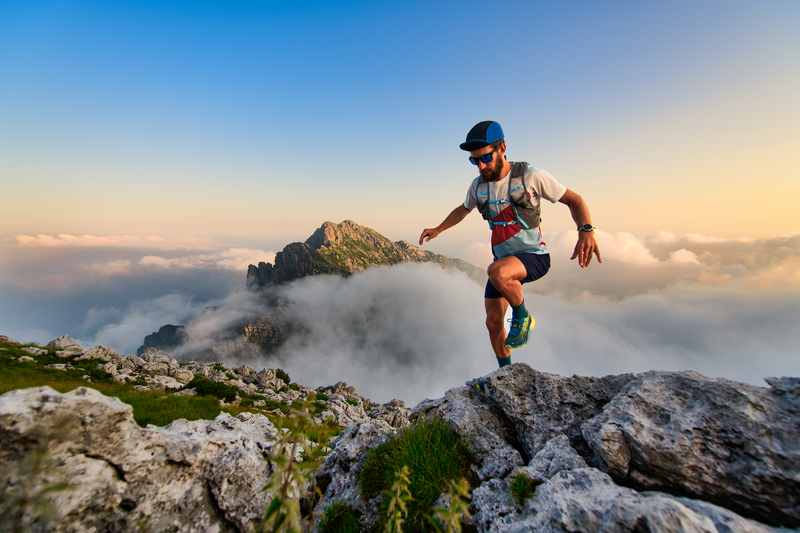
94% of researchers rate our articles as excellent or good
Learn more about the work of our research integrity team to safeguard the quality of each article we publish.
Find out more
MINI REVIEW article
Front. Mar. Sci. , 08 September 2020
Sec. Marine Biogeochemistry
Volume 7 - 2020 | https://doi.org/10.3389/fmars.2020.540165
This article is part of the Research Topic Current Topics in Marine Organic Biogeochemical Research View all 21 articles
Extensive studies in the 1980s–1990s led to the characterization of latitudinal variations in sea surface δ13C values of particulate organic carbon (δ13CPOC), and relationships were found with CO2 concentrations, temperature, growth rates, and cell geometries. Surprisingly, no large-scale efforts have been made to describe variations in δ13CPOC values over depth in the water column. Here we compile published examples demonstrating a widespread isotopic pattern in particulate organic carbon (POC) of the upper water column. In 51 vertical profiles, δ13CPOC values in the lower euphotic zone on average are 1.4‰ lower than δ13CPOC values in the upper euphotic zone of open ocean settings. In a majority of locations this vertical decrease in δ13CPOC values is >2‰ and up to 5‰, larger than the commonly recognized vertical δ13C variation in dissolved inorganic carbon over the same depths. We briefly review hypotheses and supporting evidence offered by previous studies of individual water columns: The observed patterns could result from vertical differences in photosynthetic growth rates or community composition, biochemical composition of organic matter due to degradation, isotopic disequilibrium within the dissolved inorganic carbon pool, particle dynamics, or seasonal vertical mixing. Coordinated isotopic, biological, and seawater chemistry data are sparse, and consistent drivers of this widespread isotopic pattern are currently elusive. Further work is needed to adequately characterize the environmental conditions coinciding with this pattern, to test its origins, and to determine if the magnitude of upper water column δ13CPOC variations could be a useful marker of upper ocean carbon cycle dynamics.
Particulate organic carbon (POC) is the major carrier of carbon from the surface to the deep ocean (the biological pump); therefore, quantifying POC and understanding its origins and degradation are important priorities. POC is defined as all combustible carbon captured on filters of pore size ∼0.7 μm and remaining after removal of carbonates by acidification. POC therefore encompasses diverse organic structures contained in the biomass of phytoplankton, heterotrophic and chemoautotrophic microbes, and detrital materials such as dead cells, cell fragments, fecal pellets, other aggregated material, and terrigenous or resuspended allochthonous organic matter. The naturally occurring stable carbon isotope ratio of POC (δ13CPOC) is one of the only chemical characterizations that captures the entire POC pool, since individual, measurable chemical components constitute only a small proportion of the total (Kharbush et al., 2020; this issue). Accordingly, δ13CPOC values preserved in seafloor sediments are important factors in interpreting earth’s past carbon cycle (Kump and Arthur, 1999).
δ13CPOC values in the surface ocean have been relatively well-characterized, largely motivated by the possibility of using preserved δ13CPOC values as an indicator of photosynthetic responses to past and evolving sea surface CO2 concentrations (e.g., Rau et al., 1989; Freeman and Hayes, 1992; Francois et al., 1993; Goericke and Fry, 1994; Young et al., 2013). Open-ocean δ13CPOC values vary from −35‰ at high latitudes to −16‰ at low-mid latitudes (Goericke and Fry, 1994). In contrast, the δ13C value of dissolved CO2 used as a substrate for photosynthesis only varies by ∼2‰ across surface waters (Rau et al., 1989). To explain the δ13CPOC variations, the expressed isotopic fractionation (εP) between CO2 (δ13CCO2) and photosynthetic biomass (δ13CP) (Hayes, 1993, 2001) was found to correlate to some degree with CO2 concentration, but also with phytoplankton growth rates and cell geometry (Laws et al., 1995; Popp et al., 1998, and others). Additional influence on εP can arise from the use of carbon concentrating mechanisms and the active uptake and/or fixation of bicarbonate (see Wilkes and Pearson, 2019).
In contrast, relatively few studies have focused explicitly on vertical patterns of δ13CPOC extending below near-surface depths (Jeffrey et al., 1983; Saino, 1992), and to our knowledge no global data compilation exists. Individually, several studies have noted large (2–5‰) decreases in δ13CPOC values between the near-surface ocean and the lower euphotic zone or upper pycnocline in open ocean settings, a depth range over which the values of δ13CCO2 usually vary by only 0.5–2.0‰ (Schmittner et al., 2013; Meyer et al., 2016). Hypothesized mechanisms for creating vertical δ13CPOC patterns include both photosynthetic and degradative processes. In this short review we compile data from published studies reporting δ13CPOC values in the upper 250 m of open-ocean water columns in order to explore whether global patterns or environmental drivers can be detected, briefly review existing hypotheses for the observed vertical patterns, and make suggestions for future work.
We compiled suspended δ13CPOC data from 11 published studies representative of the global open ocean and containing 51 vertical profiles of δ13CPOC extending from near-surface waters to depths of 250 m. Study locations spanned latitudes from −53° to 50° (Figure 1A and Supplementary Tables S1, S2) in the oligotrophic Atlantic (Pedrosa-Pàmies et al., 2018), equatorial Atlantic (Bishop et al., 1977; Jeffrey et al., 1983), Gulf of Mexico, Caribbean Sea, Eastern Tropical Pacific (Jeffrey et al., 1983), South Atlantic (Hurley et al., 2019), Southern Ocean (O’Leary et al., 2001; Trull et al., 2008), and North Pacific (Saino, 1992; Minagawa et al., 2001; Hernes and Benner, 2002; Druffel et al., 2003). Non-tabulated data points (Saino, 1992) and coordinates locations (Trull et al., 2008) were extracted using a built-in MATLAB function (ginput); data are compiled in Supplementary Table S2. We excluded some published studies from our numerical analysis due to a scarcity of depths sampled in the upper water column or due to likelihood of terrigenous inputs, low-oxygen metabolisms, or experimental manipulations (Williams and Gordon, 1970; Eadie and Jeffrey, 1973; Druffel et al., 1996; Benner et al., 1997; Trull and Armand, 2001; Hernes and Benner, 2006; Close et al., 2014; Krishna et al., 2018; Liu et al., 2018). We also did not include data from the Arctic region due to the prevalence of terrigenous or advected POC in these areas (e.g., Griffith et al., 2012; Xiang and Lam, 2020).
Figure 1. (A) Data locations (open circles) shown over mapped annual climatological surface ocean chlorophyll concentrations from Melin (2013). Symbols are color-coded according to the ranges in Δδ13CPOC values observed in the upper 250 m (overlying maximum minus underlying minimum; see Figure 2). Insets show examples of four vertical profiles of δ13CPOC values (filled black circles): each inset spans from the surface down to 250 m on the vertical axis and a δ13C range of −27 to −21‰ on the horizontal axis (see also Supplementary Figure S1); horizontal lines indicate depths of the mixed layer and euphotic zone as indicated in the legend. (B,C) Normalized Δδ13CPOC values among all compiled data: medians (center vertical lines), interquartile range (boxes), 99% intervals (brackets), and outliers (individual points); (B) when grouped by standardized euphotic zone depth intervals (100% Ez, depth at which irradiance is 0.1% of surface values); (C) when grouped by local mixed layer depth (MLD) and maximum annual mixed layer depth (MLDmax). Δδ13CPOC indicates δ13CPOC values normalized to the minimum δ13CPOC value found within the upper 250 m at each station, as described in the text. Depth ranges and statistical results are further outlined in Supplementary Table S3.
To compare across sites, we standardized reported sampling depth in two different ways: as a proportion of total euphotic zone depth, defined as 0.1% surface PAR (Ez, Figure 1B), and relative to the monthly and maximum annual mixed layer depths (MLD, Figure 1C). For the Ez standardization we retrieved global 1% surface PAR depths from the mapped MODIS-Aqua satellite data product (NASA Goddard Space Flight Center, 2018) produced using the method of Lee et al. (2007); determined to be within ∼14% of in situ measured values). The 4 × 4 km grid location closest to each published study site was extracted; results were similar (within 0.3 ± 1.7 m) when using an average of the surrounding data points. Ez was calculated based on first-order attenuation from 1% PAR at each location and ranged from 61 to 187 m. We retrieved monthly and maximum annual MLD from Holte et al. (2017), who compiled data from Argo float profiles and used a hybrid density algorithm to find MLD. We found the nearest points on the resulting 1 × 1° grid and used an average of the values at these points.
To account for known latitudinal variations in absolute values of δ13CPOC, the δ13CPOC data for each individual vertical profile were normalized to the minimum δ13CPOC value anywhere within the upper 250 m of the water column, i.e., the local minimum value was subtracted from each data value in an individual profile to calculate Δδ13CPOC (Figures 1B,C). After depth and isotopic data were normalized, we compiled all data to assess global vertical trends. We compared the distribution of all Δδ13CPOC values in the upper 0–50% and lower 50–100% of the Ez and below (Figure 1B and Supplementary Figure S2A). For the MLD standardization we compared all Δδ13CPOC values above the monthly MLD, between the monthly and annual maximum MLD (local pycnocline), and below the annual maximum MLD (maximum pycnocline; Figure 1C and Supplementary Figure S2B). Due to normalization of the isotopic data to a zero value, the distributions were skewed and statistical tests were chosen accordingly for non-normally distributed data. A Wilcoxon rank sum test was used to assess significance pair-wise between the different depth ranges (Supplementary Table S3). δ13CPOC values were found to be significantly lower in the lower euphotic zone compared to the upper euphotic zone, with a difference in median Δδ13CPOC values of 1.4‰ (p < 10–8; Figure 1B and Supplementary Table S3). δ13CPOC values were also significantly lower in depths between the monthly and maximum MLD when compared to those above the monthly MLD, with a difference in median Δδ13CPOC values of 0.9‰ (p < 0.01; Figure 1C and Supplementary Table S3).
Generalizing the statistical results above, within the 51 compiled profiles, δ13CPOC values in the lower euphotic zone and/or below the local mixed layer are significantly lower than values in the upper euphotic zone and/or above the local mixed layer. Further, when considering data distribution in relation to the euphotic zone depth, δ13CPOC values in the lower euphotic zone (50–100% of Ez) defined a local minimum; they are lower than δ13CPOC values both above and below this depth range (p < 0.02; Figure 1B and Supplementary Table S3).
The analysis above considered the compiled global data as a whole and examined differences between median δ13CPOC values within coarse divisions of the upper water column. We also examined the overall magnitude of variation in δ13CPOC values found within individual water columns, i.e., the upper water column max-min range in δ13CPOC values (Δδ13CPOC max-min). We calculated this range as the difference between the minimum δ13CPOC value found anywhere in the upper 250 m of individual water columns and the maximum δ13CPOC value found above this depth in order to focus on the pattern of higher δ13CPOC overlying lower δ13CPOC values. We found that 63% of individual water columns had a max-min range > 2‰, with the largest ranges >5‰ (Figure 2A). We attempted to assess whether the observed Δδ13CPOC max-min range correlated with broad scale environmental features extracted from global datasets. Using linear regressions (MATLAB function polyfit), no correlation was found between the local max-min range and the absolute depth of the euphotic zone, chlorophyll a, nor modeled surface phytoplankton size or fecal pellet export (Melin, 2013; Siegel et al., 2014; linear regression, r2 < 0.1). The only linear regressions resulting in r2 >0.15 were those comparing the local Δδ13CPOC max-min range to net primary production (Figure 2B) and maximum annual mixed layer depth (Figure 2C).
Figure 2. (A) Distribution of upper water column ranges in Δδ13CPOC values in the 51 individual water columns in this study, calculated as the overlying maximum δ13CPOC value minus subsurface minimum δ13CPOC value (i.e., maximum values occurring at depths below the minimum are not considered here). Negative values indicate profiles in which the minimum δ13CPOC value was found at the shallowest data point. (B) Comparison of Δδ13CPOC max-min ranges in (A) to climatological net primary production (NPP) at each location. (C) Comparison of Δδ13CPOC max-min ranges in (A) to maximum annual mixed layer depth (MLDmax) at each location. One extreme outlier was omitted from (B) with an NPP value of 1250 mg m–2 d–1 and Δδ13CPOC max-min range of 1.8‰.
Where possible, we extracted coinciding in situ vertical profiles of concentrations of POC, dissolved inorganic carbon (DIC), O2, chlorophyll, and nutrient concentrations from the published studies, as well as C:N ratios. We also obtained annual climatological DIC concentrations, alkalinity, salinity, pH, and temperature data mapped at 1 × 1° resolution from GLODAP and World Ocean Atlas databases (Key et al., 2004), and we calculated CO2 concentration profiles for each δ13CPOC data location using the CO2SYS tool (Lewis and Wallace, 1998). We tested for pair-wise correlations between vertical profiles of these variables and δ13CPOC values (both absolute values and Δδ13CPOC values) where possible, and we did not find any significant relationships. Measured in situ δ13CDIC values were available from O’Leary et al. (2001) and Hurley et al. (2019). We normalized δ13CDIC and δ13CPOC to near-surface values, using the data point closest to the surface to account for the known latitudinal variation in surface ocean δ13CDIC and δ13CPOC values. In the nine individual profiles where this comparison was possible, we found that the vertical range in δ13CPOC values was on average approximately four times larger than the vertical range in δ13CDIC over the same depths (slope = 4.17, r2 = 0.53, linear regression).
While our compilation of published data here is likely not exhaustive, it is to our knowledge the first global examination of vertical profiles of δ13CPOC in the upper water column. Global mapped 3-dimensional oceanographic data products for δ13CPOC currently do not exist, and most major oceanographic programs do not include vertical δ13CPOC as a standard parameter. Anecdotally, vertical δ13CPOC values might be measured much more frequently than they are published or interpreted (e.g., Bishop et al., 1999). The scarcity of published data and lack of a database for δ13CPOC values were barriers to identifying environmental commonalities underlying the observed patterns. We also note that low vertical resolution in many profiles likely means that the true minimum and maximum δ13CPOC values in a given water column were not captured (Supplementary Figure S1).
Of the 11 studies from which we compiled data, 6 collected POC using in situ or submersible pumps to filter large volumes of water (>70 L). Jeffrey et al. (1983); Saino (1992), Wu et al. (1999); Minagawa et al. (2001), and Hernes and Benner (2002) instead collected seawater in large volumes (10–30 L) from Niskin bottles and filtered the water shipboard. Filtering POC from large volumes of water helps in obtaining a statistical sampling of the particle population; small sample volumes are subject to interference from the inconsistent capture of sparse large particles as well as a larger proportional blank contribution from dissolved organic matter (DOM) sorbed to the filter when POC loading is low. Discussion of DOM sorption and differential particle retention based on filtration media or pressures has been extensive in terms of effects on POC and pigment concentrations (e.g., Gardner et al., 2003; Nayar and Chou, 2003; Bishop et al., 2012). However, the effects of sampling methods and blank correction on δ13CPOC values are under-discussed (Lorrain et al., 2003) and are of particular importance in the subsurface where POC concentrations are lower and blank contribution is proportionally higher. In addition, the most common, elemental analysis-based methods for bulk carbon isotope analysis are >99% inefficient, necessitating large sample quantities; broader use of “nano-scale” analytical methods could improve our ability to obtain better three-dimensional data coverage for oceanic δ13CPOC measurements (see Close, 2019).
The size fraction(s) of particles studied also can be important. Most studies described here collected total POC onto 0.45, 0.7, 0.8, or 1.0 μm glass or quartz fiber filters, but the studies of Bishop et al. (1977) and Hurley et al. (2019) in the mid-latitude Atlantic included a prefilter of 53 μm mesh size. In these cases, the observed patterns of δ13CPOC in the lower euphotic zone were a particular feature of small particles (∼0.7–53 μm), which constituted the majority of POC. Trull et al. (2008) included measurements of several particle size classes in a study of the Southern Ocean – where large phytoplankton and particles are more abundant that the above studies – and found heterogeneities in the δ13C values across both size and depth, possibly relating to a combination of physiological effects on εP, different sinking rates, and temporal offsets between surface production and deeper particles (see below).
Several physiological, community, and environmental features of in situ photosynthesis have been suggested as drivers of systematic variations in δ13CPOC values between the upper and lower euphotic zones. Saino (1992) noted “a subsurface minimum near the base of the euphotic zone” when examining δ13CPOC values at study sites adjacent to the Kuroshio Current and suggested photosynthetic mechanisms. Models and culture studies (e.g., Popp et al., 1998; Cassar et al., 2006) have demonstrated that εP depends on an interplay between the growth rate of an organism and the supply rate of CO2; higher CO2 supply rates and/or slower growth rates will lead to a larger εP, and therefore lower values of δ13CP. Saino (1992) suggested that both conditions likely exist in the lower euphotic zone: CO2 concentrations here are higher than the surface due to the net removal of CO2 by photosynthesis at the surface and the net respiration of CO2 in the lower euphotic zone, and growth rates are likely lower due to light limitation. O’Leary et al. (2001) also found low δ13CPOC values in the lower euphotic zone in a study of the subantarctic water column, and their model results pointed to slow photosynthetic growth rates as the cause. Decreases in photosynthetic growth rate with increasing depth in the euphotic zone are frequently observed in the open ocean (e.g., Laws, 2013), and relationships between growth rates and photosynthetic fractionation of carbon isotopes have been demonstrated in culture (Laws et al., 1995; Bidigare et al., 1997; Popp et al., 1998).
A smaller average cell size of phytoplankton in the lower euphotic zone/upper pycnocline than in the upper euphotic zone/mixed layer also is a frequent observation in open-ocean settings (e.g., Poulton et al., 2006; Barone et al., 2015). Trull and Armand (2001) demonstrated that a smaller average cell size (higher surface area: volume ratio) of phytoplankton in the lower euphotic zone could result in lower δ13CPOC values, an isotopic relationship previously established in phytoplankton cultures and models by Rau et al. (1996); Popp et al. (1998), and others, stemming from faster CO2 diffusion rates.
Conversely, targeted studies of individual photosynthetic taxa or biomarkers have sometimes suggested a pattern of increasing or unchanging δ13C values over increasing depth in the euphotic zone (Prahl et al., 2005; Popp et al., 2006; Tolosa et al., 2008; Radabaugh et al., 2014). The recent model of Wilkes and Pearson (2019) suggests that increasing δ13C values over depth could be a phenomenon specific to eukaryotic phytoplankton with intracellular CO2 partitioning or carbon concentrating strategies, with carbon isotopic fractionation varying according to whether growth is limited by low nutrient concentrations (upper euphotic zone/mixed layer) or other factors such as light [lower euphotic zone/upper pycnocline; also explored by Laws et al. (2002) and Cassar et al. (2006)]. Some phytoplankton also employ active uptake and/or fixation of bicarbonate (HCO3–), thereby also complicating relationships between cell size, CO2 concentrations, and δ13CP (e.g., Bentaleb et al., 1998; Cassar et al., 2004). Due to such variations in photosynthetic physiologies, the isotopic sensitivity of a whole phytoplankton community to its local seawater environment (CO2 concentrations, light and nutrient availability) will depend also on the taxonomic composition of photosynthesizing organisms present (e.g., Leboulanger et al., 1995; Bentaleb et al., 1998; Trull et al., 2008).
Saino (1992) also discussed how slow isotopic equilibrium relative to chemical equilibrium within the DIC system could lead to relatively high values of δ13CCO2 in the mixed layer compared to the lower euphotic zone. Notably, δ13CCO2 usually is calculated from measured δ13CDIC using constants that assume the system is at both chemical and isotopic equilibrium (Freeman and Hayes, 1992).
Some compound-specific isotope analyses of lipids have supported a photosynthetic origin of low δ13CPOC values in the lower euphotic zone. Close et al. (2014) found that δ13C values of lipid biomarkers for in situ production paralleled δ13CPOC values throughout the euphotic zone in the Eastern Tropical North Pacific, which ruled out the degradative accumulation of lipids (see section “Degradative or Dynamical Hypotheses for Origins of Low δ13CPOC Values in the Lower Euphotic Zone or Upper Pycnocline”) as a driver behind low δ13C values in the lower euphotic zone. Similarly, O’Leary et al. (2001) demonstrated that δ13C values of photosynthetic sterols paralleled vertical profiles of δ13CPOC in subantarctic waters.
Equally common in published interpretations of upper water column δ13CPOC variations are hypotheses relating to degradation or alteration processes. Jeffrey et al. (1983) noted that “the upper part of the pycnocline (i.e., below the local mixed layer) is marked by lighter POC-δ13C values at almost all the stations occupied” in a broad-ranging study of the Eastern Tropical North Pacific, Gulf of Mexico, Caribbean, and western south Atlantic. These authors suggested a degradative mechanism, following the interpretations of Eadie and Jeffrey (1973) and based on the results of Degens (1969): below the local mixed layer POC concentrations decrease rapidly due to high rates of respiration. Amino acids and sugars comprise quickly degrading, 13C-enriched components of POC, potentially leaving remaining POC at these depths with a relatively high abundance of 13C-depleted lipids. This explanation was cited by Druffel et al. (2003), who noted relatively low δ13C values of POC in the upper 200 m at study sites in the North Central Pacific and Sargasso Sea.
Bishop et al. (1977) noted “a depletion in 13C of 4‰ over the depth interval between 32 and 50 m,” but could not attribute this to the degradative explanation of Eadie and Jeffrey (1973) because a preponderance of lipids should result in an increase in C:N ratios. Instead C:N ratios were invariant over these depths, and the authors suggested that secondary production by bacteria could lead to low δ13C values. However, the proportional contribution of lipids may increase without being accompanied by an increase in C:N, if proteinaceous (low C:N) material is also abundant, as suggested by Pedrosa-Pàmies et al. (2018). There are few broad organic compositional studies of POC that are accompanied by carbon isotopic data. One such study by Sannigrahi et al. (2005) did not find a strong relationship between organic composition and δ13CPOC values in the upper water column at Station ALOHA [isotopic data previously reported by Hernes and Benner (2002)]; in particular the lipid composition was not proportionally higher at the lower euphotic δ13CPOC minimum than it was at shallower depths.
Hernes and Benner (2002, 2006) suggested that low subsurface δ13CPOC values in the oligotrophic subtropical Pacific and Sargasso Sea were the result of an advected source of terrigenous organic matter, based on a correlation with lignin concentration. However, this correlation existed mainly in POM below the euphotic zone. The local minimum in δ13CPOC values observed in the lower euphotic zone did not correlate with the proportional contribution of lignin to total POC at that depth. Advection of POC from adjacent waters often cannot explain the range of δ13CPOC values observed in a single water column (e.g., Lourey et al., 2004), although this is more commonly cited in the Arctic (Griffith et al., 2012; Xiang and Lam, 2020).
Overall, organic compositional effects on δ13CPOC values seem to be more apparent at advanced stages of organic matter degradation that occur below the euphotic zone, in deep mesopelagic and bathypelagic waters. Here decreases in δ13CPOC values are more clearly related to increasing C:N ratios or changing biochemical composition than they are in the upper water column (see Minagawa et al., 2001; Hwang and Druffel, 2003; Sannigrahi et al., 2005). Analogous to the patterns recognized for particulate δ15N values (Altabet et al., 1991), shallower, earlier degradation of macromolecules may lead first to increases in δ13CPOC values as bonds containing 12C are more quickly hydrolyzed or respired in upper mesopelagic waters (e.g., Bishop et al., 1977; Jeffrey et al., 1983; Wu et al., 1999). Accordingly, slightly higher δ13CPOC values were found below Ez compared to the lower euphotic zone in our data compilation (Figure 1B and Supplementary Table S3), and this pattern is more apparent in individual water column profiles (Supplementary Figure S1; see also Cavagna et al., 2013).
Particle, seasonal, and water column dynamics also may lead to vertical variations in δ13CPOC. Studies of size-fractionated POC or plankton frequently find size-based differences in δ13C values. Typically large particles are assumed to sink more quickly through the euphotic zone; those originating in the surface can carry their δ13CPOC values below the mixed layer, possibly also being disaggregated into small particles therein. Large phytoplankton often have higher δ13CPOC values than small phytoplankton and therefore would tend to contribute 13C-enriched rather than 13C-depleted material into the subsurface through such a mechanism (Bishop et al., 1977; Fry and Wainright, 1991; Wu et al., 1999; Trull and Armand, 2001; Trull et al., 2008). Heterogeneous sinking rates and δ13C values have also been used to explain temporal offsets in the origins of upper and lower water column δ13CPOC values, especially in locations with strong seasonality. That is, POC in the subsurface may represent export from the surface ocean during growth conditions (growth rates, CO2 concentrations, phytoplankton community composition) typical of preceding seasons (Lourey et al., 2004; Cavagna et al., 2013). In high latitude areas seasonal changes in MLD can also distribute surface biomass throughout the euphotic zone and create temporal and spatial offsets between the formation and observation of POC (Grenier et al., 2015).
Zooplankton egesta and/or methane production by gut microbiota also have been cited as possibly affecting δ13CPOC values (Cavagna et al., 2013). Fecal pellets can be highly 13C-depleted in comparison to zooplankton diet (Tamelander et al., 2006). In addition, relatively 13C-depleted methane has been observed reaching maximum concentrations in the pycnocline of oxygenated open-ocean water columns (Holmes et al., 2000; Sasakawa et al., 2008), likely linked to bacterial degradation of dissolved organic compounds (Repeta et al., 2016). Bacteria may incorporate this methane into biomass during oxidative metabolism (Sasakawa et al., 2008), but it is unclear if biomass quantities would be sufficient to affect overall δ13CPOC values.
One objective of this review is to motivate wider measurement and/or reporting of vertically resolved δ13CPOC data. Regardless of the underlying primary or degradative drivers of this pattern, better data coverage – especially at higher vertical resolution – will allow more precise definition of the depths at which δ13CPOC minima occur and the overall magnitude of variation in upper water column δ13CPOC values; both could prove to be a useful diagnostic of underlying ecosystem processes or carbon dynamics as described above.
The current data compilation suggests that there is a widespread pool of POC in the ocean with δ13C values that are significantly different from those modeled as “source” photosynthetic values in the surface ocean. POC in the lower euphotic zone or upper pycnocline is abundant and serves as a food source for subsurface biota; studies such as Liu et al. (2018) have discussed how variations in δ13CPOC values in deep chlorophyll maxima can influence how δ13C values are interpreted in food web studies. The depths of the lower euphotic zone or upper pycnocline, which usually include deep chlorophyll maxima when present, can also be a location of net particle formation and export (Kemp et al., 2000; Benitez-Nelson et al., 2001; Umhau et al., 2019). Recognizing subsurface sources for exported material can be important to the interpretation of the sedimentary geochemical record (e.g., Luo et al., 2014; Hurley et al., 2018). Interestingly, an analog exists in terrestrial systems; plant matter growing in the upper canopy of forests consistently has higher δ13C values than plant matter growing below (the “canopy effect”; Van der Merwe and Medina, 1991). The differential preservation and dietary usage of these two signatures can affect the interpretation of terrestrial soil records and food webs (e.g., Bonafini et al., 2013).
Finally, global biogeochemical models include the carbon isotope fractionation of photosynthetic organisms according to relationships with growth rate and CO2 concentrations established in culture (Tagliabue and Bopp, 2008; Schmittner et al., 2013). However, it is unclear whether current models already can adequately reproduce observed vertical δ13CPOC patterns; these models have instead focused on validation of surface ocean δ13CPOC values or vertical δ13CDIC patterns. A more comprehensive dataset of vertical δ13CPOC values could help validate such isotope-enabled carbon cycle models.
Existing published works demonstrate that δ13CPOC values can be significantly lower in the lower euphotic zone or upper pycnocline compared to the upper euphotic zone or local surface mixed layer in a wide range of global open-ocean locations. While individual studies have offered hypotheses for the origin of this vertical pattern, a global compilation of δ13CPOC profiles with coinciding oceanographic data is necessary to test whether there are consistent environmental drivers. We suggest that high-quality isotopic data coverage of the global open ocean is needed, particularly with better data density throughout the euphotic zone and with coinciding CO2 system data, cell size characterization, and δ13CDIC data. The large water sample volume needed to obtain sufficient material for δ13CPOC measurements is currently a barrier to including this parameter in many global-scale oceanographic programs, but improvements in the efficiency of isotopic measurement could mitigate this issue. Compound-specific isotope approaches, autotrophic and heterotrophic rate measurements (including substrate-specific photosynthetic uptake, bicarbonate vs. CO2), particle dynamics characterization, and relevant enzyme expression data all would aid in discerning between potential hypothesized mechanisms. Further, we suggest that an isotope-specific database such as the emergent IsoBank (Pauli et al., 2017)1 would be an appropriate place to aggregate both published and unpublished δ13CPOC data that currently may be dispersed across various oceanographic databases.
HC and LH designed the study. LH conducted the data analysis and contributed to the manuscript. HC wrote the manuscript. Both authors contributed to the article and approved the submitted version.
Publication was funded by the Deutsche Forschungsgemeinschaft (DFG, German Research Foundation) project number 422798570. This work was partially inspired by discussions at a workshop on Marine Organic Biogeochemistry that was held in Delmenhorst, Germany in April 2019 and funded by the Hanse-Wissenschaftskolleg and the Geochemical Society. LH was supported by a University of Miami Fellowship.
The authors declare that the research was conducted in the absence of any commercial or financial relationships that could be construed as a potential conflict of interest.
We thank Ellen Druffel and Rut Pedrosa-Pámies for providing tabulated data from Druffel et al. (2003) and Pedrosa-Pàmies et al. (2018), Igor Belkin for advice in recovering data from Saino (1992), and David Siegel for providing modeled climatologies from Siegel et al. (2014). We are grateful for comments by MM, Yuan Shen, and TT which resulted in considerable improvements to this mini-review.
The Supplementary Material for this article can be found online at: https://www.frontiersin.org/articles/10.3389/fmars.2020.540165/full#supplementary-material
Altabet, M. A., Deuser, W. G., Honjo, S., and Stienen, C. (1991). Seasonal and depth-related changes in the source of sinking particles in the North Atlantic. Nature 354, 136–139. doi: 10.1038/354136a0
Barone, B., Bidigare, R. R., Church, M. J., Karl, D. M., Letelier, R. M., and White, A. E. (2015). Particle distributions and dynamics in the euphotic zone of the North Pacific Subtropical Gyre. J. Geophys. Res. Oceans 120, 3229–3247. doi: 10.1002/2015jc010774
Benitez-Nelson, C., Buesseler, K. O., Karl, D. M., and Andrews, J. (2001). A time-series study of particulate matter export in the North Pacific Subtropical Gyre based on 234Th: 238U disequilibrium. Deep Sea Res. Part I Oceanogr. Res. Pap. 48, 2595–2611. doi: 10.1016/s0967-0637(01)00032-2
Benner, R., Biddanda, B., Black, B., and McCarthy, M. (1997). Abundance, size distribution, and stable carbon and nitrogen isotopic compositions of marine organic matter isolated by tangential-flow ultrafiltration. Mar. Chem. 57, 243–263. doi: 10.1016/s0304-4203(97)00013-3
Bentaleb, I., Fontugne, M., Descolas-Gros, C., Girardin, C., Mariotti, A., Pierre, C., et al. (1998). Carbon isotopic fractionation by plankton in the Southern Indian Ocean: relationship between δ13C of particulate organic carbon and dissolved carbon dioxide. J. Mar. Syst. 17, 39–58. doi: 10.1016/s0924-7963(98)00028-1
Bidigare, R. R., Fluegge, A., Freeman, K. H., Hanson, K. L., Hayes, J. M., Hollander, D., et al. (1997). Consistent fractionation of 13C in nature and in the laboratory: growth-rate effects in some haptophyte algae. Global Biogeochem. Cycles 11, 279–292. doi: 10.1029/96gb03939
Bishop, J. K., Calvert, S. E., and Soon, M. Y. (1999). Spatial and temporal variability of POC in the northeast Subarctic Pacific. Deep Sea Res. Part II Top. Stud. Oceanogr. 46, 2699–2733. doi: 10.1016/s0967-0645(99)00081-8
Bishop, J. K., Edmond, J. M., Ketten, D. R., Bacon, M. P., and Silker, W. B. (1977). The chemistry, biology, and vertical flux of particulate matter from the upper 400 m of the equatorial Atlantic Ocean. Deep Sea Res. 24, 511–548. doi: 10.1016/0146-6291(77)90526-4
Bishop, J. K., Lam, P. J., and Wood, T. J. (2012). Getting good particles: accurate sampling of particles by large volume in-situ filtration. Limnol. Oceanogr. Methods 10, 681–710. doi: 10.4319/lom.2012.10.681
Bonafini, M., Pellegrini, M., Ditchfield, P., and Pollard, A. (2013). Investigation of the ‘canopy effect’in the isotope ecology of temperate woodlands. J. Archaeol. Sci. 40, 3926–3935. doi: 10.1016/j.jas.2013.03.028
Cassar, N., Laws, E. A., Bidigare, R. R., and Popp, B. N. (2004). Bicarbonate uptake by Southern Ocean phytoplankton. Global Biogeochem. Cycles 18:GB2003.
Cassar, N., Laws, E. A., and Popp, B. N. (2006). Carbon isotopic fractionation by the marine diatom Phaeodactylum tricornutum under nutrient-and light-limited growth conditions. Geochim. Cosmochim. Acta 70, 5323–5335. doi: 10.1016/j.gca.2006.08.024
Cavagna, A.-J., Dehairs, F., Bouillon, S., Woule-Ebongué, V., Planchon, F., Delille, B., et al. (2013). Water column distribution and carbon isotopic signal of cholesterol, brassicasterol and particulate organic carbon in the Atlantic sector of the Southern Ocean. Biogeosciences 10, 2787–2801. doi: 10.5194/bg-10-2787-2013
Close, H. G. (2019). Compound-specific isotope geochemistry in the ocean. Annu. Rev Mar. Sci. 11, 27–56. doi: 10.1146/annurev-marine-121916-063634
Close, H. G., Wakeham, S. G., and Pearson, A. (2014). Lipid and 13C signatures of submicron and suspended particulate organic matter in the Eastern Tropical North Pacific: implications for the contribution of Bacteria. Deep Sea Res. Part I Oceanogr. Res. Pap. 85, 15–34. doi: 10.1016/j.dsr.2013.11.005
Degens, E. (1969). “Biogeochemistry of stable carbon isotopes,” in Organic Geochemistry, eds E. Eglington and M. T. J. Murphy (Berlin: Springer), 304–329. doi: 10.1007/978-3-642-87734-6_14
Druffel, E. R., Bauer, J. E., Griffin, S., and Hwang, J. (2003). Penetration of anthropogenic carbon into organic particles of the deep ocean. Geophys. Res. Lett. 30:1744.
Druffel, E. R., Bauer, J. E., Williams, P. M., Griffin, S., and Wolgast, D. (1996). Seasonal variability of particulate organic radiocarbon in the northeast Pacific Ocean. J. Geophys. Res. Oceans 101, 20543–20552. doi: 10.1029/96jc01850
Eadie, B. J., and Jeffrey, L. M. (1973). δ13C analyses of oceanic particulate organic matter. Mar. Chem. 1, 199–209. doi: 10.1016/0304-4203(73)90004-2
Francois, R., Altabet, M. A., Goericke, R., McCorkle, D. C., Brunet, C., and Poisson, A. (1993). Changes in the δ13C of surface water particulate organic matter across the subtropical convergence in the SW Indian Ocean. Global Biogeochem. Cycles 7, 627–644. doi: 10.1029/93gb01277
Freeman, K. H., and Hayes, J. (1992). Fractionation of carbon isotopes by phytoplankton and estimates of ancient CO2 levels. Global Biogeochem. Cycles 6, 185–198. doi: 10.1029/92gb00190
Fry, B., and Wainright, S. C. (1991). Diatom sources of 13 C-rich carbon in marine food webs. Mar. Ecol. Prog. Ser. 76, 149–157. doi: 10.3354/meps076149
Gardner, W. D., Richardson, M. J., Carlson, C. A., Hansell, D., and Mishonov, A. V. (2003). Determining true particulate organic carbon: bottles, pumps and methodologies. Deep Sea Res. Part II Top. Stud. Oceanogr. 50, 655–674. doi: 10.1016/s0967-0645(02)00589-1
Goericke, R., and Fry, B. (1994). Variations of marine plankton δ13C with latitude, temperature, and dissolved CO2 in the world ocean. Global Biogeochem. Cycles 8, 85–90. doi: 10.1029/93gb03272
Grenier, M., Della Penna, A., and Trull, T. (2015). Autonomous profiling float observations of the high-biomass plume downstream of the Kerguelen Plateau in the Southern Ocean. Biogeosciences 12, 2707–2735. doi: 10.5194/bg-12-2707-2015
Griffith, D. R., McNichol, A. P., Xu, L., McLaughlin, F. A., Brown, K. A., and Eglinton, T. I. (2012). Carbon dynamics in the western Arctic Ocean: insights from full-depth carbon isotope profiles of DIC, DOC, and POC. Biogeosciences 9, 1217–1224. doi: 10.5194/bg-9-1217-2012
Hayes, J. (1993). Factors controlling 13C contents of sedimentary organic compounds: principles and evidence. Mar. Geol. 113, 111–125. doi: 10.1016/0025-3227(93)90153-m
Hayes, J. M. (2001). Fractionation of carbon and hydrogen isotopes in biosynthetic processes. Rev. Mineral. Geochem. 43, 225–277. doi: 10.1515/9781501508745-006
Hernes, P. J., and Benner, R. (2002). Transport and diagenesis of dissolved and particulate terrigenous organic matter in the North Pacific Ocean. Deep Sea Res. Part I Oceanogr. Res. Pap. 49, 2119–2132. doi: 10.1016/s0967-0637(02)00128-0
Hernes, P. J., and Benner, R. (2006). Terrigenous organic matter sources and reactivity in the North Atlantic Ocean and a comparison to the Arctic and Pacific oceans. Mar. Chem. 100, 66–79. doi: 10.1016/j.marchem.2005.11.003
Holmes, M. E., Sansone, F. J., Rust, T. M., and Popp, B. N. (2000). Methane production, consumption, and air-sea exchange in the open ocean: an evaluation based on carbon isotopic ratios. Global Biogeochem. Cycles 14, 1–10. doi: 10.1029/1999gb001209
Holte, J., Talley, L. D., Gilson, J., and Roemmich, D. (2017). An Argo mixed layer climatology and database. Geophys. Res. Lett. 44, 5618–5626. doi: 10.1002/2017gl073426
Hurley, S. J., Close, H. G., Elling, F. J., Jasper, C. E., Gospodinova, K., McNichol, A. P., et al. (2019). CO2-dependent carbon isotope fractionation in Archaea, Part II: the marine water column. Geochim. Cosmochim. Acta 261, 383–395. doi: 10.1016/j.gca.2019.06.043
Hurley, S. J., Lipp, J. S., Close, H. G., Hinrichs, K.-U., and Pearson, A. (2018). Distribution and export of isoprenoid tetraether lipids in suspended particulate matter from the water column of the Western Atlantic Ocean. Organ. Geochem. 116, 90–102. doi: 10.1016/j.orggeochem.2017.11.010
Hwang, J., and Druffel, E. R. (2003). Lipid-like material as the source of the uncharacterized organic carbon in the ocean? Science 299, 881–884. doi: 10.1126/science.1078508
Jeffrey, A. W., Pflaum, R. C., Brooks, J. M., and Sackett, W. M. (1983). Vertical trends in particulate organic carbon 13C: 12C ratios in the upper water column. Deep Sea Res. Part A Oceanogr. Res. Pap. 30, 971–983. doi: 10.1016/0198-0149(83)90052-3
Kemp, A. E., Pike, J., Pearce, R. B., and Lange, C. B. (2000). The “Fall dump”—a new perspective on the role of a “shade flora” in the annual cycle of diatom production and export flux. Deep Sea Res. Part II Top. Stud. Oceanogr. 47, 2129–2154. doi: 10.1016/s0967-0645(00)00019-9
Key, R. M., Kozyr, A., Sabine, C. L., Lee, K., Wanninkhof, R., Bullister, J. L., et al. (2004). A global ocean carbon climatology: results from Global Data Analysis Project (GLODAP). Global Biogeochem. Cycles 18:GB4031.
Kharbush, J. J., Van Mooy, B. A., Close, H. G., Arnosti, C., Smittenberg, R. H., Le Moigne, F. A. C., et al. (2020). Particulate organic carbon deconstructed: molecular and chemical composition of particulate organic carbon in the ocean. Front. Mar. Sci. 7:518. doi: 10.3389/fmars.2020.00518
Krishna, M., Mukherjee, J., Dalabehera, H., and Sarma, V. (2018). Particulate organic carbon composition in temperature fronts of the northeastern Arabian Sea during winter. J. Geophys. Res. Biogeosci. 123, 463–478. doi: 10.1002/2018jg004387
Kump, L. R., and Arthur, M. A. (1999). Interpreting carbon-isotope excursions: carbonates and organic matter. Chem. Geol. 161, 181–198. doi: 10.1016/s0009-2541(99)00086-8
Laws, E. A. (2013). Evaluation of in situ phytoplankton growth rates: a synthesis of data from varied approaches. Annu. Rev Mar. Sci. 5, 247–268. doi: 10.1146/annurev-marine-121211-172258
Laws, E. A., Popp, B. N., Bidigare, R. R., Kennicutt, M. C., and Macko, S. A. (1995). Dependence of phytoplankton carbon isotopic composition on growth rate and [CO2) aq: theoretical considerations and experimental results. Geochim. Cosmochim. Acta 59, 1131–1138. doi: 10.1016/0016-7037(95)00030-4
Laws, E. A., Popp, B. N., Cassar, N., and Tanimoto, J. (2002). 13C discrimination patterns in oceanic phytoplankton: likely influence of CO2 concentrating mechanisms, and implications for palaeoreconstructions. Funct. Plant Biol. 29, 323–333. doi: 10.1071/pp01183
Leboulanger, C., Descolas-Gros, C., Fontugne, M., Bentaleb, I., and Jupin, H. (1995). Interspecific variability and environmental influence on particulate organic carbon δ13C in cultured marine phytoplankton. J. Plankton Res. 17, 2079–2091. doi: 10.1093/plankt/17.11.2079
Lee, Z., Weidemann, A., Kindle, J., Arnone, R., Carder, K. L., and Davis, C. (2007). Euphotic zone depth: its derivation and implication to ocean-color remote sensing. J. Geophys. Res. Oceans 112. doi: 10.1029/2006JC003802
Lewis, E., and Wallace, D. (1998). Program Developed for CO2 System Calculations. Oak Ridge, TN: US Department of Energy.
Liu, Q., Kandasamy, S., Lin, B., Wang, H., and Chen-Tung, A. C. (2018). Biogeochemical characteristics of suspended particulate matter in deep chlorophyll maximum layers in the southern East China Sea. Biogeosciences 15, 2091–2109. doi: 10.5194/bg-15-2091-2018
Lorrain, A., Savoye, N., Chauvaud, L., Paulet, Y.-M., and Naulet, N. (2003). Decarbonation and preservation method for the analysis of organic C and N contents and stable isotope ratios of low-carbonated suspended particulate material. Anal. Chim. Acta 491, 125–133. doi: 10.1016/s0003-2670(03)00815-8
Lourey, M. J., Trull, T. W., and Tilbrook, B. (2004). Sensitivity of δ13C of Southern Ocean suspended and sinking organic matter to temperature, nutrient utilization, and atmospheric CO2. Deep Sea Res. Part I Oceanogr. Res. Pap. 51, 281–305. doi: 10.1016/j.dsr.2003.10.002
Luo, G., Algeo, T. J., Huang, J., Zhou, W., Wang, Y., Yang, H., et al. (2014). Vertical δ13Corg gradients record changes in planktonic microbial community composition during the end-Permian mass extinction. Palaeogeogr. Palaeoclimat. Palaeoecol. 396, 119–131. doi: 10.1016/j.palaeo.2014.01.006
Melin, F. (2013). GMIS - SeaWiFS Monthly Climatology Sea Surface Chlorophyll-a Concentration (9km) in mg.m^-3. Available online at: http://data.europa.eu/89h/d6f9abd9-777c-4a0c-a5f7-669612f83307 (accessed July 1, 2020).
Meyer, K., Ridgwell, A., and Payne, J. (2016). The influence of the biological pump on ocean chemistry: implications for long-term trends in marine redox chemistry, the global carbon cycle, and marine animal ecosystems. Geobiology 14, 207–219. doi: 10.1111/gbi.12176
Minagawa, M., Ohashi, M., Kuramoto, T., and Noda, N. (2001). δ15N of PON and nitrate as a clue to the origin and transformation of nitrogen in the subarctic North Pacific and its marginal sea. J. Oceanogr. 57, 285–300.
NASA Goddard Space Flight Center, Ocean Ecology Laboratory, Ocean Biology Processing Group (2018). Moderate-Resolution Imaging Spectroradiometer (MODIS) Aqua Euphotic Depth Data; 2018 Reprocessing. Available online at: https://oceancolor.gsfc.nasa.gov/data/10.5067/AQUA/MODIS/L3M/ZLEE/2018 (accessed January 8, 2020).
Nayar, S., and Chou, L. (2003). Relative efficiencies of different filters in retaining phytoplankton for pigment and productivity studies. Estuar. Coast. Shelf Sci. 58, 241–248. doi: 10.1016/s0272-7714(03)00075-1
O’Leary, T., Trull, T., Griffiths, F., Tilbrook, B., and Revill, A. (2001). Euphotic zone variations in bulk and compound-specific δ13C of suspended organic matter in the Subantarctic Ocean, south of Australia. J. Geophys. Res. Oceans 106, 31669–31684. doi: 10.1029/2000jc000288
Pauli, J. N., Newsome, S. D., Cook, J. A., Harrod, C., Steffan, S. A., Baker, C. J., et al. (2017). Opinion: why we need a centralized repository for isotopic data. Proc. Natl. Acad. Sci. U.S.A. 114, 2997–3001. doi: 10.1073/pnas.1701742114
Pedrosa-Pàmies, R., Conte, M., Weber, J., and Johnson, R. (2018). Carbon cycling in the Sargasso Sea water column: insights from lipid biomarkers in suspended particles. Prog. Oceanogr. 168, 248–278. doi: 10.1016/j.pocean.2018.08.005
Popp, B. N., Laws, E. A., Bidigare, R. R., Dore, J. E., Hanson, K. L., and Wakeham, S. G. (1998). Effect of phytoplankton cell geometry on carbon isotopic fractionation. Geochim. Cosmochim. Acta 62, 69–77. doi: 10.1016/s0016-7037(97)00333-5
Popp, B. N., Prahl, F. G., Wallsgrove, R. J., and Tanimoto, J. (2006). Seasonal patterns of alkenone production in the subtropical oligotrophic North Pacific. Paleoceanography 21:PA1004.
Poulton, A. J., Holligan, P. M., Hickman, A., Kim, Y.-N., Adey, T. R., Stinchcombe, M. C., et al. (2006). Phytoplankton carbon fixation, chlorophyll-biomass and diagnostic pigments in the Atlantic Ocean. Deep Sea Res. Part II Top. Stud. Oceanogr. 53, 1593–1610. doi: 10.1016/j.dsr2.2006.05.007
Prahl, F. G., Popp, B. N., Karl, D. M., and Sparrow, M. A. (2005). Ecology and biogeochemistry of alkenone production at Station ALOHA. Deep Sea Res. Part I Oceanogr. Res. Pap. 52, 699–719. doi: 10.1016/j.dsr.2004.12.001
Radabaugh, K. R., Malkin, E. M., Hollander, D. J., and Peebles, E. B. (2014). Evidence for light-environment control of carbon isotope fractionation by benthic microalgal communities. Mar. Ecol. Prog. Ser. 495, 77–90. doi: 10.3354/meps10559
Rau, G. H., Riebesell, U., and Wolf-Gladrow, D. (1996). A model of photosynthetic 13C fractionation by marine phytoplankton based on diffusive molecular CO2 uptake. Mar. Ecol. Prog. Ser. 133, 275–285. doi: 10.3354/meps133275
Rau, G. H., Takahashi, T., and Des Marais, D. J. (1989). Latitudinal variations in plankton δ13C: implications for CO2 and productivity in past oceans. Nature 341, 516–518. doi: 10.1038/341516a0
Repeta, D. J., Ferrón, S., Sosa, O. A., Johnson, C. G., Repeta, L. D., Acker, M., et al. (2016). Marine methane paradox explained by bacterial degradation of dissolved organic matter. Nat. Geosci. 9, 884–887. doi: 10.1038/ngeo2837
Saino, T. (1992). 15N and 13C natural abundance in suspended particulate organic matter from a Kuroshio warm-core ring. Deep Sea Res. Part A Oceanogr. Res. Pap. 39, S347–S362.
Sannigrahi, P., Ingall, E. D., and Benner, R. (2005). Cycling of dissolved and particulate organic matter at station Aloha: insights from 13C NMR spectroscopy coupled with elemental, isotopic and molecular analyses. Deep Sea Res. Part I Oceanogr. Res. Pap. 52, 1429–1444. doi: 10.1016/j.dsr.2005.04.001
Sasakawa, M., Tsunogai, U., Kameyama, S., Nakagawa, F., Nojiri, Y., and Tsuda, A. (2008). Carbon isotopic characterization for the origin of excess methane in subsurface seawater. J. Geophys. Res. Oceans 113:C03012.
Schmittner, A., Gruber, N., Mix, A. C., Key, R. M., Tagliabue, A., and Westberry, T. K. (2013). Biology and air-sea gas exchange controls on the distribution of carbon isotope ratios (delta C-13) in the ocean. Biogeosciences 10, 5793–5816. doi: 10.5194/bg-10-5793-2013
Siegel, D., Buesseler, K., Doney, S., Sailley, S., Behrenfeld, M. J., and Boyd, P. (2014). Global assessment of ocean carbon export by combining satellite observations and food-web models. Global Biogeochem. Cycles 28, 181–196. doi: 10.1002/2013gb004743
Tagliabue, A., and Bopp, L. (2008). Towards understanding global variability in ocean carbon-13. Global Biogeochem. Cycles 22:GB1025.
Tamelander, T., Søreide, J. E., Hop, H., and Carroll, M. L. (2006). Fractionation of stable isotopes in the Arctic marine copepod Calanus glacialis: effects on the isotopic composition of marine particulate organic matter. J. Exp. Mar. Biol. Ecol. 333, 231–240. doi: 10.1016/j.jembe.2006.01.001
Tolosa, I., Miquel, J.-C., Gasser, B., Raimbault, P., Goyet, C., and Claustre, H. (2008). Distribution of lipid biomarkers and carbon isotope fractionation in contrasting trophic environments of the South East Pacific. Biogeosciences 5, 949–968. doi: 10.5194/bg-5-949-2008
Trull, T., and Armand, L. (2001). Insights into Southern Ocean carbon export from the δ13C of particles and dissolved inorganic carbon during the SOIREE iron release experiment. Deep Sea Res. Part II Top. Stud. Oceanogr. 48, 2655–2680. doi: 10.1016/s0967-0645(01)00013-3
Trull, T. W., Davies, D., and Casciotti, K. (2008). Insights into nutrient assimilation and export in naturally iron-fertilized waters of the Southern Ocean from nitrogen, carbon and oxygen isotopes. Deep Sea Res. Part II Top. Stud. Oceanogr. 55, 820–840. doi: 10.1016/j.dsr2.2007.12.035
Umhau, B. P., Benitez-Nelson, C. R., Close, H. G., Hannides, C. C., Motta, L., Popp, B. N., et al. (2019). Seasonal and spatial changes in carbon and nitrogen fluxes estimated using 234Th: 238U disequilibria in the North Pacific tropical and subtropical gyre. Mar. Chem. 217:103705. doi: 10.1016/j.marchem.2019.103705
Van der Merwe, N. J., and Medina, E. (1991). The canopy effect, carbon isotope ratios and foodwebs in Amazonia. J. Archaeol. Sci. 18, 249–259. doi: 10.1016/0305-4403(91)90064-v
Wilkes, E. B., and Pearson, A. (2019). A general model for carbon isotopes in red-lineage phytoplankton: interplay between unidirectional processes and fractionation by RubisCO. Geochim. Cosmochim. Acta 265, 163–181. doi: 10.1016/j.gca.2019.08.043
Williams, P., and Gordon, L. (1970). Carbon-13: carbon-12 ratios in dissolved and particulate organic matter in the sea. Deep Sea Res. Oceanogr. Abstr. 17, 19–27. doi: 10.1016/0011-7471(70)90085-9
Wu, J., Calvert, S., Wong, C., and Whitney, F. (1999). Carbon and nitrogen isotopic composition of sedimenting particulate material at Station Papa in the subarctic northeast Pacific. Deep Sea Res. Part II Top. Stud. Oceanogr. 46, 2793–2832. doi: 10.1016/s0967-0645(99)00084-3
Xiang, Y., and Lam, P. J. (2020). Size-fractionated compositions of marine suspended particles in the Western Arctic Ocean: lateral and vertical sources. J. Geophys. Res. Oceans 125:e2020J16144. doi: 10.1029/2020JC016144
Keywords: carbon isotopes, particulate organic carbon, water column, marine organic carbon, phytoplankton
Citation: Close HG and Henderson LC (2020) Open-Ocean Minima in δ13C Values of Particulate Organic Carbon in the Lower Euphotic Zone. Front. Mar. Sci. 7:540165. doi: 10.3389/fmars.2020.540165
Received: 03 March 2020; Accepted: 13 August 2020;
Published: 08 September 2020.
Edited by:
Cindy Lee, Stony Brook University, United StatesReviewed by:
Matt McCarthy, University of California, Santa Cruz, United StatesCopyright © 2020 Close and Henderson. This is an open-access article distributed under the terms of the Creative Commons Attribution License (CC BY). The use, distribution or reproduction in other forums is permitted, provided the original author(s) and the copyright owner(s) are credited and that the original publication in this journal is cited, in accordance with accepted academic practice. No use, distribution or reproduction is permitted which does not comply with these terms.
*Correspondence: Hilary G. Close, aGNsb3NlQHJzbWFzLm1pYW1pLmVkdQ==
Disclaimer: All claims expressed in this article are solely those of the authors and do not necessarily represent those of their affiliated organizations, or those of the publisher, the editors and the reviewers. Any product that may be evaluated in this article or claim that may be made by its manufacturer is not guaranteed or endorsed by the publisher.
Research integrity at Frontiers
Learn more about the work of our research integrity team to safeguard the quality of each article we publish.