- 1Department of Marine Sciences–Tjärnö, University of Gothenburg, Strömstad, Sweden
- 2Marine Evolutionary Ecology, GEOMAR Helmholtz Centre for Ocean Research Kiel, Kiel, Germany
The temperate seagrass species eelgrass Zostera marina can be infected by the wasting disease pathogen Labyrinthula zosterae, which is believed to have killed about 90% of the seagrass in the Atlantic Ocean in the 1930s. It is not known why this opportunistic pathogen sometimes becomes virulent, but the recurrent outbreaks may be due to a weakening of the Z. marina plants from adverse environmental changes. This study investigated the individual and interactive effects of multiple extrinsic factors (temperature, light, and tissue damage) on the host-pathogen interaction between Z. marina and L. zosterae in a fully crossed infection experiment. The degree of infection was measured as both lesion coverage and L. zosterae cell concentration. We also investigated if the treatment factors affect the chemical defense of the host, measured as the inhibitory capacity of seagrass extracts in bioassays with L. zosterae. Finally, gene expression of a set of targeted genes was quantified in order to investigate how the treatments change Z. marina’s response to infection. Light had a pronounced effect on L. zosterae infection measured as lesion coverage, where reduced light conditions increased lesions by 35%. The response to light on L. zosterae cell concentration was more complex and showed significant interaction with the temperature treatment. Cell concentration was also significantly affected by physical damage, where damage surprisingly resulted in a reduced cell concentration of the pathogen. No treatment factor caused detectable decrease in the inhibitory capacity of the seagrass extracts. There were several interactive effects between L. zosterae infection and the treatment factors on Z. marina growth, and on the expression of genes associated with immune defense, phenol synthesis and primary metabolism, showing that the molecular reaction toward L. zosterae infection depends on prevailing environmental conditions. Our study shows that individual or interactive effects of light, temperature and tissue damage can affect multiple aspects of host-pathogen interactions in seagrasses. These results highlight the complexity of marine host-pathogen systems, showing that more multi-factorial investigations are needed to gain a better understanding of disease in marine plants under different environmental conditions.
Introduction
Seagrass meadows are habitats dominated by one or several species of seagrasses, and they constitute one of the most important types of coastal marine ecosystems globally (Costanza et al., 2014). The meadows act as nursery grounds for commercially important fish and shellfish species (Orth et al., 1984), function as coastal protection (Barbier et al., 2011), and sequester large amounts of carbon (Duarte et al., 2005). During the last decades seagrass populations have declined sharply worldwide (Waycott et al., 2009), due to multiple stressors such as sediment and nutrient runoff, physical disturbance, invasive species, commercial fishing practices, aquaculture, overgrazing, algal blooms, global warming, and disease (Orth et al., 2006).
The dominant seagrass species in the northern hemisphere, Zostera marina L. or eelgrass, is regularly infected by the endophytic pathogen Labyrinthula zosterae Porter and Muehlstein (1991) (Bockelmann et al., 2013; Groner et al., 2016; Jakobsson-Thor et al., 2018). The pathogen is a colony-forming stramenopile that causes black necrotic lesions on its host plant, and is likely the causative organism responsible the wasting disease outbreaks in the 1930s, when about 90% of the Atlantic populations of Z. marina vanished over a span of a few years (reviewed by Sullivan et al., 2013). Labyrinthula spp. has since been detected in areas with seagrass decline on a smaller scale in e.g., New Zealand in the 1960s (Armiger, 1964), and on the north-eastern coast of the United States in the 1980s (Muehlstein et al., 1988). Presently, L. zosterae and other pathogenic Labyrinthula spp. are omnipresent in seagrass populations around the world (Martin et al., 2016), but it is unknown why they sometimes become virulent pathogens. The recurrent virulent outbreaks of L. zosterae may be due to the weakening of the Z. marina plants because of adverse environmental changes (e.g., Tutin, 1938). Stressors caused by a changing environment, e.g., heat stress, reduced light and salinity, and lowered pH, are all factors that potentially can increase pathogen susceptibility of an organism (Chakraborty et al., 2000; Anderson et al., 2004).
It is well known that plants resist pathogens through production of chemical defenses (Levin, 1976; Bennett and Wallsgrove, 1994), but in comparison to many terrestrial plants the chemical ecology of seagrasses is vastly understudied. Many marine organisms, including seagrasses, are chemically defended against herbivores and pathogens (Hay and Fenical, 1988; Pavia et al., 2012; Zidorn, 2016), and extracts from Z. marina can inhibit L. zosterae growth (Jakobsson-Thor et al., 2018). Furthermore, external stressors can have an impact on seagrass metabolite production, e.g., increased temperature and reduced light intensity both affect the concentration of phenolic acids in Z. marina (Vergeer et al., 1995). However, it is unknown if and how environmental stressors also affect the production of the compounds that are inhibitory against L. zosterae (Jakobsson-Thor et al., 2018). Plant resistance to pathogens consists of multiple defensive strategies, often including the activation of several defense-related genes (Dangl et al., 2013), and laboratory experiments show that L. zosterae infection can alter Z. marina’s expression of genes connected to a metabolic defense cascade (Brakel et al., 2014).
The influence of temperature on wasting disease dynamics has been poorly investigated, and most data on temperature effects are correlational (Rasmussen, 1977; Giesen et al., 1990; Bull et al., 2012). For example, mass mortality of eelgrass caused by wasting disease in Denmark during the 1930s was connected to unusually high water temperatures (Rasmussen, 1977). Furthermore, temperature correlates with L. zosterae abundance in natural Z. marina populations, and the pathogen is more active during warmer months of the year (Bockelmann et al., 2013). Increased summer temperature has, however, been reported to reduce lesion size caused by a pathogenic Labyrinthula sp. in laboratory experiments on the Mediterranean seagrass species Posidonia oceanica Delile (1813) and Cymodocea nodosa Acherson (1870) (Olsen and Duarte, 2015; Olsen et al., 2015).
A correlation between L. zosterae infection rate and water depth has been detected in natural Z. marina meadows (Groner et al., 2014; Jakobsson-Thor et al., 2018). Shoots growing in shallower waters, and consequently with higher light availability, have a higher degree of L. zosterae infection compared to deeper populations (Groner et al., 2014; Jakobsson-Thor et al., 2018). In contrast, petri dish experiments show increased lesions coverage on Z. marina leaves subjected to complete darkness compared to pieces kept at a diel light cycle (Dawkins et al., 2018). However, laboratory experiments with whole Z. marina shoots and artificial lights found no change in lesion size or L. zosterae cell concentrations (Brakel et al., 2019). Additional experimental studies at ecologically relevant light levels and with intact Z. marina shoots are needed to determine how light can affect L. zosterae infection in Z. marina.
Tissue wounding caused by physical/mechanical damage is correlated to increased risk of pathogen infection in terrestrial plants (Maleck and Dietrich, 1999), but this issue has not been addressed for seagrasses with one exception. In a study on Thalassia testudinum Koenig (1805), in Florida Bay, Bowles and Bell (2004) showed that plants subjected to simulated herbivory were more likely to be infected by Labyrinthula sp. The Swedish northwestern coast is a popular recreational boating area, and 74% of the docks and 91% of the marinas in this area are located in Z. marina meadows (Eriander, 2016). The effect of boat traffic and risk of damage by boat propellers on Z. marina plants is therefore potentially high, and shoots with damaged leaves are frequent in some highly trafficked areas (personal observations by Jakobsson-Thor, 2017).
Most previous studies have focused on how seagrass-pathogen interactions are affected by a single external factor, even though coastal areas can be subjected to changes in several environmental factors (e.g., temperature and light) simultaneously (e.g., Pérez and Romero, 1992). Only two studies have, to our knowledge, specifically aimed at investigating interactive effects between two or several factors (Bishop et al., 2017; Dawkins et al., 2018). Investigations of salinity, temperature, sulfide, and night-time hypoxia showed that the interaction between ambient salinity and high sulfide concentrations results in larger Labyrinthula sp. lesions on T. testudinum (Bishop et al., 2017), whereas temperature and light have no interactive effect on lesion size caused by L. zosterae infection in Z. marina in Washington, United States (Dawkins et al., 2018). This interaction was not detected in a laboratory experiment with L. zosterae and Z. marina in Germany, instead increased temperature in combination with low salinity reduced L. zosterae infection (Brakel et al., 2019). In general, interactive effects of climate change and infectious diseases have not been well studied (Sullivan et al., 2018), and more complex studies are needed to predict future outcomes of climate change on pathogen outbreaks.
The overall aim of this study was to investigate how individual and interactive effects of temperature, light, and tissue damage affect L. zosterae infection in Z. marina in a fully crossed infection experiment. The degree of infection was measured both as lesion coverage and as L. zosterae cell concentration. We also investigated if the treatment factors affect growth and the chemical defense (inhibitory capacity of tissue extracts) of the host, and the gene expression pattern of Z. marina in response to pathogen infection. We hypothesized that low light, increased temperature, and tissue damage would increase L. zosterae infection and reduce host resistance, and that interactions among these factors would have an additive or synergistic effect on L. zosterae infection in Z. marina.
Materials and Methods
Experimental Setup
The individual and interactive effects of temperature, light, tissue damage, and L. zosterae infection on Z. marina were investigated in an outdoor laboratory experiment. A total of 320 Z. marina shoots between 22 and 60 cm in length were haphazardly collected at 1–2 m depth from the Ide Fjord, Sweden, by snorkeling in August 2015 and transported to Tjärnö marine laboratory, Sweden. The sampling was carried out in meadows free from L. zosterae infection (Jakobsson-Thor et al., 2018). The shoots were planted into individual 6 L aquariums containing 600 mL sterilized sediment and connected to an outdoor flow-through seawater system (19–31 PSU, X̄ = 23 PSU). Surface seawater was pumped from 1.5 m depth, filtered (10 μm), and distributed to the individual aquariums. Four factors (temperature, light, tissue damage, and infection) with two levels each were manipulated in a fully factorial design (n = 20). Shoots received seawater at either ambient (19–20°C) or increased (25–26°C) temperatures, and light at ambient (approximately 600 μmol m–1 s–1) or reduced (approximately 100 μmol m–1 s–1) levels. The latter temperature and light treatments correspond to the expected maximum surface-seawater conditions in shallow bays on the northwest coast of Sweden during a heat wave (Infantes et al., 2016), and the measured irradiance at 5 m depth within Z. marina meadows located in the Tjärnö archipelago (Jakobsson-Thor et al., 2018). The incoming seawater for the increased temperature treatment was elevated and maintained by a sensory controlled heating system and thereafter distributed into the separate aquariums. Different light levels were achieved by placing one or several layers of fiber cloth above the aquariums. The natural light/dark cycle at Tjärnö, Sweden at the time of the experiment was approximate 14 h light:10 h dark. Shoots were acclimated to their temperature and light conditions for 1 week before the start of the experiment. Shoots receiving increased temperature were initially placed in seawater with ambient temperature that increased to the intended temperature over the course of 2 days. Treatments and replicates were randomized at the start of the experiment.
At the start of the experiment shoots were either left intact or had their leaves cut by scissors to simulate damages by boat propellers. One clean cut was made over all the leaves at approximately 10 cm from the tip of the longest leaf, resulting in all or most leaves of the plant being cut, but at different individual lengths. The L. zosterae strain for the infection treatment was isolated from Z. marina shoots collected in the Tjärnö archipelago in July 2015. To isolate L. zosterae, 3 cm pieces of Z. marina leaves with apparent lesions were surface sterilized in 0.5% sodium hypochlorite, rinsed in distilled water, soaked in filtered (0.2 μm) seawater, and finally placed upon serum seawater agar (SSA) plates. The SSA plates contained filtered (0.2 μm) seawater (30 PSU), 12 g L–1 agar, 1 g L–1 glucose L–1, 0.1 g L–1 peptone, 0.1 g L–1 yeast extract, and 3 mg L–1 germanium dioxide. The medium was autoclaved after which 10 ml L–1 horse serum and 25 ml L–1 streptomycin/penicillin (10,000 units penicillin and 10 mg streptomycin mL–1) were added. The SSA plates with L. zosterae (Figure 1A) cultures were incubated in the dark at 25°C. Inoculation of Z. marina plants in the experiment was performed by attaching L. zosterae-covered agar plugs, taken from the SSA plates (Ø = 5 mm), to the third youngest leaf with a small piece of silicone tubing. Agar plugs without L. zosterae were attached to non-inoculated shoots. All Z. marina shoots were photographed (Figure 1B) before and after the 7-day experiment. At the end of the experiment, Z. marina tissue samples were taken for measurements of gene expression, and the remaining parts of the shoots were placed in −80°C for further assessment of chemical defense and L. zosterae cell concentration (see paragraphs below).
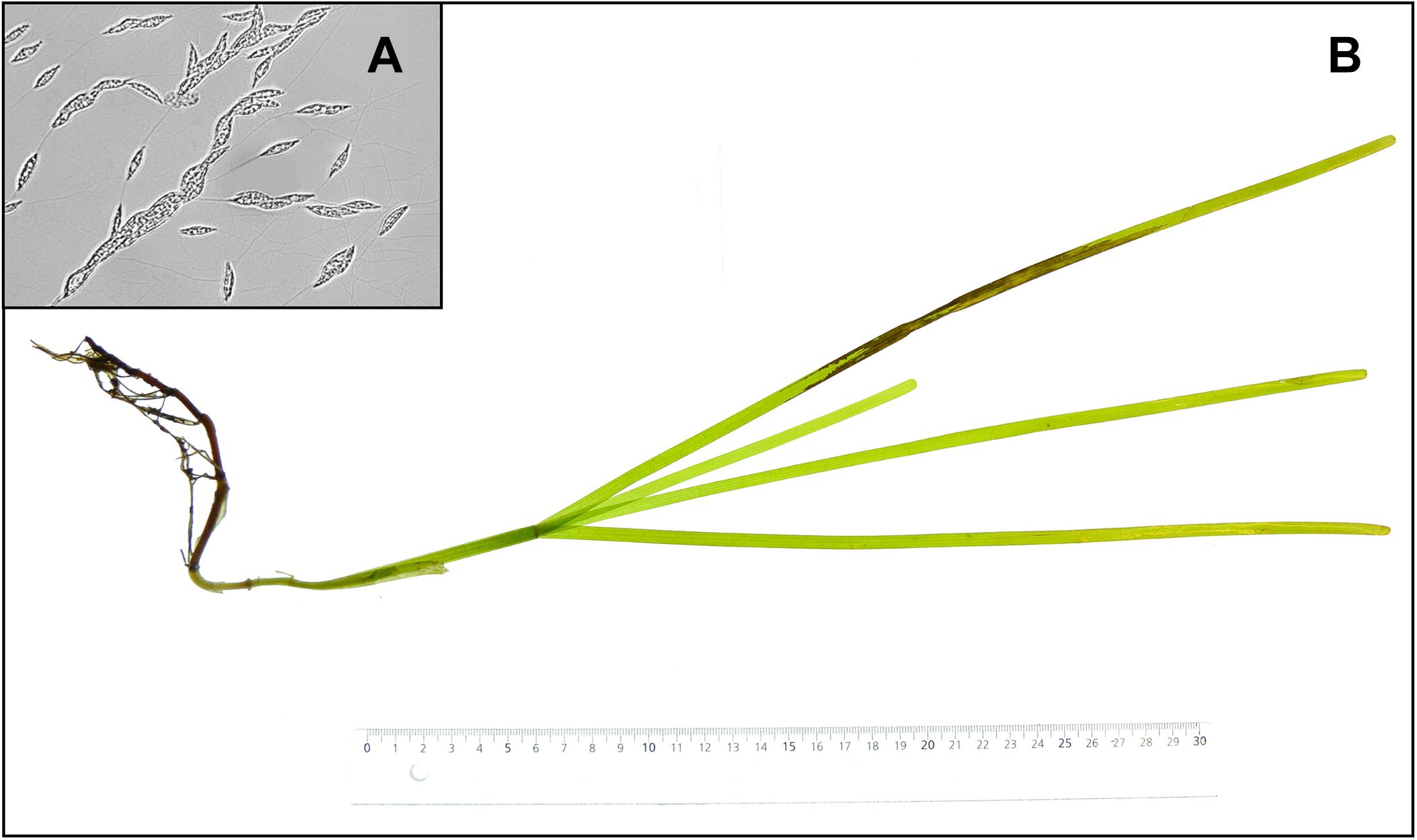
Figure 1. (A) Labyrinthula zosterae cells on an agar plate, (B) necrotic lesions on a Zostera marina shoot following inoculation with L. zosterae.
Zostera marina Growth and Lesion Coverage
ImageJ software was used to measure Z. marina growth (% of initial shoot area, n = 20) and L. zosterae disease symptoms, i.e., lesion coverage (% of final shoot area, n = 20) from the photographs taken before and after the experiment.
Labyrinthula zosterae Concentration
The pathogen load was investigated by measuring the L. zosterae cell concentration in seagrass tissue by extracting L. zosterae DNA from the whole shoots (n = 20) at the end of the experiment. Each sample was freeze-dried and homogenized with a mortar and pestle, after which 2–3 mg of each sample was extracted for L. zosterae DNA using the Invisorb Spin Plant Mini Kit (Stratec Molecular, Berlin, Germany) according to the manufacturers protocol, with the exception that 1 μL salmon sperm (Invitrogen, Waltham, MA, United States) was added to each extraction to saturate the silica columns with untargeted DNA. DNA was eluted in 100 μL elution buffer and the samples were analyzed using real-time quantitative PCR (RT-qPCR) performed on a StepOnePlus qPCR machine (Applied Biosystems, Waltham, MA, United States) as previously described by Bockelmann et al. (2013). Each sample ran in technical triplicates, and cycle threshold (CT) was calculated with a fixed threshold of 0.06. A standard curve with known L. zosterae concentrations of 0.75 cells μL–1 (CT: 36.75 ± 1.21 mean ± SD), 7.5 cells μL–1 (CT: 32.87 ± 1.04 mean ± SD), 75 cells μL–1 (CT: 29.23 ± 1.04 mean ± SD), and 750 cells μL–1 (CT: 24.59 ± 0.95 mean ± SD) ran along the samples on each plate. Samples with CT values over 39, or where the standard variation of the triplicates exceeded 0.5, were excluded from further analysis.
Chemical Defense Assay
Eelgrass shoots of each treatment (n = 15) were used to investigate if environmental stressors affect Z. marina’s production of inhibitory compounds against L. zosterae. The shoots were freeze-dried and homogenized, and individually extracted in methanol and dichloromethane 1:1 for 1 h on a shaker table. The samples were diluted to 1/16 volumetric concentration to better detect inhibitory differences between extracts from different shoots, since all Z. marina extracts at natural concentration strongly inhibit L. zosterae growth (Jakobsson-Thor et al., 2018). The plant material was filtered (1.6 μm) and solvents were evaporated using a SpeedVac.
The inhibitory effects of the Z. marina extracts on the pathogen was tested in a modified L. zosterae growth assay first described by Martin et al. (2009). Each sample was redissolved in 0.7 mL liquid growth media (SSA without agar, see above) containing 1% dimethyl sulfoxide. The samples were transferred to six well plates (Ø = 35 mm, Thermo Fisher Scientific, Waltham, MA, United States), and L. zosterae covered plugs (Ø = 7 mm) taken from the edge of the L. zosterae cultures growing on SSA plates were placed in the center of each well. L. zosterae used in the growth assays was isolated from Z. marina shoots collected in the Tjärnö archipelago in August 2016 as described in ‘Experimental setup’ above. Wells containing growth media without Z. marina extracts (n = 48) served as controls. The well plates were sealed with Parafilm and incubated at 25°C in the dark. After 11 h the size of the L. zosterae colonies were outlined with a marker and the well plates photographed. The total area of the colonies was measured using ImageJ software.
Gene Expression
A 5 cm piece from the middle part of the fourth leaf of each shoot (n = 6) was placed in RNA-later (Thermo Fisher Scientific, Waltham, MA, United States) at the end of the experiment for gene expression measurements. We chose to analyze the middle section of fourth leaf (adjacent to the originally inoculated leaf) for gene expression, thus avoiding the mostly necrotic tissue (i.e., lesions caused through L. zosterae infection) of the originally inoculated leaf. RNA was extracted with the Invitrap® Spin Plant RNA Mini kit (Stratec Molecular, Berlin, Germany) according to the manufactures protocol. Next, cDNA was synthesized using qScriptTM cDNA synthesis kit (Quanta Biosciences, Beverly, MA, United States) in combination with DNA wipe-out treatment by PerfeCTa® DNase I treatment (Quanta Biosciences, Beverly, MA, United States).
Fourteen target genes that previously have been investigated in connection with reduced light intensity, increased temperature, and L. zosterae infection (Bergmann et al., 2010; Winters et al., 2011; Brakel et al., 2014, 2017; Salo et al., 2015) were selected for gene expression analysis, along with development of two new primer pairs to target the phenol synthesis. See Table 1 for a summary of the genes and their function, and for further details on the newly developed primers.
Gene expression was measured with a Biomark HD System (Fluidigm, South San Francisco, CA, United States) on a 96.96 Dynamic Array IFC chip according to published protocols (Salo et al., 2015). Each primer pair ran in technical triplicates. If the standard deviation of a triplicate was >0.5 the value was excluded from further analysis. To normalize gene expression data five reference genes were chosen based on an algorithm geNorm implemented in the software qbase+ (Biogazelle, Zwijnaarde, Belgium). The results were cross validated by two additional algorithms, Bestkeeper (Pfaffl et al., 2004), and NormFinder (Andersen et al., 2004), see Supplementary Table S1. Based on the combined results we used 10kDa, SOD, APX, GRFb, and ubiquitin as reference genes. The cycle threshold (Ct) values of target genes were normalized with the geometric mean of the Ct of reference targets. The relative gene expression levels were calculated as: −ΔCT = Geometric mean of reference genes (CT)–CT (target gene).
Statistical Analyses
Lesion coverage (% of total shoot area) and L. zosterae cell concentration (no. of L. zosterae cells mg–1 Z. marina dry weight) were statistically analyzed in SuperANOVA (ABACUS Concepts) statistical software using three-way analysis of variance (ANOVA) with temperature (two levels), light (two levels), and tissue damage (two levels) as fixed, orthogonal factors.
Growth of Z. marina shoots (% growth of initial shoot size) and the inhibitory effect of the extracted Z. marina metabolites on L. zosterae growth (% growth of control) in the bioassays were analyzed using four-way ANOVA with temperature (two levels), light (two levels), tissue damage (two levels), and infection (two levels) as fixed, orthogonal factors.
Relative gene expression values (−ΔCT) were statistically analyzed using permutational multivariate ANOVA (Permanova) for each functional gene group (general stress genes and redox and detoxification genes, genes associated to immune response, genes of the phenol synthesis, and genes of primary metabolism), with infection (two levels), temperature (two levels), light (two levels), and tissue damage (two levels) as fixed orthogonal factors. Permanova was conducted, using Euclidian distances and 999 permutations of the package vegan (Oksanen et al., 2016), within R version 3.1.2. In order to illustrate the results of the gene expression for the different treatments a heat map was created using the mean −ΔCT values. Principal component analyses (PCA) were performed for each gene group with significant treatment effects in the Permanova, using the ade4 package (Dray and Dufour, 2007) in R (version 3.3.1). The expression of individual genes in comparison to undamaged, non-inoculated shoots at ambient conditions of light and temperature (hereafter referred to as ambient control) is reported as relative gene expression, −ΔΔCT = −ΔCT−(−ΔCT (ambient control)).
Prior to all statistical analyses, data was tested for homogeneity of variances with a Cochran’s test, and transformed when required. Data on L. zosterae growth in the chemical defense assays were square root transformed prior to statistical analysis. However, the order of the mean values changed after transformation for data on Z. marina growth. Due to the large number of groups and replicates, ANOVA is robust against heterogeneous variances (Underwood, 1997) and therefore, the data was not transformed. Multiple comparisons of means were performed using the Student–Newman–Keuls (SNK) procedure.
Results
Labyrinthula zosterae Lesion Coverage and Cell Concentration
All Z. marina shoots developed lesions when inoculated with L. zosterae cells, whereas no lesions were detected on non-inoculated shoots. After the 7 days experiment lesions covered large parts of the inoculated leaf, but also spread to a smaller extent to other leaves within the shoots. There was no significant interaction between the treatment factors (Table 2). However, reduced light conditions resulted in a significant increase of lesion coverage (Table 2), with shoots in low light treatments showing 35.0% ± 0.7 SE (mean ± SE) higher lesion coverage compared to shoots under ambient light (Figure 2). Increased temperature and tissue damage had no significant effect on lesion coverage (Table 2).
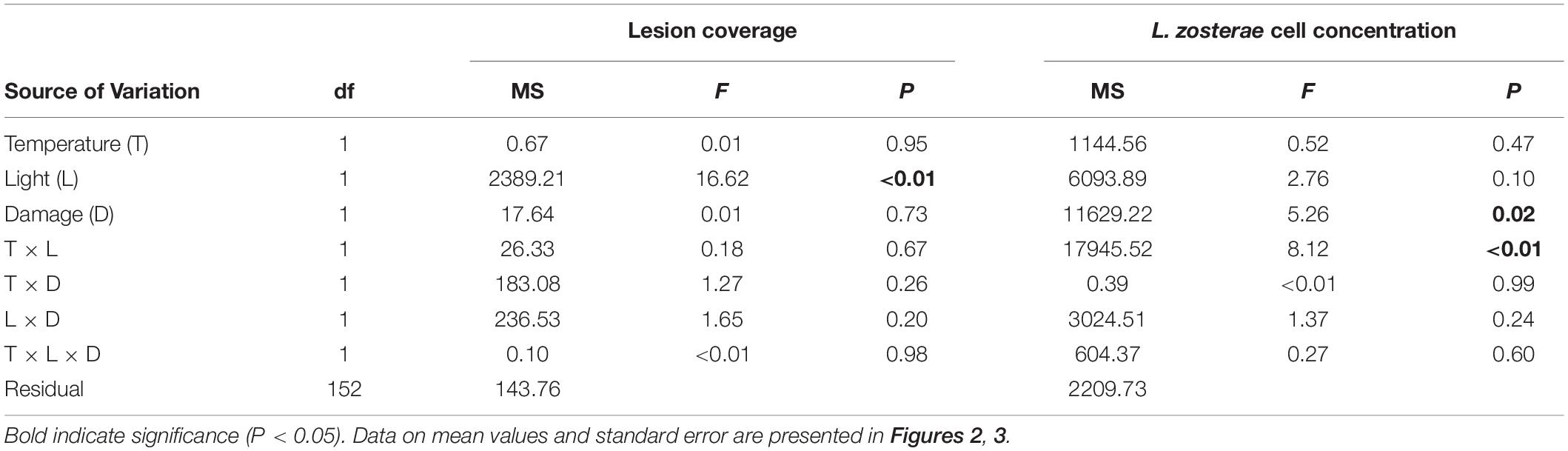
Table 2. Analysis of variance of lesion coverage (%) and Labyrinthula zosterae cell concentration (cells mg–1 Zostera marina dry weight) in Z. marina grown under different levels and combinations of temperature (ambient and increased), light (reduced and ambient), and tissue damage (damaged and undamaged).
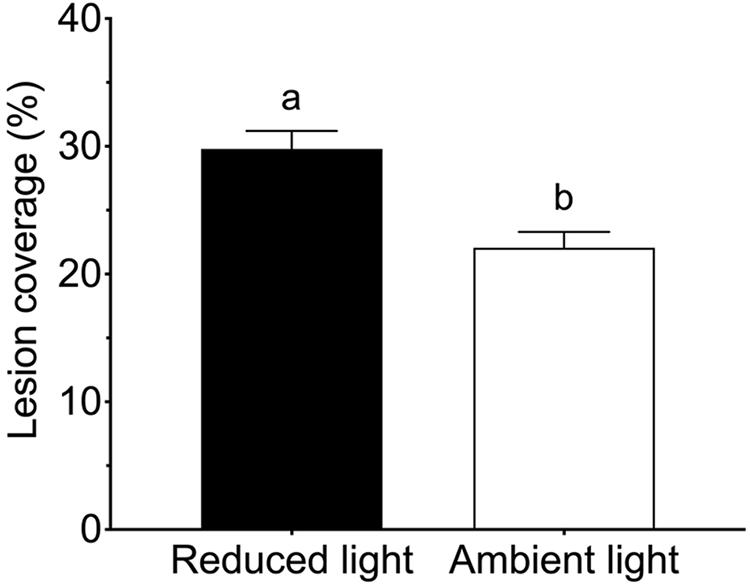
Figure 2. Lesion coverage (% of total area) following inoculation by Labyrinthula zosterae on Zostera marina shoots exposed to ambient and reduced light intensity. Different letters above bars indicate significant differences between treatments based on the Student–Newman–Keuls test (P < 0.05). Only significant results (see Table 2) are included in the figure and not all interactions. The non-significant treatments are therefore pooled giving a higher replication than 20 replicates per treatment. Error bars show standard error, n = 80.
Reduced light conditions significantly (SNK, P < 0.05) increased the L. zosterae cell concentration in Z. marina, but only under ambient temperatures, as shown by the significant interaction between the main factors light and temperature (Table 2). Shoots experiencing ambient temperature showed a slightly higher concentration of L. zosterae cells following infection under reduced light conditions compared to shoots kept at ambient light (Figure 3A). At increased temperatures, there was no significant (SNK, P > 0.05) difference in L. zosterae cell concentration between shoots exposed to different light levels. Contrary to our hypothesis, tissue damage had a significant negative effect on L. zosterae cell concentration in Z. marina leaves (Table 2 and Figure 3B).
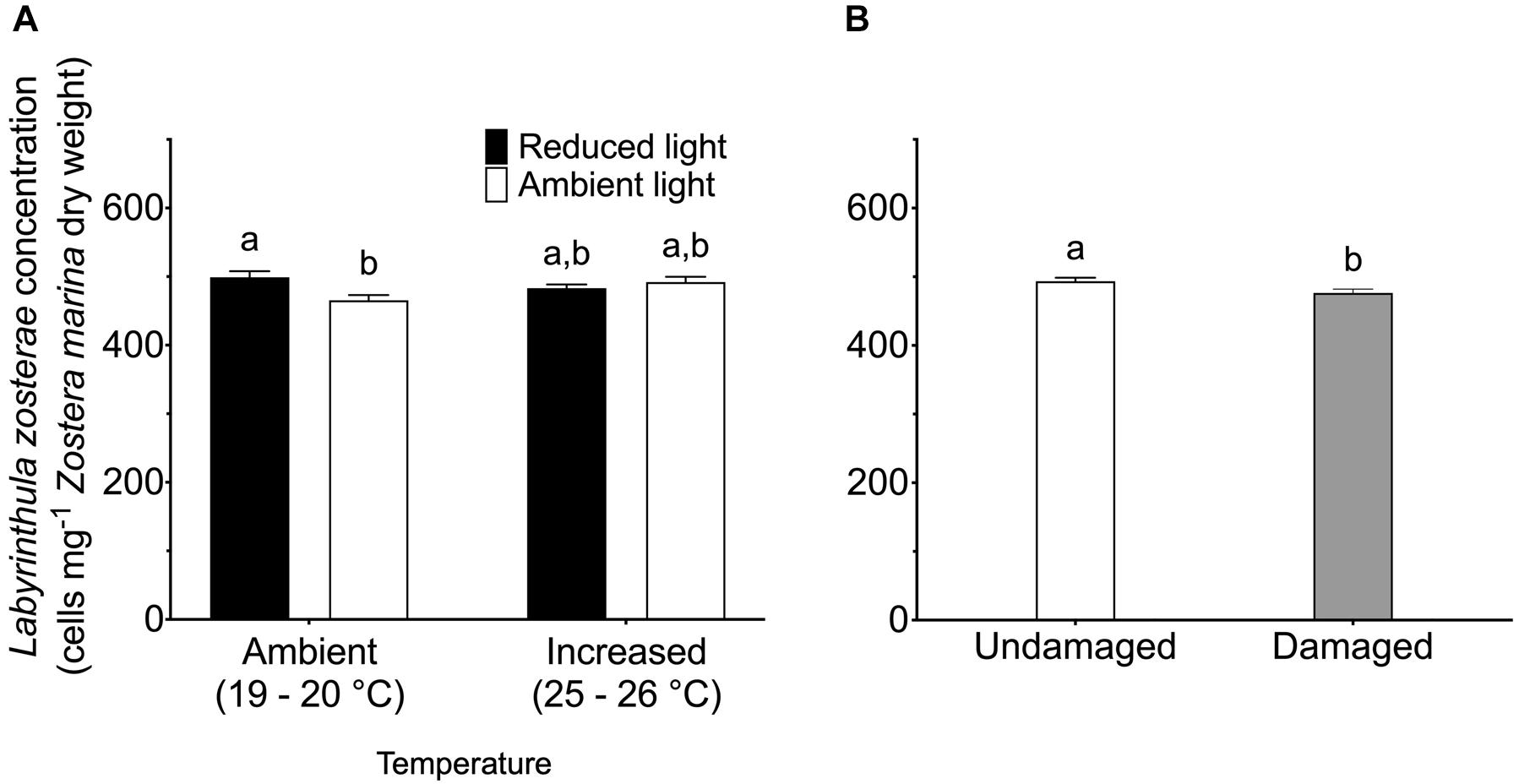
Figure 3. Labyrinthula zosterae cell concentration (cells mg– 1 Zostera marina dry weight) following inoculation by L. zosterae on Z. marina shoots (A) exposed to ambient or increased temperature and to ambient or reduced light intensity (n = 40), and (B) with damaged or undamaged tissue (n = 80). Different letters above bars indicate significant differences between treatments based on the Student–Newman–Keuls test (P < 0.05). Only significant results (see Table 2) are included in the figure and not all interactions. The non-significant treatments are therefore pooled giving a higher replication than 20 replicates per treatment. Error bars show standard error.
Zostera marina Growth
Eelgrass growth was observed under all treatment conditions and varied between 8 and 33% of the initial shoot area. When data on Z. marina growth was analyzed, we found a significant interaction between the factors damage and temperature (Table 3). Damaged Z. marina shoots grew significantly (SNK, P < 0.05) more (58.9 ± 2.1% mean ± SE) compared to undamaged shoots under ambient temperature, whereas only a trend toward a similar result was found at increased temperature (Figure 4A). Furthermore, we found a significant interaction between the factors light, temperature, and infection on Z. marina growth (Table 3). Highest growth was observed for non-inoculated Z. marina shoots exposed to increased temperature under ambient light conditions (Figure 4B). However, there was no significant (SNK, P > 0.05) difference in growth between inoculated and non-inoculated Z. marina shoots exposed to different temperatures under reduced light conditions (Figure 4B).
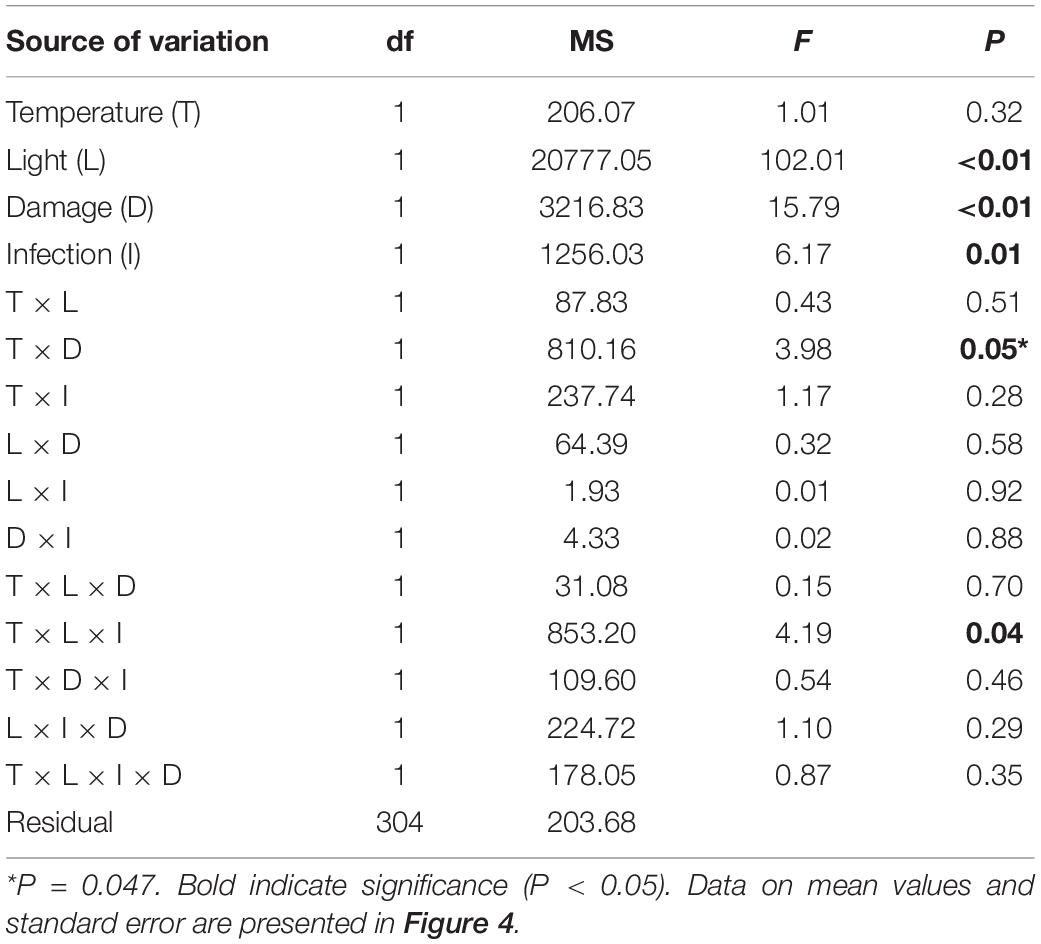
Table 3. Analysis of variance of Zostera marina growth (% growth relative to initial size) under different levels and combinations of temperature (ambient and increased), light (reduced and ambient), tissue damage (damaged and undamaged), and Labyrinthula zosterae infection (non-inoculated and inoculated).
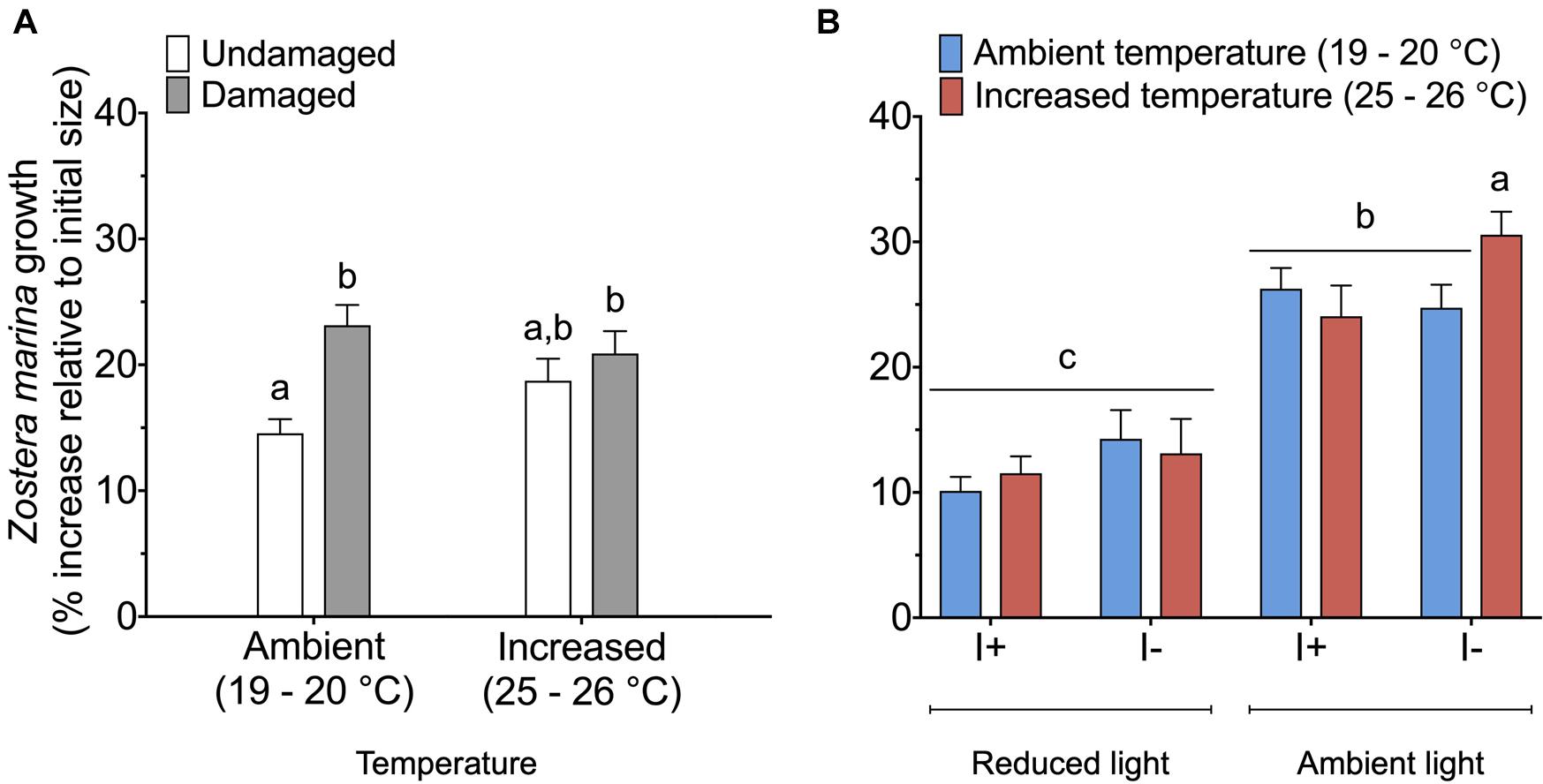
Figure 4. Growth (% increase relative to initial size) of Zostera marina exposed to (A) ambient or increased temperature, and damaged or undamaged tissue (n = 80), and (B) ambient or increased temperature, ambient or reduced light, and inoculation or non-inoculation of Labyrinthula zosterae (n = 40). I+ = inoculated, I– = non-inoculated. Different letters above bars indicate significant difference between treatments based on the Student–Newman–Keuls test (P < 0.05). Only significant results (see Table 3) are included in the figures and not all interactions. The non-significant treatments are therefore pooled giving a higher replication than 20 replicates per treatment. Error bars show standard error.
Chemical Defense Assay
The statistical analysis of data on the inhibitory effect of Z. marina extracts on L. zosterae growth revealed a significant interaction between the factors tissue damage and temperature (Table 4). The inhibitory effect on L. zosterae growth was significantly (SNK, P < 0.05) higher for extracts from undamaged Z. marina shoots exposed to ambient temperature compared to undamaged shoots at increased temperature or damaged shoots at ambient temperature (Figure 5).
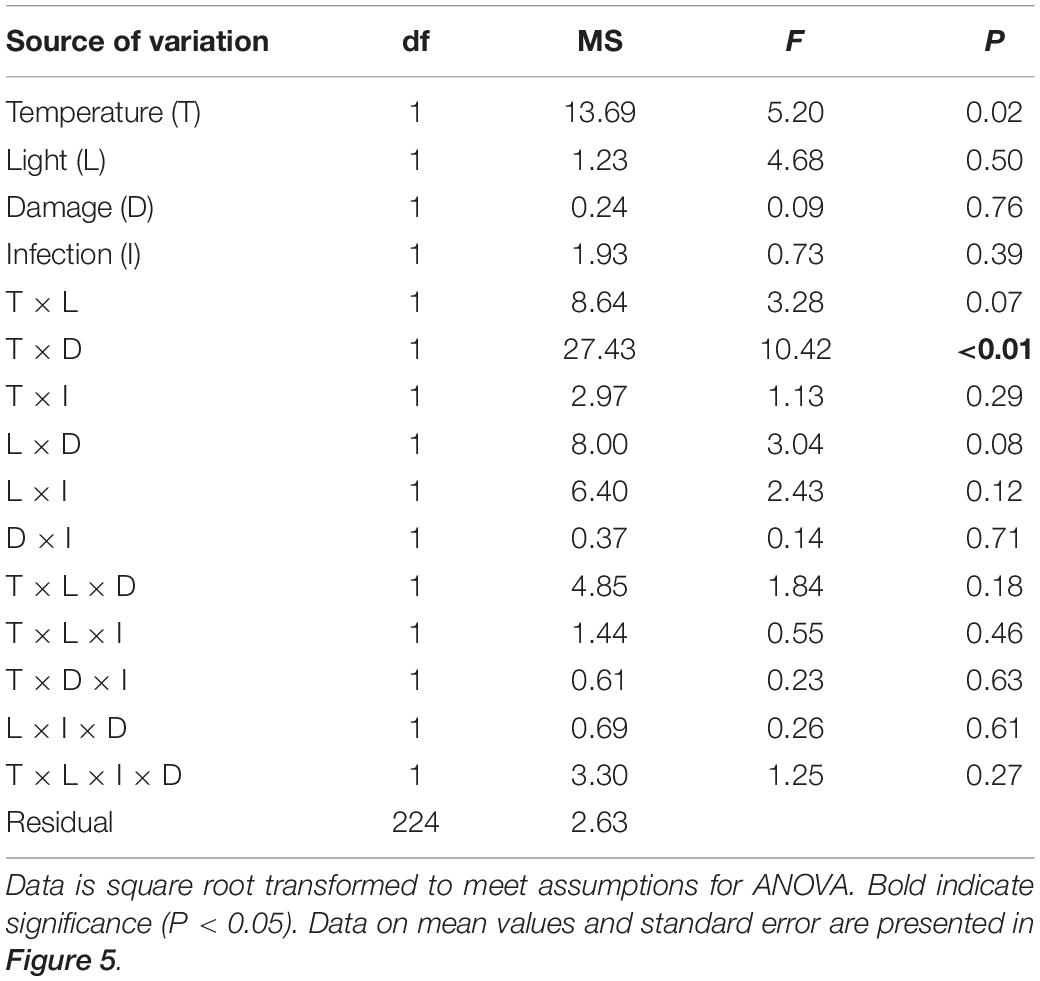
Table 4. Analysis of variance of inhibition of Labyrinthula zosterae growth by extracts of Zostera marina (% relative to controls) from shoots grown under different levels and combinations of temperature (ambient and increased), light (reduced and ambient), tissue damage (damaged and undamaged), and L. zosterae infection (non-inoculated and inoculated).
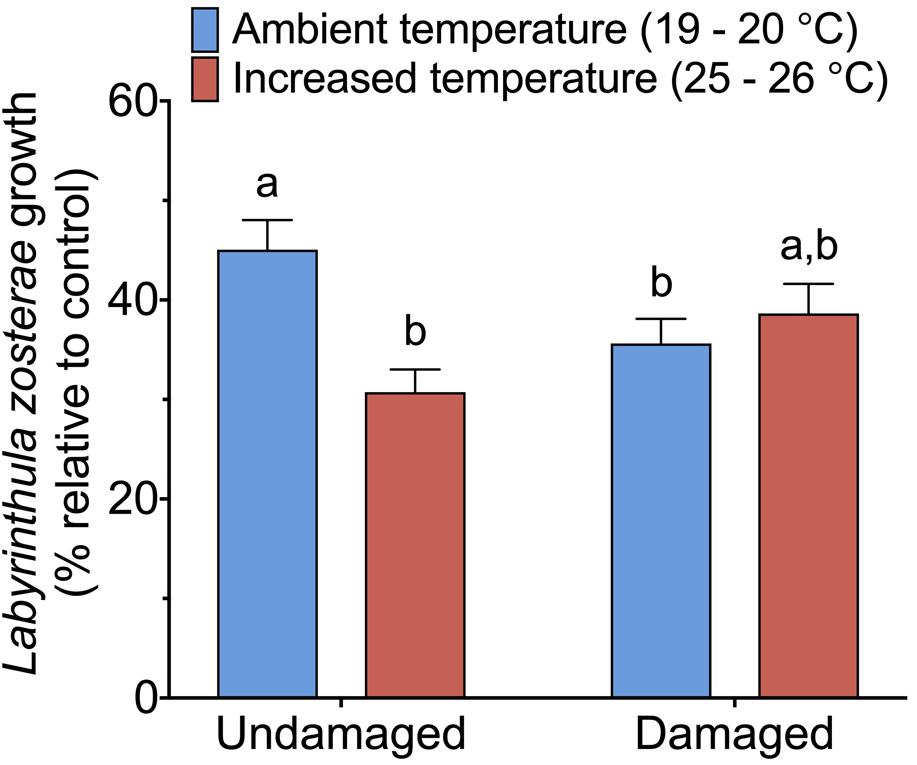
Figure 5. Inhibitory effect of Zostera marina extracts against Labyrinthula zosterae, measured as average L. zosterae growth on extracts of Z. marina shoots exposed to ambient and increased temperature and with damaged or undamaged tissue relative to L. zosterae growth on controls. Different letters above bars indicate significant differences between treatments based on the Student–Newman–Keuls test (P < 0.05). Only significant results (see Table 4) are included in the figure and not all interactions. The non-significant treatments are therefore pooled giving a higher replication than 20 replicates per treatment. Error bars show standard error, n = 60.
Gene Expression
Expression of the 16 targeted Z. marina genes following L. zosterae infection under different combinations of light, temperature and tissue damage showed a complex pattern with multiple interactive effects between treatments (Supplementary Figure S1). A summary of the expression of the different functional gene groups in relation to non-inoculated shoots at ambient conditions (ambient control) is shown for the groups with significant differences (PERMANOVA, P < 0.05) among treatments (Table 5).
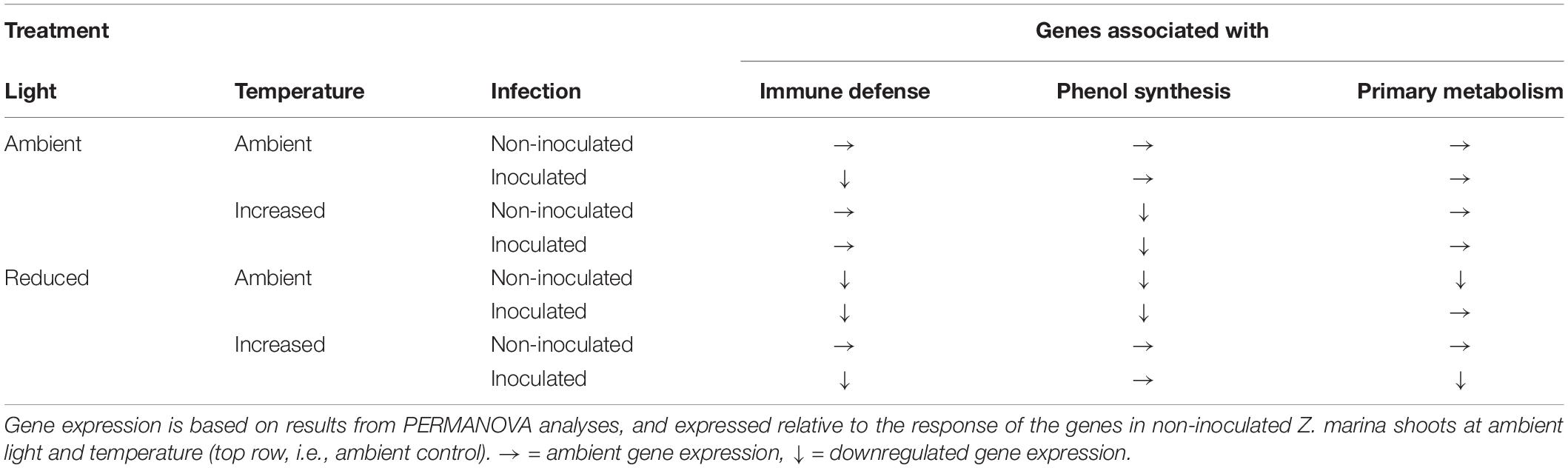
Table 5. Expression of genes associated with immune defense, phenol synthesis, and primary metabolism in Zostera marina grown under different levels and combinations of light, temperature and Labyrinthula zosterae infection.
The expression pattern of targeted immune genes was affected by a statistically significant interactive effect between temperature, light and infection (Table 6). The PCA showed that 87.7% of the total variation is explained by PC1 (53.5%) and PC2 (34.2%). PC1 is associated with the genes CLT1 and RppA and showed that inoculated shoots had a decreased expression of these genes compared to non-inoculated shoots, in both the treatment combinations ambient temperature/ambient light and increased temperature/reduced light, but not in ambient temperature/reduced light, or increased temperature/ambient light (Table 6). However, ambient temperature/reduced light led to a reduced expression of the targeted immune genes compared to non-infected shoots at ambient temperature and light (Table 6).
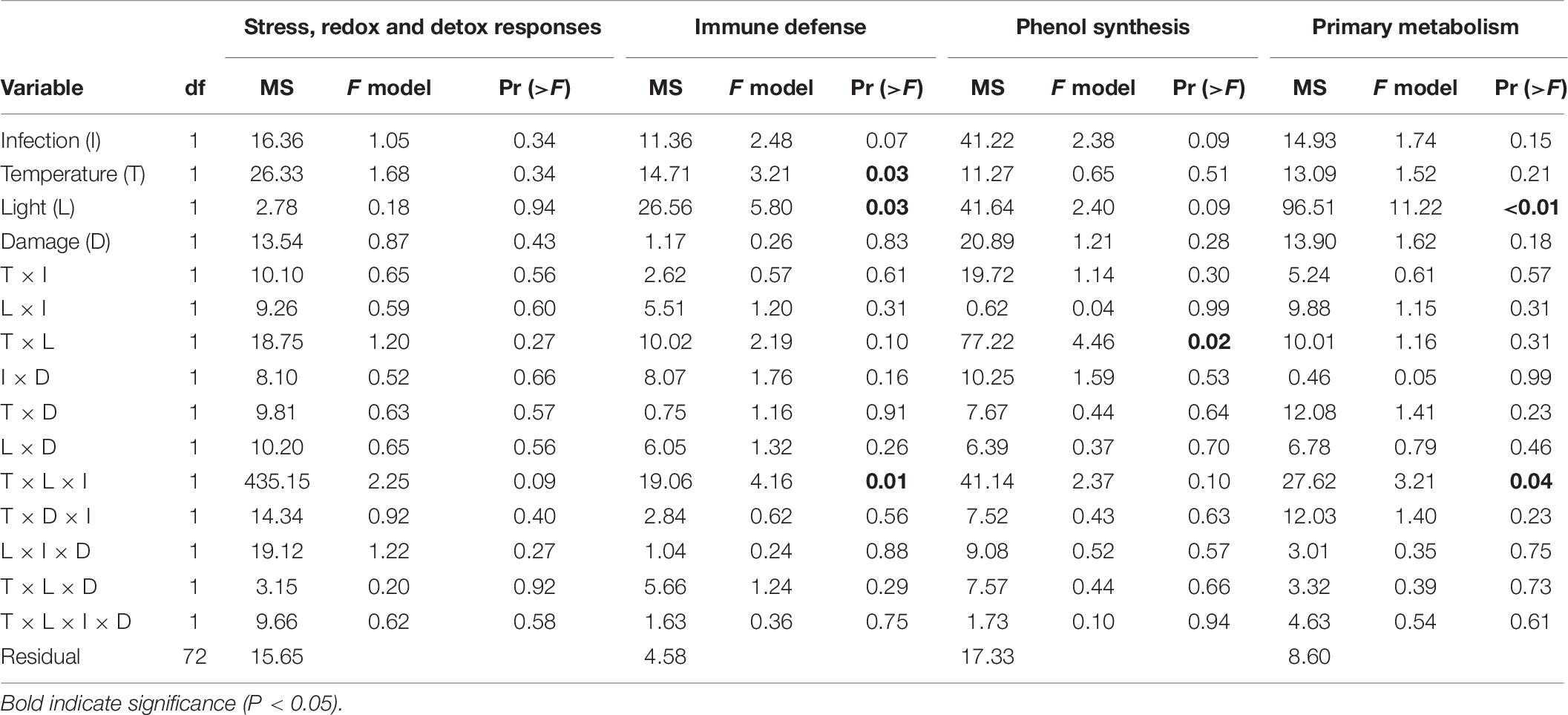
Table 6. Permutational analysis of variance of expression of genes associated with different functions (stress, redox, and detox responses, immune defense, phenol synthesis, and primary metabolism) in Zostera marina in response to different levels and combinations of temperature (ambient and increased), light (reduced and ambient), tissue damage (damaged and undamaged), and Labyrinthula zosterae infection (non-inoculated and inoculated).
The expression of genes associated with phenol synthesis was influenced by a significant interactive effect between light and temperature (Table 6). The first two principal components explained 88.6% of the total variation, and accounted for 72.6 and 16.0% of the variation, respectively. PC1 is associated with CYP73A, PAL1a, and PAL1b and showed a decreased expression of these genes in Z. marina when the shoots were subjected to combinations of ambient light/increased temperature, and reduced light/ambient temperature (Table 6).
As for the targeted immune genes, the expression of genes associated with phenol synthesis was influenced by a significant interactive effect between temperature, light and infection (Table 6). PCA showed that 88.3% of the total variation was explained by PC1 (49.3%) and PC2 (39.0%). There was a down regulation of the genes for inoculated shoots, but only at increased temperature/reduced light. In addition, non-inoculated shoots at ambient temperature/reduced light also had a decreased expression of the genes associated with the primary metabolism, compared to non-inoculated shoots at ambient conditions of temperature and light (Table 6 and Figure 6).
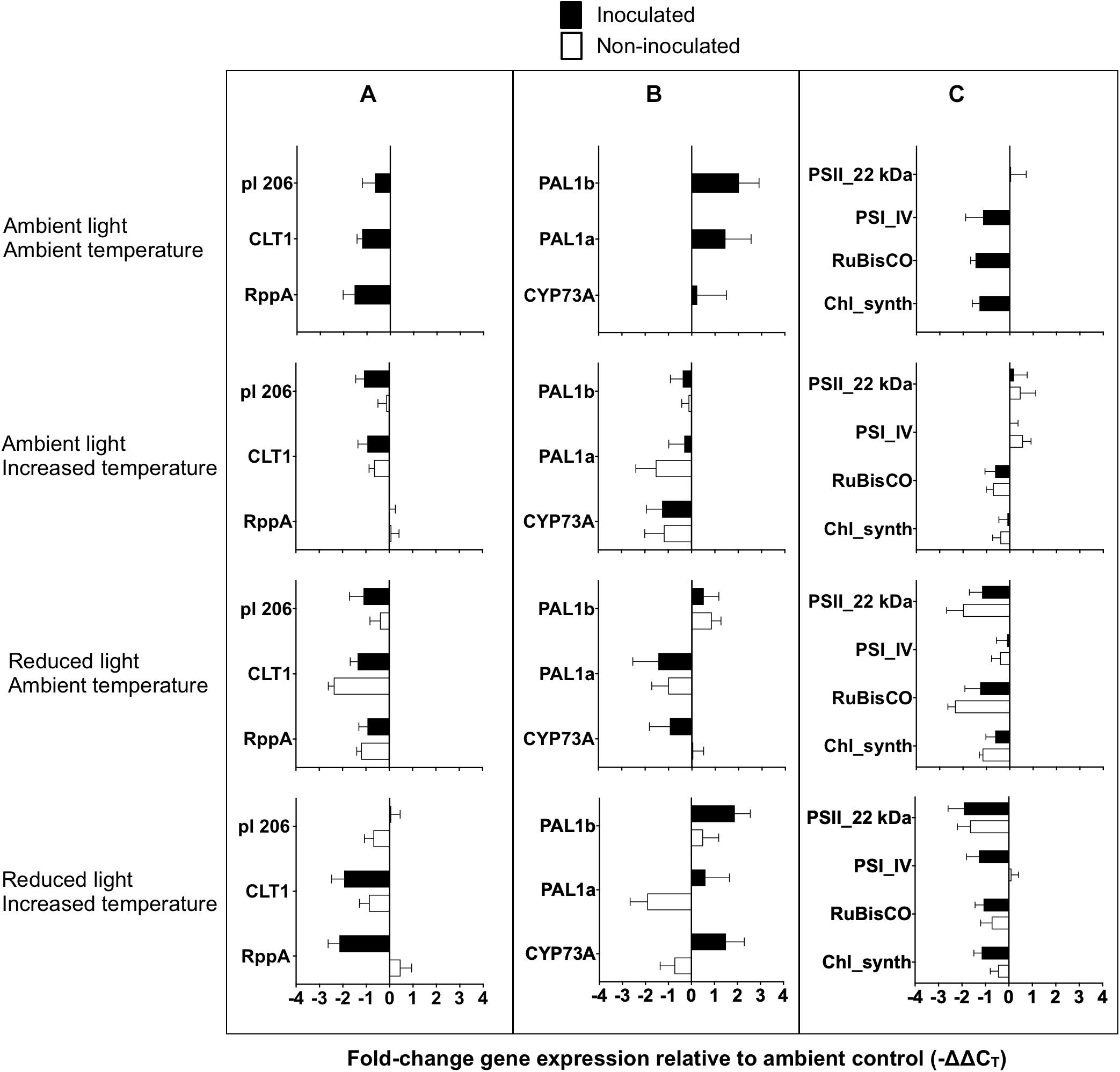
Figure 6. Gene expression of Zostera marina following experimental inoculation with Labyrinthula zosterae under different treatment conditions. Mean fold-change expression relative to ambient control (–ΔΔCT) for individual genes associated with (A) immune defense, (B) phenol synthesis, and (C) primary metabolism. Ambient control referes to undamaged, non-inoculated shoots at ambient conditions of light and temperature. Gene names are abbreviated, for full gene names see Table 1. Error bars show standard error, n = 12.
No significant effect between the treatments was detected for the expression on the general stress, and redox and detoxification genes (Table 6). Mean fold-change expression relative to ambient control (−ΔΔCT) for all individual genes are presented in Figure 6.
Discussion
This study investigated how changes in different extrinsic factors influence L. zosterae infection in Z. marina, and is one of the first studies to include interactive effects of multiple factors on Labyrinthula sp. infection in seagrass systems (see review by Sullivan et al., 2018). We show that changes in extrinsic factors can have an impact on the host-pathogen interaction on a relatively short time scale. In general, light had the largest effect on L. zosterae infection, as well as on Z. marina growth and gene expression, but there were also a large number of interactions between light and the other tested factors (temperature, tissue damage, and infection), highlighting the complexity of this host-pathogen system (Figure 7 summarizes the impact of L. zosterae infection on Z. marina shoots for the different treatment combinations).
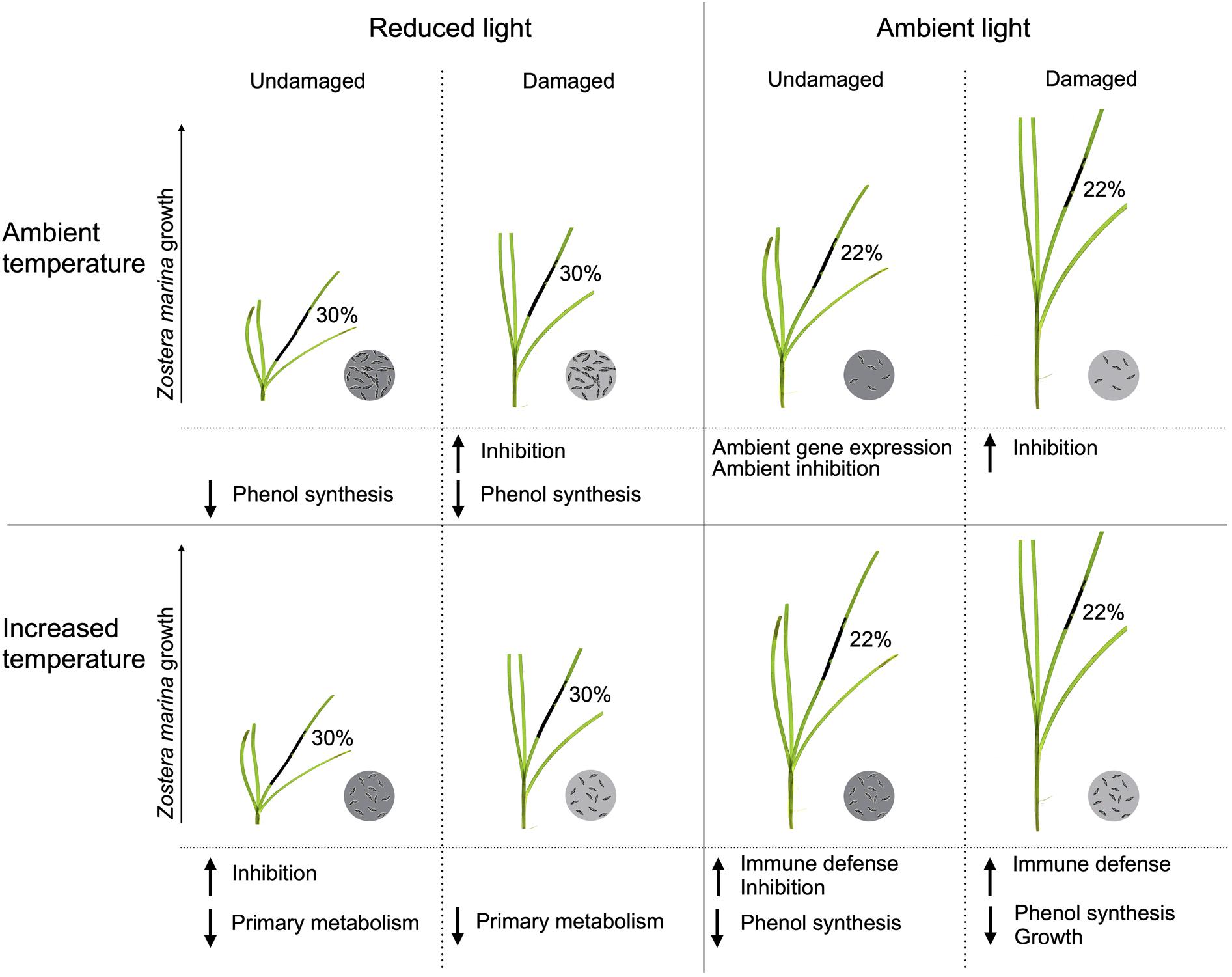
Figure 7. Summarized effects of light, temperature and tissue damage on the host-pathogen interaction between Zostera marina and Labyrinthula zosterae following 7 days of L. zosterae inoculation. The significant effects on lesion coverage, L. zosterae cell concentration, Z. marina growth, chemical defense, and gene expression demonstrated in Figures 2–6 and Table 5 are shown. Mean lesion coverage are given in percentage. L. zosterae cell concentration are indicated by the circles with L. zosterae cells: ambient = 6 cells, increased = 24 cells, intermediate = 12 cells, dark gray circles have significantly higher cell concentration than light gray circles for each light/temperature combination. L. zosterae growth inhibition by Z. marina extracts, and up or downregulation of gene groups in relation to undamaged Z. marina shoots at ambient light/ambient temperature are shown below each treatment.
Contrary to our hypothesis, reduced light, increased temperature, and tissue damage did not have additive or synergistic negative effects on L. zosterae infection in Z. marina. This corroborate the results from previous studies investigating the effect of multiple factors on Labyrinthula sp. infection in seagrass (Z. marina and T. testudinum), where the effect of single factors did not differ from the effect of cumulative stressors (Bishop et al., 2017; Brakel et al., 2019). Instead, light intensity had the strongest impact on disease lesions in the current study, and low light significantly increased lesion coverage to a moderate degree. The effects of light intensity on L. zosterae infection found in this study support previous laboratory findings, showing that reduced light increases lesion coverage (Dawkins et al., 2018). In contrast, laboratory experiments in the western Baltic Sea found no effect of light on L. zosterae infection (Brakel et al., 2019). Although our study and the study by Brakel et al. (2019) are similar in many aspects (model and pathogen species used, length of experiment, and experimental year), different light sources were used for the different experiments (Brakel et al., 2019) used artificial light, whereas our study used natural light). This could be a possible explanation to why the results differ. It is also important to consider potential differences in virulence between the pathogens used in respective experiment, since infection by more virulent L. zosterae strains can result in 25% larger lesion coverage on Z. marina plants, compared to infection by less virulent strains (Dawkins et al., 2018). As of yet, little is know about strain diversity of L. zosterae in northern Europe, and it would be beneficial to our understanding of this host-pathogen interaction to include different stains as a factor in future studies.
Whether light intensity is a primary driver for disease severity in natural seagrass populations is not clear. Increased lesion coverage can be detected on shoots in T. testudinum populations at lower light intensities (Trevathan-Tackett et al., 2013), whereas shallow-growing Z. marina shoots, exposed to relatively high light levels, have larger lesion coverage compared to deeper growing shoots receiving less light (Jakobsson-Thor et al., 2018). Although the present study shows that light can regulate L. zosterae infection in Z. marina, the results further suggest that other factors, besides light level, significantly influences the different degree of L. zosterae infection between water depths in natural Z. marina populations.
Reduced light also significantly increased pathogen cell concentrations, but only at ambient temperature. Thus, at higher temperatures, lesion coverage did not correlate with L. zosterae cell concentration. Furthermore, at ambient temperatures, the effect of reduced light was approximately four times greater on lesion coverage than on pathogen load, showing that a relatively small increase in cell concentration can have a large impact on the degree of disease symptoms in this system, or that Z. marina is more sensitive to L. zosterae cells in a light limiting environment.
Increased temperature did not increase L. zosterae infection in Z. marina in the present study, and the reported effects of temperature on Labyrinthula spp. infection in other seagrass systems are variable. A temperature increase from 11 to 18°C did not alter lesion size on Z. marina leaves following L. zosterae infection in laboratory experiments (Dawkins et al., 2018), whereas increased temperature from 22 to 27°C decreases infection, but only in combination with low salinity (12 PSU) (Brakel et al., 2019). Increased temperatures also reduced the size of lesions caused by Labyrinthula spp. in laboratory experiments with the two Mediterranean seagrass species P. oceanica and C. nodosa (Olsen and Duarte, 2015; Olsen et al., 2015). While a negative correlation between lesion size and temperature was found between 24 and 32°C for C. nodosa, P. oceanica had an upper threshold for infection with a constantly low lesion size at temperatures above 28°C (Olsen and Duarte, 2015). In the subtropical seagrass T. testudinum laboratory investigations showed that a temperature increase from 27 to 30°C had no effect on lesion size caused by Labyrinthula sp., whereas exposure to extreme heat (45°C) reduced the lesion size (Bishop, 2013). Optimum growth temperature varies among different Labyrinthula species (Olsen et al., 2015), which could explain the different effects of temperature on the Labyrinthula spp. infection reported in the literature. However, no study has reported an enhancing effect of increased temperature on lesion size. Thus, in consistence with previous investigations (Bishop, 2013; Olsen and Duarte, 2015; Olsen et al., 2015; Dawkins et al., 2018; Brakel et al., 2019), our results suggest that increased temperature is not a sole driver of increased Labyrinthula spp. virulence and wasting disease outbreaks in seagrass.
Lesion coverage was reduced in Z. marina shoots with tissue damage in this study. In contrast, field experiments with T. testudinum showed that infection by Labyrinthula sp. was more prevalent in shoots subjected to tissue damage compared to undamaged shoots (Bowles and Bell, 2004). The seagrass leaves in the study by Bowles and Bell (2004) where cut very close to the rhizome of the seagrass shoots, whereas in our experiments only moderate amounts of leaf tissue (similar to damages by boat propellers) were removed from the Z. marina shoots. The level of tissue removal in the present study potentially enabled Z. marina to manage the L. zosterae infection better than what was possible for T. testudinum in the study by Bowles and Bell (2004). Our 7-day experiment suggests that moderate tissue loss, mimicking damage by small boat propellers on the Swedish west coast, will not impact Z. marina negatively. Whether repeated tissue damage over a longer time period would affect the interaction between Z. marina and L. zosterae differently warrants further investigation.
Infection by L. zosterae did not increase the production of inhibitory compounds in Z. marina, in accordance with findings from previous field and experimental investigations (Jakobsson-Thor et al., 2018; Brakel et al., 2019), where no correlation between pathogen pressure and inhibition strength of extracted metabolites was found. The chemical identity of the inhibitory compounds is presently not known, but phenolic acids, which increase in seagrasses in response to L. zosterae infection in laboratory inoculation experiments (McKone and Tanner, 2009; Trevathan-Tackett et al., 2013), have been suggested to function as chemical defenses against Labyrinthula spp. (e.g., Buchsbaum et al., 1990; Vergeer et al., 1995). However, in contrast to previous studies (Brakel et al., 2014, 2017), infection did not upregulate genes associated with the phenol synthesis in the present study, suggesting that phenolic acids are produced at similar levels in shoots independent of infection status. The expression of trans-cinnamate 4-monooxygenase (CYP73A) as well as phenolic acid concentrations have previously been investigated in the primary leaf of inoculation (Vergeer and Develi, 1997; Steele et al., 2005; Brakel et al., 2014, 2017; Groner et al., 2016), indicating that upregulation of phenolic acids may be localized to areas of infection. In this study, gene expression was measured in a secondary leaf, which could explain why no upregulation of CYP73A was detected in the Z. marina shoots.
In contrast to results from previous studies on phenolic acids (Vergeer et al., 1995; McKone and Tanner, 2009; Hernán et al., 2017), increased temperature and reduced light intensity did not decrease Z. marina’s production of inhibitory compounds against L. zosterae. Since we did not find an upregulation of the genes associated with the phenol synthesis, it is likely that compound(s) other than phenolic acids are responsible for the inhibitory effect against L. zosterae found in this study. Little is known about how a changing environment is affecting other secondary metabolites in seagrasses (Zidorn, 2016). A review on how factors connected to global change can affect chemical defenses against insect herbivores in terrestrial plants shows that the outcome is species specific, and will depend on the compound group investigated (Bidart-Bouzat and Imeh-Nathaniel, 2008). Therefore, identification of the inhibitory compounds found in this study is needed to further investigate the effects of environmental change on chemically mediated interactions between L. zosterae and Z. marina.
Not surprisingly, Z. marina shoots kept under reduced light conditions had significantly decreased growth compared to shoots subjected to ambient light. Furthermore, increased temperature significantly enhanced growth in uninfected plants under ambient light conditions, but this effect disappeared when shoots were inoculated with L. zosterae. These results indicate that under ambient light conditions, increased temperature has a positive, and L. zosterae infection a negative, effect on Z. marina in terms of growth. Furthermore, Z. marina growth increased significantly in response to tissue damage predominantly at ambient temperatures, indicating that Z. marina shows compensatory growth responses when exposed to tissue damage. Previous studies show, similar to the results in this study, that seagrasses can compensate loss of leaf area by increasing their growth (Mitchell, 1987; Valentine et al., 1997; Moran and Bjorndal, 2005; Vergés et al., 2008). However, the response to tissue damage depends on the extent of removed tissue, and compensatory growth is not possible for seagrass with extensive physical damage (Vergés et al., 2008).
Gene expression analysis of Z. marina shoots at increased temperature, where no correlation between L. zosterae cell concentration and lesion coverage was detected, shows that shoots with a low degree of disease symptoms at increased temperature/ambient light had a higher expression of the targeted genes associated with immune defense. Whether a higher expression of these genes conveys a higher (or lower) resistance against L. zosterae is not known (Brakel et al., 2014). However, these genes encode for pathogenesis related proteins and an immune receptor, and their function in the plant immune response are well described in terrestrial plant pathosystems (van Loon et al., 2006; Spoel and Dong, 2012). Therefore, it is likely that an upregulation of these genes increases the resistance of the plants against L. zosterae, or against secondary infections of other pathogens.
Eelgrass shoots with a higher degree of lesion coverage at increased temperature/reduced light had a downregulation of genes associated with primary metabolism. Heat stress has in previous mesocosm experiments decreased gene expression of PSII_22kDa in Z. marina, although only for one of three populations investigated (Winters et al., 2011). These primary metabolism genes encode essential proteins active in photosynthesis and assimilation of glucose. Most of the proteins have a regular turnover to keep photosynthesis functional, and e. g. the targeted genes PSI_IV (Zosma35g00710) and PSII_22kDa (Zosma60g00630) have been shown to have one of the highest expression within the transcriptome in Z. marina leaves (position 32 and 9, respectively, from 22732 genes in total; Olsen et al., 2016). Thus, if the expression of these genes is decreased, we can assume that this corresponds to a decreased photosynthetic capacity.
As previously detected, expression of genes associated with immune defense is generally lower in Z. marina following L. zosterae infection (Brakel et al., 2014, 2017), although this study shows that interactive effects between L. zosterae inoculation and environmental factors can alter this relationship. This was also observed for genes associated with primary metabolism, where L. zosterae inoculation changes the expression, but only at reduced light conditions. Similar responses have been detected in Arabidopsis thaliana, where heat stress and draught can alter gene expression in virus-treated plants (Prasch and Sonnewald, 2013). It is, however, important to consider that gene expression responses toward microorganisms can be highly localized or systemic, affecting wide parts of the plant (Pozo et al., 2002). This study analyzed Z. marina leaves that were not initially inoculated, since inoculated tissue turned largely necrotic after 7 days as a response to the infection and were considered a confounding factor. Instead of sampling directly inoculated tissue we decided to look at a systemic responses in adjacent leaves to the originally inoculated leaves. Significant changes in gene expression as part of a systemic response were detected showing that the gene response toward L. zosterae infection is depending on prevailing environmental conditions. To understand gene expression responses directly in L. zosterae infected tissue and its interaction with stressors will need further studies that carefully control L. zosterae presence in non-necrotic tissue.
Although complex studies with multiple factors and interactive effects are challenging to perform and interpret, our understanding of host-pathogen interactions in the light of a changing environment is becoming increasingly important. This study shows that changing extrinsic factors, in particular light availability, can have a significant impact on the interaction between the seagrass Z. marina and the wasting disease pathogen L. zosterae on a relatively short time scale. In contrast to our experimental study, natural Z. marina meadows exhibit larger and more prevalent lesions where the light intensity is higher (Jakobsson-Thor et al., 2018), suggesting the presence of alternate uninvestigated drivers of wasting disease in the field. Along with the large number of interactive effects between the tested factors in this study (light, temperature, tissue damage, and infection) this highlights the complexity of this system, and show that more multi-factorial investigations of abiotic and biotic (e.g., different L. zosterae strains) factors are needed to better understand the impact of extrinsic factors on seagrass-Labyrinthula interactions.
Data Availability Statement
The datasets presented in this study can be found in online repositories. The names of the repository/repositories and accession number(s) can be found below: Figshare (10.6084/m9.figshare.12931247).
Author Contributions
SJ-T, HP, and GT designed the study. SJ-T carried out the fieldwork. SJ-T and GT performed experiments. SJ-T, JB, and GT handled sample and data analysis. SJ-T led the writing of the manuscript, with critical input from JB, GT, and HP. All authors contributed to the article and approved the submitted version.
Funding
This work was funded by the Swedish Research Council FORMAS (no. 2011-1193) and additional support was provided by the Linnaeus Centre for Marine Evolutionary Biology (CeMEB) and the Centre for Marine Chemical Ecology (CeMaCE) at the University of Gothenburg, and by Rådman och Fru Ernst Collianders Stiftelse. JB was funded by the Deutsche Bundesstiftung Umwelt (DBU).
Conflict of Interest
The authors declare that the research was conducted in the absence of any commercial or financial relationships that could be construed as a potential conflict of interest.
Acknowledgments
Thanks to Ylva Durland, Kristie Rigby, and Arielle Zoellin Jilliot for experimental assistance. Parts of the content of this manuscript have been printed in a thesis (Jakobsson-Thor, 2017).
Supplementary Material
The Supplementary Material for this article can be found online at: https://www.frontiersin.org/articles/10.3389/fmars.2020.575183/full#supplementary-material
References
Andersen, C. L., Jensen, J. L., and Ørntoft, T. F. (2004). Normalization of real-time quantitative reverse transcription-PCR data: a model-based variance estimation approach to identify genes suited for normalization, applied to bladder and colon cancer data sets. Cancer Res. 64, 5245–5250. doi: 10.1158/0008-5472.can-04-0496
Anderson, P. K., Cunningham, A. A., Patel, N. G., Morales, F. J., Epstein, P. R., and Daszak, P. (2004). Emerging infectious diseases of plants: pathogen pollution, climate change and agrotechnology drivers. Trends Ecol. Evol. 19, 535–544. doi: 10.1016/j.tree.2004.07.021
Barbier, E. B., Hacker, S. D., Kennedy, C., Koch, E. W., Stier, A. C., and Silliman, B. R. (2011). The value of estuarine and coastal ecosystem services. Ecol. Monogr. 81, 169–193.
Bennett, R. N., and Wallsgrove, R. M. (1994). Secondary metabolites in plant defence mechanisms. New Phytol. 127, 617–633. doi: 10.1111/j.1469-8137.1994.tb02968.x
Bergmann, N., Winters, G., Rauch, G., and Eizaguirre, C. (2010). Population-specificity of heat stress gene induction in northern and southern eelgrass Zostera marina populations under simulated global warming. Mol. Ecol. 19, 2870–2883. doi: 10.1111/j.1365-294x.2010.04731.x
Bidart-Bouzat, M. G., and Imeh-Nathaniel, A. (2008). Global change effects on plant chemical defenses against insect herbivores. J. Integr. Plant Biol. 50, 1339–1354. doi: 10.1111/j.1744-7909.2008.00751.x
Bishop, N., Martin, D. L., and Ross, C. (2017). Effects of multi-stress exposure on the infection dynamics of a Labyrinthula sp.-turtle grass pathosystem. Mar. Ecol. Prog. Ser. 581, 119–133. doi: 10.3354/meps12318
Bishop, N. D. (2013). The effects of Multiple Abiotic Stressors on the Susceptibility of the Seagrass Thalassia Testudinum to Labyrinthula sp., the Causative Agent of Wasting Disease. Master thesis, University of North Florida, Jacksonville, FL.
Bockelmann, A.-C., Tams, V., Ploog, J., Schubert, P. R., and Reusch, T. B. H. (2013). Quantitative PCR reveals strong spatial and temporal variation of the wasting disease pathogen, Labyrinthula zosterae in northern European eelgrass (Zostera marina) beds. PLoS One 8:e62169. doi: 10.1371/journal.pone.0062169
Bowles, J. W., and Bell, S. S. (2004). Simulated herbivory and the dynamics of disease in Thalassia testudinum. Mar. Ecol. Prog. Ser. 283, 127–132. doi: 10.3354/meps283127
Brakel, J., Jakobsson-Thor, S., Bockelmann, A.-C., and Reusch, T. B. H. (2019). Modulation of the eelgrass – labyrinthula zosterae interaction under predicted ocean warming, salinity change and light limitation. Front. Mar. Sci. 6:268. doi: 10.3389/fmars.2019.00268
Brakel, J., Reusch, T. B. H., and Bockelmann, A.-C. (2017). Moderate virulence caused by the protist Labyrinthula zosterae in ecosystem foundation species Zostera marina under nutrient limitation. Mar. Ecol. Prog. Ser. 571, 97–108. doi: 10.3354/meps12104
Brakel, J., Werner, F. J., Tams, V., Reusch, T. B. H., and Bockelmann, A.-C. (2014). Current European Labyrinthula zosterae are not virulent and modulate seagrass (Zostera marina) defense gene expression. PLoS One 9:e92448. doi: 10.1371/journal.pone.0092448
Buchsbaum, R. N., Short, F. T., and Cheney, D. P. (1990). Phenolic-nitrogen interactions in eelgrass, Zostera marina L.: possible implications for disease resistance. Aquat. Bot. 37, 291–297. doi: 10.1016/0304-3770(90)90075-v
Bull, J. C., Kenyon, E. J., and Cook, K. J. (2012). Wasting disease regulates long-term population dynamics in a threatened seagrass. Oecologia 169, 135–142. doi: 10.1007/s00442-011-2187-6
Chakraborty, S., Tiedemann, A. V., and Teng, P. S. (2000). Climate change: potential impact on plant diseases. Environ. Pollut. 108, 317–326. doi: 10.1016/s0269-7491(99)00210-9
Costanza, R., de Groot, R., Sutton, P., and van der Ploeg, S. (2014). Changes in the global value of ecosystem services. Glob. Environ. Change 26, 152–158.
Dangl, J. L., Horvath, D. M., and Staskawicz, B. J. (2013). Pivoting the plant immune system from dissection to deployment. Science 341, 746–751. doi: 10.1126/science.1236011
Dawkins, P., Eisenlord, M., Yoshioka, R., and Fiorenza, E. (2018). Environment, dosage, and pathogen isolate moderate virulence in eelgrass wasting disease. Dis. Aquat. Organ. 130, 51–63. doi: 10.3354/dao03263
Dray, S., and Dufour, A.-B. (2007). The ade4 Package: implementing the duality diagram for ecologists. J. Stat. Softw. 22, 1–20.
Duarte, C. M., Middelburg, J. J., and Caraco, N. (2005). Major role of marine vegetation on the oceanic carbon cycle. Biogeosciences 2, 1–8. doi: 10.5194/bg-2-1-2005
Eriander, L. (2016). Restoration and Management of Eelgrass (Zostera marina) on the West Coast of Sweden. Doctorial thesis, University of Gothenburg, Gothenburg.
Giesen, W. B. J. T., Van Katwijk, M. M., and Den Hartog, C. (1990). Temperature, salinity, insolation and wasting disease of eelgrass (Zostera marina L.) in the Dutch Wadden Sea in the 1930’s. Netherlands J. Sea Res. 25, 395–404. doi: 10.1016/0077-7579(90)90047-k
Groner, M. L., Burge, C. A., Couch, C. S., and Kim, C. J. S. (2014). Host demography influences the prevalence and severity of eelgrass wasting disease. Dis. Aquat. Organ. 108, 165–175. doi: 10.3354/dao02709
Groner, M. L., Burge, C. A., Kim, C. J. S., and Rees, E. (2016). Plant characteristics associated with widespread variation in eelgrass wasting disease. Dis. Aquat. Organ. 118, 159–168. doi: 10.3354/dao02962
Hay, M. E., and Fenical, W. (1988). Marine plant-herbivore interactions: the ecology of chemical defense. Annu. Rev. Ecol. Syst. 19, 111–145. doi: 10.1146/annurev.es.19.110188.000551
Hernán, G., Ortega, M. J., Gándara, A. M., Castejón, I., Terrados, J., and Tomas, F. (2017). Future warmer seas: increased stress and susceptibility to grazing in seedlings of a marine habitat-forming species. Glob. Change Biol. 23, 4530–4543. doi: 10.1111/gcb.13768
Infantes, E., Eriander, L., and Moksnes, P.-O. (2016). Eelgrass (Zostera marina) restoration on the west coast of Sweden using seeds. Mar. Ecol. Prog. Ser. 546, 31–45. doi: 10.3354/meps11615
Jakobsson-Thor, S. (2017). Seagrass Wasting Disease Impact of Abiotic Factors and Chemical Defense. PhD dissertation, University of Gothenburg, Gothenburg.
Jakobsson-Thor, S., Toth, G. B., Brakel, J., Bockelmann, A.-C., and Pavia, H. (2018). Seagrass wasting disease varies with salinity and depth in natural Zostera marina populations. Mar. Ecol. Prog. Ser. 587, 105–115. doi: 10.3354/meps12406
Levin, D. A. (1976). The chemical defenses of plants to pathogens and herbivores. Annu. Rev. Ecol. Syst. 7, 121–159. doi: 10.1146/annurev.es.07.110176.001005
Maleck, K., and Dietrich, R. A. (1999). Defense on multiple fronts: how do plants cope with diverse enemies? Trends Plant Sci. 4, 215–219. doi: 10.1016/s1360-1385(99)01415-6
Martin, D. L., Boone, E., Caldwell, M. M., Major, K. M., and Boettcher, A. A. (2009). Liquid culture and growth quantification of the seagrass pathogen. Labyrinthula spp. Mycologia 101, 632–635. doi: 10.3852/08-171
Martin, D. L., Chiari, Y., Boone, E., and Sherman, T. D. (2016). Functional, phylogenetic and host-geographic Signatures of Labyrinthula spp. provide for putative species delimitation and a global-scale view of seagrass wasting disease. Estuar. Coast. 39, 1403–1421. doi: 10.1007/s12237-016-0087-z
McKone, K. L., and Tanner, C. E. (2009). Role of salinity in the susceptibility of eelgrass Zostera marina to the wasting disease pathogen Labyrinthula zosterae. Mar. Ecol. Prog. Ser. 377, 123–130. doi: 10.3354/meps07860
Mitchell, C. A. (1987). Growth of Halodule wrightii in culture and the effects of cropping, light, salinity and atrazine. Aquat. Bot. 28, 25–37. doi: 10.1016/0304-3770(87)90054-4
Moran, K. L., and Bjorndal, K. A. (2005). Simulated green turtle grazing affects structure and productivity of seagrass pastures. Mar. Ecol. Prog. Ser. 305, 235–247. doi: 10.3354/meps305235
Muehlstein, L. K., Porter, D., and Short, F. T. (1988). Labyrinthula sp., a marine slime mold producing the symptoms of wasting disease in eelgrass. Zostera marina. Mar. Biol. 99, 465–472. doi: 10.1007/bf00392553
Oksanen, J., Blanchet, F. G., Kindt, R., Legendre, P., Minchin, P. R., O’Hara, R. B., et al. (2016). Vegan: Community Ecology Package. R Package. 2.3–3. Available online at: https://CRAN.R-project.org/package=vegan
Olsen, J. L., Rouzé, P., Verhelst, B., and Lin, Y.-C. (2016). The genome of the seagrass Zostera marina reveals angiosperm adaptation to the sea. Nature 530, 331–335.
Olsen, Y. S., and Duarte, C. M. (2015). Combined effect of warming and infection by Labyrinthula sp. on the Mediterranean seagrass Cymodocea nodosa. Mar. Ecol. Prog. Ser. 532, 101–109. doi: 10.3354/meps11343
Olsen, Y. S., Potouroglou, M., Garcias-Bonet, N., and Duarte, C. M. (2015). Warming reduces pathogen pressure on a climate-vulnerable seagrass species. Estuar. Coas. 38, 659–667. doi: 10.1007/s12237-014-9847-9
Orth, R. J., Carruthers, T. J. B., Dennison, W. C., and Duarte, C. M. (2006). A global crisis for seagrass ecosystems. BioScience 56, 987–996.
Orth, R. J., Heck, K. L., and Vanmontfrans, J. (1984). Faunal communities in seagrass beds - A review of the influence of plant structure and prey characteristics on predator - prey relationship. Estuaries 7, 339–350. doi: 10.2307/1351618
Pavia, H., Baumgartner, F., Cervin, G., and Enge, S. (2012). “Chemical defences against herbivores,” in Chemical Ecology in Aquatic Systems, eds C. Brönmark and L. A. Hannson (New York, NY: Oxford University Press), 210–235. doi: 10.1093/acprof:osobl/9780199583096.003.0016
Pérez, M., and Romero, J. (1992). Photosynthetic response to light and temperature of the seagrass Cymodocea nodosa and the prediction of its seasonality. Aquat. Bot. 43, 51–62. doi: 10.1016/0304-3770(92)90013-9
Pfaffl, M. W., Tichopad, A., Prgomet, C., and Neuvians, T. P. (2004). Determination of stable housekeeping genes, differentially regulated target genes and sample integrity: bestkeeper – Excel-based tool using pair-wise correlations. Biotechnol. Lett. 26, 509–515. doi: 10.1023/b:bile.0000019559.84305.47
Pozo, M. J., Cordier, C., Dumas-Gaudot, E., Gianinazzi, S., Barea, J. M., and Azcón-Aguilar, C. (2002). Localized versus systemic effect of arbuscular mycorrhizal fungi on defence responses to Phytophthora infection in tomato plants. J. Exp. Bot. 53, 525–534. doi: 10.1093/jexbot/53.368.525
Prasch, C. M., and Sonnewald, U. (2013). Simultaneous application of heat, drought, and virus to Arabidopsis plants reveals significant shifts in signaling networks. Plant Physiol. 162, 1849–1866. doi: 10.1104/pp.113.221044
Rasmussen, E. (1977). “The wasting disease of eelgrass (Zostera marina) and its effects on environmental factors and fauna,” in Seagrass Ecosystems: A Scientific Perspective, Vol. 4, eds C. P. McRoy and C. Helfferich (New York, NY: M. Dekker), 1–51.
Salo, T., Reusch, T. B. H., and Boström, C. (2015). Genotype-specific responses to light stress in eelgrass Zostera marina, a marine foundation plant. Mar. Ecol. Prog. Ser. 519, 129–140. doi: 10.3354/meps11083
Spoel, S. H., and Dong, X. (2012). How do plants achieve immunity? Defence without specialized immune cells. Nat. Rev. Immunol. 12, 89–100. doi: 10.1038/nri3141
Steele, L. T., Caldwell, M., Boettcher, A., and Arnold, T. (2005). Seagrass-pathogen interactions:‘pseudo-induction’ of turtlegrass phenolics near wasting disease lesions. Mar. Ecol. Prog. Ser. 303, 123–131. doi: 10.3354/meps303123
Sullivan, B. K., Sherman, T. D., Damare, V. S., Lilje, O., and Gleason, F. H. (2013). Potential roles of Labyrinthula spp. in global seagrass population declines. Fungal Ecol. 6, 328–338. doi: 10.1016/j.funeco.2013.06.004
Sullivan, B. K., Trevathan-Tackett, S. M., Neuhauser, S., and Govers, L. L. (2018). Host-pathogen dynamics of seagrass diseases under future global change. Mar. Pollut. Bull. 134, 75–88. doi: 10.1016/j.marpolbul.2017.09.030
Trevathan-Tackett, S. M., Lauer, N., Loucks, K., Rossi, A. M., and Ross, C. (2013). Assessing the relationship between seagrass health and habitat quality with wasting disease prevalence in the Florida Keys. J. Exp. Mar. Biol. Ecol. 449, 221–229. doi: 10.1016/j.jembe.2013.10.004
Tutin, T. G. (1938). The autecology of Zostera marina in relation to its wasting disease. New Phytol. 37, 50–71. doi: 10.1111/j.1469-8137.1938.tb06926.x
Underwood, A. J. (1997). Experiments in Ecology: Their Logical Design and Interpretation Using Analysis of Variance. Cambridge: Cambridge University Press.
Valentine, J. F., Heck, K. L., Busby, J. Jr., and Webb, D. (1997). Experimental evidence that herbivory increases shoot density and productivity in a subtropical turtlegrass (Thalassia testudinum) meadow. Oecologia 112, 193–200. doi: 10.1007/s004420050300
van Loon, L. C., Rep, M., and Pieterse, C. M. J. (2006). Significance of inducible defense-related proteins in infected plants. Annu. Rev. Phytopathol. 44, 135–162. doi: 10.1146/annurev.phyto.44.070505.143425
Vergeer, L. H. T., Aarts, T. L., and de Groot, J. D. (1995). The ‘wasting disease’ and the effect of abiotic factors (light intensity, temperature, salinity) and infection with Labyrinthula zosterae on the phenolic content of Zostera marina shoots. Aquat. Bot. 52, 35–44. doi: 10.1016/0304-3770(95)00480-n
Vergeer, L. H. T., and Develi, A. (1997). Phenolic acids in healthy and infected leaves of Zostera marina and their growth-limiting properties towards Labyrinthula zosterae. Aquat. Bot. 58, 65–72. doi: 10.1016/s0304-3770(96)01115-1
Vergés, A., Pérez, M., Alcoverro, T., and Romero, J. (2008). Compensation and resistance to herbivory in seagrasses: induced responses to simulated consumption by fish. Oecologia 155, 751–760. doi: 10.1007/s00442-007-0943-4
Waycott, M., Duarte, C. M., Carruthers, T. J. B., and Orth, R. J. (2009). Accelerating loss of seagrasses across the globe threatens coastal ecosystems. Proc. Natl. Acad. Sci. U.S.A. 106, 12377–12381. doi: 10.1073/pnas.0905620106
Winters, G., Nelle, P., Fricke, B., Rauch, G., and Reusch, T. B. H. (2011). Effects of a simulated heat wave on photophysiology and gene expression of high-and low-latitude populations of Zostera marina. Mar. Ecol. Prog. Ser. 435, 83–95. doi: 10.3354/meps09213
Keywords: chemical defense, gene expression, eelgrass, infection, Labyrinthula, multifactorial experiment, marine pathogen
Citation: Jakobsson-Thor S, Brakel J, Toth GB and Pavia H (2020) Complex Interactions of Temperature, Light and Tissue Damage on Seagrass Wasting Disease in Zostera marina. Front. Mar. Sci. 7:575183. doi: 10.3389/fmars.2020.575183
Received: 22 June 2020; Accepted: 23 September 2020;
Published: 21 October 2020.
Edited by:
Rui Rosa, University of Lisbon, PortugalReviewed by:
C. Drew Harvell, Cornell University, United StatesHung Manh Nguyen, University of Naples Federico II, Italy
Copyright © 2020 Jakobsson-Thor, Brakel, Toth and Pavia. This is an open-access article distributed under the terms of the Creative Commons Attribution License (CC BY). The use, distribution or reproduction in other forums is permitted, provided the original author(s) and the copyright owner(s) are credited and that the original publication in this journal is cited, in accordance with accepted academic practice. No use, distribution or reproduction is permitted which does not comply with these terms.
*Correspondence: Stina Jakobsson-Thor, c2pha29ic3Nvbjg3QGdtYWlsLmNvbQ==