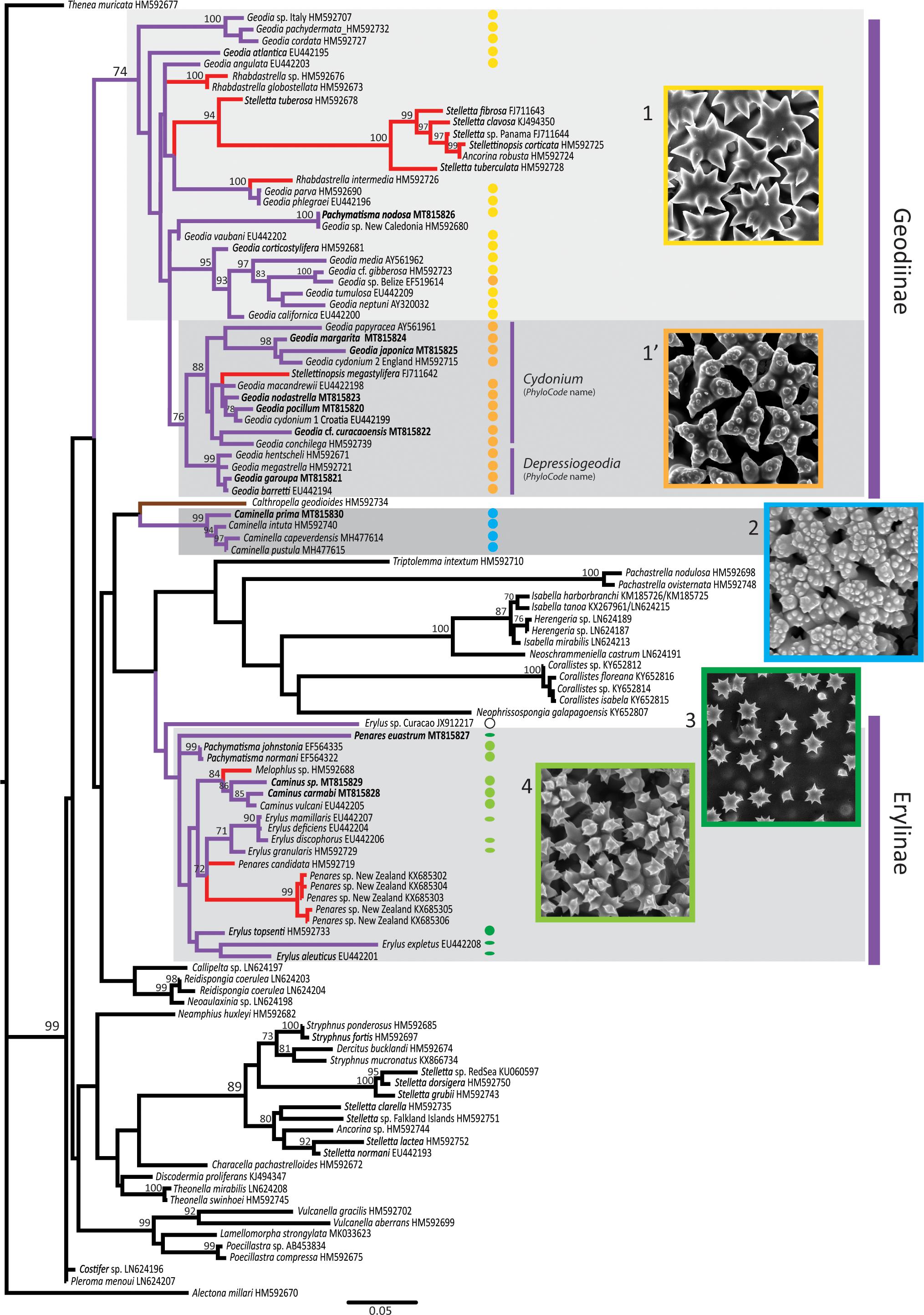
Figure 9. Astrophorina COI maximum likelihood (ML) tree reconstructed with RAxML. ML bootstrap supports (1,000 bootstrap replicates) > 70 are indicated. Genbank accession numbers are given after each taxon. In bold are new sequences produced in this study. Geodiidae species have purple branches, Calthropellidae have brown branches. Red branches indicate Geodiidae species which secondarily lost sterrasters (species previously considered to belong to the Ancorinidae). Colored dots indicate sequenced species/specimens for which previous authors or this study have observed the surface of sterrasters with SEM (see Table 3 for references); round shapes represent sterrasters, flattened shapes represent aspidasters. Image inserts illustrate the five types of sterraster surfaces observed (1. smooth rosettes, in Geodia atlantica; 1’. warty rosettes, in Geodia papyracea, holotype; 2. complex network, in Caminella pustula; 3. clear single rosettes in Penares euastrum, holotype; 4. unclear rosettes in Pachymatisma normani).
The COI and 28S phylogenetic results do not recover a monophyletic Geodiidae (Figure 9 and Supplementary Figure S1), although all the intermediate nodes between Geodiinae, Erylinae, and Caminella are not supported. Previous molecular trees show a poorly-supported Geodiidae clade (Cárdenas et al., 2011) or even fail to recover the monophyly of the Geodiidae (Redmond et al., 2013; Schuster et al., 2015; Kelly et al., 2019), with Caminella somewhat close to the Calthropellidae and the Erylinae (Cárdenas et al., 2018). Knowing how widespread spicule convergent evolution is in sponges (Cárdenas et al., 2011; Vargas et al., 2013; Schuster et al., 2015), the diversity of sterraster surfaces could represent at least three independent evolution of sterrasters—in the Geodiinae, in the Erylinae and in Caminella—thereby further questioning the monophyly of the Geodiidae. Each type of sterraster may have evolved from an astrophorin ancestor with euasters. After all, euasters themselves have appeared several times in demosponges: in Chondrilla, Tethyida, Stelligeridae, and even in some Poecilosclerida (Vacelet and Cárdenas, 2018).
As a proof of concept of the phylogenetic value of sterraster microornamentations, the phylogenetic position of two Geodiidae with doubtful genera allocations was tested. Cárdenas et al. (2018, p. 193) had already noticed that the sterraster microornamentations of Caminus primus (Sim-Smith and Kelly, 2015) from New Zealand suggested its transfer to the genus Caminella. Its well-supported phylogenetic position within the Caminella clade in our COI phylogenetic tree confirms this reallocation under the name Caminella prima (Figure 9). Likewise, the sterrasters of Pachymatisma nodosa (Sim-Smith and Kelly, 2015), from New Zealand have a typical Geodia rosette pattern, which hinted to a wrong genera assignment. The COI grouping of P. nodosa within the Geodia clade confirmed this hypothesis (Figure 9). Furthermore, after examination of slides made from the holotype (NIWA 53817) “microrhabds” as described by Sim-Smith and Kelly (2015) were observed, thereby confirming their close similarity to Pachymatisma microrhabds. However, the COI sequence of the holotype was identical to that of a deep-sea Geodia sp. (IRD-NC-R1820) from New Caledonia (Stylaster Bank) previously sequenced by Cárdenas et al. (2011), this specimen has mostly irregular strongylasters. Further work is needed to test the conspecificity of P. nodosa and our Geodia sp. but it revealed that the “microrhabds” of P. nodosa are in fact modified strongylasters, thus confirming its reallocation to Geodia. The name Geodia nodosa comb. nov. is formally proposed for this species. In a similar fashion, sterraster surface microornamentations could be useful to revise further poorly known Geodiidae species with uncertain genus allocations.
With their highly sophisticated morphology and microornamentations, sterrasters and/or selenasters may also be considered for future biotechnological or medical applications, as suggested for other types of biosilica material (Wysokowski et al., 2018). For instance, Kaya et al. (2020) propose to use partially dissolved sterrasters for bone tissue or controlled drug release applications, while Schoeppler et al. (2017) study sterrasters to understand the regularity of silica architecture in spicules.
Warty Rosettes Appearance in Geodia Evolution
Cárdenas et al. (2013, p. 50) previously recognized that sterrasters from Geodia species had either smooth (Figure 2) or warty rosettes (Figure 3) and suggested that this could potentially represent an informative phylogenetic character. This character was therefore carefully compiled from the literature and new SEM observations (Table 3) and mapped on the COI (Figure 9) and 28S trees (Supplementary Figure S1). Results showed that warty rosettes are restricted to two sister clades provisionally named Depressiogeodiap and Cydoniump by Cárdenas et al. (2011), following the rules of the PhyloCode3. The character of “warty rosettes” would therefore be a morphological synapomorphy for the Depressiogeodiap+Cydoniump clade, which always appeared monophyletic, albeit with low support (bootstrap of 53 for 28S and 76 for COI).
Again, as a proof of concept, the COI phylogenetic positions of several recently described Geodia species with warty rosettes were tested (Table 3): Geodia margarita, Geodia japonica, Geodia pocillum, Geodia garoupa, Geodia curacaoensis, and Geodia nodastrella. These species clustered with Depressiogeodiap (G. garoupa) or with Cydoniump (Geodia margarita, Geodia japonica, Geodia pocillum, Geodia garoupa, Geodia nodastrella, and Geodia curacaoensis) thereby validating the warty rosette as a synapomorphy of the Depressiogeodiap+Cydoniump clade.
A potential morphological synapomorphy of the Depressiogeodiap clade is the deep preoscule (Cárdenas et al., 2011, p. 9). Interestingly, there is no mention of deep preoscules in G. garoupa (Carvalho et al., 2016), although its COI had only a 2 bp. difference with the NW Atlantic haplotype of G. barretti (KC574389). A re-examination of the type material of G. garoupa should be done to see if the reported small oscules (∼0.5 mm) could be instead small preoscules; similar small preoscules are commonly observed in some G. barretti specimens (Cárdenas et al., 2013; Cárdenas and Moore, 2019). There is currently no morphological character supporting the Cydoniump clade.
One noticeable exception to the warty/smooth rosette pattern is the specimen Geodia sp. (USNM B27, South Reef, Belize) which has clear warty rosettes (this study, data not shown), although it groups within a smooth-rosette Geodia group and has an overall morphology fairly close to the Geodia gibberosa complex. No explanation for this exception can be put forward at this point and more specimens of that undescribed species are required to confirm this result. It, however, suggests that warty rosettes might have appeared independently in other Geodia clades. In the literature Geodia tenera (Sim-Smith and Kelly, 2015) also represents a unique case, with microspines instead of warts on its rosettes; this species should be further investigated in the future to see if it groups with the warty-rosette clade, as expected.
Fossilization of Sterrasters/Selenasters
The sterraster surface microornamentations could also be used in paleontology, to improve the taxonomic identification of fossil ball-shaped spicules. However, one needs to assess first how these microornamentations are transformed during diagenesis. Unfortunately, ball-shaped spicules (sterrasters and selenasters) tend to loose the surface microornamentations due to typical diagenetic processes of spicules: corrosion (= dissolution), substitution (silica is replaced by calcite, zeolite clinoptilolite, or pyrite) and recrystallization (the original silica, opaline silica, transforms into crystalline silica such as chalcedony) (Ba̧k et al., 2015). This makes it challenging to discriminate sterrasters from selenasters (Supplementary Table S1). As a consequence, sterraster/selenaster surface microornamentations are rarely preserved in Cenozoic fossils, very rarely in Cretaceous fossils and never (to this day) in Jurassic fossils (Supplementary Table S1). However, when sterrasters fossilize, they sometimes have a regular knobby surface when the rosette rays disappear, with each knob 3–5 μm in diameter (Rigby and Smith, 1992). When the whole rosette and knob disappear, the axial filament canals below each of them is revealed giving a regular porous surface, with pores 1–3 μm in diameter, separated by ∼2 μm, measurements made from SEM pictures (Bonci et al., 1997; Pisera et al., 2006; Vishnevskaya et al., 2009; Łukowiak, 2015). Finally, as the dissolution moves along and widens the axial canals and reaches the center of the sterraster, a central cavity often develops (Říha, 1987; Bonci et al., 1997; Frisone et al., 2014). These processes can happen rather fast, especially in modern tropical reef sediments (Rützler and Macintyre, 1978), typically alkaline and undersaturated in silica. The central cavity makes the sterraster fossils fragile and they can often break open: it is common to find pieces of fossilized sterrasters (e.g., Říha, 1987; Vishnevskaya et al., 2009). Since the actines dissolve or recrystallize more easily than the inter-actine silica (Rigby and Smith, 1992) the radial pattern of fossilized sterrasters is often retained, if the recrystallization is not too coarse. If the surface has been entirely dissolved, sterrasters can appear as spherical/subspherical smooth spicules (Łukowiak and Pisera, 2016) so even in recrystallized sterrasters, the overall spherical/subspherial shape is maintained. Likewise, sterrasters treated with different potassium hydroxide (KOH) (Sollas, 1877) or hydrofluoric acid (HF) treatments (Rützler and Macintyre, 1978; Kaya et al., 2020) show the same patterns as fossil sterrasters: surface covered with pores, 2–3 μm diameter (Kaya et al., 2020), and a central cavity.
When selenasters fossilize and as the pentoradiate surface pattern dissolves, several randomly placed “holes” appear and there is no central cavity or radial pattern, due to the absence of axial canals (Rützler and Macintyre, 1978; Łukowiak, 2015) and therefore, they do not break. Selenasters may also become more or less smooth, and retain their subspherical to bean shape.
This short review on the fossilization shows that the use of surface microornamentations as a taxonomic character is limited essentially to Cenozoic ball-shaped spicules and very rarely to Cretaceous ones. The oldest sterrasters (Vishnevskaya et al., 2009) and selenasters (Gruber and Reitner, 1991) with preserved surfaces both date from the Late Cretaceous (Santonian/Campanian) some 83 Mya. The Cretaceous sterrasters seem to have smooth rosettes but the resolution of the SEM pictures is too low to be entirely certain (Vishnevskaya et al., 2009, Plate II-1a). The smooth/warty rosette character can be observed as long as the rosettes are well preserved: clear warty rosettes are visible in fossilized sterrasters from the Early Miocene, 16 Mya (Říha, 1987).
When the surface microornamentations disappears, one could rely instead on mostly diagenetic structures mentioned earlier: sterrasters would have a regular porous surface, a radial pattern and/or a central cavity; selenasters have an irregular porous pattern without a radial pattern or central cavity. Applying this chain of thought, the paleontological record of ball-shaped spicules (Supplementary Table S1) was revised to build a timeline of Geodiidae and Placospongia (Figure 10), but also to revisit the nature of ball-shaped spicule fossils called rhaxes.
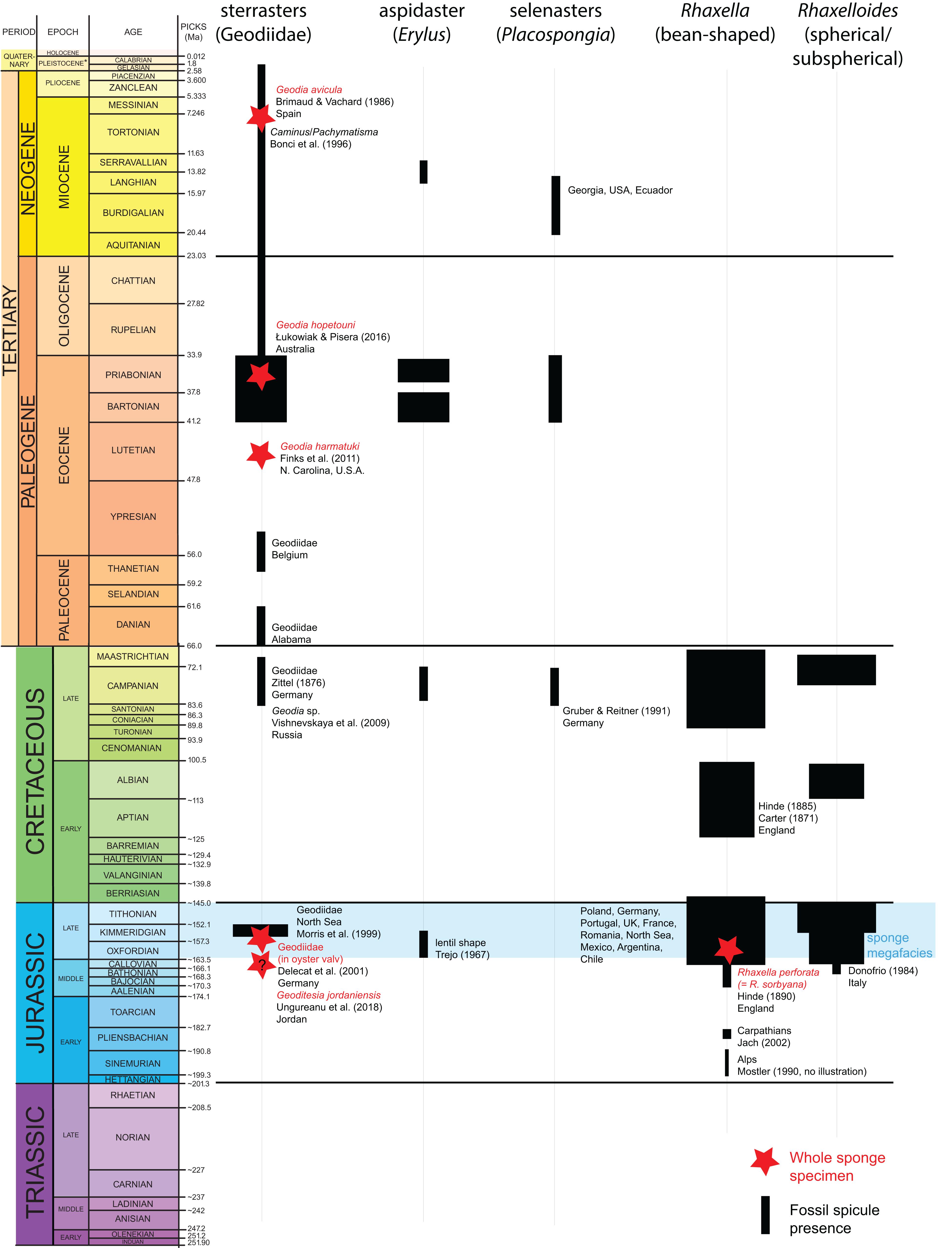
Figure 10. Timeframe of ball-shaped fossil spicules from the Trias to the Holocene. Red stars represent whole body fossils. Black lines represent presence of the respective fossils (sterraster, aspidaster, selenaster, rhax, or smooth sphere); the width of the black lines is indicative of the abundance of these fossils. For literature references used to build this timeframe, see Supplementary Table S1.
Rhaxes: Sterrasters or Selenasters? Revising the Evidence
Rhaxes are bean-to ball-shaped fossilized spicules, originally described from the Late Jurassic where they are very abundant; they are nearly equally common in the Late Cretaceous (Figure 10). After being mistaken for Foraminifera, they were considered as sterrasters, despite some acknowledged common characteristics with Placospongia (Hinde, 1890). Based on their bean shape and their presumed higher resistance, Rützler and Macintyre (1978) claim they are without a doubt etched selenasters. Following paleontologists mostly accepted this view (Wiedenmayer, 1994; Pisera, 1997; Łukowiak, 2015). However, taking advantage of this study on sterrasters, this issue is revisited here using illustrations from the literature combined with examination of the fossil Rhaxella perforata (now a junior synonym of R. sorbyana), type species of the genus Rhaxella (Figure 11); for a history of the taxonomy of Rhaxella see Trejo (1967).
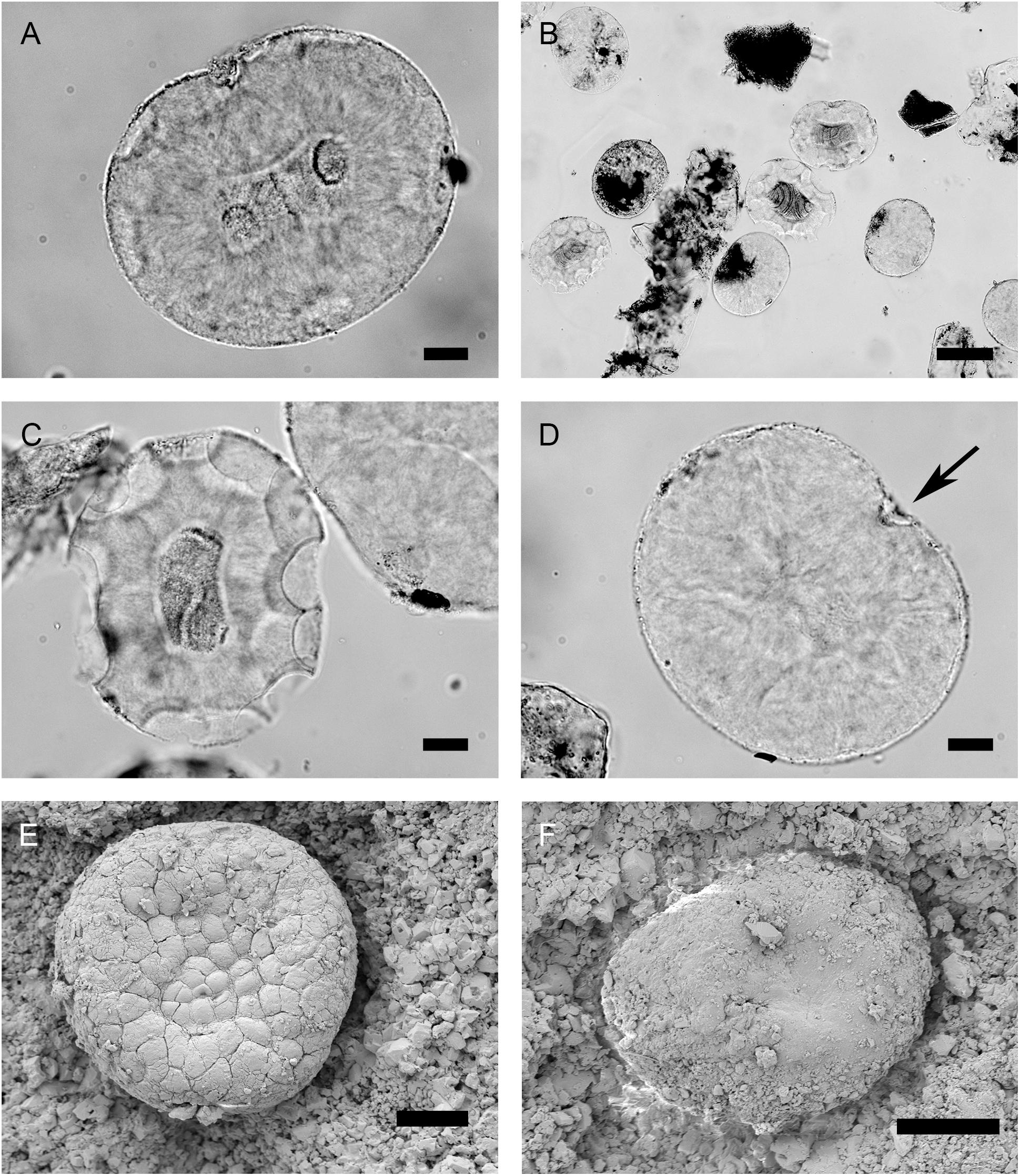
Figure 11. Rhaxes observed with an optical microscope, Rhaxella perforata (now a junior synonym of Rhaxella sorbyana) (NHMUK PI, slide S1682) from the type locality, Late Jurassic, Oxfordian (Lower Calcareous Grit Formation, Scarborough, North Yorkshire, England). (A) Rhax with typical inside double-dot pattern and fibrous chalcedony recrystallization, seen in transparency. Scale: 20 μm. (B) Rhaxes with oval to bean shapes. Scale: 100 μm. (C) Rhax with typical semicircular etching patterns, fibrous chalcedony forming a dark cavity and a radial pattern. Scale: 20 μm. (D) Bean-shaped rhax with a dent (black arrow), and an etching pattern in the dent. Scale: 20 μm. (E) Putative subspherical spicule on the surface of Geoditesia jordanensis (specimen S10–18), Callovian, Middle Jurassic. The secondary surface polygonal pattern is typical of fibrous chalcedony recrystallization. Scale: 20 μm. (F) Putative subspherical spicule on the surface of G. jordanensis (specimen S10–18). Scale: 20 μm.
Evidence 1: Size
Hinde (1890) mentions that rhaxes are 110–150 μm in size, the smallest 80 μm. Rhaxes from the NHMUK PI slide S1682 (Late Jurassic, Lower Calcareous Grit, type locality) were 129-147.9-166 × 107-120.4-124 μm (N = 25). In the literature (Supplementary Table S1), Jurassic rhaxes have size ranges (80–200 μm), within ranges of sterrasters and selenasters (Table 2). However, the upper range (> 120 μm) is more in accordance with that of Recent (25–590 μm) and Eocene (70–350 μm) sterrasters than Recent (30–100 μm) or Eocene (60–120 μm) selenasters (Supplementary Table S1). Then again, Jurassic spicules might have been larger than Recent or Eocene sponges. The very few Cretaceous unambiguous sterrasters (122–163 μm) and selenasters (120–150 μm) are within the range of rhaxes (Supplementary Table S1) including R. perforata measured here; interestingly, Cretaceous selenasters are bigger than any other known Recent selenasters (30–100 μm).
Evidence 2: Surface and Shape
Hinde (1890) mentions that rhaxes have 2 μm wide spots on the best-preserved specimens. Rhaxes with these spots were not observed on slide S1682 but they have been observed repeatedly by previous studies and explain why R. sorbyana was originally described as Foraminifera. Trejo (1967, plate II) confirms that rhaxes have pores about 2 μm wide and 2 μm apart. This pattern and sizes of pores fits with the above mentioned porous pattern observed on some fossilized as well as artificially dissolved sterrasters (∼2 μm spacing between pores). They could also, however, fit with the selenaster knobby pattern: in recent selenasters knobs are spaced 2.5–5 μm from each other, and 2.5–3 μm from each other in Cretaceous ones (our measurements on SEM picture from Gruber and Reitner, 1991). Hinde (1890) also observes rhaxes which have lost semicircular portions; these etching patterns were fairly common on slide S1682 (Figure 11C) but also on Cenozoic Rhaxella chert sections (S7502).
One source of confusion on the nature of rhaxes is linked to the nature of the notch in the bean-shaped spicules. Hinde (1890) miscalls the notch a “hilum,” which was followed by some subsequent authors (Haslett, 1992; Kozur et al., 1996) while most following authors have considered this notch to derive instead from the bent of selenasters. In that case, one should potentially be able to see a selenaster hilum in the best-preserved rhaxes, but neither previous studies, nor ours were able to find hila on rhaxes, even on the ones with the preserved regular surface spots. This absence of hilum could be due to dissolution of the surface and/or recrystallization, but there is yet another possibility that the spiculogenesis of Jurassic rhaxes took place in larger cells (with no need for the spicule to grow pressed against the nucleus).
Finally, it should be emphasized that the shape of rhaxes also varies from “ellipsoid to subspherical” (Hinde, 1890) so they are not all bean shaped. This is also the case in Recent Placospongia species where selenasters can be more or less subspherical and bean-shape (Figure 7). Importantly, sterrasters are never bean-shaped, even after erosion or artificial dissolution of spicules (Sollas, 1877; Rützler and Macintyre, 1978).
Evidence 3: Inside Structure
Hinde (1890) mentions that some rhaxes have a central recrystallized cavity with fibrous chalcedony: it appears dark and banded, it can have a slightly bent shape sometimes (Figures 11A–D). Usually, a clear radial pattern is visible around this cavity (Figures 11A,C), which is probably indicative of fibrous quartz recrystallization, typical in fossilized spicules (Ba̧k et al., 2015). In the literature, the dark center and clear fibrous corona are recurrent characteristics of rhaxes from the Late Jurassic (e.g., Gramann, 1962; Supplementary Table S1). This secondary silicification means that it is unfortunately not possible to know the original inside structure of rhaxes (radial vs. non-radial).
Evidence 4: Associated Megascleres and Whole Sponge Fossils
Hinde (1890) already questioned the total absence of megascleres in the whole sponge R. perforata: one would expect associated triaenes if this is a Geodiidae, tylostyles if this is a Placospongiidae. Some argued that megascleres could have been dissolved more easily than the more resistant ball-shaped spicules, others that megascleres were simply absent in R. perforata (Hinde, 1890). Only two whole sponge fossils from the Jurassic actually associated triaenes with ball-shaped spicules (Figure 10; Delecat et al., 2001; Ungureanu et al., 2018), thereby suggesting they are part of the Geodiidae. Surprisingly, the sterrasters reported are said to be bean-shaped. Delecat et al. (2001) describe a whole sponge fossil from the Late Jurassic, living in reef-building oysters. This sponge associates large calthrops, occasional small triaenes, oxeas and rhaxes; the calthrops and triaenes leave no doubt that this is a Tetractinellida. Furthermore, Delecat et al. (2001) examined the ball-shaped spicules in thin sections so that a sterraster cut transversally in the hilum will appear bean-shaped in section. The “notch” in those rhaxes is 10 μm wide (Delecat et al., 2001) fitting with the hilum size of a sterraster. Therefore, this fossil is a credible Late Jurassic Geodiidae, although no Recent genera has calthrops associated with triaenes; this fossil cannot currently fit into any known genus. Ungureanu et al. (2018) also state that Geoditesia jordanensis from the Middle Jurassic has fairly large “reniform sterrasters” (140–330 μm). However, the spicules observed with SEM were not clearly bean shaped (Figures 11E,F) and were smaller (∼66–88 μm) than the sizes reported. Their surfaces were smooth, so that it was impossible to decide if they were sterrasters or selenasters (Figures 11E,F). The nature of these ball-shaped spicules is uncertain, and so is the nature of this fossil (see section “Discussion”).
Evidence 5: Paleogeography and the Abundance of Rhaxes
Late Jurassic rhaxes have a wide distribution, from Argentina, Chile, Mexico, Europe (= Tethys Ocean at the time) to Japan (Supplementary Table S1). During the Late Jurassic, all of these locations were in the temperate zone (Argentina, Central Chile, Japan), tropical/temperate zone (Europe) and tropical zone (Mexico) (Paleomap Maker4, accessed 3rd of September 2020). Late Jurassic rhax records may come from shallow-water paleoenvironments, but also from relatively deep-sea environments, as in the case of the “sponge megafacies” from the Tethys Ocean, considered to be ∼100–600 m depth (Pisera, 1997; Supplementary Table S1). At these locations, the large dominance of rhaxes, often representing from 49.7 (Reif, 1967), to 75% (Pisera, 1997) or even 80–90% (Afşar et al., 2014) of all loose spicules is striking. These amounts make rhaxes rock-forming fossils leading to cherts and rhax spiculitic sandstones. Three hypothesis could explain these amounts: either (i) these species were dominant, (ii) these spicules were more resistant than others and accumulated in the sediments or (iii) these spicules were sorted based on size and morphology during transportation.
Selenasters are very numerous in the cortex of Placospongia. This genus includes today only a dozen of species, and most are restricted to very shallow tropical waters, in reefs, marine lakes, caves, and anchialine pools (Becking, 2013); only one species, Placospongia anthosigma (Tanita and Hoshino, 1989) was recorded from slightly deeper waters (62–67 m), in Sagami Bay, Japan. Recent Placospongia species never dominate their habitats or form large aggregations.
Sterrasters are also usually very abundant spicules in Geodiidae, packed in the cortex in the numbers of thousands (Cárdenas et al., 2013). More than 340 species Geodiidae species are found worldwide (except in Antarctica), in shallow and deep waters, up to 3,100 m depth (Cárdenas and Rapp, 2015). Today, large quantities of sterrasters could accumulate in sponge grounds dominated by massive Geodia species. Indeed, some North Atlantic Geodia species are dominant species in deep-sea boreo-arctic sponge grounds, on continental shelves, slopes, and seamounts (Klitgaard and Tendal, 2004; Murillo et al., 2012; McIntyre et al., 2016). Sterrasters and triaenes from decaying sponges are among the few spicules that remain in deep-sea spicule mats from these boreo-arctic sponge grounds (Wagoner et al., 1989; Murillo et al., 2016). Massive Geodia cydonium aggregate in shallow waters of the Western Mediterranean Sea (Mercurio et al., 2006; Grech et al., 2020). Based on modern ecosystems, Geodia species are therefore more likely to contribute to large accumulations of ball-shaped spicules. To support this, sterrasters are by far the dominant spicules, over selenasters in the Middle Eocene of NE Italy, a rocky temperate shore at the time (Frisone et al., 2014). In modern tropical shallow-water reef sediments, sterrasters, and selenasters are usually overrepresented: they represent nearly half of all spicules although they represent only a minor portion of the living biomass (Łukowiak et al., 2013). One reason may be that they are amongst the most resistant microscleres (Rützler and Macintyre, 1978) and, as mentioned earlier, they are produced in abundance. To sum up, the abundance of rhaxes in the Late Jurassic may indeed reflect the abundance of Geodiidae and/or Placospongia (the former being more likely), and a bias of better preservation. Whether sorting of these spicules during transportation could explain their accumulation remains to be proven, in modern shallow-water reef sediments for instance. Modern studies in sediments also raise the possibility that rhaxes could be a mix of ball-shaped spicules.
Rhaxes, a Mix of Sterrasters and Selenasters
Since Recent sterrasters are never bean-shaped, shape alone is a strong argument in favor of rhaxes being connected to selenasters; the surface and inside structure cannot confirm this because of substitutions or recrystallizations. Such a conclusion implies that some Placospongia ancestors were common in much deeper waters than today since rhaxes were fairly abundant in the Tethys Ocean at important depths (sponge megafacies, ∼100–600 m depth). In any case, more work on the taphonomy and diagenesis of these spicule fossils is necessary to fully understand their nature.
The recurrent wide range of sizes (80–200 μm) given for rhaxes (Supplementary Table S1) as well as the diversity of morphology sometimes described (spherical to subspherical, to bean-shaped) suggest that many of the reported rhaxes or rhax spiculites probably originate from mixes of selenasters and sterrasters, as observed in Recent shallow-water tropical sediments. It is also possible that the terms “rhax” or “bean-shape” have been overused, without a proper definition due to the parataxonomic status of “rhax,” as a loose spicule, without being really representative of a species. Some researchers use it for oval shapes (e.g., de Geyter and Willems, 1982) or for just any ball-shaped spicules, especially when only thin sections of rocks were studied, and without realizing that sterrasters could potentially appear bean shape in section, as illustrated in Delecat et al. (2001). Consequently, rhaxes are possibly overrepresented in the literature thus giving the impression that selenasters were more common than they really were in the Late Jurassic, although sterrasters could have been more abundant.
Actually, many studies have shown that one can distinguish in the Late Jurassic different ball-shaped spicules with similar sizes: rhaxes sensu stricto ( = bean-shaped), spherasters and what some paleontologists call sterrasters (spherical spicule) (Supplementary Table S1). Since Recent selenasters are never spherical, spherasters and other spherical spicules are credible sterraster ancestors. In addition to R. perforata and its typical bean-shape spicules, Trejo (1967) and Haslett (1992) have actually started to describe different rhax morphologies giving them other parataxonomic names, in order to distinguish different potential species. This is Rhaxelloides sphaerica (Trejo, 1967) and Rhaxelloides cilindricas (Trejo, 1967), Rhaxella elongata (Haslett, 1992) and Rhaxella winspitensis (Haslett, 1992). According to the overall shape of selenasters and sterrasters, R. sphaerica and R. cilindricas would be sterrasters while R. elongata and R. winspitensis would be selenasters. In accordance, the genus Rhaxelloides should be allocated to the Geodiidae, this genus should be used to describe single spherical/subspherical to oval/cylindrical spicules which have lost their surface microornamentations. Rhaxella should be used for bean-shaped fossils which have also lost their surface microornamentations (putative selenasters). R. elongata looks very much like the selenasters of P. decorticans (Figure 7E) while R. winspitensis cannot be linked to a modern selenaster at this point.
Conversely, some sterraster fossils described under different genera names are not any different from those of modern Geodia. Silicosphaera asteroderma (Hughes, 1985) (Miocene-Recent) are loose sterrasters from the Sedili River mud (West Malaysia), with clear rosettes. Therefore, Silicosphaera (Hughes, 1985) becomes a junior synonym of Geodia (Lamarck, 1815). Conciliaspongia rarus (Robinson and Haslett, 1995) from the Miocene of Ecuador is clearly a sterraster with rosettes therefore it is formally proposed here that Conciliaspongia (Robinson and Haslett, 1995) becomes a junior synonym of Geodia (Lamarck, 1815). The Late Miocene-Pliocene Hataina ovata (Huang, 1967) from Japan previously described as a radiolarian has been shown to be sterrasters (Inoue and Iwasaki, 1975), with no formal taxonomical act taken. Since the sterraster surface of H. ovata clearly has “flower like ornamentation” (Inoue and Iwasaki, 1975), in other words “rosettes,” it is formally proposed here that Hataina (Huang, 1967) becomes a junior synonym of Geodia (Lamarck, 1815). Silicosphaera asteroderma and Hataina ovata were described under the false premise that these sterrasters could suffice for the description of the total organism. Therefore, the descriptions given are insufficient to differentiate these species from other species of Geodia. Conciliaspongia rarus was described as a loose spicule but again, its description and its characters alone do not permit an unambiguous identification. A revision of the type material would not help either because in general, the description of a sterraster cannot be enough to describe a new species of Geodia, which has several other types of spicules. According to requirement 13.1.1 of the International Code of Zoological Nomenclature (International Commission on Zoological Nomenclature [ICZN], 1999), names published after 1930 must “be accompanied by a description or definition that states in words characters that are purported to differentiate the taxon.” Therefore, S. asteroderma, C. rarus, and H. ovata species become unavailable names.
Geoditesia and Geodiopsis Fossils Are Tetractinellida Incertae sedis
The Cretaceous genus Geoditesia (previously Geodites) was founded on arbitrary groupings of loose triaenes and bean-shaped spicules found in the same fine sand layer: Upper Greensand of Albian age in Devon, England (Carter, 1871). Because of this, they have traditionally been placed, albeit with doubt, in the Geodiidae (Rigby, 2004). Slides of type and non-type material of Geodites species have been re-examined in this study, including from the type species of the genus (Geodites haldonensis), a species defined by an arbitrary association of protriaenes, prodichotriaenes, anatriaenes and ball-shaped spicules. Following authors were more cautious in their groupings (e.g., Geodites cretacea Sollas, 1880a) or took the more reasonable approach of defined the species by only one type of characteristic triaene (Hinde, 1885, 1888; Weller, 1930). As noted previously (Finks et al., 2011, p. 70), Geodites triaenes are similar to what can be today observed in several families of Tetractinellida: Geodiidae, Ancorinidae, Tetillidae or even lithistids (Corallistidae, Pleromidae, Phymatellidae, Isoraphiniidae). It makes this genus probably polyphyletic, and used for any loose triaenes; this explains the very wide stratigraphic range of this genus with species described from the Carboniferous (Hinde, 1888; Weller, 1930) to the Late Eocene (Hinde and Holmes, 1892). There is no evidence that the rhaxes included in G. haldonensis actually belong to G. haldonensis, and according to our previous conclusions they are more probably selenasters. For these reasons, Geoditesia should formally leave the Geodiidae and be moved to Tetractinellida incertae sedis. Furthermore, since some of these species may be mixes of spicules coming from different species, and to avoid any future confusion a full revision of the genus is necessary to eventually restrict Geoditesia species definitions to their main triaene, a task beyond the scope of this study.
The first claimed whole specimen of Geoditesia is Geoditesia jordanensis from the Middle Jurassic (Callovian), two specimens of which have been examined in the present study. After sectioning of these subspherical 2 cm fossils, the absence of internal structure was confirmed (Ungureanu et al., 2018); just a few oxeas with no particular orientation in a homogeneous matrix; no sterraster cortex could be observed either; at the surface, a disorganized assemblage of oxeas, dichotriaenes and ball-shaped spicules. The absence of internal structure is troubling since this non-lithistid sponge must have been buried quickly to retain its original shape and in that case, some sort of skeleton organization would have been retained. It also contrasts with the preservation of delicate details such as the so-called “osculum,” a round structure that was also observed on one of our specimens. This calls into question the whole body nature of these specimens, which would require a full and in-depth revision of the type series to understand their taphonomy. Furthermore, the presence of subspherical spicules on the surface of G. jordanensis does not prove their belonging to the fossil either: these microscleres are sometime so abundant that they cover all sponge fossils, and have often confused paleontologists (e.g., Zittel, 1876; Hurcewicz, 1966). Unfortunately, the surface of these ball-shaped spicules had disappeared, and their subspherical shape was also unclear (Figures 11E,F). To conclude, the nature and identification of this species remains questionable; its Geodiidae affiliation cannot be confirmed.
Geodiopsis was created for sponge whole fossils from the Campanian (Late Cretaceous) associating oxeas, chubby triaenes and anatriaenes. Although he also finds sterrasters, Schrammen (1910, p. 116) stresses that he cannot associate them with confidence to the Geodiopsis specimens. For this reason, the current classification of Geodiopsis in the Geodiidae remains doubtful, the combination of triaenes and anatriaenes could also be found in some Ancorinidae genera (Stelletta, Ancorina, Ecionemia, Stellettinopsis, Stryphnus) or even Tetillidae. For these reasons, Geodiopsis should be transferred from the Geodiidae to the Tetractinellida incertae sedis.
As for the Cretaceous Pachymatisma virga (Hinde, 1885), it is based only on loose strongyles, which could belong to many different Heteroscleromorpha genera; there is no evidence that this species belongs to Pachymatisma and the description is insufficient to identify this species. According to the article 13.1.1. (International Commission on Zoological Nomenclature [ICZN], 1999) cited previously, the species name P. virga becomes an unavailable name.
Timing the Emergence of the Geodiidae, Geodia, and Placospongia
Geodiidae species belong to the Tetractinellida order, defined by the presence of triaene megascleres. The Tetractinellida order is deeply rooted in the Paleozoic with isolated triaene-like fossils already reported from the Middle Cambrian, Georgina Basin, in Australia (van Kempen, 1990; Mehl, 1998) and later sparsely found throughout the Paleozoic periods. Just like the Paleozoic lithistid desmas or microscleres such as toxas and forceps (Mostler, 1996), it is difficult to link these Paleozoic triaenes to the Mesozoic ones which appear only in the Middle Trias (Upper Anisian, ∼242 Mya), and are at this stage already very diverse (Mostler, 1976). According to a time-calibrated molecular phylogeny, the crown-group Tetractinellida would have appeared in the Early Jurassic (Plese et al., 2020). However, (i) the divergence estimate range for this node is between the Early Trias and the Late Jurassic and (ii) Plese et al. (2020) are missing in their calibrated phylogeny deep-diverging groups such as rhizomorine lithistids or Stupenda (Kelly and Cárdenas, 2016) which may push back in time this average divergence date. All in all, an emergence of crown-group tetractinellids around the Middle Trias is plausible, and means that Geodiidae must have appeared later. Importantly, the crown-group Clionaida order possibly appeared around Late Trias (Plese et al., 2020) meaning that the Placospongiidae diverged later.
Accordingly, the three Paleozoic reports of sterrasters/rhaxes (Supplementary Table S1) are currently not convincing. The Cambrian putative “sterraster” fossils are poorly illustrated and studied (Gruber and Reitner, 1991; Reitner and Mehl, 1995); they are entirely recrystallized so that their original structure/surface is unknown. The report of a single rhax from the Early Ordovician is doubtful since it is more than 1 mm large (Kozur et al., 1996), a size far larger than any of the recent sterrasters/selenasters. As for the two rhaxes found by Hinde (1888) in the Carboniferous, Wiedenmayer (1994, p. 31) suggests they are petrosiid microstrongyles (Haplosclerida order).
Two reports of rhaxes in the Early Jurassic (Mostler, 1990; Jach, 2002) suggest that the Placospongiidae were already present then. In the Middle Jurassic, Rhaxella and Rhaxelloides are more present: Rhaxella have been reported from the Bajocian (Haslett, 1992), Rhaxella and spherasters in the Bathonian (Donofrio, 1984), while Rhaxella and/or Rhaxelloides are already fairly abundant in the Upper Callovian (Trejo, 1967; Block Vagle et al., 1994). In the Late Jurassic (Oxfordian, Kimmeridgian and Tithonian), Rhaxella/Rhaxelloides are abundant and widespread in the sponge megafacies. In this study, the first sterrasters from the Kimmeridgian were formally identified in the North Sea where they were reported as “Praeconocaryomma (?) sp.2” radiolarians (Morris et al., 1999, Figure 9k); the rosettes are gone but these spicules look very similar to the fossil sterrasters described by Rigby and Smith (1992). Putative aspidasters are reported from the Late Jurassic in Mexico, although not much information is given (Trejo, 1967). The first formally identified Geodia sterraster from the Santonian/Campanian boundary, Late Cretaceous were identified in a study by Vishnevskaya et al. (2009, plates II and III) thanks to a clear rosette SEM illustration. Later in the Campanian, sterrasters are fairly common (Zittel, 1876; Schrammen, 1924). At the same time, the first formally identified Placospongia selenaster is reported from the Campanian, Late Cretaceous, misidentified as a sterraster in the article (Gruber and Reitner, 1991). To conclude, the fossil record suggests the emergence of Placospongiidae in the Early Jurassic, followed by the emergence of Geodiidae in the Middle Jurassic. The time-calibrated phylogeny suggests a younger emergence since Plese et al. (2020) suggest the Geodiidae stem-group appear in the Albian, Early Cretaceous (112 Mya), although with a divergence estimate range between the Middle Jurassic (174 Mya) and the Paleocene (61 Mya). The appearance of the Geodiidae crown-group would be in the Santonian, Late Cretaceous, around 85 Mya (Plese et al., 2020) with a range from the Barremian, Early Cretaceous (128 Mya) to the Oligocene (24 Mya). This is well in accordance with the first identification of typical Geodia sterrasters, from the Santonian/Campanian boundary and their following abundance in the Campanian. No whole specimens are known from this period unfortunately; the Lower Campanian Geodia sp. described by Hurcewicz (1966, p. 25) is likely a result of sterraster contamination (Wiedenmayer, 1994, p. 32) and is probably specimens of Stelletta (Stolleya) (E. Swierczewska Gladysz, pers. comm.).
While reviewing the paleontological literature, the oldest warty rosettes were observed in fossilized sterrasters from the Early Miocene, 16 Mya (Říha, 1987) so the Depressiogeodiap+Cydoniump clade had probably diverged by then. Future observations of fossilized sterraster should pay close attention to the morphology of rosettes in order to better determine the timing of divergence of this clade. No Caminella sterrasters were found in the paleontological literature, but an SEM picture of a Pachymatisma or Caminus sterraster was identified, from the Late Miocene in Italy (Bonci et al., 1997, plate 5, Figure 1); without other microscleres one cannot discriminate both genera. This is to our knowledge the first fossil ever reported for this group of Geodiidae.
Estimating the origin of the Geodiidae in the Middle Jurassic is relevant with respect to the “sponge biomarker hypothesis” whereby unique unconventional sterols found in some Recent Geodia and Rhabdastrella species (both part of the Geodiinae), were connected to the sterane biomarker 26-methylstigmastane (26-mes) from a sequence ∼100 My long of Neoproterozoic-Cambrian rocks and oils (Zumberge et al., 2018). The fact that the Geodiidae diverged some 500 My later than the 26-mes records suggests either that (i) these unique sterols appeared independently in the Geodiidae (by convergent evolution) or that (ii) the capacity to make these sterols was inherited from demosponge ancestors since the Neoproterozoic, and secondarily lost in most groups. This second scenario is currently supported by the presence of 26-mes precursors in other demosponge orders than the Tetractinellida (Geodia/Rhabdastrella): they are found in large amounts in Haplosclerida [Petrosia (Strongylophora) sp.] and in trace amounts in the orders Agelasida (Cymbaxinella corrugata) and Verongiida (Aplysina spp.) (Bortolotto et al., 1978; Zumberge et al., 2018). Further screening of demosponges for 26-mes precursors in parallel with a revision of their fossil record is required to better evaluate this second scenario.
Conclusion
In this study, a new spicule morphological character was explored: the surface microornamentations of sterraster spicules. Collagen fiber-rosette connections may give the cortex its solidity and flexibility, an attachment structure with biomimetic potential deserving to be further studied. Four types of microornamentations were revealed which, for some of them, matched specific Geodiidae clades: type I characteristic of Geodia, type II characteristic of Pachymatisma, Caminus and some Erylus; type III characteristic of other Erylus; type IV characteristic of Caminella. Within the type I microornamentations of Geodia, sterrasters had smooth or warty rosettes; all species with warty rosettes grouped in the “Depressiogeodiap+Cydoniump” clade. Therefore, the surface microstrucure character alone could be used to identify isolated Recent or fossil sterrasters as belonging to Geodia (smooth), Geodia (warty), Caminella, “Pachymatisma/Caminus//E. granularis/Erylus discophorus complex” or the rest of the Erylus.
Using this character, this study was able to sort uncertain genus allocations of some modern Geodiidae, and formally identify Geodiidae sterrasters from the Late Jurassic, Geodia sterrasters from the Late Cretaceous, as well as for the first time a Pachymatisma/Caminus sterraster from the Late Miocene. Evidence from the literature review suggests that the Geodiidae originated in the Middle Jurassic, after the Placospongiidae for which we have some evidence in the Early Jurassic. So-called “rhaxes” probably represent mixes of sterrasters and selenasters: while Rhaxella seems to belong to the Placospongiidae, Rhaxelloides belongs to the Geodiidae. Miocene-Pliocene fossils sterrasters Hataina, Silicosphaera, and Conciliaspongia become junior synonyms of Geodia. Other traditional Geodiidae fossil genera, Geoditesia, and Geodiopsis, are reallocated to the Tetractinellida incertae sedis.
Data Availability Statement
The datasets presented in this study can be found in online repositories. The names of the repository/repositories and accession number(s) can be found in the article/Supplementary Material.
Author Contributions
PC conceived and designed the study, performed the observations, and the data analyses and literature surveys. PC wrote the manuscript.
Funding
PC received support by the European Union’s Horizon 2020 Research and Innovation Program under Grant Agreement No. 679849 (the SponGES project). This document reflects only the authors’ view and the Executive Agency for Small and Medium-sized Enterprises (EASME) is not responsible for any use that may be made of the information it contains. Visits to the NHM and the HBOI collections were funded by “Inez Johanssons” travel grants to PC (Uppsala University, HT2012, and HT2014).
Conflict of Interest
The authors declare that the research was conducted in the absence of any commercial or financial relationships that could be construed as a potential conflict of interest.
Acknowledgments
I am very grateful to Ana Riesgo and Vasiliki Koutsouveli (NHM London) for sharing SEM pictures from a cryofractured G. barretti and to A. Riesgo for help with fishing the 28S sequences of G. atlantica and G. phlegraei in transcriptomes. I would like to thank Consuelo Sandino for giving access to the NHM paleontology collections. Thanks to Fayez Ahmad (The Hashemite University, Zarqa, Jordan) for sharing two Geoditesia jordanensis specimens; thanks to Christian Marshall (Station Marine d’Endoume, Marseille, France) for his help with the preparation of this specimen. Thanks to Marzia Bo (Università degli Studi di Genova, Italy), Sadie Mills, and Michelle Kelly (NIWA, New Zealand), Nicole de Voogd (Naturalis, Leiden, The Netherlands), Shirley Pomponi (HBOI, Fort Pierce, FL, U.S.A.), and Pilar Ríos (IEO, Gijón, Spain) for collecting and/or sharing the material. Thanks to Klaus Rützler, Sophie Carteron, Jean Vacelet, Pilar Ríos, Rob van Soest, Patricia Gómez/Diana Ugalde for sharing SEM pictures of Geodia, Erylus or Placospongia species which were used for the plates or Table 3. Thanks to all the Institutes where I was able to do SEM images throughout these last 12 years: University of Bergen (Norway), MNHN (Paris, France), IMBE (Station Marine d’Endoume, Marseille, France), and Uppsala University (Sweden). Thanks to David Rees (University of Bergen, BIO) for help sequencing the COI of some of these species. Thanks to Andrzej Pisera (Institute of Paleobiology, Polish Academy of Sciences, Warsawa, Poland) for discussions on sponge fossils and paleontology literature. Thanks to Karin Steffen (Uppsala University) for helping with the translation of paleontology articles in German. Caminus sp. (MNHN-IP-2015-1144) in Figure 4 originates from the deep-sea cruise BIOMAGLO conducted jointly by French National Museum of Natural History (MNHN) as part of the Tropical Deep-Sea Benthos program, the French Research Institute for Exploitation of the Sea (IFREMER), the French Southern and Antarctic Lands (TAAF), the Departmental Council of Mayotte and the French Development Agency (AFD), with the financial support of the European Union. I am grateful to the crew of R/V Antèa, especially C. Debitus in charge of collecting and documenting sponge specimens, as well as the cruise leaders of BIOMAGLO: L. Corbari, K. Olu-Le Roy, and S. Samadi. Geodia vaubani (MNHN-IP-2015-1667) in Figure 2 originates from the deep-sea cruise KANACONO expedition in New Caledonia (convention MNHN-Province Sud, APA_N_2016_012; PI: N. Puillandre and S. Samadi). KANACONO and KANADEEP2 expeditions operated under the regulations then in force in the country in question and satisfies the conditions set by the Nagoya Protocol for access to genetic resources. NIWA material was provided by the NIWA Invertebrate Collection, Wellington, New Zealand collected on the research programme: “Seamounts: their importance to fisheries and marine ecosystems,” undertaken by NIWA and funded by the former New Zealand Foundation for Research, Science and Technology with additional funding from the former NZ Ministry of Fisheries and NOAA Satellite Operations Facility. Research was co-funded under Coasts and Oceans Research Programme 2 Marine Biological Resources: Discovery and definition of the marine biota of New Zealand (2019/2020 SCI). I am grateful to the Porifera Tree of Life (PorToL) project (Dr. Bob Thacker) and Dr. David Newman for providing permission to access the U.S. National Cancer Institute’s (NCI) collection of sponges, housed at the U.S. National Museum of Natural History, Smithsonian Institution. These specimens were originally collected under contract to NCI by Dr. Pat Colin and Lori Bell Colin of the Coral Reef Research Foundation (CRRF), Koror, Palau; I thank CRRF for providing access to metadata for these specimens.
Supplementary Material
The Supplementary Material for this article can be found online at: https://www.frontiersin.org/articles/10.3389/fmars.2020.613610/full#supplementary-material
Supplementary Figure 1 | Astrophorina 28S (C1–D2) maximum likelihood (ML) tree reconstructed with RAxML. ML bootstrap supports (1,000 bootstrap replicates) > 70 are indicated. Genbank accession numbers are given after each taxon. In bold are new sequences produced in this study. Geodiidae species have purple branches, Calthropellidae have brown branches. Red branches indicate Geodiidae species which secondarily lost sterrasters (species previously considered to belong to the Ancorinidae). Colored dots indicate sequenced species/specimens for which previous authors or this study have observed the surface of sterrasters with SEM (see Table 3 for references); round shapes represent sterrasters, flattened shapes represent aspidasters.
Supplementary Table 1 | List of paleontological records of ball-shaped spicules (sterrasters, aspidasters, selenasters, rhaxes). Whenever the term “rhax” is used in publications it entails that it is a reniform-shaped spicule. “?” means missing data. Colors stand for periods: blue for Jurassic, green for Cretaceous and yellow/orange for Paleogene. In purple, comments on the identifications given in the papers.
Supplementary Dataset 1 | Astrophorina COI alignment in FASTA format.
Supplementary Dataset 2 | Astrophorina 28S (C1–D2) alignment in FASTA format.
Abbreviations
CASIZ, California Academy of Sciences Invertebrate Zoology, San Francisco, CA, USA; CNPGG, Colección Nacional del Phylum Porifera “Gerardo Green,” Instituto de Ciencias del Mar y Limnología, Universidad Nacional Autónoma de México, Mexico; HBOI, Harbor Branch Oceanographic Institute, Florida Atlantic University, Fort Pierce, FL, USA; MNHN, Muséum National d’Histoire Naturelle, Paris, France; MNRJ, Museu Nacional Rio de Janeiro, Brazil; NHM, Natural History Museum, London, UK; NHMUK PI, Palaeontology collection, Natural History Museum, London, UK; NIWA, National Institute of Water and Atmospheric Research, New Zealand; NTM, Museum and Art Gallery of the Northern Territory, Darwin, Australia; PC, personal collection (P. Cárdenas); RMNH, Rijksmuseum van Natuurlijke Historie, Naturalis Biodiversity Center, Leiden, The Netherlands; SAMA, South Australian Museum, Adelaide, Australia; SME, Station Marine d’Endoume, Marseille, France; UPSZMC, Zoological Museum of Uppsala, Uppsala, Sweden; USNM, Smithsonian National Museum of Natural History, USA; ZMAPOR, Amsterdam Porifera collection, now stored at Naturalis, Leiden, The Netherlands; ZMBN, Bergen Museum, Bergen, Norway.
Footnotes
- ^ https://poriferatreeoflife.org
- ^ http://www.phylo.org
- ^ http://phylonames.org/code/
- ^ http://portal.gplates.org/map
References
Adams, C. L., and Hooper, J. N. A. (2001). A revision of Australian Erylus (Porifera : Demospongiae : Astrophorida : Geodiidae) with a tabular review of worldwide species. Invertebr. Syst. 15, 319–340. doi: 10.1071/IT00028
Afşar, F., Duda, J.-P., Zeller, M., Verwer, K., Westphal, H., and Eberli, G. P. (2014). “First report of sponge rhaxes in the Picuùn Leufuù Formation (Tithonian–Berriasian), Neuqueèn Basin, Argentina,” in ”Spongy, slimy, cosy & more.”. Commemorative volume in celebration of the 60th birthday of Joachim Reitner, eds F. Wiese, M. Reich, and G. Arp (Güttingen: Saint Philip Street Press), 49–56.
Ba̧k, M., Górny, Z., Ba̧k, K., Wolska, A., and Stożek, B. (2015). Successive stages of calcitization and silicification of Cenomanian spicule-bearing turbidites based on microfacies analysis, Polish Outer Carpathians. Ann. Soc. Geol. Poloniae 85, 187–203. doi: 10.14241/asgp.2015.003
Becking, L. (2013). Revision of the genus Placospongia (Porifera, Demospongiae, Hadromerida, Placospongiidae) in the Indo-West Pacific. ZooKeys 298, 39–76. doi: 10.3897/zookeys.298.1913
Block Vagle, G., Hurst, A., and Dypvik, H. (1994). Origin of quartz cements in some sandstones from the jurassic of the inner moray firth (UK). Sedimentology 41, 363–377. doi: 10.1002/9781444304459.ch22
Bonci, M. C., Magnino, G., Radrizzani, C. P., and Pronzato, R. (1997). Finding of Geodia (Demospongiae) sterrasters in the Late Miocene of Capella Montei (Alessandria) and comparison with living forms. Bollettino della Società Paleontol. Ital. 35, 245–256. doi: 10.1080/00364820410002451
Bortolotto, M., Braeckman, J. C., Daloze, D., and Tursch, B. (1978). Chemical studies of marine invertebrates. XXXVI. Strongylosterol, a novel C-30 sterol from the sponge Strongylophora durissima (Dendy). Bull. Soc. Chimiques Belges 87, 539–543. doi: 10.1002/bscb.19780870706
Boury-Esnault, N., Pansini, M., and Uriz, M. J. (1994). Spongiaires bathyaux de la mer d’Alboran et du golfe ibéro-marocain. Mémoires Muséum Natl. d’Histoire Nat. 160, 1–174.
Cárdenas, P., Menegola, C., Rapp, H. T., and Díaz, M. C. (2009). Morphological description and DNA barcodes of shallow-water Tetractinellida (Porifera: Demospongiae) from Bocas del Toro, Panama, with description of a new species. Zootaxa 2276, 1–39. doi: 10.11646/zootaxa.2276.1.1
Cárdenas, P., and Moore, J. A. (2019). First records of Geodia demosponges from the New England Seamounts, an opportunity to test the use of DNA mini-barcodes on museum specimens. Mar. Biodivers. 49, 163–174. doi: 10.1007/s12526-017-0775-3
Cárdenas, P., and Rapp, H. T. (2013). Disrupted spiculogenesis in deep-water Geodiidae (Porifera, Demospongiae) growing in shallow waters. Invertebr. Biol. 132, 173–194. doi: 10.1111/ivb.12027
Cárdenas, P., and Rapp, H. T. (2015). Demosponges from the Northern Mid-Atlantic Ridge shed more light on the diversity and biogeography of North Atlantic deep-sea sponges. J. Mar. Biol. Assoc. U.K. 95, 1475–1516. doi: 10.1017/S0025315415000983
Cárdenas, P., Rapp, H. T., Klitgaard, A. B., Best, M., Thollesson, M., and Tendal, O. S. (2013). Taxonomy, biogeography and DNA barcodes of Geodia species (Porifera, Demospongiae, Tetractinellida) in the Atlantic boreo-arctic region. Zool. J. Linn. Soc. 169, 251–311. doi: 10.1111/zoj.12056
Cárdenas, P., Rapp, H. T., Schander, C., and Tendal, O. S. (2010). Molecular taxonomy and phylogeny of the Geodiidae (Porifera, Demospongiae, Astrophorida) — combining phylogenetic and Linnaean classification. Zool. Scripta 39, 89–106. doi: 10.1111/j.1463-6409.2009.00402.x
Cárdenas, P., Vacelet, J., Chevaldonné, P., Pérez, T., and Xavier, J. R. (2018). From marine caves to the deep sea, a new look at Caminella (Demospongiae, Geodiidae) in the Atlanto-Mediterranean region. Zootaxa 4466, 174–196. doi: 10.11646/zootaxa.4466.1.14
Cárdenas, P., Xavier, J. R., Reveillaud, J., Schander, C., and Rapp, H. T. (2011). Molecular phylogeny of the Astrophorida (Porifera, Demospongiae) reveals an unexpected high level of spicule homoplasy. PLoS One 6:e18318. doi: 10.1371/journal.pone.0018318
Carter, H. J. (1871). On fossil sponge-spicules of the Greensand compared with those of existing species. Ann. Magazine Nat. History 7, 112–141. doi: 10.1080/00222937108696328
Carter, H. J. (1876). Descriptions and figures of deep-sea sponges and their spicules, from the Atlantic Ocean, dredged up on board H.M.S.‘Porcupine’, chiefly in 1869 (concluded). Ann. Magazine Nat. History 4, 226–240. doi: 10.1080/00222937608682035
Carvalho, M. D. S., Lopes, D. A., Cosme, B., and Hajdu, E. (2016). Seven new species of sponges (Porifera) from deep-sea coral mounds at Campos Basin (SW Atlantic). Helgoland Mar. Res. 70:10. doi: 10.1186/s10152-016-0461-z
Chanas, B., and Pawlik, J. R. (1995). Defenses of Caribbean sponges against predatory reef fish. II. Spicules, tissue toughness, and nutritional quality. Mar. Ecol. Prog. Ser. 127, 195–211. doi: 10.3354/meps127195
Chombard, C., Boury-Esnault, N., and Tillier, S. (1998). Reassessment of homology of morphological characters in Tetractinellid sponges based on molecular data. Syst. Biol. 47, 351–366. doi: 10.1080/106351598260761
Corriero, G. (1987). (1989). The sponge fauna from the Stagnone di Marsala (Sicily): taxonomic and ecological observations. Bollettino dei musei e degli istituti biologici dell’. Univ. Genova 53, 101–113.
da Silva, C. M. M. (2002). Revisão das espécies de Geodia (Porifera, Astrophorida, Geodiidae) do Atlântico Ocidental e Pacífico Oriental. Ph.D. thesis, Instituto de Biociências da Universidade de São Paulo, Butantã.
da Silva, C. M. M., and Mothes, B. (2000). Three new species of Geodia (Porifera, Demospongiae) from the bathyal depths off Brazilian coast, Southwestern Atlantic. Revue Suisse de Zool. 107, 31–48. doi: 10.5962/bhl.part.80117
de Geyter, G., and Willems, W. (1982). Sponge spicules from the Landen Formation in Belgium. Tertiary Res. 3, 153–160.
de Laubenfels, M. W. (1953). Sponges from the Gulf of Mexico. Bull. Mar. Sci. Gulf Caribbean 2, 511–557.
Delecat, S., Peckmann, J., and Reitner, J. (2001). Non-rigid cryptic sponges in oyster patch reefs (Lower Kimmeridgian, Langenberg/Oker, Germany). Facies 45, 231–254. doi: 10.1007/bf02668115
Donofrio, D. A. (1984). Microfaune triassiche e giurassiche della serie calcareo-silico-marnosa dellaLucania, facies S. Fele (Appennino Campano-Lucano, Italia meridionale). Geol. Paleont. Mitt. Innsbruck 13, 177–179.
Finks, R. M., Hollocher, K., and Thies, K. J. (2011). A major Eocene sponge fauna (Castle Hayne Formation, North Carolina). J. Carolina Acad. Sci. 127, 39–175. doi: 10.7572/2167-5880-127.2.39
Frisone, V., Pisera, A., Hajdu, E., Preto, N., Zorzi, F., and Zorzin, R. (2014). Isolated spicules of Demospongiae from Mt. Duello (Eocene, Lessini Mts., northern Italy): preservation, taxonomy, and depositional environment. Facies 60, 883–904. doi: 10.1007/s10347-014-0407-3
Geller, J., Meyer, C., Parker, M., and Hawk, H. (2013). Redesign of PCR primers for mitochondrial cytochrome c oxidase subunit I for marine invertebrates and application in all-taxa biotic surveys. Mol. Ecol. Resour. 13, 851–861. doi: 10.1111/1755-0998.12138
González-Farías, F. (1989). Estudio al microscopio electrónico de barrido de la estructura esquelética de la esponja Placospongia melobesioides Gray. Anales del Instituto de Ciencias del Mar y Limnologia Universidad Nacional Autónoma de México 16, 81–90.
Gramann, F. (1962). Schwamm-Rhaxen und Schwamm-Gesteine (Spongiolithe, Spiculite) aux dem Oxford NW-Deutschlands. Geologisches Jahrbuch 80, 213–220.
Grech, D., van de Poll, B., Bertolino, M., Rosso, A., and Guala, I. (2020). Massive stranding event revealed the occurrence of an overlooked and ecosystem engineer sponge. Mar. Biodivers. 50:82. doi: 10.1007/s12526-020-01105-4
Gruber, G., and Reitner, J. (1991). Isolated poriferan micro- and megascleres from the lower Campanian of Höver (northern Germany) and remarks on the phylogeny of the taxon Geodiidae (Demospongiae). Berliner Geowissenschaftliche Abhandlungen 134, 107–117.
Hajdu, E., Peixinho, S., and Fernandez, J. C. C. (2011). Esponjas Marinhas da Bahia - Guia de Campo e Laboratório. Série Livros 45. Rio de Janeiro: Museu Nacional/UFRJ.
Hanitsch, R. (1895). Notes on a collection of sponges from the West Coast of Portugal. Trans. Liverpool Biol. Soc. 9, 205–219.
Haslett, S. K. (1992). Rhaxellid sponge microscleres from the Portlandian of Dorset, UK. Geol. J. 27, 339–347. doi: 10.1002/gj.3350270404
Hill, M. S., and Hill, A. L. (2002). Morphological plasticity in the tropical sponge Anthosigmella varians: responses to predators and wave energy. Biol. Bull. 202, 86–95. doi: 10.2307/1543225
Hinde, G. J. (1885). VII. On beds of sponge-remains in the lower and upper greensand of the South of England. Philos. Trans. R. Soc. Lond. 176, 403–453. doi: 10.1098/rstl.1885.0007
Hinde, G. J. (1888). A monograph of the british fossil sponges. Part II. (Pages 93–188; Plate IX.). Monogr. Palaeontogr. Soc. 41, 6–188. doi: 10.1080/02693445.1888.12027997
Hinde, G. J. (1890). On a new genus of siliceous sponges from the lower calcareous grit of Yorkshire. Q. J. Geol. Soc. 46, 54–61. doi: 10.1144/gsl.jgs.1890.046.01-04.06
Hinde, G. J., and Holmes, W. M. (1892). On the sponge-remains in the lower tertiary strata near oamaru, Otago, New Zealand. Zool. J. Linn. Soc. 24, 177–262. doi: 10.1111/j.1096-3642.1892.tb02480.x
Huang, T. (1967). 531. A new radiolaria from the Somachi Formation, Kikai-Jima, Kagoshima perfecture, Japan. Trans. Proc. Paleontol. Soc. Jpn. 1967, 177–184. doi: 10.14825/prpsj1951.1967.68_177
Hughes, G. W. (1985). Silicosphaera asteroderma (Porifera), a new siliceous microfossil from the South China Sea. Neues Jahrb Geol. Palaeontol. Monatsh. 1985, 599–604. doi: 10.1127/njgpm/1985/1985/599
Hurcewicz, H. (1966). Siliceous sponges from the upper cretaceous of Poland. Part I. Tetraxonia. Acta Palaeontol. Polonica 11, 1–129.
Inoue, M., and Iwasaki, Y. (1975). A problematic micro-organism similar to the sterraster of sponges. Proc. Jpn. Acad. 51, 273–278. doi: 10.2183/pjab1945.51.273
International Commission on Zoological Nomenclature [ICZN] (1999). International Code of Zoological Nomemclature (ICZN), 4th Edn. Available online at: https://www.iczn.org/the-code/the-international-code-of-zoological-nomenclature/the-code-online/ (accessed November 10, 2020).
Jach, R. (2002). Lower Jurassic spiculite series from the Križna Unit in the Western Tatra Mts, Western Carpathians, Poland. Ann. Soc. Geol. Poloniae 72, 131–144.
Katoh, K., and Standley, D. M. (2013). MAFFT multiple sequence alignment software version 7: improvements in performance and usability. Mol. Biol. Evol. 30, 772–780. doi: 10.1093/molbev/mst010
Kaya, M., Bilican, I., Mujtaba, M., Sargin, I., Erginer Haskoylu, M., Toksoy Oner, E., et al. (2020). Sponge-derived natural bioactive glass microspheres with self-assembled surface channel arrays opening into a hollow core for bone tissue and controlled drug release applications. Chem. Eng. J. doi: 10.1016/j.cej.2020.126667 [Epub ahead of print].
Kelly, M., and Cárdenas, P. (2016). An unprecedented new genus and family of Tetractinellida (Porifera, Demospongiae) from New Zealand’s Colville Ridge, with a new type of mitochondrial group I intron. Zool. J. Linn. Soc. 177, 335–352. doi: 10.1111/zoj.12365
Kelly, M., Cárdenas, P., Rush, N., Sim-Smith, C., McPherson, D., Page, M., et al. (2019). Molecular study supports the position of New Zealand endemic genus Lamellomorpha in family Vulcanellidae (Demospongiae, Tetractinellida, Astrophorina), with the description of three new species. Eur. J. Taxonomy 506, 1–25. doi: 10.5852/ejt.2019.506
Klitgaard, A. B., and Tendal, O. S. (2004). Distribution and species composition of mass occurrences of large-sized sponges in the northeast Atlantic. Prog. Oceanogr. 61, 57–98. doi: 10.1016/j.pocean.2004.06.002
Kozur, H. W., Mostler, H., and Repetski, J. E. (1996). ‘Modern’ siliceous sponges from the lowermost Ordovician (Early Ibexian - Early Tremadocian) Windfall Formation of the Antelope Range, Eureka County, Nevada, U.S.A. Geol. Paläeontol. Mitteilungen Innsbruck 21, 201–221.
Lamarck, J. B. P. (1815). Suite des polypiers empâtés. Mémoires du Muséum d’Histoire Naturelle 1, 69–80.
Larsson, A. (2014). AliView: a fast and lightweight alignment viewer and editor for large datasets. Bioinformatics 30, 3276–3278. doi: 10.1093/bioinformatics/btu531
Lehnert, H. (1993). The sponges from Cozumel (Mexico). Inventory, critical comparison of taxonomic characters and description of a new species. Acta Biol. Benrodis 5, 35–127.
Lehnert, H., Stone, R., and Heimler, W. (2006). Erylus aleuticus sp. nov. (Porifera: Demospongiae: Astrophorida: Geodiidae) from the Aleutian Islands, Alaska, USA. J. Mar. Biol. Assoc. U.K. 86, 971–975. doi: 10.1017/S0025315406013944
Lehnert, H., and Stone, R. P. (2016). A comprehensive inventory of the Gulf of Alaska sponge fauna with the description of two new species and geographic range extensions. Zootaxa 4144:18. doi: 10.11646/zootaxa.4144.3.5
Lindgren, N. G. (1898). Beitrag zur Kenntniss der Spongienfauna des Malayischen Archipels und der chinesischen Meere. Zool. Jahrbücher 11, 283–378.
Łukowiak, M. (2015). Late Eocene siliceous sponge fauna of southern Australia: reconstruction based on loose spicules record. Zootaxa 3917, 1–65. doi: 10.11646/zootaxa.3917.1.1
Łukowiak, M., and Pisera, A. (2016). Bodily preserved Eocene non-lithistid demosponge fauna from southern Australia: taxonomy and affinities. J. Syst. Palaeontol. 15, 473–497. doi: 10.1080/14772019.2016.1197329
Łukowiak, M., Pisera, A., and O’Dea, A. (2013). Do spicules in sediments reflect the living sponge community? A test in a Caribbean shallow-water lagoon. PALAIOS 28, 373–385. doi: 10.2110/palo.2012.p12-082r
McIntyre, F. D., Drewery, J., Eerkes-Medrano, D., and Neat, F. C. (2016). Distribution and diversity of deep-sea sponge grounds on the Rosemary Bank Seamount, NE Atlantic. Mar. Biol. 163, 1–11. doi: 10.1007/s00227-016-2913-z
Mehl, D. (1998). Porifera and chancelloridae from the middle cambrian of the georgina basin, Australia. Palaeontology 41, 1153–1182.
Mercurio, M., Corriero, G., and Gaino, E. (2006). Sessile and non-sessile morphs of Geodia cydonium (Jameson) (Porifera, Demospongiae) in two semi-enclosed Mediterranean bays. Mar. Biol. 148, 489–501. doi: 10.1007/s00227-005-0092-4
Meylan, A. (1988). Spongivory in hawksbill turtles: a diet of glass. Science 239, 393–395. doi: 10.1126/science.239.4838.393
Miller, M. A., Pfeiffer, W., and Schwartz, T. (2010). “Creating the CIPRES Science Gateway for inference of large phylogenetic trees,” in Proceedings of the Gateway Computing Environments Workshop (GCE), 14 Nov. 2010, (New Orleans, LA), 1–8.
Moraes, F. C. (2011). Esponjas das Ilhas Oceânicas Brasileiras. Série Livros 44. Rio de Janeiro: Museu Nacional/UFRJ.
Morris, P. H., Payne, S. N. J., and Richards, D. P. J. (1999). “Micropalaeontological biostratigraphy of the magnus sandstone member (Kimmeridgian-Early Volgian), Magnus Field, UK North Sea,” in Biostratigraphy in Production and Development Geology, eds R. W. Jones and M. D. Simmons (London: Geological Society), 55–73. doi: 10.1144/gsl.sp.1999.152.01.04
Mostler, H. (1976). Poriferenspiculae der alpinen Trias. Geol. Paläeontol. Mitteilungen Innsbruck 6, 1–42.
Mostler, H. (1990). Mikroskleren von Demospongien (Porifera) aus dem basalen Jura der Nördlichen Kalkalpen. Geol. Paläeontol. Mitteilungen Innsbruck 17, 119–142.
Mostler, H. (1996). Ein beitrag zur genese Liassischer toxa und forcipes (skelettelemente von demospongien). Geol. Paläeontol. Mitteilungen Innsbruck 21, 185–199.
Mothes, B., Lerner, C. B., and da Silva, C. M. M. (1999). Revision of Brazilian Erylus (Porifera: Astrophorida: Demospongiae) with description of a new species. Mem. Queensland Museum 44, 369–380.
Müller, W. E. G., Schloßmacher, U., Eckert, C., Krasko, A., Boreiko, A., Ushijima, H., et al. (2007). Analysis of the axial filament in spicules of the demosponge Geodia cydonium: different silicatein composition in microscleres (asters) and megascleres (oxeas and triaenes). Eur. J. Cell Biol. 86, 473–487. doi: 10.1016/j.ejcb.2007.06.002
Murillo, F. J., Kenchington, E., Lawson, J. M., Li, G., and Piper, D. J. W. (2016). Ancient deep-sea sponge grounds on the Flemish Cap and Grand Bank, northwest Atlantic. Mar. Biol. 163, 1–11. doi: 10.1007/s00227-016-2839-5
Murillo, F. J., Muñoz, P. D., Cristobo, J., Ríos, P., González, C., Kenchington, E., et al. (2012). Deep-sea sponge grounds of the Flemish Cap, Flemish Pass and the Grand Banks of Newfoundland (Northwest Atlantic Ocean): distribution and species composition. Mar. Biol. Res. 8, 842–854. doi: 10.1080/17451000.2012.682583
Pisera, A. (1997). Upper jurassic siliceous sponges from the swabian alb: taxonomy and paleoecology. Palaeontol. Polonica 57, 3–216.
Pisera, A., Cachao, M., and Da Silva, C. M. (2006). Siliceous sponge spicules from the Miocene Mem Moniz marls (Portugal) and their environmental significance. Rivista Italiana Di Paleontologia E Stratigrafia 112, 287–299. doi: 10.13130/2039-4942/6342
Plese, B., Kenny, N., Rossi, M. E., Cárdenas, P., Schuster, A., Taboada, S., et al. (2020). Mitochondrial evolution in the demospongiae (porifera): phylogeny, divergence time, and genome biology. Mol. Phylogenet. Evol. doi: 10.1186/s12862-018-1230-1 [Epub ahead of print].
Redmond, N. E., Morrow, C. C., Thacker, R. W., Diaz, M. C., Boury-Esnault, N., Cárdenas, P., et al. (2013). Phylogeny and systematics of demospongiae in light of new small subunit ribosomal DNA (18S) sequences. Integr. Compar. Biol. 53, 388–415. doi: 10.1093/icb/ict078
Reif, W.-E. (1967). Schwammspicula aus dem weissen Jura zeta von Nattheim (Schwäbische Alb). Palaeontogr. Abteilung A 127, 85–102.
Reitner, J., and Mehl, D. (1995). Early Paleozoic diversification of sponges: new data and evidences. Geol. Paläontol. Mitteilungen Innsbruck 20, 335–347.
Rezvoi, P. D., Zhuravleva, I. T., and Koltun, V. M. (1962). “Phylum Porifera,” in Osnovy Paleontologii [Fundamentals of Paleontology], vol.1, number 2, Porifera, Archaeocyatha, Coelenterata, Vermes, ed. B. S. Sokolov (Moscow: Izdatel’stvo Akademii Nauk SSSR), 17–74.
Rigby, J. K. (2004). “Part E. Porifera,” in Treatise on Invertebrate Paleontology, ed. R. L. Kaesler (Boulder, CO: The Geological Society of America and the University of Kansas), 185–192.
Rigby, J. K., and Smith, C. C. (1992). Microscleres of a Paleocene Geodia from Western Alabama. J. Paleontol. 66, 406–413. doi: 10.1017/S0022336000033965
Říha, J. (1987). Problematicky tvar jehlic hub Sterraster (Rhax) v Miocenu CSSR. Časopis Moravského muzea. Vědy přírodní 72, 75–80.
Robinson, P. D., and Haslett, S. K. (1995). A radiolarian dated sponge microsclere assemblage from the Miocene Dos Bocas Formation of Ecuador. J. S. Am. Earth Sci. 8, 195–200. doi: 10.1016/0895-9811(95)00005-Z
Rützler, K., and Macintyre, I. G. (1978). Siliceous sponge spicules in coral reef sediments. Mar. Biol. 49, 147–159. doi: 10.1007/BF00387114
Rützler, K., Piantoni, C., Van Soest, R. W. M., and Díaz, M. C. (2014). Diversity of sponges (Porifera) from cryptic habitats on the Belize barrier reef near Carrie Bow Cay. Zootaxa 3805:129. doi: 10.11646/zootaxa.3805.1.1
Santucci, R. (1922). La Geodia cydonium come centro di associazione biologica. Mem. Comitato Talassografico ltaliano 103, 5–19.
Schmidt, O. (1868). Die Spongien der Küste von Algier. Mit Nachträgen zu den Spongien des Adriatischen Meeres (Drittes Supplement). Leipzig: Wilhelm Engelmann.
Schoeppler, V., Reich, E., Vacelet, J., Rosenthal, M., Pacureanu, A., Rack, A., et al. (2017). Shaping highly regular glass architectures: a lesson from nature. Sci. Adv. 3:eaao2047. doi: 10.1126/sciadv.aao2047
Schrammen, A. (1899). Beitrag zur Kenntnis der obersenonen Tetractinelliden. Mittheilungen aus dem Roemer-Museum Hildesheim 10, 1–9.
Schrammen, A. (1910). Die Kieselspongien der oberen Kreide von Nordwestdeutschland. I. Teil. Tetraxonia, Monaxonia und Silicea incertae sedis. Palaeontographica 5(Suppl.), 1–175.
Schrammen, A. (1924). Die Kieselspongien der oberen Kreide von Nordwestdeutschland. III. und letzter Teil. Monogr. Geol. Paläontol. 1, 1–159.
Schuster, A., Erpenbeck, D., Pisera, A., Hooper, J. N. A., Bryce, M., Fromont, J., et al. (2015). Deceptive desmas: molecular phylogenetics suggests a new classification and uncovers convergent evolution of lithistid demosponges. PLoS One 10:e116038. doi: 10.1371/journal.pone.0116038
Shim, E. J., and Sim, C. J. (2012). A new species of the genus Caminus (Astroporida: Geodiidae) from Korea. J. Anim. Syst. Evol. Divers. 28, 208–211. doi: 10.5635/ASED.2012.28.3.208
Sim-Smith, C., and Kelly, M. (2015). The marine fauna of New Zealand: sponges in the family Geodiidae (Demospongiae: Astrophorina). NIWA Biodivers. Mem. 128, 1–102.
Sollas, W. J. (1877). On the changes produced in the siliceous skeletons of certain sponges by the action of caustic potash. Ann. Magazine Nat. History 20, 285–300.
Sollas, W. J. (1880a). On the flint nodules of the Trimmingham chalk. Ann. Magazine Nat. Hist. 6, 384–395. doi: 10.1080/00222938009458956
Sollas, W. J. (1880b). The sponge-fauna of Norway; a report on the Rev. A.M. Norman’s collection of sponges from the Norwegian Coast. Ann. Magazine Nat. Hist. 5, 396–409.
Sollas, W. J. (1880c). The sponge-fauna of Norway; a report on the Rev. A.M. Norman’s Collection of sponges from the Norwegian Coast. Ann. Magazine Nat. Hist. 5, 130–144.
Sollas, W. J. (1882). The sponge-fauna of Norway; a Report on the Rev. A.M. Norman’s Collection of Sponges from the Norwegian Coast. Ann. Magazine Nat. Hist. 5, 141–165.
Sollas, W. J. (1888). Report on the Tetractinellida collected by H.M.S. Challenger, during the years 1873-1876. Report on the Scientific Results of the Voyage of H.M.S. Challenger, 1873-1876. Zoology 25, 1–458.
Stamatakis, A. (2014). RAxML version 8: a tool for phylogenetic analysis and post-analysis of large phylogenies. Bioinformatics 30, 1312–1313. doi: 10.1093/bioinformatics/btu033
Stephens, J. (1915). Sponges of the Coasts of Ireland. I.- The Triaxonia and part of the Tetraxonida. Fish. Ireland Sci. Investig. 1914, 1–43.
Tanita, S., and Hoshino, T. (1989). The Demospongiae of Sagami Bay. Japan: Biological Laboratory, Imperial Household, 1–197.
Thacker, R. W., Hill, A. L., Hill, M. S., Redmond, N. E., Collins, A. G., Morrow, C. C., et al. (2013). Nearly complete 28S rRNA gene sequences confirm new hypotheses of sponge evolution. Integr. Compar. Biol. 53, 373–387. doi: 10.1093/icb/ict071
Todt, C., Cárdenas, P., and Rapp, H. T. (2009). The chiton Hanleya nagelfar (Polyplacophora, Mollusca) and its association with sponges in the European Northern Atlantic. Mar. Biol. Res. 5, 408–411. doi: 10.1080/17451000802572394
Trejo, M. (1967). La esponja fosil Rhaxella sorbyana (Blake) y su significacion estratigraphica. Boletín de la Asociación Mexicana de Geólogos Petroleros 48, 33–38.
Ugalde, D., Gómez, P., and Simões, N. (2015). Marine sponges (Porifera: Demospongiae) from the Gulf of México, new records and redescription of Erylus trisphaerus (de Laubenfels, 1953). Zootaxa 3911, 33. doi: 10.11646/zootaxa.3911.2.1
Ungureanu, D., Ahmad, F., and Farouk, S. (2018). A Callovian (Middle Jurassic) poriferan fauna from northwestern Jordan: taxonomy, palaeoecology and palaeobiogeography. Historical Biol. 30, 577–592. doi: 10.1080/08912963.2017.1304935
Vacelet, J., and Cárdenas, P. (2018). When is an aster not an aster? A new deep-sea Discorhabdella (Demospongiae, Poecilosclerida) with asters, from the Mozambique Channel. Zootaxa 4466, 197–204. doi: 10.11646/zootaxa.4466.1.15
van Kempen, T. M. G. (1990). “On the oldest tetraxon megascleres,” in New Perspectives in Sponge Biology. Third International Conference on the Biology of Sponges, ed. K. Rützler (Washington, D.C: Smithsonian Institution Press), 9–16.
Van Soest, R. W. M. (2017). Sponges of the Guyana Shelf. Zootaxa 4217, 1–225. doi: 10.11646/zootaxa.4217.1.1
Van Soest, R. W. M., Boury-Esnault, N., Hooper, J. N. A., Rützler, K., de Voogd, N. J., Alvarez, B., et al. (2020). World Porifera Database [Online]. Available online at: http://www.marinespecies.org/porifera (accessed September 29, 2020).
Van Soest, R. W. M., Meesters, E. H., and Becking, L. E. Z. (2014). Deep-water sponges (Porifera) from bonaire and klein curaçao, Southern Caribbean. Zootaxa 3878, 401–443. doi: 10.11646/zootaxa.3878.5.1
Vargas, S., Erpenbeck, D., Göcke, C., Hall, K. A., Hooper, J. N. A., Janussen, D., et al. (2013). Molecular phylogeny of Abyssocladia (Cladorhizidae: Poecilosclerida) and Phelloderma (Phellodermidae: Poecilosclerida) suggests a diversification of chelae microscleres in cladorhizid sponges. Zool. Scripta 42, 106–116. doi: 10.1111/j.1463-6409.2012.00560.x
Vishnevskaya, V. S., Dubinina, E. O., and Mokhov, A. V. (2009). Phenomenal structure of some spicule elements of sponge, radiolarians and nanotechnologies [in Russian]. Collection Sci. Works Instit. Geol. Sci. NAS Ukraine 2, 78–80. doi: 10.30836/igs.2522-9753.2009.147924
von Lendenfeld, R. (1894). Die Tetractinelliden der Adria. (Mit einem Anhange über die Lithistiden). Denkschriften der Kaiserlichen Akademie der Wissenschaften.Wien. Math. Naturwissenschaftliche Klasse 61, 91–204.
von Lendenfeld, R. (1910). The Sponges. 2. The erylidae. in: reports on the scientific results of the expedition to the eastern tropical pacific, in charge of Alexander Agassiz, by the U.S. Fish Commission Steamer ‘Albatross’, from October, 1904, to March, 1905, Lieut. Commander L.M. Garrett, U.S.N., Commanding, and of other Expeditions of the Albatross, 1888-1904. (21). Mem. Museum Comp. Zool. 41, 261–324.
Vosmaer, G. C. J., and Vernhout, J. H. (1902). “The porifera of the siboga-expedition. I. The genus Placospongia,” in Siboga-Expeditie. Uitkomsten op zoologisch, botanisch, oceanographisch en geologisch gebied verzameld in Nederlandsch Oost-lndië 1899-1900aan boord H.M. ‘Siboga’ onder commando van Luitenant ter Zee 1e kl. G.F. Tydeman. 9 (Monographie VIa), ed. M. Weber (Leiden: E. J. Brill), 1–17.
Wagoner, N. A. V., Mudie, P. J., Cole, F. E., and Daborn, G. (1989). Siliceous sponge communities, biological zonation, and Recent sea-level change on the Arctic margin: Ice Island results. Can. J. Earth Sci. 26, 2341–2355. doi: 10.1139/e89-200
Weller, J. M. (1930). Siliceous sponge spicules of pennsylvanian age from illinois and Indiana. J. Paleontol. 4, 233–251.
Wiedenmayer, F. (1994). Contributions to the knowledge of post-Palaeozoic neritic and archibenthal sponges (Porifera). The stratigraphic record, ecology, and global distribution of intermediate and higher taxa. Schweizerische Paläontol. Abhandlungen 116, 1–147.
Wysokowski, M., Jesionowski, T., and Ehrlich, H. (2018). Biosilica as a source for inspiration in biological materials science. Am. Mineral. 103, 665–691. doi: 10.2138/am-2018-6429
Zittel, K. A. (1876). Ueber Coeloptychium. Ein Beitrag zur Kenntniss der Organisation fossiler Spongien. München: Abhandlungen der k. bayr. Akad. der Wissenschaften.
Keywords: Porifera, systematics, Geodia, Placospongia, Rhaxella, microfossils, sterrasters, selenasters
Citation: Cárdenas P (2020) Surface Microornamentation of Demosponge Sterraster Spicules, Phylogenetic and Paleontological Implications. Front. Mar. Sci. 7:613610. doi: 10.3389/fmars.2020.613610
Received: 02 October 2020; Accepted: 16 November 2020;
Published: 14 December 2020.
Edited by:
Joana R. Xavier, University of Porto, PortugalReviewed by:
Hermann Ehrlich, Freiberg University of Mining and Technology, GermanyAndrzej Pisera, Institute of Paleobiology (PAN), Poland
Copyright © 2020 Cárdenas. This is an open-access article distributed under the terms of the Creative Commons Attribution License (CC BY). The use, distribution or reproduction in other forums is permitted, provided the original author(s) and the copyright owner(s) are credited and that the original publication in this journal is cited, in accordance with accepted academic practice. No use, distribution or reproduction is permitted which does not comply with these terms.
*Correspondence: Paco Cárdenas, cGFjby5jYXJkZW5hc0BmYXJtYmlvLnV1LnNl