- 1School of Environmental Science and Engineering, Guangzhou University, Guangzhou, China
- 2Department of Biological Sciences, University of Southern California, Los Angeles, CA, United States
- 3State Key Laboratory of Marine Environmental Science, College of Ocean and Earth Sciences, Xiamen University, Xiamen, China
Dissolution of anthropogenic CO2 into the oceans results in ocean acidification (OA), altering marine chemistry with consequences for primary, secondary, and tertiary food web producers. Here we examine how OA could affect the food quality of primary producers and subsequent trophic transfer to second and tertiary producers. Changes in food quality induced by OA are often related to secondary metabolites in primary producers, such as enriched phenolics in microalgae and iodine in brown algae. These biomolecules can then be transferred to secondary producers, potentially affecting seafood quality and other marine ecosystem services. Furthermore, shifts in dominant functional groups of primary producers under the influence of OA would also impact higher trophic levels through food web interactions. It is challenging to understand how these complex food chain effects of OA may be expressed under the influence of fluctuating environments or multiple drivers, and how these effects can be scaled up through marine food webs to humans.
Introduction
The oceans have absorbed about one-third of the CO2 released into the atmosphere by humans, altering marine carbonate chemistry with a continuous decline of pH, resulting in ocean acidification (OA) (Caldeira and Wickett, 2003). The pH of the surface ocean has dropped by ∼0.1 since pre-industrial times, which is a rate tenfold higher than in the past 300 million years (Hönisch et al., 2012). By the end of this century, proton concentrations in the surface ocean will be more than twice those of the pre-industrial period (IPCC, 2014; Gattuso et al., 2015). Carbonate chemistry changes associated with OA include increases in concentrations of CO2 and bicarbonate and decreases in levels of carbonate ions and pH. Although a wide range of altered carbonate chemistry effects have been observed at each trophic level of marine food webs (Kroeker et al., 2013; Nagelkerken and Munday, 2016; Hutchins and Fu, 2017; Hurd et al., 2018; Gao et al., 2019), how OA affects the food quality of primary producers and trophic transfer to secondary and tertiary producers is still controversial. Here, we present an overview of the up-to-date advances regarding this specific issue and highlight the challenges for future research in this area.
Food Chain Effects Associated With Fatty Acid Changes
Primary producers synthesize lipids, fatty acids (FA), and in particular polyunsaturated FA (PUFA). These biomolecules are crucial cellular components of marine organisms, in that they play critical roles in cell membrane function, physiological processes for energy storage, and trophic interactions in aquatic food webs (Dalsgaard et al., 2003; Guschina and Harwood, 2009). PUFA cannot be synthesized de novo by metazoans, which therefore must acquire these compounds via their diet (Hixson et al., 2015). In aquatic food webs, PUFA are exclusively synthesized by phytoplankton, and thus algal PUFA composition is an important determinant of food quality, consequently affecting the health and optimal functioning of marine and freshwater ecosystems (Dalsgaard et al., 2003).
Ocean acidification is likely to affect all of these interactions; for instance, it has been reported that the FA composition of phytoplankton is highly dependent on CO2 concentrations. PUFA content decreased by ∼3% in the diatom Cylindrotheca fusiformis grown at a pCO2 of 750 μatm (representing OA), compared to a contemporary pCO2 treatment of 380 μatm CO2 (Bermúdez et al., 2015). This suggests a decline of food quality in primary producers under future OA conditions. However, it is worth noting that there is strong spatiotemporal variability in marine carbonate chemistry. This is especially true for coastal seawater due to multiple drivers such as tidal cycles (Dai et al., 2009; Jiang et al., 2011; Wang et al., 2014), upwelling (Capone and Hutchins, 2013), anthropogenic nutrient inputs, aquaculture activities, and changes in ecosystem structure and metabolism (Duarte et al., 2013; Waldbusser and Salisbury, 2014). Elevated photosynthesis and respiration also drive large diel pH fluctuations in coastal and coral reef ecosystems (Santos et al., 2011), and it has been suggested that OA-induced effects on the food quality of primary producers can be dampened in these waters with major natural pCO2 fluctuations (Bermúdez et al., 2016). Moreover, OA is likely to impact different phytoplankton taxa differently in terms of their growth, metabolic activity, and organism size (Flynn et al., 2012). Therefore, investigation of OA effects on food quality has to take into account phytoplankton species of various taxonomic groups and natural fluctuations in pCO2 (Flynn et al., 2012).
Approximately 10–20% of essential biomolecules in primary producers are incorporated into new biomass at the next trophic level, and thus the decline of food quality in primary producers will have potential impacts on the zooplankton which prey on them. In one striking study by Rossoll et al. (2012), declines in both total FAs and the ratio of long-chain polyunsaturated to saturated FAs, constrained growth and reproduction of the copepod Acartia tonsa fed the diatom Thalassiosira pseudonana grown under OA conditions. Further support for this food chain effect of OA was provided by a mesocosm experiment using a natural plankton community. A declining proportion of PUFA in the natural phytoplankton assemblage was mirrored by a reduction in the relative PUFA content of the dominant copepod Calanus finmarchicus (Bermúdez et al., 2016). Another study found that changes in ratios of lipid to protein and/or lipid to carbohydrate in phytoplankton under the influence of OA decreased trophic transfer efficiency from phytoplankton to zooplankton by about 50%, and caused a commensurate decrease in zooplankton recruitment (Cripps et al., 2016). On the other hand, OA has sometimes been shown to increase food availability, and thus help alleviate the negative effects of acidification on invertebrates (Thomsen et al., 2013).
Besides the indirect effects of OA on zooplankton via trophic transfer (bottom up effects), it can also directly affect zooplankton differentially according to gender and/or developmental stage (Cripps et al., 2014). In addition, it is not only the food quality of primary producers that can be altered by OA. Higher trophic levels such as some commercially valuable oysters can become less nutritious due to lower levels of proteins, lipids, carbohydrates, and caloric content under OA conditions (Lemasson et al., 2018). Overall, the available data suggest that FA and PUFA in phytoplankton can be influenced by OA, and these compositional changes will also affect transfer to higher trophic levels which rely on their prey as a source of these essential macromolecules.
Phenolics and Iodine Changes in Algae and Their Grazers
Besides lipids and FA, other secondary metabolites in primary producers such as phenolic compounds can also be affected by OA. As reported in pioneering work by Arnold et al. (2012), polymeric phenolics decreased in the seagrasses Cymodocea nodosa, Ruppia maritima, and Potamogeton perfoliatus under OA conditions. In contrast, phytoplankton species (diatoms and coccolithophores) increased their phenolic compounds when grown in acidified seawater (Jin et al., 2015). This accumulation of phenolics under OA has been suggested to be caused by alterations in β-oxidation metabolic pathways (Figure 1). Upregulation of β-oxidation and the Krebs cycle, with enhanced degradation of phenolics, may provide extra energy to cope with acidic stress caused by OA. Since phenolic compounds are highly toxic to most grazers, an increase in their content in primary producers has the potential to drive significant consequences for food webs (Boyd and Carlucci, 1993; Ianora et al., 2006). Interestingly, Jin et al. (2015) demonstrated that copepods fed with OA-grown phytoplankton had higher phenolics content compared to the control (Figure 1), suggesting a food chain effect of OA from primary to secondary producers. The increased phenolic compounds in primary producers associated with FA metabolism via β-oxidation would further decrease the nutritional value of these organisms. This might provide a tentative explanation for the observation that shrimp grown under OA in the presence of natural phytoplankton and zooplankton tasted bitter (Dupont et al., 2014). In addition, as phenolics are known to possess antimicrobial properties (Arnold and Targett, 2002), biogeochemical cycles in the oceans may be affected as well. Food chain effects of OA were also observed in the brown alga Saccharina japonica, in which OA increased the accumulation of iodide and the amounts of iodine released into seawater, while abalone fed on the kelp, exhibited increased levels of iodine (Xu et al., 2019). Altogether, a limited number of studies show that OA can influence accumulation of secondary metabolites and their transfer to higher trophic levels, thereby producing cascading impacts on marine food webs.
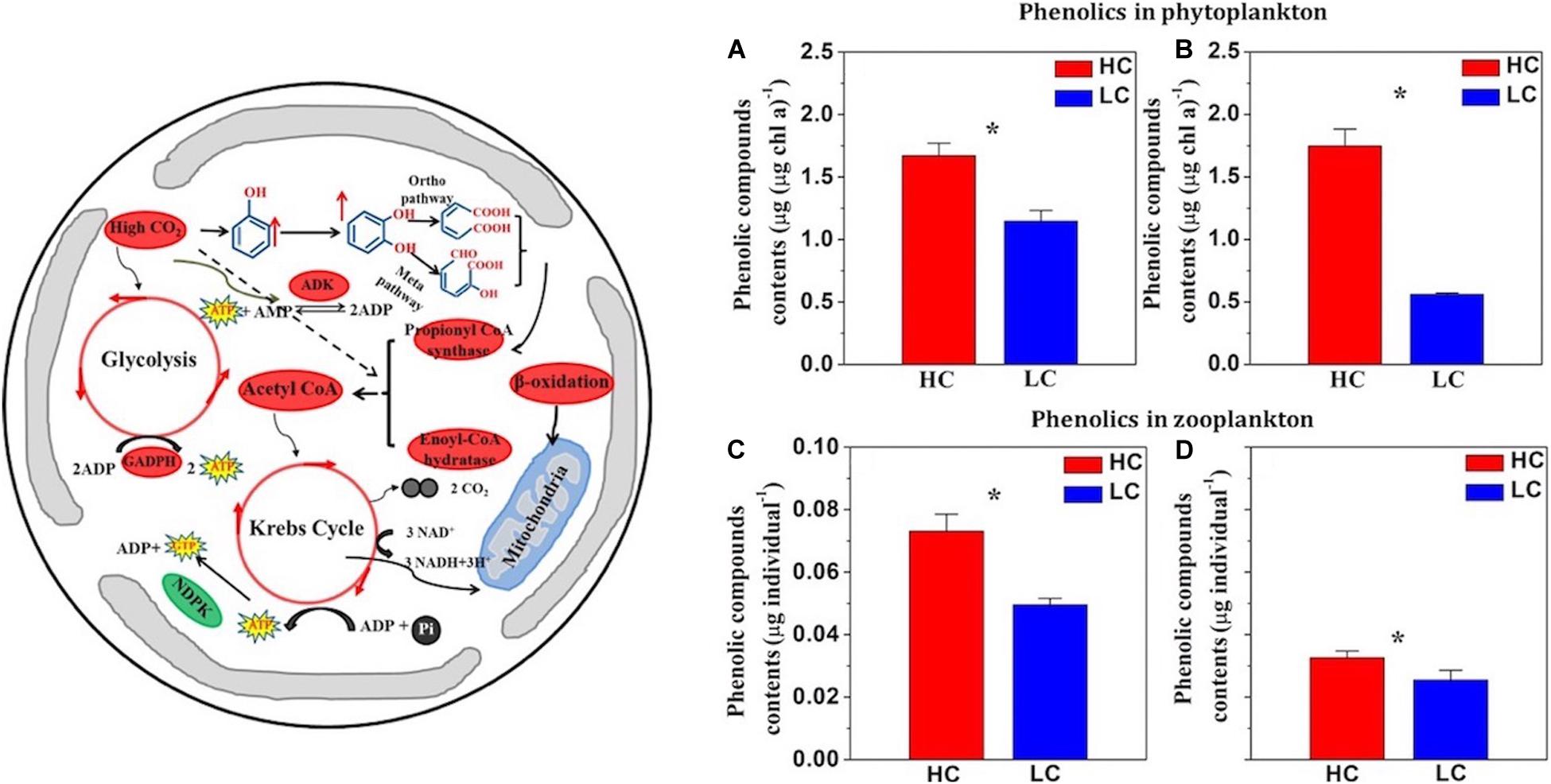
Figure 1. Left panel: Ocean acidification (OA) up regulates (red) or down regulates (green) metabolic pathways of the calcified phytoplankton species Emilianiahuxleyi. The cells up regulate several metabolic pathways such as β-oxidation, the TCA cycle, and glycolysis which is required for the degradation of phenolics, in order to gain more energy to resist OA. Right panels: OA significantly increased the concentrations of phenolics of phytoplankton assemblages in 30-L microcosm (A) and in 4000-L mesocosm experiments (B). Content of phenolic compounds (Îijg per individual) in zooplankton assemblages that were fed on high CO2 (HC, 1000 Îijatm, Red bar) or low pCO2 grown (LC, 395 Îijatm, Blue bar) phytoplankton cells collected from the microcosms (C) or mesocosms (D) (Jin et al., 2015). Vertical lines represent standard deviation of the means. *Significance at the p < 0.05 level. (reconstructed from Jin et al., 2015).
Consequences of Altered Community Composition of Primary Producers
In addition to biochemical changes in primary producers, the influence of OA on their composition may also impact energetic and nutritional transfer to higher trophic levels. For instance, OA may selectively favor an increase in the growth rates of larger versus smaller phytoplankton species (Wu et al., 2014; Bach and Taucher, 2019). OA-driven shifts toward larger diatoms have been observed in natural phytoplankton communities (Tortell et al., 2008; Feng et al., 2010; Eggers et al., 2014; Bach et al., 2017; Taucher et al., 2017). Such phytoplankton community shifts to larger species may increase the trophic transfer of energy to marine animals by shortening food chains (Sommer et al., 2002) and promote production in higher trophic levels. At a natural CO2 seep in the North Pacific Ocean, the large chain-forming diatom Biddulphia biddulphiana greatly increases in abundance as CO2 increases along a seawater concentration gradient. Along this same gradient, the abundance of calcified grazers such as gastropods and sea urchins decreased (Harvey et al., 2019). This observation suggests that OA can alter the food-web structure and ecosystem productivity by shifting the community composition of primary producers.
In a mesocosm experiment with an oligotrophic plankton community in the subtropical North Atlantic Ocean, the toxic microalga Vicicitus globosus increased its abundance at CO2 levels higher than 600 μatm and developed into blooms above 800 μatm CO2. This strongly inhibited the development of the zooplankton community, thereby disrupting the trophic transfer of organic matter from primary producers (Riebesell et al., 2018). This food chain alteration prolonged the residence of particulate matter in the water column and caused a strong decline in export flux. In another mesocosm study in a sill fjord located on the Swedish west coast, enhanced primary production under OA increased the survival rate of Atlantic herring (Clupea harengus) larvae (Sswat et al., 2018). Similarly, a positive bottom-up effect of OA from primary to secondary producers and from secondary producers to secondary consumers has been reported in benthic fish (Goldenberg et al., 2017).
Together with other climate change drivers, coastal eutrophication can promote harmful algal blooms (Fu et al., 2012; Gao et al., 2012), which may lead to seawater basification (increase in pH) and thus override the signal from OA (Flynn et al., 2015). Basification during bloom development would act independently or interactively with other factors to influence algal growth and blooms succession (Macedo et al., 2001; Hansen, 2002; Hinga, 2002; Flynn et al., 2015). Therefore, the impacts of OA on phytoplankton community composition and thus on trophic transfer are somewhat dependent on levels of eutrophication in coastal waters. Moreover, these responses may also vary across different seasons and/or in different regions (Tortell et al., 2002; Rost et al., 2003; Hare et al., 2007; Grear et al., 2017). It is apparent that changes in phytoplankton community succession and/or primary productivity caused by OA will inevitably impact higher trophic levels through food-web interactions, although these effects may differ spatiotemporally with different levels of adaptation to regionally unique environments.
While marine secondary producers such as zooplankton and tertiary producers such as fish can be indirectly affected by OA, through trophic transfer or food-web interactions, additional direct effects of OA often cannot be ruled out. As summarized in the comprehensive review by Nagelkerken and Munday (2016), behaviors of secondary and tertiary producers such as olfaction, phototaxis, lateralization, shelter, and escape can be altered by OA through multiple, non-exclusive mechanisms (also see recent studies by Schunter et al., 2016, 2018; Spady et al., 2018; Jarrold and Munday, 2019; Clark et al., 2020). This in turn will affect species interactions, population dynamics, community structure, and ultimately, biodiversity (Nagelkerken and Munday, 2016). Changes in species interactions are most commonly implicated as proximate causes of population declines and extinctions due to ocean climate changes.
Perspectives
A lot of progress has been made in understanding how OA affects marine primary, secondary, and tertiary producers directly, yet we are still far from understanding how these effects may transfer across multiple trophic levels (Figure 2). There have been only a handful of studies that investigated how the deterioration in nutritional values of primary producers due to OA may affect secondary producers via food chain effects. Considering that fish are a critical natural resource (Food and Agriculture Organization of the United Nations [FAO], 2014), there is a potential that the food chain effects of OA can reach humans, who rely on fisheries as an important protein source. However, this has not yet been examined in detail, especially with full consideration of how food chain effects could scale up through multiple trophic levels (e.g., primary to secondary to tertiary and then to humans) (Figure 2). Because our understanding of the effects of multiple marine environmental changes on phytoplankton from different functional groups is still poor, more studies from monocultures to community levels are expected. Furthermore, since primary producers are known to exhibit evolutionary responses to OA (Jin et al., 2013; Hutchins et al., 2015; Collins et al., 2020), how these responses will alter the trophic transfer in food webs should also be considered.
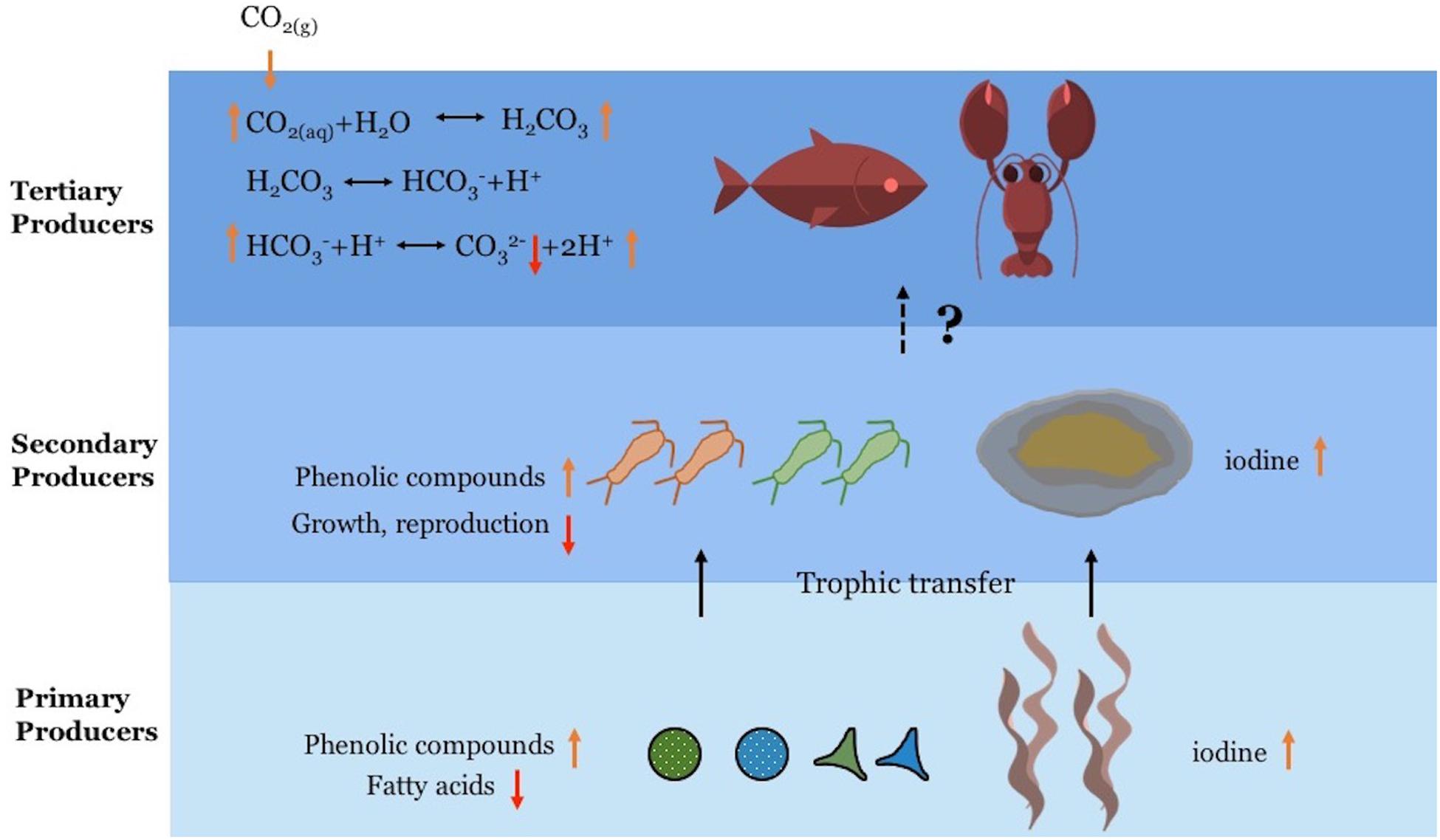
Figure 2. Conceptual diagram showing the food chain effects of ocean acidification (OA). OA increased the contents of phenolic compounds and iodine in the primary producers (phytoplankton and kelp), and these changes lead to accumulation in the secondary producers that feed on them. Whether these food chain effects can scale up in multiple trophic levels (e. g. primary to secondary to tertiary producers) needs further investigation. The orange arrows indicate increased and red ones indicate decreased levels of the compounds, growth or reproduction.
Author Contributions
PJ and KG contributed to the theoretical designs and data analysis. PJ wrote the manuscript with major contributions from KG and DH. All authors contributed to the article and approved the submitted version.
Funding
This study was supported by the National Natural Science Foundation (41806141 and 41720104005), the Innovation Program of Guangdong Province (No. 2018KTSCX173), and the MEL Visiting Fellowship (MELRS1903).
Conflict of Interest
The authors declare that the research was conducted in the absence of any commercial or financial relationships that could be construed as a potential conflict of interest.
References
Arnold, T., Mealey, C., Leahey, H., Miller, A. W., Hall-Spencer, J. M., Milazzo, M., et al. (2012). Ocean acidification and the loss of phenolic substances in marine plants. PLoS One 7:e35107. doi: 10.1371/journal.pone.0035107
Arnold, T. M., and Targett, N. M. (2002). Marine tannins: the importance of a mechanistic framework for predicting ecological roles. J. Chem. Ecol. 28, 1919–1934.
Bach, L. T., Alvarez-Fernandez, S., Hornick, T., Stuhr, A., and Riebesell, U. (2017). Simulated ocean acidification reveals winners and losers in coastal phytoplankton. PLoS One 12:e0188198. doi: 10.1371/journal.pone.0188198
Bach, L. T., and Taucher, J. (2019). CO2 effects on diatoms: a synthesis of more than a decade of ocean acidification experiments with natural communities. Ocean Sci. 15, 1159–1175.
Bermúdez, J. R., Winder, M., Stuhr, A., Almén, A.-K., Engström-Öst, J., and Riebesell, U. (2016). Effect of ocean acidification on the structure and fatty acid composition of a natural plankton community in the Baltic Sea. Biogeosciences 13, 6625–6635.
Bermúdez, R., Feng, Y., Roleda, M. Y., Tatters, A. O., Hutchins, D. A., Larsen, T., et al. (2015). Long-term conditioning to elevated pCO2 and warming influences the fatty and amino acid composition of the diatom Cylindrotheca fusiformis. PLoS One 10:e0123945. doi: 10.1371/journal.pone.0123945
Boyd, T. J., and Carlucci, A. (1993). Degradation rates of substituted phenols by natural populations of marine bacteria. Aquat. Toxicol. 25, 71–82.
Capone, D. G., and Hutchins, D. A. (2013). Microbial biogeochemistry of coastal upwelling regimes in a changing ocean. Nat. Geosci. 6, 711–717. doi: 10.1038/ngeo1916
Clark, T. D., Raby, G. D., Roche, D. G., Binning, S. A., Speers-Roesch, B., Jutfelt, F., et al. (2020). Ocean acidification does not impair the behaviour of coral reef fishes. Nature 577, 370–375.
Collins, S., Boyd, P. W., and Doblin, M. A. (2020). Evolution, microbes, and changing ocean conditions. Annu. Rev. Mar. Sci. 12, 181–208.
Cripps, G., Flynn, K. J., and Lindeque, P. K. (2016). Ocean acidification affects the phyto-zoo plankton trophic transfer efficiency. PLoS One 11:e0151739. doi: 10.1371/journal.pone.0151739
Cripps, G., Lindeque, P., and Flynn, K. J. (2014). Have we been underestimating the effects of ocean acidification in zooplankton? Global Change Biol. 20, 3377–3385. doi: 10.1111/gcb.12582
Dai, M., Lu, Z., Zhai, W., Chen, B., Cao, Z., Zhou, K., et al. (2009). Diurnal variations of surface seawater pCO2 in contrasting coastal environments. Limnol. Oceanogr. 54, 735–745.
Dalsgaard, J., St. John, M., Kattner, G., Müller-Navarra, D., and Hagen, W. (2003). Fatty acid trophic markers in the pelagic marine food environment. Adv. Mar. Biol. 46, 225–234.
Duarte, C. M., Hendriks, I. E., Moore, T. S., Olsen, Y. S., Steckbauer, A., Ramajo, L., et al. (2013). Is ocean acidification an open-ocean syndrome? Understanding anthropogenic impacts on seawater pH. Estuar. Coast. 36, 221–236.
Dupont, S., Hall, E., Calosi, P., and Lundve, B. (2014). First evidence of altered sensory quality in a shellfish exposed to decreased pH relevant to Ocean acidification. J. Shellfish. Res. 33, 857–861.
Eggers, S. L., Lewandowska, A. M., Barcelos, E., Ramos, J., Blanco-Ameijeiras, S., Gallo, F., et al. (2014). Community composition has greater impact on the functioning of marine phytoplankton communities than ocean acidification. Glob. Change Biol. 20, 713–723.
Feng, Y., Hare, C. E., Rose, J. M., Handy, S. M., DiTullio, G. R., Lee, P. A., et al. (2010). Interactive effects of iron, irradiance and CO2 on Ross Sea phytoplankton. Deep Sea Res. Part Oceanogr. Res. Pap. 57, 368–383.
Flynn, K. J., Blackford, J. C., Baird, M. E., Raven, J. A., Clark, D. R., Beardall, J., et al. (2012). Changes in pH at the exterior surface of plankton with ocean acidification. Nat. Clim. Change 2, 510–513. doi: 10.1038/nclimate1489
Flynn, K. J., Clark, D. R., Mitra, A., Fabian, H., Hansen, P. J., Glibert, P. M., et al. (2015). Ocean acidification with (de)eutrophication will alter future phytoplankton growth and succession. Proc. R. Soc. B. 282:20142604.
Food and Agriculture Organization of the United Nations [FAO] (2014). The State of World Fisheries and Aquaculture, 1st Edn, Vol. 1. Rome: FAO, 62–68.
Fu, F.-X., Tatters, A. O., and Hutchins, D. A. (2012). Global change and the future of harmful algal blooms in the ocean. Mar. Ecol. Prog. Ser. 470, 207–233. doi: 10.3354/meps10047
Gao, K., Beardall, J., Häder, D. P., Hall-Spencer, J. M., Gao, G., and Hutchins, D. A. (2019). Effects of ocean acidification on marine photosynthetic organisms under the concurrent influences of warming, UV radiation and deoxygenation. Front. Mar. Sci. 6:322. doi: 10.3389/fmars.2019.00322
Gao, K., Helbling, E. W., Häder, D.-P., and Hutchins, D. A. (2012). Responses of marine primary producers to interactions between ocean acidification, solar radiation, and warming. Mar. Ecol. Prog. Ser. 470, 167–189. doi: 10.3354/meps10043
Gattuso, J.-P., Magnan, A., Billé, R., Cheung, W., Howes, E., Joos, F., et al. (2015). Contrasting futures for ocean and society from different anthropogenic CO2 emissions scenarios. Science 349:aac4722. doi: 10.1126/science.aac4722
Goldenberg, S. U., Nagelkerken, I., Ferreira, C. M., Ullah, H., and Connell, S. D. (2017). Boosted food web productivity through ocean acidification collapses under warming. Glob. Change Biol. 23, 4177–4184.
Grear, J. S., Rynearson, T. A., Montalbano, A. L., Govenar, B., and Menden-Deuer, S. (2017). pCO2 effects on species composition and growth of an estuarine phytoplankton community. Estuar. Coast. Shelf Sci. 190, 40–49. doi: 10.1016/j.ecss.2017.03.016
Guschina, I. A., and Harwood, J. L. (2009). “Algal lipids and effect of the environment on their biochemistry,” in Lipids in Aquatic Ecosystems, eds M. T. Arts, M. T. Brett, and M. J. Kainz (New York: Springer), 1–24.
Hansen, P. J. (2002). The effect of high pH on the growth and survival of marine phytoplankton: implications for species succession. Aquat. Microb. Ecol. 28, 279–288. doi: 10.3354/ame028279
Hare, C., Leblanc, K., DiTullio, G., Kudela, R., Zhang, Y., Lee, P., et al. (2007). Consequences of increased temperature and CO2 for phytoplankton community structure in the Bering Sea. Mar. Ecol. Prog. Ser. 35, 9–16.
Harvey, B. P., Agostini, S., Kon, K., Wada, S., and Hall-Spencer, J. M. (2019). Diatoms dominate and alter marine food-webs when CO2 rises. Diversity 12:242.
Hinga, K. R. (2002). Effect of high pH on coastal marine phytoplankton. Mar. Ecol. Prog. Ser. 238, 281–300. doi: 10.3354/meps238281
Hixson, S. M., Sharma, B., Kainz, M. J., Wacker, A., and Arts, M. T. (2015). Production, distribution, and abundance of long-chain omega-3 polyunsaturated fatty acids: a fundamental dichotomy between freshwater and terrestrial ecosystems. Environ. Rev. 23, 414–424.
Hönisch, B., Ridgwell, A., Schmidt, D. N., Thomas, E., Gibbs, S. J., Sluijs, A., et al. (2012). The geological record of ocean acidification. Science 335, 1058–1063.
Hurd, C. L., Lenton, A., Tilbrook, B., and Boyd, P. W. (2018). Current understanding and challenges for oceans in a higher-CO2 world. Nat. Clim. Chang. 8, 686–694.
Hutchins, D. A., and Fu, F. (2017). Microorganisms and ocean global change. Nat. Microbiol. 2:17058.
Hutchins, D. A., Walworth, N., Webb, E. A., Saito, M. A., Moran, D., McIlvin, M. R., et al. (2015). Irreversibly increased N2 fixation in Trichodesmium experimentally adapted to high CO2. Nat. Commun. 6:8155. doi: 10.1038/ncomms9155
Ianora, A., Boersma, M., Casotti, R., Fontana, A., Harder, J., Hoffmann, F., et al. (2006). New trends in marine chemical ecology. Estuar. Coast. 29, 531–551.
IPCC (2014). Synthesis Report. Contribution of Working Groups I. II and III to the Fifth Assessment Report of the Intergovernmental Panel on Climate Change. Geneva: Intergovernmental Panel on Climate Change, 151.
Jarrold, M. D., and Munday, P. L. (2019). Diel CO2 cycles and parental effects have similar benefits to growth of a coral reef fish under ocean acidification. Biol. Lett. 15:20180724. doi: 10.1098/rsbl.2018.0724
Jiang, Z.-P., Huang, C. Jr., Dai, M., Kao, S. J., Hydes, D. J., Chou, W. C., et al. (2011). Short-term dynamics of oxygen and carbon in productive nearshore shallow seawater systems off Taiwan: observations and modelling. Limnol. Oceanogr. 56, 1832–1849.
Jin, P., Gao, K., and Beardall, J. (2013). Evolutionary responses of a coccolithophorid Gephyrocapsa oceanica to ocean acidification. Evolution 67, 1869–1878.
Jin, P., Wang, T., Liu, N., Dupont, S., Beardall, J., Boyd, P. W., et al. (2015). Ocean acidification increases the accumulation of toxic phenolic compounds across trophic levels. Nat. Commun. 6:8714. doi: 10.1038/ncomms9714
Kroeker, K. J., Kordas, R. L., Crim, R., Hendriks, I. E., Ramajo, L., Singh, G. S., et al. (2013). Impacts of ocean acidification on marine organisms: quantifying sensitivities and interaction with warming. Glob. Change Biol. 19, 1884–1896.
Lemasson, A. J., Hall-Spencer, J. M., Kuri, V., and Knights, A. M. (2018). Changes in the biochemical and nutrient composition of seafood due to ocean acidification and warming. Mar. Environ. Res. 143, 82–92. doi: 10.1016/j.marenvres.2018.11.006
Macedo, M. F., Duarte, P., Mendes, P., and Ferreira, J. G. (2001). Annual variation of environmental variables, phytoplankton species composition and photosynthetic parameters in a coastal lagoon. J. Plankt. Res. 23, 719–732.
Nagelkerken, I., and Munday, P. L. (2016). Animal behaviour shapes the ecological effects of ocean acidification and warming: moving from individual to community−level responses. Glob. Change Biol. 22, 974–989. doi: 10.1111/gcb.13167
Riebesell, U., Aberle-Malzahn, N., Achterberg, E. P., Algueró-Muñiz, M., Alvarez-Fernandez, S., Arístegui, J., et al. (2018). Toxic algal bloom induced by ocean acidification disrupts the pelagic food web. Nat. Clim. Chang. 8, 1082–1086.
Rossoll, D., Bermúdez, R., Hauss, H., Schulz, K., and Riebesell, U. (2012). Ocean acidification-induced food quality deterioration constrains trophic transfer. PLoS One 7:e34737. doi: 10.1371/journal.pone.0034737
Rost, B., Riebesell, U., Burkhardt, S., and Sultemeyer, D. (2003). Carbon acquisition of bloom-forming marine phytoplankton. Limnol Oceanogr. 48, 55–67.
Santos, I. R., Glud, R. N., Maher, D., Erler, D., and Eyre, B. D. (2011). Diel coral reef acidification driven by porewater advection in permeable carbonate sands, Heron Island, Great Barrier Reef. Geophys. Res. Lett. 38:L03604.
Schunter, C., Welch, M. J., Nilsson, G. E., Rummer, J. L., Munday, P. L., and Ravasi, T. (2018). An interplay between plasticity and parental phenotype determines impacts of ocean acidification on a reef fish. Nat. Ecol. Evol. 2, 334–342.
Schunter, C., Welch, M. J., Ryu, T., Zhang, H., Berumen, M. L., Nilsson, G. E., et al. (2016). Molecular signatures of transgenerational response to ocean acidification in a species of reef fish. Nat. Clim. Chang. 6, 1014–1018.
Sommer, U., Stibor, H., Katechakis, A., Sommer, F., and Hansen, T. (2002). Pelagic food web configurations at different levels of nutrient richness and their implications for the ratio fish production:primary production. Hydrobiologia 484, 11–20.
Spady, B. L., Munday, P. L., and Watson, S. A. (2018). Predatory strategies and behaviors in cephalopods are altered by elevated CO2. Glob. Change Biol. 24, 2585–2596.
Sswat, M., Stiasny, M. H., Taucher, J., Algueró-Muñiz, M., Bach, L. T., Jutfelt, F., et al. (2018). Food web changes under ocean acidification promote herring larvae survival. Nat. Ecol. Evol. 2, 836–840.
Taucher, J., Bach, L. T., Boxhammer, T., Nauendorf, A., Achterberg, E. P., Algueró-Muñiz, M., et al. (2017). Influence of ocean acidification and deep water upwelling on oligotrophic plankton communities in the subtropical North Atlantic: insights from an in situ mesocosm study. Front. Mar. Sci. 4:85. doi: 10.3389/fmars.2017.00085
Thomsen, J., Casties, I., Pansch, C., Körtzinger, A., and Melzner, F. (2013). Food availability outweighs ocean acidification effects in juvenile Mytilus edulis: laboratory and field experiments. Glob. Change Biol. 19, 1017–1027.
Tortell, P. D., DiTullio, G. R., Sigman, D. M., and Morel, F. M. M. (2002). CO2 effects on taxonomic composition and nutrient utilization in an Equatorial Pacific phytoplankton assemblage. Mar. Ecol. Prog. Ser. 236, 37–43.
Tortell, P. D., Payne, C. D., Li, Y., Trimborn, S., Rost, B., Smith, W. O., et al. (2008). CO2 sensitivity of Southern Ocean phytoplankton. Geophys. Res. Lett. 35:L04605. doi: 10.1029/2007GL032583
Waldbusser, G. G., and Salisbury, J. E. (2014). Ocean acidification in the coastal zone from an organism’s perspective: multiple system parameters, frequency domains, and habitats. Annu. Rev. Mar. Sci. 6, 221–247. doi: 10.1146/annurev-marine-121211-172238
Wang, G., Jing, W., Wang, S., Xu, Y., Wang, Z., Zhang, Z., et al. (2014). Coastal acidification induced by tidal-driven submarine groundwater discharge in a coastal coral reef system. Environ. Sci. Technol. 48, 13069–13075.
Wu, Y., Campbell, D. A., Irwin, A. J., Suggett, D. J., and Finkel, Z. V. (2014). Ocean acidification enhances the growth rate of larger diatoms. Limnol. Oceanogr. 59, 1027–1034.
Keywords: ocean acidification, fatty acids, food quality, primary producers, trophic transfer, secondary producers
Citation: Jin P, Hutchins DA and Gao K (2020) The Impacts of Ocean Acidification on Marine Food Quality and Its Potential Food Chain Consequences. Front. Mar. Sci. 7:543979. doi: 10.3389/fmars.2020.543979
Received: 19 March 2020; Accepted: 27 August 2020;
Published: 23 October 2020.
Edited by:
Silvia Franzellitti, University of Bologna, ItalyReviewed by:
Shane B. Kanatous, Colorado State University, United StatesWayne R. Fitzgibbon, Medical University of South Carolina, United States
Copyright © 2020 Jin, Hutchins and Gao. This is an open-access article distributed under the terms of the Creative Commons Attribution License (CC BY). The use, distribution or reproduction in other forums is permitted, provided the original author(s) and the copyright owner(s) are credited and that the original publication in this journal is cited, in accordance with accepted academic practice. No use, distribution or reproduction is permitted which does not comply with these terms.
*Correspondence: Kunshan Gao, a3NnYW9AeG11LmVkdS5jbg==