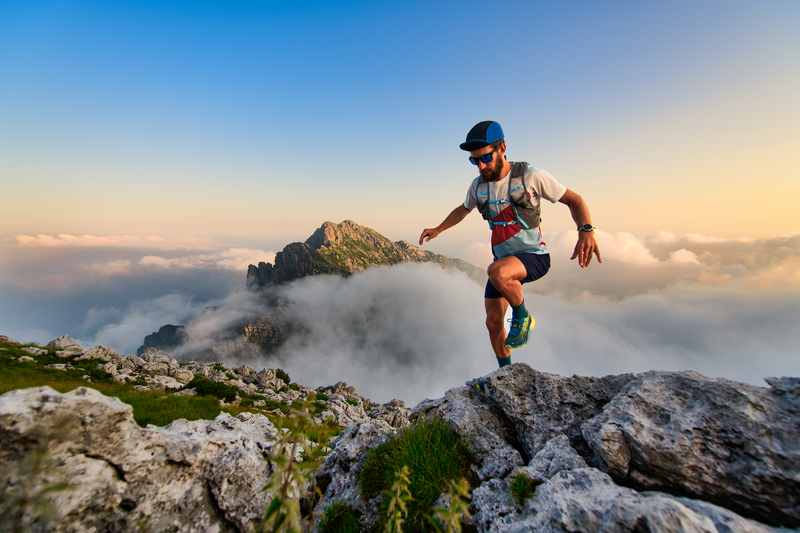
95% of researchers rate our articles as excellent or good
Learn more about the work of our research integrity team to safeguard the quality of each article we publish.
Find out more
ORIGINAL RESEARCH article
Front. Mar. Sci. , 28 August 2020
Sec. Marine Biotechnology and Bioproducts
Volume 7 - 2020 | https://doi.org/10.3389/fmars.2020.543523
Drug resistance to the classically used chemotherapeutic drugs is the major challenge in their treatment of cancer needing the discovery of novel anticancer drugs. In terms of finding novel therapeutics, endophytes are quite promising as they are an excellent source of novel structures, which exhibit bioactivity. The present study demonstrated a dose-dependent antiproliferative activity of mycelial-derived secondary metabolites from a macroalgae associated endophyte Aspergillus unguis AG 1.1 (G). The antiproliferative activity of A. unguis mycelial extract (AUME) was observed on different human cancer cell lines. PI live/dead assay further confirmed the cytotoxic potential of the mycelial extract. Furthermore, AUME caused mitochondrial membrane aberration and generated ROS production as well indicating its potential to induce cell death by apoptosis. The metabolic profiling of the mycelial extract using GC-MS and LC-MS/MS revealed the presence of fatty acids, a benzoquinolinone derivative, imidazolidinedione derivative, diethyl phthalate and phthalate acid ester, a difuraxanthone, two prenylxanthone analogs and a phthalide derivative and some unknown metabolites. Presence of 4-(4-Hydroxy-3,5-dimethoxy-phenyl)-3,4-dihydro-1H-benzo[h]quinolin-2-one, 1-hydroxy-3,5-dimethoxy-2-prenylxanthone, 1,6-dihydroxy-3-methoxy-2-prenylxanthone and 3-butylidene-7-hydroxyphthalide in AUME could be correlated to the notable cytotoxicity exhibited by the endophyte. The additional presence of many unidentified compounds heightened the prospects of finding some novel bioactive metabolites. Our results indicated that secondary metabolites produced by A. unguis AG 1.1 (G) have therapeutic potential as anticancer agents.
Cancer is the major cause of morbidity and mortality worldwide. Global statistics estimated the death of 9.6 million people due to cancer in 2018 (Bray et al., 2018). Survival rate and quality of life of cancer patients are drastically improved by anticancer drugs, thanks to advances in cancer biology and medical biotechnology (Ma and Adjei, 2009). A major challenge in the successful treatment of cancer is the drug resistance to the chemotherapeutic drugs due to the various intrinsic and extrinsic factors such as drug inactivation by cancer cells, apoptosis suppression and epigenetic modification (Mansoori et al., 2017). Hence, the discovery of novel anticancer drug targets is an utmost priority.
Natural products derived from plants, marine organisms and microorganism contribute to more than 60% of the currently used anticancer agents. The bioactive metabolites such as alkaloids, terpenoids, flavonoids, sterols and polysaccharide from natural sources are widely reported for their anticancer activity (Seelinger et al., 2012; Rayan et al., 2017). Endophytes are the promising tools for red biotechnology as they are the rich source of bioactive compounds of therapeutic value. Some of these compounds are identical natural products synthesized by the associated host plant. The importance of endophytes was brought into the limelight by the discovery of taxol producing endophyte Taxomyces andreanae, isolated from the bark of Taxus brevifolia (Kusari et al., 2014). The genetic possibility for the secondary metabolite production and the stimuli of the production basically determine the biotrophic relationship between fungi and host plant as the fungal partner has to alter its lifestyle to assimilate within a plant host. Secondary metabolites serve complex roles; some are involved in cross-kingdom talk, others are in conjunction with fungal life cycle such as sporulation, while some suppress the host immune system (Pusztahelyi et al., 2015; Zeilinger et al., 2016).
Considering the high diversity of marine ecosystem, the marine fungi still remain untapped. Endophytic fungi are ubiquitous in all marine habitats such as marine plants, marine vertebrates and invertebrates. There is a huge knowledge gap in the marine derived endophytes and their secondary metabolite production. Bioactive metabolites produced by macroalgal endophytes play a role in chemical defense of the host by conferring protection against the various pathogens, which explains their heterogeneity and functionality (Vallet et al., 2018).
Enteromorpha species are widely distributed green algae in the saline coastal region. They were reported to produce bioactive carotenoids, fucoidans, phlorotannins and bioactive polysaccharide with potential application as functional ingredients in pharmaceuticals, nutraceuticals and cosmeceuticals industries (Zhao et al., 2016). Wardomyces anomalus isolated from Enteromorpha species produced potent antibacterial and antioxidant molecules (Abdel-Lateff et al., 2003). Aspergillus genus is an excellent chemical factory that is capable of producing biotechnologically important compounds such as enzymes, organic acid and bioactive metabolites of therapeutic potential. Endophytes belonging to the Aspergillus genus were reported to produce pharmaceutically relevant compounds such as deoxy podophyllotoxin and taxol (Kusari et al., 2009; Zhao et al., 2009). In our previous study, we reported that Aspergillus species, the predominantly present endophytes in marine macroalgae isolated from the Konkan coast are producing secondary metabolites with potential antibacterial, antioxidant and cytotoxic activities. One of the isolate, A. unguis AG 1.2 exhibited excellent cytotoxicity against various human cancer cell lines by inducing apoptosis. The total culture crude extract of another isolate, A. unguis AG 1.1 (G) demonstrated significant cytotoxic activity and antibacterial activity (Kamat et al., 2020). In the present study, we investigated whether the cytotoxic secondary metabolites produced by the potential isolate, A. unguis AG 1.1 (G) were mycelial bound or cell-free. We aimed to study the mechanism behind the cytotoxic activity and secondary metabolite profiling of A. unguis AG 1.1 (G).
Potato dextrose agar and broth (PDA and PDB) were procured from Himedia, India. Dulbecco’s modified Eagle’s medium (DMEM) and 2,2-Diphenyl-1-picrylhydrazyl (DPPH) were purchased from Sigma Aldrich, United States. Fetal Bovine Serum (FBS) and trypsin-EDTA were obtained from GIBCO-BRL. Antibiotics and 3-(4, 5-dimethylthiazole-2yl)-2, 5-diphenyl tetrazolium bromide (MTT) reagent were procured from SRL-Ranbaxy. TLC plates and organic solvents like ethyl acetate and methanol were obtained from Merck-Millipore. All other chemicals and reagents used were of analytical grade.
The endophytic fungus, A. unguis AG 1.1 (G) was isolated from a green macroalga, Enteromorpha sp. The preservation and maintenance of stock cultures were carried in PDA slants at 4°C and for long-term storage as spores and mycelium in 15% (v/v) glycerol at -70°C. Phylogenetic analysis was performed for the strain identification. The cytotoxic activity of the total culture crude extract was initially screened by MTT assay on different human cancer cell lines (Kamat et al., 2020).
To determine whether the cytotoxic compounds produced by A. unguis AG 1.1 (G) are mycelial bound or extracellular, ethyl acetate extract of the mycelium and culture filtrate were tested separately for cytotoxicity by MTT assay on different human cancer cell lines- HeLa, MCF-7, A-431, and COLO 205 as described below. Cytotoxicity of fungal extracts was also tested on normal human embryonic kidney cell line (HEK 293). For the preparation of crude extracts, A. unguis AG 1.1 (G) was grown on PDA plates at 25°C for 7 days. Two agar discs (0.5 × 0.5 cm) were used as inoculum. A. unguis AG 1.1 (G) was then cultivated in potato dextrose broth (PDB) by incubation for 28 days in dark and static conditions. At the end of the incubation period, the fungal mycelia were separated from the culture medium by filtration through cheesecloth. The biomass was dried in an oven for 48 h. The dried mycelial biomass was ground in liquid nitrogen, and extracted twice with double volume of ethyl acetate. Culture filtrate was also extracted twice with double volume of ethyl acetate. The ethyl acetate fraction of mycelial and culture filtrate extracts was concentrated in a rotary evaporator under reduced pressure at 40°C. The extracts were further concentrated using a speed vacuum chamber. For bioactivity assays, the extracts were dissolved in dimethyl sulfoxide.
Cells were cultured in DMEM supplemented with 10% FBS, 100 mg/l penicillin, 250 mg/l streptomycin and 2 mM glutamine and incubated at 37°C in a humidified chamber with 5% CO2. 100 μl of cells at a density of 1 × 104 cells/well were seeded in 96-well plates and grown for 24 h at 37°C in a 5% CO2 incubator. When cells reached ∼70% confluency, fungal extracts were added at various concentrations, ranging from 10 to 100 μg/ml. After 48 h, MTT solution (10 μl of 5 mg/ml stock prepared in PBS) was added to each well and incubated for 90 min. Thereafter, the media containing MTT was removed, and DMSO (100 μl) was added to each well to dissolve the formazan crystals. The absorbance was measured at 595 nm using a microplate reader (Infinite 200Pro, Tecan Life Science). Cells treated with 0.1% DMSO were kept as a control group.
For viability assay, HeLa cells were seeded at a density of 1 × 105 cells/well in 24 well plates and allowed to grow for 24 h. HeLa cells were then treated with different concentrations of A. unguis mycelial extract (AUME) (10–100 μg/ml) and incubated for 48 h at 37°C. Then cells were harvested using trypsin-EDTA, washed with PBS, and suspended in PBS. 10 μl PI (10 μg/ml) was added to cell suspension just prior to analysis (Kumari et al., 2018). Cells treated with paclitaxel (100 nM) were taken as positive control and untreated cells were taken as negative control. PI fluorescence was acquired with CytoFLEX S flow cytometer (Beckman Coulter, USA) and analyzed by CytExpert 2.0 software.
For cell cycle analysis, HeLa cells (1 × 106) were seeded into 6-well plates in 1 ml DMEM with 10% FBS and allowed to grow for 24 h at 37°C. Then, AUME was added at different concentration (10–100 μg/ml) and incubated for 48 h. Cells were harvested and fixed in cold 70% ethanol for overnight. Then, cells were treated with RNase A for 5 h and stained with PI (50 μg/ml) (Crowley et al., 2016). Cells treated with paclitaxel (100 nM) were taken as positive control and untreated cells as negative control. Cells in different phases were investigated by CytoFLEX S flow cytometer (Beckman Coulter, United States) and data was analyzed by CytExpert 2.0 software.
HeLa cells (1 × 104 cells) were seeded in 24 well plates, incubated for 24 h and treated with AUME at the concentrations of 10, 25, 50, and 100 μg/ml for 48 h. Further, cells were washed with PBS and stained with 2.5 μg/ml of JC-1 dye for 15 min at 37°C in dark and analyzed by flow cytometry (CytoFLEX S, Beckman Coulter, United States). The emission of JC-1 monomers peak at 530 nm (FL-1 channel -green fluorescence) and J-aggregate peaks at 590 nm (FL-2 channel- red fluorescence) were recorded and the data was analyzed by CytExpert 2.0 software. Carbonyl cyanide m-chlorophenyl hydrazone (CCCP 50μM) treated cells were served as positive control and untreated cells were served as negative control (Chazotte, 2011).
Reactive oxygen species (ROS) generated after treatment with AUME were quantified with 2’,7’-dichlorodihydrofluorescein diacetate (DCFH-DA) (Sigma Aldrich, United States). In this assay, HeLa cells (1 × 104 cells) were seeded in 24 well plates, incubated for 24 h and treated with different concentrations of 10, 25, 50, and 100 μg/mL AUME for 48 h. Further, cells were washed with PBS and stained with 50 μM of DCFH-DA for 15 min at 37°C in dark. Fluorescence generated due to oxidation of DCFH was measured by flow cytometry at 500 nm using CytoFLEX S (Beckman Coulter, United States) and analyzed by CytExpert 2.0 software. H2O2 (100 nM) treated cells served as positive control and untreated cells served as a negative control (Eruslanov and Kusmartsev, 2010).
Antioxidative activity of AUME was determined by its ability to scavenge stable 1,1- diphenyl-2-picrylhydrazyl (DPPH) free radical following the protocol of scavenging activity (Herald et al., 2012). Hundred microliter of fungal extract at different concentration (10–100 μg/ml) prepared in methanol was added to 100 μl of 100 μM DPPH prepared in methanol. The mixture was mixed well and incubated in dark for 30 min at room temperature. Absorbance values were measured at 517 nm using a microplate reader (Infinite 200Pro, Tecan Life Science). The scavenging activity of DPPH radicals was calculated by assessing the decrease in purple color of DPPH. Ascorbic acid (10–100 μg/ml) was taken as a positive control and methanol as a negative control.
The GC–MS analysis of bioactive compounds of AUME was done using Agilent Technologies GC systems with GC-7890A/MS-5975C (Agilent Technologies, Santa Clara, CA, United States) equipped with HP-5MS column (30 m length × 250 μm internal diameter × 0.25 μm film thickness). Spectroscopic detection by GC–MS involved electron impact ionization system which utilized high energy electrons (70 eV). Pure helium gas (99.995%) was used as the carrier gas with a flow rate of 1 ml/min. The initial temperature was set at 50–150°C with an increasing rate of 3°C/min and holding time of about 10 min. Finally, the temperature was increased to 250°C at 10°C/min. For derivatization, 20 μl sample was taken in a glass vial and dried with pure N2 gas. 100 μl derivatizing reagent [BSTFA: TMCS (99:1)] was added and incubated at 60°C again for 60 min, derivatized samples were diluted and subjected (1 μl) for GC-MS analysis. All the compounds obtained are trimethylsilyl aducts/derivatives. Derivatized samples were diluted 100 times with ethyl acetate and 1 μl of the derivatized extract was injected into the GC–MS using a microsyringe. The scanning was done at 30–600 m/z range. The compounds were identified by mass library search (AMDIS and NIST, 2011) with their relative retention indices. The relative quantity of the chemical compounds present in the extracts of endophytic fungi was expressed as a percentage based on peak area observed in the chromatogram.
AUME was subjected to liquid chromatography-mass spectrometry (LC-MS/MS) for secondary metabolites profiling. The analysis was performed using Dionex Ultimate 3000 Micro LC instrument fitted with an analytical column, Agilent poroshell 120 (4.6∗150 mm) SB-C18, 2.7 μm particle size and a guard column, Agilent Zorbax Eclipse Plus C8 (4.6∗12.5 mm). The mobile phase consisted of solvent A: Solvent B. Solvent A was 0.2% formic acid in 5 mM ammonium acetate and solvent B was 100% acetonitrile. The column temperature was set to 40°C. The separation was performed using gradient elution with a flow rate of 0.3 ml/min. MS/MS was performed using the instrument ESI-Qtof (Impact HD from Bruker) in the negative mode. Acquisition range was from 50 to 1700 m/z at the spectral Rate of 1 Hz. LC-MS interface used was electrospray ionization (ESI). The sample and mobile phases were filtered through 0.22 μm polyvinylidene fluoride (PVDF) filters before injecting into the column. Metabolites were identified using Metfrag1 and Metlin tandem MS/MS2 databases.
All experiments were carried out at least in triplicates and data obtained presented as means ± SD. All the data were subjected to one-way ANOVA post hoc analysis using the SPSS 11.0 Software. Results were considered significant at p < 0.05 throughout the present study.
Figure 1 shows the growth of A. unguis PDA. On the phylogenetic tree, the isolate was positioned close to A. unguis with 99% similarity on BLAST search. The partial gene sequence has been submitted to GenBank with accession number MH654997 (Kamat et al., 2020).
The MTT assay is based on the cellular reduction of tetrazolium salts to their intensely colored formazans and the amount of formazan produced is proportional to the number of living cells present in culture (Florento et al., 2012). AUME exhibited significant antiproliferative activity against tumor cell lines HeLa, MCF-7, A-431, and COLO-205 in a dose-dependent manner (Figure 2), while culture filtrate had not exhibited any activity (data not shown). This suggested that the bioactive metabolites are mycelial bound. Furthermore, the mycelial extract showed negligible cytotoxicity against normal cell line, HEK 293. The calculated IC50 values ranged from 13.46 ± 0.89 to 19.97 ± 0.21 for different cancer cell lines and > 100 μg/ml for HEK 293 cells, respectively (Table 1). The relative low cytotoxicity of A. unguis derived secondary metabolites against normal cell line HEK 293 makes it an attractive source for an anticancer drug candidate. Since the proliferation of HeLa cells was inhibited to a greater extent, further investigation was performed on the same cell line.
Figure 2. Antiproliferative activity of A. unguis AG 1.1 (G) mycelial extract (AUME) on different cell lines estimated using MTT assay, expressed in percentage growth inhibition. The data shown are average ± SD of values obtained from three replicates, p < 0.05.
To evaluate the performance of secondary metabolites, quantification of the viability of the cell population after treatment with the mycelial extract is necessary. Propidium Iodide is a membrane impermeant dye that stains dead cells. In the presence of AUME, cell viability decreased significantly and followed a dose-response relationship (Figure 3). The dead cell percent raised from 6% in untreated cells to 90% in treated cells with 100 μg/ml AUME, which confirmed the concentration dependent cytotoxicity of AUME.
Figure 3. (A) Cell viability studies of HeLa cells treated with indicated concentration of AUME estimated using PI staining followed by FACS analysis. (B) The bar diagram represents the percentage of dead cells in response to the AUME treatment. The data shown are average ± SD of values obtained from three replicates, p < 0.05, *statistically significant when compared to control.
Cell cycle phase arrest is an extensively observed phenomenon in response to treatment with cytotoxic drugs (Kamat et al., 2020). Figure 4 illustrates the cell cycle distribution of HeLa cells treated with different concentration of AUME. This was then quantified with PI staining and analyzed by FACS. Treatment with AUME resulted in a dose-dependent increase of G0 peak (also called sub-G1), which represents the apoptotic cell population. The percentage of cells in G0 increased from 8% in control group to 9, 31, 44, and 46% in cells treated with 10, 25, 50, and 100 μg/ml AUME, respectively. There was a corresponding decrease in the cell population of other cell cycle phases G1, S, and G2. Hence, the results indicated that AUME induced apoptosis in HeLa cells to a significant extent. In cells treated with paclitaxel, there was a remarkable increase in the G2 phase and G0 phase.
Figure 4. (A) Flow cytometric analysis of HeLa cells treated with indicated concentration of AUME estimated using PI staining. There was an increase in the apoptotic Go phase in a dose-dependent manner. (B) The bar diagram represents the percentage of cells in each phase of cell cycle. Values are given as means ± SD of three replicates in each group, p < 0.05, *statistically significant when compared to control.
One of the significant events that happens during apoptosis is the loss of MMP. Hence, measuring the dynamics of MMP is an effective way to determine apoptosis induction. JC1 is a cationic, lipophilic dye which can be used to assess the mitochondrial membrane potential (ΔΨm). In healthy cells, JC1 enters negatively charged and polarized mitochondria and forms red fluorescent aggregates whereas JC1 monomers do not aggregate and retain their original green fluorescence in apoptotic cells as a result of the loss of MMP (Sivandzade et al., 2019). Treatment with AUME resulted in the loss of mitochondrial membrane potential as measured by the green fluorescence of JC1 monomers, which is shown in the Figure 5. Dose-dependent increase in cells with collapsed mitochondria when treated with AUME revealed the its induction of mitochondria-mediated apoptosis.
Figure 5. (A) Loss of mitochondrial membrane potential by AUME as measured by the percentage of JC-1 monomers. HeLa cells were treated with indicated concentration of AUME for 48 h. After JC1 staining, cells were analyzed by FACS. (B) The bar diagram represents the percentage of loss in MMP in HeLa cells. Values are given as means ± SD of three replicates in each group, p < 0.05, *statistically significant when compared to control.
Quantification of oxidative stress by DCFH makes use of oxidation of non-fluorescent 2’,7’-dihydrodichlorofluorescin diacetate (DCFH-DA) to fluorescent DCF by intracellular ROS (Jarolim et al., 2018). FACS analysis revealed a steady increase in DCF fluorescence in a dose-dependent manner on treatment with AUME at concentrations up to 50 μg/ml (Figure 6A). The ROS production increased from 36.60 to 52.12% at a concertation range from 10 to 50 μg/ml (Figure 6B). However, at higher concentration (100 μg/ml), fluorescence reduced, which could be due to cell death. This revealed that treatment with AUME induced ROS production.
Figure 6. (A) Intracellular ROS generation by AUME. HeLa cells were treated with indicated concentration of AUME for 48 h. DCFH-DA assay was performed to quantify the level of ROS by flow cytometry. (B) The bar diagram represents percentage of ROS produced in HeLa cells. Values are given as means ± SD of three replicates in each group, p < 0.05, *statistically significant when compared to control.
Antioxidant activity of AUME was evaluated by its ability to reduce the free radical DPPH. AUME showed moderate antioxidant activity with an IC50 value of 73.91 ± 0.91 μg/ml (Figure 7). The IC50 value of ascorbic acid was found to be 28.01 ± 0.5 μg/ml (data not given). AUME contains a number of bioactive compounds and some of which could have moderate free radical scavenging activity.
Figure 7. DPPH radical scavenging activity of AUME at different concentrations measured using spectrophotometry. Values are the means ± SD of three replicates, p < 0.05.
The GC-MS analysis was performed to investigate the bioactive compounds present in AUME (Figure 8). Out of 11 compounds detected, an unknown compound had the highest peak area, which did not have a significant match in the NIST database. Other major constituents were fatty acids such as trans-9-octadecenoic acid, hexadecanoic acid and octadecanoic acid, cis-4,7,10,13,16,19-docosahexaenoic acid and oleic acid. Apart from fatty acids, 4-(4-Hydroxy-3,5-dimethoxy-phenyl)-3,4-dihydro-1H-benzo[h]quinolin-2-one and imidazolidinedione derivative were also present. A prostaglandin, prosta-5,13-dien-1-oic acid was also detected on GC-MS chromatogram. Remaining compounds were glycerol and 1,4- benzenedicarboxylic acid. List of metabolites with retention time and area percentage of total are summarized in Table 2.
Figure 9 represents the base peak chromatogram of AUME and fragmentation pattern of the peaks and representative metabolites. Mass-based search and MS/MS spectra of each peak analyzed using Metfrag revealed the presence of some known compounds such as 01-ethyl 04-(2-hydroxyethyl) benzene-1,4-dicarboxylate, diethyl phthalate, asperxanthone, 1-hydroxy-3,5-dimethoxy-2-prenylxanthone, 3-butylidene-7-hydroxyphthalide, and 1,6-dihydroxy-3-methoxy-2-prenylxanthone. Only highest scoring MetFrag candidates were chosen, which were further cross-checked by Metlin database search. 13 out of 19 peaks were not tentatively identified as they had come up with zero hits of natural products in mass search. Table 3 summarizes LC-MS/MS result.
Figure 9. (A) Base peak chromatogram of AUME analyzed by LC-MS/MS. (B) Fragmentation pattern of the peaks and representative metabolites.
Table 3. List of secondary metabolites in AUME with retention time and MetFrag score identified by LC-MS/MS analysis.
The endophytic community of macroalgae is underexplored due to the difficulty in sampling and isolation. The complex and dynamic relationship between algicolous endophytic fungi and its host determines the production of secondary metabolites. There is a large number of secondary metabolites discovered so far serving many roles. These compounds contribute to the adaptive or defensive mutualism against biotic and abiotic stress incurred by algae. In addition to this, horizontal gene transfer between algae and endophytic fungi can result in the production of host secondary metabolites by endophytes. Secondary metabolites possess unique structural features, which contribute to the various bioactivities such as antibacterial, antifungal, antiviral, antitumor, antioxidant, anti-inflammatory, anticoagulant, antidiabetic activity and so forth (Tadjch and White, 2012; Barzkar et al., 2019). Furthermore, these molecules can act as the structural scaffolds for synthetic chemistry to develop more potent and efficient therapeutics.
The chemotherapeutic potential of the compounds can be evaluated by in vitro cell-based cytotoxicity assay such as MTT, which is quite rapid and reliable. The potency and effectiveness of anticancer agents are determined by half-maximal inhibitory concentration (IC50 value) derived from the dose-response curve. Furthermore, the anticancer compound should be selective between cancerous and non-cancerous cells in the context of cytotoxicity (Popiołkiewicz et al., 2005). AUME treatment demonstrated significantly higher cytotoxicity (IC50-13.46 μg/ml) in HeLa cells in comparison with other tested cell lines, while exhibiting low cytotoxicity on normal cells. Kamat et al. (2020) reported that total culture crude extract of A. unguis AG 1.2 exhibited high cytotoxicity in A431 cell with an IC50 value of 5.94 μg/ml. Both studies inferred that macroalgal derived A. unguis could be a principal source of cytotoxic secondary metabolites.
Cytotoxicity of AUME was further validated by PI live dead assay. Estimation of cell survival using flow cytometry to assess the effect of the chemotherapeutic agent on the cancer cells is a facile and relatively rapid method and is based on the cellular fluorescence after staining with fluorescent dyes (Ross et al., 1989). PI live dead assay result confirmed the cytotoxic potential of AUME. To elucidate the cellular mechanism by which extract exerts anticancer activity, the effect of AUME on cell cycle progression, mitochondrial membrane potential and intracellular ROS production was also studied.
The noteworthy observation on cell cycle analysis was the presence of dose-dependently increasing Go or sub-G1 peak, which indicated the occurrence of apoptosis brought about by the treatment with AUME. Darzynkiewicz et al. (2010) reported that apoptotic cell population is manifested as the sub-G1 peak on DNA content histogram, due to the lowest DNA content. The cell cycle arrest at G2/M phase leading to apoptosis is one of the mechanisms by which paclitaxel exerts cytotoxicity (Wang et al., 2000). In comparison with paclitaxel, it was observed that AUME treatment increased the number of cells in Go phase, while decreased the number of cells in G1, S, and G2/M phases. A similar observation of apoptotic phase increase was reported in leukemic cells when treated with Greensporone C, a fungal derived secondary metabolite (Prabhu et al., 2018). A. unguis AG 1.2 total culture crude extract exhibited G1 phase cell cycle arrest in A431 cells (Kamat et al., 2020). Anticancer agents induce cell death by either causing DNA lesions or hamper cell division mechanisms, leading to the activation of DNA damage checkpoints, eventually causing cell cycle arrest followed by cell death (Visconti et al., 2016).
Our results suggest that AUME exerts the cytotoxic effect on HeLa cells by inducing mitochondrial-mediated apoptosis. The role of MMP in the induction of apoptosis is still unclear as there are a lot of conflicting results suggesting that loss of ΔΨm can trigger apoptosis or ΔΨm can be dissipated as a consequence of apoptosis (Ly et al., 2003). Still, loss of ΔΨm is a key step in apoptosis. Our results confirmed that AUME was causing loss of ΔΨm and induced apoptosis.
We have further demonstrated that AUME triggered oxidative stress. Most of the anticancer agents induce ROS, which leads to oxidative-stress mediated cell death (Singh et al., 2016). ROS are highly reactive molecules, serving a number of crucial roles at a low to moderate concentration, particularly in regulations of physiological functions and maintaining a normal immune system. Excess intracellular ROS induces damage to nucleic acids, proteins, lipids, membranes and organelles leading to the activation of apoptosis, which is mediated by mitochondria, death receptors and the ER signaling pathway (Redza-Dutordoir and Averill-Bates, 2016). Additionally, cancer stem cell maintains a low level of intracellular reactive oxygen species (ROS) level to maintain its survival and proliferation during tumorigenesis. Increasing oxidative stress to cause ROS-mediated cell injury by the exogenous agent is an effective anticancer therapy, which selectively kills cancer cells without affecting normal cells (Liu and Wang, 2015). The molecular mechanism of antiproliferative activity of endophytic fungal derived cytotoxic secondary metabolites could be by the induction of apoptosis through ROS dependent MMP loss. Similar observations were reported from macroalgal associated endophytes isolated from the Indian coastline (Kumari et al., 2018; Kamat et al., 2020). Aspergillus protuberus and Talaromyces flavus, marine derived fungi isolated from Indian coastline also showed cytotoxicity and antibacterial activity (Mathan et al., 2011; Anand et al., 2016).
Though reactive oxygen species (ROS) are an integral part of the intracellular signaling pathway, high ROS production is usually deleterious and involved in inflammatory diseases (Henrotin et al., 2003). It has been shown that antioxidant supplementation can alleviate oxidative damage caused by high ROS production (Liu et al., 2018). Metabolites produced by endophytic fungi can be a potential source of novel natural antioxidants (Huang et al., 2007). Many synthetic analogs of natural compounds show potent antioxidant activity. The synthetic analog of the metabolite derived from fungus Colletotrichum gloeosporioides exhibits excellent free radical scavenging activity which is comparable to protocatechuic acid (Femenía-Ríos et al., 2006). Various biotic and abiotic stresses in plants generate ROS and adversely affect plant growth. Endophytes have the ability to alleviate the drought and metal stress by modulating ROS scavenging system and thus improving the plant viability (Ma et al., 2019; Sadeghi et al., 2020). A diverse array of plants has been in a symbiotic relationship with endophytes. Secondary metabolites produced by endophytes heavily contribute to this mutualistic relationship through herbivore and oxidative stress protection (White and Torres, 2010). In comparison with the antioxidant activity of the total culture crude extract reported by Kamat et al. (2020), a better antioxidant activity of AUME revealed the mycelial origin of antioxidant metabolites.
Metabolic profiling is critical to identify the bioactive compounds contributing for the interesting biological activities exhibited by A. unguis AG 1.1 (G). GC-MS and LC-MS/MS are robust dereplicatory tools for metabolite identification. GC-MS analysis performed on AUME revealed the presence of therapeutically important compounds such as trans-9-Octadecenoic acid, hexadecanoic acid, octadecanoic acid, 4-(4-Hydroxy-3,5-dimethoxy-phenyl)-3,4-dihydro-1H-benzo[h]quinolin-2-one, imidazolidinedione derivative, 1,4-benzene dicarboxylic acid and a prostaglandin, prosta-5,13-dien-1-oic acid. A critical observation was the presence of a major peak of an unknown compound. Octadecanoid acid and hexadecenoic acid exhibit antiproliferative activity, antioxidant activity, and anti-microbial activities (Habib et al., 1987; Ravi and Krishnan, 2017; Ashraf et al., 2018). In our study, the presence of above-mentioned fatty acids in A. unguis mycelium could be greatly contributing to its antioxidant activity. A number of compounds belonging to 5-hydroxy-4-aryl-quinolin-2(1H)-one alkaloids are reported from Aspergillus and Pencillium species. A prenylated quinoline, aspoquinolones produced by Aspergillus species exhibited good cytotoxicity due to isoprenyl derived sidechain in the structure (Uchida et al., 2006; Simonetti et al., 2016). 5-methyl- 2,4-imidazolidinedione was detected in the bioactive fraction of Lactobacillus plantarum VTTE-78076, contributing to its antibacterial activity against Gram negative test organism (Niku-Paavola et al., 1999). In our previous study, we observed that A. unguis AG 1.1 (G) ethyl acetate extract demonstrated antimicrobial activity against Gram negative bacterial pathogens, E. coli, P. aeruginosa, and X. campestris (Kamat et al., 2020). A high concentration of 1,2-benzene dicarboxylic acid mono(2-ethylhexyl) ester contributed to the antifungal activity of the endophytic fungi, Aspergillus flavipes strain (Verma et al., 2014). Many pathogenic fungi have the ability to produce prostaglandins that act as virulence factors (Noverr et al., 2002). Due to the fact that bioactive compounds such as trans-9-Octadecenoic acid, hexadecanoic acid, and octadecanoic acid are present at relatively low concentration and are less cytotoxic, we hypothesize that the bioactive compounds, 4-(4-Hydroxy-3,5-dimethoxy-phenyl)-3,4-dihydro-1H-benzo[h]quinolin-2-one or the unknown compound present in the mycelial extract of A. unguis may be directly involved in the strong anticancer activity that we have observed. GC-MS analysis of cytotoxic A. unguis AG 1.2 total culture crude extract detected the presence of azelaic acid, azetidine and furopyrans, which could be contributing to the excellent cytotoxicity (Kamat et al., 2020). The metabolic profile of A. unguis AG 1.1 (G) was quite distinct from that of A. unguis AG 1.2, though both belong to the same species, demonstrated significant cytotoxicity and were isolated from the same algal sample. This reveals that secondary metabolite diversity of A. unguis species is strain specific. Therefore, it is necessary to investigate the natural productome of each fungal isolate with respect to its potential bioactivity.
LC-MS/MS analysis revealed that A. ungis AG 1.1 (G) is capable of producing another phthalate acid ester, O1-ethyl O4-(2-hydroxyethyl) benzene-1,4-dicarboxylate and diethyl phthalate, apart from 1,2-benzene dicarboxylic acid mono(2-ethylhexyl) ester. A few filamentous fungi have been reported to produce phthalate acid esters (Tian et al., 2016). Asperxanthone, a difuraxanthone reported from marine-derived Aspergillus species exhibited moderate activity against tobacco mosaic virus (Wu et al., 2009). Some prenylxanthone analogs with various bioactive functions such as antibacterial, antifungal and cytotoxic activities have been reported from the Aspergillus species (Zhu et al., 2018). Butylidenephthalide, present in many medicinal herbs possesses various bioactivities including antitumor activity (Chang et al., 2020). A cytotoxic 3- butyl-7-hydroxyphthalide was reported from Penicillium vulpinum (Ohseki and Mori, 2003). The production of 3-butylidene-7-hydroxyphthalide has not been reported from a fungal source to the best of our knowledge. Based on LC-MS/MS results, 1-hydroxy-3,5-dimethoxy-2-prenylxanthone, 1,6-dihydroxy-3-methoxy-2-prenylxanthone and 3-butylidene-7-hydroxyphthalide in AUME are presumed to contribute to the cytotoxic activity exhibited by AUME, owing to the known bioactivity of their related metabolites. Additionally, many unknown metabolites are also present, which may be contributing to the cytotoxicity of AUME. Hence, bioassay-guided purification followed by the experimental verification can only pinpoint the molecule possessing excellent cytotoxic activity of AUME in view of developing an anticancer drug candidate. Though numerous bioactive secondary metabolites have been identified from endophytic fungi, lack of their MS spectra in the public databases is a major constraint in the dereplication of fungal secondary metabolite. Availability of an open natural product database with all relevant chemical and taxonomic information could facilitate the bioprospecting of novel natural products (Nielsen and Larsen, 2015; Zhou et al., 2013).
In conclusion, the mutualistic relationship of an endophytic fungus with its host influences the secondary metabolite diversity of the fungus. Macroalgal derived A. unguis could be a principal source of cytotoxic secondary metabolites. Even the identical endophytic fungal species isolated from a common source can have a unique set of therapeutically significant compounds, which empowers the diversity of marine natural productome. Induction of apoptosis by markedly increasing the intracellular ROS production and severely aberrating the mitochondrial function could be the major molecular mechanism behind the cytotoxic activity of A. unguis derived secondary metabolites. The strong cytotoxicity of AUME warrants further investigation to purify and characterize the cytotoxic compounds with the prospect of developing novel anticancer therapeutics. Our study highlights the importance of bioprospecting of macroalgal derived fungal endophytic community for the discovery of novel anticancer therapeutics.
The datasets generated for this study can be found in GenBank with accession number MH654997.
KS carried out the designed research, collected, analyzed, and interpreted the scientific data, wrote the article. SK did the sampling, isolation and identification of the fungus, analyzed and interpreted the data, made critical revisions, and edited the article. CJ designed the study and did the supervision, project administration, and funding acquisition. All authors read and approved the final manuscript.
This project was funded by the Department of Biotechnology (Ref. no. BT/PR/14569).
The authors declare that the research was conducted in the absence of any commercial or financial relationships that could be construed as a potential conflict of interest.
All authors would like to acknowledge IISc for all the infrastructure and the support. All authors acknowledge FACS and GC-MS facility of IISc. KS would like to acknowledge Dr. D.S. Kothari Post-doctoral Fellowship Scheme of University Grant Commission, New Delhi, India for the fellowship. SK would like to acknowledge University Grant Commission, New Delhi, India for the senior research fellowship.
Abdel-Lateff, A., Klemke, C., König, G. M., and Wright, A. D. (2003). Two new xanthone derivatives from the algicolous marine fungus Wardomyces anomalus. J. Nat. Prod. 66, 706–708. doi: 10.1021/np020518b
Anand, B. G., Thomas, C. N., and Prakash, S. (2016). In vitro cytotoxicity and antimicrobial activity of Talaromyces flavus SP5 inhabited in the marine sediment of Southern Coast of India. Chin. J. Nat. medicines. 14, 913–921. doi: 10.1016/s1875-5364(17)30016-x
Ashraf, I., Zubair, M., Rizwan, K., Rasool, N., Jamol, M., Khan, S. A., et al. (2018). Chemical composition, antioxidant and antimicrobial potential of essential oils from different parts of Daphne mucronata Royle. Chem. Cent. J. 12:135. doi: 10.1186/s13065-018-0495-1
Barzkar, N., Jahromi, S. T., Poorsaheli, H. B., and Vianello, F. (2019). Metabolites from marine microorganisms, micro, and macroalgae: immense scope for pharmacology. Mar. Drugs 17:464. doi: 10.3390/md17080464
Bray, F., Ferlay, J., Soerjomataram, I., Siegel, R. L., Torre, L. A., and Jemal, A. (2018). Global cancer statistics 2018: GLOBOCAN estimates of incidence and mortality worldwide for 36 cancers in 185 countries. CA Cancer J. Clin. 68, 394–424. doi: 10.3322/caac.21492
Chang, K. F., Chang, J. T., Huang, X. F., Lin, Y. L., Liao, K. W., Huang, C. W., et al. (2020). Antitumor effects of N-butylidenephthalide encapsulated in lipopolyplexs in colorectal cancer cells. Molecules 25:2394. doi: 10.3390/molecules25102394
Chazotte, B. (2011). Labeling mitochondria with JC-1. Cold Spring Harb. Protoc. 2011:pdb.prot065490. doi: 10.1101/pdb.prot065490
Crowley, L. C., Chojnowski, G., and Waterhouse, N. J. (2016). Measuring the DNA content of cells in apoptosis and at different cell-cycle stages by propidium iodide staining and flow cytometry. Cold Spring Harb. Protoc. 10:087247. doi: 10.1101/pdb.prot087247
Darzynkiewicz, Z., Halicka, H. D., and Zhao, H. (2010). Analysis of cellular DNA content by flow and laser scanning cytometry. Adv. Exp. Med. Biol. 676, 137–147. doi: 10.1007/978-1-4419-6199-0_9
Eruslanov, E., and Kusmartsev, S. (2010). “Identification of ROS using oxidized DCFDA and flow-cytometry,” in Advanced Protocols in Oxidative Stress II. Methods in Molecular Biology (Methods and Protocols), ed. D. Armstrong (Totowa, NJ: Humana Press), 57–72. doi: 10.1007/978-1-60761-411-1_4
Femenía-Ríos, M., García-Pajón, C. M., Hernández-Galán, R., Macías-Sánchez, A. J., and Collado, I. G. (2006). Synthesis and free radical scavenging activity of a novel metabolite from the fungus Colletotrichum gloeosporioides. Bioorg. Med. Chem. Lett. 16, 5836–5839. doi: 10.1016/j.bmcl.2006.08.071
Florento, L., Matias, R., Tuaño, E., Santiago, K., Dela Cruz, F., and Tuazon, A. (2012). Comparison of cytotoxic activity of anticancer drugs against various human tumor cell lines using in vitro cell-based approach. Int. J. Biomed. Sci. 8, 76–80.
Habib, N. A., Wood, C. B., Apostolov, K., Barker, W., Hershman, M. J., Aslam, M., et al. (1987). Stearic acid and carcinogenesis. Br. J. Cancer. 56, 455–458. doi: 10.1038/bjc.1987.223
Henrotin, Y. E., Bruckner, P., and Pujol, J. P. (2003). The role of reactive oxygen species in homeostasis and degradation of cartilage. Osteoarthritis Cartilage 11, 745–755.
Herald, T. J., Gadgil, P., and Tilley, M. (2012). High-throughput micro plate assays for screening flavonoid content and DPPH-scavenging activity in sorghum bran and flour. J. Sci. Food Agric. 92, 2326–2331. doi: 10.1002/jsfa.5633
Huang, W.-Y., Cai, Y.-Z., Xing, J., Corke, H., and Sun, M. (2007). A potential antioxidant resource: endophytic fungi from medicinal plants. Econ. Bot. 61, 14–30. doi: 10.1663/0013-0001(2007)61[14:aparef]2.0.co;2
Jarolim, K., Wolters, K., Woelflingseder, L., Pahlke, G., Beisl, J., Puntscher, H., et al. (2018). The secondary fusarium metabolite aurofusarin induces oxidative stress, cytotoxicity and genotoxicity in human colon cells. Toxicol. Lett. 284, 170–183. doi: 10.1016/j.toxlet.2017.12.008
Kamat, S., Kumari, M., Tartitla, S., and Jayabaskaran, C. (2020). Endophytic fungi of marine alga from Konkan coast, India -a rich source of bioactive material. Front. Mar. Sci. 7:31. doi: 10.3389/fmars.2020.00031
Kumari, M., Taritla, S., Sharma, A., and Jayabaskaran, C. (2018). Antiproliferative and antioxidative bioactive compounds in extracts of marine-derived endophytic fungus Talaromyces purpureogenus. Front. Microbiol. 9:1777. doi: 10.3389/fmicb.2018.01777
Kusari, S., Lamshöft, M., and Spiteller, M. (2009). Aspergillus fumigatus Fresenius, an endophytic fungus from Juniperus communis L. horstmann as a novel source of the anticancer pro-drug deoxypodophyllotoxin. J. Appl. Microbiol. 10, 1019–1030. doi: 10.1111/j.1365-2672.2009.04285.x
Kusari, S., Singh, S., and Jayabaskaran, C. (2014). Rethinking production of Taxol® (paclitaxel) using endophyte. Trends Biotechnol. 32, 304–311. doi: 10.1016/j.tibtech.2014.03.011
Liu, J., and Wang, Z. (2015). Increased oxidative stress as a selective anticancer therapy. Oxid. Med. Cell Longev. 2015:294303. doi: 10.1155/2015/294303
Liu, Z., Ren, Z., Zhang, J., Chuang, C. C., Kandaswamy, E., Zhou, T., et al. (2018). Role of ROS and nutritional antioxidants in human diseases. Front Physiol. 17:477. doi: 10.3389/fphys.2018.00477
Ly, J. D., Grubb, D. R., and Lawn, A. (2003). The mitochondrial membrane potential (Δψm) in apoptosis; an update. Apoptosis 8, 115–128.
Ma, L., Li, X., Wang, L., Li, Y., Ning, B., and Cuimei, Y. (2019). Endophytic infection modulates ROS scavenging systems and modifies cadmium distribution in rice seedlings exposed to cadmium stress. Theor. Exp. Plant Physiol. 31:463. doi: 10.1007/s40626-019-00159-5
Ma, W. W., and Adjei, A. A. (2009). Novel agents on the horizon for cancer therapy. CA Cancer J. Clin. 59, 111–137. doi: 10.3322/caac.20003
Mansoori, B., Mohammadi, A., Davudian, S., Shirjang, S., and Baradaran, B. (2017). The different mechanisms of cancer drug resistance: a brief review. Adv. Pharm. Bull. 7, 339–348. doi: 10.15171/apb.2017.041
Mathan, S., Smith, A. A., Kumaran, J., and Prakash, S. (2011). Anticancer and antimicrobial activity of Aspergillus protuberus SP1 isolated from marine sediments of South Indian coast. Chin. J. Nat. medicines. 9, 286–292. doi: 10.1016/s1875-5364(11)60066-6
Nielsen, K. F., and Larsen, T. O. (2015). The importance of mass spectrometric dereplication in fungal secondary metabolite analysis. Front.Microbiol. 6:71. doi: 10.3389/fmicb.2015.00071
Niku-Paavola, M. L., Laitila, A., Mattila-Sandholm, T., and Haikara, A. (1999). New types of antimicrobial compounds produced by Lactobacillus plantarum. J. Appl. Microbiol. 86, 29–35. doi: 10.1046/j.1365-2672.1999.00632.x
Noverr, M. C., Toews, G. B., and Huffnagle, G. B. (2002). Production of prostaglandins and leukotrienes by pathogenic fungi. Infect Immun. 70, 400–402. doi: 10.1128/iai.70.1.400-402.2002
Ohseki, T., and Mori, K. (2003). Synthesis and absolute configuration of (-)-3-Butyl-7-hydroxyphthalide, a cytotoxic metabolite of Penicillium vulpinum. Biosci. Biotechnol. Biochem. 67, 2240–2244. doi: 10.1271/bbb.67.2240
Popiołkiewicz, J., Polkowski, K., Skierski, J., and Mazurek, A. P. (2005). In vitro toxicity evaluation in the development of new anticancer drugs-genistein glycosides. Cancer Lett. 229, 67–75. doi: 10.1016/j.canlet.2005.01.014
Prabhu, K. S., Siveen, K. S., Kuttikrishnan, S., Iskandarani, A. N., Khan, A. Q., and Merhi, M. (2018). Greensporone C, a freshwater fungal secondary metabolite induces mitochondrial-mediated apoptotic cell death in leukemic cell lines. Front. Pharmacol. 9:720. doi: 10.3389/fphar.2018.00720
Pusztahelyi, T., Holb, I. J., and Pócsi, I. (2015). Secondary metabolites in fungus-plant interactions. Front. Plant Sci. 6:573. doi: 10.3389/fpls.2015.00573
Ravi, L., and Krishnan, K. (2017). Cytotoxic potential of N-hexadecanoic acid extracted from Kigelia pinnata leaves. Asian J. Cell Biol. 12, 20–27. doi: 10.3923/ajcb.2017.20.27
Rayan, A., Raiyn, J., and Falah, M. (2017). Nature is the best source of anticancer drugs: indexing natural products for their anticancer bioactivity. PLoS One 12:e0187925. doi: 10.1371/journal.pone.0187925
Redza-Dutordoir, M., and Averill-Bates, D. A. (2016). Activation of apoptosis signalling pathways by reactive oxygen species. Biochim. Biophys. Acta 1863, 2977–2992. doi: 10.1016/j.bbamcr.2016.09.012
Ross, D. D., Joneckis, C. C., Ordonez, J. V., Sisk, A. M., Wu, R. K., Hamburger, A. W., et al. (1989). Estimation of cell survival by flow cytometric quantification of fluorescein diacetate/propidium iodide viable cell number. Cancer Res. 49, 3776–3782.
Sadeghi, F., Samsampour, D., Sevahooei, M. A., Bagheri, A., and Soltani, J. (2020). Fungal endophytes alleviate drought-induced oxidative stress in mandarin (Citrus reticulata L.): toward regulating the ascorbate–glutathione cycle. Sci. Hort. 261:108991. doi: 10.1016/j.scienta.2019.108991
Seelinger, M., Popescu, R., Giessrigl, B., Jarukamjorn, K., Unger, C., Wallnofer, B., et al. (2012). Methanol extract of the ethnopharmaceutical remedy Smilax spinosa exhibits anti-neoplastic activity. Int. J. Oncol. 41, 1164–1172. doi: 10.3892/ijo.2012.1538
Simonetti, S. O., Larghi, E. L., and Kaufman, T. S. (2016). The 3,4-dioxygenated 5-hydroxy-4-aryl-quinolin-2(1H)-one alkaloids. Results of 20 years of research, uncovering a new family of natural products. Nat. Prod. Rep. 33, 1425–1446. doi: 10.1039/c6np00064a
Singh, A. K., Singh, S., Garg, G., and Rizvi, S. I. (2016). Rapamycin alleviates oxidative stress-induced damage in rat erythrocytes. Biochem. Cell Biol. 94, 471–479. doi: 10.1139/bcb-2016-0048
Sivandzade, F., Bhalerao, A., and Cucullo, L. (2019). Analysis of the mitochondrial membrane potential using the cationic JC-1 dye as a sensitive fluorescent probe. Biol. Protoc. 9:e3128. doi: 10.21769/BioProtoc.3128
Tadjch, M., and White, J. F. (2012). “Endophyte Microbes,” in Topics in Ecological and Environmental Microbiology, eds T. M. Schmidt and M. Schaechter (Amsterdam: Elsevier), 133–146.
Tian, C., Ni, J., Chang, F., Liu, S., Xu, N., Sun, W., et al. (2016). Bio-Source of di-n-butyl phthalate production by filamentous fungi. Sci. Rep. 6:19791. doi: 10.1038/srep19791
Uchida, R., Imasato, R., Tomoda, H., and Omura, S. (2006). Yaequinolones, new insecticidal antibiotics produced by Penicillium sp. FKI-2140. II. Structural elucidation. J. Antibiot. 59, 652–658. doi: 10.1038/ja.2006.87
Vallet, M., Strittmatter, M., Murúa, P., Lacoste, S., Dupont, J., Hubas, C., et al. (2018). Chemically-mediated interactions between macroalgae, their fungal endophytes, and protistan pathogens. Front. Microbiol. 9:3161. doi: 10.3389/fmicb.2018.03161
Verma, A., Johri, B. N., and Prakash, A. (2014). Antagonistic evaluation of bioactive metabolite from endophytic fungus, Aspergillus flavipes KF671231. J. Mycol. 2014:371218. doi: 10.1155/2014/371218
Visconti, R., Della Monica, R., and Grieco, D. (2016). Cell cycle checkpoint in cancer: a therapeutically targetable double-edged sword. J. Exp. Clin. Cancer Res. 35:153.
Wang, T. H., Wang, H. S., and Soong, Y. K. (2000). Paclitaxel-induced cell death: where the cell cycle and apoptosis come together. Cancer 88, 2619–2628. doi: 10.1002/1097-0142(20000601)88:11<2619::aid-cncr26>3.0.co;2-j
White, J. F. Jr., and Torres, M. (2010). Is plant endophyte-mediated defensive mutualism the result of oxidative stress protection? Physiol. Plant 138, 440–446. doi: 10.1111/j.1399-3054.2009.01332.x
Wu, Z. J., Ouyang, M. A., and Tan, Q. W. (2009). New asperxanthone and asperbiphenyl from the marine fungus Aspergillus sp. Pest Manag. Sci. 65, 60–65. doi: 10.1002/ps.1645
Zeilinger, S., Gupta, V. K., Dahms, T. E., Silva, R. N., Singh, H. B., Upadhyay, R. S., et al. (2016). Friends or foes? Emerging insights from fungal interactions with plants. FEMS Microbiol. Rev. 40, 182–207. doi: 10.1093/femsre/fuv045
Zhao, C., Yang, C., and Liu, B. (2016). Biological activities of green macroalgae Enteromorpha prolifera for potential applications. MOJ Food Process. Technol. 2, 153–155. doi: 10.15406/mojfpt.2016.02.00048
Zhao, K., Ping, W., Li, Q., Hao, S., Zhao, L., Gao, T., et al. (2009). Aspergillus niger var. taxi, a new species variant of taxol-producing fungus isolated from Taxus cuspidata in China. J. Appl. Microbiol. 107, 1202–1207. doi: 10.1111/j.1365-2672.2009.04305.x
Zhou, B., Xiao, J. F., and Ressom, H. W. (2013). Prioritization of putative metabolite identifications in LC-MS/MS experiments using a computational pipeline. Proteomics 13, 248–260. doi: 10.1002/pmic.201200306
Keywords: secondary metabolites, anticancer, Aspergillus unguis, GC-MS, LC-MS/MS, endophytes
Citation: Sajna KV, Kamat S and Jayabaskaran C (2020) Antiproliferative Role of Secondary Metabolites From Aspergillus unguis AG 1.1 (G) Isolated From Marine Macroalgae Enteromorpha sp. by Inducing Intracellular ROS Production and Mitochondrial Membrane Potential Loss Leading to Apoptosis. Front. Mar. Sci. 7:543523. doi: 10.3389/fmars.2020.543523
Received: 17 March 2020; Accepted: 12 August 2020;
Published: 28 August 2020.
Edited by:
Periasamy Anbu, Inha University, South KoreaReviewed by:
Bin Wu, Zhejiang University, ChinaCopyright © 2020 Sajna, Kamat and Jayabaskaran. This is an open-access article distributed under the terms of the Creative Commons Attribution License (CC BY). The use, distribution or reproduction in other forums is permitted, provided the original author(s) and the copyright owner(s) are credited and that the original publication in this journal is cited, in accordance with accepted academic practice. No use, distribution or reproduction is permitted which does not comply with these terms.
*Correspondence: Chelliah Jayabaskaran, Y2piQGlpc2MuYWMuaW4=
Disclaimer: All claims expressed in this article are solely those of the authors and do not necessarily represent those of their affiliated organizations, or those of the publisher, the editors and the reviewers. Any product that may be evaluated in this article or claim that may be made by its manufacturer is not guaranteed or endorsed by the publisher.
Research integrity at Frontiers
Learn more about the work of our research integrity team to safeguard the quality of each article we publish.