- 1Laboratorio de Mamíferos Marinos, Centro para el Estudio de los Sistemas Marinos (CESIMAR) CCT CONICET-CENPAT, Puerto Madryn, Argentina
- 2Instituto de Investigaciones Marinas y Costeras, Universidad Nacional de Mar del Plata (IIMyC-CONICET-UNMdP) – CONICET, Buenos Aires, Argentina
- 3Instituto Patagónico de Ciencias Sociales y Humanas (IPCSH) CCT CONICET-CENPAT, Puerto Madryn, Argentina
In cetaceans, increased body flexibility is associated with increased maneuverability, this affects the animal’s swimming speed and foraging behavior. A more stable body form is associated with fast swimming and wide turns. One factor that affects the flexibility of a cetacean’s body is the structure and interaction of its vertebrae. Differences in vertebral morphology confer different muscular insertion sites and affect mechanical properties of swimming muscles. We studied vertebral morphology in four closely related and partially sympatric dolphin species from the Southern Hemisphere: Commerson’s dolphin (Cephalorhynchus commersonii), Peale’s dolphin (Lagenorhynchus australis), the dusky dolphin (Lagenorhynchus obscurus) and the hourglass dolphin (Lagenorhynchus cruciger). The former two species are usually considered coastal, associated with complex habitats where foraging strategies require greater maneuverability; they also show plasticity in their prey preferences. The latter two species are considered fast-swimming cooperative feeders, with long distance movements reflecting prey availability in pelagic habitats. We employed three-dimensional (3D) geometric morphometric techniques and multivariate analyses to evaluate differences in vertebral morphology. Our analyses tested whether particular morphologies that limit or enhance flexibility were associated with preferred habitats and feeding strategies. We established links between morphology and behavioral patterns based on the biomechanical significance of specific vertebral morphological features. Principal component analyses (PCA) showed great differentiation between species in all the studied regions along the vertebral column. This was especially evident in the middle area, except in the case of dusky and hourglass dolphins which showed no discernible morphological difference in their mid-column vertebrae. PCA results were supported by statistically significant Mahalanobis distances (MD) between species. Species associated with complex habitats and behaviors possessed morphological features associated with greater flexibility of the column (i.e., spool-shaped vertebrae with short erect processes), whereas cooperative-feeder species possessed features associated with greater stability (i.e., disk-shaped vertebrae with long strongly bent processes). In these closely related and partially sympatric dolphins, vertebral morphology is distinctive and varies with the differential foraging strategies and habitat of each species. These findings reveal morphological plasticity among these dolphin species, highlighting the importance of behavioral complexity and of habitat use in the evolutionary development of morphological adaptations.
Introduction
Feeding strategies in cetaceans are associated with particular foraging habitats and the movement and locomotive abilities that they require. Cetacean morphologies that have evolved in different habitats may therefore reflect the particular selective pressures of those habitats (Ballance, 2018) and, specifically, the rigidity and flexibility of a cetacean’s body might reflect the locomotive demands of its habitat. Considering that cetacean bodies are not rigid and show variable flexibility (Pabst, 1993, 2000; Long et al., 1997), greater flexibility has been associated with greater maneuverability (Fish and Rohr, 1999; Fish, 2002). Conversely, a stable morphology would minimize energy costs and increase efficiency during prolonged swimming (Fish and Rohr, 1999). The more stable the body the more favored these animals are to inhabit pelagic habitats (Fish, 2002). Body stabilization systems can be active or passive: active systems involve neurological activation of musculoskeletal components at expense of energy, whereas passive systems include morphological traits, such as vertebral morphology, and physical tissue properties that do not incur energetic costs (Fish et al., 2003).
The vertebral column of a dolphin distributes forces that contribute to the movement of its body and controls its bodily deformation pattern (Gal, 1992, 1993a; Long, 1992, 1995; Pabst, 1993). The flexibility of a dolphin’s vertebral column is determined by many traits including development and composition of muscles and ligaments, the composition and size of intervertebral disks, and the structure and interactions of the vertebrae (Gal, 1993b; Long et al., 1997; Koob and Long, 2000). Vertebral morphology and flexural mechanisms vary regionally along the vertebral column and might help determine the pattern of force transference and deformation along the body axis (Long et al., 1997).
Variations in vertebral structure can reinforce or limit movements between adjacent vertebrae. These variations occur mainly in the shape of the centrum, its spacing, the structure and orientation of the vertebral processes, the number of vertebrae, and the development of accessory structures (Buchholtz and Schur, 2004; Marchesi et al., 2017). The vertebral centrum is the primary mechanical support of the column and its shape affects the angle of rotation and displacement of one vertebra relative to its adjacent vertebrae (Slijper, 1936, 1946; Crovetto, 1991; Long et al., 1997; Buchholtz, 2001; Buchholtz and Schur, 2004; Rommel and Reynolds, 2018). Both neural and transverse processes are sites of origin and insertion for muscle fibers and series of long tendons (Pabst, 1990). The development of these processes affects the insertion area, and their length and orientation determine the distance between insertion sites along the longitudinal axis of the body (Slijper, 1936, 1961; Pabst, 2000). The larger the distance, the greater lever arm and mechanical advantage of the muscle. The main accessory structures are the zygapophyses (articulation facets among vertebrae). In the cervical region, these are included in the neural arches; in the anterior half of the thoracic region, pre- and post-zygapophyses are distinguishable. They are located on the transverse processes and their horizontal orientation indicates a potential for axial rotation and lateral movements (Rommel and Reynolds, 2018). From the mid-thorax to the peduncle, the zygapophyses are located on the anterior edge of the neural spine and have a vertical orientation allowing dorso-ventral movements (Rommel and Reynolds, 2018). These types of zygapophyses are known as metapophyses (Buchholtz and Schur, 2004). They provide insertion sites for the deep tendon; a tendinous system for the m. multifidus and m. longissimus, the main effectors of column extension (Pabst, 2000). Variations in the height of the metapophyses affect the mechanics of their associated muscles systems (Buchholtz and Schur, 2004; Marchesi et al., 2017). In certain areas along the column, the metapophyses may overlap with the neural process of the vertebra cranially adjacent to them and restrict rotation movements between these vertebrae (Buchholtz and Schur, 2004).
Flexible and stable areas of the column show particular combinations of these anatomical features (Table 1; Buchholtz and Schur, 2004; Woodward, 2006). Vertebrae from flexible areas are characterized by a long centrum, small centrum faces with high curvature, short but strongly inclined neural processes, metapophyses that do not overlap with adjacent vertebrae, and short transverse processes. Complementarily, vertebrae from stable regions have short centra with large flat or concave faces, tall neural processes that are perpendicular to the body’s longitudinal axis, long transverse processes, and high metapophyses that overlap with the neural processes of adjacent vertebrae (Table 1). Woodward (2006) suggests that slow swimming species have features characteristic of a more flexible vertebral column whereas pelagic, fast-swimming species have osteological features characteristic of a more stable column. This pattern has been documented for two dolphin species (Marchesi et al., 2017). Moreover, certain features such as low vertebral count and long centra have been associated with species that occupy coastal habits; predicting that a high vertebral count and disk-shaped centra would be associated with species that occupy pelagic habits (Buchholtz and Schur, 2004).
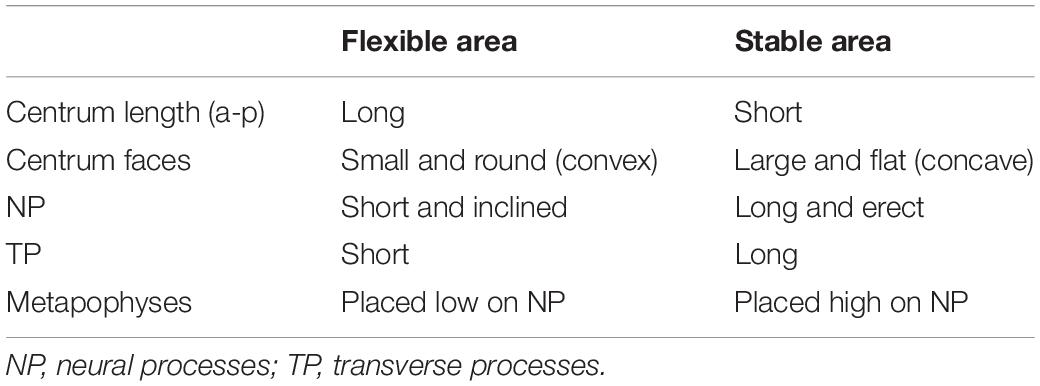
Table 1. Combination of vertebral features in flexible and stable areas of the vertebral column for cetaceans based on Buchholtz and Schur (2004) and Woodward (2006).
It is expected that sympatric species should show behavioral and physiological strategies and accompanying morphological adaptations that minimize competition and maximize the possibility of occupying different niches (see Gross et al., 2009). Commerson’s dolphin (Cephalorhynchus commersonii), Peale’s dolphin (Lagenorhynchus australis), the dusky dolphin (Lagenorhynchus obscurus) and the hourglass dolphin (Lagenorhynchus cruciger) are closely related species belonging to the subfamily Lissodelphininae (Figure 1; see Vollmer et al., 2019; Committee on Taxonomy, 2020; McGowen et al., 2020). They inhabit the Southern Hemisphere with partially sympatric distributions overlapping in different areas of their geographic ranges but differing in their habitat preferences. The former two species are considered coastal associated with complex habitats where foraging strategies require greater maneuverability; and accordingly, they show plasticity in their prey preference (see Goodall, 1994; Goodall et al., 1997b, c; Coscarella et al., 2010; Riccialdelli et al., 2010; Garraffo et al., 2011; Dellabianca et al., 2016; Franchini et al., 2020). The latter two species are considered fast-swimming cooperative feeders that forage on pelagic prey exhibiting long-distance displacements that reflect prey availability in pelagic habitats (see Würsig and Würsig, 1980; Goodall et al., 1997a; Schiavini et al., 1999; Riccialdelli et al., 2010; Degrati et al., 2012).
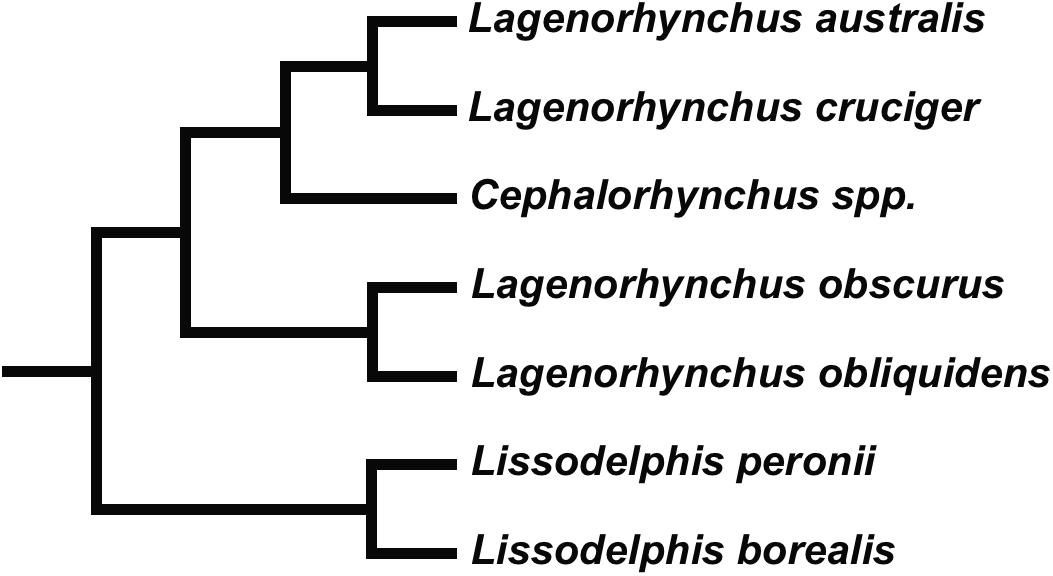
Figure 1. Relationships within Lissodelphininae (see Vollmer et al., 2019; McGowen et al., 2020).
In this study we compare the vertebral morphology of these four closely related Southern Hemisphere dolphin species with different feeding strategies and prey preferences. We showed how differences in vertebral morphology suggest particular selective pressures imposed by the biomechanical demands of particular habitats and associated foraging strategies.
Materials and Methods
Specimens
We studied a total of 100 specimens including 37 Commerson’s dolphins, 24 Peale’s dolphins, 29 dusky dolphins, and 10 hourglass dolphins. All the specimens are housed in mammalogy collections and listed in Supplementary Material 1. Due to a low number of adult specimens, we included both sub-adult and adults in the analyses. Specimens were classified into ontogenetic classes based on the degree of fusion of the vertebral epiphyses, according to the criterion proposed by Perrin (1975) and modified by Goodall et al. (1988) and Lockyer et al. (1988).
Functional Subdivision of the Vertebral Column and Vertebrae Selection
Regional morphological differences along the vertebral columns of different dolphins may indicate how swimming style may varies between species (Buchholtz, 2001; Buchholtz and Schur, 2004; Marchesi et al., 2018). Buchholtz and Schur (2004) suggested an approach to vertebral column functional analyses, specific to dolphins, that redefines regions of the column into functional subdivisions. This approach yields a detailed analysis of the morphological variation within each region, focusing on traits with functional implications that could be masked under the traditional regional criterion (e.g., Buchholtz and Schur, 2004; Marchesi et al., 2020b). Based on this functional approach, traditional lumbar and caudal regions are divided into three functional regions: torso, tailstock, and fluke (Figure 1). The torso includes all vertebrae between the thoracic region (last rib bearing vertebra) and the first vertebra that is taller than wide, which is thereby the anterior boundary of the tailstock (Figure 2; see also Marchesi et al., 2018). The torso is further divided into three sub-regions: anterior, mid-, and posterior torso. Vertebrae on which the neural spine inverts its inclination define the anterior and posterior boundaries of the mid-torso (see Buchholtz and Schur, 2004; Marchesi et al., 2017, 2018). Tailstock vertebrae are those that are taller than wide, and fluke vertebrae are much wider than tall, and have rectangular cross sections. For our four studied species, boundaries for the functional regions were determined following Marchesi et al. (2017, 2018).
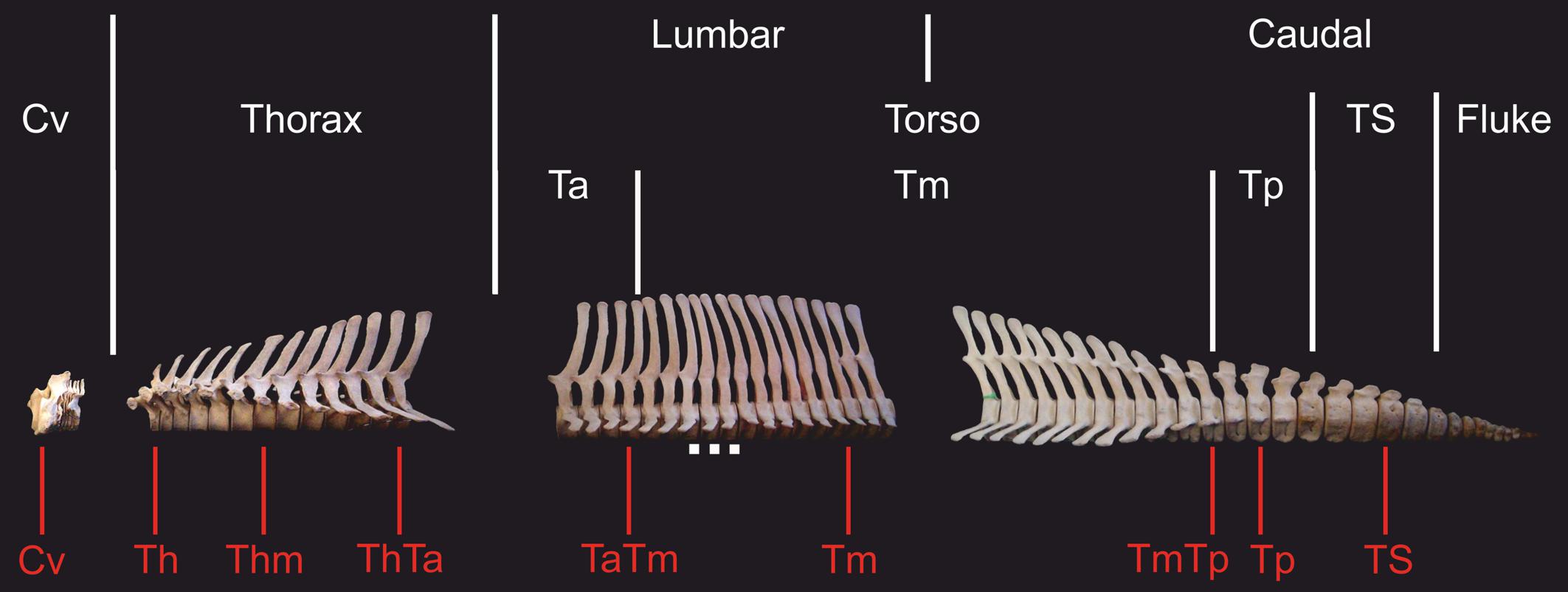
Figure 2. Skeleton of the hourglass dolphin (Lagenorhynchus cruciger) showing traditional and functional subdivision of the vertebral column in dolphins. In red are shown the vertebrae selected from each vertebral column. Cv, cervical; Th, anterior thorax; Thm, mid-thorax; ThTa, thorax and anterior torso boundary; TaTm, anterior and mid-torso boundary; Tm, mid-torso; TmTp, mid-posterior torso boundary, Tp: posterior torso; TS, tailstock; Scale, 5 cm.
We selected a maximum of nine vertebrae from each specimen, representing those functional regions and their boundaries, yielding a total of 595 vertebrae (Table 2). Some specimens were incomplete (lacking caudal vertebrae) or damaged (lacking epiphyses). For this reason, the number of vertebrae included for each region does not correspond with the number of specimens studied. As the number of vertebrae varied both within regions (especially the torso) and between species, we chose the vertebrae based on their position with respect to the functional regions described previously (Table 3 and Figure 2). The atlas-axis complex was chosen to represent the cervical region (Cv). The first thoracic vertebra was selected to represent the anterior thorax (Th), the sixth thoracic vertebra represented the mid-thorax (Thm), the last thoracic vertebra represented the thorax-anterior torso boundary (ThTa), and the first vertebra with a neural spine perpendicular to the longitudinal body axis signaled the anterior and mid-torso boundary (TaTm). We chose the central vertebra in the mid-torso (Tm) to represent that region, and the second vertebra with a neural spine perpendicular to the body axis (synclinal point sensu Slijper, 1946) to represent the mid- and posterior torso boundary (TmTp). We chose the central vertebra in the posterior torso (Tp) to represent that region and, finally the central vertebra of the tailstock (TS) was chosen (Figure 2). If a region contained an even number of vertebrae and a central vertebrae could not be chosen, we selected the vertebra immediately anterior to the center of the region; for example, in a region with four vertebrae, the second vertebra was chosen.
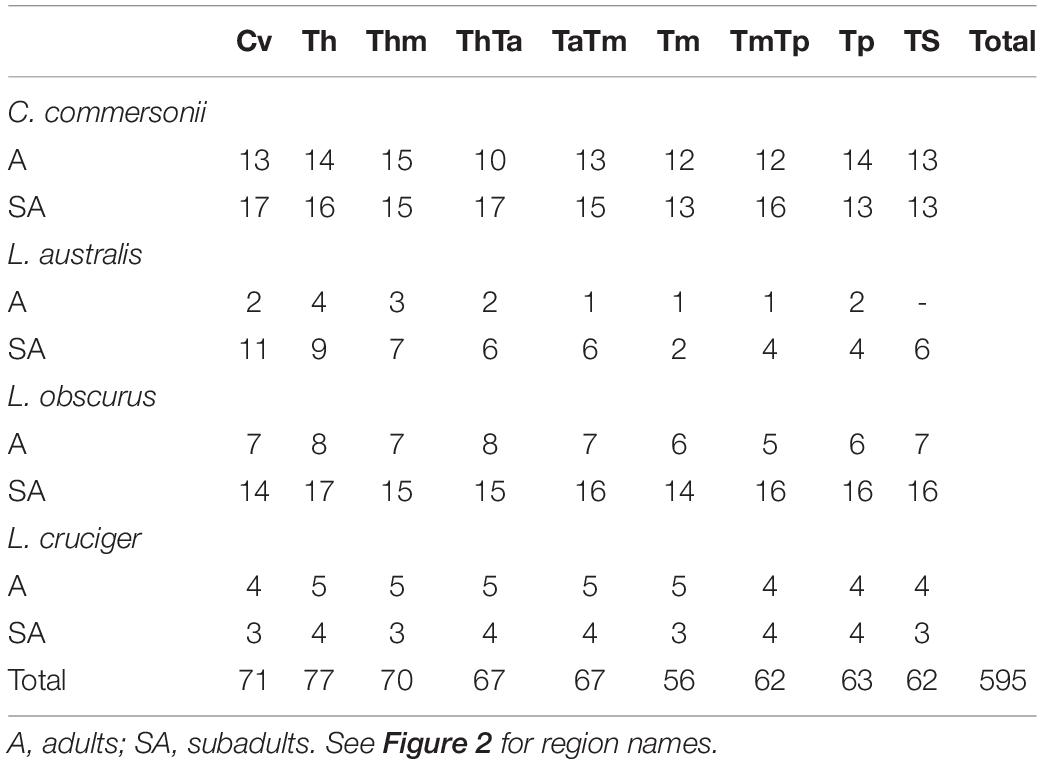
Table 2. Sample size for each region of the four studied species: Commerson’s dolphin (Cephalorhynchus commersonii), Peale’s dolphin (Lagenorhynchus australis), dusky dolphin (Lagenorhynchus obscurus), and hourglass dolphin (Lagenorhynchus cruciger).
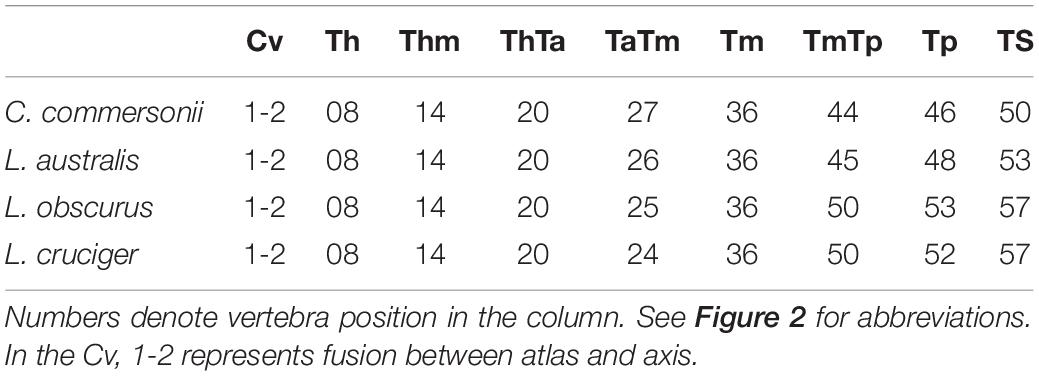
Table 3. Vertebrae employed to characterize each particular region or boundary in Commerson’s dolphin (Cephalorhynchus commersonii), Peale’s dolphin (Lagenorhynchus australis), dusky dolphin (Lagenorhynchus obscurus), and hourglass dolphin (Lagenorhynchus cruciger).
3D Geometric Morphometrics
Geometric morphometric tools allow the visualization of shapes in complex structures (Zelditch et al., 2004). These methods are based on the spatial displacement of landmarks (Bookstein, 1996a,b). Sometimes the use of landmarks may not be sufficient, either because they cannot be identified on structures, or because morphological features lack anatomically homologous points (e.g., curved surfaces, outlines; Oxnard, 1978). This is the case of vertebrae, where the primary homology of vertebral structure is based on the outline of the vertebra. When landmarks are too scarce, outlines can be digitized as series of discrete points (semi-landmarks, see Pérez et al., 2006). This outline method does not differ fundamentally from conventional landmark methods in the way it handles biological homology (MacLeod, 1999; Sheets et al., 2004). The semi-landmarks must be slid along a tangential direction so as to remove tangential variation because contours should be homologous from subject to subject, whereas their individual points need not be (Pérez et al., 2006). After Procrustes superimposition (see below), semi-landmarks can be considered homologous (see Pérez et al., 2006) and for simplicity, we refer to them as landmarks. We used a similar approach to that employed by Maddux and Franciscus (2009) and Paschetta et al. (2016). In order to maximize the information on shape and preserve the 3D nature of the vertebrae, we developed three radiated figures to be projected on the vertebrae in order to allocate landmarks (Supplementary Figure 1; see also Marchesi et al., 2020b). We projected an “asterisk” (Supplementary Figure 1A) on each face of the centrum in order to allocate landmarks. This projection was positioned so that its center would coincide with the anatomical landmark on the center of the centrum face. Accordingly, the vertical line of the “asterisk” passed through the vertical axis of the neural spine (see also Marchesi et al., 2020a). After positioning the projection, we placed one landmark in the center of the face and landmarks on the centrum outline at the intersection of the equiangular radial arrangement and the centrum face outline. This was done first as we digitized the anterior face, then we rotated the vertebra 180 and digitized the posterior face. For the neural process, we projected a radii arrangement (Supplementary Figure 1B) laterally from the left in such a way that the most external radii passed through the junction between the neural arch and the neural spine. We placed four landmarks (two anterior and two posterior) at the intersection of the inner radii and the process outline. In a similar manner, we projected a radii arrangement dorsally (Supplementary Figure 1C) in such a way that the most external radii passed through the tip of the transverse processes and the middle radius passed through the neural process. Finally, we placed four landmarks (two anterior and two posterior) on each process.
By using the methods described above, we digitized five original 3D landmark configurations, containing 28–41 landmarks depending on the region (Supplementary Figure 2 and Supplementary Table 1) using a Microscribe G2X digitizer. These landmark configurations were superimposed by generalized procrustes analysis (GPA; Rohlf and Slice, 1990; Goodall, 1991) using the routine implemented in MorphoJ 1.06d (Klingenberg, 2011). After GPA, the size variable is the centroid size (CS), which is the square root of the summed squared distances of each landmark from the centroid of the landmark configuration (Klingenberg, 2011). Shape variables are the new coordinates that describe the location of each specimen in a curved space related to Kendall’s shape space and represent the difference between the consensus (mean shape) and each sample (Slice, 2001). In all cases, we employed the symmetric component of shape (Klingenberg, 2011).
Our sample included adult and sub-adult specimens, so in the multivariate analysis, we removed the effect of size-dependent shape variation due to ontogenetic size scaling by performing a multivariate linear regression of shape on the logarithm of centroid size pooled by species. The shape variation not affected by allometric scaling remains preserved in the residuals of such regression, these residuals are used as allometry-free shape variables in further analyses (Klingenberg, 2016). Regressions were performed in MorphoJ 1.06d (Klingenberg, 2011). For each region, we assessed qualitatively the major components of variation among species by using the principal components analysis (PCA) function included in the MorphoJ software. Landmarks were not congruent between regions, so we analyzed the PCA of each region separately. We determined the statistical significance of differences between species by computing Mahalanobis distances (MD) between groups, and we calculated their associated p-values through permutation tests using the free software R v3.3.3 (R Core Team, 2017) and the package “Morpho” v2.6 (Schlager, 2017). For this, we employed principal components (PCs) selected using the Kaiser-Guttman criterion (Guttman, 1954; Cliff, 1988; Jackson, 1993). The number of PCs selected varied depending on the region: there were 11 PC for the Cv, nine for the Th, eight for the Thm, seven for the ThTa, TmTp, and TS, five both for the TaTm and Tm, and ten for the Tp.
Results
Vertebral regions differed from one another with respect to the percentage of variance that the first two principal components explained (Figure 3). This percentage of explained variance was smaller for regions at the extremes of the vertebral column than in the mid-area, with the first two principal components explaining most variance for the Tm (Figure 3).
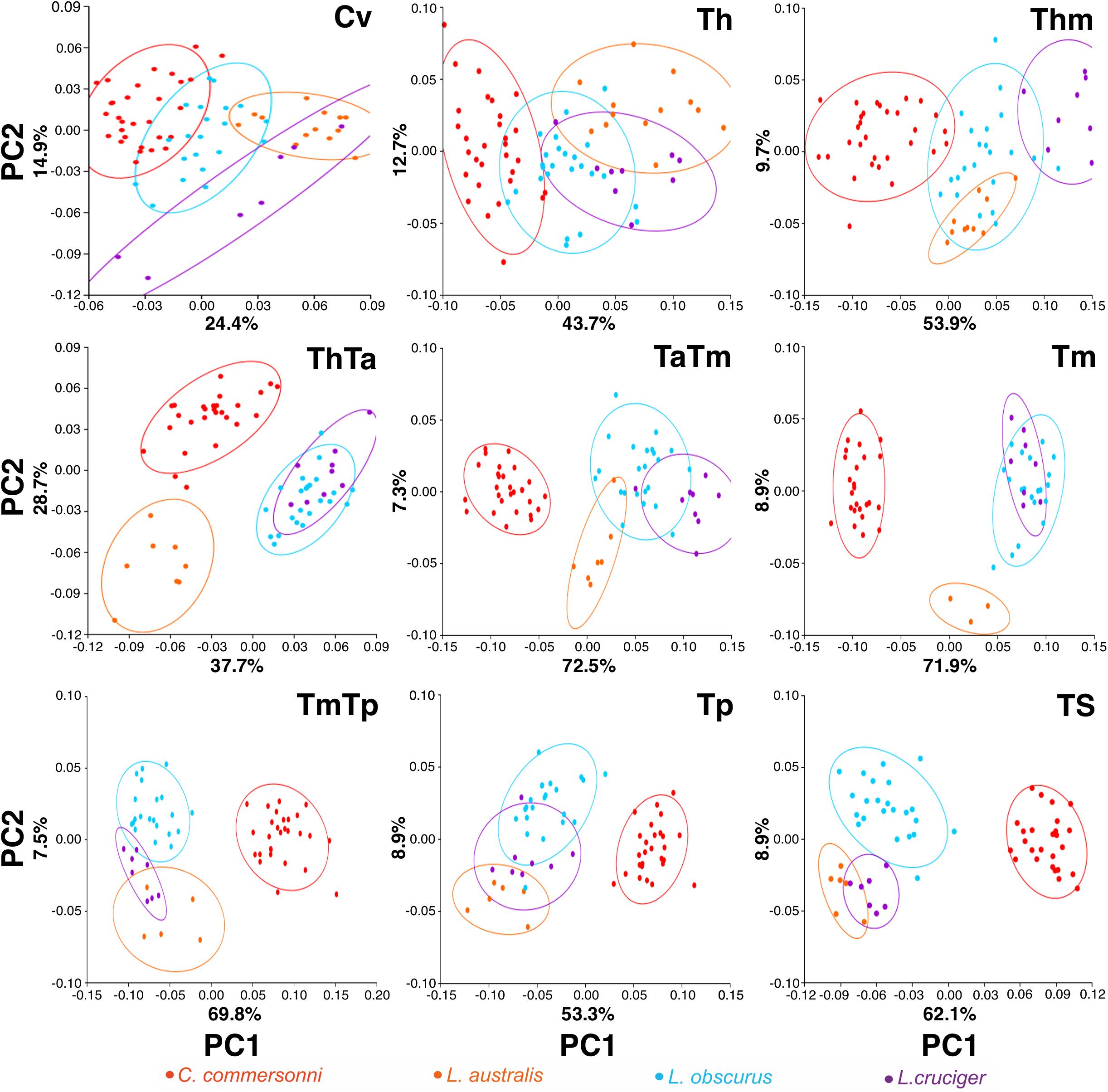
Figure 3. Plot of the first two principal components (PC1 and PC2) scores for the four species: Commerson’s dolphin (Cephalorhynchus commersonii; red), Peale’s dolphin (Lagenorhynchus australis; orange), dusky dolphin (Lagenorhynchus obscurus; light blue), and hourglass dolphin (Lagenorhynchus cruciger; purple) for each studied region (see Figure 2 for region names). Equal frequency ellipses are shown for each species. Percentage of explained variance is depicted for each PC and region.
Detailed analysis of shape changes and anatomical interpretation of different morphologies are described in Supplementary Material 2. Shape changes associated with minimum and maximum values of the first two components are described in Supplementary Table 2, and shown in Supplementary Figure 3. Accordingly, shape changes for each species have been compiled in Table 4. This table contains the descriptions of particular morphologies of vertebral structures. Within each region species are organized in such a way that they show an increase in flexibility (Table 4 and Figure 4). Mean morphology of each region for the four species is depicted in Figures 5A–I.
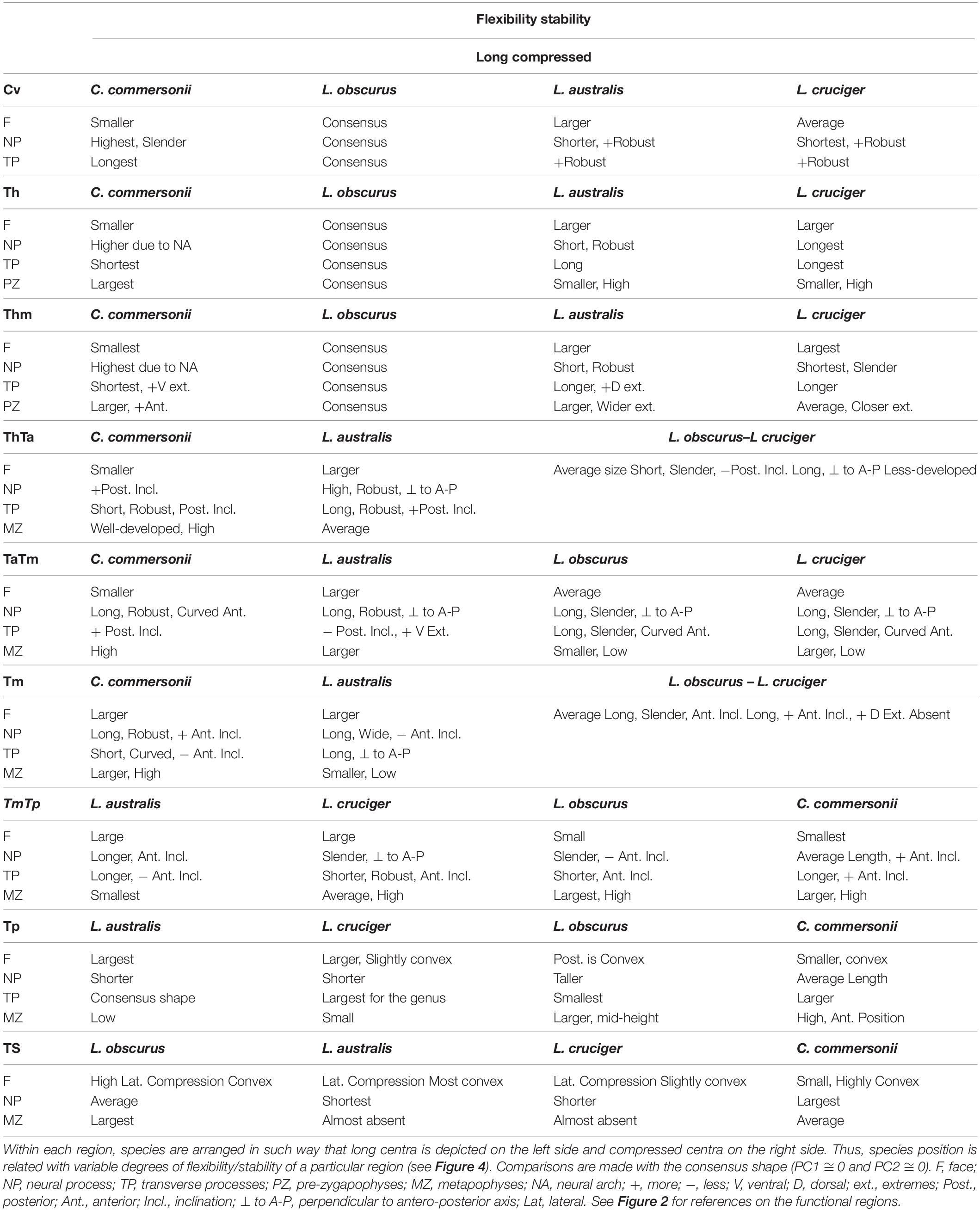
Table 4. Shape changes for each region of the four species: Commerson’s dolphin (Cephalorhynchus commersonii), Peale’s dolphin (Lagenorhynchus australis), dusky dolphin (Lagenorhynchus obscurus), and hourglass dolphin (Lagenorhynchus cruciger).
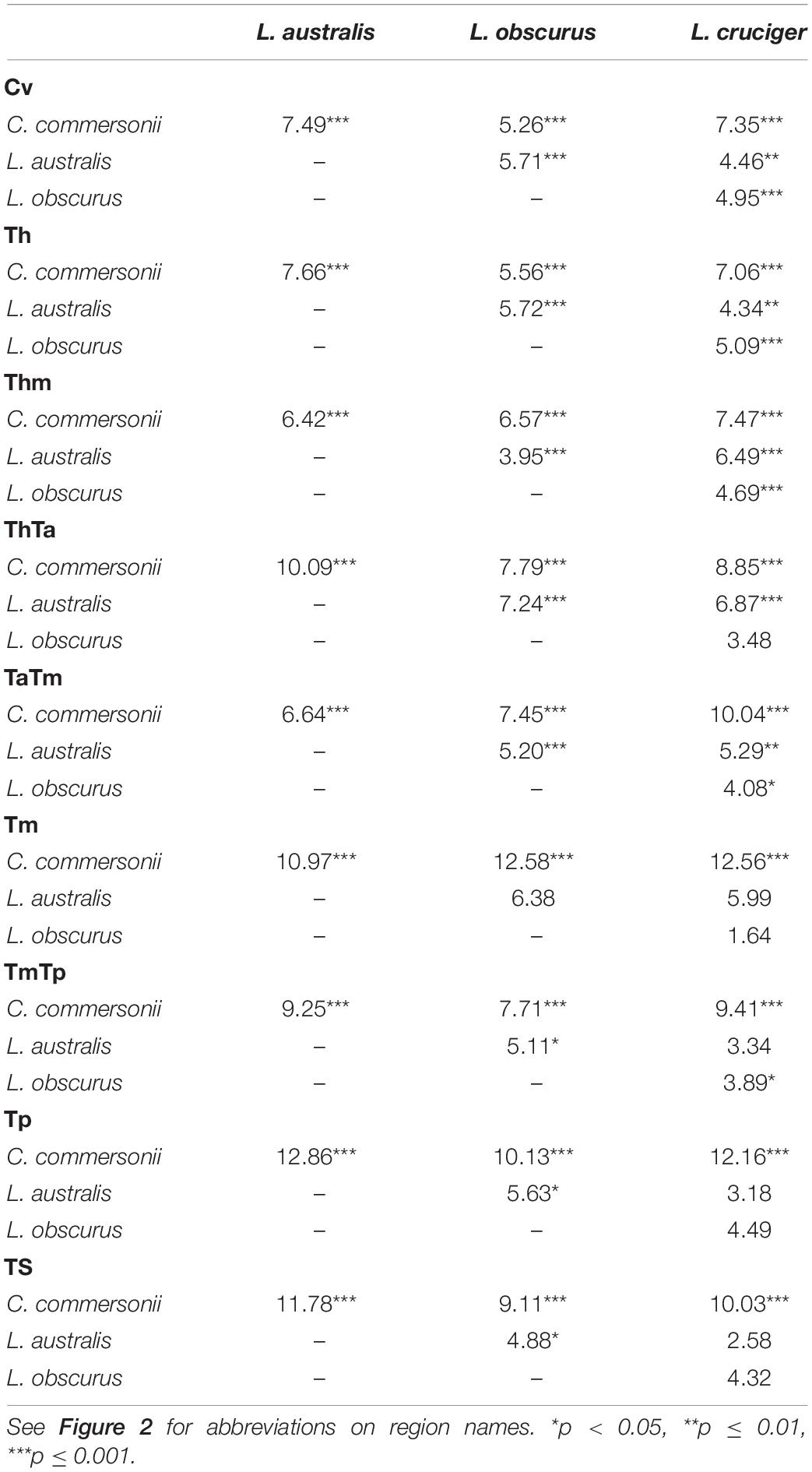
Table 5. Mahalanobis distances among Commerson’s dolphin (Cephalorhynchus commersonii), Peale’s dolphin (Lagenorhynchus australis), dusky dolphin (Lagenorhynchus obscurus), and hourglass dolphin (Lagenorhynchus cruciger), for each functional region and their statistical significance.
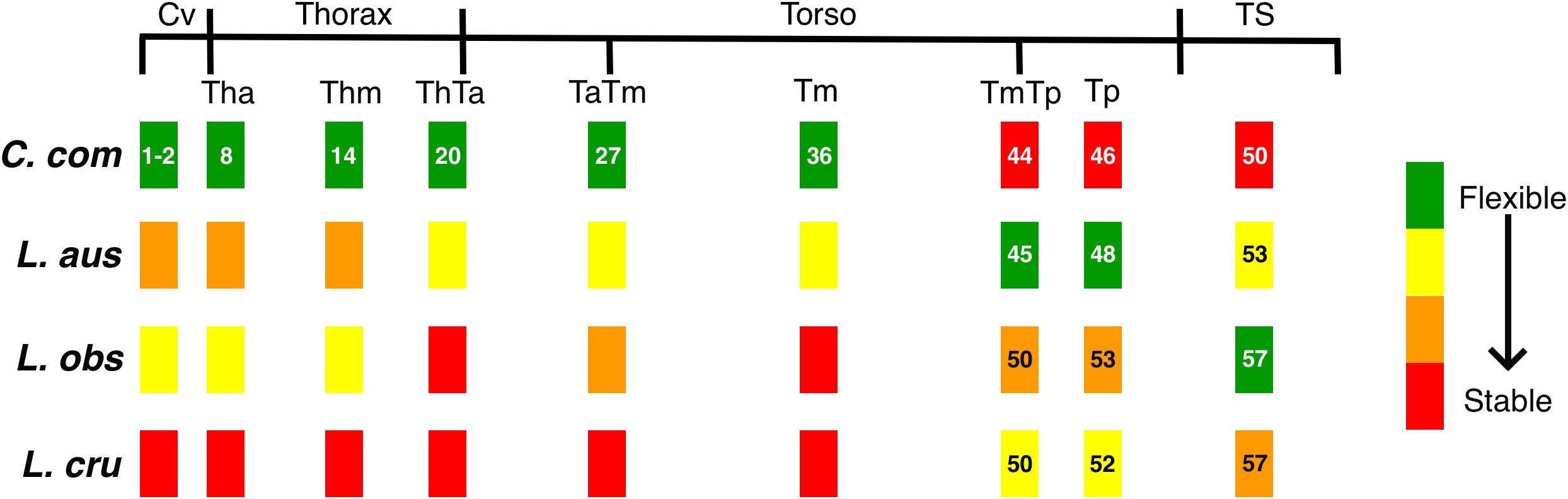
Figure 4. Schematics representing the variable degrees of flexibility/stability along the vertebral column of the four species studied: Commerson’s dolphin (Cephalorhynchus commersonii), Peale’s dolphin (Lagenorhynchus australis), dusky dolphin (Lagenorhynchus obscurus), and hourglass dolphin (Lagenorhynchus cruciger; see Table 4). Black lines depict functional regions. Colored rectangles correspond to position of the studied vertebra with respect to functional regions. Numbers indicate the position of the studied vertebra for each species (see Table 3). Absence of numbers indicate lack with difference with Commerson’s dolphin. See Figure 2 for references on functional regions.
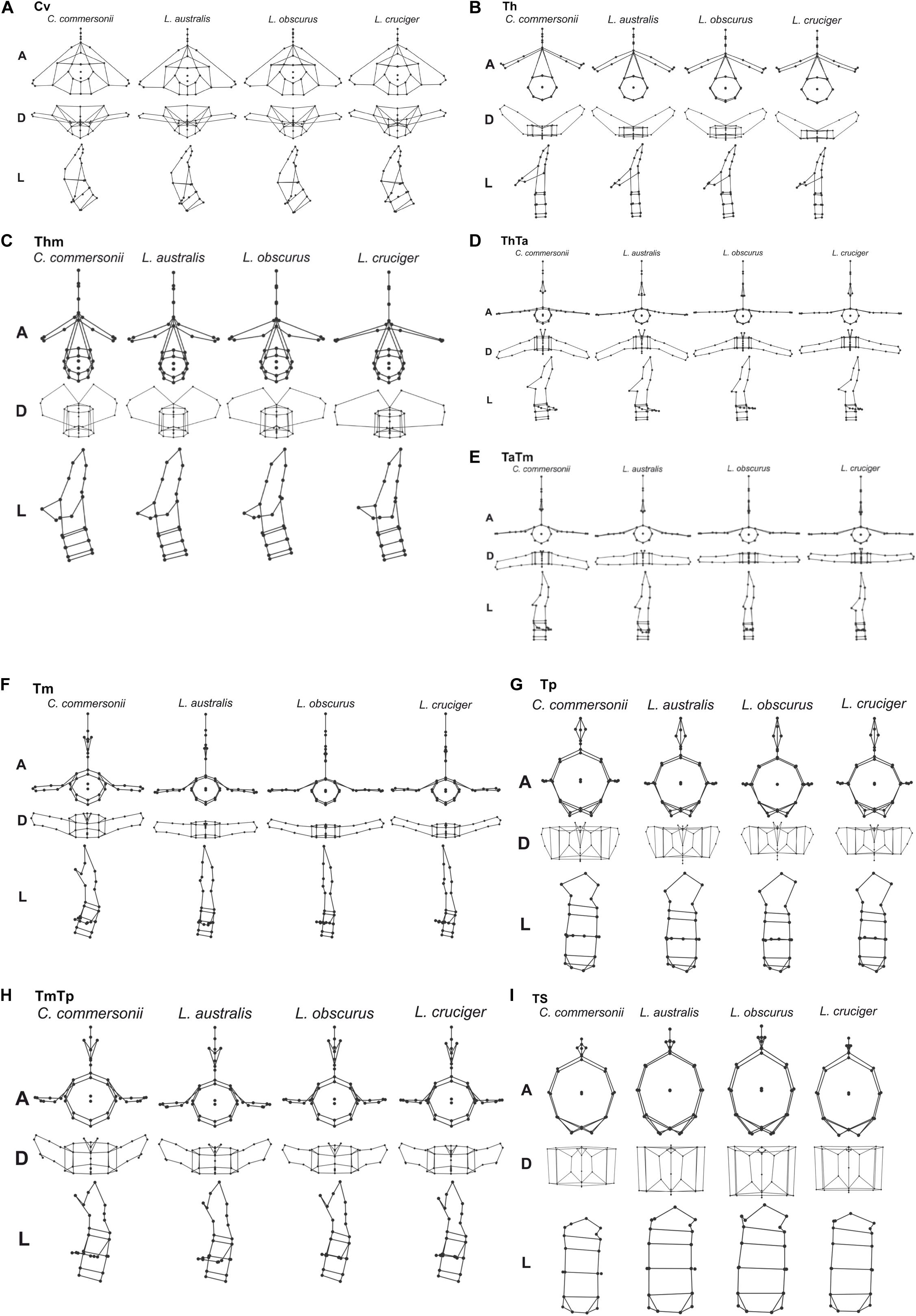
Figure 5. Mean vertebral morphology of the four species: Commerson’s dolphin (Cephalorhynchus commersonii), Peale’s dolphin (Lagenorhynchus australis), dusky dolphin (Lagenorhynchus obscurus), and hourglass dolphin (Lagenorhynchus cruciger) for each studied region (A–I) in anterior (A), dorsal (D), and left lateral (L) view.
In this study, the cervical region corresponded to the atlas-axis complex, thus inferences did not include the remaining five cervical vertebrae. In all these species, Cv3 through Cv7 are highly compressed and in contact with each other (MCM, personal observation). Slight morphological differences were evident between species in our analyses of the cervical region. These interspecific differences were mostly distributed along PC1 and interspecific differences were less distinct along PC2. The dusky dolphin specimens had PC values closest to the consensus shape (PC1 ≅ 0, PC2 ≅ 0). Vertebral morphology of the hourglass dolphin was highly variable. Commerson’s dolphin showed the greatest potential flexibility and the hourglass dolphin showed the greatest potential stability; the dusky dolphin was less flexible than Commerson’s dolphin and more flexible than Peale’s dolphin (Table 4 and Figure 4). This pattern in flexibility degrees was also observed for the two following regions: the thorax and the mid-thorax.
At the beginning of the thorax (Th), two groups were differentiated along PC1: one group consisting of only Commerson’s dolphin (PC1 < 0, PC2 ≅ 0), and the other group consisting of Peale’s and the hourglass dolphin; the dusky dolphin’s equal frequency ellipse (PC1 ≅ 0, PC2 ≅ 0) partially overlapped both groups (Figure 3). PC2 allowed further separation between Peale’s dolphin (PC1 > 0, PC2 > 0) and the hourglass dolphin (PC1 > 0, PC2 ≤ 0).
In the mid-thorax the analyses showed three groups along PC1. One group consisted of Commerson’s dolphin (PC1 > 0, PC2 ≅ 0), the second group was formed by Peale’s dolphin and the dusky dolphin (PC1 ≅ 0), and the third group included only the hourglass dolphin (PC1 > 0, PC2 > 0; Figure 3). PC2 allowed further separation between Peale’s dolphin and the dusky dolphin (PC2 < 0 and PC2 ≅ 0, respectively). The dusky dolphin showed morphologies associated with the consensus shape for the Tm region.
At the thorax-torso boundary (ThTa) three groups were observed. Commerson’s dolphin and Peale’s dolphin, had negative PC1 values (PC1 < 0) but opposite values in PC2 (PC2 > 0 and PC2 < 0, respectively). The third group was formed by dusky and hourglass dolphins (PC1 > 0, PC2 ≅ 0; Figure 3). Species showed different degrees of stability: Commerson’s dolphin was the most flexible, and the group formed by the dusky dolphin and hourglass dolphin the most stable. Peale’s dolphin had features indicating intermediate flexibility between these two groups (Table 4 and Figure 4).
In the anterior-mid-torso boundary (TaTm) we identified two distinct groups along PC1. The first group consisted only of Commerson’s dolphin with negative PC1 values (PC1 < 0), and the second group included partially overlapping equal frequency ellipses for Peale’s dolphin, the dusky dolphin, and the hourglass dolphin (Figure 3). There were slight differences in PC1 values among these three species, increasing from Peale’s dolphin to dusky, and to hourglass dolphin. Peale’s dolphin also differed in its PC2 values (PC2 < 0). The pattern of flexibility observed in this vertebral region was similar to that observed in anterior regions of the column (Cv, Th, and Thm): Commerson’s dolphin showed morphological features associated with the greatest flexibility and the hourglass dolphin exhibited features associated with greater stability in the vertebral column (Table 4 and Figure 4).
Principal component analysis of the mid-torso showed three distinct groups along the first component. One group contained Commerson’s dolphin (PC1 < 0, PC2 ≅ 0), the second group consisted of Peale’s dolphin (PC1 ≅ 0, PC2 < 0), and the third group was formed by the dusky dolphin and the hourglass dolphin (PC1 > 0, PC2 ≅ 0; Figure 3). Once again, Commerson’s dolphin showed features related with greater flexibility whereas the dusky dolphin and the hourglass dolphin showed features associated with greater stability (Table 4 and Figure 4).
For the two studied regions involving the posterior torso (TmTp and Tp) two groups were distinguishable along PC1: one group comprising Peale’s dolphin, the dusky dolphin and the hourglass dolphin (PC1 < 0), and the other group consisting of Commerson’s dolphin (PC1 > 0; Figure 3). Species within the first group could be further separated according to PC2 values: the ellipse for the hourglass dolphin (PC2 ≅ 0) only partially overlapped the ellipses of Peale’s (PC2 < 0) and the dusky dolphin (PC2 > 0). In both these regions, Peale’s dolphin showed the most potentially flexible area whilst Commerson’s dolphin was the most stable (Table 4 and Figure 4).
Principal components analysis analyses for the most caudal area that we analyzed, the tailstock (TS), showed a similar pattern of interspecific differences as was seen in the two previous regions (TmTp and Tp). There were two distinguishable groups along PC1, and PC2 values allowed separation between the dusky dolphin and Peale’s dolphin and hourglass dolphin (Figure 3). The dusky dolphin showed the greatest degree of flexibility and Commerson’s dolphin had the most stable tailstock.
Mahalanobis distances indicated statistical differences among all species for the first three analyzed regions (Cv, Th, and Thm). Vertebrae at the beginning of the thorax (ThTa) did not show significant morphological differences between the dusky dolphin and the hourglass dolphins. In the mid-torso (Tm) vertebral morphology was significantly different between Commerson’s dolphin and the Lagenorhynchus species, but not between the three Lagenorhynchus species. At the mid-posterior torso boundary (TmTp) vertebral morphology was not significantly different between Peale’s dolphin and the hourglass dolphin. In the final two regions analyzed, the posterior torso (Tp) and the tailstock (TS), vertebrae showed no significant morphological differences between Peale’s and hourglass dolphins and also between the dusky and hourglass dolphins but did show significant morphological differences between Peale’s and dusky dolphins.
Discussion
Morphological Differences and Factors Affecting Swimming
A comprehensive morphological characterization of the vertebral column contributes greatly to an understanding of the locomotor performance of different cetacean species that inhabit different habitats and exhibit different foraging strategies. Factors that affect locomotion involve a complex interaction between the axial skeleton and its associated muscles, tendons, ligaments, and the subdermal connective tissue sheath (SDS; see Pabst, 1990). Here, we performed a complete characterization of the major functional regions (Figure 2) of the vertebral columns of four closely related and partially sympatric dolphin species, with an emphasis on biomechanically important morphological differences.
For delphinids, high stability in the anterior area of the body reduces pitching movements that are produced in response to forces generated at the caudal end of the body (Fish et al., 2003), ultimately reducing the energetic requirements of swimming (Long et al., 1997; Fish et al., 2003). This stable region determines the degree to which the head can move, based on the shape of its articular faces. Vertebral processes of the studied vertebrae (fused first and second cervical vertebra) act as attachment sites for muscles associated with the head but also for the most anterior region of epaxial swimming muscles (Pabst, 1993, 2000; Cozzi et al., 2017). Thus, morphology of this stable region affects the most anterior sites of the muscles affecting the extension of the column. In Commerson’s dolphin, a species usually considered to be coastal, the cervical region showed a higher degree of flexibility and development potential for muscles involved in lateral movements of the head. This region in Peale’s dolphin showed morphology that indicated less flexibility (Figures 4, 5A).
One of the main functions of the thorax is to assist with breathing during locomotion (Cotten et al., 2008). Piscitelli et al. (2010) did not find differences in thorax flexibility between the shallow-diving coastal bottlenose dolphins (Tursiops truncatus) and deep-diving pelagic pygmy and dwarf sperm whales (Kogia breviceps and Kogia. sima). In contrast, Marchesi et al. (2020a) found morphological differences related to potential differences in thorax flexibility in the four species studied here. This finding was also supported here, as the thoracic region showed heterogeneity in vertebral morphology, allowing to identify two potentially stable areas (Th and ThTa). Mechanical analyses in common dolphins (Delphinus delphis) showed that intervertebral joints from the thoracic and the caudal region have similar rigidity, less than that observed at an intervertebral joint from the lumbar region. In contrast, Pabst (2000) states that the thoracic region of the bottlenose dolphin does not show quantifiable bending during steady swimming, whereas the tailstock does. The typical rigidity of the thoracic region is caused mainly by development and morphology of the processes and zygapophyses of thoracic vertebrae. The ribs, especially those with sternal connections, stabilize the thorax and limit rotation among vertebrae (Filler, 1986); further restricting bending in the anterior region of cetaceans (Fish et al., 2003). Despite this region being highly conserved among cetacean species, our analysis established varying degrees of flexibility among our study species, from the potentially highly flexible thorax of Commerson’s dolphins to a potentially highly stable thorax in the hourglass dolphins (Figures 4, 5B,C). The morphology of the vertebral processes in the hourglass dolphin could be related to a greater development of the m. longissimus; which could allow more potential movement of the anterior region with respect to the middle region of the body.
In delphinids, the torso includes numerous vertebrae with discoidal centra (Buchholtz and Schur, 2004). This stable area provides skeletal support (e.g., long neural processes) for muscles involved in the production of forces acting on the tailstock, the m. longissimus and its caudal extension, the extensor caudae lateralis (Pabst, 1990). The more stable this area is, the greater the force produced by the tailstock. Particularly, the anterior torso (anterior lumbar area) is where the m. longissimus develops most of the forces that are transmitted to the posterior region of the column (Pabst, 1993). Our results showed that, starting at the beginning of the torso (ThTa), both the anterior and mid-torso vertebral morphology resulted in more stable regions for the pelagic species, the dusky dolphin and the hourglass dolphin, the latter of which had the highest stability (Figures 5D–F). Less potential stability was observed in the species considered to be coastal, with Commerson’s dolphin being the most flexible (Figure 4). Peale’s dolphin processes inclination could indicate an area with a relatively higher potential for rotation than in the other species. In our study, patterns of flexibility/stability are inversely expressed along the different regions of the torso of the different species (Figure 4). The anterior half of the torso (ThTa, TaTm, and Tm) is notably more stable in the cooperative feeders, the dusky and hourglass dolphins. The caudal half of the torso (TmTp and Tp) is more stable for Commerson’s dolphin and more flexible for Peale’s dolphin and the hourglass dolphin (see Table 4 and Figures 4, 5G–H). Posterior to the TmTp boundary flexibility increases as processes shorten. The posterior torso (Tp) therefore represents the transition between the stable mid-torso and the flexible tailstock, representing the area where muscle forces are produced needed to change the fluke’s angle of attack (Pabst, 1990; Marchesi et al., 2017). In the caudal torso regions (TmTp, and Tp), Peale’s dolphin and the hourglass dolphin were the species with the greatest potential flexibility, followed by the dusky dolphin, and finally Commerson’s dolphin whose posterior torso (TmTp and Tp) showed morphologies that suggest high stability. For Commerson’s dolphin, the great development of processes, and the position and orientation of metapophyses indicates a probable mechanical advantage in the lateral movement of the fluke. Overall, morphological features are observed in different areas of the posterior torso for each species suggesting mechanical advantage for muscles that rotate and flex (see Table 4 and Figures 4, 5G–H).
The tailstock has a high degree of flexibility and rotation potential in comparison with other regions of the vertebral column (Buchholtz and Schur, 2004; Marchesi et al., 2017). In this region vertebrae are long and taller than wide, with markedly convex faces; interaction between vertebrae is reduced by the absence or reduction of processes (Buchholtz and Schur, 2004; Marchesi et al., 2017). The dorso-ventral elongation of the centra maximizes the potential vertical displacement of the fluke (Long et al., 1997; Buchholtz and Schur, 2004). Vertebral morphology of the tailstock showed differences between species, with Commerson’s dolphin showing features associated with less flexibility but large insertion sites; the hourglass dolphin and Peale’s dolphin had morphologies associated with high flexibility (big convex faces and short neural processes); and the vertebral morphology of the dusky dolphin suggests that this species has the greatest flexibility of the four species we compared, both in terms of rotation and in terms of vertical displacement (see Table 4 and Figures 4, 5I).
Morphological Differences in Relation to Habits and Habitat
Phylogenetic relationships and species names within the subfamily Lissodelphininae are still a matter of debate, with some genera currently recognized as being polyphyletic (Figure 5; see Vollmer et al., 2019; Committee on Taxonomy, 2020; McGowen et al., 2020). One group consisting of sister species, the Pacific white-side dolphin (Lagenorhynchus obliquidens) and the dusky dolphin, is well distinguished from the clade formed by Cephalorhynchus spp., Peale’s dolphin, and the hourglass dolphin; these latter being sister species (Figure 1). Lissodelphininae species are thought to have undergone a rapid adaptive radiation during the Late Miocene/Pliocene (∼5–3.5 Ma) in the South Atlantic Ocean, mostly attributed to differential adaptation to local habitats and to dispersal processes (Banguera-Hinestroza et al., 2014; Galatius and Goodall, 2016; McGowen et al., 2020). In this study, we suggest that morphological differences in the vertebral column of these closely related and partially sympatric species might be accordingly related to selective pressures arising from differential foraging strategies and preferred habitats, yielding a direct impact on each species biomechanical properties and behavioral performance. As suggested by Fish (2002), features that affect flexibility and maneuverability in cetaceans might be associated with both feeding behavior and habitat characteristics. Cetacean body morphology is subject to a trade-off between drag during routine movements and the work required to maneuver (Weihs, 2002).
In their analyses on delphinid osteology, Buchholtz and Schur (2004) state that the vertebral column of derived species shows greater regionalization, with features that limit movement in the region anterior to the torso (cervical and thoracic region) and increase flexibility at the synclinal point, increasing the vertical displacement of the pre-caudal vertebrae, the tailstock. According to these authors, the species in our study could be considered highly derived with respect to vertebral count and vertebral morphology. The Pacific white-side dolphin, closely related to the dusky dolphin (Figure 1), and Commerson’s dolphin have restricted flexibility and produce faster and wider turns compared to cetaceans with high maneuverability such as the river dolphin (Inia sp.; Fish, 2002). In our study, the general morphological pattern was found to be similar among species; they each have vertebral columns with features that are considered highly derived and typical of relatively fast swimmers in comparison with other odontocetes (Buchholtz and Schur, 2004; Gillet et al., 2019). At the same time, our detailed analyses allowed us to detect considerable morphological differences between species with expected effects on the biomechanical properties of the vertebral column that can be associated with differences in foraging strategies. Among our studied species, the two species known to prefer more heterogeneous environments (Commerson’s and Peale’s dolphin) have a relatively longer thorax and anterior torso that contain more vertebrae; and they have a smaller mid-torso with fewer vertebrae when compared to species that occupy the continental shelf (the dusky dolphin) or oceanic habitats (the hourglass dolphin) which is consistent with our findings (see Marchesi et al., 2018). The biomechanical significance of a higher number of vertebrae in a region depends on the morphology of the vertebra. In regions with disk-shaped vertebrae, a high vertebral count can enhance stability and store elastic energy (see Pabst, 1996). On the contrary, in regions with spool-shaped vertebrae, a higher number of intervertebral joints can contribute more possible bending sites, thus increasing flexibility (Buchholtz and Schur, 2004). Accordingly, some species that are considered coastal (here, Commerson’s dolphin and Peale’s dolphin) have a greater number of intervertebral joints in vertebral regions such as the anterior torso (Marchesi et al., 2018), which showed morphological features associated with greater flexibility, compared to pelagic species (here, the dusky dolphin and the hourglass dolphin). Conversely, species that are considered to occupy offshore habitats have a greater number of intervertebral joints in regions that are considered highly stable, such as the mid-torso (Marchesi et al., 2018; Gillet et al., 2019) presumably contributing further to stability of this region.
Vertebral morphology of Commerson’s dolphin (C. commersonii) indicates a high degree of flexibility in the anterior regions of the body but a high degree of stability in the posterior regions of the body caudal to the mid-posterior torso boundary (Figure 4). This particular combination of features agrees with the ecological plasticity of this species. A high flexibility of the anterior body could contribute to capturing benthic prey in shallow waters, allowing a great diversity of movements and a high potential maneuverability. The highly stable posterior torso and tailstock would function in conjunction to produce forces that move the fluke. In addition, a less flexible tailstock could reduce the dissipation of energy from the mid-torso to the fluke, by restricting movement between adjacent vertebrae. This might contribute more efficiency for swimming long distances when Commerson’s dolphins feed or locomote in shelf waters. The relatively larger development of transverse processes in the posterior torso suggest a high potential for generating force to move the fluke laterally; simultaneously they suggest a high rotational potential in this region and toward the caudal end of the body; with a small portion of the vertebral column participating in rotation and orientation movements of the fluke.
In the case of Peale’s dolphin (L. australis), greater body flexibility is linked to increased maneuverability (Marchesi et al., 2017), which could be beneficial when feeding in heterogeneous environments (Woodward, 2006). In this species, flexibility is potentially high in both the anterior and posterior torso regions, and in the tailstock (Figure 4). The degree of potential flexibility noted in the anterior body fits the highly complex and heterogeneous environment that the species frequents. This flexibility would be translated into a greater “regionalization” of the vertebral column, with marked morphological differences from one region to the other, that would contribute greater movement potential. In this species, contrasting with Commerson’s dolphin, the mid-torso region shows high rotational potential, indicating that a large proportion of the vertebral column participates in movements that change the position of the fluke. Despite this characteristic, however, both Commerson’s dolphin and Peale’s dolphin have relatively high flexibility of the anterior body, and the differentiation of the torso into morphological sub-regions could signal that a relatively larger part of the vertebral column is involved in movement to a greater degree than seen in the other species we studied here. Osteological features in Peale’s dolphin suggest that it trades-off speed for maneuverability, the latter of which is a necessary trait in coastal habitats. Both the kelp beds of Macrocystis pyrifera where this species is frequently found feeding, and coastal topography in general pose numerous barriers and challenges to fast, straight line movements. Moreover, capturing benthic prey often requires tight turns in limited spaces that would almost certainly be impaired by a more stable vertebral column.
In the dusky dolphin (L. obscurus) vertebral morphology showed a trade-off between a high potential flexibility of the anterior column, which would probably be required to feed in shallow waters, and a high stability in the mid-body (torso) that likely reduces the energy required for moving and feeding in open shelf waters (see Figure 4). At the same time, the greater flexibility of the tailstock, not seen in the other three species, could partially counteract the stability of the torso when feeding in shallow waters.
The hourglass dolphin (L. cruciger) showed vertebral morphologies that indicate greater stability than in the other three species studied here. As in the case of the dusky dolphin, the hourglass dolphin also showed features that indicate high stability in the whole middle region, from the thorax-torso boundary to the end of the mid-torso (Figure 4). In both species, stability in the mid-body might help reduce recoil movements and minimize drag forces, whereas long neural processes provide a mechanical advantage for attached swimming muscles. In these species, the torso could also be acting as an oscillatory beam, storing potential energy (Pabst, 1996; Marchesi et al., 2017, 2020a). This elastic energy could complementarily replace some of the muscle work that is required to accelerate or decelerate the fluke, functioning as an elastic spring (Pabst, 1996). In these species, fluke displacement movements that are required for swimming are produced by flexion of the tailstock, which oscillates away from a highly stable region. These results are consistent with propositions made by Fish and Rohr (1999) and by Fish (2002), who suggested that a more stable body enables animals to more efficiently move and feed in pelagic habitats. A body specially adapted toward stability during swimming would minimize energy expenditure and increase efficiency in propulsion for prolonged swimming during foraging or migration (Fish and Rohr, 1999; Fish, 2002). The high stability of the hourglass dolphin’s skeleton might also reduce the effect of external forces on the animal’s swimming direction in the turbulent waters it frequents.
By employing a 3D geometric morphometric method on cetacean vertebrae, in conjunction with our functional subdivision of the vertebral column, we demonstrated that vertebral morphology is distinctive for each species studied here, with biomechanical implications that likely correspond to differences in feeding behaviors and habitat associations. In this study, both vertebral morphology and some biomechanics patterns suggest a possible evolutionary convergence between the dusky dolphin and the hourglass dolphin (Figure 1). The dusky dolphin would be retaining morphological features from an oceanic ancestor (see Banguera-Hinestroza et al., 2014; McGowen et al., 2020). The close relationship between the coastal Peale’s dolphin, with its flexible vertebral column, and the oceanic hourglass dolphin, with its stable vertebral column, suggests that the latter species has perhaps re-acquired ancestral morphological features during speciation. The species studied here are closely related (McGowen et al., 2020), and their vertebral columns reflect of plasticity in morphology, highlighting the importance of environmental complexity in the particular development of adaptations to minimize energetic costs according to foraging strategies and particular habitats requirements. Future studies targeting a larger number of small odontocete species could reveal the degree of phylogenetic signal that is present in vertebral morphology, and could further substantiate whether habitat and foraging strategies influence morphology in these taxa of aquatic life and axial locomotion.
Data Availability Statement
The datasets presented in this study can be found in online repositories, doi: 10.6084/m9.figshare.13017470.
Author Contributions
MCM, MSM, and RG-J: conceptualization and methodology. MCM and RG-J: formal analysis and writing – original draft. MCM: funding acquisition. MCM, MSM, MC, SD, and RG-J: investigation. SD, MC, and RG-J: resources. MCM, MSM, SD, MC, and RG-J: writing – review and editing. All authors contributed to the article and approved the submitted version.
Funding
This research is part of MCM’s Ph.D. thesis financed by a doctoral fellowship from the Consejo Nacional de Investigaciones Científicas y Técnicas (CONICET). Funding for travel and accommodation during data collection was provided by the Society for Marine Mammalogy (Small Grants in Aid of Research) and the Cetacean Society International. Moreover, a stay at the University of North Carolina Wilmington (UNCW) was possible due to accommodations provided by the UNCW. Funders had no role in study design, data collection and analysis, decision to publish, or preparation of the manuscript.
Conflict of Interest
The authors declare that the research was conducted in the absence of any commercial or financial relationships that could be construed as a potential conflict of interest.
Acknowledgments
MCM would like to specially thank Dr. Ann Pabst and Bill McLellan, and all the people at their lab, for receiving her at the University of North Carolina Wilmington and teaching her muscle dissection techniques. MCM thanks museums and collection curators for assistance during specimen preparation and data collection. MCM would like to dedicate this paper to the memory of Dr. Natalie R. Prosser Goodall: a pioneer in marine mammals’ research in southernmost Argentina, a true mentor and friend.
Supplementary Material
The Supplementary Material for this article can be found online at: https://www.frontiersin.org/articles/10.3389/fmars.2020.581762/full#supplementary-material
References
Ballance, L. T. (2018). “Cetacean ecology,” in Encyclopedia of Marine Mammals, 3 Edn, eds B. Würsig, J. G. M. Thewissen, and K. M. Kovacs (Cambridge, MA: Academic Press), 172–180. doi: 10.1016/b978-0-12-804327-1.00087-x
Banguera-Hinestroza, E., Hayano, A., Crespo, E. A., and Hoelzel, A. R. (2014). Delphinid systematics and biogeography with a focus on the current genus Lagenorhynchus: multiple pathways for antitropical and trans-oceanic radiation. Mol. Phylogenet. Evol. 80, 217–230. doi: 10.1016/j.ympev.2014.08.005
Bookstein, F. L. (1996a). Biometrics, biomathematics and the morphometric synthesis. Bull. Math. Biol. 58, 313–365. doi: 10.1016/0092-8240(95)00329-0
Bookstein, F. L. (1996b). “Combining the tools of geometric morphometrics,” in Advances in Morphometrics, Nato ASI Series, Series A: Life Science, Vol. 284, eds L. F. Marcus, M. Corti, A. Loy, G. J. P. Nylor, and D. E. Slice (New York, NY: Plenum Press), 131–152. doi: 10.1007/978-1-4757-9083-2_12
Buchholtz, E. A. (2001). Vertebral osteology and swimming style in living and fossil whales (Order: Cetacea). J. Zool. 253, 175–190. doi: 10.1017/s0952836901000164
Buchholtz, E. A., and Schur, S. A. (2004). Vertebral osteology in Delphinidae (Cetacea). Zool. J. Linn. Soc. 140, 383–401. doi: 10.1111/j.1096-3642.2003.00105.x
Cliff, N. (1988). The eighenvalues-greater-than-one rule and the reliability of components. Psyc. Bull. 103, 276–279. doi: 10.1037/0033-2909.103.2.276
Committee on Taxonomy (2020). List of Marine Mammal Species and Subspecies. Society for Marine Mammalogy. Available online at: www.marinemammalscience.org (accessed September 20, 2020).
Coscarella, M. A., Pedraza, S. N., and Crespo, E. A. (2010). Behavior and seasonal variation in the relative abundance of Commerson’s dolphin (Cephalorhynchus commersonii) in northern Patagonia, Argentina. J. Ethol. 28, 463–470. doi: 10.1007/s10164-010-0206-4
Cotten, P. B., Piscitelli, M. A., McLellan, W. A., Rommel, S. A., and Pabst, D. A. (2008). The gross morphology and histochemistry of respiratory muscles in bottlenose dolphins, Tursiops truncatus. J. Morphol. 269, 1520–1538. doi: 10.1002/jmor.10668
Cozzi, B., Huggenberg, S., and Oelschäler, H. (2017). Anatomy of Dolphins: Insights into Body Structure and Function. London: Academic Press.
Crovetto, A. (1991). Etude osteometrique et anatomo-funcionelle de la colonne vertebrale chez grans cetaces [Osteometric and anatomo-functional study of the vertebral column in large cetaceans]. Invest. Cetacea 23, 71–89.
Degrati, M., Dans, S. L., Garaffo, G. V., and Crespo, E. A. (2012). Diving for food: a switch of foraging strategy of dusky dolphins in Argentina. J. Ethol. 30, 361–367. doi: 10.1007/s10164-012-0333-1
Dellabianca, N., Pierce, G. J., Raya Rey, A., Scioscia, G., Miller, D. L., Torres, M., et al. (2016). Spatial models of abundance and habitat preferences of Commerson’s and Peale’s Dolphin in Southern Patagonian waters. PLoS One 11:e0163441. doi: 10.1371/journal.pone.0163441
Filler, A. G. (1986). Axial Character Seriation in Mammals: An Historical and Morphological Exploration of the Origin, Development, Use and Current Collapse of the Homology Paradigm. Ph. D thesis, Harvard University, Cambridge, MA.
Fish, F. E. (2002). Balancing requirements for stability and maneuverability in cetaceans. Int. Comp. Biol. 42, 85–93. doi: 10.1093/icb/42.1.85
Fish, F. E., Peacock, J., and Rohr, J. (2003). Stabilization mechanism in swimming odontocete cetaceans by phased movements. Mar. Mamm. Sci. 19, 515–528. doi: 10.1111/j.1748-7692.2003.tb01318.x
Fish, F. E., and Rohr, J. (1999). Review of Dolphin Hydrodynamics and Swimming Performance. Technical Report 1801. San Diego, CA: SPAWARS System Center.
Franchini, F., Smout, S., Blight, C., Boehme, L., Munro, G., Costa, M., et al. (2020). Habitat partitioning in sympatric delphinids around the Falkland islands: predicting distributions based on a limited data set. Front. Mar. Sci. 7:277. doi: 10.3389/fmars.2020.00277
Gal, J. (1992). Spinal flexion and locomotor energetics in kangaroo, monkey, and tiger. Can. J. Zool. 70, 2444–2451. doi: 10.1139/z92-329
Gal, J. (1993a). Mammalian spinal biomechanics. I. Static and dynamic mechanical properties of intact intervertebral joints. J. Exp. Biol. 174, 247–280.
Gal, J. (1993b). Mammalian spinal biomechanics. II. Intervertebral lesion experiments and mechanisms of bending resistance. J. Exp. Biol. 174, 281–297.
Galatius, A., and Goodall, R. N. P. (2016). Skull shapes of the Lissodelphininae: radiation, adaptation and asymmetry. J. Morphol. 277, 776–785. doi: 10.1002/jmor.20535
Garraffo, G. V., Dans, S. L., Pedraza, S. N., Degrati, M., Schiavini, A., González, R., et al. (2011). Modeling habitat use for dusky dolphin and Commerson’s dolphin in patagonia. Mar. Ecol. Prog. Ser. 421, 217–227. doi: 10.3354/meps08912
Gillet, A., Frédérich, B., and Parmentier, E. (2019). Divergent evolutionary morphology of the axial skeleton as a potential key innovation in modern cetaceans. Proc. R. Soc. B 286:20191771. doi: 10.1098/rspb.2019.1771
Goodall, C. (1991). Procrustes methods in the statistical analysis of shape. J. R. Stat. Soc. 53, 285–339. doi: 10.1111/j.2517-6161.1991.tb01825.x
Goodall, R. N. P. (1994). “Commerson’s dolphin Cephalorhynchus commersonii (Lacépèd 1804),” in Handbook of Marine Mammals, Volume 5, The First Book of Dolphin, ed. S. H. Ridgway (London: Academic Press), 241–267.
Goodall, R. N. P., Galeazzi, R. A., Leatherwood, S., Miller, K. W., Cameron, I. S., Kastelein, R. K., et al. (1988). “Studies of Commerson’s dolphins, Cephalorhynchus commersonii, off Tierra del Fuego, 1976-1984, with a review of information of the species in South Atlantic,” in Reports of the International Whaling Commission, Special Issue 9, eds R. L. Brownell and G. P. Donovan (Cambridge. MA: International Whaling Commission), 3–70.
Goodall, R. N. P., Baker, A. N., Best, P. B., Mayer, M., and Miyazaki, N. (1997a). On the biology of the hourglass dolphin, Lagenorhynchus cruciger (Quoy and Gaimard, 1824). Rep. Int. Whal. Comm. 47, 985–999.
Goodall, R. N. P., de Haro, J. C., Fraga, F., Iñiguez, M. A., and Norris, K. S. (1997b). Sightings and behaviour of the Peale’s dolphins, Lagenohrynchus australis, with notes on dusky dolphins, L. obscurus, off southernmost South America. Rep. Int. Whal. Comm. 47, 757–775.
Goodall, R. N. P., Norris, K. S., Schevill, W. E., Fraga, F., Praderi, R., Iñiguez, M. A., et al. (1997c). Review and update on the biology of Peale’s dolphin, Lagenorhynchus australis. Rep. Int. Whal. Comm. 47, 777–796.
Gross, A., Kiszka, J., Cannyet, O. V., Richard, P., and Ridoux, V. (2009). A preliminary study of habitat and resource partitioning among co-occurring tropical dolphins around Mayotte, southwest Indian Ocean. Estuar. Coast Shelf S. 84, 367–374. doi: 10.1016/j.ecss.2009.05.017
Guttman, L. (1954). Some necessary conditions for common factor analysis. Psycometrica 19, 149–161. doi: 10.1007/bf02289162
Jackson, D. A. (1993). Stopping rules in principal components analysis: a comparison of heuristical and statistical approaches. Ecology 74, 2204–2214. doi: 10.2307/1939574
Klingenberg, C. P. (2011). MorphoJ: an integrated software package for geometric morphometrics. Mol. Ecol. Resour. 11, 353–357. doi: 10.1111/j.1755-0998.2010.02924.x
Klingenberg, C. P. (2016). Size, shape and form: concepts of allometry in geometric morphometrics. Dev. Gene. Evol. 226, 113–137. doi: 10.1007/s00427-016-0539-2
Koob, T. J., and Long, J. H. Jr. (2000). The vertebrate body axis: evolution and mechanical function. Am. Zool. 40, 1–18. doi: 10.1093/icb/40.1.1
Lockyer, C., Goodall, R. N. P., and Galeazzi, R. A. (1988). “Age and body length characteristics of Cephalorhynchus commersonii from incidentally-caught specimens off Tierra del Fuego,” in Reports of the International Whaling Commission, Special Issue 9, eds R. L. Brownell and G. P. Donovan (Cambridge: International Whaling Commission), 103–118.
Long, J. H. Jr. (1992). Stiffness and damping forces in the intervertebral joints of blue marlin, (Makaira nigricans). J. Exp. Biol. 162, 131–155.
Long, J. H. Jr. (1995). Morphology, mechanics, and locomotion: the relation between the notochord and swimming speed in sturgeon. Environ. Biol. Fish. 44, 199–211. doi: 10.1007/bf00005916
Long, J. H. Jr., Pabst, D. A., Shepherd, W. R., and McLellan, W. (1997). Locomotor design of dolphin vertebral columns: bending mechanics and morphology of Delphinus delphis. J. Exp. Biol. 200, 65–81.
MacLeod, N. (1999). Generalizing and extending the eigen shape method of shape space visualization and analysis. Paleobiology 25, 107–108.
Maddux, S. D., and Franciscus, R. G. (2009). Allometric scaling of infraorbital surface topography in Homo. J. Hum. Evol. 56, 161–174. doi: 10.1016/j.jhevol.2008.10.003
Marchesi, M. C., Boy, C. C., Dans, S. L., Mora, M. S., and González-José, R. (2020a). Morphology of the vertebral centra in dolphins off the south western South Atlantic: a 3D morphometric approach and functional implications. Mar. Mamm. Sci. 36, 548–564. doi: 10.1111/mms.12660
Marchesi, M. C., Dans, S. L., Mora, M. S., and González-José, R. (2020b). Allometry and ontogeny in the vertebral column of southern hemisphere dolphins: a 3D approach. J. Mamm. Evol.
Marchesi, M. C., Mora, M. S., Crespo, E. A., Boy, C. C., González-José, R., and Goodall, R. N. P. (2018). Functional subdivision of the vertebral column in four South American dolphins. J. Neotrop. Mamm. 25, 329–343. doi: 10.31687/saremmn.18.25.2.0.12
Marchesi, M. C., Mora, M. S., Pimper, L. E., and Goodall, R. N. P. (2017). Can habitat characteristics shape vertebral morphology in dolphins? An example of two phylogenetically related species from southern South America. Mar. Mamm. Sci. 33, 1126–1148. doi: 10.1111/mms.12432
McGowen, M. R., Tsagkogeorga, G., Álvarez-Carretero, S., dos Reis, M., and Struebig, M. (2020). Phylogenomic resolution of the cetacean tree of life using target sequence capture. Syst. Biol. 69, 479–501. doi: 10.1093/sysbio/syz068
Oxnard, C. E. (1978). One biologist’s view of morphometrics. Ann. Rev. Ecol. Evol. Syst. 9, 219–241. doi: 10.1146/annurev.es.09.110178.001251
Pabst, D. A. (1990). “Axial muscles and connective tissues of the bottlenose dolphin,” in The Bottlenose Dolphin, eds S. Leatherwood and R. R. Reeves (San Diego: Academic Press), 51–67. doi: 10.1016/b978-0-12-440280-5.50007-x
Pabst, D. A. (1993). Intramuscular morphology and tendon geometry of the epaxial swimming muscles of dolphins. J. Zool. 230, 159–176. doi: 10.1111/j.1469-7998.1993.tb02679.x
Pabst, D. A. (2000). To bend a dolphin: convergence of force transmission designs in cetaceans and scombrid fishes. Am. Zool. 40, 146–155. doi: 10.1093/icb/40.1.146
Paschetta, C., De Azevedo, S., González, M., Quito-Sánchez, M., Cintas, C., Varela, H., et al. (2016). Shifts in subsistence type and its impact on the human skull’s morphological integration. Am. J. Hum. Biol. 28, 118–128. doi: 10.1002/ajhb.22746
Pérez, S. I., Bernal, V., and González, P. V. (2006). Differences between sliding semi-landmark methods in geometric morphometrics, with an application to human craniofacial and dental variation. J. Anat. 208, 769–784. doi: 10.1111/j.1469-7580.2006.00576.x
Perrin, W. F. (1975). Variation of spotted and spinner porpoises (genus Stenella) in the eastern tropical Pacific and Hawaii. Bull. Scripps. Inst. Oceanogr. 21:206.
Piscitelli, M. A., McLellan, W. A., Rommel, S. A., Blum, J., Barco, S. G., and Pabst, D. A. (2010). Lung size and thoracic morphology in shallow (Tursiops truncatus) and deep (Kogia spp.) diving cetaceans. J. Morphol. 271, 654–673.
R Core Team (2017). R: A Language and Environment for Statistical Computing. Vienna: R Foundation Statistical Computing.
Riccialdelli, L., Newsome, S. D., Fogel, M. L., and Goodall, R. N. P. (2010). Isotopic assessment of prey and habitat preferences of a cetacean community in the southwestern South Atlantic Ocean. Mar. Ecol. Prog. Ser. 418, 235–248. doi: 10.3354/meps08826
Rohlf, F. J., and Slice, D. E. (1990). Extensions of the Procrustes method for the optimal superimposition of landmarks. Syst. Zool. 39, 40–59. doi: 10.2307/2992207
Rommel, S. A., and Reynolds, J. E. (2018). “Skeleton,” in Encyclopedia of Marine Mammals, 3 Edn, eds B. Würsig, J. G. M. Thewissen, and K. M. Kovacs (London: Academic Press), 861–871.
Schiavini, A. C. M., Pedraza, S. N., Crespo, E. A., González, R., and Dans, S. L. (1999). Abundance of dusky dolphins (Lagenorhynchus obscurus) off north and central Patagonia, Argentina, in spring and comparison with incidental catch in fisheries. Mar. Mamm. Sci. 15, 828–840. doi: 10.1111/j.1748-7692.1999.tb00845.x
Schlager, S. (2017). “Morpho and Rvcg – shape analysis in R,“ in Statistical Shape and Deformation Analysis, eds G. Zheng, S. Li and G. Szekely (London: Academic Press), 217–256.
Sheets, H. D., Keonho, K., and Mitchell, C. E. (2004). “A combined landmark and outline-based approach to ontogenetic shape change in the ordovician trilobite Triarthrus becki,” in Applications of Moprhometrics in Paloentology and Biology, ed. A. Elewa (New York, NY: Springer), 67–81. doi: 10.1007/978-3-662-08865-4_6
Slice, D. E. (2001). Landmark coordinates aligned by Procrustes analysis do not lie in Kendall’s shape space. Syst. Biol. 50, 141–149. doi: 10.1080/10635150119110
Slijper, E. J. (1936). Die Cetaceen, Vergleichend-Anatomisch und Systematisch [The Cetaceans, Compared Anatomy and Systematics]. New York, NY: Asher.
Slijper, E. J. (1946). Comparative biologic anatomical investigations on the vertebral column and spinal musculature of mammals. Tweede Sect. 17:128.
Slijper, E. J. (1961). Locomotion and locomotory organs in whales and dolphins (Cetacea). Symp. Zool. Soc. Lond. 5, 77–94.
Vollmer, N. L., Ashe, E., Brownell, R. L. Jr., Cipriano, F., and Mead, J. G. (2019). Taxonomic revision of the dolphin genus Lagenorhynchus. Mar. Mamm. Sci. 35, 957–1057. doi: 10.1111/mms.12573
Weihs, D. (2002). Stability versus maneuverability in aquatic locomotion. Int. Comp. Biol. 42, 127–134. doi: 10.1093/icb/42.1.127
Woodward, B. (2006). Locomotory Strategies, Dive Dynamics, and Functional Morphology of the Mysticetes: Using Morphometrics, Osteology, and DTAG Data to Compare Swim Performance in Four Species of Baleen Whales. Ph. D thesis, University of Maine, Orono.
Würsig, B., and Würsig, M. (1980). Behavior and ecology of dusky dolphins, Lagenorhynchus obscurus, in the South Atlantic. U.S. Fish. Bull. 77, 871–890.
Keywords: dolphin, flexibility, geometric morphometrics, maneuverability, southern hemisphere, vertebral morphology
Citation: Marchesi MC, Mora MS, Dans SL, Coscarella MA and González-José R (2020) Vertebral Morphology in Partially Sympatric Dolphins: A 3D Approach. Front. Mar. Sci. 7:581762. doi: 10.3389/fmars.2020.581762
Received: 09 July 2020; Accepted: 14 October 2020;
Published: 10 November 2020.
Edited by:
Lars Bejder, University of Hawai‘i at Mānoa, United StatesReviewed by:
Anders Galatius, Aarhus University, DenmarkRobert William Boessenecker, College of Charleston, United States
Copyright © 2020 Marchesi, Mora, Dans, Coscarella and González-José. This is an open-access article distributed under the terms of the Creative Commons Attribution License (CC BY). The use, distribution or reproduction in other forums is permitted, provided the original author(s) and the copyright owner(s) are credited and that the original publication in this journal is cited, in accordance with accepted academic practice. No use, distribution or reproduction is permitted which does not comply with these terms.
*Correspondence: Maria C. Marchesi, bWFyY2hlc2ltY0BnbWFpbC5jb20=; orcid.org/0000-0002-9926-6719