- 1British Antarctic Survey, Natural Environment Research Council, Cambridge, United Kingdom
- 2Alfred Wegener Institute, Helmholtz Centre for Polar and Marine Research, Bremerhaven, Germany
- 3Instituto Antártico Chileno, Punta Arenas, Chile
- 4Cedric Cotte Sorbonne Université, CNRS-IRD-MNHN, LOCEAN-IPSL, Paris, France
- 5School of GeoSciences, University of Edinburgh, Edinburgh, United Kingdom
- 6Escuela de Ciencias del Mar, Pontificia Universidad Católica de Valparaíso, Valparaiso, Chile
- 7Centro FONDAP de Investigación Dinámica de Ecosistemas Marinos de Altas Latitudes (IDEAL), Valdivia, Chile
- 8Tristan da Cunha Government, Edinburgh of the Seven Seas, Tristan da Cunha
- 9School of Marine Science and Policy, University of Delaware, Newark, DE, United States
- 10Department of Geography, University of California, Los Angeles, Los Angeles, CA, United States
- 11Institute of Arctic and Alpine Research (INSTAAR), University of Colorado, Boulder, CO, United States
- 12Department of Fisheries, Animal and Veterinary Science, University of Rhode Island, Kingston, RI, United States
- 13Department of Forest & Conservation Sciences, University of British Columbia, Vancouver, BC, Canada
- 14Department of Biological and Marine Sciences, Faculty of Science and Engineering, University of Hull, Hull, United Kingdom
- 15Institute for Marine and Antarctic Studies, University of Tasmania, Hobart, TAS, Australia
The manuscript assesses the current and expected future global drivers of Southern Ocean (SO) ecosystems. Atmospheric ozone depletion over the Antarctic since the 1970s, has been a key driver, resulting in springtime cooling of the stratosphere and intensification of the polar vortex, increasing the frequency of positive phases of the Southern Annular Mode (SAM). This increases warm air-flow over the East Pacific sector (Western Antarctic Peninsula) and cold air flow over the West Pacific sector. SAM as well as El Niño Southern Oscillation events also affect the Amundsen Sea Low leading to either positive or negative sea ice anomalies in the west and east Pacific sectors, respectively. The strengthening of westerly winds is also linked to shoaling of deep warmer water onto the continental shelves, particularly in the East Pacific and Atlantic sectors. Air and ocean warming has led to changes in the cryosphere, with glacial and ice sheet melting in both sectors, opening up new ice free areas to biological productivity, but increasing seafloor disturbance by icebergs. The increased melting is correlated with a salinity decrease particularly in the surface 100 m. Such processes could increase the availability of iron, which is currently limiting primary production over much of the SO. Increasing CO2 is one of the most important SO anthropogenic drivers and is likely to affect marine ecosystems in the coming decades. While levels of many pollutants are lower than elsewhere, persistent organic pollutants (POPs) and plastics have been detected in the SO, with concentrations likely enhanced by migratory species. With increased marine traffic and weakening of ocean barriers the risk of the establishment of non-indigenous species is increased. The continued recovery of the ozone hole creates uncertainty over the reversal in sea ice trends, especially in the light of the abrupt transition from record high to record low Antarctic sea ice extent since spring 2016. The current rate of change in physical and anthropogenic drivers is certain to impact the Marine Ecosystem Assessment of the Southern Ocean (MEASO) region in the near future and will have a wide range of impacts across the marine ecosystem.
Introduction
The Southern Ocean (SO) physical environment is shaped by permanent cold and predictable seasonal cycles. However, despite its relative isolation within the Antarctic Circumpolar Current (ACC) inter-annual variation of the SO is strongly influenced through atmospheric and oceanic teleconnections. In an era of rapid climate change it is vital to identify the global drivers of the SO marine ecosystems. It is important to understand the regions within the SO where their effects are greatest, the expected impacts of any changes in these drivers, and crucially their interactions. For the working group, Marine Ecosystem Assessment of the SO (MEASO), global drivers are classified as those that influence the whole of the SO, even though their affects may manifest more strongly in some regions than others. Local drivers are defined as those that influence a particular location or series of locations within the SO (Grant et al., to be published in this research topic). While there is a global demand for protein to feed the ever increasing human population, southern ocean fisheries have regional impacts on stocks and ecosystems. A key difference is that local drivers can be managed with local interventions, e.g., regional regulation of fisheries. Yet, global drivers can only be managed externally to the region, e.g., climate change, ozone and plastic. Global tourism is driven by global drivers such as increasing wealth and demand for wilderness experiences. However, the regional impacts of tourism can also be managed through local regulation. Tourism therefore appears as both a global and local driver.
The manuscript outlines the global phenomena that impact the SO now and are expected to continue to impact it in the future. Atmospheric drivers considered here include changes in ozone, trends in Southern Annular Mode (SAM), variability in El Niño Southern Oscillation (ENSO), elevated air and ocean temperatures and ongoing increases in atmospheric CO2. Global oceanic connections considered here include the global thermohaline (overturning) circulation as well as changes in the SO, including eddies currents and the exchange of water masses (Figure 1). Other global anthropogenic drivers include pollution and tourism.
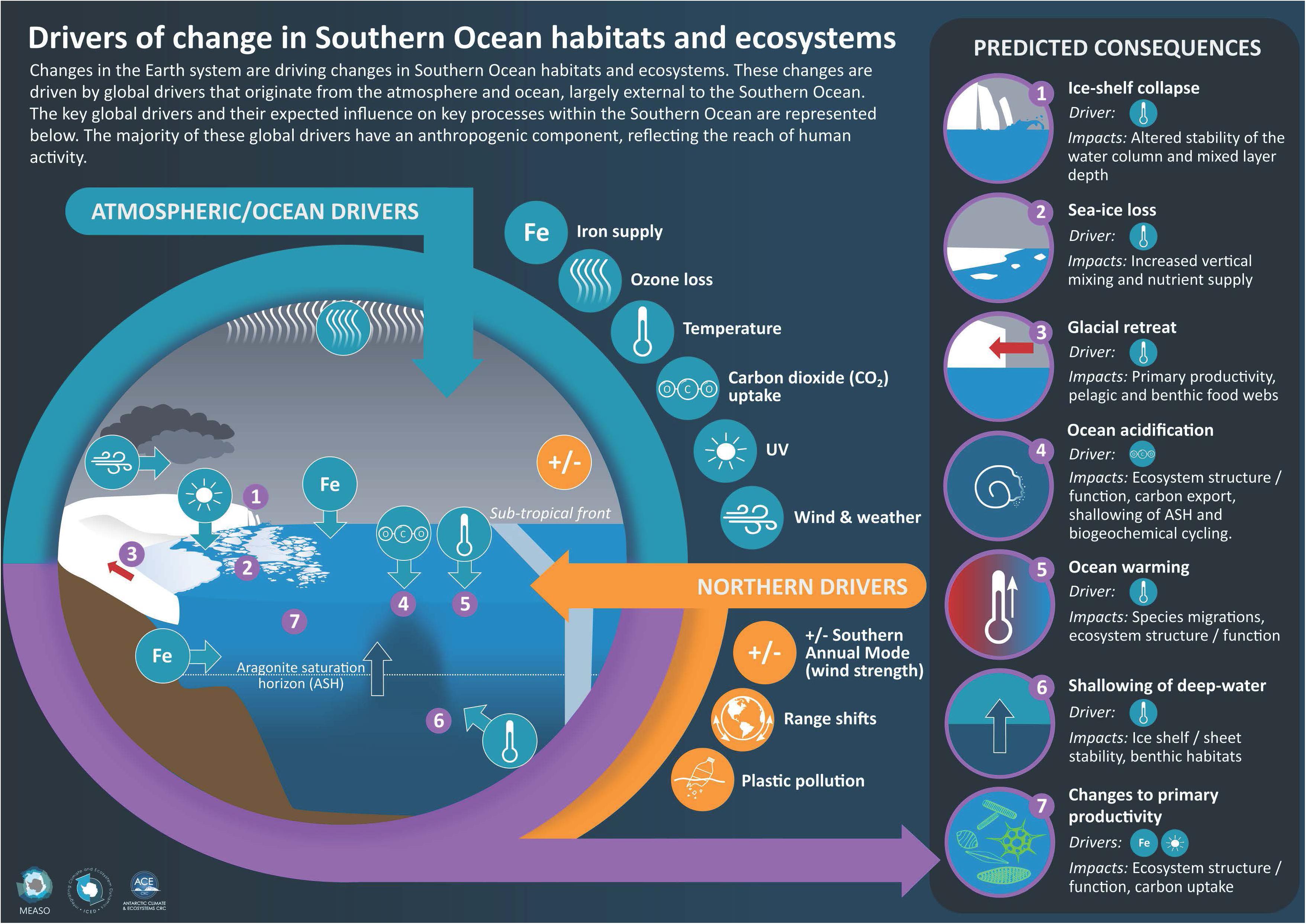
Figure 1. Description of the global drivers affecting the Southern Ocean. Northern drivers are global drivers whose influence reaches from North of the Southern Ocean.
These drivers, and their interactions, affect a range of attributes of the SO environment. Those considered here are: the cryosphere including marine ice, ice shelves and glaciers as well as salinity. For the purposes of MEASO the SO is separated into five sectors and three zones (Figure 2)
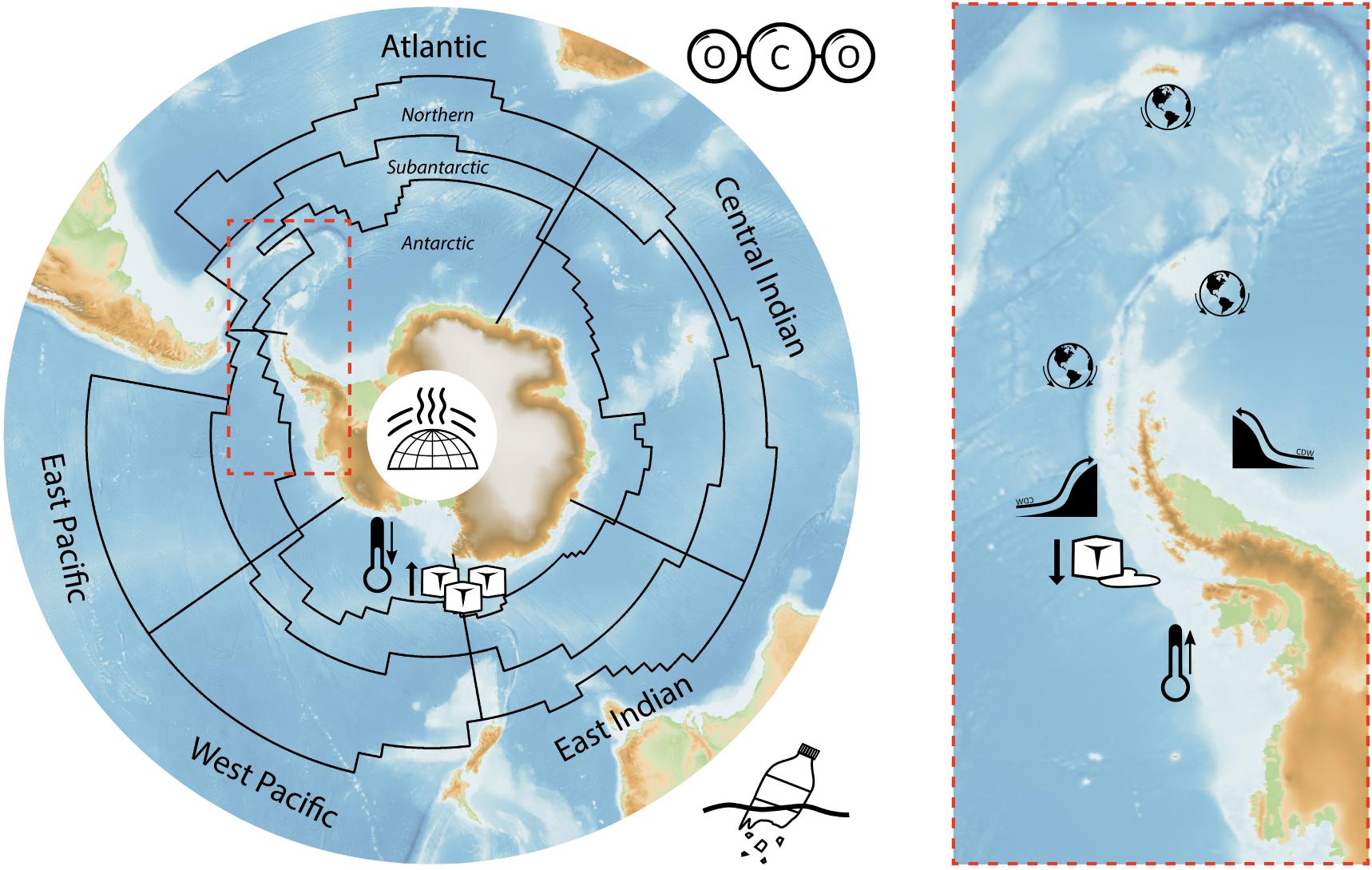
Figure 2. Areas for assessing effects of global drivers in the Marine Ecosystem Assessment of the Southern Ocean (black lines) with the Antarctic Peninsula highlighted (red dashed box). The five sectors (Atlantic, Central Indian, East Indian, West Pacific and East Pacific) and zones (Antarctic, Subantarctic and Northern) are labeled. Zonal boundaries are the Southern ACC Front between Antarctic and Subantarctic zones, and the Subantarctic Front between Subantarctic and Northern zones, with the Subtropical Front to the north. Icons indicate currently identified critical locations for increase and decrease in temperature and sea ice, potential for invasive species, and where circumpolar deep water is increasingly shoaling onto the continental shelf. They also illustrate general effects of ozone depletion, pollution and ocean acidification.
The majority of the biological consequences within pelagic and benthic marine ecosystem are discussed in the specific MEASO biota papers (as described in Constable et al., 2014 to be published in this research topic), but the impact of changes in the biogeochemical cycles, including the impacts of CO2 and ocean acidification are included. The increased risk of non-indigenous species establishing in the SO (range extensions in Figures 1, 2, 4) and the external influences on species that migrate in and out of the SO are also discussed as external drivers in this manuscript.
There have been several recent attempts to assess the dynamism of stressors impacting the Southern Ocean and its inhabitants (Constable et al., 2014; Gutt et al., 2015; Rogers et al., 2020). Where the current work differs is in taking a detailed look at large scale, global, influences on the physical environment, and to set these into a MEASO context. Where appropriate, the level of confidence in a conclusion is given according to the approach of the IPCC (Mastrandrea et al., 2011). These are given in italics in parentheses, with an appropriate reference where the confidence level has been judged elsewhere. When not accompanied by a reference, we judged confidence from the levels of agreement we observe in the scientific literature and the amount of evidence presented to support the conclusion, including consideration of any contrary evidence. Sometimes, we only report on the scope of the evidence in terms of agreement and amount. The levels of confidence are not to be used as the inverse of confidence for alternative hypotheses, as those alternatives may not be addressed in the literature. The future prognosis of these drivers is assessed and gap analysis is used to define future priorities.
Physical Drivers
SAM/ENSO
Stratospheric ozone concentration in the middle and high latitudes of the southern hemisphere has been decreasing in spring, summer and winter since the 1970s (e.g., WMO, 2011). Termed stratospheric ozone depletion, it is considered the single largest driver of change in summer, tropospheric (atmospheric) circulation in the southern high latitudes (Polvani et al., 2011). Normally, ozone absorbs UV radiation, radiatively warming the stratosphere. Its depletion, caused by chlorofluorocarbon (CFC) emissions, that began in the 1970s, means reduced absorption when sunlight returns to the southern high latitudes in the southern spring, and results in radiative cooling of the stratosphere over Antarctica. The dynamic response is increased shear in the zonal wind field and a strengthened stratospheric polar vortex as the atmosphere moves to restore the thermal wind balance (Thompson and Solomon, 2002). These changes in the stratosphere propagate to the troposphere, in approximately 2 months. The tropospheric effect is therefore observed in summer and is seen as a poleward shift of the mid-latitude jet and the accompanying storm track (Thompson and Solomon, 2002).
The tropospheric effect is an indication that stratospheric ozone depletion directly influences the leading mode of atmospheric circulation variability in the southern extratropical regions, the Southern Annular Mode (SAM). A measure of the SAM is an index calculated as the pressure gradient between mid-latitudes and Antarctica (Marshall, 2003), which when strongly positive, results in westerlies that are stronger than average and shifted poleward (e.g., Swart et al., 2015). Since 1957 there has been a significant increase in positive SAM in the austral summer and autumn (Marshall, 2003, 2007). The summer trend is thought to be forced primarily by stratospheric polar ozone depletion (Perlwitz et al., 2008). The variability of the SAM has a significant impact on Antarctic surface temperature (Marshall and Thompson, 2016), precipitation (Marshall et al., 2017), and sea ice (e.g., Hobbs et al., 2016; Doddridge and Marshall, 2017). The strong circumpolar westerlies associated with a positive SAM contributes to a cooler, drier, continental plateau (Eastern Antarctica) as they prevent the southward penetration of warmer air. Conversely, the Antarctic Peninsula warms because of warm air advection by the northwesterly flow, which also induces orographic precipitation on the western slopes and a rain shadow on the eastern slopes (Thompson and Solomon, 2002; Marshall and Thompson, 2016; Marshall et al., 2017). The increase in sea ice extent observed in the satellite era is attributed, in part, to increased northward Ekman drift associated with stronger westerlies over the Southern Ocean, facilitating the spread of the ice.
The SAM interacts with ENSO during the austral summer, particularly in the Pacific sector (e.g., Carleton, 2003; L’Heureux et al., 2006; Lim et al., 2019). When a La Niña phase in the tropical Pacific (cooler than average sea surface temperatures in the central and eastern tropical Pacific Ocean) co-occurs with a positive SAM phase at mid-high latitudes, this condition amplifies the effect of stratospheric ozone depletion. SAM and the high latitude ENSO both exert an influence on the Amundsen Sea Low (ASL), a climatological low pressure system centered off the coast of West Antarctica, the central pressure of which has deepened in recent years in response to an increase in the number of cyclones entering the region. This increase is primarily due to the more positive SAM driven by ozone depletion (Grieger et al., 2018). This effect on the ASL may have far reaching consequences since the ASL plays a role in altering ocean currents, allowing warm Circumpolar Deep Water (CDW) to reach more frequently under the Pine Island and Thwaites glaciers that drain much of West Antarctica (Thoma et al., 2008). This has coincided with a period of thinning of these glaciers (Thoma et al., 2008).
Temperature
Atmospheric Warming
Intensifying warm and moist westerly winds during more frequent positive phases of the summer SAM cause pronounced atmospheric warming of the Northern and central sectors of the West Antarctic Peninsula (WAP). Strong interannual variations of atmospheric warming/cooling patterns are strongly related to climatic modes of SAM and ENSO (Bers et al., 2013). The strengthening of the westerly winds has also resulted in the warm air passing over the mountains of the Antarctic Peninsula, which has contributed to the melting of the east Antarctic Peninsula ice shelves (Cape et al., 2015).
Oceanic Warming
The SO has been a stable cold environment for millions of years, shaping unique ecosystems of cold adapted Antarctic biota. Within this stable regime there have been 100’s to 1000’s year time scale variations of 2–4°C on the WAP, which are associated with reconstructions of the SO westerly winds (Shevenell et al., 2011). The increased intensity of positive summer SAM events are correlated with significant changes in seawater temperature that have been recorded in recent decades (high confidence; Hellmer et al., 2017; Moffat and Meredith, 2018). Circumpolar deep water (CDW), the temperature maximum layer of Antarctic Continental Shelf Bottom Water (ASBW) is warming (0.1°C decade–1) and shoaling (−30 m decade–1) in most regions around the Antarctic (high confidence; Schmidtko et al., 2014) and both hydrographic and climatic forcing, modify the atmospheric and ocean heat transport.
Regional Patterns
At the northwestern tip of Bransfield Strait and the South Shetland Islands (East Pacific), ocean temperatures are cooled by water entering from the Weddell Sea, while mean air temperatures increased by 0.4°C per decade between 1991 and 2012 (Schloss et al., 2012). Melt events at the Northern WAP are regional and episodic, and thus amplify environmental and ecological variability in coastal and shelf systems (Meredith et al., 2018). In contrast, the central/south WAP is influenced by atmospheric warming and by upwelling of warm CDW onto the shelf, which has driven thinning of about 75% of the ice shelves of the AP and the Amundsen Sea. Mean atmospheric temperatures increased by 3°C (0.6°C per decade) and sea surface temperatures by 1°C (SST in the upper 100 m) between 1955 and 2004 (Moffat and Meredith, 2018). In spite of a recent superimposed cooling phase recorded since the early 2000s, which appears to be associated with shifts in decadal forcing in this region (Turner et al., 2016), net warming of the WAP atmosphere is still significant and the ocean has continued to warm (Martinson et al., 2008). Similarly, increases in seawater temperature have been reported for the Atlantic Sector, with warming averaging over 2°C in the upper 150 m over the past 81 years (Whitehouse et al., 2008) and 0.3–1.5°C in subsurface temperature (50–400 m) at the Eastern Antarctic Peninsula (Etourneau et al., 2019).
Other regions of the SO either remained stable or cooled in the last two-three decades. These differences originate from varying patterns in the ocean heat transport around the Antarctic (Schmidtko et al., 2014) and shoaling of warm deep water (WDW) up onto the continental shelf. For instance, relatively warm CDW entering the eastern Weddell Sea gyre causes shoaling of WDW (+0.8°) flowing along the continental shelf break in the Southern Weddell Sea. Models project intrusion of WDW into the Filchner-Ronne Ice Shelf cavity as part of a tipping point scenario, which would dramatically increase basal melt rates, threatening the stability of the largest floating ice mass fringing the Antarctic continent (Timmermann and Hellmer, 2013; Hellmer et al., 2017). The warming has been observed in most sectors, but the vertical displacement of the CDW core shows a more complex pattern, with deepening in the Cosmonaut and Northern Weddell Seas and shoaling elsewhere (Schmidtko et al., 2014). It is interesting to note that while the general trend of CDW also persists in the Ross Sea, no shoaling and intrusions of CDW onto the shelf have been observed so far (H. Hellmer, pers. communication). In contrast to the warming of the AP and CDW in the Weddell and Ross Seas/gyres, the Eastern sections of the continental SO have shown a cooling trend (Rogers et al., 2020). With continued warming of the earth’s atmosphere, future warming between now and 2100, is expected across the whole of the SO (Rogers et al., 2020).
Many SO marine ectotherms are adapted to a narrow annual thermal range of only 2–4°C, and are therefore vulnerable to the effects of warming (medium confidence). Increasing ocean temperature is also correlated with the secondary effects of warming on summer sea ice dynamics and glacial melting in coastal and near-shore regions (high confidence). Increasing temperature is therefore projected to shape open ocean ecosystems and affect coastal communities down to the level of species ecological performance and molecular composition (high confidence, Ashton et al., 2017; Peck, 2018).
Currents and Eddies
The SO circulation forms a major horizontal connection between ocean basins as well as a vertical link between shallow and deep water masses. The ACC consists of a strong, multi-jet, and turbulent eastward flow around Antarctica, transporting approximately 173.3 ± 10.7 × 106 m3.s–1 (Donohue et al., 2016). The ACC, driven by westerly winds and surface buoyancy fluxes, is also identified by three main circumpolar fronts, separating distinct water masses, which contribute to the isolation of the SO (Sokolov and Rintoul, 2009).
As indexed by the SAM, a clear trend in strengthening of westerly winds has been observed in recent decades (Swart et al., 2015), yet there is no evidence of a significant increase in the ACC transport (medium confidence; Chidichimo et al., 2014). Although the response of the ACC transport and eddy field to wind forcing is still uncertain (low confidence), the presence of active ocean eddy dynamics, and its saturation suggested by modeling (Munday et al., 2013), buffers the oceanic response to atmospheric changes. This apparent cascade of energy into small scale eddies is supported by the satellite derived measurements, which indicate an increase in eddy kinetic energy over the last two decades. This is especially significant in the Pacific and Indian Ocean sectors of the SO (medium confidence; Hogg et al., 2015).
The use of sea surface temperature (SST) and sea surface height (SSH) contours to track front locations has suggested there has been a latitudinal change in the mean location of the ACC. This could have major impacts on marine ecosystems by modifying the environmental conditions experienced by numerous marine organisms (Bost et al., 2015; Cristofari et al., 2018; Meijers et al., 2019). However, this approach was challenged because these parameters are affected by the large-scale thermal expansion and steric sea-level rise occurring as a result of the warming of this circumpolar region. Recent studies found no significant long-term trend in either the annual mean latitude of zonal wind jets between 1979 and 2009 (Swart et al., 2015) or the zonally averaged latitude of ACC transport, which seem insensitive to changes in the SO’s broad-scale structure (Gille, 2014; Chapman et al., 2020). The location of the ACC may be constrained by sea floor and land mass topography, particularly close to narrow gaps between land masses, such as Drake’s Passage (high confidence; Moore et al., 1999).
The assessments of trends in the overturning circulation is challenging due to the high inter-decadal variability related to wind stress (low confidence; Waugh et al., 2013; DeVries et al., 2017; Ting and Holzer, 2017). While the production and export of Antarctic Bottom Water in the global ocean has decreased (medium confidence; Purkey and Johnson, 2013; Desbruyeres et al., 2017), this may reflect the same inter-decadal variability (low confidence; Abrahamsen et al., 2019).
Future projections for the SO under different warming scenarios from CMIP5 and CMIP6 models suggest that the trends observed over the last few decades will continue in the coming century at a rate that depends on future emission scenarios (Meredith et al., 2019). Projections include the strengthening of the westerly winds for all scenarios, except under stringent mitigation (Bracegirdle et al., 2020), and as a response, increased eddy activity (Downes and Hogg, 2013). Warming of the ACC and the freshening of surface water from increasing precipitation is projected to continue for all emission scenarios (Sallée et al., 2013; Bracegirdle et al., 2020). Due to their inability to explicitly resolve eddy processes (Gent, 2016; Downes et al., 2018) and increased water input from melting ice (Bronselaer et al., 2018), the projections from CMIP5 or CMIP6 models have low confidence. Evidence from other models suggests that glacial meltwater from ice sheet might continue in the future (medium confidence; Levermann et al., 2020; Seroussi et al., 2020), with important impacts on ocean circulation, including cooling of surface subpolar ocean (Bronselaer et al., 2018; Rye et al., 2020) and warming of subsurface subpolar waters (Sadai et al., 2020).
Seawater Circulation and Exchange
The observed changes in ocean properties and circulation in Antarctica reveals the central role that regional variability plays in understanding the evolution of this system (Thompson and Solomon, 2002). At depth, warm waters around Antarctica supply heat and nutrients to the continental shelf. This supply to the coastal ocean varies greatly around the continent. Circumpolar Deep Water (CDW) has both warmed significantly since the late 70s and its core has shallowed by about 30 m per decade (Schmidtko et al., 2014). The expression of these changes in open ocean properties is not straightforward, however, as strong shelf currents, the Antarctic Slope Front (ASF) in particular, act as a barrier for cross-slope exchange. In the regions where the ASF is weak or absent, i.e., the Bellingshausen and Amundsen Seas, the warming of shelf waters more closely follows the changes in the open ocean (Schmidtko et al., 2014; Thompson et al., 2018).
The rapid warming of the WAP (Meredith and King, 2005) has impacted ocean properties as a result of increased freshwater input to the coast (Meredith and King, 2005; van Wessem et al., 2017) altering the structure of the upper layers of the ocean (Martinson et al., 2008; Welhouse et al., 2016). This region also shows strong impacts from ENSO and its interaction with SAM variability, which has been shown to modulate wind forcing, which in turns modulates the depth of the mixed layer and marine productivity on interannual scales (Venables and Meredith, 2014). Warming of the subsurface layers of the Peninsula is representative of similar trends along the Bellingshausen and Amundsen Seas, where the weak presence or absence of the Antarctic Slope Front, found along the shelf break elsewhere in Antarctica, facilitates the flooding of the shelf by the warming CDW (Thompson et al., 2018). Deep, steep canyons and other topographic features play a key role in the across-shelf exchange of properties along this and other Antarctic shelves (Moffat et al., 2009; Martinson and McKee, 2012). This exchange is strongly modulated by small ocean eddies that play a role on both the supply of heat and nutrients to the shelf (Moffat et al., 2009) as well as the export of shelf-modified waters to the open ocean (Brearley et al., 2019). Eddy-driven exchange is a key modulating factor of ocean properties around the continent, and this process is modulated by wind forcing (Thompson et al., 2014; Stewart and Thompson, 2015). The WAP shelf has experienced a build-up of subsurface heat (Martinson et al., 2008), and while full attribution of this change remains unresolved, both the changes in open ocean water properties and the increase of coastal stratification as a result of increased freshwater discharge from the coast likely play a role in this process.
The combination of strong regional and temporal variability in observed ocean properties, combined with what are still relatively limited datasets, result in significant challenges when trying to attribute the origin of the changes. Elsewhere in Antarctica, teasing out anthropogenic change from natural variability has proven challenging for many variables, suggesting that the observed change is consistent with the pattern expected from natural variability (Jones et al., 2016). This implies that process studies to understand the underlying mechanisms of variability as well as continued efforts to improve the observational records around Antarctica are critical to understand the nature of change around the continent and the impacts on marine ecosystems.
Southern Ocean Attributes
Marine Ice
Every year Antarctic seasonal sea ice undergoes a sixfold change in ice-covered area; it is one of the largest seasonal signals on Earth, with consequent effects on air-sea exchanges of heat, momentum and gases (most notably CO2), water mass properties and finally, on marine ecosystems. Antarctic sea ice is, however, highly variable and frequently exposed to strong storms, high winds and waves. These factors affect Antarctic sea ice growth and melt processes, thickness evolution and drift, and impart high regional and seasonal variability.
Over the past four decades there has been a modest increase in spatially averaged Antarctic sea ice extent. However, this Antarctic-wide average hides important regional and seasonal details, as Antarctic sea ice is not increasing everywhere and shows high seasonal and regional variability (e.g., Parkinson, 2019). The two regions showing the strongest but opposing sea ice trends are the east Pacific, Bellingshausen-Amundsen Sea, sector (where sea ice decreased over 1979–2015) and the west Pacific, Ross Sea Sector (where sea ice increased over 1979–2015). Although the changes in sea ice were opposite, the rates were comparable to those observed in the Arctic (Cavalieri and Parkinson, 2012; Parkinson and Cavalieri, 2012; Stammerjohn et al., 2012).
These contrasting regional sea ice distributions can in part be explained by the SAM and ENSO changes detailed previously. The westerly winds have strengthened mostly in austral summer and autumn, i.e., when SAM shows the strongest positive trends (Marshall, 2003, 2007), thus impacting sea ice distributions mostly in summer and autumn (Stammerjohn et al., 2008; Hobbs et al., 2016). ENSO also effects regional and seasonal sea ice distributions, with the strongest signal being an Antarctic Dipole between the Amundsen/Ross and Bellingshausen/Weddell sea regions, consisting of positive/negative sea ice anomalies during a La Niña, and vice-versa for El Niño (e.g., Yuan, 2004). Together, strong phases in SAM and ENSO can either amplify or dampen the effect on sea ice distributions, along with other atmospheric perturbations, e.g., Pacific Decadal Oscillation, the Atlantic Multi-decadal Oscillation, greenhouse gas increases, not to mention ocean changes in heat and freshwater content and stratification, making attribution quite challenging.
This last decade, 2010–2020, has been most notable for showing the strongest changes in Antarctic-wide sea ice. During 2012–2014 numerous records were broken for Antarctic-wide high sea ice extent (based on satellite observations since 1979) (Turner et al., 2013; Reid and Massom, 2015; Reid et al., 2015). There appeared to be no one dominant factor behind the record maxima, but instead, for each of these 3 years (2012–2014), there were different regions and seasons contributing to the record high sea ice extent (Reid and Massom, 2015). Then, just as abruptly, Antarctic-wide sea ice extent since spring 2016 has shown numerous record lows, for which several explanations have also been proposed, including anomalies in both the atmosphere and ocean, with a possible deep ocean driver acting on decadal or longer timescales (e.g., Stuecker et al., 2017; Turner et al., 2017; Schlosser et al., 2018; Meehl et al., 2019; Wang et al., 2019). Whether this recent decline in Antarctic sea ice extent will continue or not is still unknown, and once again points to the challenges in attributing and predicting Antarctic sea ice changes.
Previous work has highlighted the effects of changes in sea ice and how they can transform shallow water benthic ecosystems associated with changes in light regimes (Clark et al., 2013) and also by producing longer phytoplankton blooms and increased iceberg disturbance (Barnes et al., 2018a). Importantly for SO foodwebs, biodiversity and nutrient cycling and the abundance of Antarctic krill (Euphausia superba) all depend on the extent of winter sea ice, which is varying between sectors within the SO (Atkinson et al., 2019). Sea ice is one of the major drivers of energy flow into the SO and the effects of future sea ice extent on SO productivity are difficult to predict (low confidence).
Ice Shelf Disintegration
The floating ice shelves shape unique conditions in the SO for marine life living below them. Ice shelves are floating masses of ice slowly advancing from the glaciers of the interior toward the ocean, at least regionally and temporarily since the late Oligocene (Hochmuth and Gohl, 2019). They range from 100 to 1,400 m thick. The distance between the grounding line, where they lose contact with the land and the edge facing the open ocean, ranges from a very short distance to approximately 600 km. By far the largest two, which are each roughly the size of Spain are the Ross and Filchner-Rönne Ice shelves in the Pacific and Atlantic Ocean, respectively. With a total area of 1.63 × 106 km2 ice shelves cover 36% of the continental shelf being equivalent to 11% of the size of the continent (Clarke and Johnston, 2003). Their existence and dynamics demand a replenishment of ice from the hinterland, permanently low air and water temperatures and depend on specific geomorphological structures. Ice shelves also seem to stabilize glacier draining velocity (Miles et al., 2018) and interact with sea-ice and ocean dynamics (Jourdain et al., 2017). The Larsen A ice shelf in the North of the Antarctic Peninsula most likely disintegrated more than once during the Holocene due to natural temperature changes (Domack et al., 2005). Further south the Larsen B on the east coast of the Peninsula (2002) as well as Wordie (2009) ice shelves on the west coast of the Peninsula, collapsed due to recent regional atmospheric and ocean warming, respectively (Massom et al., 2018). Ice shelves shape almost 50% of the coastline and icebergs as large as the size of Jamaica calve from the seaward edges. Despite this dynamism, the long-term mass balance currently seems to remain stable. However, large-scale long-term disintegration and melting is predicted under ongoing climate-change scenarios (high confidence; Naughten et al., 2018).
Besides impacts on ocean physics the disintegration of large ice shelves causes one of the most effective changes in marine ecosystems (medium confidence). The covering of ice acts as a barrier to energy exchange between the water and the atmosphere. Once this barrier is gone then there will be increased transfer of wind, heat and light energy, affecting the stability of the water column and the mixed layer depth. When light penetrates into the water it initiates the production of algal biomass (medium confidence, Bertolin and Schloss, 2009; Peck et al., 2010), which is the basis for almost all life in the oceans, in a previously totally dark and, thus, food-poor environment (Raes et al., 2010; Gutt et al., 2011). Icebergs calving naturally and more frequently under climate-change scenarios from ice shelves (high confidence for the next decades; e.g., Rack and Rott, 2004) drift in the ACC, scour the sea-floor (see e.g., Post et al., 2020) and eventually run aground before they disintegrate. These processes also have local to regional impact on pelagic and benthic systems (medium confidence; Gutt and Piepenburg, 2003).
Glacier Retreat
A third, smaller but important area of marine ice loss is glacier retreat. Like sea ice and ice shelves, glaciers are also showing decreases, and in fairly complex geographic patterns. Nearly 90% of glaciers along the WAP are now retreating, and their retreat rates are also increasing (Cook et al., 2016). CDW upwelling has driven frontline retreat of nearly all glaciers South of the Bransfield Strait (Cook et al., 2016; Etourneau et al., 2019). There is also evidence of thinning in southern glaciers around the East Pacific Sector (Hogg et al., 2017 and references therein). Until they reach grounding lines this glacier retreat generates icebergs and therefore coastal ice scouring and is opening up new fjordic habitats and carbon sinks (see Barnes et al., 2020). Although the high sedimentation in such environments can be harsh for new colonists (Sahade et al., 2015), they could be important for a number of reasons. Unlike elsewhere in the world, Antarctica has no other low wave energy-high productivity habitats, e.g., salt marshes, seagrass meadows and mangrove swamps, that provide hotspot potential for fast track from carbon capture to burial and sequestration. However, they also provide a, previously missing, key habitat which may provide opportunity for invasion of these newly exposed areas by non-indigenous species.
Meltwater and Freshening
Seawater salinity in the whole SO has decreased during the last 60–70 years (e.g., Jacobs et al., 2002; Jacobs and Giulivi, 2010; Hellmer et al., 2011; Durack et al., 2012). Surface waters (i.e., above 100 m depth) south of the ACC have shown stronger freshening rates, 0.0011 ± 0.0004 yr–1 (de Lavergne et al., 2014), than intermediate waters, 0.0002–0.0008 yr–1 (Skliris et al., 2014), or bottom waters, 0.0004 ± 0.0001 yr–1 (medium confidence; Menezes et al., 2017). Higher freshening rates have been recorded over Antarctic continental shelves, although there are large spatial differences with the Ross and Cosmonaut Sea displaying intense freshening, whereas the Bellingshausen and Amundsen Sea (East Pacific sector) are showing the opposite trend, i.e., increasing salinity, (Schmidtko et al., 2014). The latter may be the consequence of more frequent and shallower intrusions of CDW (Schmidtko et al., 2014), a saltier and warmer water mass (high confidence; Moffat et al., 2009). Despite the high melting rates of glaciers in west Antarctica (Paolo et al., 2015), enough CDW intrusions seem to reach the shelf in order to prevent a steady freshening of these shelf waters. This may be the consequence of changes in large scale atmospheric and ocean circulation patterns (e.g., the increasing frequency and strength of SAM), since these drive the intrusions of CDW, rather than shelf hydrographic conditions (Nakayama et al., 2018). Similarly, a relationship between positive SAM and increased freshening has been registered for other areas (e.g., Atlantic sector: eastern Antarctic Peninsula and Weddell Gyre) although atmospheric warming seems to drive this relationship instead of hydrographic conditions (Dickens et al., 2019). Although similar freshening trends have been registered in different Antarctic regions, the drivers and mechanisms involved vary spatially (high confidence). For example, except for the WAP, positive SAM has increased northward Ekman drift of sea ice that is formed close to the continent (Holland and Kwok, 2012), increasing the transport of freshwater to the open ocean and promoting the freshening recorded for offshore waters in the SO (Haumann et al., 2016).
Freshening has other effects besides salinity. Stratification has a key controlling influence on phytoplankton blooms as do sources of iron from glacial meltwater and icebergs (De Baar et al., 1995; Loscher et al., 1997; Dierssen et al., 2002; Höfer et al., 2019; Hopwood et al., 2019). These inputs may, however, be more restricted to coastal waters than previously thought (Hopwood et al., 2019). In contrast, there is limited evidence that meltwater inputs can dilute the macronutrient concentrations of seawater (Henley et al., 2017; Höfer et al., 2019). Meltwater may also carry a high amount of inorganic suspended matter (e.g., glacial flour) that may kill, or impair the functions of, pelagic (Pakhomov et al., 2003; Fuentes et al., 2016; Giesecke et al., 2019) and benthic organisms (high agreement, limited evidence; Torre et al., 2014; Sahade et al., 2015). Finally, although there is medium confidence that the volume of Antarctic Bottom Water has decreased (Purkey and Johnson, 2013; Desbruyeres et al., 2017), there is almost no direct record of freshening hindering the formation of Antarctic Bottom Water (Silvano et al., 2018). Besides, recent studies suggest that the formation and northward export of Antarctic Bottom Water depends on several processes varying on multi-annual time scales (Abrahamsen et al., 2019), which need longer time series in order to detect any long term trend.
Biogeochemistry
CO2 Uptake and Ocean Acidification
One of the most important functions of the SO in the Earth System is its uptake of atmospheric CO2 by physical and biological processes and its release of oceanic CO2 to the atmosphere (Sarmiento and Le Quere, 1996; Marinov et al., 2006; Gruber et al., 2009, 2019; Lenton et al., 2013; Henley et al., 2020 this volume). However, this uptake is leading to changes in ocean biogeochemistry, which could have devastating consequences for the ecosystem. Ocean acidification driven by oceanic uptake of atmospheric CO2 is expected to occur earlier in the SO than most other ocean regions, due to enhanced solubility of CO2 at low temperature and upwelling of deep waters with high CO2 concentrations (Orr et al., 2005; McNeil and Matear, 2008; Feely et al., 2009). Ongoing changes in upper ocean temperature and salinity are very likely to impact the solubility of CO2 in SO surface waters, and projected increases in the Revelle factor are expected to increase the biologically mediated uptake of CO2 over the twenty-first century (Hauck and Völker, 2015; Hauck et al., 2015; Henley et al., 2020).
At the global scale, oceans have absorbed ∼30% of the anthropogenic atmospheric CO2 and this has already caused shifts in seawater carbonate chemistry by reducing seawater pH, carbonate ion concentrations and therefore saturation states of the carbonate minerals aragonite and calcite (Ω, where values < 1 indicate undersaturated conditions that result in the dissolution of carbonate structures; Doney et al., 2009; Orr, 2011; McKinley et al., 2017; IPCC, 2019). Prior to the industrial period, atmospheric CO2 was approximately 280 μatm with global average surface seawater pH of 8.17 and Ωarag ∼3.5. Atmospheric pCO2 has now reached 380–400 ppm, which has reduced surface seawater pH to 8.01–8.05, equivalent to a 100-fold increase in hydrogen ion concentration (Guinotte and Fabry, 2008; Doney et al., 2009; IPCC, 2019). Associated decreases in carbonate saturation have led to a shallowing of the saturation horizon by 30–200 m (Feely et al., 2002, 2004; Sabine et al., 2004). These trends are likely to continue and to accelerate in the coming decades, based on the current RCP 8.5 worst case “business as usual” emissions scenario. Further reductions of 0.3–0.4 pH units and Ωarag ∼1.2 are projected (high confidence) at atmospheric pCO2 of 851–1,370 by the year 2100 associated with further shallowing of both aragonite and carbonate saturation horizons (ASH and CSH) (Houghton et al., 2001; Caldeira and Wickett, 2003; IPCC, 2019).
The SO has taken up around 40% of the total oceanic uptake of anthropogenic CO2 (Orr et al., 2001; Fletcher et al., 2006; Devries, 2014). The Ωarag is in SO surface waters is largely below 1.5, with particularly low values in winter (Jones et al., 2017; IPCC, 2019). Trends of ocean acidification have already been observed in many of the MEASO areas, such as the east Pacific Antarctic and Subantarctic zones, Atlantic Antarctic zone, west Pacific Antarctic zone and across the East Indian sector (Hauck et al., 2010; van Heuven et al., 2011; Midorikawa et al., 2012; Takahashi et al., 2014; Munro et al., 2015; Williams et al., 2015; Lencina-Avila et al., 2018). The ASH resides at ∼730 m depth southwards of 60’S, cross-cutting the steep Antarctic continental shelf (McNeil and Matear, 2008; IPCC, 2019), whilst the CSH resides at approximately 3,000 m (Sewell and Hofmann, 2011). The ASH is expected to shoal to the surface during winter periods from ∼2,030 and across all seasons by 2100 (Orr et al., 2005; McNeil and Matear, 2008; McNeil et al., 2010; IPCC, 2019). This is likely to impact upon phytoplankton abundance, growth rates and species composition as well as zooplankton growth, reproductive success and survival, especially of ecologically important species of pteropods and krill (Tortell et al., 2008; Kawaguchi et al., 2013; Trimborn et al., 2013; Gardner et al., 2018; Henley et al., 2020). Mg-calcites are more soluble than aragonite and Southern Ocean surface waters are already undersaturated for ≥ 12 mol% MgCO3 and are predicted to become undersaturated for 4–5% mol% MgCO3 by 2100 (Andersson et al., 2008). Areas of upwelling CDW (from 2 to 3 km depth) can bring seawater with low Ωarag (∼0.9–1.08, ∼490–570 μatm pCO2) toward the upper ocean in the ACC, leading to conditions that are corrosive for aragonite-secreting organisms such as pteropods (Bednaršek et al., 2012). Models suggest that upwelling could become stronger in the near future but the severity and timeframe with respect to carbonate undersaturation remain poorly constrained (Constable et al., 2014; Rintoul, 2018). Potential impacts of ocean acidification on microbial communities could lead to faster recycling of organic matter and overall declines in carbon export (Maas et al., 2013; Deppeler et al., 2018; Westwood et al., 2018), with implications for large-scale biogeochemical cycling and potentially climate, but more research is required.
The coastal regions around Antarctica are characterized by highly variable topography, bathymetry, hydrodynamics and carbonate chemistry, which leads to highly variable seawater pH, pCO2 and Ω. Present day variations include Ross Sea surface pCO2 values ranging from ∼100 to 450 μatm, equivalent to aragonite saturation of 3–4, between the autumn and summer, which then decline > 4-fold during autumn/winter to near-undersaturation of aragonite at 1.1–1.2, with an associated reduction of pH by 0.6 units (McNeil et al., 2010). Summer periods are characterized by high primary production, whilst entrainment of carbonate-depleted CDW occurs onto the Ross Sea Shelf during winter (Sweeney, 2004; McNeil et al., 2010). Similar seasonal patterns have been observed in surface waters at coastal sites of McMurdo Sound, McMurdo Jetty and Cape Evans with changes in seawater pH of 0.3 units (Kapsenberg et al., 2015) and in Prydz Bay with changes of 0.6 pH units (Gibson and Trull, 1999). Continued ocean acidification is projected to show large and uncertain regional and local variations, particularly in coastal regions (high confidence; IPCC, 2019). Nevertheless, model simulations have projected a pronounced increase in aragonite undersaturation around 2030, affecting around 30% of Southern Ocean surface waters by 2060 (Hauri et al., 2016). There is also medium certainty that the current rates of change are the fastest observed for at least 300 million years and high confidence that these changes will continue to rise at an accelerating rate (Canadell et al., 2007; Hönisch et al., 2012; IPCC, 2019), making ocean acidification likely to become one of the most important drivers of Southern Ocean ecosystem structure and functioning in the near future (Gutt et al., 2015).
Increasing Iron Inputs and Freshwater Flux From Glacial Ice Melting
The Southern Ocean is a large high nutrient low chlorophyll (HNLC) zone where primary production is not limited by the availability of macronutrients, as in other oceans, but of the essential micronutrient, iron (e.g., De Baar et al., 1990; Moore et al., 2013). As such, iron supply to Southern Ocean surface waters has a profound impact on primary production and ecosystem functioning, and is changing in response to changes in Earth’s climate, large-scale ocean circulation and regional environmental conditions (Henley et al., 2020 and references therein). Increases in iron supply are expected due to increases in dust flux from lower latitudes (IPCC, 2019), oceanic iron transport to the sub-Antarctic in strengthening western boundary currents (Bowie et al., 2009) and enhanced release of terrigenous iron sources from glacial ice and the Antarctic continent (Lin et al., 2011; Gerringa et al., 2012; Sherrell et al., 2015; Winton et al., 2016; van Der Merwe et al., 2019). However, these may be offset to an extent by increased stratification of the upper ocean linked to a weakening overturning circulation, which could lead to a reduction in iron delivery from underlying waters (Tagliabue et al., 2009; Rintoul, 2018), and the ongoing declines in sea ice, which can store iron (Lannuzel et al., 2016). Changes in iron speciation, solubility, scavenging, internal cycling and remineralization—as well as phytoplankton dynamics, iron requirements and the impacts of ocean acidification—further complicate the net effect of climate and environmental changes on iron availability to phytoplankton blooms (e.g., Planquette et al., 2013; Blain and Tagliabue, 2016; Hutchins and Boyd, 2016; Andrew et al., 2019). Nevertheless, there is good agreement amongst CMIP5 models that Southern Ocean NPP will increase overall as a result of increased iron supply, changes in mixed layer depth, declining sea ice cover, increasing SST and altered westerly winds (Bopp et al., 2013; Leung et al., 2015; Fu et al., 2016; Moore et al., 2018). Increased iron supply and temperature could also lead to a floristic shift to diatoms and potentially Phaeocystis antarctica, with consequences for benthic and pelagic consumers as well as nutrient uptake, carbon export and large-scale biogeochemical cycling (Henley et al., 2020).
Impact of Climate-Induced Physical Changes on Nutrient and Carbon Supply and Distribution
The ongoing and projected increase in wind speeds and storminess over large parts of the Southern Ocean is likely to increase upwelling and upper ocean mixing, thus micro- and macronutrient flux from the CDW source waters into the mixed layer (Moore et al., 2018; Rintoul, 2018). As regional warming continues, anticipated sea ice losses would also increase vertical mixing and nutrient supply by removing the barrier to wind-driven mixing of the surface ocean, as has been observed at the Antarctic Peninsula (Venables et al., 2013; Henley et al., 2017). The increased vertical flux of iron would be more important than that of macronutrients for biological productivity in the iron-limited open Southern Ocean (e.g., Leung et al., 2015). Increasing wind speeds, storminess and ice losses may also increase the outgassing of oceanic CO2, as has occurred in the past (Le Quere et al., 2007; Lenton et al., 2013). Shoaling of carbon- and nutrient-rich CDW in most regions around Antarctica (Schmidtko et al., 2014) is also likely to increase the flux of nutrients and carbon toward the ocean surface. In contrast, projected increases in glacial meltwater inputs may increase stratification around Antarctica with the potential to reduce vertical nutrient fluxes and availability to phytoplankton blooms (see section on freshening). This could limit primary production in locations and at times of year when surface macronutrients are drawn down to limiting concentrations (Henley et al., 2017, 2018; Höfer et al., 2019). Ice shelf disintegration could have complex effects on upper ocean mixing and vertical carbon and nutrient fluxes depending on the relative forcing of increased exposure to winds and altered strength and position of the buoyant meltwater plume (e.g., Randall-Goodwin et al., 2015). Projected increases in eddy activity within the ACC and the anticipated changes in ocean currents and frontal positions will influence the transport and distribution of nutrients and carbon around the Southern Ocean, as well as air-sea exchange of CO2 (Sallée et al., 2012; Rintoul, 2018; Chapman et al., 2020).
Biogeochemical Consequences of Climate-Driven Changes to Pelagic and Benthic Food Webs
The large-scale climatic and environmental drivers of changes in the abundance and distribution of marine organisms will also have important consequences for Southern Ocean biogeochemistry through export fluxes, carbon storage in pelagic and benthic food webs, and for benthic-pelagic coupling (Henley et al., 2020). For instance, the southward contraction of krill populations in the Atlantic sector and linked increases in salp abundance may reduce carbon transfer through the pelagic food web, with implications for carbon storage and export (Atkinson et al., 2004, 2019; Murphy et al., 2016). Phytoplankton, zooplankton and higher organisms also play an important role in benthic-pelagic coupling and the transport of nutrients and carbon vertically and horizontally (e.g., Lavery et al., 2010; Belcher et al., 2017; Cavan et al., 2019), which could be interrupted by shifts in abundance, distribution or trophic interactions. In contrast, increases in primary production and benthic biomass in response to reduced sea ice duration and longer growing seasons on the Antarctic Peninsula (Peck et al., 2010; Moreau et al., 2015) have the potential to increase carbon storage and invigorate benthic nutrient and carbon cycling as well as benthic-pelagic coupling (Barnes et al., 2018a). Benthic-pelagic coupling and water column transport are key to the (re)distribution of nutrients in the Southern Ocean, such that climate-forced changes in these mechanisms could hold severe consequences for the entire ecosystem. Because the microbial loop plays a decisive role in the fate of organic carbon in pelagic and benthic food webs (Azam et al., 1991; Sailley et al., 2013), climate-driven shifts in microbial communities and processes are very likely to have a strong impact on ecosystem carbon storage, benthic-pelagic coupling and regional biogeochemistry (Henley et al., 2019 and references therein).
Anthropogenic Drivers
Tourism
Antarctic Tourism started over 100 years ago (Headland, 1994) but the modern trend for ecotourism started in 1950’s and the first dedicated expedition cruise ship for the Southern Ocean was the Lindblad Explorer launched in 19691. There was a steep increase in shipborne tourism in Antarctic from around 100 visitors in the late 1950’s to a peak in the 2007–2008 season of 46,000. Visitor demographics are heavily affected by global socio-economic factors and the sharp decline following 2008 is attributed to the world economic crisis and the International Maritime Organization (IMO) ban on the use and carriage of heavy fuel oil in Antarctica. Traditionally Antarctic tourism is seen as an emerging market due to increasing global wealth. The industry began growing again steadily after 2011, reaching a new peak of 55,000 visitors in 2018–2019, mostly within the East Pacific and subAntarctic zone of the Atlantic MEASO areas, with most passenger landings and traffic concentrated at a few locations (Bender et al., 2016). Expedition cruising is the fastest growing market within the entire cruise industry, with new expedition cruise ships being built at the fastest rate in history, suggesting that, unless disease outbreaks pause the industry in the long term, this trend in visitor numbers is expected to continue (high confidence).
The nature of Antarctic ecotourism is also changing with the increased number of itineraries expanding the market to attract more active tourists, with options to kayak, camp on the ice, dive and snorkel with wildlife as well as skiing, including heli-skiing2. Additionally, fly cruising options are increasing with passengers flying into King George Island and starting their trip from there (Bastmeijer, 2009), spending more of their trip within the Southern Ocean. New vessels with the latest X-bow design are expected to increase the ability to push into ice and therefore expected to open up the West Pacific MEASO area (Ross Sea) to more tourism (medium confidence).
Today the large majority of all tour operators operating in Antarctica are members of the International Association of Antarctic Tour Operators (IAATO), a self-governing body, including all commercial SOLAS passenger ship operators (see footnote). The association’s membership comprises 105 companies and organizations from all over the world. The future protection of Antarctica from the impacts of human activity requires collaboration on a global scale. To promote effective visitor management, IAATO annually shares detailed information on its activities with Antarctic Treaty Parties. Without further regulation the geographic spread of visits is virtually certain to increase.
All of these developments are highly likely to increase the footprint of Antarctic tourism, potentially increasing the impact on marine ecosystems, particularly through the risk of the introduction of non-indigenous species. There are also concerns about the risk of tourists transferring anthropengic diseases to Antarctic seabirds. However, the greatest health concern for the cruise ship industry are over human disease transmission within the close quarters of cruise ships (Hill, 2019). These concerns have been amplified by the COVID-19 pandemic and the trajectory of SO tourism is now much less certain.
Pollutants
Many anthropogenic pollutants are resistant to environmental degradation and can be transported from human population sources to remote regions of the world, such as the Southern Ocean. Heavy metals have been detected in ice core samples, with, for example, lead from Australian mining operations appearing at concentrations of up to 6 pg.g–1 after the start of the industrial revolution (McConell et al., 2014). Radioactive isotopes of elements such as chlorine (36Cl) are, also, still being released from the cryosphere after nuclear bomb tests in the 50’s and 60’s (Pivot et al., 2019). The persistent organic pollutants (POPs; Krasnobaev et al., 2020) and marine debris, amongst which the plastics have become a focus for policy makers and the public alike (Rochman et al., 2016), will be discussed in detail here. Increase in atmospheric CO2 is covered within the previous biogeochemistry section and the impact of CFCs are covered in discussions about SAM/ENSO.
POPs consist of a number of different chemicals, including polychlorinated biphenyls (PCBs), organochloride pesticides (O) and polybrominated diphenyl ethers (PBDEs). A recent study of these compounds in the East Pacific region found comparable PCB and OCP concentrations to those detected 10 years earlier, but detected PBDEs for the first time (Krasnobaev et al., 2020). In contrast to some previous studies, there was no indication of local sources for this pollution, e.g., from research stations (Wild et al., 2015), rather that the pollution was highly likely to have come from atmospheric sources (Krasnobaev et al., 2020). This atmospheric origin is supported by the highest concentration of POPs being recorded in the surface phytoplankton, early in the summer, soon after the sea ice, and any snow cover has melted (Casal et al., 2018, 2019; Krasnobaev et al., 2020). With changes in the cryosphere, particularly the reduction in sea ice duration, and the associated changes in phytoplankton bloom dynamics, the seasonal pattern of POPs is projected to become less pronounced (high confidence). Global legislation, such as the Montreal protocol can only control chemicals that are widely used and once their effects are clearly demonstrated. History shows that novel industrial chemicals will continue to be developed with a considerable time lag before they are recognized as POPs.
Plastics are a global problem with levels in remote regions of the Atlantic. There has been a 10 fold increase in the quantity of plastic debris on beaches in the remote Falkland, Tristan da Cunha, St Helena and Ascension Islands in the last decade (Barnes et al., 2018b). The number of items increasing from approximately 1 to 10 per meter of beach (Barnes et al., 2018b). Large items of plastic drifting onto the Antarctic Peninsula were first reported nearly 20 years ago (Barnes and Fraser, 2003). These were first recognized as vectors for the introduction of non-indigenous species but in more recent times, the break down products of waste plastic, the micro- and nanoplastics have become a major concern as they can affect suspension and deposit feeders which are dominant members of the Antarctic benthos (Waller et al., 2017; Horton and Barnes, 2020), even affecting the immune system, as recently described in the sea urchin, Sterechinus neumayeri (Bergami et al., 2019). A recent study has also demonstrated effects of nanoplastics on keystone species such as the Antarctic krill (Bergami et al., 2020). The local sources of microplastics are discussed in the local drivers manuscript but there is also recent evidence of global transport of microplastics to the SO in the ocean currents and precipitation (Åslund, 2018).
Biological Drivers
Non-indigenous Species
The shallow seas of the SO and its outer lying archipelagos have high levels of endemism in the region; up to 70% in some taxa (David and Saucède, 2015). Here we term species not native to the region as non-indigenous species – NIS. Its relative historic isolation, means that it still has an almost entirely native biota (Convey et al., 2014; de Broyer et al., 2014), and is the only large surface environment on the planet where this is still the case (Bax et al., 2003; Early et al., 2016). There are thought to be many factors causing this, including its large size, historic geographic and environmental isolation by the ACC, and long periods of relatively constant environment (Ruiz and Hewitt, 2009). These attributes mean that NIS that are able to establish are likely to outcompete or prey upon native biota and entire native communities could be disrupted.
However, there are “barriers” to species movement. The Polar Front is both semi-porous and migratory over time (Clarke et al., 2005). Species can cross the Polar Front in short ecological time frames in eddies and rafting as well as over longer time frames with glaciation-interglacial shifts of ocean fronts and crawling along the seafloor (Sands et al., 2015; Fraser et al., 2018). Recent human activity, including increasing tourist visitation and more widespread national Antarctic operator logistical and research activity (Tin et al., 2014; Bender et al., 2016; Pertierra et al., 2017), have increased the types of vectors and opportunities, including ship hulls and plastic in the sea (Barnes, 2002; Lewis et al., 2003, 2004). Furthermore changes in the cryosphere and sea temperatures described above have also changed the potential survivorship of NIS propagules in transit to the region, their establishment and spread (Clarke et al., 2007; Turner et al., 2009; McCarthy et al., 2019). Risk of NIS transport and establishment in marine environments is not evenly spread around the southern polar region (Barnes et al., 2009) and threat levels are strongly coincident with human transport routes and destinations (Hughes et al., 2019; McCarthy et al., 2019). One of the main threats is seen as the bivalve mollusc genus Mytilus which are already well documented NIS elsewhere in the world (Hughes et al., 2019).
Preventative/biosecurity measures have been considerably ramped up and enforced within the last decade, including with the production of a Non-native Species Manual by the Committee for Environmental Protection (CEP, 2017) and biosecurity checklists by the Council of Manager of National Antarctic Programs (COMNAP/SCAR, 2010). Some steps have been taken to address NIS within ballast water, with the Antarctic Treaty Consultative Meeting and International Maritime Organisation (IMO) having agreed ballast water exchange protocols for ships entering the Southern Ocean (ATCM, 2006; International Maritime Organisation Marine Environment Protection Committee [IMO], 2007) and more stringent internationally applicable ballast water regulations, having entered into force in 2017, which require ships to treat or exchange ballast water (International Maritime Organisation [IMO], 2004). However, there is still little progress to reduce marine NIS risk associated with hull fouling, possibly due to the perceived expense and impact on itineraries of hull cleaning activities. There may also be the perception that traveling though sea ice will scour the hulls free of NIS, thereby removing the risk, but research has shown fouling species persisting in sea chests and water intakes ports on the ship hull (Lee and Chown, 2007; Hughes and Ashton, 2017) and many vessels operating in polar water actively avoid sea ice, removing any potential biosecurity benefit (McCarthy et al., 2019). Marine invasions are harder to detect and much harder (if not impossible) to reverse (Ojaveer et al., 2014) and effective biosecurity practices depend more on national operator measures rather than the action of individuals (e.g., the cost and coordinated effort required to clean a ship’s hull).
The majority of NIS records have been in the Atlantic and East Pacific regions where the majority of boat transits into the Southern Ocean occur (Figure 3). For example, a NIS marine algae (Ulva intestinalis) arrived and established at Deception Island (Clayton et al., 1997). It has also been reported from other frequently visited locations, Half Moon, Livingston, Robert, Nelson, King George, Penguin and Elephant Islands and sub-Antarctic Macquarie Island (Figure 3). There have been concerted efforts to bring experts together for NIS risk assessment, to identify most likely invader species and vectors and to provide stronger prevention and management advice (Key, 2018; Roy et al., 2019), which could be usefully undertaken within other regions of Antarctica.
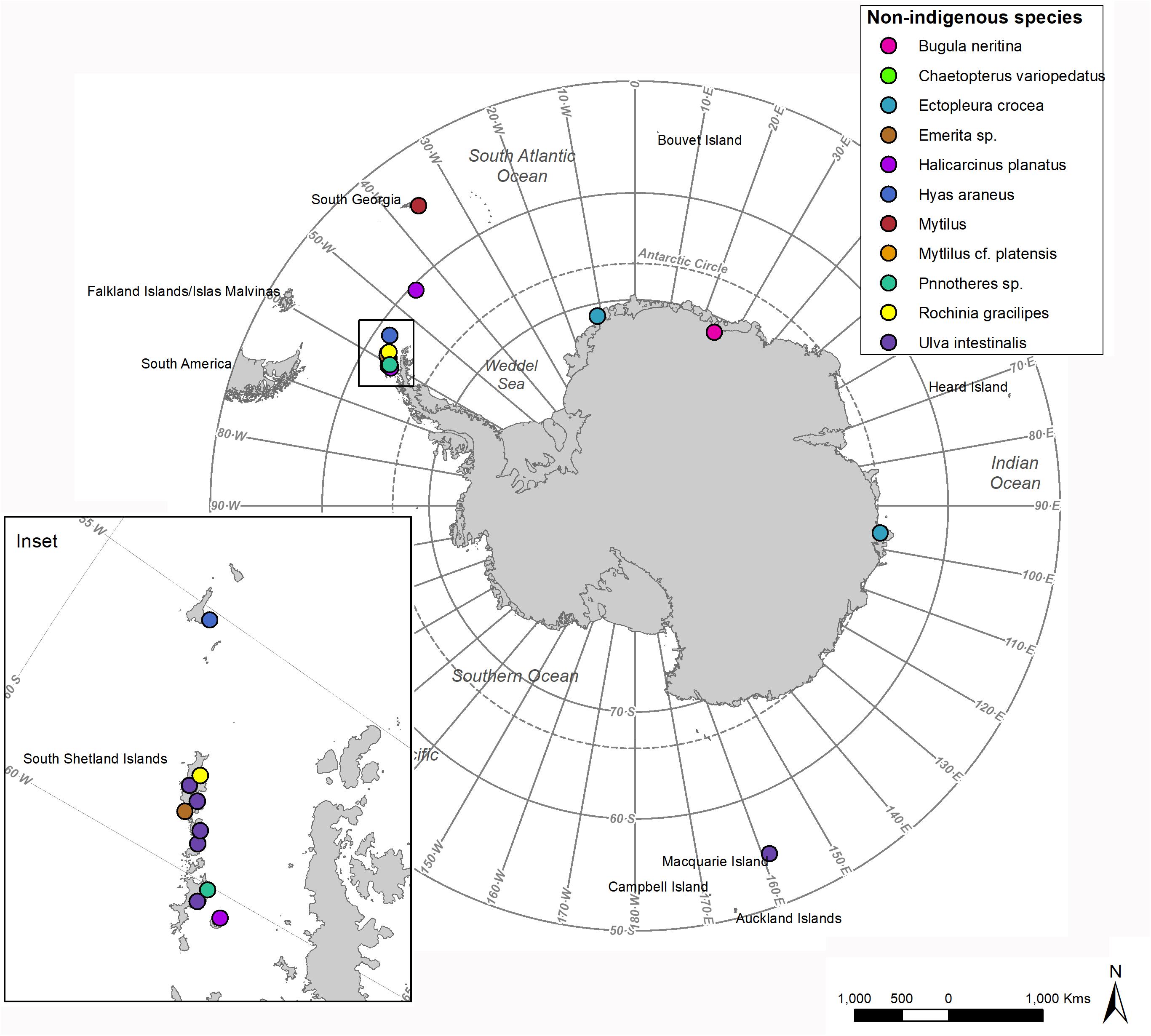
Figure 3. Locations of records of non-indigenous species within the MEASO region. References cited in text and McCarthy et al. (2019). Map produced using data from the SCAR Antarctic Digital Database.
New approaches to threats to native species (Morley et al., 2019) and most likely invaders (Hughes et al., 2019) have been through risk analysis. More than a hundred likely potential invaders were considered, mainly likely to come from southern South America and establish in the northern Antarctic Peninsula and Scotia Arc locations. Mytelid mussels were highest on the NIS risk, because they are abundant in ports, widely successful invaders elsewhere, have survived journeys to the SO (Lee and Chown, 2007) and tend to smother coastal life on establishment (Hughes et al., 2019). The strength of these approaches has been illustrated by the recently discovered population of Mytlilus cf. platensis which settled in a shallow subtidal habitat of the South Shetland Islands (Cárdenas et al., 2020).
Poleward movement of species to bottlenecks such as Patagonia, South Africa and Australasia is likely to intensify over the coming decades, increasing propagule pressure of potential NIS (Ruiz and Hewitt, 2009). The number of vessels, people and water-born debris set to reach the region also seems likely to continue to increase, as does climate-mediated environmental change (Barnes, 2002; Barnes et al., 2018b; McCarthy et al., 2019). Consideration of the problem and preventative action have both been stepped up for some pathways, but the challenge is considerable and the impacts and costs of NIS can clearly be seen in every other major non-polar environment.
Migratory Species and External Impacts
Migratory species in Southern Ocean ecosystems include pinnipeds, cetaceans and seabirds. Many of these species travel long distances to Antarctic and sub-Antarctic waters during the austral summer to forage on low-trophic level prey. Migratory species are uniquely vulnerable to changing environmental conditions due to their long-distance travel and reliance on a number of unique integrated factors (Leaper et al., 2006; Tulloch et al., 2017). These include whole suites of discrete sites remaining intact, the resources they require at those sites being available at the right time, and the connections between sites remaining within reach given a species’ movement capability (Newson et al., 2009).
Migratory species moving through Southern Ocean ecosystems face numbers of direct and indirect pressures from changing physical environments and human activities across their range. Within Antarctic waters, changing climate drivers will alter productivity regimes, which will very likely affect low trophic prey such as krill (Flores et al., 2012), with possible flow-on effects to dependent migratory fur seal (medium confidence; Croll and Tershy, 1998), baleen whale (high confidence; Tulloch et al., 2019) and seabird (medium confidence; Barbraud et al., 2012) predators that travel to Antarctic waters to forage. Many of these species are particularly vulnerable due to low abundances and slow population growth. Historical commercial exploitation of migratory fur seals and whales across the Southern Oceans pushed many species almost to extinction. Although some heavily depleted populations of seals and whales have begun to recover (Hucke-Gaete et al., 2004; Tulloch et al., 2017), populations of whale species including blue, fin and Southern rights are still well below their pre-exploitation numbers despite international protection. Some populations of Southern Ocean seabirds are very low numbers due to historical fisheries interactions, and are likely vulnerable to changes in adult mortality given their life history characteristics (high confidence; Phillips et al., 2016).
The long distance movements of Southern Ocean migratory species make them susceptible to other external anthropogenic impacts, such pollution, ship strikes fishing gear interactions diminishing food supplies, and reduced breeding habitats, all of which can reduce population numbers or negatively affect the fitness of an animal (Rosenbaum et al., 2014; Clapham, 2016).
While pollutant exposure is generally low within the SO, pollutants pose a serious risk to SO migratory species. Migratory seabirds such as south polar skuas (Catharacta maccormicki) and brown skua (Catharacta skua lönnbergi) have up to 20 times higher levels of the persistent organic pollutant (polybrominated diphenyl ethers) than endemic species that live year round within the SO, likely due to exposure to contamination events at lower latitudes during the non-breeding season when they migrate northward (high confidence; Yogui and Sericano, 2009; Corsolini et al., 2011).
Due to this exposure when outside of the SO, migratory species likely act as vectors for transporting pollutants into the SO (medium confidence; Yogui and Sericano, 2009; Corsolini et al., 2011). Marine debris can also enter the Antarctic ecosystem carried by nektonic animals such as seabirds and seals (Barnes et al., 2004). Fur seals can become entangled on the South American continental shelf or along populated shores (Laist, 1997; do Sul et al., 2011). During the austral winter migratory marine biota reach lower latitudes, when seabirds ingest floating plastics in the open ocean; on nesting areas, plastics may then be regurgitated to chicks (medium confidence; Fry et al., 1987; van Franeker and Bell, 1988; Wilcox et al., 2015). Greater quantities of plastics traveling in the SO likely increase the possibility of transport of colonists to Antarctica (medium confidence; Barnes, 2002). Terrestrial threats such as disease and predation may affect migratory seabirds nesting on land (Phillips et al., 2016). Introduced mammals are the foremost land-based threat to seabirds on sub-Antarctic islands (high confidence; Jouventin and Weimerskirch, 1991; Baker et al., 2002).
Since the early 90s, efforts conducted by the Committee for the Conservation of Antarctic Living Marine Resources (CCAMLR), via a series of conservation measures, have been very successful in addressing the problem of incidental mortality, especially seabirds, in the Convention area. However, during migrations outside of the SO bycatch and entanglement in fisheries gear are recognized as the most significant threat to the survival of cetacean and seabird species and populations globally (Read, 2008; IWC, 2010; Barbraud et al., 2012). Continued industrialization of fisheries have led to intensification of fishing effort in many regions (Pauly, 2009), including increased presence of fishing gear in whale habitat (Lewison et al., 2004; Read et al., 2006), increasing the risk of interactions with such gear (Cassoff et al., 2011). Binding legal requirements to reduce bycatch exist in the national legislation of many countries. However, despite new technologies and industry recognition of the issue, monitoring and management can be costly, and/or ineffective, and bycatch remains a major global problem (Tulloch et al., 2020). Unregulated or increased longline and trawl fishing in key breeding areas for vulnerable species of albatross (e.g., Thalassarche melanophrys, Diomedea spp.) is likely to lead to continued population declines (high confidence; Tuck et al., 2003; Phillips et al., 2016). Some baleen whale populations (e.g., southern right whales in the southeastern Pacific off Chile and Peru) are small and endangered, and, for them, even small numbers of fisheries gear entanglements are potentially significant (low confidence; Reeves et al., 2013).
Other external drivers affecting SO migratory species include vessel traffic and mining activities. Ship strike/collision can result in injury, displacement, disturbance, and behavioral modification (high confidence; van der Hoop et al., 2015; Pirotta et al., 2019). The number of reported vessel strike incidents has increased over the last 2 decades (Peel et al., 2018), but reporting biases exist related to species identification, spatial coverage of reports and type of vessels involved, and quantifying the rate of occurrence of these collisions is difficult because many incidents are not detected. Southern right whale (Eubalaena australis) populations off South Africa and off eastern South America (Brazil, Uruguay and Argentina) have historically suffered high mortality due to vessel strikes (56 reported cases before 2007, high confidence; van Waerebeek et al., 2007). Studies show likely behavioral modifications of migratory whales including southern right and humpback whales (Megaptera novaeangliae) with reduced foraging and shifting of traditional use areas in response to noise from human activities such as shipping and seismic surveys (high confidence; Parks et al., 2007; Dunlop et al., 2010; Blair et al., 2016).
Some migratory animal populations may also be susceptible to diminishing food supplies in regions where fisheries are poorly managed, or where climate change impacts may affect lower trophic prey populations. Overfishing can have profound implications for higher-order predators. Population-level impacts on seabirds such as albatrosses and petrels may occur through direct competition with fisheries for prey (medium confidence; Croxall and Gales, 1998; Baker et al., 2002). The level (and the effects) of competition for food resources between seabird populations and fisheries, however, is uncertain. Lower trophic prey such as krill are expected to be affected by future warming oceans given climate change (high confidence; Flores et al., 2012). It is uncertain how climate change may facilitate or hinder the recovery of species that forage heavily on krill or rely on stable sea-ice conditions in rapidly warming regions of the SO (Simmonds and Isaac, 2007). Blue (Balaenoptera musculus), fin (Balaenoptera physalus) and humpback whales that forage in mid-latitudes may be the most at risk from climate-induced impacts on their prey (medium confidence; Tulloch et al., 2019).
Assessment
Main Drivers and Their Interactions
The global physical and human drivers, along with their interactions, were summarized from the proceeding text into a qualitative network diagram (Figure 4). The Northern drivers, those whose influence on the SO comes from North of the region, dominate the changes happening within the SO. The major effect of the ozone hole on atmospheric circulation and wind strength which, in turn, have had contrasting regional effects on the cryosphere of the West Pacific (Ross Sea) and East Pacific (western Antarctic Peninsula) sectors of the SO, were a consistent theme throughout the assessment. The importance of the additive interactive effects of SAM and ENSO were also repeated. The other key human driver is the increase in CO2, which is not only linked to warming, and its impact on the cryosphere, but will also lead to ocean acidification and affect the carbon cycle (high confidence). This is expected to have an impact on both benthic and pelagic ecosystems (high confidence). Unless human emissions are controlled, the comparatively limited current impact of pollution and non-indigenous species is expected to increase into the future (high confidence).
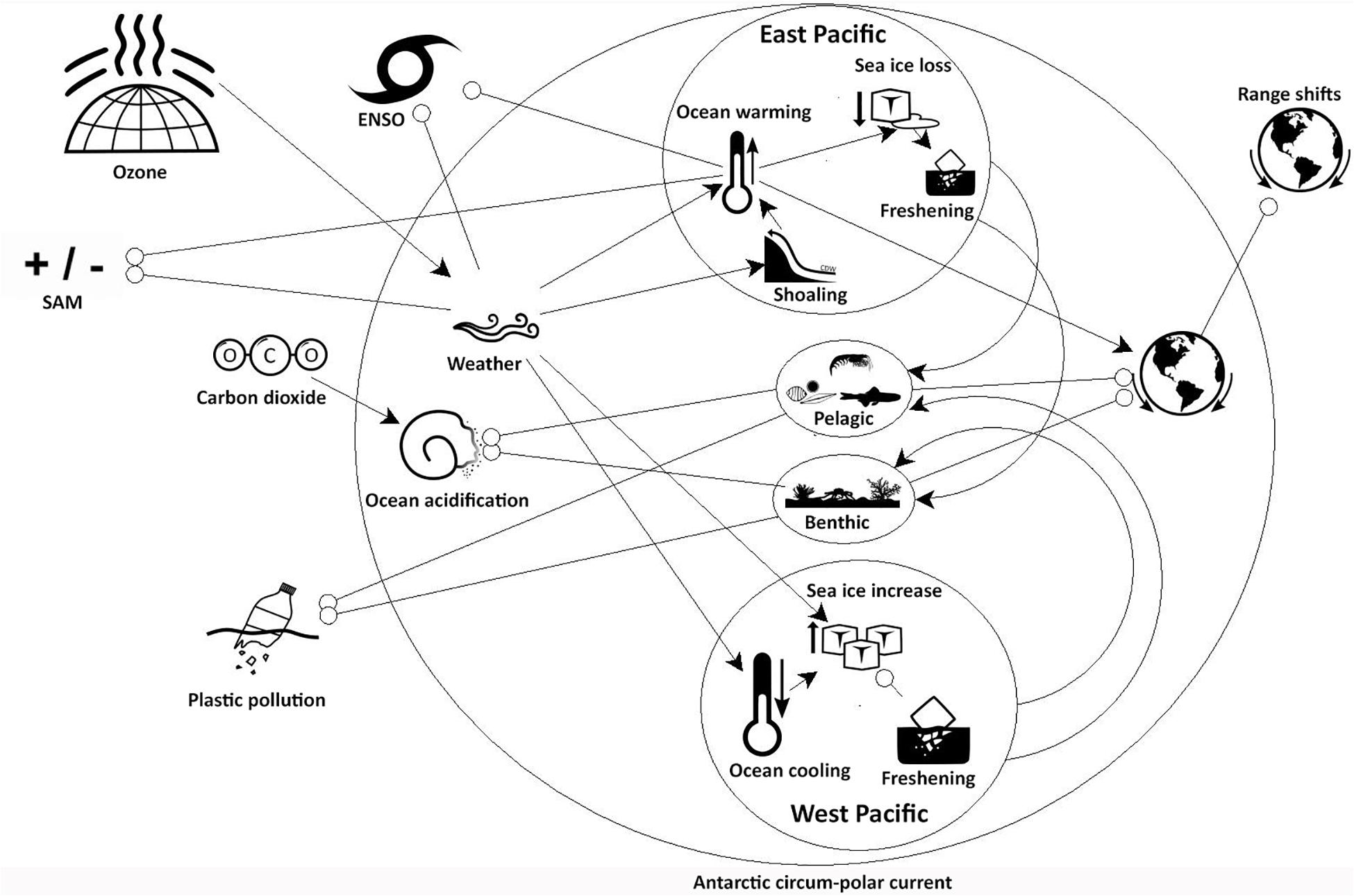
Figure 4. Qualitative network diagram describing the linkages between the global drivers and the benthic and pelagic Southern Ocean assemblages. Northern drivers are global drivers whose influence comes from North of the Antarctic cirucm-polar current (ACC). Contrasting effects are highlighted by separate west Pacific (western Antarctic Peninsula) and east Pacific regional networks. Range shifts from north of the ACC (Non-indigenous species) and within the Southern Ocean are indicated separately. An arrow head indicates a positive influence, an open circle indicates a negative influence.
Future Prognosis and Priorities
The future projections of the global drivers highlighted in the proceeding literature review are summarized through the assessment in Table 1. One of the main themes running through this assessment are the large uncertainties in the models that are used to make these projections. Only a concerted international effort will enable these uncertainties to be reduced (Box 1). International frameworks, working groups and grants, such as the Antarctic Treaty System and CCAMLR, the Southern Ocean Observing System (SOOS) and MEASO, and the EU Rise network project CoastCarb, are essential to ensure consistent approaches are applied across the region.
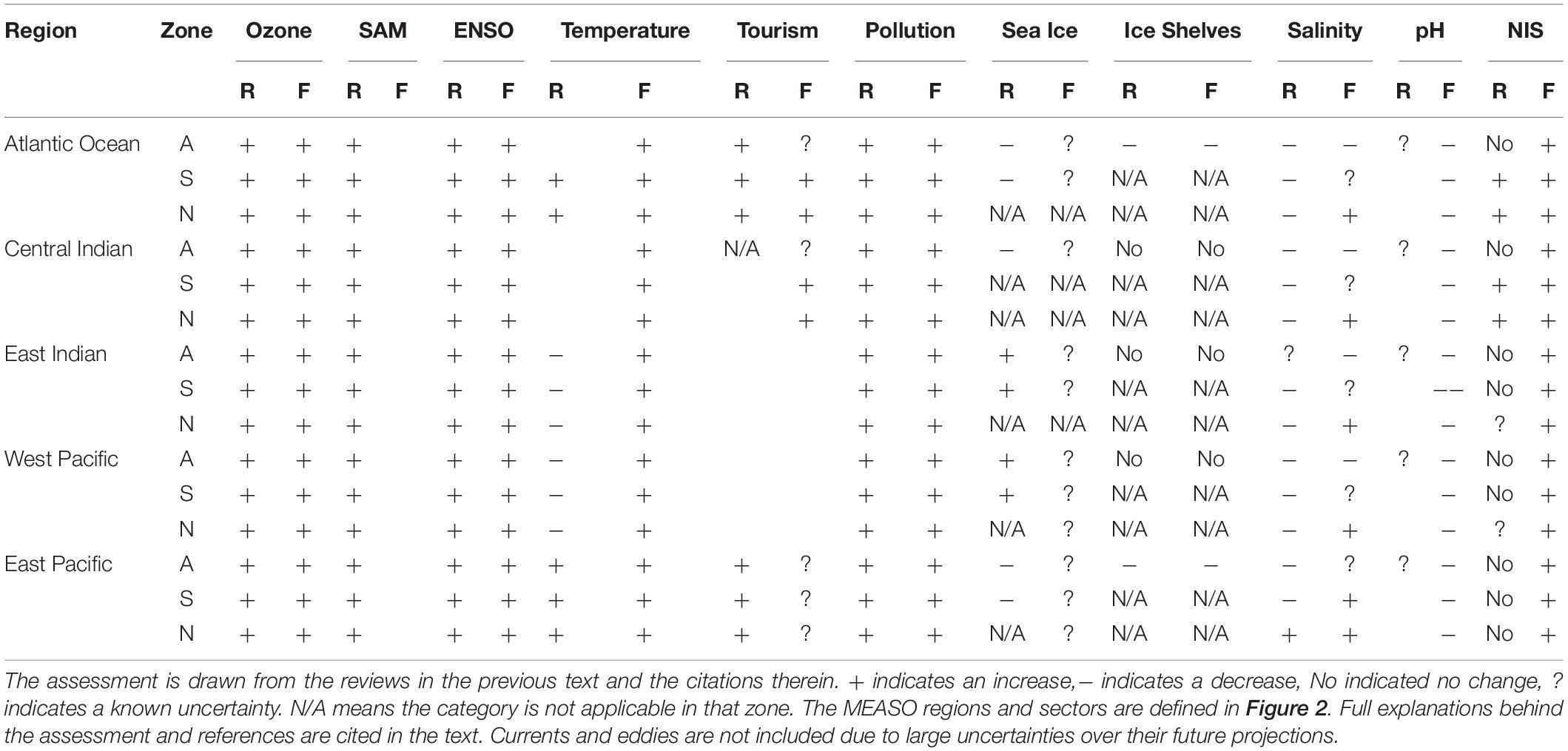
Table 1. Assessment of the current direction of each of the identified global drivers within the MEASO sectors, in recent times (R) and in the future (F).
Box 1. Research Gaps and Priorities.
Modeling the trajectories of climate change is one of the biggest challenges to understanding the future impacts of drivers on the Southern Ocean and a key limitation to future projections. Whereas arctic environmental responses to climate over the last decade have been continuous and homogeneous, Antarctic and SO changes are regional and variable (Stammerjohn et al., 2012; Parkinson, 2019), resulting in ambiguous effects when looking at the global scale.
There is also a degree of uncertainty over when tipping points will be reached (e.g., Timmermann and Hellmer, 2013; Hellmer et al., 2017). Current model predictions for sea ice extent and duration have been poor despite the mechanisms driving sea ice formation/melting having such a profound impact on the SO (Reid and Massom, 2015). This is typified by the retreat of tidewater glaciers and floating ice shelves and the number of icebergs that will be present to scour the sea floor. At a certain point the trend will move from increasing to reducing numbers of icebergs being present, starting to reverse the trends in ice scour (Barnes et al., 2020). Whether this will happen before irrecoverable change has occurred within benthic communities is hard to predict.
The very limited availability of long-term time series hinders our ability to discern between interannual/interdecadal changes and long-term trends in ecosystem shift. It is difficult to inform policy and decision makers without a clear distinction between natural variability and global change impacts. Funding agencies must allocate money to maintain long-term sampling programs. Continued and improved data collection through international effort to obtain standardized datasets, developments in modeling and integrated work programs, such as the Southern Ocean Observing System (Newman et al., 2019) are allowing improved coverage and better predictions of future drivers across the Southern Ocean. Only through funding of extended time series can trajectories of change be monitored and tipping points be better constrained.
As with all remote regions, data coverage is better near locations with the greatest human footfall. This footfall is also higher in summer than winter. Remote sensing from satellites and remote monitoring stations are key tools to increase the spatio-temporal coverage and improve the reliability of projections that can significantly reduce the cost of long term sampling programs. The increase in continuous observing systems will be key to improving data coverage. Innovation, technological advancements on autonomous vehicles and other platforms (e.g., oceanographic moorings), including the ability to measure under the seasonal ice cover and floating ice sheets should contribute towards improving our understanding of processes occurring during the winter, and how they change in longer time-scales.
Understanding if human behavior will change, and how international treaties will limit the pace of climate change, will be key. Industrial process are developing novel chemicals, some of these are designed to replace substances that are currently controlled, such as CFCs. However, some of these replacements, such as SF6, are known to be potent climate gases (Widger and Haddad, 2018) which could markedly change rates of atmospheric warming. The fate of new industrial chemicals needs to be tracked and understood so that their impacts can be determined and impacts controlled before they have major impacts.
Many of the changes in global drivers that have affected SO marine ecosystems over recent decades were strongly linked to the loss of atmospheric ozone since the 1970s (very high confidence; Table 1 and Figure 4). One of the key future uncertainties about how global drivers of SO marine ecosystems will change, therefore, relates to the impact of the expected reversal of the ozone hole. Will this start to reverse some of the recent physical changes, or will global and regional increases in temperature override this? The best estimates suggest that within decades the global increase in temperature is highly likely to override any cessation of warming in the Eastern Pacific sector, particularly the Antarctic Peninsula (high confidence; Turner et al., 2016; Rogers et al., 2020). Depending on the trend in SAM, the reversal of the stronger winds may reduce the cooling influence in the East Indian and West Pacific zones, ultimately leading to warming across the whole of the SO (medium confidence).
While the reversal of the winds may slow the shoaling of warm water onto SO shelves (medium confidence), the increase in global air temperatures is very likely to continue the disintegration (giant iceberg formation) and melting of ice shelves. The number or glaciers in retreat and their retreat rate will further increase and many will soon reach ground lines (very high confidence). To make predictions for sea ice change is much less clear. The mechanisms underlying the recent drop from near recent maximum to minimum sea ice extent since spring 2014 including the time-scale over which these drivers are operating, is uncertain (low confidence). As one of the key drivers for SO marine ecosystems, and crucial for key SO species such as krill and some top predators, this leaves a large area of uncertainty around the future of sea ice.
Some pollutants such as CFC’s, are subject to global agreements, their levels are controlled and their impacts are reversing (medium confidence). As the impact of other industrial climate gases are identified (e.g., sulfur hexafluoride, SF6; Widger and Haddad, 2018) research on which pollutants need to be controlled will need to keep pace with technological developments. However, even under the best case future emission scenarios atmospheric CO2 will continue to increase (high confidence), adding to global increases in temperature. CO2 will continue to dissolve into the ocean, lowering the pH. Year round measurements from a wider variety of locations, are required to constrain uncertainties in the models.
Other anthropogenic drivers also have degrees of uncertainty. Until very recently global tourism was expected to increase in parallel with economic prosperity, however, growing health concerns over mass tourism (Hill, 2019) have been amplified by the COVID-19 pandemic and the trajectory of near future SO tourism is now highly uncertain. There are also concerns about the risk of tourists transferring anthropengic diseases to Antarctic seabirds which may become reflected in future recommendations of IAATO and the Antarctic Treaty (Cerdà-Cuéllar et al., 2019).
In particular the necessity of determining between trends that are the result of natural variability and those that are anthropogenic or the result of climate change requires an increased number of extended time series. Process studies, to understand the underlying mechanisms of this variability, must be a priority. Efforts to improve the observational records around Antarctica are critical to understand the nature of change around the continent and to predict the impacts on marine ecosystems.
Author Contributions
All authors listed have made a substantial, direct and intellectual contribution to the work, and approve it for publication.
Funding
All authors contributions were funded by their institutions except for the following. JH was funded through FONDECYT (POSTDOCTORADO 3180152) and ANID (FONDAP IDEAL 15150003). SH was supported by the UK Natural Environment Research Council through grant NE/K010034/1. CS was partly funded by the United States Department of Agriculture, National Institute of Food and Agriculture (Project #RI0019- H020). SS was supported by NSF grant PLR1440435. CM was supported by NSF-OPP grant 1703310.
Conflict of Interest
The authors declare that the research was conducted in the absence of any commercial or financial relationships that could be construed as a potential conflict of interest.
Acknowledgments
This work is a core contribution to the first Marine Ecosystem Assessment for the Southern Ocean (MEASO). We thank the MEASO Support Group and Steering Committee for assisting with figures, coordination and editing of the text.
Footnotes
- ^ www.iaato.org
- ^ https://www.adventuresmithexplorations.com/cruises/antarctica/new-expedition-ships/
References
Abrahamsen, E. P., Meijers, A. J. S., Polzin, K. L., Naveira Garabato, A. C., King, B. A., Firing, Y. L., et al. (2019). Stabilization of dense Antarctic water supply to the Atlantic Ocean overturning circulation. Nat. Clim. Chang. 9, 742–746. doi: 10.1038/s41558-019-0561-2
Andersson, A., Mackenzie, F. T., and Bates, N. R. (2008). Life on the margin: implications of ocean acidification on Mg-calcite, high latitude and cold water marine calcifiers. Mar. Ecol. Prog. Ser. 373, 265–273. doi: 10.3354/meps07639
Andrew, S. M., Morell, H. T., Strzepek, R. F., Boyd, P. W., and Ellwood, M. J. (2019). Iron availability influences the tolerance of southern ocean phytoplankton to warming and elevated irradiance. Front. Mar. Sci. 6:681. doi: 10.3389/fmars.2019.00681
Ashton, G. V., Morley, S. A., Barnes, D. K. A., Clark, M. S., and Peck, L. S. (2017). Warming by 1°C drives species and assemblage level responses in Antarctica’s marine shallows. Curr. Biol. 27, 2698–2705. doi: 10.1016/j.cub.2017.07.048
Åslund, C. (2018). Microplastics and Persistent Fluorinated Chemicals in the Antarctic. Amsterdam: Greenpeace International, 1–11.
ATCM (2006). Practical Guidelines for Ballast Water Exchange in the Antarctic Treaty Area. Annex to Resolution 3. Available at: https://www.ats.aq/e/ep_marine.htm (accessed February 4, 2020).
Atkinson, A., Hill, S. L., Pakhomov, E. A., Siegel, V., Reiss, C. S., Loeb, V. J., et al. (2019). Krill (Euphausia superba) distribution contracts southward during rapid regional warming. Nat. Clim. Change 9, 142–147. doi: 10.1038/s41558-018-0370-z
Atkinson, A., Siegel, V., Pakhomov, E., and Rothery, P. (2004). Long-term decline in krill stock and increase in salps within the Southern Ocean. Nature 432, 100–103. doi: 10.1038/nature02996
Azam, F., Smith, D. C., and Hollibaugh, J. T. (1991). The role of the microbial loop in antarctic pelagic ecosystems. Pol. Res. 10, 239–243. doi: 10.1111/j.1751-8369.1991.tb00649.x
Baker, G. B., Gales, R., Hamilton, S., and Wilkinson, V. (2002). Albatrosses and petrels in Australia: a review of their conservation and management. EMU 102, 71–97. doi: 10.1071/mu01036
Barbraud, C., Rolland, V., Jenouvrier, S., Nevoux, M., Delord, K., and Weimerskirch, H. (2012). Effects of climate change and fisheries bycatch on Southern Ocean seabirds: a review. Mar. Ecol. Prog. Ser. 454, 285–307. doi: 10.3354/meps09616
Barnes, D. K. (2002). Biodiversity: invasions by marine life on plastic debris. Nature 416, 808–809. doi: 10.1038/416808a
Barnes, D. K. A., Fleming, A., Sands, C. J., Quartino, M. L., and Dereqibus, D. (2018a). Icebergs, Sea ice, blue carbon and Antarctic climate feedbacks. Philos. Trans. R. Soc. A 376:20170176. doi: 10.1098/rsta.2017.0176
Barnes, D. K. A., and Fraser, K. P. P. (2003). Rafting by five phyla on man-made flotsam in the Southern Ocean. Mar. Ecol. Prog. Ser. 262, 289–291. doi: 10.3354/meps262289
Barnes, D. K. A., Kaiser, S., Griffiths, H. J., and Linse, K. (2009). Marine, intertidal, freshwater and terrestrial biodiversity of an isolated polar archipelago. J. Biogeogr. 36, 756–769. doi: 10.1111/j.1365-2699.2008.02030.x
Barnes, D. K. A., Morley, S. A., Bell, J., Brewin, P., Brigden, K., Collins, M., et al. (2018b). Marine plastics threaten giant atlantic marine protected areas. Curr. Biol. 28, R1137–R1138.
Barnes, D. K. A., Sands, C. J., Cook, A., Howard, F., Roman Gonzalez, A., Muñoz–Ramirez, C., et al. (2020). Blue carbon gains from glacial retreat along Antarctic fjords: What should we expect? Glob. Change Biol. 26, 2750–2755. doi: 10.1111/GCB.15055
Barnes, D. K. A., Warren, N. L., Webb, K., Phalan, B., and Reid, K. (2004). Polar pedunculate barnacles piggy-back on pycnogona, penguins, pinniped seals and plastics. Mar. Ecol. Prog. Ser. 284, 305–310. doi: 10.3354/meps284305
Bastmeijer, K. (2009). “A long term strategy for Antarctic tourism: the key to decision making within the antarctic treaty system?,” in Polar Tourism: Human, Environmental and Governance Dimensions, Cognizant Communication Corporation, eds P. Maher, E. Stewart, and M. Lück, (Elmsford, NY: Taylor & Francis).
Bax, N., Williamson, A., Aguero, M., Gonzalez, E., and Geeves, W. (2003). Marine invasive alien species: a threat to global biodiversity. Mar. Policy 27, 313–323. doi: 10.1016/s0308-597x(03)00041-1
Bednaršek, N., Tarling, G. A., Bakker, D. C., Fielding, S., Jones, E. M., Venables, H. J., et al. (2012). Extensive dissolution of live pteropods in the Southern Ocean. Nat. Geosci. 5, 881–885. doi: 10.1038/ngeo1635
Belcher, A., Tarling, G. A., Manno, C., Atkinson, A., Ward, P., Skaret, G., et al. (2017). The potential role of Antarctic krill faecal pellets in efficient carbon export at the marginal ice zone of the South Orkney Islands in spring. Pol. Biol. 40, 2001–2013. doi: 10.1007/s00300-017-2118-z
Bender, N. A., Crosbie, K., and Lynch, H. J. (2016). Patterns of tourism in the Antarctic Peninsula region: a 20-year analysis. Ant. Sci. 28, 194–203. doi: 10.1017/s0954102016000031
Bergami, E., Krupinski, E. A., González-Aravena, M., Cárdenas, C. A., Hernández, P., Silva, J. R. M. C., et al. (2019). Polystyrene nanoparticles affect the innate immune system of the Antarctic sea urchin Sterechinus neumayeri. Pol. Biol. 42, 743–757. doi: 10.1007/s00300-019-02468-6
Bergami, E., Manno, C., Capello, S., Vannnuccini, M. L., and Corsi, I. (2020). Nanoplastics affect moulting and faecal pellet sinking in Antarctic krill (Euphausia superba) juveniles. Environ. Int. 143:105999. doi: 10.1016/j.envint.2020.105999
Bers, A. V., Momo, F., Schloss, I. R., and Abele, D. (2013). Analysis of trends and sudden changes in long-term environmental data from King George Island (Antarctica): relationships between global climatic oscillations and local system response. Clim. Change 116, 789–803. doi: 10.1007/s10584-012-0523-4
Bertolin, M. L., and Schloss, I. R. (2009). Phytoplankton production after the collapse of the Larsen A Ice Shelf, Antarctica. Polar Biol. 32, 1435–1446. doi: 10.1007/s00300-009-0638-x
Blair, H. B., Merchant, N. D., Friedlaender, A. S., Wiley, D. N., and Parks, S. E. (2016). Evidence for ship noise impacts on humpback whale foraging behaviour. Biol. Lett. 12, 1–5.
Bopp, L., Resplandy, L., Orr, J. C., Doney, S. C., Dunne, J. P., Gehlen, M., et al. (2013). Multiple stressors of ocean ecosystems in the 21st century: projections with CMIP5 models. Biogeosciences 10, 6225–6245. doi: 10.5194/bg-10-6225-2013
Bost, C., Cotté, C., Terray, P., Barbraud, C., Bon, C., Delord, K., et al. (2015). Large-scale climatic anomalies affect marine predator foraging behaviour and demography. Nat. Commun. 6:8220. doi: 10.1038/ncomms9220
Bowie, A. R., Lannuzel, D., Remenyi, T. A., Wagener, T., Lam, P. J., Boyd, P. W., et al. (2009). Biogeochemical iron budgets of the Southern Ocean south of Australia: Decoupling of iron and nutrient cycles in the subantarctic zone by the summertime supply. Glob. Biogeochem. Cycles 23:GB4034.
Bracegirdle, T. J., Krinner, G., Tonelli, M., Haumann, F. A., Naughten, K. A., Rackpw, T., et al. (2020). Twenty first century changes in Antarctic and Southern Ocean surface climate in CMIP6. Atmos. Sci. Lett. 21:e984. doi: 10.1002/asl.984
Brearley, J. A., Moffat, C., Venables, H. J., Meredith, M. P., and Dinniman, M. S. (2019). The role of eddies and topography in the export of shelf waters from the West Antarctica Peninsula shelf. J. Geophys. Res. 124:2018JC014679. doi: 10.1029/2018JC014679
Bronselaer, B., Winton, M., Griffies, S. M., Hurlin, W. J., Rodgers, K. B., Sergienko, O. V., et al. (2018). Change in future climate due to Antarctic meltwater. Nature 564, 53–58. doi: 10.1038/s41586-018-0712-z
Caldeira, K., and Wickett, M. E. (2003). Oceanography: anthropogenic carbon and ocean pH. Nature 425:365. doi: 10.1038/425365a
Canadell, J. G., Le Quéré, C., Raupach, M. R., Field, C. B., Buitenhuis, E. T., Ciais, P., et al. (2007). Contributions to accelerating atmospheric CO2 growth from economic activity, carbon intensity, and efficiency of natural sinks. Proc. Nat. Acad. Sci. U.S.A. 104, 18866–18870. doi: 10.1073/pnas.0702737104
Cape, M. R., Vernet, M., Skvarca, P., Marinsek, S., Scambos, T., and Domack, E. (2015). Foehn winds link climate-driven warming to ice shelf evolution in Antarctica. J. Geophys. Res. Atmos. 120, 11–037.
Cárdenas, L., Leclerc, J., Bruning, P., Garrido, I., Détrée, C., Figueroa, A., et al. (2020). First mussel settlement observed in Antarctica reveals the potential for future invasions. Sci. Rep. 10:5552.
Carleton, A. M. (2003). Atmospheric teleconnections involving the Southern Ocean. J. Geophys. Res. 108:JC000379. doi: 10.1029/2000JC000379
Casal, P., Cabrerizo, A., Vila-Costa, M., Pizarro, M., Jiménez, B., and Dachs, J. (2018). Pivotal role of snow deposition and melting driving fluxes of polycyclic aromatic hydrocarbons at Coastal Livingston Island (Antarctica). Environ. Sci. Technol. 52, 12327–12337. doi: 10.1021/acs.est.8b03640
Casal, P., Casas, G., Vila-Costa, M., Cabrerizo, A., Pizarro, M., Jiménez, B., et al. (2019). Snow amplification of persistent organic pollutants at Coastal Antarctica. Environ. Sci. Technol. 53, 8872–8882. doi: 10.1021/acs.est.9b03006
Cassoff, R. M., Moore, K. M., McLellan, W. A., Barco, S. G., Rotstein, D. S., and Moore, M. J. (2011). Lethal entanglement in baleen whales. Dis. Aquat. Organ. 96, 175–185. doi: 10.3354/dao02385
Cavalieri, D. J., and Parkinson, C. L. (2012). Arctic sea ice variability and trends, 1979-2010. Cryosphere 6, 881–889. doi: 10.5194/tc-6-881-2012
Cavan, E. L., Belcher, A., Atkinson, A., Hill, S. L., Kawaguchi, S., Mccormack, S., et al. (2019). The importance of Antarctic krill in biogeochemical cycles. Nat. Commun. 10:4742.
Cerdà-Cuéllar, M., Moré, E., Ayats, T., Aguilera, M., Muñoz-González, S., Antilles, N., et al. (2019). Do humans spread zoonotic enteric bacteria in Antarctica? Sci. Total Environ. 654, 190–196. doi: 10.1016/j.scitotenv.2018.10.272
Chapman, C. C., Lea, M.-A., Meyer, A., Sallée, J.-B., and Hindell, M. (2020). Defining Southern Ocean fronts and their influence on biological and physical processes in a changing climate. Nat. Clim. Chang. 10, 209–219. doi: 10.1038/s41558-020-0705-4
Chidichimo, M. P., Donohue, K. A., Watts, D. R., and Tracey, K. L. (2014). Baroclinic transport time series of the antarctic circumpolar current measured in Drake Passage. J. Phys. Oceanogr. 44, 1829–1853. doi: 10.1175/JPO-D-13-071.1
Clapham, P. J. (2016). Managing leviathan: conservation challenges for the great whales in a post-whaling world. Oceanography 29, 214–225.
Clark, G. F., Stark, J. S., Johnston, E. L., Runcie, J. W., Goldsworthy, P. M., Raymond, B., et al. (2013). Light-driven tipping points in polar ecosystems. Glob. Change Biol. 19, 3749–3761. doi: 10.1111/gcb.12337
Clarke, A., Barnes, D. K. A., and Hodgson, D. A. (2005). How isolated is Antarctica? Trends Ecol. Evol. 20, 2221–2223.
Clarke, A., and Johnston, N. M. (2003). Antarctic marine benthic diversity. Oceanogr. Mar. Biol. 41, 47–114.
Clarke, A., Murphy, E. J., Meredith, M. P., King, J. C., Peck, L. S., Barnes, D. K. A., et al. (2007). Climate change and the marine ecosystem of the western Antarctic Peninsula. Phil. Trans. Soc. B 362, 149–166. doi: 10.1098/rstb.2006.1958
Clayton, M. N., Wiencke, C., and Klöser, H. (1997). New records of temperate and sub-Antarctic marine benthic macroalgae from Antarctica. Pol. Biol. 17, 141–149. doi: 10.1007/s003000050116
COMNAP/SCAR (2010). Checklists for Supply Chain Managers of National Antarctic Programs to Reduce the Risk of Transfer of Non-Native Species. Available at: https://www.comnap.aq/Shared%20Documents/nnschecklists.pdf (accessed February 5, 2020).
Constable, A. J., Melbourne-Thomas, J., Conrey, S. P., Arrigo, K. R., Barbraud, C., Barnes, D. K., et al. (2014). Climate change and Southern Ocean ecosystems I: how changes in physical habitats directly affect marine biota. Glob. Chang. Biol. 20, 3004–3015.
Convey, P., Chown, S. L., Clarke, A., Barnes, D. K. A., Cummings, V., Ducklow, et al. (2014). The spatial structure of Antarctic biodiversity. Ecol. Monogr. 84, 203–244.
Cook, A. J., Holland, P. R., Meredith, M. P., Murray, T., Luckman, A., and Vaughan, D. G. (2016). Ocean forcing of glacier retreat in the western Antarctic Peninsula. Science 353, 283–286. doi: 10.1126/science.aae0017
Corsolini, S., Borghesi, N., Ademollo, N., and Focardi, S. (2011). Chlorinated biphenyls and pesticides in migrating and resident seabirds from East and West Antarctica. Environ. Int. 37, 1329–1335. doi: 10.1016/j.envint.2011.05.017
Cristofari, R., Liu, X., Bonadonna, F., Cherel, Y., Pistorius, P., Le Maho, Y., et al. (2018). Climate-driven range shifts of the king penguin in a fragmented ecosystem. Nat. Clim. Change 8, 245–251. doi: 10.1038/s41558-018-0084-2
Croll, D. A., and Tershy, B. R. (1998). Penguins, fur seals, and fishing: prey requirements and potential competition in the South Shetland Islands, Antarctica. Polar Biol. 19, 365–374. doi: 10.1007/s003000050261
Croxall, J. P., and Gales, R. (1998). “An assessment of the conservation status of albatrosses,” in The Albatross Biology and Conservation, eds R. Robertson and R. Gales, (Chipping Norton, UK: Surrey Beatty and Sons), 46–65.
David, B., and Saucède, T. (2015). “Southern Ocean biogeography and communities,” in Biodiversity of the Southern Ocean, eds T. Saucède and B. David, (Amsterdam: Elsevier), 43–57. doi: 10.1016/b978-1-78548-047-8.50004-7
De Baar, H. J. W., Buma, A. G. J., Nolting, R. F., Cadée, G. C., Jacques, G., and Tréguer, P. J. (1990). On iron limitation of the Southern Ocean: experimental observations in the Weddell and Scotia Seas. Mar. Ecol. Prog. Ser. 65, 105–122. doi: 10.3354/meps065105
De Baar, H. J. W., De Jong, J. T. M., Bakker, D. C. E., Loscher, B. M., Veth, C., Bathmann, U., et al. (1995). Importance of iron for plankton blooms and carbon dioxide drawdown in the Southern Ocean. Nature 373, 412–415. doi: 10.1038/373412a0
de Broyer, C., Koubbi, P., Griffiths, H. J., Raymond, B., d’Udekem d’Acoz, C., and Van de Putte, A. P., et al. (eds) (2014). Biogeographic Atlas of the Southern Ocean. Cambridge, MA: Scientific Committee on Antarctic Research.
de Lavergne, C., Palter, J., Galbraith, E. D., Bernardello, R., and Marinov, I. (2014). Cessation of deep convection in the open Southern Ocean under anthropogenic climate change. Nat. Clim. Change 4, 278–282. doi: 10.1038/nclimate2132
Deppeler, S., Petrou, K., Schulz, K. G., Westwood, K., Pearce, I., Mckinlay, J., et al. (2018). Ocean acidification of a coastal Antarctic marine microbial community reveals a critical threshold for CO2 tolerance in phytoplankton productivity. Biogeosciences 15, 209–231. doi: 10.5194/bg-15-209-2018
Desbruyeres, D., McDonagh, E. L., King, B. A., and Thierry, V. (2017). Global and full-depth ocean temperature trends during the early twenty-first century from argo and repeat hydrography. J. Clim. 30, 1985–1997. doi: 10.1175/JCLI-D-16-0396.1
Devries, T. (2014). The oceanic anthropogenic CO2 sink: storage, air-sea fluxes, and transports over the industrial era. Glob. Biogeochem. Cycles 28, 631–647. doi: 10.1002/2013gb004739
DeVries, T., Holzer, M., and Primeau, F. (2017). Recent increase in oceanic carbon uptake driven by weaker upper-ocean overturning. Nature 542:215. doi: 10.1038/nature21068
Dickens, W. A., Kuhn, G., Leng, M. J., Graham, A. G. C., Dowdeswell, J. A., Meredith, M. P., et al. (2019). Enhanced glacial discharge from the eastern Antarctic Peninsula since the 1700s associated with a positive Southern Annular Mode. Scientific Rep. 9, 1–11. doi: 10.1038/s41598-019-50897-4
Dierssen, H. M., Smith, R. C., and Vernet, M. (2002). Glacial meltwater dynamics in coastal waters West of the Antarctic Peninsula. Proc. Natl. Acad. Sci. U.S.A. 99, 1790–1795. doi: 10.1073/pnas.032206999
do Sul, J. A. I., Barnes, D. K. A., Costa, M. F., Convey, P., Costa, E. S., and Campos, L. S. (2011). Plastics in the Antarctic environment: are we looking only at the tip of the iceberg? Oecol. Austral. 15, 150–170. doi: 10.4257/oeco.2011.1501.11
Doddridge, E. W., and Marshall, J. (2017). Modulation of the seasonal cycle of Antarctic sea ice extent related to the Southern Annular Mode. Geophys. Res. Lett. 44, 9761–9768. doi: 10.1002/2017GL074319
Domack, E., Duran, D., Leventer, A., Ishman, S., Doane, S., and McCallum, S. (2005). Stability of the Larsen B ice shelf on the Antarctic Peninsula during the Holocene epoch. Nature 436, 481–685. doi: 10.1038/nature03908
Doney, S. C., Fabry, V. J., Feely, R. A., and Kleypas, J. A. (2009). Ocean acidification: the other CO2 problem. Annu. Rev. Mar. Sci. 1, 169–192.
Donohue, K. A., Tracey, K. L., Watts, D. R., Chidichimo, M. P., and Chereskin, T. K. (2016). Mean antarctic circumpolar current transport measured in drake passage. Geophys. Res. Lett. 43, 760–711. doi: 10.1002/2016GL070319
Downes, S., Spence, P., and Hogg, A. (2018). Understanding variability of the Southern Ocean overturning circulation in CORE-II models. Ocean Modelling 123, 98–109. doi: 10.1016/j.ocemod.2018.01.005
Downes, S. M., and Hogg, A. M. (2013). Southern Ocean circulation and eddy compensation in CMIP5 models. J. Clim. 26, 7198–7220. doi: 10.1175/JCLI-D-12-00504.1
Dunlop, R. A., Cato, D. H., and Noad, M. J. (2010). Your attention please: increasing ambient noise levels elicits a change in communication behaviour in humpback whales (Megaptera novaeangliae). Proc. Roy. Soc. B 277, 2521–2529. doi: 10.1098/rspb.2009.2319
Durack, P. J., Wijffels, S. E., and Matear, R. J. (2012). Ocean salinities reveal strong global water cycle intensification during 1950 to 2000. Science 336, 455–458. doi: 10.1126/science.1212222
Early, R., Bradley, B. A., Dukes, J. S., Lawler, J. J., Olden, J. D., and Blumenthal, D. M. (2016). Global threats from invasive alien species in the twenty-first century and national response capacities. Nat. Commun. 7: 12485.
Etourneau, J., Sgubin, G., Crosta, X., Swingedouw, D., Willmott, V., Barbara, L., et al. (2019). Ocean temperature impact on ice shelf extent in the eastern Antarctic Peninsula. Nat. Commun. 10:304. doi: 10.1038/s41467-018-08195-6
Feely, R. A., Doney, S. C., and Cooley, S. R. (2009). Ocean acidification: present conditions and future changes in a high-CO2 world. Oceanography 22, 36–47. doi: 10.5670/oceanog.2009.95
Feely, R. A., Sabine, C. L., Lee, K., Berelson, W., Kleypas, J., Fabry, V. J., et al. (2004). Impact of anthropogenic CO2 on the CaCO3 system in the oceans. Science 305, 362–371. doi: 10.1126/science.1097329
Feely, R. A., Satttebin, C. L., Lee, K., Millero, F. J., Lamb, M. F., Greeley, D., et al. (2002). In situ calcium carbonate dissolution in the Pacific Ocean. Glob. Biogeochem. Cycle 16:1144. doi: 10.1029/2002GB001866
Fletcher, S. E. M., Gruber, N., Jacobson, A. R., Doney, S. C., Dutkiewicz, S., Gerber, M., et al. (2006). Inverse estimates of anthropogenic CO2 uptake, transport, and storage by the ocean. Glob. Biogeochem. Cycle 20:GB2002. doi: 10.1029/2005GB002530
Flores, H., Atkinson, A., Kawaguchi, S., Krafft, B. A., Milinevsky, G., Nicol, S., et al. (2012). Impact of climate change on Antarctic krill. Mar. Ecol. Prog. Ser. 458, 1–19.
Fraser, C. I, Morrison, A. K., Hogg, A., Hogg, M. C. C., Macaya, E. C., van Sebille, E., et al. (2018). Antarctica’s ecological isolation will be broken by storm-driven dispersal and warming. Nat. Clim. Change 8, 704–708. doi: 10.1038/s41558-018-0209-7
Fry, M. D., Fefer, S. I., and Sileo, L. (1987). Ingestion of plastic debris by Laysan albatrosses and wedge-tailed shearwaters in the Hawaiian islands. Mar. Pollut. Bull. 18, 339–343. doi: 10.1016/s0025-326x(87)80022-x
Fu, W. W., Randerson, J. T., and Moore, J. K. (2016). Climate change impacts on net primary production (NPP) and export production (EP) regulated by increasing stratification and phytoplankton community structure in the CMIP5 models. Biogeosciences 13, 5151–5170. doi: 10.5194/bg-13-5151-2016
Fuentes, V., Alurralde, G., Meyer, B., Aguirre, G. E., Canepa, A., Wölfl, A. C., et al. (2016). Glacial melting: an overlooked threat to Antarctic krill. Sci. Rep. 6:27234.
Gardner, J., Manno, C., Bakker, D. C. E., Peck, V. L., and Tarling, G. A. (2018). Southern Ocean pteropods at risk from ocean warming and acidification. Mar. Biol. 165:8.
Gent, P. R. (2016). Effects of Southern Hemisphere wind changes on the meridional overturning circulation in ocean models. Ann. Rev. Mar. Sci. 8, 79–94. doi: 10.1146/annurev-marine-122414-033929
Gerringa, L. J. A., Alderkamp, A. C., Laan, P., Thuroczy, C. E., De Baar, H. J. W., Mills, M. M., et al. (2012). Iron from melting glaciers fuels the phytoplankton blooms in Amundsen Sea (Southern Ocean): iron biogeochemistry. Deep Sea Res. II 71-76, 16–31. doi: 10.1016/j.dsr2.2012.03.007
Gibson, J. A., and Trull, T. W. (1999). Annual cycle of fCO2 under sea-ice and in open water in Prydz Bay, East Antarctica. Mar. Chem. 66, 187–200. doi: 10.1016/s0304-4203(99)00040-7
Giesecke, R., Höfer, J., Vallejos, T., and Gonzalez, H. E. (2019). Death in southern Patagonian fjords: copepod community structure and mortality in land- and marine-terminating glacier-fjord systems. Prog. Oceanogr. 174, 162–172. doi: 10.1016/j.pocean.2018.10.011
Gille, S. T. (2014). Meridional displacement of the antarctic circumpolar current. Philos. Trans. R. Soc. A 372:20130273. doi: 10.1098/rsta.2013.0273
Grieger, J., Leckebusch, G. C., Raible, C. C., Rudeva, I., and Simmond, I. (2018). Subantarctic cyclones identified by 14 tracking methods, and their role for moisture transports into the continent. Tellus A 70, 1–18. doi: 10.1080/16000870.2018.1454808
Gruber, N., Gloor, M., Fletcher, S. E. M., Doney, S. C., Dutkiewicz, S., Follows, M. J., et al. (2009). Oceanic sources, sinks, and transport of atmospheric CO2. Glob. Biogeochem. Cycle 23:GB1005.
Gruber, N., Landschützer, P., and Lovenduski, N. S. (2019). The variable southern ocean carbon sink. Ann. Rev. Mar. Sci. 11, 159–186. doi: 10.1146/annurev-marine-121916-063407
Guinotte, J. M., and Fabry, V. J. (2008). Ocean acidification and its potential effects on marine ecosystems. Ann. N. Y. Acad. Sci. 1134, 320–342. doi: 10.1196/annals.1439.013
Gutt, J., Barratt, I., Domack, E., d’Udekem d’Acoz, C., and Dimmler, W. (2011). Biodiversity change after climate-induced ice-shelf collapse in the Antarctic. Deep Sea Res. II 58, 74–83.
Gutt, J., Bertler, N., Bracegirdle, T. J., Buschmann, A., Comiso, J., Hosie, G., et al. (2015). The Southern Ocean ecosystem under multiple climate change stresses - an integrated circumpolar assessment. Glob. Change Biol. 21, 1434–1453. doi: 10.1111/gcb.12794
Gutt, J., and Piepenburg, D. (2003). Scale-dependent impact on diversity of Antarctic benthos caused by grounding of icebergs. Mar. Ecol. Prog. Ser. 253, 77–83. doi: 10.3354/meps253077
Hauck, J., Hoppema, M., Bellerby, R. G. J., Volker, C., and Wolf-Gladrow, D. (2010). Data-based estimation of anthropogenic carbon and acidification in the Weddell Sea on a decadal timescale. J. Geophys. Res. Oceans 115:C03004.
Hauck, J., and Völker, C. (2015). Rising atmospheric CO2 leads to large impact of biology on Southern Ocean CO2 uptake via changes of the Revelle factor. Geophys. Res. Letts. 42, 1459–1464. doi: 10.1002/2015gl063070
Hauck, J., Völker, C., Wolf-Gladrow, D. A., Laufkötter, C., Vogt, M., Aumont, O., et al. (2015). On the Southern Ocean CO2 uptake and the role of the biological carbon pump in the 21st century. Glob. Biogeochem. Cycle 29, 1451–1470. doi: 10.1002/2015gb005140
Haumann, F. A., Gruber, N., Münnich, M., Frenger, I., and Kern, S. (2016). Sea-ice transport driving Southern Ocean salinity and its recent trends. Nature 537, 89–92. doi: 10.1038/nature19101
Hauri, C., Friedrich, T., and Timmermann, A. (2016). Abrupt onset and prolongation of aragonite undersaturation events in the Southern Ocean. Nat. Clim. Chang. 6, 172–176. doi: 10.1038/nclimate2844
Headland, R. K. (1994). Historical development of Antarctic tourism. Ann. Tourism Res. 21, 269–280. doi: 10.1016/0160-7383(94)90044-2
Hellmer, H. H., Huhn, O., Gomis, D., and Timmermann, R. (2011). On the freshening of the northwestern Weddell Sea continental shelf. Ocean Sci. 7, 305–316. doi: 10.5194/os-7-305-2011
Hellmer, H. H., Kauker, F., Timmermann, R., and Hattermann, T. (2017). The fate of the southern Weddell Sea continental shelf in a warming climate. J. Climate 30, 4337–4350. doi: 10.1175/jcli-d-16-0420.1
Henley, S. F., Cavan, E. L., Fawcett, S. E., Kerr, R., Monteiro, T., Sherrell, R. M., et al. (2020). Changing biogeochemistry of the Southern Ocean and its ecosystem implications. Front. Mar. Sci. 7:581. doi: 10.3389/fmars.2020.00581
Henley, S. F., Tuerena, R. E., Annett, A. L., Fallick, A. E., Meredith, M. P., Venables, H. J., et al. (2017). Macronutrient supply, uptake and recycling in the coastal ocean of the west Antarctic Peninsula. Deep Sea Res. II 139, 58–76. doi: 10.1016/j.dsr2.2016.10.003
Henley, S. F., Jones, E. M., Venables, H. J., Meredith, M. P., Firing, Y. L., Dittrich, R., et al. (2018). Macronutrient and carbon supply, uptake and cycling across the Antarctic Peninsi shelf during summer. Philios. Trans. R. Soc. 376:20170168. doi: 10.1098/rsta.2017.0168
Henley, S. F., Schofield, O. M., Hendry, K. R., Schloss, I. R., Steinberg, D. K., Moffat, C., et al. (2019). Variability and change in the west Antarctic Peninsula marine system: research priorities and opportunities. Prog. Oceanogr. 173, 208–237.
Hill, C. D. (2019). Cruise Ship Travel in Travel Medicine, Fourth Edn. London, UK: Elsevier, 377–382.
Hobbs, W., Massom, R., Stammerjohn, S., Reid, P., Williams, G. D., and Meier, W. N. (2016). A Review of Recent Changes in Southern Ocean Sea Ice, their Drivers and Forcings. Glob. Planet. Change 143, 228–250. doi: 10.1016/j.gloplacha.2016.06.008
Hochmuth, K., and Gohl, K. (2019). Seaward growth of Antarctic continental shelves since establishment of a continent-wide ice sheet: patterns and mechanisms. Palaeogeography 520, 44–54. doi: 10.1016/j.palaeo.2019.01.025
Höfer, J., Giesecke, R., Hopwood, M. J., Carrera, V., Alaræon, E., and González, H. E. (2019). The role of water column stability and wind mixing in the production/export dynamics of two bays in the Western Antarctic Peninsula. Prog. Oceanogr. 174, 105–116. doi: 10.1016/j.pocean.2019.01.005
Hogg, A., Meredith, M. P., Chambers, D. P., Abrahamsen, E. P., Hughes, C. W., and Morrison, A. K. (2015). Recent trends in the Southern Ocean eddy field. J. Geophys. Res. Oceans 120, 257–267. doi: 10.1002/2014JC010470
Hogg, A. M., Spence, P., Saenko, O. A., and Downes, S. M. (2017). The energetics of Southern Ocean upwelling. J. Phys. Oceanogr. 47, 135–153. doi: 10.1175/jpo-d-16-0176.1
Holland, P. R., and Kwok, R. (2012). Wind-driven trends in Antarctic sea-ice drift. Nat. Geosci. 5, 872–875. doi: 10.1038/Ngeo1627
Hönisch, B., Ridgwell, A., Schmidt, D. N., Thomas, E., Gibbs, S. J., Sluijis, A., et al. (2012). The geological record of ocean acidification. Science 335:6072.
Hopwood, M. J., Carroll, D., Höfer, J., Achterberg, E. P., Meire, L., Frédéric, A. C., et al. (2019). Highly variable iron content modulates iceberg-ocean fertilisation and potential carbon export. Nat. Commun. 10:5261. doi: 10.1038/s41467-019-13231-0
Horton, A. A., and Barnes, D. K. A. (2020). Microplastic pollution in a rapidly changing world: implications for remote and vulnerable marine ecosystems. Sci. Total Environ. 738:140249.
Houghton, J. T., Ding, Y., Griggs, D. J., Noguer, N., Van der Linden, P. J., Xiaosu, D., et al. (2001). Climate Change 2001: The Scientific Basis. Cambridge, MA: Cambridge University Press.
Hucke-Gaete, R., Osman, L. P., Moreno, C. A., and Torres, D. (2004). Examining natural population growth from near extinction: the case of the Antarctic fur seal at the South Shetlands, Antarctica. Polar Biol. 27, 304–311. doi: 10.1007/s00300-003-0587-8
Hughes, K. A., and Ashton, G. V. (2017). Breaking the ice: the introduction of biofouling organisms to Antarctica on vessel hulls. Aquat. Conserv. 27, 158–164. doi: 10.1002/aqc.2625
Hughes, K. A., Convey, P., Vega, G. C., Aragón, P., and Olalla-Tárraga, M. Á, and Pertierra, L. R. (2019). Human-mediated dispersal of terrestrial species between Antarctic biogeographic regions: a preliminary risk assessment. J. Environ. Manage. 232, 73–89. doi: 10.1016/j.jenvman.2018.10.095
Hutchins, D. A., and Boyd, P. W. (2016). Marine phytoplankton and the changing ocean iron cycle. Nat. Clim. Change 6, 1072–1079. doi: 10.1038/nclimate3147
International Maritime Organisation Marine Environment Protection Committee [IMO] (2007). Guidelines for Ballast Water Exchange in The Antarctic Treaty Area. Annex 4, Resolution MEPC.163(56). Available at: http://www.imo.org/en/KnowledgeCentre/IndexofIMOResolutions/Marine-Environment-Protection-Committee-(MEPC)/Documents/MEPC.163(56).pdf (accessed February 5, 2020).
International Maritime Organisation [IMO] (2004). International Convention for the Control and Management of Ships’ Ballast Water and Sediments. BWM/CONF/36. Available at: http://library.arcticportal.org/1913/ International Convention for the Control and Management of Ships’ Ballast Water and Sediments.pdf (accessed February 4, 2020).
IWC (2010). Report of the Workshop on Welfare Issues Associated with the Entanglement of Large Whales. Australia: IWC.
Jacobs, S. S., and Giulivi, C. F. (2010). Large multidecadal salinity trends near the Pacific–Antarctic continental margin. J. Clim. 23, 4508–4524. doi: 10.1175/2010jcli3284.1
Jacobs, S. S., Giulivi, C. F., and Mele, P. A. (2002). Freshening of the Ross Sea during the late 20th century. Science 297, 386–389. doi: 10.1126/science.1069574
Jones, E. M., Fenton, M., Meredith, M. P., Clargo, N. M., Ossebaar, S., Ducklow, H. W., et al. (2017). Ocean acidification and calcium carbonate saturation states in the coastal zone of the West Antarctic Peninsula. Deep Sea Res. II 139, 181–194. doi: 10.1016/j.dsr2.2017.01.007
Jones, J. M., Gille, S. T., Goosse, H., Abram, N. J., Canziani, P. O., Charman, D. J., et al. (2016). Assessing recent trends in high-latitude Southern Hemisphere surface climate. Nat. Clim. Change 6, 917–926. doi: 10.1038/nclimate3103
Jourdain, N. C., Mathiot, P., Merino, N., Durand, G., Le Sommer, J., Spence, P., et al. (2017). Ocean circulation and sea-ice thinning induced by melting ice shelves in the Amundsen Sea. J. Geophys. Res. 122, 2550–2573. doi: 10.1002/2016JC012509
Jouventin, P., and Weimerskirch, H. (1991). “Changes in the population size and demography of southern seabirds: management implications,” in Bird Population Studies: Their Relevance to Conservation and Management, eds C. M. Perrins, J. D. Lebreton, and G. M. Hirons, (Oxford: Oxford University Press), 297–316.
Kapsenberg, L., Kelley, A. L., Shaw, E. C., Martz, T. R., and Hofmann, G. E. (2015). Near-shore Antarctic pH variability has implications for the design of ocean acidification experiments. Sci. Rep. 5:9638.
Kawaguchi, S., Ishida, A., King, R., Raymond, B., Waller, N., Constable, A., et al. (2013). Risk maps for Antarctic krill under projected Southern Ocean acidification. Nat. Clim. Change 3, 843–847. doi: 10.1038/nclimate1937
Key, G. (2018). Tackling Invasive Non-Native Species in the UK Overseas Territories - Pathway Analyses. Unpublished Report to the UK Non-Native Species Secretariat. Available at: http://www.nonnativespecies.org/downloadDocument.cfm?id=1648 (accessed February 4, 2020).
Krasnobaev, A., ten Dam, G., Boerrigter-Eenling, R., van Leeuwen, S. P. J., Morley, S. A., Peck, L. S., et al. (2020). Seasonal variability in concentrations of persistent organic pollutants in phytoplankton near Rothera Point, Western Antarctic Peninsula. Environ. Sci. Toxicol. 54, 2763–2771. doi: 10.1021/acs.est.9b06622
Laist, D. W. (1997). “Impacts of marine debris: entanglement of marine life in marine debris including a comprehensive list of species with entanglement and ingestion records,” in Marine Debris: Sources, Impacts, and Solutions, eds J. M. Coe and D. B. Rogers, (New York: Springer-Verlag), 99–140. doi: 10.1007/978-1-4613-8486-1_10
Lannuzel, D., Vancoppenolle, M., Van Der Merwe, P., De Jong, J., Meiners, K. M., Grotti, M., et al. (2016). Iron in sea ice: review and new insights. Elem. Sci. Anth. 4:000130.
Lavery, T. J., Roudnew, B., Gill, P., Seymour, J., Seuront, L., Johnson, G., et al. (2010). Iron defecation by sperm whales stimulates carbon export in the Southern Ocean. Proc. R. Soc. B 277, 3527–3531. doi: 10.1098/rspb.2010.0863
Le Quere, C., Rodenbeck, C., Buitenhuis, E. T., Conway, T. J., Langenfelds, R., Gomez, A., et al. (2007). Saturation of the Southern Ocean CO2 sink due to recent climate change. Science 316, 1735–1738. doi: 10.1126/science.1136188
Leaper, R., Cooke, J., Trathan, P., Reid, K., Rowntree, V., and Payne, R. (2006). Global climate drives southern right whale (Eubalaena australis) population dynamics. Biol. Lett. 2, 289–292. doi: 10.1098/rsbl.2005.0431
Lee, J. E., and Chown, S. L. (2007). Mytilus on the move: transport of an invasive bivalve to the Antarctic. Mar. Ecol. Prog. Ser. 339, 307–310. doi: 10.3354/meps339307
Lencina-Avila, J. M., Goyet, C., Kerr, R., Orselli, I. B. M., Mata, M. M., and Touratier, F. (2018). Past and future evolution of the marine carbonate system in a coastal zone of the Northern Antarctic Peninsula. Deep Sea Res. II 149, 193–205. doi: 10.1016/j.dsr2.2017.10.018
Lenton, A., Tilbrook, B., Law, R. M., Bakker, D., Doney, S. C., Gruber, N., et al. (2013). Sea-air CO2 fluxes in the Southern Ocean for the period 1990-2009. Biogeosciences 10, 4037–4054.
Leung, S., Cabre, A., and Marinov, I. (2015). A latitudinally banded phytoplankton response to 21st century climate change in the Southern Ocean across the CMIP5 model suite. Biogeosciences 12, 5715–5734. doi: 10.5194/bg-12-5715-2015
Levermann, A., Winkelmann, R., Albrecht, T., Goelzer, H., Golledge, N. R., Greve, R., et al. (2020). Projecting Antarctica’s contribution to future sea level rise from basal ice shelf melt using linear response functions of 16 ice sheet models (LARMIP-2). Earth Syst. Dyn. 11, 35–76. doi: 10.5194/esd-11-35-2020
Lewis, P. N., Hewitt, C. L., Riddle, M., and McMinn, A. (2003). Marine introductions in the Southern Ocean: an unrecognised hazard to biodiversity. Mar. Pollut. Bull. 46, 213–223. doi: 10.1016/s0025-326x(02)00364-8
Lewis, P. N., Riddle, M., and Hewitt, C. L. (2004). Management of exogenous threats to Antarctica and the sub-Antarctic Islands: balancing risks from TBT and non-indigenous marine organisms. Mar. Pollut. Bull. 49, 999–1005. doi: 10.1016/j.marpolbul.2004.07.001
Lewison, R. L., Crowder, L. B., Read, A. J., and Freeman, S. A. (2004). Understanding impacts of fisheries bycatch on marine megafauna. Trends Ecol. Evol. 19, 598–604. doi: 10.1016/j.tree.2004.09.004
L’Heureux, M. L., Thompson, D. W. J., L’Heureux, M. L., and Thompson, D. W. J. (2006). Observed relationships between the El Niño–Southern oscillation and the extratropical zonal-mean circulation. J. Clim. 19, 276–287. doi: 10.1175/jcli3617.1
Lim, E.-P., Hendon, H. H., Hope, P., Chung, C., Delage, F., and McPhaden, M. J. (2019). Continuation of tropical Pacific Ocean temperature trend may weaken extreme El Niño and its linkage to the Southern Annular Mode. Sci. Rep. UK 9:17044. doi: 10.1038/s41598-019-53371-3
Lin, H., Rauschenberg, S., Hexel, C. R., Shaw, T. J., and Twining, B. S. (2011). Free-drifting icebergs as sources of iron to the Weddell Sea. Deep Sea Res. II 58, 1392–1406. doi: 10.1016/j.dsr2.2010.11.020
Loscher, B. M., De Baar, H. J. W., De Jong, J. T. M., Veth, C., and Dehairs, F. (1997). The distribution of Fe in the antarctic circumpolar current. Deep Sea Res. II 44, 143–187.
Maas, E. W., Law, C. S., Hall, J. A., Pickmere, S., Currie, K. I., Chang, F. H., et al. (2013). Effect of ocean acidification on bacterial abundance, activity and diversity in the Ross Sea. Antarctica. Aquat. Microb. Ecol. 70, 1–15. doi: 10.3354/ame01633
Marinov, I., Gnanadesikan, A., Toggweiler, J. R., and Sarmiento, J. L. (2006). The Southern Ocean biogeochemical divide. Nature 441, 964–967. doi: 10.1038/nature04883
Marshall, G. J. (2003). Trends in the Southern Annular Mode from observations and reanalyses. J. Clim. 16, 4134–4143. doi: 10.1175/1520-0442(2003)016<4134:titsam>2.0.co;2
Marshall, G. J. (2007). Half-century seasonal relationships between the Southern Annular Mode and Antarctic temperatures. Int. J. Climatol. 27, 373–383. doi: 10.1002/joc.1407
Marshall, G. J., and Thompson, D. W. J. (2016). The signatures of large-scale patterns of atmospheric variability in Antarctic surface temperatures. J. Geophys. Res. Atmos. 121, 3276–3289. doi: 10.1002/2015JD024665
Marshall, G. J., Thompson, D. W. J., and van den Broeke, M. R. (2017). The signature of Southern Hemisphere atmospheric circulation patterns in Antarctic precipitation. Geophys. Res. Lett. 44, 580–589. doi: 10.1002/2017GL075998
Martinson, D. G., and McKee, D. C. (2012). Transport of warm Upper Circumpolar Deep Water onto the western Antarctic Peninsula continental shelf. Ocean Sci. 8, 433–442. doi: 10.5194/os-8-433-2012
Martinson, D. G., Stammerjohn, S. E., Iannuzzi, R. A., Smith, R. C., and Vernet, M. (2008). Western Antarctic Peninsula physical oceanography and spatio–temporal variability. Deep Sea Res. II 55, 1964–1987. doi: 10.1016/j.dsr2.2008.04.038
Massom, R. A., Scambos, T. A., Bennetts, L. G., Reis, P., Squire, V. A., and Stammerjohn, S. E. (2018). Antarctic ice shelf disintegration triggered by sea ice loss and ocean swall. Nature 558, 383–389. doi: 10.1038/s41586-018-0212-1
Mastrandrea, M. D., Mach, K. J., Plattner, G.-K., Edenhofer, O., Stocker, T. F., Field, C. B., et al. (2011). The IPCC AR5 guidance note on consistent treatment of uncertainties: a common approach across the working groups. Clim. Change 108, 675–691. doi: 10.1007/s10584-011-0178-6
McCarthy, A., Peck, L. S., Hughes, K. A., and Aldridge, D. (2019). Antarctica: the final frontier for marine biological invasions. Glob. Change Biol. 25, 2221–2241.
McConell, J. R., Maselli, O. J., Sigl, M., Vallelonga, P., Neumann, T., Anschütz, H., et al. (2014). Antarctic-wide array of high-resolution ice core records reveals pervasive lead pollution began in 1889 and persists today. Sci. Rep. 4:5848.
McKinley, G. A., Fay, A. R., Lovenduski, N. S., and Pilcher, D. J. (2017). Natural variability and anthropogenic trends in the ocean carbon sink. Ann. Rev. Mar. Sci. 9, 125–150. doi: 10.1146/annurev-marine-010816-060529
McNeil, B., Tagliabue, A., and Sweeney, C. (2010). A multi-decadal delay in the onset of corrosive ‘acidified’ waters in the Ross Sea of Antarctica due to strong air-sea CO2 disequilibrium. Geophys. Res. Lett. 37:L19607.
McNeil, B. I., and Matear, R. (2008). Southern Ocean acidification: a tipping point at 450 pp, atmospheric CO2. Proc. Nat. Acad. Sci. U.S.A. 105, 18860–18864. doi: 10.1073/pnas.0806318105
Meehl, G. A., Arblaster, J. M., Chung, C. T. Y., Holland, M. M., DuVivier, A., Thompson, L., et al. (2019). Sustained ocean changes contributed to sudden Antarctic sea ice retreat in late 2016. Nat. Commun. 10:14. doi: 10.1038/s41467-018-07865-9
Meijers, A. J. S., Meredith, M. P., Murphy, E. J., Chambers, D. P., Belchier, M., Young, E. F., et al. (2019). The role of ocean dynamics in king penguin range estimation. Nat. Clim. Change 9, 120–121. doi: 10.1038/s41558-018-0388-2
Menezes, V. V., Macdonald, A. M., and Schatzman, C. (2017). Accelerated freshening of Antarctic Bottom Water over the last decade in the Southern Indian Ocean. Sci. Adv. 3:e1601426. doi: 10.1126/sciadv.1601426
Meredith, M. P., Falk, U., Bers, A. V., Mackensen, A., Schloss, I. R., Ruiz Barlett, E., et al. (2018). Anatomy of a glacial meltwater discharge event in an Antarctic cove. Philos. Trans. Soc. A 376:20170163. doi: 10.1098/rsta.2017.0163
Meredith, M. P., and King, J. C. (2005). Rapid climate change in the ocean west of the Antarctic Peninsula during the second half of the 20th century. Geophys. Res. Lett. 32:L19604. doi: 10.1029/2005GL024042
Meredith, M. P., Sommerkorn, M., Cassotta, S., Derksen, C., Ekaykin, A., Hollowed, A., et al. (2019). “Polar Regions,” in Intergovernmental Panel on Climate Change (IPCC) Special Report on the Ocean and Cryosphere in a Changing Climate, eds R. Hock, J.-P. Gattuso, and N. Abram, (Geneva: IPCC).
Midorikawa, T., Inoue, H. Y., Ishii, M., Sasano, D., Kosugi, N., Hashida, G., et al. (2012). Decreasing pH trend estimated from 35-year time series of carbonate parameters in the Pacific sector of the Southern Ocean in summer. Deep Sea Res. I 61, 131–139. doi: 10.1016/j.dsr.2011.12.003
Miles, B. W. J., Stokes, C. R., and Jamieson, S. S. R. (2018). Velocity increases at Cook Glacier, East Antarctica, linked to ice shelf loss and a subglacial flood event. Cryosphere 12, 3123–3136. doi: 10.5194/tc-.12-3123-2018
Moffat, C., and Meredith, M. P. (2018). Shelf–ocean exchange and hydrography west of the Antarctic Peninsula: a review. Philos. Trans. R. Soc. A 376:20170164. doi: 10.1098/rsta.2017.0164
Moffat, C., Owens, B., and Beardsley, R. C. (2009). On the characteristics of circumpolar deep water intrusions to the west antarctic peninsula continental shelf. J. Geophys. Res. 114:C05017. doi: 10.1029/2008JC004955
Morley, S. A., Barnes, D. K. A., and Dunn, M. J. (2019). Predicting which species succeed in climate-forced polar seas. Front. Mar. Sci. 5:507. doi: 10.3389/fmars.2018.00507
Moore, C. M., Mills, M. M., Arrigo, K. R., Berman-Frank, I., Bopp, L., Boyd, P. W., et al. (2013). Processes and patterns of oceanic nutrient limitation. Nat. Geoscience 6, 701–710.
Moore, J. K., Abbott, M. R., and Richman, J. G. (1999). Location and dynamics of the Antarctic Polar Front from satellite sea surface temperature data. J. Geophys. Res. 104, 3059–3073. doi: 10.1029/1998jc900032
Moore, J. K., Fu, W. W., Primeau, F., Britten, G. L., Lindsay, K., Long, M., et al. (2018). Sustained climate warming drives declining marine biological productivity. Science 359, 1139–1142. doi: 10.1126/science.aao6379
Moreau, S., Mostajir, B., Belanger, S., Schloss, I. R., Vancoppenolle, M., Demers, S., et al. (2015). Climate change enhances primary production in the western Antarctic Peninsula. Glob. Change Biol. 21, 2191–2205. doi: 10.1111/gcb.12878
Munday, D. R., Johnson, H. L., and Marshall, D. P. (2013). Eddy saturation of equilibrated circumpolar currents. J. Phys. Oceanogr. 43, 507–532. doi: 10.1175/JPO-D-12-095.1
Munro, D. R., Lovenduski, N. S., Takahashi, T., Stephens, B. B., Newberger, T., and Sweeney, C. (2015). Recent evidence for a strengthening CO2 sink in the Southern Ocean from carbonate system measurements in the Drake Passage (2002-2015). Geophys. Res. Lett. 42, 7623–7630. doi: 10.1002/2015gl065194
Murphy, E. J., Cavanagh, R. D., Drinkwater, K. F., Grant, S. M., Heymans, J. J., Hofmann, E. E., et al. (2016). Understanding the structure and functioning of polar pelagic ecosystems to predict the impacts of change. Proc. R. Soc. B 283:20161646. doi: 10.1098/rspb.2016.1646
Nakayama, Y., Menemenlis, D., Zhang, H., Schodlok, M., and Rignot, E. (2018). Origin of circumpolar deep water intruding onto the amundsen and Bellingshausen Sea continental shelves. Nat. Commun. 9:3403. doi: 10.1038/s41467-018-05813-1
Naughten, K. A., Meissner, K. J., Galton-Fenzi, B. K., England, M. H., Timmermann, R., and Helmer, H. H. (2018). Future projections of Antarctic ice shelf melting based on CMIP5 scenarios. J. Clim. 31, 5243–5261. doi: 10.1175/JCLI-D-17-0854.1
Newman, L., Heil, P., Trebilco, R., Katsumata, K., Constable, A., van Wijk, E., et al. (2019). Delivering sustained, coordinated, and integrated observations of the Southern Ocean for Global Impact. Front. Mar. Sci. 6:433. doi: 10.3389/fmars.2019.00433
Newson, S. E., Mendes, S., Crick, H. Q., Dulvy, N. K., Houghton, J. D., Hays, G. C., et al. (2009). Indicators of the impact of climate change on migratory species. Endanger. Species Res. 7, 101–113. doi: 10.3354/esr00162
Ojaveer, H., Galil, B., Minchin, D., Olenin, S., Amorim, A., Canning-Clode, J., et al. (2014). Ten recommendations for advancing the assessment and management of non-indigenous species in marine ecosystems. Mar. Pollut. Bull. 44, 160–165. doi: 10.1016/j.marpol.2013.08.019
Orr, J. C. (2011). “Recent and future changes in ocean carbonate chemistry,” in Ocean Acidification, eds J.-P. Gattuso and L. Hansson, (Oxford: Oxford University Press), 41–66.
Orr, J. C., Fabry, V. J., Aumont, O., Bopp, L., Doney, S. C., and Feely, R. A. (2005). Anthropogenic ocean acidification over the twenty-first century and its impact on calcifying organisms. Nature 437, 681–686. doi: 10.1038/nature04095
Orr, J. C., Maier-Reimer, E., Mikolajewicz, U., Monfray, P., Sarmiento, J. L., Toggweiler, J. R., et al. (2001). Estimates of anthropogenic carbon uptake from four three-dimensional global ocean models. Glob. Biogeochem. Cycle 15, 43–60. doi: 10.1029/2000gb001273
Pakhomov, E. A., Fuentes, V., Schloss, I. R., Atencio, A., and Esnal, G. B. (2003). Beaching of the tunicate Salpa thompsoni at high levels of suspended particulate matter in the Southern Ocean. Polar Biol. 26, 427–431. doi: 10.1007/s00300-003-0494-z
Paolo, F. S., Fricker, H. A., and Padman, L. (2015). Volume loss from Antarctic ice shelves is accelerating. Science 348, 327–331. doi: 10.1126/science.aaa0940
Parkinson, C. L. (2019). A 40-y record reveals gradual Antarctic sea ice increases followed by decreases at rates far exceeding the rates seen in the Arctic. Proc. Nat. Acad. Sci. U.S.A. 116, 14414–14423. doi: 10.1073/pnas.1906556116
Parkinson, C. L., and Cavalieri, D. J. (2012). Antarctic sea ice variability and trends, 1979-2010. Cryosphere Discuss 6, 931–956. doi: 10.5194/tcd-6-931-2012
Parks, S. E., Clark, C. W., and Tyack, P. L. (2007). Short-and long-term changes in right whale calling behavior: the potential effects of noise on acoustic communication. J. Acoust. Soc. Am. 122, 3725–3731. doi: 10.1121/1.2799904
Pauly, D. (2009). Beyond duplicity and ignorance in global fisheries. Sci. Mar. 73, 215–224. doi: 10.3989/scimar.2009.73n2215
Peck, L. S., Barnes, D. K. A., Cook, A. J., Fleming, A. H., and Clarke, A. (2010). Negative feedback in the cold: ice retreat produces new carbon sinks in Antarctica. Glob. Change Biol. 16, 2614–2623. doi: 10.1111/j.1365-2486.2009.02071.x
Peel, D., Smith, J. N., and Childerhouse, S. (2018). Vessel strike of whales in Australia: the challenges of analysis of historical incident data. Front. Mar. Sci. 5:69. doi: 10.3389/fmars.2018.00069
Perlwitz, J., Pawson, S., Fogt, R. L., Nielsen, J. E., and Neff, W. D. (2008). Impact of stratospheric ozone hole recovery on Antarctic climate. Geophys. Res. Lett. 35:L08714. doi: 10.1029/2008GL033317
Pertierra, L. R., Hughes, K. A., Vega, G. C., and Olalla-Tárraga, M. A. (2017). High resolution spatial mapping of human footprint across Antarctica and its implications for the strategic conservation of avifauna. PLoS One 12:e0168280. doi: 10.1371/journal.pone.0168280
Phillips, R. A., Gales, R., Baker, G. B., Double, M. C., Favero, M., Quintana, F., et al. (2016). The conservation status and priorities for albatrosses and large petrels. Biol. Conserv. 201, 169–183. doi: 10.1016/j.biocon.2016.06.017
Pirotta, V., Grech, A., Jonsen, I. D., Laurance, W. F., and Harcourt, R. (2019). Marine roads: consequences of global shipping traffic for marine giants. Front. Ecol. Environ. 17, 39–47. doi: 10.1002/fee.1987
Pivot, S., Baroni, M., Bard, E., Giraud, X., and ASTER Team, (2019). A comparison of 36 Cl nuclear bomb inputs deposited in snow from Vostok and Talos Dome, Antarctica, using the 36 Cl/Cl - ratio. J. Geophys. Res. 124, 10973–10988. doi: 10.1029/2018JD030200
Planquette, H., Sherrell, R. M., Stammerjohn, S., and Field, M. P. (2013). Particulate iron delivery to the water column of the Amundsen Sea, Antarctica. Mar. Chem. 153, 15–30. doi: 10.1016/j.marchem.2013.04.006
Polvani, L. M., Waugh, D. W., Correa, G. J., and Son, S. (2011). Stratospheric ozone depletion: the main driver of twentieth-century atmospheric circulation changes in the Southern Hemisphere. J. Climate 24, 795–812. doi: 10.1175/2010JCLI3772.1
Post, A. L., O’Brien, P. E., Edwards, S., Carroll, A. G., Malakoff, K., and Armand, L. K. (2020). Upper slope processes and seafloor ecosytems on the Sabrina continental slope, East Antarctica. Mar. Geol. 422:106091. doi: 10.1016/j.margeo.2019.106091
Purkey, S. G., and Johnson, G. C. (2013). Antarctic Bottom Water warming and freshening: contributions to sea level rise, ocean freshwater budgets, and global heat gain. J. Clim. 26, 6105–6122. doi: 10.1175/JCLI-D-12-00834.1
Rack, W., and Rott, H. (2004). Pattern of retreat and disintegration of the Larsen B ice shelf, Antarctic Peninsula. Ann. Glaciol. 39, 505–510. doi: 10.3189/172756404781814005
Raes, M., Rose, A., and Vanreusel, A. (2010). Response of nematode communities after large-scale ice-shelf collapse events in the Antarctic Larsen area. Glob. Change Biol. 16, 1618–1631. doi: 10.1111/j.1365-2486.2009.02137.x
Randall-Goodwin, E., Meredith, M. P., Jenkins, A., Yager, P. L., Sherrell, R. M., Abrahamsen, E. P., et al. (2015). Freshwater distributions and water mass structure in the Amundsen Sea Polynya region, Antarctica. Elem. Sci. Anth. 3:000065.
Read, A. J. (2008). The looming crisis: interactions between marine mammals and fisheries. J. Mammal. 89, 541–548. doi: 10.1644/07-mamm-s-315r1.1
Read, A. J., Drinker, P., and Northridge, S. (2006). Bycatch of marine mammals in US and global fisheries. Conserv. Biol. 20, 163–169. doi: 10.1111/j.1523-1739.2006.00338.x
Reeves, R. R., McClellan, K., and Werner, T. B. (2013). Marine mammal bycatch in gillnet and other entangling net fisheries, 1990 to 2011. Endanger. Species Res. 20, 71–97. doi: 10.3354/esr00481
Reid, P., and Massom, R. A. (2015). “Successive Antarctic sea ice extent records during 2012, 2013, and 2014,” in State of the Climate 2014. Bull. Am. Meteor. Soc. 96, S163–S164.
Reid, P., Stammerjohn, S., Massom, R., Scambos, T., and Lieser, J. (2015). The record 2013 Southern Hemisphere sea-ice extent maximum. Ann. Glaciol. 56, 99–106. doi: 10.3189/2015AoG69A892
Rintoul, S. R. (2018). The global influence of localized dynamics in the Southern Ocean. Nature 558, 209–218. doi: 10.1038/s41586-018-0182-3
Rochman, C. M., Cook, A.-M., and Koelmans, A. A. (2016). Plastic debris and policy: using current scientific understanding to invoke positive change. Environ. Toxicol. Chem. 35, 1617–1626. doi: 10.1002/etc.3408
Rogers, A. D., Frinault, B. A. V., Barnes, D. K. A., Bindoff, N. L., Downie, R., Ducklow, H. W., et al. (2020). Antarctic futures: an assessment of climate-driven changes in ecosystem structure, function, and service provisioning in the Southern Ocean. Annu. Rev. Mar. Sci. 12, 87–120. doi: 10.1146/annurev-marine-010419-011028
Rosenbaum, H. C., Maxwell, S. M., Kershaw, F., and Mate, B. (2014). Long-range movement of humpback whales and their overlap with anthropogenic activity in the South Atlantic Ocean. Conserv. Biol. 28, 604–615. doi: 10.1111/cobi.12225
Roy, H. E., Peyton, J. M., Pescott, O. L., and Rorke, S. L. (2019). Prioritising Invasive Non-Native Species Through Horizon Scanning on the UK Overseas Territories. Lancaster: UK Centre for Ecology & Hydrology.
Ruiz, G. M., and Hewitt, C. L. (2009). “Latitudinal patterns of biological invasions in marine ecosystems: a polar perspective,” in Smithsonian at the Poles: Contributions to International Polar Year Science, eds I. Krupnik, M. A. Lang, and S. E. Miller, (Washington, DC: Smithsonian Institution Scholarly Press), 347–358. doi: 10.5479/si.097884601x.26
Rye, C. D., Marshall, J., Kelley, M., Russell, G., Nazarenko, L. S., Kostov, Y., et al. (2020). Antarctic glacial melt as a driver of recent Southern Ocean climate trends. Geophys. Res. Lett. 47:e2019GL086892. doi: 10.1029/2019GL086892
Sabine, C. L., Feely, R. A., Gruber, N., Key, R. M., Lee, K., Bullister, J. L., et al. (2004). The oceanic sink for anthropogenic CO2. Science 305, 367–371. doi: 10.1126/science.1097403
Sadai, S., Condron, A., DeConto, R., and Pollard, D. (2020). Future climate response to Antarctic Ice Sheet melt caused by anthropogenic warming. Sci. Adv. 6:eaaz1169. doi: 10.1126/sciadv.aaz1169
Sahade, R., Lagger, C., Torre, L., Momo, F., Monien, P., Schloss, I., et al. (2015). Climate change and glacier retreat drive shifts in an Antarctic benthic ecosystem. Sci. Adv. 1:e1500050. doi: 10.1126/sciadv.1500050
Sailley, S. F., Ducklow, H. W., Moeller, H. V., Fraser, W. R., Schofield, O. M., Steinberg, D. K., et al. (2013). Carbon fluxes and pelagic ecosystem dynamics near two western Antarctic Peninsula Adelie penguin colonies: an inverse model approach. Mar. Ecol. Prog. Ser. 492, 253–272. doi: 10.3354/meps10534
Sallée, J. B., Matear, R. J., Rintoul, S. R., and Lenton, A. (2012). Localized subduction of anthropogenic carbon dioxide in the Southern Hemisphere oceans. Nat. Geosci. 5, 579–584. doi: 10.1038/ngeo1523
Sallée, J. B., Shuckburgh, E., Bruneau, N., Meijers, A. J. S., Bracegirdle, T. J., Wang, Z., et al. (2013). Assessment of Southern Ocean water mass circulation and characteristics in CMIP5 models: historical bias and forcing response. J. Geophys. Res. Oceans 118, 1830–1844. doi: 10.1002/jgrc.20135
Sands, C. J., O’Hara, T. D., Barnes, D. K. A., and Martín-Ledo, R. (2015). Against the flow: evidence of multiple recent invasions of warmer continental shelf waters by a Southern Ocean brittle star. Front. Ecol. Evol. 3:63. doi: 10.3389/fevo.2015.00063
Sarmiento, J. L., and Le Quere, C. (1996). Oceanic carbon dioxide uptake in a model of century-scale global warming. Science 274, 1346–1350. doi: 10.1126/science.274.5291.1346
Schloss, I. R., Abele, D., Moreau, S., Demers, S., Bers, A. V., González, O., et al. (2012). Response of Potter Cove phytoplankton dynamics to 19 year (1991-2009) climate trends. J. Mar. Syst. 92, 53–66. doi: 10.1016/j.jmarsys.2011.10.006
Schlosser, E., Haumann, F. A., and Raphael, M. N. (2018). Atmospheric influences on the anomalous (2016). Antarctic sea ice decay. Cryosphere 12, 1103–1119. doi: 10.5194/tc-12-1103-2018
Schmidtko, S., Heywood, K. J., Thompson, A. F., and Aoki, S. (2014). Multidecadal warming of Antarctic waters. Science 346, 1227–1231. doi: 10.1126/science.1256117
Seroussi, H., Nowicki, S., Payne, A. J., Goelzer, H., Lipscomb, W. H., Abe-Ouchi, A., et al. (2020). ISMIP6 Antarctica: a multi-model ensemble of the Antarctic ice sheet evolution over the 21st century. Cryosphere 14, 3033–3070. doi: 10.5194/tc-14-3033-2020
Sewell, M. A., and Hofmann, G. E. (2011). Antarctic echinoids and climate change: a major impact on the brooding forms. Glob. Chang. Biol. 17, 734–744. doi: 10.1111/j.1365-2486.2010.02288.x
Sherrell, R. M., Lagerstrom, M. E., Forsch, K. O., Stammerjohn, S. E., and Yager, P. L. (2015). Dynamics of dissolved iron and other bioactive trace metals (Mn, Ni, Cu, Zn) in the Amundsen Sea Polynya, Antarctica. Elem. Sci. Anth. 3, 1–27.
Shevenell, A. E., Ingalls, A. E., Domack, E. W., and Kelly, C. (2011). Holocene Southern Ocean surface temperature variability west of the Antarctic Peninsula. Nature 470, 250–254. doi: 10.1038/nature09751
Silvano, A., Rintoul, S. R., Peña-Mollino, B., Hobbs, W. R., van Wijk, E., Aoki, S., et al. (2018). Freshening by glacial meltwater enhances melting of ice shelves and reduces formation of Antarctic Bottom Water. Sci. Adv. 4:eaa9467. doi: 10.1126/sciadv.aap9467
Simmonds, M. P., and Isaac, S. J. (2007). The impacts of climate change on marine mammals: early signs of significant problems. Oryx 41, 19–26. doi: 10.1017/s0030605307001524
Skliris, N., Marsh, R., Josey, S. A., Good, S. A., Liu, C., and Allan, R. P. (2014). Salinity changes in the World Ocean since 1950 in relation to changing surface freshwater fluxes. Clim. Dynam. 43, 709–736. doi: 10.1007/s00382-014-2131-7
Sokolov, S., and Rintoul, S. R. (2009). Circumpolar structure and distribution of the Antarctic circumpolar current fronts: 1. Mean circumpolar paths. J. Geophys. Res. 114:11018. doi: 10.1029/2008JC005108
Stammerjohn, S., Massom, R., Rind, D., and Martinson, D. (2012). Regions of rapid sea ice change: an inter-hemispheric seasonal comparison. Geophys. Res. Lett. 39:L06501. doi: 10.1029/2012gl050874
Stammerjohn, S. E., Martinson, D. G., Smith, R. C., Yuan, X., and Rind, D. (2008). Trends in Antarctic annual sea ice retreat and advance and their relation to ENSO and Southern Annular Mode Variability. J. Geophys. Res. 113:JC004269 doi: 10.1029/2007JC004269
Stewart, A. L., and Thompson, A. F. (2015). Eddy-mediated transport of warm circumpolar deep water across the antarctic shelf break. Geophys. Res. Lett. 42, 432–440. doi: 10.1002/2014GL062281
Stuecker, M. F., Bitz, C. M., and Armour, K. C. (2017). Conditions leading to the unprecedented low Antarctic sea ice extent during the 2016 austral spring season. Geophys. Res. Lett. 44, 9008–9019. doi: 10.1002/2017GL074691
Swart, N. C., Fyfe, J. C., Gillett, N., and Marshall, G. J. (2015). Comparing trends in the Southern Annular Mode and surface westerly jet. J. Clim. 28, 8840–8859. doi: 10.1175/JCLI-D-15-0334.1
Sweeney, C. (2004). “The annual cycle of surface CO2 and O2 in the Ross Sea: a model for gas exchange on the continental shelves of Antarctica,” in Biogeochemistry of the Ross Sea Antarc. Res. Ser, Vol. 78, eds G. R. DiTullio and R. B. Dunbar, (Washington, DC: AGU), 295–312. doi: 10.1029/078ars19
Tagliabue, A., Bopp, L., and Aumont, O. (2009). Evaluating the importance of atmospheric and sedimentary iron sources to Southern Ocean biogeochemistry. Geophys. Res. Lett. 36:L13601.
Takahashi, T., Sutherland, S. C., Chipman, D. W., Goddard, J. G., Ho, C., Newberger, T., et al. (2014). Climatological distributions of pH, pCO2, total CO2, alkalinity, and CaCO3 saturation in the global surface ocean, and temporal changes at selected locations. Mar. Chem. 164, 95–125. doi: 10.1016/j.marchem.2014.06.004
Thoma, M., Jenkins, A., Holland, D., and Jacobs, S. (2008). Modelling circumpolar deep water intrusions on the Amundsen Sea continental shelf, Antarctica. Geophys. Res. Lett. 35:L18602. doi: 10.1029/2008GL034939
Thompson, A. F., Heywood, K. J., Schmidtko, S., and Stewart, A. L. (2014). Eddy transport as a key component of the Antarctic overturning circulation. Nat. Geosci. 7, 879–884. doi: 10.1038/ngeo2289
Thompson, A. F., Stewart, A. L., Spence, P., and Heywood, K. J. (2018). The antarctic slope current in a changing climate. Rev. Geophys. 56, 741–770. doi: 10.1029/2018RG000624
Thompson, D. W. J., and Solomon, S. (2002). Interpretation of recent southern hemisphere Climate Change. Science 296, 895–899. doi: 10.1126/science.1069270
Timmermann, R., and Hellmer, H. H. (2013). Southern Ocean warming and increased ice shelf basal melting in the twenty-first and twenty-second centuries based on coupled ice-ocean finite-element modelling. Ocean Dyn. 63, 1011–1026. doi: 10.1007/s10236-013-0642-0
Tin, T., Liggett, D., Maher, P. T., and Lamers, M. (2014). Antarctic Futures. Human Engagement with the Antarctic Environment. Dordrecht: Springer.
Ting, Y.-H., and Holzer, M. (2017). Decadal changes in Southern Ocean ventilation inferred from deconvolutions of repeat hydrographies. Geophys. Res. Lett. 44, 5655–5664. doi: 10.1002/2017gl073788
Torre, L., Abele, D., Lagger, C., Momo, F., and Sahade, R. (2014). When shape matters: strategies of different Antarctic ascidians morphotypes to deal with sedimentation. Mar. Environ. Res. 99, 179–187. doi: 10.1016/j.marenvres.2014.05.014
Tortell, P. D., Payne, C. D., Li, Y. Y., Trimborn, S., Rost, B., Smith, W. O., et al. (2008). CO2 sensitivity of Southern Ocean phytoplankton. Geophys. Res. Lett. 35:L04605.
Trimborn, S., Brenneis, T., Sweet, E., and Rost, B. (2013). Sensitivity of Antarctic phytoplankton species to ocean acidification: growth, carbon acquisition, and species interaction. Limnol. Oceanogr. 58, 997–1007. doi: 10.4319/lo.2013.58.3.0997
Tuck, G. N., Polacheck, T., and Bulman, C. M. (2003). Spatiotemporal trends of longline fishing effort in the Southern Ocean and implications for seabird bycatch. Biol. Conserv. 114, 1–27. doi: 10.1016/s0006-3207(02)00378-6
Tulloch, V. J. D., Plagányi, ÉE., Matear, R., Brown, C. J., and Richardson, A. J. (2017). Ecosystem modelling to quantify the impact of historical whaling on Southern Hemisphere baleen whales. Fish Fish. 19, 117–137. doi: 10.1111/faf.12241
Tulloch, V. J. D., Plagányi, É. E., Brown, C. J., Matear, R., and Richardson, A. J. (2019). Future recovery of baleen whales is imperiled by climate change. Glob. Chang. Biol. 25 1263–1281. doi: 10.1111/gcb.14573
Tulloch, V., Grech, A., Jonsen, I., Pirotta, V., and Harcourt, R., (2020). Cost-effective mitigation strategies to reduce bycatch threats to cetaceans identified using return-on-investment analysis. Conserv. Biol. 34, 168–179. doi: 10.1111/cobi.13418
Turner, J., Bindschadler, R. A., Convey, P., Di Prisco, G., Fahrbach, E., Gutt, J., et al. (2009). Antarctic Climate Change and the Environment. Cambridge: SCAR.
Turner, J., Hosking, J. S., Phillips, T., and Marshall, G. J. (2013). Temporal and spatial evolution of the Antarctic sea ice prior to the September 2012 record maximum extent. Geophys. Res. Lett. 40, 5894–5898. doi: 10.1002/2013gl058371
Turner, J., Lu, H., White, I., King, J. C., Phillips, T., Hosking, J. S., et al. (2016). Absence of 21st century warming on Antarctic Peninsula consistent with natural variability. Nature 535, 411–415. doi: 10.1038/nature18645
Turner, J., Phillips, T., Marshall, G. J., Hosking, J. S., Pope, J. O., Bracegirdle, T. J., et al. (2017). Unprecedented springtime retreat of Antarctic sea ice in 2016. Geophys. Res. Lett. 44, 6868–6875. doi: 10.1002/2017GL073656
van der Hoop, J. M., Vanderlaan, A. S., Cole, T. V., Cole, T. V. N., Henry, A. G., Hall, L., et al. (2015). Vessel strikes to large whales before and after the 2008 ship strike rule. Conserv. Lett. 8, 24–32. doi: 10.1111/conl.12105
van Der Merwe, P., Wuttig, K., Holmes, T., Trull, T. W., Chase, Z., Townsend, A. T., et al. (2019). High lability Fe particles sourced from glacial erosion can meet previously unaccounted biological demand: heard Island, Southern Ocean. Front. Mar. Sci. 6:332. doi: 10.3389/fmars.2019.00332
van Franeker, J. A., and Bell, P. J. (1988). Plastic ingestion by petrels breeding in Antarctica. Mar. Pollut. Bull. 19, 672–674. doi: 10.1016/0025-326x(88)90388-8
van Heuven, S. M. A. C., Hoppema, M., Huhn, O., Slagter, H. A., and De Baar, H. J. W. (2011). Direct observation of increasing CO2 in the Weddell Gyre along the Prime Meridian during 1973-2008. Deep Sea Res. II 58, 2613–2635. doi: 10.1016/j.dsr2.2011.08.007
van Waerebeek, K. O. E. N., Baker, A. N., Félix, F., Gedamke, J., Iñiguez, M., Sanino, G. P., et al. (2007). Vessel collisions with small cetaceans worldwide and with large whales in the Southern Hemisphere, an initial assessment. LAJAM 6, 43–69.
van Wessem, J. M., Meredith, M. P., Reijmer, C. H., Van den Broeke, M. R., and Cook, A. J. (2017). Characteristics of the modelled meteoric freshwater budget of the western Antarctic Peninsula. Deep Sea Res. II 139, 31–39. doi: 10.1016/j.dsr2.2016.11.001
Venables, H. J., Clarke, A., and Meredith, M. P. (2013). Wintertime controls on summer stratification and productivity at the western Antarctic Peninsula. Limnol. Oceanogr. 58, 1035–1047. doi: 10.4319/lo.2013.58.3.1035
Venables, H. J., and Meredith, M. P. (2014). Feedbacks between ice cover, ocean stratification, and heat content in Ryder Bay, western Antarctic Peninsula. J. Geophys. Res. 119, 5323–5336. doi: 10.1002/2013JC009669
Waller, C. L., Griffiths, H. J., Waluda, C. M., Thorpe, S. E., Loaiza, I., Moreno, B., et al. (2017). Microplastics in the Antarctic marine system: an emerging area of research. Sci. Tot. Environ. 598, 220–227. doi: 10.1016/j.scitotenv.2017.03.283
Wang, G., Hendon, H. H., Arblaster, J. M., Lim, E.-P., Abhik, S., and van Rensch, P. (2019). Compounding tropical and stratospheric forcing of the record low Antarctic sea-ice in 2016. Nat. Commun. 10, 1–9. doi: 10.1038/s41467-018-07689-7
Waugh, D. W., Primeau, F., DeVries, T., and Holzer, M. (2013). Recent changes in the ventilation of the Southern Oceans. Science 339, 568–570. doi: 10.1029/2008JC004864
Welhouse, L. J., Lazzara, M. A., Keller, L. M., Tripoli, G. J., and Hitchman, M. H. (2016). Composite analysis of the effects of ENSO Events on Antarctica. J. Clim. 29, 1797–1808. doi: 10.1175/JCLI-D-15-0108.1
Westwood, K. J., Thomson, P. G., Van Den Enden, R. L., Maher, L. E., Wright, S. W., and Davidson, A. T. (2018). Ocean acidification impacts primary and bacterial production in Antarctic coastal waters during austral summer. J. Exp. Mar. Biol. Ecol. 498, 46–60. doi: 10.1016/j.jembe.2017.11.003
Whitehouse, M. J., Meredith, M. P., Rothery, P., Atkinson, A., Ward, P., and Korb, R. E. (2008). Rapid warming of the ocean around South Georgia, Southern Ocean, during the 20th century: forcings, characteristics and implications for lower trophic levels. Deep Sea Res. I 55, 1218–1228. doi: 10.1016/j.dsr.2008.06.002
Widger, P., and Haddad, A. (2018). Evaluation of SF6 leakage from gas insulated equipment on electricity networks in Great Britain. Energies 11:2037. doi: 10.3390/en11082037
Wilcox, C., Van Sebille, E., and Hardesty, B. D. (2015). Threat of plastic pollution to seabirds is global, pervasive, and increasing. Proc. Nat. Acad. Sci. U.S.A. 112, 11899–11904. doi: 10.1073/pnas.1502108112
Wild, S., McLagan, D., Schlabach, M., Bossi, R., Hawker, D., Cropp, R., et al. (2015). An antarctic research station as a source of brominated and perfluorinated persistent organic pollutants to the local environment. Environ. Sci. Technol. 49, 103–112. doi: 10.1021/es5048232
Williams, N. L., Feely, R. A., Sabine, C. L., Dickson, A. G., Swift, J. H., Talley, L. D., et al. (2015). Quantifying anthropogenic carbon inventory changes in the Pacific sector of the Southern Ocean. Mar. Chem. 174, 147–160. doi: 10.1016/j.marchem.2015.06.015
Winton, V. H. L., Edwards, R., Delmonte, B., Ellis, A., Andersson, P. S., Bowie, A., et al. (2016). Multiple sources of soluble atmospheric iron to Antarctic waters. Glob. Biogeochem. Cycle 30, 421–437. doi: 10.1002/2015gb005265
WMO (2011). World Meteorological Organization Scientific Assessment of Ozone Depletion: 2010 Global Ozone Research and Monitoring Project Report No. 52. Geneva: WMO.
Yogui, G., and Sericano, J. (2009). Levels and pattern of polybrominated diphenyl ethers in eggs of Antarctic seabirds: endemic versus migratory species. Environ. Pollut. 157, 975–980. doi: 10.1016/j.envpol.2008.10.016
Keywords: Southern Annular Mode, ozone hole, cryosphere and climate change, biogeochemsitry, carbon dioxde, non-indigenous species, warming, freshening
Citation: Morley SA, Abele D, Barnes DKA, Cárdenas CA, Cotté C, Gutt J, Henley SF, Höfer J, Hughes KA, Martin SM, Moffat C, Raphael M, Stammerjohn SE, Suckling CC, Tulloch VJD, Waller CL and Constable AJ (2020) Global Drivers on Southern Ocean Ecosystems: Changing Physical Environments and Anthropogenic Pressures in an Earth System. Front. Mar. Sci. 7:547188. doi: 10.3389/fmars.2020.547188
Received: 30 March 2020; Accepted: 23 November 2020;
Published: 15 December 2020.
Edited by:
Monica M. C. Muelbert, Federal University of São Paulo, BrazilReviewed by:
Fabien Roquet, University of Gothenburg, SwedenLeticia Cotrim Da Cunha, Rio de Janeiro State University, Brazil
Copyright © 2020 Morley, Abele, Barnes, Cárdenas, Cotté, Gutt, Henley, Höfer, Hughes, Martin, Moffat, Raphael, Stammerjohn, Suckling, Tulloch, Waller and Constable. This is an open-access article distributed under the terms of the Creative Commons Attribution License (CC BY). The use, distribution or reproduction in other forums is permitted, provided the original author(s) and the copyright owner(s) are credited and that the original publication in this journal is cited, in accordance with accepted academic practice. No use, distribution or reproduction is permitted which does not comply with these terms.
*Correspondence: Simon A. Morley, c21vckBiYXMuYWMudWs=