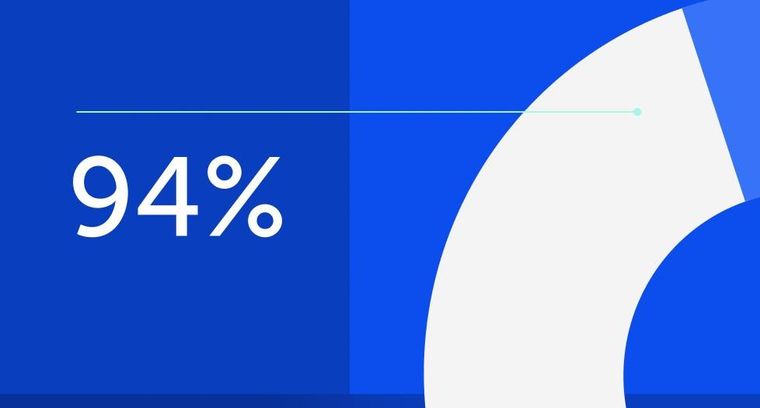
94% of researchers rate our articles as excellent or good
Learn more about the work of our research integrity team to safeguard the quality of each article we publish.
Find out more
ORIGINAL RESEARCH article
Front. Mar. Sci., 27 January 2021
Sec. Marine Megafauna
Volume 7 - 2020 | https://doi.org/10.3389/fmars.2020.608848
Marine protected areas (MPAs) have emerged as potentially important conservation tools for the conservation of biodiversity and mitigation of climate impacts. Among MPAs, a large percentage has been created with the implicit goal of protecting shark populations, including 17 shark sanctuaries which fully protect sharks throughout their jurisdiction. The Commonwealth of the Bahamas represents a long-term MPA for sharks, following the banning of commercial longlining in 1993 and subsequent designation as a shark sanctuary in 2011. Little is known, however, about the long-term behavior and space use of sharks within this protected area, particularly among reef-associated sharks for which the sanctuary presumably offers the most benefit. We used acoustic telemetry to advance our understanding of the ecology of such sharks, namely Caribbean reef sharks (Carcharhinus perezi) and tiger sharks (Galeocerdo cuvier), over two discrete islands (New Providence and Great Exuma) varying in human activity level, over 2 years. We evaluated which factors influenced the likelihood of detection of individuals, analyzed patterns of movement and occurrence, and identified variability in habitat selection among species and regions, using a dataset of 23 Caribbean reef sharks and 15 tiger sharks which were passively monitored in two arrays with a combined total of 13 acoustic receivers. Caribbean reef sharks had lower detection probabilities than tiger sharks, and exhibited relatively low habitat connectivity and high residency, while tiger sharks demonstrated wider roaming behavior across much greater space. Tiger sharks were associated with shallow seagrass habitats where available, but frequently transited between and connected different habitat types. Our data support the notion that large MPAs afford greater degrees of protection for highly resident species such as Caribbean reef sharks, yet still may provide substantial benefits for more migratory species such as tiger sharks. We discuss these findings within the context of species-habitat linkages, ecosystem services, and the establishment of future MPAs.
Marine protected areas (MPAs) are key instruments for conserving and rebuilding marine biodiversity and mitigating human impacts, including those resulting from climate change and habitat loss (Bates et al., 2019; Maestro et al., 2019; Duarte et al., 2020). The benefits of MPAs are widespread, ranging from social to ecological and economic (McClure et al., 2020), and they can also play a key role in the conservation of threatened, mobile marine fauna, especially slow growing, highly migratory species such as sharks (Graham et al., 2016; Duce et al., 2019; Jacoby et al., 2020). Indeed, declines in many shark populations worldwide have spurred growing interest in establishing MPAs to aid in their recovery (Knip et al., 2012), and current estimates suggest up to one-third of all ocean protected habitat has been designated exclusively for sharks (Marine Conservation Institute, 2016; MacKeracher et al., 2019).
Protective management addresses key threats to the species and habitat of interest, and the effectiveness of any MPA will depend largely the extent to which they overlap with the biology - specifically the behavior and ecological niche of species targeted for protection (Dwyer et al., 2020; Hanson et al., 2020). Designing suitable MPAs for the protection of target species requires knowledge of the habitat these species are associated with to ensure they are included within the MPAs (Espinoza et al., 2014; Lea et al., 2016). This information can reveal which habitats may be most suitable for incorporation into future MPAs (Birkmanis et al., 2020) and may assist with understanding how existing MPAs could be managed adaptively. However, long-term behavioral data required to characterize the residency, coastal space use, and the drivers of movement are surprisingly lacking for most shark species, particularly reef-associated species that are likely to benefit from MPAs (Heupel et al., 2019; Dwyer et al., 2020).
Shark MPAs, broadly defined as any spatial protection where extractive activities have been partially restricted or completely prohibited (MacKeracher et al., 2019), have continued to evolve and develop in recent decades. Most recently, shark sanctuaries, a type of shark MPA, have emerged as a popular management tool to reduce shark mortality among island nations, with a total of 17 countries worldwide currently designating their national waters as such (Ward-Paige, 2017). Sanctuary designations typically prohibit the commercial fishing of all sharks, the retention of sharks caught as bycatch, and the possession, trade, and sale of sharks and shark products within a country's full exclusive economic zone (EEZ, Ward-Paige and Worm, 2017; MacKeracher et al., 2019). Shark sanctuaries have received criticism, primarily because our understanding of their effectiveness remains limited – and, in many cases, these MPAs have been created without a robust understanding of the focal species' behavior (Ward-Paige and Worm, 2017). While the creation of shark MPAs (inclusive of shark sanctuaries) has outpaced our understanding of how sharks may use them, telemetry techniques offer many advantages for generating applied information (Hussey et al., 2015; White et al., 2017), and are well-suited for tracking and monitoring mobile elasmobranch species passively over large expanses of ocean (Andrzejaczek et al., 2020; Jacoby et al., 2020).
The Greater Caribbean contains some of the highest records of marine biodiversity in the Atlantic (Roberts et al., 2002) and was recently identified as a priority region for establishing MPAs to meet ambitious spatial conservation targets to protect 30% of oceans by 2030 (Zhao et al., 2020). Yet, historical and recent overfishing, combined with the consumption of sharks in the region (e.g., Ali et al., 2020), have rendered its shark populations patchy and variable among countries (Ward-Paige et al., 2010; Bakker et al., 2017; MacNeil et al., 2020). As a result, there is a need for exploring and creating large scale MPAs in the region to aid in the conservation of sharks and their movements (Gallagher et al., 2020). The Commonwealth of The Bahamas is known for its relatively robust shark populations (Brooks et al., 2011; Gallagher and Hammerschlag, 2011; MacNeil et al., 2020), which benefited from the prohibition of commercial longlining and gillnetting in 1993 and the declaration of The Bahamas exclusive economic zone (EEZ, > 600,000 km2) as a shark sanctuary in 2011 (Haas et al., 2017; Talwar et al., 2020). As a result, the waters of The Bahamas represent a nearly 30-year case study for evaluating how large MPAs are used by a natural and stable population of sharks, and, ultimately, whether existing spatial conservation measures are adequate. However, research into the behavior and movements of sharks in The Bahamas is surprisingly lacking, with the existing published studies either focusing on local monitoring efforts (Shipley et al., 2018) or describing seasonal residency and migration of single species in relation to a discrete tagging location (Hammerschlag et al., 2012; Howey-Jordan et al., 2013; Guttridge et al., 2017; Shipley et al., 2017). For commonly encountered, reef-associated sharks, regional efforts to describe long-term behavior may provide critical information to advance our understanding of how these threatened species utilize the shark sanctuary in The Bahamas, and the pertinent drivers of their behavior.
The overarching goal of this study was to advance our understanding of the behavioral ecology of reef-associated sharks in The Bahamas shark MPA through a multi-region application of passive acoustic monitoring. Specifically, we (1) analyzed which factors influenced our ability to detect and monitor sharks over two discrete islands varying in human activity level, separated by large spatial scales (~250 km); (2) evaluated the temporal patterns of shark movement and occurrence; and (3) identified variability in habitat selection between species and among regions, to infer which habitat types were of importance for ongoing and future spatial conservation strategies. We focused our analyses on two medium-to-large bodied species that are abundant throughout the coastal waters of The Bahamas, the tiger shark (Galeocerdo cuvier, Péron and Lesueur, 1822) and Caribbean reef shark (Carcharhinus perezi, Poey, 1876). Combined, both species generate significant income to The Bahamian economy through dive tourism (a cumulative industry value of >$110 million/year, Haas et al., 2017). Thus, effective conservation of these species promotes both long-term ecological and economic benefits to The Bahamas and could be a model for other countries in the region.
Research was conducted within the coastal waters of two islands in The Bahamas, New Providence Island (25.0443° N, 77.3504° W) and Great Exuma (23.6193° N, 75.9695° W) from February 2018 to February 2020. The Bahamas archipelago is a carbonate platform comprised of over 700 islands and cays, characterized by a diversity of marine habitats such as coral reefs, shallow seagrass beds, mangroves, deep open ocean, and inshore flats (Buchan, 2000). New Providence is a 208 km2 island located in the center of the archipelago, hosting the capital, Nassau, and nearly one-third of the national population (~250,000 people). The north and west portions of New Providence are characterized by sloping walls and coral reef habitats dropping off into deep water in the Tongue of the Ocean, whereas the south and east are dominated shallow seagrass and sand with scattered patch reefs, comprising the northernmost portion of the Great Bahamas Bank (Buchan, 2000). Great Exuma, which is part of the Exuma Cays chain of islands, is a 158 km2 island located roughly 230 km south of New Providence. Great Exuma/The Exuma Cays are characterized by a reef tract separating the deep waters of the Exuma Sound to the east, from and shallow sand and seagrass meadows of the Great Bahamas Bank to the west. To evaluate patterns of shark habitat use and network connectivity within islands, we used passive acoustic telemetry, whereby sharks were implanted with acoustic transmitters and monitored by an array of underwater hydrophone stations.
From February 2018 to June 2019, Caribbean reef sharks (n = 23) and tiger sharks (n = 15) were captured using standardized circle-hook research drum lines (see Gallagher et al., 2014 for detailed description of capture methodology). All animal tagging and capture was ethically approved by the Carleton University Animal Care Committee. Upon capture, animals were secured alongside the research vessel while remaining submerged, and sex and morphometric measurements were taken. Sharks were then inverted to induce a state of tonic immobility and surgically implanted with coded acoustic transmitters (V16, 69 kHz, transmission interval of ~60–120 s, Vemco Ltd., Innovasea, Halifax, Canada). Tags were implanted into the peritoneal cavity by making a 2.5 cm incision in the abdominal wall, which was subsequently closed using dissolvable sutures. Shark diving provisioning occurs regularly in one specific location in the southwest corner of the New Providence Array (Maljković and Côté, 2011), and as a result no sharks were tagged within 3 km of this area to avoid tagging provisioned sharks. An identification tag was also placed externally at the base of the dorsal fin, and each shark was quickly sampled for various tissues for separate investigations (blood, muscle, fin clip), before they were released. The time from securing the shark at the boat until its release ranged between 4 and 10 min.
A total of 13 acoustic receivers (Vemco VR2W, Vemco Ltd., Innovasea, Halifax, Canada) were deployed across the two islands, resulting in two arrays: New Providence (eight receivers) and Great Exuma (five receivers) (Figure 1). Receivers were installed on three separate occasions: May 2018 (New Providence, five initial receivers), November 2018 (New Providence, three receivers added; Great Exuma three initial receivers), and February 2019 (Great Exuma, two receivers added). Receivers were either secured to light-weight concrete moorings or attached to large metal auger screws. Concrete moorings were constructed using large aluminum basting pans filled with 10 kg of dried concrete, housing a 0.75 m piece of rebar, where the VR2W receiver was attached using industrial strength cable ties. Metal augers were screwed into sandy substrates such that ~1 m was sticking above the seafloor, where a bracketed VR2W receiver was then attached using bolts and nuts. Depths of receiver locations ranged from 5 to 18 meters, and were placed in seagrass, sand, and reef habitats. Acoustic receivers were downloaded, and batteries were replaced in November 2018 and again in January / February 2020 (receivers were added here as well). Two temperature loggers (HOBO Water Temp Pro v2), set to record continuously once every hour, were attached to the metal auger frame at one receiver in each array (New Providence = Southwest Reef; Great Exuma = Rat Cay) in November 2018, which recorded water temperature data for up to 1 year. Detection range testing was conducted with fixed sentinel tags deployed between 1 May 18 and 30 November 18 (see Supplementary Materials for full details). Effective detection range for this study was defined as the distance at which 50 % of transmissions were detected and logged based on the Weibull 3-parameter peak curve (Kessel et al., 2014) and was found to be 360 m (Supplementary Figure 1).
Figure 1. Map of Lucayan archipelago, focusing on The Commonwealth of Bahamas with Exclusive Economic Zone Boundary (EEZ, designated with green border) and spatial extent of study site. Acoustic receiver locations (green) and tagging locations of tiger sharks (yellow) and Caribbean reef sharks (red) in proximity to New Providence and Exuma, The Bahamas. The size of circle indicates the number of sharks tagged in each location, ranging 1–10.
Detections of tiger sharks and Caribbean reef sharks were first corrected for receiver time drift in the program VUE (Vemco) and exported for further analysis with R (R Core Team, 2018) using the interface RStudio (RStudio Team, 2016). Prior to analysis, shark detections were filtered to remove potential false detections (Simpfendorfer et al., 2015), including those that occurred prior to tag deployment, repeated detections that occurred within less than the minimum tag transmission delay, and single detections that occurred within a 2-h time period (i.e., minimum lag filter). Detection data were then visually inspected to assess any potential animal mortalities / anomalies, resulting in tags not attached to the target animals being detected on acoustic receivers. This can result in consistent detections of a transmitter on a single receiver over extended periods of time, without being subsequently detected on any other receivers (Matley et al., 2020). Two tags that were implanted in Caribbean reef sharks had these characteristics, which we attributed to natural predation events from tiger sharks that occurred weeks after release, and as such we removed these from subsequent analyses due to potential unreliability (Appendix I; Figures 1, 2). Since shark detections were very low from May – November 2018, we began our formal analysis on receiver data from November 2018.
Figure 2. (A) Proportion of sharks tagged with acoustic transmitters within 50 km of study sites that were detected on acoustic receivers in New Providence Island and Exuma, The Bahamas in days since tagging (crosses indicate censored observations), (B) Predictor importance (mean decrease in accuracy) of shark detection probability. TLm, shark total length; mindistkm, distance from tagging location to the nearest acoustic receiver. (C) Marginal effects of shark species and total length on detection probability estimated with a random forests model, (D) Marginal effects of shark species and distance from tagging to the nearest receiver on detection probability estimated with a random forests model.
Because a considerable proportion of sharks tagged within proximity to the two study sites were undetected during the study period, the factors influencing detection probability were explored, with the data aggregated at the individual shark level. Only sharks that were tagged within 5 km of the study areas were included in this analysis, excluding one outlier, tagged 63 km from the nearest receiver at a location in between the two study sites. To address detection probability, a Cox proportional hazards model using the “survival” package (Therneau, 2015) was fit with detection date (the first date each individual shark was detected) as the response, and species, distance from the tagging site to the closest receiver, shark total length, and shark sex as predictors. Detection date was censored to the day when acoustic receivers were last downloaded. The final model structure was determined using backward model selection selecting the model with the lowest Akaike information criterion (AIC; Appendix I, Table 1). To further explore factors influencing detection probability, a random forests (RF) model (Breiman, 2001) was fit to detection presence (detected, undetected) with the same set of predictors. RF models use classification and regression trees to recursively apply binary partitions in the data with varied combinations of predictors to optimize prediction of the response (Breiman et al., 1984; De'ath and Fabricius, 2000). With RF, these trees are fit numerous times with randomly selected subsets of data and predictors to reduce overfitting and improve prediction accuracy (Breiman, 2001; Cutler et al., 2007). The RF model was run with 70:30% training: test data split with 10 iterations to generate estimated mean decrease in model accuracy (MDA) to assess predictor importance (Olden et al., 2008). The RF model was fit with the “randomForest” package (Liaw and Wiener, 2002) and the marginal effects (y) of each predictor (e.g., the predicted value holding other predictors constant) were calculated using the “pdp” package (Greenwell, 2017).
Table 1. Random forests model fit metrics for shark detection probability (Detected ~ species + total length + sex + minimum distance to receiver), as well as tiger shark and Caribbean reef shark presence/absence at acoustic telemetry receivers in New Providence Island and Exuma (Presence ~ season + lunar phase + habitat type + depth + temperature).
To examine shark connectivity and space use patterns, network analysis was used - a technique based in graph theory that treats discrete locations (acoustic receivers) as nodes connected by edges in a network to reveal ecologically relevant patterns (Jacoby et al., 2012; Jacoby and Freeman, 2016). Edges are also associated with weights that are defined by the number of intra- and inter-node detections (i.e., self-loops and movements between nodes). Prior to fitting networks to each species and study location, detection data were filtered to only include individuals with at least five detections and those with tracking durations > 1 day, resulting in 29 individuals (17 Caribbean reef sharks, 12 tiger sharks). Networks were fit using the “igraph” R package (Csardi and Nepusz, 2006). Network metrics were derived from directed movements (i.e., to- and from-edges) and included node degree (the sum number of ingoing and outgoing edges), path number (the sum number of edges), network density (the ratio of the number of edges and the number of possible edges, including nodes where individuals were never detected at but excluding self-loops), network diameter (the longest path length), average path length (shortest paths between all pairs of connected vertices), average betweenness (the number of shortest paths going through each node), which were aggregated at the species level. Additionally, node degree, node strength (the sum edge weights of the adjacent edges for each node), betweenness centrality (the number of shortest paths going through each node), and eigenvector centrality (calculated as the dominant eigenvector of the network and is equal to the weighted proportion of the total number of in- and out- paths at a given node; Newman, 2004; Stehfest et al., 2015) were summarized by habitat type (seagrass, sand, coral reef) which was defined based on visual observation of the seafloor at each receiver. To test whether shark movement networks were different from random - and hence - whether receiver array design was heavily biasing derived network metrics, a subset of shark network metrics (sum node degree, network paths, network density, and average betweenness) for each individual shark were compared to 1,000 randomized networks following the approach outlined in Novak et al. (2020). For all sharks and metrics tested, at least one or more network metrics were significantly different from random (p < 0.001), suggesting observed animal networks were non-random.
To explore the factors influencing the habitat use of both shark species, RF models were applied to daily shark presence/absence for each species at the acoustic receiver level with habitat type (seagrass, sand, coral reef), water depth, season, water temperature, and lunar phase as predictors, following Brownscombe et al. (2019). Separate models were fit to each shark species and receiver array (New Providence, Exuma). Data included in this analysis spanned from 2018-11-16 to 2020-02-21 when detection and corresponding environmental data were available. To reduce the potential for large sample sizes to influence variable importance and marginal effects assessment, the model was fit with 10-fold cross validation, using a single fold for each training iteration (n = 10), and the remaining 9 folds as test sets. Separate RF models were fit to each species and location separately. For all RF models, class prediction was balanced through iterative optimization to achieve optimal balance between positive and negative class accuracy, model sensitivity, specificity, and overall accuracy balance (Brownscombe et al., 2019). All data are reported as mean ± 95% confidence interval unless otherwise specified.
The New Providence Island (NPI) and Exuma (EX) receiver arrays jointly recorded 96,750 filtered detections from 15 tiger sharks (NPI = 8, EX = 7; 270 ± 60 cm overall total length, 160–320 cm range, hereafter TS for analytical reporting) and 20 Caribbean reef sharks (NPI = 9, EX = 11; 170 ± 10 cm overall total length, 110 ± 220 cm range, hereafter CRS for analytical reporting) from 2018-05-02 to 2020-01-24, with a range of tracking periods amongst individuals (247 ± 220 days, 1–629 day range; 80 ± 83 detection days, 1–261 day range; Figure 1, see Supplementary Table 2). Detected sharks were almost entirely those tagged within proximity of the two study locations in The Bahamas, except for one individual Caribbean reef shark tagged in the central Exuma cays, ~63 km from the nearest receiver (Figure 1). This was the only shark in the dataset that made large (>20 km) movements during the monitoring period; no sharks tagged within the New Providence array were detected in the Exuma array, and vice versa.
Of the 61 sharks tagged within 5 km of the two study areas, 34 were detected on acoustic receivers. The final model of shark detection probability did not include shark total length, sex, or tagging distance, but there was a significant effect of shark species on detection probability (Cox proportional hazards; z = 4.0, p < 0.001; Figure 2A). Random forests also found species to be the most important predictor (18.0 [13.4–22.6]; mean [95%CI] MDA) of detection probability, and a positive, but unimportant influence of shark total length (2.0 [−2.7–6.7]) (Figure 2B; Table 1). Caribbean reef sharks had lower detection probabilities than tiger sharks, while relatively small Caribbean reef sharks, and relatively large tiger sharks were detected less often (Figure 2C). Distance from tagging to the nearest receiver showed no notable patterns (Figure 2D).
Caribbean reef sharks and tiger sharks exhibited highly variable movements between species, locations, and amongst individuals (Figure 3). Caribbean reef sharks visited few receiver locations relative to tiger sharks, which were more wide ranging in both New Providence Island and Exuma. This result was confirmed with network analysis, which showed tiger sharks had greater network connectedness across all metrics examined in both study locations (Figures 4A,B). This was reflected in a range of network metrics in New Providence Island (node degree: CRS: 4.0 [2.1–5.9], TS: 38 [19.3–56.7]; path number: CRS: 2.0 [1.0–3.0], TS: 19 [9.6–28.4]; network density: CRS: 0.01 [−0.0002–0.02], TS: 0.2 [0.1–0.4]; network diameter: CRS: 0.6 [−0.01–1.2], TS: 13 [6.0–20.0]; average path length: CRS: 0.4 [0.03–0.8], TS: 1.5 [1.2–1.7]; average betweenness: CRS: 0.0 [0.0–0.0], TS: 3.8 [0.5–7.1]). Network metrics followed a similar trend in Exuma (node degree: CRS: 4.8 [1.9–7.7], TS: 15 [0.4–29.6]; path number: CRS: 2.4 [1.0–3.9], TS: 7.5 [0.2–14.8]; network density: CRS: 0.05 [−0.002–0.09], TS: 0.3 [0.06–0.6]; network diameter: CRS: 4.6 [−1.2–10.4], TS: 4.7 [0.4–9.0]; average path length: CRS: 0.4 [0.007–0.8], TS: 0.7 [0.3–1.2]; average betweenness: CRS: 0.1 [−0.03–0.2], TS: 0.5 [−0.3–1.4]). Network differences were more pronounced in New Providence Island, because Caribbean reef sharks generally exhibited higher connectivity in Exuma, where there is more homogeneous habitat and the acoustic receiver array was also less expansive (Figures 3, 4; see Supplementary Tables 3, 4 for full network metric outputs). Amongst habitat types, in New Providence Island tiger sharks exhibited the highest connectivity (node degree, strength, and eigenvector centrality scores) in seagrass habitats, while Caribbean reef sharks showed greater connectivity in coral reefs, but also relatively high site fidelity and repeated visits to the same sites (node strength; Figure 4C). In contrast, our study sites in Exuma had little seagrass habitat available and hence no acoustic receiver coverage in this habitat type; yet tiger sharks still exhibited higher spatial connectivity in sand and coral reef habitats in terms of node degree and strength but not eigenvector centrality (Figure 4D). In Exuma, Caribbean reef sharks exhibited high node strength and eigenvector centrality, reflecting high site fidelity and repeated visits to the same sites (Figures 3, 4D). Overall, tiger sharks moved frequently between all habitat types, with movements most frequently to seagrass habitats in New Providence Island, where they were available. Caribbean reef sharks exhibited higher site fidelity and repeated visits to the same sites but were wider ranging in Exuma where the acoustic receiver array was smaller and was restricted to the outer bank of the coastline.
Figure 3. Map of detections of individual Caribbean reef sharks (red) and tiger sharks (yellow) at acoustic receivers (green) in (A) New Providence Island and (B) Exuma. Degree (circle size) indicates the number of locations visited (for individual sharks) or the number of sharks visited (for receivers). Edge thickness indicates the relative number of detections at each receiver. Shark locations reflect their mean position in the receiver array ± 0–0.5 degrees to reduce node overlap.
Figure 4. Network analysis metrics for the movements of Caribbean reef sharks (red) and tiger sharks (yellow) in (A) New Providence Island, (B) Exuma, and aggregated by habitat type in (C) New Providence Island and (D) Exuma. Node strength was log transformed, and error bars represent SEM.
Consistent with the variable movement patterns described above, Caribbean reef sharks and tiger sharks also exhibited different spatial and temporal patterns of habitat use (Figure 5). In New Providence Island, random forests models found water depth was the most important predictor of space use for Caribbean reef sharks (CRS: 28.2 [26.0–30.4]; mean [95%CI] MDA) and tiger sharks (TS: 35.3 [31.6–39.1]), followed by habitat type (CRS: 24.8 [19.5–30.0]; TS: 23.0 [20.9–25.1]), with moderate effects of season (CRS: 12.0 [7.8–16.1]; TS:6.1 [1.9–10.4]) and water temperature (CRS: 9.4 [4.9–13.8]; TS:13.0[6.9–19.0]), and no influence of lunar phase (CRS:1.6 [−0.8–4.02]; TS: −2.3 [−2.3–1.76]) (Table 1; Figure 5). In Exuma, the drivers of space use were similar in importance structure, with an important influence of water depth (CRS: 56.7 [50.9–62.5]; TS: 28.1 [23.8–32.4]) and habitat type (CRS: 16.8 [13.0–20.5]; TS: 20.1 [17.2–22.9]). There were more moderate effects of season (CRS: 12.7 [4.8–20.6]; TS: 7.0 [4.0–10.1]) and temperature (CRS: 3.2[−2.5–8.9]; TS: 9.1 [5.3–12.9]), and no significant influence of lunar phase (CRS: −0.9 [−6.1–4.3]; TS: 2.9 [−3.6–9.3]). The effect of water temperature was stronger on tiger sharks than Caribbean reef sharks, while the latter showed stronger seasonal variations in habitat use, detected more frequently in the spring and summer seasons (Figure 5). In New Providence Island, where seagrass habitats are abundantly available on the Great Bahamas Bank to the south, tiger sharks associated most frequently with receivers placed in shallow seagrass habitats, while Caribbean reef sharks associated most frequently with receivers with deeper-water reefs (Figure 5). However, in Exuma, where seagrass habitat is available on the western bank, but was not covered with our acoustic receivers in this study, Caribbean reef sharks occupied shallow water most frequently, and tiger sharks were more generalist amongst depths and habitats (Figure 5). The effects of water temperature, although low relative to habitat and depth, were different between study sites, with both species generally occupying relatively cooler water in New Providence Island, and warmer water in Exuma (Figure 5).
Figure 5. Random forests outputs for the presence/absence of Caribbean reef sharks (red) and tiger sharks (yellow) in New Providence (left) and Exuma (right), including variable importance scores (mean decrease accuracy; ± 95% CI; top) and marginal effects of predictors (predicted values holding other predictors constant). Confidence intervals were generated using 10-fold cross validation.
The conservation of coastal and semi-coastal shark species is a primary goal and intended outcome for establishing MPAs and facilitating their long-term persistence among island nations (Heupel et al., 2019; Gallagher et al., 2020). Few studies have investigated the behavior of reef-associated sharks in the Caribbean, and this study is the largest spatial and temporal evaluation into the movements of multiple shark species in The Bahamas shark MPA. We provide contrasting patterns of movement between Caribbean reef sharks and tiger sharks, the former exhibiting relatively low habitat connectivity and high residency. Tiger sharks, however, demonstrated wider roaming behavior across much greater space and are likely important vectors of ecosystem connectivity and nutrient transfer across subtropical seascapes in the study area. These findings have important implications for understanding the benefits of shark MPAs for The Bahamas, the Greater Caribbean, and for other island nations interested in shark conservation.
We found that species was the strongest predictor of detection probability after tagging, with some potential influence of body size. Smaller Caribbean reef sharks (i.e., < ~150 cm) and larger tiger sharks approaching size-at-maturity (i.e., > ~300 cm, Sulikowski et al., 2016) were less likely to be detected throughout our arrays. The distance at which animals were tagged from acoustic receivers also appeared to impact detection probability for Caribbean reef sharks, with a greater chance of detection for animals tagged closer to the array, mirroring size-based effects on residency for similar reef shark species in the Indo-Pacific (Chin et al., 2013). Despite the paucity of information on regional and local residency of reef sharks in the Greater Caribbean, they are known to have strong site fidelity across small spatial scales (e.g., Bond et al., 2012), which our findings support (at least for those reef sharks that were detected over time). While long-term movements of reef sharks outside of the current array designs cannot be discounted, it is likely that the overall lower detection probability of Caribbean reef sharks, relative to tiger sharks, was indeed associated with the lack of sufficient receiver coverage in areas where those individuals were tagged. This trend was not apparent in tiger sharks, suggesting that relatively smaller arrays can perform well for this species within certain geographic contexts (e.g., Nassau is centrally located in the Bahamas archipelago).
Some large sharks have been recently shown to induce strong changes in the behavior and distribution of prey species (Phenix et al., 2019; Lester et al., 2020), including smaller sharks (Shea et al., 2020), across large ocean landscapes. Predation risk of mortality from large tiger sharks may also be a potential driver of lower detection probability for Caribbean reef sharks, where tiger sharks use the entire area of the islands studied here (Figure 2), thus pushing smaller reef sharks into landscapes with greater potential for escape (Gallagher et al., 2017). Tiger sharks can exert top-down forcing on prey species (Heithaus et al., 2009), and preliminary data from “predation sensor” tags deployed by our team on a subset of smaller reef sharks (~150 cm total length) in the New Providence array revealed two inferred mortalities occurring roughly 2 weeks after release, both being detected on a receiver situated near reef habitat (A. Gallagher, Unpublished Data). We have also commonly observed evidence for large diameter bite marks (suggestive of tiger sharks) on reef sharks, and we have also observed several natural predation events on Caribbean reef sharks and other smaller Carcharhinids by tiger sharks throughout the sampling period, as well as evidence of depredation of two line-caught reef-sharks. Similarly, video monitoring has provided evidence for predation of experimentally captured Caribbean reef sharks by tiger sharks in proximate locations, such as Eleuthera (O'Shea et al., 2015). Thus, on the basis of these lines of evidence, we posit that smaller Caribbean reef sharks may comprise at least some proportion of tiger shark diets, particularly if they foray into “risky” habitats preferentially selected by tiger sharks (e.g., seagrass banks, Figure 5), thereby contributing to lower detection probabilities of tagged Caribbean reef sharks (e.g., Papastamatiou et al., 2006). Further investigation is required to empirically test this hypothesis, but it highlights the potential for acoustic telemetry to reveal new insights into potential predator-prey interactions and the functional role of large sharks (Hammerschlag, 2019), while offering a logical avenue for future work utilizing non-lethal tools such as isotopic analyses (Hussey et al., 2012; Shipley and Matich, 2020) and DNA metabarcoding (Berry et al., 2017). While sex appeared to be an important factor for detectability, we caution the interpretation of this result due to the lack of control resulting in bias in the sex of our tagged sharks.
Tiger sharks displayed more extensive connectivity and space use across receivers, although home range could not be defined here, with expansive and highly connected networks on both islands (Figure 3). This finding is not surprising as broadscale movements have been commonly documented in tiger sharks from The Bahamas and Greater Caribbean (e.g., Hammerschlag et al., 2012, 2015; Lea et al., 2015), and other locations worldwide (e.g., Fitzpatrick et al., 2012; Papastamatiou et al., 2013; Holmes et al., 2014; Afonso et al., 2017). Novel to this study, however, was the ability to infer habitat selection for tiger sharks, based on the results from Random Forests models (Figure 5). Within the New Providence array, where habitat is highly varied, tiger sharks frequented all habitat types but showed a strong preference for seagrass habitats (Figure 3), nearly double that of sand and reef habitats (Figure 5). This preference for shallower seagrass habitats was also corroborated by the strong effect of shallow depth in driving space use patterns (Figure 5).
Seagrass habitats are widely recognized as keystone ecosystems for their high productivity their role in promoting biodiversity and offering nursery sites as well as feeding grounds for a number of species of conservation interest, such as sirenians and green turtles (Hemminga and Duarte, 2000), and their intense capacity to sequester and store carbon in their sediments (Fourqurean et al., 2012; Duarte et al., 2013). The Great Bahama Bank, which was partially covered here by our study design, lies mostly in 5 m or less of water (Harris et al., 2015), is dominated by moderate and dense seagrass meadows (Thalassia testudinum; Garcia et al., 2020) which contribute up to 80% of the net primary productivity in the region (Dierssen et al., 2010). This area is also important for lobster and conch fisheries which are vital to the Bahamian economy and culture (Sherman et al., 2018). Whereas the importance of seagrass as habitat to tiger sharks is well-established from studies in the Indian Ocean (e.g., Heithaus et al., 2014), where tiger sharks have been shown to prefer shallow seagrass habitats for prey-searching and foraging activities (e.g., Heithaus et al., 2006; Andrzejaczek et al., 2019), the importance of the role of tiger sharks in maintaining the role of tropical seagrass meadows in supporting large blue carbon stocks has only been theoretically proposed (Atwood et al., 2015). Indeed, tiger sharks may function as sentinels for the conservation of keystone habitats serving as central nodes in foraging networks, as seen on insular shelves and reefs off Hawaii (Meyer et al., 2009, 2018). Nevertheless, current knowledge regarding the relationship between this apex predator and keystone habitats has been predominantly generated from studies in the Indian Ocean as well as the Pacific, thus leaving a gap in other ocean regions. Our data shed new light into tiger sharks and seagrass as a central node for their life history in the Atlantic Ocean, specifically the Great Bahama Bank where some of this study was conducted. From an ecological perspective, the high mobility of tiger sharks throughout multiple ecosystems and habitat types, as documented here, further solidifies the role of large apex predators as important vectors of ecosystem connectivity (Papastamatiou et al., 2015; Williams et al., 2018), transporting nutrients and exhibiting top-down control across ocean seascapes (Hammerschlag et al., 2019), with seagrass meadows as a central node in the network of connected habitats. This type of network-driven habitat use may be of value for future management seeking to conserve critical shark habitat using MPAs.
The diversity of movement patterns between species sharing the same island habitats support the notion that broader-scale measures such as large MPAs may actually provide the greatest overall biodiversity conservation benefits, although their efficacy is likely to be context dependent. For example, large shark MPAs are likely to be most effective in areas of high shark abundance where there are low levels of historical shark fishing mortality, as seen in The Bahamas and supported by work done on reef sharks living amongst atolls in the Pacific Ocean (White et al., 2017). Our data support the notion that no-take marine reserves are likely to be effective at protecting Caribbean reef sharks, corroborating results from a recent modeling approach which found that shark MPAs spanning at least 100 km would provide full protection for ~70% for this species (Dwyer et al., 2020). However, our results also suggest that small, well-designed patches will likely not be sufficient to capture the space use of all species, and that larger spatial measures will be needed to effectively tackle the greater issue of the loss of shark biodiversity, especially for nations with multiple, connected islands. The relatively low seasonality for tiger sharks suggests moderate to high residency or potential partial migration and contests the more highly migratory nature of tiger sharks that has been observed at other locations in The Bahamas, such as Grand Bahama, which is thought to serve reproductive purposes (Sulikowski et al., 2016). A parallel investigation into the long-range movements of tiger sharks in both arrays suggests that at least some individuals will embark on long distance migrations (A. Gallagher, Unpublished data), but they do not appear to be as common or wide-ranging than other subpopulations in the region (e.g., Hammerschlag et al., 2012; Graham et al., 2016). Mature individuals are present in our study regions, although less common than places like a known aggregation site, nicknamed “Tiger Beach,” off The Little Bahama Bank (Hammerschlag et al., 2017). As such, there may be fine-scale genetic underpinnings to these differential migration dynamics, as seen in other elasmobranchs (e.g., winter skate, Frisk et al., 2019), although this remains unknown. Caribbean reef sharks in the present study exhibited high site fidelity to relatively constricted regions of fringing coral reef habitat adjacent to the continental shelf drop off (Tongue of the Ocean in New Providence, Exuma Sound in the Exumas), which supports previous work on this species (Chapman et al., 2005; Shipley et al., 2018). The observed differences in space use, connectivity, and dispersal between Caribbean reef sharks and tiger sharks as described here, may in-part explain observed trends in relative abundances over the last 50 years. Through comparing catch data from scientific long-line surveys undertaken before and after MPA designation in 2011, Talwar et al. (2020) illustrated a relative increase in catch rates of Caribbean reef sharks, yet no change in catch rates for tiger sharks. Taken together, these findings may illustrate the disproportionate benefits of shark MPAs for highly resident species, compared to a lesser effect on more transient species that move beyond the Bahamas EEZ boundary (Howey-Jordan et al., 2013; Graham et al., 2016; Guttridge et al., 2017). Nevertheless, due to the diversity of behavioral types in both species described here (e.g., some individuals exhibit high site fidelity, whereas others may be roaming), local and regional scale measures such as MPAs and the shark sanctuary will have added conservation benefits such as promoting population resilience to future stressors.
While large MPAs and shark sanctuaries have been criticized in the past, these data shine new light on the notion that these large MPAs can effectively safeguard existing populations of reef-associated sharks, for which there is still a general lack of detailed information on their movement and habitat use worldwide. Indeed, the abundance of reef-associated sharks inferred via underwater camera surveys has been shown to be among the highest in nations where shark sanctuaries have been established (MacNeil et al., 2020). Therefore, the long-term conservation significance of these species-specific protected areas should not be overlooked or unfairly critiqued until there is a greater body of studies to reference. Indeed, shark MPAs, including shark sanctuaries, provide researchers arguably the greatest undisturbed opportunity to study the biology, life-history, and behavior of multiple shark species where few external stressors exist, which also providing a temporal buffer against harvest by which spatial conservation measures or even non-place-based management can be refined.
Our study provided an interannual, multi-species comparison of the space use, connectivity, and drivers of movement for two abundant shark species in the largest shark MPA in the Atlantic Ocean at two distinct sites. The information presented here suggests that The Bahamas shark sanctuary affords protection to reef sharks during critical life stages, such as juveniles and their growth into adults, and that large, protected areas provide substantial benefits to reef sharks in the Caribbean. Tiger sharks, in contrast, are more highly migratory and may spend some portion of time outside of The Bahamas EEZ. Given that there was no connectivity between our two arrays, no detections from these sharks on outside arrays (either in the Bahamas or United States) and high long-term site fidelity to tagging locations, our data suggest that large marine protected areas will most benefit tiger sharks on an island-to-island basis. Furthermore, our findings suggest that the effective conservation of Caribbean reef sharks in the region is therefore tied to the continued implementation and enforcement of the shark MPA in The Bahamas and is likely to further increase ecological and economic benefits to The Bahamas in future years. We also demonstrated important species-habitat linkages, particularly for linking tiger sharks and seagrass meadows in New Providence, a trend that probably holds for other areas of the Great Bahamas Bank, including other areas of the Exumas (Garcia et al., 2020). Although the ultimate functionality of tiger sharks on Bahamian ecosystems was beyond the scope of this study, the removal of this species from these habitats may have detrimental effects across many ecosystems as previously hypothesized (Heithaus et al., 2014). Large MPAs should play a pivotal role in pursuing the goal of restoring the abundance of marine life in our oceans by 2050 (Duarte et al., 2020). Large MPAs can effectively conserve resident and migratory sharks in the Greater Caribbean, including the network of habitats they use, and should likewise be a valuable tool in other countries bearing similar characteristics (Gallagher et al., 2020).
The raw data supporting the conclusions of this article will be made available by the authors, without undue reservation.
The animal study was conducted in accordance with the guidelines set by the Canadian Council on Animal Care under administration of the Carleton University Animal Care Committee.
AG conceived and facilitated all aspects of the study. AG, OS, MZ, NH, SKa, YP, BS, and SKe conducted the field work. JB, LG, BS, and AG analyzed the data. All authors wrote the manuscript and approved of the final version.
This work was supported by grants to BTW from the following funders: The Wanderlust Fund, The King Family, Sternlicht Family Foundation, Lush, Maverick1000, National Geographic Wild, Seaworld and Busch Gardens Conservation Fund, Thayer Academy, WCPD Foundation, and Anomaly Entertainment. These funders were not involved in the study design, collection, analysis, interpretation of data, the writing of this article or the decision to submit it for publication.
The authors declare that the research was conducted in the absence of any commercial or financial relationships that could be construed as a potential conflict of interest.
We thank our Bahamian partners and stakeholders who have enabled and supported this work: L. Gittens and the Dept. Marine Resources, E. Carey and S. Cant from Bahamas National Trust, S. Cove from Stuart Cove's, J. Todd, P. Nicholson, R. Sands from Grand Isle Resort, A. Phillips and A. Musgrove from Bahamas Dive Guides, Dive Exuma, and The Exuma Foundation. We are particularly grateful to the following partners for logistical and operational support: Stuart Cove's, The International Seakeepers Association, The Grand Isle Resort, GIR Bahamas, M/Y Marcato, J. and M. McClurg, Fleet Miami, R/V Sharkwater, Atlantis, Bahamas Dive Guides, Dive Exuma, The Exuma Foundation, Vemco, and the Ocean Tracking Network. For field work support, we thank J. Halvorsen, E. Staaterman, E. Quintero, J. Sternlicht, T. Gilbert, J. Roth, S. Moorhead, E. Sudal, and M. Adunni. This work was covered under a permit to AG from the Department of Marine Resources.
The Supplementary Material for this article can be found online at: https://www.frontiersin.org/articles/10.3389/fmars.2020.608848/full#supplementary-material
Afonso, A. S., Garla, R., and Hazin, F. H. (2017). Tiger sharks can connect equatorial habitats and fisheries across the Atlantic Ocean basin. PLoS ONE 12:e0184763. doi: 10.1371/journal.pone.0184763
Ali, L., Grey, E., Singh, D., Mohammed, A., Tripathi, V., Gobin, J., et al. (2020). An evaluation of the public's knowledge, attitudes and practices (KAP) in trinidad and tobago regarding sharks and shark consumption. PLoS ONE 15:e0234499. doi: 10.1371/journal.pone.0234499
Andrzejaczek, S., Chapple, T. K., Curnick, D. J., Carlisle, A. B., Castleton, M., Jacoby, D. M., et al. (2020). Individual variation in residency and regional movements of reef manta rays Mobula alfredi in a large marine protected area. Marine Ecol. Progr. Series 639, 137–153. doi: 10.3354/meps13270
Andrzejaczek, S., Gleiss, A. C., Lear, K. O., Pattiaratchi, C. B., Chapple, T. K., and Meekan, M. G. (2019). Biologging tags reveal links between fine-scale horizontal and vertical movement behaviors in tiger sharks (Galeocerdo cuvier). Front. Marine Sci. 6:229. doi: 10.3389/fmars.2019.00229
Atwood, T. B., Connolly, R. M., Ritchie, E. G., Lovelock, C. E., Heithaus, M. R., Hays, G. C., et al. (2015). Predators help protect carbon stocks in blue carbon ecosystems. Nat. Climate Change 5, 1038–1045. doi: 10.1038/nclimate2763
Bakker, J., Wangensteen, O. S., Chapman, D. D., Boussarie, G., Buddo, D., Guttridge, T. L., et al. (2017). Environmental DNA reveals tropical shark diversity in contrasting levels of anthropogenic impact. Sci. Rep. 7, 1–11. doi: 10.1038/s41598-017-17150-2
Bates, A. E., Cooke, R. S., Duncan, M. I., Edgar, G. J., Bruno, J. F., Benedetti-Cecchi, L., et al. (2019). Climate resilience in marine protected areas and the protection paradox. Biol. Conserv. 236, 305–314. doi: 10.1016/j.biocon.2019.05.005
Berry, T. E., Osterrieder, S. K., Murray, D. C., Coghlan, M. L., Richardson, A. J., Grealy, A. K., et al. (2017). DNA metabarcoding for diet analysis and biodiversity: a case study using the endangered Australian sea lion (Neophoca cinerea). Ecol. Evolution 7, 5435–5453. doi: 10.1002/ece3.3123
Birkmanis, C. A., Partridge, J. C., Simmons, L. W., Heupel, M. R., and Sequeira, A. M. (2020). Shark conservation hindered by lack of habitat protection. Global Ecol. Conserv. 21:e00862. doi: 10.1016/j.gecco.2019.e00862
Bond, M. E., Babcock, E. A., Pikitch, E. K., Abercrombie, D. L., Lamb, N. F., and Chapman, D. D. (2012). Reef sharks exhibit site-fidelity and higher relative abundance in marine reserves on the Mesoamerican Barrier Reef. PLoS ONE 7:e32983. doi: 10.1371/journal.pone.0032983
Breiman, L., Friedman, J., Stone, C. J., and Olshen, R. A. (1984). Classification and Regression Trees. Pacific Grove, CA: Wadsworth & Brooks.
Brooks, E. J., Sloman, K. A., Sims, D. W., and Danylchuk, A. J. (2011). Validating the use of baited remote underwater video surveys for assessing the diversity, distribution and abundance of sharks in the Bahamas. Endangered Species Res. 13, 231–243. doi: 10.3354/esr00331
Brownscombe, J. W., Griffin, L. P., Gagne, T. O., Haak, C. R., Cooke, S. J., Finn, J. T., et al. (2019). Environmental drivers of habitat use by a marine fish on a heterogeneous and dynamic reef flat. Marine Biol. 166:18. doi: 10.1007/s00227-018-3464-2
Buchan, K. C. (2000). The Bahamas. Marine Pollut. Bull. 41, 94–111. doi: 10.1016/S0025-326X(00)00104-1
Chapman, D. D., Pikitch, E. K., Babcock, E., and Shivji, M. S. (2005). Marine reserve design and evaluation using automated acoustic telemetry: a case-study involving coral reef-associated sharks in the Mesoamerican Caribbean. Marine Technol. Soc. J. 39, 42–55. doi: 10.4031/002533205787521640
Chin, A., Tobin, A. J., Heupel, M. R., and Simpfendorfer, C. A. (2013). Population structure and residency patterns of the blacktip reef shark Carcharhinus melanopterus in turbid coastal environments. J. Fish Biol. 82, 1192–1210. doi: 10.1111/jfb.12057
Csardi, G., and Nepusz, T. (2006). The igraph software package for complex network research. InterJ. Complex Syst. 1695:1–9.
Cutler, D. R., Edwards Jr, T. C., Beard, K. H., Cutler, A., Hess, K. T., Gibson, J., et al. (2007). Random forests for classification in ecology. Ecology 88, 2783–2792. doi: 10.1890/07-0539.1
De'ath, G., and Fabricius, K. E. (2000). Classification and regression trees: a powerful yet simple technique for ecological data analysis. Ecology 81, 3178–3192. doi: 10.1890/0012-9658(2000)081[3178:CARTAP]2.0.CO;2
Dierssen, H. M., Zimmerman, R. C., Drake, L. A., and Burdige, D. (2010). Benthic ecology from space: optics and net primary production in seagrass and benthic algae across the Great Bahama Bank. Marine Ecol. Progr. Series 411, 1–15. doi: 10.3354/meps08665
Duarte, C. M., Agusti, S., Barbier, E., Britten, G. L., Castilla, J. C., Gattuso, J. P., et al. (2020). Rebuilding marine life. Nature 580, 39–51. doi: 10.1038/s41586-020-2146-7
Duarte, C. M., Losada, I. J., Hendriks, I. E., Mazarrasa, I., and Marbà, N. (2013). The role of coastal plant communities for climate change mitigation and adaptation. Nat. Climate Change 3, 961–968. doi: 10.1038/nclimate1970
Duce, S., Pressey, R. L., Simpfendorfer, C. A., Weeks, R., and Diedrich, A. (2019). Global opportunities and challenges for shark large marine protected areas. Biol. Conserv. 234, 107–115. doi: 10.1016/j.biocon.2019.03.026
Dwyer, R. G., Krueck, N. C., Udyawer, V., Heupel, M. R., Chapman, D., Pratt Jr, H. L., et al. (2020). Individual and population benefits of marine reserves for reef sharks. Curr. Biol. 30, 480–489. doi: 10.1016/j.cub.2019.12.005
Espinoza, M., Cappo, M., Heupel, M. R., Tobin, A. J., and Simpfendorfer, C. A. (2014). Quantifying shark distribution patterns and species-habitat associations: implications of marine park zoning. PLoS ONE 9:e106885. doi: 10.1371/journal.pone.0106885
Fitzpatrick, R., Thums, M., Bell, I., Meekan, M. G., Stevens, J. D., and Barnett, A. (2012). A comparison of the seasonal movements of tiger sharks and green turtles provides insight into their predator-prey relationship. PLoS ONE 7:e51927. doi: 10.1371/journal.pone.0051927
Fourqurean, J. W., Duarte, C. M., Kennedy, H., Marbà, N., Holmer, M., Mateo, M. A., et al. (2012). Seagrass ecosystems as a globally significant carbon stock. Nat. Geosci. 5, 505–509. doi: 10.1038/ngeo1477
Frisk, M. G., Shipley, O. N., Martinez, C. M., McKown, K. A., Zacharias, J. P., and Dunton, K. J. (2019). First observations of long-distance migration in a large skate species, the winter skate: implications for population connectivity, ecosystem dynamics, and management. Marine Coastal Fisher. 11, 202–212. doi: 10.1002/mcf2.10070
Gallagher, A. J., Amon, D. J., Bervoets, T., Shipley, O. N., Hammerschlag, N., and Sims, D. W. (2020). The Caribbean needs big marine protected areas. Science 367:749. doi: 10.1126/science.abb0650
Gallagher, A. J., Creel, S., Wilson, R. P., and Cooke, S. J. (2017). Energy landscapes and the landscape of fear. Trends Ecol. Evolut. 32, 88–96. doi: 10.1016/j.tree.2016.10.010
Gallagher, A. J., and Hammerschlag, N. (2011). Global shark currency: the distribution, frequency, and economic value of shark ecotourism. Curr. Issues Tourism 14, 797–812. doi: 10.1080/13683500.2011.585227
Gallagher, A. J., Serafy, J. E., Cooke, S. J., and Hammerschlag, N. (2014). Physiological stress response, reflex impairment, and survival of five sympatric shark species following experimental capture and release. Marine Ecol. Progr. Series 496, 207–218. doi: 10.3354/meps10490
Garcia, R. A., Lee, Z., Barnes, B. B., Hu, C., Dierssen, H. M., and Hochberg, E. J. (2020). Benthic classification and IOP retrievals in shallow water environments using MERIS imagery. Remote Sensing Environ. 249:112015. doi: 10.1016/j.rse.2020.112015
Graham, F., Rynne, P., Estevanez, M., Luo, J., Ault, J. S., and Hammerschlag, N. (2016). Use of marine protected areas and exclusive economic zones in the subtropical western North Atlantic Ocean by large highly mobile sharks. Diversity Distribut. 22, 534–546. doi: 10.1111/ddi.12425
Greenwell, B. (2017). pdp: an R package for constructing partial dependence plots. R J. 9, 421–436. doi: 10.32614/RJ-2017-016
Guttridge, T. L., Van Zinnicq Bergmann, M. P., Bolte, C., Howey, L. A., Finger, J. S., Kessel, S. T., et al. (2017). Philopatry and regional connectivity of the great hammerhead shark, Sphyrna mokarran in the US and Bahamas. Front. Marine Sci. 4:3. doi: 10.3389/fmars.2017.00003
Haas, A. R., Fedler, T., and Brooks, E. J. (2017). The contemporary economic value of elasmobranchs in The Bahamas: reaping the rewards of 25 years of stewardship and conservation. Biol. Conserv. 207, 55–63. doi: 10.1016/j.biocon.2017.01.007
Hammerschlag, N. (2019). Quantifying shark predation effects on prey: Dietary data limitations and study approaches. Endanger. Species Res. 38, 147–151. doi: 10.3354/esr00950
Hammerschlag, N., Broderick, A. C., Coker, J. W., Coyne, M. S., Dodd, M., Frick, M. G., et al. (2015). Evaluating the landscape of fear between apex predatory sharks and mobile sea turtles across a large dynamic seascape. Ecology 96:2117–2126. doi: 10.1890/14-2113.1
Hammerschlag, N., Gallagher, A. J., Wester, J., Luo, J., and Ault, J. S. (2012). Don't bite the hand that feeds: assessing ecological impacts of provisioning ecotourism on an apex marine predator. Funct. Ecol. 26, 567–576. doi: 10.1111/j.1365-2435.2012.01973.x
Hammerschlag, N., Gutowsky, L. F. G., Gallagher, A. J., Matich, P., and Cooke, S. J. (2017). Diel habitat use patterns of a marine apex predator (tiger shark, Galeocerdo cuvier) at a high use area exposed to dive tourism. J. Exp. Mar. Biol. Ecol. 495, 24–34. doi: 10.1016/j.jembe.2017.05.010
Hammerschlag, N., Schmitz, O. J., Flecker, A. S., Lafferty, K. D., Sih, A., Atwood, T. B., et al. (2019). Ecosystem function and services of aquatic predators in the Anthropocene. Trends Ecol. Evol. 34, 369–383. doi: 10.1016/j.tree.2019.01.005
Hanson, J. O., Rhodes, J. R., Butchart, S. H., Buchanan, G. M., Rondinini, C., Ficetola, G. F., et al. (2020). Global conservation of species' niches. Nature 580:232–234. doi: 10.1038/s41586-020-2138-7
Harris, P. M., Purkis, S. J., Ellis, J., Swart, P. K., and Reijmer, J. J. (2015). Mapping bathymetry and depositional facies on Great Bahama Bank. Sedimentology 62, 566–589. doi: 10.1111/sed.12159
Heithaus, M. R., Alcoverro, T., Arthur, R., Burkholder, D. A., Coates, K. A., Christianen, M. J., et al. (2014). Seagrasses in the age of sea turtle conservation and shark overfishing. Front. Marine Sci. 1:28. doi: 10.3389/fmars.2014.00028
Heithaus, M. R., Hamilton, I. M., Wirsing, A. J., and Dill, L. M. (2006). Validation of a randomization procedure to assess animal habitat preferences: microhabitat use of tiger sharks in a seagrass ecosystem. J. Animal Ecol. 75, 666–676. doi: 10.1111/j.1365-2656.2006.01087.x
Heithaus, M. R., Wirsing, A. J., Burkholder, D., Thomson, J., and Dill, L. M. (2009). Towards a predictive framework for predator risk effects: the interaction of landscape features and prey escape tactics. J. Animal Ecol. 78, 556–562. doi: 10.1111/j.1365-2656.2008.01512.x
Heupel, M. R., Papastamatiou, Y. P., Espinoza, M., Green, M. E., and Simpfendorfer, C. A. (2019). Reef shark science–key questions and future directions. Front. Marine Sci. 6:12. doi: 10.3389/fmars.2019.00012
Holmes, B. J., Pepperell, J. G., Griffiths, S. P., Jaine, F. R., Tibbetts, I. R., and Bennett, M. B. (2014). Tiger shark (Galeocerdo cuvier) movement patterns and habitat use determined by satellite tagging in eastern Australian waters. Mar. Biol. 161, 2645–2658. doi: 10.1007/s00227-014-2536-1
Howey-Jordan, L. A., Brooks, E. J., Abercrombie, D. L., Jordan, L. K., Brooks, A., Williams, S., et al. (2013). Complex movements, philopatry and expanded depth range of a severely threatened pelagic shark, the oceanic whitetip (Carcharhinus longimanus) in the western North Atlantic. PLoS ONE 8:e56588. doi: 10.1371/journal.pone.0056588
Hussey, N. E., Kessel, S. T., Aarestrup, K., Cooke, S. J., Cowley, P. D., Fisk, A. T., et al. (2015). Aquatic animal telemetry: a panoramic window into the underwater world. Science 348:6240. doi: 10.1126/science.1255642
Hussey, N. E., MacNeil, M. A., Olin, J. A., McMeans, B. C., Kinney, M. J., Chapman, D. D., et al. (2012). Stable isotopes and elasmobranchs: tissue types, methods, applications and assumptions. J. Fish Biol. 80, 1449–1484. doi: 10.1111/j.1095-8649.2012.03251.x
Jacoby, D. M., Brooks, E. J., Croft, D. P., and Sims, D. W. (2012). Developing a deeper understanding of animal movements and spatial dynamics through novel application of network analyses. Methods Ecol. Evolut. 3, 574–583. doi: 10.1111/j.2041-210X.2012.00187.x
Jacoby, D. M., Ferretti, F., Freeman, R., Carlisle, A. B., Chapple, T. K., Curnick, D. J., et al. (2020). Shark movement strategies influence poaching risk and can guide enforcement decisions in a large, remote Marine protected area. J. Appl. Ecol. 57, 1782–1792. doi: 10.1111/1365-2664.13654
Jacoby, D. M., and Freeman, R. (2016). Emerging network-based tools in movement ecology. Trends Ecol. Evol. 31, 301–314. doi: 10.1016/j.tree.2016.01.011
Kessel, S. T., Cooke, S. J., Heupel, M. R., Hussey, N. E., Simpfendorfer, C. A., Vagle, S., et al. (2014). A review of detection range testing in aquatic passive acoustic telemetry studies. Rev. Fish Biol. Fish. 24, 199–218. doi: 10.1007/s11160-013-9328-4
Knip, D. M., Heupel, M. R., and Simpfendorfer, C. A. (2012). Evaluating marine protected areas for the conservation of tropical coastal sharks. Biol. Conserv. 148:200–209. doi: 10.1016/j.biocon.2012.01.008
Lea, J. S., Humphries, N. E., von Brandis, R. G., Clarke, C. R., and Sims, D. W. (2016). Acoustic telemetry and network analysis reveal the space use of multiple reef predators and enhance marine protected area design. Proc. R. Soc. B: Biol. Sci. 283:20160717. doi: 10.1098/rspb.2016.0717
Lea, J. S., Wetherbee, B. M., Queiroz, N., Burnie, N., Aming, C., Sousa, L. L., et al. (2015). Repeated, long-distance migrations by a philopatric predator targeting highly contrasting ecosystems. Sci. Rep. 5:11202. doi: 10.1038/srep11202
Lester, E. K., Langlois, T. J., Simpson, S. D., McCormick, M. I., and Meekan, M. G. (2020). The hemisphere of fear: the presence of sharks influences the three dimensional behaviour of large mesopredators in a coral reef ecosystem. Oikos 129, 731–739. doi: 10.1111/oik.06844
MacKeracher, T., Diedrich, A., and Simpfendorfer, C. A. (2019). Sharks, rays and marine protected areas: a critical evaluation of current perspectives. Fish Fisher. 20, 255–267. doi: 10.1111/faf.12337
MacNeil, M. A., Chapman, D. D., Heupel, M., Simpfendorfer, C. A., Heithaus, M., Meekan, M., et al. (2020). Global status and conservation potential of reef sharks. Nature 583, 801–806. doi: 10.1038/s41586-020-2519-y
Maestro, M., Pérez-Cayeiro, M. L., Chica-Ruiz, J. A., and Reyes, H. (2019). Marine protected areas in the 21st century: current situation and trends. Ocean Coast. Manag 171, 28–36. doi: 10.1016/j.ocecoaman.2019.01.008
Maljković, A., and Côté, I. M. (2011). Effects of tourism-related provisioning on the trophic signatures and movement patterns of an apex predator, the Caribbean reef shark. Biol. Conserv. 144, 859–865. doi: 10.1016/j.biocon.2010.11.019
Marine Conservation Institute (2016). MPAtlas [Online]. Seattle, WA: Marine Conservation Institute. Available online at: www.mpatlas.org (accessed June 1, 2020).
Matley, J. K., Faust, M. D., Raby, G. D., Zhao, Y., Robinson, J., MacDougall, T., et al. (2020). Seasonal habitat-use differences among Lake Erie's walleye stocks. J. Great Lakes Res. 46, 609–621. doi: 10.1016/j.jglr.2020.03.014
McClure, E. C., Sievers, K. T., Abesamis, R. A., Hoey, A. S., Alcala, A. C., and Russ, G. R. (2020). Higher fish biomass inside than outside marine protected areas despite typhoon impacts in a complex reefscape. Biol. Conserv. 241:108354. doi: 10.1016/j.biocon.2019.108354
Meyer, C. G., Anderson, J. M., Coffey, D. M., Hutchinson, M. R., Royer, M. A., and Holland, K. N. (2018). Habitat geography around Hawaii's oceanic islands influences tiger shark (Galeocerdo cuvier) spatial behaviour and shark bite risk at ocean recreation sites. Sci. Rep. 8:4945. doi: 10.1038/s41598-018-23006-0
Meyer, C. G., Clark, T. B., Papastamatiou, Y. P., Whitney, N. M., and Holland, K. N. (2009). Long-term movement patterns of tiger sharks Galeocerdo cuvier in Hawaii. Mar. Ecol. Prog. Ser. 381, 223–235. doi: 10.3354/meps07951
Newman, M. E. (2004). Analysis of weighted networks. Phys. Rev. E 70:056131. doi: 10.1103/PhysRevE.70.056131
Novak, A. J., Becker, S. L., Finn, J. T., Danylchuk, A. J., Pollock, C. G., Hillis-Starr, Z., et al. (2020). Inferring residency and movement patterns of horse-eye jack Caranx latus in relation to a Caribbean marine protected area acoustic telemetry array. Animal Biotelemetry 8, 1–13. doi: 10.1186/s40317-020-00199-8
Olden, J. D., Lawler, J. J., and Poff, N. L. (2008). Machine learning methods without tears: a primer for ecologists. Q. Rev. Biol 83, 171–193. doi: 10.1086/587826
O'Shea, O. R., Mandelman, J., Talwar, B., and Brooks, E. J. (2015). Novel observations of an opportunistic predation event by four apex predatory sharks. Mar. Freshw. Behav. Physiol. 48, 374–380. doi: 10.1080/10236244.2015.1054097
Papastamatiou, Y. P., Meyer, C., Carlvaho, F., Dale, J., Hutchinson, M, and Holland, K. (2013). Telemetry and random walk models reveal complex patterns of partial migration in a marine predator. Ecology. 94, 2595–2606. doi: 10.1890/12-2014.1
Papastamatiou, Y. P., Meyer, C. G., Kosaki, R. K., Wallsgrove, N. J., and Popp, B. N. (2015). Movements and foraging of predators associated with mesophotic coral reefs and their potential for linking ecological habitats. Mar. Ecol. Prog. Ser. 521, 155–170. doi: 10.3354/meps11110
Papastamatiou, Y. P., Wetherbee, B. M., Lowe, C. G., and Crow, G. L. (2006). Distribution and diet of four species of carcharhinid shark in the Hawaiian Islands: evidence for resource partitioning and competitive exclusion. Mar. Ecol. Prog. Ser. 320, 239–251. doi: 10.3354/meps320239
Phenix, L. M., Tricarico, D., Quintero, E., Bond, M. E., Brandl, S. J., and Gallagher, A. J. (2019). Evaluating the effects of large marine predators on mobile prey behavior across subtropical reef ecosystems. Ecol. Evol. 9, 13740–13751. doi: 10.1002/ece3.5784
Roberts, C. M., McClean, C. J., Veron, J. E., Hawkins, J. P., Allen, G. R., McAllister, D. E., et al. (2002). Marine biodiversity hotspots and conservation priorities for tropical reefs. Science 295, 1280–1284. doi: 10.1126/science.1067728
Shea, B. D., Benson, C. W., de Silva, C., Donovan, D., Romeiro, J., Bond, M. E., et al. (2020). Effects of exposure to large sharks on the abundance and behavior of mobile prey fishes along a temperate coastal gradient. PLoS ONE 15:e0230308. doi: 10.1371/journal.pone.0230308
Sherman, K. D., Shultz, A. D., Dahlgren, C. P., Thomas, C., Brooks, E., Brooks, A., et al. (2018). Contemporary and emerging fisheries in The Bahamas—conservation and management challenges, achievements and future directions. Fish. Manag. Ecol. 25, 319–331. doi: 10.1111/fme.12299
Shipley, O. N., Brownscombe, J. W., Danylchuk, A. J., Cooke, S. J., O'Shea, O. R., and Brooks, E. J. (2018). Fine-scale movement and activity patterns of Caribbean reef sharks (Carcharhinus perezi) in the Bahamas. Environ. Biol. Fishes. 101, 1097–1104. doi: 10.1007/s10641-017-0656-4
Shipley, O. N., Howey, L. A., Tolentino, E. R., Jordan, L. K., Ruppert, J. L., and Brooks, E. J. (2017). Horizontal and vertical movements of Caribbean reef sharks (Carcharhinus perezi): conservation implications of limited migration in a marine sanctuary. R. Soc. Open Sci. 4:160611. doi: 10.1098/rsos.160611
Shipley, O. N., and Matich, P. (2020). Studying animal niches using bulk stable isotope ratios: an updated synthesis. Oecologia 193, 27–51. doi: 10.1007/s00442-020-04654-4
Simpfendorfer, C. A., Huveneers, C., Steckenreuter, A., Tattersall, K., Hoenner, X., Harcourt, R., et al. (2015). Ghosts in the data: false detections in VEMCO pulse position modulation acoustic telemetry monitoring equipment. Animal Biotelemetry 3:55. doi: 10.1186/s40317-015-0094-z
Stehfest, K. M., Patterson, T. A., Barnett, A., and Semmens, J. M. (2015). Markov models and network analysis reveal sex-specific differences in the space-use of a coastal apex predator. Oikos 124, 307–318. doi: 10.1111/oik.01429
Sulikowski, J. A., Wheeler, C. R., Gallagher, A. J., Prohaska, B. K., Langan, J. A., and Hammerschlag, N. (2016). Seasonal and life-stage variation in the reproductive ecology of a marine apex predator, the tiger shark Galeocerdo cuvier, at a protected female-dominated site. Aquat. Biol. 24, 175–184. doi: 10.3354/ab00648
Talwar, B. S., Stein, J. A., Connett, S. M., Liss, S. A., and Brooks, E. J. (2020). Results of a fishery-independent longline survey targeting coastal sharks in the eastern Bahamas between 1979 and 2013. Fish. Res. 230:105683. doi: 10.1016/j.fishres.2020.105683
Therneau, T. (2015). A Package for Survival Analysis in S. Available online at: https://CRAN.R-project.org/package=survival.
Ward-Paige, C. A. (2017). A global overview of shark sanctuary regulations and their impact on shark fisheries. Marine Policy 82, 87–97. doi: 10.1016/j.marpol.2017.05.004
Ward-Paige, C. A., Mora, C., Lotze, H. K., Pattengill-Semmens, C., McClenachan, L., Arias-Castro, E., et al. (2010). Large-scale absence of sharks on reefs in the greater-Caribbean: a footprint of human pressures. PLoS ONE 5:e11968. doi: 10.1371/journal.pone.0011968
Ward-Paige, C. A., and Worm, B. (2017). Global evaluation of shark sanctuaries. Global Environ. Change 47, 174–189. doi: 10.1016/j.gloenvcha.2017.09.005
White, T. D., Carlisle, A. B., Kroodsma, D. A., Block, B. A., Casagrandi, R., De Leo, G. A., et al. (2017). Assessing the effectiveness of a large marine protected area for reef shark conservation. Biol. Conserv. 207, 64–71. doi: 10.1016/j.biocon.2017.01.009
Williams, J. J., Papastamatiou, Y. P., Caselle, J. E., Bradley, D., and Jacoby, D. M. (2018). Mobile marine predators: an understudied source of nutrients to coral reefs in an unfished atoll. Proc. R. Soc. B: Biol. Sci. 285:20172456. doi: 10.1098/rspb.2017.2456
Keywords: shark, acoustic telemetry, marine protected area, MPA, seagrass, coral reef, Bahamas
Citation: Gallagher AJ, Shipley ON, van Zinnicq Bergmann MPM, Brownscombe JW, Dahlgren CP, Frisk MG, Griffin LP, Hammerschlag N, Kattan S, Papastamatiou YP, Shea BD, Kessel ST and Duarte CM (2021) Spatial Connectivity and Drivers of Shark Habitat Use Within a Large Marine Protected Area in the Caribbean, The Bahamas Shark Sanctuary. Front. Mar. Sci. 7:608848. doi: 10.3389/fmars.2020.608848
Received: 21 September 2020; Accepted: 18 December 2020;
Published: 27 January 2021.
Edited by:
Alastair Martin Mitri Baylis, South Atlantic Environmental Research Institute, Falkland IslandsReviewed by:
Luis Cardona, University of Barcelona, SpainCopyright © 2021 Gallagher, Shipley, van Zinnicq Bergmann, Brownscombe, Dahlgren, Frisk, Griffin, Hammerschlag, Kattan, Papastamatiou, Shea, Kessel and Duarte. This is an open-access article distributed under the terms of the Creative Commons Attribution License (CC BY). The use, distribution or reproduction in other forums is permitted, provided the original author(s) and the copyright owner(s) are credited and that the original publication in this journal is cited, in accordance with accepted academic practice. No use, distribution or reproduction is permitted which does not comply with these terms.
*Correspondence: Austin J. Gallagher, YXVzdGluQGJlbmVhdGh0aGV3YXZlcy5vcmc=
Disclaimer: All claims expressed in this article are solely those of the authors and do not necessarily represent those of their affiliated organizations, or those of the publisher, the editors and the reviewers. Any product that may be evaluated in this article or claim that may be made by its manufacturer is not guaranteed or endorsed by the publisher.
Research integrity at Frontiers
Learn more about the work of our research integrity team to safeguard the quality of each article we publish.