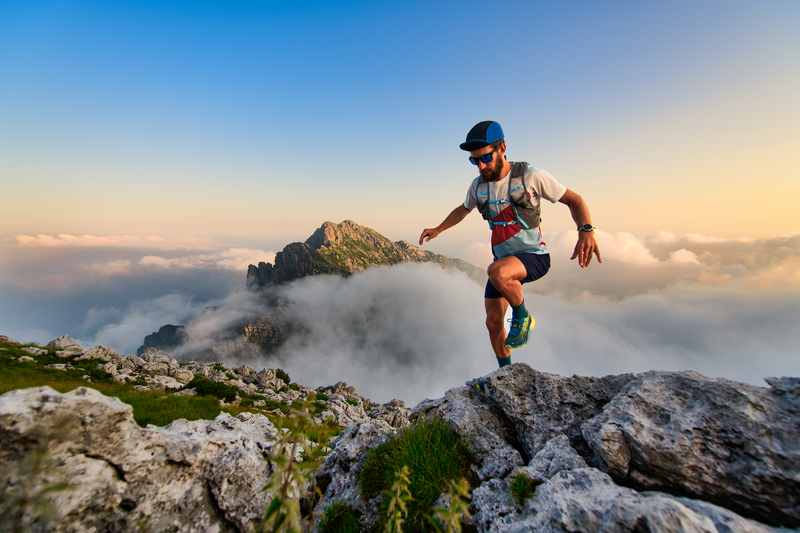
94% of researchers rate our articles as excellent or good
Learn more about the work of our research integrity team to safeguard the quality of each article we publish.
Find out more
ORIGINAL RESEARCH article
Front. Mar. Sci. , 15 December 2020
Sec. Marine Biology
Volume 7 - 2020 | https://doi.org/10.3389/fmars.2020.597899
Increasing green house gas emissions are expected to raise surface seawater temperatures and lead to locally intensified ocean acidity in the U.S. Pacific Northwest. Pacific herring (Clupea pallasi) are ecologically and economically important forage fish species native to this region. While the impacts of ocean acidification and ocean warming on organism physiology have been extensively studied, less is known on how concurrent climate change stressors will affect marine fish. Therefore, our study focused on the combined effects of ocean acidification and warming on Pacific herring early life history stages. Pacific herring embryos were incubated under a factorial design of two temperature (10°C or 16°C) and two pCO2 (600 μatm or 1200 μatm) treatments from fertilization until hatch (6 to 15 days depending on temperature). Elevated pCO2 was associated with a small increase in embryo mortality. Elevated temperature, as a single stressor, generated greater embryo mortality and embryo heart rates, larger yolk areas upon hatch, lower hatching success, and shorter larval lengths; compared with the same parameters measured under ambient temperature. The interaction of elevated temperature and pCO2 was associated with greater embryo heart rates and yolk areas compared to ambient conditions. This study suggests that while temperature is the primary global change stressor affecting Pacific herring embryology, interaction effects with pCO2 could introduce additional physiological challenges.
Pacific herring are key prey for ecologically and commercially important finfish, sea birds and marine mammals in the U.S. Pacific Northwest (PNW) (Fresh et al., 1981), and their eggs are a lipid-rich resource for many marine invertebrate and vertebrate foragers (Penttila, 2007). Herring spawn in shallow, vegetated habitats (Penttila, 2007), where developing embryos may be exposed to more extreme abiotic variability than in offshore habitats. Pacific herring, which spawn in distinct, local populations that can differ in genetic structure (Stick et al., 2014), are declining across much of their PNW range (Schweigert et al., 2010; Shelton et al., 2014; Thompson et al., 2017). This is especially notable in Puget Sound, the southern half of the Salish Sea in the PNW (Stick et al., 2014), where some populations in this region are declining by as much as 97% from their historical highs (Francis and Lowry, 2018; Sandell et al., 2019). Forage fish are known for showing high interannual variability in spawning population recruitment (Schweigert, 1994; Williams and Quinn, 2000), but what is causing the long-term trend of decline in herring populations located in the PNW is likely a complex amalgamation of factors that include, but are not limited to, trophic interactions, maternal fitness, human over-exploitation, and climate variability and change (Houde, 2008; Siple et al., 2018).
In coastal waters, variable environmental conditions may lead to early or more extreme exposure of organisms to high temperature or elevated pCO2 waters (Feely et al., 2008; Cai, 2011; IPCC, 2019), leading to reduced organismal fitness. However, organisms in these systems may also have evolved greater tolerance to a wider range of conditions. Attempts to gain an understanding about the effects of higher temperatures and elevated pCO2 on coastal marine species have been under investigation for some time (Doney et al., 2012; Harvey et al., 2013), and the effects of these climate variables are context and species-dependent (Harvey et al., 2013). The influence that these climate stressors will have on marine fish are beginning to be understood (e.g., Munday et al., 2009a; Hurst et al., 2013; Sswat et al., 2018), but to date little is known about the effects of elevated pCO2 on Pacific herring (Clupea pallasii), especially the combined effects of temperature and pCO2 on their early life history stages. Stressors such as climate change and habitat loss can have strong effects on early life stages, and loss of fitness or increased mortality in these stages can have serious impacts on population trends.
Within the context of climate change, it is well known that temperature affects the development and survival of marine teleosts at early developmental stages. For instance, shortened time to hatching is one common effect of increased temperature (Pankhurst and Munday, 2011). Abnormalities in the formation of pectoral fins and spinal cords were associated with temperature increases in Atlantic and Pacific herring (Purcell et al., 1990; Johnston et al., 1998; Dinnel et al., 2007; Sswat et al., 2018). These fin and spinal abnormalities may affect the growth and survival of later stages through diminished swimming performance, leading to greater mortality from an inability to capture prey or avoid predators (Vieira and Johnston, 1992; Johnston, 1993; Johansen and Jones, 2011; Kawakami et al., 2011).
Under elevated pCO2 adult marine fish are often resilient, having fully developed gills, they have effective ion exchange and acid-base regulation to prevent acidosis (Deigweiher et al., 2008). Early life stages of marine fish are hypothesized to be more susceptible to increases in pCO2 as they have not yet developed these mechanisms to mitigate the effects of hypercapnia (Kikkawa et al., 2003; Ishimatsu et al., 2008). Poor ion exchange could lead to shifting energy allocations, respiration sensitivities, and metabolic depressions (Deigweiher et al., 2010). Early life stage responses to elevated pCO2 include mortality (Baumann et al., 2011), loss of sensory ability (Munday et al., 2009b), changes in behavior (Dixson et al., 2010; Chung et al., 2014), and, at the molecular level, plasticity in gene regulation of metabolic processes (Tseng et al., 2013). Mechanisms driving these effects may include increased cost of osmoregulation (Kreiss et al., 2015) and changes in biochemical pathways, and metabolic rates (Dahlke et al., 2017).
In order to better predict the effects of climate change on coastal fish, the range of temperature and pCO2 exposures in experiments should be established in the context of the water chemistry associated with their environments. Predictions for surface seawater for open ocean systems indicate an increase in pCO2 from about 400 to 1,000 μatm (decrease from pH 8.1 to pH 7.7) by the year 2100, driven primarily by equilibrium of atmospheric CO2 with seawater (Caldeira and Wickett, 2003; IPCC, 2019, RCP 8.5). In coastal ecosystems of the U.S. Pacific Northwest, factors such as high photosynthesis and community respiration, eutrophication, vertical mixing associated with tidal exchange over complex bottom topography, and coastal upwelling amplify pCO2 fluctuations, bringing the high pCO2 excursions currently observed well beyond predicted levels for the end of this century over short time scales (Feely et al., 2008, 2010; Sunda and Cai, 2012; Duarte et al., 2013; Pacella et al., 2018). Ocean acidity extreme events such as these, are also predicted to increase dramatically, as overall variability in conditions and baseline acidity increases as anthropogenic CO2 is taken up into the global oceans (Burger et al., 2020).
Models and measurements on the regional scale can also provide some important context. A recent biogeochemical and hydrodynamic model predicted bottom water pH in the Salish Sea will decrease between 0.1 and 0.3 units depending on season and location under the RCP 8.5 emissions scenario (Khangaonkar et al., 2018). An in situ sensor deployed in the nearshore at Cherry Point, WA, the herring-spawning site from which fish in this study were collected, recorded a mean pH of 8.1 and 8.0 during May and June of 2019 (Department of Natural Resources (DNR), 2019). These means are consistent with open ocean values and with atmospheric equilibrium, however, the standard deviation of pH in June 2019 was 0.39 and daily low pH readings regularly fell below 7.7 during peak spawning periods. This suggests pCO2 close to 1,000 μatm is present during low pH periods of daily fluctuations under current conditions at Cherry Point.
Temperature varies seasonally across the spawning season for Pacific herring, which peaks in March for some populations in the Salish Sea and as late as June for the Cherry Point population (Sandell et al., 2019). Modeling results indicate that temperatures in the Salish Sea are between 6–8°C in March and 10–15°C in June and that overall seawater temperatures can be expected to increase by 1.5°C under the RCP 8.5 emission scenario (Khangaonkar et al., 2018). However, these changes may be larger in nearshore areas with temperature predictions increasing up to 4°C, depending on the proximity to freshwater inputs (Khangaonkar et al., 2018). If peak flows of the Fraser River in June were reduced, or the river temperature increased, then seawater temperatures at Cherry Point could increase markedly (Khangaonkar et al., 2018). In 2019, an in situ sensor package at Cherry Point in an eelgrass meadow recorded a mean temperature of 15.5°C during June and just over 14°C in May. Standard deviation of temperature measurements was about 2.5°C during both months, resulting primarily from substantial diurnal fluctuations (Department of Natural Resources (DNR), 2019).
As both temperature and pCO2 are expected to increase in concert, investigation of multiple stressor interactions on teleost early life stages is necessary. Interacting temperature and pCO2 may cause additive, synergistic, or antagonistic effects on a range of physiological factors depending on fish life stage and species (Pimentel et al., 2014; Dahlke et al., 2017; Sswat et al., 2018). Individual factors were also investigated, as temperature and CO2 do not always vary in concert with each other. For instance, upwelling events bring cold, CO2 rich water to the surface and as sea surface temperatures increase during summer months after upwelling events occur. Our study was designed to assess the effects that elevated temperature and pCO2 have on Pacific herring embryo development. While we may expect developmental differences to occur under temperature stress, the interaction of temperature and pCO2 may provide a more representative scenario of developmental impacts under multiple environmental stressors; which may reveal significant reductions in viable Pacific herring embryos and larvae that were not observable under single environmental stressors. Overall, the potential for reduced embryo survival and hatching success will limit the population growth of this critical forage fish species in the PNW. Given that negative effects from high temperature are well established and effects of high pCO2 are not, Pacific herring embryos were reared in seawater under two pCO2 conditions and two temperatures to determine if high pCO2 alone, or when combined high temperature would result in greater mortality and other sub-lethal physiological outcomes in embryonic herring than temperature stress alone.
All experimental procedures were carried out in accordance with the recommendations and approval of the Animal Care and Use Committee at Western Washington University (protocol approval 16-004). Pacific herring spawn in the Puget Sound region from early January through late June, depending on the stock. The Washington Department of Fish and Wildlife collected adult Pacific herring via gill netting from Cherry Point, Puget Sound, WA in May 2017. Male and female gonads were dissected in the field, placed on ice, and transported on the same day to the Shannon Point Marine Center (SPMC) in Anacortes, WA, where fertilization occurred.
Upon same day arrival to the SPMC, ova from a female gonad were lifted using a micro-spatula and dispersed in a single layer onto groups of four glass microscope slides (25 × 75 × 0.1 mm) under treatment combinations. Each slide had an average of 72 eggs and while some egg clusters were present this is a natural occurrence in the field during spawning season. Testis samples (∼1 cm3) from four males were combined and macerated in one of four 200 mL bowls at the 4 treatment conditions. A 1 mL aliquot of the homogenized sperm suspension was used to fertilize each egg slide. After 10 min of gamete contact, slides were gently rinsed with their respective treatment water to remove sperm and ovarian remnants. Each slide with eggs from a single female, was then transferred into a 200 mL glass bowl filled with treatment water and covered with fine mesh to allow water circulation and prevent escapement. This process was repeated for each of the other three female gonads. Following fertilization, slides were photographed to determine initial counts of ova and assess fertilization (Stereomicroscope: Olympus SZ40, 6.7× magnification; Camera: Nikon DSLR D3300 55 mm lens). The presence of a raised membrane around the embryo was recorded as an indication of successful fertilization. Bowls were then distributed into their respective treatment basins, each basin receiving a bowl with eggs from each of the 4 females.
Embryos were incubated in a triplicated 2 × 2 factorial framework consisting of two water temperatures (10°C, 16°C), and two pCO2 treatments (∼600 μatm, pH 7.8; ∼1200 μatm, pH 7.5). The temperature range in this study (10–16°C) represents a reasonable range for spawning temperatures currently present in the Salish Sea. The high temperature of 16°C is only slightly higher than the average temperature recorded last year during probable spawning periods at Cherry Point, and the lower temperature is representative of more southerly sites, which may experience temperature increases in the future. The pH difference of about 0.3 tested here is similar to the higher end of predictions for the Salish Sea (Khangaonkar et al., 2018). Fluctuations currently bring pH regularly to 7.7 in nearshore waters (Feely et al., 2010). The low pH of 7.6 used in this study, while lower than typical pH conditions found currently, will likely be present in bottom waters in the Salish Sea by the year 2100 (Khangaonkar et al., 2018). It is also likely to be common during low pH periods of nearshore fluctuations given that it is even now sometimes reached during these fluctuations (DNR). Therefore, the pCO2 and temperature levels chosen for our study are appropriate in this context, and the high treatments are reasonable to establish the existence of physiological effects of high pCO2 when combined with temperature stress.
A header tank (170 L) received filtered seawater drawn from the Salish Sea. Seawater was then gravity fed into twelve, 40 L mixing tanks at 4 L min–1. Within each mixing tank, submersible power head pumps circulated the seawater. Six pumps continuously received food grade CO2 gas (40 mL min–1), which was regulated by an 8 channel Masterflex® L/S Digital Drive peristaltic pump. Gas was fed directly into the intakes of the power head pumps, where the gas stream was broken into fine bubbles that quickly dissolved into the water (Jokiel et al., 2014). Water flowed from the mixing tanks into treatment basins (n = 3 basins per treatment x 4 treatments = 12 total basins) by gravity through tubing restricted to 1/16” ID at the lower end. The basin volume (11 L) and water flow rate into the basins (3.6 mL s–1) allowed a residence time (50 min) that permitted heaters (Aqueon® 100 W Submersible Aquarium Heater) to raise the ambient seawater temperature (10°C) to 16°C in half of the basins. Water circulation pumps (Hydor Koralia Nano 240) ensured uniform temperatures within all the basins. Standpipes within the basins maintained sufficient water depth and allowed excess seawater to flow to waste. A single header tank and individual mixing tanks for each basin reduced pseudoreplication (Cornwall and Hurd, 2015). Four glass bowls fitted with mesh lids containing the developing embryos were submerged in each basin. It is possible that gradients could develop across the mesh lids or within the bowls, but water circulation was vigorous in the basins, and bowls were refreshed with new water daily during data collection.
Water chemistry was monitored throughout the study. Daily temperature and pH values were recorded using a hand-held, Orion StarTM A329 pH conductivity meter calibrated with NBS-buffers at 10°C. Basin seawater samples were drawn on days 2, 7, and 12 for carbonate chemistry analyses. Samples were passed through a 0.6 μm glass fiber filter before filling 20 mL scintillation vials with no headspace for duplicate pH and duplicate total dissolved inorganic carbon (DIC) samples. Samples were preserved with 10 μL of mercuric chloride (HgCl2) and refrigerated at 2°C before being analyzed with a DIC analyzer (Apollo SciTech AS-C3) a week after collection. Room temperature and sample salinity (measured with refractometer) were used to calculate water density and convert DIC measurements from μmol L–1 to μmol kg–1. DIC values were calibrated against a standard curve generated using certified reference material (CRM, Batch 149, Dickson, Scripps Institute of Oceanography). Seawater samples for pH measurements were analyzed within hours of collection using a diode array spectrophotometer (Agilent 8453A UV-VIS). These samples were brought to 25°C in a water bath before transferring, via syringe, into a jacketed 5 cm cuvette also maintained at the same temperature. One 30 μL aliquot of m-cresol indicator dye was added to each sample, and the pH was calculated from absorbance at 730 nm, 578 nm, and 434 nm and corrected for the dye addition (Dickson et al., 2007). DIC and pH measurements were used to calculate pCO2, pH (total scale), and aragonite saturation states (Ω) using CO2SYS (Lewis and Wallace, 2012) with K1 and K2 equilibrium constants (Dickson and Millero, 1987). Mixing tank salinity was measured during carbonate chemistry sampling, using an YSI Pro-20.
Fertilization success for each treatment was quantified as the percentage of the initial egg count that was fertilized. Unfertilized eggs were carefully removed from the slide with a micro-spatula when not attached to a fertilized embryo. Dead embryos post-fertilization were counted and removed from the slides every 24 h until hatching commenced. To determine hatching success, daily counts of malformed and successfully hatched larvae were recorded. Hatching success was calculated as the percentage of the initial egg count that hatched as larvae with no deformities. Larvae were anesthetized in a 10 mL bath of tricaine methanesulfonate (MS-222) and then fixed in a 5% formalin solution. Fixed larvae were preserved with 15 mL of 200-proof ethyl alcohol in high-density polyethylene vials and were later dried and measured using the tracing tools of ImageJ analysis software.
Developing embryos and larvae rely heavily on yolk provisions and increased metabolic costs associated with environmental stress could cause the reallocation of this energy source to maintain other physiological processes, at the cost of growth or development (Dahlke et al., 2017). The amount of yolk in Pacific herring embryos was characterized by measuring the areal extent. Beginning on day 2, three embryos for which positioning made the embryo stage easily visible were chosen from each slide. These selected embryos were photographed daily using identical camera and microscope settings described for fertilization. The stage of embryo development was categorized for each of the embryos selected for heart rate analysis (Kawakami et al., 2011). Embryo yolk areas were measured from 2 days of digital photographs taken before the developing embryo obscured the yolk sac (days 9–10 at 10°C and days 3–4 at 16°C). Yolk areas were measured using ImageJ analysis software. Heart visibility depended on embryo development stage and position, which contributed to the difference in sample size. Video recordings of embryo heart rates were taken as soon as hearts were visible, which occurred on day 4 for the 16°C treatment and days 5 and 6 in the 10°C treatment. One-minute video recordings were taken from haphazardly selected embryos from each slide in the basins (n = 62 videos at 16°C, and n = 143 videos at 10°C). Videos were recorded by angling a 55-mm Nikon D3300 lens into an Olympus SZ40 Stereomicroscope viewfinder (6.7×). Heartbeats were visually counted during video analysis. Measurement of oxygen uptake was also attempted, but technical issues resulted in compromised data that could not be used.
Larval size at hatch was measured using standard length. The number of larvae per sample varied depending on daily hatch numbers, where a sample consisted of all larvae hatched from each bowl on a given day. Larvae were rinsed and photographed with a Nikon D3300 DSLR 55-mm lens. Each sample was then dried at 70°C for 24 h minimum and reweighed on the analytical scale to determine dry weight. Digital photographs were analyzed using ImageJ analysis software to measure larval standard length (from the tip of the snout to the last vertebrae) to the nearest 0.1 mm.
A linear mixed-effects model with fixed factors including temperature, pCO2 and their interaction effect was generated for each measured parameter (fertilization and hatching success, embryo mortality, yolk area, embryo heart rates, larval length and dry weight). Random factors (basin and parental female) were added to the models, fit by restricted maximum likelihood (REML) with individual slopes and intercepts, to determine if random factors improved the model fit. Linear mixed-effects models and random effects models were compared and model quality was chosen based on Akaike information criterion (AIC) values, with lower AIC values indicating a better model fit. A summary of statistical results from these linear models is presented in Supplementary Table 1. Random factors were found to be not significant and the additional flexibility of the linear mixed-effects models were not needed. We present the results of multifactorial analysis of variance (two-way ANOVA) for each variable for simplicity. Measurement endpoints were expressed as means ± standard deviations and tested for equal variance and normality using Levene’s and Shapiro-Wilk methods, respectively. A multifactorial analysis of variance (two-way ANOVA) was performed to evaluate the effects of temperature and pCO2 on each measurement endpoint (n = 7). Tukey’s HSD post hoc comparisons were used to detect significant differences between the means. Linear models were generated using the nlme package and the multcomp package for ANOVA’s and post hoc comparisons in RStudio (version 3.1.2, 2014). Statistical significance was determined by p ≤ 0.05.
Treatment levels are referred by their target pCO2 and temperature combination. The ambient pCO2 (∼ 600 μatm) treatments deviated less than 20 μatm over the course of experiments. The difference from “current-day” pCO2 (∼400 μatm) conditions, from our ambient pCO2, may be due to CO2 accumulation from respiration in the seawater system and from slightly elevated pCO2 in the waters of the Salish Sea. The high pCO2 treatments (∼ 1200 μatm) showed greater variability than the 600 μatm (Table 1). Since DIC in the high pCO2 treatments at 10°C and 16°C did not differ (p > 0.05), CO2 outgassing was not higher at 16°C, and the pCO2 differences between the 1200 μatm:10°C and 1200 μatm:16°C treatments can be attributed to the temperature effect on solubility of pCO2. Temperature, pH, and salinity were consistent within replicate basins for each treatment (Table 1).
Table 1. In situ seawater parameters and calculated discrete carbonate chemistry values during experiments.
Fertilization success was greater than 80 ± 6% in all dishes and was not affected by temperature, pCO2, or their interaction (p > 0.05, two-way ANOVA, Table 2). Within the ambient pCO2 (600 μatm), at elevated temperature (16°C) hatching success was 31 ± 6% (F1,44 = 55.1, p < 0.01, η2p = 0.55, two-way ANOVA, Figure 1 and Table 2), a significant decrease compared to 61 ± 10% hatch success at the ambient temperature (10°C). The difference in hatching success at elevated pCO2 was driven by temperature, with 50 ± 12% at 1200 μatm:10°C compared to 22 ± 7% at 1200 μatm:16°C. Embryo mortality increased with temperature, from 16 ± 10% at 600 μatm:10°C to 42 ± 16% at 600 μatm:16°C (F1,44 = 39.8, p < 0.01, η2p = 0.47, two-way ANOVA). Elevated pCO2 also had an effect on embryo mortality, which increased from 42 ± 16% at 600 μatm:16°C to 58 ± 19% at 1200 μatm:16°C (Figure 2 and Table 2) (F1,44 = 4.5, p < 0.05, η2p = 0.09, two-way ANOVA). While both stressors significantly affected embryo mortality the effect size of pCO2 was small, accounting for only 9% of the overall variance, and mortality was primarily driven by temperature.
Table 2. Two-way analysis of variance of each measured parameter (in bold) of Pacific herring early life stages as a function of temperature and pCO2.
Figure 1. Treatment effects on Pacific herring hatching success. Data represent the mean ± 1 SD of the percentage per slide, with 12 slides per 3 basins for each treatment. Lower-case letters indicate statistically different treatments based on a Tukey’s HSD post hoc comparison.
Figure 2. Treatment effects on Pacific herring embryo mortality. Data represent the mean ± 1 SD of the percentage per slide, with 12 slides per 3 basins for each treatment. Lower-case letters indicate statistically different treatments based on a Tukey’s HSD post hoc comparison.
Under ambient pCO2, elevated temperature was associated with an a mean area of 0.75 ± 0.12 yolk area (F1,691 = 80.5, p < 0.01, η2p = 0.10, two-way ANOVA), and embryo yolk areas were larger (mean area 0.82 ± 0.13) in the combined treatment of 1200 μatm:16°C (F1,691 = 4.7, p < 0.05, η2p < 0.00, two-way ANOVA) (Figure 3 and Table 2). Embryo heart rates were significantly faster (78 ± 10 beats per minute) in the 600 μatm:16°C treatment (F1,201 = 650.4, p < 0.01, η2p = 0.76, two-way ANOVA) than embryo heart rates in the 600 μatm:10°C (46 ± 7 beats per minute, Figure 4). The 1200 μatm:16°C had the highest heart rates among all treatment combinations at an average of 86 ± 10 beats per minute (F1,201 = 5.63, p < 0.01, η2p = 0.02, two-way ANOVA, Figure 4 and Table 2).
Figure 3. Mean yolk area (mm2) for Pacific herring embryos under treatment conditions. Data are from images collected over 2 days before the developing embryo obscured the yolk sac (days 9–10 at 10°C and 3–4 at 16°C. Data are based on the mean ± 1 SD of (n) measurements: 600 μatm:10°C (n = 60), 1200 μatm:10°C (n = 54), 600 μatm:16°C (n = 49), and 1200 μatm:16°C (n = 43). Lower-case letters indicate statistically different treatments based on a Tukey’s HSD post hoc comparison.
Figure 4. Treatment effects of Pacific herring embryo heart rates (beats min–1). Heartbeats were recorded during 1-min intervals on either day 4 (16°C) or day 5 and 6 (10°C). Data are based on the mean ± 1 SD of (n) measurements 600 μatm:10°C (n = 72), 1200 μatm:10°C (n = 71), 600 μatm:16°C (n = 32), and 1200 μatm:16°C (n = 30). Lower-case letters indicate statistically different treatments based on a Tukey’s HSD post hoc comparison.
Larvae reared at 600 μatm:16°C were shorter (5.6 ± 7 mm mean length) than larvae in the 600 μatm:10°C treatment (F1,41 = 75.5, p < 0.01, η2p = 0.34, two-way ANOVA), but were not affected by pCO2 nor the treatment interaction (p > 0.05, two-way ANOVA, Figure 5 and Table 2). Lengths were also on average 16% shorter between 1200 μatm:10°C than 1200 μatm:16°C. The magnitude of the temperature effect did not change with pCO2 treatment as indicated by the lack of a significant interaction effect of temperature and pCO2. Average dry weights did not significantly differ among treatments, averaging 0.08 mg per larvae (p > 0.05, two-way ANOVA). Larvae in the 16°C treatment hatched earlier (between 6 and 11 days post fertilization) than those in the 10°C treatment (between 10 and 15 days post fertilization).
Figure 5. Mean Pacific herring larval lengths (mm) upon hatch. Length data are represented by the mean ± 1 SD of (n) measurements 600 μatm:10°C (n = 414), 1200 μatm:10°C (n = 425), 600 μatm:16°C (n = 338), and 1200 μatm:16°C (n = 271). Lower-case letters indicate statistically different treatments based on a Tukey’s HSD post hoc comparison.
The principle environmental variable affecting Pacific herring embryos and larvae in this study was elevated temperature. This finding is congruent with the understanding of temperature’s effect on developing Pacific herring, in which development was accelerated along with a greater embryo mortality and reduced hatching success (Alderdice and Velsen, 1971; Alderdice and Hourston, 1985; Pepin, 1991; Dinnel et al., 2007; Kawakami et al., 2011). Given that the mean temperature measured at Cherry Point during spawning season is already within 1 degree of the high temperature investigated here (DNR), and that further increases of between 1.5 and 4°C can be expected (Khangaonkar et al., 2018), temperature stress may become critical for larval herring at Cherry Point and in the Salish Sea. Elevated pCO2 as a single stressor did not elicit a discernable effect on the metrics of Pacific herring fitness surveyed here. Pacific herring in this region may be desensitized to the elevated pCO2 level used in this study. The carbonate system in coastal oceans is dynamic and in the Salish Sea daily and seasonal pCO2 variability can be extreme, due to freshwater inputs, respiratory activity from biological communities, and the intrusion of pCO2-rich deep water into the Salish Sea basin (Feely et al., 2010; Hoffman et al., 2011; Moore-Maley et al., 2016).
These environmental conditions may influence the relative sensitivity of species-specific responses to ocean acidification. For example, Leo et al. (2018) suggest that the differing responses to high pCO2 observed in Atlantic herring, a congener species to Pacific herring, may be explained by environmental acclimation. High pCO2 is regularly observed in the Kiel Fjord, and little to no effect of highly elevated pCO2 was observed in Atlantic herring larvae from this location (Franke and Clemmesen, 2011), compared to significant effects in larvae from populations spawning in relatively low pCO2 in the Oslo Fjord (Frommel et al., 2011; Leo et al., 2018). Diel and tidal cycles in coastal environments may also contribute to a lack of embryo sensitivity to elevated pCO2 (Cross et al., 2019). Coastal species, such as the Pacific herring, that experience short-term pCO2 fluctuations could produce offspring that are tolerant of these conditions, as the cost of acid-base regulation decreased during diel pCO2 cycles in several teleost species (Jarrold et al., 2017; Cross et al., 2019). The possibility for parental acclimation to elevated pCO2 on larval survival is a fundamental parameter for population recruitment. A recent study on Atlantic cod (Gadus morhua) found that parental acclimation, along with high food availability, alleviated some negative effects of high pCO2 on larval mortality (Stiasny et al., 2018). Atlantic silverside offspring were more susceptible to acidified conditions early in the spawning season, compared to later in the season, perhaps because of better parental conditioning later in the season when these conditions are more common (Murray et al., 2014).
While our study solely focused on the early life stages, until hatch, of Pacific herring embryos from one spawning event under static conditions, the results are suggestive that effects may be present under more fully realized simulations including variable conditions and with more widely sampled individuals. The potential for parental acclimation in this species, or varying adaption between populations is another area of research that could yield valuable information about potential responses to ocean acidification in Pacific herring populations. Below we discuss how the synergy of these climate variables affected the various aspects of Pacific herring early life stages in this study.
As an individual stressor, high pCO2 did not affect hatching success, which is a congruent finding with a previous study on Atlantic herring. Franke and Clemmesen (2011) found no linear relationship between high pCO2 and negative effects on Atlantic herring larval total length, dry weight, yolk sac and otolith area. While temperature has clear effects on Pacific herring embryo mortality and hatching success (Alderdice and Velsen, 1971; Alderdice and Hourston, 1985), this study demonstrates that high pCO2 when combined with temperature stress, may further increase mortality. The increased mortality at combined high temperature and pCO2 observed here calls for further investigation.
The broad temperature effects measured in this study are similar to those observed in other investigations (Alderdice and Velsen, 1971; Johnston et al., 1998, 2001; Dinnel et al., 2007). For instance, Pacific herring hatching success at a salinity of 30 PSU is predicted to be 80% and 36% at 10°C and 16°C, respectively by Alderdice and Velsen (1971), compared to measured values of 70% and 32% is this study. Predicted mortality rates are also similar to our observed values (Alderdice and Velsen, 1971). Mechanisms for increased mortality in the high temperature treatment may be related to oxygen supply, respiratory activity and metabolic activity, as suggested by heart rate and yolk sac data combined with results of previous studies (Alderdice and Hourston, 1985; Rombough, 2011; Dahlke et al., 2017). The interaction of pCO2 and temperature was not significant for hatching success. This metric is closely related to mortality, but includes larvae that hatch, yet are not likely to survive due to malformations.
While the effects of increased embryo mortality on Pacific herring populations are obvious, subtle effects on elevated heart rates and changes in size and development at hatch can have important survival impacts at later life stages, and can provide insight into mechanisms that may be driving the changes in embryo mortality. We found that Pacific herring incubated at higher temperatures hatched earlier and were smaller in length than those incubated at lower temperatures, which is consistent with patterns found in Atlantic herring, including length association with age (Geffen, 2002; Leo et al., 2018). Larval lengths at hatch may have an indirect effect on swimming performance. Shorter lengths (∼ 7 mm total length difference) decreased swimming velocity (m s–1) by 24% of Atlantic herring larvae (Johnston et al., 2001). Energetic tradeoffs may also continue into later life stages. For example, elevated temperature was associated with increased swimming ability, but decreased growth and survival in Atlantic herring larvae (Sswat et al., 2018).
Pacific herring larval dry weights did not statistically differ between treatments and an examination of embryo yolk sacs may provide an explanation. Yolk conversion efficiency reaches a maximum within the thermal tolerance range of a given species, and tends to decrease near the upper and lower boundaries of tolerated temperatures (Galloway et al., 1998). For example, Atlantic cod embryos incubated at a low temperature (1°C) produced smaller larvae with larger yolk sacs, than embryos incubated at 5°C or 8°C (Galloway et al., 1998); suggesting that the reduced larval length obtained at the low temperatures may be a sub-lethal response an unfavorable environment. Conversely, under increased temperature (12°C) and pCO2 (1100 μatm), Atlantic cod embryos experienced higher metabolic rates and reduced larval length at hatch, while the consumption of yolk reserves remained unaffected; indicating embryos were not able to convert yolk energy to other physiological functions (Dahlke et al., 2017).
In this study, Pacific herring embryos reared in the 1200 μatm:16°C treatment had the largest yolk area. Greater yolk areas in Pacific herring embryos reared at increased temperature and pCO2 indicate less energy was used for other developmental processes, such as growth. This may explain why no differences were detected in larval dry weights, with mass either remaining within the yolk sac for larvae under the high temperature or converted into growth for larvae in the ambient temperature treatment.
The effects of increased temperature on metabolism are well known and have been widely studied on marine fish, while the pCO2 effects on metabolic rates and processes are only now beginning to be explored. The increase in heart rate in both 16°C treatments observed in this study could be caused by increased oxygen demand at higher temperature, and appears to be exacerbated by high pCO2. The effect of temperature on oxygen availability may help to explain these results. Thermally regulated limits to oxygen supply can be a major driver in determining the thermal window through both limited circulatory capacity and solubility driven reductions in O2 availability (Pörtner and Knust, 2007). While elevated pCO2 conditions can impose additional metabolic demands through acid-base regulation, basal oxygen demand and standard metabolic rate have not been found to increase in marine fish under existing and projected globally relevant pCO2 levels (Lefevre, 2016; Esbaugh, 2018).
While oxygen consumption rates appear to respond to increased temperature in Atlantic herring larvae (Leo et al., 2018), enzyme activity – which indicates overall aerobic capacity – did not exhibit a strong temperature response (Overnell and Batty, 2000). Increased oxygen uptake in Atlantic herring appears to be partially linked to a reduction in the efficiency of mitochondrial ATP production as temperature increases (Leo et al., 2018). When efficiency in energy conversion decreases, both oxygen uptake and respiration must increase, potentially reallocating energy resources away from growth and development when energy becomes limiting. A similar mechanism for reduced metabolic scope with increased temperature was detected in Atlantic cod, and in this case, elevated pCO2 caused an earlier onset of the thermal stress to metabolic rates (Dahlke et al., 2017). This pairing of increased respiration with impaired energy conversion fits with the elevated heart rates and slower yolk utilization observed in the Pacific herring embryos exposed to high temperature and elevated pCO2 in this study. Direct oxygen uptake measurements were not successful here, but future investigation of mitochondrial oxygen uptake could determine if the interaction effects of temperature and pCO2 on Pacific herring heart rates are driven by this mechanism as well.
Resilience to pCO2 stress may result from acclimation to the dynamic environment in the Salish Sea, where the pH and pCO2 regularly fluctuate to extreme values on diurnal and tidal cycles during early to mid-summer months in nearshore eelgrass meadows where Pacific herring regularly spawn. These fluctuations often exceed the magnitude of predicted climate change related shifts for the end of the century in both temperature and pH. However, as climate changes progresses and the additional inputs from upwelling events, freshwater inputs, and other natural processes within the Salish Sea further reduces environmental pH, Pacific herring embryos may exhibit a reduced tolerance to elevated pCO2, particularly with the co-occurrence of warmer temperatures. The high temperature and high pCO2 conditions tested in this study are present currently, at least during some periods of the diurnal and tidal cycles for late spawning Cherry Point population. They may become increasingly common during those cycles at this location, and for earlier spawning populations in the coming decades, perhaps bringing increased metabolic stress and mortality as thresholds for harmful temperature and pCO2 are more frequently crossed.
Our study demonstrated that pCO2 had little effect on developmental endpoints in Pacific Herring as an individual stressor, but mortality and metabolic effects of pCO2 on yolk sac area and heart rate were distinct when combined with increased temperature. Therefore, the potential for negative effects of ocean acidification must be considered along with the known effects of increasing temperature on reproductive success.
The ecological outcomes for Pacific herring populations in the Salish Sea will depend on how the effects of environmental stresses on their early life stages interact with potential behavioral changes, direct or indirect food web effects, reproductive and ecosystem level drivers. So, further investigation of the larger implications of these findings is needed.
The raw data supporting the conclusions of this article will be made available by the authors, without undue reservation.
The animal study was reviewed and approved by Western Washington University Animal Care and Use Committee.
CV conceptualized and designed the experiment with modifications directed by BL and BO. CV led the writing of the manuscript with contributions by BL and BO. CV preformed all data analysis with statistical analysis support by BL. All authors reviewed and contributed to the writing of the final manuscript.
This work was funded by the National Science Foundation (NSF) Graduate Research Fellowship Program (GRFP) and the Huxley College of the Environment at Western Washington University.
The authors declare that the research was conducted in the absence of any commercial or financial relationships that could be construed as a potential conflict of interest.
We thank Dr. Paul Dinnel, Dr. Katherina L. Schoo, and Kelley Bright for their research support. We also thank the Washington Department of Fish and Wildlife for their assistance in obtaining Pacific herring. CV would like to thank Jocelyn Wensloff, Max Miner, Katey Williams, Hillary Thalmann, Darby Finnegan, Lynne Nowak, and Tyler Tran for their valuable assistance during experiments. Lastly, we thank the faculty and staff at the Shannon Point Marine Center for providing support, facilities, and equipment necessary to conduct this research.
The Supplementary Material for this article can be found online at: https://www.frontiersin.org/articles/10.3389/fmars.2020.597899/full#supplementary-material
Alderdice, D., and Hourston, A. (1985). Factors influencing development and survival of Pacific herring (Clupea harengus pallasi) eggs and larvae to beginning of exogenous feeding. Can. J. Fish. Aquat. Sci. 42, 56–68. doi: 10.1139/f85-262
Alderdice, D., and Velsen, F. (1971). Some effects of salinity and temperature on early development of Pacific herring (Clupea pallasi). J. Fish. Res. Board Can. 28, 1545–1562. doi: 10.1139/f71-234
Baumann, H., Talmage, S. C., and Gobler, C. J. (2011). Reduced early life growth and survival in a fish in direct response to increased carbon dioxide. Nat. Clim. Change 2, 38–41. doi: 10.1038/nclimate1291
Burger, F. A., John, J. G., and Frölicher, T. L. (2020). Increase in ocean acidity variability and extremes under increasing atmospheric CO2. Biogeosciences 17, 4633–4662. doi: 10.5194/bg-17-4633-2020
Cai, W. J. (2011). “Estuarine and costal ocean carbon paradox: CO2 sinks or sites of terrestrial carbon incineration?,” in Annual Review of Marine Science, Vol. 3, eds C. A. Carlson and S. J. Giovannoni (Palo Alto, CA: Annual Reviews), 123–145. doi: 10.1146/annurev-marine-120709-142723
Caldeira, K., and Wickett, M. E. (2003). Anthropogenic carbon and ocean pH. Nature 423:365. doi: 10.1038/425365a
Chung, W. S., Marshall, N. J., Watson, S. A., Munday, P. L., and Nilsson, G. E. (2014). Ocean acidification slows retinal function in a damselfish through interference with GABAA receptors. J. Exp. Biol. 217, 323–326. doi: 10.1242/jeb.092478
Cornwall, C. E., and Hurd, C. L. (2015). Experimental design in ocean acidification research: problems and solutions. ICES J. Mar. Sci. 73, 572–581. doi: 10.1093/icesjms/fsv118
Cross, E. L., Murray, C. S., and Baumann, H. (2019). Diel and tidal pCO2 x O2 fluctuations provide physiological refuge to early life stages of a coastal forage fish. Sci. Rep. 9:18146. doi: 10.1038/s41598-019-53930-8
Dahlke, F. T., Leo, E., Mark, F. C., Pörtner, H. O., Bickmeyer, U., Frickenhaus, S., et al. (2017). Effects of ocean acidification increase embryonic sensitivity to thermal extremes in Atlantic cod, Gadus morhua. Glob. Chan. Biol. 23, 1499–1510. doi: 10.1111/gcb.13527
Deigweiher, K., Hirse, T., Bock, C., Lucassen, M., and Pörtner, H. O. (2010). Hypercapnia induced shifts in gill energy budgets of Antarctic notothenioids. J. Comp. Physiol. B 180, 347–359. doi: 10.1007/s00360-009-0413-x
Deigweiher, K., Koschnick, N., Pörtner, H. O., and Lucassen, M. (2008). Acclimation of ion regulatory capacities in gills of marine fish under environmental hypercapnia. Am. J. Physiol. Regul. Integr. Comp. Physiol. 295, R1660–R1670. doi: 10.1152/ajpregu.90403.2008
Department of Natural Resources (DNR) (2019). Acidification Nearshore Monitoring Network. Available online at: https://esri.maps.arcgis.com/apps/webappviewer/index.html?id=17a44f434a7c48fa8fe992d1bb933345 (accessed October 5, 2020).
Dickson, A. G., and Millero, F. J. (1987). A comparison of the equilibrium constants for the dissociation of carbonic acid in seawater media. Deep Sea Res. Part A Oceanogr. Res. Pap. 34, 1733–1743. doi: 10.1016/0198-0149(87)90021-5
Dickson, A. G., Sabine, C. L., and Christian, J. R. (2007). Guide to best practices for ocean CO2 measurements. PICES Spec. Publ. 3, 1–191. doi: 10.1201/b10717-2
Dinnel, P. A., Hoover, R., and Lechuga, L. (2007). Development of Larval Pacific Herring, Clupea pallasi, Bioassay Protocols: Refinement, Validation, Refinery Effluent and Cherry Point Ambient Water Testing During 2007. (Anacortes, WA: Western Washington University), 82.
Dixson, D. L., Munday, P. L., and Jones, G. P. (2010). Ocean acidification disrupts the innate ability of fish to detect predator olfactory cues. Ecol. Lett. 13, 68–75. doi: 10.1111/j.1461-0248.2009.01400.x
Doney, S. C., Ruckelshaus, M., Duffy, E., Barry, J. P., Chan, F., English, C. A., et al. (2012). Climate change impacts on marine ecosystems. Annu. Rev. Mar. Sci. 4, 11–37. doi: 10.1146/annurev-marine-041911-111611
Duarte, C. M., Hendriks, I. E., Moore, T. S., Olsen, Y. S., Steckbauer, A., Ramajo, L., et al. (2013). Is ocean acidification an open-ocean syndrome? Understanding anthropogenic impacts on seawater pH. Estuar. Coast. 36, 221–236. doi: 10.1007/s12237-013-9594-3
Esbaugh, A. J. (2018). Physiological implications of ocean acidification for marine fish: emerging patterns and new insights. J. Comp. Phys. B 188, 1–13. doi: 10.1007/s00360-017-1105-6
Feely, R. A., Alin, S. R., Newton, J., Sabine, C. L., Warner, M., Devol, A., et al. (2010). The combined effects of ocean acidification, mixing, and respiration on pH and carbonate saturation in an urbanized estuary. Estuar. Coast Shelf Sci. 88, 442–449. doi: 10.1016/j.ecss.2010.05.004
Feely, R. A., Sabine, C. L., Hernandez-Ayon, J. M., Ianson, D., and Hales, B. (2008). Evidence for upwelling of corrosive “acidified” water onto the continental shelf. Science 320, 1490–1492. doi: 10.1126/science.1155676
Francis, T., and Lowry, D. (2018). Assessment and Management of Pacific Herring in the Salish Sea: Conserving and Recovering a Culturally Significant and Ecologically Critical Component of the Food Web. The SeaDoc Society, 74. Available online at: http://static1.squarespace.com/static/5b071ddea2772cebc1662831/t/5c18489e032be4b073ad9d86/1545095350054/frances-et-al-herring-assessment-management-of-salish-sea.pdf (accessed May 27, 2020).
Franke, A., and Clemmesen, C. (2011). Effect of ocean acidification on early life stages of Atlantic herring (Clupea harengus L.). Biogeosciences 8, 3697–3707. doi: 10.5194/bg-8-3697-2011
Fresh, K. L., Cardwell, R. D., and Koons, R. R. (1981). Food Habitats of Pacific Salmon, Baitfish, and their Potential Competitors and Predators in the Marine Waters of Washington, August 1978 to September 1979. State of Washington, Department of Fisheries, Progress Report No. 145. (Olympia, WA: Washington State Department of Fish and Wildlife).
Frommel, A. Y., Maneja, R., Lowe, D., Malzahn, R. M., Geffen, A. J., Folkvord, A., et al. (2011). Severe tissue damage in Atlantic cod larvae under increasing ocean acidification. Nat. Clim. Change. 2, 42–46. doi: 10.1038/NCLIMATE1324
Galloway, T. F., Kjørsvik, E., and Kryvi, H. (1998). Effect of temperature on viability and axial muscle development in embryos and yolk sac larvae of the Northeast Arctic cod (Gadus morhua). Mar. Biol. 132, 559–567. doi: 10.1007/s002270050421
Geffen, A. (2002). Length of herring larvae in relation to age and time of hatching. J. Fish. Biol. 60, 479–485. doi: 10.1111/j.1095-8649.2002.tb00295.x
Harvey, B. P., Gwynn-Jones, D., and Moore, P. J. (2013). Meta-analysis reveals complex marine biological to the interactive effects of ocean acidification and warming. Ecol. Evol. 3, 1016–1030. doi: 10.1002/ece3.516
Hoffman, G. E., Smith, J. E., Johnson, K. S., Send, U., Levin, L. A., Micheli, F., et al. (2011). High-frequency dynamics of ocean pH: a multi-ecosystem comparison. PLoS One 6:e28983. doi: 10.1371/journal.pone.0028983
Houde, E. D. (2008). Emerging from Hjort’s shadow. J. Northw. Atl. Fish. Sci. 41, 53–70. doi: 10.2960/J.v41.m634
Hurst, T. P., Fernandez, E. R., and Mathis, J. T. (2013). Effects of ocean acidification on hatch size and larval growth of walleye pollock (Theragra chalcogramma). ICES J. Mar. Sci. 70, 812–822. doi: 10.1093/icesjms/fst053
IPCC (2019). “Summary for Policymakers,” in IPCC Special Report on the Ocean and Cryosphere in a Changing Climate, eds H.-O. Pörtner, D. C. Roberts, V. Masson-Delmotte, P. Zhai, M. Tignor, E. Poloczanska, et al. (Geneva: IPCC).
Ishimatsu, A., Hayashi, M., and Kikkawa, T. (2008). Fishes in high-CO2, acidified oceans. Mar. Eco. Prog. Ser. 373, 295–302. doi: 10.3354/meps07823
Jarrold, M. D., Humphrey, C., McCormick, M. I., and Munday, P. L. (2017). Diel CO2 cycles reduce severity of behavioural abnormalities in coral reef fish under ocean acidification. Sci. Rep. 7:10153. doi: 10.1038/s41598-017-10378-y
Johansen, J. L., and Jones, G. P. (2011). Increasing ocean temperature reduces the metabolic performance and swimming ability of coral reef damselfishes. Glob. Chan. Biol. 17, 2971–2979. doi: 10.1111/j.1365-2486.2011.02436.x
Johnston, I. A. (1993). Temperature influences muscle differentiation and the relative timing of organogenesis in herring (Clupea harengus) larvae. Mar. Biol. 116, 363–379. doi: 10.1007/bf00350053
Johnston, I. A., Cole, N. J., Abercromby, M., and Vieira, V. L. A. (1998). Embryonic temperature modulates muscle growth characteristics in larval and juvenile herring. J. Exp. Biol. 201, 623–646.
Johnston, I. A., Vieira, V. L. A., and Temple, G. K. (2001). Functional consequences and population differences in the developmental plasticity of muscle to temperature in Atlantic herring Clupea harengus. Mar. Ecol. Prog. Ser. 213, 285–300. doi: 10.3354/meps213285
Jokiel, P. L., Bahr, K. D., and Rodgers, K. S. (2014). Low-cost, high-flow mesocosm system for simulation ocean acidification with CO2 gas. Limnol. Oceanogr. Methods 12, 313–322. doi: 10.4319/lom.2014.12.313
Kawakami, T., Okouchi, H., Aritaki, M., Aoyama, J., and Tsukamoto, K. (2011). Embryonic development and morphology of eggs and newly hatched larvae of Pacific herring (Clupea pallasii). Fish. Sci. 77, 183–190. doi: 10.1007/s12562-010-0317-4
Khangaonkar, T., Nugraha, A., Xu, W., Long, W., Bianucci, L., Ahmed, A., et al. (2018). Analysis of hypoxia and sensitivity to nutrient pollution in Salish Sea. J. Geophys. Res. 123, 4735–4761. doi: 10.1029/2017JC013650
Kikkawa, T., Ishimatsu, A., and Kita, J. (2003). Acute CO2 Tolerance during the Early Developmental Stages of fur Marine Teleosts. (Hoboken, NJ: Wiley InterScience). doi: 10.1002/tox.10139
Kreiss, C. M., Michael, K., Jutfelt, F., Motyka, R., Dupont, S., and Pörtner, H. O. (2015). Ocean warming and acidification modulate energy budget and gill ion regulatory mechanisms in Atlantic cod (Gadus morhua). J. Comp. Phys. B 185, 767–781. doi: 10.1007/s00360-015-0923-7
Lefevre, S. (2016). Are global warming and ocean acidification conspiring against marine ectotherms? A meta-analysis of the respiratory effects of elevated temperature, high CO2 and their interaction. Conserv. Physiol. 4:cow009. doi: 10.1093/conphys/cow009
Leo, E., Dahlke, F. T., Storch, D., Pörtner, H. O., and Mark, F. C. (2018). Impact of ocean acidification and warming on the bioenergetics of developing eggs of Atlantic herring Clupea harengus. Conserv. Physiol. 6:coy050. doi: 10.1093/conphys/coy050
Lewis, E., and Wallace, D. W. R. (2012). Program Developed for CO2 System Calculations. ORNL/CDIAC- 105. Oak Ridge, TN: US Department of Energy.
Moore-Maley, B. L., Allen, S. E., and Ianson, D. (2016). Locally driven interannual variability of near-surface pH and ΩA in the Strait of Georgia. J. Geophys. Res. Oceans 121, 1600–1625. doi: 10.1002/2015JC011118
Munday, P. L., Donelson, J. M., Dixson, D. L., and Endo, G. G. K. (2009a). Effects of ocean acidification on the early life history of a tropical marine fish. Proc. R. Soc. B 276, 3275–3283. doi: 10.1098/rsbp.2009.0784
Munday, P. L., Dixson, D. L., Donelson, J. M., Jones, G. P., Pratchett, M. S., Devitsina, G. V., et al. (2009b). Ocean acidification impairs olfactory discrimination and homing ability of a marine fish. Proc. Natl. Acad. Sci. U.S.A. 106, 1848–1852. doi: 10.1073/pnas.0809996106
Murray, C. S., Malvezzi, A., Gobler, C. J., and Baumann, H. (2014). Offspring sensitivity to ocean acidification changes seasonally in a coastal marine fish. Mar. Ecol. Prog. Ser. 504, 1–11. doi: 10.3354/meps107191
Overnell, J., and Batty, R. S. (2000). Scaling of enzyme activity in larval herring and plaice: effects of temperature and individual growth rate on aerobic and anaerobic capacity. J. Fish. Biol. 56, 577–589. doi: 10.1006/jfbi.1999.1176
Pacella, S. R., Brown, C. A., Waldbusser, G. G., Labiosa, R. G., and Hales, B. (2018). Seagrass habitat metabolism increases short-term extremes and long-term offset of CO2 under future ocean acidification. Proc. Natl. Acad. Sci. U.S.A. 115, 3870–3875. doi: 10.1073/pnas.1703445115
Pankhurst, N. W., and Munday, P. L. (2011). Effects of climate change on fish reproduction and early life history stages. Mar. Fresh. Res. 62, 1015–1026. doi: 10.1071/MF10269
Penttila, D. (2007). Marine Forage Fishes in Puget Sound. Washington Department of Fish and Wildlife Technical Report 2007-03. (Olympia, WA: Washington Department of Fish and Wildlife), 23.
Pepin, P. (1991). Effect of temperature and size on development, mortality, and survival rates of the pelagic early life history stages of marine fish. Can. J. Fish. Aquat. Sci. 48, 503–518. doi: 10.1139/f91-065
Pimentel, M. S., Faleiro, F., Dionísio, G., Repolho, T., Pousão-Ferreira, P., Machado, J., et al. (2014). Defective skeletogeneis and oversized otoliths in fish early stages in a changing ocean. J. Exp. Biol. 217, 2062–2070. doi: 10.1242/jeb.092635
Pörtner, H. O., and Knust, R. (2007). Climate change affects marine fishes through the oxygen limitation of thermal tolerance. Science 315, 95–97. doi: 10.1126/science.1135471
Purcell, J. E., Grosse, D., and Grover, J. J. (1990). Mass abundances of abnormal Pacific herring larvae at a spawning ground in British Columbia. Trans. Am. Fish. Soc. 119, 463–469. doi: 10.1577/1548-8659(1990)119<0463:maoaph>2.3.co;2
Rombough, P. (2011). The energetics of embryonic growth. Respir. Physiol. Neurobiol. 178, 22–29. doi: 10.1016/j.resp.2011.04.026
Sandell, T., Lindquist, A. P., Dionne, P., and Lowry, D. (2019). 2016 Washington State Herring Stock Status Report. Species Status Reports. Fish Program Technical Report No. FPT 19–07. (Olympia, WA: Washington Department of Fish and Wildlife).
Schweigert, J. (1994). “Evaluation of harvesting policies for the management of Pacific herring stocks, Clupea harengus pallasi,” in Symposium of the Management Strategies for Exploited Fish Populations, eds G. Kruse, D. Eggers, R. J. Marasco, C. Pautzke, and T. J. Quinn (Fairbanks, AK: University of Alaska Press), 167–190.
Schweigert, J. F., Boldt, J. L., Flostrand, L., and Cleary, J. S. (2010). A review of factors limiting recovery of Pacific herring stocks in Canada. ICES J. Mar. Sci. 67, 1903–1913. doi: 10.1093/icesjms/fsq134
Shelton, A. O., Francis, T. B., Williams, G. D., Feist, B., Stick, K., and Levin, P. S. (2014). Habitat limitation and spatial variation in Pacific herring egg survival. Mar. Ecol. Prog. Ser. 514, 231–245. doi: 10.3354/meps10941
Siple, M. C., Shelton, A. O., Francis, T. B., Lowry, D., Lindquist, A. P., and Essington, T. E. (2018). Contributions of adult mortality to declines of Puget Sound Pacific herring. ICES J. Mar. Sci. 75, 319–329. doi: 10.1093/icesjms/fsx094
Sswat, M., Stiasny, M. H., Jutfelt, F., Riebesell, U., and Clemmesen, C. (2018). Growth performance and survival of larval Atlantic herring, under the combined effects of elevated temperatures and CO2. PLoS One 13:e0191947. doi: 10.1371/journal.pone.0191947
Stiasny, M. H., Mittermayer, F. H., Göttler, G., Bridges, C. R., Falk-Petersen, I.-B., Puvanendran, V., et al. (2018). Effects of parental acclimation and energy limitation in response to high CO2 exposure in Atlantic cod. Sci. Rep. 8, 8348. doi: 10.1038/s41598-018-26711-y
Stick, K. C., Lindquist, A., and Lowry, D. (2014). 2012 Washington State Herring Stock Status Report. Washington Department of Fish and Wildlife, Fish Program Technical Report No. FPA. (Olympia, WA: Washington Department of Fish and Wildlife), 14–19.
Sunda, W. G., and Cai, W. J. (2012). Eutrophication induced CO2-acidification of subsurface coastal waters: interactive effects of temperature, salinity, and atmospheric pCO2. Environ. Sci Technol. 46, 10651–10659.
Thompson, S. A., Sydeman, W. J., Thayer, J. A., Weinstein, A., Krieger, K. L., and Hay, D. (2017). Trends in the Pacific herring (Clupea pallasii) metapopulation in the California current ecosystem. CalCOFI Rep. 58, 1–18.
Tseng, Y. C., Hu, M. Y., Stump, M., Lin, L. Y., Melzner, F., and Hwang, P. P. (2013). CO2-driven seawater acidification differentially affects development and molecular plasticity along life history of fish (Oryzias latipes). Comp. Biochem. Phys. A 165, 119–130. doi: 10.1016/j.cbpa.2013.02.005
Vieira, V. I. A., and Johnston, I. A. (1992). Influence of temperature on muscle-fibre development in larvae of the herring Clupea harangus. Mar. Biol. 112, 333–341. doi: 10.1007/bf00702480
Keywords: Pacific herring, ocean acidification, ocean warming, early life, teleost
Citation: Villalobos C, Love BA and Olson MB (2020) Ocean Acidification and Ocean Warming Effects on Pacific Herring (Clupea pallasi) Early Life Stages. Front. Mar. Sci. 7:597899. doi: 10.3389/fmars.2020.597899
Received: 22 August 2020; Accepted: 24 November 2020;
Published: 15 December 2020.
Edited by:
Pedro Morais, University of Algarve, PortugalReviewed by:
Ana M. Faria, MARE-ISPA, PortugalCopyright © 2020 Villalobos, Love and Olson. This is an open-access article distributed under the terms of the Creative Commons Attribution License (CC BY). The use, distribution or reproduction in other forums is permitted, provided the original author(s) and the copyright owner(s) are credited and that the original publication in this journal is cited, in accordance with accepted academic practice. No use, distribution or reproduction is permitted which does not comply with these terms.
*Correspondence: Cristina Villalobos, Y3ZpbGxhbG9ib3NAY3N1bWIuZWR1
Disclaimer: All claims expressed in this article are solely those of the authors and do not necessarily represent those of their affiliated organizations, or those of the publisher, the editors and the reviewers. Any product that may be evaluated in this article or claim that may be made by its manufacturer is not guaranteed or endorsed by the publisher.
Research integrity at Frontiers
Learn more about the work of our research integrity team to safeguard the quality of each article we publish.