- 1Geochemistry and Isotope BioGeoChemistry Group, Department of Marine Geology, Leibniz Institute for Baltic Sea Research (IOW), Warnemünde, Germany
- 2Department of Biological Sciences, Institute of Environment, Florida International University, Miami, FL, United States
- 3Institute for Coastal Research, Helmholtz-Zentrum Geesthacht (HZG), Geesthacht, Germany
Seagrasses can enhance carbonate sediment dissolution on diel timescales through oxidation of the rhizosphere and production of acidic exudates of dissolved organic matter (DOM). Carbonates can also associate with DOM either from biogenesis or later adsorption. However, the impact of mineral dissolution on the release of carbonate-associated DOM and on surface water DOM quantity and quality is unclear. We analyzed sub-daily changes in EEMS-PARAFAC components (excitation-emission matrices with parallel factor analysis), fluorescence, and absorbance properties of surface waters over adjacent low- and high-density (LD and HD) Thalassia testudinum seagrass meadows in Florida Bay, United States. We compared fluorescent DOM characteristics of seagrass leaves, acidified (dissolved) sediment leachates, and surface water samples collected from the HD and LD sites with surface water from a nearby mangrove island. The HD site was higher in humic-like PARAFAC components, specific ultraviolet absorbance, and humification index. We did not observe changes in EEMs indices or PARAFAC components with cumulative photosynthetically active radiation, indicating that photodegradation was unlikely to contribute to temporal variability in DOM. Similarities among DOM optical properties from acidified sediment leachates and surface waters at both sites suggest the importance of carbonate dissolution/reprecipitation for DOM cycling, while seagrass leaf leachates were markedly dissimilar to surface waters. We observed similarities among the acidified sediment leachate, surface water, and porewater elsewhere in Florida Bay, indicating dynamic coupling between these DOM pools. From this short study, Florida Bay DOM cycling appears to be more sensitive to carbonate dissolution than to additional photodegradation or authigenic seagrass leaching.
Introduction
Seagrass ecosystems are important players in the global carbon cycle, via the “blue carbon” mechanism of organic carbon storage in underlying sediments (Fourqurean et al., 2012a). Sedimentary organic carbon in seagrass meadows contains both autochthonous and allochthonous organic material from terrestrial or marine origins (Kennedy et al., 2010), and can be protected from further degradation as seagrasses can stabilize sediments, reducing resuspension and oxidative loss (Serrano et al., 2020). In carbonate sediments, however, the processes of calcium carbonate precipitation and dissolution causes the production or consumption of CO2 in excess of net ecosystem metabolism (Mazarrasa et al., 2015; Howard et al., 2018). This carbonate dissolution can be enhanced in seagrass meadows via seagrass pumping of oxygen into the sediments during photosynthesis (Eldridge and Morse, 2000; Burdige and Zimmerman, 2002; Burdige et al., 2008, 2010), and can follow seasonal (Barrón et al., 2006; Egea et al., 2019) and diel patterns (Ziegler and Benner, 1999; Maher and Eyre, 2010), as demonstrated by lower porewater sulfide in the middle of the day (Lee and Dunton, 2000). However, the impact of this carbonate dissolution on “blue carbon” organic matter remains uncertain.
In addition to particulate organic matter, carbonate-associated organic matter contributes to the “blue carbon” stock of carbonate seagrass meadows (Howard et al., 2018). In laboratory studies, carbonates can adsorb and remove from solution aspartic acid rich fulvic acids (Carter, 1978), stearic acids, and egg albumin (Suess, 1970). In carbonate sediments, organic matter containing contain acidic functional groups can adsorb onto carbonate minerals, and autochthonous organic matter can be protected in the intracrystalline network of carbonate minerals at the point of biogenesis (Müller and Suess, 1977; Ingalls et al., 2004). It is unknown whether seagrass-mediated carbonate dissolution also results in the release of sediment-associated organic matter, or if this affects water column dissolved organic matter (DOM) quality and quantity.
Excitation-emission matrices (EEMs) with parallel factor analysis (PARAFAC) is a useful tool for assessing natural organic matter cycling and dynamics in aquatic environments (Jaffé et al., 2014), and has been implemented in an adsorption study of terrestrial DOM to Florida carbonate aquifer samples (Jin and Zimmerman, 2010). In Florida Bay, the site for this study, EEMs has been implemented to explore seasonal and episodic drivers of variations in DOM (Maie et al., 2006, 2012; Ya et al., 2015). However, the contributions of diel forcing, benthic fluxes, seagrass density, or associations with carbonate sediments to the fluorescent DOM (FDOM) signal remains uncertain. We hypothesized that surface water FDOM in seagrass meadows would vary on diel timescales because of the combined processes of sediment dissolution, seagrass exudation, and photodegradation. To test this, we used a diel sampling framework to quantify dissolved organic carbon (DOC) concentrations and FDOM at two sites of high- and low-density Thalassia testudinum seagrass meadows in Florida Bay. In a related experiment, we investigated the optical properties of FDOM released from acidified sediment leachates and porewater, in order to understand the contribution of sediment-associated organic matter and sediment dissolution to FDOM variability.
Methods
Study Site
Florida Bay (FB) is a carbonate rich, seagrass-dominated, shallow estuary in southern Florida. Primary productivity in this oligotrophic ecosystem is supported in part by the release of nutrients (Nitrogen, N, and Phosphorus, P) stored in sediments (Fourqurean et al., 2012b). The primary source of P is from the Gulf of Mexico, where seagrass productivity is higher, while the main freshwater source is through Taylor Slough from the Everglades in the northeastern bay, and a network of numerous mudbanks restrict the flow of water between basins (Fourqurean and Robblee, 1999). The sediments range from 70 to 95% calcium carbonate (Caccia et al., 2003), and associations between inorganic P and carbonates contribute to the P limitation of the ecosystem (Koch et al., 2001). While carbonate dissolution is variable, it tends to be higher in regions of increased P limitation and during periods of hyper salinity (Yates and Halley, 2006) which is likely related to the strategies employed by seagrasses to release limited carbonate-associated P through organic acid exudates (Long et al., 2008) or enhanced sulfide oxidation through oxygen pumping in the rhizosphere (Ku et al., 1999; Jensen et al., 2009) or bioturbation (Walter et al., 1993). Diurnal variations in carbonate dissolution and precipitation processes can lead to strong variations in water column alkalinity, dissolved CO2, and pH in Florida Bay (Yates and Halley, 2006; Yates et al., 2007).
We visited the same high- and low-density seagrass sites (HD and LD, respectively) as a concurrent study into net ecosystem productivity of Florida Bay seagrasses, which were both found to be net dissolving during the study period (Van Dam et al., 2019), and are separated from each other by mudbanks and a mangrove island (M, Figure 1). Both HD and LD sites are located in northeastern FB, and share similar sedimentary organic matter sources (Xu et al., 2006), surface water organic matter characteristics (Maie et al., 2012), and sedimentary CaCO3 content (Caccia et al., 2003). The five additional sites throughout FB have variable seagrass presence and span the productivity gradient (TC, DK, LM, BA, and SB, Figure 1).
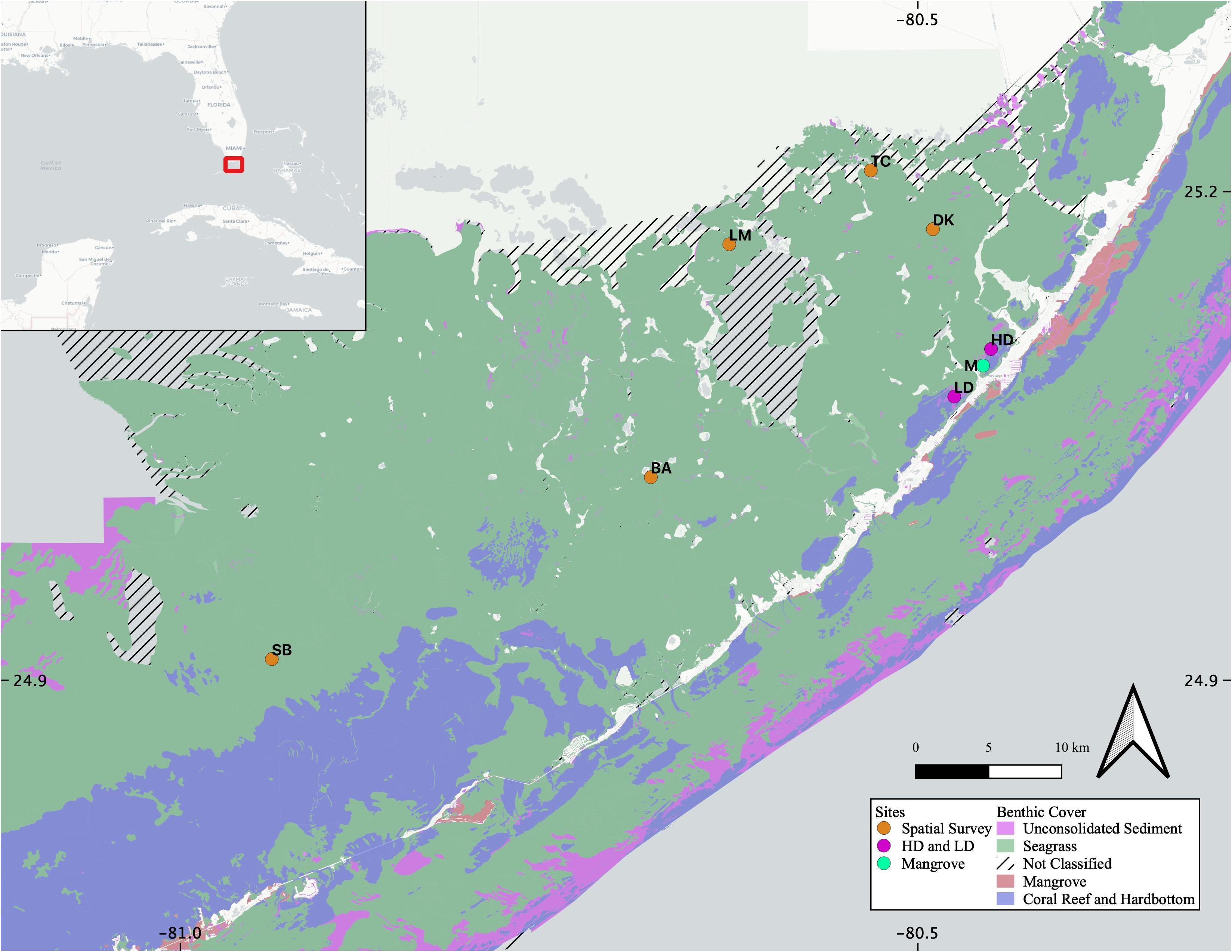
Figure 1. Site map, including the location of the study area within the broader southeastern United States (inset, red box). High density (HD) and low density (LD) seagrass sites were sampled for the diel portion of the study, as well as sediment and seagrass leaching. The Mangrove site (M) was sampled for surface water comparison. The five additional sites sampled for porewater, surface water, and sediment leaching comparisons cover the entire range of Florida Bay (DK, TC, LM, BA, and SB). Benthic coverage data was acquired from the Florida Fish and Wildlife Conservation Commission- Fish and Wildlife Research Institute (FWRI FWC), Unified Reef Map.
Sampling Campaigns
Two multi-day diel sampling campaigns (8 days total) were conducted at HD and LD in late 2018 (Figure 1). Surface water (SW) samples were collected on a dawn-noon-dusk cycle during the first campaign (Oct. 28 through Nov. 01, 2018), and 4 times per day during the second campaign (November 25 through November 29, 2018). At each time point, 60 mL SW was filtered (0.45 μm) and stored in dark, acid washed, 60-mL HDPE dark bottles triple rinsed with site water. These samples were placed immediately on ice, then refrigerated at 4°C until further analysis. Excitation emission matrices (EEMs) and absorbance spectra were collected within 2 weeks, and all remaining sample water was acidified to Ph < 2 with HCl and stored at 4°C until dissolved organic carbon (DOC) analysis within 4 months of collection. Sediment grab samples and seagrass leaf clippings were collected at the HD and LD sites. Three SW samples were also collected from M (Figure 1) during the first campaign. Photosynthetically active radiation (PAR) was measured in the water column at both sites using a submerged Sea-bird ECO-PAR sensor (Van Dam et al., 2019), and was integrated over the day to obtain cumulative PAR (E/m2) for our analyses.
A third sampling campaign was conducted in April 2019, in which filtered (0.45 μm) pore water (PW), filtered SW, and sediment grab samples were collected in a spatial survey of five additional sites (TC, DK, LM, BA, and SB, Figure 1). PW was collected using a porewater sipper with a surface shield from 10 cm sediment depth.
Sediment and Seagrass Leaf Leachates
Wet carbonate sediments were divided into two equal portions of 5–10 grams each, then diluted with ∼60 mL deionized water (DI). One portion was acidified with enough 12N HCl (about 20 drops) to give appreciable but incomplete dissolution, so as to maintain buffering and not significantly alter the supernatant pH. The sediment and supernatant solution were allowed to leach at 4°C for 48 h, upon which time the sample was filtered (0.45 μm) prior to EEMS and DOC analysis. This material constitutes the “SEDHCl” and “SEDDI” described in the results. Due to the cold leaching temperature and the pH of deionized water, a small fraction of the carbonate sediment would also be dissolved in the SEDDI treatment, however, SEDHCl represents substantially higher sediment dissolution. Seagrass leaves from both the HD and LD sites (150–500 mg) were leached with 60 mL DI water at 4°C for 48 h; we term this leachate “SG.” EEMs and Absorbance data were collected on all leachates, however, DOC was analyzed for the SEDHCl and SEDDI leachates from the third campaign only. Sedimentary metals have been shown to quench the fluorescence of humic substances (Haider and Guggenberger, 2004), however, the low amount of metals such as Fe, Al, and Mn in our sediments (Caccia et al., 2003), and the incomplete dissolution of the SEDHCl method suggests that the metal concentrations of the leachates would not have a large effect on FDOM signal (Poulin et al., 2014; Liu et al., 2018).
DOC, EEMs, and Absorbance Acquisition
Dissolved organic carbon was determined on acidified and filtered (0.45 μm) samples using a Shimadzu TOC-V total organic carbon analyzer. Absorbance spectra were collected (240–621 nm, 3 nm interval) concurrently with the EEMs using an Aqualog spectrofluorometer (HORIBA Scientific, HORIBA New Jersey Optical Spectroscopy Center, United States) equipped with a 150 W continuous output Xenon arc lamp and a 1 cm quartz cuvette. The emission scans were collected from 250 to 621 nm, with intervals of ∼1.5 nm, and were acquired at excitation wavelengths of 240–621 nm at intervals of 3 nm. Post-acquisition data were blank-subtracted with Milli-Q water, and corrected for inner filter effects (McKnight et al., 2001). EEMs are provided in Raman units (RU).
Specific UV absorbance at 254 (SUVA254) is defined as the absorbance at 254 nm (m–1) normalized to DOC in mg-C L–1 (Weishaar et al., 2003). A254 was estimated by linear interpolation between A255 and A252. Absorbance at 351 (A351) was used instead of A350, and scaled to DOC. The slope ratio (SR) is the ratio of the spectral slopes S275–295 to S350–400, which in turn were calculated by fitting a linear regression to the natural log-transformed absorbance between 275–295 and 350–400 nm (Helms et al., 2008). The fluorescence-derived indices of humification index (HIX), fluorescence index (FI) and biological (freshness) index (BIX) were calculated directly from the EEM spectra (Wagner et al., 2015). HIX tends to increase with humification and microbial processing of DOM (Wickland et al., 2007), FI has been used to assess microbial vs. terrestrial contributions to DOM (Cory et al., 2005), and BIX has been correlated with recently produced DOM (Parlanti et al., 2000). Statistical analyses were carried out in MatLab R2017a and R Studio. The relationship between each index and cumulative PAR were quantified with a linear model in R, and the slopes are provided in Supplementary Table S1. Differences between sites and campaigns for each index were calculated from one-way analysis of variance (one-way ANOVA), and are represented graphically in Supplementary Figure S5.
Parallel factor analysis modeling and were carried out using the DrEEM 3.0 toolbox in MatLab R2017a (Murphy et al., 2013). The PARAFAC model was run on n = 97 of the 100 samples using an excitation wavelength range of 258 to 498 nm, and the zap function was used to remove anomalous peaks individually. Three samples were removed as outliers, as the high leveraging had the potential to distort the model results. A 5-component model was validated using the split-half analysis S4C4T2 and was then applied to the non-normalized full dataset. When comparisons are made between pools (PW, SW, SEDDI, and SEDHCl) PARAFAC components are expressed in percent, while temporal variations in PARAFAC components are expressed in RU. Due to the potential for matrix effects, especially the presence of halides, impacting the range of samples differently (i.e., SW vs. SEDDI vs. SEDHCl), comparisons between these sample types are made in a qualitative manner (Grebel et al., 2009).
Results and Discussion
Description of PARAFAC Components
A 5-component PARAFAC model was validated for our data set (Supplementary Figure S1). Previously, an 8-component PARAFAC model was fit to south Florida surface water samples (Chen et al., 2010; Murphy et al., 2014), but this model could not be applied to the present dataset because of the differences in wavelength intervals between the spectrophotometer used in this study (Aqualog), and that used in Chen et al., 2010 (FluoroMax 3). A visual comparison of the PARAFAC components (Supplementary Figure S2) shows that our 5-component PARAFAC model captures the same general FDOM groups as the 8-component model, summarized as follows: Our C1 is similar to the reported components 3, 4, and 6, and is humic-like (Yamashita and Jaffé, 2008). Our C2 is similar to the reported component 1 and component 5, and is also humic-like. Our C3 is analogous to the reported component 2, and is humic-like. Our C4 is similar to the reported component 8 and is tryptophan protein-like. Finally, our C5 is similar to the reported component 7, and is tyrosine protein-like. The similarity between these components allows us to set our findings in the context of prior research, and the implications will be discussed in subsequent sections.
Diel Variability in Surface Water FDOM
The moderate growth in SR and FI, and moderate reduction in HIX, observed during the second half of the second campaign (Supplementary Figure S3) corresponds temporally with an increase in wind speed and a drop in sea surface temperature (Van Dam et al., 2019), but otherwise temporal trends in fluorescence indices, absorbance indices, and PARAFAC components were not detected. DOC was variable at both sites throughout the study period, but was comparable with DOC values observed in central Florida Bay through a decade of monthly monitoring (Jaffé, 2018; Supplementary Figure S4). We hypothesized that photodegradation could be a predominant factor contributing to diel variability in surface water FDOM given that a previous study applying the 8-component model (Supplementary Figure S2) found that all of their components exhibited some response to light (Chen and Jaffé, 2014). Interestingly, their component 2 (similar to our C3), appeared to be a product of photodegradation, and their component 7 (similar to our C5), appeared highly photolabile (Chen and Jaffé, 2014, 2016). Despite this photo-lability and photo-production, we detected no changes in any PARAFAC component including C3 and C5 with varying cumulative PAR (Figures 2A,B and Supplementary Table S1).
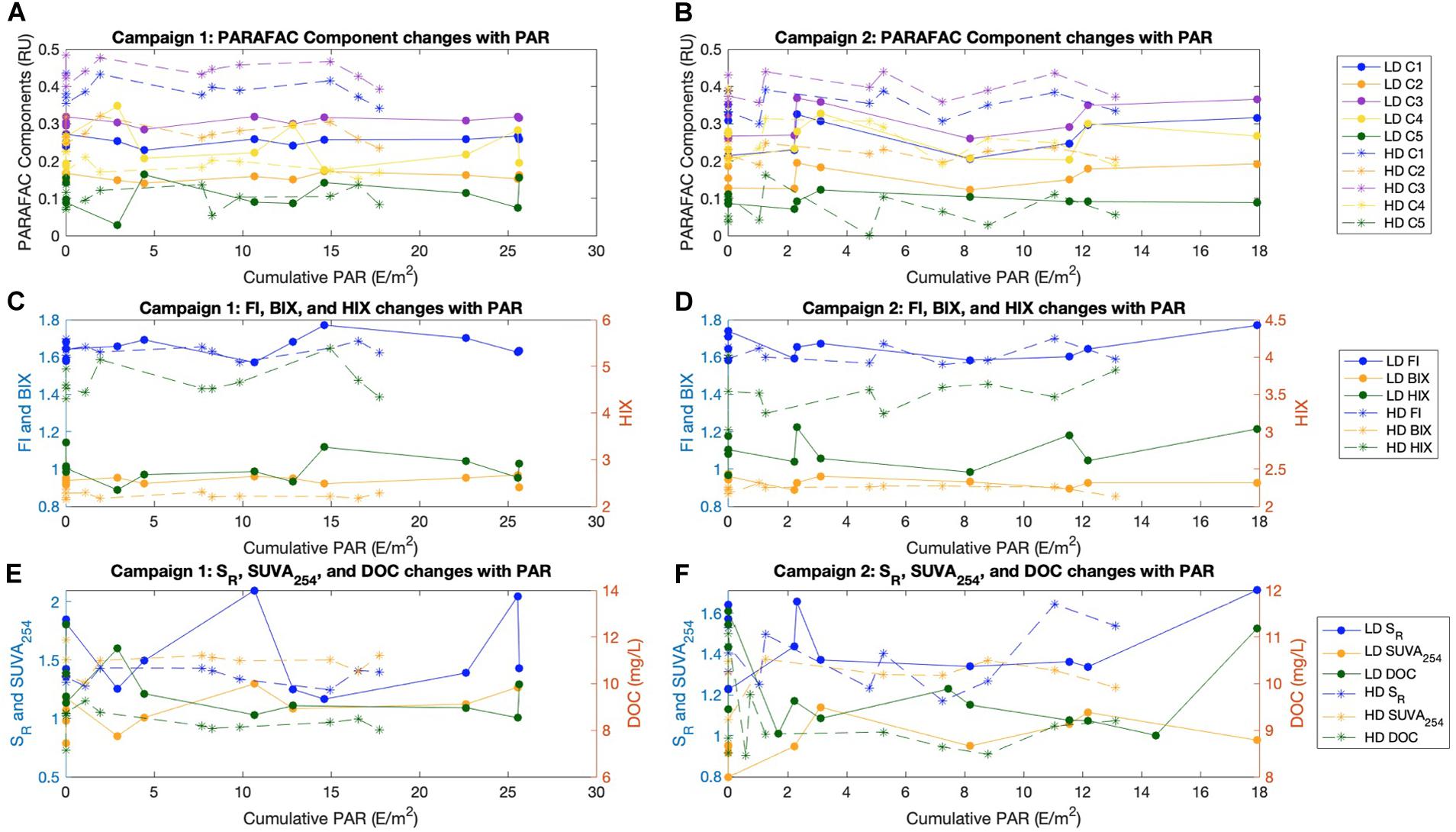
Figure 2. All indices and PARAFAC components plotted against cumulative PAR (E/m2) for the diel study, separated between Campaign 1 (Left) and Campaign 2 (Right), as well as by site (LD circles and lines, HD asterisk and dashed). (A,B) PARAFAC components in Raman units (RU). (C,D) FI and BIX on the left axis, HIX on the right axis. (E,F) SR and SUVA254 on the left axis and DOC (mg/L) on the right axis.
Reductions in SUVA254 are often considered as evidence for photodegradation, but we did not measure variation in SUVA254 with PAR. However, our low SUVA254 values of ∼1.0 (LD) to ∼1.6 (HD) suggest that this material is already highly photodegraded (Figures 2E,F). In fact, no measured index exhibited a significant increase or decrease with cumulative PAR (p > 0.05; Supplementary Table S1, Figure 2). Taken together, our results suggest that DOM in FB is already significantly photodegraded, such that fluorescence and absorbance indices are temporally stable on diel timescales. This is consistent with the sequential photo- and bio- degradation of neutral sediment leachates from SB (TS/Ph11 in reference), which approached the optical signature of FB surface waters (Chen and Jaffé, 2014).
We expected that the HD site would exhibit greater variability in optical properties and DOC concentration with PAR than at the LD site, as prior studies had linked seagrass density with benthic DOC flux (Ziegler and Benner, 1999) and carbonate dissolution (Burdige and Zimmerman, 2002; Yates and Halley, 2006; Burdige et al., 2010). Instead, we did not see any such diel variability for either the HD or LD site. However, this does not mean that seagrass density and sediment dissolution do not affect surface water optical properties, for two reasons. First, estimates of net carbonate dissolution/precipitation made during the coinciding study were also not strongly related to PAR, and while the HD site was more net dissolving than the LD site, these differences were not large (Van Dam et al., 2019). So, even if a benthic flux of sediment-associated FDOM is facilitated by sediment dissolution, we would not necessarily expect to see a relationship between FDOM indices and PAR for this particular short study period. Second, it is plausible that our water column approach was not sensitive enough, and that a more dedicated experiment such as core incubations or benthic chambers would be better suited to address this question.
Spatial Variability in Surface Waters Between HD and LD Sites
Although we observed limited diel variability in FDOM and related indices, there were strong spatial differences between the HD and LD sites, as well as between sampling campaigns, which were only 1 month apart during the wet (first campaign) and late wet (second campaign) season (Ya et al., 2015). All indices were similar at the LD site for both campaigns, however, at the HD site BIX and %C4 were lower and HIX, %C1, %C2, %C3, and A351/DOC were higher during the first campaign (Supplementary Figure S5). Between sites, the HD site was significantly higher in HIX, SUVA254, %C1, %C2, and %C3 which are all “humic-like” (Supplementary Figures S1, S5, p < 0.05). Other “non-humic” indices, such as FI and SR, were not statistically different across sites. Since the HD and LD sites were adjacent (Figure 1), any differences we observed could indicate fine-scale heterogeneity in DOM that was missed with broader spatial surveys (Maie et al., 2006, 2012). Furthermore, since the HD site was also higher in sedimentary organic carbon (Van Dam et al., 2019), it is plausible that variations in the “humic” DOM fraction may be related to differences in sedimentary organic matter between the two sites. This hypothesis motivated our sediment leachate assay.
Potential FDOM Source Attribution
We hypothesized the existence of three predominant FDOM sources to the HD and LD surface waters: (1) seagrass leachates/exudates, (2) nearby mangroves, and (3) sediment dissolution. Prior studies (Chen and Jaffé, 2014, 2016) used the 8-component FCE PARAFAC model to explore seagrass leaf leachates and explicitly non-dissolving sediment leachates from one site in western FB (SB, or TS/Ph11 in the reference). We expand on this work by comparing the surface water FDOM at both HD and LD sites with that of SG leachates, mangrove SW collected from M (Figure 1), and the SEDDI and SEDHCl leachates. Both HD and LD SG were dominated by C5, minimal C4, C2, and C1, and no C3, and contrasted sharply from that of HD and LD SW, which contained all components, but were dominated by C1, C3, and C4 (Figure 3A). Our SG leachates show similar fluorescence to SG in Chen and Jaffé (2014), who found that their seagrass leaf leachates at SB were also dominated by C5 (their component 7), and showed little or no C3 (their component 2). Although C5 was also likely highly photodegradable, these results suggest that seagrasses are not themselves predominant contributors to the surface water FDOM signal.
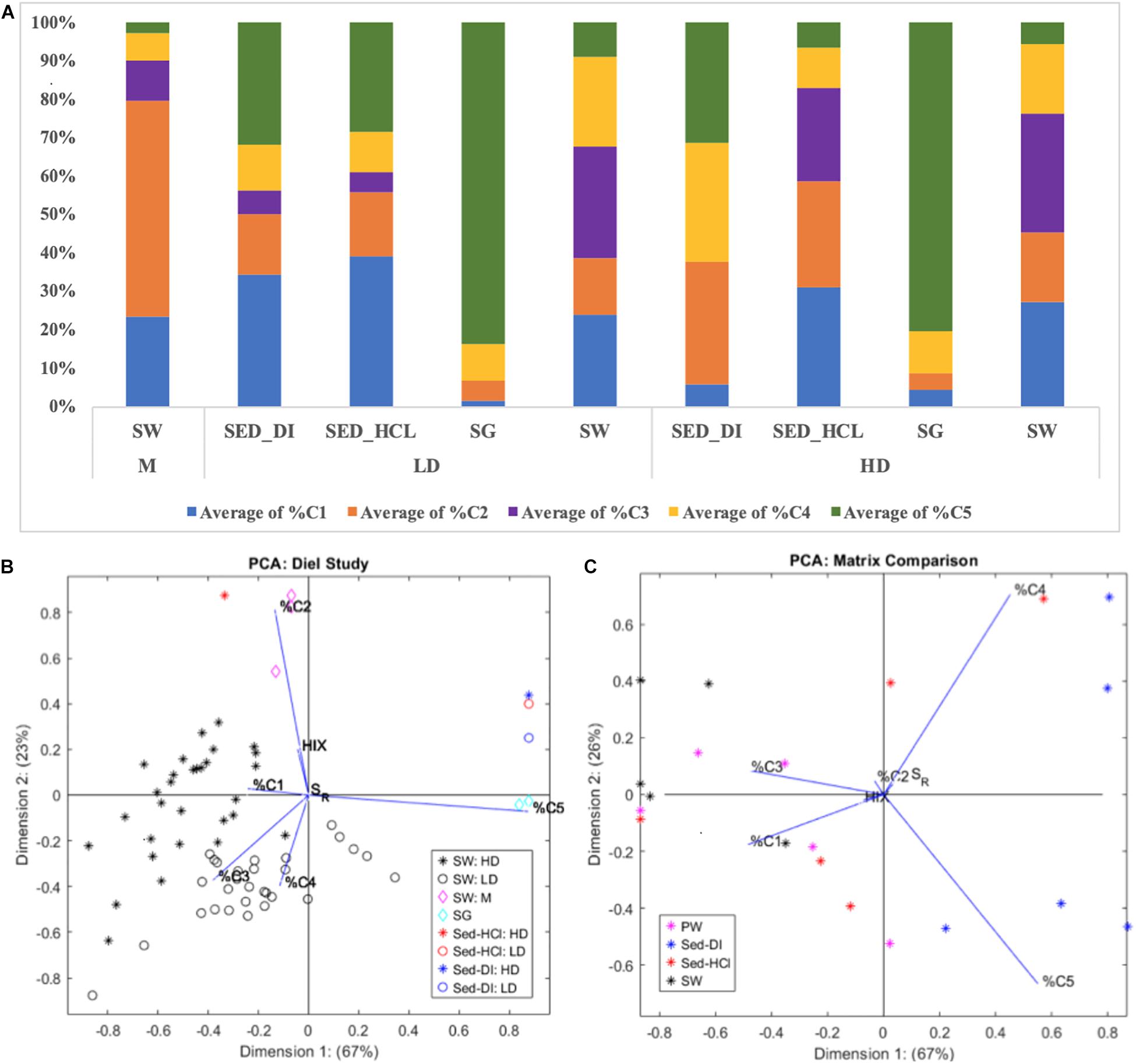
Figure 3. (A) PARAFAC component makeup of the different matrixes (SW, SG, SEDDI, and SEDHCl) from the HD and LD sites, compared to the nearby Mangrove (M) site. (B) PCA of the data shown in the top figure, from the diel portion of this study. (C) PCA of the data from the five additional sites throughout FB, separated by matrix (PW, SW, SEDDI, and SEDHCl).
The M site is situated between the HD and LD sites (Figure 1), allowing us to identify any presence of a “mangrove” source of FDOM. Surface waters at M were dominated by C2, and to a lesser extent C1 and C3, with only small amounts, if any, of C4 and C5 (Figure 3A). Conversely, C2 was only a minor component of HD and LD SW. While Chen and Jaffé (2014) did not explicitly consider mangroves in Florida Bay, they did collect surface waters from the mangrove-dominated section of Shark River Slough (SRS5 in their report), which is located north of FB on the western coast of south Florida. They found mangrove SW dominated by their components 1 and 5, and to a lesser extent, components 2, 3, 4, and 6, which are similar to our components C2, C1, and C3. Interestingly, their components 1, 3, 4, 5, and 6 were found to be much more photo-reactive than bio-reactive. Thus, the differences between SW collected from M, HD, and LD sites does not rule out mangroves as a possible source for surface water FDOM, as the optical signal could be modified due to photodegradation in clear FB waters. However, our diel study does not show a change in C1, C2, or C3 as a function of cumulative PAR (Figure 2).
Our third hypothetical source of FDOM was from the sediments. We conducted two sediment leachate treatments, one intended to minimize the impact of carbonate dissolution (SEDDI) and one with enhanced sediment dissolution (SEDHCl). Our SEDDI protocol is most comparable to the passive leaching with DOC-reduced site water demonstrated in Chen and Jaffé (2014). When considering the percent component makeup of these leachates, the HD SEDHCl is much more similar to HD SW than the HD SEDDI, while both of the sediment leachates at the LD site are more similar to LD SW than either M or LD SG (Figure 3A). Our PCA analysis demonstrates that %C5, and to a lesser extent %C2 and %C3, are most important in explaining the variability between the different pools. HD SEDHCl was more closely related to HD SW than HD SEDDI, however, due to the prevalence of the highly photolabile C5 (the main explainer variable of Dimension 1), the LD SEDHCl and LD SEDDI both clustered closer to LD SG than LD SW (Figure 3B). In the Chen and Jaffé (2014) study, mangrove SW was more similar to their Florida Bay SW than the neutrally leached sediment. This indicates that if sediment dissolution is not explicitly considered, the role of sediment dissolution or reprecipitation processes in contributing to DOM cycling in Florida Bay could be overlooked.
The leachate study suggests that seagrasses or mangroves are not necessarily the major direct sources of FDOM to overlying water. Instead, we highlight the process of sediment carbonate dissolution as a potential source of FDOM to surface waters. This sediment carbonate dissolution could be impacted by seagrasses, and we attempted to elucidate this connection by studying seagrass meadows of high and low density. However, rates of carbonate dissolution were similarly low between HD and LD sites during this study period (Van Dam et al., 2019), challenging our ability to distinguish its influence on DOM variability in space or time.
Sediment, Porewater, and Surface Water FDOM Throughout Florida Bay
To further investigate this apparent similarity between the FDOM of SW and SEDHCl, we collected samples from 5 additional sites (TC, DK, LM, BA, and SB; Figure 1), consisting of a single sample for each site and each pool (PW, SW, SEDDI, and SEDHCl). When considering carbonate dissolution as a potential source of organic matter, PW can be considered an intermediary pool between SEDHCl and SW. For this reason, it can be useful to compare SW to PW, as well as SEDHCl and SEDDI to PW. Across all sites, the FDOM and absorbance indices clustered around the 1:1 line, suggesting rapid exchange between PW and SW DOM pools. We observed deviations from the 1:1 line for %C5, which was much higher in PW than in SW for most sites, likely due to its photo-lability (Chen and Jaffé, 2014). SUVA254 tended to be higher in SW than PW, but differences in SW and PW SR were less clear (Figures 4A,D). Decreases in PW SUVA254 were potentially related to releases of labile but not chromophoric DOC from seagrasses in the rhizosphere.
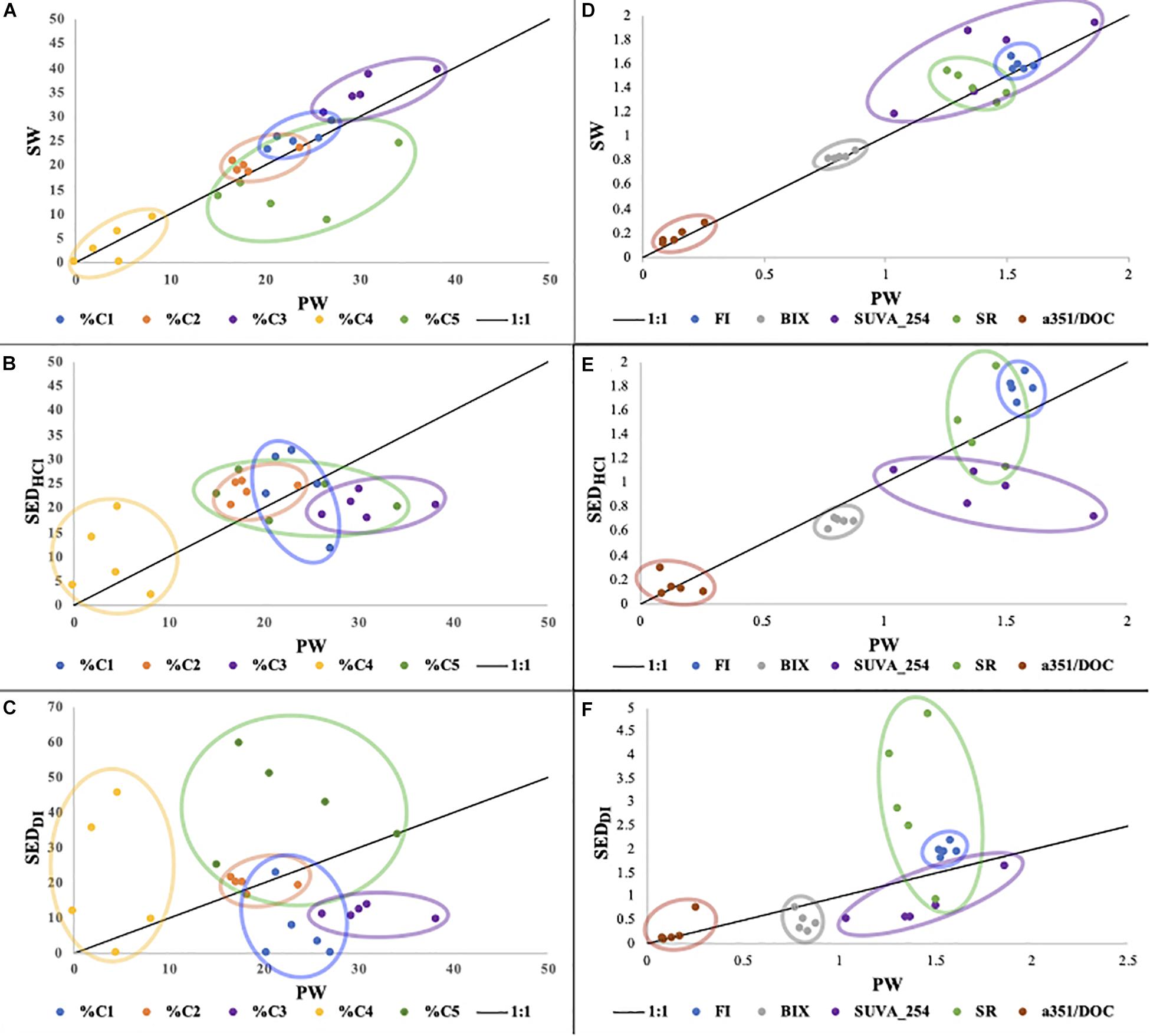
Figure 4. Comparison of PARAFAC component composition (A–C) and other optical properties (D–F) for the 5 Florida Bay sites (DK, SB, LM, BA, and TC) and 4 pools (SW, PW, SEDHCl, and SEDDI). PW is on the x-axis, with either SW (A,D), SEDHCl (B,E), or SEDDI (C,F) on the y-axis. One sample was used for each site and each pool (n = 20).
We also observed a strong relationship between SEDHCl leachates and PW (Figures 4B,E) for most components and indices, indicating a biogeochemical link between the DOM in PW and that associated with carbonate sediments. Deviations observed for %C3 and SUVA254, which behaved similarly due to overlapping spectral properties, and were higher in PW than SEDHCl, imply that C3 was less strongly associated with carbonates. In contrast, the SEDDI leachates appeared much less related to PW (Figures 4C,F). This is consistent with the prior leachate study in Florida Bay, which found that FB sediment leachates using non-acidified waters were very dissimilar to surface waters (Chen and Jaffé, 2014), and clearly contrasts with our SEDHCl leachates. Both their study, and our SEDDI leachates, attempted to minimize sediment dissolution, whereas our SEDHCl approach attempted to highlight sediment dissolution. The relationships among these pools can also be seen in our PCA analysis, which shows 4 of the SEDHCl clustering near the PW or SW along with the variables %C1 and %C3, whereas the SEDDI is more dissimilar and clusters nearer the variables %C5 and %C4 (Figure 3C). The SEDHCl leaching released large quantities of DOC relative to SEDDI, enough to increase the DOC concentration in the leachates by 145 to 260% across the 5 sites, illustrating the potential contribution of this pool in in situ seagrass meadows (Supplementary Table S2).
Concluding Remarks
The results of our unique HCl-leaching procedure indicate that carbonate sediment dissolution could be an important mechanism, linking sediment-associated organic matter to the broader dissolved organic matter cycle in carbonate seagrass systems like Florida Bay. We suggest that the exchange between SEDHCl and PW pools are facilitated by sediment dissolution and reprecipitation, and subsequently PW and SW pools mix upon periodic sediment resuspension events, bioturbation, or slow diffusive exchange. Although our diel study was not able to elucidate this laboratory derived relationship in the field, this study lays the groundwork for future investigations to measure the sediment-water FDOM flux alongside estimates of carbonate dissolution, to address the reversibility of the process, and investigate the alteration of DOM by its association with carbonates.
Data Availability Statement
The dataset generated for this study is available on Figshare (10.6084/m9.figshare.12611897.v2). PAR data used for this article is already published and is available at Figshare (10.6084/m9.figshare.7707029.v1). The PARAFAC model is available on OpenFluor (OpenFluor ID 2859).
Author Contributions
MZ wrote the manuscript, developed the PARAFAC model, and was instrumental in the sediment leachate study design. BV and CL created the diel study, were instrumental in field work, and provided detailed edits to the manuscript. JK was instrumental in the study design and also provided detailed edits and comments to the manuscript. All authors contributed to the article and approved the submitted version.
Funding
Funding for research was supported by a National Science Foundation grant to the Florida Coastal Everglades Long-Term Ecological Research Program (DEB-1237517). The publication of this article was funded by the Open Access Fund of the Leibniz Association. MZ is currently supported within the BMBF-project “DAM-MGF.”
Conflict of Interest
The authors declare that the research was conducted in the absence of any commercial or financial relationships that could be construed as a potential conflict of interest.
Acknowledgments
We thank Sarah Wilson for help during the sample collection and Matthew Smith for help in DOC analysis. This is contribution #221 from the Coastlines and Oceans Division of the Institute of Environment at Florida International University.
Supplementary Material
The Supplementary Material for this article can be found online at: https://www.frontiersin.org/articles/10.3389/fmars.2020.580284/full#supplementary-material
References
Barrón, C., Duarte, C. M., Frankignoulle, M., and Borges, A. V. (2006). Organic carbon metabolism and carbonate dynamics in a Mediterranean seagrass (Posidonia oceanica) meadow. Estuar. Coast. 29, 417–426. doi: 10.1007/BF02784990
Burdige, D. J., and Zimmerman, R. C. (2002). Impact of sea grass density on carbonate dissolution in Bahamian sediments. Limnol. Oceanogr. 47, 1751–1763. doi: 10.4319/lo.2002.47.6.1751
Burdige, D. J., Hu, X., and Zimmerman, R. C. (2010). The widespread occurrence of coupled carbonate dissolution/reprecipitation in surface sediments on the Bahamas Bank. Am. J. Sci. 310, 492–521. doi: 10.2475/06.2010.03
Burdige, D. J., Zimmerman, R. C., and Hu, X. (2008). Rates of carbonate dissolution in permeable sediments estimated from pore-water profiles: The role of sea grasses. Limnol. Oceanogr. 53, 549–565. doi: 10.4319/lo.2008.53.2.0549
Caccia, V. G., Millero, F. J., and Palanques, A. (2003). The distribution of trace metals in Florida Bay sediments. Mar. Pollut. Bull. 46, 1420–1433. doi: 10.1016/S0025-326X(03)00288-1
Carter, P. W. (1978). Adsorption of amino acid-containing organic matter by calcite and quartz. Geochim. Cosmochim. Acta 42, 1239–1242. doi: 10.1016/0016-7037(78)90117-5
Chen, M., and Jaffé, R. (2014). Photo- and bio-reactivity patterns of dissolved organic matter from biomass and soil leachates and surface waters in a subtropical wetland. Water Res. 61, 181–190. doi: 10.1016/j.watres.2014.03.075
Chen, M., and Jaffé, R. (2016). Quantitative assessment of photo- and bio-reactivity of chromophoric and fluorescent dissolved organic matter from biomass and soil leachates and from surface waters in a subtropical wetland. Biogeochemistry 129, 273–289. doi: 10.1007/s10533-016-0231-7
Chen, M., Price, R. M., Yamashita, Y., and Jaffé, R. (2010). Comparative study of dissolved organic matter from groundwater and surface water in the Florida coastal Everglades using multi-dimensional spectrofluorometry combined with multivariate statistics. Appl. Geochem. 25, 872–880. doi: 10.1016/j.apgeochem.2010.03.005
Cory, R. M., Mcknight, D. M., and Mcknight, D. M. (2005). Fluorescence Spectroscopy Reveals Ubiquitous Presence of Oxidized and Reduced Quinones in Dissolved Organic Matter Fluorescence Spectroscopy Reveals Ubiquitous Presence of Oxidized and Reduced Quinones in Dissolved Organic Matter. Environ. Sci. Technol. 39, 8142–8149. doi: 10.1021/es0506962
Egea, L. G., Barrón, C., Jiménez-Ramos, R., Hernández, I., Vergara, J. J., Pérez-Lloréns, J. L., et al. (2019). Coupling carbon metabolism and dissolved organic carbon fluxes in benthic and pelagic coastal communities. Estuar. Coast. Shelf Sci. 227:106336. doi: 10.1016/j.ecss.2019.106336
Eldridge, P. M., and Morse, J. W. (2000). A diagenetic model for sediment-seagrass interactions. Mar. Chem. 70, 89–103. doi: 10.1016/S0304-4203(00)00018-9
Fourqurean, J. W., and Robblee, M. B. (1999). Florida Bay: A history of recent ecological changes. Estuaries 22, 345–357. doi: 10.2307/1353203
Fourqurean, J. W., Duarte, C. M., Kennedy, H., Marbà, N., Holmer, M., Mateo, M. A., et al. (2012a). Seagrass ecosystems as a globally significant carbon stock. Nat. Geosci. 5:505. doi: 10.1038/NGEO1477
Fourqurean, J. W., Kendrick, G. A., Collins, L. S., Chambers, R. M., and Vanderklift, M. A. (2012b). Carbon, nitrogen and phosphorus storage in subtropical seagrass meadows: Examples from Florida Bay and Shark Bay. Mar. Freshwater Res. 63, 967–983. doi: 10.1071/MF12101
Grebel, J. E., Pignatello, J. J., Song, W., Cooper, W. J., and Mitch, W. A. (2009). Impact of halides on the photobleaching of dissolved organic matter. Mar. Chem. 115, 134–144. doi: 10.1016/j.marchem.2009.07.009
Haider, K. M., and Guggenberger, G. (2004). Organic Matter–Genesis and Formation. Encyc. Soils Environ. 4, 93–101. doi: 10.1016/B0-12-348530-4/00510-5
Helms, J. R., Kieber, D. J., Minor, E. C., Ritchie, J. D., Stubbins, A., and Mopper, K. (2008). Absorption spectral slopes and slope ratios as indicators of molecular weight, source, and photobleaching of chromophoric dissolved organic matter. Limnol. Oceanogr. 53, 955–969. doi: 10.4319/lo.2008.53.3.0955
Howard, J. L., Creed, J. C., Aguiar, M. V. P., and Fouqurean, J. W. (2018). CO 2 released by carbonate sediment production in some coastal areas may offset the benefits of seagrass “Blue Carbon” storage. Limnol. Oceanogr. 63, 160–172. doi: 10.1002/lno.10621
Ingalls, A. E., Aller, R. C., Lee, C., and Wakeham, S. G. (2004). Organic matter diagenesis in shallow water carbonate sediments. Geochim. Cosmochim. Acta 68, 4363–4379. doi: 10.1016/j.gca.2004.01.002
Jaffé, R. (2018). Monthly monitoring fluorescence data for Shark River Slough and Taylor Slough, Everglades National Park (FCE) for October 2004 to February 2014. Available online at: https://doi.org/10.6073/pasta/3938d3bb664d57584afc749c6a768f31 (accessed Oct 19, 2020).
Jaffé, R., Cawley, K. M., and Yamashita, Y. (2014). Applications of excitation emission matrix fluorescence with parallel factor analysis (EEM-PARAFAC) in assessing environmental dynamics of natural dissolved organic matter (DOM) in aquatic environments: A review. ACS Sympos. Ser. 1160, 27–73. doi: 10.1021/bk-2014-1160.ch003
Jensen, H. S., Nielsen, O. I., Koch, M. S., and De Vicente, I. (2009). Phosphorus release with carbonate dissolution coupled to sulfide oxidation in Florida Bay seagrass sediments. Limnol. Oceanogr. 54, 1753–1764. doi: 10.4319/lo.2009.54.5.1753
Jin, J., and Zimmerman, A. R. (2010). Abiotic interactions of natural dissolved organic matter and carbonate aquifer rock. Appl. Geochem. 25, 472–484. doi: 10.1016/j.apgeochem.2009.12.012
Kennedy, H., Beggins, J., Duarte, C. M., Fourqurean, J. W., Holmer, M., Marbá, N., et al. (2010). Seagrass sediments as a global carbon sink: Isotopic constraints. Glob. Biogeochem. Cyc. 24, 1–8. doi: 10.1029/2010GB003848
Koch, M. S., Benz, R. E., and Rudnick, D. T. (2001). Solid-phase phosphorus pools in highly organic carbonate sediments of northeastern Florida Bay. Estuar. Coast. Shelf Sci. 52, 279–291. doi: 10.1006/ecss.2000.0751
Ku, T. C. W., Walter, L. M., Coleman, M. L., Blake, R. E., and Martini, A. M. (1999). Coupling between sulfur recycling and syndepositional carbonate dissolution: Evidence from oxygen and sulfur isotope composition of pore water sulfate, South Florida Platform, U.S.A. Geochim. Cosmochim. Acta 63, 2529–2546. doi: 10.1016/S0016-7037(99)00115-5
Lee, K. S., and Dunton, K. H. (2000). Diurnal changes in pore water sulfide concentrations in the seagrass Thalassia testudinum beds: The effects of seagrasses on sulfide dynamics. J. Exp. Mar. Biol. Ecol. 255, 201–214. doi: 10.1016/S0022-0981(00)00300-2
Liu, S., Zhu, Y., Liu, L., He, Z., Giesy, J. P., Bai, Y., et al. (2018). Cation-induced coagulation of aquatic plant-derived dissolved organic matter: Investigation by EEM-PARAFAC and FT-IR spectroscopy. Environ. Pollut. 234, 726–734. doi: 10.1016/j.envpol.2017.11.076
Long, M. H., Mcglathery, K. J., Zieman, J. C., Berg, P., and Long, H. (2008). The role of organic acid exudates in liberating phosphorus carbonate sediments. Limnol.Oceanogr. 53, 2616–2626. doi: 10.4319/lo.2008.53.6.2616
Maher, D. T., and Eyre, B. D. (2010). Benthic fluxes of dissolved organic carbon in three temperate Australian estuaries: Implications for global estimates of benthic DOC fluxes. J. Geophys. Res. 115:G04039. doi: 10.1029/2010JG001433
Maie, N., Boyer, J. N., Yang, C., and Jaffé, R. (2006). Spatial, geomorphological, and seasonal variability of CDOM in estuaries of the Florida Coastal Everglades. Hydrobiologia 569, 135–150. doi: 10.1007/s10750-006-0128-x
Maie, N., Yamashita, Y., Cory, R. M., Boyer, J. N., and Jaffé, R. (2012). Application of excitation emission matrix fluorescence monitoring in the assessment of spatial and seasonal drivers of dissolved organic matter composition: Sources and physical disturbance controls. Appl. Geochem. 27, 917–929. doi: 10.1016/j.apgeochem.2011.12.021
Mazarrasa, I., Marbà, N., Lovelock, C. E., Serrano, O., Lavery, P. S., Fourqurean, J. W., et al. (2015). Seagrass meadows as a globally significant carbonate reservoir. Biogeosciences 12, 4993–5003. doi: 10.5194/bg-12-4993-2015
McKnight, D. M., Boyer, E. W., Westerhoff, P. K., Doran, P. T., Kulbe, T., and Andersen, D. T. (2001). Spectrofluorometric characterization of dissolved organic matter for indication of precursor organic material and aromaticity. Limnol. Oceanogr. 46, 38–48. doi: 10.4319/lo.2001.46.1.0038
Müller, P. J., and Suess, E. (1977). Interaction of organic compounds with calcium carbonate-III. Amino acid composition of sorbed layers. Geochim. Cosmochim. Acta 41, 941–949. doi: 10.1016/0016-7037(77)90153-3
Murphy, K. R., Stedmon, C. A., Graeber, D., and Bro, R. (2013). Fluorescence spectroscopy and multi-way techniques. PARAFAC. Analytic. Methods 5, 6557–6566. doi: 10.1039/c3ay41160e
Murphy, K. R., Stedmon, C. A., Wenig, P., and Bro, R. (2014). OpenFluor- an online spectral library of auto-fluorescence by organic compounds in the environment. Analytic. Methods 6, 658–661. doi: 10.1039/C3AY41935E
Parlanti, E., Wörz, K., Geoffroy, L., and Lamotte, M. (2000). Dissolved organic matter Fluorescence spectroscopy as a tool to estimate biological activity in a coastal zone submitted to anthropogenic inputs. Organ. Geochem. 31, 1765–1781. doi: 10.1016/S0146-6380(00)00124-8
Poulin, B. A., Ryan, J. N., and Aiken, G. R. (2014). Effects of iron on optical properties of dissolved organic matter. Environ. Sci. Technol. 48, 10098–10106. doi: 10.1021/es502670r
Serrano, O., Rozaimi, M., Lavery, P. S., and Smernik, R. J. (2020). Organic chemistry insights for the exceptional soil carbon storage of the seagrass Posidonia australis. Estuar. Coast. Shelf Sci. 237:106662. doi: 10.1016/j.ecss.2020.106662
Suess, E. (1970). Interaction of organic compounds with calcium carbonate-I. Association phenomena and geochemical implications. Geochim. Cosmochim. Acta 34, 157–168. doi: 10.1016/0016-7037(70)90003-7
Van Dam, B. R., Lopes, C., Osburn, C. L., and Fourqurean, J. W. (2019). Net heterotrophy and carbonate dissolution in two subtropical seagrass meadows. Biogeosciences 16, 4411–4428. doi: 10.5194/bg-16-4411-2019
Wagner, S., Jaffé, R., Cawley, K., Dittmar, T., and Stubbins, A. (2015). Associations Between the Molecular and Optical Properties of Dissolved Organic Matter in the Florida Everglades, a Model Coastal Wetland System. Front. Chem. 3:66. doi: 10.3389/fchem.2015.00066
Walter, L. M., Bischof, S. A., Patterson, W. P., and Lyons, T. W. (1993). Dissolution and recrystallization in modern shelf carbonates: evidence from pore water and solid phase chemistry. Philosop. Trans. 344, 27–36. doi: 10.1098/rsta.1993.0072
Weishaar, J. L., Aiken, G. R., Bergamaschi, B. A., Fram, M. S., Fujii, R., and Mopper, K. (2003). Evaluation of specific ultraviolet absorbance as an indicator of the chemical composition and reactivity of dissolved organic carbon. Environ. Sci. Technol. 37, 4702–4708. doi: 10.1021/es030360x
Wickland, K. P., Neff, J. C., and Aiken, G. R. (2007). Dissolved organic carbon in Alaskan boreal forest: Sources, chemical characteristics, and biodegradability. Ecosystems 10, 1323–1340. doi: 10.1007/s10021-007-9101-4
Xu, Y., Mead, R. N., and Jaffé, R. (2006). A molecular marker-based assessment of sedimentary organic matter sources and distributions in Florida Bay. Hydrobiologia 569, 179–192. doi: 10.1007/s10750-006-0131-2
Ya, C., Anderson, W., and Jaffé, R. (2015). Assessing dissolved organic matter dynamics and source strengths in a subtropical estuary: Application of stable carbon isotopes and optical properties. Continent. Shelf Res. 92, 98–107. doi: 10.1016/j.csr.2014.10.005
Yamashita, Y., and Jaffé, R. (2008). Characterizing the interactions between trace metals and dissolved organic matter using excitation-emission matrix and parallel factor analysis. Environ. Sci. Technol. 42, 7374–7379. doi: 10.1021/es801357h
Yates, K. K., and Halley, R. B. (2006). Diurnal variation in rates of calcification and carbonate sediment dissolution in Florida Bay. Estuar. Coast. 29, 24–39. doi: 10.1007/BF02784696
Yates, K. K., Dufore, C., Smiley, N., Jackson, C., and Halley, R. B. (2007). Diurnal variation of oxygen and carbonate system parameters in Tampa Bay and Florida Bay. Mar. Chem. 104, 110–124. doi: 10.1016/j.marchem.2006.12.008
Keywords: sediment dissolution, carbonate dissolution, organic matter, excitation-emission matrices, seagrass ecosystem
Citation: Zeller MA, Van Dam BR, Lopes C and Kominoski JS (2020) Carbonate-Associated Organic Matter Is a Detectable Dissolved Organic Matter Source in a Subtropical Seagrass Meadow. Front. Mar. Sci. 7:580284. doi: 10.3389/fmars.2020.580284
Received: 05 July 2020; Accepted: 16 October 2020;
Published: 12 November 2020.
Edited by:
Xinping Hu, Texas A&M University-Corpus Christi, United StatesReviewed by:
Laodong Guo, University of Wisconsin–Milwaukee, United StatesHussain A. Abdulla, Texas A&M University-Corpus Christi, United States
Copyright © 2020 Zeller, Van Dam, Lopes and Kominoski. This is an open-access article distributed under the terms of the Creative Commons Attribution License (CC BY). The use, distribution or reproduction in other forums is permitted, provided the original author(s) and the copyright owner(s) are credited and that the original publication in this journal is cited, in accordance with accepted academic practice. No use, distribution or reproduction is permitted which does not comply with these terms.
*Correspondence: Mary A. Zeller, maryazeller@gmail.com
†ORCID: Mary A. Zeller orcid.org/0000-0001-6952-4273; Bryce R. Van Dam, orcid.org/0000-0003-0876-1392; Christian Lopes, orcid.org/0000-0001-9568-9223; John S. Kominoski, orcid.org/0000-0002-0978-3326