- 1Graduate School of Agricultural Science, Tohoku University, Sendai, Japan
- 2Riken Food Co., Ltd., Tagajo, Japan
- 3Nishina Center for Accelerator-Based Science, RIKEN, Saitama, Japan
Heat stress is known to induce photoinhibition, leaf senescence, and nutrient remobilization in terrestrial plants with apical growth, however, its effect on blade erosion and associated-changes in chemical compositions has rarely been studied in marine macroalgae with intercalary growth such as kelp. The present study examined the combined effects of ocean warming (23 and 26°C), irradiance (30 and 180 μmol m–2 s–1), and nutrient enrichment (enriched and non-enriched) on photosystem II maximum efficiency (Fv/Fm) in the kelp Eisenia bicyclis. It also investigated the effect of ocean warming on the kelp’s relative growth rates based on five morphological parameters and three chemical compositions (carbon, nitrogen, and phlorotannnins). A warming effect on photoinhibition (i.e., decline in Fv/Fm) was only detected under the higher irradiance combined with nutrient-enrichment condition. Under this condition, elevated temperature decreased relative growth rates to negative values, indicating occurrence of apical blade erosion. Temperature elevation also caused increases in nitrogen and phlorotannin contents within the whole body, but not carbon content. Moreover, nitrogen content in the meristems at 26°C was higher than that at 23°C, although such a difference was not observed with phlorotannin content. These results suggested that heat-induced apical blade erosion promoted nitrogen accumulation in the meristems, located in the lower part of the blade, in E. bicyclis.
Introduction
Heat stress is one of the major environmental factors affecting biomass and productivity of ecosystem primary producers, including terrestrial plants and marine macroalgae, in the warming world (e.g., Lobell et al., 2012; Bita and Gerats, 2013; Wernberg et al., 2013). In these photosynthetic organisms, abiotic stressors, including warm and cold temperatures, combined with excess light energy result in an increase of reactive oxygen species production in chloroplasts, and consequently cause photoinhibition, which is described as a decline of photosystem II (PSII) efficiency (Murata et al., 2007; Takahashi and Badger, 2011; Bita and Gerats, 2013; Pintó-Marijuan and Munné-Bosch, 2014). To cope with the oxidative stress, these organisms have developed several photoprotective systems, including the production of antioxidant compounds such as phenols and carotenoids (Takahashi and Badger, 2011; Bita and Gerats, 2013; Pintó-Marijuan and Munné-Bosch, 2014). In terrestrial plants, heat stress is known to induce leaf senescence via the promotion of chlorophyll degradation (Pintó-Marijuan and Munné-Bosch, 2014). During senescence, macromolecules, such as proteins, are broken down into small mobile molecules, such as amino acids, and these compounds are translocated to sink tissues through the vascular system (Avila-Ospina et al., 2014). Heat stress can also result in decreased plant nitrogen content (Wu et al., 2019). The remobilization and accumulation of nitrogenous compounds in plant bodies may contribute to the maintenance of photosynthetic apparatus under heat stress, because nitrogen is a primary constituent of chloroplast proteins, including photosynthetic enzymes such as RuBisCo (Hörtensteiner and Feller, 2002; Suzuki et al., 2009; Avila-Ospina et al., 2014).
Marine macroalgae, including brown, red, and green algae, are the major primary producers in coastal marine ecosystems globally (Hurd et al., 2014). Specifically, kelp (Laminariales), large brown algae, are the dominant taxa in temperate reef ecosystems and play an ecologically important role providing food, habitat, nursery, and spawning grounds for various marine organisms (Steneck et al., 2002; Graham, 2004; Christie et al., 2009). However, kelp beds around the world have recently been experiencing regional declines due to ocean warming (Smale, 2020). Previous studies have shown that above-average temperature combined with low nutrient availability during summer causes physiological stresses, such as decreases in nitrogen content, photosynthetic pigments, photosynthetic rates, growth rates, and survival rates in kelp species (Dayton and Tegner, 1984; Gerard, 1997; Tegner et al., 1997; Edwards and Estes, 2006; Gao et al., 2013a, 2016, 2017). Moreover, recent studies have shown that the negative effect of ocean warming on the growth of macroalgae, including kelp, is synergized by nutrient enrichment or eutrophication (Endo et al., 2017; Gouvêa et al., 2017). Elevated temperature and increased nutrient availability have also been found to have interactive effects on the contents of several antioxidant compounds in a red alga (Gouvêa et al., 2017). These results imply that a temperature-elevated and nutrient-enriched condition combined with strong irradiance might cause photoinhibition of macroalgae, because abiotic stresses combined with excess light energy generally result in an increased production of reactive oxygen species in photosynthetic organisms (Murata et al., 2007; Bita and Gerats, 2013; Pintó-Marijuan and Munné-Bosch, 2014). However, little is known about the combined effects of these three abiotic stressors on PSII efficiency, an indicator of photoinhibition, in macroalgal species (however, see Mabin et al., 2019).
Kelp are distantly related to terrestrial plants but have convergently evolved a plant-like body plan and a vascular system for transporting internal resources, such as carbohydrates (mannitol, glucose, and mannose), amino acids, and heavy metals (Drobnitch et al., 2015). They have a holdfast (analogous to roots), a stipe (analogous to stems), and several blades (analogous to leaves), and translocate carbon and nitrogen from old tissues to actively growing tissues (i.e., meristems) through trumpet-shaped sieve elements (Lüning et al., 1973; Lobban, 1978; Davison and Stewart, 1983). However, in contrast to the majority of plants, kelp exhibit intercalary growth; their meristem is located in the lower part of the blades and therefore blade erosion occurs from the apical part (Krumhansl and Scheibling, 2012). Several studies have reported that the erosion rate of kelp species increased during warm seasons, and nitrogen and/or carbon content in meristems increased at the same time (Yoshikawa et al., 2001; Li et al., 2009; Sato and Agatsuma, 2016). Because nitrogen and carbon contents are naturally higher in the meristems than in apical blades (Li et al., 2009; Sato and Agatsuma, 2016), erosion of apical blades with lower contents might result in increased contents within whole blades in the eroded sporophytes. There is also a possibility that remobilization of nitrogenous and carbonous compounds might occur during blade erosion, because this occurs during leaf senescence in terrestrial plants (Avila-Ospina et al., 2014). However, to the best of our knowledge, the direct effect of increased temperature on blade erosion and associated-changes in chemical compositions has rarely been studied in macroalgae.
Brown algae, including kelp, contain unique polyphenols called phlorotannins, which have antioxidant, antibacterial, antifouling, and antiherbivore properties (Ragan and Glombitza, 1986; Amsler and Fairhead, 2006; Cruces et al., 2017). Although a significant effect of high- temperature acclimation on brown algal phlorotannin content has rarely been detected (Hay et al., 2010; Poore et al., 2013; Endo et al., 2017), Hargrave et al. (2017) found that elevated temperature from 12 to 18°C resulted in increased phenolic content (i.e., phlorotannin content) in the kelp Laminaria digitata, and speculated that this might be a photoprotective response to heat-induced photoinhibition. However, as phlorotannin contents tend to be higher in the meristem than in the apical part (Amsler and Fairhead, 2006), as well as nitrogen and carbon contents (Li et al., 2009; Sato and Agatsuma, 2016), apical blade erosion under high temperature might result in increased contents within whole blades in eroded kelp. There is also a possibility that remobilization of carbonous compounds during blade erosion might result in the increased content of carbonous phlorotannins, although this hypothesis needs to be tested.
The kelp Eisenia bicyclis (Kjellman) Setchell is one of the dominant species on temperate reefs around Japan (Kawai et al., 2020; Terada et al., 2020), and is one of the most phlorotannin-rich kelp globally (Ragan and Glombitza, 1986). In the present study, two different experiments were conducted; experiment 1 tested the combined effects of warming, irradiance, and nutrient enrichment on maximum quantum yield of PSII (Fv/Fm) to determine the irradiance and nutrient conditions in which warming induces photoinhibition in this kelp species. Under this condition, experiment 2 examined the warming effect on five relative growth rates (RGRs) based on blade length (BL), blade width (BW), blade area (BA), wet weight (WW), and dry weight (DW), and the content of three chemical compositions, including carbon, nitrogen, and phlorotannins, to address the following questions: (1) does warming result in blade erosion?; and (2) do chemical compositions increase as a result of blade erosion?
Materials and Methods
Experiment 1
Eisenia bicyclis juvenile sporophytes were collected in June 2013 at a site in Kitsune-zaki (39°51′ 20″N, 139° 48′ 56″E), Oshika Peninsula, Miyagi Prefecture, northeastern Japan. They were transported to the laboratory in insulated cool boxes and each specimen was immediately placed in a flask containing 500 mL of sterile seawater. These flasks were maintained in four incubators under aeration with a 12 h light (L): 12 h dark (D) photoperiod, and at an irradiance of 70 μmol photon m–2 s–1 at 20 °C for 3 d until the start of the experiment. The WW of 68 specimens (initial wet weight) were measured at the start of experiment using an electronic balance (0.1 mg accuracy) after removal of excess moisture by blotting on paper towels. A total of 48 specimens of similar size were then selected and randomized into eight groups of six specimens with a similar size distribution. These eight groups were each subjected to one of the eight different treatments.
The experiment had a three-way factorial design (2 × 2 × 2 treatments) comprising two temperature levels (23 and 26°C), two nutrient levels (enriched and non-enriched seawater), and two light levels (30 and 180 μmol photon m–2 s–1). The ambient and elevated temperature was set at 23 and 26°C, respectively. This is because average seawater temperatures during the summer of the years 2011–2014 were 22.3, 23.8, 23.2, and 22.0°C, respectively, in an E. bicyclis kelp bed in Benten-zaki (38° 39′ 31″N, 141° 26′ 56″E), Shizugawa Bay, near the Kitsune-zaki site (Endo, unpublished data), and average temperatures have been predicted to increase 2–3°C by 2100 under the most serious climate change scenario (IPCC, 2013). Low and high irradiance levels were set at 30 and 180 μmol photon m–2 s–1, respectively, because critical irradiance for E. bicyclis growth has been estimated to be between 0.8 and 1.6 mol photon m–2 d–1 (Kurashima et al., 1996); these values can be converted into 18.5 and 37.0 μmol photon m–2 s–1, respectively, for a 12 h L: 12 h D photoperiod, and light saturation of adult sporophytes reportedly occurs at 185 μmol photon m–2 s–1 (Maegawa et al., 1987). Enriched and non-enriched seawater comprised 25% PESI-enriched seawater (Tatewaki, 1966) and non-enriched sterile seawater, respectively. This was because interactive effects of nutrient enrichment using a 25% PESI and elevated temperature from 23 to 26°C on the growth of E. bicyclis were detected in a previous study (Endo et al., 2017). Mean (± SD) ammonia, nitrate, nitrite, and phosphate concentrations in the nutrient-enriched medium were reportedly 8.71 ± 0.39, 131.34 ± 12.04, 0.40 ± 0.01, and < 0.16 μmol l–1, respectively, while those in the non-enriched medium were < 0.71, 2.11 ± 0.13, 0.41 ± 0.01, and < 0.16 μmol l–1, respectively (Endo et al., 2017).
Sporophytes were maintained in incubators under aeration and with a photoperiod of 12 h L: 12 h D for 9 days. The culture medium in each flask was changed every 3 days. At the end of experiment, the PSII maximum efficiency (Fv/Fm) of each specimen was measured using a Diving-PAM (Heinz Walz GmbH, Germany), after 5 min of dark acclimation to exclude energy-dependent fluorescence quenching and quenching by state transitions (Hanelt, 1998; Bischof et al., 1999). Photographs of these specimens were also taken using a digital camera (Supplementary Figure S1).
Experiment 2
Sixty E. bicyclis juvenile sporophytes were collected in June 2014 from the Benten-zaki coast and transported to the laboratory in insulated cool boxes. Each specimen was immediately placed in a flask containing 500 mL of sterile seawater. The 60 flasks were maintained in five incubators under aeration with a 12 h L: 12 h D photoperiod at 70 μmol photons m–2 s–1, and at a temperature of 20°C for 24 h. After incubation, the WW of each specimen (initial WW) was measured. Photographs of these specimens were taken using a digital camera, and BL, BW, and BA of all specimens were measured photographically using the software Image J (Schneider et al., 2012). A total of 40 specimens of similar size were selected and randomized into five groups of eight specimens with a similar size distribution.
One of the five groups was used to measure initial carbon, nitrogen, and phlorotannin content. The eight sporophytes were cut in half (right and left). One half was weighed before and after drying for 72 h at 80°C in a convection oven, and the carbon and nitrogen content of the specimens was measured using an organic elemental analyzer (FLASH2000, Thermo Fisher Scientific, Waltham, MA, United States). The other half was immediately placed in a 15 mL conical tube with 4 mL of 80% aqueous methanol at 4°C in the dark, and phlorotannin content was measured by quantifying the total level of polyphenolics according to the Folin-Ciocalteu method (Van Alstyne, 1995; Kamiya et al., 2010; Endo et al., 2017), as the phlorotannins are the major polyphenolics in the brown algae (Amsler and Fairhead, 2006).
Each specimen from the other four groups was placed in a flask containing 500 mL of 6.25% PESI nutrient-enriched culture medium (Tatewaki, 1966). Two of the four groups were assigned to one of two temperature treatments at 23 and 26°C under aeration and with a photoperiod of 12 h L: 12 h D at 180 μmol photons m–2 s–1 for 24 days. The culture medium in each flask was changed every 3 d. The concentration of ammonia, nitrate, nitrite, and phosphate in the medium was measured with five replications. At the end of the culture experiment, juvenile WW (final WW) was measured and photographs were taken to measure final BW, BL, and BA of all specimens. RGRs (% d–1) based on the BW, BL, BA, WW, and DW were calculated as 100 × ln (final value/initial value)/24 days.
The sporophytes in each two groups cultured at 23 or 26°C were cut in half (right and left, as illustrated in Figures 1A–D), and the carbon, nitrogen, phlorotannin contents within the whole sporophytes were measured using the same methods mentioned above. The sporophytes in another group cultured at 23°C were cut in half into upper and lower parts at the center of the BL (Figure 1C). The three chemical compositions of these two different parts in the 23°C treatment and whole (eroded; see section “Results”) sporophytes cultured at 26°C were also measured to compare contents between lower parts of the blades (i.e., meristems) cultured at 23 and 26°C, and between upper and lower parts of the blades in the 23°C treatment.
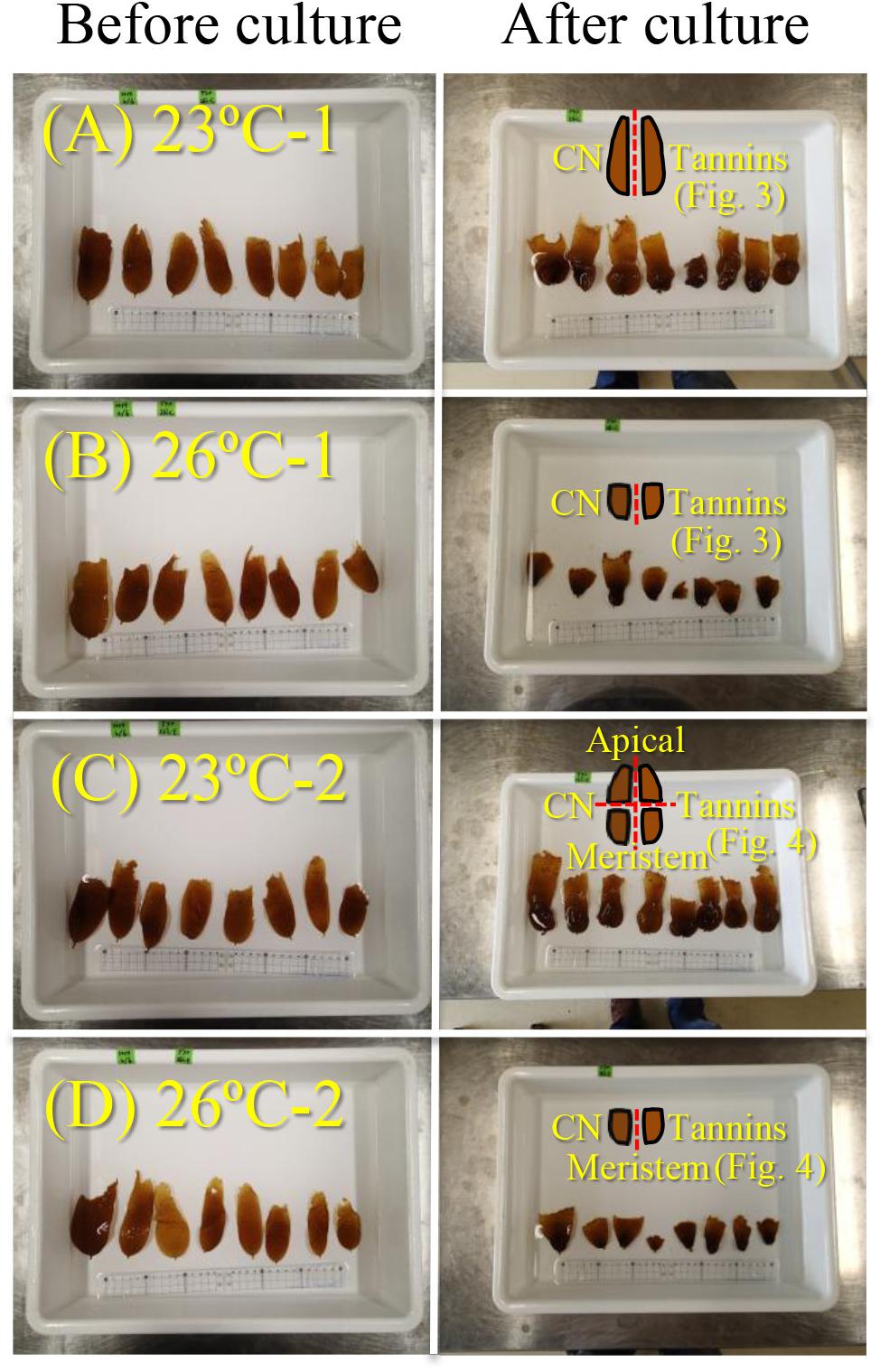
Figure 1. Photographs of E. bicyclis juvenile sporophytes before and after culture at 23°C (A,C) and 26°C (B,D), with illustrations showing the cutting methods (red dotted line) for the chemical composition measurements in experiment 2. All kelps (A–D) were cut in half (right and left) and each half was used to measure carbon and nitrogen (indicated as “CN”), and phlorotannin contents (indicated as “Tannins”) within whole sporophytes. Eight kelps cultured at 23°C (C) were also cut into upper and lower parts (i.e., apical and meristems, respectively) at the center of the BL in order to measure the chemical compositions separately. Results of the measurements are shown in Figures 3, 4.
Statistical Analysis
For experiment 1, the combined effects of temperature, nutrient availability, and irradiance on Fv/Fm were tested using a three-way analysis of variance (ANOVA). When significant interactions between two or three factors were found, Tukey’s multiple comparison tests were used to determine if the non-additive effect was synergistic or antagonistic. For experiment 2, differences in initial WW, DW, BL, BW, and BA between the five groups were analyzed by one-way ANOVA. A principle component analysis was conducted to examine the relationship between the eight variables (five RGRs and three chemical compositions). Correlations between the RGRs and chemical compositions were confirmed using Pearson’s test. The effect of temperature on RGRs and chemical compositions were analyzed by multivariate analysis of variance (MANOVA), followed by univariate tests, due to the correlations between these variables. Differences in chemical compositions between the upper and lower parts in the 23°C treatment and the whole (eroded) sporophytes in the 26°C treatment were analyzed by one-way ANOVA and Tukey’s multiple comparison test. All analyses were performed using SPSS software version 20.0 (IBM, Armonk, NY, United States).
Results
Experiment 1
The results of the three-way ANOVA revealed significant individual effects of temperature and irradiance on the Fv/Fm of E. bicyclis juvenile sporophytes, and significant interactions between temperature and irradiance, and between temperature and nutrient enrichment (Table 1 and Figure 2). Tukey’s multiple comparison tests showed that Fv/Fm was significantly lower in the high irradiance treatments than in the low irradiance treatments. Moreover, Fv/Fm was significantly lower at 26°C than at 23°C in the high irradiance combined with nutrient enriched treatment. There was no significant difference in Fv/Fm between 23 and 26°C in the high irradiance combined with non-enriched treatment, and in the low irradiance treatments. This indicated that the effect of elevated temperature was synergized by increased irradiance combined with nutrient enrichment.
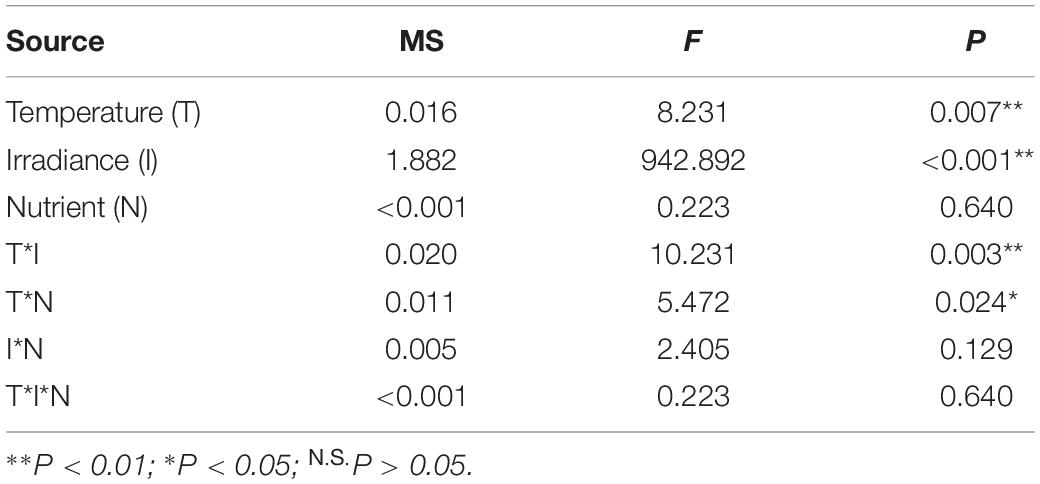
Table 1. Results of three-way ANOVA on the effects of temperature, irradiance, and nutrient enrichment on maximum PSII efficiency (Fv/Fm) of E. bicyclis juvenile sporophytes.
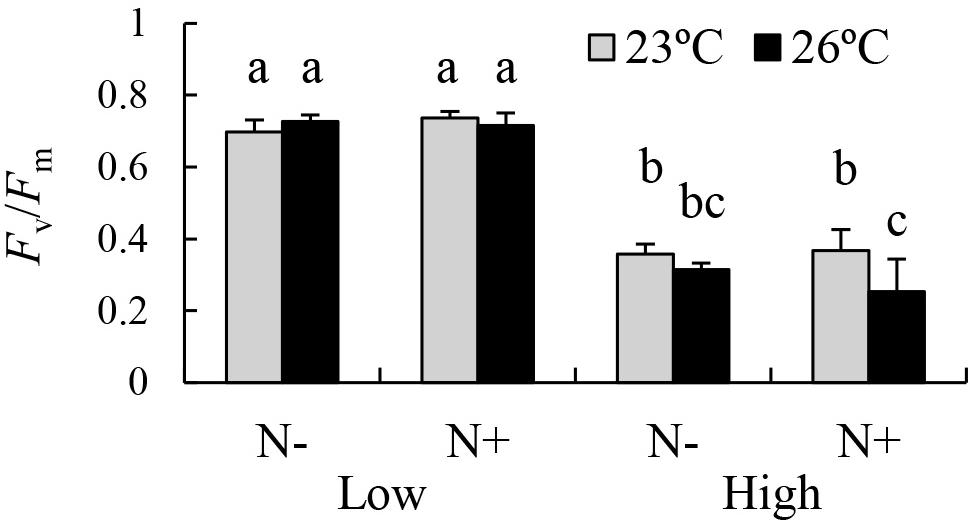
Figure 2. PSII Maximum efficiency (Fv/Fm) of the brown alga E. bicyclis cultured in eight different treatments (mean ± SD, n = 6). N+ and N– indicate nutrient-enriched and non-enriched treatments, respectively. Low and High indicate low and high irradiance treatments, respectively. Different small letters indicate significant differences among different treatments.
Experiment 2
There was no significant difference in WW (MS < 0.001, F = 0.001, P = 1.000), DW (MS < 0.001, F = 0.001, P = 1.000), BL (MS = 1.367, F = 1.021, P = 0.410), BW (MS = 0.217, F = 0.578, P = 0.681), and BA (MS = 5.084, F = 0.149, P = 0.962) among the five groups. Mean (± SD) WW, DW, BL, BW, and BA of the sporophytes at the beginning of the experiment were 694.5 ± 225.0 mg, 92.8 ± 29.2 mg, 7.95 ± 1.16 cm, 3.36 ± 0.60 cm, and 22.08 ± 5.57 cm2, respectively. Mean (± SD) nitrogen, carbon, and phlorotannin contents of the sporophytes at the beginning of the experiment were 1.83 ± 0.14%, 28.07 ± 1.28%, and 11.99 ± 4.54%, respectively. Nitrate and phosphate concentrations in the 6.25% PESI nutrient-enriched medium were 57.39 ± 7.91 and 1.72 ± 0.40 μmol L–1, respectively. Ammonia and nitrite were not detected.
Principal component analysis results showed that principal component 1 was positively correlated with RGRs and negatively correlated with nitrogen and phlorotannin contents (Table 2). In contrast, principal component 2 was highly correlated with carbon content. Significant negative correlations between RGRs and nitrogen content were detected (Table 3). The phlorotannin content correlated negatively with RGR-WW and RGR-DW but not with RGR-BL, RGR-BW, and RGR-BA. There was no significant correlation between RGRs and carbon content.
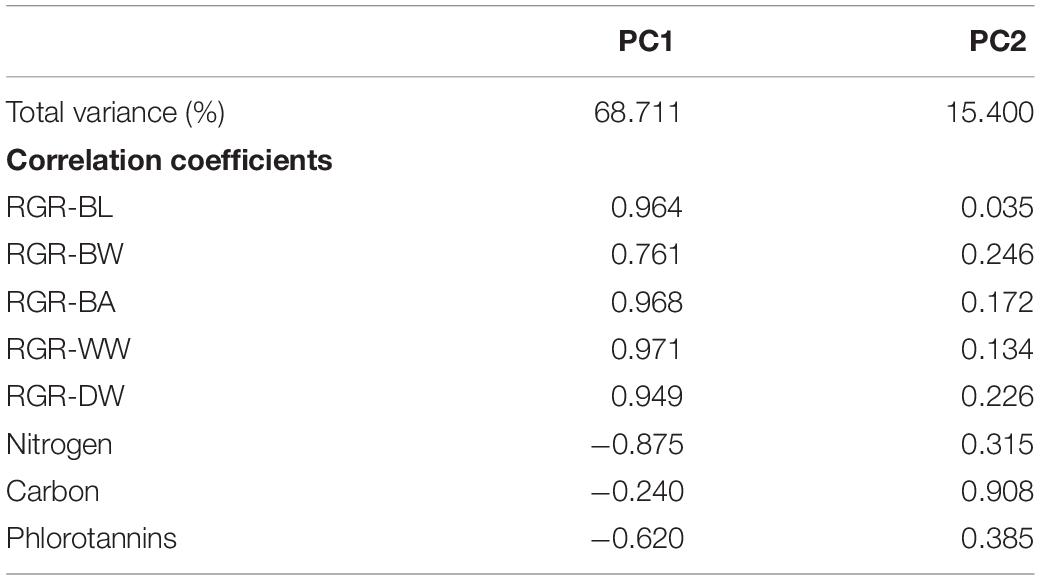
Table 2. Percentage of total variance and correlations of two principal components of E. bicyclis juvenile sporophytes cultured at two different temperatures.
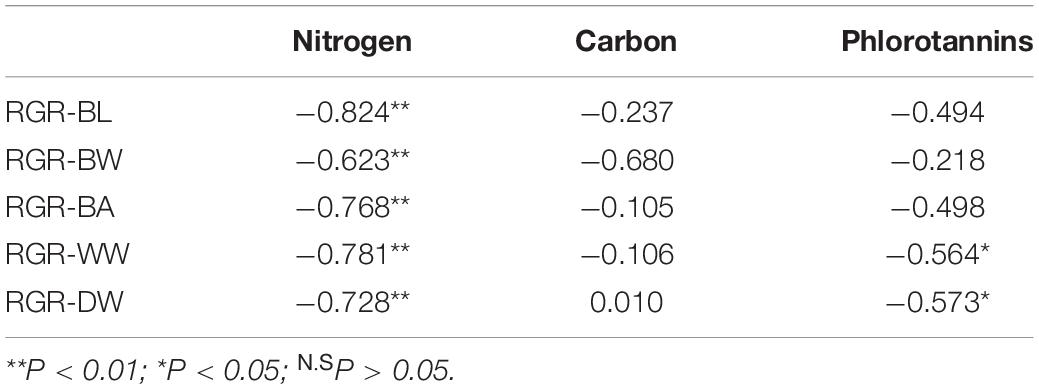
Table 3. Correlation coefficients between RGRs and chemical compositions of E. bicyclis juvenile sporophytes cultured at two different temperatures.
MANOVA results showed that variables were significantly different between two temperature treatments (Table 4). Post-MANOVA univariate tests detected significant differences in the five RGRs, nitrogen content, and phlorotannin content among the treatments but not in carbon content. The five RGRs at 26°C were significantly lower than those at 23°C (Figure 3). In addition, RGR-BL, RGR-BW, RGR-BA, and RGR-WW at 26°C were negative, whereas RGR-DW was positive even at 26°C. Nitrogen contents at 23°C (1.13 ± 0.13%DW) were significantly higher than those at 26°C (1.60 ± 0.22%DW). Carbon contents did not differ significantly between 23°C (34.57 ± 0.71%DW) and 26°C (35.25 ± 1.35%DW). Phlorotannin contents at 26 °C (21.68 ± 4.23%DW) were significantly higher than those at 23°C (14.93 ± 2.66%DW).
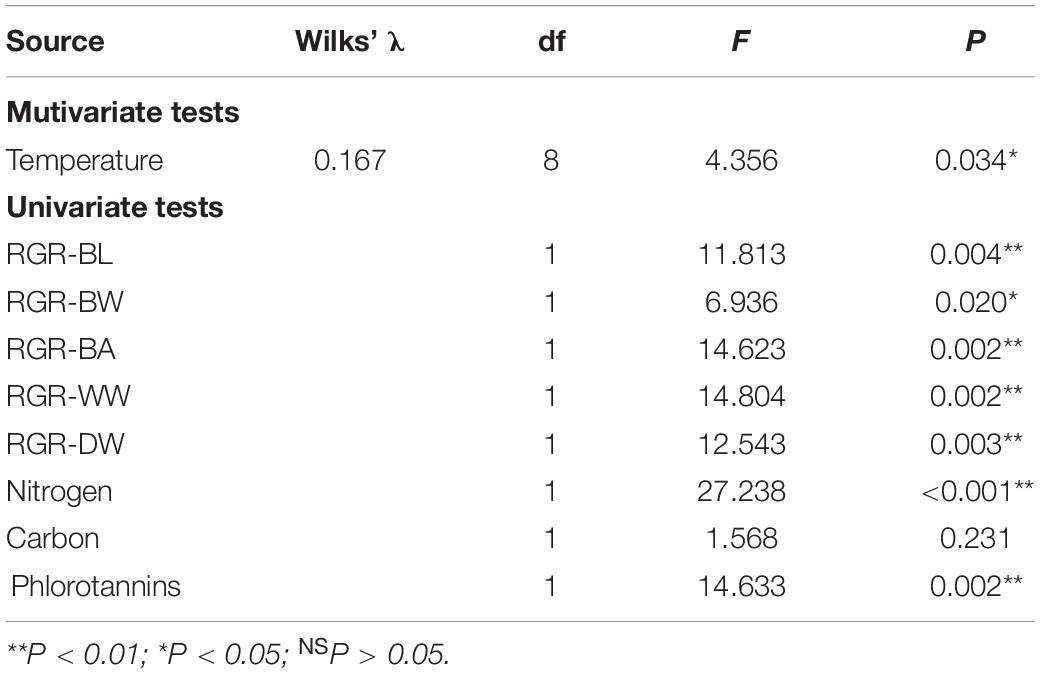
Table 4. Results of MANOVA on the effect of temperature on the RGRs and chemical compositions of E. bicyclis juvenile sporophytes.
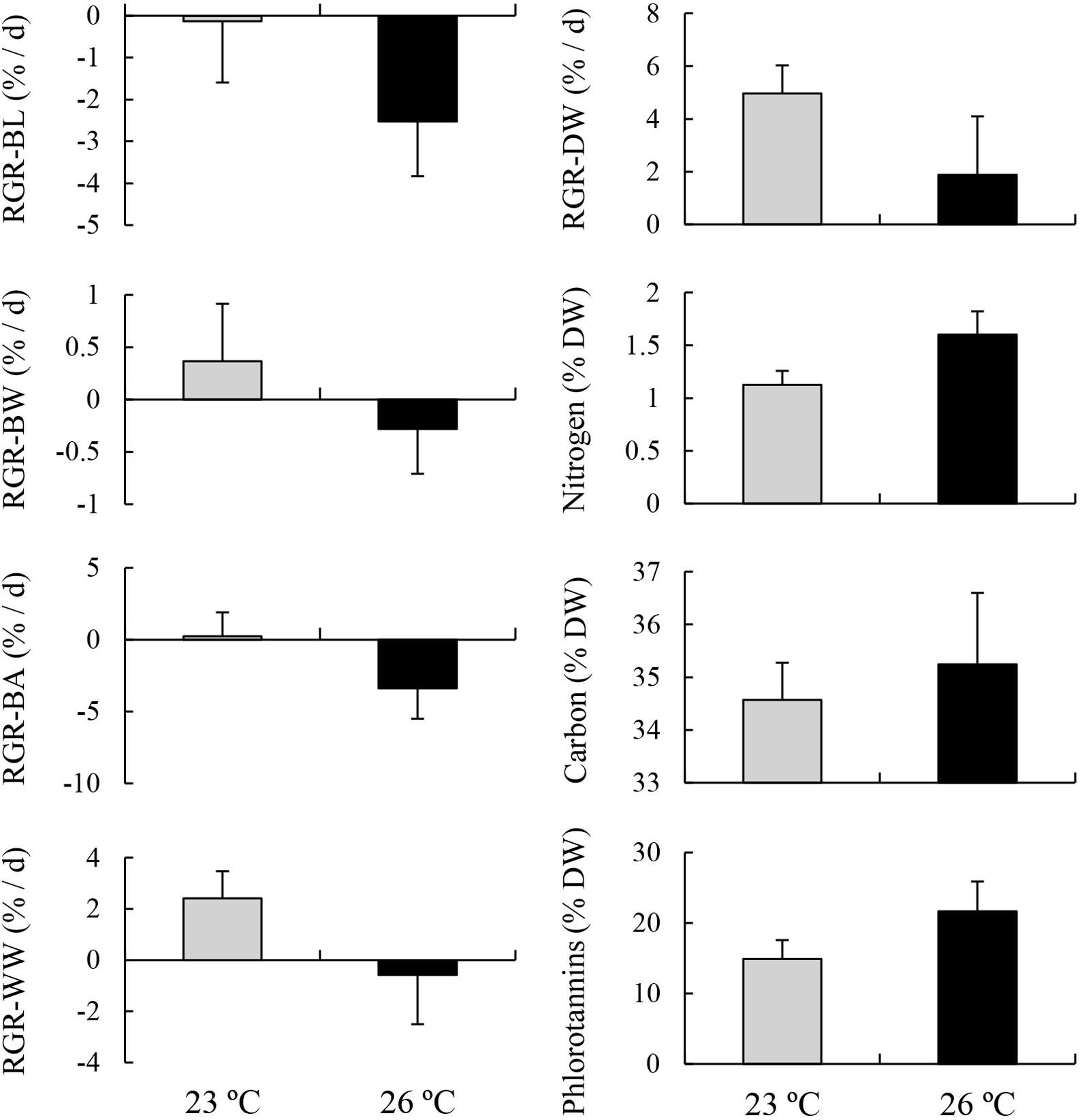
Figure 3. Relative growth rates (RGRs) based on blade length (BL), blade width (BW), blade area (BA), wet weight (WW), and dry weight (DW), and contents of chemical compositions including nitrogen, carbon, and phlorotannins of E. bicyclis juvenile sporophytes cultured in two different temperature treatments (mean ± SD, n = 8).
Nitrogen contents in the lower part of the blades (i.e., meristem) cultured at 23°C were significantly higher than those in the upper part of the same blades, but were significantly lower than those of the eroded sporophytes cultured at 26°C (Figure 4; MS = 1.241, F = 30.826, P < 0.001). No significant difference was found in carbon content among these treatments (MS = 0.997, F = 0.528, P = 0.598). Phlorotannin contents of the lower part of the sporophytes cultured at 23°C were significantly higher than those of the upper part of the same sporophytes, whereas no significant difference was detected between the value of lower part of the sporophytes cultured at 23°C and those of the eroded sporophytes cultured at 26°C (MS = 0.070, F = 3.919, P = 0.036).
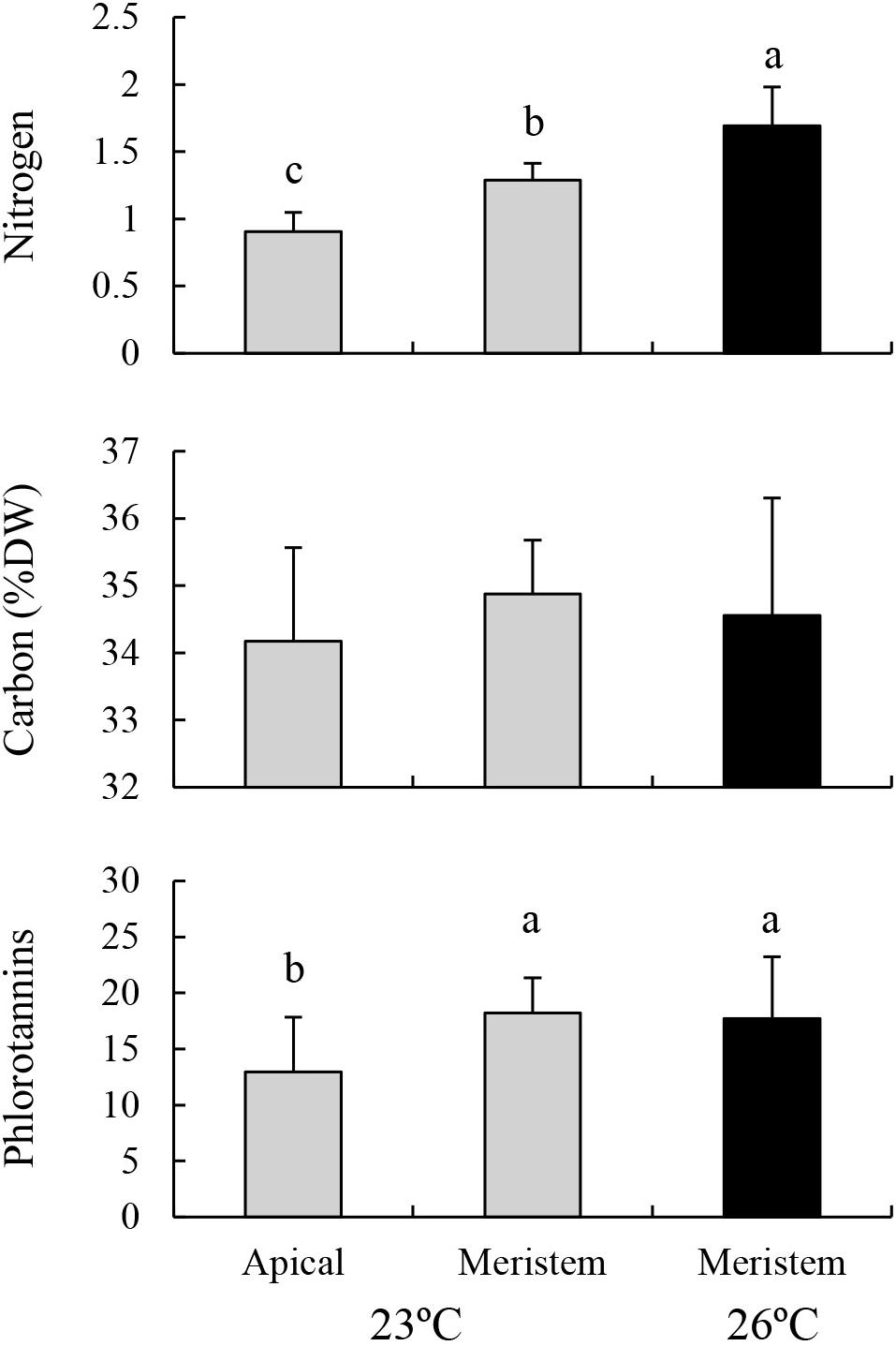
Figure 4. Nitrogen, carbon, and phlorotannin contents of the apical part and meristems (i.e., lower part) of the blades of E. bicyclis juvenile sporophytes cultured at 23°C, and of erose blades (i.e., meristem only) cultured at 26°C (mean ± SD, n = 8). Different small letters indicate significant differences among different treatments.
Discussion
The maximum PSII efficiency (Fv/Fm) of macroalgae is known to decrease diurnally in response to increased irradiance around midday and recover during the afternoon (i.e., dynamic photoinhibition, Hanelt, 1998; Cruces et al., 2017). Although an acclimation to relatively strong irradiance for several days can result in a reduced degree of photoinhibition and an increased rate of recovery (Bischof et al., 1999), long-term exposure to strong irradiance causes a gradual decrease in Fv/Fm (i.e., chronic photoinhibition, Hanelt, 1998), because excess light energy causes inhibition of the PSII repair process via the production of reactive oxygen species (Takahashi and Badger, 2011). In the present study, the Fv/Fm of E. bicyclis juvenile sporophytes cultured for 9 days was lower in the high irradiance treatments than in the low irradiance treatments, suggesting that this 9 days culture caused chronic photoinhibition of the algae rather than a reduction in degree of photoinhibition. Moreover, there was a significant interactive effect of elevated temperature, increased irradiance, and nutrient enrichment on the Fv/Fm. This interaction indicated that the temperature-elevated and nutrient-enriched condition combined with strong irradiance caused photoinhibition in this alga. Although our previous study showed that the negative effect of elevated temperature (23–26°C) on RGRs, based on the BW of this species, was synergized by nutrient enrichment, this synergistic effect was independent of irradiance condition (Endo et al., 2017). Therefore, this reduction in RGRs under warm and nutrient-rich conditions cannot be fully explained by the irradiance-dependent photoinhibition found in the present study. Further research is needed to reveal the physiological mechanism that causes the synergistic effects of warming and eutrophication on macroalgal growth. Nevertheless, there is no doubt that the negative effects of global stressors (i.e., ocean warming) on E. bicyclis production can vary with local nutrient concentration.
Our previous study also showed that elevated temperature from 23 to 26°C resulted in decreases in the RGRs based on the WW and BW of E. bicyclis juvenile sporophytes in a 9 days experiment, while RGRs based on BL and BA were unaffected by changing temperature (Endo et al., 2017). In the present 24 days experiment, temperature elevation under nutrient-enriched and strong-irradiance conditions decreased the four RGRs, based on BL, BW, BA, and WW of juvenile sporophytes, from positive values (at 23°C) to negative values (at 26°C). These results suggested that apical blade erosion occurred in the 26°C treatment. However, surprisingly, the RGRs based on DW were positive values not only at 23°C but also at 26°C. This indicated that this species synthesized and accumulated organic compounds even during active erosion at 26°C.
The present study also showed that increased temperature from 23 to 26°C resulted in a significant increase in the nitrogen content of E. bicyclis juvenile sporophytes. Additionally, significant negative correlations between RGRs and nitrogen content were detected. Moreover, surprisingly, the nitrogen content in the meristem of the eroded blades cultured at 26°C was higher than that in the meristem of the blades cultured at 23°C. These results suggested that nitrogen accumulation in the meristems was promoted during blade erosion. In contrast, Gerard (1997) reported that the nitrogen content of the kelp Saccharina latissima (formerly Laminaria saccharina) decreased as a result of a 4 days exposure to a high temperature of 22°C, compared with a control at 12°C. Moreover, Hay et al. (2010) showed that a temperature elevation from 21 to 28°C decreased the nitrogen content of the fucoid (Fucales) brown alga Sargassum flavicans in an 8 days culture experiment. Thus, the heat-induced nitrogen accumulation observed in the present study does not appear to occur in kelp specimens without apical blade erosion and in fucoid species that have meristems in the apical part of the thallus. Kelp sporophytes are known to translocate nitrogen and carbon from their apical blades to the meristems through trumpet-shaped sieve elements (Lüning et al., 1973; Lobban, 1978; Davison and Stewart, 1983). However, there was no significant effect of temperature on carbon content, and no significant correlation between RGRs and carbon contents detected in this study. Hence, carbon accumulation in kelp meristems does not appear to be promoted by warming and blade erosion.
Hargrave et al. (2017) reported that elevated temperature from 12 to 18°C resulted in increased phenolic content in the kelp Laminaria digitata, and speculated that this might be a photoprotective response. The present study also recorded increased phlorotannin content of E. bicyclis juvenile sporophytes in response to temperature elevation. However, there was no significant difference in phlorotannin content within the meristems between eroded (at 26°C) and non-eroded (at 23°C) sporophytes. Moreover, phlorotannin content was higher in the meristems than in the apical blades of the non-eroded sporophytes cultured at 23°C, as reported in other kelp species (Amsler and Fairhead, 2006). Therefore, increased phlorotannin content in response to elevated temperature in the present study was due to the loss of apical blades with a lower content, rather than an antioxidant response or the result of carbon translocation. In addition, this study detected a significant negative correlation between RGRs and phlorotannin content in this kelp species. Such a negative relationship has often been recorded in other brown algae, and has been attributed to the fact that measurable phlorotannin levels can increase in slow-growing tissue in which rates of phlorotannin synthesis outpace rates of decomposition and incorporation of phlorotannins into cell walls (Amsler and Fairhead, 2006). The results of the present study provide a new insight that increased erosion rate can also induce an increase in the phlorotannin content of kelp species. In contrast, there was no detectable effect of temperature on phlorotannin content recorded in fucoid brown algae (Hay et al., 2010; Poore et al., 2013), which are also dominant taxa in temperate reef ecosystems along with kelp (Schiel and Foster, 2006; Coleman and Wernberg, 2017). This may be explained by the lack of heat-induced erosion in these algae, because their meristems are located in the apical part of the thallus.
The kelp life cycle involves an alternation of heteromorphic generations, with a macroscopic sporophyte and a microscopic gametophyte (Schiel and Foster, 2006). Whereas annual kelp species survive during summer in the form of gametophytes, which have higher-temperature tolerance compared with sporophytes (Bolton and Lüning, 1982; tom Dieck, 1993); the sporophytes of perennial species survive during the summer by using internal nitrogen reserves, which are accumulated during winter when nutrient availability is high (Chapman and Craigie, 1977; Chapman and Lindley, 1980). Moreover, kelp sporophytes have the ability to acclimatize their physiological traits to high-temperatures in the short-term (Davison, 1991), and genetic differentiation of high-temperature tolerance occurs by natural selection in the long-term, even within a species (Gerard, 1997; Gao et al., 2013b). Gerard (1997) compared the physiological traits of kelp species between two ecotypes with different high-temperature tolerances, and suggested that the high-temperature tolerance of kelp species is associated with the ability to accumulate and maintain internal nitrogen reserves rather than the thermal stability of their photosynthetic apparatus. The present study showed that nitrogen accumulation in the meristem is promoted by heat-induced blade erosion in E. bicylis juvenile sporophytes. This property may contribute to survival during summer with warm and nutrient-poor conditions for these sporophytes, which often germinate between spring and summer (Suzuki et al., 2020). However, it is uncertain whether erose sporophytes recover and survive after summer; further research is necessary to determine this. tom Dieck (1993) found that the gametophytes of this species had the highest- temperature tolerance among kelp globally, as well as Undaria pinnatifida, which also grows along the coast of Japan. It is speculated that this may be due to the large temperature variations among seasons and latitudes in Japan, which has a large north-south latitude extension (tom Dieck, 1993).
The erosion rate of kelp is known to differ among species and populations (Krumhansl and Scheibling, 2012). For example, the erosion rate of Saccharina longissima, which is distributed along the eastern coast of Hokkaido Island, subarctic Japan, where it is strongly affected by the nutrient-rich Oyashio cold current, is lower compared with that of S. japonica, which is distributed along the coasts less affected by cold current (Li et al., 2009; Sato and Agatsuma, 2016). The present study showed that the temperate kelp E. bicylis, distributed from northern Honshu Island to northern Kyushu Island where it is affected by the nutrient-poor Kuroshio and/or Tsushima warm currents, also exhibits blade erosion and associated nitrogen accumulation. These results suggested that the ability to accumulate nitrogen through blade erosion differs among species; species inhabiting unstable environments in terms of temperature and nutrient availability have a stronger ability than those in more stable environments. Globally, kelp beds have recently been experiencing regional declines associated with ocean warming (Smale, 2020). Although this has been explained by the interactive effects of this global stressor and local environments on kelp productivity (Endo et al., 2017, 2020; Gao et al., 2017), it can also be explained by the differing species responses to warming, because nitrogen accumulation ability varies among kelp species.
Data Availability Statement
The raw data supporting the conclusions of this article will be made available by the authors, without undue reservation, to any qualified researcher.
Author Contributions
HE and YA conceptualized and designed the present study. HE and XG cultured the algae. EI measured the nitrogen and carbon contents of the algae. HE and JK measured the phlorotannin content of the algae. HE and YS prepared the manuscript. All authors checked and approved the manuscript for submission.
Funding
This work was supported by the project “Tohoku Ecosystem-Associated Marine Sciences (TEAMS) and JSPS KAKENHI Grant Number 20K06187.”
Conflict of Interest
YS was employed by the company Riken Food Co., Ltd.
The remaining authors declare that the research was conducted in the absence of any commercial or financial relationships that could be construed as a potential conflict of interest.
Acknowledgments
We thank Drs. M. Aoki, A. Horikoshi, and H. Suzuki of Tohoku University for their assistance with sample collection.
Supplementary Material
The Supplementary Material for this article can be found online at: https://www.frontiersin.org/articles/10.3389/fmars.2020.575721/full#supplementary-material
Supplementary Figure 1 | Photographs of E. bicyclis juvenile sporophytes cultured at 23°C (A,C,E,G) and 26°C (B,D,F,H) combined with two nutrient levels and two irradiance levels in the experiment 1. N− (A,B,E,F) and N+ (C,D,G,H) indicate non-enriched and enriched treatments, respectively. I− (A–D) and I+ (E–H) indicate low and high irradiance treatments, respectively.
References
Amsler, C. D., and Fairhead, V. A. (2006). Defensive and sensory chemical ecology of brown algae. Adv. Bot. Res. 43, 1–91. doi: 10.1016/s0065-2296(05)43001-3
Avila-Ospina, L., Moison, M., Yoshimoto, K., and Masclaux-Daubresse, C. (2014). Autophagy, plant senescence, and nutrient recycling. J. Exper. Bot. 65, 3799–3811. doi: 10.1093/jxb/eru039
Bischof, K., Hanelt, D., and Wiencke, C. (1999). Acclimation of maximal quantum yield of photosynthesis in the brown alga Alaria esculenta under high light and UV radiation. Plant Biol. 1, 435–444. doi: 10.1111/j.1438-8677.1999.tb00726.x
Bita, C., and Gerats, T. (2013). Plant tolerance to high temperature in a changing environment: scientific fundamentals and production of heat stress-tolerant crops. Front. Plant Sci. 4:273. doi: 10.3389/fpls.2013.00273
Bolton, J. J., and Lüning, K. (1982). Optimal growth and maximal survival temperatures of Atlantic Laminaria species (Phaeophyta) in culture. Mar. Biol. 66, 89–94. doi: 10.1007/bf00397259
Chapman, A. R. O., and Craigie, J. S. (1977). Seasonal growth in Laminaria longicruris: relations with dissolved inorganic nutrients and internal reserves of nitrogen. Mar. Biol. 40, 197–205. doi: 10.1007/bf00390875
Chapman, A. R. O., and Lindley, J. E. (1980). Seasonal growth of Laminaria solidungula in the Canadian High Arctic in relation to irradiance and dissolved nutrient concentrations. Mar. Biol. 57, 1–5. doi: 10.1007/bf00420961
Christie, H., Norderhaug, K. M., and Fredriksen, S. (2009). Macrophytes as habitat for fauna. Mar. Ecol. Prog. Ser. 396, 221–233. doi: 10.3354/meps08351
Coleman, M. A., and Wernberg, T. (2017). Forgotten underwater forests: the key role of fucoids on Australian temperate reefs. Ecol. Evol. 7, 8406–8418. doi: 10.1002/ece3.3279
Cruces, E., Rautenberger, R., Rojas-Lillo, Y., Cubillos, V. M., Arancibia-Miranda, N., Ramírez-Kushel, E., et al. (2017). Physiological acclimation of Lessonia spicata to diurnal changing PAR and UV radiation: differential regulation among down-regulation of photochemistry, ROS scavenging activity and phlorotannins as major photoprotective mechanisms. Photosynth. Res. 131, 145–157. doi: 10.1007/s11120-016-0304-4
Davison, I. R. (1991). Environmental effects on algal photosynthesis: temperature. J. Phycol. 27, 2–8. doi: 10.1111/j.0022-3646.1991.00002.x
Davison, I. R., and Stewart, W. D. P. (1983). Occurrence and significance of nitrogen transport in the brown alga Laminaria digitata. Mar. Biol. 77, 107–112. doi: 10.1007/bf00396307
Dayton, P. K., and Tegner, M. J. (1984). Catastrophic storms, El Niño, and patch stability in a southern California kelp community. Science 224, 283–285. doi: 10.1126/science.224.4646.283
Drobnitch, S. T., Jensen, K. H., Prentice, P., and Pittermann, J. (2015). Convergent evolution of vascular optimization in kelp (Laminariales). Proc. R. Soc. B Biol. Sci. 282:20151667. doi: 10.1098/rspb.2015.1667
Edwards, M. S., and Estes, J. A. (2006). Catastrophe, recovery and range limitation in NE Pacific kelp forests: a large-scale perspective. Mar. Ecol. Prog. Ser. 320, 79–87. doi: 10.3354/meps320079
Endo, H., Sato, Y., Kaneko, K., Takahashi, D., Nagasawa, K., Okumura, Y., et al. (2020). Ocean warming combined with nutrient enrichment increases the risk of herbivory during cultivation of the marine macroalga Undaria pinnatifida. ICES J. Mar. Sci. 2020:fsaa069. doi: 10.1093/icesjms/fsaa069
Endo, H., Suehiro, K., Gao, X., and Agatsuma, Y. (2017). Interactive effects of elevated summer temperature, nutrient availability, and irradiance on growth and chemical compositions of juvenile kelp, Eisenia bicyclis. Phycol. Res. 65, 118–126. doi: 10.1111/pre.12170
Gao, X., Endo, H., Nagaki, M., and Agatsuma, Y. (2016). Growth and survival of juvenile sporophytes of the kelp Ecklonia cava in response to different nitrogen and temperature regimes. Fish. Sci. 82, 623–629. doi: 10.1007/s12562-016-0998-4
Gao, X., Endo, H., Nagaki, M., and Agatsuma, Y. (2017). Interactive effects of nutrient availability and temperature on growth and survival of different size classes of Saccharina japonica (Laminariales, Phaeophyceae). Phycologia 56, 253–260. doi: 10.2216/16-91.1
Gao, X., Endo, H., Taniguchi, K., and Agatsuma, Y. (2013a). Combined effects of seawater temperature and nutrient condition on growth and survival of juvenile sporophytes of the kelp Undaria pinnatifida (Laminariales; Phaeophyta) cultivated in northern Honshu, Japan. J. Appl. Phycol. 25, 269–275. doi: 10.1007/s10811-012-9861-x
Gao, X., Endo, H., Taniguchi, K., and Agatsuma, Y. (2013b). Genetic differentiation of high-temperature tolerance in the kelp Undaria pinnatifida sporophytes from geographically separated populations along the Pacific coast of Japan. J. Appl. Phycol. 25, 567–574. doi: 10.1007/s10811-012-9891-4
Gerard, V. A. (1997). The role of nitrogen nutrition in high-temperature tolerance of the kelp, Laminaria saccharina (Chromophyta). J. Phycol. 33, 800–810. doi: 10.1111/j.0022-3646.1997.00800.x
Gouvêa, L. P., Schubert, N., Martins, C. D. L., Sissini, M., Ramlov, F., Rodrigues, E. R. D. O., et al. (2017). Interactive effects of marine heatwaves and eutrophication on the ecophysiology of a widespread and ecologically important macroalga. Limnol. Oceanogr. 62, 2056–2075. doi: 10.1002/lno.10551
Graham, M. H. (2004). Effects of local deforestation on the diversity and structure of southern California giant kelp forest food webs. Ecosystems 7, 341–357.
Hanelt, D. (1998). Capability of dynamic photoinhibition in Arctic macroalgae is related to their depth distribution. Mar. Biol. 131, 361–369. doi: 10.1007/s002270050329
Hargrave, M. S., Foggo, A., Pessarrodona, A., and Smale, D. A. (2017). The effects of warming on the ecophysiology of two co-existing kelp species with contrasting distributions. Oecologia 183, 531–543. doi: 10.1007/s00442-016-3776-1
Hay, K. B., Millers, K. A., Poore, A. G., and Lovelock, C. E. (2010). The use of near infrared reflectance spectrometry for characterization of brown algal tissue. J. Phycol. 46, 937–946. doi: 10.1111/j.1529-8817.2010.00890.x
Hörtensteiner, S., and Feller, U. (2002). Nitrogen metabolism and remobilization during senescence. J. Exper. Bot. 53, 927–937. doi: 10.1093/jexbot/53.370.927
Hurd, C. L., Harrison, P. J., Bischof, K., and Lobban, C. S. (2014). Seaweed Ecology and Physiology. Cambridge: Cambridge University Press.
IPCC (2013). “Climate change 2013: the physical science basis,” in Contribution of Working Group I to the Fifth Assessment Report of the Intergovernmental Panel on Climate Change (IPCC), eds T. F. Stocker, D. Qin, G.-K. Plattner, M. Tignor, S. K. Allen, J. Boschung, et al. (Cambridge: Cambridge University Press).
Kamiya, M., Nishio, T., Yokoyama, A., Yatsuya, K., Nishigaki, T., Yoshikawa, S., et al. (2010). Seasonal variation of phlorotannin in sargassacean species from the coast of the Sea of Japan. Phycol. Res. 58, 53–61. doi: 10.1111/j.1440-1835.2009.00558.x
Kawai, H., Akita, S., Hashimoto, K., and Hanyuda, T. (2020). A multigene molecular phylogeny of Eisenia reveals evidence for a new species, Eisenianipponica (Laminariales), from Japan. Eur. J. Phycol. 55, 234–241. doi: 10.1080/09670262.2019.1692911
Krumhansl, K. A., and Scheibling, R. E. (2012). Production and fate of kelp detritus. Mar. Ecol. Prog. Ser. 467, 281–302. doi: 10.3354/meps09940
Kurashima, A., Yokohama, Y., and Aruga, Y. (1996). Physiological characteristics of Eisenia bicyclis Setchell and Ecklonia cava Kjellman (Phaeophyta). Jpn. J. Phycol. 44, 87–94.
Li, J. Y., Agatsuma, Y., Nagai, T., Sato, Y., and Taniguchi, K. (2009). Differences in resource storage pattern between Laminaria longissima and Laminaria diabolica (Laminariaceae; Phaeophyta) reflecting their morphological characteristics. J. Appl. Phycol. 21, 215–224. doi: 10.1007/s10811-008-9352-2
Lobban, C. S. (1978). Translocation of 14C in Macrocystis pyrifera (giant kelp). Plant Physiol. 61, 585–589. doi: 10.1104/pp.61.4.585
Lobell, D. B., Sibley, A., and Ortiz-Monasterio, J. I. (2012). Extreme heat effects on wheat senescence in India. Nat. Clim. Chang. 2, 186–189. doi: 10.1038/nclimate1356
Lüning, K., Schmitz, K., and Willenbrink, J. (1973). CO2 fixation and translocation in benthic marine algae. III. Rates and ecological significance of translocation in Laminaria hyperborea and L. saccharina. Mar. Biol. 23, 275–281. doi: 10.1007/bf00389334
Mabin, C. J., Johnson, C. R., and Wright, J. T. (2019). Physiological response to temperature, light, and nitrates in the giant kelp Macrocystis pyrifera from Tasmania, Australia. Mar. Ecol. Prog. Ser. 614, 1–19. doi: 10.3354/meps12900
Maegawa, M., Yokohama, Y., and Aruga, Y. (1987). Critical light conditions for young Ecklonia cava and Eisenia bicyclis with reference to photosynthesis. Hydrobiologia 151–152, 447–455. doi: 10.1007/bf00046166
Murata, N., Takahashi, S., Nishiyama, Y., and Allakhverdiev, S. I. (2007). Photoinhibition of photosystem II under environmental stress. Biochim. Biophys. Acta Bioenerget. 1767, 414–421. doi: 10.1016/j.bbabio.2006.11.019
Pintó-Marijuan, M., and Munné-Bosch, S. (2014). Photo-oxidative stress markers as a measure of abiotic stress-induced leaf senescence: advantages and limitations. J. Exper. Bot. 65, 3845–3857. doi: 10.1093/jxb/eru086
Poore, A. G., Graba-Landry, A., Favret, M., Brennand, H. S., Byrne, M., and Dworjanyn, S. A. (2013). Direct and indirect effects of ocean acidification and warming on a marine plant-herbivore interaction. Oecologia 173, 1113–1124. doi: 10.1007/s00442-013-2683-y
Ragan, M. A., and Glombitza, K. W. (1986). Phlorotannins, brown algal polyphenols. Prog. Phycol. Res. 4, 129–241. doi: 10.1016/j.fitote.2013.02.013
Sato, Y., and Agatsuma, Y. (2016). Resource accumulation of the kelp Saccharina ochotensis based on photosynthetic rate and specific nutrient uptake kinetics. J. Appl. Phycol. 28, 499–509. doi: 10.1007/s10811-015-0578-5
Schiel, D. R., and Foster, M. S. (2006). The population biology of large brown seaweeds: ecological consequences of multiphase life histories in dynamic coastal environments. Annu. Rev. Ecol. Evol. Syst. 37, 343–372. doi: 10.1146/annurev.ecolsys.37.091305.110251
Schneider, C. A., Rasband, W. S., and Eliceiri, K. W. (2012). NIH Image to ImageJ: 25 years of image analysis. Nat. Methods 9, 671–675. doi: 10.1038/nmeth.2089
Smale, D. A. (2020). Impacts of ocean warming on kelp forest ecosystems. New Phytol. 225, 1447–1454. doi: 10.1111/nph.16107
Steneck, R. S., Graham, M. H., Bourque, B. J., Corbett, D., Erlandson, J. M., Estes, J. A., et al. (2002). Kelp forest ecosystems: biodiversity, stability, resilience and future. Environ. Conserv. 29, 436–459. doi: 10.1017/s0376892902000322
Suzuki, H., Aoki, T., Inomata, E., Agatsuma, Y., and Aoki, M. N. (2020). Effect of breakwater restoration work following the subsidence caused by the 2011 Tohoku Earthquake on the subtidal kelp population. Phycol. Res. doi: 10.1111/pre.12376
Suzuki, Y., Miyamoto, T., Yoshizawa, R., Mae, T., and Makino, A. (2009). Rubisco content and photosynthesis of leaves at different positions in transgenic rice with an overexpression of RBCS. Plant Cell Environ. 32, 417–427. doi: 10.1111/j.1365-3040.2009.01937.x
Takahashi, S., and Badger, M. R. (2011). Photoprotection in plants: a new light on photosystem II damage. Trends Plant Sci. 16, 53–60. doi: 10.1016/j.tplants.2010.10.001
Tatewaki, M. (1966). Formation of a crustaceous sporophyte with unilocular sporangia in Scytosiphon lomentaria. Phycologia 6, 62–66. doi: 10.2216/i0031-8884-6-1-62.1
Tegner, M. J., Dayton, P. K., Edwards, P. B., and Riser, K. L. (1997). Large-scale, low-frequency oceanographic effects on kelp forest succession: a tale of two cohorts. Mar. Ecol. Prog. Ser. 146, 117–134. doi: 10.3354/meps146117
Terada, R., Abe, M., Abe, T., Aoki, M., Dazai, A., Endo, H., et al. (2020). Japan’s nationwide long-term monitoring survey of seaweed communities known as the “Monitoring Sites 1000”: Ten-year overview and future perspectives. Phycol. Res. doi: 10.1111/pre.12395
tom Dieck, I. (1993). Temperature tolerance and survival in darkness of kelp gametophytes (Laminariales, Phaeophyta): ecological and biogeographical implications. Mar. Ecol. Prog. Ser. 100, 253–253. doi: 10.3354/meps100253
Van Alstyne, K. L. (1995). Comparison of three methods for quantifying brown algal polyphenolic compounds. J. Chem. Ecol. 21, 45–58. doi: 10.1007/bf02033661
Wernberg, T., Smale, D. A., Tuya, F., Thomsen, M. S., Langlois, T. J., De Bettignies, T., et al. (2013). An extreme climatic event alters marine ecosystem structure in a global biodiversity hotspot. Nat. Clim. Chang. 3, 78–82. doi: 10.1038/nclimate1627
Wu, T., Qu, C., Li, Y., Li, X., Zhou, G., Liu, S., et al. (2019). Warming effects on leaf nutrients and plant growth in tropical forests. Plant Ecol. 220, 663–674. doi: 10.1007/s11258-019-00943-y
Keywords: climate change, non-additive effect, high-temperature tolerance, foundation species, kelp
Citation: Endo H, Inomata E, Gao X, Kinoshita J, Sato Y and Agatsuma Y (2020) Heat Stress Promotes Nitrogen Accumulation in Meristems via Apical Blade Erosion in a Brown Macroalga With Intercalary Growth. Front. Mar. Sci. 7:575721. doi: 10.3389/fmars.2020.575721
Received: 24 June 2020; Accepted: 07 September 2020;
Published: 25 September 2020.
Edited by:
Manoj Kumar, University of Technology Sydney, AustraliaReviewed by:
Pushp Sheel Shukla, Dalhousie University, CanadaAlok Arun, KU Leuven, Belgium
Narendra Singh Yadav, University of Lethbridge, Canada
Asish Kumar Parida, Central Salt & Marine Chemicals Research Institute (CSIR), India
Copyright © 2020 Endo, Inomata, Gao, Kinoshita, Sato and Agatsuma. This is an open-access article distributed under the terms of the Creative Commons Attribution License (CC BY). The use, distribution or reproduction in other forums is permitted, provided the original author(s) and the copyright owner(s) are credited and that the original publication in this journal is cited, in accordance with accepted academic practice. No use, distribution or reproduction is permitted which does not comply with these terms.
*Correspondence: Hikaru Endo, h-endo@fish.kagoshima-u.ac.jp
†Present address: Hikaru Endo, Faculty of Fisheries, Kagoshima University, Kagoshima, Japan; United Graduate School of Agricultural Sciences, Kagoshima University, Kagoshima, Japan; Xu Gao, The Swire Institute of Marine Science, School of Biological Sciences, The University of Hong Kong, Hong Kong, China; Junji Kinoshita, Pelagic Fish Resources Division, Fisheries Stock Assessment Center, Fisheries Resources Institute, Japan Fisheries Research and Education Agency, Kanagawa, Japan