- 1Kepley Biosystems Incorporated, Greensboro, NC, United States
- 2Department of Nanoscience, Joint School of Nanoscience and Nanoengineering, University of North Carolina at Greensboro, Greensboro, NC, United States
- 3ClienTell® Consulting, LLC, The Valley, Anguilla
- 4Archer Daniels Midland Company, Decatur, IL, United States
- 5Ontario Veterinary College, University of Guelph, Guelph, ON, Canada
- 6Department of Nanoengineering, Joint School of Nanoscience and Nanoengineering, North Carolina A&T State University, Greensboro, NC, United States
The Limulus amebocyte lysate (LAL) isolated from cells in the horseshoe crab (HSC) hemolymph is a critical resource for global biomedical and pharmaceutical quality control and sterility testing. Given the necessity of and limitations associated with wild capture, a conservational approach to LAL harvesting would benefit the medical community that relies on the raw material while helping ensure species viability. We posited that aquaculture and year-round collection represented a sustainable alternative for the production of LAL from a finite HSC cohort, thereby averting the impact of current practices on wild populations. Given the specter of captivity diseases linked to diet, such as panhypoproteinemia, this work, at the outset, focused on optimizing a feed formulation to ensure animal vitality. In turn, each preparation required evaluation with respect to effects on LAL, as well as vital HSC health markers, so as to meet or exceed industry requirements and establish a new supply chain paradigm. In this controlled husbandry study, we conducted three 8-week feeding trials and demonstrated a ∼7-fold LAL reactivity range among the HSC feed groups. Relative protein abundance patterns of HSC amebocyte clotting factors (i.e., Factor C, Factor B, and proclotting enzyme) were influenced by diet in particular, and the up-regulation of specific LAL factors correlated with enhanced reactivity. These results also cite the discovery that coagulation Factor C, the LPS-sensitive serine protease proenzyme, may be a phosphoprotein.
Introduction
Marine pharmacognosy focuses on the development of clinically significant products obtained from marine species (Malve, 2016). A common limitation of this sector is the lack of viable supplies of unique compounds (Lindequist, 2016). In recent decades, aquaculture has advanced significantly and currently plays a prominent role in global food and environmental sustainability. Similarly, aquaculture represents an opportunity to establish a reliable source for production of marine-derived biomedical compounds.
The HSC, Limulus polyphemus, is one such marine species that has been utilized by pharmaceutical and device manufacturers, worldwide (Krisfalusi-Gannon et al., 2018; Bolden, 2019). Annually, some 600,000 Atlantic HSCs are captured and bled to meet the demand for Limulus amebocyte lysate (LAL) testing (Atlantic States Marine Fisheries Commission, 2019). The assay is the primary FDA-approved method for bacterial endotoxin testing (BET) of biomedical products and devices (Wachtel and Tsuji, 1977; Hochstein, 1990; Bryans et al., 2004; Novitsky, 2009; Food and Drug Administration, 2014). Aquaculture husbandry represents a sustainable alternative to wild-capture bleeding practices associated with an estimated 30% mortality rate (Anderson et al., 2013). Establishing a finite cohort (∼5–10% of one year’s catch) of carefully monitored and consistently nourished HSCs in managed habitats would allow year-round, low-impact harvesting to meet current LAL demand for the majority of the typical HSC life expectancy of up to ∼20 years while helping to replenish wild populations (Grady and Valiela, 2006).
Derived from HSC blood cells (amebocytes), LAL is exceptionally sensitive (parts per trillion) to lipopolysaccharides (LPS). Also known as endotoxin, LPS is a constituent of the cell wall of gram-negative bacteria (Roslansky and Novitsky, 1991). Activation of the LAL pathway results in cleavage of three serine protease zymogens: Factor C, Factor B and proclotting enzyme, as well as the secretion of transglutaminase (TGase) from the amebocyte cytosol (Osaki et al., 2002; Osaki and Kawabata, 2004). Endotoxins trigger autocatalytic activation of Factor C zymogen into its active form, which in turn activates Factor B in an LPS-dependent manner, converting the proclotting enzyme to the clotting enzyme that cleaves coagulogen (Iwanaga et al., 1992, 1998; Muta and Iwanaga, 1996; Kawabata and Muta, 2010; Kobayashi et al., 2015). The endpoint is the conversion of coagulogen into coagulin, an altered fibrin-like protein gel (Iwanaga, 2007). In a Ca++-dependent pathway, TGase helps to stabilize the clot by cross-linking coagulin with two other proteins, proxin and stablin (Osaki et al., 2002; Matsuda et al., 2007).
Previous studies have determined the diet and food preferences of HSCs in their natural habitat, but there is relatively little information available regarding the diet of HSCs in aquaculture, with most captive diets composed of a natural feed sources, such as shrimp, krill, mussels, sprat, sand eels, squid, mackerel, and trash fish (Botton, 1984). Three decades of adult HSC aquaculture have yet to define optimal parameters required for long-term management in captivity (Carmichael and Brush, 2012). Sustained aquaculture (exceeding 6 months) has been associated with low survival rates, principally linked to poor water quality (due to high stocking density) and inadequate nutrition, both of which place the HSCs under stress and increase their susceptibility to pathogens and vulnerability to captivity-induced disease (Kautsky, 1982; Smith and Berkson, 2005; Defoirdt et al., 2007; Carmichael et al., 2009; Nolan and Smith, 2009; Schreibman and Zarnoch, 2009; Kwan et al., 2014). Specifically, the most notable malady of captive adult HSCs has been panhypoproteinemia; whereby, hemolymph protein concentrations drop below 3.4–11.7 mg/mL after 3–4 months (Nolan and Smith, 2009). This syndrome is thought to be caused by nutritional deficiencies and results in protein-losing enteropathy and nephropathy, as well as hepatic insufficiency (Nolan and Smith, 2009).
Studies of HSC feed formulations have also been largely lacking, defaulting to costly natural resources (e.g., fish, crustaceans, and mollusks) for short-term indoor research. Many factors affect the nutrient composition of natural feeds, further complicating nutritional parameters and requiring frequent adjustment. In the wild, most aquatic animals are opportunistic and consume a diverse array of prey. However, captive diets are typically limited in complexity due to species availability and consist of frozen and thawed bivalves, cephalopods, or pelagic fish, often exposed to prolonged storage. Freezing and thawing natural feeds can also cause water-soluble vitamin loss through diffusion at erratic, non-linear rates (Rigdon and Drager, 1955; Dierenfeld et al., 1991).
Alternatively, synthetic options provide affordable and nutritionally defined aquaculture feedstocks. Manufactured feed is typically formulated for the type and life stage of each species. To date, however, HSC-specific commercial feed has not been developed, and the suitability of adapting current feedstocks for captive HSCs has not been extensively studied. Given that such products are typically intended to stimulate consumption and rapid growth, they nonetheless may not be appropriate for long-term HSC maintenance. Current aquatic feed trends have also transitioned to alternative, terrestrial sources of proteins and lipids to keep prices competitive and growth rates high, whereas an optimal HSC product would center on animal vigor and cellular health.
One circulating cell type, the amebocyte, confers HSC immunity. In response to microbial exposure, amebocytes initiate formation of coagulin, a polymer of protein, to envelop endotoxins (LPS) and prevent systemic infection. This single-cell simplicity of the HSC hemolymph (copper-rich blood) offers a unique model to investigate the impact of various diets at the cellular level, not feasible in most species.
Ultimately, this aspect of our work focused on the effects of diet on HSC wellbeing, cellular health, and LAL quality in the greater context of establishing prototypical nutritional and aquaculture standards for adult HSC husbandry and year-round LAL production (Tinker-Kulberg et al., 2020a, b). Specifically, these efforts concentrated on developing feed formulations enriched with natural sources of vitamins, minerals, and probiotics to support long-term HSC aquaculture management and establish a new, sustainable supply paradigm for a critical industrial raw material (LAL). This work may also provide a foundation for applications in a variety of sectors.
Materials and Methods
Recirculating Aquaculture System for HSC Husbandry
The HSC studies were conducted in a recirculating aquaculture system (RAS; Clear Flow Aquaponic System, Nelson and Pade, Montello, WI, United States) equipped with four holding tanks (4′ × 6′ × 1′); a biofiltration tank with K1 medium (Pentair, Minneapolis, MN, United States); and a clarifying (solids separation) tank (Figure 1). Ambient air supplied oxygen to the water via a piston pump with supplemental air stones positioned in each tank (Nelson and Pade, Montello, WI, United States). Water was recirculated through biological filtration tanks, allowing for purification, hygiene and disease prevention. The tanks were brought to a salinity of 20–22‰ using a commercial sea salt preparation (Crystal Sea®, Marine Enterprise International, Baltimore, MD, United States), an aquatic salinity refractometer (AEROway, ImagitariumTM, San Diego, CA, United States), and an electric conductivity (EC) meter (Bluelab Corporation, San Dimas, CA, United States). Weekly saltwater exchanges were performed between 1 and 2%.
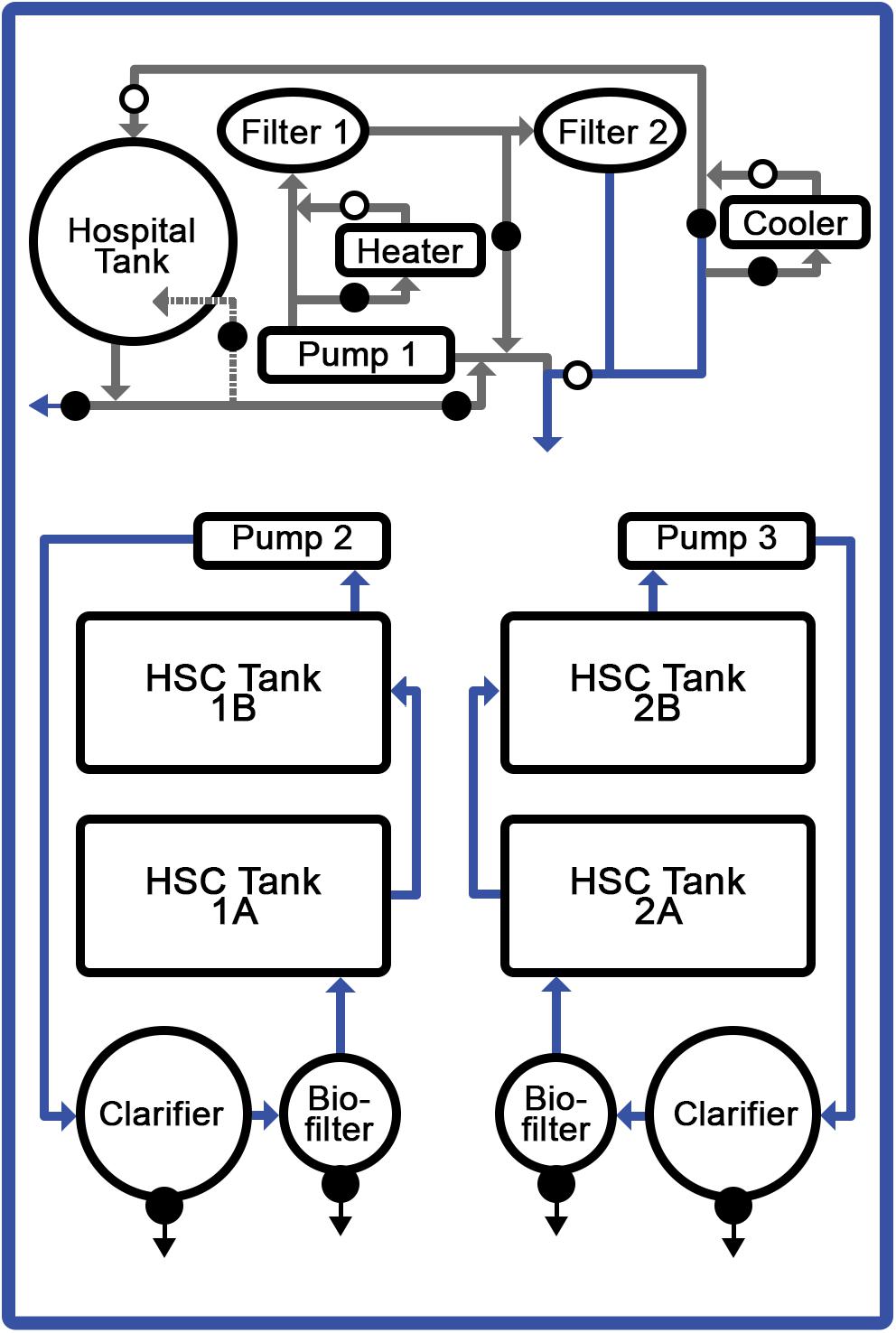
Figure 1. Recirculating aquaculture system (RAS). Temperature-controlled, saltwater system optimized for HSC comprising: four discrete tanks, filtration units, and aeration system. Respective feed formulations were provided to specific tanks with 6 HSCs each.
Target water-quality parameters were determined based on standard saltwater aquaculture methods (Timmons, 1994; Schreibman and Zarnoch, 2009; Ebeling and Timmons, 2010; Shelley and Lovatelli, 2011), and comprehensive daily, weekly and monthly analyses were conducted. Daily water conditions were optimized for: temperature (18.5–20°C); salinity (20–22‰); pH (7.5–8.2); dissolved oxygen (6–9 mgL–1); photoperiod (12-h of natural light); ammonia (0–1.5 ppm); nitrates (<150 ppm); nitrites (2–5 ppm), and alkalinity (90–150 ppm). Water parameters were measured and adjusted as needed six times per week and did not vary significantly throughout the course of the study.
HSCs (n = 24) were procured (Dynasty Marine Associates, Marathon, FL, United States) or obtained via a collection permit and then maintained in accordance with an aquaculture operation permit (North Carolina Department of Environmental Quality, Division of Marine Fisheries; #1946771 and #1947050, respectively). A health assessment was recorded for each HSC upon intake (i.e., sex, weight, carapace length/width, as well as an appendage, carapace, eye, and mouth evaluation). HSCs were stocked at a density of 1.0-lb per square foot, resulting in approximately one HSC per 4-ft3 of tank space.
Feed Studies, Sources and Key Parameters
For the feed studies, ∼6 adult HSCs were held in each tank, such that their combined weight was similar between the four cohorts. Mature HSCs used in this study on average weighed 1.77 ± 0.77 kg (3.91 ± 1.58 pounds). Each cohort had the same number of males and female HSCs to avoid differences in feeding behavior or data bias. To ensure HSC wellbeing and avoid captive-induced panhypoproteinemia, diet and feeding rates were established over a 6-week period prior to initiation of the trials. The feed rates, crude protein (CP) intake, nutrient composition, and daily energy requirements were determined for natural feed preparations and a commercial aquaculture feed (Tables 1–3). Initial observations revealed that HSCs fed at different rates per day (expressed as the percent of b/w of the HSC) depending on the diet. Feed rates were calculated as a percentage of HSC body weight eaten within 8 h (any feed not consumed was removed from the tanks), with a total daily feed rate of 3%, consistent with Carmichael and Brush (2012). We found that at this rate, feed was in slight excess and not limiting, and thus HSCs were more or less feeding at ad libitum throughout the day. The average gross energy of each diet was calculated by summing the content of its protein (23.6 kJ/g), lipid (39.5 kJ/g), and carbohydrate (1.2 kJ/g) components (National Research Council, 1993). The nutrient content of natural sourced diets (Diets B–D; Tables 2, 3) was estimated from published references (McRoberts Sales Co. Inc, 2014; Food and Agriculture Organization, 2019; United States Department of Agriculture, 2019). Chemical analyses of experimental gelatin-based diets were performed by an external independent laboratory (i.e., dry matter, DM; Carolina Analytical Services, LLC, Bear Creek, NC, United States). Dry weight was determined by comparison before and after dehydration of feeds at 125–140°C for 48 h. The nutrient content of the wet feed (AF, As Fed) used in the feeding trials was determined by multiplying the DM nutrient content by the DM ratio (dried/wet feed weight).
Six feed sources were combined into the three evaluations, including: natural sources (three variations); a commercial hard pellet feed (Classic Brood 46/12 Complete Feed, Skretting, Tooele, UT, United States); and two experimental formulations shown in Table 2 [amino acid composition (%); Supplementary Table 1]. The commercial and experimental feeds were modified with gelatin (5% w/v) to retain moisture, limit nutrient leaching and facilitate sinking to allow characteristic HSC bottom foraging while providing the same stability between feeds. The gelatin-based matrix conferred an ability to easily and economically encapsulate the nutritional inputs, while readily retaining palatability, consistency, and stability (in storage, as well as in the aquaculture system). Natural, frozen feeds were purchased commercially (Aylesworth’s Fish and Bait, St Petersburg, FL, United States) or sourced locally and fed directly. Except for natural feeds that were given whole, the experimental feeds were processed through a meat grinder, mixed with hot gelatin (65–70°C) and allowed to solidify at room temperature. They were measured for each cohort based on daily consumption rates and then frozen at −20°C to preserve freshness. Feed was thawed only once and fed daily to each cohort. Fresh batches were made at the beginning of each trial to safeguard vitamins that are susceptible to oxidation during prolonged frozen storage. Diets were optimized to ensure that macronutrients (protein and lipids) and micronutrients (Vitamins B, C, E, and K, copper, etc.) were available for hemocyanin production, as hemocyanin loss initially follows hemolymph harvest. Some feed formulations (Diets B–F, Table 2) naturally contained or were enriched with probiotics and prebiotics from natural sources to ensure healthy gut microbiota and improve digestion and extraction of energy and nutrients from the feed (Abelli et al., 2009; Qi et al., 2009; Fukuda and Ohno, 2014; Torrecillas et al., 2014; Carnevali et al., 2017; Gibson et al., 2017; Egerton et al., 2018).
Respective feed trials, HSC cohorts, and diet compositions are shown in Tables 1, 2. The feeds were dispersed in individual tanks, and each group received the same feed at ∼3% of the total body weight per tank each day throughout the 8-week periods, with the exception of Cohort 2 (Table 1). The variety of natural feed sources was increased with each successive trial for the latter cohort (Tables 1, 2) to investigate dietary complexity and diversity. After each study, HSCs were also physically examined with respect to the carapace, joints, eyes, and book gills, as well as weight and size. Total serum protein concentration, amebocyte density (cells/mL), and LAL reactivity were also analyzed at the beginning and conclusion of each feed trial.
Hemolymph Collection and Analysis
Hemolymph was collected at the start and culmination of each feed study via previously established methods (Coates et al., 2012; Anderson et al., 2013). The pericardial membrane was cleaned twice with 70% ethanol prior to hemolymph collection with a 22-gauge needle (Becton Dickinson, NJ) using a standard SOP (Armstrong and Conrad, 2008). An aliquot of the hemolymph was diluted (1:1) in a pre-chilled anticoagulant mixture (pH 7.3) of N-ethylmaleimide (NEM; 0.125%), NaCl (3%), and Tris-HCl (0.5M, pH 7.5) for amebocyte density analysis. Sterile tubes containing the balance of each harvest were centrifuged (1000 rcf/5 min), and the supernatant was removed and stored at −70°C pending hemocyanin analysis. Any samples that appeared clotted after centrifugation were discarded. Amebocyte pellets were washed with endotoxin-free NaCl (3% v/v) and then stored at -70°C pending LAL extraction.
Hemocyanin and amebocyte density were measured throughout the study as HSC health indicators (Nagai et al., 2001). Total serum protein concentration was used as a proxy for hemocyanin (Hc) by diluting the hemolymph supernatant in 0.1M Tris-HCl (pH 7.5) and measuring absorbance at 280nm (UV-Vis Spectrometer; Cary 6000i, Agilent Technologies, Santa Clara, CA, United States). Dioxygen-bound Hc (Oxy-Hc) was likewise measured at 340nm. The total protein concentration was calculated according to Nickerson and Van Holde (1971), using the absorption coefficient A280 = 1.39 mg–1mLcm–1 for hemocyanin.
Amebocyte density was calculated by adding 10 μL of the NEM-stabilized cells to a hemocytometer and analyzed microscopically. Since amebocytes are susceptible to rapid degranulation, a digital camera was used to record bright field images from the hemocytometer, and counts were processed offline (ImageJ Software, NIH, Bethesda, MD, United States).
LAL Preparation and Reactivity
Limulus amebocyte lysate reactivity was also evaluated throughout the study as an HSC health indicator and to investigate the effects of aquaculture diets on quality. LAL was prepared by thawing frozen amebocyte pellets on ice and then lysing them in pyrogen-free water at a 1:1 ratio to original hemolymph collection volume under gentle agitation overnight at 4°C. Next, a chloroform extraction (1:1 v/v) of the lysate was performed, and the aqueous phase was measured for total protein using a BCA kit (Pierce, Thermo Fisher Scientific, Waltham, MA, United States). All LAL extracts were aliquoted and frozen at −80°C pending analysis (consistent LAL reactivity was observed in frozen samples for up to 6-months). LAL samples were also limited to a single freeze-thaw cycle to preserve reactivity.
Limulus amebocyte lysate from HSCs fed different diets was evaluated turbidimetrically. Equal protein amounts of amebocyte lysate (LAL) isolated from individual HSCs in each feed cohort (n = 6) were pooled and analyzed for their relative reactivity. LAL from commercial kits (E-ToxateTM, Sigma Aldrich, St. Louis, MO, United States) served as a reference. For each LAL assay, an equal amount of protein from pooled aquaculture lysates and reference LAL were brought to a total volume of 100 μL using endotoxin-free water; these were mixed gently with 100 μL of endotoxin solution (final concentration: 0 – 50 EU/mL) in a pyrogen-free, 96-well microplate and incubated at 37°C for 1 h. The conversion of coagulogen to coagulin was measured at 340 nm on a microplate reader (BioTek 800, BioTek Instruments, Winooski, VT, United States). The turbidity of the blank (endotoxin-free lysates) was subtracted from test values, and the relationship between endotoxin concentration and clot formation was measured. The reactivity of the aquaculture LAL was normalized to the reference LAL, and relative absorbance was measured.
Protein Analyses
Purified protein extracts (10 μg) from each feed group were subjected to SDS-PAGE in the presence of β-mercaptoethanol. Each sample was diluted in a loading buffer (NuPageTM, Invitrogen, Carlsbad, CA, United States) containing 2.5% β-mercaptoethanol and heated to 85°C for 3 min. Electrophoresis was performed on 8% Tris-Glycine gels (NovecTM Wedge Well, Invitrogen, Carlsbad, CA, United States). A pre-stained protein standard (SeeBlue® Plus2, Invitrogen, Carlsbad, CA, United States) was used as a molecular weight marker, and commercial LAL was used as a reference (E-ToxateTM, Sigma-Aldrich, St. Louis, MO, United States). The gel was either stained (Coomassie Brilliant Blue R-250; Bio-Rad, Hercules, CA, United States) or transferred to a polyvinylidene fluoride (PVDF) membrane (Invitrogen, Carlsbad, CA, United States) for Western blot analysis.
For Western blotting, the PVDF membranes were blocked with PBS (pH 7.4) containing 5% BSA (or 5% non-fat milk) and 0.1% Tween 20 for 1 h at room temperature. Primary rabbit polyclonal antibodies [COCH (A6562), Factor IX (A1578) and KLKB1 (A5318)] were used for the detection of LAL clotting factors (ABclonal Inc., Woburn, MA) by overnight incubation at 4°C (1:1,000 – 1:3,000; determined experimentally). Membranes were washed 3X with PBS/Tween 20 (0.1%) for 7 min with agitation and then incubated with a goat anti-rabbit secondary antibody (1:10,000 dilution) labeled with horseradish-peroxidase (HRP; ABclonal Inc, Woburn, MA, United States) for 1 h at room temperature. Residual secondary antibody was removed by washing, as described above. The membranes were incubated with ECL reagent (Thermo Fisher Scientific, Waltham, MA, United States) for 5 min at room temperature, and consequent target protein luminescence was measured (AmershamTM Imager 600; GE Life Sciences, Piscataway, NJ, United States). Relative protein abundance within the linear dynamic range was quantified using densitometry.
Post-translational protein modification of the LAL extracts was measured by Coomassie staining or Western blot, as described above. Prior to electrophoresis, LAL extracts were treated with either glycopeptidase F (PNGase F; ∼36 kDa; New England BioLabs, Ipswich, MA, United States), under denaturing conditions at a final concentration of ∼0.4 units of enzyme (20 ng/μg of lysate) or alkaline phosphatase (calf intestine AP; ∼54 kDa; Roche, Indianapolis, IN, United States) at a final concentration of 2 units of enzyme (2 μg/μg of lysate) according to the manufacturer’s instructions. Protein deglycosylation and dephosphorylation were assessed by analyzing mobility shifts (AmershamTM Imager 600, Buckinghamshire, United Kingdom).
Data Analysis
Individual HSC cohorts were used for each respective analysis (Table 4). Standard deviation between individual HSCs (n = 6) within each cohort was calculated. For simplicity and assay accuracy, LAL reactivity was measured using pooled samples from HSCs within each feeding cohort (n = 6), comprised of equal amounts of proteins from each one. The standard deviation of LAL reactivity represents each assay performed in triplicate. LAL reactivity derived from individual HSCs was close to the mean of pooled samples from each cohort. All trials represent the mean ± standard deviation, where n equals the number of HSCs per cohort. If a difference was determined, a Student’s t-test was used for comparison, assuming equal variance, with a significant difference set at p ≤ 0.05.
Results
Feed Rate Determination
Three separate 8-week feed trials were conducted to investigate the effect of diet on health parameters of respective HSC cohorts (n = 6 per study) as described in Table 1. The nutritional composition and economic analyses were determined for each experimental feed (Tables 2, 3). CP intake for adult HSCs ranged between 3.6 and 6.9 mg per gram of HSC b/w per day [(CP (mg)/(HSC body weight (g) per day)] when fed an 11–23% protein diet at a 3% daily feed rate. Experimental feeds were developed to provide the target energy requirements (Joules) from 153 to 244 kJ [HSC body weight (kg) per day] determined in the pre-trial feed studies (Table 3). These values are aligned with growth trials conducted with juvenile HSCs that were shown to require 224 kJ of digestible energy and 8.7 mg of digestible protein for maintenance per gram b/w per day (Tzafrir-Prag et al., 2010).
Irrespective of feed type, adult HSCs consumed similar amounts of food energy per day across all groups (i.e., mean = 150 kJ per kg of HSC b/w per day), and the previously cited 3% feed rate was thus validated and employed (Carmichael and Brush, 2012; Table 1). Maintenance levels of CP for adult HSCs were found to be ∼5.25 mg per gram b/w per day when fed an 11–23% protein diet, which aligned with various fish, shrimp, and juvenile HSC aquaculture studies (Kuresh and Davis, 2000; Blanc and Margraf, 2002; Lupatsch et al., 2008; Berkson et al., 2009; Tzafrir-Prag et al., 2010). The daily feed rate and diet formulations sustained HSC weight (±1.2%) and serum protein levels (>5.0 mg/mL) over the course of the feed study with a 100% survival rate (Supplementary Table 2). All HSCs were mature and no longer prone to molting; thus, their growth rate did not increase over the course of the study.
Feed Trial 1: Effect of Diet on LAL Reactivity and Protein Levels
Horseshoe crabs were provided three different diets over a period of 8 weeks (Diet A, Diet B and Diet E; Tables 1–3). At the outset of Feed Trial 1, the aquaculture HSC serum protein baselines (hemocyanin concentration, Hc) ranged from 44.49 to 65.15 mg/mL. Upon trial conclusion, HSCs fed Diet E had the highest LAL reactivity (2.1X higher than reference LAL) but showed the lowest Hc concentration (Diet E = 21.2 + 1.22 mg/mL vs. Diet A and B = 43.6 + 8.9 and 65.2 + 11.8 mg/mL, respectively); whereas, amebocyte density remained constant across the three groups, ranging between ∼2 × 107 to 3 × 107 cells/mL.
The reactivity of LAL extracts (150 μg) for each feed group and a reference LAL are shown in Figure 2A. LAL incubation with low concentrations of LPS (0.5 EU/mL) revealed similar reactivity (clot formation) for aquaculture-derived LAL from HSCs fed Diet E and reference LAL as a standard control (E-ToxateTM; Sigma-Aldrich, St. Louis, MO, United States). However, the LAL reactivity derived from HSCs fed Diets A and E was ∼2X more responsive than reference LAL at high concentrations (saturation levels) of LPS (50 EU/mL) (Figure 2B). Diet B, the least-complex feed (Table 2) showed the lowest reactivity at 0.5 and 5.0 EU/mL concentrations of LPS, but it was equivalent to that of reference LAL at 50 EU/mL.
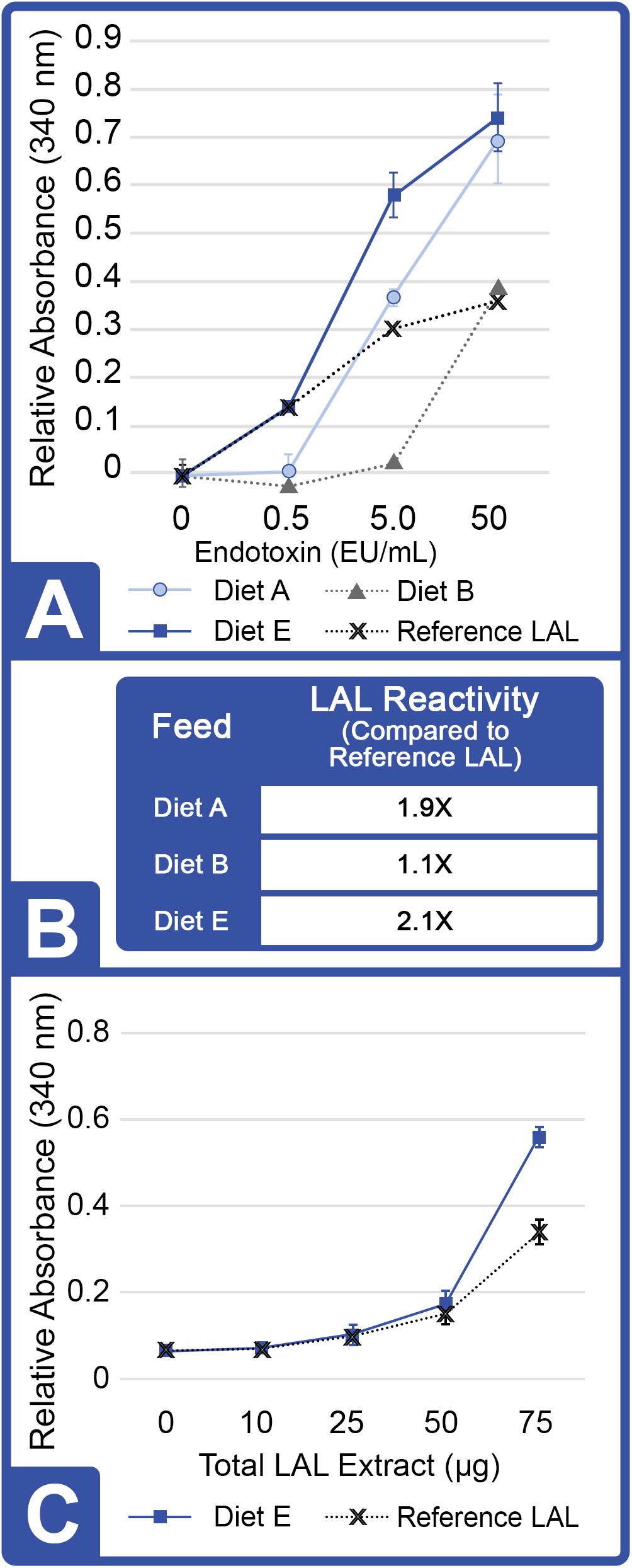
Figure 2. Effect of diet on LAL reactivity to endotoxins (Feed Study 1). (A) Gel formation measured by absorbance at 340 nm. Hundred and fifty μg of aquaculture LAL was evaluated at a range of endotoxin concentrations: 0, 0.5, 5.0, and 50.0 EU/mL with a reference LAL. Results are represented as mean ± SD between individual LAL assays performed in triplicate. (B) LAL reactivity to endotoxin from each diet cohort was normalized to reference LAL reactivity at 50 EU/mL of LPS. (C) Reactivity (gelation) of increasing concentrations of LAL (0, 10 25, 50, and 75 μg total protein) from HSCs fed Diet E vs. reference LAL at 50 EU/mL of LPS measured by absorbance at 340 nm. Results are represented as mean ± SD between individual LAL assays performed in triplicate.
The reactivity of LAL from the Diet E cohort and the reference LAL were evaluated at increasing protein concentrations (0, 10, 25, 50, and 75 μg, Figure 2C) to assess gelation (ΔOD) between LAL extracts in the presence of saturation levels of LPS (50 EU/mL). Both extracts required at least 50 μg of total protein (or 250 μg/mL) for absorbance to increase 0.200 units above the background (indicative of a positive reaction/gel formation). At 75 μg of LAL protein, the Diet E cohort also showed greater reactivity (2.1X higher) per μg of total protein compared to control LAL after 1 h of incubation with a saturation-level (50 EU/mL) of the LPS substrate.
Feed Trial 2: Effect of Increased Diet Diversity on LAL Reactivity
In the first feed trial, HSCs in Cohort 2 were provided a natural feed diet (Diet B; Tables 1, 2) as previously reported (Carmichael and Brush, 2012), and their LAL demonstrated the lowest reactivity (Figures 2A,B). During the second feed trial, the nutrient complexity and diversity of the natural feed sources were increased by adding a greater variety of marine-derived proteins [Table 2: Diet B (2 sources) compared to Diet C (6 sources)]. Additionally, a new experimental feed (Diet F) was developed with different sources of proteins and lipids than those used for Diet E (Table 1), in an effort to avoid declining Hc (21.2 mg/mL) values observed in Trial 1 for Diet E. In Feed Trial 2, HSC Cohorts 1 and 3 were provided the same diets as in Trial 1 (Table 1: Diets A and E, respectively).
At the conclusion of Feed Trial 2, hemolymph was harvested from each HSC cohort, and LAL reactivity was measured. A titration was performed with increasing concentrations of LAL protein (37.5, 75.0, and 150 μg) derived from each diet and a reference LAL (E-ToxateTM, Sigma-Aldrich, St. Louis, MO, United States) incubated with saturation levels of LPS at 50 EU/mL (Figure 3A). Similar to Feed Study 1, the aquaculture LAL had slightly greater reactivity per μg of total protein compared to the reference LAL (1.4–1.6X higher; Figure 3B). The degree of nutrient diversity affected LAL: Diet C (Feed Trial 2; 6 sources) outperformed Diet B (Feed Trial 1; 2 sources) in terms of reactivity (Diet B = 1.1X versus Diet C = 1.4X more sensitive when normalized to the control LAL).
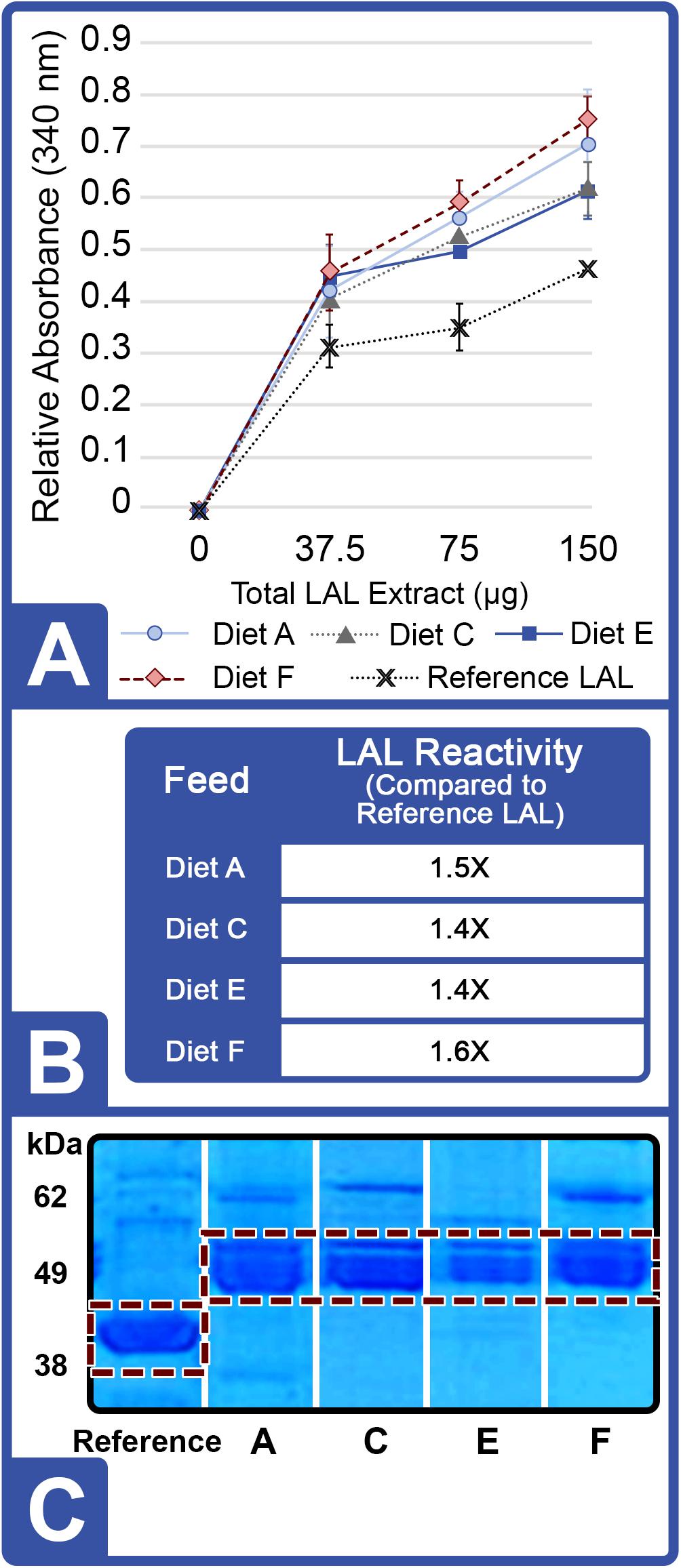
Figure 3. Effect of diet on LAL reactivity to endotoxins (Feed Study 2). (A) Standard curves of aquaculture LAL reactivity to endotoxins by diet vs. reference LAL with 50 EU/mL LPS measured by absorbance at 340 nm. Results are represented as mean ± SD between individual LAL assays performed in triplicate. (B) Aquaculture LAL from each diet was normalized to reference LAL reactivity in the presence of 50 EU/mL of LPS. (C) SDS-PAGE of crude amebocyte lysates from aquaculture HSCs (Lanes 3 – 6) compared to reference LAL. Each sample was loaded at 5 μg protein per lane on an 8% Tris-Glycine gel and stained with Coomassie blue; the See Blue+2 molecular weight marker was loaded as a ladder. Factor C (L-chain) is highlighted in white and appeared as a well-defined band at ∼43 kDa in reference LAL and at ∼52 kDa in the aquaculture LAL.
Feed Trial 3: Effect of Diet on LAL Reactivity, Hc Concentrations and Amebocyte Density
In Feed Trial 3, a significantly more diverse natural feed diet (Diet D; 12 ingredients; Table 2) was compared with Diets B and C (2 and 6 ingredients, respectively). HSCs fed diet D yielded more reactive LAL than HSCs fed the other natural diets: 45% greater than diet B (the lowest-complexity diet) and 14% greater than diet C (moderately complex diet) (Table 4).
Overall, the HSC cohort fed Diet F over an 8-week period appeared to outperform all feeds with 2.9X greater LAL reactivity over the control; and across two successive feed trials (16 weeks), sensitivity increased (Table 4). Notably, the overall LAL reactivity of extracts derived from HSCs fed Diet F was 7.25X greater than those given Diet E (Figure 4A).
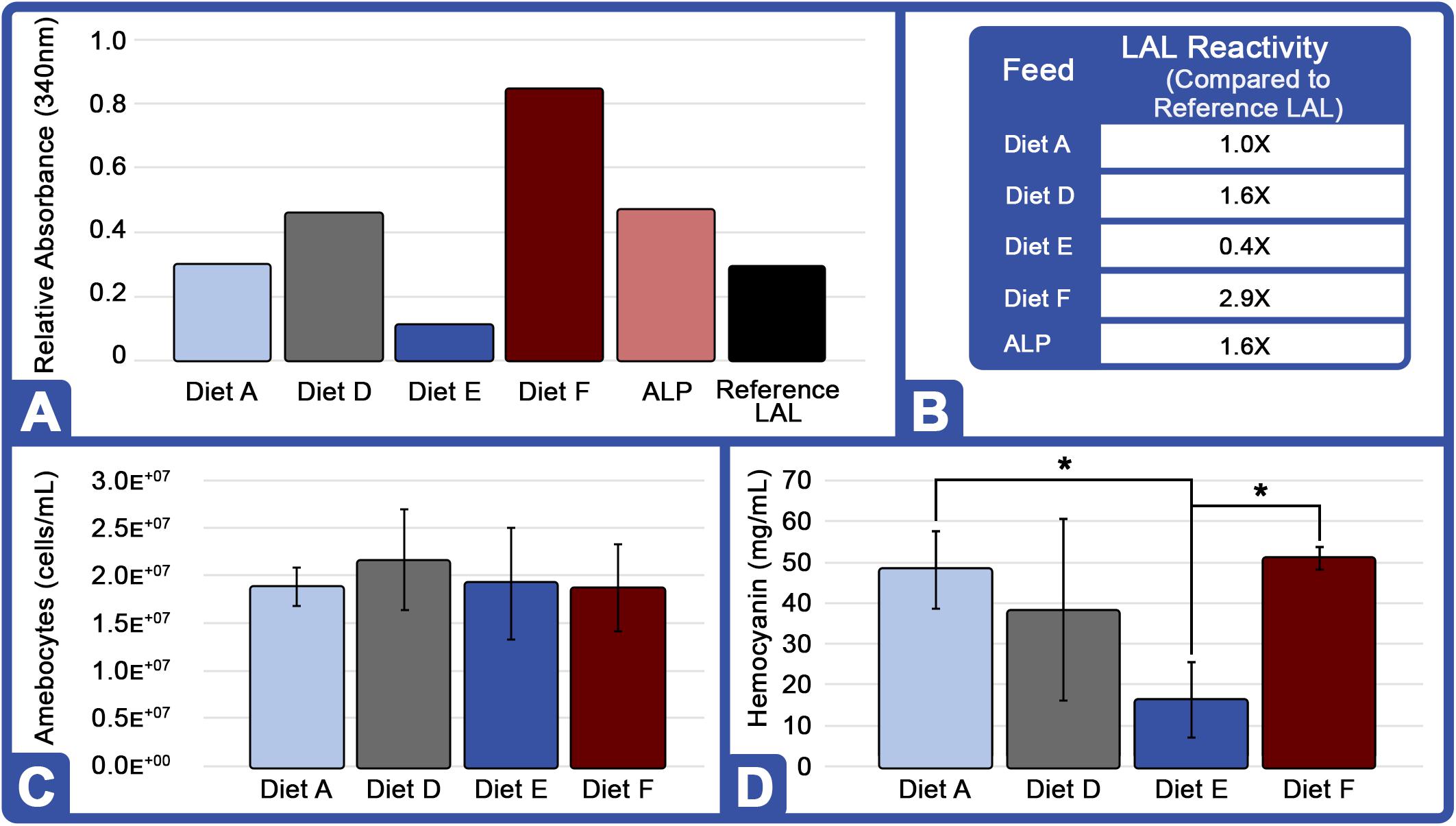
Figure 4. Effect of diet on LAL reactivity, amebocyte density, and hemocyanin concentration (Feed Study 3): (A) Aquaculture LAL reactivity from four diets over 8-week period vs. reference LAL at 50 EU/mL LPS concentration. Equal protein amounts from each Diet (75 μg; total = 300 μg) were pooled and tested for clotting [ALP: Aquaculture Lysate Pool (Diet A, D, E, F)] and reactivity to endotoxins was measured by absorbance at 340 nm. (B) Aquaculture LAL reactivity to endotoxins vs. reference LAL. (C) Amebocyte density of aquaculture HSC determined microscopically on a hemocytometer. (D) Hc concentration of aquaculture HSC hemolymph measured by UV spectrophotometry (280 nm). Results are represented as mean ± SD between individual HSCs in each cohort (n = 6). *p < 0.05 compared to Diet E.
In contrast, the LAL reactivity of the HSC cohorts fed Diets A and E in all three trials (24 weeks) declined over time (Table 4). In Feed Trial 1, Diet E resulted in a 2.1X increase over baseline (Figure 4), which fell to 1.4- and 0.4X in Feed Trials 2 and 3, respectively. The LAL sensitivity from HSCs fed Diet E dropped 33% after the second 8-week trial and 71% after the third 8-week trial.
Horseshoe crabs from each cohort demonstrated consistent total amebocyte counts (Figure 4C). The low LAL reactivity observed in HSCs fed Diet E was thus not related to lower amebocyte concentrations. Rather, in Feed Trial 3, it correlated with low Hc levels (Figure 4D). HSCs offered Diet F also had the highest Hc levels and produced the most reactive LAL. Conversely, Diet E resulted in the lowest Hc and LAL response among all evaluated feed cohorts.
In Feed Trial 3, the LAL reactivity varied between the different cohorts more than observed in the previous studies (e.g., Figures 3A vs. 4A). Equal amounts of LAL protein (μg) pooled from each group (aquaculture LAL pool; ALP) had ∼1.6X higher reactivity than reference LAL (Figure 4B). This reactivity is approximately equivalent to the average of the LAL response derived from individual diet cohorts (∼1.5X). Therefore, pooling the LAL protein had no effect on reactivity. The LAL sensitivity of each HSC cohort relative to the reference LAL is shown in Table 4.
Effects of Diet on LAL Clotting Factors
LAL Factor C protein characteristically generates an 80 kDa (H-chain) and a 43 kDa subunit (L-chain) under denaturing electrophoresis (Figure 5A). LPS activation of Factor C results in the conversion of the L-chain into the A (9 kDa) and B-chain (34 kDa) fragments, thereby initiating the reaction cascade (Figure 5B). The Factor C L-chain subunit in the aquaculture-derived LAL extracts notably migrated as a higher molecular weight species compared to that of reference LAL (Figures 3C, 6A,C).
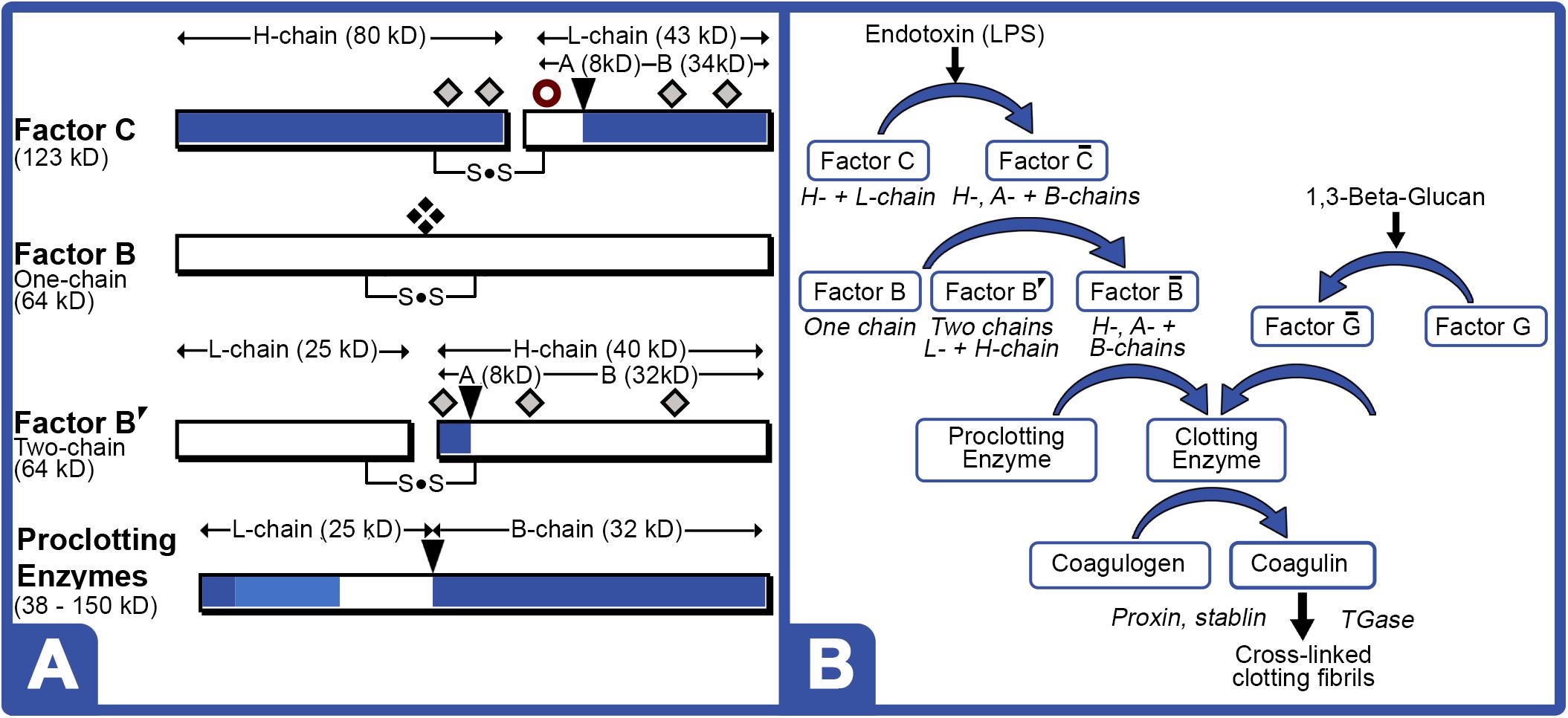
Figure 5. LAL clotting factors structures and coagulation cascade. (A) Cleavage sites [] for activation; [
] nicking site; [
] potential glycosylation sites; and [
] newly proposed phosphorylation site in the protein zymogen. Zymogen Factor B is a single-chain form; whereas, “nicked” double-chain form is composed of L- and H-chains, both containing internal disulfide bond. (B) Schematic of LPS and 1,3-Beta-Glucan LAL coagulation cascade. LPS converts phosphorylated or glycosylated Factor C zymogen (H- + L-chain subunits) into activated Factor enzyme (A- + B-chain subunits). Activated Factor C nicks single-chain Factor B zymogen into Factor B′ (H-chain) glycosylated precursor that is converted to activated Factor enzyme (B-chain). Activated Factor B and activated (1–3)-D-glucan binding factor G, initiate cleavage of various proclotting enzymes to form the clotting enzyme, which hydrolyzes coagulogen into coagulin, resulting in insoluble clot.
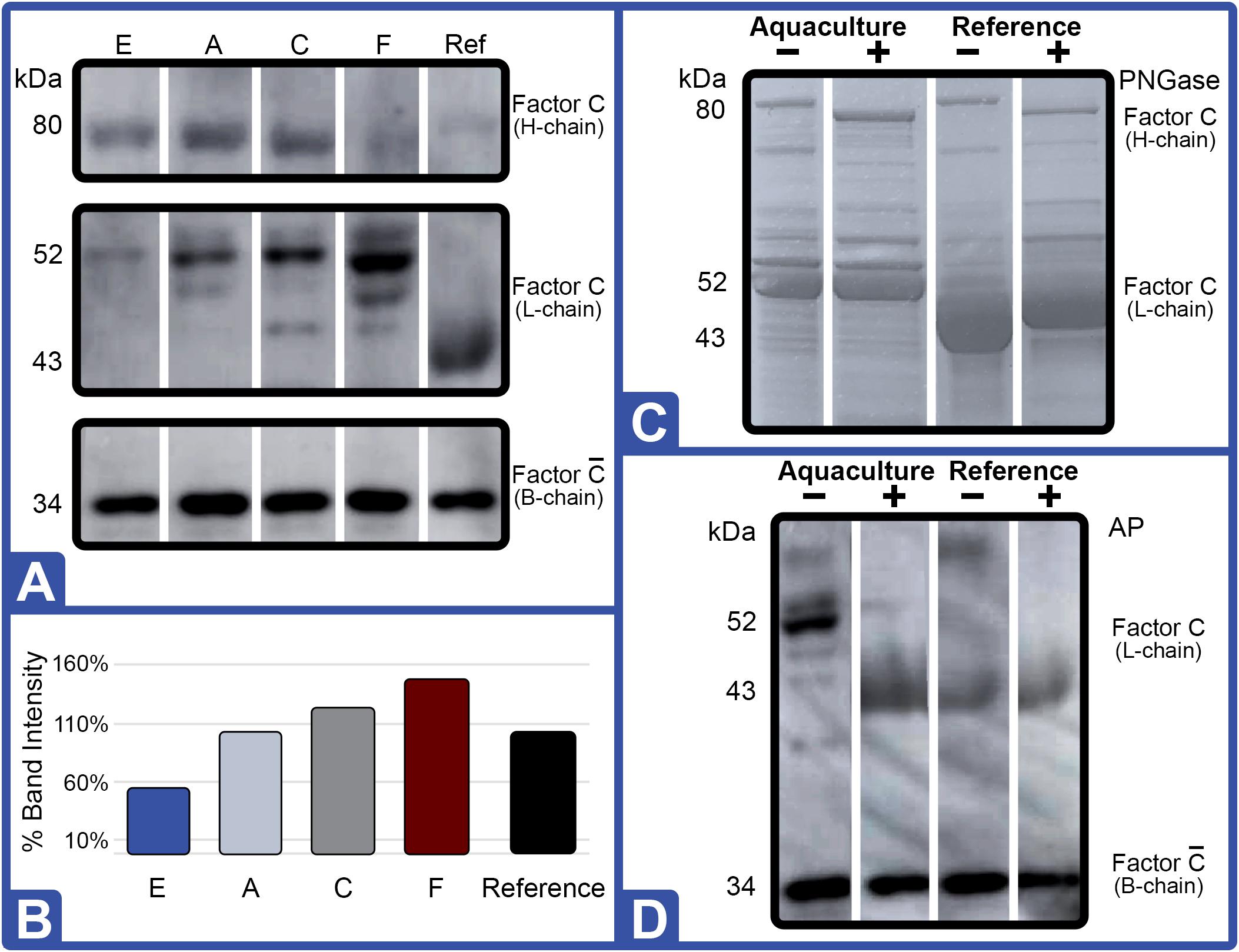
Figure 6. Effect of diet on Factor C protein expression (Feed Study 3). (A) Western blot of crude lysates (5 μg) of LAL from each diet vs. reference LAL. Extracts from Diet E (Lane 1), Diet A (Lane 2), Diet C (Lane 3), Diet F (Lane 4), and reference LAL (Ref; Lane 5) with See Blue+2 molecular weight marker. Each sample was resolved across 8.0% Tris-Glycine gel and transferred to PVDF membrane. Proteins analyzed by membrane incubation with anti-Factor C polyclonal rabbit antibodies (COCH). (B) Total Factor C protein abundance (% band intensity) quantified on an imaging device. Zymogen and activated Factor C were observed. (C) Coomassie-blue staining of equal protein amounts from each diet were pooled [ALP: Aquaculture Lysate Pool (Diet A, D, E, and F)]; treated with (+) or without (–) PNGase. (D) Western blot of crude LAL lysate (ALP) and reference LAL treated with (+) or without (–) alkaline phosphatase (AP). Ref: Reference LAL.
Gel electrophoresis of crude LAL from the different feed groups also revealed distinct differences in Factor C patterns relative to other protein bands with Western blot testing (Figure 6). In fact, Western blot analysis of Factor C suggested that relative abundance of this protein correlated to LAL reactivity.
For example, the Diet E cohort yielded 40% lower LAL reactivity and 50% less Factor C than reference LAL per μg of total protein; whereas, Factor C protein levels and LAL reactivity were equivalent to the reference (Figures 4, 6A,B). The HSCs fed Diet D showed 1.2X higher Factor C levels and 1.6X greater LAL reactivity compared to the reference; while the Diet F cohort had 1.5X higher Factor C protein levels and 2.9X greater LAL response (Figures 4, 6A,B).
Western blot analysis with polyclonal antibodies specific to Limulus Factor B (Figure 7) and proclotting enzyme (Figure 8) was also conducted to determine the relative concentrations of these clotting factors. The single-chain form of Factor B (Figure 5A) was observed only in the aquaculture LAL (Figure 7A, L- + H-chain). The total amount of Factor B in the LAL of HSCs fed Diet E was 2.4X higher than reference LAL, despite this lysate revealing 40% lower LAL reactivity (Figures 4B, 7B and Table 5). Factor B protein abundance was between 2 and 4X higher in all LAL derived from the aquaculture cohorts in comparison to the reference. In contrast to Factor B, the relative protein levels (Figure 8) of the proclotting enzymes corresponded to the observed LAL reactivity of the respective groups (Figures 4B, 8B and Table 5). However, Western blotting showed variations in the molecular weights of the proclotting enzyme(s) among the diet groups.
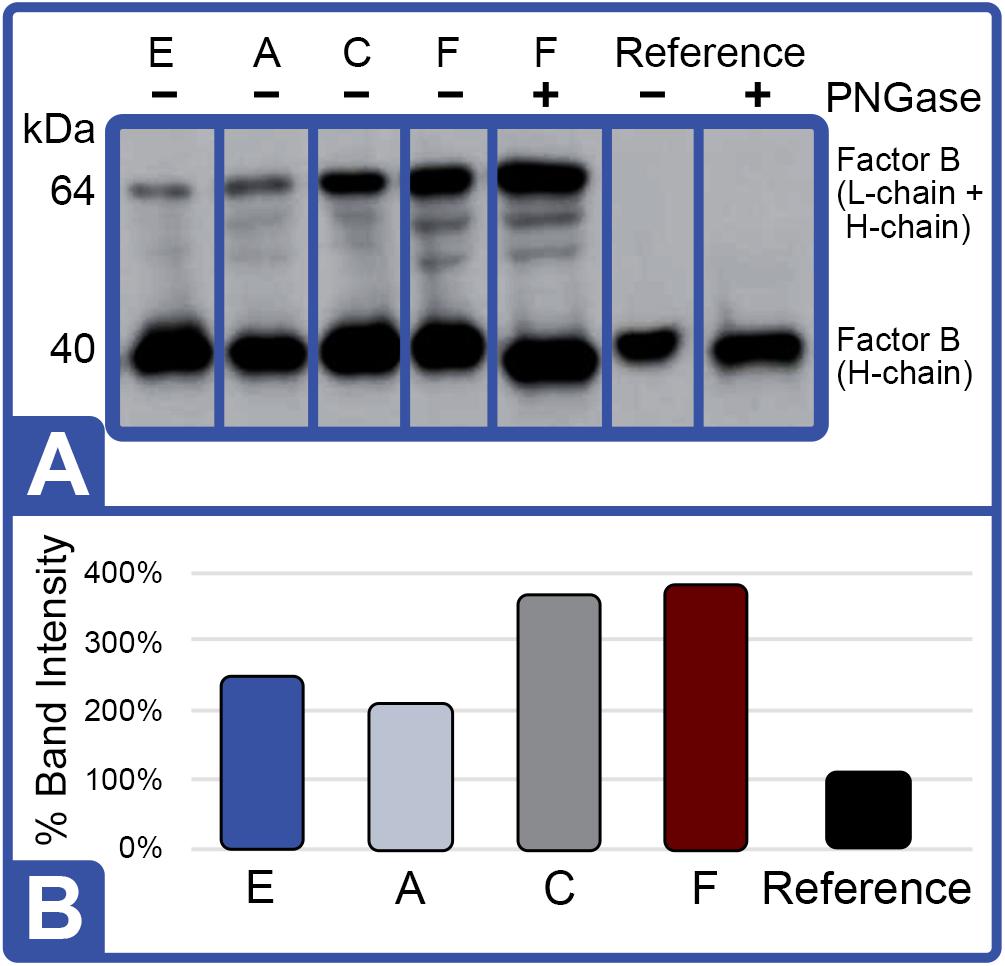
Figure 7. Effect of diet on Factor B protein expression (Feed Study 3). (A) Western blot of crude lysates (5 μg) of LAL from each diet analyzed vs. reference LAL. Extracts from Diet E (Lane 1), Diet A (Lane 2), Diet C (Lane 3), Diet F (Lane 4), Diet F + PNGase (Lane 5), reference LAL (Lane 6), and reference LAL + PNGase (control; Lane 7) with See Blue+2 molecular weight marker. Each sample was resolved across 8.0% Tris-Glycine gel and transferred to PVDF membrane. Proteins analyzed by membrane incubation with anti-Factor B polyclonal rabbit antibodies (KLKB1). Both aquaculture (Diet F) and reference LAL revealed Factor B H-chain glycosylation as shown by slight molecular weight shift in Lanes 4 and 5 of aquaculture LAL and Lanes 6 and 7 in reference LAL. Single-chain Factor B (L- + H-chain) was resolved only in the aquaculture LAL (Lanes 1–5 vs. Lanes 6 and 7); it was not sensitive to PNGAse treatment (Lane 4 vs. 5). Activated Factor (A- and B-chains) was not observed. (B) Total Factor B protein abundance (% band intensity) quantified on an imaging device. Ref, reference.
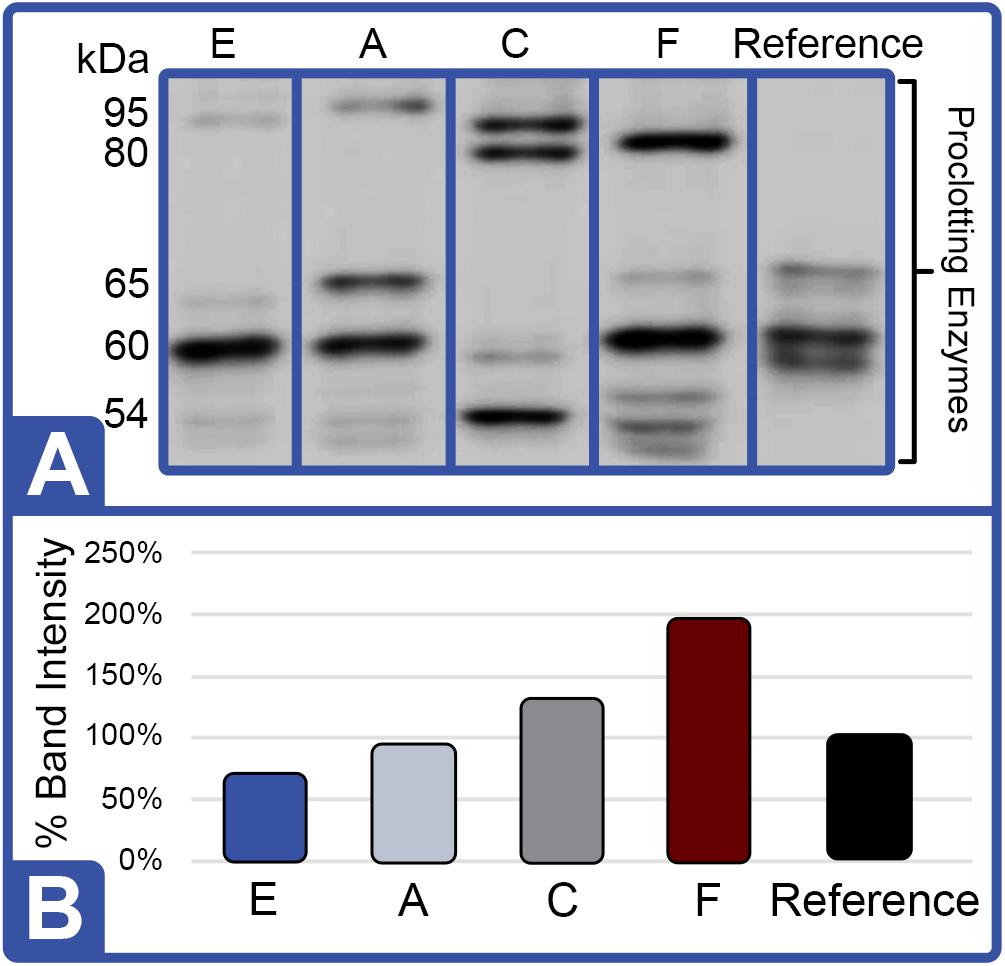
Figure 8. Effect of Diet on Proclotting Enzyme Protein Expression (Feed Study 3). (A) Western blot of crude lysates (5 μg) of LAL from each diet analyzed vs. reference LAL. Extracts from Diet E (Lane 1), Diet A (Lane 2), Diet C (Lane 3), Diet F (Lane 4), and reference LAL (Lane 5) with See Blue+2 molecular weight marker. Each sample was resolved across 8.0% Tris-Glycine gel and transferred to PVDF membrane. Proteins analyzed by membrane incubation with anti-proclotting enzyme polyclonal rabbit antibodies (Factor IX). (B) Total proclotting enzyme protein abundance (% band intensity) quantified on an imaging device.
The highest LAL reactivity observed in the HSCs fed Diet F (2.9-fold; Figure 4B and Table 4) also correlated to the greatest abundance of clotting factor proteins relative to the reference LAL baseline [Table 5: Factor C: 1.5X (Figure 6B); Factor B: 3.8X (Figure 7B); and proclotting enzyme: 2X (Figure 8B)]. HSCs provided Diet E produced the least reactive LAL (0.4X; Figure 4B and Table 5); had the lowest protein expression for Factor C (0.5X; Figure 6B and Table 5), and of proclotting enzyme (0.7X; Figure 8B). However, Factor B in this cohort had 2.4X higher protein levels (Figure 7B and Table 5) compared to reference LAL. In fact, comparison of the highest (Diet F) and lowest (Diet E) LAL reactivity revealed that Factor B may not be the limiting component of the coagulation cascade.
Additionally, the Diet A cohort had similar LAL response (Figure 4B), levels of Factor C protein expression (Figure 6B), and levels/banding patterns of proclotting enzyme (Figure 8B) as the reference LAL. However, this group (Diet A) had 2X higher levels of Factor B protein (Figure 7B) than reference LAL, further signifying that Factor B is not the limiting component. In Diet D-derived LAL, Factor C and proclotting enzyme protein levels were directly related to LAL response.
In all feed groups, Factor C and proclotting enzyme protein levels correlated more closely to LAL reactivity; whereas, the relative abundance of Factor B did not.
Protein Analysis: Glycosylation and Phosphorylation of Clotting Factor Subunits
The most abundant protein bands produced by the crude LAL derived from all aquaculture cohorts were similar, but they differed from the reference LAL as shown via Coomassie gel staining (Figure 3C). Specifically, the L-chain of Factor C (subsequently determined via Western blot in Figure 7) migrated faster (∼43 kDa) in the reference LAL than in the aquaculture LAL (∼52 kDa) (Figure 3C). Both subunits of Factor C have multiple glycosylation sites. The glycosylation sites of the Factor C A-chain subunit have been shown to be partially modified; whereas, the B-chain has two potential glycosylation sites (Figure 5A).
The shift in mobility of the Factor C L-chain subunit between aquaculture and reference LAL was evaluated to determine if the difference in migration was related to glycosylation. Equal amounts (μg) of aquaculture LAL protein (pooled from all diet cohorts; ALP) and the reference LAL were treated with a glycopeptidase (peptide:N-glycosidase F; PNGase) to remove N-linked glycans from glycoproteins. PNGase-treated LAL was then analyzed using Coomassie staining (Figure 6C). Both ALP and the reference LAL revealed a mobility shift in the H-chain subunit of Factor C, thus verifying glycosylation (Figure 6C). The PNGase-induced mobility shift caused an increase in migration that aligned with the expected 80 kDa protein subunit size. Western blotting with an anti-Factor C antibody revealed similar results (data not shown). PNGase treatment of reference LAL also caused an unexpected upward shift in the mobility of the Factor C L-chain subunit after removal of the N-glucan (Figure 6C), and the Factor C L-chain of PNGase-treated reference LAL continued to migrate faster than the ALP Factor C L-chain.
To test whether the higher molecular weight of the Factor C L-chain in aquaculture-derived LAL relative to reference LAL was caused by post-translational phosphorylation, LAL was treated with alkaline phosphatase (AP). As shown in Figure 6D, Western blot analysis revealed that the Factor C L-chain was phosphorylated in the aquaculture LAL; as treatment with AP resulted in a migration pattern of the Factor C L-chain subunit that aligned with reference LAL (∼43 kDa). The mobility of the B-chain subunit of activated aquaculture LAL Factor C (Figure 6D) was unaffected by AP treatment. Factor C in the reference LAL was also unaffected by AP treatment.
Western blotting of residual Factor C B-chain in LAL preparations, presumably generated from LPS activation of Factor C, showed that this segment was not glycosylated or phosphorylated, since PNGase and AP treatment did not change band migration (data not shown). Low levels of this “activated” Factor C B-chain subunit were found in both ALP and reference LAL and may be present naturally in HSC hemolymph (Figure 6A).
As shown in Figure 5A, Factor B and the proclotting enzyme also have characteristic glycosylation sites. Factor B is known to contain two molecular species: a single-chain form (64 kDa; L- + H-chain) and a “nicked” double-chain form (40 and 25 kDa) tethered by a disulfide bond (Figure 6). The reference LAL contained the double-chain but not the single-chain form of Factor B (L- + H-chain) found in aquaculture LAL (Figures 5B, 8A). Glycosylation of the Factor B H-chain was present in both the aquaculture and reference LAL; however, the single-chain form of Factor B (L- + H-chain) was not sensitive to PNGAse treatment (Figure 7A). The banding patterns of the proclotting enzyme(s) were also not affected by PGNase treatment (data not shown). Lastly, both Factor B and proclotting enzyme were insensitive to AP treatment.
Effect of Divalent Cations on LAL Reactivity
The inclusion of divalent cations (MgCl2 and CaCl2) has reportedly enhanced LAL reactivity (Sullivan and Watson, 1974, 1978). Given that LAL preparation methods by commercial sources are proprietary, the inclusion of divalent cations were evaluated to determine the potential influence of processing techniques on the LAL reaction. Addition of MgCl2 at 10 and 25 mM to the assay using aquaculture LAL (Diet F) reduced reactivity (−32% and −30%, respectively; Figure 9A); whereas, the inclusion of 2 mM CaCl2 revealed a modest increase (5.8%), but blunted response (−7.8%) at 10 mM (Figure 9A). In contrast, the addition of MgCl2 (10 and 25 mM) and CaCl2 (2 and 10 mM) in reference LAL increased sensitivity at all concentrations (Figure 9A). As shown in Figure 9B, aquaculture LAL revealed greater response than reference LAL preparations under optimal salt conditions for each lysate of 2 mM CaCl2 and 25 mM MgCl2, respectively.
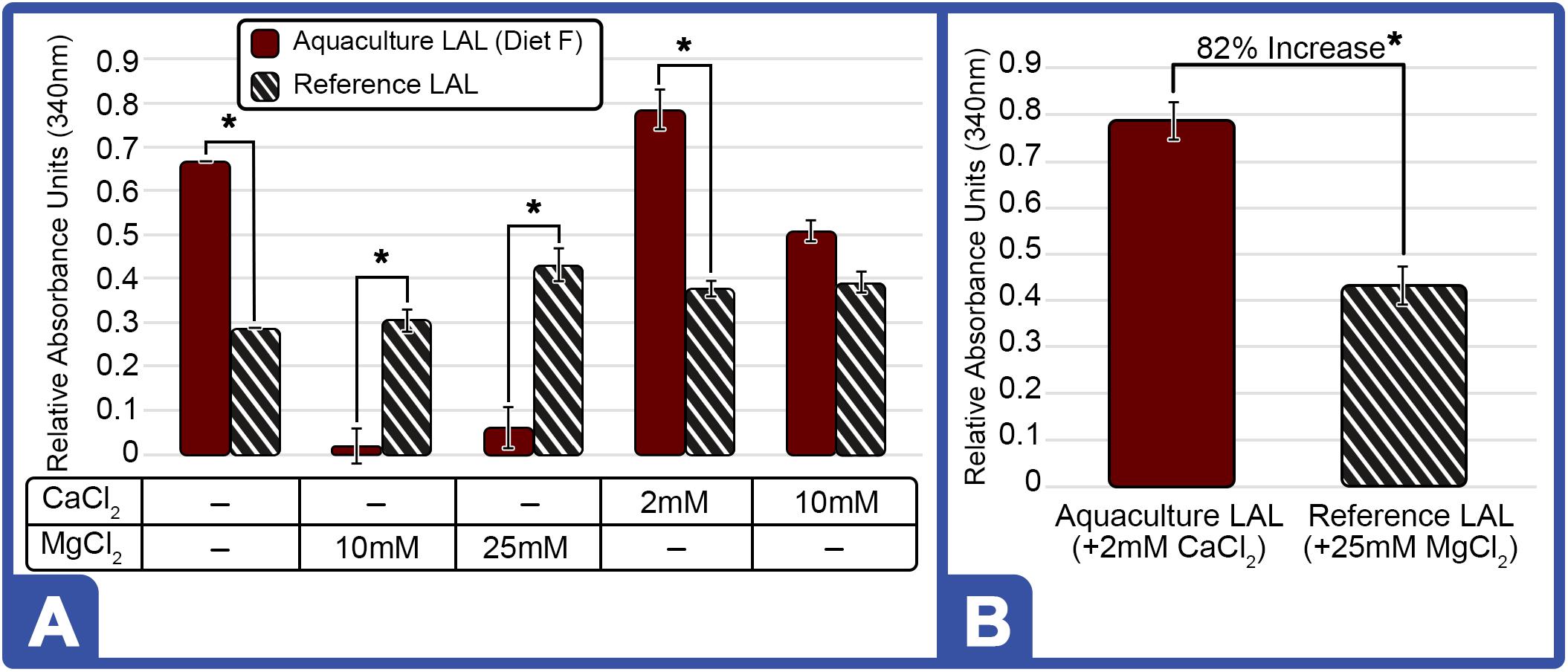
Figure 9. Effect of Different Cations on LAL-LPS Reactivity of Aquaculture and Reference LAL. (A) Effect of MgCl2 and CaCl2 addition on LAL (300 μg) from HSCs fed Diet F in Feed Study 3 vs. reference LAL at 5.0 EU/mL of LPS. LAL reactivity to endotoxins evaluated for aquaculture (Solid Bars) and reference (Striped Bars) at 340 nm on microplate reader after 1-h incubation at 37°C (B) Maximum LAL reactivity of aquaculture LAL (Diet F; Feed Study 3) vs. reference LAL at 2 mM CaCl2 and 25 mM MgCl2, respectively. Results are represented as mean ± SD between individual LAL assays performed in triplicate. *p < 0.05 of aquaculture LAL compared to the reference LAL for the respective cation conditions evaluated.
Experimentally derived concentrations of divalent cation effects were also investigated across a range of endotoxin concentrations (0.5 – 50 EU/mL). Incubation of aquaculture LAL with divalent cations increased sensitivity at low endotoxin concentrations (0.5 EU/mL; Figure 10). As presented in Figure 9A, aquaculture LAL reactivity to moderate LPS levels (5.0 EU/mL) was also amplified by CaCl2 (2 mM); but it was reduced with MgCl2 (10 and 25 mM), as well as CaCl2 (10 mM). Notably, the addition of divalent cations at both concentrations reduced LAL reactivity at high levels of LPS (50 EU/mL; Figure 10). While slight improvements were shown at lower levels of LPS with 2 mM Ca++, inclusion of divalent cations did not significantly enhance aquaculture LAL assay performance.
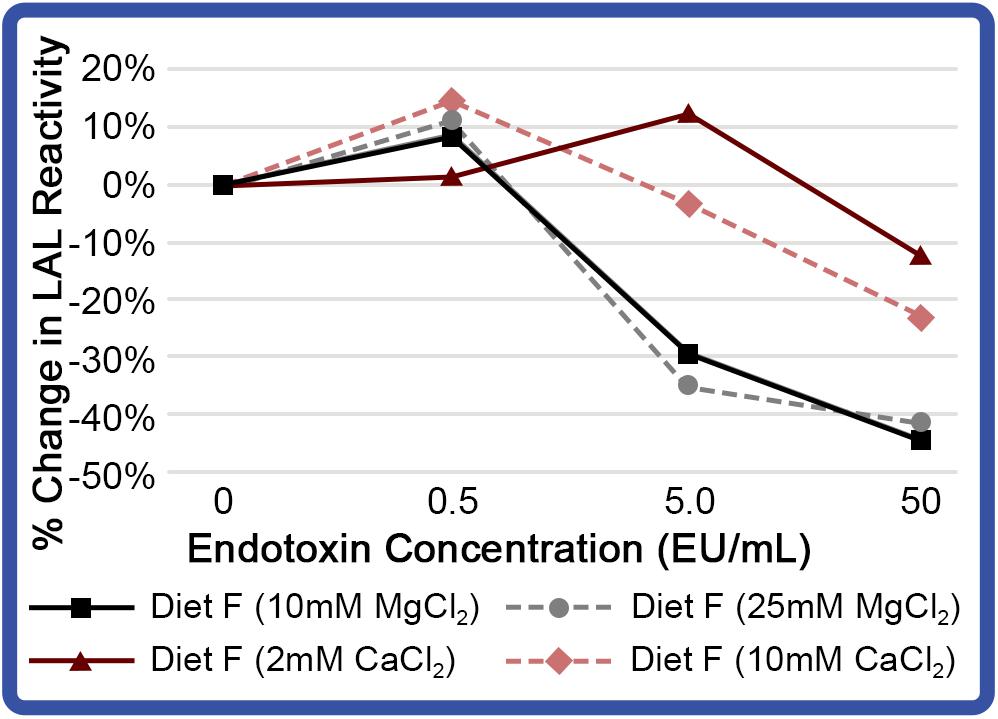
Figure 10. Effect of different cations on reactivity of aquaculture LAL at different endotoxin concentrations. LAL extract (300 μg) derived from HSCs fed Diet F in Feed Study 3 analyzed to determine effect of MgCl2 (10 and 25 mM) and CaCl2 (2 and 10 mM). LAL was incubated with a range of endotoxin concentrations (0 – 50 EU/mL) for 1 h at 37°C. Background absorbance was subtracted from each at 0 EU/mL, as addition of CaCl2 and MgCl2 resulted in minor shifts in absorption. Percent change in LAL reactivity shown vs. Diet F LAL free of divalent cations.
Discussion
Globally, four HSC species have suffered population declines as a result of increased harvesting for biomedical sterility testing and bait, commercial fishing bycatch, loss of spawning habitat, pollution, and declining oceanic ecosystems (Berkson et al., 2009; Gauvry, 2015; Krisfalusi-Gannon et al., 2018). Furthermore, commercial LAL reactivity (from various suppliers) has been shown to vary from batch to batch due to spawning-induced stress and diet limitations that reduce sensitivity (Sullivan and Watson, 1974; Owings et al., 2019). Sustainable HSC aquaculture could afford a more consistent LAL supply, eliminate HSC mortality associated with wild-capture bleeding practices (10–30%), and provide the potential to enhance reagent quality and reactivity. This study suggests that highly reactive LAL can be produced from HSC aquaculture on a year-round basis. The HSCs have continued to thrive in aquaculture after completion of this feed evaluation and after more than 1 year of captivity on routine feedings of Diet F. All HSCs have maintained their weight (+3.2% change) and the LAL isolated from this cohort remains 2.36 ± 0.44 times higher than reference LAL. To date, a key barrier to successful husbandry has been ascribed to nutritional imbalances, dietary deficiencies, captivity-induced diseases, such as panhypoproteinemia, and increased mortality rates over time (Smith and Berkson, 2005; Nolan and Smith, 2009; Carmichael and Brush, 2012). These feed studies suggest that diet directly affects LAL sensitivity and amebocyte protein production and/or prevention of breakdown.
In the wild, most aquatic animals are opportunistic feeders and consume a diverse array of prey; whereas, captive animal diets characteristically lack relative diversity and quality of feed products and can affect animal health through digestive microbiota. Captive adult HSCs reportedly have been provided natural sources of feed to mimic wild foraging; however, such diets can be costly, seasonally limited, and yield variable nutrient quality and composition due to storage and other variables. Specifically, in this study a comprehensive strategy was developed to leverage affordable, sustainable, and nutritionally diverse feed sources to satisfy metabolic requirements, promote cellular health, aid in harvest recovery, and support long-term husbandry. Wherein, some experimental diets promoted overall cellular health throughout the course of the trial by maintaining sufficient HSC hemocyanin levels and LAL sensitivity, both of which play a central role in immunity to defend against pathogens (Nagai et al., 2001).
Conversely, manufactured feeds are typically economical, abundant and shelf-stable; however, they are generally formulated to foster rapid growth rates in lieu of animal vitality, per se. As illustrated in this study, LAL reactivity from HSCs given manufactured feed declined over the three 8-week feeding trials. Another recent aquaculture study has also validated the link between diet and adult HSC hemolymph enzyme activity, indicating that serum hemocyanin levels increased at a faster rate with low levels of copper than when higher levels were added to feed formulations (Xu et al., 2020).
The feeding rationale for this study focused on economical, diverse sources that met the nutritional requirements of other aquatic invertebrates with respect to maintenance, digestibility, and utilization (i.e., 15–32% protein and 4–8% lipid composition; Aranyakananda and Lawrence, 1993; Unnikrishnan and Paulraj, 2010). HSC diets were also optimized to ensure that macronutrients (i.e., protein and lipids) and micronutrients (e.g., Vitamins B, C, E, and K, copper) were available for proper hemocyanin production, as such any deficiencies may be exacerbated with hemolymph extraction. All trial diets, with the exception of Diet A (commercially manufactured aquafeed), contained natural probiotics. However, further studies are required to characterize such probiotics in the nutritional blend to understand whether and how each enhances the HSC immune response.
Study feeds were also developed to satisfy required daily protein levels before reaching maximum energy requirements (Joules). The energy maintenance requirement for adult HSCs ranged between 153 and 235 kJ per kg of body weight per day as determined by pre-trial feed studies, which was similar to that of juvenile HSCs (224 kJ) and Pacific White Shrimp (23 kJ for maintenance and up to 345 kJ for maximum growth) (Kuresh and Davis, 2000; Lupatsch et al., 2008; Tzafrir-Prag et al., 2010). For comparison, adult humans require 135–195 kJ/kg/day and fish, 17–75 kJ/kg/day (Wilson and Halver, 1986; National Research Council, 1993; United Nations University, and World Health Organization, 2004; National Research Council, 2011). The protein levels in the hemolymph did not drop below the protein reference interval (3.4–11.7 mg/mL) over the feeding trial (or to date) and, all of the animals remained healthy (100% survival), suggesting that HSCs can be maintained in long-term captivity without risk of panhypoproteinemia using these diet formulations.
In the presence of high LPS concentrations (50 EU/mL, saturation levels), aquaculture LAL titrations displayed greater reactivity than the reference LAL (Figures 2–5 and Table 2). LAL derived from HSCs fed Diets A and E in Feed Study 1 also had higher levels of clotting factors per μg than the reference LAL and revealed 2X greater clot formation at the same total protein concentrations after 1-h of incubation. Thus, the LAL coagulation cascade appeared to demonstrate a zero-order enzyme kinetic reaction in which rates were constant; such that, at a saturation substrate level, the reaction was directly proportional to the amount of enzyme present. This finding suggests that the aquaculture LAL had greater LPS-reactivity at higher endotoxin concentrations due to higher amounts of one or more of the clotting factors than in the reference.
Further, the lag in coagulin formation from LAL derived from the Diet A and B cohorts may have been caused by the slow disassociation of an enzyme-bound inhibitor upon dilution of the clotting enzymes in the assay. However, the lower reactivity in the reference LAL was not consistent with the presence of an inhibitor, as the reaction rate at lower LPS concentrations (e.g., 0.5 EU/mL) was similar to that of the aquaculture LAL (Figure 2A).
Notably, the HSC cohort fed Diet F showed the greatest level of LAL reactivity and concentration of clotting factors among all feed groups in Feed Study 3 (Figures 4, 6–9). Results in Figures 3, 4 indicate lower levels of one or more of the clotting enzymes, Factor C, Factor B, or the proclotting enzymes in reference LAL compared to aquaculture LAL; whereby, clot formation (product) was weaker after a 1-h LPS saturation-level incubation (50 EU/mL). Western blot analysis also yielded lower clotting factors in the reference LAL than in the aquaculture LAL (Diet F).
In Feed Study 3, low LAL response also corresponded to low hemocyanin levels (Figures 4A,D), yet the highest LAL response in Feed Study 1 was observed in the HSC cohort fed Diet E with the lowest hemocyanin levels. Across all feed cohorts, amebocyte density remained consistent irrespective of changes in LAL reactivity. These results suggest that LAL reactivity may not be linked to hemocyanin levels or amebocyte density; rather, it appeared to be connected to diet, such that healthier amebocytes produced higher concentrations of immunologically essential protein factors. The variance between the 6 HSCs within the same tank was low; given that the amebocyte levels stayed consistent within each cohort between each feed trial, this health parameter served as a consistent internal control in the experimental design. Thus, variations in LAL between trials appear to be significant and did not arise from random environmental factors.
A gradual decline in LAL response (Table 4) was also observed in HSCs that were provided the same initial feed source across all three 8-week studies (Table 1; Cohort 1 – Diet A and Cohort 3 – Diet E). Whereas, the increase in diversity of natural sources between Feed Trials 1 through 3 (Tables 1, 2; HSC Cohort 2) corresponded to a consistent increase in LAL reactivity [Diet B (2 sources): 1.1X < Diet C (6 sources): 1.4X < Diet D (12 sources): 1.6X] over the 6-month period. Without optimization, long term rearing on a fixed feed source may not deliver the complexity or essential nutrients needed to maintain animal vigor, as frequently observed in captive animals (Worthy, 2001).
While the natural feed diets and Diet F contained fewer calories (Table 2; gross energy) and higher moisture content than the manufactured commercial feed, they resulted in higher quality LAL (as measured by LPS-reactivity and protein concentration). While Diet E was higher in caloric levels than natural feeds (Diet B–D) and Diet F but had a similar moisture content, hemocyanin levels and LAL reactivity from that cohort declined over the course of multiple feed trials. These findings suggest that nutritional complexity, and the bioavailability of the feed ingredients, may be key drivers to maintaining and safeguarding HSC wellbeing, rather than total caloric density, at least until the nutritional requirements of the HSC have been fully elucidated.
Divalent cations play an essential role in stabilizing LPS dimerization via cross-links, which is thought to be required for LPS biological activity. In the presence of cation chelating agents in vitro, LPS dimers are liberated into monomers, which have been shown to be unresponsive to LAL (Tsuchiya, 2019). In this study, aquaculture LAL was prepared following standard SOPs; whereby, amebocytes were washed in 0.5 M NaCl prior to lysis in water. The presence of 0.15 M NaCl has been shown to reduce LAL reactivity; however, the addition of divalent cations (CaCl2 or MgCl2) can reverse this inhibition and increase its reactivity (Sullivan and Watson, 1974). In fact, the addition of divalent cations to aquaculture LAL preparations resulted in greater reactivity at low endotoxin levels; but remarkably, a slight decrease was seen with specific cations and concentrations as endotoxin levels increased above 5.0 EU/mL. As expected, the addition of divalent cations also increased the reactivity of the reference LAL preparations, as previously described (Sullivan and Watson, 1974). However, under optimal salt conditions for each lysate, aquaculture LAL nonetheless outperformed the reference LAL (Figures 2, 9 mM CaCl2 and 25 mM MgCl2, respectively). It appears that variables in amebocyte lysate preparations can affect the biochemical properties of LAL; therefore, the optimization of LAL extraction methods, buffer formulation, and lyophilization (for shelf-life stability) are planned for future studies to increase LAL sensitivity.
These observations of dietary effects on coagulation and HSC hemolymph clotting factors appear to share similarities with those of other organisms. For example, Limulus Factor C, Factor B, and proclotting enzyme suggest functional and evolutionary parallels with vertebrate complement factors and certain Vitamin-K-dependent coagulation factors (e.g., mammalian Factors VII, IXa, and X). The activity of human coagulation Factor VII (a homolog to Limulus Factor C) is regulated by macronutrients with respect to total lipid intake (Miller et al., 1998). Vitamin K is required for normal human blood coagulation and tyrosine kinase activation (and phosphorylation), which plays a central role in cell growth and metabolism (Saxena et al., 1997; Vermeer, 2012). In another vitamin-dependent metabolic process associated with coagulation, calcium, cholecalciferol (Vitamin D3) and retinoic acid (Vitamin A) control the protein expression of Kallikrein (a homolog to Limulus Factor B) in human epidermal keratinocytes (Morizane et al., 2010). Similarly, enhanced human coagulation Factor IXa (a homolog to Limulus proclotting enzyme) activity has been demonstrated in the presence of calcium and magnesium (Sekiya et al., 1996; Agah and Bajaj, 2009).
In this study, diet was specifically shown to influence the biochemical properties, concentration, and reactivity of LAL clotting factors. The relative abundance and ratios of Factor C, Factor B, and proclotting enzyme varied across diets and corresponded to protein expression of amebocyte clotting factors. Factor B was present at a higher concentration in the aquaculture LAL from all cohorts relative to reference LAL. However, the amount of Factor B did not directly correlate to LAL reactivity and may not be a rate-limiting factor in the HSC coagulation cascade. Whereas, higher Factor C and proclotting enzyme protein levels correlated to increased LAL reactivity. The LAL derived from HSCs fed Diet E demonstrated the least response and contained the lowest levels of Factor C and proclotting enzyme; thus, suggesting that these clotting factors may be rate-limiting component(s) of the LAL cascade and therefore determinants of the overall LAL reaction.
Diet also appeared to affect proclotting enzymes in the aquaculture HSC amebocytes. While a range of sizes (38, 54, 60, and >150 kDa) and amino acid compositions of the proclotting enzymes from North American (L. polyphemus) and Asian (T. gigas, T. tridentatus, and C. rotundicauda) HSCs have been described (Roth and Levin, 1992), variations of the proclotting factors were detected among the different feed groups. Neither glycosylation nor phosphorylation were responsible for the observed differences (Figure 9). However, an evolutionary basis for clotting enzyme size variations between different HSC species might explain why the beta-glucan-sensitive Factor G pathway is active in some HSC species and not others.
When Factor C is bound to the amebocyte membrane and activated by LPS in vivo, it can trigger a G-protein-coupled receptor (GPCR) signal transduction cascade, leading to the degranulation of the amebocyte and the release of defense molecules, including the zymogens of the coagulation cascade (Ariki et al., 2008). Most GPCR and receptor-mediated signaling pathways are regulated by phosphorylation (Lefkowitz and Whalen, 2004). For example, in HSCs the phosphorylation of both visual arrestin and its respective receptor (via a calcium/calmodulin-dependent protein kinase) plays a critical role in uncoupling membrane receptors from triggering GPCR-dependent cascades (Calman et al., 1996).
To date, a possible role of phosphorylation of Factor C-mediated amebocyte exocytosis has not been fully characterized, but this work has now shown that Factor C is a target of phosphorylation (Figure 5B). As illustrated in Figure 6, the L-chain of Factor C in the aquaculture LAL migrated at a higher molecular weight in SDS-Tris glycine gels than the Factor C (L-chain) in the reference LAL. The difference in migration was attributed to the phosphorylation of Factor C in aquaculture LAL (Figure 6D). In addition, AP treatment of the aquaculture-derived LAL did not affect the mobility of the B-chain subunit of Factor C, suggesting that the phosphorylation site(s) in the L-chain of Factor C is located in the A-chain subunit (Figures 6, 7). Notably, LAL protein phosphorylation modification of Factor C was present in all aquaculture HSC cohorts regardless of feed inputs, but not in the reference LAL. Factor C in LAL sourced directly from the wild (“wild type”) HSCs and collected within 1 h of habitat removal was also shown to be phosphorylated (data not shown), suggesting that this modification is not a consequence of indoor aquaculture or experimentally diets, per se. The observation that Factor C was not phosphorylated in the reference lysate would require additional information with respect to product preparation and possible additives to draw further conclusions. Future analysis is directed toward complete characterization of Factor C phosphorylation observed in the aquaculture LAL.
The role of glycosylation in protein folding, stability, reactivity, and macromolecular interactions has been well documented (Medzihradszky, 2005; Kosloski et al., 2009), including increased sensitivity to endotoxins with glycosylation of recombinant Factor C (Mizumura et al., 2017). As expected, Factor C in both reference and aquaculture LAL was glycosylated in the H-chain region (Figures 5B, 6C). Since the activated Factor C B-chain was not found to be glycosylated in the reference LAL, Factor C L-chain glycosylation may occur in the A-chain subunit. The B-chain of intact Factor C has two predicted glycosylation sites (Figure 5A), but whether glycosylation modifications remain on the B-chain after Factor C activation has not been reported.
Remarkably, PNGase treatment of the Factor C L-chain caused an upward shift (not the expected downward shift) in mobility of this subunit derived from the reference LAL but not in the aquaculture LAL (Figure 6C). Post-translational modifications like phosphorylation or glycosylation of proteins typically change protein hydrophobicity and result in a slower migration. In some circumstances, highly glycosylated proteins that retain globularity (less frictional drag) have migrated faster; whereby, the glycosylation branches cannot be linearized after denaturation (Rath et al., 2009; Schwarz and Aebi, 2011). This may be the case for the Factor C L-chain in the reference LAL in this study (Figure 6C).
It further appears that Factor C can be modified by either glycosylation or phosphorylation and that it most likely occurs in the A-chain region of the L-chain, since these post-translational modifications were not detected in the B-chain subunit of activated Factor C (i.e., the A-chain was not detectable by Western blot analysis). Conversely, these modifications could instead reside in the B-chain and be removed after Factor C activation and L-chain conversion to the A- and B-chain forms (Figures 5, 6). Future studies will be directed at describing the observed modifications of the Factor C protein.
The differences in the type of post-translational modification of Factor C (e.g., glycosylation and phosphorylation) in aquaculture versus reference LAL may also be directly related to those seen in clotting activity between the preparations (Table 2). As the experimental feeds are high in protein and lipids but low in carbohydrates (Table 1), it would follow that the quantity and quality of carbohydrates in the diets could lead to lower blood glucose levels. Whereas, diets low in carbohydrates have been shown to reduce in vivo (non-enzymatic) protein glycation (Guilbaud et al., 2016). The significance of glycosylation and phosphorylation of Factor C in aquaculture-derived LAL and the relationship to the diet of the HSC nonetheless remains to be elucidated.
While Factor B was found to be a glycoprotein in both aquaculture and reference LAL, glycosylation was detected only in the double-chain form of Factor B in the H-chain and not in the intact single-chain form of Factor B (Figures 5B, 7). Whether glycosylation of Factor B triggers the Factor C dependent nicking of the full-length single-chain form into the double-chain form of Factor B, or whether glycosylation is downstream of the nicking process that generates the double-chain form remains to be determined (Figure 5B). The presence of the double-chain form of Factor B typically found in standard LAL preparations is thought to be a result of trace contamination of Factor C generated during the purification process (Nakamura et al., 1986).
Conclusion
We consider this work to be important in the HSC global context and the potential role of aquaculture for the future of species viability. That is, given the valuable resources derived from these arthropods and the potential for long-term aquaculture solutions, a meticulous assessment of alternative feed strategies with respect to the quality of the resources for which they have been selected is both rational and necessary. This study demonstrated that protein-dense amebocytes, optimal hemocyanin levels, and high-quality LAL derived from HSCs in aquaculture can be produced with functional nutrient-rich feed. Aquaculture LAL contained higher amounts of clotting factors per μg of total protein compared to reference LAL preparations and could thus yield higher reactivity and therefore more tests per unit of harvested hemolymph. HSC sustentation and reproductive activities also continued during captivity, such that all aspects of natural behavior were observed without apparent stress or decline in wellbeing.
This study highlights the use of amebocyte protein components as novel biomarkers to objectively measure HSC nutritional status with respect to the intake of dietary constituents for usefulness in future studies. The data show that the expression levels and chemical modifications of such biomarkers are directly affected by feed inputs and therefore may provide a new tool to evaluate dietary patterns, especially with HSCs and possibly other species. We found that LAL reactivity to an endotoxin standard was similar in the indoor HSCs compared to LAL sourced directly from wild-type HSC collected within 1 h of habitat removal. However, LAL prepared from husbanded HSCs had higher reactivity compared to commercially available LAL preparations (reference) that are also sourced from wild type HSCs. Additional studies would be needed to identify and assess possible variations in reference LAL preparation compared to these methods that do not involve lyophilization. In addition, batch-to-batch and seasonal variations in commercial LAL prepared from wild harvested HSC populations have been shown to differ in their sensitivity to LPS (Lindberg et al., 1972), although these findings may not reflect differences in sensitivity to LPS per se but reactivity related to the potency of LAL. Controlled-environmental aquaculture using diverse feedstocks may alleviate these LAL variations and result in more reproducibility and batch-to-batch product consistencies while producing more robust LAL reactivity.
Ultimately, this work has shown that nutrition imparts an effect on the LAL derived from an aquaculture cohort. The research further suggests that highly reactive LAL can be reproducibly prepared from aquaculture HSCs on a year-round basis if a properly defined and economical dietary regimen is established. As such, aquaculture and an enriched feeding strategy for a finite cohort of HSCs representing a fraction of the current annual wild catch could satisfy the global demand for LAL biomedical testing over the long term. Thus, enduring HSC aquaculture and the resulting raw material quality represent a promising, sustainable new supply paradigm for the biomedical industry.
In turn, HSC husbandry also promises unparalleled conservation advantages for dwindling wild populations and dependent species, including myriad migrating shorebirds.
With respect to future research, the elemental HSC immune system based on a single circulating cell also provides an elegant model to investigate the effects of nutrition on immunity and vigor at an ingredient and cellular level. Such studies will center on further examination of the relationship between nutrition and production and modification of specific, bioactive LAL proteins. Notably, these strategies to ensure wellbeing in captivity could also be applied in other sectors, from agriculture stocks to companion animals and zoos, to human medicine, etc.
Following the submission of this manuscript and during the peer-review process, it was noteworthy that the United States Pharmacopeia (USP) ruled to retain LAL as the industry BET amid new demands for drug and vaccine development and manufacturing emerging from the novel coronavirus pandemic, suggesting that LAL will remain the standard for the foreseeable future (United States Pharmacopeia, 2020a, b).
Data Availability Statement
The datasets generated for this study are available on request to the corresponding author.
Author Contributions
RT-K, AD, TB, MG, and KD conceived the project. RT-K, AD, TB, and KD developed the research objectives and experimental plan. RT-K, KD, CK, and TB verified the materials and methods. RT-K, AD, LR, and KD conducted the research. KD and AD supervised the work. All the authors prepared analysis of the primary research and contributed to the final writing of the manuscript. JB and SA provided the research support for the nutritional effects on performance and physiology and alternative feedstuff development for sustainable production and safety. MG performed the editing of the final manuscript.
Funding
The authors would like to acknowledge the following funding sources: National Science Foundation SBIR Grant program (Grant # 1819562); North Carolina Board of Science & Technology; One North Carolina SBIR Matching Funds Program; and North Carolina Sea Grant (Grant # 2017-R/MG-1712).
Conflict of Interest
AD, RT-K, TB, and LR are employed by Kepley BioSystems, Inc. CK was a founding member of Kepley Biosystems, Inc. KD is a former employee, who was working for Kepley BioSystems, Inc. during the experimental planning, data acquisition and analysis portions of the project. MG was employed by the company ClienTell Consulting, LLC and provides consultation for Kepley BioSystems, Inc. JB is a Senior Research Scientist of Aquaculture at Archer Daniels Midland (ADM) in Decatur, IL, United States. SA is an owner of Sit, Stay, Speak Nutrition, LLC; SA serves as a scientific advisor for Kepley Biosystems, Inc. The authors alone are responsible for the content and writing of the manuscript.
Acknowledgments
We thank our peers and collaborators at: Gateway Research Park; Ecological Research and Development Group (Mayor Glenn Gauvry); Joint School of Nanoscience and Nanoengineering; National Science Foundation; North Carolina Board of Science & Technology; North Carolina Sea Grant; and the University of Georgia Marine Extension and Georgia Sea Grant. We are grateful to Dr. Eric Josephs for materials contribution and to Dr. Peter Geiduschek for technical manuscript review. We also thank Lauren Perdue, a dedicated senior at North East Guilford High School (McLeansville, NC, United States), for her daily help in measuring aquaculture system water parameters and HSC feeding.
Supplementary Material
The Supplementary Material for this article can be found online at: https://www.frontiersin.org/articles/10.3389/fmars.2020.541604/full#supplementary-material
Abbreviations
Δ, delta; ‰, parts per thousand; A-chain, alpha-chain; AF, As fed; AP, alkaline phosphatase; ALP, aquaculture lysate pool; ASMFC, Atlantic States Marine Fisheries Commission; β, beta; B-chain, beta-chain; BET, bacterial endotoxin test; BCA, bicinchoninic acid assay; b/w, body weight; BSA, bovine serum albumin; Ca++, calcium; CaCl2, calcium chloride; ° C, celsius; COCH, cochlin; dL, deciliter; DM, dry matter; CP, crude protein; HSC, Horseshoe crab; EC, electric conductivity; EU, endotoxin unit; ft, feet; g/mol, grams per moles; kDa, kilodalton; kJ, kilojoules; kJ/g, kilojoules per gram; H-chain, heavy chain; Hc, hemocyanin; LAL, Limulus amebocyte lysate; lb, pound; L-chain, light chain; LPS, lipopolysaccharide; MgCl2, magnesium chloride; mmol/L, millimoles per liter; NaCl, sodium chloride; nm, nanometer; OD, optical density; Oxy-Hc, oxyhemocyanin; g/mol, molar mass; GPCR, G-Protein-Coupled Receptor; PNGase, glycopeptidase F; ppm, parts per million; RAS, recirculating aquaculture system; rcf, relative centrifugal force; SOP, standard operating procedure; TGase, transglutaminase; Tris-HCl, tris(hydroxymethyl)aminomethane hydrochloride; μ, micro; UV-Vis, ultraviolet-visible; v/v, volume/volume; w/v, weight/volume.
References
Abelli, L., Randelli, E., Carnevali, O., and Picchietti, S. (2009). Stimulation of gut immune system by early administration of probiotic strains in Dicentrarchus labrax and Sparus aurata. Ann. N. Y. Acad. Sci. 1163, 340–342. doi: 10.1111/j.1749-6632.2008.03670.x
Agah, S., and Bajaj, S. P. (2009). Role of magnesium in factor XIa catalyzed activation of factor IX: calcium binding to factor IX under physiologic magnesium. J. Thromb. Haemost. 7:1426. doi: 10.1111/j.1538-7836.2009.03506.x
Anderson, R. L., Watson, W. H. III, and Chabot, C. C. (2013). Sublethal behavioral and physiological effects of the biomedical bleeding process on the American horseshoe crab, Limulus polyphemus. Biol. Bull. 225, 137–151. doi: 10.1086/BBLv225n3p137
Aranyakananda, P., and Lawrence, A. L. (1993). Dietary Protein and Energy Requirements of the White-Legged Shrimp, Penaeus vannamei, and the Optimal Protein to Energy Ratio. From Discovery to Commercialization. Oostende: European Aquaculture Soc, 21.
Ariki, S., Takahara, S., Shibata, T., Fukuoka, T., Ozaki, A., Endo, Y., et al. (2008). Factor C acts as a lipopolysaccharide-responsive C3 convertase in horseshoe crab complement activation. J. Immunol. 181, 7994–8001. doi: 10.4049/jimmunol.181.11.7994
Armstrong, P., and Conrad, M. (2008). Blood collection from the American horseshoe crab, Limulus polyphemus. J. Vis. Exp. 20:e958.
Atlantic States Marine Fisheries Commission (2019). Horseshoe Crab Stock Benchmark Stock Assessment and Peer Review Report. Prepared by the ASMFC Horseshoe Crab Stock Assessment Review Panel: Dr. Lawrence Jacobson (Chair), Dr. Ruth Carmichael, and Dr. Matthew Cieri. Arlington, VA: Atlantic States Marine Fisheries Commission.
Berkson, J., Chen, C. P., Mishra, J., Shin, P., Spear, B., and Zaldívar-Rae, J. (2009). “A discussion of horseshoe crab management in five countries: Taiwan, India, China, United States, and Mexico,” in Biology and Conservation of Horseshoe Crabs, eds J. Tanacredi, M. Botton, and D. Smith (Boston, MA: Springer), 465–475. doi: 10.1007/978-0-387-89959-6_29
Blanc, T. J., and Margraf, F. J. (2002). Effects of nutrient enrichment on channel catfish growth and consumption in Mount Storm Lake, West Virginia. Lakes Reserv. 7, 109–123. doi: 10.1046/j.1440-169X.2002.00184.x
Bolden, J. (2019). “Recombinant factor C in endotoxin detection and control,” in Pharma, Limulus, and Mammalian Systems, ed. K. Williams (Cham: Springer), 497–521. doi: 10.1007/978-3-030-17148-3_13
Botton, M. L. (1984). Diet and food preferences of the adult horseshoe crab Limulus polyphemus in Delaware Bay, New Jersey, USA. Mar. Biol. 81, 199–207. doi: 10.1007/BF00393118
Bryans, T. D., Braithwaite, C., Broad, J., Cooper, J. F., Darnell, K. R., Hitchins, V. M., et al. (2004). Bacterial endotoxin testing: a report on the methods, background, data, and regulatory history of extraction recovery efficiency. Biomed. Instr. Technol. 38, 73–78.
Calman, B. G., Andrews, A. W., Rissler, H. M., Edwards, S. C., and Battelle, B. A. (1996). Calcium/calmodulin-dependent protein kinase II and arrestin phosphorylation in Limulus eyes. J. Photochem. Photobiol. Biol. 35, 33–44. doi: 10.1016/1011-1344(96)07312-5
Carmichael, R. H., and Brush, E. (2012). Three decades of horseshoe crab rearing: a review of conditions for captive growth and survival. Rev. Aquacult. 4, 32–43. doi: 10.1111/j.1753-5131.2012.01059.x
Carmichael, R. H., Gaines, E., Sheller, Z., Tong, A., Clapp, A., and Valiela, I. (2009). “Diet composition of juvenile horseshoe crabs: implications for growth and survival of natural and cultured stocks,” in Biology and Conservation of Horseshoe Crabs, eds J. Tanacredi, M. Botton, and D. Smith (Boston, MA: Springer), 521–534.
Carnevali, O., Maradonna, F., and Gioacchini, G. (2017). Integrated control of fish metabolism, wellbeing and reproduction: the role of probiotic. Aquaculture 472, 144–155. doi: 10.1016/j.aquaculture.2016.03.037
Coates, C. J., Bradford, E. L., Krome, C. A., and Nairn, J. (2012). Effect of temperature on biochemical and cellular properties of captive Limulus polyphemus. Aquaculture 334, 30–38. doi: 10.1016/j.aquaculture.2011.12.029
Defoirdt, T., Boon, N., Sorgeloos, P., Verstraete, W., and Bossier, P. (2007). Alternatives to antibiotics to control bacterial infections: luminescent vibriosis in aquaculture as an example. Trends Biotechnol. 25, 472–479. doi: 10.1016/j.tibtech.2007.08.001
Dierenfeld, E. S., Katz, N., Pearson, J., Murru, F., and Asper, E. D. (1991). Retinol and α-tocopherol concentrations in whole fish commonly fed in zoos and aquariums. Zool. Biol. 10, 119–125.
Ebeling, J. M., and Timmons, M. B. II (2010). Recirculating Aquaculture. New York, NY: Cayuga Aqua Ventures LLC.
Egerton, S., Culloty, S., Whooley, J., Stanton, C., and Ross, R. P. (2018). The gut microbiota of marine fish. Front. Microbiol. 9:873. doi: 10.3389/fmicb.2018.00873
Food and Agriculture Organization (2019). Anti-Nutritional Factors within Feed Ingredients. Silver Spring, MD: Food and Drug Administration.
Food and Drug Administration (2014). Bacterial Endotoxins/Pyrogens. Inspection Technical Guides, 40. Silver Spring, MD: Food and Drug Administration.
Fukuda, S., and Ohno, H. (2014). Gut microbiome and metabolic diseases. Semin. Immunopathol. 36, 103–114. doi: 10.1007/s00281-013-0399-z
Gauvry, G. (2015). “Current horseshoe crab harvesting practices cannot support global demand for TAL/LAL: the pharmaceutical and medical device industries’ role in the sustainability of horseshoe crabs,” in Changing Global Perspectives on Horseshoe Crab Biology, Conservation and Management, eds R. Carmichael, M. Botton, P. Shin, and S. Cheung (Cham: Springer), 475–482. doi: 10.1007/978-3-319-19542-1_27
Gibson, G. R., Hutkins, R., Sanders, M. E., Prescott, S. L., Reimer, R. A., Salminen, S. J., et al. (2017). Expert consensus document: the International Scientific Association for Probiotics and Prebiotics (ISAPP) consensus statement on the definition and scope of prebiotics. Nat. Rev. Gastroenterol. Hepatol. 14:491. doi: 10.1038/nrgastro.2017.75
Grady, S. P., and Valiela, I. (2006). Stage-structured matrix modeling and suggestions for management of Atlantic horseshoe crab, Limulus polyphemus, populations on Cape Cod, Massachusetts. Estuar. Coasts 29, 685–698. doi: 10.1007/BF02784293
Guilbaud, A., Niquet-Leridon, C., Boulanger, E., and Tessier, F. J. (2016). How can diet affect the accumulation of advanced glycation end-products in the human body? Foods 5:84. doi: 10.3390/foods5040084
Hochstein, H. D. (1990). “Role of the FDA in regulating the Limulus amoebocyte lysate test,” in Clinical Applications of the Limulus Amoebocyte Lysate Test, ed. R. B. Prior (Boca Raton: CRC Press), 37–50.
Iwanaga, S. (2007). Biochemical principle of Limulus test for detecting bacterial endotoxins. Proc. Jpn. Acad. Ser. B 83, 110–119. doi: 10.2183/pjab.83.110
Iwanaga, S., Kawabata, S. I., and Muta, T. (1998). New types of clotting factors and defense molecules found in horseshoe crab hemolymph: their structures and functions. J. Biochem. 123, 1–15. doi: 10.1093/oxfordjournals.jbchem.a021894
Iwanaga, S., Miyata, T., Tokunaga, F., and Muta, T. (1992). Molecular mechanism of hemolymph clotting system in Limulus. Thromb. Res. 68, 1–32. doi: 10.1016/0049-3848(92)90124-S
Kautsky, N. (1982). Growth and size structure in a Baltic Mytilus edulis population. Mar. Biol. 68, 117–133. doi: 10.1007/BF00397599
Kawabata, S., and Muta, T. (2010). Sadaaki Iwanaga: discovery of the lipopolysaccharide-and β-1, 3-d-glucan-mediated proteolytic cascade and unique proteins in invertebrate immunity. J. Biochem. 147, 611–618. doi: 10.1093/jb/mvq026
Kobayashi, Y., Takahashi, T., Shibata, T., Ikeda, S., Koshiba, T., Mizumura, H., et al. (2015). Factor B is the second lipopolysaccharide-binding protease zymogen in the horseshoe crab coagulation cascade. J. Biol. Chem. 290, 19379–19386. doi: 10.1074/jbc.M115.653196
Kosloski, M. P., Miclea, R. D., and Balu-Iyer, S. V. (2009). Role of glycosylation in conformational stability, activity, macromolecular interaction and immunogenicity of recombinant human factor VIII. J. Am. Assoc. Pharm. Sci. 11:424. doi: 10.1208/s12248-009-9119-y
Krisfalusi-Gannon, J., Ali, W., Dellinger, K., Robertson, L., Brady, T. E., Goddard, M. K., et al. (2018). The role of horseshoe crabs in the biomedical industry and recent trends impacting species sustainability. Front. Mar. Sci. 5:185. doi: 10.3389/fmars.2018.00185
Kuresh, N., and Davis, D. A. (2000). “Metabolic requirement for protein by pacific white shrimp Litopenaeus vannamei,” in Advances in Aquaculture Nutrition V. Memories of the V International Symposium on Aquaculture Nutrition, eds L. E. Cruz-Suárez, D. Ricque-Marie, M. Tapia-Salazar, M. A. Olvera-Novoa, and R. Civera-Cerecedo (Mérida: Auburn University).
Kwan, B. K., Chan, A. K., Cheung, S. G., and Shin, P. K. (2014). Hemolymph quality as indicator of health status in juvenile Chinese horseshoe crab Tachypleus tridentatus (Xiphosura) under laboratory culture. J. Exp. Mar. Biol. Ecol. 457, 135–142. doi: 10.1016/j.jembe.2014.04.011
Lefkowitz, R. J., and Whalen, E. J. (2004). β-arrestins: traffic cops of cell signaling. Curr. Opin. Cell Biol. 16, 162–168. doi: 10.1016/j.ceb.2004.01.001
Lindberg, R. B., Pruit, B., Inge, W., and Mason, A. (1972). Natural variation in sensitivity of Limulus amoebocyte lysate to endotoxin. Fed. Proc. 31:A791.
Lindequist, U. (2016). Marine-derived pharmaceuticals–challenges and opportunities. Biomol. Therap. 24:561. doi: 10.4062/biomolther.2016.181
Lupatsch, I., Cuthbertson, L., Davies, S., and Shields, R. J. (2008). “Studies on energy and protein requirements to improve feed management of the Pacific white shrimp, Litopenaeus vannamei. Management, 281-295,” in Advances in Aquaculture Nutrition VII, eds L. E. Cruz Suárez, D. R. Marie, M. T. Salazar, M. G. N. López, D. V. A. Cavazos, J. P. Lazo, et al. (Monterrey: Universidad Autonoma de Nuevo Leon), 281–295.
Malve, H. (2016). Exploring the ocean for new drug developments: Marine pharmacology. J. Pharm. Bioall. Sci. 8:83. doi: 10.4103/0975-7406.171700
Matsuda, Y., Koshiba, T., Osaki, T., Suyama, H., Arisaka, F., Toh, Y., et al. (2007). An arthropod cuticular chitin-binding protein endows injured sites with transglutaminase-dependent mesh. J. Biol. Chem. 282, 37316–37324. doi: 10.1074/jbc.M705731200
McRoberts Sales Co. Inc (2014). Zoo and Aquarium Species Directory. Ruskin, FL: McRoberts Sales Co. Inc.
Medzihradszky, K. F. (2005). Characterization of protein N−glycosylation. Methods Enzymol. 405, 116–138. doi: 10.1016/S0076-6879(05)05006-8
Miller, G. J., Mitropoulos, K. A., Nanjee, M. N., Howarth, D. J., Martin, J. C., Esnouf, M. P., et al. (1998). Very low activated Factor VII and reduced Factor VII antigen in familial abetalipoproteinaemia. Thromb. Haemost. 80, 233–238. doi: 10.1055/s-0037-1615179
Mizumura, H., Ogura, N., Aketagawa, J., Aizawa, M., Kobayashi, Y., Kawabata, S. I., et al. (2017). Genetic engineering approach to develop next-generation reagents for endotoxin quantification. Innate Immun. 23, 136–146. doi: 10.1177/1753425916681074
Morizane, S., Yamasaki, K., Kabigting, F. D., and Gallo, R. L. (2010). Kallikrein expression and cathelicidin processing are independently controlled in keratinocytes by calcium, vitamin D3, and retinoic acid. J. Invest. Dermatol. 130, 1297–1306. doi: 10.1038/jid.2009.435
Muta, T., and Iwanaga, S. (1996). The role of hemolymph coagulation in innate immunity. Curr. Opin. Immunol. 8, 41–47. doi: 10.1016/S0952-7915(96)80103-8
Nagai, T., Osaki, T., and Kawabata, S. I. (2001). Functional conversion of hemocyanin to phenoloxidase by horseshoe crab antimicrobial peptides. J. Biol. Chem. 276, 27166–27170. doi: 10.1074/jbc.M102596200
Nakamura, T., Horiuchi, T., Morita, T., and Iwanaga, S. (1986). Purification and properties of intracellular clotting factor, Factor B, from horseshoe crab (Tachypleus tridentatus) hemocytes. J. Biochem. 99, 847–857. doi: 10.1093/oxfordjournals.jbchem.a135545
National Research Council (1993). Nutrient Requirements of Fish, Nutrient Requirements of Domestic Animals. Washington, DC: National Academy Press.
National Research Council (2011). Nutrient Requirements of Fish and Shrimp. Washington, DC: National Academy Press.
Nickerson, K. W., and Van Holde, K. E. (1971). A comparison of molluscan and arthropod hemocyanin-I. Circular dichroism and absorption spectra. Comp. Biochem. Physiol. B Biochem. 39, 855–872.
Nolan, M. W., and Smith, S. A. (2009). “Clinical evaluation, common diseases, and veterinary care of the horseshoe crab, Limulus polyphemus,” in Biology and Conservation of Horseshoe Crabs, eds J. Tanacredi, M. Botton, and D. Smith (Boston, MA: Springer), 479–499. doi: 10.1007/978-0-387-89959-6_30
Novitsky, T. J. (2009). “Biomedical applications of Limulus amebocyte lysate,” in Biology and Conservation of Horseshoe Crabs, eds J. T. Tanacredi, M. L. Botton, and D. Smith (Boston, MA: Springer), 315–329. doi: 10.1007/978-0-387-89959-6_20
Osaki, T., and Kawabata, S. (2004). Structure and function of coagulogen, a clottable protein in horseshoe crabs. Cell. Mol. Life Sci. 61, 1257–1265. doi: 10.1007/s00018-004-3396-5
Osaki, T., Okino, N., Tokunaga, F., Iwanaga, S., and Kawabata, S. I. (2002). Proline-rich cell surface antigens of horseshoe crab hemocytes are substrates for protein cross-linking with a clotting protein coagulin. J. Biol. Chem. 277, 40084–40090. doi: 10.1074/jbc.M206773200
Owings, M., Chabot, C., and Watson, W. III (2019). Effects of the biomedical bleeding process on the behavior of the American horseshoe crab, Limulus polyphemus, in its natural habitat. Biol. Bull. 236, 207–223. doi: 10.1086/702917
Qi, Z., Zhang, X. H., Boon, N., and Bossier, P. (2009). Probiotics in aquaculture of China—current state, problems and prospect. Aquaculture 290, 15–21. doi: 10.1016/j.aquaculture.2009.02.012
Rath, A., Glibowicka, M., Nadeau, V. G., Chen, G., and Deber, C. M. (2009). Detergent binding explains anomalous SDS-PAGE migration of membrane proteins. Proc. Natl. Acad. Sci. U.S.A. 106, 1760–1765. doi: 10.1073/pnas.0813167106
Rigdon, R. H., and Drager, G. A. (1955). Thiamine deficiency in sea lions (Otaria californiana) fed only frozen fish. J. Am. Vet. Med. Assoc. 127, 453–455.
Roslansky, P. F., and Novitsky, T. J. (1991). Sensitivity of Limulus amebocyte lysate (LAL) to LAL-reactive glucans. J. Clin. Microbiol. 29, 2477–2483. doi: 10.1128/JCM.29.11.2477-2483.1991
Roth, R. I., and Levin, J. (1992). Purification of Limulus polyphemus proclotting enzyme. J. Biol. Chem. 267, 24097–24102.
Saxena, S. P., Fan, T., Li, M., Israels, E. D., and Israels, L. G. (1997). A novel role for vitamin K1 in a tyrosine phosphorylation cascade during chick embryogenesis. J. Clin. Invest. 99, 602–607. doi: 10.1172/JCI119202
Schreibman, M. P., and Zarnoch, C. B. (2009). “Aquaculture methods and early growth of juvenile horseshoe crabs (Limulus polyphemus),” in Biology and Conservation of Horseshoe Crabs, eds J. T. Tanacredi, M. L. Botton, and D. Smith (Boston, MA: Springer), 501–511. doi: 10.1007/978-0-387-89959-6_31
Schwarz, F., and Aebi, M. (2011). Mechanisms and principles of N-linked protein glycosylation. Curr. Opin. Struct. Biol. 21, 576–582. doi: 10.1016/j.sbi.2011.08.005
Sekiya, F., Yoshida, M., Yamashita, T., and Morita, T. (1996). Magnesium (II) is a crucial constituent of the blood coagulation cascade potentiation of coagulant activities of factor IX by Mg ions. J. Biol. Chem. 271, 8541–8544. doi: 10.1074/jbc.271.15.8541
Shelley, C., and Lovatelli, A. (2011). Mud Crab Aquaculture: A Practical Manual. FAO Fisheries and Aquaculture Technical Paper. Rome: FAO, 567.
Smith, S. A., and Berkson, J. (2005). Laboratory culture and maintenance of the horseshoe crab (Limulus polyphemus). Lab. Anim. 34, 27–35. doi: 10.1038/laban0705-27
Sullivan, J. D., and Watson, S. W. (1974). Factors affecting the sensitivity of Limulus lysate. Appl. Environ. Microbiol. 28, 1023–1026. doi: 10.1128/AEM.28.6.1023-1026.1974
Sullivan, J. D., and Watson, S. W. (1978). U.S. Patent No. 4,107,077. Washington, DC: U.S. Patent and Trademark Office.
Timmons, M. B. (1994). Aquaculture reuse systems: engineering design and management. Dev. Aquacult. Fish. Sci. 27:346.
Tinker-Kulberg, R., Dellinger, A., Gentit, L. C., Fluech, B. A., Wilder, C. A., Spratling, I. L., et al. (2020a). Evaluation of indoor and outdoor aquaculture systems as alternatives to harvesting hemolymph from random wild capture of horseshoe crabs. Front. Mar. Sci. doi: 10.3389/fmars.2020.00153 [Epub ahead of print].
Tinker-Kulberg, R., Dellinger, K., Brady, T., Robertson, L., Levy, J., Abood, S., et al. (2020b). Horseshoe crab aquaculture as a sustainable endotoxin testing resource. Front. Mar. Sci. 7:153.
Torrecillas, S., Montero, D., and Izquierdo, M. (2014). Improved health and growth of fish fed mannan oligosaccharides: potential mode of action. Fish Shellfish Immunol. 36, 525–544. doi: 10.1016/j.fsi.2013.12.029
Tsuchiya, M. (2019). Mechanism of low endotoxin recovery caused by a solution containing a chelating agent and a detergent. Immun. Res. 15, 1–10. doi: 10.35248/1745-7580.19.15.166
Tzafrir-Prag, T., Schreibman, M. P., Lupatsch, I., and Zarnoch, C. B. (2010). Preliminary studies of energy and protein requirements of Atlantic horseshoe crabs, Limulus polyphemus, grown in captivity. J. World Aquacult. Soc. 41, 874–883. doi: 10.1111/j.1749-7345.2010.00430.x
United Nations University, and World Health Organization (2004). Human Energy Requirements: Report of a Joint FAO/WHO/UNU Expert Consultation: Rome, 17-24 October 2001 (Vol. 1). Rome: Food and Agriculture Organization.
United States Department of Agriculture (2019). FoodData Central. Washington, DC: United States Department of Agriculture.
United States Pharmacopeia (2020a). USP Provides Guidelines for Recombinant Factor C (rFC) A Non-Animal-Derived Reagent Critical to Development of Vaccines and Other Sterile Pharmaceutical Products. Rockville, MD: United States Pharmacopeia.
United States Pharmacopeia (2020b). General Chapter Prospectus: Use of Recombinant Reagents in Bacterial Endotoxins Test. Rockville, MD: United States Pharmacopeia.
Unnikrishnan, U., and Paulraj, R. (2010). Dietary protein requirement of giant mud crab Scylla serrata juveniles fed iso-energetic formulated diets having graded protein levels. Aquacult. Res. 41, 278–294. doi: 10.1111/j.1365-2109.2009.02330.x
Vermeer, C. V. (2012). Vitamin K: the effect on health beyond coagulation–an overview. Food Nutr. Res. 56:5329. doi: 10.3402/fnr.v56i0.5329
Wachtel, R. E., and Tsuji, K. (1977). Comparison of Limulus amebocyte lysates and correlation with the United States Pharmacopeial pyrogen test. Appl. Environ. Microbiol. 33, 1265–1269. doi: 10.1128/AEM.33.6.1265-1269.1977
Wilson, R. P., and Halver, J. E. (1986). Protein and amino acid requirements of fishes. Annu. Rev. Nutr. 6, 225–244.
Worthy, G. A. (2001). “Nutrition and energetics,” in CRC Handbook of Marine Mammal Medicine, Vol. 2, eds L. Dierauf and F. Gulland (Boca Raton, FL: CRC Press), 791–817. doi: 10.1201/9781420041637.ch36
Keywords: amebocyte, aquaculture, horseshoe crab, Limulus amebocyte lysate, nutrition, protein expression, sustainable
Citation: Tinker-Kulberg R, Dellinger A, Brady TE, Robertson L, Goddard MKM, Bowzer J, Abood SK, Kepley C and Dellinger K (2020) Effects of Diet on the Biochemical Properties of Limulus Amebocyte Lysate From Horseshoe Crabs in an Aquaculture Setting. Front. Mar. Sci. 7:541604. doi: 10.3389/fmars.2020.541604
Received: 09 March 2020; Accepted: 22 September 2020;
Published: 29 October 2020.
Edited by:
Marty Riche, Florida Atlantic University, United StatesReviewed by:
Shun-ichiro Kawabata, Kyushu University, JapanWinsor Hays Watson, University of New Hampshire, United States
Copyright © 2020 Tinker-Kulberg, Dellinger, Brady, Robertson, Goddard, Bowzer, Abood, Kepley and Dellinger. This is an open-access article distributed under the terms of the Creative Commons Attribution License (CC BY). The use, distribution or reproduction in other forums is permitted, provided the original author(s) and the copyright owner(s) are credited and that the original publication in this journal is cited, in accordance with accepted academic practice. No use, distribution or reproduction is permitted which does not comply with these terms.
*Correspondence: Kristen Dellinger, a2RlbGxpbmdlckBuY2F0LmVkdQ==; Anthony Dellinger, YWRlbGxpbmdlckBrZXBsZXliaW9zeXN0ZW1zLmNvbQ==; YWRlbGxpbmdlckBnbWFpbC5jb20=