- 1Commonwealth Scientific and Industrial Research Organisation, Oceans and Atmosphere, Hobart, TAS, Australia
- 2Institute for Marine and Antarctic Studies, University of Tasmania, Hobart, TAS, Australia
- 3Department of Chemical Engineering, Monash University, Clayton, VIC, Australia
- 4Centre for Marine Socioecology & The School of Social Sciences, University of Tasmania, Hobart, TAS, Australia
Interest in understanding the extent of plastic and specifically microplastic pollution has increased on a global scale. However, we still know relatively little about how much plastic pollution has found its way into the deeper areas of the world’s oceans. The extent of microplastic pollution in deep-sea sediments remains poorly quantified, but this knowledge is imperative for predicting the distribution and potential impacts of global plastic pollution. To address this knowledge gap, we quantified microplastics in deep-sea sediments from the Great Australian Bight using an adapted density separation and dye fluorescence technique. We analyzed sediment cores from six locations (1–6 cores each, n = 16 total samples) ranging in depth from 1,655 to 3,062 m and offshore distances ranging from 288 to 356 km from the Australian coastline. Microplastic counts ranged from 0 to 13.6 fragments per g dry sediment (mean 1.26 ± 0.68; n = 51). We found substantially higher microplastic counts than recorded in other analyses of deep-sea sediments. Overall, the number of microplastic fragments in the sediment increased as surface plastic counts increased, and as the seafloor slope angle increased. However, microplastic counts were highly variable, with heterogeneity between sediment cores from the same location greater than the variation across sampling sites. Based on our empirical data, we conservatively estimate 14 million tonnes of microplastic reside on the ocean floor.
Introduction
Plastic pollution of the world’s oceans is an internationally recognized environmental issue (UNEP, 2018). The extent of this pollution, and increasing understanding of its potential impacts, make it a matter of increasing public concern (Bonanno and Orlando-Bonaca, 2018). Millions of tonnes of plastic enter the marine environment annually, and quantities are expected to increase in coming years (Jambeck et al., 2015; Geyer et al., 2017; Lebreton and Andrady, 2019). Over time, plastic items in the marine environment can degrade or break down into smaller pieces predominantly through weathering and mechanical forces such as wave action and abrasion with sand (Thompson et al., 2004; Corcoran et al., 2009). Once a plastic item is between 5 mm and 1 μm, it is defined as microplastic (MP) (GESAMP, 2015). Due to their size, MPs are easily ingested by an extensive range of marine species from high to low trophic species (Wright et al., 2013) and can cause negative effects on organisms (Teuten et al., 2009; Galloway, 2015; Auta et al., 2017; Hermabessiere et al., 2017).
Research has shown that plastic pollution (including MPs) accumulate in seafloor sediments either directly by sinking through the water column or indirectly via currents and sediments transported down continental slopes (Clark et al., 2016). However, the extent of MP accumulation in deep-sea sediments is poorly understood due to numerous logistical factors. Namely, the ocean is deep and vast, and there are significant costs and operational challenges associated with obtaining samples from deep-sea environments. Regardless of these limitations, it is important to investigate MPs in the benthic environment as the deep seabed may be harboring a large amount of plastic pollution that is unaccounted for Woodall et al. (2014). To date, MPs have been found in deep-sea sediments in surveyed areas of all major oceans (e.g., Van Cauwenberghe et al., 2013; Bergmann et al., 2017; Zhang et al., 2020). Although all major oceans have been explored, the survey areas are small and scarce, and to date, there have been no studies of deep-sea sediments in Australian waters.
To fill this knowledge gap, we sampled deep-sea sediment from the Great Australian Bight (GAB), a large ocean area to the south of Australia that is home to a range of iconic marine species (CSIRO, 2018). The risk of plastic pollution on the seabed here via local land-based sources is extremely low due to the low population density in adjacent coastal areas (Hardesty et al., 2017). However, when considering the estimated movements of ocean surface particles on a global scale (UNEP, 2016), the GAB is not immune to land-based sourced of plastic pollution and may be receiving plastic pollution from as far away as Africa and Asia (Lebreton et al., 2012; van Sebille et al., 2015). Furthermore, ocean-based anthropogenic activity due to the GAB supporting Australia’s largest fishing industry (CSIRO, 2018) and mining exploration (O’Neil, 2003), sea-based sources of pollution are not unlikely. Despite the region’s ecological and economic importance, the GAB has only been previously surveyed in one marine plastics study, which focused on the ocean surface and found low levels of floating plastic pollution (Reisser et al., 2013).
In addition to investigating the quantity and distribution of seabed MPs in the region, we used oceanographic models to assess the potential origin sources of MP we observed. Finally, we extrapolated from our empirical data to provide a conservative global and mass of MPs on the seabed floor.
Materials and Methods
Sample Collection
Deep-sea sediments were collected from six locations in the GAB in March and April 2017 (Figure 1). Sample locations ranged from 288 to 356 km off the Australian coastline with ocean depths ranging from 1,655 to 3,062 m (Table 1). A remotely operated vehicle deployed from the REM Etive (Voyage RE2017_C01) was used to press polycarbonate sediment cores (500 mm long, 100 mm internal diameter) into the seafloor, with between one and six cores collected at each of the six survey sites (Table 1). Onboard the vessel, each core was subsampled using two to three polypropylene mini-cores (91 mm long, 29 mm wide), and one bulk sample was taken from the surface of each main core. In total, 35 mini-core subsamples and 16 bulk subsamples were collected, totaling 51 subsamples for analysis (Table 1). All subsamples were wrapped in aluminum foil and immediately frozen for storage. Sampling personnel wore cotton fiber overalls to minimize sampling contamination through air-borne plastic fibers from clothing.
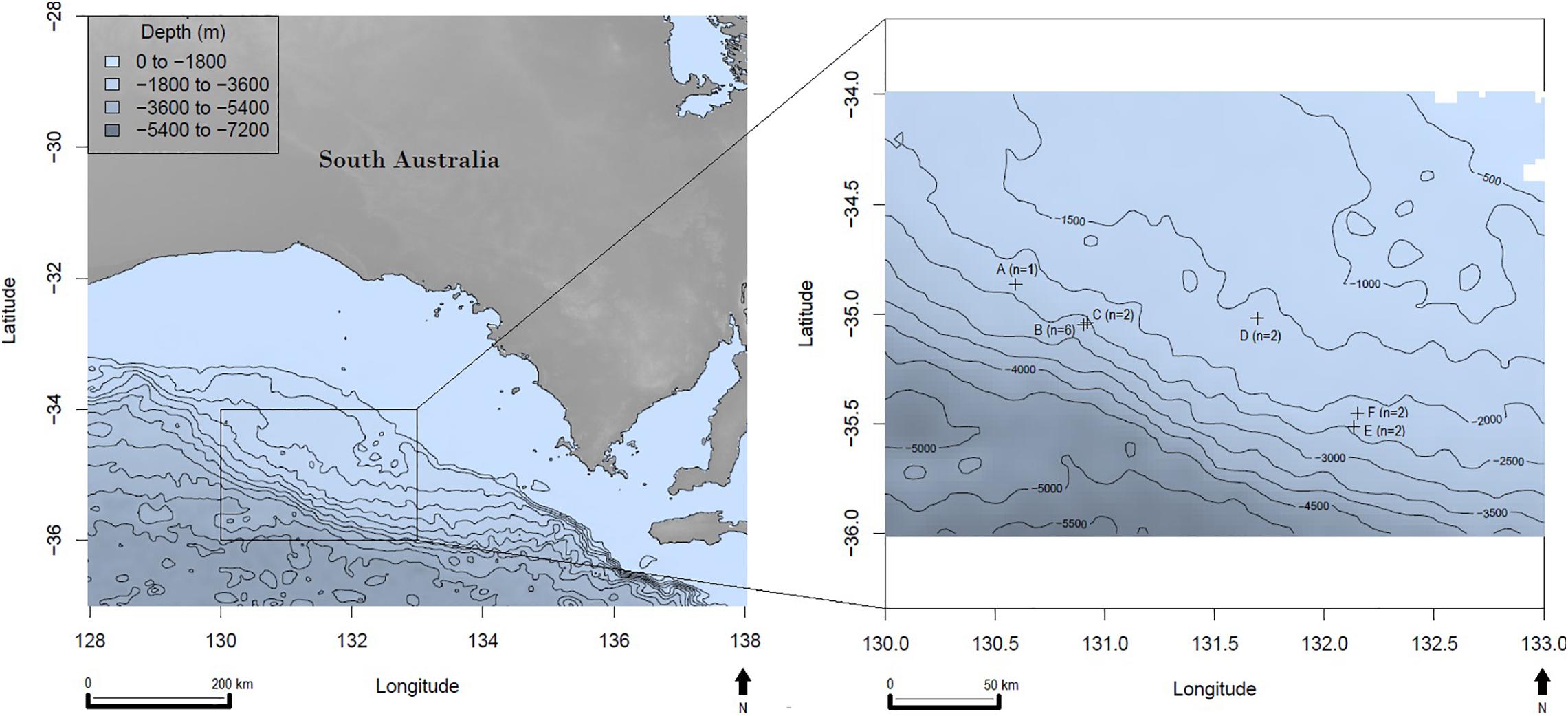
Figure 1. Great Australian Bight sampling site locations with bathymetry; n = the number of sediment cores collected per site, latitude, and longitude are in decimal degrees.
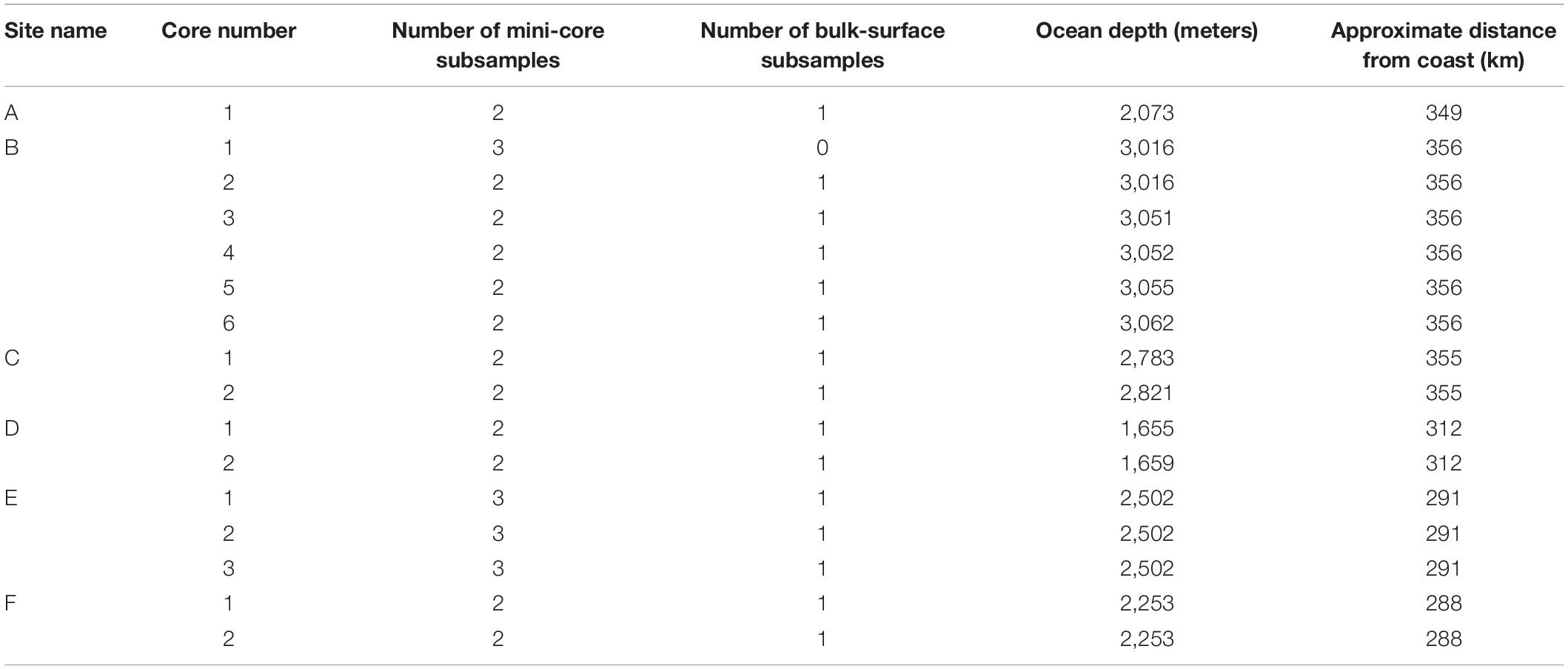
Table 1. Sediment core and subsample information, including ocean depth at the sample location and the distance from the nearest Australian coastline.
Laboratory Analysis
The laboratory analysis method was adapted from an MP fluorescence technique developed by Maes et al. (2017). Sediment subsamples were defrosted and homogenized with filtered deionized water. Each subsample was homogenized via gentile agitation. Approximately 15 g (about a third of each mini-core) of each homogenized subsample was added to 30 mL of zinc chloride (ZnCl2) solution (density of 1.37 g mL–1) and 400 μL of Nile Red dye solution (Nile Red 1 mg: n-propanol 1 mL) to give a final concentration of approximately 10 μg mL–1 Nile Red. Each subsample was treated individually in this way. These subsamples were agitated on an orbital shaker for 30 min and centrifuged at 1,800 × g for 5 min. Following centrifuging, the supernatant was vacuum filtered through a 0.22 μm mixed cellulose ester filter membrane. The sediment was rinsed with another 30 mL of ZnCl2 solution and centrifuged again at 1,800 × g for 5 min. The rinsing and centrifuging step was repeated a second time; hence, a total of three supernatant volumes were collected per sediment subsample. The supernatant from each subsample was then filtered through a single filter paper for microscopy analyses. The specific density of the ZnCl2 solution, combined with the centrifuging step, reduced the possibility of organic matter in the collected supernatant per subsample. This was an important step because organic matter is also known to take up Nile Red dye (Shim et al., 2016) and can potentially lead to false-positive results. A ratio of wet to dry sediment mass (air-dried while covered with pre-rinsed aluminum foil, with mass measured to four decimal places) was determined for each subsample and used to convert the number of MPs per wet sediment to a dry sediment result (per gram of dry sediment).
Quantification Analysis Using Fluorescence Microscopy
The MPs present on each subsample filter were counted using a Leica MZ16FA microscope viewed at 25 times magnification with a fluorescent light source and a GFP2 filter. Microscope camera settings: exposure 240.9 mS, gain 4.9×, saturation 0.25, and gamma 0.73. MPs were categorized as being fragments or fibers. We counted all MPs that were ≥50 μm in size (see section “Methodological Considerations” for more information about the size limitation applied). A qualitative verification analysis was performed to confirm if the fluorescing fragments were, in fact, plastic (see section Verification of Microplastics).
Prevention of Microplastic Contamination During Laboratory Analysis
Contamination is a major issue in MP laboratory-based work. Therefore, we took several measures to prevent environmental contamination from impacting our results. First, during sampling onboard the vessel, a cotton over suit was worn by all personnel involved, and the mini-core sampling tubes and wrapping foil were pre-rinsed in deionized water. In the laboratory, we pre-rinsed all equipment three times with deionized water prior to use, and glass equipment was used where possible. All solutions were filtered through 0.22 μm mixed cellulose ester membranes. The laboratory analyst wore dark-colored merino wool clothing with their hair tied back, and the laboratory floor, benchtops, and fume cabinet were cleaned daily prior to analysis using a natural fiber (bamboo) paper towel and water. Where possible, laboratory processes were carried out within a fume cabinet to minimize air-borne contamination. Finally, only essential people were in the laboratory during the sediment analysis to minimize the introduction of any clothing or air-borne fibers into the sediment samples. During method trials, wet-mount filter membranes were placed in the fume cabinet for the duration of the analysis. We observed zero MPs on the wet-mount filter membranes following adjustment for the MP counts from negative control samples. This meant that environmental MP contamination was unlikely to be influential on the sample results.
Method Verification
Laboratory Method Verification
The following steps were taken to enable the detection of any potential contamination in the laboratory analysis;
• The 51 sediment subsamples were randomly assigned a laboratory analysis order.
• Approximately every five subsamples (n = 10), a duplicate was taken from the same sediment subsample and analyzed the same as all other subsamples.
• Experimental replicates were included in the analyses as the subsamples from each main sediment core.
• Negative controls were processed approximately every eight samples (n = 6) using the same method as the subsamples (minus the sediment). Meaning the zinc chloride and Nile Red dye solution was added to an empty tube, centrifuged and filtered repeatably, then analyzed under microscopy as per the subsamples. The mean MP count observed in the negative controls was subtracted from each subsample MP count to account for environmental contamination.
• Positive controls were analyzed approximately every eight subsamples (n = 6) using known common polymers (PP, PVC, LDPE, HDPE, and PS). These polymers were cut into 2 mm × 2 mm squares and added to each positive control at a concentration of 20 pieces per 15 g sediment. The near-perfect square shape of the known plastic pieces meant they were unlikely to be mistaken for MPs already in the sediment. The mean MP count from the positive controls was used to calculate a recovery percentage, which was factored into the MP count for each sediment subsample.
• The laboratory analyst was not aware of the sample or control order when counting MPs.
Due to the negative control and recovery adjustments, the final MP count for each subsample was not always a positive number. In this instance, the MP count was reported as 0 (for example, if, after adjustments, an MP count was −1, it was reported as 0).
Verification of Microplastics
Following microscopy, the fluorescing MP fragments were analyzed with Optical photothermal infrared (O-PTIR) spectroscopy to verify them as being a polymer. O-PTIR spectroscopy is a novel method for qualitative analysis of MPs with a previous study indicating it may be a better technique for detecting environmental plastics (nano) than other IR techniques (Merzel et al., 2020). The O-PTIR was used with an mIRage IR microscope (Photothermal Spectroscopy Corp., Santa Barbara, CA, United States) equipped with a Quantum Cascade Laser (QCL) with a tunable range from 790 to 1890 cm–1, an Optical Parametric Oscillators (OPO) laser with a tunable range from 2700 to 3600 cm–1 and a continuous-wave probe laser at 532 nm with adjustable output powers.
Spectra and images were collected through a 40×, 0.78 NA, 8 mm-working-distance, all-reflective Cassegrain objective. The MP fragments samples were placed on a standard 75 mm × 25 mm microscope slide. O-PTIR was operated at 80% (QCL) or 16% (OPO) IR power, 5% probe power, and the system was purged with dry nitrogen to maintain a constant humidity at 0%. O-PTIR spectra were collected on MP surfaces with an effective spectral resolution of 2 cm–1 and co-averaged for 10–20 spectral scans. O-PTIR data were processed using PTIR studio software (Photothermal Spectroscopy Corp., Santa Barbara, CA, United States). Spectra were “deglitched” to remove laser intensity jumps arising around the transition wavelength between QCL laser stages. Savitzky-Golay smoothing (polynomial order 3, side points 6) was applied to the point spectra. The spectra obtained from each MP fragment analyzed were matched to the closest known spectra using SpectraBase (Jung et al., 2018; Bio-Rad Laboratories Inc, 2020). A minimum of five matching absorption bands were required for acceptable identification.
As our study was focused on the quantity of MPs rather than the plastic material of which they were comprised, only a portion of MPs underwent O-PTIR. Eight sediment subsamples were prepared for O-PTIR verification (at least one from each major sampled site location) with 24 fragments identified as MPs through the staining technique. A random subset of 8 (30%) of these fragments were analyzed (discussed in section “Verification of Microplastics”).
Statistical Analyses
We restricted statistical analyses to fragment counts as these were the data of highest quantity. We acknowledge that fiber counts were few in number and could have resulted from contamination (see section “Laboratory Method Considerations”). Hence, they were excluded from analyses. To investigate sampling and experimental bias, we looked for a correlation between results (MP fragment counts) and sediment sampling order, and laboratory analysis order using the respective coefficients of determination. Method verification was investigated by comparing results from the replicate samples (subsamples from the same sediment core) and the larger cores from the same sample site location using the statistics software R (R Core Team, 2017). We performed a Generalized Additive Model (GAM) between MP fragment counts of the replicate samples, and applying a Tweedie distribution, to look for a statistically significant relationship. Tweedie was selected as the best-suited distribution family because the experiment results (MPs) were in the form of count data where the mean and variance were not considered to be equal. The MP count variance was also investigated using a GAM where a fixed effect was used to test for significant differences in subsample types (mini-cores versus bulk subsamples), core identification, and site location.
We investigated the potential causes of MPs observed in the sediment by identifying natural site attributes and potential anthropogenic associations of relevance. The following factors were considered to have a potential causal effect on MP fragment counts in GAB sediments: ocean depth, the distance from the nearest coastal town with regard to town population [distance in km × (town population/total population of South Australia)], fishing effort (the number of fishing vessels adjacent to the sampling site in a given year), location (latitude and longitude), proximity to the nearest oil exploration site (anthropogenic activity investigating site suitability for an oil and gas well), shipping activity (the number of transit ships passing through the ocean area adjacent to the sampling site), surface plastic plume (the estimated amount of plastic floating on the ocean surface above the sediment sample sites), and seafloor slope (derived from detailed seafloor bathymetry information obtained during the sampling voyage). Although the list of explanatory variables included seems extensive, we wanted to explore which factors could potentially influence MP counts: local anthropogenic variables or greater environmental variables.
To investigate which combination of variables had the strongest effect on the MP fragment counts, we implemented a dredge function in the R statistical language (2017). This was done using the Akaike’s Information Criterion (AIC) to determine the variable effect compared to the null hypothesis (no variables having any effect on MP counts). To control for correlation among the explanatory variables, we removed from the model any two variables with a correlation >0.7 within the same model. This combination of variables was then analyzed against MP fragment counts using a GAM in R (2017), again with a Tweedie distribution. The best GAM provided the likelihood of the selected explanatory variables effect on MP counts in the sediments (Table 2). We then compared the effect size of the variables within the selected model by multiplying the regression coefficient for a variable by the median value of that variable in our data.
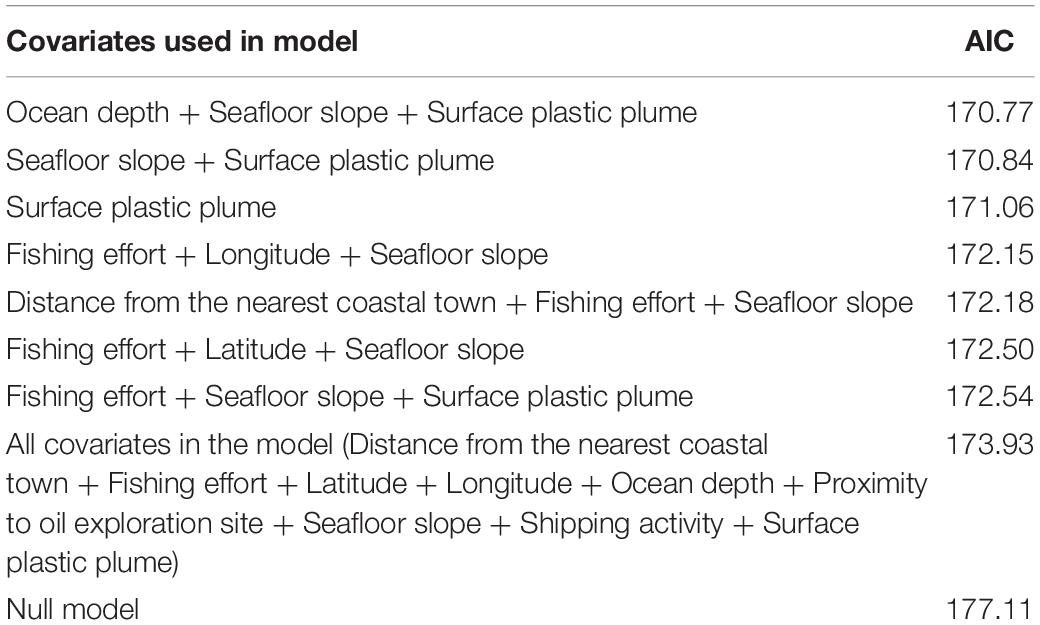
Table 2. The best fit GAM (based on the lowest AIC value) for investigating potential explanatory variable effects on MP counts.
Results
Method Verification
For analysis and verification of the robustness of the method we employed, we ran positive and negative controls (as outlined in the methods). The mean MP count in the negative control samples was 0.175 ± 0.046 fragments per gram of dry sediment. The mean MP recovery of positive controls was 71%. Sediment sampling order, laboratory sample processing order, and subsample type (mini-core or bulk) were found to have no influence on MP results. The duplicate samples had a mean count difference of 0.26 ± 0.052 fragments g–1 of dry sediment. A significant relationship was found between MP counts from the sample replicates (subsamples of each sediment core) (p-value < 0.05). All of the MP fragments that underwent O-PTIR analysis were confirmed as being MPs. Specifically, we observed cis-polyisoprene (n = 4), polyurethane (n = 2), polyester (n = 1), and polypropylene (n = 1) (Supplementary Material, Table S1 and Figures S1–S6).
Microplastic Counts
Based on the Nile Red staining, the MP counts from the 51 deep-sea samples analyzed ranged from 0 to 13.6 fragments (mean of 1.26 ± 0.68 fragments) g–1 dry sediment. The average MP counts for the six sediment locations were 0.97, 0.12, 0.17, 0.62, 2.90, and 0.10 fragment g–1 dry sediment (sites A to F, respectively, Figure 1). By comparison, fibers made up only 10% of all MPs detected in the sediments, thus fibers were excluded from the statistical analysis.
Sample Variability
The range of MP counts amongst cores was highly variable with the overall mean fragment variance being 13.1 MPs g–1 dry sediment. The standard error for MP fragment counts ranged from ±0 to ±4.5 g–1 dry sediment, with an overall mean standard error of ±0.68 fragments. Core B6 had the greatest variability of MPs ranging from 0 to 13.6 g–1 dry sediment among subsamples (Figure 2). The overall variance in MP counts at a site location level was not significantly different. However, a significant variance (p-value 0.002) was detected in results at a core level (cores from the same location).
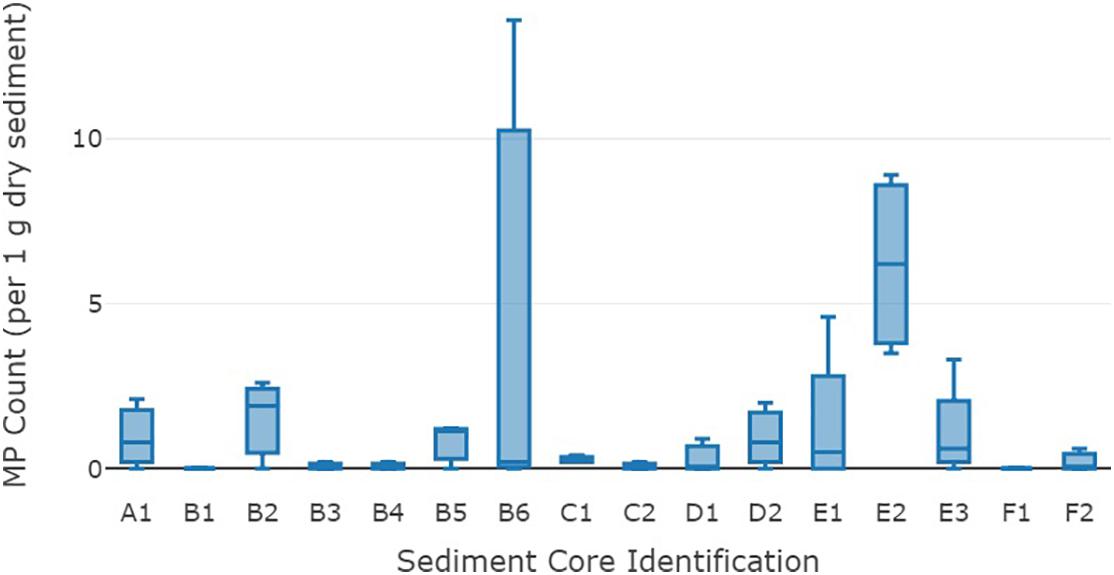
Figure 2. Mean microplastic fragment counts (g–1 dry sediment) from the 16 sediment cores from the deep-sea Great Australian Bight. The middle line on each box is the median, the lower and upper lines are the first and third quartiles, and the whiskers represent the minimum and maximum MP counts.
Spatial and Causal Association
Potential explanatory variables were added to a statistical model (GAM) in differing combinations and compared for best fit through the model AIC value. The explanatory variables present in the model with the lowest AIC and those within two units of the lowest AIC (Table 2) were the distance from the nearest coastal town, fishing effort, latitude, longitude, ocean depth, seafloor slope, and the surface plastic plume at the sediment sample locations.
The best fit model included ocean depth, seafloor slope, and surface plastic plume. These three variables in the model explained 23.3% of the deviance in MP fragment counts. When considering best fit model, a significant relationship was evident between MP counts and the variables surface plastic plume (p = 0.031) and seafloor slope (p = 0.002) (Table 3). MP counts increased as surface plastic plume increased, and as the angle of the seafloor slope increased. The median × coefficient was used to compare the effect of the explanatory variables on MP counts in the sediments. The surface plastic plume showed the greatest effect on MP counts in sediments.

Table 3. A summary of the best fit model (GAM) outputs for the explanatory variable effect on MP counts.
Discussion
Microplastic Fragments in Deep-Sea Sediments
The global ocean is expansive, covering more than 70% of the earth’s surface. Given its vastness, and the cost associated with sampling in remote areas, sampling of the seafloor is variable and typically limited to small areas. From our work, the average number of MPs from the GAB was 1.26 MPs g–1 dry sediment. While there are no other deep-sea sediment studies in Australia, two studies have investigated MP counts in coastal ocean sediment in southern Australia. In the Derwent Estuary in Tasmania, MP counts were 2–3 times higher than those we observed (2.43 and 4.2 fragments g–1 sediment; Willis et al., 2017). In another study, authors reported an average MP count of 3.4 mL–1 of sediment from 42 sites across south-eastern Australia (Ling et al., 2017). However, these sediments were from coastal environments, and the majority of their findings were filaments (Ling et al., 2017). Given the lack of contamination prevention procedures, their higher counts may be in part due to contamination issues. However, since both these studies are from coastal areas with higher local population densities, it is not surprising they reported substantially higher numbers of MPs.
While low compared to coastal sediment studies in Australia, the MP counts from our study are high compared to other deep-sea sediment analyses globally (Table 4). Four other studies of MPs in deep-sea sediment reported MP counts similar to ours including samples from the Arctic (Bergmann et al., 2017), the Mediterranean (Woodall et al., 2014; Kane et al., 2020), the North Atlantic (Woodall et al., 2014), and the Western Pacific (Zhang et al., 2020; Table 4). Even in these instances, however, the MP counts we observed were more than double those reported in other studies, in spite of our careful measures to exclude contamination and not including fibers.
Scaling up our empirical data to the global approximation, we estimate that there could be as much as 14.4 million tonnes of MP in the top 9 cm of sediment throughout the world’s ocean (9 cm being the depth of our sediment cores and subsequent results used for this estimation). Based on the MP count of global deep-sea sediment studies (0.72 MP g–1 dry sediment; Table 4) we estimate a mass of 8.4 million tonnes of MPs [determined by first calculating the average MP count per wet sediment volume, then multiplying by the average density of MP (1.099 g per cm3; Kooi and Koelmans, 2019), per 100 μm (the average MP size we observed), and finally scaling up to a global level based on the ocean size estimate of 361,132,000 km2 (Pidwirny, 2006)]. Overall, given the much higher density of MPs reported in sediment in coastal areas, both global estimates are extremely conservative. The estimated global mass we calculated suggests that the bottom of the ocean has between 34 and 57 times the standing stock of plastic at the surface (based on the surface estimate of 250,000 tonnes; Eriksen et al., 2014). However the estimated mass is only a fraction of the annual input from coastal pollution (between 1 and 1.7 times) based on 8.5 million tonnes per year (Jambeck et al., 2015).
Variability in Microplastics
Interestingly, the variability in MPs was much higher within cores at the same site (merely meters away) than we observed from sediment sampled hundreds of kilometers away. It is likely that this difference would be highlighted further if a greater number of samples were collected and analyzed. The high variance at a core level points to the high heterogeneity of MP deposition on the seabed and the likely influence of oceanographic conditions at a very fine scale. The small size and variable density of MPs suggest these small particles are easily transported via ocean currents (Lusher, 2015; Kane et al., 2020), climate and weather changes (Welden and Lusher, 2017), fish and animal behavior (Davidson, 2012; Cózar et al., 2014), biofouling (Ye and Andrady, 1991; Fazey and Ryan, 2016), and buoyant rise velocity (Reisser et al., 2013).
Additional implications of this variability suggest that the MP counts in one sediment location are unlikely to be a reliable predictor of MP counts nearby. We suggest that MP sediment studies without replication at all levels (sample, site, and region) should be treated with great caution. This is particularly important when estimating global hotspots for MP deposition.
Explanatory Factors Associated With Microplastic Deposition
While the high variance made the detection of spatial and causal associations challenging, we found surface plastic plume and seafloor slope angle were both significantly associated with MP density. As plastic floating on the surface near the sampling site increased, so too did the MP counts in the seabed sediments. We also found that where the seafloor slope angle increased, there was a corresponding increase in the number of MPs in the sediment. Due to logistical difficulties, no samples were collected from the bottom of a steep slope, where it might be expected that MP fragments would accumulate even more.
Other studies have predicted the movement of surface particles (including plastic pollution) to water south of Australia from as far as Asia and Africa from a western direction (Lebreton et al., 2012; van Sebille et al., 2015; UNEP, 2016). To investigate a potential relationship between ocean currents and MP counts in our study, the explanatory factor of site location longitude was used because the main currents in the GAB (both local and global) move in a longitudinal manner (Middleton and Bye, 2007). If plastic pollution driven by current had a significant impact on MP counts in deep-sea sediments from the GAB, then a statistical relationship would have been observed between MP counts and longitude. However, no association was detected, suggesting there was no significant relationship between current driven plastic pollution and sediment MP density. The GAB is a tumultuous body of water where current directions and circulation patterns vary seasonably (Middleton and Bye, 2007; Middleton et al., 2017). These oceanographic events are likely to contribute to the highly variable MP counts in GAB sediments.
Methodological Considerations
Laboratory Method Considerations
Upon analysis of the 51 sediment subsamples, it was clear that fibers were many fewer in number compared to fragments. No correlation was detected between MP fiber and fragment counts (r2 = 0.0002) within the sediment subsamples, and the variation between fibers (in total) was only a tenth of the variation among fragments. Thus we took the conservative perspective that MP fibers resulted from contamination (sensu Wesch et al., 2017; Willis et al., 2017), and we did not include them in further analysis.
We verified our method based on the presence of an association between MP results in sediment subsamples, the low difference in the number of fragments between duplicates, as well as the absence of an effect of MP counts and sampling order and laboratory processing order. In addition, no statistically significant difference was detected in MP counts between subsample types (mini-core and bulk). However, there were still two methodological issues to consider.
Firstly, the mean result of 71% of plastic fragments and fibers recovered in the positive experimental controls was much lower than expected given that laboratory equipment was triple rinsed with deionized water where possible throughout the method, thus creating ample opportunity for MPs to be washed onto the membrane filter for microscopic observation. One reason for the low recovery may have been due to the low-density nature of many MPs (Chubarenko et al., 2016), including those used in the positive control. The low density of MPs means they were likely subject to water surface tension tendencies, causing them to remain fixed to the surface of laboratory equipment rather than being carried onto membrane filters for microscopic analysis. To increase the recovery of MPs from sediment in future analyses, a drop of detergent could be added to laboratory solutions and equipment rinsing water to reduce the presence of water surface tension bonds (sensu Mani et al., 2015).
The second consideration is that of size reporting. In our study, we only reported on MPs ≥50 μm (and ≤5 mm). Implementing a size limitation in MP studies is not unusual (e.g., Kanhai et al., 2019), and in our case was applied due to the presence of fragments of approximately 5–10 μm in size, which could not be reliably counted. Furthermore, these fragments were also too small to undergo qualitative analysis for confirmation of material type. Given our focus on data quality, we opted for a conservative, consistent size range where we could have high confidence. We also thought it possible that the smaller fragments were organic false-positive fragments because they increased substantially when the laboratory analyst wore white cotton overalls. To explore the potential false-positive fragments further, atmospheric particulate matter (0.3–10 μm) was monitored. The monitoring revealed that the laboratory space showed high levels of atmospheric particulates (e.g., maximum of 47.7 × 103 per m3 particles of 5 μm in size) (3.3–5.9 × 106 per m3) compared to a laboratory space that is under strict cleanliness and quarantine regulations (e.g., an accredited ISO Class 4 laboratory space has 0 particles of 5 μm in size) (International Organization for Standardization [ISO], 2015). Although many of these atmospheric particulates were not likely MPs, air monitoring suggested that efforts could be made to increase the cleanliness of the laboratory environment, for example, using a laminar flow workspace (Wesch et al., 2017). Anecdotally, we observed that changing the laboratory analyst’s attire to dark-colored merino wool fiber clothing reduced the number of suspected false-positive fragments. Setting a fragment size limit well above the size of the suspected false-positive fragments and as per other studies (e.g., Frias et al., 2018) meant that smaller and suspected false-positive fragments were not counted and had no impact on the overall results and subsequent interpretation of our findings.
Verification of Microplastics
We used O-PTIR analysis to verify the Nile Red staining technique with eight samples. Because the instrument was located at a laboratory in another state, and given that fluorescence fades with time, only a third of the samples intended for verification were still fluorescing at the time of O-PTIR analysis. An obvious remedy is to undertake all analyses at the same facility, if possible.
Even with the small proportion of fragments analyzed, the O-PTIR verification step gave confidence that the fragments identified as MPs through the Nile Red staining technique were indeed MPs: 100% of the O-PTIR analyzed fragments were confirmed as plastic. The four different plastic polymers identified [cis-polyisoprene (rubber/latex), polyurethane, polyester, and polypropylene] are all used in and may originate from anthropogenic activities. However, cis-polyisoprene also naturally occurs in a variety of ocean plants (Broadgate et al., 2004). At this time, we cannot definitively identify the cis-polyisoprene source. However, it’s unlikely that the cis-polyisoprene came from natural sources given the depth of the sediment studied and the absence of plant material in any of the sediment cores. In the future, further research to investigate the polymer types of each of the MPs found in the sediment would be greatly beneficial to provide a clearer picture of potential plastic origins.
Conclusion
The presence of MPs in sediment from each of the deep-sea locations in the GAB has highlighted both the ubiquity and the heterogeneity of MPs in the marine environment. We found that the distribution of MPs at a deep-sea level correlated with surface plastic plume and seafloor slope (at least in the absence of deeper sinks such as submarine canyons which are known to have higher MP densities (Mordecai et al., 2011; Pham et al., 2014). This suggests that plastic fragments floating in the ocean surface layers may, in fact, settle to the bottom, making the benthic sediments a sink for this material. However, our estimated 14.4 million tonnes of MPs in deep-sea sediment does not account for the estimated 8 million tonnes of plastic lost from the world’s coast annually (Jambeck et al., 2015). In spite of claims that the seabed floor is a major “sink” (Woodall et al., 2014; Koelmans et al., 2017; Chiba et al., 2018) our results suggest that while MPs were numerous (14 million tonnes), sediments account for but a minuscule proportion of the ocean’s “missing plastic” (Thompson et al., 2004).
Data Availability Statement
The raw data supporting the conclusions of this article will be made available by the authors, without undue reservation.
Author Contributions
JB conducted the laboratory analysis, the statistical analyses, and wrote the manuscript. BH, CW, and ZC co-supervised the project and assisted with various methodological, statistical, and writing aspects of the project and associated manuscript. KW assisted with method development, statistical analyses, and editing. MH and JZ conducted the spectral analysis on MP fragments. AW secured funding, carried out ship-based research, collected sediment samples, and contributed to editing. All authors contributed to the article and approved the submitted version.
Conflict of Interest
The authors declare that the research was conducted in the absence of any commercial or financial relationships that could be construed as a potential conflict of interest.
Acknowledgments
We wish to thank the CSIRO Oceans and Atmosphere and the Great Australian Bight Deepwater Marine Program (GABDMP) for funding this project. The GABDMP is a CSIRO-led research program sponsored by Chevron Australia, with data generated to be made publicly available. We thank Andy Ross for providing the opportunity and then engineering the ROV sampling program so that these extra samples could be taken. We also thank several seagoing staff from CSIRO, notably Mark Green, for collecting the samples. We also thank Qamar Schuyler for her assistance with statistical analysis and Robert Walch from Walch Optics for his assistance with microscopy matters. Finally, we thank T. J. Lawson, Peter Thompson, Pascal Craw, and Andrew Revill for the loan of laboratory equipment to undertake this project.
Supplementary Material
The Supplementary Material for this article can be found online at: https://www.frontiersin.org/articles/10.3389/fmars.2020.576170/full#supplementary-material
References
Auta, H. S., Emenike, C. U., and Fauziah, S. H. (2017). Distribution and importance of microplastics in the marine environment: a review of the sources, fate, effects, and potential solutions. Environ. Int. 102, 165–176. doi: 10.1016/j.envint.2017.02.013
Bergmann, M., Wirzberger, V., Krumpen, T., Lorenz, C., Primpke, S., Tekman, M. B., et al. (2017). High quantities of microplastic in Arctic deep-sea sediments from the Hausgarten observatory. Environ. Sci. Technol. 51, 11000–11010. doi: 10.1021/acs.est.7b03331
Bio-Rad Laboratories Inc (2020). SpectraBase [Online]. Available online at: http://spectrabase.com/ (accessed April 15, 2020).
Bonanno, G., and Orlando-Bonaca, M. (2018). Ten inconvenient questions about plastics in the sea. Environ. Sci. Policy 85, 146–154. doi: 10.1016/j.envsci.2018.04.005
Broadgate, W. J., Malin, G., Küpper, F. C., Thompson, A., and Liss, P. S. (2004). Isoprene and other non-methane hydrocarbons from seaweeds: a source of reactive hydrocarbons to the atmosphere. Mar. Chem. 88, 61–73. doi: 10.1016/j.marchem.2004.03.002
Chiba, S., Saito, H., Fletcher, R., Yogi, T., Kayo, M., Miyagi, S., et al. (2018). Human footprint in the abyss: 30 year records of deep-sea plastic debris. Mar. Policy 96, 204–212. doi: 10.1016/j.marpol.2018.03.022
Chubarenko, I., Bagaev, A., Zobkov, M., and Esiukova, E. (2016). On some physical and dynamical properties of microplastic particles in the marine environment. Mar Pollut. Bull. 108, 105–112. doi: 10.1016/j.marpolbul.2016.04.048
Clark, J. R., Cole, M., Lindeque, P. K., Fileman, E., Blackford, J., Lewis, C., et al. (2016). Marine microplastic debris: a targeted plan for understanding and quantifying interactions with marine life. Front. Ecol. Environ. 14:317–324. doi: 10.1002/fee.1297
Corcoran, P. L., Biesinger, M. C., and Grifi, M. (2009). Plastics and beaches: a degrading relationship. Mar. Pollut. Bull. 58, 80–84. doi: 10.1016/j.marpolbul.2008.08.022
Cordova, M., and Wahyudi, A. (2016). Microplastic in the deap-sea sediment of southwestern Sumatran waters. Mar. Res. Indones. 41, 27–36. doi: 10.14203/mri.v41i1.99
Courtene-Jones, W., Quinn, B., Ewins, C., Gary, S. F., and Narayanaswamy, B. E. (2020). Microplastic accumulation in deep-sea sediments from the Rockall Trough. Mar. Pollut. Bull. 154:111092. doi: 10.1016/j.marpolbul.2020.111092
Cózar, A., Echevarría, F., González-Gordillo, J. I., Irigoien, X., Úbeda, B., Hernández-León, S., et al. (2014). Plastic debris in the open ocean. Proc. Natl. Acad. Sci. U.S.A. 111, 10239–10244. doi: 10.1073/pnas.1314705111
CSIRO (2018). Understanding the Great Australian Bight [Online]. Available online at: https://www.csiro.au/en/Research/OandA/Areas/Marine-resources-and-industries/Great-Australian-Bight (accessed July 12, 2018).
Davidson, T. M. (2012). Boring crustaceans damage polystyrene floats under docks polluting marine waters with microplastic. Mar. Pollut. Bull. 64, 1821–1828. doi: 10.1016/j.marpolbul.2012.06.005
Eriksen, M., Lebreton, L. C. M., Carson, H. S., Thiel, M., Moore, C. J., Borerro, J. C., et al. (2014). Plastic pollution in the world’s oceans: more than 5 trillion plastic pieces weighing over 250,000 tons afloat at sea. PLoS One 9:e111913. doi: 10.1371/journal.pone.0111913
Fazey, F. M. C., and Ryan, P. G. (2016). Biofouling on buoyant marine plastics: An experimental study into the effect of size on surface longevity. Environ. Pollut. 210, 354–360. doi: 10.1016/j.envpol.2016.01.026
Fischer, V., Elsner, N. O., Brenke, N., Schwabe, E., and Brandt, A. (2015). Plastic pollution of the Kuril–Kamchatka Trench area (NW pacific). Deep Sea Res. II Top. Stud. Oceanogr. 111(Suppl. C), 399–405. doi: 10.1016/j.dsr2.2014.08.012
Frias, J., Pagter, E., Nash, R., O’Connor, I., Carretero, O., Filgueiras, A., et al. (2018). Standardised Protocol for Monitoring Microplastics in Sediments. Deliverable 4.2. Brussels: JPI-Oceans BASEMAN Project, 24. doi: 10.25607/OBP-723
Galloway, T. S. (2015). “Micro- and nano-plastics and human health,” in Marine Anthropogenic Litter, eds M. Bergmann, L. Gutow, and M. Klages (Cham: Springer), 343–366. doi: 10.1007/978-3-319-16510-3_13
GESAMP (2015). Sources, Fate and Effects of Microplastics in the Marine Environment: a Global Assessment. IMO/FAO/UNESCO-IOC/UNIDO/WMO/IAEA/UN/UNEP/UNDP Joint Group of Experts on the Scientific Aspects of Marine Environmental Protection. London: International Maritime Organization (IMO). doi: 10.1007/978-3-319-16510-3_13
Geyer, R., Jambeck, J. R., and Law, K. L. (2017). Production, use, and fate of all plastics ever made. Sci. Adv. 3:e1700782. doi: 10.1126/sciadv.1700782
Hardesty, B. D., Lawson, T. J., van der Velde, T., Lansdell, M., and Wilcox, C. (2017). Estimating quantities and sources of marine debris at a continental scale. Front. Ecol. Environ. 15:18–25. doi: 10.1002/fee.1447
Hermabessiere, L., Dehaut, A., Paul-Pont, I., Lacroix, C., Jezequel, R., Soudant, P., et al. (2017). Occurrence and effects of plastic additives on marine environments and organisms: a review. Chemosphere 182, 781–793. doi: 10.1016/j.chemosphere.2017.05.096
International Organization for Standardization [ISO] (2015). Cleanrooms, and Associated Controlled Environments - Part 1: Classification of Air Cleanliness by Particle Concentration (ISO 14644-1:2015). Geneva: International Organization for Standardization [ISO].
Jambeck, J. R., Perryman, M., Geyer, R., Wilcox, C., Siegler, T. R., Andrady, A., et al. (2015). Plastic waste inputs from land into the ocean. Science 347, 768–771. doi: 10.1126/science.1260352
Jung, M. R., Horgen, F. D., Orski, S. V., Rodriguez, C. V., Beers, K. L., Balazs, G. H., et al. (2018). Validation of ATR FT-IR to identify polymers of plastic marine debris, including those ingested by marine organisms. Mar. Pollut. Bull. 127, 704–716. doi: 10.1016/j.marpolbul.2017.12.061
Kane, I. A., Clare, M. A., Miramontes, E., Wogelius, R., Rothwell, J. J., Garreau, P., et al. (2020). Seafloor microplastic hotspots controlled by deep-sea circulation. Science 368:1140. doi: 10.1126/science.aba5899
Kanhai, L. D. K., Johansson, C., Frias, J. P. G. L., Gardfeldt, K., Thompson, R. C., and O’Connor, I. (2019). Deep sea sediments of the Arctic Central Basin: a potential sink for microplastics. Deep Sea Res. Part I Oceanogr. Res. Pap. 145, 137–142. doi: 10.1016/j.dsr.2019.03.003
Koelmans, A. A., Kooi, M., Law, K. L., and van Sebille, E. (2017). All is not lost: deriving a top-down mass budget of plastic at sea. Environ. Res. Lett. 12:114028. doi: 10.1088/1748-9326/aa9500
Kooi, M., and Koelmans, A. A. (2019). Simplifying microplastic via continuous probability distributions for size, shape, and density. Environ. Sci. Technol. Lett. 6, 551–557. doi: 10.1021/acs.estlett.9b00379
Lebreton, L., and Andrady, A. (2019). Future scenarios of global plastic waste generation and disposal. Palgrave Commun. 5:6. doi: 10.1057/s41599-018-0212-7
Lebreton, L. C. M., Greer, S. D., and Borrero, J. C. (2012). Numerical modelling of floating debris in the world’s oceans. Mar. Pollut. Bull. 64, 653–661. doi: 10.1016/j.marpolbul.2011.10.027
Ling, S. D., Sinclair, M., Levi, C. J., Reeves, S. E., and Edgar, G. J. (2017). Ubiquity of microplastics in coastal seafloor sediments. Mar Pollut. Bull. 121, 104–110. doi: 10.1016/j.marpolbul.2017.05.038
Lusher, A. (2015). “Microplastics in the marine environment: distribution, interactions and effects,” in Marine Anthropogenic Litter, eds M. Bergmann, L. Gutow, and M. Klages (Cham: Springer International Publishing), 245–307. doi: 10.1007/978-3-319-16510-3_10
Maes, T., Jessop, R., Mayes, A. G., Wellner, N., and Haupt, K. (2017). A rapid-screening approach to detect and quantify microplastics based on fluorescent tagging with Nile Red. Sci. Rep. 7:44501. doi: 10.1038/srep44501
Mani, T., Hauk, A., Walter, U., and Burkhardt-Holm, P. (2015). Microplastics profile along the Rhine River. Sci. Rep. 5:17988. doi: 10.1038/srep17988
Martin, J., Lusher, A., Thompson, R. C., and Morley, A. (2017). The Deposition and accumulation of microplastics in marine sediments and bottom water from the Irish continental shelf. Sci. Rep. 7:10772. doi: 10.1038/s41598-017-11079-2
Merzel, R. L., Purser, L., Soucy, T. L., Olszewski, M., Colón-Bernal, I., Duhaime, M., et al. (2020). Uptake and retention of nanoplastics in quagga mussels. Glob. Chall. 4:1800104. doi: 10.1002/gch2.201800104
Middleton, J. F., and Bye, J. A. T. (2007). Review: a review of the shelf-slope circulation along Australia’s southern shelves: Cape Leeuwin to Portland. Progr. Oceanogr. 75, 1–41. doi: 10.1016/j.pocean.2007.07.001
Middleton, J. F., Griffin, D., Luick, J., Herzfeld, M., Hemer, M., James, C., et al. (2017). Theme 1: Physical Oceanography of the Great Australian Bight. GABRP Research Report Series Number 31. Bedford Park, SA: Flinders University.
Mordecai, G., Tyler, P. A., Masson, D. G., and Huvenne, V. A. I. (2011). Litter in submarine canyons off the west coast of Portugal. Deep Sea Res. Part II Top. Stud. Oceanogr. 58, 2489–2496. doi: 10.1016/j.dsr2.2011.08.009
O’Neil, B. J. (2003). “History of petroleum exploration,” in Bight Basin, eds G. W. O’Brien, E. Paraschiviou, and J. E. Hibbert (Adelaide, SA: Government of South Australia).
Peng, G., Bellerby, R., Zhang, F., Sun, X., and Li, D. (2020). The ocean’s ultimate trashcan: Hadal trenches as major depositories for plastic pollution. Water Research 168, 115121. doi: 10.1016/j.watres.2019.115121
Pham, C. K., Ramirez-Llodra, E., Alt, C. H. S., Amaro, T., Bergmann, M., Canals, M., et al. (2014). Marine litter distribution and density in European seas, from the shelves to deep basins. PLoS One 9:e95839. doi: 10.1371/journal.pone.0095839
Pidwirny, M. (2006). Introduction to the Oceans. [Online]. Available: http://www.physicalgeography.net/fundamentals/8o.html (accessed August 26, 2020).
R Core Team (2017). R: A Language and Environment for Statistical Computing, 3.4.0 Edn. Vienna: R Foundation for Statistical Computing.
Reisser, J., Thums, M., Pattiaratchi, C., Wilcox, C., Hardesty, B. D., Shaw, J., et al. (2013). Marine plastic pollution in waters around Australia: Characteristics, concentrations, and pathways. PLoS One 8:e80466. doi: 10.1371/journal.pone.0080466
Sanchez-Vidal, A., Thompson, R. C., Canals, M., and de Haan, W. P. (2018). The imprint of microfibres in southern European deep seas. PLoS One 13:e0207033. doi: 10.1371/journal.pone.0207033
Shim, W. J., Song, Y. K., Hong, S. H., and Jang, M. (2016). Identification and quantification of microplastics using Nile Red staining. Mar. Pollut. Bull. 113, 469–476. doi: 10.1016/j.marpolbul.2016.10.049
Tenzer, R., and Gladkikh, V. (2014). Assessment of density variations of marine sediments with ocean and sediment depths. Sci. World J. 2014:823296. doi: 10.1155/2014/823296
Teuten, E. L., Saquing, J. M., Knappe, D. R. U., Barlaz, M. A., Jonsson, S., Björn, A., et al. (2009). Transport and release of chemicals from plastics to the environment and to wildlife. Philos. Trans. R. Soc. B Biol. Sci. 364, 2027–2045. doi: 10.1098/rstb.2008.0284
Thompson, R. C., Olsen, Y., Mitchell, R. P., Davis, A., Rowland, S. J., John, A. W. G., et al. (2004). Lost at sea: Where is all the plastic? Science 304, 838–838. doi: 10.1126/science.1094559
UNEP (2016). Marine Litter – a Modelling Study. Nairobi: United Nations Environment Programme (UNEP).
UNEP (2018). Marine Litter: The Issue [Online]. Available online at: http://www.unenvironment.org/explore-topics/oceans-seas/what-we-do/addressing-land-based-pollution/marine-litter-issue (accessed May 20, 2020).
Van Cauwenberghe, L., Vanreusel, A., Mees, J., and Janssen, C. R. (2013). Microplastic pollution in deep-sea sediments. Environ. Pollut. 182, 495–499. doi: 10.1016/j.envpol.2013.08.013
van Sebille, E., Wilcox, C., Hardesty, B. D., Lebreton, L., Maximenko, N., Van Franeker, J. A., et al. (2015). A global inventory of small floating plastic debris. Environm. Res. Lett. 10:124006. doi: 10.1088/1748-9326/10/12/124006
Welden, N. A. C., and Lusher, A. L. (2017). Impacts of changing ocean circulation on the distribution of marine microplastic litter. Integr. Environ. Assess. Manag. 13, 483–487. doi: 10.1002/ieam.1911
Wesch, C., Elert, A. M., Wörner, M., Braun, U., Klein, R., and Paulus, M. (2017). Assuring quality in microplastic monitoring: About the value of clean-air devices as essentials for verified data. Sci. Rep. 7:5424. doi: 10.1038/s41598-017-05838-4
Willis, K., Eriksen, R., Wilcox, C., and Hardesty, B. (2017). Microplastic distribution at different sediment depths in an urban estuary. Front. Mar. Sci. 4:419. doi: 10.3389/fmars.2017.00419/full
Woodall, L. C., Sanchez-Vidal, A., Canals, M., Paterson, G. L. J., Coppock, R., Sleight, V., et al. (2014). The deep sea is a major sink for microplastic debris. R. Soc. Open Sci. 1:8. doi: 10.1098/rsos.140317
Wright, S. L., Thompson, R. C., and Galloway, T. S. (2013). The physical impacts of microplastics on marine organisms: a review. Environ. Pollut. 178(Suppl. C), 483–492. doi: 10.1016/j.envpol.2013.02.031
Ye, S., and Andrady, A. L. (1991). Fouling of floating plastic debris under Biscayne Bay exposure conditions. Mar. Pollut. Bull. 22, 608–613. doi: 10.1016/0025-326X(91)90249-R
Keywords: deep-sea, fiber, fragment, microplastics, pollution, sediment
Citation: Barrett J, Chase Z, Zhang J, Holl MMB, Willis K, Williams A, Hardesty BD and Wilcox C (2020) Microplastic Pollution in Deep-Sea Sediments From the Great Australian Bight. Front. Mar. Sci. 7:576170. doi: 10.3389/fmars.2020.576170
Received: 25 June 2020; Accepted: 03 September 2020;
Published: 05 October 2020.
Edited by:
Maria Grazia Pennino, Spanish Institute of Oceanography, SpainReviewed by:
Atsuhiko Isobe, Kyushu University, JapanWinnie Courtene-Jones, University of Plymouth, United Kingdom
Copyright © 2020 Barrett, Chase, Zhang, Holl, Willis, Williams, Hardesty and Wilcox. This is an open-access article distributed under the terms of the Creative Commons Attribution License (CC BY). The use, distribution or reproduction in other forums is permitted, provided the original author(s) and the copyright owner(s) are credited and that the original publication in this journal is cited, in accordance with accepted academic practice. No use, distribution or reproduction is permitted which does not comply with these terms.
*Correspondence: Justine Barrett, anVzdGluZS5iYXJyZXR0QGNzaXJvLmF1