- Biological and Environmental Science and Engineering Division, Red Sea Research Centre and Computational Bioscience Research Center, King Abdullah University of Science and Technology, Thuwal, Saudi Arabia
Giant clams (Subfamily Tridacninae), are important members of Indo-Pacific coral reefs, playing multiple roles in the framework of these communities. Although they are prominent species in Red Sea reefs, data on their distribution and densities in the region are scarce. The present study provides the first large-scale survey of Red Sea Tridacna spp. densities, where we examined a large proportion of the Saudi Arabian Red Sea coast (1,300 km; from 18° to 29°N). Overall, Tridacninae were found at densities of 0.19 ± 0.43 individuals m–2 (±SD). Out of the total 4,002 observed clams, the majority (89%) were Tridacna maxima, with 0.17 ± 0.37 individuals m–2, while only 11% were Tridacna squamosa clams with 0.02 ± 0.07 individuals m–2. We also report on a few (total 6) Tridacna squamosina specimens, found at a single reef. We identified different geographical parameters (i.e., latitude and distance to shore) and local environmental factors (i.e., depth and reef zone) as the main drivers for local Tridacna spp. densities. Our results show that the drivers influencing the densities of Red Sea giant clams are complex due to their co-occurrence and that this complexity might explain the high variation in Tridacninae abundances across the Indo-Pacific, but also within a given reef. We also estimate that giant clam calcification likely contributes to an average of 0.7%, but potentially up to 9%, of the overall mean calcium carbonate budget of Red Sea coral reef communities.
Introduction
Giant clams of the Tridacninae subfamily, ranking among the largest (Beckvar, 1981) and fastest-growing bivalves on Earth, are charismatic and important components of Indo-Pacific coral reef communities (Bonham, 1965; Neo et al., 2015). They are an important ecosystem engineer, contributing to the reef carbonate structure (Andréfouët et al., 2005; Neo et al., 2015) and play multiple other roles in this ecosystem. They provide food for predators and scavengers (Alcazar, 1986), shelter for commensal organisms (De Grave, 1999), and serve as a settling substrate for epibionts (Vicentuan-Cabaitan et al., 2014). To date, this bivalve subfamily comprises 12 extant species (Neo et al., 2017; Fauvelot et al., 2020), found in greatest diversity in the Central Indo-Pacific, especially Polynesia, but also in Australian waters (both, Pacific and Indian Ocean), and the Red Sea (bin Othman et al., 2010; Neo et al., 2017). However, their taxonomical status, as well as their distribution ranges and phylogeography are continuously revised (Fauvelot et al., 2020).
All giant clam species are currently listed on the IUCN Red List of Threatened Species (IUCN, 2016) and protected under Appendix II of the Convention on International Trade in Endangered Species of Wild Fauna and Flora (CITES). Most of them are considered to be at a lower risk/conservation-dependent status; however, according to Neo et al. (2017), the IUCN status of Tridacninae is in urgent need of updating. For the Red Sea, three species of giant clams have been previously reported (e.g., by Roa-Quiaoit, 2005; Richter et al., 2008; Huber and Eschner, 2010; Ullmann, 2013), namely Tridacna squamosa Lamarck, 1819, Tridacna squamosina Sturany, 1899 (previously described as T. costata by Richter et al., 2008) and Tridacna maxima Röding, 1789. The latter, also called the “small giant clam,” is assumed to be the most abundant giant clam species in the Red Sea (Jantzen et al., 2008; Pappas et al., 2017; Lim et al., 2020). Previously reported densities of T. maxima vary substantially, with typical averages ranging between 10–4 and 10–5 individuals per meter square (m2), in different regions of the Indo-Pacific (Neo et al., 2017). Only in very few cases, T. maxima has been reported in much higher densities, for example for some reefs in French Polynesia with averages of 1.6–2.7 individuals m–2 (Andréfouët et al., 2009) and a maximum of 544 individuals per m2 (Gilbert et al., 2005).
Large variability of the abundance of Tridacninae could be explained by several environmental and biogeographic factors. The availability of light is crucial for these clams, as they stand out among bivalve mollusks due to their symbiotic relationship with dinoflagellates of the Symbiodiniaceae family (Yonge, 1936; Taylor, 1969; LaJeunesse et al., 2018). Due to their photo-symbiotic relationship, and the resulting mixotrophic feeding strategy (Jantzen et al., 2008), Tridacninae can even reach a net photoautotrophic metabolism (Klumpp et al., 1992; Klumpp and Griffiths, 1994). Processes of light-enhanced calcification are presumed to be the main reason why Tridacninae show high calcification rates (Ip et al., 2017b, 2018; Boo et al., 2019; Chew et al., 2019; Rossbach et al., 2019) and their subsequent impressive sizes. The requirement for photosynthetically active radiation (PAR, 400–700 nm) of their algal symbionts restricts giant clams to sunlit, shallow waters, where they are – at the same time – exposed to potentially high levels of ultraviolet (UV) radiation (Overmans and Agustí, 2020; Rossbach et al., 2020b) and high water temperatures (Andréfouët et al., 2013).
Existing studies on densities of giant clams across depth report the highest numbers of Tridacninae per m2 in shallow reefs (<10 m), principally reef flats and the reef edge (Van Wynsberge et al., 2016; Rossbach et al., 2019). The recently described, strong light-dependency of calcification in Red Sea giant clams (Rossbach et al., 2019), may therefore be among the main drivers which restrict the depth-distribution of Tridacninae within the reef. In addition to the availability of light, sea temperatures (Apte et al., 2019) and wave exposure are also important environmental factors for symbiotic benthic organisms (Williams et al., 2013), and are potentially impacting the overall abundances of Tridacninae. Other habitat parameters, for example the substrate and overall geomorphology of the reef could influence larval settlement success, as giant clam larvae have been reported to favor high substrate rugosity (e.g., on coral rubble) to settle (Alcazar and Solis, 1986; Neo et al., 2009). Furthermore, at a regional spatial scale, the “reef-type” seems to have a major influence on giant clam abundances (Andréfouët et al., 2009). For example, higher densities are found in enclosed vs. open atoll reefs, and inside lagoons rather than at the outer reef slope (Van Wynsberge et al., 2016). Due to their specific habitat preference (Yonge, 1975; Hart et al., 1998) and their presumed longevity (Chambers, 2007), giant clams are exceedingly vulnerable to exploitation (e.g., harvest for food and souvenirs) and environmental degradation, caused by human activities and climate change (Ashworth et al., 2004; Van Wynsberge et al., 2016). Consequently, giant clam densities are reported to show a negative correlation with local human population size per surface area of shallow reef, which could be mainly explained by a higher fishing pressure, usually associated with a larger population (Van Wynsberge et al., 2016). Yet, in general, the extent to which all these different factors potentially affect the distribution and local abundances of giant clams, has been rather poorly assessed so far (Van Wynsberge et al., 2016). This holds true for the entire geographical Indo-Pacific distribution range of Tridacninae, but especially for poorly scientifically surveyed coral reefs, such as those in the Red Sea (as reviewed by Van Wynsberge et al., 2016).
The Red Sea presents distinct natural latitudinal gradients of temperature (Chaidez et al., 2017), salinity (Arz et al., 2003) and nutrient availability (Sawall et al., 2015), all of them displaying a high spatial variability (Ngugi et al., 2012). Surface water temperatures are overall high and increase toward the South, with maximum sea surface temperatures ranging from 33.0 to 33.9°C during the summer (Chaidez et al., 2017; Agulles et al., 2020). Red Sea coral reefs have also repeatedly faced thermal stress associated to heatwaves, especially in the southern region (Monroe et al., 2018; Osman et al., 2018; Anton et al., 2020). Although waters in the North are more transparent to wavelengths of the UV spectrum than those in the southern Red Sea, they receive in general less intense solar radiation due to the higher latitude (Overmans and Agustí, 2019). Thus, overall maximum UV doses, received in the water column at both, northern and southern Red Sea reefs might be comparable (Overmans and Agustí, 2019). There are also two wind patterns in the region: In the North, the wind systems remains relatively constant throughout the year, with winds coming predominantly from the North-West (Sofianos and Johns, 2002; Zarokanellos et al., 2017). In the South, the wind system is additionally regulated by the dynamics of the Indian monsoon, and the wind changes the direction to South-East during the northern hemisphere winter (Al Saafani and Shenoi, 2004; Sofianos and Johns, 2015).
Red Sea coral reefs also face a number of anthropogenic threats, including unsustainable tourism (Gladstone et al., 2013), habitat destruction (Badr et al., 2009), and overfishing (Hasan, 2005; Spaet and Berumen, 2015). Unlike the Asian Pacific region, where Tridacninae are still commercially harvested (Lucas, 1994; Larson, 2016), the collection of giant clams was prohibited by the Saudi Arabian Wildlife Commission in the early 2000s (Gladstone, 2000; AbuZinada et al., 2004), thus protecting local Tridacninae communities in the eastern Red Sea for the past two decades.
To date, densities of giant clams in the Red Sea have been mostly assessed for northern latitudes, including the Egyptian Red Sea coast (Zuschin et al., 2001; Ullmann, 2013) and the Gulf of Aqaba (Roa-Quiaoit, 2005), as well as at a few scattered reefs along the Saudi Arabian Red Sea coast (Roa-Quiaoit, 2005; Rossbach et al., 2019). However, except for Rossbach et al. (2019), none of these previous studies assessed the depth-related distribution of Tridacna spp., although previous research clearly indicates that depth can have a significant impact on the density estimates of Tridacninae (Van Wynsberge et al., 2016; Rossbach et al., 2019).
Here we present a large-scale survey of the density of Tridacna spp. in the eastern Red Sea, where we assessed the number of individuals m–2 of reef, along approximately 1,300 km of the Saudi Arabian Red Sea coastline (ranging from 29 to 18°N). We further aim to identify the most important environmental and biogeographical factors (e.g., light availability, water temperature, wave exposure, and distance to shore) that could potentially influence the overall densities of Tridacninae in the eastern Red Sea.
Materials and Methods
Abundance Surveys
Between December 2016 and April 2019, we visited 58 different reefs along the Saudi Arabian Red Sea coast (Figure 1A, see Supplementary Figure 1 for a more detailed map), and performed a total of 661 belt transects on Tridacna spp. densities, either via snorkeling or SCUBA diving. The surveyed reefs were categorized according to their geographical location, ranging from reefs at 29°N in the Gulf of Aqaba to 18°N at the southern Farasan Banks, and grouped into the northern (25°, 27°, and 29°N), central (21°, 22°, and 24°N), and southern (18°, 19°, and 20°N) Red Sea (according to Raitsos et al., 2013; Figure 1A). We also considered the distance to shore, as reefs were located either nearshore (<10 km away from shore), midshore (10–20 km), or offshore (>20 km) (according to Furby et al., 2013; Figure 1B). At a respective reef, we would further differentiate between the windward or leeward site of the reef, dependent on the predominant exposure to wind (according to Al Saafani and Shenoi, 2004; Figure 1B). At both sites (i.e., windward and leeward), we conducted 30 m long belt transects, recording all Tridacna specimens within a 1 m2 wide corridor (i.e., 30 m2 for each transect; Figure 1C), covering a total Red Sea coral reef area of 20,976 m2. Belt transects have been established as a standard surveying method, as their ease of use and adaptable length has been shown to be most suitable to assess the density range of Tridacna spp., and its habitat substratum configurations (Van Wynsberge et al., 2016). The belt transects were conducted at different depths, between 0.5 and 15 m, depending on the depth extension of the respective reef, with a minimum of six and a maximum of 21 transects per site (Figure 1D). We grouped the transects then into three categories, i.e., conducted at depths shallower than 2 m, between 2 and 6.5 m and deeper than 6.5 m (Figure 1D) and also considered the reef architecture, as transects covered the reef flat, the edge, and the slope (Figure 1D), with slopes having an inclination of more than 50 degrees being considered as “steep slopes.”
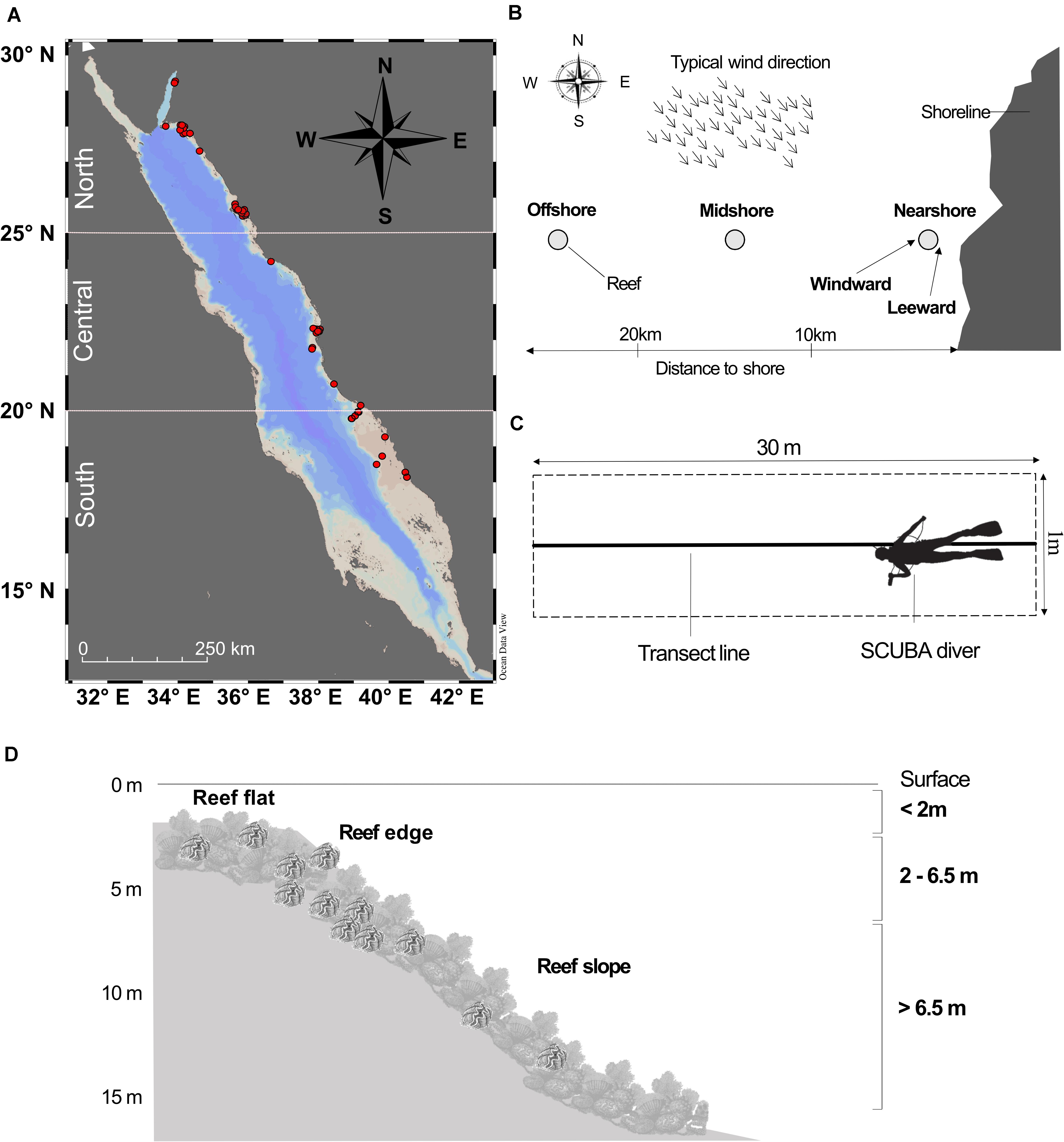
Figure 1. (A) Map of Red Sea, highlighted in red are the 58 surveyed reefs along the Saudi Arabian coastline, (B) Schematic of surveyed reefs located nearshore (<10 km from the shore), midshore (10–20 km), and offshore (>20 km), indicating the windward and leeward side (according to predominant northwesterly winds in the Red Sea). (C) Illustration of a SCUBA diver conducting a belt transect. (D) Schematic reef with reef flat, edge and slope, highlighting the three surveyed depth zones, >2, 2–6.5, and >6.5 m.
Temperature Data
Annually averaged water temperatures of the last 10 years (2007–2017) were extracted from the corresponding data set of Agulles et al. (2020). Their 3-D gridded temperature product (TEMPERSEA), with a spatial resolution of 0.25° × 0.25°, compares satellite observations of sea surface temperature and reconstructs sea temperatures in the upper layer (for depths <100 m). We used these averaged annually water temperatures from depths shallower than 5 m, at 5–10 m, and in those deeper than 10 m, an additional factor in the analysis of potential drivers for Tridacna spp. densities in the Red Sea.
Data Filtering
For the potential regional predictors of clam densities, we used the following broad-scale physical variables for each of the surveyed reefs: (A) latitude, i.e., southern (18°, 19°, and 20°N), central (21°, 22°, and 24°N) and northern Red Sea (25°, 27°, and 29°N), (B) distance to shore, i.e., nearshore (<10 km away from shore), midshore (10–20 km), and offshore reefs (>20 km), (C) predominant exposure to wind, i.e., windward and leeward sites, (D) annually averaged water temperatures of the last ten years, (E) depth, i.e., <2, 2–6.5, and >6.5 m, and (F) reef zone, i.e., reef flat, edge, slope, and steep slope (where the inclination was more than 50 degrees).
Statistical Analyses
We used generalized mixed-effects linear models with the lme() function from the nlme R package (Version 3.6.1.; Pinheiro et al., 2015) adding reef ID as a random factor because several transects were deployed on each site. We first performed simple mixed-effects models for each potential driver of the densities of Tridacna spp. independently to assess their effects at the basin level. All these simple mixed-models were linear regressions (y∼x) of categorical variables except for temperature, which was included as a continuous variable, and analyzed using a polynomial type 2 model (y∼x + x2). We used a likelihood ratio test to assess the significance of the fixed variables at p-values <0.05. To assess differences across levels of treatment we performed a post hoc Tuckey test using the lsmeans () package (Lenth and Hervé, 2015). Statistical significance was reported at p-values <0.05.
We then performed a more complex exploration of the potential drivers for Tridacna spp. densities, by combining their effects in a single model. To do this, we first examined potential correlations between independent predictors (Supplementary Figure 2) and, if correlated, selected the best independent variable based on the Akaike Information Criterion (AIC; Supplementary Table 1). We started with a model that included all independent variables and the paired interactions between them, and reef as a random factor (Supplementary Table 2). After that, we followed a step-wise model simplification approach to find the most parsimonious model (Crawley, 2012), using AIC to exclude predictors in the model (Burnham and Anderson, 2004; Supplementary Table 2). The R-square of the model was calculated using the r.squaredGLMM() function from the MuMIn R package. Again, we used a likelihood ratio test to assess the significance of the fixed variables and a post hoc Tuckey test to assess differences across levels of treatment at p-values <0.05. All graphs were created using the ggplot2 R package (Wickham, 2009).
Results
We recorded a total of 4,002 Tridacna spp. clams, with an overall average (±SD) density of 0.19 ± 0.43 individuals m–2 and a median density of 0.07 individuals m–2 (The full dataset can be assessed in the PANGEA repository at doi.org/10.1594/PANGAEA.921114).
The majority of the individuals (89%) were identified as T. maxima (Figure 2A), while only about 11% were T. squamosa specimens (Figure 2B). In addition, we found a few specimens (n = 6) of the extremely rare T. squamosina (Figure 2C) at one single reef in the southern Red Sea. On average, and for the entire surveyed coastline, the density of the ‘small giant clam’ T. maxima was 0.17 ± 0.37 individuals m–2 (Figure 2D). However, densities varied substantially between reefs, with up to 3.03 ± 1.28 individuals m–2 at a few reefs located in the Al Wajd Lagoon (northern Red Sea), to no clams being present at some reefs in the Farasan Banks (southern Red Sea). Specimens of T. squamosa were present at 45 reefs and with an overall density of 0.02 ± 0.07 individuals m–2.
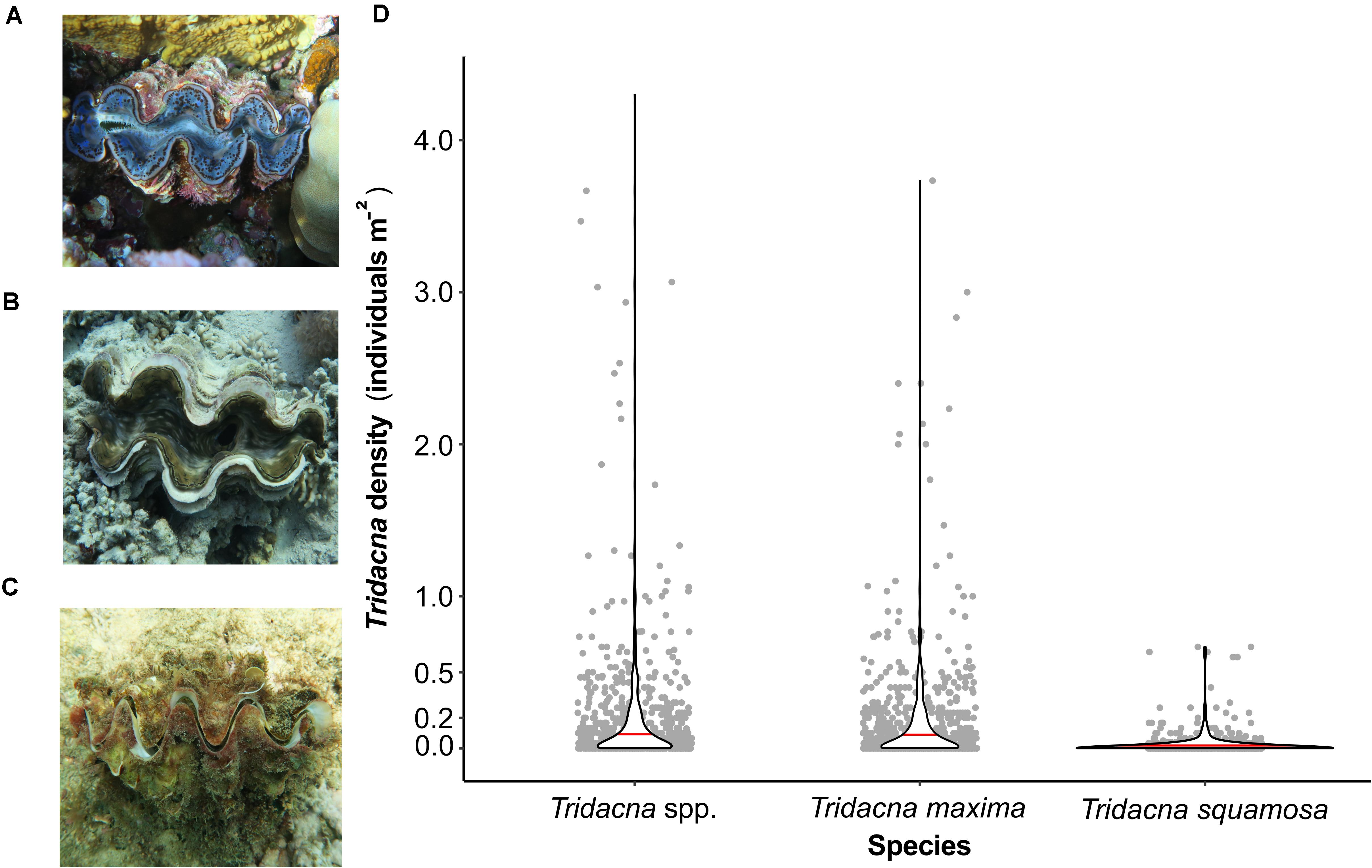
Figure 2. Photos of Tridacna spp. specimens with (A) Tridacna maxima, (B) Tridacna squamosa, and (C) Tridacna squamosina. (D) Densities of Tridacna spp., T. maxima and T. squamosa as individuals per m– 2 along the Saudi Arabian Red Sea coast. Gray dots symbolize densities of Tridacninae at each conducted transect, the red line indicates the averaged density.
Drivers of Tridacna spp. Density
Densities (±SD) of Tridacna spp. showed a strong North-South gradient, with significantly more clams present in the northern reefs (>25°) with 0.46 ± 0.84 individuals m–2, than in the South (18°, 19°, and 20°N), where the average density was 0.10 ± 0.20 individuals m–2 (F-value = -3.28, p-value = 0.005, Figure 3A and Supplementary Tables 2, 3). In the central Red Sea (21°–24°N), averaged Tridacna spp. density was 0.17 ± 0.23 individuals m–2. The distance to the shore had a significant influence on giant clam densities, with more clams being present in reefs located nearshore (i.e., <10 km away from shore) than at reefs located offshore (i.e., >20 km away from the coast) (Figure 3B, F-value = 2.84, p-value = 0.017, Supplementary Tables 2, 3).
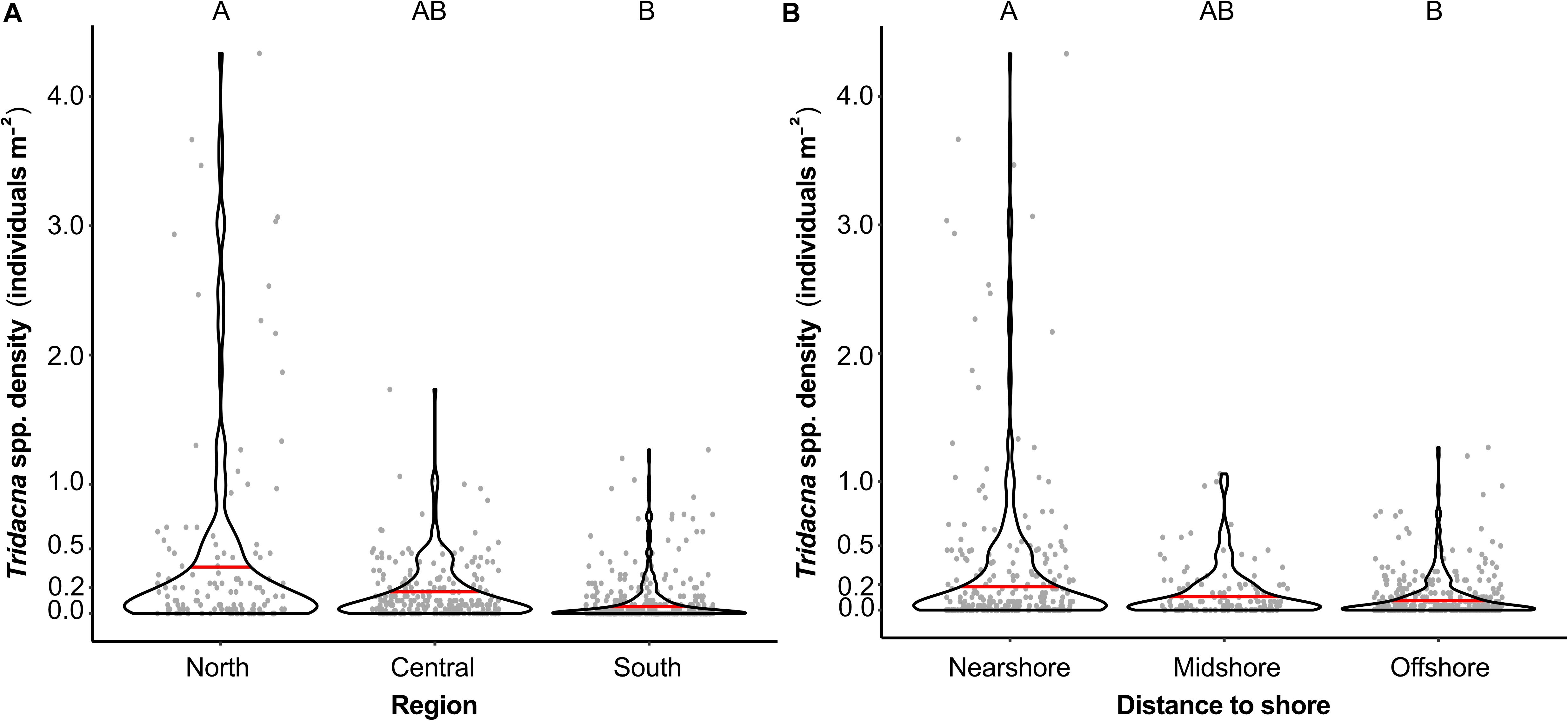
Figure 3. Tridacna spp. densities as individuals per m2 at (A) reefs in the northern (25°, 27°, and 29°), central (21°, 22°, and 24°) and southern (18°, 19°, and 20°) Red Sea and at (B) surveyed reefs located nearshore (<10 km), midshore (10–20 km), and offshore (>20 km). Gray dots symbolize densities of Tridacninae at each conducted transect, the red line indicates the averaged density. Different capital letters indicate statistically significant differences in density (p < 0.05).
Within a given reef, we found a number of drivers that, mostly due to their correlation with each other and/or co-occurrence, had a significant influence on the density of Tridacna spp. giant clams in Red Sea coral reefs (Supplementary Tables 2, 3). When examining the specific environmental factors of wind exposure (i.e., sites located wind - or leeward), we observed significantly higher densities of Tridacna spp. at the leeward side of reefs (Figure 4A) (F = 23.14, p < 0.001). There was also a relationship between annually averaged temperature and Tridacna spp. density (Figure 4B), and our empirical data suggest that the thermal optimum for Tridacna spp. densities in Red Sea coral reefs is at 26.3°C (Figure 4B). This is also reflecting the North-South gradients in the Red Sea, as all of the reefs experiencing mean annual temperatures above 27°C were located in the southern Red Sea (Supplementary Figure 2). When comparing Tridacna spp. densities between different depths (Figure 4C), we found that the giant clams were significantly more abundant in waters shallower than 6.5 m (i.e., at <2 m and between 2 and 6.5 m; p < 0.001; respective F values can be found in Supplementary Table 3) in comparison to deeper depths (i.e., >6.5 m). Furthermore, we found significantly less Tridacna spp. specimens at the reef slope than at the flat (F = 4.77, p < 0.001) and edge (F = 5.59, p < 0.001) (Figure 4D), and even fewer clams at steep reef slopes, in comparison to the reef flat (F = 5.32, p < 0.001) and edge (F = 6.09, p < 0.001).
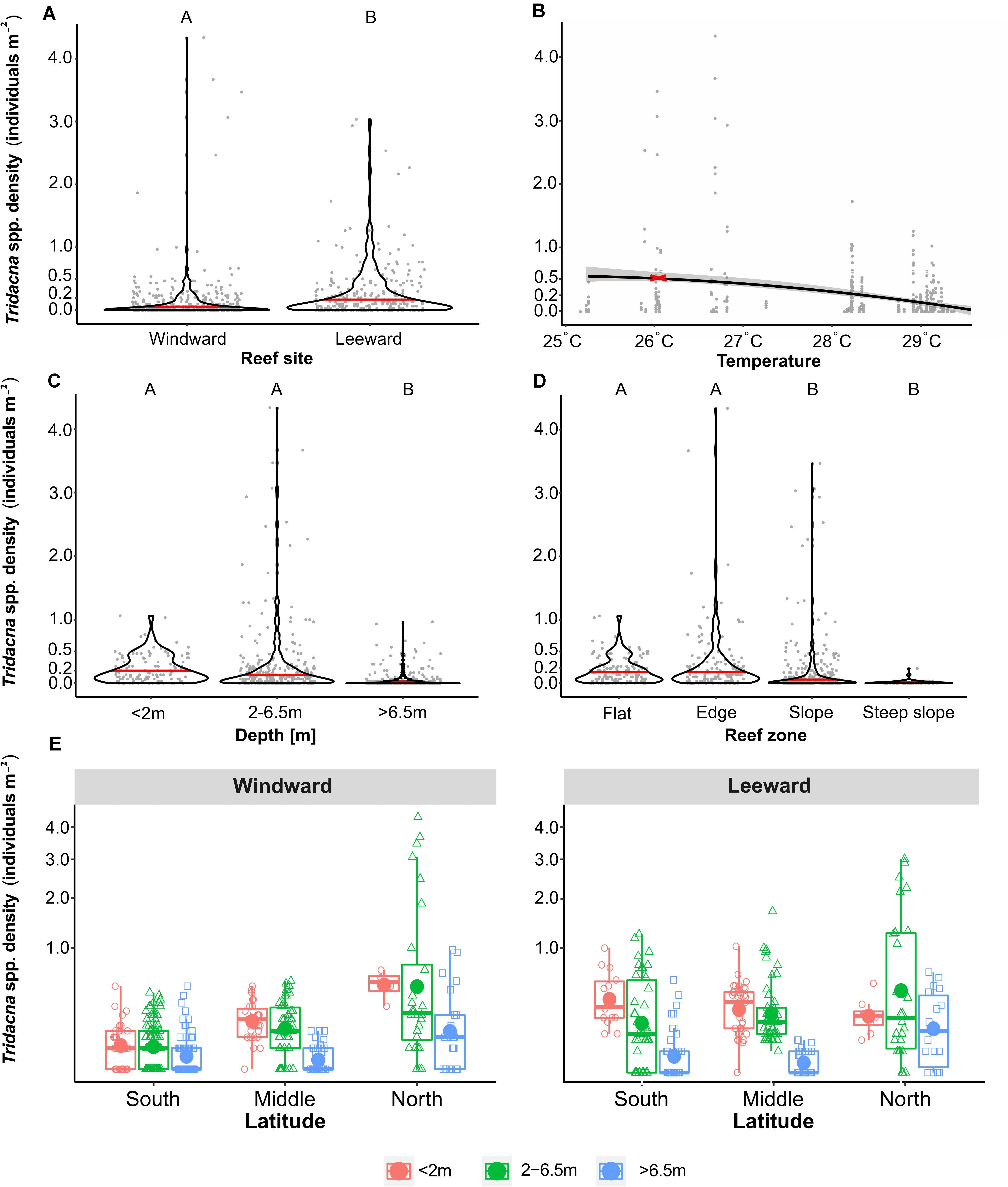
Figure 4. Tridacna spp. densities as individuals per m2, plotted for (A) wind exposure (windward and leeward reef site), (B) annually averaged ambient water temperatures (°C) with an optimum temperature at 26.3°C, highlighted with an red cross, (C) depth (<2, 2–6.5, and >6.5 m), as well as the (D) reef zone (flat, edge, slope, and steep slope). Gray dots symbolize densities of Tridacninae at each conducted transect, the red line indicates the averaged density. Different capital letters indicate statistically significant differences in density (p < 0.05). Panel (E) shows data for each individual belt transect and for the different latitudes (southern, central, and northern Red Sea), wind exposure (leeward and windward), and depth zone (<2, 2–6.5, and >6.5 m) as boxplots, including the median (line) and average (dot) density.
To address the correlation and co-occurrence of the independent drivers, which we identified to have a significant influence on the densities of Red Sea Tridacna spp. (Supplementary Tables 2, 3) we ran a model testing the effect of geographical location, meaning the latitude at which the reef was located (i.e., southern, central, and northern), the effect of the site, depending on the wind exposure (i.e., leeward or windward), and the depth (i.e., <2, 2–6.5, and >6.5 m), as well as their interactions (Figure 4E and Table 1) on clam density. The model revealed Tridacna spp. densities to vary significantly with latitude, wind exposure and depth, as well as significant interactions between these three factors (Table 1 and Supplementary Table 2). Overall, the generalized linear model including these variables, interaction terms and random factor, accounted for 86% of the variability in Tridacninae densities in the eastern Red Sea (R2 = 0.859).
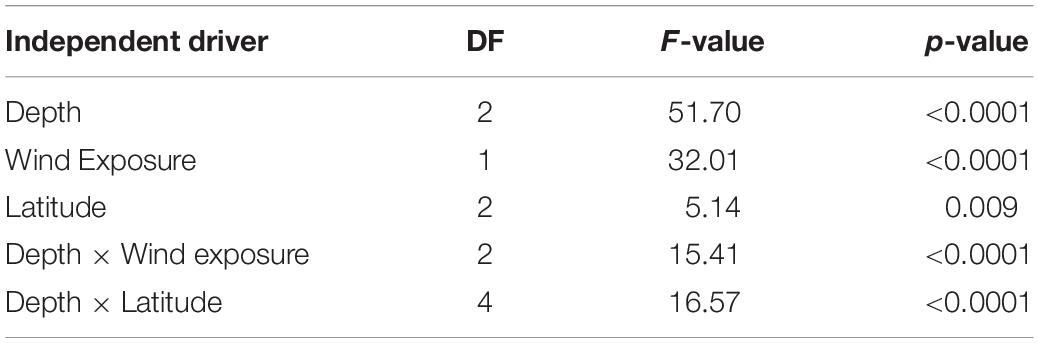
Table 1. Results of the generalized mixed-effects linear models, testing the independent drivers of Tridacna spp. Densities.
Discussion
Observed Tridacninae Species
During the fieldwork and SCUBA dives for the present study, we encountered all three giant clam species that had been previously reported for the Red Sea, i.e., T. maxima, T. squamosa, and T. squamosina (Figures 2A–C). While we observed T. maxima and T. squamosa along the entire study area (i.e., Saudi Arabian Red Sea coastline from the northern part of the Gulf of Aqaba to the southern Farasan banks), we found T. squamosina exclusively in a single location in the southern Red Sea. To date, there are only two other reports on the presence of this species in the region, from the Gulf of Aqaba (Richter et al., 2008) and from a single location at the Farasan Islands (Fauvelot et al., 2020). In accordance to our findings, these previous surveys report on the extremely low density of this species (e.g., 0.9 ± 0.4 individuals per 1,000 m2 in the Gulf of Aqaba; Richter et al., 2008) and although it has been reported that T. squamosina presents up to >80% of the fossil Tridacninae shells in the Red Sea (Richter et al., 2008), nowadays this species presumably represents less than 1% of the present stocks. It is not yet clear what caused this putatively steep population decline of T. squamosina, but the extremely rare sightings of this species may warrant legal protection, such as being added to the IUCN Red List. Densities of, and species allocation between the two other species, T. maxima (89% of individuals), and T. squamosa (11%) were consistent with previous reports on Red Sea Tridacninae (e.g., Roa-Quiaoit, 2005; Ullmann, 2013; Pappas et al., 2017).
Overall Abundance of Tridacna spp.
Densities of Tridacna spp. in the Red Sea showed a strong latitudinal gradient, decreasing nearly 5-fold from North to South. Locally, densities were occasionally very high, for example with up to 3.03 ± 1.28 T. maxima individuals m–2 at a reef in the Al Wajd lagoon in the northern Red Sea, matching the highest Tridacna spp. densities reported in the literature (Table 2; Andréfouët et al., 2005, 2009).
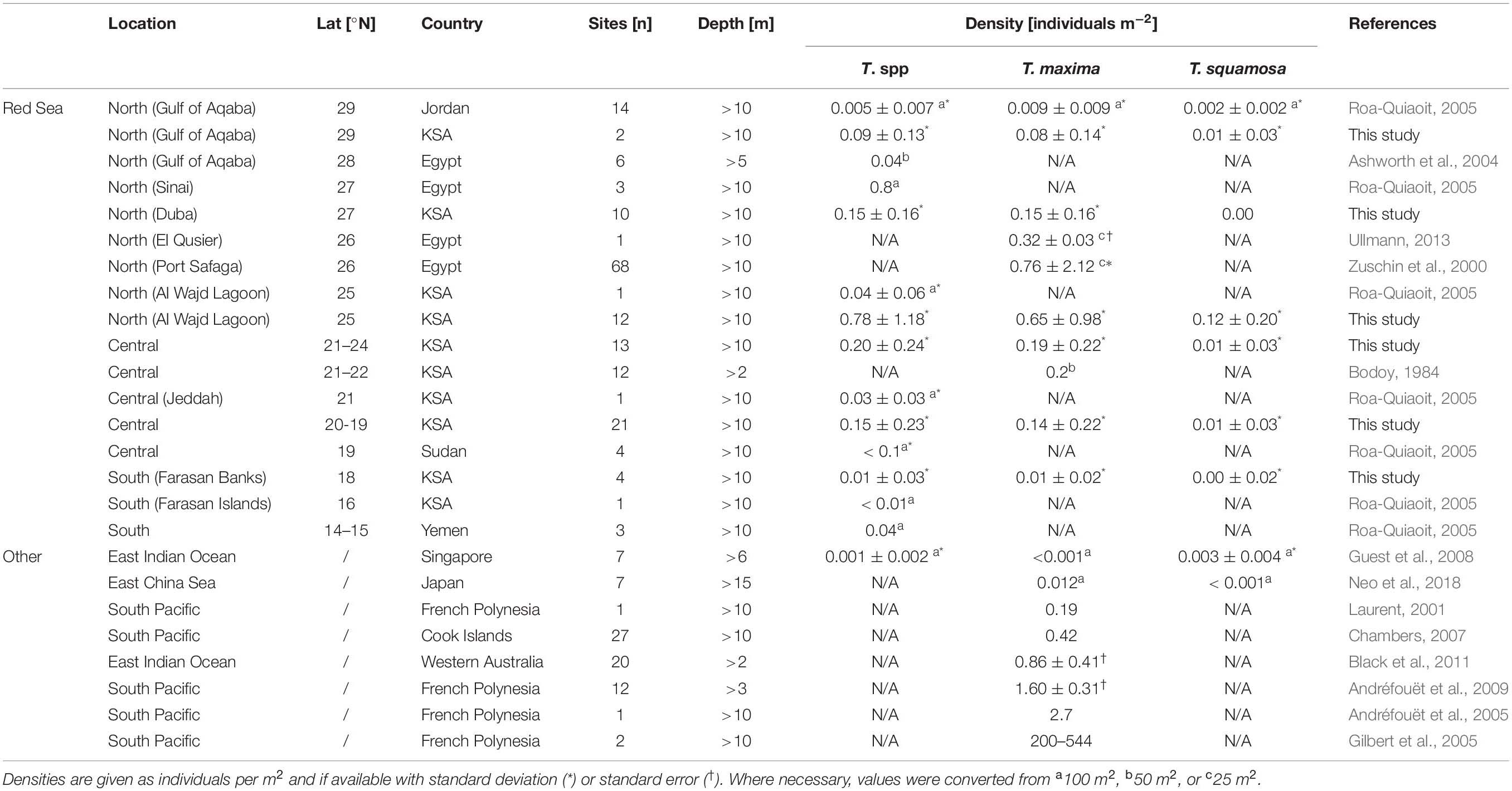
Table 2. Comparison of reports on average densities of Tridacna maxima, Tridacna squamosa, and Tridacna spp. (pooled data for both species) in the Red Sea and globally.
In the northern and central part of the Saudi Arabian Red Sea coast, the reef systems are typically characterized by fringing reefs with steep drop-offs and interrupted offshore barrier reefs (Montaggioni et al., 1986; Edwards et al., 1987). In contrast, the southern region is more characterized by less-developed fringing reefs, and shallow bays and lagoons, which are often bordered by mangroves, extensive reef lagoons and nutrient-rich waters (Edwards et al., 1987; Raitsos et al., 2013). The observed latitudinal North-South gradient of giant clam abundance is consistent with previous reports on Tridacna spp. in the Red Sea (Roa-Quiaoit, 2005). However, comparing the present survey data with previous studies from the region also highlights the occasionally strong variances between and among different sites (Table 2). In some occasion, for example when comparing the assessed abundances within the Al Wajd Lagoon in the northern Red Sea, reported densities of Tridacna spp., by Roa-Quiaoit (2005), are approximately 15 times lower (0.04 ± 0.06 clams m–2; ±SD) than those recorded in the present study (0.60 ± 1.03 clams m–2). This difference is most likely due to two reasons: (1) the specific variability of environmental factors (e.g., wave exposure and light availability) among sites, which probably influences the suitability of the reef for settlement and the thriving of Tridacna spp., as discussed hereafter; and (2) the differences in sampling effort, especially with regard to survey depths, since most previous studies only surveyed at one or few depths (e.g., Bodoy, 1984; Ashworth et al., 2004; Roa-Quiaoit, 2005), while the present study covered seven different depths from the surface to 15 m. These differences may explain the discrepancy in observed numbers, as it has been shown that the depth of these surveys can significantly impact abundance estimates (Van Wynsberge et al., 2016).
Globally, the averaged densities of T. maxima typically range between 10–4 and 10–5 individuals m–2 (Neo et al., 2017). Therefore, the data obtained for the present study suggest that the averaged densities of T. maxima in the Saudi Arabian Red Sea are well within the upper range, with 0.17 ± 0.37 individuals m–2, but with locally up to 3.03 ± 1.28 clams m–2 (e.g., at some reefs in the Al Wajd lagoon). Only a few exceptional studies report higher densities of this species, for example in some reefs of French Polynesia, with reports of averaged densities between 1.6 to 2.7 individuals m–2 (Andréfouët et al., 2009), but locally up to 544 individuals m–2 (Gilbert et al., 2005), as well as for the Ningaloo Marine Park in Western Australia with 0.86 ± 0.41 individuals m–2 (Black et al., 2011), and 0.42 individuals m–2 in Kiribati (Chambers, 2007; Table 2). For T. squamosa, surveys on abundances are in general scarce, but the few available reports present numbers that were 40–95% (Guest et al., 2008; Neo et al., 2018; respectively) lower compared to the average number found in eastern Red Sea reefs in this present study (Table 2).
Yet, when comparing Tridacna spp. densities on a global scale and across regions, it is important to consider the latest revisions of the Tridacninae taxonomy, including the recent evidence for high cryptic diversity (Kubo and Iwai, 2007; Huelsken et al., 2013; Fauvelot et al., 2020). Especially for regions that are inhabited by a number of different giant clam species (e.g., the Coral Triangle, Western Pacific and the Eastern Indian Ocean), previous density estimates for T. maxima, the species with the broadest geographical distribution (bin Othman et al., 2010), may thus be under- or over- estimated (Johnson et al., 2016; Van Wynsberge et al., 2017). This could be particularly true where reefs are also inhabited by the recently resurrected Tridacna noae (Su et al., 2014), as this species has been reported to be frequently misidentified as T. maxima (Su et al., 2014; Borsa et al., 2015; Neo and Low, 2017). Occasional confusion of T. maxima and T. squamosa have been also previously suspected for the Red Sea (Pappas et al., 2017). However, as the same observers as in the present study, reached a very high and genetically confirmed accuracy in previous species-targeted sampling (Lim et al., 2020), and since the presented ratio of 89% T. maxima and 11% T. squamosa clams corresponds to past reports on species allocation in the region (Jantzen et al., 2008; Pappas et al., 2017), we are confident that presented numbers actually reflect what can be found in Red Sea coral reefs.
Drivers for Abundances of Tridacna spp. in the Red Sea
To compare observed densities from the present study with previous assessments from the Red Sea (Zuschin et al., 2000; Ashworth et al., 2004; Roa-Quiaoit, 2005; Ullmann, 2013) which mainly report on genus level only, we then pooled densities of T. maxima and T. squamosa, considering them as Tridacna spp. for the analysis on potential drivers influencing the abundances of Red Sea Tridacninae.
We identified a number of drivers that, mostly in combination and/or due to their correlation and co-occurrence, have a significant influence on the densities of Tridacna spp. in Red Sea coral reefs (Supplementary Figure 2). The Red Sea area is characterized by a relatively stable and uniform wind system, with consistent northwesterly winds blowing over the sea throughout the year, except for the reversal in the South during the northern hemisphere winter (Sofianos and Johns, 2002; Zarokanellos et al., 2017). Red Sea coral reefs are mainly of the fringing type (Montaggioni et al., 1986), and grow close to the mainland (Mergner and Schuhmacher, 1974; Brachert and Dullo, 1991), with a predominantly windward located fore reef zone and a leeward located back reef (Montaggioni et al., 1986). We found Tridacna spp. to be significantly more abundant in these leeward, sheltered parts of the reef, which is consistent with previous reports on giant clam densities in other regions worldwide (Andréfouët et al., 2009; Van Wynsberge et al., 2016). In general, also the “reef type” may have a substantial effect on the densities of giant clams (Andréfouët et al., 2009; Van Wynsberge et al., 2016).
There are a number of environmental factors that can potentially influence the density of clams in the shallow waters of lagoons and the back-reef. For instance, a potentially reduced water-exchange could lead to a decrease in oxygenation. In fact, it was suggested that the mass mortality events of giant clams in French Polynesia in 2009/2010 were the result of prolonged periods of low swell and low winds that prevented a renewal of the lagoonal water (Andréfouët et al., 2015; Van Wynsberge et al., 2017). For the back-reef of Red Sea fringing reefs, however, it was reported that there are no, or only very infrequent, long-term stagnations (leading to an irregular inflow of oxygenated water) in the innermost parts of the reefs (Montaggioni et al., 1986). Thus, giant clams in shallow Red Sea reefs are less likely to be exposed to occasional low-oxygen levels (Giomi et al., 2019). Sheltered conditions inside the reef lagoon may even support the larval supply, resulting in higher densities inside these sheltered lagoons, in comparison to the outer reef slope, as suggested by Van Wynsberge et al. (2016). This would be further supported by our findings that Red Sea Tridacna spp. can be found in highest densities at reefs located inside the Al Wajd lagoon, located in the central/northeastern part of the Red Sea, which is characterized by extensive coral reef formations with a live coral cover that ranges between ∼30 and ∼70% (Chalastani et al., 2020). Occasionally, however, the temperature in shallow waters can become very high, especially during the summer months, which can potentially cause bleaching (i.e., the expulsion of the symbiotic microalgae) by the clams (Addessi, 2001; Leggat et al., 2003). Additionally, high water temperatures have been previously shown to result in lower overall survival of Tridacninae larvae (Neo et al., 2013; Enricuso et al., 2018) and a general increase in mortality (Addessi, 2001; Junchompoo et al., 2013; Apte et al., 2019). Indeed, we also identified water temperature as a potential driver influencing the densities of Tridacna spp. in the Red Sea. The highest density of clams was estimated at an average yearly water temperature of 26.3°C, which was reflected in a decrease in densities from North to South, where the average annual temperatures are higher than in the northern Red Sea (Supplementary Figure 1; Chaidez et al., 2017; Agulles et al., 2020). The recent experimental findings that water temperatures above 30.7°C can lead to a strong bleaching response in T. maxima (Brahmi et al., 2019) and the reported negative effect of elevated seawater temperatures on the embryonic and larval development of T. gigas (Enricuso et al., 2019) and T. squamosa clams (Eckman et al., 2019) further support our findings of a rather low yearly mean thermal optimum of Tridacninae. In the oligotrophic waters of the Red Sea, giant clams in shallow waters are potentially also exposed to high levels of incident radiation, in particular highly energetic UV (Overmans and Agustí, 2020). Yet, recent studies suggest that giant clams are well adapted to these short wavelengths and developed different photo-defensive mechanisms (Ishikura et al., 1997; Rossbach et al., 2020a, b) allowing them to thrive even under high solar irradiances.
The availability of photosynthetically active radiation within the PAR spectrum (400–700 nm), however, has been previously suggested to influence the depth-related abundances of Tridacninae (Jantzen et al., 2008; Rossbach et al., 2019). Our data likewise demonstrate a strong depth-dependency of giant clam densities, with significantly less individuals in depths below 6.5 m. As the survey depth can have a significant impact on the estimates of Tridacna spp. abundances, with expected higher densities reported for shallow reefs (i.e., 0–5 m; Van Wynsberge et al., 2016), this might again explain the differences between previously reported densities of Red Sea giant clams (e.g., Roa-Quiaoit, 2005) and those in the present study. As photosymbiotic organisms, and occasionally even functional phototrophs (Klumpp and Griffiths, 1994; Jantzen et al., 2008), Tridacna spp. rely on their symbionts and their photosynthetic activity to yield their carbon respiratory requirements (Trench et al., 1981; Klumpp et al., 1992) and promote their high calcification rates (Ip et al., 2017a, b; Boo et al., 2019; Chew et al., 2019; Rossbach et al., 2019). Their distribution may, therefore, also be influenced by underwater light penetration (Schuhmacher, 1973), as variations in suspension load in the reefs has been shown to fundamentally influence the abundances of Tridacna spp. (Zuschin and Piller, 1997). This could also provide a further explanation for the latitudinal decrease in abundances from North to South. Tridacninae in the northern and central Red Sea can be found on narrow shelves, where the reefs are exposed to clear oceanic waters (Richter and Abu-Hilal, 2006) while the reef waters in the southern Red Sea are more turbid due to higher amounts of suspended particles (Kotb et al., 2004) in these nutrient- and plankton-rich waters.
Particularly the significant differences in densities of Tridacna spp. according to the reef zone (i.e., flat, edge, slope, and steep slope) highlights the intertwining of drivers that together modulate their abundance. The clams can be mainly found at reef flats and the edge, where they receive sufficiently high incident light levels, which are required by their algal symbionts to support and maintain their high metabolic rates. However, the high temperature and irradiance, which the clams might occasionally experience in shallow waters, could explain why most of the clams can be found on the reef edge. Here, the clams receive sufficient incident light, while being simultaneously exposed to a constant flow of well-oxygenated and cooler water. This pattern concurs with the previous report by Ashworth et al. (2004), who also observed higher numbers of Tridacna spp. at the reef edge. We found that giant clam numbers decreased with depth along the reef slope and that clams were significantly less abundant at steep reef slopes. There are a number of possible explanations and potential causes. For example the topography of the settling substrate, as it has been reported that giant clam larvae, like those of corals, prefer to settle on substrates which provide shelter via grooves, pits and crevices (Petersen et al., 2005; Neo et al., 2009), where they (as they further develop) penetrate the substrates (mostly mechanically but probably supported by chemical boring) and attach themselves permanently with their byssus threads (Yonge, 1962). The slopes of a coral reef might thus be less suitable for their settlement. At steep slopes, reef organisms also receive only limited amounts of incident light (Vermeij and Bak, 2002; Lesser et al., 2018), due to shading of the reef wall, which may influence their densities. Furthermore, in the Red Sea, steep outer reef slopes are mostly found at offshore reefs, which is where we observed lower clam density. It is therefore not possible to unravel if the abundances of Tridacna spp. are reduced at offshore reefs because of a co-occurrence of factors, including higher proportion of steep slopes at these reefs.
Globally, giant clam populations have been reported to be highly vulnerable to anthropogenic over-exploitation (Lewis et al., 1988; Lucas, 1994; Gilbert et al., 2006; Larson, 2016), as they can be easily accessed, especially in shallow reefs (Ashworth et al., 2004). Indeed, fishing pressure may have been a driver for Red Sea Tridacna spp. abundances in previous times (as reported by Bodoy, 1984; Gladstone, 2000; and Ashworth et al., 2004). Yet, since the collection of giant clams has been banned by the Saudi Arabian Wildlife Commission since the early 2000s (Gladstone, 2000; AbuZinada et al., 2004), and after consultation with several local fishermen at various sites along the Saudi Arabian coastline (S.R., personal communication), confirming that Tridacninae are not targeted in local fisheries since the ban, we presume that harvesting pressure is most likely not among the drivers for giant clam densities in the eastern Red Sea.
Contribution of Tridacninae to Calcium Carbonate Budgets
Coral reefs, due to the biological activity of their communities, play an important role in the oceanic carbon budget. Although scleractinian corals are certainly the main contributors to the calcium carbonate budget of reefs, other calcifying organisms, including giant clams are also contributing. As reported previously, the average net calcification of T. maxima in shallow reefs (<10 m) is about 0.47 ± 0.03 μmol CaCO3 cm–2 of mantle surface per hour, and averaged mantle surface area of clams in these depths is 140 cm2 (Rossbach et al., 2019). Therefore, considering the average density of T. maxima of 0.21 ± 0.40 clams m–2 in shallow depths (<10 m) in Red Sea reefs reported here, giant clams account for approximately 0.3% of the total reef area. Their estimated contribution to the overall reef net calcification would be therefore about 14 μmol CaCO3 m–2 h–1, resulting in 114 kg CaCO3 ha–1 year–1. Given that overall calcification rates of entire Red Sea coral reefs communities are estimated to be around 16.425 kg CaCO3 ha–1 year–1 (Silverman et al., 2007), giant clams may thus contribute to about 0.7% to overall reef calcification. Locally, at those reefs with highest abundances (i.e., in the Al Wajd lagoon), they may even contribute up to 9%.
Conclusion
In summary, it is evident that there is not one main, but a number of different geographical (i.e., latitude and distance to shore) and local environmental drivers (i.e., depth and reef zone) that influence Tridacna spp. densities in the Red Sea. Their co-occurrence and the complexity of many drivers might also explain the high variation in Tridacninae densities across different sites, which is congruent with existing reports on Tridacna spp. densities from other parts of the world, where censuses revealed comparably large variations among sites (e.g., in Western Australia; Black et al., 2011). Only the latest insights on their overall abundances, provided by this study, in combination with the recent efforts in understanding the population structures and dynamics of Tridacninae in the region (Lim et al., 2020) can give a comprehensive picture of their status in the Red Sea. The presented data on the large-scale abundances of Tridacna spp. in Saudi Arabian Red Sea waters may therefore serve as a baseline to understand the importance of this charismatic reef invertebrate for Red Sea coral reefs, and to assess future trends. It may also contribute to the conservation efforts from local to regional scales and eventually aid the protection of Tridacninae in the entire Red Sea and elsewhere.
Data Availability Statement
The datasets presented in this study can be found in online repositories. The names of the repository/repositories and accession number(s) can be found below: doi: 10.1594/PANGAEA.921114.
Author Contributions
CD and SR conceptualized the research. SR performed the abundance surveys. AA and SR conducted the data curation and ran formal statistical analyses. SR prepared the first draft of the manuscript and all the co-authors contributed substantially to subsequent versions, including the final draft. All authors contributed to the article and approved the submitted version.
Funding
This work was funded by King Abdullah University of Science and Technology (KAUST), through baseline funding to CD (BAS/1/1071-01-01).
Conflict of Interest
The authors declare that the research was conducted in the absence of any commercial or financial relationships that could be construed as a potential conflict of interest.
Acknowledgments
We thank Aislinn Dunne, Alejandra Ortega, Cecilia Martin, Felix Ivo Rossbach, Janna Leigh Randle, Silvia Arossa, Vincent Saderne, and Walther Rich IV and for assistance with the abundance transects, and the KAUST Coastal and Marine Resources Core Lab for logistical support in the field. We also thank Sebastian Overmans for his help with an early version of the manuscript. Further, we thank Gabriel Jorda and Miguel Agulles for the provision of the average water temperature data. We also thank Beacon Development Company for the provision of some of their transect data in the Red Sea.
Supplementary Material
The Supplementary Material for this article can be found online at: https://www.frontiersin.org/articles/10.3389/fmars.2020.592852/full#supplementary-material
References
AbuZinada, A., Robinson, E., Nader, I., and Al Wetaid, Y. (2004). First Saudi Arabian National Report on the Convention on Biological Diversity. Riyadh: The National Commission for Wildlife Conservation and Development.
Addessi, L. (2001). Giant clam bleaching in the lagoon of Takapoto atoll (French Polynesia). Coral Reefs 19:220. doi: 10.1007/pl00006957
Agulles, M., Jordà, G., Jones, B., Agustí, S., and Duarte, C. M. (2020). Temporal evolution of temperatures in the Red Sea and the Gulf of Aden based on in situ observations (1958–2017). Ocean Sci. 16, 149–166. doi: 10.5194/os-16-149-2020
Al Saafani, M., and Shenoi, S. (2004). Seasonal cycle of hydrography in the Bab el Mandab region, southern Red Sea. J. Earth Syst. Sci. 113, 269–280. doi: 10.1007/bf02716725
Alcazar, S., and Solis, E. (1986). Spawning, larval rearing and early growth of Tridacna maxima (Röding) (Bivalvia: Tridacnidae). Silliman J. 33, 65–73.
Alcazar, S. N. (1986). Observations on predators of giant clams (Bivalvia: Family Tridacnidae). Silliman J. 33, 54–57.
Andréfouët, S., Friedman, K., Gilbert, A., and Remoissenet, G. (2009). A comparison of two surveys of invertebrates at Pacific Ocean islands: the giant clam at Raivavae Island, Australes Archipelago, French Polynesia. ICES J. Mar. Sci. 66, 1825–1836. doi: 10.1093/icesjms/fsp148
Andréfouët, S., Dutheil, C., Menkes, C. E., Bador, M., and Lengaigne, M. (2015). Mass mortality events in atoll lagoons: environmental control and increased future vulnerability. Glob. Chang. Biol. 21, 195–205. doi: 10.1111/gcb.12699
Andréfouët, S., Gilbert, A., Yan, L., Remoissenet, G., Payri, C., and Chancerelle, Y. (2005). The remarkable population size of the endangered clam Tridacna maxima assessed in Fangatau Atoll (Eastern Tuamotu, French Polynesia) using in situ and remote sensing data. ICES J. Mar. Sci. 62, 1037–1048. doi: 10.1016/j.icesjms.2005.04.006
Andréfouët, S., Van Wynsberge, S., Gaertner-Mazouni, N., Menkes, C., Gilbert, A., and Remoissenet, G. (2013). Climate variability and massive mortalities challenge giant clam conservation and management efforts in French Polynesia atolls. Biol. Conserv. 160, 190–199. doi: 10.1016/j.biocon.2013.01.017
Anton, A., Randle, J. L., Garcia, F. C., Rossbach, S., Ellis, J. I., Weinzierl, M., et al. (2020). Differential thermal tolerance between algae and corals may trigger the proliferation of algae in coral reefs. Glob. Chang. Biol. 26, 4316–4327. doi: 10.1111/gcb.15141
Apte, D., Narayana, S., and Sutirtha, D. (2019). Impact of sea surface temperature anomalies on giant clam population dynamics in Lakshadweep reefs: inferences from a fourteen years study. Ecol. Indic. 107:105604. doi: 10.1016/j.ecolind.2019.105604
Arz, H. W., Lamy, F., Pätzold, J., Müller, P. J., and Prins, M. (2003). Mediterranean moisture source for an early-Holocene humid period in the northern Red Sea. Science 300, 118–121. doi: 10.1126/science.1080325
Ashworth, J. S., Ormond, R. F., and Sturrock, H. T. (2004). Effects of reef-top gathering and fishing on invertebrate abundance across take and no-take zones. J. Exp. Mar. Biol. Ecol. 303, 221–242. doi: 10.1016/j.jembe.2003.11.017
Badr, N. B., El-Fiky, A. A., Mostafa, A. R., and Al-Mur, B. A. (2009). Metal pollution records in core sediments of some Red Sea coastal areas, Kingdom of Saudi Arabia. Environ. Monit. Assess. 155, 509–526. doi: 10.1007/s10661-008-0452-x
Beckvar, N. (1981). Cultivation, spawning, and growth of the giant clams Tridacna gigas, T. derasa, and T. squamosa in Palau, Caroline Islands. Aquaculture 24, 21–30. doi: 10.1016/0044-8486(81)90040-5
bin Othman, A. S., Goh, G. H., and Todd, P. A. (2010). The distribution and status of giant clams (family Tridacnidae)- a short review. Raffles Bull. Zool. 58, 103–111.
Black, R., Johnson, M. S., Prince, J., Brearley, A., and Bond, T. (2011). Evidence of large, local variations in recruitment and mortality in the small giant clam, Tridacna maxima, at Ningaloo Marine Park, Western Australia. Mar. Freshw. Res. 62, 1318–1326. doi: 10.1071/mf11093
Bodoy, A. (1984). “Assessment of human impact on giant clams, Tridacna maxima, near Jeddah, Saudi Arabia,” in Proceedings of the Symposium on Coral Reef Environment of the Red Sea, Jeddah, Saudi Arabia, January 1984, Jeddah, 472–490.
Bonham, K. (1965). Growth rate of giant clam Tridacna gigas at Bikini Atoll as revealed by radioautography. Science 149, 300–302. doi: 10.1126/science.149.3681.300
Boo, M. V., Hiong, K. C., Wong, W. P., Chew, S. F., and Ip, Y. K. (2019). Shell formation in the giant clam, Tridacna squamosa, may involve an apical Na+/Ca 2+ exchanger 3 homolog in the shell-facing epithelium of the whitish inner mantle, which displays light-enhanced gene and protein expression. Coral Reefs 38, 1173–1186. doi: 10.1007/s00338-019-01848-y
Borsa, P., Fauvelot, C., Andréfouët, S., Chai, T.-T., Kubo, H., and Liu, L.-L. (2015). On the validity of Noah’s giant clam Tridacna noae (Röding, 1798) and its synonymy with Ningaloo giant clam Tridacna ningaloo Penny & Willan, 2014. Raffles Bull. Zool. 63, 484–489.
Brachert, T. C., and Dullo, W.-C. (1991). Laminar micrite crusts and associated foreslope processes, Red Sea. J. Sediment. Res. 61, 354–363.
Brahmi, C., Chapron, L., Le Moullac, G., Soyez, C., Beliaeff, B., Lazareth, C. E., et al. (2019). Effects of temperature and pCO2 on the respiration, biomineralization and photophysiology of the giant clam Tridacna maxima. bioRxiv [Preprint]. 672907,Google Scholar
Burnham, K. P., and Anderson, D. R. (2004). Multimodel inference: understanding AIC and BIC in model selection. Sociol. Methods Res. 33, 261–304. doi: 10.1177/0049124104268644
Chaidez, V., Dreano, D., Agusti, S., Duarte, C. M., and Hoteit, I. (2017). Decadal trends in Red Sea maximum surface temperature. Sci. Rep. 7:8144.
Chalastani, V. I., Manetos, P., Al-Suwailem, A. M., Hale, J. A., Vijayan, A. P., Pagano, J., et al. (2020). Reconciling tourism development and conservation outcomes through marine spatial planning for a Saudi giga-project in the Red Sea (The Red Sea Project, Vision 2030). Front. Mar. Sci. 7:168. doi: 10.3389/fmars.2020.00168
Chambers, C. N. (2007). Pasua (Tridacna maxima) size and abundance in Tongareva Lagoon, Cook Islands. SPC Trochus Inform. Bull. 13, 7–12.
Chew, S. F., Koh, C. Z., Hiong, K. C., Choo, C. Y., Wong, W. P., Neo, M. L., et al. (2019). Light-enhanced expression of Carbonic Anhydrase 4-like supports shell formation in the fluted giant clam Tridacna squamosa. Gene 683, 101–112. doi: 10.1016/j.gene.2018.10.023
De Grave, S. (1999). Pontoniinae (Crustacea: Decapoda: Palaemonidea) associated with bivalve molluscs from Hansa Bay, Papua New Guinea. Bull. Institut R. Sci. Nat. Belgique Biol. 69, 125–141.
Eckman, W., Vicentuan, K., and Todd, P. A. (2019). Effects of low light and high temperature on pediveligers of the fluted giant clam Tridacna squamosa. Mar. Freshw. Behav. Physiol. 52, 255–264. doi: 10.1080/10236244.2019.1700117
Edwards, A., Head, S., Braithwaite, C., Edwards, F., Karbe, L., Weikert, H., et al. (1987). Key Environments Red Sea, ed. A. Edwards (Oxford: Pergamon Press), 45–68.
Enricuso, O. B., Conaco, C., Sayco, S. L. G., Neo, M. L., and Cabaitan, P. C. (2018). Elevated seawater temperatures affect embryonic and larval development in the giant clam Tridacna gigas (Cardiidae: Tridacninae). J. Molluscan Stud. 85, 66–72. doi: 10.1093/mollus/eyy051
Enricuso, O. B., Conaco, C., Sayco, S. L. G., Neo, M. L., and Cabaitan, P. C. (2019). Elevated seawater temperatures affect embryonic and larval development in the giant clam Tridacna gigas (Cardiidae: Tridacninae). J. Molluscan Stud. 85, 66–72.
Fauvelot, C., Zuccon, D., Borsa, P., Grulois, D., Magalon, H., Riquet, F., et al. (2020). Phylogeographical patterns and a cryptic species provide new insights into Western Indian Ocean giant clams phylogenetic relationships and colonization history. J. Biogeogr. 47, 1086–1105. doi: 10.1111/jbi.13797
Furby, K. A., Bouwmeester, J., and Berumen, M. L. (2013). Susceptibility of central Red Sea corals during a major bleaching event. Coral Reefs 32, 505–513. doi: 10.1007/s00338-012-0998-5
Gilbert, A., Remoissenet, G., Yan, L., and Andrefouet, S. (2006). Special traits and promises of the giant clam (Tridacna maxima) in French Polynesia. Fish. Newsl. South Pac. Comm. 118, 44.
Gilbert, A., Yan, L., Remoissenet, G., Andrefouët, S., Payri, C., and Chancerelle, Y. (2005). Extraordinarily high giant clam density under protection in Tatakoto atoll (Eastern Tuamotu archipelago, French Polynesia). Coral Reefs 24, 495–495. doi: 10.1007/s00338-005-0494-2
Giomi, F., Barausse, A., Duarte, C. M., Booth, J., Agusti, S., Saderne, V., et al. (2019). Oxygen supersaturation protects coastal marine fauna from ocean warming. Sci. Adv. 5:eaax1814. doi: 10.1126/sciadv.aax1814
Gladstone, W. (2000). The ecological and social basis for management of a Red Sea marine-protected area. Ocean Coast. Manag. 43, 1015–1032. doi: 10.1016/s0964-5691(00)00070-3
Gladstone, W., Curley, B., and Shokri, M. R. (2013). Environmental impacts of tourism in the Gulf and the Red Sea. Mar. Pollut. Bull. 72, 375–388. doi: 10.1016/j.marpolbul.2012.09.017
Guest, J. R., Todd, P. A., Goh, E., Sivalonganathan, B. S., and Reddy, K. P. (2008). Can giant clam (Tridacna squamosa) populations be restored on Singapore’s heavily impacted coral reefs? Aquat. Conserv. 18, 570–579. doi: 10.1002/aqc.888
Hart, A. M., Bell, J. D., and Foyle, T. P. (1998). Growth and survival of the giant clams, Tridacna derasa, T. maxima and T. crocea, at village farms in the Solomon Islands. Aquaculture 165, 203–220. doi: 10.1016/s0044-8486(98)00255-5
Hasan, M. H. (2005). Destruction of a Holothuria scabra population by overfishing at Abu Rhamada Island in the Red Sea. Mar. Environ. Res. 60, 489–511. doi: 10.1016/j.marenvres.2004.12.007
Huber, M., and Eschner, A. (2010). Tridacna (Chametrachea) costata Roa-Quiaoit, Kochzius, Jantzen, Al-Zibdah & Richter from the Red Sea, a junior synonym of Tridacna squamosina Sturany, 1899 (Bivalvia, Tridacnidae). Ann. Nat. Mus. Wien Ser. B Bot. Zool. 112, 153–162.
Huelsken, T., Keyse, J., Liggins, L., Penny, S., Treml, E. A., and Riginos, C. (2013). A novel widespread cryptic species and phylogeographic patterns within several giant clam species (Cardiidae: Tridacna) from the Indo-Pacific Ocean. PLoS One 8:e80858. doi: 10.1371/journal.pone.0080858
Ip, Y. K., Koh, C. Z., Hiong, K. C., Choo, C. Y., Boo, M. V., Wong, W. P., et al. (2017a). Carbonic anhydrase 2−like in the giant clam, Tridacna squamosa: characterization, localization, response to light, and possible role in the transport of inorganic carbon from the host to its symbionts. Physiol. Rep. 5:e13494. doi: 10.14814/phy2.13494
Ip, Y. K., Hiong, K. C., Goh, E. J. K., Boo, M. V., Choo, C. Y. L., Ching, B., et al. (2017b). The whitish inner mantle of the giant clam, Tridacna squamosa, expresses an apical plasma membrane Ca2+-ATPase (PMCA) which displays light-dependent gene and protein expressions. Front. Physiol. 8:781. doi: 10.3389/fphys.2017.00781
Ip, Y. K., Hiong, K. C., Lim, L. J., Choo, C. Y., Boo, M. V., Wong, W. P., et al. (2018). Molecular characterization, light-dependent expression, and cellular localization of a host vacuolar-type H+-ATPase (VHA) subunit A in the giant clam, Tridacna squamosa, indicate the involvement of the host VHA in the uptake of inorganic carbon and its supply to the symbiotic zooxanthellae. Gene 659, 137–148. doi: 10.1016/j.gene.2018.03.054
Ishikura, M., Kato, C., and Maruyama, T. (1997). UV-absorbing substances in zooxanthellate and azooxanthellate clams. Mar. Biol. 128, 649–655. doi: 10.1007/s002270050131
IUCN (2016). Red List of Threatened Species. Available online at: wwwiucnredlistorg Version 2016-3 (accessed August 1, 2020).
Jantzen, C., Wild, C., El-Zibdah, M., Roa-Quiaoit, H. A., Haacke, C., and Richter, C. (2008). Photosynthetic performance of giant clams, Tridacna maxima and T. squamosa, Red Sea. Mar. Biol. 155, 211–221. doi: 10.1007/s00227-008-1019-7
Johnson, M. S., Prince, J., Brearley, A., Rosser, N. L., and Black, R. (2016). Is Tridacna maxima (Bivalvia: Tridacnidae) at Ningaloo Reef, Western Australia? Molluscan Res. 36, 264–270. doi: 10.1080/13235818.2016.1181141
Junchompoo, C., Sinrapsasan, N., Penpain, C., and Patsorn, P. (2013). “Changing seawater temperature effects on giant clams bleaching, Mannai Island, Rayong province, Thailand,” in Proceedings of the Design Symposium on Conservation of Ecosystem 2013, BANGKOK, 71–76.
Klumpp, D. W., and Griffiths, C. L. (1994). Contributions of phototrophic and heterotrophic nutrition to the metabolic and growth requirements of four species of giant clam (Tridacnidae). Mar. Ecol. Prog. Ser. 115, 103–115. doi: 10.3354/meps115103
Klumpp, D. W., Bayne, B. L., and Hawkins, A. J. S. (1992). Nutrition of the giant clam Tridacna gigas (L.) I. Contribution of filter feeding and photosynthates to respiration and growth. J. Exp. Mar. Biol. Ecol. 155, 105–122. doi: 10.1016/0022-0981(92)90030-e
Kotb, M., Abdulaziz, M., Al-Agwan, Z., Alshaikh, K., Al-Yami, H., Banajah, A., et al. (2004). Status of Coral Reefs in the RED Sea and Gulf of Aden in 2004, Vol. 70. Townsville, QLD: Global Coral Reef Monitoring Network and Australian Institute of Marine Science, 137–139.
Kubo, H., and Iwai, K. (2007). On two sympatric species within Tridacna “maxima”. Ann. Rep. Okinawa Fish. Ocean Res. Cent. 68, 205–210.
LaJeunesse, T. C., Parkinson, J. E., Gabrielson, P. W., Jeong, H. J., Reimer, J. D., Voolstra, C. R., et al. (2018). Systematic revision of symbiodiniaceae highlights the antiquity and diversity of coral endosymbionts. Curr. Biol. 28, 2570–2580. doi: 10.1016/j.cub.2018.07.008
Laurent, V. (2001). Etude de Stocks, Relations Biométriques et Structure des Populations de Bénitiers, Tridacna Maxima, dans trois lagons de Polynésie Française (Moorea, Takapoto et Anaa), Rapport de fin d’études. Rennes: ENSAR, 45.
Larson, C. (2016). Shell trade pushes giant clams to the brink. Science 351, 323–324. doi: 10.1126/science.351.6271.323
Leggat, W., Buck, B. H., Grice, A., and Yellowlees, D. (2003). The impact of bleaching on the metabolic contribution of dinoflagellate symbionts to their giant clam host. Plant Cell Environ. 26, 1951–1961. doi: 10.1046/j.0016-8025.2003.01111.x
Lesser, M. P., Slattery, M., and Mobley, C. D. (2018). Biodiversity and functional ecology of mesophotic coral reefs. Ann. Rev. Ecol. Evol. Syst. 49, 49–71. doi: 10.1146/annurev-ecolsys-110617-062423
Lewis, A., Adams, T., and Ledua, E. (1988). Fiji’s giant clam stocks- a review of their distribution, abundance, exploitation and management. ACIAR Monogr. Ser. 98, 66–72.
Lim, K. K., Rossbach, S., Geraldi, N. R., Schmidt-Roach, S., Serrao, E. A., and Duarte, C. M. (2020). The small giant clam, Tridacna maxima exhibits minimal population genetic structure in the Red Sea and genetic differentiation from the Gulf of Aden. Front. Mar. Sci. 7:889. doi: 10.3389/fmars.2020.570361
Lucas, J. S. (1994). The biology, exploitation, and mariculture of giant clams (Tridacnidae). Rev. Fish. sci. 2, 181–223. doi: 10.1080/10641269409388557
Mergner, H., and Schuhmacher, H. (1974). Morphologie, ökologie und zonierung von korallenriffen bei aqaba,(Golf von Aqaba, Rotes Meer). Helgoländer Wissenschaftliche Meeresuntersuchungen 26, 238–358. doi: 10.1007/bf01627619
Monroe, A. A., Ziegler, M., Roik, A., Röthig, T., Hardenstine, R. S., Emms, M. A., et al. (2018). In situ observations of coral bleaching in the central Saudi Arabian Red Sea during the 2015/2016 global coral bleaching event. PLoS One 13:e0195814. doi: 10.1371/journal.pone.0195814
Montaggioni, L., Behairy, A., El-Sayed, M. K., and Yusuf, N. (1986). The modern reef complex, Jeddah area, Red Sea: a facies model for carbonate sedimentation on embryonic passive margins. Coral Reefs 5, 127–150. doi: 10.1007/bf00298180
Neo, M., Todd, P., Teo, S., and Chou, L. (2013). The effects of diet, temperature and salinity on survival of larvae of the fluted giant clam, Tridacna squamosa. J. Conchol. 4, 369–376.
Neo, M. L., and Low, J. K. (2017). First observations of Tridacna noae (Röding, 1798)(Bivalvia: Heterodonta: Cardiidae) in Christmas Island (Indian Ocean). Mar. Biodivers. 48, 2183–2185. doi: 10.1007/s12526-017-0678-3
Neo, M. L., Todd, P. A., Teo, S. L.-M., and Chou, L. M. (2009). Can artificial substrates enriched with crustose coralline algae enhance larval settlement and recruitment in the fluted giant clam (Tridacna squamosa)? Hydrobiologia 625, 83–90. doi: 10.1007/s10750-008-9698-0
Neo, M. L., Liu, L.-L., Huang, D., and Soong, K. (2018). Thriving populations with low genetic diversity in giant clam species, Tridacna maxima and Tridacna noae, at Dongsha Atoll, South China Sea. Reg. Stud. Mar. Sci. 24, 278–287. doi: 10.1016/j.rsma.2018.09.001
Neo, M. L., Eckman, W., Vicentuan, K., Teo, S. L. M., and Todd, P. A. (2015). The ecological significance of giant clams in coral reef ecosystems. Biol. Conserv. 181, 111–123. doi: 10.1016/j.biocon.2014.11.004
Neo, M. L., Wabnitz, C. C., Braley, R. D., Heslinga, G. A., Fauvelot, C., Van Wynsberge, S., et al. (2017). Giant clams (Bivalvia: Cardiidae: Tridacninae): a comprehensive update of species and their distribution, current threats and conservation status. Oceanogr. Mar. Biol. 55, 87–388. doi: 10.1201/b21944-5
Ngugi, D. K., Antunes, A., Brune, A., and Stingl, U. (2012). Biogeography of pelagic bacterioplankton across an antagonistic temperature–salinity gradient in the Red Sea. Mol. Ecol. 21, 388–405. doi: 10.1111/j.1365-294x.2011.05378.x
Osman, E. O., Smith, D. J., Ziegler, M., Kürten, B., Conrad, C., El−Haddad, K. M., et al. (2018). Thermal refugia against coral bleaching throughout the northern Red Sea. Glob. Change Biol. 24, e474–e484.
Overmans, S., and Agustí, S. (2019). Latitudinal gradient of UV attenuation along the highly transparent Red Sea Basin. Photochem. Photobiol. 95, 1267–1279. doi: 10.1111/php.13112
Overmans, S., and Agustí, S. (2020). Unraveling the seasonality of UV exposure in reef waters of a rapidly warming (sub-) tropical sea. Front. Mar. Sci. 7:111. doi: 10.3389/fmars.2020.00111
Pappas, M. K., He, S., Hardenstine, R. S., Kanee, H., and Berumen, M. L. (2017). Genetic diversity of giant clams (Tridacna spp.) and their associated Symbiodinium in the central Red Sea. Mar. Biodiv. 47, 1–14. doi: 10.1007/978-3-319-23534-9_1
Petersen, D., Laterveer, M., and Schuhmacher, H. (2005). Spatial and temporal variation in larval settlement of reefbuilding corals in mariculture. Aquaculture 249, 317–327. doi: 10.1016/j.aquaculture.2005.04.048
Pinheiro, J., Bates, D., DebRoy, S., Sarkar, D., and Team, R. C. (2015). nlme: Linear and Nonlinear Mixed Effects Models. R Package Version 3.
Raitsos, D. E., Pradhan, Y., Brewin, R. J., Stenchikov, G., and Hoteit, I. (2013). Remote sensing the phytoplankton seasonal succession of the Red Sea. PLoS One 8:e64909. doi: 10.1371/journal.pone.0064909
Richter, C., and Abu-Hilal, A. (2006). “Seas of the Arabian Region,” in The Sea, Ideas and Observations on Progress in the Study of the Seas. 14, The Global Coastal Ocean. Interdisciplinary Regional Studies and Syntheses, Part B: The Coasts of Africa, Europe, Middle East, Oceania and Polar Regions, eds A. R. Robinson, and K. H. Brink (New York, NY: John Wiley & Sons), 1373–1412.
Richter, C., Roa-Quiaoit, H., Jantzen, C., Al-Zibdah, M., and Kochzius, M. (2008). Collapse of a new living species of giant clam in the Red Sea. Curr. Biol. 18, 1349–1354. doi: 10.1016/j.cub.2008.07.060
Roa-Quiaoit, H. A. F. (2005). The Ecology and Culture of Giant Clams (Tridacnidae) in the Jordanian Sector of the Gulf of Aqaba, Red Sea. Ph D. thesis, Universitat Bremen, Bremen.
Rossbach, S., Saderne, V., Anton, A., and Duarte, C. M. (2019). Light-dependent calcification in Red Sea giant clam Tridacna maxima. Biogeosciences 16, 2635–2650. doi: 10.5194/bg-16-2635-2019
Rossbach, S., Subedi, R. C., Ng, T. K., Ooi, B. S., and Duarte, C. M. (2020a). Iridocytes mediate photonic cooperation between giant clams (Tridacninae) and their photosynthetic symbionts. Front. Mar. Sci. 7:465. doi: 10.3389/fmars.2020.00465
Rossbach, S., Overmans, S., Kaidarova, A., Kosel, J., Agustí, S., and Duarte, C. M. (2020b). Giant clams in shallow reefs: UV-resistance mechanisms of Tridacninae in the Red Sea. Coral Reefs 39, 1345–1360. doi: 10.1007/s00338-020-01968-w
Sawall, Y., Al-Sofyani, A., Hohn, S., Banguera-Hinestroza, E., Voolstra, C. R., and Wahl, M. (2015). Extensive phenotypic plasticity of a Red Sea coral over a strong latitudinal temperature gradient suggests limited acclimatization potential to warming. Sci. Rep. 5:8940.
Schuhmacher, H. (1973). Light-dependent colonization of pillars by sessile animals and algae from coral reef at Eilat (Red Sea). Helgolander Wissenschaftliche Meeresuntersuchungen 24, 307–326. doi: 10.3354/meps263307
Silverman, J., Lazar, B., and Erez, J. (2007). Effect of aragonite saturation, temperature, and nutrients on the community calcification rate of a coral reef. J. Geophys. Res. 112:C05004.
Sofianos, S., and Johns, W. E. (2015). “Water mass formation, overturning circulation, and the exchange of the Red Sea with the adjacent basins,” in The Red Sea, eds N. Rasul and I. Stewart (Berlin: Springer), 343–353. doi: 10.1007/978-3-662-45201-1_20
Sofianos, S. S., and Johns, W. E. (2002). An oceanic general circulation model (OGCM) investigation of the Red Sea circulation, 1. Exchange between the Red Sea and the Indian Ocean. J. Geophys. Res. 107, 17-1-17-11.
Spaet, J. L., and Berumen, M. L. (2015). Fish market surveys indicate unsustainable elasmobranch fisheries in the Saudi Arabian Red Sea. Fish. Res. 161, 356–364. doi: 10.1016/j.fishres.2014.08.022
Su, Y., Hung, J.-H., Kubo, H., and Liu, L.-L. (2014). Tridacna noae (Röding, 1798) –a valid giant clam species separated from T. maxima (Röding, 1798) by morphological and genetic data. Raffles Bull. Zool. 62, 143–154.
Taylor, D. L. (1969). Identity of zooxanthellae isolated from some Pacific Tridacnidae. J. Phycol. 5, 336–340. doi: 10.1111/j.1529-8817.1969.tb02623.x
Trench, R., Wethey, D., and Porter, J. (1981). Observations on the symbiosis with zooxanthellae among the Tridacnidae (Mollusca, Bivalvia). Biol. Bull. 161, 180–198. doi: 10.2307/1541117
Ullmann, J. (2013). Population status of giant clams (Mollusca: Tridacnidae) in the northern Red Sea, Egypt. Zool. Middle East 59, 253–260. doi: 10.1080/09397140.2013.842307
Van Wynsberge, S., Andréfouët, S., Gaertner-Mazouni, N., Wabnitz, C. C. C., Gilbert, A., Remoissenet, G., et al. (2016). Drivers of density for the exploited giant clam Tridacna maxima: a meta-analysis. Fish Fish. 17, 567–584. doi: 10.1111/faf.12127
Van Wynsberge, S., Andréfouët, S., Gaertner-Mazouni, N., Wabnitz, C. C., Menoud, M., Le Moullac, G., et al. (2017). Growth, survival and reproduction of the giant clam Tridacna maxima (Röding 1798, Bivalvia) in two contrasting Lagoons in French Polynesia. PLoS One 12:e0170565. doi: 10.1371/journal.pone.0170565
Vermeij, M., and Bak, R. (2002). How are coral populations structured by light? Marine light regimes and the distribution of Madracis. Mar. Ecol. Prog. Ser. 233, 105–116. doi: 10.3354/meps233105
Vicentuan-Cabaitan, K., Neo, M. L., Eckman, W., Teo, S. L. M., and Todd, P. A. (2014). Giant clam shells host a multitude of epibionts. Bull. Mar. Sci. 90, 795–796. doi: 10.5343/bms.2014.1010
Wickham, H. (2009). ggplot2: Elegant Graphics for Data Analysis. New York, NY: Springer-Verlag, 2009.
Williams, G. J., Smith, J. E., Conklin, E. J., Gove, J. M., Sala, E., and Sandin, S. A. (2013). Benthic communities at two remote Pacific coral reefs: effects of reef habitat, depth, and wave energy gradients on spatial patterns. PeerJ 1:e81. doi: 10.7717/peerj.81
Yonge, C. M. (1936). Mode of life, feeding, digesting and symbiosis with zooxanthellae. Sci. Rep. Great Barrier Reef Expedition 1, 283–321.
Yonge, C. M. (1962). On the primitive significance of the byssus in the Bivalvia and its effects in evolution. J. Mar. Biol. Assoc. U K 42, 113–125. doi: 10.1017/S0025315400004495
Zarokanellos, N. D., Papadopoulos, V. P., Sofianos, S. S., and Jones, B. H. (2017). Physical and biological characteristics of the winter-summer transition in the Central Red Sea. J. Geophys. Res. 122, 6355–6370. doi: 10.1002/2017jc012882
Zuschin, M., and Piller, W. E. (1997). Bivalve distribution on coral carpets in the northern Bay of Safaga (Red Sea, Egypt) and its relation to environmental parameters. Facies 37, 183–194. doi: 10.1007/bf02537378
Zuschin, M., Hohenegger, J., and Steininger, F. F. (2000). A comparison of living and dead molluscs on coral reef associated hard substrata in the northern Red Sea—implications for the fossil record. Palaeogeogr. Palaeoclimatol. Palaeoecol. 159, 167–190. doi: 10.1016/s0031-0182(00)00045-6
Keywords: Tridacna, giant clam, Red Sea, abundance, reef
Citation: Rossbach S, Anton A and Duarte CM (2021) Drivers of the Abundance of Tridacna spp. Giant Clams in the Red Sea. Front. Mar. Sci. 7:592852. doi: 10.3389/fmars.2020.592852
Received: 08 August 2020; Accepted: 14 December 2020;
Published: 14 January 2021.
Edited by:
Yehuda Benayahu, Tel Aviv University, IsraelReviewed by:
Dominic A. Andradi-Brown, World Wildlife Fund, United StatesSimon Van Wynsberge, Institut Français de Recherche pour l’Exploitation de la Mer (IFREMER), France
S. F. Chew, Nanyang Technological University, Singapore
Copyright © 2021 Rossbach, Anton and Duarte. This is an open-access article distributed under the terms of the Creative Commons Attribution License (CC BY). The use, distribution or reproduction in other forums is permitted, provided the original author(s) and the copyright owner(s) are credited and that the original publication in this journal is cited, in accordance with accepted academic practice. No use, distribution or reproduction is permitted which does not comply with these terms.
*Correspondence: Susann Rossbach, c3VzYW5ucm9zc2JhY2hAaG90bWFpbC5jb20=