- 1School of Marine and Environmental Programs, University of New England, Biddeford, ME, United States
- 2School of Marine Science, University of Maine, Walpole, ME, United States
- 3Department of Ocean Sciences, Memorial University of Newfoundland, St. John’s, NL, Canada
Detritus is a frequent, poorly defined, component of bivalve growth and carrying capacity models. The purpose of this study was to determine the proportional contributions of detrital material derived from primary producers (phytoplankton, macroalgae, Spartina alterniflora, and terrestrial leaf litter) to particulate organic matter (POM) and blue mussel’s (Mytilus edulis) diet within a temperate bay (Saco Bay, ME, United States). We assessed which detrital sources, if any, warranted incorporation into modeling efforts. Stable isotopes (δ13C and δ15N) and fatty acid biomarkers (FA) of mussels, size fractionated (<100 μm) POM, and primary producer endmembers (phytoplankton, Saccharina latissima, Ascophyllum nodosum, Chondrus crispus, Spartina alterniflora and leaf litter) collected between 2016 and 2017 were used to estimate endmember contributions to POM and mussel diets. Based on FAs dinoflagellates were the most abundant phytoplankton in Saco Bay, even during the fall diatom bloom. Diatoms within the bay were primarily centric, but pennate diatoms were at times present in the water column (e.g., in September). Following abundances of dinoflagellates, and centric and pennate diatoms, 22:6ω3 (DHA) was the most abundant essential FA (8.6 ± 0.1% total FAs), followed by 20:5ω3 (EPA: 7.0 ± 0.1%) and 20:4ω6 (ARA: 0.3 ± 0.1%). On average, phytoplankton derived organic matter contributed 22.1 ± 0.3% of the total POM in the bay. The concentration of non-fresh phytoplankton organic matter, or remaining organic matter (REMORG), was positively correlated with all endmember biomarkers. However, the proportion (%) of vascular plant, macroalgal, and detrital FAs was negatively correlated with the concentration of REMORG. This finding suggests in periods of low productivity, vascular plant and macroalgal detritus are proportionally more important contributors to POM. Mussels were broad spectrum omnivores, consuming phytoplankton, zooplankton, and detrital material. Detrital contributions to mussel diets were important (minimum of 16% of diet). Although small, macroalgae’s dietary contribution (8%) to M. edulis may be important. Macroalgal detritus contained essential FAs (20:5ω3 and 20:4ω6) that could supplement mussel diets, as M. edulis in Saco Bay were likely limited by 20:5ω3. Consideration of how macroalgal detritus affects the availability of essential FAs in POM may be useful to incorporate into aquaculture site selection.
Introduction
Bivalves, such as the blue mussel (Mytilus edulis), are an ecologically and economically important group of filter-feeding animals whose growth (Grant, 1996; Hawkins et al., 2002, 2013) and ecological carrying capacity (Byron et al., 2011a, b; Kluger et al., 2016; Outeiro et al., 2018) have been modeled based on food availability and other environmental variables. Detritus is often incorporated into food web and bivalve growth models because a large proportion of ecosystem energy moves through detrital food webs (Cebrian and Lartigue, 2004). We operationally define detritus as any dead or decaying matter shed from a parent organism along with associated bacteria. Detritus is difficult to measure, so models frequently must indirectly estimate the size of the detrital pool. For example, organic matter (OM) that is not live phytoplankton defined as remaining organic matter (REMORG) in the bivalve growth model Shellsim, which is treated as a homogeneous entity (Hawkins et al., 2013).
Of course, detritus is not a homogeneous pool of organic matter. The physical and biochemical composition of different detrital particulates determines their rate of degradation and residence time in ecosystems (Cebrian and Lartigue, 2004) as well as their bioavailability for bivalve consumers (Grant and Cranford, 1991; Duggins and Eckman, 1997; Arambalza et al., 2010; Dethier et al., 2014). Not incorporating this complexity into models may cause under or overestimations of detrital importance. The magnitude of the discrepancy caused by the assumptions of a homogeneous detrital pool of organic matter may be small enough relative to other sources of variability to not cause a major problem in bivalve growth models. However, when used in ecosystem models to determine carrying capacities intended for regulators and decision makers such over-simplifications can have real repercussions. For example, intensive bivalve aquaculture exceeding carrying capacity can result in bay-wide seston depletion (Grant et al., 2005; Comeau et al., 2008). By assuming all detrital material is equally valuable for a bivalve consumer we risk overestimating or underestimating the available food resources.
The major primary producers that contribute to detrital pools in temperate estuaries are phytoplankton, macroalgae and salt marsh grasses. A common proxy for nutritional quality of detrital material is the ratio of carbon-to-nitrogen, which correlates with herbivory rate on the parent material (Cebrian, 1999) as well as predicts decomposition rate (Swift et al., 1979; Coleman et al., 1983; Melillo et al., 1984; Cebrian, 1999; Moore et al., 2004). Phytoplankton, the primary diet for most bivalves, represents the highest quality material with relatively low C:N ratios (9 ± 5 weight weight–1) (Cranford and Grant, 1990; Enríquez et al., 1993; Kitazato et al., 2000; Beaulieu, 2002) while macroalgae and marsh grass detritus represent progressively lower and more variable quality material with corresponding C:N ratios of 30 ± 20 and 61 ± 34 respectively (Enríquez et al., 1993; Krumhansl and Scheibling, 2012). Although lower in quality than phytoplankton based on C:N, both marsh grass detritus (Lucas and Newell, 1984; Peterson et al., 1985; Newell and Langdon, 1986; Mann, 1988; Langdon and Newell, 1990; Decottignies et al., 2007) and macroalgal detritus (Bustamante and Branch, 1996; Fredriksen, 2003; Allan et al., 2010) have been shown to contribute to bivalve diets. Macroalgal detritus in particular can play an important role in the food webs of nearshore ecosystems (Duggins et al., 1989; Hill et al., 2006; Kaehler et al., 2006; Tallis, 2009; Von Biela et al., 2016) and may represent a valuable resource for bivalves during periods of low phytoplankton production.
Laboratory feeding trials using macroalgal detritus suggest current models of bivalve feeding may be underestimating this potential food source. For example, bivalves display moderate to high absorption efficiencies (41–87%) which increases with age of detrital particulates (Stuart et al., 1982; Cranford and Grant, 1990). Detrital particles consisting of a single cell formed from macroalgae, single celled detritus (SCD; Uchida, 1996), were capable of supporting equivalent or greater growth compared to phytoplankton diets when comprising 50–90% of larval diets in a shellfish hatchery, suggesting a synergistic effect between macroalgal detritus and phytoplankton in the diet (Camacho et al., 2004; Carboni et al., 2016). The synergy of phytoplankton and macroalgal detritus when used together, suggests that macroalgal detritus may supplement something which is lacking in phytoplankton which could be related to their fatty acid (FA) profiles (Carboni et al., 2016). While laboratory examinations of macroalgal detritus use by bivalves looks promising, demonstrating the importance of macroalgal detritus to bivalves in situ is far more difficult.
The interplay between aquaculture growth and carrying capacity models, which are reductionist by necessity, and the ecological understanding that bivalves can consume a wide variety of material is interesting and potentially informative. On one hand, if models can adequately predict the growth and carrying capacity of bivalves using solely phytoplankton, why bother with the unnecessary complexity of detrital food sources? On the other hand, if phytoplankton is the only resource required for bivalve growth, why do they ingest detrital food sources? Are detrital food sources an unnecessary component of bivalve diets, and merely ingested accidentally? Or do detrital foods supplement bivalve diets with something lacking in phytoplankton? For example, could inclusion of macroalgal detritus into a bivalve’s diet lead to a more optimized diet than solely phytoplankton as previously suggested? Though answers to these questions are likely to be species, and even location, specific, they are ecologically relevant questions and their answers could help further our understanding of bivalve nutritional requirements while also improving our capacity to model the growth and interactions of bivalve aquaculture with the environment.
Because directly tracking detrital material use by bivalves in situ is exceedingly difficult, many studies rely on stable isotope analysis (SIA) (Duggins et al., 1989; Hill et al., 2006; Kaehler et al., 2006; Tallis, 2009; Von Biela et al., 2016). SIA is well suited to distinguishing between C3 and C4 photosynthetic plants, as well as between marine and terrestrial primary producers (O’leary, 1988; Ehleringer and Cerling, 2001; Mortazavi et al., 2005; Fry, 2006). In addition, because fractionation causes δ15N values of consumers to become more enriched than their prey, δ15N values are useful for estimating trophic level (Post, 2002; Fry, 2006). However, one potential complexity when interpreting δ13C is that some key primary producers in marine ecosystems can display a wide range of δ13C values. For example, diatoms display a wide range of δ13C values depending on their phase of growth (Fry and Wainright, 1991) which can overlap with macroalgal values and lead to ambiguities about the importance of macroalgal detritus (Miller and Page, 2012).
Another powerful tool to track organic matter in ecosystems is lipid biomarkers. Lipid classes (composition, quantity, and ratios) have a long history of use as biomarkers in ecological and biogeochemical studies (see review by Parrish, 2013) and individual FA have been used in oceanographic studies as biomarkers for a large variety of organisms (Kelly and Scheibling, 2012; Parrish, 2013 and references within). For example, lipid biomarkers have been used to determine the shifting contributions of diatoms and dinoflagellates within the water column, as well as detrital inputs into marine sediments (Budge et al., 2001). Lipid FA biomarkers have also been used to determine the contribution of mangrove detritus to suspended particulate matter (Bachok et al., 2003), and compound specific SIA of FA biomarkers has been used to determine the source and age of particulate organic matter (POM; McIntosh et al., 2015; Taipale et al., 2015). Additionally, lipid biomarkers have frequently been used to determine the composition of bivalve diets (Bachok et al., 2003, 2009; Guest et al., 2008; Allan et al., 2010; Ezgeta-Balić et al., 2012; Irisarri et al., 2014; Wang et al., 2014). When used in conjunction with stable isotopes FA biomarkers become a versatile tool that can help determine primary producer contributions to food webs (Carreón-Palau et al., 2013).
We contend that by using SIA and FA biomarkers together, complex organic matter pathways in coastal ecosystems can be parsed more accurately. The purpose of this study was to determine the contributions of detrital particulates from phytoplankton, macroalgae, and vascular plants (marsh grass and terrestrial leaf litter) within a northern temperate bay to the diet of intertidal M. edulis using a combination of stable isotopes and lipid biomarkers. Our aim was to more accurately represent organic matter pathways for consideration in bivalve feeding and carrying capacity models.
To determine the contributions of macroalgae and vascular plants to mussel diets, we collected fresh samples of each from Saco Bay, ME, United States, along with live M. edulis. Lipids were extracted from M. edulis, vascular plant, and macroalgal endmembers and quantified via Iatroscan analysis before being derivatized into fatty acid methyl esters (FAME) and identified with gas chromatography. Multivariate statistics were used to determine the relative contributions of each endmember to the diet of M. edulis, based on the proportions of FA biomarkers from each producer (identified via SIMPER analysis and previous literature). In addition, the stable isotopic composition (δ13C and δ15N) of M. edulis and each endmember was used to construct a dual-isotope Bayesian mixing model, which estimated primary producer contributions to the diet of M. edulis.
Materials and Methods
Study Site and Sample Collection
We collected samples from Saco Bay (Figure 1), which is located in southern Maine, United States, with its northern-most border framed by Scarborough marsh and Biddeford Pool as the southern boundary (Reynolds and Casterlin, 1985). Saco Bay has a mean tidal range of 2.7 m (Jensen, 1983; Kelley et al., 2005) and the primary source of freshwater is the Saco River. The Saco River is the sixth largest river discharging into the Gulf of Maine (Tilburg et al., 2011) with discharge rates varying between 40 and 620 m3 s–1 and a mean of 100 m3 s–1 (Barber, 1995; Kelley et al., 2005). The only other significant river discharging into the bay is the Scarborough River, which has an average discharge of only 3.1 m3 s–1 (Figure 1; Jacobson et al., 1987; Kelley et al., 2005).
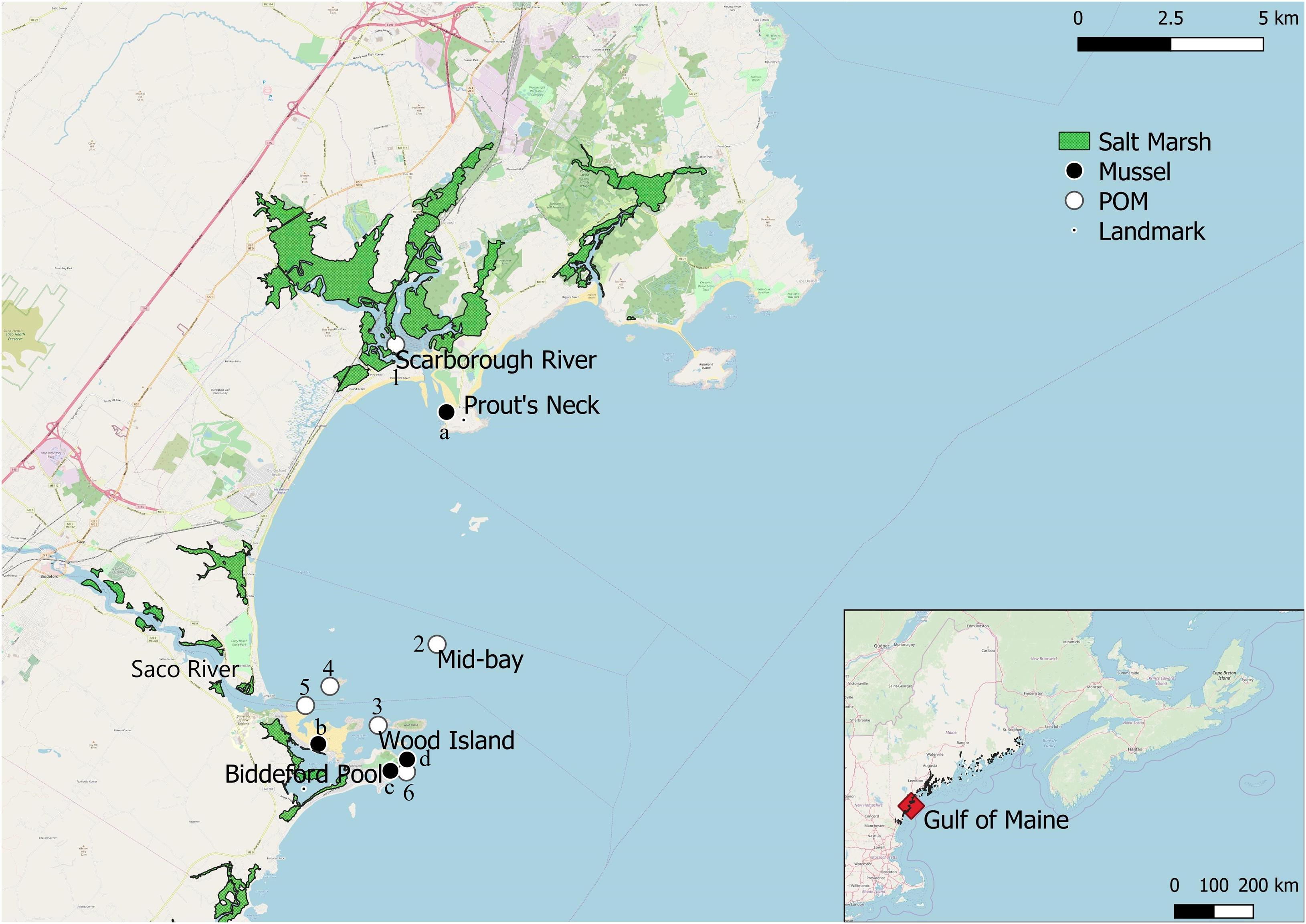
Figure 1. Map of Saco Bay, ME, United States, including location of fringing salt marshes, particulate organic matter (POM) sampling sites (1: Scarborough River, 2: Mid-bay, 3: Wood Island, 4: Ram Island, 5: Saco River, 6: East coast), and sampling sites for mussels (Mytilus edulis; a: Scarborough, b: Hill’s beach, c: East coast, d: Wood Island).
Water samples for POM were obtained from six sites throughout Saco Bay in 2016 and 2017 (Figure 1). POM was size fractionated (<100 μm) in situ in the water column with a custom made sampling device. Although M. edulis is capable of ingesting particulates > 100 μm, we targeted POM < 100 μm in this study because we were interested in small detrital particulates, which are able to remain in the water column for extended periods and be exported to other ecosystems. Additionally, the 100 μm cut-off excluded large zooplankton which may have overwhelmed any detrital signal. The sampling device filtered water through a 100 μm pre-screen and a 1 μm collection mesh while being slowly towed vertically through the water column, suction was provided by a small pump aboard the vessel. Water depth for all sampling sites was <10 m and the entirety of the water column was sampled, with exception to the mid-bay site which had a depth of 31 m and only the top 15 m of the water column was sampled due to limitations of the sampling device. On average, 374 ± 146 l were filtered per location when collecting < 100 μm POM. Once captured on the 1 μm mesh, POM was gently rinsed into a Nalgene bottle using filtered (0.45 μm) seawater. Separate 1 μm meshes were used per sample location, after sampling meshes were dried at 60°C for 24 h and weighed to account for any particulates that may have adhered to them. Concentrated slurries of POM were stored in a cooler of ambient water until subsampled on land for each analysis. Replicate subsamples were filtered onto 1.2 μm GF/C Whatman filters for each analysis. Samples were analyzed for dry weight, ash-free dry weight, chlorophyll-α, stable isotopes (δ13C and δ15N), and FA biomarkers. In 2016 POM was collected biweekly from all six sites, while in 2017 POM samples were only collected monthly from three sites: Wood Island, the mid-bay site, and the mouth of the Scarborough River. For number of samples analyzed per site for each analysis and sampling date see Supplementary Table S1.
Intertidal blue mussels and macroalgae (Saccharina latissima, Ascophyllum nodosum and Chondrus crispus) as well as a C4 photosynthetic marsh grass Spartina alterniflora, were collected biweekly from four sites in 2016 and monthly in 2017: the outer northern perimeter of Biddeford pool, the outer eastern perimeter of Biddeford pool, near Wood island, and Prout’s Neck near the Scarborough River (Figure 1). All mussels, A. nodosum, and C. crispus samples were collected by hand from the intertidal zone during low-tide, no depth was recorded but the mean tidal range for Saco Bay is 2.7 m (Jensen, 1983; Kelley et al., 2005). Kelp samples were obtained opportunistically when washed into the inter-tidal zone via wave action proceeding from storm events and collected from an experimental aquaculture lease near Wood Isle. S. alterniflora samples were collected from the Scarborough marsh near Prout’s neck. Terrestrial leaf litter (composed primarily of Quercus spp.) was sampled once in the fall of 2016 to represent C3 photosynthetic plants. For a breakdown of the number of samples analyzed for mussels and each endmember per site and sampling location see Supplementary Tables S2, S3. Macroalgae sampled within this study represent system dominants, fucoid algae, such as A. nodosum, and C. crispus dominate the intertidal area in the North western Atlantic and kelps, such as S. latissima, dominate the subtidal zone (Stephenson and Stephenson, 1972; Chapman and Johnson, 1990). Macroalgal and Spartina samples were scraped with a razor to remove epiphytes and rinsed in deionized water prior to processing for stable isotope (δ13C and δ15N) and FA analysis. Zooplankton were collected with vertical tows of a 200 μm zooplankton net. Afterward, zooplankton were phototaxically separated from settling detritus in a graduated cylinder, by shining a beam of light horizontally through the water surface and decanting off aggregating photophilic zooplankton. For a breakdown of the number of zooplankton samples analyzed per site, per sample date see Supplementary Table S4.
Chlorophyll-α
Chlorophyll-α samples were collected on pre-combusted 1.2 μm GF/C Whatman filters and stored at −20°C until analysis. Chlorophyll-α content was determined fluorometrically using a Turner Designs TD700 fluorometer calibrated with quantified standards (part # 10-850). Chlorophyll-α was extracted using 90% acetone and acidified with 5% hydrochloric acid (Strickland and Parsons, 1972; Parsons et al., 1984; Welschmeyer, 1994; Arar and Collins, 1997). Selected organic matter (SELORG; or organic matter associated with phytoplankton), and remaining organic matter (REMORG; or non-phytoplankton associated organic matter), were calculated as and REMORG = POM−SELORG as described by Hawkins et al. (2013). Hawkins et al. (2013) recommended using a carbon-to-chlorophyll (C:CHL) ratio of 12 when calculating REMORG to avoid SELORG estimates exceeding total POM; however, we used a more conservative and widely used C:CHL ratio of 50 (Taylor et al., 1997) to avoid underestimating phytoplankton contributions to POM.
Lipid Class and Fatty Acid Analysis
Modified Folch extractions were used to extract lipids from tissues or filtered POM using a chloroform-to-methanol ratio of 2:1 as described by Parrish (2013). Lipid quantification was determined using an Iatroscan Mark V TLC-FID. Heat (100°C for 1 h) and concentrated sulfuric acid were used to transesterify subsamples of total lipid extracts into FAME. An Agilent 7890A Series GC with an FID detector equipped with a 30 m (0.25 μm internal diameter) ZB wax + column (Phenomenex, US) was used to determine FAME composition; retention times were determined with a Supelco 37 component FAME mix (Product number 47885-U). Helium was used as the carrier gas at 2 ml min–1 while column temperature began at 65°C for 0.5 min then ramped to 195°C at a rate of 40°C min–1 and held for 15 min. Column temperature was then ramped to 220°C at a rate of 2°C min–1 and held for 3.25 min. Injector temperature started at 150°C and ramped at a rate of 200°C min–1 until reaching a final temperature of 250°C, while the detector remained a constant 260°C.
We used Primer 7 with the PERMANOVA + package (ver. 7.0.13, Quest Research Limited) to perform principal coordinates analysis (PCoA), similarity percentages (SIMPER), cluster analysis, permutational multivariate analysis of variance (PERMANOVA) and homogeneity of dispersion tests (PERMDISP). Data were plotted with PCoA while SIMPER and cluster analysis were used to determine similarity and dissimilarity within and among endmember groups. Statistically significant groupings (α = 0.05) were determined using PERMANOVA and prior to multivariate tests PERMDISP was used to test for homogeneity of multivariate dispersion. When necessary, FA data were square root transformed to improve homogeneity. Biomarker FAs for each endmember group as identified from previous studies, are given in Table 1. Data shown are mean ± 1 standard error, unless otherwise indicated. Percentage (%) of diatom and macroalgal FA markers, identified based on PCoA and SIMPER analysis, were used to separate the combined diatom/macroalgal estimates from stable isotope mixing models as follows:
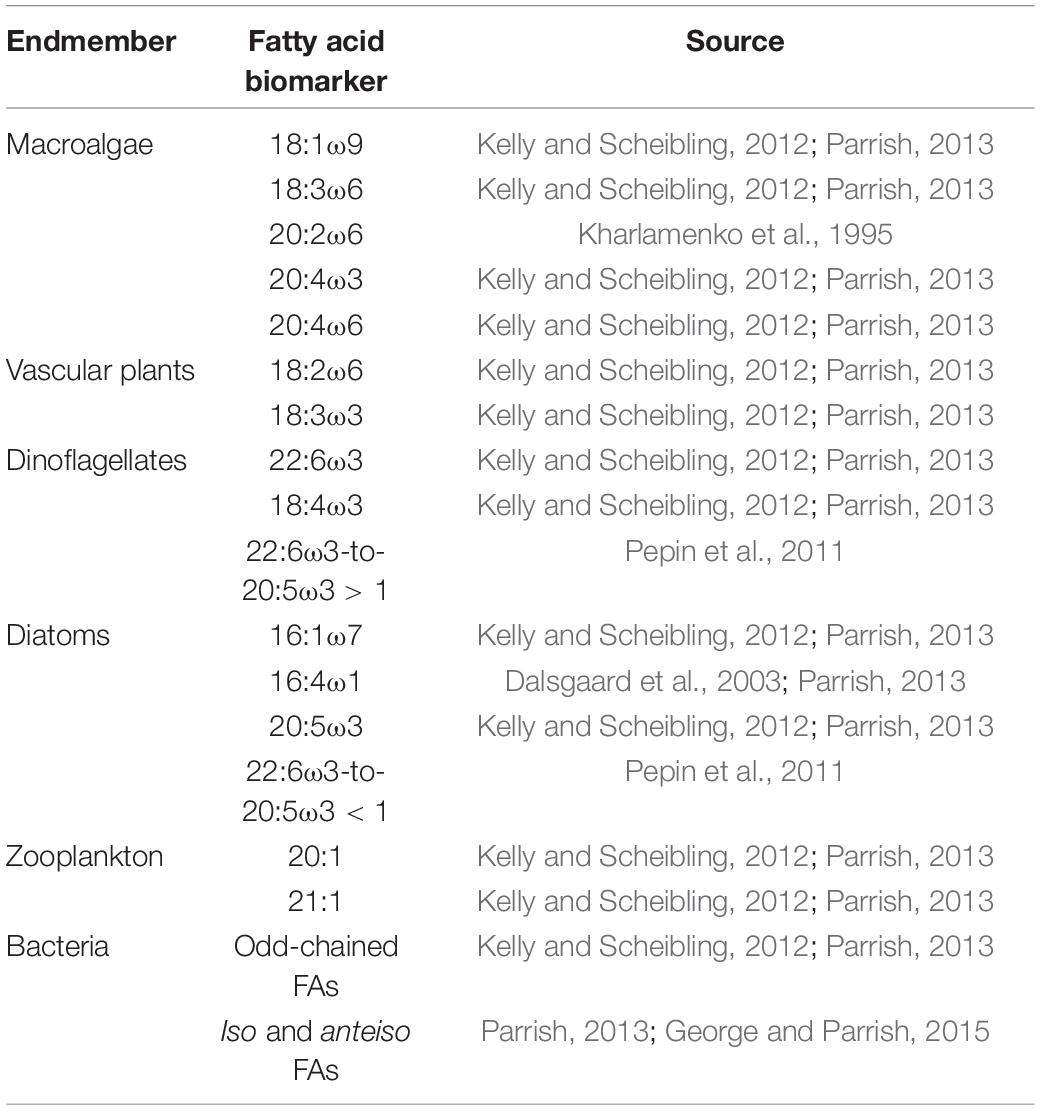
Table 1. Fatty acid biomarkers used for each endmember in this study along with literature source of previous use.
Stable Isotope Analysis
Initial processing of stable isotope samples for POM was identical to dry weights processing. POM was filtered onto pre-combusted and pre-weighed 1.2 μm GF/C Whatman filters, dried for 24 h (60°C), or until a constant weight, and stored in desiccation chambers until processing and sending for analysis at University of California’s Davis Stable Isotope facility. Hydrochloric acid fumes were introduced to dried POM samples for 24 h to remove carbonate carbon prior to encapsulation in tin. Due to difficulties in obtaining enough POM for all analyses, we did not send duplicate unacidified samples of POM for analysis. Mussel and endmember tissues were prepared by drying for 24 h at 60°C, or until a constant weight. Once dry, tissues were crushed into a fine powder and subsamples (1.0 ± 0.2 mg) of powder were encapsulated and sent for analysis. Samples were compared to laboratory reference materials, which were calibrated against international reference materials (IAEA-600, USGS-40, USGS-41, USGS-42, USGS-43, USGS-61, USGUS-64, and USGS-65). Prior to processing endmember tissues were stored at −20°C.
Analysis of variance tests were conducted using R-Studio (Version 3.6.1). Additionally, we constructed Bayesian stable isotope mixing models, which use Markov-Chain Monte Carlo methods to generate probability distributions for possible dietary contributions (Parnell et al., 2010), using the SIA package in R (SIAR, ver. 4.2). Fractionation factors of 0.4 ± 1.0‰ and 3.4 ± 1.0‰ for δ13C and δ15N respectively established by Post (2002), were used when determining mussel diets and no fractionation factors were used when determining POM composition. Phytoplankton δ15N values were estimated by subtracting one trophic level (3.4‰) from zooplankton δ15N values. Previously reported δ13C values from George’s Banks in the Gulf of Maine were used, −18 ± 2‰ and −24 ± 1‰ for diatoms and dinoflagellates respectively (Fry and Wainright, 1991). Diatoms and macroalgae were combined in mixing models due to similarities in their isotopic values. Modeled endmember contributions are reported as mean, minimum, and maximum contributions of 95% Bayesian credibility intervals, which represent the range of values within which an estimate from a single iteration of the model has a 95% probability of falling. All stable isotope data, measured and modeled, are reported as mean ± 1 standard deviation, while all other data are reported as mean ± 1 standard error. Regressions were constructed using SigmaPlot (2008; version 11.2.0.5 Systat Software, Inc.).
Our stable isotope mixing model did not account for the effects of lipid depletion on mussel δ13C values and assumed 1 trophic level (3.4‰δ15N) difference between the δ15N values of phytoplankton and zooplankton, this assumes no heterotrophic feeding by zooplankton. To test the validity of these assumptions, we constructed an alternative model incorporating a 1.75 trophic level (5.95‰δ15N) difference between phytoplankton and zooplankton, to account for heterotrophic feeding by zooplankton, as well as mussel δ13C values corrected for lipid content (based on a lipid-to-protein depletion of 6.5‰: Logan et al., 2008). Additionally, as lower fractionation rates (2.3‰) have been reported for poikilotherms (McCutchan et al., 2003), we constructed another mixing model with a lower (2.3‰) fractionation between zooplankton and phytoplankton, as well as for mussel consumers. The estimates of all models were compared to assess the effects of our assumptions on our conclusions.
Results
Phytoplankton Community and Detrital Contributions to POM
The phytoplankton community of Saco Bay was dominated by dinoflagellates, followed by centric and then pennate diatoms, which directly affected the availability of essential FAs. The average concentration of dinoflagellates (9.3 ± 0.3 μg C l–1) was significantly greater (one-way ANOVA; p < 0.01, n = 30) than that of diatoms (1.7 ± 0.1 μg C l–1) and the concentration of dinoflagellates was almost always greater than diatoms (Figure 2A). The ratio of 22:6ω3-to-20:5ω3 confirmed dinoflagellates as the dominant phytoplankton (Figure 2B). A 22:6ω3-to-20:5ω3 ratio > 1 indicates a greater abundance of dinoflagellates than diatoms and the 22:6ω3-to-20:5ω3 ratio of Saco Bay was almost always > 1. Based on volume estimates, centric diatoms (0.1 ± 0.01 mm3 l–1) were statistically more prevalent (t-test; p = 0.02) in the water column than pennate diatoms (0.02 ± 0.04 mm3 l–1; Figure 2C). Both centric and pennate diatoms bloomed in the fall which was followed by an increase in the volume of zooplankton (Figure 2C). However, even during the fall diatom bloom when chlorophyll levels reached their maximum (12.1 ± 3.9 μg l–1), the biomass of dinoflagellates (17.5 ± 6.0 μg C l–1) was greater than that of diatoms (4.9 ± 0.4 μg C l–1). Consistent with the hierarchy of phytoplankton abundance, 22:6ω3 (DHA: 8.6 ± 0.1%) was significantly (one-way ANOVA, post hoc Tukey; p < 0.001, n = 101) the most abundant essential FA, followed by 20:5ω3 (EPA: 7.0 ± 0.1%) and 20:4ω6 (ARA: 0.3 ± 0.1%).
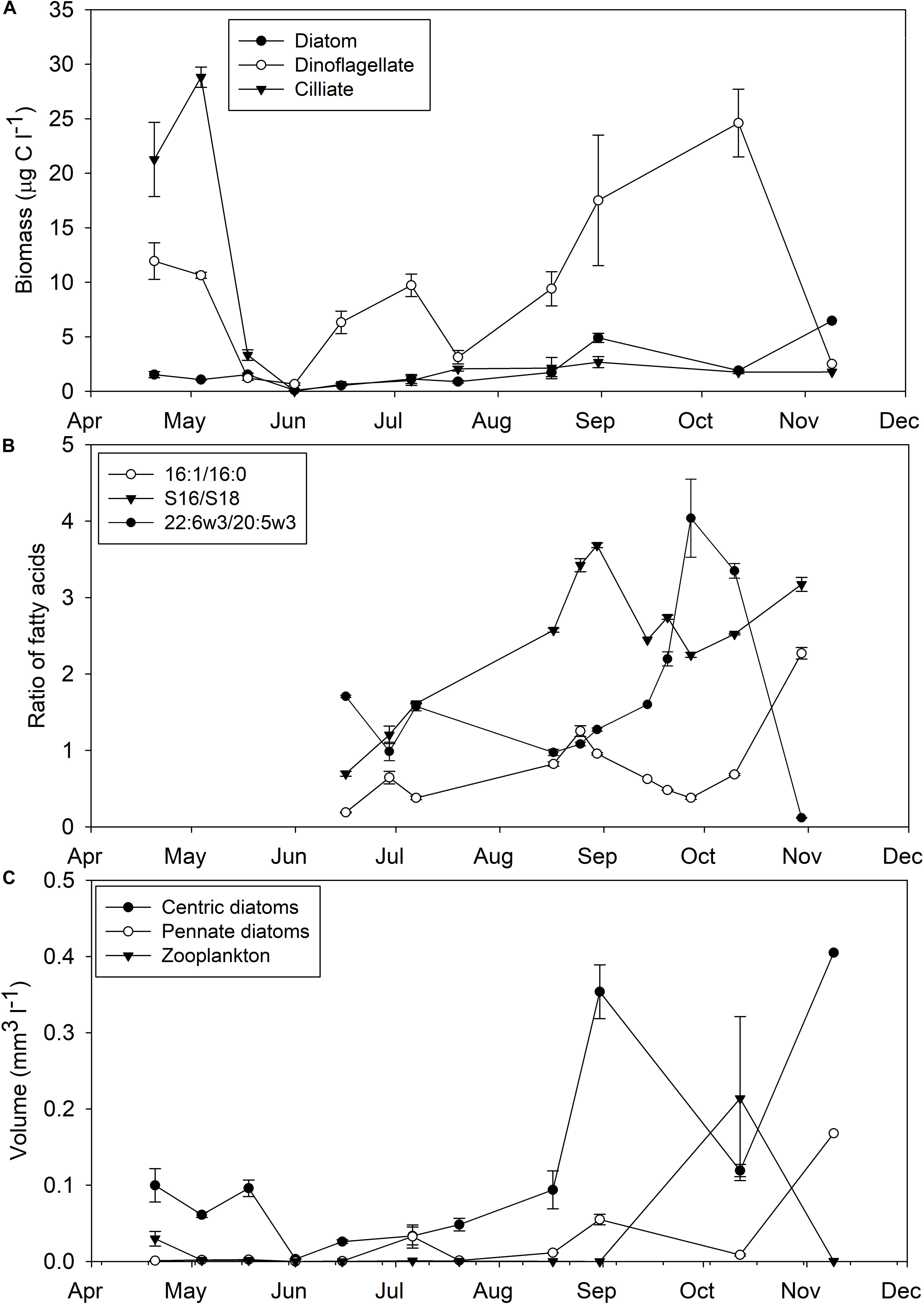
Figure 2. Selected components of the plankton community composition in Saco Bay, ME, United States, throughout 2016. (A) Biomass (μg C l− 1) of diatoms, dinoflagellates, and ciliates. (B) Diatom fatty acid indices (16:1-to-16:0 and Σ16-to-Σ18) and a dinoflagellate index (22:6ω3-to-20:5ω3). (C) Volume (mm3 l− 1) of centric diatoms, pennate diatoms, and zooplankton. Error bars represent ± 1 standard error.
REMORG comprised material from all primary producers and was most abundant during and preceding the fall phytoplankton bloom. The concentrations of all endmember biomarker FAs were significantly (p < 0.05) positively correlated with the concentration of REMORG (Figure 3A). Although REMORG comprised a significantly (t-test, p < 0.001) larger proportion of POM in summer (84 ± 0.4%, n = 40) than during the fall (69 ± 0.7%, n = 34), the average concentration of REMORG during the fall (0.5 ± 0.02 mg l–1) was significantly higher (t-test, p < 0.001) than summer concentrations (0.1 ± 0.01 mg l–1). Higher REMORG concentrations during the fall suggest that phytoplankton were more important contributors to REMORG than vascular plants and macroalgae. The proportion of vascular plant (18:3ω3 and 18:2ω6; p = 0.02, R2 = 0.16), macroalgal (18:1ω9, 18:3ω6, 20:2ω6, 20:4ω3, 20:4ω6; p = 0.01, R2 = 0.21), and detrital FAs (iso, anteiso, odd-chained, 18:1ω9, 18:2ω6, 18:3ω3, 18:3ω6, 20:2ω6, 20:4ω3, 20:4ω6; p = 0.01, R2 = 0.20) were significantly negatively correlated with REMORG concentration (Figure 3B).
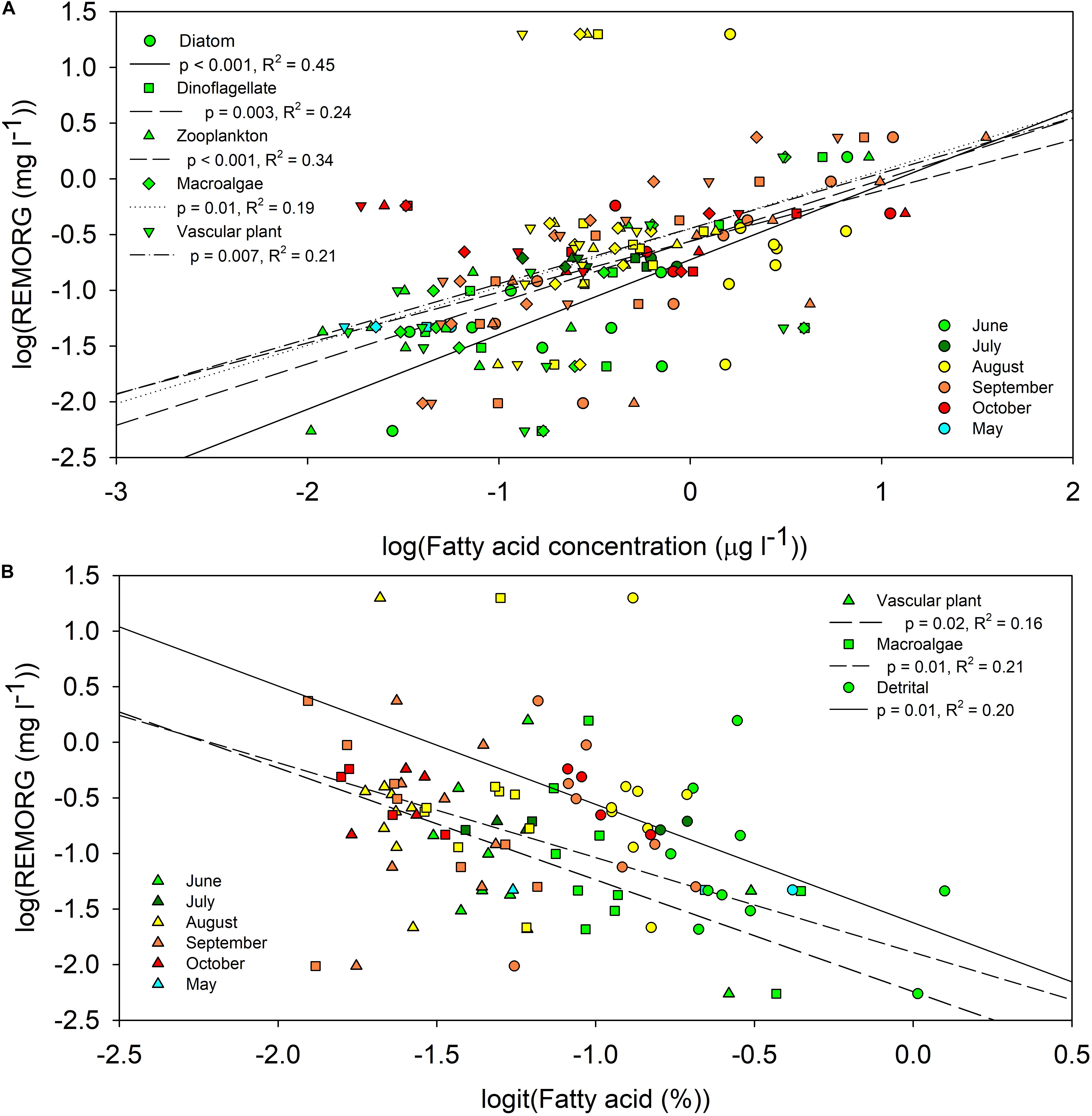
Figure 3. Significant (p < 0.05) regressions among the REMORG concentration [log(mg l− 1)] and the (A) concentration [log(μg l− 1)] and (B) proportion [logit(%)] of endmember biomarker fatty acids in <100 μm particulate organic matter from Saco Bay, ME, United States, throughout 2016 and 2017. Endmembers: diatoms (16:1ω7, 16:4ω1 and 20:5ω3), dinoflagellate (22:6ω3 and 18:4ω3), zooplankton (Σ20:1 and Σ22:1), macroalgal (18:1ω9, 18:3ω6, 20:2ω6, 20:4ω3 and 20:4ω6), vascular plant (18:2ω6 and 18:3ω3), and detrital (18:1ω9, 18:3ω6, 20:2ω6, 20:4ω3 and 20:4ω6, 18:2ω6 and 18:3ω3, iso, anteiso, odd-chained).
The concentration of macroalgal FAs was significantly (t-test, p = 0.02) higher during the spring (0.6 ± 0.1 μg l–1, n = 25) than fall (0.4 ± 0.1 μg l–1, n = 40) and macroalgal detritus became a more important source of essential FAs during late spring and early summer due to significantly (t-test, p < 0.001) smaller contributions from phytoplankton FAs (diatom: 0.3 ± 0.1 μg l–1, dinoflagellate: 0.7 ± 0.1 μg l–1) compared to the fall (diatom: 2.4 ± 0.1 μg l–1, dinoflagellate: 1.1 ± 0.1 μg l–1). All three species of macroalgae contained large proportions of the essential FA 20:4ω6 (A. nodosum: 11.5 ± 0.1%, S. latissima: 17.7 ± 0.3, C. crispus: 7.1 ± 0.3%) as well as smaller proportions of 20:5ω3 (A. nodosum: 7.7 ± 0.1%, S. latissima: 12.9 ± 0.2%, C. crispus: 25.9 ± 0.9%). Macroalgal detritus contributions to POM in June and July increased the proportion of 20:4ω6 relative to other essential FAs (20:5ω3). During June and July when macroalgal FAs comprised a larger proportion of POM FAs, the 20:4ω6-to-20:5ω3 ratio was significantly (t-test, p < 0.001) higher in early summer (0.1 ± 0.01, n = 27) than fall (0.03 ± 0.01, n = 40; Figure 4A). Although pennate diatoms can also be sources of 20:4ω6, the ratio of 20:4ω6 to a diatom biomarker (16:1ω7) was also significantly higher (t-test, p < 0.001) in late spring and early summer (5.5 ± 0.1) than fall (0.2 ± 0.1; Figure 4B) in addition another macroalgal marker (18:1ω9) to 16:1ω7 (Figure 4C) was also significantly higher (t-test, p < 0.001) in late spring and early summer (0.11 ± 0.01) than fall (0.02 ± 0.01) suggesting 20:4ω6 within the water column was from macroalgal detritus and not diatoms.
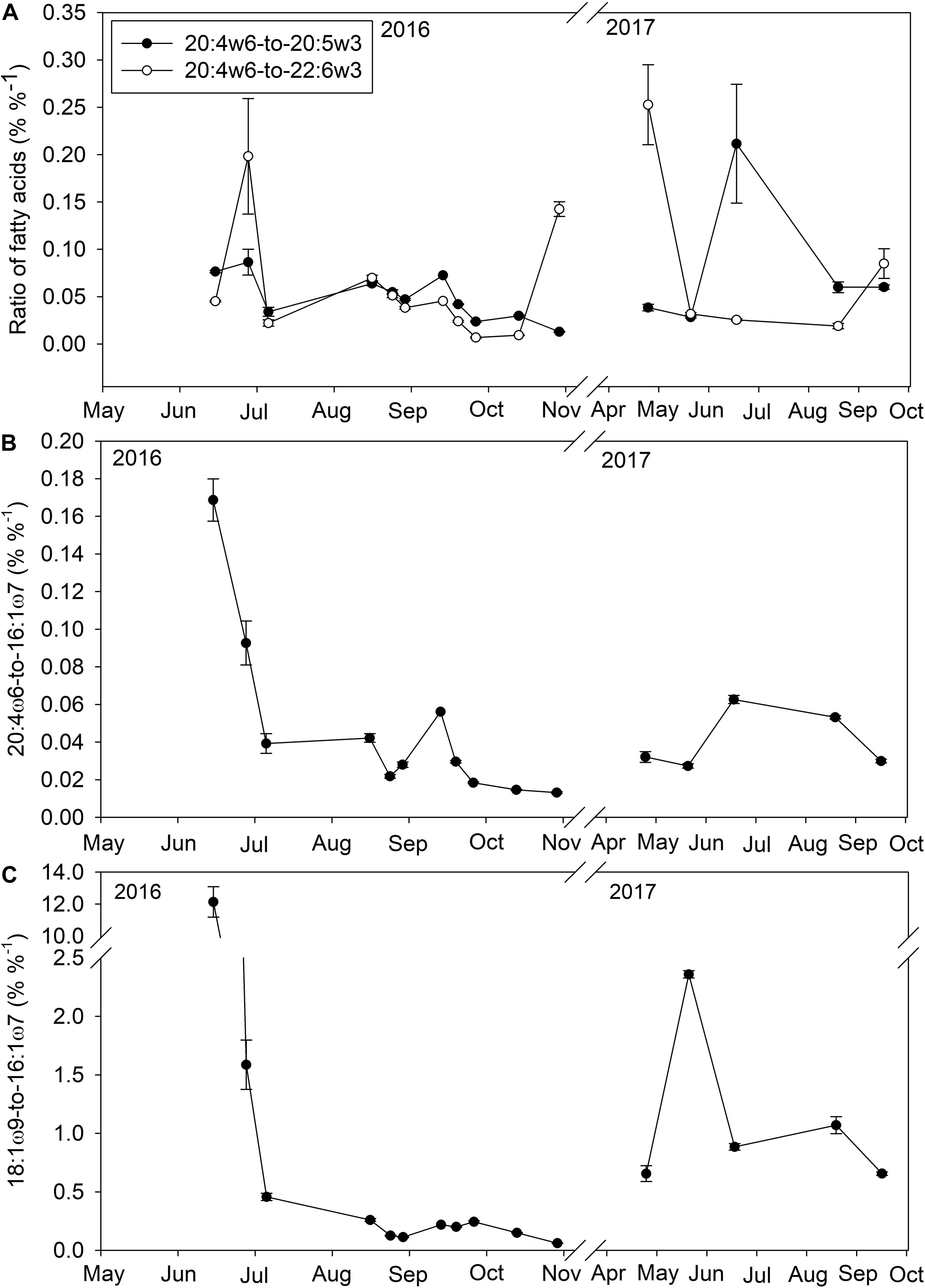
Figure 4. The ratio (%%− 1) of the essential fatty acid 20:4ω6 to other essential fatty acids (20:5ω3 and 22:6ω3; A), the ratio of 20:4ω6 to a diatom fatty acid (16:1ω7; B), and the ratio of a macroalgal fatty acid (18:1ω9) to a diatom fatty acid (16:1ω7; C) in <100 μm particulate organic matter within Saco Bay, ME, United States, throughout 2016 and 2017.
Isotopic and FA Composition of Mytilus edulis
Mussels within Saco Bay lost lipid and selectively retained certain FAs during summer months. Throughout the entire study, lipid content of M. edulis ranged from 0.8 to 5.4% wet weight (WW) with an average of 3.3 ± 0.1% (Figure 5A), consistent with previously reported lipid content of M. edulis (Table 2). Lipid content in mussels was significantly lower (one-way ANOVA, post hoc Tukey; p < 0.01) in summer (20.0 ± 0.8 mg g–1, n = 22) than during spring (41.5 ± 2.7 mg g–1, n = 31) or fall (34.9 ± 0.6 mg g–1, n = 46; Figure 5A). Additionally, concentrations (mg g–1) of total FAs and all essential FAs (20:4ω6, 20:5ω3, and 22:6ω3) were significantly lower (one-way ANOVA, post hoc Tukey; p < 0.001) during summer (Figure 5A). Although M. edulis experienced across the board decreases in FAs, the proportion of two essential FAs (20:4ω6 and 22:6ω3) and non-methylene interrupted dienes (NMID) were significantly higher (one-way ANOVA, post hoc Tukey; p < 0.001) throughout summer (Figure 5B), suggesting the importance of these FAs to mussels. Additionally, there were significant decreases (one-way ANOVA, post hoc Tukey; p < 0.001) in the proportion of 20:5ω3 throughout the summer (Figure 5B), and 20:5ω3 was significantly (p < 0.01, R2 = 0.2) negatively correlated with the proportion of NMIDs (Figure 6), suggesting mussels may use NMIDs to compensate for 20:5ω3 deficiency.
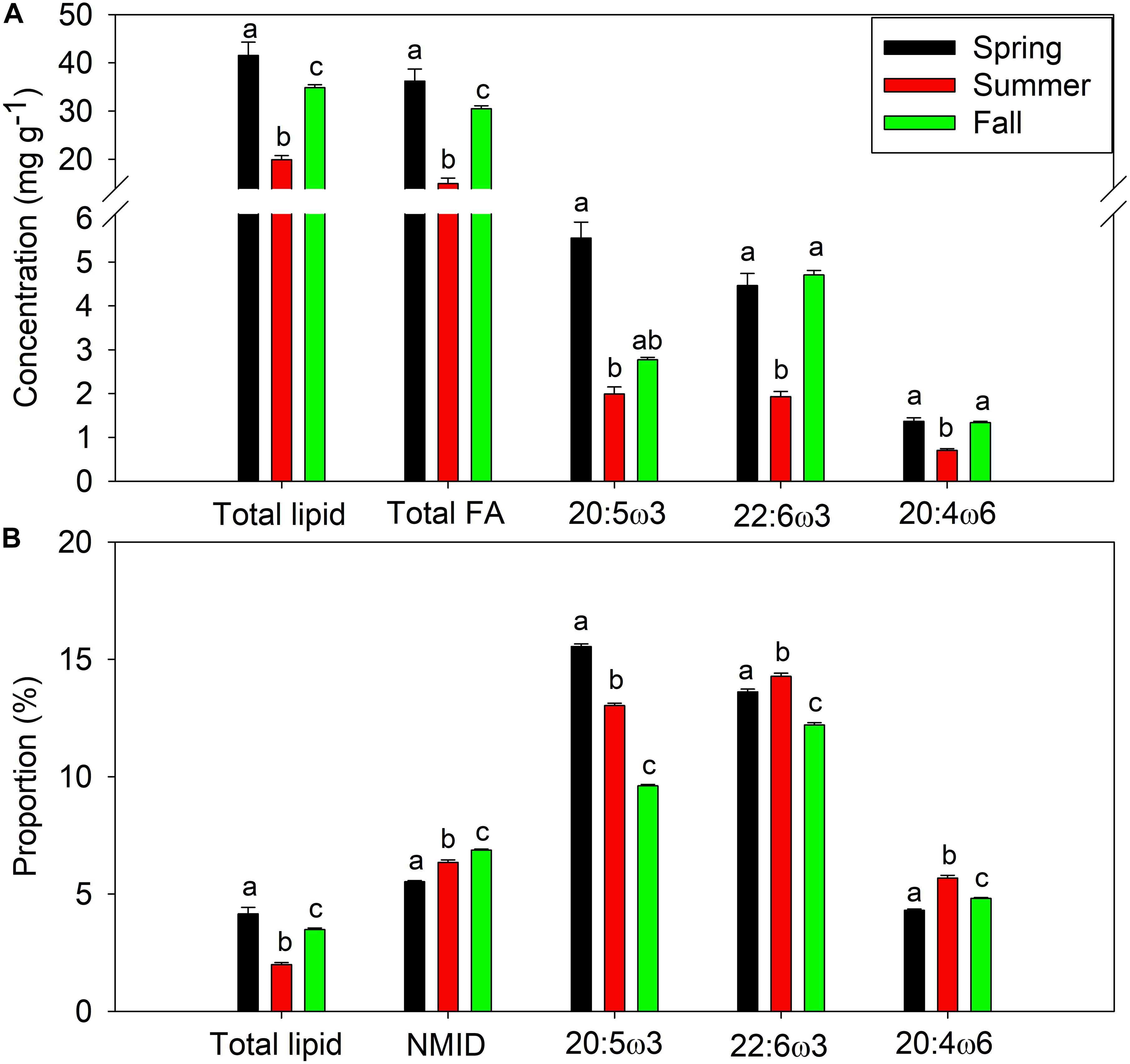
Figure 5. Average seasonal (spring, summer, and fall) lipid and essential fatty acid content of Mytilus edulis from Saco Bay, ME, United States, collected between 2016 and 2017. (A) Concentration (mg g− 1) of total lipid, total fatty acid (FA), and essential fatty acids (20:5ω3, 22:6ω3 and 20:4ω6) (B) Proportion (%) of total lipid, non-methylene interrupted dienes (NMID), and essential fatty acids (20:5ω3, 22:6ω3, and 20:4ω6). Data shown are average ± standard error and statistically significant (p < 0.05) differences across seasons are denoted by abc.
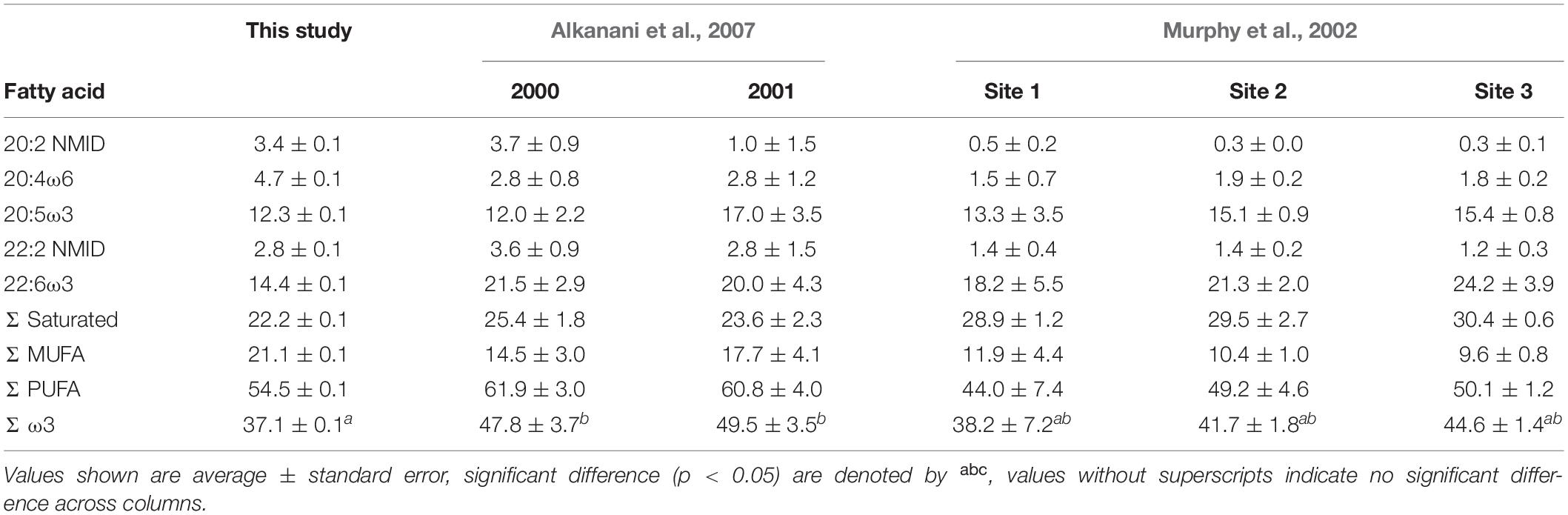
Table 2. Proportions (%) of fatty acids in Mytilus edulis measured in this study compared to literature values.
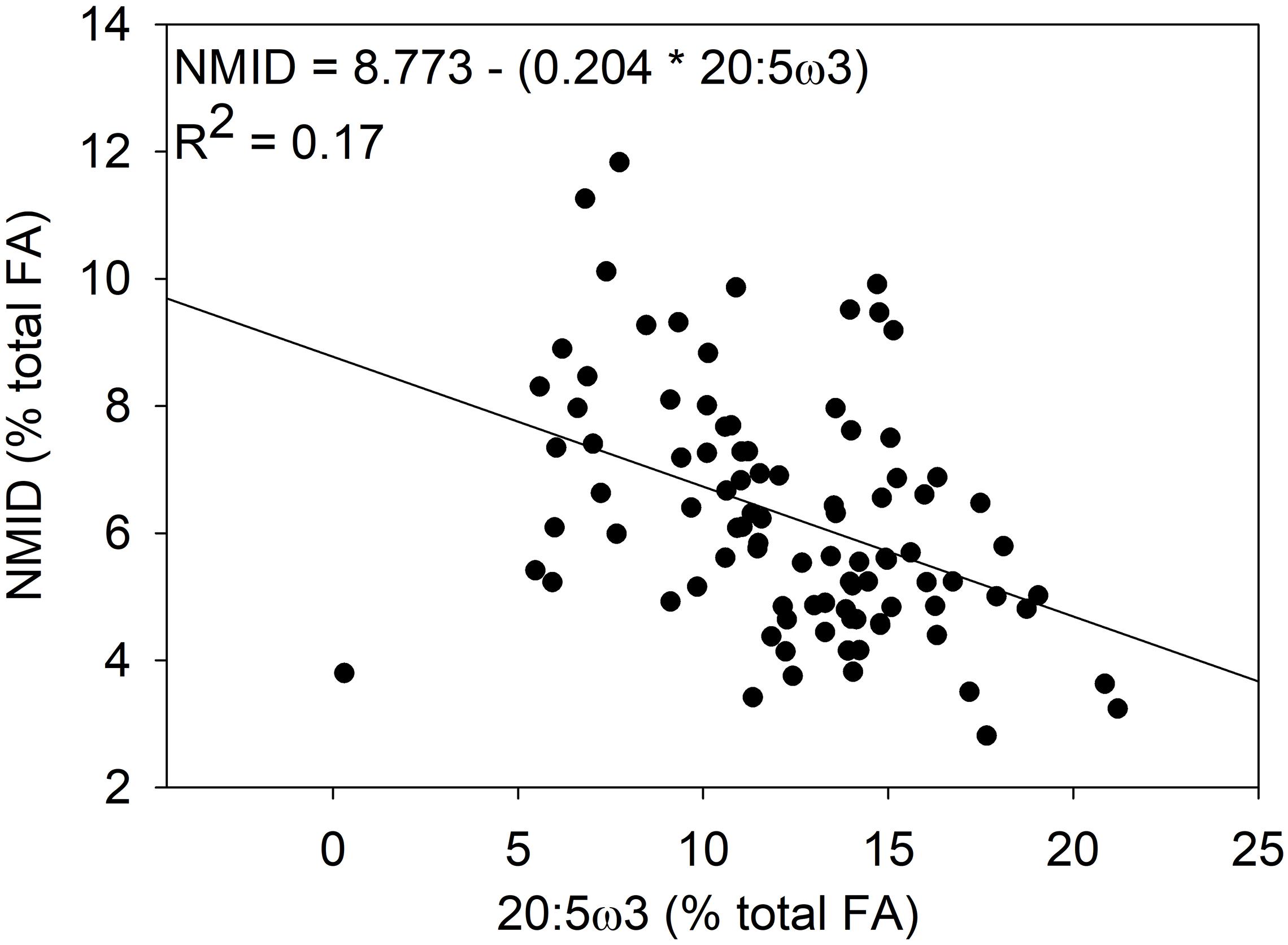
Figure 6. Significant (p < 0.05) linear regression of the proportion (%) of non-methylene interrupted dienes (NMID) and the essential fatty acid 20:5ω3 in Mytilus edulis from Saco Bay throughout 2016 and 2017.
The FA composition of M. edulis was distinctly different from any individual primary producer endmember (see Supplementary Table S5 for more detail). The three major FAs (> 10% of total FA) identified in M. edulis were 16:0 along with two essential FAs 20:5ω3 and 22:6ω3. This FA profile was significantly different [PERMANOVA, p(perm) < 0.05] than all endmembers. There were several defining FAs for each endmember group (Table 3). The most defining difference between macroalgal sources and other endmembers was the amount of 20:4ω6, which comprised a large proportion of macroalgal FAs (A. nodosum; 11.5 ± 0.1%, S. latissima; 17.7 ± 0.3%, C. crispus; 7.1 ± 0.3%) and only small proportions in vascular plants (S. alterniflora; 0.3 ± 0.1%, leaf litter; <0.1%) and consumers (M. edulis; 4.7 ± 0.1%, zooplankton; 0.5 ± 0.1%). Additionally, macroalgae (especially A. nodosum) had large proportions of 18:1ω9 (A. nodosum; 33.6 ± 0.3%, S. latissima; 9.0 ± 0.3%, C. crispus; 5.7 ± 0.3%) compared to other endmembers (<5%). Both vascular plant endmembers had large proportions of 18:3ω3 (S. alterniflora; 37.7 ± 0.4%, Leaf litter; 18.6 ± 2.5%) and 18:2ω6 (S. alterniflora; 19.0 ± 0.2%, Leaf litter; 6.0 ± 0.1%). Unsurprisingly, while the M. edulis FA profile was distinctly different than any primary producer, the FA profile of zooplankton was very similar to M. edulis. The three major FAs in zooplankton were the same as M. edulis (16:0, 20:5ω3, and 22:6ω3). Although M. edulis contained FAs from all endmembers, phytoplankton (diatom and dinoflagellate) were the largest contributors. Diatom (16:1ω7, 16:4ω1 and 20:5ω3) and dinoflagellate (22:6ω3 and 18:4ω3) FAs comprised the largest proportions of M. edulis FAs, 17.2 ± 0.1% and 16.9 ± 0.1% respectively, followed by macroalgae (7.9 ± 0.1%) and zooplankton (20:1 and 21:1; 6.2 ± 0.1%) FAs. Vascular plant FAs comprised the smallest proportion (3.3 ± 0.1%) of M. edulis FAs with bacterial FAs (iso, anteiso and odd-chained) comprising another 4.8 ± 0.1%.
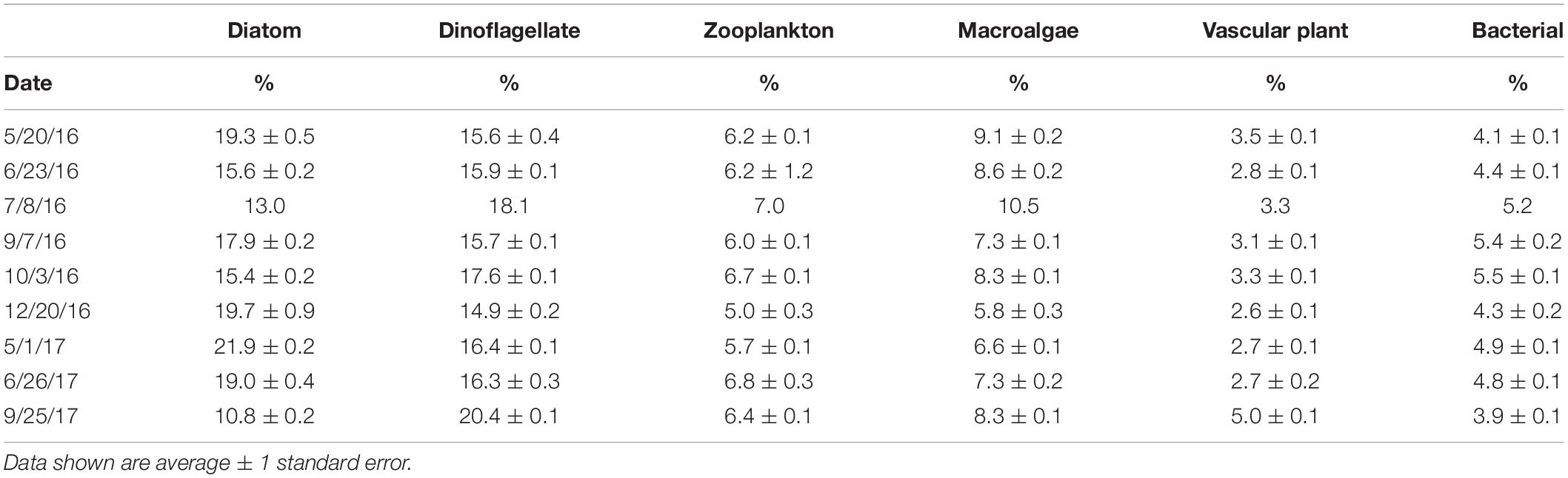
Table 3. Proportion (%) of endmember fatty acids in Mytilus edulis collected from Saco Bay, ME, United States, throughout 2016 and 2017. Endmembers: Zooplankton (20:1 and 21:1), Vascular plants (18:2ω6 and 18:3ω3), Macroalgae (18:1ω9, 18:3ω6, 20:2ω6, 20:4ω3, and 20:4ω6), Diatoms (16:1ω7, 16:4ω1 and 20:5ω3), Dinoflagellates (22:6ω3), and Bacteria (iso, anteiso and odd-chained).
The isotopic composition of M. edulis closely resembled that of < 100 μm POM (Figure 7). The δ13C (−19.4 ± 3.7‰) and δ15N (7.0 ± 2.8‰) of POM < 100 μm varied the most among all endmembers, ranging from −12 to −25‰δ13C and 2.9 to 15.2‰δ15N. In contrast, the δ13C (−20.0 ± 1.1‰) and δ15N (7.0 ± 0.9‰) of M. edulis occupied a very narrow range between −18 to −21‰ and 6.0 to 7.6‰ respectively. All three macroalgal species had similar δ13C and δ15N values (combined average −18.8 ± 2.0‰ and 5.3 ± 1.0‰ respectively). Macroalgal isotopic values were very close to the δ13C and δ15N values for diatoms (−18.5‰ and 5.3‰ respectively) based on literature sources and estimated δ15N values from zooplankton, which were the most enriched in δ15N in this study (Figure 7). Both C3 and C4 vascular plants were isotopically distinct from marine endmembers. Leaf litter (C3) was the most depleted source of both δ13C and δ15N (−30.5 ± 1.2‰ and −2.5 ± 0.6‰, respectively) while S. alterniflora (C4) was the most enriched in δ13C (−13.3 ± 1.1‰) compared to other endmembers. The δ13C of all marine endmembers fell between the values of C3 and C4 vascular plants and were more enriched in δ15N (Figure 7). The tight clustering of marine endmembers at roughly the mid-point between C3 and C4 vascular plants based on δ13C made it difficult to determine endmember contributions to POM and mussel diets based on stable isotopes alone.
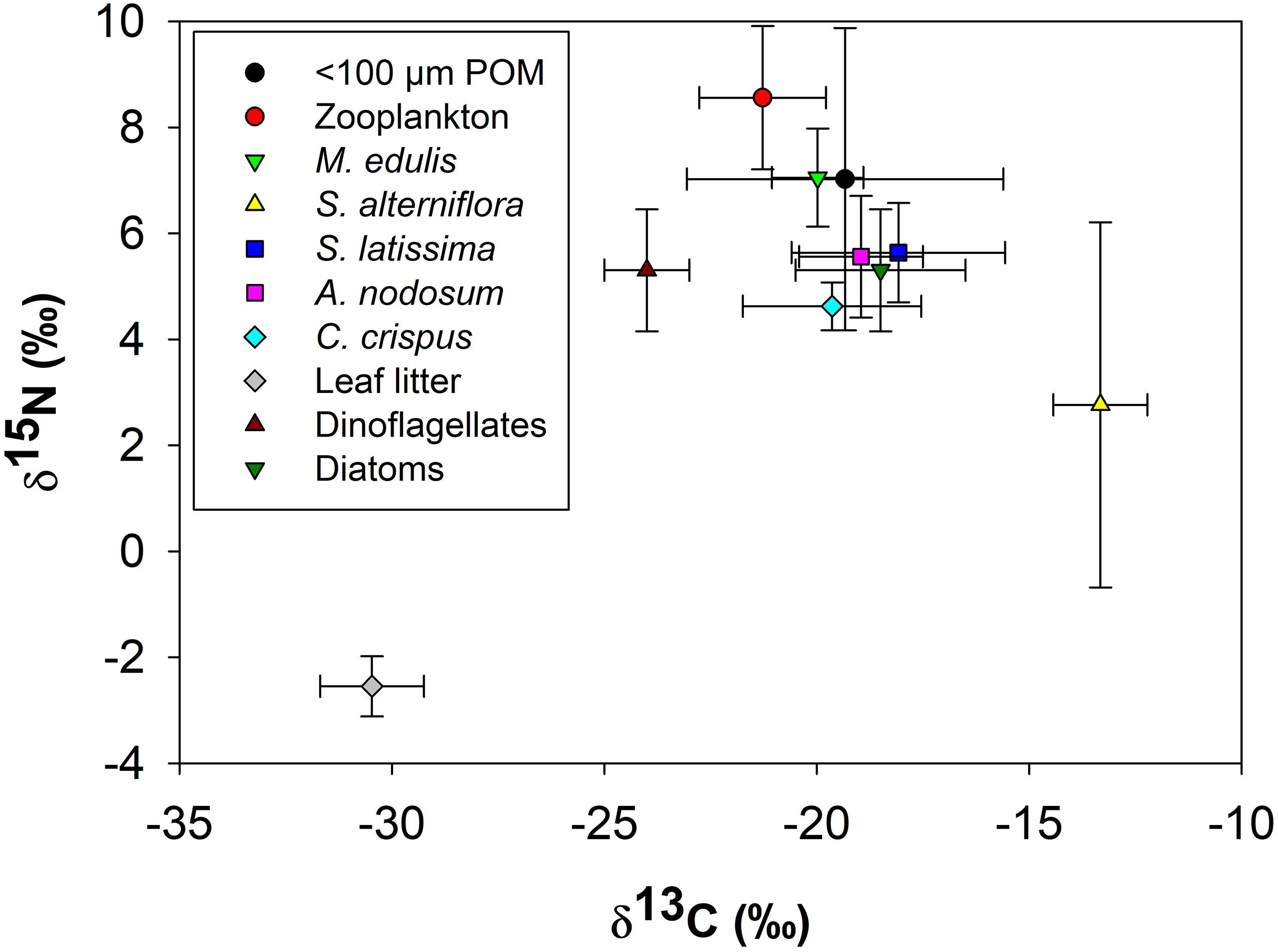
Figure 7. Carbon (δ13C) and nitrogen (δ15N) biplot of Mytilus edulis and all endmembers (<100 μm POM, zooplankton, Spartina alterniflora, Saccharina latissima, Ascophyllum nodosum, Chondrus crispus, leaf litter, dinoflagellates and diatoms), prior to consolidation, used in the stable isotope mixing model. Data shown are average ± 1 standard deviation of endmember δ13C and δ15N.
Endmember Contributions to POM and Mussel Diets
Principal coordinates analysis separated all endmember groups based on their FA profiles (Figure 8). Samples within each endmember group were highly similar (>75%), except for POM (61% similarity), likely a result of its variable composition. The FA profiles of consumers (zooplankton and M. edulis) were similar (65%) to each other, both groups of vascular plants (S. alterniflora and leaf litter) also resembled each other (65%), while the three macroalgal groups were more loosely grouped together. POM was the most variable group interspersed between all the other groups. However, there was a larger proportion of POM samples grouped closer to zooplankton than either macroalgae or vascular plants, suggesting zooplankton (and presumably phytoplankton) more strongly influenced POM FAs.
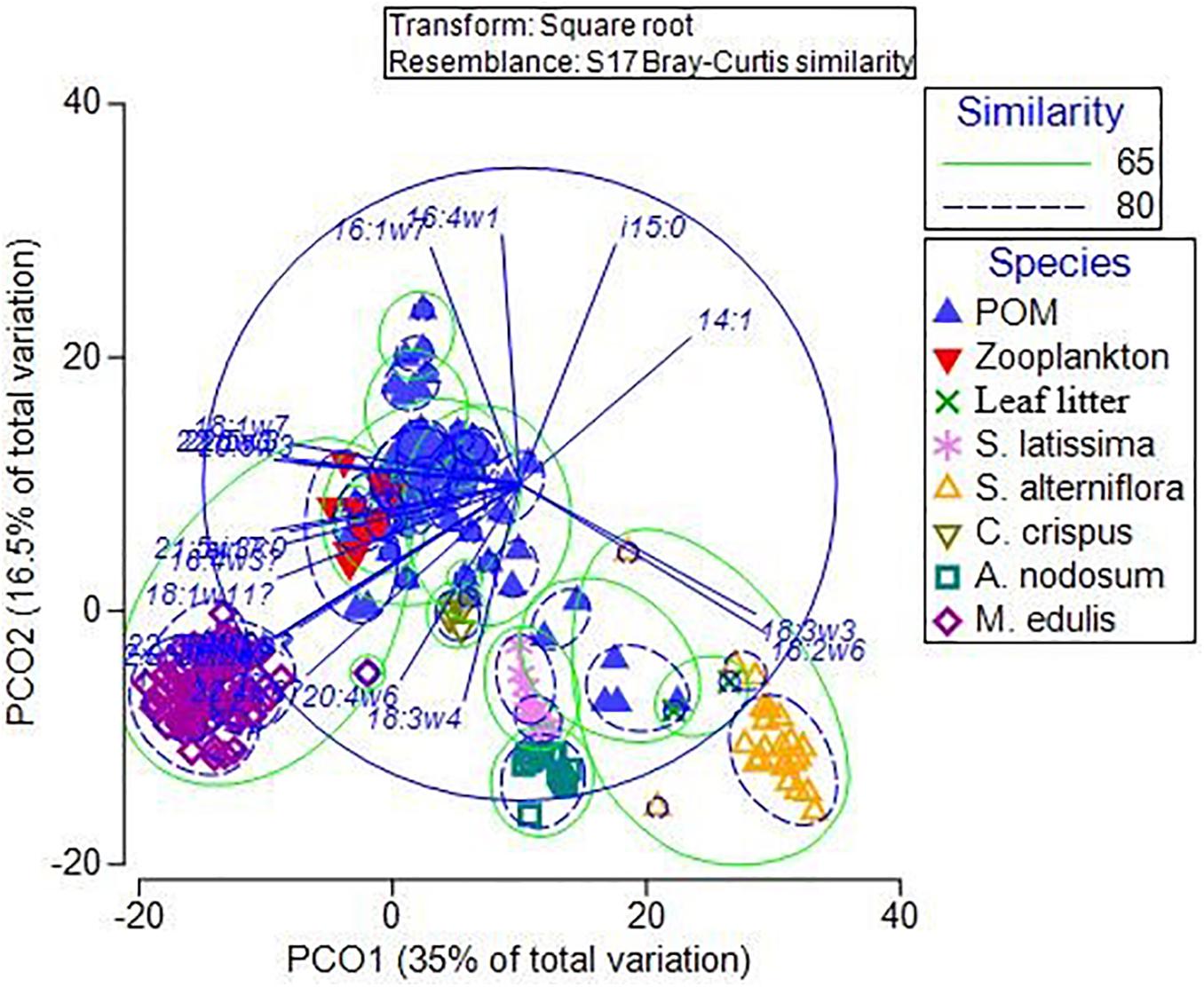
Figure 8. Principal coordinates analysis of fatty acids in <100 μm particulate organic matter (POM), zooplankton, leaf litter, Spartina alterniflora, Saccharina latissima, Ascophyllum nodosum, Chondrus crispus and Mytilus edulis from Saco Bay, ME, United States, throughout 2016 and 2017. Circled groupings are based on similarity (%) determined from similarity percentage analysis (SIMPER) and cluster analysis.
Credibility intervals (95%) from the dual isotope (δ13C and δ15N) Bayesian mixing models estimating endmember contributions to POM and the diet of M. edulis were relatively large, suggesting uncertainty in the model (Figures 9, 10). Because diatoms and macroalgae are traditionally difficult to separate based on their δ13C (−18 ± 2.0‰ and −18.8 ± 2.0‰ respectively), they were initially combined for the model. Taking the combined macroalgal and diatom model output, we used the relative proportions of diatom and macroalgal FAs to estimate their separate contributions to POM and mussel diets. In the updated model, based on mean credibility interval estimates, zooplankton (33.6 ± 8.2%) were the largest contributor to the POM pool, followed by diatoms (16.8 ± 6.5%), S. alterniflora (15.6 ± 8.4%), dinoflagellates (19.4 ± 7.9%), leaf litter (9.8 ± 8.1%), and finally macroalgal detritus (6.3 ± 3.7%; Figure 9). The largest proportion (25.3 ± 5.4%) of M. edulis diet was comprised of S. alterniflora based on the dual isotope mixing model followed by dinoflagellates (18.6 ± 2.3%), zooplankton (17.2 ± 3.0%), diatoms (16.7 ± 2.6%), leaf litter (14.8 ± 2.1%), and macroalgae (8.3 ± 2.1%; Figure 10).
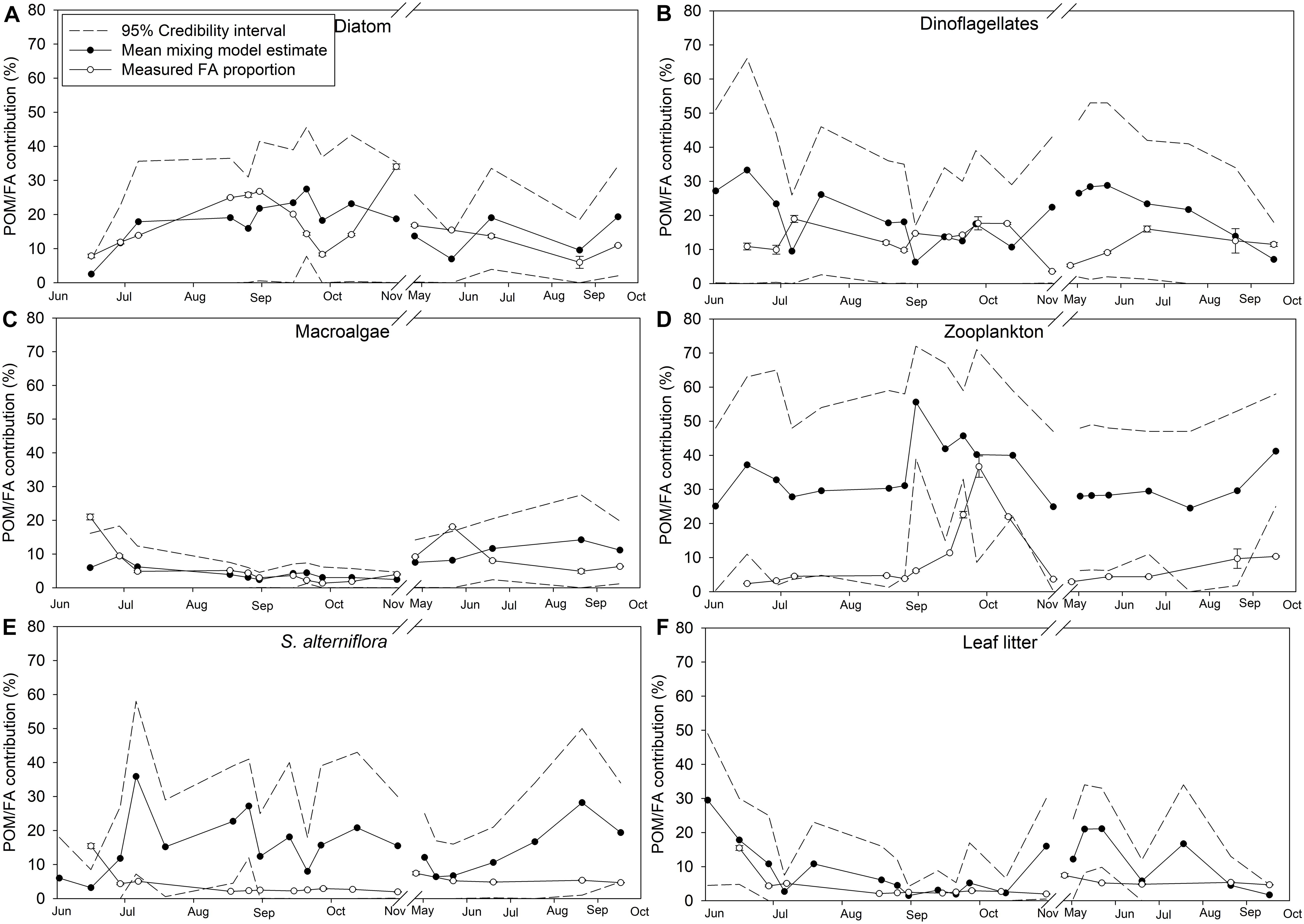
Figure 9. Endmember contribution (%) to particulate organic matter (POM) in Saco Bay, ME, United States, throughout 2016 and 2017. Values shown are the mean POM contribution (%) estimated from the dual isotope (δ13C and δ15N) Bayesian mixing model along with accompanying credibility interval (95%) and the proportion (% total FAs) of endmember biomarker FAs measured in POM. Endmembers: (A) Diatom. (B) Dinoflagellates. (C) Macroalgae. (D) Zooplankton. (E) S. alterniflora. (F) Leaf litter. Values shown are average ±1 standard error.
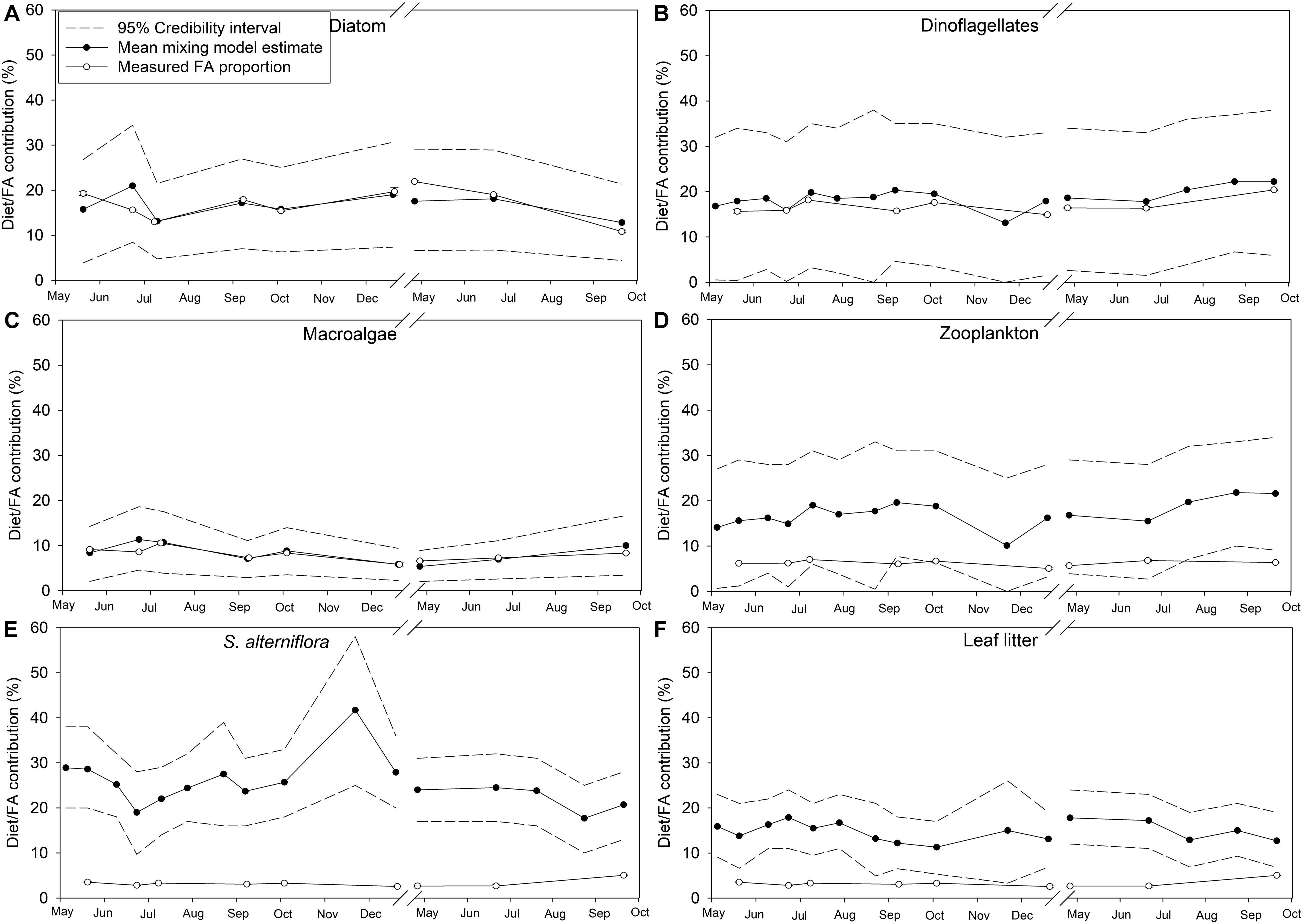
Figure 10. Endmember diet contribution (%) to Mytilus edulis in Saco Bay, ME, United States, throughout 2016 and 2017. Values shown are the mean diet contribution (%) estimated from the dual isotope (δ13C and δ15N) Bayesian mixing model along with accompanying credibility interval (95%) and the proportion (% total FAs) of endmember biomarker FAs measured in M. edulis. Endmembers: (A) Diatoms (16:1ω7, 16:4ω1, and 20:5ω3), (B) Dinoflagellates (22:6ω3), (C) Macroalgae (18:1ω9, 18:3ω6, 20:2ω6, 20:4ω3, and 20:4ω6), (D) Zooplankton (20:1 and 21:1), (E) Spartina alterniflora (18:2ω6 and 18:3ω3), and (F) leaf litter (18:2ω6 and 18:3ω3). Values shown are average ± 1 standard error.
Importantly, the stable isotope mixing model and the FA composition analyses largely agree regarding the contributions of diatoms, dinoflagellates, and macroalgae to both POM and mussel diets (Figures 9A–C, 10A–C). However, there were more discrepancies between the estimated contributions of zooplankton (Figures 9D, 10D) and vascular plant detritus (Figures 9E,F, 10E,F) between the two methods. To test the assumptions of our model, we compared our original estimates with estimates of a revised models incorporating δ13C corrections based on mussel lipid content and larger or smaller fractionation values between zooplankton and phytoplankton, as well as between mussels and their diet (Supplementary Table S6). Based on the comparison, we concluded that our model assumptions did not affect our conclusions. To address uncertainty in the model from wide credibility intervals, which frequently approached zero, we used the raw model output (distribution of estimates from all 3 × 104 model iterations) to determine the probability of zero contribution (<1%) to the diet of M. edulis for each endmember (Table 4). In general, the probability of zero contribution for most endmembers was low (<1%), with the exception of leaf litter which had significantly higher probabilities (p < 0.01) of zero diet contribution than any other endmember.
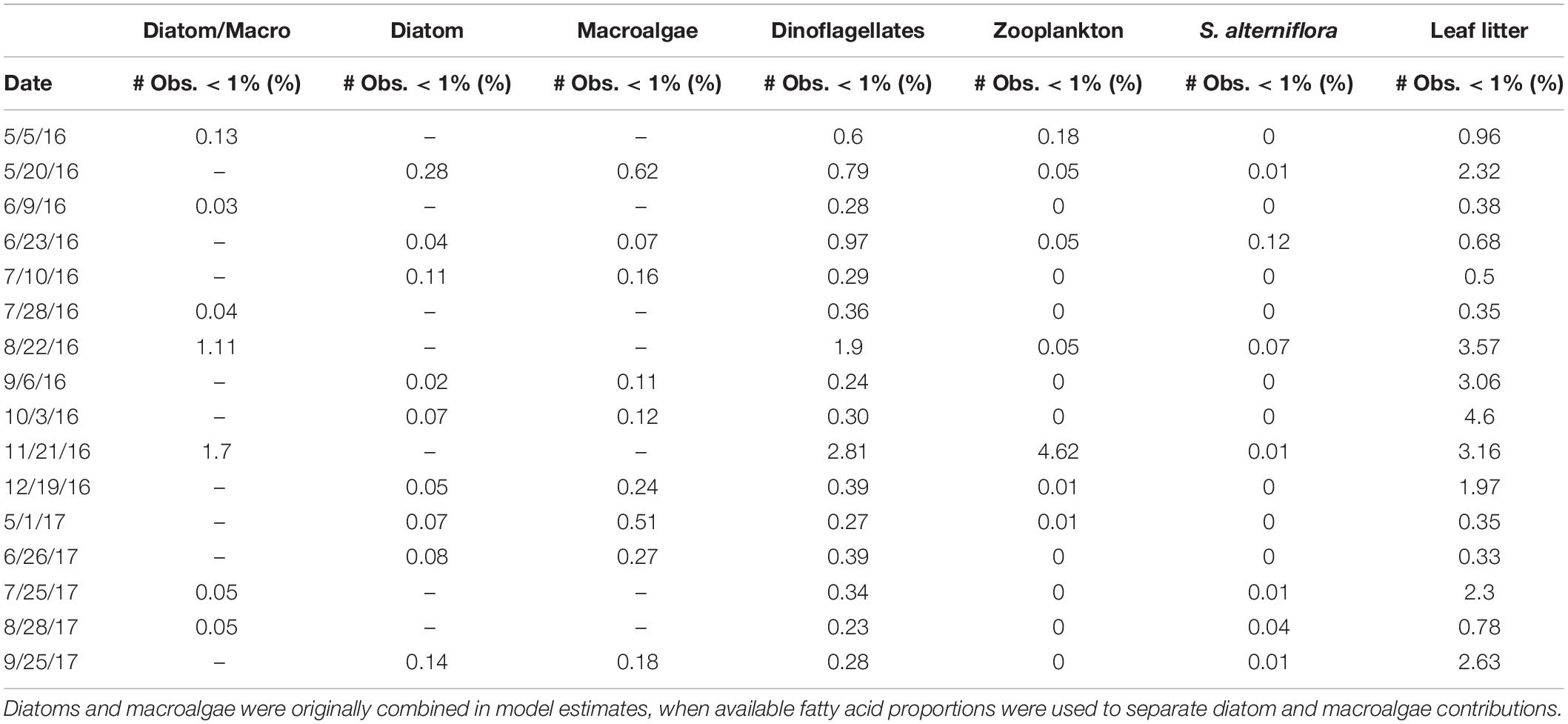
Table 4. The proportion (%) of Bayesian mixing model iterations (30 × 103) that estimated zero contribution (<1%) for each endmember (Diatom, dinoflagellate, zooplankton, macroalgae, Spartina alterniflora, and leaf litter) to the diet of Mytilus edulis in Saco Bay, ME, United States, throughout 2016 and 2017.
Discussion
It has long been hypothesized that detritus food chains predominate in salt-marsh ecosystems (Odum, 1980), and more recently that differential utilization of POM allows filter feeders to partition into trophic niches to reduce competition (Lefebvre et al., 2009; Tallis, 2009; Antonio and Richoux, 2016). Our forensic investigation of intertidal mussel diets clearly shows mussels were broad spectrum opportunistic omnivores and incorporated phytoplankton, zooplankton, macroalgal detritus, and vascular plant detritus into their diets to varying degrees. Using the measured FA biomarkers, along with insights from the Bayesian stable isotope mixing model results, we characterized the potential roles of each food source for M. edulis (Figure 11). Unsurprisingly, diatoms, dinoflagellates, and zooplankton were the primary contributors to the diet of mussels. These three food sources explained 40% of M. edulis FAs and 53% of their isotopic signature. Mussels primarily obtained the essential FAs 20:5ω3, 22:6ω3, and to a lesser extent 20:4ω6 (depending on resuspension of benthic pennate diatoms) from phytoplankton and zooplankton. In addition to their primary food sources, mussels also incorporated macroalgal detritus into their diet, which accounted for 8% of their FAs and isotopic composition (Figure 10). We suspect that although macroalgal detritus comprised a small proportion of their diet, macroalgal detritus could be important to M. edulis in supplying the essential FAs, 20:4ω6 and 20:5ω3. Although mussels could obtain 20:5ω3 from both diatoms and zooplankton, our results suggest mussels were likely limited by 20:5ω3 during summer months and would have incentive to obtain this essential FA from additional sources. In contrast, although bacteria and vascular plants also contributed to the FAs of M. edulis (5 and 3%, respectively), they do not supply essential FAs. As such, the role of bacteria and vascular plant detritus is likely only to aid mussels in meeting their energetic or carbon requirements. There were only very low probabilities (<1%) that macroalgal and S. alterniflora detritus did not contribute to the diet of M. edulis in Saco Bay based on our stable isotope model outputs (Table 4). Although it is likely that M. edulis did make some use of leaf litter, the significantly (p < 0.01) higher likelihood (<5%) and frequency that leaf litter had zero-contribution to mussel diets compared to all other endmembers suggests leaf litter use may be less important and a more sporadically used food source.
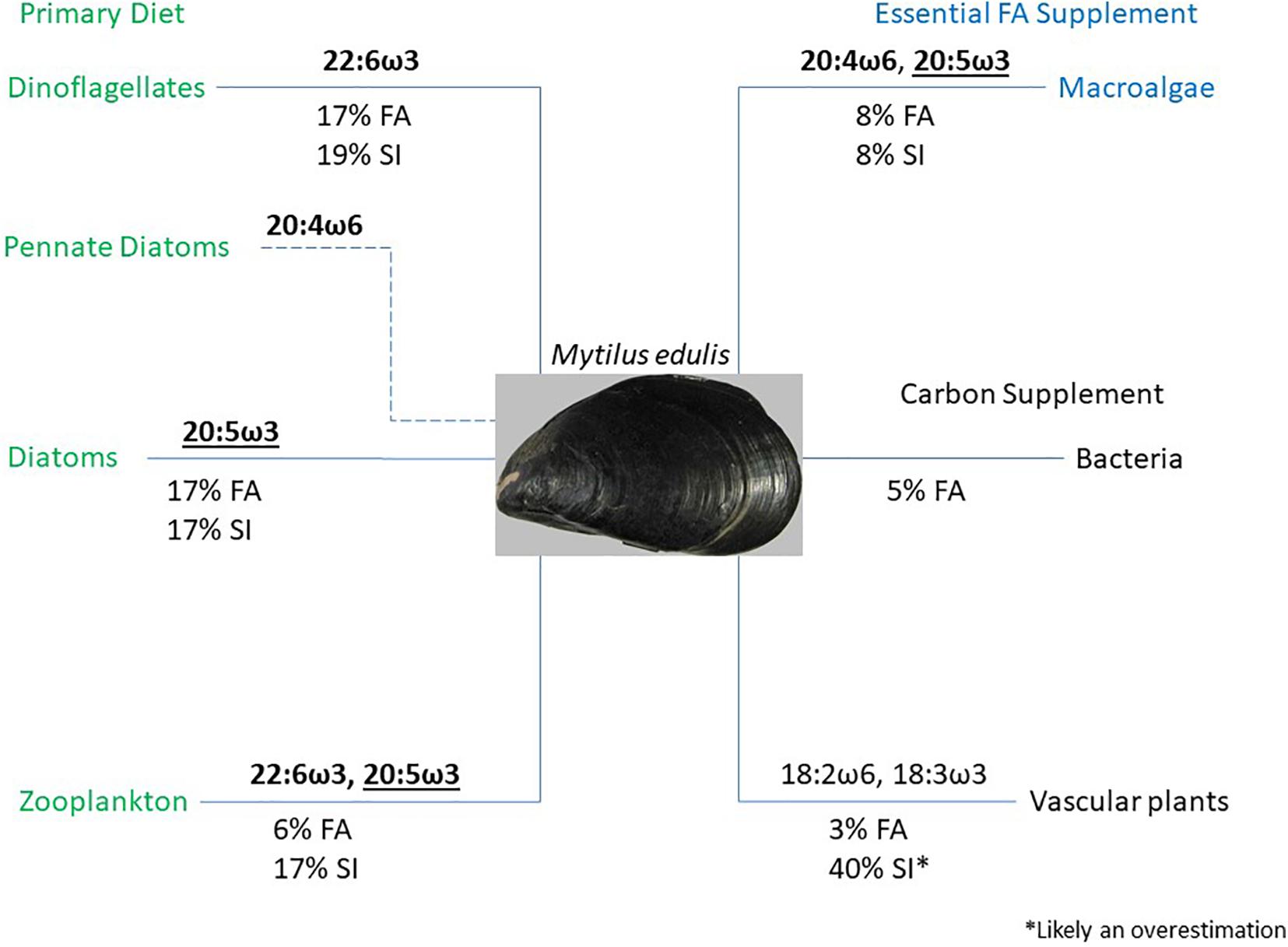
Figure 11. Flowchart depicting the contributions of dinoflagellates, diatoms, zooplankton, macroalgal detritus, bacteria, and vascular plant detritus to the diet of Mytilus edulis. Contributions are shown based on measured fatty acid (FA) biomarkers and model estimates from stable isotope (SI) composition. Important FA contributions are also shown, bold indicates essential FA, underline denotes limiting FA, and ∗ denotes likely model overestimations.
Mussels in Saco Bay were omnivores that obtained some of their dietary requirements through detrital feeding. Although M. edulis FAs primarily resembled those of dinoflagellates (22:6ω3) and diatoms (20:5ω3 and 16:1ω7) based on their biomarker content in both PCoA and SIMPER analyses, mussels did contain zooplankton (20:1 and 22:1), vascular plant detritus (18:2ω6 and 18:3ω3), and macroalgal detritus (18:1ω9, 18:3ω6, 20:2ω6, 20:4ω3, and 20:4ω6) markers (Table 3). Zooplankton were the most similar group to M. edulis, based on dinoflagellate and diatom markers, similarities which are likely due to a shared diet of phytoplankton. However, M. edulis did contain zooplankton FAs (6.2 ± 0.1%) which could only be obtained by direct consumption of either live zooplankton or zooplankton derived detritus (Dalsgaard et al., 2003). We assume that vascular plant (salt marsh and leaf litter) and macroalgal material present in POM and assimilated by mussels are detrital in origin. In addition to phytoplankton and zooplankton markers, M. edulis contained small proportions of macroalgal (7.9 ± 0.1%), bacterial (4.8 ± 0.1%), and vascular plant FAs (3.3 ± 0.1%), suggesting that M. edulis on average obtains 22.2% of their FAs from a combination of detritivory and carnivory. Mussels and oysters have previously been reported to make use of non-phytoplankton food sources such as bacteria, protozoans, zooplankton and detritus based on a variety of analyses, including stable isotopes and FA (Newell and Langdon, 1986; Baldwin and Newell, 1991; Bustamante and Branch, 1996; Davenport et al., 2000; Xu and Yang, 2007; Zhao et al., 2013). Our results further support M. edulis not being solely herbivorous. Detrital contributions to the diet of M. edulis in this study are comparable to estimated salt marsh detritus contributions to the carbon requirement of oysters (5.5%) and ribbed mussels (31%; Langdon and Newell, 1990), but are lower than previous contributions of kelp detritus (50%) to carbon requirements of Mytilus galloprovincialis (Bustamante and Branch, 1996). The latter discrepancy is perhaps the result of Saco Bay’s adjacency to Scarborough Marsh, the largest salt marsh in the region, and the abundance of marsh detritus in POM. High contributions of kelp detritus to the diet of M. galloprovincialis were in areas where kelp detritus composed large proportions (>65%) of POM, which is not the case in Saco Bay and may explain lower macroalgal contributions to the diet of M. edulis.
Although there is little doubt that M. edulis consumes phytodetritus, our methods cannot differentiate between contributions of live vs. dead phytoplankton to their diets. By assuming all phytoplankton ingested are fresh our estimates of detrital usage are inherently conservative and underestimate the importance of detritus. Diatom FAs comprised 17.2 ± 0.1% of M. edulis FAs, which agrees with the range of modeled contributions estimated with stable isotopes (Figure 10A). Proportions of dinoflagellate FAs in M. edulis averaged 16.9 ± 0.1%, which also fell within the range of modeled dietary contributions (Figure 10B). Taken together, these results suggest that M. edulis obtains at least 34% of their diet from herbivorous grazing. As such, phytoplankton contribute more FAs, and presumably total lipids, to the diet of M. edulis than any single other detrital source. This contribution of phytoplankton FAs is slightly lower than previously reported for M. edulis (39–46%; Alkanani et al., 2007), again possibly due to the proximity of Saco Bay to Scarborough Marsh. Proportions of saturated FAs, PUFA, MUFA, ω3, essential FAs and non-methylene interrupted dienes (NMID) in M. edulis were also comparable to previously reported values (Table 2; Murphy et al., 2002; Alkanani et al., 2007).
While vascular plant FAs contributed only a small proportion to M. edulis FAs (3.3 ± 0.1%) and POM (4.8 ± 0.1%), vascular plant detritus, particularly S. alterniflora detritus, comprised a significant portion of mussel diets (25.3 ± 5.4%) and POM (15.6 ± 8.4%) based on stable isotopes (Figures 9E,F, 10E,F). There are several possible explanations for this discrepancy: (1) Degraded vascular plant detritus is relatively depleted in lipids compared to their parent material and contribute more to POM and M. edulis C than lipids. (2) Vascular plant detritus contributions based on FAs are underestimates due to conversion of vascular plant FAs into bacterial FAs. (3) Diet contributions of diatoms and dinoflagellates are overestimations due to selective retention of FAs (e.g., 22:6ω3 and 20:5ω3) by M. edulis. (4) Inherent differences in what each method measures; FA biomarkers are only a subset of dietary lipids (do not sum to 100%), whereas isotope mixing models consider all C and N sources (an explicit assumption in some Bayesian models). (5) Overlap in endmember stable isotope signatures could mislead the Bayesian mixing model. For example, using an isotopically heavier δ13C for diatoms closer to −16 or −14‰, still within the reported range (Fry and Wainright, 1991), would result in lower estimations of S. alterniflora contributions. In our stable isotope mixing model S. alterniflora and macroalgae/diatoms were highly negatively correlated (r = −0.77), so improper estimation of macroalgae/diatom contributions would lead to overestimations of S. alterniflora detritus. Similarly, benthic pennate diatoms are enriched in δ13C (−13‰) and their contributions to POM and mussel diets would be misinterpreted as S. alterniflora detritus in our isotope model (Currin et al., 1995). Although less prevalent than centric diatoms, pennate diatoms do contribute to the water column of Saco Bay, particularly in September (Figure 2C).
It is important to note that due to the difficulty in collecting enough <100 μm POM for analysis, we were unable to analyze duplicate unacidified samples for δ15N. Although acidification has little effect on δ15N values of animal samples (Bosley and Wainright, 1999; Jaschinski et al., 2008), acidification can affect δ15N values of POM samples (Barrios-Guzmán et al., 2019). Because our POM samples were acidified, effects of acidification on POM δ15N values is a source of error for this study. However, we suspect that it is primarily differences in δ13C values driving the results of our mixing model, we base our suspicion on the relatively small changes in estimates when comparing alternative models with both smaller (2.3‰) and larger (5.95‰) fractionation values (Supplementary Table S6). As the shift in δ15N values due to acidification is usually minor (0.5‰; Barrios-Guzmán et al., 2019), smaller than the difference in δ15N among our alternative models, error due to the effects of acidification on POM δ15N values is unlikely to shift model estimates enough to alter the conclusions of this study.
While it is surprising that isotope modeled contributions of S. alterniflora detritus to M. edulis diets were relatively high, it is not necessarily implausible. Although the eastern oyster (Crassostrea virginica) and the ribbed mussel (Geukensia demissa) only obtained small amounts of their required carbon from S. alterniflora detritus (0.7 and 8.6% respectively), they obtained larger proportions of their carbon requirements (5.5 and 31% respectively) from the associated bacteria (Langdon and Newell, 1990). Minimum isotope modeled contributions of S. alterniflora detritus in this study (9.7–25.0%) fall within the upper range of Langdon and Newell’s (1990) values suggesting they are feasible. However, the mean (17.7–41.7%) and maximum (25.0–58.0%) estimates seem less likely. Combined bacterial and vascular plant FAs represented 8.1% of mussel FAs, much lower than the 19–29% minimum modeled contributions of vascular plants based on stable isotopes. Attributing bacterial FAs to vascular plant detritus consumption, assumes that all bacterial FAs measured in M. edulis were from their tissue and not from bacteria growing on them, as well as that all bacterial FAs ingested by M. edulis were from vascular plant sources. Both assumptions are unlikely and, even considering that detritus is likely to be lipid depleted, our model almost certainly over-estimated S. alterniflora contributions to mussel diets. Higher proportional contributions of vascular plant FAs (∼11% total FAs) in the Mediterranean mussel (Mytilus galloprovincialis; Zhao et al., 2013), further suggest that lipid depletion of vascular plant detritus is unlikely to fully explain the higher contributions of vascular plant detritus in our model. Due to the close proximity of Scarborough marsh, the largest salt marsh in Maine, and the “outwelling hypothesis” we expected an abundance of S. alterniflora detritus for M. edulis to consume (Odum and de la Cruz, 1967). Both our measured FAs and model estimates confirm that S. alterniflora contributed to POM (Figure 9E), although our model estimates for POM are also likely overestimations. Our minimum stable isotope estimates of S. alterniflora (9.7–25.0%) are comparable to isotope modeled S. alterniflora contributions to mussel diets (10–12%) near other large marshes (Riera, 2007). However, the mean (17.7–41.7%) and maximum (25.0–58.0%) estimates are again much higher, further corroborating they are likely overestimations.
Macroalgae comprised a modest proportion (∼10%) of the diet of M. edulis (Figure 10C). Consumption of macroalgal detritus by M. edulis is unsurprising given that macroalgal detritus is known to contribute to nearshore food webs and bivalve diets elsewhere (Duggins et al., 1989; Fredriksen, 2003; Kaehler et al., 2006; Tallis, 2009; Allan et al., 2010; Von Biela et al., 2016). Additionally, macroalgae have been shown to be a long-term dietary component for bivalves in areas with strong seasonal production of phytoplankton. The Antarctic scallop (Adamussium colbecki) utilized phytoplankton and zooplankton in the short-term during productive summer seasons, based on gut δ13C and δ15N, but their tissue δ13C and δ15N indicated the use of the red algae Iridea cordata as a long-term dietary item (Calizza et al., 2018). Detrital contributions from macroalgae were on the lower end of previously reported values for other bivalves. Macroalgal detritus can contribute small (10–20%) to large (>50%) proportions of bivalve diets (Bustamante and Branch, 1996; Fredriksen, 2003; Allan et al., 2010) and have also been successfully incorporated into hatchery diets at rations of 50–90% (Camacho et al., 2007; Carboni et al., 2016). Lower contributions of macroalgal detritus in this study, could be due to differences in the quality of detrital particulates, or the abundance of salt marsh detritus. Quality of macroalgal detritus varies with species, age, and concentration of secondary metabolites, such as polyphenols (Cranford and Grant, 1990; Duggins and Eckman, 1997). There are large amounts of polyphenols (5–15% DW) in both A. nodosum and S. latissima (Ragan and Jensen, 1978; Wang et al., 2009). These concentrations are equivalent to or greater than those previously shown to inhibit bivalve grazing of fresh material (Duggins and Eckman, 1997), suggesting polyphenols could have deterred ingestion by M. edulis.
Although macroalgal detritus did not comprise a large proportion of M. edulis diets, its role may still be significant. Macroalgae have large proportions (7–18%) of the essential FA arachidonic acid (20:4ω6; see Supplementary Table S1), which is found only in small amounts (∼1%) in dinoflagellates and most diatoms (Ackman and Tocher, 1968; Nichols et al., 1984; Dunstan et al., 1994; Mansour et al., 1999, 2003; Leblond and Chapman, 2000; Arts et al., 2001). Arachidonic acid, when used in conjunction with other ω6 FAs, has been significantly correlated with mussel growth (Alkanani et al., 2007) and, importantly, was selectively retained by mussels in the current study (Figure 5). Supplementation of 20:4ω6 by macroalgal detritus could potentially explain why bivalves fed mixtures of macroalgal detritus and phytoplankton performed better than bivalves fed solely phytoplankton in previous studies (Carboni et al., 2016).
Decreases in lipid and total FA concentrations in M. edulis throughout the summer, accompanied by an increase in the proportion of NMIDs and essential FAs (20:4ω6 and 22:6ω3), suggests that mussels were physiologically stressed and preferentially retaining essential FAs (Figures 5, 6). Between spring and summer mussel lipid content decreased by 52%, a decrease that was generally mirrored across all FAs (SAT: 61%, MUFA: 58%, PUFA: 58%, ω3: 59%) including essential FAs, such as 20:5ω3, which decreased 64%. However, the decreases in 20:4ω6 (48%) and 22:6ω3 (57%) were lower, suggesting selective retention of these FAs. Growth of M. edulis has been negatively correlated with the proportion of NMIDs present in their FAs and NMIDs are negatively correlated with essential FAs (Alkanani et al., 2007). Mussels are capable of synthesizing NMIDs de novo and are believed to do so to substitute for essential ω3 FAs lacking in their diet (Zhukova and Svetashev, 1986; Zhukova et al., 1992; Alkanani et al., 2007). The negative correlations between NMID and growth of mussels suggests their synthesis is metabolically costly, considering co-occurring reductions in mussel 20:5ω3, mussels were likely stressed and either unable to completely compensate with NMID production, or 20:5ω3 was more dispensable to mussels than 20:4ω6 and 22:6ω3. In finfish, 20:5ω3 is often more dispensable than 22:6ω3 and its supplementation into diets does not improve growth (Trushenski et al., 2012), if also true for mussels, supplementation of 20:5ω3 may not result in better performance. However, the negative correlations of NMIDs between mussel growth and 20:5ω3 suggests supplementing 20:5ω3 could improve mussel growth and warrants further study.
Selective retention of FAs could affect interpretation of diet contributions based on biomarker FAs. Both selectively retained FAs (20:4ω6 and 22:6ω3) were used as biomarkers in this study, for macroalgae and diatoms respectively. By selectively retaining these biomarker FAs, the contribution of both macroalgae and diatoms to mussel diets would appear larger. Effects of consumer metabolism on the interpretation of biomarker FAs is well known and has led to the development of Quantitative Fatty Acid Signature Analysis (QFASA; Iverson et al., 2004). In QFASA, calibration coefficients are used to account for the lipid metabolism of consumers, like trophic retention factors account for changes in consumer stable isotopes. Although useful for dealing with effects of consumer lipid metabolism, controlled feeding experiments are required to compute QFASA calibration coefficients. Due to a lack of controlled feeding experiments, we are unable to calculate calibration coefficients for M. edulis consuming diatoms and macroalgae. As such, it is important to recognize that contributions of macroalgal detritus and diatoms to the diet of mussels in this study may be slight over-estimations because mussels selectively retain 20:4ω6 and 22:6ω3.
Mussels may have been limited by dietary 20:5ω3 and consequently used macroalgal detritus to supplement their dietary requirements. Macroalgae contained the essential FA 20:5ω3 in large proportions (8–26%; see Supplementary Material). Eicosapentaenoic acid (EPA; 20:5ω3) is important for maintaining membrane fluidity and there is a strong relationship between 20:5ω3 and temperature in the gills of scallops (Hall et al., 2002). In the current study, only the proportion of the essential FA 20:5ω3 decreased in conjunction with increasing NMIDs (Figures 5, 6), suggesting that NMIDs were synthesized to replace 20:5ω3. Based on this negative correlation, 20:5ω3 may have been a limiting FA for mussels. The phytoplankton community of Saco Bay is dominated by dinoflagellates (Figure 2), so it is possible that limitations in diatom availability caused the limitation of 20:5ω3 for mussels. Thus mussels could make use of macroalgal detritus to supplement their diet with 20:4ω6 and 20:5ω3; this supplemental effect might explain why bivalve growth was found to increase when supplied with a mixed diet of macroalgal detritus and live phytoplankton in previous studies (Camacho et al., 2007). The period in late spring and early summer when mussels were physiologically stressed coincides with when macroalgal detritus contributed proportionally more to POM (Figure 9). Stressed mussels, potentially limited in 20:5ω3, would be incentivized to make use of macroalgal detritus as an alternative essential FA source. Higher proportional contributions of macroalgal detritus to POM in late spring and early summer also resulted in higher 20:4ω6-to-22:6ω3 and 20:4ω6-to-20:5ω3 ratios (Figure 4). Macroalgae have a history of use as a supplemental diet in agriculture. Although considered an inferior ingredient, based on proximate composition and low digestibility, macroalgae is still supplemented into animal diets in low amounts (3–10%) due to prebiotic effects which result in increased health and productivity (Evans and Critchley, 2014). Could macroalgal detritus serve a similar role for bivalves and provide small amounts of micronutrients such as essential FAs?
While 20:4ω6 only occurs in small proportions in most diatoms, some pennate diatoms can have larger proportions (2–6%: Dunstan et al., 1994). Microphytobenthos production can represent a significant portion of total productivity, which when resuspended can directly contribute to the water column phytoplankton assemblage (MacIntyre et al., 1996). Pacific oysters, Crassostrea gigas, are capable of selectively feeding on resuspended pennate diatoms from the microphytobenthos (Cognie et al., 2001). Additionally, microphytobenthos have been shown to contribute (0.4–4.4%) to the diets of both wild and cultivated bivalves (Riera, 2007). Another bivalve, Macoma balthica, has also been shown to consume benthic diatoms in another Maine estuary connected to the Damariscotta river (Incze et al., 1982). It was hypothesized based on δ13C values that M. balthica were feeding on the isotopically heavy (−12.4‰) Amphipleura rutilans. Pennate diatoms were present in the water column of Saco Bay in September, a time when POM δ13C values became enriched (Figure 2C; see Both et al., 2020 for more details) It therefore seems likely that pennate diatoms contributed 20:4ω6 to the diet of M. edulis during September, potentially explaining some of the discrepancy between stable isotope modeled estimates and measured FAs. However, at other times (e.g., June) when pennate diatoms were less abundant, macroalgal detritus may be a more important source of 20:4ω6 (Figure 2C). M. edulis does not always maximize energy gain in the short-term and can rely upon energy reserves for gametogenesis, or to avoid feeding inefficiently upon largely inorganic particulates (Hawkins et al., 1985). Although macroalgal detritus consumption is unlikely to be as energy efficient as fresh phytoplankton consumption, when physiologically stressed and potentially limited by essential FAs, M. edulis may feed on less energetically optimized macroalgal detritus to obtain physiologically important micronutrients such as essential FAs.
Ratios of FA biomarkers in POM confirm that macroalgal detritus was present in the water column during early summer. A well-known FA biomarker for diatoms is 16:1ω7 (Parrish, 2013), and because of their higher proportion of 20:4ω6, pennate diatoms (0.01–0.25) have a higher ratio of 20:4ω6-to-16:1ω7 than centric diatoms (0.01–0.07; Dunstan et al., 1994). With higher proportional abundances of 20:4ω6 and lower proportional abundances of 16:1ω7 than pennate diatoms, macroalgae have an even higher ratio of 20:4ω6-to-16:1ω7 (A. nodosum: 6.8 ± 0.1, S. latissima: 4.8 ± 1.3, C. crispus: 0.8 ± 0.1). In this study, 18:1ω9 was an abundant FA in macroalgae (especially A. nodosum) which led to its use as a biomarker (see Supplementary Material). By comparing the ratio of the macroalgal marker 18:1ω9 to the diatom marker 16:1ω7, we can estimate whether there were macroalgae or diatom FAs present in the water column. The ratio of 18:1ω9-to-16:1ω7 is low in both centric (0.01–0.43) and pennate (0.01–0.27) diatoms (Dunstan et al., 1994), while the ratio of 18:1ω9-to-16:1ω7 is much higher in macroalgae (A. nodosum: 20.1 ± 0.4, S. latissima: 2.4 ± 0.1, C. crispus: 0.6 ± 0.1). Consequently, an increase in the ratio of both 20:4ω6-to-16:1ω7 and 18:1ω9-to-16:1ω7 indicates the presence of macroalgae, whereas an increase in the ratio of only 20:4ω6-to-16:1ω7 indicates pennate diatoms. In our study, both 20:4ω6-to-16:1ω7 and 18:1ω9-to-16:1ω7 are elevated in late spring and early summer confirming the presence of macroalgal detritus in the water column, while in the fall only the ratio of 20:4ω6-to-16:1ω7 was elevated suggesting pennate diatoms were the source of 20:4ω6 (Figures 4B,C). Contributions of macroalgal detritus to POM represent an additional avenue for mussels to obtain 20:4ω6 which is not reliant on resuspension of benthic pennate diatoms. Through multiple lines of evidence, our study makes it clear that mussels have a diverse diet with multiple pathways to obtain essential FAs.
It should be noted that, detrital use by M. edulis is likely to vary depending on their habitat and the available detrital resources. Different ecosystems will have different types, but also proportions, of detrital material in their POM for M. edulis to ingest. For example, the diet of the scallop (A. colbecki) varied with both depth and season as available POM shifted (Calizza et al., 2018). This may be particularly important for offshore aquaculture, where nearshore detrital inputs are likely to be reduced. Additionally, although some areas may have similar proportions of detrital inputs from various taxonomic groups (e.g., vascular plant, macroalgae, phytodetritus), the species within those groups may vary (e.g., different species of macroalgae). Detritus produced from different species will have different compositions (e.g., C:N, presence of secondary metabolites, and different FA profiles) which will affect the ability of M. edulis to exploit them. For example, the food quality of macroalgal particulates is known to vary with species for M. edulis consumers (Duggins and Eckman, 1997), so macroalgal detritus contributions to M. edulis will likely vary between ecosystems with different species of macroalgae. As such, it is important to take into consideration both the available sources (taxonomic groups and temporal availability) and composition of detrital resources within an ecosystem when considering their potential value for bivalve consumers.
We show that M. edulis in Saco Bay are omnivores and diversify their diet by incorporating multiple detrital sources. However, more work is needed to understand not only when, but why, and in what environments mussels make use of detrital subsidies. Feeding trials to confirm the ingestion and bioavailability of macroalgal FAs would help validate macroalgal detritus as an avenue for mussels to obtain essential FAs. Compound specific SIA of detrital FA biomarkers could also be used to more clearly trace organic matter within ecosystems. Additionally, more work is needed to determine whether detrital subsidies are required to meet mussel metabolic demands. These are important ecological questions and regarding aquaculture siting, if M. edulis does not require detrital inputs then siting strictly based on available chlorophyll is valid. However, if detrital inputs are required, estimates of detrital abundance and quality will be needed to properly predict growth, carrying capacities, and environmental interactions of bivalve aquaculture. Understanding detrital requirements could be particularly important for offshore aquaculture, which may result in growing areas with minimal contributions from nearshore detrital inputs and water depths that inhibit the ability of resuspended material to contribute to bivalve diets.
Conclusion
Mytilus edulis obtained at least 22.2% of their assimilated lipid from omnivorous feeding, of which at least 16% was detrital feeding based on FAs. Of all the detrital sources ingested, macroalgal detritus arguably warrants the most attention for further studies. Although macroalgal detritus only comprised a small proportion (5–11%) of M. edulis diet, its contribution to M. edulis growth could be significant. Macroalgal detritus had large amounts of the essential FAs 20:4ω6 and 20:5ω3 and could be an avenue for mussels to supplement their essential FA requirements. Despite phytoplankton being a more important food source for bivalves, diversifying their organic matter consumption through detrital food sources offer alternate pathways for mussels to supplement their catabolic energy and essential FA requirements. Although the importance of detrital subsidies is still unclear in an ecological context, detrital material may be a useful resource to consider when culturing bivalves or siting bivalve aquaculture (e.g., macroalgal detritus increasing availability of essential FA in POM).
Data Availability Statement
The raw data supporting the conclusions of this article will be made available by the authors, without undue reservation.
Author Contributions
AB designed the study, collected and processed the data, and wrote the manuscript. CB, DB, BC-P, and CP advised and guided the development of the study, provided insight and input during data processing, and edited the manuscript. All the authors contributed to the article and approved the submitted version.
Funding
This study was funded by the Sustainable Ecological Aquaculture Network (SEANET; Grant #1355457) and an NSF Maine EPSCoR grant (1355457) (https://www.nsf.gov/awardsearch/showAward?AWD_ID=1355457&HistoricalAwards=false). Publication fees for this article were covered by the Maine Agricultural and Forest Experiment Station (MAFES). Funding from USDA ARS Project NACA 58-8030-0-004 made this work possible.
Conflict of Interest
The authors declare that the research was conducted in the absence of any commercial or financial relationships that could be construed as a potential conflict of interest.
Acknowledgments
We would like to thank Lawrence Mayer for his advice, expertise, and guidance throughout the development of this study. We would like to acknowledge all the staff at the University of New England’s Marine Science Center, ME, United States, for their help collecting samples as well as Ursula Roese for her technical expertise and support. We would also like to thank the Maine Agriculture and Field Experiment Station (MAFES) for funding the publication of this manuscript.
Supplementary Material
The Supplementary Material for this article can be found online at: https://www.frontiersin.org/articles/10.3389/fmars.2020.561073/full#supplementary-material
References
Ackman, R. G., and Tocher, C. S. (1968). Marine phytoplanktoner fatty acids. Fish. Res. Bp. Canada 25, 1603–1620. doi: 10.1139/f68-145
Alkanani, T., Parrish, C. C., Thompson, R. J., and McKenzie, C. H. (2007). Role of fatty acids in cultured mussels, Mytilus edulis, grown in Notre Dame Bay, Newfoundland. J. Exp. Mar. Bio. Ecol. 348, 33–45. doi: 10.1016/J.JEMBE.2007.02.017
Allan, E. L., Ambrose, S. T., Richoux, N. B., and Froneman, P. W. (2010). Determining spatial changes in the diet of nearshore suspension-feeders along the South African coastline: stable isotope and fatty acid signatures. Estuar. Coast. Shelf Sci. 87, 463–471. doi: 10.1016/j.ecss.2010.02.004
Antonio, E. S., and Richoux, N. B. (2016). Tide-induced variations in the fatty acid composition of estuarine particulate organic matter. Estuar. Coasts 39, 1072–1083. doi: 10.1007/s12237-015-0049-x
Arambalza, U., Urrutia, M. B., Navarro, E., and Ibarrola, I. (2010). Ingestion, enzymatic digestion and absorption of particles derived from different vegetal sources by the cockle Cerastoderma edule. J. Sea Res. 64, 408–416. doi: 10.1016/J.SEARES.2010.06.003
Arar, E. J., and Collins, G. B. (1997). In Vitro Determination of chlorophyll a and pheophytin a in marine and freshwater algae by fluorescence. EPA Method 445:22. doi: 10.2216/i0031-8884-32-1-22.1
Arts, M. T., Ackman, R. G., and Holub, B. J. (2001). Essential fatty acids” in aquatic ecosystems: a crucial link between diet and human health and evolution. Can. J. Fish. Aquat. Sci. 58, 122–137. doi: 10.1139/cjfas-58-1-122
Bachok, Z., Meziane, T., Mfilinge, P. L., and Tsuchiya, M. (2009). Fatty acid markers as an indicator for temporal changes in food sources of the bivalve Quidnipagus palatum. Aquat. Ecosyst. Health Manag. 12, 390–400. doi: 10.1080/14634980903347589
Bachok, Z., Mfilinge, P. L., and Tsuchiya, M. (2003). The diet of the mud clam Geloina coaxans (Mollusca, Bivalvia) as indicated by fatty acid markers in a subtropical mangrove forest of Okinawa, Japan. J. Exp. Mar. Bio. Ecol. 292, 187–197. doi: 10.1016/S0022-0981(03)00160-6
Baldwin, B. S., and Newell, R. I. E. (1991). Omnivorous feeding by planktotrophic larvae of the eastern oyster Crassostrea virginica. Mar. Ecol. Prog. Ser. 78, 285–301. doi: 10.3354/meps078285
Barber, D. C. (1995). Holocene evolution and modern sand budget of inner Saco Bay, Maine. Orono, ME: University of Maine.
Barrios-Guzmán, C., Sepúlveda, M., Docmac, F., Zarate, P., Reyes, H., and Harrod, C. (2019). Sample acidification has a predictable effect on isotopic ratios of particulate organic matter along the Chilean coast. Rapid Commun. Mass Spectrom 33, 1652–1659. doi: 10.1002/rcm.8511
Beaulieu, S. E. (2002). “Accumulation and fate of phytodetritus on the sea floor,” in Oceanography and Marine Biology: an Annual Review, eds R. N. Gibson, M. Barnes, and R. J. A. Atkinson (Milton Park: Taylor & Francis), 171–232. doi: 10.1201/9780203180594
Bosley, K. L., and Wainright, S. C. (1999). Effects of preservatives and acidification on the stable isotope ratios (15N: 14N, 13C: 12C) of two species of marine animals. Can. J. Fish. Aquat. Sci. 56, 2181–2185. doi: 10.1139/f99-153
Both, A., Byron, C. J., Brady, D. C., Costa-Pierce, B., Mayer, L. M., and Parrish, C. C. (2020). Sourcing and evaluating the use of detritus as a supplementary diet for bivalve aquaculture using stable isotopes and fatty acid biomarkers. Electron. Theses Diss. 3159:130.
Budge, S. M., Parrish, C. C., and McKenzie, C. H. (2001). Fatty acid composition of phytoplankton, settling particulate matter and sediments at a sheltered bivalve aquaculture site. Mar. Chem. 76, 285–303. doi: 10.1016/S0304-4203(01)00068-8
Bustamante, R. H., and Branch, G. M. (1996). The dependence of intertidal consumers on kelp-derived organic matter on the west coast of South Africa. J. Exp. Mar. Bio. Ecol. 196, 1–28. doi: 10.1016/0022-0981(95)00093-3
Byron, C., Link, J., Costa-Pierce, B., and Bengtson, D. (2011a). Calculating ecological carrying capacity of shellfish aquaculture using mass-balance modeling: narragansett Bay. Rhode Island. Ecol. Model. 222, 1743–1755. doi: 10.1016/j.ecolmodel.2011.03.010
Byron, C., Link, J., Costa-Pierce, B., and Bengtson, D. (2011b). Modeling ecological carrying capacity of shellfish aquaculture in highly flushed temperate lagoons. Aquaculture 314, 87–99. doi: 10.1016/j.aquaculture.2011.02.019
Calizza, E., Careddu, G., Sporta Caputi, S., Rossi, L., and Costantini, M. L. (2018). Time- and depth-wise trophic niche shifts in Antarctic benthos. PLoS One 13:e0194796. doi: 10.1371/journal.pone.0194796
Camacho, P., Salinas, J. M., Fuertes, C., and Delgado, M. (2004). Preparation of single cell detritus from Laminaria saccharina as a hatchery diet for bivalve mollusks. Mar. Biotechnol. 6, 642–649. doi: 10.1007/s10126-004-2901-z
Camacho, P. A., Salinas, J. M., Delgado, M., and Fuertes, C. (2007). Use of single cell detritus (SCD) produced from Laminaria saccharina in the feeding of the clam Ruditapes decussatus (Linnaeus, 1758). Aquaculture 266, 211–218. doi: 10.1016/j.aquaculture.2006.12.033
Carboni, S., Clegg, S. H., and Hughes, A. D. (2016). The use of biorefinery by-products and natural detritus as feed sources for oysters (Crassostrea gigas) juveniles. Aquaculture 464, 392–398. doi: 10.1016/j.aquaculture.2016.07.021
Carreón-Palau, L., Parrish, C. C., del Angel-Rodríguez, J. A., Pérez-España, H., and Aguiñiga-García, S. (2013). Revealing organic carbon sources fueling a coral reef food web in the Gulf of Mexico using stable isotopes and fatty acids. Limnol. Oceanogr. 58, 593–612. doi: 10.4319/lo.2013.58.2.0593
Cebrian, J. (1999). Patterns in the fate of production in plant communities. Am. Nat. 154, 449–468. doi: 10.1086/303244
Cebrian, J., and Lartigue, J. (2004). Patterns of herbivory and decomposition in aquatic and terrestrial ecosystems. Ecol. Monogr. 74, 237–259. doi: 10.1890/03-4019
Chapman, A. R. O., and Johnson, C. R. (1990). Disturbance and organization of macroalgal assemblages in the Northwest Atlantic. Hydrobiologia 192, 77–121. doi: 10.1007/BF00006228
Cognie, B., Barillé, L., and Rincé, Y. (2001). Selective feeding of the oyster Crassostrea gigas fed on a natural microphytobenthos assemblage. Estuar. Res. Fed. Estuaries 24, 126–131. doi: 10.2307/1352819
Coleman, D. C., Reid, C. P. P., and Cole, C. V. (1983). Biological strategies of nutrient cycling in soil systems. Adv. Ecol. Res. 13, 1–55. doi: 10.1016/S0065-2504(08)60107-5
Comeau, L. A., Drapeau, A., Landry, T., and Davidson, J. (2008). Development of longline mussel farming and the influence of sleeve spacing in Prince Edward Island, Canada. Aquaculture 281, 56–62. doi: 10.1016/j.aquaculture.2008.05.031
Cranford, P. J., and Grant, J. (1990). Particle clearance and absorption of phytoplankton and detritus by the sea scallop Placopecten magellanicus (Gmelin). J. Exp. Mar. Bio. Ecol. 137, 105–121. doi: 10.1016/0022-0981(90)90064-J
Currin, C., Newell, S., and Paerl, H. (1995). The role of standing dead Spartina alterniflora and benthic microalgae in salt marsh food webs: considerations based on multiple stable isotope analysis. Mar. Ecol. Prog. Ser. 121, 99–116. doi: 10.3354/meps121099
Dalsgaard, J., St John, M., Kattner, G., Müller-Navarra, D., and Hagen, W. (2003). Fatty acid trophic markers in the pelagic marine environment. Adv. Mar. Biol. 46, 225–340. doi: 10.1016/S0065-2881(03)46005-7
Davenport, J., Smith, R. J. J. W., and Packer, M. (2000). Mussels Mytilus edulis: significant consumers and destroyers of mesozooplankton. Mar. Ecol. Prog. Ser. 198, 131–137. doi: 10.3354/meps198131
Decottignies, P., Beninger, P. G., Rincé, Y., and Riera, P. (2007). Trophic interactions between two introduced suspension-feeders, Crepidula fornicata and Crassostrea gigas, are influenced by seasonal effects and qualitative selection capacity. J. Exp. Mar. Bio. Ecol. 342, 231–241. doi: 10.1016/j.jembe.2006.10.005
Dethier, M. N., Brown, A. S., Burgess, S., Eisenlord, M. E., Galloway, A. W. E., Kimber, J., et al. (2014). Degrading detritus: changes in food quality of aging kelp tissue varies with species. J. Exp. Mar. Bio. Ecol. 460, 72–79. doi: 10.1016/j.jembe.2014.06.010
Duggins, D., Simenstad, C., and Estes, J. (1989). Magnification of secondary producition by kelp detritus in coastal marine ecosystems. Sci. New Ser. 245, 170–173. doi: 10.1126/science.245.4914.170
Duggins, D. O., and Eckman, J. E. (1997). Is kelp detritus a good food for suspension feeders? Effects of kelp species, age and secondary metabolites. Mar. Biol. 128, 489–495. doi: 10.1007/s002270050115
Dunstan, G. A., Volkman, J. K., Barrett, S. M., Leroi, J.-M., and Jeffrey, S. W. (1994). Essential polyunsaturated fatty acids from 14 species of diatom (Bacillariophyceae). Phytochemistry 35, 155–161. doi: 10.1016/s0031-9422(00)90525-9
Ehleringer, J. R., and Cerling, T. E. (2001). “C3 and C4 photosynthetis,” in Encylopedia of Global Environmental Change, Vol. II, eds H. A. Mooney and J. Canadell (New York: John Wiley and Sons), 186–190.
Enríquez, S., Duarte, C. M., and Sand-Jensen, K. (1993). Patterns in decomposition rates among photosynthetic organisms: the importance of detritus C:N:P content. Oecologia 94, 457–471. doi: 10.1007/BF00566960
Evans, F. D., and Critchley, A. T. (2014). Seaweeds for animal production use. J. Appl. Phycol. 26, 891–899. doi: 10.1007/s10811-013-0162-9
Ezgeta-Balić, D., Najdek, M., Peharda, M., and Blažina, M. (2012). Seasonal fatty acid profile analysis to trace origin of food sources of four commercially important bivalves. Aquaculture 334–337, 89–100. doi: 10.1016/j.aquaculture.2011.12.041
Fredriksen, S. (2003). Food web studies in a Norwegian kelp forest based on stable isotope (δ13C and δ15N) analysis. Mar. Ecol. Prog. Ser. 260, 71–81. doi: 10.3354/meps260071
Fry, B. (2006). Stable Isotope Ecology. New York, NY: Springer Science and Business Media LLC, doi: 10.1007/0-387-33745-8
Fry, B., and Wainright, S. C. (1991). Diatom sources of 13C-rich carbon in marine food webs. Mar. Ecol. Prog. Ser. 76, 149–157. doi: 10.3354/meps076149
George, E. M., and Parrish, C. C. (2015). Invertebrate uptake of lipids in the vicinity of Atlantic salmon (Salmo salar) aquaculture sites in British Columbia. Aquac. Res. 46, 1044–1065. doi: 10.1111/are.12259
Grant, J. (1996). The relationship of bioenergetics and the environment to the field growth of cultured bivalves. J. Exp. Mar. Bio. Ecol. 200, 239–256. doi: 10.1016/S0022-0981(96)02660-3
Grant, J., Cranford, P., Hargrave, B., Carreau, M., Schofield, B., Armsworthy, S., et al. (2005). A model of aquaculture biodeposition for multiple estuaries and field validation at blue mussel (Mytilus edulis) culture sites in eastern Canada. Can. J. Fish. Aquat. Sci. 62, 1271–1285. doi: 10.1139/F05-033
Grant, J., and Cranford, P. J. (1991). Carbon and nitrogen scope for growth as a function of diet in the sea scallop Placopecten Magellanicus. J. Mar. Biol. Assoc. U.K. 71:437. doi: 10.1017/S0025315400051699
Guest, M. A., Nichols, P. D., Frusher, S. D., and Hirst, A. J. (2008). Evidence of abalone (Haliotis rubra) diet from combined fatty acid and stable isotope analyses. Mar. Biol 153, 579–588. doi: 10.1007/s00227-007-0831-9
Hall, J. M., Parrish, C. C., and Thompson, R. J. (2002). Eicosapentaenoic acid regulates scallop (Placopecten magellanicus) membrane fluidity in response to cold. Biol. Bull. 202, 201–203. doi: 10.2307/1543469
Hawkins, A. J. S., Duarte, P., Fang, J. G., Pascoe, P. L., Zhang, X. L., and Zhu, M. Y. (2002). A functional model of responsive suspension-feeding and growth in bivalve shellfish, configured and validated for the scallop Chlamys farreri during culture in China. J. Exp. Mar. Bio. Ecol. 281, 13–40. doi: 10.1016/S0022-0981(02)00408-2
Hawkins, A. J. S., Pascoe, P. L., Parry, H., Brinsley, M., Black, K. D., McGonigle, C., et al. (2013). Shellsim: a generic model of growth and environmental effects validated across contrasting habitats in bivalve shellfish. J. Shellfish Res. 32, 237–253. doi: 10.2983/035.032.0201
Hawkins, A. J. S., Salkeld, P. N., Bayne, B. L., Gnaiger, E., and Lowe, D. M. (1985). Feeding and resource allocation in the mussel Mytilus edulis: evidence for time-averaged optimization. Mar. Ecol. Prog. Ser. 20, 273–287. doi: 10.3354/meps020273
Hill, J., McQuaid, C., and Kaehler, S. (2006). Biogeographic and nearshore–offshore trends in isotope ratios of intertidal mussels and their food sources around the coast of southern Africa. Mar. Ecol. Prog. Ser. 318, 63–73. doi: 10.3354/meps318063
Incze, L. S., Mayer, L. M., Sherr, E. B., and Macko, S. A. (1982). Carbon inputs to bivalve mollusks: a comparison of two estuaries. Can. J. Fish. Aquat. Sci. 39, 1348–1352. doi: 10.1139/f82-181
Irisarri, J., Fernández-Reiriz, M.-J., De Troch, M., and Labarta, U. (2014). Fatty acids as tracers of trophic interactions between seston, mussels and biodeposits in a coastal embayment of mussel rafts in the proximity of fish cages. Comp. Biochem. Physiol. Part B 172-173, 105–115. doi: 10.1016/j.cbpb.2014.04.006
Iverson, S. J., Field, C., Don Bowen, W., and Blanchard, W. (2004). Quantitative fatty acid signature analysis: a new method of estimating predator diets. Ecol. Monogr. 74, 211–235. doi: 10.1890/02-4105
Jacobson, H. A., Jacobson, G. L., and Kelley, J. T. (1987). Distribution and abundance of tidal marshes along the coast of Maine. Estuaries 10:126. doi: 10.2307/1352176
Jaschinski, S., Hansen, T., and Sommer, U. (2008). Effects of acidification in multiple stable isotope analyses. Limnol. Oceanogr. Methods 6, 12–15. doi: 10.4319/lom.2008.6.12
Jensen, R. E. (1983). Atlantic Coast Hindcast, Shallow-Water, Significant Wave Information. Washington DC: National Technical Information Service.
Kaehler, S., Pakhomov, E. A., Kalin, R. M., and Davis, S. (2006). Trophic importance of kelp-derived suspended particulate matter in a through-flow sub-Antarctic system. Mar. Ecol. Prog. Ser. 316, 17–22. doi: 10.3354/meps316017
Kelley, J. T., Barber, D. C., Belknap, D. F., Fitzgerald, D. M., Van Heteren, S., Dickson, S. M., et al. (2005). Sand budgets at geological, historical and contemporary time scales for a developed beach system, Saco Bay, Maine, USA. Mar. Geol. 214, 117–142. doi: 10.1016/j.margeo.2004.10.027
Kelly, J. R., and Scheibling, R. E. (2012). Fatty acids as dietary tracers in benthic food webs. Mar. Ecol. Prog. Ser. 446, 1–22. doi: 10.3354/meps09559
Kharlamenko, V. I., Zhukova, N. V., Khotimchenko, S. V., Svetashev, V. I., and Kamenev, G. M. (1995). Fatty acids as markers of food sources in a shallow-water hydrothermal ecosystem (Kraternaya Bight. Yankich Island, Kurile Islands). Mar. Ecol. Prog. Ser. 120, 231–241. doi: 10.3354/meps120231
Kitazato, H., Shirayama, Y., Nakatsuka, T., Fujiwara, S., Shimanaga, M., Kato, Y., et al. (2000). Seasonal phytodetritus deposition and responses of bathyal benthic foraminiferal populations in Sagami Bay, Japan: preliminary results from “Project Sagami 1996-1999. Mar. Micropaleontol. 40, 135–149. doi: 10.1016/S0377-8398(00)00036-0
Kluger, L. C., Taylor, M. H., Mendo, J., Tam, J., and Wolff, M. (2016). Carrying capacity simulations as a tool for ecosystem-based management of a scallop aquaculture system. Ecol. Model. 331, 44–55. doi: 10.1016/j.ecolmodel.2015.09.002
Krumhansl, K. A., and Scheibling, R. E. (2012). Production and fate of kelp detritus. Mar. Ecol. Prog. Ser. 467, 281–302. doi: 10.3354/meps09940
Langdon, C. J., and Newell, R. I. E. (1990). Utilization of detritus and bacteria as food sources by two bivalve suspension-feeders, the oyster Crassostrea virginica and the mussel Geukensia demissa. Mar. Ecol. Prog. Ser. 58, 299–310. doi: 10.3354/meps058299
Leblond, J. D., and Chapman, P. J. (2000). Lipid class distribution of highly unsaturated long chain fatty acids in marine dinoflagellates. J. Phycol. 36, 1103–1108. doi: 10.1046/j.1529-8817.2000.00018.x
Lefebvre, S., Marín Leal, J. C., Dubois, S., Orvain, F., Blin, J. L., Bataillé, M. P., et al. (2009). Seasonal dynamics of trophic relationships among co-occurring suspension-feeders in two shellfish culture dominated ecosystems. Estuar. Coast. Shelf Sci. 82, 415–425. doi: 10.1016/j.ecss.2009.02.002
Logan, J. M., Jardine, T. D., Miller, T. J., Bunn, S. E., Cunjak, R. A., and Lutcavage, M. E. (2008). Lipid corrections in carbon and nitrogen stable isotope analyses: comparison of chemical extraction and modelling methods. J. Anim. Ecol. 77, 838–846. doi: 10.1111/j.1365-2656.2008.01394.x
Lucas, M. L., and Newell, R. C. (1984). Utilization of saltmarsh grass detritus by two estuarine bivalves: carbohydrase activity of crystalline style enzymes of the oyster Crassostrea virginica (Gmelin) and the mussel Geukensia demissa (Dillwyn). Mar. Biol. Lett. 5, 275–290.
MacIntyre, H. L., Geider, R. J., and Miller, D. C. (1996). Microphytobenthos: the ecological role of the “secret garden” of unvegetated, shallow-water marine habitats. I. Distribution, abundance and primary production. Estuaries 19, 186–201. doi: 10.2307/1352224
Mann, K. H. (1988). Production and use of detritus in various freshwater, estuarine, and coastal marine ecosystems. Limnol. Ocean. 33, 910–930. doi: 10.4319/lo.1988.33.4_part_2.0910
Mansour, M. P., Volkman, J. K., and Blackburn, S. I. (2003). The effect of growth phase on the lipid class, fatty acid and sterol composition in the marine dinoflagellate, Gymnodinium sp. in batch culture. Phytochemistry 63, 145–153. doi: 10.1016/S0031-9422(03)00052-9
Mansour, M. P., Volkman, J. K., Jackson, A. E., and Blackburn, S. I. (1999). The fatty acid and sterol composition of five marine dinoflagellates. J. Phycol. 35, 710–720. doi: 10.1046/j.1529-8817.1999.3540710.x
McCutchan, J. H., Lewis, W. M., Kendall, C., and McGrath, C. C. (2003). Variation in trophic shift for stable isotope ratios of carbon, nitrogen, and sulfur. Oikos 102, 378–390. doi: 10.1034/j.1600-0706.2003.12098.x
McIntosh, H. A., McNichol, A. P., Xu, L., and Canuel, E. A. (2015). Source-age dynamics of estuarine particulate organic matter using fatty acid δ13C and Δ14C composition. Limnol. Oceanogr. 60, 611–628. doi: 10.1002/lno.10053
Melillo, J. M., Naiman, R. J., Aber, J. D., and Linkins, A. E. (1984). Factors controlling mass loss and nitrogen dynamics of plant litter decaying in northern streams. Bull. Mar. Sci. 35, 341–356.
Miller, R. J., and Page, H. M. (2012). Kelp as a trophic resource for marine suspension feeders: a review of isotope-based evidence. Mar. Biol. 159, 1391–1402. doi: 10.1007/s00227-012-1929-2
Moore, J. C., Berlow, E. L., Coleman, D. C., De Suiter, P. C., Dong, Q., Hastings, A., et al. (2004). Detritus, trophic dynamics and biodiversity. Ecol. Lett. 7, 584–600. doi: 10.1111/j.1461-0248.2004.00606.x
Mortazavi, B., Chanton, J. P., Prater, J. L., Oishi, A. C., Oren, R., and Katul, G. (2005). Temporal variability in 13C of respired CO2 in a pine and a hardwood forest subject to similar climatic conditions. Oecologia 142, 57–69. doi: 10.1007/s00442-004-1692-2
Murphy, K. J., Mooney, B. D., Mann, N. J., Nichols, P. D., and Sinclair, A. J. (2002). Lipid, FA, and sterol composition of New Zealand green lipped mussel (Perna canaliculus) and tasmanian blue mussel (Mytilus edulis). Lipids 37, 587–595. doi: 10.1007/s11745-002-0937-8
Newell, R. I. E., and Langdon, C. J. (1986). Digestion and absorption of refractory carbon from the plant Spartina alterniflora by the oyster Crassostrea virginica. Mar. Ecol. Prog. Ser. 34, 105–115. doi: 10.2307/24824957
Nichols, P. D., Jones, G. J., De Leeuw, J. W., and Johns, R. B. (1984). The fatty acid and sterol composition of two marine dinoflagellates. Phytochemistry 23, 1044–1047. doi: 10.1016/S0031-9422(00)82605-9
Odum, E. P. (1980). The status of three ecosystem-level hypotheses regarding salt marsh estuaries: tidal subsidy, outwelling, and detritus-based food chains. Estuar. Perspect. 1980, 485–495. doi: 10.1016/b978-0-12-404060-1.50045-9
Odum, E. P., and de la Cruz, A. A. (1967). “Particulate organic detritus in a Georgia salt-marsh estuarine system,” in Estuaries, ed. G. H. Lauff (Washington, DC: AAAS), 383–388.
O’leary, M. H. (1988). Carbon Isotopes in Photosynthesis. Bioscience 38, 328–336. doi: 10.2307/1310735
Outeiro, L., Byron, C., and Angelini, R. (2018). Ecosystem maturity as a proxy of mussel aquaculture carrying capacity in Ria de Arousa (NW Spain): a food web modeling perspective. Aquaculture 496, 270–284. doi: 10.1016/j.aquaculture.2018.06.043
Parnell, A. C., Inger, R., Bearhop, S., and Jackson, A. L. (2010). Source partitioning using stable isotopes: coping with too much variation. PLoS One 5:e0009672. doi: 10.1371/journal.pone.0009672
Parrish, C. C. (2013). “Lipids in Marine Ecosystems,” in ISRN Oceanography. New York, NY: Hindawi Publishing Corporation, 1–16. doi: 10.5402/2013/604045
Parsons, T. R., Maita, Y., and Lalli, C. M. (1984). A manual of chemical and biological methods for seawater analysis. Mar. Ecol. Prog. Ser. 199, 43–53.
Pepin, P., Parrish, C. C., and Head, E. J. H. (2011). Late autumn condition of Calanus finmarchicus in the northwestern Atlantic: evidence of size-dependent differential feeding. Mar. Ecol. Prog. Ser. 423, 155–166. doi: 10.3354/meps08952
Peterson, B. J., Howarth, R. W., and Garritt, R. H. (1985). Multiple stable isotopes used to trace the flow of organic-matter in estuarine food webs. Science 227, 1361–1363. doi: 10.1126/science.227.4692.1361
Post, D. M. (2002). Using stable isotopes to estimate trophic position: models, methods, and assumptions. Ecology 83, 703–718. doi: 10.2307/3071875
Ragan, M. A., and Jensen, A. (1978). Quantitative studies on brown algal phenols. II. Seasonal variation in polyphenol content of Ascophyllum nodosum Le Jol. and Fucus vesiculosus (L.). J. Exp. Mar. Biol. Ecol. 34, 245–258. doi: 10.1016/S0022-0981(78)80006-9
Reynolds, W. W., and Casterlin, M. E. (1985). Vagile macrofauna and the hydrographic environment of the Saco River Estuary and adjacent waters of the Gulf of Maine. Hydrobiologia 128, 207–215. doi: 10.1007/BF00006816
Riera, P. (2007). Trophic subsidies of Crassostrea gigas, Mytilus edulis and Crepidula fornicata in the Bay of Mont Saint Michel (France): a δ13C and δ15N investigation. Estuar. Coast. Shelf Sci. 72, 33–41. doi: 10.1016/j.ecss.2006.10.002
Stephenson, T. A., and Stephenson, A. (1972). Life Between the Tidemarks on Rocky Shores. San Francisco: W.H. Freeman and Co.
Strickland, J. D. H., and Parsons, T. R. (1972). A Practical Handbook of Seawater Analysis. Second. Ottawa: Fisheries research board of Canada.
Stuart, V., Field, J., and Newell, R. (1982). Evidence for absorption of kelp detritus by the ribbed mussel Aulacomya ater using a new 51Cr-labelled microsphere technique. Mar. Ecol. Prog. Ser. 9, 263–271. doi: 10.3354/meps009263
Swift, M. J., Heal, O. W., and Anderson, J. M. (1979). Decomposition in Terrestrial Ecosystems, 5th Edn. Berkeley, CA: University of California Press.
Taipale, S. J., Peltomaa, E., Hiltunen, M., Jones, R. I., Hahn, M. W., Biasi, C., et al. (2015). Inferring phytoplankton, terrestrial plant and bacteria bulk δ13C values from compound specific analyses of lipids and fatty acids. PLoS One 10:e0133974. doi: 10.1371/journal.pone.0133974
Tallis, H. (2009). Kelp and rivers subsidize rocky intertidal communities in the pacific northwest (USA). Mar. Ecol. Prog. Ser. 389, 85–96. doi: 10.3354/meps08138
Taylor, A. H., Geider, R. J., and Gilbert, F. J. H. (1997). Seasonal and latitudinal dependencies of phytoplankton carbon-to-chlorophyll a ratios: results of a modelling study. Mar. Ecol. Prog. Ser. Mar. Ecol. Prog. Ser. 152, 51–66. doi: 10.3354/meps152051
Tilburg, C. E., Gill, S. M., Zeeman, S. I., Carlson, A. E., Arienti, T. W., Eickhorst, J. A., et al. (2011). Characteristics of a shallow river plume: observations from the saco river coastal observing system. Estuar. Coasts 34, 785–799. doi: 10.1007/s12237-011-9401-y
Trushenski, J., Schwarz, M., Bergman, A., Rombenso, A., and Delbos, B. (2012). DHA is essential, EPA appears largely expendable, in meeting the n-3 long-chain polyunsaturated fatty acid requirements of juvenile cobia Rachycentron canadum. Aquaculture 32, 81–89. doi: 10.1016/j.aquaculture.2011.11.033
Uchida, M. (1996). Formation of single cell detritus densely covered with bacteria during experimental degradation of Laminaria japonica thalli. Fish. Sci. 62, 731–736. doi: 10.2331/fishsci.62.731
Von Biela, V. R., Newsome, S. D., Bodkin, J. L., Kruse, G. H., and Zimmerman, C. E. (2016). Widespread kelp-derived carbon in pelagic and benthic nearshore fishes suggested by stable isotope analysis. Estuar. Coast. Shelf Sci. 181, 364–374. doi: 10.1016/j.ecss.2016.08.039
Wang, S., Chu, T., Huang, D., Li, B., and Wu, J. (2014). Incorporation of exotic Spartina alterniflora into diet of deposit-Feeding snails in the Yangtze River Estuary salt marsh: stable isotope and fatty acid Analyses. Ecosystems 17, 567–577. doi: 10.1007/s10021-013-9743-3
Wang, T., Jónsdóttir, R., and Ólafsdóttir, G. (2009). Total phenolic compounds, radical scavenging and metal chelation of extracts from Icelandic seaweeds. Food Chem. 116, 240–248. doi: 10.1016/j.foodchem.2009.02.041
Welschmeyer, N. A. (1994). Fluorometric analysis of chlorophyll a in the presence of chlorophyll b and pheopigments. Limnol. Oceanogr. 39, 1985–1992. doi: 10.4319/lo.1994.39.8.1985
Xu, Q., and Yang, H. (2007). Food sources of three bivalves living in two habitats of Jiaozhou Bay (Qingdao, China): indicated by lipid biomarkers and stable isotope analysis. J. Shellfish Res. 26, 561–567. doi: 10.2983/0730-8000(2007)26[561:fsotbl]2.0.co;2
Zhao, L., Yang, F., and Yan, X. (2013). Stable isotopes and fatty acids as dietary tracers of intertidal bivalves. Fish. Sci. 79, 749–756. doi: 10.1007/s12562-013-0645-2
Zhukova, N. V., Kharlamenko, V. I., Svctashev, V. I., and Rodionov, I. A. (1992). Fatty acids as markers of bacterial symbionts of marine bivalve molluscs. Mar. Biol. Ecol. 162, 253–263. doi: 10.1016/0022-0981(92)90205-O
Keywords: Mytilus edulis, stable isotopes, fatty acids, detritus, macroalgae, aquaculture, particulate organic matter
Citation: Both A, Byron CJ, Costa-Pierce B, Parrish CC and Brady DC (2020) Detrital Subsidies in the Diet of Mytilus edulis; Macroalgal Detritus Likely Supplements Essential Fatty Acids. Front. Mar. Sci. 7:561073. doi: 10.3389/fmars.2020.561073
Received: 11 May 2020; Accepted: 16 November 2020;
Published: 07 December 2020.
Edited by:
Alberto Basset, University of Salento, ItalyReviewed by:
Jan Marcin Weslawski, Institute of Oceanology, Polish Academy of Sciences, PolandEdoardo Calizza, Sapienza University of Rome, Italy
Copyright © 2020 Both, Byron, Costa-Pierce, Parrish and Brady. This is an open-access article distributed under the terms of the Creative Commons Attribution License (CC BY). The use, distribution or reproduction in other forums is permitted, provided the original author(s) and the copyright owner(s) are credited and that the original publication in this journal is cited, in accordance with accepted academic practice. No use, distribution or reproduction is permitted which does not comply with these terms.
*Correspondence: Adrianus Both, YWRyaWFudXMuYm90aEBtYWluZS5lZHU=