- 1CNRS, OCA, IRD, Université Côte d’Azur, Géoazur, Valbonne, France
- 2Laboratoire d’Océanographie de Villefranche, CNRS UMR 7093, Sorbonne Universités, Villefranche-sur-Mer, France
- 3Center for Marine Biotechnology and Biomedicine, Scripps Institution of Oceanography, University of California, San Diego, La Jolla, CA, United States
- 4Laboratoire MAPIEM, Université de Toulon, Toulon, France
- 5Marine Biodiscovery, School of Chemistry and Ryan Institute, National University of Ireland Galway, Galway, Ireland
Macroalgae constitute one of the preferred substrates of the benthic dinoflagellate Ostreopsis cf. ovata. Across the Mediterranean Sea, this toxic microalga has been shown to thrive on the surface of various species of macroalgae, including Chlorophyta, Rhodophyta, and Phaeophyceae. Interestingly, some Dictyotaceae are characterized by a low abundance of cells of O. cf. ovata on their surface. Based on the antifouling properties of some specialized metabolites produced by seaweeds, macroalgal metabolites have been proposed to contribute to the settlement and development of O. cf. ovata. To address this question, the composition of the surface of four Dictyotaceae, Dictyota dichotoma, Dictyota spiralis, Taonia atomaria, and Padina pavonica was investigated through an integrative approach combining the analysis of their eukaryotic diversity (18S rRNA gene metabarcoding), their surface metabolome (untargeted LC-MS-based metabolomics) as well as the bioactivity of their surface extracts on O. cf. ovata. Altogether, the data suggest an influence of the macroalgal surface chemistry on the growth of the dinoflagellate, with D. dichotoma being the most bioactive. Some metabolites are proposed to be involved in the observed bioactivity. Other biotic factors are also likely to be entailed in the control of the O. cf. ovata population and they may even prevail on the influence of the macroalgal surface chemistry.
Introduction
Recurrent outbreaks of the toxic dinoflagellate Ostreopsis cf. ovata have been reported over the past 15 years in Mediterranean coastal waters (Ciminiello et al., 2006; Mangialajo et al., 2011). Despite recent research efforts (reviewed in Pistocchi et al., 2011; Carnicer et al., 2015), the abiotic factors fostering the proliferation of this species remain unclear (Cohu et al., 2011; Meroni et al., 2018) although high temperature, low hydrodynamic conditions seem to constitute a pre-requisite (Totti et al., 2010; Pezzolesi et al., 2012; Meroni et al., 2018). O. cf. ovata is a benthic species that colonizes a variety of substrates, including macrophytes (Totti et al., 2010; Mangialajo et al., 2011). The surface of these seaweeds can constitute an ideal microenvironment for the growth of highly diverse prokaryotic and eukaryotic communities, including benthic microalgae. While the interactions between bacteria and macroalgae have been investigated to some extent during the past decades (Egan et al., 2013; Hollants et al., 2013), less information has been provided about the drivers guiding the settlement of microalgae on the surface of macroalgae.
Ostreopsis cf. ovata has been shown to thrive on the surface of various species of macroalgae across the Mediterranean Sea, including Chlorophyta (Battocchi et al., 2010; Accoroni et al., 2015; Hosny and Labib, 2019), Rhodophyta (Vila et al., 2001; Battocchi et al., 2010; Cohu et al., 2013; Blanfuné et al., 2015; Hosny and Labib, 2019; Gémin et al., 2020) and Phaeophyceae (Vila et al., 2001; Battocchi et al., 2010; Cohu et al., 2013; Blanfuné et al., 2015; Hosny and Labib, 2019; Gémin et al., 2020), without any clear species preference. Remarkably, some Phaeophyceae of the family Dictyotaceae are usually characterized by a low abundance of O. cf. ovata as for instance Taonia atomaria, Dictyota fasciola, or Padina pavonica (Blanfuné et al., 2015; Hosny and Labib, 2019; Gémin et al., 2020). The antifouling properties of some specialized metabolites produced by Dictyotaceae have been proposed to drive the settlement and development of O. cf. ovata (Cohu et al., 2013; Blanfuné et al., 2015; Gémin et al., 2020). Approaches in chemical ecology have revealed that metabolites produced by macroalgae can be detrimental or beneficial to the settlement of bacteria and microalgae (Goecke et al., 2010; Hellio et al., 2002; Wichard and Beemelmanns, 2018). In particular, several metabolites produced by species of the family Dictyotaceae have been shown to inhibit the growth of bacteria (e.g., dictyol C and fucoxanthin; Salvador Soler et al., 2007; Viano et al., 2009), microalgae (e.g., dictyolactone and sanadaol; Kim et al., 2006), bryozoan (e.g., dictyol E, pachydictyol A, dictyodial; Schmitt et al., 1998) and various other benthic species (e.g., diterpenoids, gleenol, and geranylgeranylglycerol; Bianco et al., 2009; Othmani et al., 2016b).
To the best of our knowledge, only one study has investigated the effect of the metabolites from brown, red and green macroalgae (Dictyota dichotoma, Rhodymenia pseudopalmata, and Ulva rigida, respectively) on the growth of the dinoflagellate O. cf. ovata (Accoroni et al., 2015). The exposure to dried algal cells, fresh thalli and dissolved metabolites of D. dichotoma caused an intense stress to O. cf. ovata cells yielding to growth inhibition as well as formation of double-walled cysts. Conversely, this inhibiting activity of D. dichotoma metabolites on the toxic dinoflagellate does not mirror the frequent development of O. cf. ovata on D. dichotoma observed in previous studies performed in the NW Mediterranean Sea (Cohu et al., 2013; Blanfuné et al., 2015; Gémin et al., 2020). Following common practices in the chemical ecology of macroalgae (Puglisi et al., 2014), the authors used whole-cell macroalgal extracts which are not representative of real ecological situations occurring at the surface of macroalgae. Therefore, the influence of the surface metabolome of brown algae on the growth and settlement of O. cf. ovata remains largely to be assessed. Results of such studies will provide valuable information on the pattern of colonization of this toxic dinoflagellate along the NW Mediterranean shoreline that leads to ecological (Gorbi et al., 2013; Giussani et al., 2016; Pavaux et al., 2019) and health (Ciminiello et al., 2006; Casabianca et al., 2013) concerns.
The epiphytic community colonizing the surface of macroalgae is not only expected to contribute to the surface metabolome but also to directly interact with O. cf. ovata, adding another level of complexity to its settlement preferences. Among co-occurring species on surfaces colonized by O. cf. ovata, Roseobacter bacteria (Vanucci et al., 2016; Guidi et al., 2018), diatoms of the genera Licmophora, Navicula, and Amphora, and the dinoflagellates Prorocentrum lima, Amphidinium carterae, and Coolia monotis (Accoroni et al., 2015; Marro et al., 2019) have been reported to be the most abundant. Recent evidences of chemically mediated interactions between microalgae and their predators (Ianora et al., 2004; Selander et al., 2015), competitors (Tillmann and Hansen, 2009; Poulson-Ellestad et al., 2014; Ternon et al., 2018), and the bacterial community (Wichard and Beemelmanns, 2018 and references therein) suggest that biotic interactions within members of the epiphytic community may also influence the settlement of O. cf. ovata on macroalgal surfaces.
This study aims to investigate the influence of the surface metabolome of four brown algae of the Dictyotaceae family (T. atomaria, P. pavonica, D. dichotoma, and Dictyota spiralis) on the settlement of the toxic dinoflagellate O. cf. ovata in the NW Mediterranean. An integrative approach was used to investigate both the chemical and eukaryotic compositions at the surface of each algal species combining untargeted LC-MS-based metabolomics and high throughput sequencing (18S rRNA Illumina MiSeq sequencing), respectively. Applications of dedicated experimental protocols allowed the selective extraction of the surface metabolites of each algal species (Othmani et al., 2016a) and the activity of these extracts were further tested on monocultures of the toxic dinoflagellate.
Materials and Methods
Field sampling was performed at 9 a.m. on the 7th of July 2017 during an O. cf. ovata bloom at the Rochambeau site in the bay of Villefranche-sur-Mer (France, Ligurian Sea; 43°41′34.83″ N, 7°18′31.66″ E) known for the recurrence of such events (Cohu et al., 2013; Jauzein et al., 2018; Gémin et al., 2020). The seawater temperature and salinity were 23.9°C and 39, respectively, at the time of the collection. Thirteen specimens of each macroalgal species (D. dichotoma, D. spiralis, P. pavonica, and T. atomaria) were collected at 0.5 m depth by snorkeling. The collection of the macroalgae followed the protocol recommended by Totti et al. (2010) and consisted in holding specimens of each species with zip bags avoiding any agitation of the surrounding seawater and resuspension of benthic cells, followed by a careful removing of their holdfast from the rocky substrate using tweezers. All zip bags containing macroalgae specimens and surrounding seawater were immediately closed underwater and processed in the lab the same morning using: (i) four specimens of each species to measure the abundance of O. cf. ovata and diatoms at the algal surface, (ii) six specimens of each species to extract the surface and the whole macroalgal metabolomes, and (iii) three specimens of each species for the analysis of epiphytic eukaryotic communities through 18S rRNA gene high-throughput sequencing. After sampling for macroalgae, a volume of 1 L of seawater was also collected from the water column at the sampling site for 18S rRNA gene high-throughput sequencing.
Microalgal Cell Abundance at the Surface of the Macroalgae
Four specimens of each macroalga were transferred from their zip bag to 15 mL Falcon tubes filled with 4 mL of filtered seawater (0.2 μm) and 150 μL of acidic Lugol’s iodine solution. The tubes were vigorously shaken and then vortexed during 5 s to remove epiphytes. Supernatants were kept in the Falcon tubes while the macroalgal thalli were removed with tweezers and air-dried on a filter paper. No pre-filtration of the supernatant was needed (Totti et al., 2010) as sediments were not abundant. Pictures of the macroalgae were taken to further estimate their surface area using the Mesurim® software and to allow the expression of the cell abundance in cell cm–2. Epiphytic cell abundances in the rinsed water were determined in a 1 mL volume Sedgwick Rafter counting chamber under an inverted phase contrast microscope (Zeiss Axiovert 40C) at a magnification of ×40. Identification of diatoms and dinoflagellates was performed using data from the literature (Bertalot et al., 2000; Horiguchi, 2014, respectively).
Chemical Extraction and Analysis of Macroalgal Samples
The surface metabolome of the four seaweed species was investigated on six replicates by a dipping method previously developed for T. atomaria (Othmani et al., 2016a) that caused no disruption of its membranes. P. pavonica is expected to have similar membrane strength as its surface is calcified (Benita et al., 2018). The integrity of the cell walls of the two softer Dictyota species was confirmed after soaking using an optical microscope. Briefly, surface extracts (SEs) were obtained by soaking each algal thallus during 5s in an 8 mL glass vial filled with 5 mL of methanol (MeOH). The total extracts (TEs) were obtained by soaking each thallus in 7 mL of MeOH during 18 h. Both resulting extracts (SEs and TEs) were concentrated under reduced pressure and stored at −20°C until analyses and bioassays. The approximate extracted surface area of each thallus was measured therefore leading to an estimation of the amount of extracts per cm2 of macroalga (Table 1).
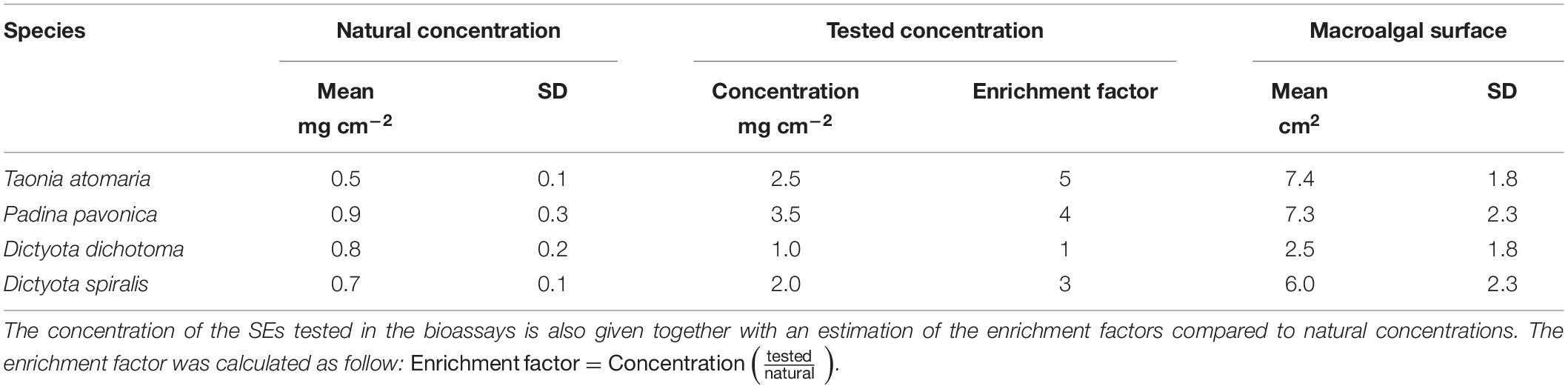
Table 1. Mean concentration (mg cm–2) of the SEs obtained from each macroalgal species given with the mean surface area measured for each species (n = 4).
DNA Extraction and Amplification of the Epiphytic Communities
The epiphytic microbiota was sampled using a sterile scalpel, introduced into plastic Eppendorf tubes (2 mL) and flash frozen in liquid nitrogen. DNA extraction was carried out using the PowerBiofilm DNA isolation Kit (Qiagen) and samples were preserved at −80°C. After DNA extraction, the V7 region of 18S rRNA gene was amplified using 960F and NSR1438R primers following Debroas et al. (2017). Amplicons were sent to GeT Platform (Toulouse, France) for MiSeq Illumina sequencing (2 × 250 bp).
18S rRNA Gene Metabarcoding Data Processing and Analysis
18S rRNA gene reads were processed using the FROGS workflow under Galaxy environment (Escudié et al., 2017). Sequences were quality filtered by removing those for which primers sequences were not present. The primer search accepts 10% of differences. Primers sequences were then removed in the remaining sequence using “cutadapt.” Clustering step was performed using SWARM with a clustering aggregation distance set to 3 (Mahé et al., 2014). Chimeric sequences were removed de novo using VSEARCH (Rognes et al., 2016). Rare OTUs representing less than 0.005% of all sequences were removed. OTUs were affiliated with the Silva 132 18S rRNA. The final matrix was obtained by performing a rarefaction to the minimum library size (8975 reads) using the “phyloseq” R package (McMurdie and Holmes, 2013). Eukaryotic β-diversity was analyzed with a non-metric multidimensional scaling (NMDS) using Jaccard distances with the “phyloseq” package (McMurdie and Holmes, 2013). PERMANOVA test was performed using the distance matrix constructed with the Jaccard index, using the “vegan” R package (Oksanen et al., 2013).
Bioassays
A bioassay was designed to test the activity of the SEs in 48-well plates for which the well surface is ∼1 cm2. The low amounts of SEs available (0.2–5 mg) allowed only one bioassay. The activity of compounds of the SEs being unknown, the highest concentrations prepared from the SEs were tested to ensure a biological response: 2.5, 3.5, 1, and 2 mg cm–2 for T. atomaria, P. pavonica, D. dichotoma, and D. spiralis, respectively. These concentrations are higher than those naturally encountered at the surface of the macroalgae by a factor 5, 4, 1, and 3, respectively (Table 1). All SEs were re-suspended in dimethyl sulfoxide (DMSO) to reach concentrations of 50, 70, 20, and 40 mg mL–1, respectively for each alga. A volume of 50 μL of each SE was added to the wells containing a thin layer of fresh unsolidified agar and further dried at room temperature (0.3% of DMSO final volume). A same volume of DMSO was similarly added to six more wells as a control.
A monoclonal strain of O. cf. ovata obtained from the MCCV (Mediterranean Culture Collection of Villefranche, MCCV054) was grown in L1 medium (Guillard and Ryther, 1962) prepared with autoclaved aged and filtered seawater (from the Bay of Villefranche), adjusted to a salinity of 38 and maintained at 24°C under a 14:10 light/dark cycle with a light intensity of 250 μmol m–2 s–1. After four days of growth (early exponential phase), 2 mL of the microalgal culture were sampled and fixed with acidic Lugol’s iodine solution (4% v/v) and the cell abundance was determined using a Sedgwick rafter counting chamber and an inverted phase contrast microscope (Zeiss Axiovert 40C) at a magnification of ×40. Appropriate volumes of culture and L1 medium were added to each well previously filled with SEs or DMSO (blank) to obtain a concentration of 100 cell mL–1 of O. cf. ovata cells with a final volume of 1.4 mL. All well plates were incubated 24 h in the same conditions as for the cultures.
To monitor the bioactivity of the SEs on O. cf. ovata, both the physiological state and the abundance of the cells were measured after 24 h of incubation. All well plates were placed in the dark for 15 min and the well content are successively transferred to a 2 mL glass cuvette immediately moved to a MC-PAM (Multi-Color Pulse-Amplitude-Modulated, Heinz Walz Gmbh, Effeltrich, Germany) equipped with a blue LED (440 nm) as a source for the actinic light and a white LED used for the saturating pulses. The maximum quantum yield (Fv/Fm) of the photosystem II (PSII) was used as a proxy of the microalga physiological state. It was calculated as (Fm−F0)/Fm, where F0 is the fluorescence of a dark-adapted sample and Fm is measured after application of a saturation pulse of light (intensity 431 μE m–2 s–1, 300 ms duration). Curve-fitting software provided with the instrument (PAMwin V3.20W) was used to obtain Fv/Fm. All curve fits and fluorescence transients were manually inspected in real time. Each sample was placed back in its well after the PAM measurement and immediately fixed with acidic Lugol’s iodine solution (4% v/v) for cell counting under microscope as described earlier. The growth rate of O. cf. ovata was calculated for each well as follows:
where N1 and N2 are the cell abundances at times t1 (beginning of the experiment) and t2 (sampling after 24 h).
UHPLC-HRMS Analysis of Macroalgal Extracts
Before injection, samples were solubilized in 1 mL of LC-MS grade MeOH. Eleven quality control samples (QCs) and four analytical blanks were also prepared as described in Paix et al. (2020), and all samples were randomly injected to avoid systematic errors. Acquisition was performed by UHPLC-HRMS using a Dionex Ultimate 3000 rapid Separation chromatographic system (Thermo Fisher Scientific, Waltham, MA, United States) coupled to a QToF Impact II mass spectrometer (Bruker Daltonics, Bremen, Germany) equipped with an electrospray source. A volume of 5 μL of each sample was injected and analyzed on a reversed phase column (150 × 2.1 mm, 1.7 μm, Kinetex Phenyl-Hexyl equipped with a SecurityGuard cartridge; Phenomenex, Le Pecq, France) with a column temperature of 40°C and a flow rate of 0.5 mL min–1. Mobile phases were (A) water and (B) acetonitrile (Chromasolv; Sigma-Aldrich-Merck, Darmstadt, Germany) containing each 0.1% (v/v) of formic acid (Ultra grade; Fluka, Fischer Scientific, Illkirch, France). The elution gradient started at 5% B and kept for 2 min, then to 100% B (linear ramp) in 8 min and kept for 4 min; then back to 5% B (linear ramp) over 0.01 min and maintained 1.99 min, for a total run time of 16 min. Mass spectrometry (MS) analyses were performed in the full scan positive mode (ESI+). The capillary voltage of the MS spectrometer was set at 4500 V and the nebulizing parameters were set as follows: nebulizing gas (N2) pressure at 0.4 bar, drying gas (N2) flow at 4 L min–1, and drying temperature at 180°C. Mass spectra were recorded from m/z 50 to 1200. Tandem mass spectrometry analyses were performed with a collision induced dissociation (CID) and collision energy of 25 eV. A solution of formate/acetate forming clusters was automatically injected before each sample for internal mass calibration, and the mass spectrometer was calibrated with the same solution before each sequence of samples. Raw UHPLC-HRMS data were analyzed using DataAnalysis (version 4.3; Bruker Daltonics), converted into netCDF files and processed for peak finding, integration and alignment using the open source XCMS package (version 1.46.0; Smith et al., 2006) in the R 3.2.3 environment. The resulting variables list was filtered using three successive steps (signal/noise ratio, coefficient of variation and coefficient of autocorrelation) with an in-house script running on R. These variables were log10-transformed, mean-centerd and normalized using the sum of the chromatographic peak areas as described in Paix et al. (2020), and analyzed by Principal Component Analysis (PCA) and partial least-square discriminant analysis (PLS-DA) using the MetaboAnalyst 3.5 online resource1. The global annotation was assessed by comparison of MS/MS spectra with those of an in-house database (purified compounds and commercial standards), and public databases (MarinLit, MetLin, MoNA, or Lipidmaps). Subsequently, the annotation procedure of analogs of known compounds was facilitated by molecular networking using the GNPS platform2.
Results
Assessment of the Natural Abundance of Benthic Microalgae Using Microscopy
All four macroalgal species had their surface colonized by diatoms, including species of the genera Licmophora, Amphora., Navicula, Cylindrotheca, Striatella, and Gyrosigma, as well as the dinoflagellate O. cf. ovata (Figure 1). O. cf. ovata showed a lower abundance than diatoms, representing 14–23% of the total number of counted cells, with densities ranging from 1.5 to 7.9 × 103 cell cm–2. Significant different concentrations of O. cf. ovata cells were observed between macroalgal hosts (ANOVA: p = 0.0175). Post hoc analysis revealed a significantly higher concentration of cells on D. dichotoma compared to D. spiralis and P. pavonica, while no significant differences were detected between T. atomaria, P. pavonica, and D. spiralis, and neither between T. atomaria and D. dichotoma – due to one outlier sample.
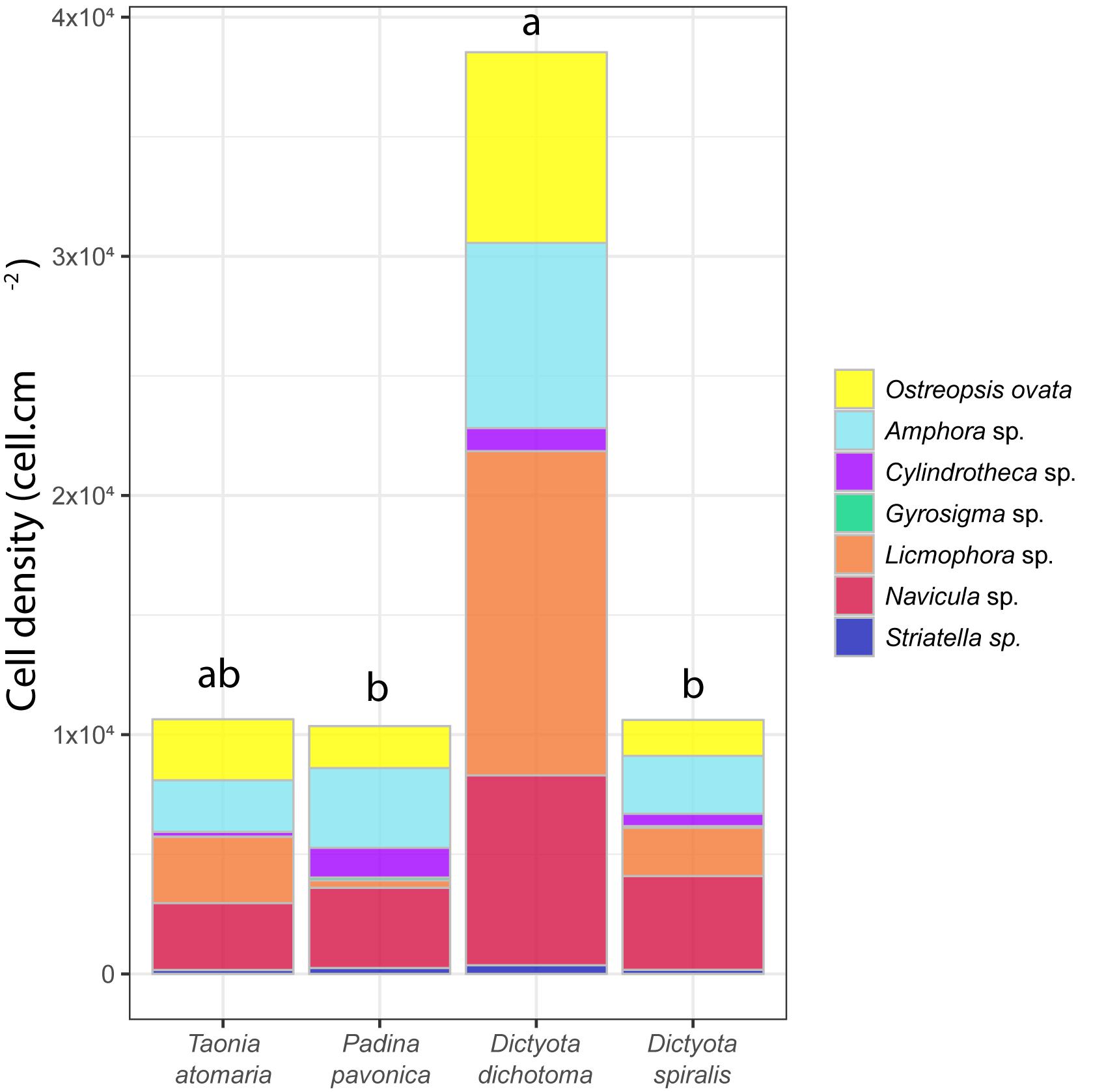
Figure 1. Cell density (cell cm–2) of major diatoms and O. cf. ovata at the surface of the four macroalgae. Statistical significance was evaluated only for O. cf. ovata abundances using an ANOVA test (*p = 0.0175) followed by a post hoc Tukey’s test (results indicated by a,b indexes).
Diversity of Epiphytic Eukaryotes Through 18S rRNA Gene HTS
When compared to the number of OTUs, the Chao1 index reflects the richness that could be reached if a higher number of sequences has been obtained. Here, Chao1 indicated that the highest richness of eukaryotic communities was observed in seawater as well as on the surface of T. atomaria and P. pavonica (Supplementary Figure S1). Considering the β-diversity, seawater communities were clearly dissimilar from those found on the macroalgal surfaces (Figure 2 and Supplementary Figure S2). The dinobiontes seemed to constitute the major taxa in seawater, with Amphidinium being the most represented genus (54%) followed by Alexandrium (9%), while Ostreopsis only represented 3%. Among epiphytes, a clear clustering at the algal species level could be noticed, with the two Dictyota species communities on the one hand, and T. atomaria and P. pavonica communities on the other hand (Supplementary Figure S2). Using the Jaccard index with a NMDS analysis, the β-diversity of the eukaryotic communities revealed clear differences between the epiphytic and the planktonic communities, but also between macroalgal hosts (Figure 2 and Supplementary Figure S2, PERMANOVA: p = 0.001).
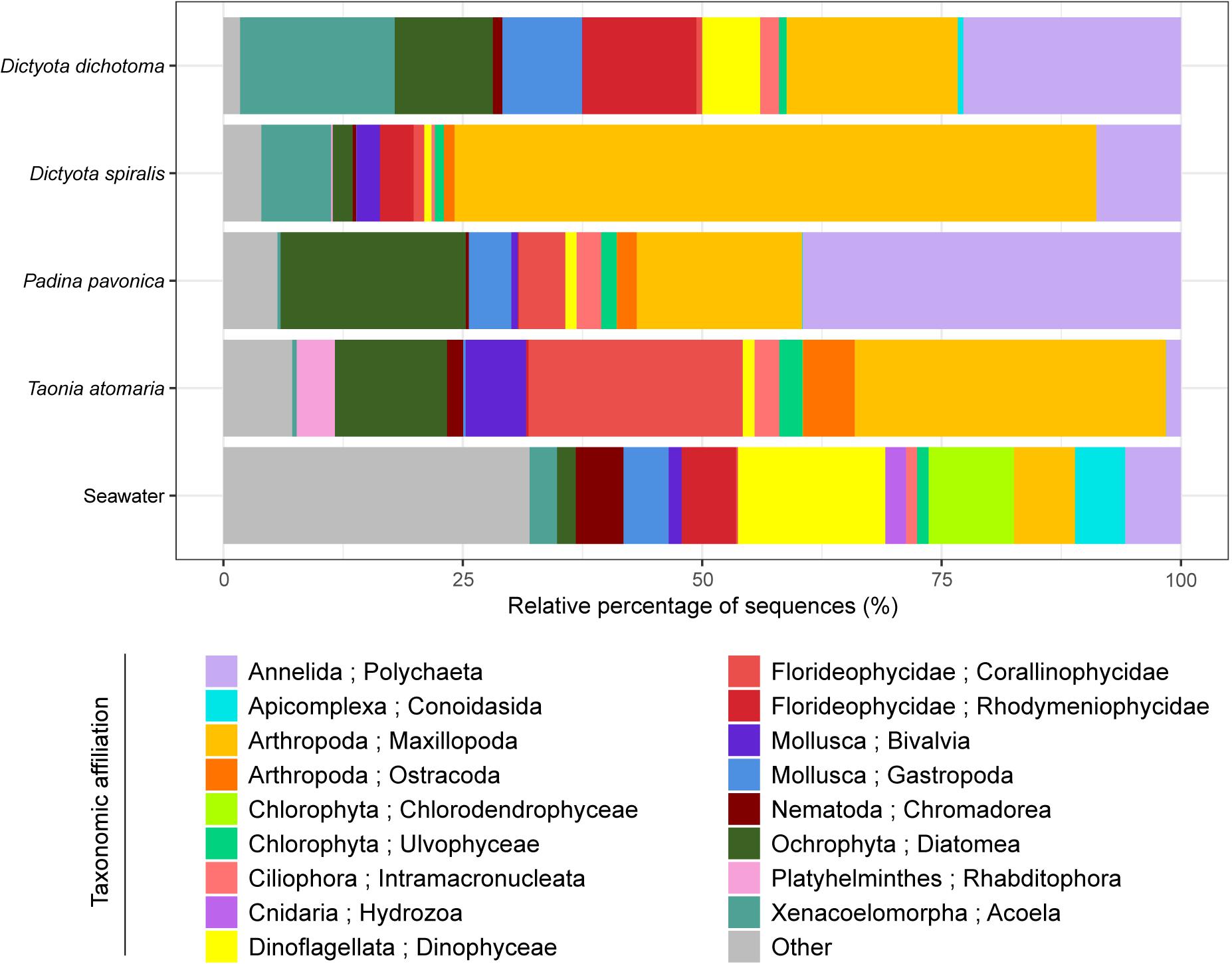
Figure 2. Eukaryotic communities colonizing the surface of the four macroalgae and present in the surrounding seawater. Replicate samples of a same species were averaged (n = 3) and relative colonization to all sequences is given (%). Taxonomic affiliation corresponds to the Phylum and Class level. “Other” combines Classes that represent less than 2% of the total diversity.
Even though they serve as support of common taxa, like Dinoflagellata, Arthropoda, Ochrophyta, Annelida, Florideophycideae, and Mollusca, the four macroalgae species exhibited a contrasted composition of their epiphytic eukaryotic communities. Among the major detected taxa, two belonged to microalgae (Ochrophyta and Dinoflagellata), one to copepods (Arthropoda), two to red algae (Florideophycideae) and two to upper benthic organisms (Annelida and Mollusca). The major species of diatoms present on the macroalgae belonged to the genera Berkeleya, Navicula, Haslea, Nitzschia, Actinocyclus, Amphora, Cylindrotheca, Cocconeis, Psammoneis, Hyalosira, and Frustulia and their relative abundance was the highest on P. pavonica (19%) and the lowest on D. spiralis (2%). The abundance of Dinoflagellata in the epiphytic community determined by cell counting was highly consistent with the results obtained by 18S rDNA gene sequencing. The major OTU identified belonged to the genus Ostreopsis, while other dinoflagellate taxa, without specific affiliation at the genus level, were also detected with very few sequences. Dinoflagellates represented only 1% of the total eukaryotic diversity on T. atomaria, P. pavonica, and D. spiralis while it reached 6% on D. dichotoma. The Harpacticoids, Amphiascoides, and Paramphiascella were the main Arthropoda families and the species Amonardia coreana, Parastenhelia sp., Amphiascoides atopus, and Paramphiascella fulvofasciata were annotated. Several Annelida species of the Phyllodocida family were detected, including Platynereis dumerilii and Syllis pigmentata; they were mainly distributed on D. dichotoma and P. pavonica. The major platyhelminthe species was Convoluta convoluta which was reported on the two Dictyota spp. The Arthropoda were particularly represented on D. spiralis (67%) and T. atomaria (32.5%) while P. pavonica showed the highest Annelida community (39.5%). The Florideophycideae also largely contributed to the eukaryotic diversity on T. atomaria (22.4%). These same taxa were shown to colonize D. dichotoma, none of them being predominant: Dinoflagellata (6%), Arthropoda (18%), Ochrophyta (10%), Annelida (23%), Florideophycideae (12%), Mollusca (8%), and Xenacoelomorpha (16%).
Chemical Analysis of Macroalgal Extracts
The chemical diversity of the four macroalgal extracts was assessed by untargeted LC-MS-based metabolomics and led to 375 features after filtering. The PCA score plot of the surface and total (SEs and TEs) macroalgal extracts (Figure 3) showed a clear separation between the two Dictyota spp. and the two other species (T. atomaria and P. pavonica), mainly on the first component (48% of the total variance) highlighting a distinct chemical composition between these species. Furthermore, the second component (18.5% of the total variance) highlighted differences between SEs and TEs within each of these two algal groups. A list of the features responsible for the differences between SEs and TEs for each species is provided in Supplementary Table S1. The vast majority of these metabolites being minor ions on the chromatograms, very few could be annotated. Most metabolites specific to the SEs were small/polar compounds as suggested by their retention time (RT < 1 min) whereas TEs were more enriched in less polar metabolites (RT > 8 min). Dimethylsulfoniopropionate (DMSP), Lyso-diacylglyceryl-3-O-carboxyhydroxymethylcholine (C16:0) and a compound, putatively annotated as acetyl-L-cysteine, were found to be enriched in the SEs of T. atomaria, P. pavonica, and D. dichotoma, respectively.
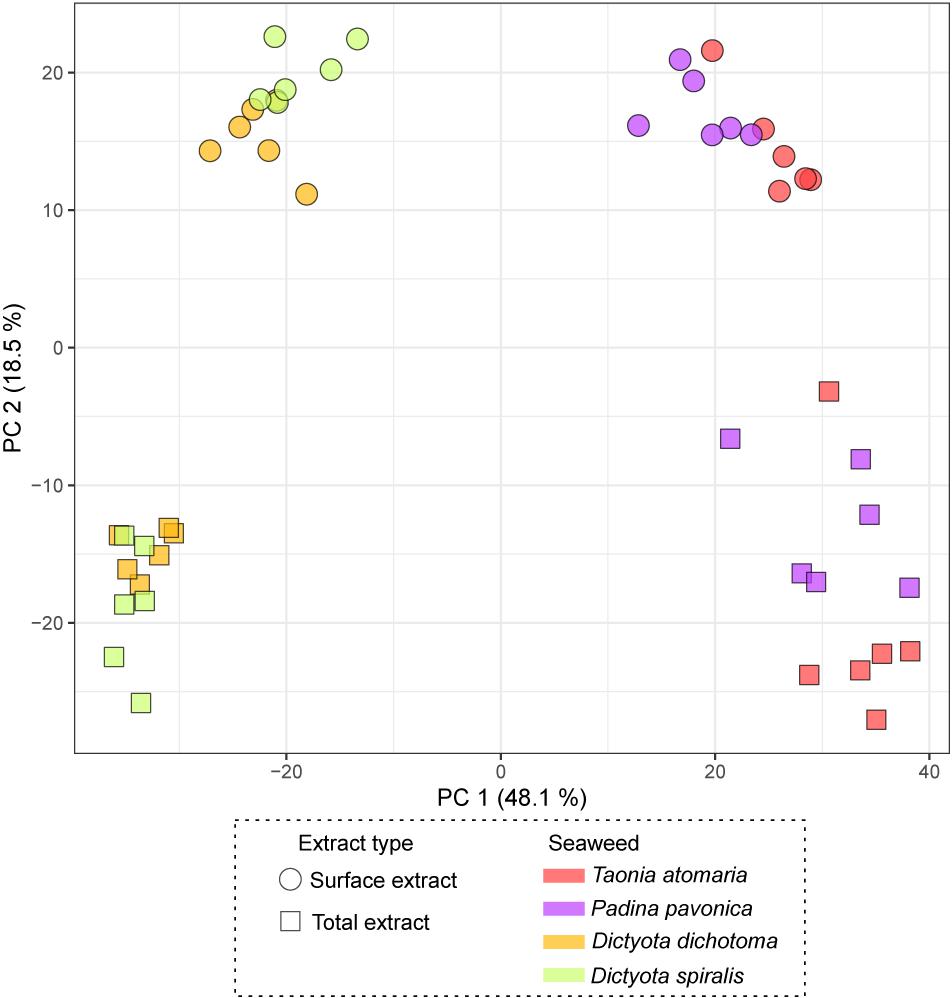
Figure 3. PCA score plot obtained from LC-(+)-ESI-MS metabolomics analyses of both the total extracts (TEs) and surface extracts (SEs) of the four macroalgae.
The score plot of the PLS-DA only built with SEs (Figure 4) offered a more focused view of the distribution of these extracts. None of the SEs overlapped with 95% confidence, suggesting significant differences in their chemical content, even for the two Dictyota spp. The distribution of the samples on both the PCA and the PLS-DA score plots was highly driven by the large number of metabolites produced by D. dichotoma and D. spiralis. The first 50 Variable Importance of Projection (VIPs) responsible for the separation of the samples on the PLS-DA score plot (VIP score >1.1) are listed in Table 2.
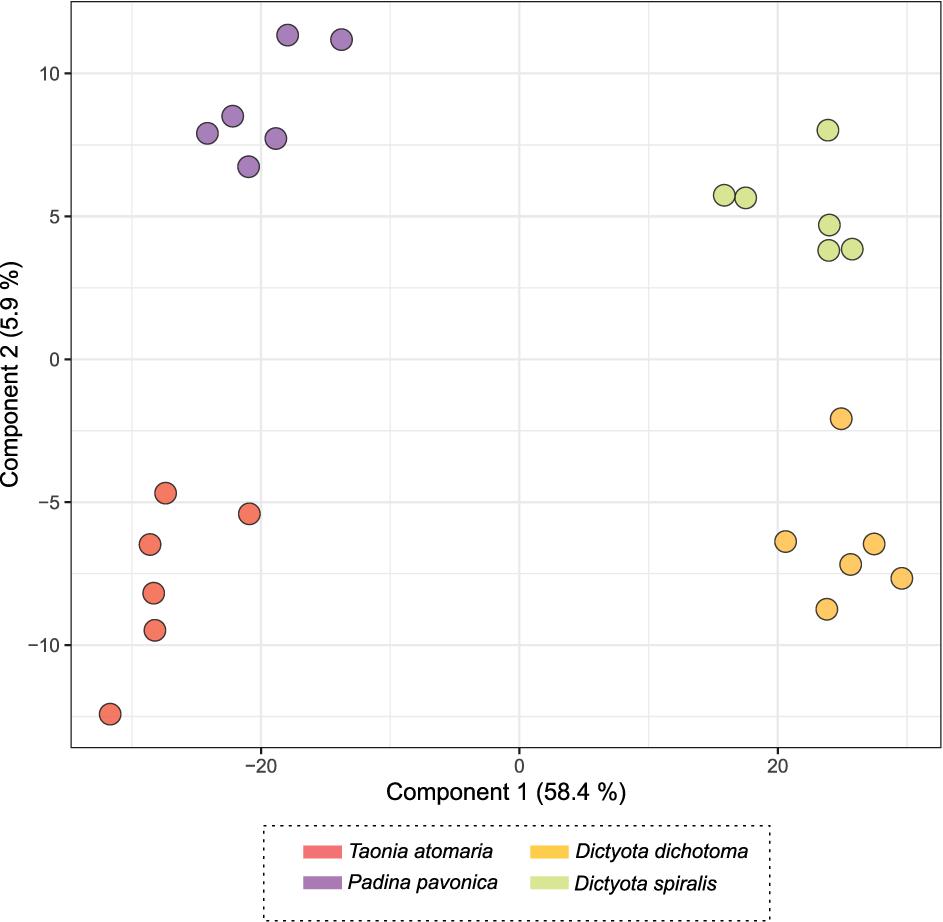
Figure 4. PLS-DA score plot obtained from LC-(+)-ESI-MS metabolomics analyses of the surface extracts (SEs) of the four macroalgae.
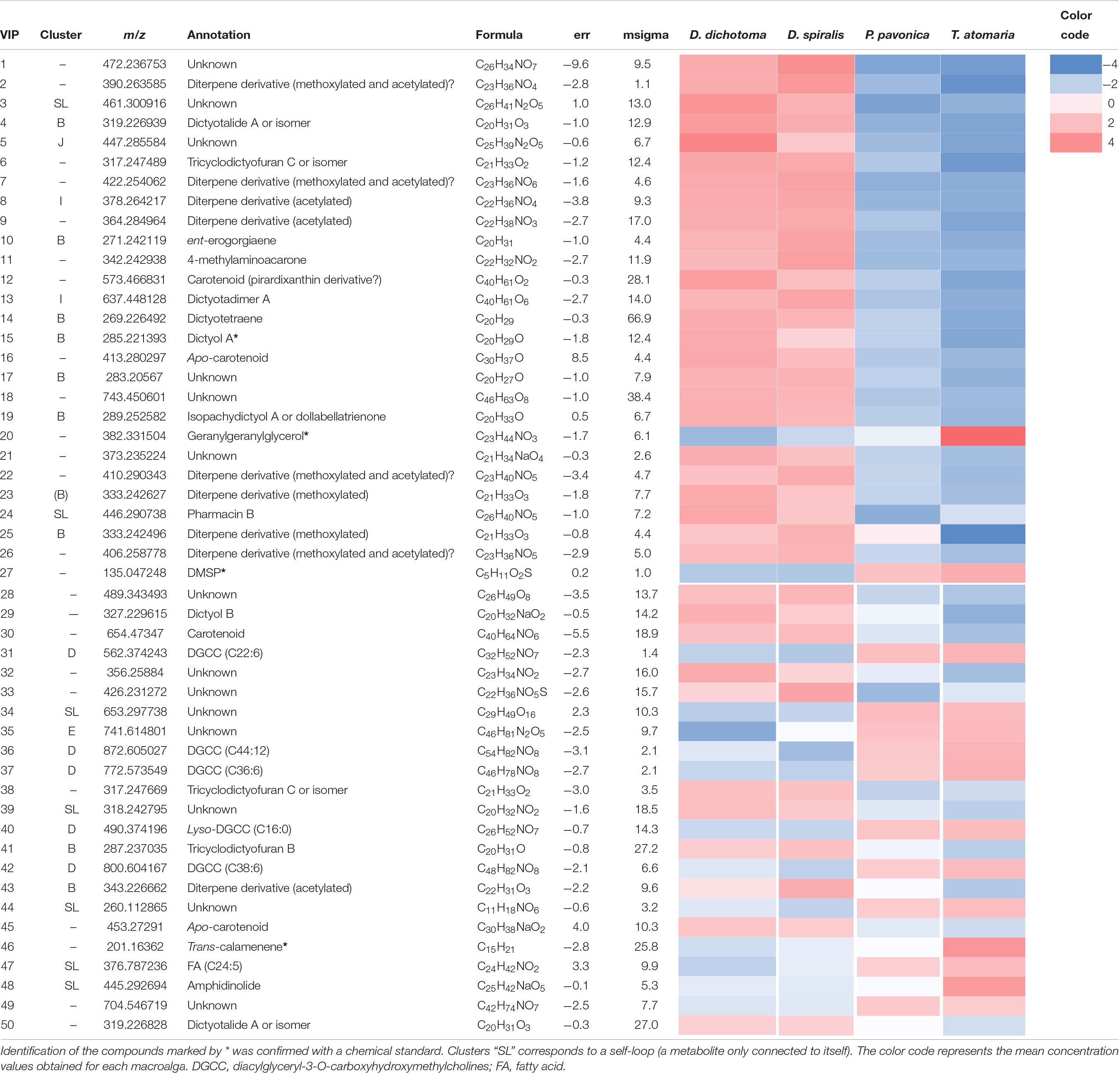
Table 2. Putative annotation of the first 50 chemical markers (VIP score >1.1) driving the distribution of the samples on the PLS-DA score plot obtained from LC-(+)-ESI-MS metabolomics analyses of the SEs of the four macroalgae.
The Search of matches of MS data from compounds of our in-house database, the MarinLit database and molecular network built from MS/MS spectra analysis (Figure 5) allowed the annotation of several of the VIPs characteristic of each macroalga (Table 2).
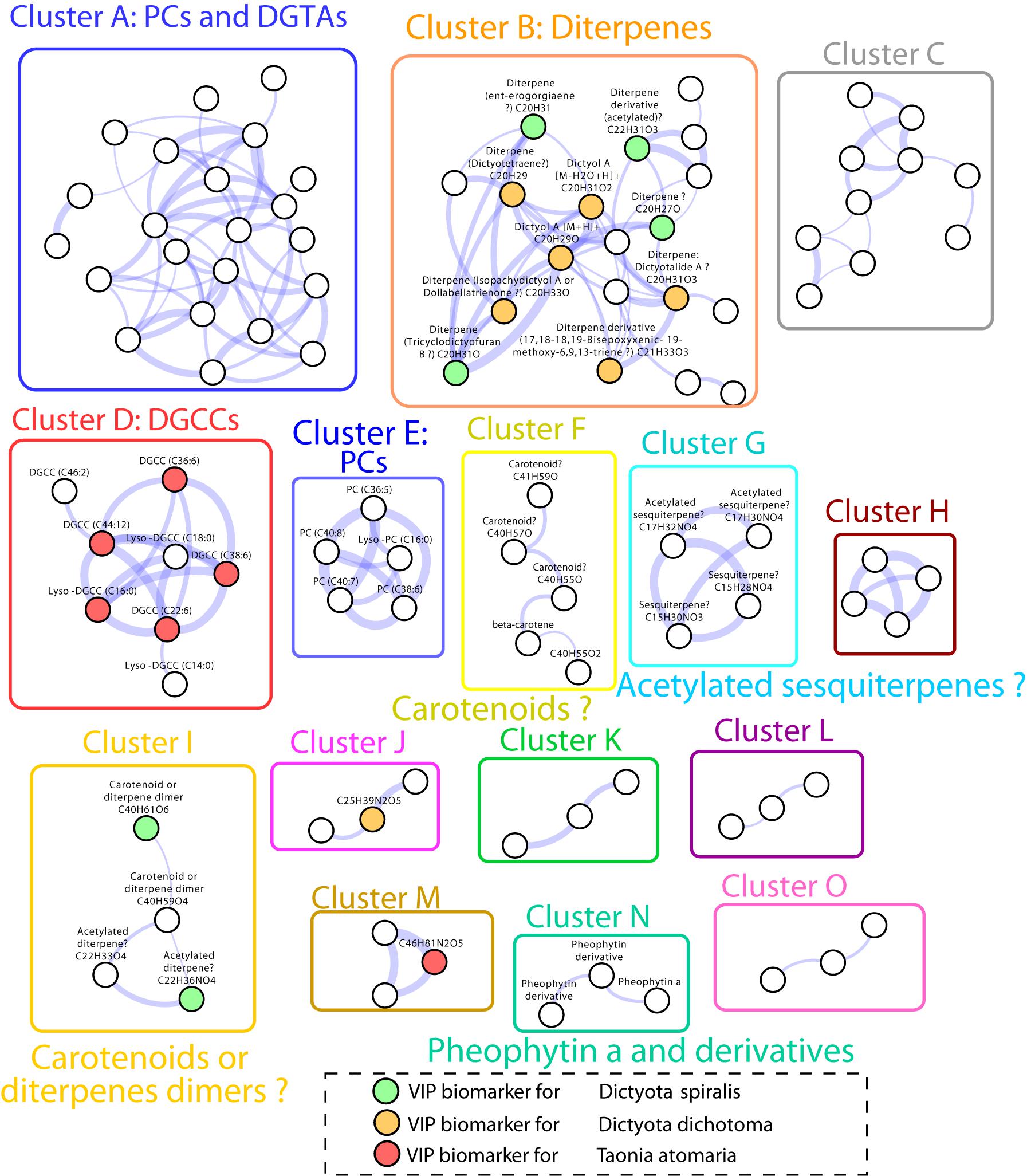
Figure 5. Molecular network obtained from LC-(+)-ESI-MS/MS data of the SEs of the four macroalgal species. Only clusters with three or more nodes are represented. Nodes represent MS/MS spectra which are connected based on their spectral similarity. Colored nodes indicate biomarkers according to the four macroalgae (orange: D. dichotoma, green: D. spiralis, red: T. atomaria, no VIPs for P. pavonica were observed). The edge width is proportional to the cosine score.
Several chemical markers enriched in D. dichotoma and D. spiralis SEs were detected in cluster B and I of the molecular network, allowing their putative annotation as diterpene derivatives. More precisely, the diterpenes dictyotalide A (or an isomer) (VIP n°4), ent-erogorgiaene (VIP n°10), dictyotetraene (VIP n°14), dictyol A (VIP n°15), isopachydictyol A (VIP n°19), 17,18-18,19-bisepoxyxenic-19-methoxy-6,9,13-triene (VIP n°25) and tricyclodictyofuran C (VIP n°6 and 41) were putatively identified in cluster B, and dictyotadimer A (VIP n°13) in cluster I. Finally, even if not appearing in the molecular network, dictyol B (VIP n°29) was also putatively annotated. Several other VIPs were not identified but were characterized as diterpenes or diterpene derivatives using their MS and MS/MS data.
Both the lipid geranylgeranylglycerol and the sulfur compound DMSP (VIPs n°20 and 27, respectively) were identified by the mean of chemical standards as the major markers found in T. atomaria SEs. Five other compounds (VIPS n°31, 36, 37, 40, and 42), putatively identified as diacylglyceryl-3-O-carboxyhydroxymethylcholines [DGCCs (C22:6), (C44:12), (C36:6); Lyso-DGCCs (C16:0), and (C38:6), respectively), were also detected in T. atomaria and gathered in cluster D. Finally, the VIP n°46 was putatively annotated as the sesquiterpene trans-calamenene using an in-house standard.
Padina pavonica was the algal species that showed the least chemical richness on its surface with only two unidentified markers (VIPs n°34 and 49) being found in its corresponding SEs.
More compounds not listed as VIPs were identified from other clusters of the molecular network: diacylglycerylhydroxymethyl-N,N,N-trimethyl-β-alanines (DGTAs) and phosphatidylcholines (PCs) present in cluster A, other PCs in cluster E, carotenoids in cluster F, sesquiterpenoids in cluster G, and pheophytin a derivatives in cluster N.
Bioactivity of Surface Extracts on O. cf. ovata
To ensure a measurable effect on O. cf. ovata cells, the maximal concentration that could be obtained from the SEs was tested for their bioactivity. Therefore, tested concentrations varied from 1 to 3.5 mg cm–2 according to the species and comparison between bioactivities should be treated cautiously. Three macroalgal SEs out of four induced a significant growth reduction of O. cf. ovata (p < 0.001; Figure 6A), with D. spiralis SE found to be not statistically active. Despite tested at the lowest concentration (1 mg cm–2), the inhibition of O. cf. ovata growth induced by D. dichotoma SEs was similar to those of P. pavonica while being significantly lower than the one of T. atomaria. Although similar concentrations were used for T. atomaria and D. spiralis SEs (2.5 and 2 mg cm–2, respectively), the inhibition on O. cf. ovata growth induced by T. atomaria SEs was significantly higher.
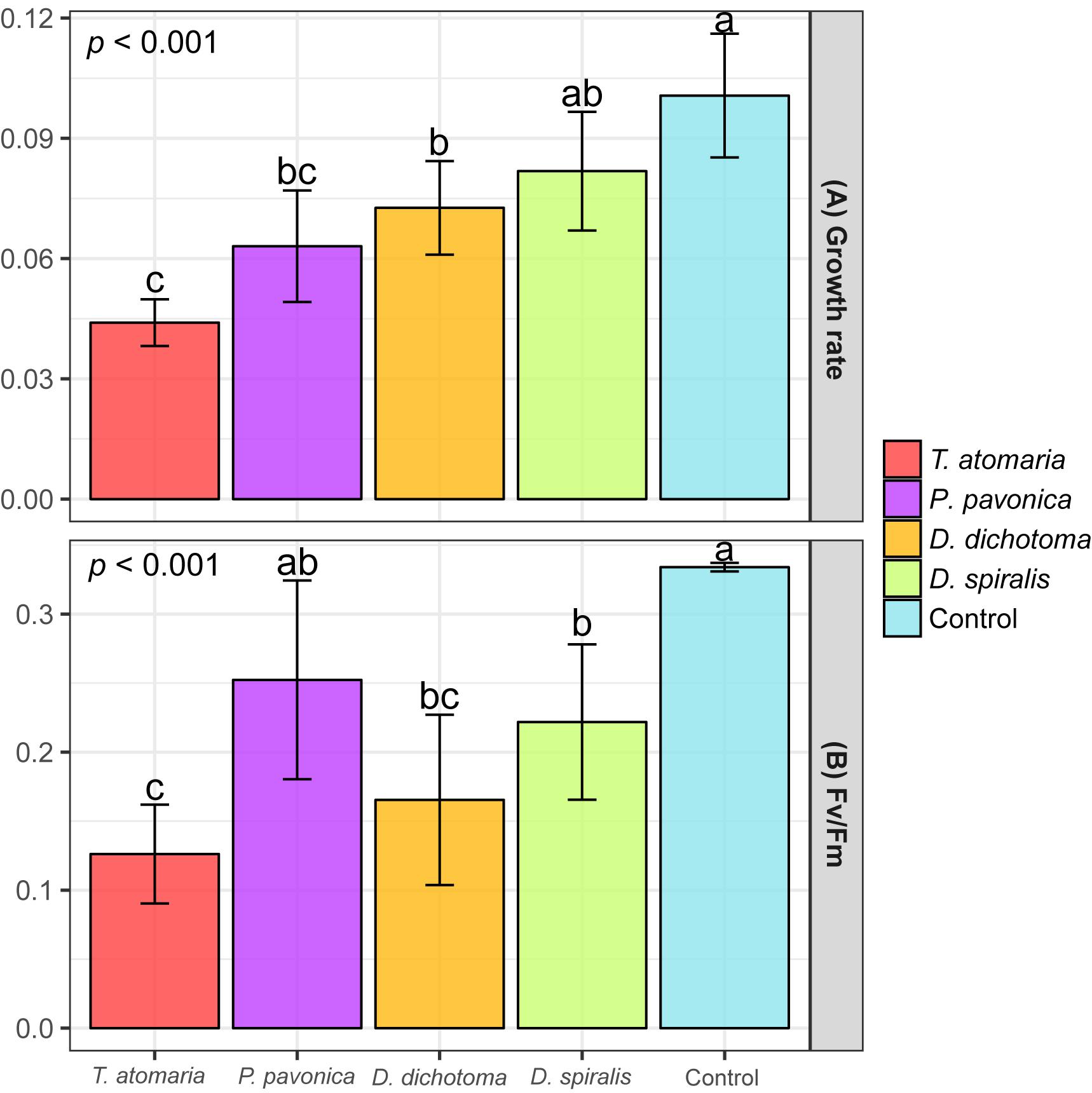
Figure 6. (A) Growth rate (μ) of O. cf. ovata and (B) Maximum quantum yield of PSII (Fv/Fm) of dark-adapted samples of O. cf. ovata after exposure of 24 h to macroalgal surface extracts (SEs) and blanks (n = 6 per condition). p-values and a, b, c indexes correspond to results of the one-way ANOVA and Tukey’s tests, respectively.
All but P. pavonica macroalgal SEs induced a significant stress on O. cf. ovata physiology as conveyed by the significant alteration of the efficiency of the PSII shown by the Fv/Fm ratio (p < 0.001; Figure 6B). Despite being tested at the lowest concentration, the SEs of D. dichotoma caused among the most intense inhibition of the PSII efficiency. Such an inhibition was comparable to those observed for D. spiralis and T. atomaria SEs. Whether the growth rate of O. cf. ovata was not affected by D. spiralis, the PSII efficiency was significantly altered to a similar extent than with D. dichotoma. Mirroring the effects on O. cf. ovata growth rate, T. atomaria SEs tested at 2.5 mg cm–2 also caused an intense stress on the PSII of the dinoflagellate. The related reduction in the Fv/Fm ratio measured was significantly higher than for D. spiralis and P. pavonica, although tested at a similar and lower concentration, respectively.
Discussion
The simultaneous monitoring of both the abundance and toxin content of benthic O. cf. ovata cells undertaken by Gémin et al. (2020) at the same sampling site in the bay of Villefranche showed that the development of this bloom followed a classic trend. In terms of intensity, the bloom peaked at 9.2 ± 7.5 105 cells g–1 FW (Gémin et al., 2020) corresponding to a moderate bloom for this site (Guidi-Guilvard et al., 2012; Cohu et al., 2013) or other Mediterranean sampling sites (Totti et al., 2010). Consistently with our results but also with other observations from the NW Mediterranean Sea (Cohu et al., 2013; Blanfuné et al., 2015), Gémin et al. (2020) found the highest cell concentration of O. cf. ovata on Dictyota spp. and the lowest on P. pavonica.
Potential Control of the Abundance of O. cf. ovata by the Epiphytic Community
A preferred colonization by O. cf. ovata on branched, three-dimensional thalli with a high surface/volume ratio has been previously suggested by several authors (Vila et al., 2001; Totti et al., 2010; Gémin et al., 2020) but such an hypothesis is not fully supported by the low abundance of O. cf. ovata measured on the highly branched T. atomaria or by settlement differences observed between morphologically similar Dictyota species (this study and Blanfuné et al., 2015). The 18S rRNA gene sequencing revealed a high diversity of eukaryotes colonizing the macroalgae, suggesting that various biotic interactions may take place within the epiphytic community having the potential to regulate the colonization of O. cf. ovata.
Inter-specific competition for nutrients, light and space may occur between the dinoflagellate O. cf. ovata and the various species of diatoms that co-occurred within the epiphytes of the macroalgae. Species of the genera Licmophora, Navicula, and Amphora were the most abundant diatoms co-occurring with O. cf. ovata, in line with previous findings (Accoroni et al., 2016), while Coscinodiscus spp. usually detected in the Catalan Sea (Vila et al., 2001; Carnicer et al., 2015) was missing. Other dinoflagellate species were barely detectable conversely to previous studies that reported the co-occurrence of P. lima and C. monotis in various sampling sites across the Mediterranean Sea, including the bay of Villefranche (Vila et al., 2001; Aligizaki and Nikolaidis, 2006; Cohu et al., 2011; Blanfuné et al., 2015; Accoroni et al., 2016). In recent co-culture experiments, weak deleterious allelopathic effects induced by Licmophora paradoxa toward O. cf. ovata were highlighted (Ternon et al., 2018). A chemical control of the colonization of O. cf. ovata by co-occurring benthic microalgae is therefore possible although it may be counterbalanced by the existence of an additional weak inhibiting allelopathic effects applied by O. cf. ovata on co-occurring microalgae (Monti and Cecchin, 2012; García-Portela et al., 2016).
Predation by benthic copepods is another factor that may modulate the colonization pattern of benthic microalgae. Benthic harpacticoids, such as Sphaeroma serratum, Tigriopus fulvus, Acartia clausi, and Sarsamphiascus cf. propinquus, have been recently shown to feed on O. cf. ovata (Prato et al., 2011; Faimali et al., 2012; Furlan et al., 2013; Pavaux et al., 2019) with similar ingestion rates as for diatoms (Boisnoir et al., 2020). The resistance of benthic copepods to O. cf. ovata’s toxicity was found to be highly variable among species, with LC50 ranging from 10 to 20,000 cells mL–1 (Prato et al., 2011; Faimali et al., 2012; Pavaux et al., 2019). Ingestion of O. cf. ovata leads to reduced fecal pellets production and fecundity rates indicating a reprotoxic effect (Pavaux et al., 2019) that may cause a reduction in nauplii abundance (Guidi-Guilvard et al., 2012) but not on adults’ abundance. Our study was conducted at the peak of the bloom, when the highest concentrations of O. cf. ovata and toxin content per cell were reported (Gémin et al., 2020). In situ cell concentrations reported in cells per g of macroalga fresh weight (9.2 ± 7.5 105 cells g–1 FW; Gémin et al., 2020) likely exceeded the toxic level of 20,000 cells per mL (Pavaux et al., 2019). Nevertheless, the amount of toxins per cell reported in situ was lower compared to the MCCV054 strain of O. cf. ovata used in Pavaux et al. study (5–10 against 13 pg cell–1; Gémin et al., 2020; Pavaux et al., 2020, respectively). Additionally, Gémin et al. (2020) showed that the toxin content in O. cf. ovata varied according to the macroalgal species they colonized. The toxicity of O. cf. ovata toward grazers may therefore vary according to the substrate. Therefore, it is difficult to conclude whether the maxillopods are suffering from the natural concentrations of O. cf. ovata encountered on the four macroalgae. Moreover, the resistance of the copepod species (P. fulvofasciata, A. atopus, A. coreana, Harpaticus spp., and Nemesis spp.) detected on the four macroalgae to O. cf. ovata has not been experimentally tested and may differ from already tested species. It is worth noting that the relative abundance of benthic copepods and O. cf. ovata did not show any obvious correlation. In contrast, if maxillopods have the capacity to feed on O. cf. ovata, they unlikely are the major factor that regulates its colonization on the macroalgae. Indeed, the toxic dinoflagellate was found to be similarly abundant on D. spiralis, T. atomaria, and P. pavonica (1,505; 2,251, and 1,759 cells cm–2, respectively), not mirroring their different ratio Ostreopsis:maxillopods (1:67, 1:33, and 1:17, respectively) in term of comparison of 18S rRNA gene sequences.
Other predators, like polychaetes, were also shown to graze O. cf. ovata despite an important sensitivity to the ovatoxins (Simonini et al., 2011). Concentrations of O. cf. ovata as low as 200 cells mL–1 caused the death of 100% of a population of Dinophylus gyrociliatus in less than 48 h (Simonini et al., 2011). However, similarly to copepods, the capacity to feed on O. cf. ovata as well as the resistance to the ovatoxins may differ among species and according to the toxicity of O. cf. ovata cells linked with the substrate they colonize (Gémin et al., 2020). The species S. pigmentata, P. dumerilii, and Neodexiospira brasiliensis, for which no LC50 is available yet, were the most abundant while D. gyrociliatus was not detected on the macroalgae.
The eukaryotic richness of the epiphytic macroalgal communities was high, particularly on T. atomaria and P. pavonica, and interactions that may result from it have not been fully investigated yet. For instance, the bacterial community which was not described in this study may play an important role in the regulation of epiphytic communities (Tait et al., 2005; Wheeler et al., 2006; Saha and Weinberger, 2019), including O. cf. ovata. The present data set did not highlight a specific biotic interaction within the epiphytic community that may favor or inhibit the settlement of O. cf. ovata on the different macroalgal species.
Algal Surface Chemistry Influences the Growth of O. cf. ovata
Several studies have shown deleterious effects of macroalgal metabolites on microalgae using whole cells dried and grounded (Tang and Gobler, 2011; Accoroni et al., 2015) or organic whole-cell extracts (Hellio et al., 2002; Nagayama et al., 2003). To our knowledge, this study is the first attempt in evaluating the bioactivity of SEs on settlement and growth of a microalgal species. Previous study on T. atomaria demonstrated a satisfying extraction of surface metabolites without disturbance of the macroalgal membranes by the soaking protocol used in the present study (Othmani et al., 2016a). The differences observed in the chemistry of the SEs and TEs for all four macroalgae species further validated the soaking protocol as an efficient method to extract surface metabolites of the three other species P. pavonica, D. spiralis, and D. dichotoma. Most chemical markers characteristics of the SEs were found to be polar metabolites (RT < 1 min), including DMSP, whereas TEs were characterized by less polar compounds (RT > 8 min).
The chemical fingerprint of the four macroalgae species draws two distinct groups: the two Dictyota spp. clustering together and P. pavonica and T. atomaria gathering in the second group. In terms of eukaryotic β-diversity, the same two groups emerged, suggesting that the metabolites at the surface of the macroalgae are influencing the settlement of eukaryotes. Since both T. atomaria and P. pavonica showed a higher richness of their overall epiphytic eukaryotic community, their surface metabolites could be considered less bioactive toward these communities, although higher antibacterial activities have been previously observed for Taonia and Dictyota spp. among Phaeophyceae species (Salvador Soler et al., 2007). When looking specifically at O. cf. ovata, the surface chemistry of the four macroalgae has the potential to influence the settlement of the toxic dinoflagellate by altering both its physiology and its growth. Nevertheless, the use of different concentrations (1–3.5 mg cm–2) in the bioassay does not allow to order the bioactivity of the four macroalgae, however D. dichotoma clearly induced a strong inhibition of both the growth and the PSII efficiency of O. cf. ovata at ecological concentrations (1 mg cm–2) and can therefore be considered as the most bioactive species. Many diterpenes isolated from Dictyota spp. have been found to exhibit antifouling properties as for instance the algicidal dictyolactone toward the red-tide microalgae Heterosigma akashiwo (Kim et al., 2006), dictyol C that reduces the adhesion of Pseudoalteromonas spp. (Viano et al., 2009), as well as dictyol E, dictyol B acetate, pachydictyol A and dictyodial that all cause significant larval mortalities of invertebrates (Schmitt et al., 1998). Although none of the cited compounds were major chemical markers in any of the two Dictyota spp., the high diterpenes content of their SEs suggests that this family of metabolites could be responsible for the observed bioactivity of both species. Nevertheless, considering the higher stress induced by D. dichotoma compared to D. spiralis on O. cf. ovata, chemical markers more specific to D. dichotoma were sought. Two metabolites listed as VIPs n°5 and 12 were significantly more enriched in D. dichotoma SEs (Supplementary Figure S3) with the molecular formulae C25H39N2O5 and C40H61O2 corresponding to an unidentified compound (cluster J) and a carotenoid-like compound likely to belong to the pirardixanthin family (Tsushima et al., 2001), respectively. While the activity of these families of compounds has not been determined so far, some carotenoids like fucoxanthin are known to possess inhibiting properties against bacteria (Viano et al., 2009; Saha et al., 2011) which could also target benthic microalgae.
Taonia atomaria showed a higher bioactivity than D. spiralis and P. pavonica, tested at a similar and higher concentration, respectively. This bioactivity is, however, likely overestimated compared to an ecological situation (enrichment factor of 5). Both geranylgeranylglycerol and DMSP were the major biomarkers in T. atomaria SEs, in agreement with previous findings (Othmani et al., 2016a; Paix et al., 2019), and were therefore enriched in this species compared to the three others (Supplementary Figure S3). These two metabolites have shown activity against bacterial settlement (Saha et al., 2012; Othmani et al., 2016a,b) and may have the potential for inhibiting the growth of O. cf. ovata. However, DMSP is a metabolite largely biosynthesized by O. cf. ovata itself (personal data; Chen et al., 2020), leaving geranylgeranylglycerol as the most likely candidate. The present dataset did not allow to propose any metabolite for P. pavonica that could explain the unspecific bioactivity of its SEs. If all four macroalgae species have the ability to affect O. cf. ovata’s growth or physiology, bidirectional effects may also be considered since some macroalgae have been shown to rely on microbial metabolites for cell division and differentiation (Wichard and Beemelmanns, 2018). The bioactive metabolites produced by the benthic dinoflagellate (Pavaux et al., 2020) may be detrimental or beneficial to the macroalgal host.
The bioactivity of the SEs combined to the natural settlement of O. cf. ovata suggests that the colonization preferences of O. cf. ovata are not driven by the sole bioactivity of the surface chemistry of macroalgae. Indeed, the highest abundance of O. cf. ovata’s cells were found on D. dichotoma that simultaneously presented the highest bioactivity at ecological concentrations (1 mg cm–2). The toxicity of D. dichotoma surface chemistry is likely faded by other unknown factors that may include reduced grazing and interspecific competition as shown by the lower ratio dinoflagellates:maxillopods and dinoflagellates:diatoms observed at the surface of D. dichotoma compared to other macroalgae. Diterpenes of Dictyota spp. have been shown to have deterrent effects on a wide range of herbivores (Hay and Steinberg, 1992; Pereira et al., 2000) and could also target grazers like copepods since they have been shown to be sensitive to various allelochemicals (Ianora et al., 2004). The lower bioactivity of D. spiralis SEs compared to those of D. dichotoma (tested at 2 mg cm–2) could not explain the low abundance of O. cf. ovata on its surface, suggesting the existence of other inhibiting factors controlling the colonization of the dinoflagellate on this macroalga as for instance the large relative abundance of copepods compared to that found at the surface of D. dichotoma.
The outcome of this experiment considered neither a spatial nor temporal variability, and rather describes an interaction between macroalgae and O. cf. ovata at high temperature (∼24°C) and salinity (∼39) conditions commonly found in the NW Mediterranean coastal waters in summer (Vila et al., 2001). If O. cf. ovata mostly blooms in summer across the Mediterranean Sea (Ciminiello et al., 2006; Cohu et al., 2013), late blooms are also reported in several areas (Aligizaki and Nikolaidis, 2006; Accoroni et al., 2012). The macroalgae colonized by O. cf. ovata were shown to differ throughout the year (Aligizaki and Nikolaidis, 2006; Battocchi et al., 2010; Hosny and Labib, 2019) likely in link to the macroalgal seasonality that modifies both their distribution and chemotypes (Paix et al., 2019) as well as their related bioactivity (Salvador Soler et al., 2007). In addition, the growth of O. cf. ovata was shown to be salinity sensitive (Pistocchi et al., 2011) and is optimal at salinity 36. Salinity also determines the distribution of seaweeds species (Martins et al., 1999) by altering various physiological aspects, including the production of metabolites (Polo et al., 2015). Therefore, at lower salinities areas (e.g., Northern Adriatic) a modified macroalgal bioactivity may lead to a different colonization pattern by O. cf. ovata (Accoroni et al., 2016).
Conclusion
The present dataset confirms that some Phaeophyceae of the family Dictyotaceae like T. atomaria, Dictyota spp. or P. pavonica may be characterized by a lower abundance of O. cf. ovata cells. Whether the surface chemistry of the macroalgae have the potential to handicap the growth of O. cf. ovata, it is not enough to explain the settlement preference on D. dichotoma that was found to be the most bioactive species. Complex interlacing factors involving several members of the epiphytic community are likely to modulate the growth of the toxic benthic dinoflagellate O. cf. ovata on macroalgae.
Data Availability Statement
The datasets presented in this study can be found in online repositories. The names of the repository/repositories and accession number(s) can be found below: NCBI Sequence Read Archive (SRA) (accession: PRJNA612893).
Author Contributions
ET, BP, J-FB, and GC designed the experiments. ET and BP conducted the experiments and treated the data. All the authors participated in the analysis of the data, contributed to the manuscript and approved the submitted version.
Funding
This work benefited from the support of the project OCEAN-15 (ANR-15-CE35-0002-01) of the French National Research Agency (ANR) and from the project CMAPO (2017) of the French GdR Mediatec. This work was also funded by the French “Sud Provence-Alpes-Côte d’Azur (Sud PACA)” regional council (Ph.D. grant of BP). The open access publication was supported by the MSCA project CHEMICROS (H2020-MSCA-IF-841051).
Conflict of Interest
The authors declare that the research was conducted in the absence of any commercial or financial relationships that could be construed as a potential conflict of interest.
Acknowledgments
The authors are grateful to Anaïs Lebrun for her help with bioassays and to Dr. Stéphane Greff (Aix-Marseille University, IMBE) for his help during the acquisition of LC-MS profiles. LC-MS experiments were conducted on the regional platform MALLABAR funded by the Institute of Ecology and Environment (INEE) of the French National Centre for Scientific Research (CNRS) and the French Sud PACA regional council.
Supplementary Material
The Supplementary Material for this article can be found online at: https://www.frontiersin.org/articles/10.3389/fmars.2020.00683/full#supplementary-material
Footnotes
References
Accoroni, S., Percopo, I., Cerino, F., Romagnoli, T., Pichierri, S., Perrone, C., et al. (2015). Allelopathic interactions between the HAB dinoflagellate Ostreopsis cf. ovata and macroalgae. Harmful Algae 49, 147–155. doi: 10.1016/j.hal.2015.08.007
Accoroni, S., Romagnoli, T., Pichierri, S., Colombo, F., and Totti, C. (2012). Morphometric analysis of Ostreopsis cf. ovata cells in relation to environmental conditions and bloom phases. Harmful Algae 19, 15–22. doi: 10.1016/j.hal.2012.05.003
Accoroni, S., Romagnoli, T., Pichierri, S., and Totti, C. (2016). Effects of the bloom of harmful benthic dinoflagellate Ostreopsis cf. ovata on the microphytobenthos community in the northern Adriatic Sea. Harmful Algae 55, 179–190. doi: 10.1016/j.hal.2016.03.003
Aligizaki, K., and Nikolaidis, G. (2006). The presence of the potentially toxic genera Ostreopsis and Coolia (Dinophyceae) in the North Aegean Sea. Greece. Harmful Algae 5, 717–730. doi: 10.1016/j.hal.2006.02.005
Battocchi, C., Totti, C., Vila, M., Masó, M., Capellacci, S., Accoroni, S., et al. (2010). Monitoring toxic microalgae Ostreopsis (dinoflagellate) species in coastal waters of the Mediterranean Sea using molecular PCR-based assay combined with light microscopy. Mar. Pollut. Bull. 60, 1074–1084. doi: 10.1016/j.marpolbul.2010.01.017
Benita, M., Dubinsky, Z., and Iluz, D. (2018). Padina pavonica: morphology and calcification functions and mechanism. Am. J. Plant Sci. 9, 1156–1168.
Bertalot, H. L., Witowski, A., and Metzeltin, D. (2000). “Diatom flora of marine coasts, Vol. 1 iconographia diatomologica: annotated diatom micrographs vol 7 Diversity - Taxonomy - Identification,” in Iconographia Diatomologica, Ed. H. Lange (Grafenau: Koeltz).
Bianco, ÉM., Rogers, R., Teixeira, V. L., and Pereira, R. C. (2009). Antifoulant diterpenes produced by the brown seaweed Canistrocarpus cervicornis. J. Appl. Phycol. 21, 341–346. doi: 10.1007/s10811-008-9374-9
Blanfuné, A., Boudouresque, C. F., Grossel, H., and Thibaut, T. (2015). Distribution and abundance of Ostreopsis spp. and associated species (Dinophyceae) in the northwestern Mediterranean: the region and the macroalgal substrate matter. Environ. Sci. Pollut. Res. 22, 12332–12346. doi: 10.1007/s11356-015-4525-4
Boisnoir, A., Pavaux, A.-S., Schizas, N. V., Marro, S., Blasco, T., Lemée, R., et al. (2020). The use of stable isotopes to measure the ingestion rate of potentially toxic benthic dinoflagellates by harpacticoid copepods. J. Exp. Mar. Biol. Ecol. 524, 151285. doi: 10.1016/j.jembe.2019.151285
Carnicer, O., Guallar, C., Andree, K. B., Diogène, J., and Fernández-Tejedor, M. (2015). Ostreopsis cf. ovata dynamics in the NW Mediterranean Sea in relation to biotic and abiotic factors. Non-Regul. Environ. Contam. Seaf. Contrib. ECsafeSEAFOOD EU Proj. 143, 89–99. doi: 10.1016/j.envres.2015.08.023
Casabianca, S., Casabianca, A., Riobó, P., Franco, J. M., Vila, M., and Penna, A. (2013). Quantification of the Toxic Dinoflagellate Ostreopsis spp. by qPCR Assay in Marine Aerosol. Environ. Sci. Technol. 47, 3788–3795. doi: 10.1021/es305018s
Chen, L., Kieber, D. J., Amato, F., Vila, M., Viure, L., Alastuey, A., et al. (2020). Dimethylsulfoniopropionate Concentrations in Mediterranean Coastal Water and Ambient Aerosols During Ostreopsis cf. ovata Blooms. San Diego: Ocean Sciences-Meeting.
Ciminiello, P., Dell’Aversano, C., Fattorusso, E., Forino, M., Magno, G. S., Tartaglione, L., et al. (2006). The Genoa 2005 outbreak. Determination of putative palytoxin in Mediterranean Ostreopsis ovata by a new liquid chromatography tandem mass spectrometry method. Anal. Chem. 78, 6153–6159.
Cohu, S., Mangialajo, L., Thibaut, T., Blanfuné, A., Marro, S., and Lemée, R. (2013). Proliferation of the toxic dinoflagellate Ostreopsis cf. ovata in relation to depth, biotic substrate and environmental factors in the North West Mediterranean Sea. Harmful Algae 24, 32–44. doi: 10.1016/j.hal.2013.01.002
Cohu, S., Thibaut, T., Mangialajo, L., Labat, J. P., Passafiume, O., Blanfune, A., et al. (2011). Occurrence of the toxic dinoflagellate Ostreopsis cf. ovata in relation with environmental factors in Monaco (NW Mediterranean). Mar. Pollut. Bull. 62, 2681–2691. doi: 10.1016/j.marpolbul.2011.09.022
Debroas, D., Mone, A., and Ter Halle, A. (2017). Plastics in the North Atlantic garbage patch: a boat-microbe for hitchhikers and plastic degraders. Sci. Total Environ. 599–600, 1222–1232. doi: 10.1016/j.scitotenv.2017.05.059
Egan, S., Harder, T., Burke, C., Steinberg, P., Kjelleberg, S., and Thomas, T. (2013). The seaweed holobiont: understanding seaweed–bacteria interactions. FEMS Microbiol. Rev. 37, 462–476. doi: 10.1111/1574-6976.12011
Escudié, F., Auer, L., Bernard, M., Mariadassou, M., Cauquil, L., Vidal, K., et al. (2017). FROGS: find. Rapidly, OTUs with Galaxy Solution. Bioinformatics 34, 1287–1294. doi: 10.1093/bioinformatics/btx791
Faimali, M., Giussani, V., Piazza, V., Garaventa, F., Corrà, C., Asnaghi, V., et al. (2012). Toxic effects of harmful benthic dinoflagellate Ostreopsis ovata on invertebrate and vertebrate marine organisms. Mar. Environ. Res. 76, 97–107. doi: 10.1016/j.marenvres.2011.09.010
Furlan, M., Antonioli, M., Zingone, A., Sardo, A., Blason, C., Pallavicini, A., et al. (2013). Molecular identification of Ostreopsis cf. ovata in filter feeders and putative predators. Harmful Algae 21–22, 20–29. doi: 10.1016/j.hal.2012.11.004
García-Portela, M., Riobó, P., Franco, J. M., Bañuelos, R. M., and Rodríguez, F. (2016). Genetic and toxinological characterization of North Atlantic strains of the dinoflagellate Ostreopsis and allelopathic interactions with toxic and non-toxic species from the genera Prorocentrum, Coolia and Gambierdiscus. Harmful Algae 60, 57–69. doi: 10.1016/j.hal.2016.10.007
Gémin, M., Réveillon, D., Hervé, F., Pavaux, A., Tharaud, M., Séchet, V., et al. (2020). Toxin content of Ostreopsis cf. ovata depends on bloom phases, depth and macroalgal substrate in the NW Mediterranean Sea. Harmful Algae 92, 101727. doi: 10.1016/j.hal.2019.101727
Giussani, V., Costa, E., Pecorino, D., Berdalet, E., De Giampaulis, G., Gentile, M., et al. (2016). Effects of the harmful dinoflagellate Ostreopsis cf. ovata on different life cycle stages of the common moon jellyfish Aurelia sp. Harmful Algae 57, 49–58. doi: 10.1016/j.hal.2016.05.005
Goecke, F., Labes, A., Wiese, J., and Imhoff, J. (2010). Chemical interactions between marine macroalgae and bacteria. Mar. Ecol. Prog. Ser. 409, 267–299.
Gorbi, S., Avio, G. C., Benedetti, M., Totti, C., Accoroni, S., Pichierri, S., et al. (2013). Effects of harmful dinoflagellate Ostreopsis cf. ovata exposure on immunological, histological and oxidative responses of mussels Mytilus galloprovincialis. Fish Shellfish Immunol. 35, 941–950. doi: 10.1016/j.fsi.2013.07.003
Guidi, F., Pezzolesi, L., and Vanucci, S. (2018). Microbial dynamics during harmful dinoflagellate Ostreopsis cf. ovata growth: bacterial succession and viral abundance pattern. MicrobiologyOpen 7:e00584. doi: 10.1002/mbo3.584
Guidi-Guilvard, L. D., Gasparini, S., and Lemée, R. (2012). The Negative Impact of Ostreopsis cf. Ovata on Phytal Meiofauna from the Coastal NW Mediterranean. Cryptogam. Algol. 33, 121–128. doi: 10.7872/crya.v33.iss2.2011.121
Guillard, R. R. L., and Ryther, J. H. (1962). Studies of marine planktonic diatoms; I. Cyclotella nana Hustedt, and Detonula Confervacea (Cleve) Gran. Can. J. Microbiol. 8, 229–239. doi: 10.1139/m62-029
Hay, M., and Steinberg, P. (1992). “The chemical ecology of plant-herbivore interactions in marine versus terrestrial communities,” in Herbivores: Their Interaction with Secondary Plant Metabolites., Evolutionary and Ecological Processes, eds G. A. Rosenthal and M. R. Berenbaum (New York, NY: Elsevier Science), 371–413.
Hellio, C., Berge, J. P., Beaupoil, C., Le Gal, Y., and Bourgougnon, N. (2002). Screening of Marine Algal Extracts for Anti-settlement Activities against Microalgae and Macroalgae. Biofouling 18, 205–215. doi: 10.1080/08927010290010137
Hollants, J., Leliaert, F., De Clerck, O., and Willems, A. (2013). What we can learn from sushi: a review on seaweed–bacterial associations. FEMS Microbiol. Ecol. 83, 1–16. doi: 10.1111/j.1574-6941.2012.01446.x
Horiguchi, E. (ed.) (2014). Marine Benthic Dinoflagellates - Unveiling Their Worldwide Biodiversity. Stuttgart: Schweizerbart Science Publishers.
Hosny, S., and Labib, W. (2019). Ecology of the Epiphytic Potentially Harmful Dinoflagellate Ostreopsis cf. ovata (Fukuyo) from Coastal Waters off Alexandria Egypt. J. Ocean Mar. Res. 7:2.
Ianora, A., Miralto, A., Poulet, S. A., Carotenuto, Y., Buttino, I., Romano, G., et al. (2004). Aldehyde suppression of copepod recruitment in blooms of a ubiquitous planktonic diatom. Nature 429, 403–407. doi: 10.1038/nature02526
Jauzein, C., Açaf, L., Accoroni, S., Asnaghi, V., Fricke, A., Hachani, M. A., et al. (2018). Optimization of sampling, cell collection and counting for the monitoring of benthic harmful algal blooms: application to Ostreopsis spp. blooms in the Mediterranean Sea. Ecol. Indic. 91, 116–127. doi: 10.1016/j.ecolind.2018.03.089
Kim, J. Y., Alamsjah, M. A., Hamada, A., Fujita, Y., and Ishibashi, F. (2006). Algicidal Diterpenes from the Brown Alga Dictyota dichotoma. Biosci. Biotechnol. Biochem. 70, 2571–2574. doi: 10.1271/bbb.60281
Mahé, F., Rognes, T., Quince, C., de Vargas, C., and Dunthorn, M. (2014). Swarm: robust and fast clustering method for amplicon-based studies. PeerJ 2:e593.
Mangialajo, L., Ganzin, N., Accoroni, S., Asnaghi, V., Blanfune, A., Cabrini, M., et al. (2011). Trends in Ostreopsis proliferation along the Northern Mediterranean coasts. Toxicon Off. J. Int. Soc. Toxinology 57, 408–420. doi: 10.1016/j.toxicon.2010.11.019
Marro, S., Pavaux, A.-S., Drouet, K., and Lemée, R. (2019). “Diversity of benthic microphytoplankton associated to Ostreopsis cf. ovata bloom in the NW Mediterranean Sea,” in Poster at French National Conference on HAB, (Brest: PHYCOTOX Group), 1.
Martins, I., Oliveira, J. M., Flindt, M. R., and Marques, J. C. (1999). The effect of salinity on the growth rate of the macroalgae Enteromorpha intestinalis (Chlorophyta) in the Mondego estuary (west Portugal). Acta Oecol. 20, 259–265. doi: 10.1016/S1146-609X(99)00140-X
McMurdie, P. J., and Holmes, S. (2013). phyloseq: an R Package for Reproducible Interactive Analysis and Graphics of Microbiome Census Data. PLoS One 8:e61217. doi: 10.1371/journal.pone.0061217
Meroni, L., Chiantore, M., Petrillo, M., and Asnaghi, V. (2018). Habitat effects on Ostreopsis cf. ovata bloom dynamics. Harmful Algae 80, 64–71. doi: 10.1016/j.hal.2018.09.006
Monti, M., and Cecchin, E. (2012). Comparative growth of three strains of Ostreopsis ovata at different light intensities with focus on inter-specific allelopathic interactions. Cryptogam. Algol. 33, 113–119. doi: 10.7872/crya.v33.iss2.2011.113
Nagayama, K., Shibata, T., Fujimoto, K., Honjo, T., and Nakamura, T. (2003). Algicidal effect of phlorotannins from the brown alga Ecklonia kurome on red tide microalgae. Aquaculture 218, 601–611. doi: 10.1016/S0044-8486(02)00255-7
Oksanen, J., Blanchet, F. G., Kindt, R., Legendre, P., Minchin, P. R., O’hara, R., et al. (2013). Package ‘vegan.’. Commun. Ecol. Package Version 2, 1–295.
Othmani, A., Briand, J.-F., Ayé, M., Molmeret, M., and Culioli, G. (2016a). Surface metabolites of the brown alga Taonia atomaria have the ability to regulate epibiosis. Biofouling 32, 801–813. doi: 10.1080/08927014.2016.1198954
Othmani, A., Bunet, R., Bonnefont, J.-L., Briand, J.-F., and Culioli, G. (2016b). Settlement inhibition of marine biofilm bacteria and barnacle larvae by compounds isolated from the Mediterranean brown alga Taonia atomaria. J. Appl. Phycol. 28, 1975–1986. doi: 10.1007/s10811-015-0668-4
Paix, B., Carriot, N., Barry-Martinet, R., Greff, S., Misson, B., Briand, J.-F., et al. (2020). A Multi-Omics analysis suggests links between the differentiated surface metabolome and epiphytic microbiota along the thallus of a mediterranean seaweed holobiont. Front. Microbiol. 11:494. doi: 10.3389/fmicb.2020.00494
Paix, B., Othmani, A., Debroas, D., Culioli, G., and Briand, J.-F. (2019). Temporal covariation of epibacterial community and surface metabolome in the Mediterranean seaweed holobiont Taonia atomaria. Environ. Microbiol. 21, 3346–3363. doi: 10.1111/1462-2920.14617
Pavaux, A.-S., Rostan, J., Guidi-Guilvard, L., Marro, S., Ternon, E., Thomas, O. P., et al. (2019). Effects of the toxic dinoflagellate Ostreopsis cf. ovata on survival, feeding and reproduction of a phytal harpacticoid copepod. J. Exp. Mar. Biol. Ecol. 516, 103–113. doi: 10.1016/j.jembe.2019.05.004
Pavaux, A.-S., Ternon, E., Dufour, L., Marro, S., Gémin, M.-P., Thomas, O. P., et al. (2020). Efficient, fast and inexpensive bioassay to monitor benthic microalgae toxicity: application to Ostreopsis species. Aquat. Toxicol. 223:105485. doi: 10.1016/j.aquatox.2020.105485
Pereira, R. C., Cavalcanti, D. N., and Teixeira, V. L. (2000). Effects of secondary metabolites from the tropical Brazilian brown alga Dictyota menstrualis on the amphipod Parhyale hawaiensis. Mar. Ecol. Prog. Ser. 205, 95–100.
Pezzolesi, L., Guerrini, F., Ciminiello, P., Dell’Aversano, C., Dello Iacovo, E., Fattorusso, E., et al. (2012). Influence of temperature and salinity on Ostreopsis cf. ovata growth and evaluation of toxin content through HR LC-MS and biological assays. Water Res. 46, 82–92. doi: 10.1016/j.watres.2011.10.029
Pistocchi, R., Pezzolesi, L., Guerrini, F., Vanucci, S., Dell’aversano, C., and Fattorusso, E. (2011). A review on the effects of environmental conditions on growth and toxin production of Ostreopsis ovata. Toxicon Off. J. Int. Soc. Toxinol. 57, 421–428. doi: 10.1016/j.toxicon.2010.09.013
Polo, L. K., Felix, M. R. L., Kreusch, M., Pereira, D. T., Costa, G. B., Simioni, C., et al. (2015). Metabolic profile of the brown macroalga Sargassum cymosum (Phaeophyceae, Fucales) under laboratory UV radiation and salinity conditions. J. Appl. Phycol. 27, 887–899. doi: 10.1007/s10811-014-0381-8
Poulson-Ellestad, K. L., Jones, A., Roy, J., Viant, M. R., Fernandez, F. M., Kubanek, J., et al. (2014). Metabolomics and proteomics reveal impacts of chemically mediated competition on marine phytoplankton. PNAS 111, 9009–9014. doi: 10.1073/pnas.1413432111
Prato, E., Biandolino, F., Bisci, A. P., and Caroppo, C. (2011). Preliminary assessment of Ostreopsis cfr. ovata acute toxicity by using a battery bioassay. Chem. Ecol. 27, 117–125. doi: 10.1080/02757540.2011.625930
Puglisi, M. P., Sneed, J. M., Sharp, K. H., Ritson-Williams, R., and Paul, V. J. (2014). Marine chemical ecology in benthic environments. Nat. Prod. Rep. 31, 1510–1553. doi: 10.1039/C4NP00017J
Rognes, T., Flouri, T., Nichols, B., Quince, C., and Mahé, F. (2016). VSEARCH: a versatile open source tool for metagenomics. PeerJ 4:e2584.
Saha, M., Rempt, M., Gebser, B., Grueneberg, J., Pohnert, G., and Weinberger, F. (2012). Dimethylsulphopropionate (DMSP) and proline from the surface of the brown alga Fucus vesiculosus inhibit bacterial attachment. Biofouling 28, 593–604. doi: 10.1080/08927014.2012.698615
Saha, M., Rempt, M., Grosser, K., Pohnert, G., and Weinberger, F. (2011). Surface-associated fucoxanthin mediates settlement of bacterial epiphytes on the rockweed Fucus vesiculosus. Biofouling 27, 423–433. doi: 10.1080/08927014.2011.580841
Saha, M., and Weinberger, F. (2019). Microbial “gardening” by a seaweed holobiont: Surface metabolites attract protective and deter pathogenic epibacterial settlement. J. Ecol. 107, 2255–2265. doi: 10.1111/1365-2745.13193
Salvador Soler, N., Gomez Garreta, M. A., Lavelli, L., and Ribera Siguan, M.-A. (2007). Antimicrobial Activity of Iberian macroalgae. Sci. Mar. 71, 101–113.
Schmitt, T. M., Lindquist, N., and Hay, M. E. (1998). Seaweed secondary metabolites as antifoulants: effects of Dictyota spp. diterpenes on survivorship, settlement, and development of marine invertebrate larvae. Chemoecology 8, 125–131. doi: 10.1007/s000490050017
Selander, E., Kubanek, J., Hamberg, M., Andersson, M. X., Cervin, G., and Pavia, H. (2015). Predator lipids induce paralytic shellfish toxins in bloom-forming algae. Proc. Natl. Acad. Sci. U.S.A. 112, 6395–6400. doi: 10.1073/pnas.1420154112
Simonini, R., Orlandi, M., and Abbate, M. (2011). Is the toxic dinoflagellate Ostreopsis cf. ovata harmful to Mediterranean benthic invertebrates? Evidences from ecotoxicological tests with the polychaete Dinophilus gyrociliatus. Mar. Environ. Res. 72, 230–233. doi: 10.1016/j.marenvres.2011.08.009
Smith, C. A., Want, E. J., O’Maille, G., Abagyan, R., and Siuzdak, G. (2006). XCMS: Processing Mass Spectrometry Data for Metabolite Profiling Using Nonlinear Peak Alignment. Matching, and Identification. Anal. Chem. 78, 779–787. doi: 10.1021/ac051437y
Tait, K., Joint, I., Daykin, M., Milton, D. L., Williams, P., and Cámara, M. (2005). Disruption of quorum sensing in seawater abolishes attraction of zoospores of the green alga Ulva to bacterial biofilms. Environ. Microbiol. 7, 229–240. doi: 10.1111/j.1462-2920.2004.00706.x
Tang, Y. Z., and Gobler, C. J. (2011). The green macroalga, Ulva lactuca, inhibits the growth of seven common harmful algal bloom species via allelopathy. Harmful Algae 10, 480–488. doi: 10.1016/j.hal.2011.03.003
Ternon, E., Pavaux, A.-S., Marro, S., Thomas, O. P., and Lemée, R. (2018). Allelopathic interactions between the benthic toxic dinoflagellate Ostreopsis cf. ovata and a co-occurring diatom. Harmful Algae 75, 35–44. doi: 10.1016/j.hal.2018.04.003
Tillmann, U., and Hansen, P. (2009). Allelopathic effects of Alexandrium tamarense on other algae: evidence from mixed growth experiments. Aquat. Microb. Ecol. 57, 101–112.
Totti, C., Accoroni, S., Cerino, F., Cucchiari, E., and Romagnoli, T. (2010). Ostreopsis ovata bloom along the Conero Riviera (northern Adriatic Sea): relationships with environmental conditions and substrata. Harmful Algae 9, 233–239. doi: 10.1016/j.hal.2009.10.006
Tsushima, M., Maoka, T., and Matsuno, T. (2001). Structures of Carotenoids with 5,6-Dihydro-β-End Groups from the Spindle Shell Fusinus perplexus. J. Nat. Prod. 64, 1139–1142. doi: 10.1021/np010060y
Vanucci, S., Guidi, F., Pistocchi, R., and Long, R. A. (2016). Phylogenetic structure of bacterial assemblages co-occurring with Ostreopsis cf. ovata bloom. Harmful Algae 55, 259–271. doi: 10.1016/j.hal.2016.04.003
Viano, Y., Bonhomme, D., Camps, M., Briand, J.-F., Ortalo-Magné, A., Blache, Y., et al. (2009). Diterpenoids from the Mediterranean Brown Alga Dictyota sp. Evaluated as Antifouling Substances against a Marine Bacterial Biofilm. J. Nat. Prod. 72, 1299–1304. doi: 10.1021/np900102f
Vila, M., Garcés, E., and Maso, M. (2001). Potentially toxic epiphytic dinoflagellate assemblages on macroalgae in the NW Mediterranean. Aquat. Microb. Ecol. 26, 51–60.
Wheeler, G. L., Tait, K., Taylor, A., Brownlee, C., and Joint, I. (2006). Acyl-homoserine lactones modulate the settlement rate of zoospores of the marine alga Ulva intestinalis via a novel chemokinetic mechanism. Plant Cell Environ. 29, 608–618. doi: 10.1111/j.1365-3040.2005.01440.x
Keywords: Ostreopsis cf. ovata, Dictyotaceae, surface colonization, metabolomics, metabarcoding, eukaryotes, biofilms
Citation: Ternon E, Paix B, Thomas OP, Briand J-F and Culioli G (2020) Exploring the Role of Macroalgal Surface Metabolites on the Settlement of the Benthic Dinoflagellate Ostreopsis cf. ovata. Front. Mar. Sci. 7:683. doi: 10.3389/fmars.2020.00683
Received: 13 March 2020; Accepted: 28 July 2020;
Published: 21 August 2020.
Edited by:
Elisa Berdalet, Institute of Marine Sciences (CSIC), SpainReviewed by:
Stefano Accoroni, Marche Polytechnic University, ItalyOlga Carnicer, Dalhousie University, Canada
Leo Lai Chan, City University of Hong Kong, Hong Kong
Copyright © 2020 Ternon, Paix, Thomas, Briand and Culioli. This is an open-access article distributed under the terms of the Creative Commons Attribution License (CC BY). The use, distribution or reproduction in other forums is permitted, provided the original author(s) and the copyright owner(s) are credited and that the original publication in this journal is cited, in accordance with accepted academic practice. No use, distribution or reproduction is permitted which does not comply with these terms.
*Correspondence: Eva Ternon, ZXZhLnRlcm5vbkBpbWV2LW1lci5mcg==; Gérald Culioli, Y3VsaW9saUB1bml2LXRsbi5mcg==
†These authors have contributed equally to this work