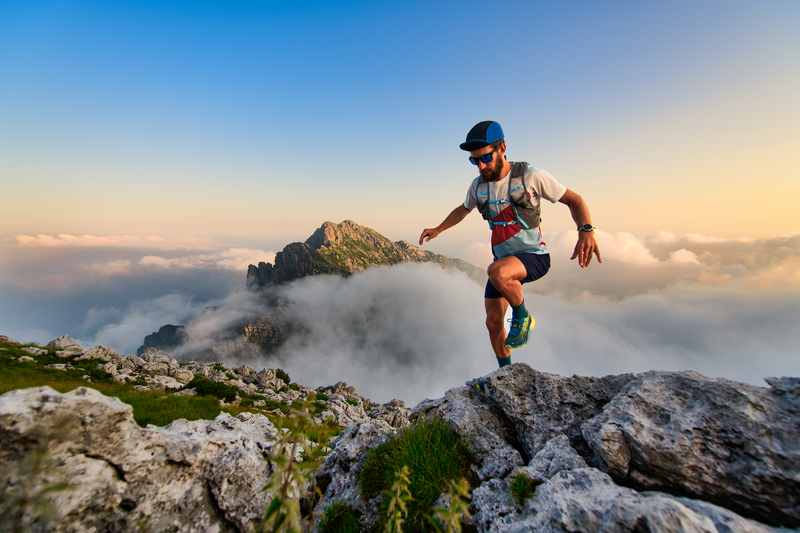
94% of researchers rate our articles as excellent or good
Learn more about the work of our research integrity team to safeguard the quality of each article we publish.
Find out more
REVIEW article
Front. Mar. Sci. , 14 August 2020
Sec. Global Change and the Future Ocean
Volume 7 - 2020 | https://doi.org/10.3389/fmars.2020.00671
This article is part of the Research Topic Marine Ecosystem Assessment for the Southern Ocean: Meeting the Challenge for Conserving Earth Ecosystems in the Long Term View all 25 articles
Our review of the literature has revealed Southern Ocean subsurface chlorophyll-a maxima (SCMs) to be an annually recurrent feature throughout the basin. Most of these SCMs are different to the “typical” SCMs observed in the tropics, which are maintained by the nutrient-light co-limitation of phytoplankton growth. Rather, we have found that SCMs are formed by other processes including diatom aggregation, sea-ice retreat, eddies, subduction events and photo-acclimation. At a local scale, these SCMs can facilitate increased downward carbon export, primary production and food availability for higher trophic levels. A large proportion of Southern Ocean SCMs appear to be sustained by aggregates of large diatoms that form under severe iron limitation in the seasonal mixed layer. The ability of large diatoms to regulate their buoyancy must play a role in the development of these SCMs as they appear to increase buoyancy at the SCM and thus avoid further sinking with the decline of the spring bloom or naturally iron fertilized blooms. These SCMs remain largely unobserved by satellites and it seems that ship-based sampling may not be able to fully capture their biomass. In the context of the Marine Ecosystem Assessment of the Southern Ocean it is important to consider that this phenomenon is missing in our current understanding of Southern Ocean ecology and future climate scenarios. The broader implications of SCMs for Southern Ocean ecology will only be revealed through basin-wide observations. This can only be achieved through an integrated observation system that is able to harness the detailed information encapsulated in ship-based sampling, with the increased observational capacity of fluorometers on autonomous platforms such as those in the biogeochemical Argo (BGC-Argo) and the Marine Mammals Exploring the Ocean Pole to pole (MEOP) programs. The main challenge toward achieving this is the uncertainties associated with translating fluorescence to chlorophyll-a concentrations. Until this translation is resolved, the reporting of subsurface fluorescence maxima (SFMs) in place of SCMs could still yield valuable insights with careful interpretation.
Southern Ocean phytoplankton are essential for Antarctic food-webs and the regulation of global climate through the marine carbon cycle (Deppeler and Davidson, 2017). Here, phytoplankton blooms are dominated by large silica-forming diatoms which have unique physiology that is adapted to the low iron, light and temperature conditions they live in (Cermeño and Falkowski, 2009; Soppa et al., 2014; Arteaga et al., 2018, 2019; Tréguer et al., 2018; Schallenberg et al., 2019; Strzepek et al., 2019). Residing in the mixed layer of the surface ocean, phytoplankton stocks can be observed from space using satellites due to the visibility of chlorophyll-a (McClain, 2009; Johnson et al., 2013). Satellite chlorophyll-a composites are routinely used in Southern Ocean ecosystem assessments of primary production, downward carbon export and food availability (Boyd et al., 2012; Siegel et al., 2014; Lee et al., 2015; Constable et al., 2016).
In the Southern Ocean, ship-based studies have presented evidence of subsurface chlorophyll-a maxima (SCMs), observing a build-up of chlorophyll-a below the penetration depth of satellites (10–40 m; Parslow et al., 2001; Holm-Hansen et al., 2005; Carranza et al., 2018). This phenomenon can be sustained by several ecological processes, although most of what is currently known comes from observing the tropical oceans (Cullen, 1982, 2015). In the tropics high irradiances, warm temperatures and low supply of macro-nutrients lead to the dominance of smaller picoplankton communities (Acevedo-Trejos et al., 2013, 2015). Most commonly tropical SCMs are associated with a typical stable water structure which persists through both summer and winter (Cullen, 1982, 2015). This SCM forms just above the pycnocline and is directly related to an increase in phytoplankton abundance (and biomass) that is stimulated by changes in light and macro-nutrients with depth (Figure 1). This mechanism of SCM formation – as a niche specialization in a stratum that receives just enough light from above and nutrient supply from below – is well understood and widely studied in the tropics and the Mediterranean Sea (Cullen, 1982; Furuya, 1990; Estrada et al., 2016; Barbieux et al., 2019).
Figure 1. A comparison of typical upper water column structure between the macronutrient limited tropical ocean and the iron limited Southern Ocean over summer and winter. Distributions of downward irradiance (yellow), macronutrients (red) and iron (brown) are shown on a low (L) to high (H) scale. Also shown are indications of mixed layer depths (dashed gray line) – note that mixed layer depths are highly variable and are dependent on the thresholds used to define them. Phytoplankton distributions (green) are shown for the picophytoplankton (circular) community of the tropical ocean and the high silicate community (diamond) of the polar Southern Ocean. The optimum depth of phytoplankton growth (solid black line) is determined by the distribution of light and the limiting nutrient according to the theory of nutrient light co-limitation. Supplementary Table S1 lists supporting information used in the construction of this graphical summary.
Southern Ocean SCMs are often located at or deeper than the pycnocline, rather than above it, making them distinguishable from those of the tropics (Quilty et al., 1985; Bathmann et al., 1997; Fiala et al., 1998; Kopczynska et al., 2001; Parslow et al., 2001; Garibotti et al., 2003; Armand et al., 2008a; Gomi et al., 2010; Westwood et al., 2011; Demidov et al., 2013; Tripathy et al., 2015). The deeper SCM coupled with the macro-nutrient replete surface ocean, suggest that Southern Ocean SCMs are different to that of the tropics (Figure 1; Louanchi and Najjar, 2000; Moore et al., 2001; Arteaga et al., 2019). These deeper SCMs are characterized by a marked community shift toward large diatoms across the pycnocline and are referred to as “diatom SCMs” herein (Bathmann et al., 1997; Cailliau et al., 1997; Kopczynska et al., 2001; Parslow et al., 2001; Quéguiner, 2001; Armand et al., 2008a; Gomi et al., 2010; Westwood et al., 2011; Tripathy et al., 2015). Similar diatom SCMs have also been observed in other stratified regions including northern-temperate and coastal areas (Estrada et al., 1993; Kemp and Villareal, 2013, 2018). The study of how SCMs form in the Southern Ocean will help to reveal the distribution of deep phytoplankton communities which are currently hidden from satellites.
We provide a comprehensive Review of what is currently known about Southern Ocean SCMs (those that form south of the circumpolar Sub-tropical front). This includes (1) methods for their observation (2) their reported occurrences (3) the ecological processes responsible for their formation and (4) the role of SCMs in Southern Ocean ecology. In doing so, we highlight how ship-based data have been able to capture annually recurrent and widespread Southern Ocean SCMs and the ocean properties that together distinguish them from the SCMs observed in the tropics. The ecological implications of this deep phytoplankton biomass, which are not captured in satellite chlorophyll-a composites, are further discussed to inform the Marine Ecosystem Assessment of the Southern Ocean (MEASO).
This Review is timely in the wake of the increased sampling of chlorophyll-a by autonomous platforms such as those in the biogeochemical Argo (BGC-Argo) and the Marine Mammals Exploring the Ocean Pole to pole (MEOP) programs in the Southern Ocean. These programs have the capacity to fill the limited spatiotemporal resolutions of ship-based sampling (Figure 2; Guinet et al., 2013; Claustre et al., 2020). From this new wealth of data, the large-scale processes that control the formation of Southern Ocean SCMs can be delineated, complementing the detailed understanding of the phenomenon presented in this Review.
Figure 2. The distribution of available fluorescence-derived chlorophyll-a data collected (blue dots) south of 30°S overlaid on bathymetry, alongside images of autonomous platforms used in two programs that facilitate large deployments of fluorometers in the Southern Ocean; (A) the biogeochemical Argo (BGC-Argo) and (B) the Marine mammals Exploring the Oceans Pole to pole (MEOP) programs. The distribution plots correspond to BGC-Argo data (produced by Kimberlee Baldry) that is freely available and MEOP data (produced by Professor Mark Hindell) available from the Integrated Marine Observing System through program leaders (Professor Mark Hindell and Dr. Christophe Guinet). Data shown was that available on the 28/03/2020. Credits for instrumentation images go to Christoph Gerigk at© Sea-Bird Electronics for BGC-Argo and Clive McMahon for MEOP.
We define an SCM by the existence of a local maximum in chlorophyll-a concentrations (i.e., chlorophyll-a concentration in subsurface > chlorophyll-a concentration at surface). An SCM should form over spatial scales greater than one meter and be maintained on the timescales of a day or longer. This definition is adapted from Cullen (2015), who defines SCMs as subsurface chlorophyll-a maximum layers which are maintained by ecological processes. We do not follow this exact definition, since we have found some SCMs in the Southern Ocean that may be maintained by purely physical processes (see Along-shelf subduction). See Cullen (2015) for further discussion on terminology and its variants.
Most Southern Ocean ship-based studies measure ex situ chlorophyll-a by the direct determination of extracted chlorophyll-a pigments from vertical seawater samples. SCMs are then identified subjectively, by plotting depth profiles of chlorophyll-a (Wright and van den Enden, 2000; Parslow et al., 2001; Gomi et al., 2007; Whitehouse et al., 2008; Tripathy et al., 2015). Usually this is feasible based on the experience of the investigator as the presence (or absence) of an SCM is often visually clear.
In these studies, seawater samples are filtered through glass fiber filters to remove phytoplankton cells. Pigments are subsequently extracted from these cells using an organic solvent for measurement in the laboratory (Valente et al., 2016; Davies et al., 2018) and analyzed by either high-performance liquid chromatography (HPLC) (Shoaf, 1978; Valente et al., 2016), fluorometry or spectrophotometry (Yentsch and Menzel, 1963; Holm-Hansen et al., 1965; Strickland and Parsons, 1972; Zeng and Li, 2015). These methods relate the absorption spectra of extracted pigments to that of chlorophyll-a. The HPLC method is the most accurate method, as pigments are first separated through a chromatographic column (Figure 3). Pigments are not separated before analysis in fluorometry or spectrophotometry and thus chlorophyll-a measurements are subjected to the additive effects of pigments that absorb (or emit) at similar wavelengths (Davies et al., 2018). Fluorometry is the method that has been most widely used in the Southern Ocean. This method often significantly underestimates chlorophyll-a concentrations which is reportedly due to the presence of chlorophyll-b pigments (Daemen, 1986; Murray et al., 1986; Pinckney et al., 1994; Davies et al., 2018). This underestimation has no consequence for detecting SCMs and can be corrected for by regressing measurements against coincidental HPLC derived chlorophyll-a.
Figure 3. A schematic of (A) the different methods of measuring chlorophyll-a in the ocean discussed within this review and (B) a representation of differences in precision and accuracy for these methods. The gray concentric circles illustrate targets, where the center is indicative of an accurate and precise measurement, with the red dots illustrating the differences between accuracy and precision. Four targets are presented to illustrate varying degrees of precision and accuracy.
Recent Southern Ocean studies have used in situ deployments of fluorometers on elephant seals and BGC-Argo floats to observe SCMs over larger spatial scales. These studies have had to adapt to automatically detecting SCMs using preselected criteria to accommodate a new wealth of data (Grenier et al., 2015; Carranza et al., 2018). Supervised classification methods applied to chlorophyll fluorescence have offered a solution for studying thousands of chlorophyll fluorescence profiles and can provide an added advantage of distinguishing between different types of SCMs. They can do this by automatically identifying characteristic features of a vertical structure using prescribed algorithms. These methods have only been applied in two Southern Ocean studies (Grenier et al., 2015; Carranza et al., 2018), the Mediterranean Sea (Lavigne et al., 2015; Barbieux et al., 2019) and the Arctic (Ardyna et al., 2013).
In situ fluorometers measure chlorophyll fluorescence from live phytoplankton cells. As measurements are not performed ex situ on extracted pigments, yields of chlorophyll fluorescence (i.e., the ratio of emitted light to absorbed light) become important by controlling fluorescence to chlorophyll-a concentration ratios (Falkowski and Kolber, 1995; Chekalyuk and Hafez, 2011; Ostrowska et al., 2012). Shifts in phytoplankton communities, photophysiological state or nutrient regimes lead to variations in chlorophyll fluorescence yields of up to 7-fold over the global ocean (Behrenfeld et al., 2009; Cheah et al., 2013; Roesler et al., 2017). In addition, a physiological process whereby phytoplankton under light stress divert energy from their photosynthetic systems, called non-photochemical quenching (NPQ), introduces a further challenge for determining chlorophyll-a concentration by decreasing chlorophyll fluorescence yield by up to 100% in surface measurements (Behrenfeld et al., 2009; Doblin et al., 2011; Thomalla et al., 2018; Xing et al., 2018; Schallenberg et al., 2019).
Correcting fluorescence-derived chlorophyll-a in the Southern Ocean for observed variations in chlorophyll fluorescence yields is done by applying a regional scaling known as the Roesler factor (Haëntjens et al., 2017; Roesler et al., 2017). The application of a single scaling factor to the entire Southern Ocean does not seem viable considering the recent observations from Schallenberg et al. (2019) which show large changes in chlorophyll fluorescence yields across the Sub-Antarctic front. The use of satellite-derived chlorophyll-a as a surface reference to correct fluorescence-derived chlorophyll-a may be a more promising approach, although consideration that the two measurements are made on vastly different spatiotemporal scales is needed (Guinet et al., 2013). This approach comes possibly at the expense of accuracy, as the accuracy of Southern Ocean satellite-derived chlorophyll-a algorithms has repeatedly been questioned (Figure 3; Mitchell and Holm-Hansen, 1991; Sullivan et al., 1993; Dierssen, 2010; Johnson et al., 2013).
NPQ decreases chlorophyll fluorescence yield toward the surface, causing a subsurface fluorescence maximum (SFM) measured by a fluorometer to appear where an SCM may not exist (Falkowski and Kolber, 1995; Biermann et al., 2015; Carranza et al., 2018; Thomalla et al., 2018; Xing et al., 2018). In the Southern Ocean, NPQ is widespread likely due to iron limitation and high summertime light levels which have led to communities lacking the nutrients to photosynthesize when light is readily available (Roesler et al., 2017; Strzepek et al., 2019; Schallenberg et al., 2020). Many methods have been suggested to correct surface fluorescence in the presence of NPQ, but an evaluation of these corrections across the Southern Ocean has not been performed. NPQ corrections have been shown to perform differently and most fail to accurately correct fluorescence when an SCM is present in the Southern Ocean (Carranza et al., 2018; Thomalla et al., 2018; Xing et al., 2018). The comparison of day-time corrected fluorescence with night has been used in studies as a good test of the performance of an NPQ correction (Carranza et al., 2018; Thomalla et al., 2018; Xing et al., 2018). However, this test could be subject to the effects of diurnal controls on ‘real’ SCM formation which are largely unknown, as well as differences in situ sampling locations between day and night (Cullen, 2015; Carranza et al., 2018).
As a consequence of variable chlorophyll fluorescence yields, Southern Ocean in-situ fluorescence measurements are not inter-comparable with measurements derived from extracted chlorophyll-a (i.e., HPLC, fluorometry, spectrophotometry) and are best interpreted in terms of subsurface fluorescence until disparities are quantified and better understood (Carranza et al., 2018). From an alternative perspective, chlorophyll fluorescence has the potential to provide further insight on the photophysiological state of phytoplankton and could be used to distinguish the amount of light energy being used in primary production. This would allow a more accurate representation of basin primary production in ecosystem assessments, rather than assuming a constant photosynthetic efficiency per unit chlorophyll-a for in estimates (Behrenfeld et al., 2009; Boss et al., 2018; Schallenberg et al., 2019, 2020).
The type of supervised classification method chosen, the post-processing method for fluorescence measurements (e.g., despiking, smoothing), sampling resolution and measurement error are all expected to affect the detection of SCMs (Grenier et al., 2015; Carranza et al., 2018). To remain comparable, future studies should clearly consider the effect of these variables on their results. Furthermore, targeted study on the accuracy of fluorometers in the Southern Ocean is needed to assess the impacts of chlorophyll fluorescence yields and NPQ on ecosystem assessments of primary production, downward carbon flux and food-webs made through large scale deployments of fluorometers.
Southern Ocean SCMs were suggested to be both prevalent and annually recurrent by two early studies. Parslow et al. (2001) reported the first evidence of annually recurrent SCMs south of Australia around the Polar front, along the WOCE SR3 line (140°E). Here, SCMs form in late spring around 50 m and deepen to 100 m by early autumn (Parslow et al., 2001). Following this Holm-Hansen et al. (2005) reported geographically wide occurrences of SCMs in the Southern Ocean by synthesizing ship-based studies. The authors associated SCMs with low surface chlorophyll, iron limitation and with the temperature minimum layer of Antarctic waters.
A more recent study by Carranza et al. (2018) confirmed both the earlier reported annually recurrent and widespread SCMs. Their study identified SCMs using SFMs collected by an array of in situ fluorometers on BGC-Argo floats and elephant seals. SFMs begin to form in late-spring and decline from late-summer to early-autumn. At the peak of summer SFMs are detected in 60% of the dataset which is constrained mostly to the open Southern Ocean around the Polar front. These SFMs appear to show synergies with episodic wind events, forming only during calm conditions, and may coincide with increased phytoplankton biomass. This study demonstrated the capabilities of fluorometers to provide a basin-wide view of SCMs from the interpretation of SFMs, despite the widespread day-time NPQ of fluorescence, by considering only measurements made at night.
In addition to SCMs in the vicinity of the Polar front (Kopczynska et al., 2001; Parslow et al., 2001; Armand et al., 2008a; Westwood et al., 2011; Tripathy et al., 2015; Rembauville et al., 2016a), ex situ measurements have found summer SCMs around the continental slopes of Antarctica and Southern Ocean islands (Garibotti et al., 2003; Holm-Hansen and Hewes, 2004; Demidov et al., 2007; Armand et al., 2008a; Whitehouse et al., 2008; Erickson et al., 2016), around eddies (Clementson et al., 1998; Daly et al., 2001) and in the Seasonal Ice Zone (Mikaelyan and Belyaeva, 1995; Bathmann et al., 1997; Cailliau et al., 1997; Wright and van den Enden, 2000; Gomi et al., 2007; de Villiers et al., 2015). During summer, a recurrent and notable absence of SCMs has only been reported in the permanently open ocean zone of Antarctic waters (Cailliau et al., 1997; Gomi et al., 2007; Rigual-Hernández et al., 2015a, b) and the Sub-Antarctic zone south of Australia (Figure 4; Clementson et al., 1998; Parslow et al., 2001; Westwood et al., 2011).
Figure 4. The number of studies that identify a mechanism of formation discussed in this review presented by region of the Southern Ocean. The evidence each study has given for these mechanisms is given in Supplementary Table S2. Regions are as defined in Deppeler and Davidson (2017), however, note that the location of fronts and sea-ice extent are highly variable. ACZ, Antarctic Coastal Zone (including continental slope to 2000 m); SIZ, Seasonal Ice Zone; POOZ, Permanently Open Ocean Zone; PFZ, Polar Frontal Zone; SAZ, Sub-Antarctic Zone; SOI, Southern Ocean Islands. The 2000 m isobar is shown as a gray line.
This is the limited viewpoint that the literature to date offers on the prevalence and persistence of Southern Ocean SCMs. The field is at a tipping point of observational power through the BGC-Argo and the MEOP programs (Figure 2; Guinet et al., 2013; Claustre et al., 2020). This power will see an unprecedented increase in reported observations of SFMs and SCMs (Carranza et al., 2018).
Identifying the ecological and physical processes (sensu Cullen, 2015) that maintain SCMs is difficult. Ancillary environmental data are required to disentangle these processes and to test hypotheses. Eighteen Southern Ocean studies have been identified to contain ancillary data that could be used to evaluate processes that form SCMs (Figure 4 and Supplementary Table S2).
The studies were identified through a literature search that revealed 145 scholarly articles published between 1991 and 2019. The search was performed using the Web of Science Core Collection and Scopus databases together with the key search terms “Subsurface chlorophyll-a maxima” and “Southern Ocean.” Derivatives of “subsurface chlorophyll-a maxima” were also implemented (Supplementary Text S1). Results from these studies are discussed below in the context of Southern Ocean biogeochemistry. We have summarized this information in new conceptual models for Southern Ocean regions to guide future research (Figure 5).
Figure 5. Proposed conceptual models for the formation of Southern Ocean SCMs and diatom SCMs (dSCMs) (A) in the oceanic HNLC regions, (B) downstream of Southern Ocean Islands (SOI), (C) following sea-ice retreat, (D) in warm-core eddies in the Sub-Antarctic Zone, (E) in cold-core eddies in the Sub-Antarctic Zone and (F) by subduction along the continental shelf (as in Erickson et al., 2016). Conceptual models are presented as schematics, with examples of what vertical measurements of chlorophyll-a and limiting nutrients might look like.
The stability of the tropical SCM relies on vertically mixed inputs of a limiting nutrient across a pycnocline into the base of the mixed layer combined with a light field that decreases exponentially with depth. This creates a depth of optimum growth for phytoplankton, at the SCM, usually just above the pycnocline (Figure 1). Below the SCM, growth is limited by light, and by nutrients above the SCM. At the SCM community shifts can occur due to low rates of mixing as different phytoplankton groups respond differently to changing light, nutrient availability and disproportionate grazing pressures (Strom, 2001; Cullen, 2015; McKie-Krisberg et al., 2015; Latasa et al., 2016, 2017). Despite these shifts, the community is dominated by picoplankton through the water column (Furuya, 1990; Estrada et al., 2016; Latasa et al., 2017). In the darker environment of the SCM phytoplankton may also undergo photo-acclimation, increasing intracellular chlorophyll-a concentration (Cullen, 2015; Estrada et al., 2016; Latasa et al., 2017). If there are high rates of surface mixing, the SCM is destroyed (see Cullen, 2015 for further discussion).
Sufficient subsurface inputs of the limiting micro-nutrient iron are not widely present across the Southern Ocean to sustain the light-nutrient co-limitation characteristic of tropical SCMs. Iron-depleted mixed layers have been shown to coincide with SCMs in the open Southern Ocean (Boyd et al., 2001; Holm-Hansen et al., 2005), but iron concentrations are still remarkably low below the mixed layer from late-spring to summer leading to small diffusive fluxes (Sohrin et al., 2000; Tagliabue et al., 2012, 2014; Klunder et al., 2014; Bowie et al., 2015). This can be attributed to the rapid use of winter iron repositories as the mixed layer shoals during the initial spring bloom (Tagliabue et al., 2014). The presence of SCMs at or deeper than the pycnocline and a consistent, marked community shift toward large diatoms at the SCM are further features which do not fit with the conceptual model of the tropical SCM (Kopczynska et al., 2001; Parslow et al., 2001; Holm-Hansen et al., 2005; Westwood et al., 2011; Moore et al., 2013; Carranza et al., 2018). Instead we find that an assortment of Southern Ocean processes may be important for maintaining diatom SCMs including stratification, buoyancy regulation by large diatoms, persistent iron limitation and grazing.
Theoretically, iron fertilization could relieve iron limitation in the mixed layer to induce an SCM sustained by light-nutrient co-limitation, by allowing silicate or nitrate, which have vertically mixed inputs, to become the limiting nutrient. The primary sources of iron to the Southern Ocean are coastal margins, shallow bathymetry, dust, hydrothermal venting and sea-ice melt (Blain et al., 2008; Raiswell et al., 2008; Boyd and Ellwood, 2010; Boyd et al., 2012; Graham et al., 2015; Ardyna et al., 2019). Coastal inputs of iron and sea-ice melt have been associated with the development of SCMs which we explore further (see Iron fertilization by land masses and Sea-ice retreat), however the role of other iron sources in SCM formation is purely speculative due to a lack of coincident observations.
Large diatoms find an environmental niche in the pycnocline under severe iron limitation and high stratification, remaining in the upper ocean when other phytoplankton taxa cannot. This leads to their accumulation forming the diatom SCM which have been observed around the Polar front (Bathmann et al., 1997; Kopczynska et al., 2001; Parslow et al., 2001; Armand et al., 2008a; Gomi et al., 2010; Westwood et al., 2011; Tripathy et al., 2015), on the Kerguelen Plateau (Armand et al., 2008a), off the continental slope of Antarctica (Quilty et al., 1985; Fiala et al., 1998; Garibotti et al., 2003) and in the Seasonal Ice zone (Cailliau et al., 1997; Wright and van den Enden, 2000; Gomi et al., 2007). The most striking observation of this phenomenon was made in the Seasonal Ice Zone by Quilty et al. (1985) who encountered a diatom SCM of remarkably high biomass, dominated by Thalassiothrix antarctica.
The tendency of large diatoms to dominate the diatom SCM has been revealed by microscopy and CHEMTAX analysis of accessory pigments (Quilty et al., 1985; Bathmann et al., 1997; Fiala et al., 1998; Wright and van den Enden, 2000; Kopczynska et al., 2001; Gomi et al., 2007, 2010; Armand et al., 2008a; Westwood et al., 2011). Further measurements of the accessory pigment fucoxanthin and biogenic silica have indicated increased diatom dominance at the SCM compared to coincident surface communities (Parslow et al., 2001; Quéguiner, 2001; Armand et al., 2008a).
Diatom SCMs only appear to form in the absence of a surface bloom and have been linked to severe iron depletion of surface waters (Parslow et al., 2001; Holm-Hansen and Hewes, 2004; Holm-Hansen et al., 2005). Further, the taxa that dominate diatom SCMs are found in upstream surface blooms, where upstream refers to island or coastal blooms (Armand et al., 2008a; Gomi et al., 2010; Westwood et al., 2011; Tripathy et al., 2015). We speculate that the diatom SCM is formed following the decline of the surface bloom as conditions fade under persistent iron or silicate limitation (Figure 5A). This could explain the regime shifts from a well-mixed surface bloom to a diatom SCM across continental shelves (Fiala et al., 1998; Garibotti et al., 2003; Holm-Hansen and Hewes, 2004), downstream from Southern Ocean Islands (Armand et al., 2008a) and with sea-ice retreat (Cailliau et al., 1999; Wright and van den Enden, 2000; Gomi et al., 2007).
The connectivity of phytoplankton communities between coastal and oceanic regions is most evident from studies conducted in the Indian sector of the Southern Ocean. Around the Polar front diatom SCMs were found to consistently contain significant communities of the large diatoms Fragilariopsis kerguelensis and Dactyliosolen antarcticus, which are both observed to bloom in the surface waters around the Kerguelen Plateau (Kopczynska et al., 2001; Armand et al., 2008a; Gomi et al., 2010; Westwood et al., 2011). In the Seasonal Ice Zone community composition is significantly different to that around the Polar front. While Fragilariopsis kerguelensis is often present in the mixed layer (downstream from the Kerguelen plateau), large diatoms Pseudo-nitzschia prolongatoides, Pseudo-nitzschia spp., Corenthon pennatum and Chaetoceros spp. dominate in the Seasonal Ice Zone (Fiala et al., 1998; Gomi et al., 2007, 2010). The decline over time and dominance in the diatom SCM of Pseudo-nitzschia spp. and Chaetoceros spp. (sea-ice diatoms), over Fragilariopsis kerguelensis suggests that SCMs formed in the Seasonal Ice Zone are most likely short lived and independent of SCMs formed around the Polar front (Gomi et al., 2007).
Ecological and physiological adaptions of large diatoms must be responsible for the ability of these taxa to survive for long periods at the pycnocline when conditions are not suitable for a surface bloom. The ability of large diatoms to regulate buoyancy over nutrient gradients, using vacuolar control of solute balance, should play a large role in sustaining their dominance at the SCM and prevent their export into the mesopelagic ocean (Waite et al., 1992; Fisher and Harrison, 1996; Kemp and Villareal, 2013). Interestingly, Southern Ocean large diatoms have been observed to increase their buoyancy under bloom conditions, iron addition and decreased light, leading to reduced downward carbon export (Waite et al., 1992; Fisher and Harrison, 1996; Waite and Nodder, 2001; Acuña et al., 2010). Diatoms efficiently use nutrients, due to their ability to take up nutrients rapidly and maintain large nutrient reserves (Lomas and Glibert, 2000; Litchman et al., 2006; Kemp and Villareal, 2013, 2018; Hansen and Visser, 2019). This coupled with adaptions to low-light environments – including optimized photosystems and photonic crystals that enhance light harvesting - allows large diatoms to be active producers in the pycnocline which receives pulses of nutrients via internal waves (Holligan et al., 1985; Kemp and Villareal, 2013; Strzepek et al., 2019; Goessling et al., 2020).
Heavy silicification and large spines to resists heavy grazing pressure also confer some protection against the depletion of these aggregations (Smetacek et al., 2004; Assmy et al., 2013). A disproportionate grazing pressure across species may also aid bloom-decline and contribute to the maintenance of the diatom SCM in the open ocean (Assmy et al., 2013). However the diatom SCM is not immune to grazing pressure as targeted grazing by microzooplankton, copepods and heterotrophic dinoflagellates is indicated by the presence of empty frustules and a maximum of zooplankton abundance (Froneman and Perissinotto, 1996; Kopczynska et al., 2001; Parslow et al., 2001; Armand et al., 2008; Gomi et al., 2010; Rembauville et al., 2016).
It is unknown if silicate, iron or light is most important in buoyancy regulation of Southern Ocean large diatoms. It may depend on the limiting nutrient of these communities which is not completely resolved (Waite et al., 1992; Fisher and Harrison, 1996; Boyd et al., 2005). Shallow bathymetry could support large subsurface reservoirs of lithogenic silica, potentially aiding in the persistence of diatoms at depth as they are carried around with the Antarctic circumpolar current (Parslow et al., 2001; Quéguiner, 2001; Robinson et al., 2016). Hopkinson et al. (2007) is the only study that has conducted an iron-addition experiment to observe iron limitation in an oceanic Southern Ocean SCM. This study found that the SCM community in the West-Antarctic Peninsula was iron limited and in situ iron was still remarkably low below the pycnocline. Unfortunately, few studies that observe an SCM have reported vertical profiles of iron and silicate concentrations.
The stratification of the water column to form a shallow pycnocline over spring and summer is important for the maintenance of diatom SCMs (Figure 5A). In late-autumn the deepening of the pycnocline leads to the destruction of the diatom SCM and a “fall dump” event leads to the export of the community as light levels become too low for survival (Smetacek, 1985; Kemp et al., 2000). Observations of SFMs further suggest that disturbing the pycnocline via episodic storms likely leads to the re-suspension of communities and the subsequent re-formation of the diatom SCM when calm conditions prevail (Carranza et al., 2018). If these SFMs correspond to diatom SCMs around the Polar front, this may point toward the survival of the diatom SCM community during episodic storm events.
In the high-energy environments of Southern Ocean islands in the path of the Antarctic circumpolar current, lateral flow is strongly zonal. A persistent supply of iron in the vicinity of and downstream of these islands is observed to be in excess of the demand of phytoplankton growth (Blain et al., 2001, 2008; Planquette et al., 2007; Chever et al., 2010; Bowie et al., 2015; Robinson et al., 2016; Schlosser et al., 2018). This iron supply facilitates large oceanic surface blooms that decline with distance from a land mass, as a limiting nutrient becomes depleted in the surface layer (Graham et al., 2015; Robinson et al., 2016). SCMs have been observed to form above the pycnocline in the wake of high chlorophyll plumes around the Kerguelen Plateau and South Georgia Island (Blain et al., 2001; Armand et al., 2008a; Whitehouse et al., 2008; Grenier et al., 2015). These SCMs are likely a result of a transient light-nutrient co-limited state as the surface bloom declines (Figure 5B; Whitehouse et al., 2008; Grenier et al., 2015). The limiting nutrient is silicate downstream from South Georgia Island, but may be silicate or iron downstream from the Kerguelen Plateau (Blain et al., 2007; Mongin et al., 2007; Whitehouse et al., 2008; Closset et al., 2014; Robinson et al., 2016).
Over the Antarctic continental shelf, high levels of iron persist through the water column due to high inputs of iron from sediments and the movement of circumpolar deep water to the surface (Blain et al., 2008; Tagliabue et al., 2012; Klunder et al., 2014; Dinniman et al., 2020). Even in this iron rich region surface waters can exhibit persistent iron limitation due to the demands of rapid phytoplankton growth in spring and summer (Martin et al., 1990; Coale et al., 2003; Bertrand et al., 2007). There is an absence of SCMs above the pycnocline in these surface waters which suggests that phytoplankton deplete subsurface sources of iron over shallow bathymetry more quickly than it is supplied (Martin et al., 1990; Coale et al., 2003; Bertrand et al., 2007; Alderkamp et al., 2015). This is further evident from records of iron limitation near the pycnocline in the Amundsen Sea (Alderkamp et al., 2015). This key difference from Southern Ocean Islands near the ACC is likely due to relatively weak surface currents in the low-energy, sheltered region and the transient nature of sea-ice as an iron source (Fahrbach et al., 1992; Dinniman et al., 2011, 2020; Stewart and Thompson, 2013; Park et al., 2014; Robinson et al., 2016).
Southern Ocean studies have often suggested iron-light co-limitation as a driver of SCM formation over the continental shelf due to decreasing iron supply by land masses (Garibotti et al., 2003; Holm-Hansen et al., 2005; Kahl et al., 2010). These oceanic SCMs develop below the pycnocline, suggesting that light-nutrient co-limitation plays no part in facilitating their formation. We reframe these observations by acknowledging that while iron limitation may play a role in maintaining these SCMs, the distribution of nutrients through the water column does not control their formation, leading us to suggest that these are diatom SCMs.
Sea-ice retreat facilitates intense phytoplankton blooms in surface waters (Raiswell et al., 2008; Death et al., 2014; Duprat et al., 2016). These blooms are thought to be initiated by seed phytoplankton released from sea-ice (Grossi et al., 1987; Suzuki et al., 2001; Gomi et al., 2007; Mangoni et al., 2009; Lizotte, 2015), the release of light limitation from sea-ice cover (Lancelot et al., 1993; Taylor et al., 2013) or the release of bio-available iron as the ice melts (Lannuzel et al., 2016), or a combination of all these mechanisms. Following surface blooms, diatom SCMs have been observed to trail sea-ice retreat (Figure 5C; Cailliau et al., 1999; Wright and van den Enden, 2000; Gomi et al., 2007). These diatom SCMs could be related to the diatom SCMs that are frequently observed over the continental slope of Antarctica.
Surface blooms at the sea-ice edge rapidly sink when nutrients quickly become depleted from meltwater (Smetacek et al., 1992; Wright and van den Enden, 2000; Ambrose et al., 2005; Wright et al., 2010; Boetius et al., 2013) or and are heavily grazed (Stretch et al., 1988; Smetacek et al., 1990; Scharek et al., 1994; Michel et al., 1996; Gomi et al., 2007). After sea-ice retreat, phytoplankton community composition is initially uniform with depth and dominated by diatoms and Phaeocystis spp., as seen by microscopy and pigment analysis (Cailliau et al., 1999; Wright and van den Enden, 2000; Gomi et al., 2007). It is at this stage, Gomi et al. (2007) detected sea-ice algae (Phaeocystis spp. and Pseudo-nitzschia prolongatoides). Cailliau et al. (1999) used a combination of pigment and lipid analysis to suggest that the subsequent diatom SCM in a sea-ice zone was formed by a selective export of nanoflagellates (inc. Phaeocystis spp.) due to grazing, while diatoms are left behind in the SCM. The ability of diatoms to regulate their buoyancy across nutrient and light gradients, thus preventing their sinking could prevent their immediate export and survival at the diatom SCM as bloom conditions decline (Waite et al., 1992; Fisher and Harrison, 1996; Moore and Villareal, 1996; Waite and Nodder, 2001; Kemp et al., 2006; Acuña et al., 2010).
The fate and spatial extent of these diatom SCMs associated with sea-ice retreat has not been explored. Further, the ability of sea-ice to fertilize blooms is inconsistent, as demonstrated in Bathmann et al. (1997). They followed the retreat of sea-ice for 6 weeks and observed little phytoplankton growth at the ice-edge, even though ice-algae and meltwater were detected. This may have been due to high wind conditions inhibiting the ice-edge blooms (Lancelot et al., 1993; Fitch and Moore, 2007; Pellichero et al., 2020) or intense zooplankton grazing at the ice-edge like that exerted by large krill swarms (Stretch et al., 1988; Smetacek et al., 1990; Scharek et al., 1994). Consequently, these diatom SCMs may not be observed around the entire marginal ice zone.
Eddies are abundant and ubiquitous in the Southern Ocean, resulting from the instabilities at high energy fronts. Particularly intense eddy activity occurs where fronts interact with shallow bathymetry (Bryden and Heath, 1985). A small number of SCMs have been attributed to eddies spinning off the Subtropical front, the Sub-Antarctic front and the Antarctic Divergence (Clementson et al., 1998; Wright and van den Enden, 2000; Daly et al., 2001; Westwood et al., 2011; de Villiers et al., 2015). To date, eddies are speculated to facilitate Southern Ocean SCMs by two mechanisms; (1) nutrient-light co-limitation within warm-core eddies from the Subtropical front and (2) increased growth at the pycnocline cold-core eddies from the Sub-Antarctic Front, likely due to the injection of nutrients (Figures 5D,E).
An SCM observed by Clementson et al. (1998) exhibited a nitrate limited tropical SCM at the center of a warm-core eddy that had come from the nitrate-poor Subtropical zone into the nitrate-rich Sub-Antarctic zone, where SCMs are consistently not observed (Clementson et al., 1998; Wright and van den Enden, 2000; Daly et al., 2001; Westwood et al., 2011). Westwood et al. (2011) also detected an eddy associated SCM above the pycnocline, revealing the warm-core eddy’s capacity to transport iron-rich waters into the Sub-Antarctic zone. This study observed higher concentrations of iron at the surface in the eddy, compared to below which would allow a nitrate limited tropical SCM to exist (Figure 5D). The fate of this type of eddy-associated SCMs and their biogeochemical implications is unknown.
Another SCM that was uncharacteristically observed in the sub-Antarctic zone at 120–160 m depth by Daly et al. (2001) was in a cold-core eddy spun off the Sub-Antarctic front in summer. The SCM community likely originated from near the Polar front, where diatom SCMs can be observed at similar depths. The SCM within the cold-core eddy was observed to be of higher biomass than those around the Polar front, which was speculated to be sustained by an injection of nutrients from shoaled isopycnals (Figure 5E). This is supported by evidence from diatom SCMs in the Arctic which thrive under nutrient pulses via internal waves and eddies (Kemp and Villareal, 2013). The fate of the SCM was likely to sink with the weakening of the eddy, and contributing to downward carbon export, as communities were not well sustained in the Sub-Antarctic zone.
Detecting eddy-associated SCMs with autonomous platforms could further reveal the fate of these SCMs and their significance in the Southern Ocean, providing insight into this narrow view displayed by the literature.
SCMs could be formed by the subduction of productive surface waters off the coastal shelf (Figure 5F). Wright and van den Enden (2000) observed a band of SCMs along a subduction zone associated with the Antarctic Divergence. This mechanism has merit but is difficult to prove with one dimensional ship-based data.
Erickson et al. (2016) provided the strongest evidence that productive waters move along isopycnals that are subducted along the continental shelf to below the pycnocline as seen by glider transects. This community was also speculated to remain productive below the upper mixed layer. This leads to the question of whether the large diatom aggregation (comprised mostly of Thalassiothrix antarctica) observed by Quilty et al. (1985) was also formed as a result of this subduction combined with the ability of large diatoms to regulate buoyancy (Waite and Nodder, 2001; Kemp and Villareal, 2013).
Photo-acclimation enables phytoplankton to harvest light more efficiently in low light environments by increasing cellular chlorophyll-a. This has been observed in the pycnocline in the Seasonal Ice Zone and around the Polar front in the Southern Ocean (Mikaelyan and Belyaeva, 1995; Rembauville et al., 2016a). This process can also occur in the mixed layer if mixing rates are slower than the rate of photo-acclimation (Cailliau et al., 1997; Dusenberry et al., 2000).
Observations conducted at the cellular level under a microscope by Mikaelyan and Belyaeva (1995) were made in the absence of a deep diatom-dominated SCM, confirming photo-acclimation by Southern Ocean phytoplankton communities. Increased cellular concentrations of chlorophyll-a could be an indication of a community shift (diatoms often have high cellular chlorophyll-a), when chlorophyll-a concentrations are compared to particulate organic carbon concentrations (as in Rembauville et al., 2016a). Consequently, it is difficult to distinguish photo-acclimation from community shift in chlorophyll-a concentration profiles unless additional pigment or microscopic analysis is performed.
Evidence of SCM formation by top-down grazing pressure was not evident in the key literature but has been explored through models. Mixotrophs exert uneven grazing pressure through the water column with higher grazing rates observed at the surface due to light-aided digestion and photooxidative stress (Strom, 2001; McKie-Krisberg et al., 2015). Thus, it has been shown through a model that this uneven grazing pressure could lead to the formation of a SCM in the tropics (Moeller et al., 2019). Mixotrophs may play a significant role in Southern Ocean ecosystems, suggesting the validity of these hypothesis in this region (Froneman and Perissinotto, 1996; Christaki et al., 2008; Moorthi et al., 2009; Norbury et al., 2019). However, their relative abundance is significantly less compared to the tropics and so the importance of this process in controlling SCM formation as revealed by the tropical model may be subdued (Edwards, 2019).
Without extensive observations of SCMs, assessments of their impact in Southern Ocean ecology is limited. Regional process studies observed that SCMs contribute to water column primary production (Parslow et al., 2001; Westwood et al., 2011; Tripathy et al., 2014, 2015), facilitate large downward carbon export events (Kemp et al., 2000; Llort et al., 2018), are sites of macrofauna foraging (Dragon et al., 2010; Kahl et al., 2010; Williams et al., 2011; Saijo et al., 2017; Rivière et al., 2019; Siegelman et al., 2019) and are sites of intense zooplankton grazing (Atkinson et al., 1992; Froneman and Perissinotto, 1996; Kopczynska et al., 2001; Parslow et al., 2001; Gomi et al., 2010; Rembauville et al., 2016a). The ecological significance of an SCM is enhanced when they also represent a subsurface biomass maximum, or high primary production, or both. SCMs can exist independently of subsurface biomass maxima and increased primary production, due to changes in cellular chlorophyll-a concentrations either by photo-acclimation or a community shift (Cullen, 2015).
To date, diatom SCMs are the most widely observed type of SCM in the pelagic Southern Ocean. They have been recurrently observed south of Tasmania, Australia over several years (Parslow et al., 2001) as well as in the Atlantic and Indian sectors (Bathmann et al., 1997; Tripathy et al., 2015). From fluorometers mounted on BGC-Argo profiling floats and elephant seals, mostly around the Polar front, summertime SFMs occurring at the pycnocline have been found in 60% of observations (Carranza et al., 2018). This begs the question; Are diatom SCMs responsible for all SCMs/SFMs around the Polar front, and if so, what is the significance of this seasonal and wide-spread persistence?
Southern Ocean SCMs often occur in very low light environments so their contribution to water column integrated primary production may be small. If formed above the pycnocline, SCMs are expected to be active contributors to water column production because they will likely be exposed to sufficient light (Herbland and Voituriez, 1979; Estrada et al., 1993; Cullen, 2015). It is difficult to discern if an SCM that forms below the pycnocline is a productive or senescent feature without measurements of primary production, because communities shift, and communities below the pycnocline function differently to those above (Parslow et al., 2001; Westwood et al., 2011; Tripathy et al., 2014, 2015). Basin-wide observations of SCMs, alongside a better understanding of the photosynthetic efficiencies and biomass of deep communities, are required to assess the potential impacts of SCMs on total water column primary production and the regulation of the global carbon cycle.
Regardless of their contribution to primary production and biomass, diatom SCMs at the pycnocline in the Southern Ocean support unique food webs and contribute to downward carbon export. The ability of a diatom SCM to act as a subsurface biomass maxima is variable and the subsequent relationship of biomass to primary production is complex (Kopczynska et al., 2001; Parslow et al., 2001; Armand et al., 2008; Gomi et al., 2010). Diatom SCMs are active contributors to total summer water column productivity around the Polar front (Parslow et al., 2001; Westwood et al., 2011; Tripathy et al., 2014, 2015). This productivity supports selective grazing by microzooplankton, copepods and heterotrophic dinoflagellates at the SCM, creating a unique trophic structure (Froneman and Perissinotto, 1996; Kopczynska et al., 2001; Parslow et al., 2001; Gomi et al., 2010; Rembauville et al., 2016a). While their growth may be light limited for long periods, diatoms are a form of new production with a high downward carbon export efficiency. Their unique photosynthetic apparatuses have large light harvesting complexes, enhancing their ability to photosynthesize at <1% surface light (Parslow et al., 2001; Tripathy et al., 2015; Goessling et al., 2020). These light levels are extremely low and would halt primary production for most species of phytoplankton. Adding to their remarkable photosynthetic capacities, some species can survive long periods of darkness by forming a resting spore (Ferrario et al., 1998; Armand et al., 2008a, b; Salter et al., 2012). The mass formation of these resting spores off the Kerguelen plateau is a source of large downward carbon export events to the seafloor (Armand et al., 2008b; Salter et al., 2012; Rembauville et al., 2016b). In addition to this, large “fall dump” events occur as the deepening of the pycnocline in autumn forces these deep diatom aggregates to sink (Kemp et al., 2000; Rigual-Hernández et al., 2015b).
The diatom SCM is likely an ecological stage between the coastal bloom and mass export event, apparently employing unique physiological and ecological adaptions to survive in turbulent, low-iron oceanic conditions, where other taxa cannot. It is well established that this subsurface biomass only persists in iron depleted waters where low surface chlorophyll-a is observed (Parslow et al., 2001; Garibotti et al., 2003; Holm-Hansen et al., 2005), but these communities may display successional connectivity with coastal areas driven by ocean physics (Kopczynska et al., 2001; Graham et al., 2015) and diatom life stage (Ferrario et al., 1998; Armand et al., 2008b; Salter et al., 2012). This is suggested by the common coastal occurrence of phytoplankton associated with oceanic SCMs, their eventual deposition on the seafloor (Kemp et al., 2000, 2006; Kemp and Villareal, 2013) and regional differences in community structure (Gomi et al., 2007, 2010).
Other observations of SCMs, not necessarily attributed to diatom SCMs, have shown that Adelie penguins and elephant seals target krill and mesopelagic fish that congregate around SCMs (Dragon et al., 2010; Kahl et al., 2010; Williams et al., 2011; Saijo et al., 2017; Rivière et al., 2019; Siegelman et al., 2019). For elephant seals, this is true both in oceanic regions around the Kerguelen plateau and around the ice-edge. Elephant seals particularly target areas of high kinetic energy, and their prey may be supported by high downward carbon export to the mesopelagic zone via eddies (Saijo et al., 2017; Rivière et al., 2019; Siegelman et al., 2019).
This Review presents evidence of Southern Ocean SCMs as a widespread and recurrent summer feature. The formation of these SCMs differs from the processes that form the better-known tropical SCM (Figure 1). The most common Southern Ocean SCMs are widespread diatom SCMs which potentially contribute to downward carbon export and primary production. Satellite composites of chlorophyll-a miss these features, introducing biases toward surface communities in ecosystem assessments of downward carbon export, primary production and food-webs that will likely be used in MEASO. Going forward, an integrated platform approach is needed that draws on detailed understandings available from ship-based observations – as presented in this Review – alongside the new wealth of observations from widespread deployments of fluorometers through the BGC-Argo and MEOP programs.
Diatom SCMs are not only missing from ecosystem assessments of the present Southern Ocean but are also missing in future biogeochemical trajectories. Paleo-sediment records have identified increased deposits of opal (a proxy for diatom concentration) around the Polar front, coincident with periods of increasing atmospheric carbon dioxide as seen in Antarctic ice cores (Gottschalk et al., 2016). This negative feedback mechanism, which may be stimulated by increased stratification promoted by warmer and fresher surface waters, is not captured in current biogeochemical models used to make ecosystem assessments of future ocean trajectories (Kemp and Villareal, 2013, 2018).
Incorporating these diatom SCMs, and the processes that lead to their formation, into models has been limited by past observational capacities. These relatively thin features (1–10 m thick) are not fully captured by coarse ship-based observations (Kemp et al., 2006; Kemp and Villareal, 2013, 2018). Further, knowledge on the active buoyancy regulation of large diatoms is fragmented with the ability to buoyancy regulate and active sinking only recorded for experiments on a few species of large diatoms (Villareal, 1988; Waite et al., 1992; Moore and Villareal, 1996; Fisher and Harrison, 1996; Waite and Nodder, 2001; Boyd et al., 2005; Acuña et al., 2010; Gemmell et al., 2016). Parameterizations for the control of light and nutrients on buoyancy regulation in large diatoms are needed in order to model the behavior of these communities and their interaction with ocean mixing.
Translating widespread observations of SFMs made by the BGC-Argo and MEOP programs to diatom SCMs is essential to understanding the environmental controls of these biogeochemical niches. Key studies discussed here suggest that the composition and prevalence of diatom SCMs communities may be delineated by bathymetry, fronts and sea-ice (Figure 4). BGC-Argo can observe these deep diatom-dominated communities as floats routinely samples at <2 m resolution, whilst the ability of MEOP to capture these features under the broken stick sampling method – which represents profiles using a subsample of data based on points of high variability - has not yet been assessed (Guinet et al., 2013).
With increased observation density in time and space, coupled with ocean models, the role of environmental drivers including ocean physics, bathymetry and sea-ice can be explored as it has been for surface chlorophyll-a concentrations from satellites (Grant et al., 2006; Graham et al., 2015; Ardyna et al., 2017; Dawson et al., 2018). Connecting coastal processes to diatom SCMs may also be possible by testing the possible links to fertilization of iron by land-masses, sea-ice retreat and along-shelf subduction that have been revealed in this Review. Further the role of frontal structures, eddies, subduction and stratification in controlling other types of SCMs can also be explored.
The greatest limitation in studying subsurface phytoplankton distributions in the Southern Ocean is the translation of chlorophyll fluorescence to chlorophyll-a and biomass concentrations. Specifically, the presence of day-time NPQ is the greatest source of inaccuracy to the study of SCMs, causing a SFM to exist where an SCM may not. The variability of fluorescence and chlorophyll to carbon also presents a challenge for conversions to biomass estimates. Further uncertainties introduced along with variations in surface community Chl:C ratios (Behrenfeld et al., 2005; Thomalla et al., 2017), are community shifts with depth to diatoms which contain higher intracellular chlorophyll and more gradual increases of intracellular chlorophyll due to photoacclimation (Mikaelyan and Belyaeva, 1995; Kopczynska et al., 2001; Armand et al., 2008; Gomi et al., 2010; Rembauville et al., 2016a). Understanding these ratios is important for representing Southern Ocean SCMs in biogeochemical models (Galbraith et al., 2010; Aumont et al., 2015; Verdy and Mazloff, 2017).
Until the accuracy of fluorescence-derived chlorophyll-a has been resolved, an integrated framework for carefully studying SCMs, through SFMs measured at night is needed. Their translatability to SCMs could be assessed using sparse ship-based data possibly equipped with fluorometers for paired assessments. The potential benefits of this is highlighted by our discussion of Carranza et al. (2018), who may have observed widespread deep diatom-dominated communities around the Polar front through SFMs. When considering ship-based observations in the literature it seems likely that these are oceanic diatom SCMs. Combining the evidence from process studies and autonomous platforms, the role of episodic storms in destroying the stability of diatom SCMs and their subsequent re-formation under calm conditions becomes apparent.
Consequently, ship-based observations will still play a large part in the future discussion on subsurface distribution and production of phytoplankton. Particularly, the role of iron and silicate in mediating SCMs and subsurface production at the pycnocline cannot be studied autonomously and we have found this to be still largely enigmatic. Iron and silicate addition experiments that include deep-diatom communities, as well as cooccurring measurements of iron, silicate and chlorophyll through the water column are needed in the future. These measurements will be particularly important for resolving the role of nutrients in sustaining stable diatom SCMs and SCMs above the pycnocline found near retreating sea-ice and Southern Ocean islands.
Southern Ocean SCMs can no longer be ignored in ecosystem assessments. Our review presents evidence of widespread diatom SCMs that may play an important role in Southern Ocean ecology and climate. Further progress on this topic will only be made through extensive data sharing and collaboration between international researchers to achieve an integrated observation framework (Brett et al., 2020). This framework must use both ship-based studies and autonomous technologies.
KB reviewed the literature and led the production of the manuscript. PS, NH, and PB assisted in conceptual developments and production of the manuscript. All authors contributed to the article and approved the submitted version.
This research was supported under Australian Research Council’s Special Research Initiative for Antarctic Gateway Partnership (Project ID: SR140300001).
The authors declare that the research was conducted in the absence of any commercial or financial relationships that could be construed as a potential conflict of interest.
We acknowledge the enormous amount of effort conducted by multiple institutions, including ship-time and analysis, to deliver the numerous studies highlighted in this review. Thanks to the continuing international collaborations and funding bodies that sustain the BGC-Argo (https://biogeochemical-argo.org/) program. We further thank the following for sustaining the Southern Ocean MEOP fluorescence program (1) Australia’s Integrated Marine Observing System (IMOS) Tracking Facility (R. Harcourt and C. McMahon) – IMOS is enabled by the National Collaborative Research Infrastructure Strategy (NCRIS). It is operated by a consortium of institutions as an unincorporated joint venture, with the University of Tasmania as Lead Agent – (2) the CEBEC/CNRS France, through the Mammals as samplers of the Ocean Environment (MEMO) for the French data, (3) the French Polar Institute (program 109: PI. H. Weimerskirch and 1201: PI. C. Gilbert and C. Guinet), the SNO-MEMO and CNES-TOSCA, and (4) Professor Mark Hindell and Dr. Christophe Guinet. Additionally, thanks to Professor Mark Hindell for providing the data distribution map for from MEOP and Prof. Fabien Roquet for maintaining this database. Thank you to two reviewers for their comments which lead to improvements in the review. Finally, thanks to Harris J. Anderson, Lennart T. Bach, Joan Llort, Christina Schallenberg, and Svenja Halfter for valuable specialist communications that aided in the development of parts of the manuscript.
The Supplementary Material for this article can be found online at: https://www.frontiersin.org/articles/10.3389/fmars.2020.00671/full#supplementary-material
Acevedo-Trejos, E., Brandt, G., Bruggeman, J., and Merico, A. (2015). Mechanisms shaping size structure and functional diversity of phytoplankton communities in the ocean. Sci. Rep. 5:8918. doi: 10.1038/srep08918
Acevedo-Trejos, E., Brandt, G., Merico, A., and Smith, S. L. (2013). Biogeographical patterns of phytoplankton community size structure in the oceans. Glob. Ecol. Biogeogr. 22, 1060–1070. doi: 10.1111/geb.12071
Acuña, J. L., López-Alvarez, M., Nogueira, E., and González-Taboada, F. (2010). Diatom flotation at the onset of the spring phytoplankton bloom: an in situ experiment. Mar. Ecol. Progr. Ser. 400, 115–125. doi: 10.3354/meps08405
Alderkamp, A.-C., Van Dijken, G. L., Lowry, K. E., Connelly, T. L., Lagerström, M., Sherrell, R. M., et al. (2015). Fe availability drives phytoplankton photosynthesis rates during spring bloom in the Amundsen Sea Polynya, Antarctica. Elementa Sci. Anthropocene 3:000043. doi: 10.12952/journal.elementa.000043
Ambrose, W. G., Quillfeldt, C. V., Clough, L. M., Tilney, P. V. R., and Tucker, T. (2005). The sub-ice algal community in the Chukchi sea: large- and small-scale patterns of abundance based on images from a remotely operated vehicle. Polar Biol. 28, 784–795. doi: 10.1007/s00300-005-0002-8
Ardyna, M., Babin, M., Gosselin, M., Devred, E., Bélanger, S., Matsuoka, A., et al. (2013). Parameterization of vertical chlorophyll a in the Arctic Ocean: impact of the subsurface chlorophyll maximum on regional, seasonal, and annual primary production estimates. Biogeosciences 10, 4383–4404. doi: 10.5194/bg-10-4383-2013
Ardyna, M., Claustre, H., Sallée, J.-B., D’Ovidio, F., Gentili, B., van Dijken, G., et al. (2017). Delineating environmental control of phytoplankton biomass and phenology in the Southern Ocean. Geophys. Res. Lett. 44, 5016–5024. doi: 10.1002/2016GL072428
Ardyna, M., Lacour, L., Sergi, S., d’Ovidio, F., Sallée, J.-B., Rembauville, M., et al. (2019). Hydrothermal vents trigger massive phytoplankton blooms in the Southern Ocean. Nat. Commun. 10:2451. doi: 10.1038/s41467-019-09973-6
Armand, L. K., Cornet-Barthaux, V., Mosseri, J., and Quéguiner, B. (2008a). Late summer diatom biomass and community structure on and around the naturally iron-fertilised Kerguelen Plateau in the Southern Ocean. Deep Sea Res. Part II Top. Stud. Oceanogr. 55, 653–676. doi: 10.1016/j.dsr2.2007.12.031
Armand, L. K., Crosta, X., Quéguiner, B., Mosseri, J., and Garcia, N. (2008b). Diatoms preserved in surface sediments of the northeastern Kerguelen Plateau. Deep Sea Res. Part II Top. Stud. Oceanogr. 55, 677–692. doi: 10.1016/j.dsr2.2007.12.032
Arteaga, L., Haëntjens, N., Boss, E., Johnson, K. S., and Sarmiento, J. L. (2018). Assessment of Export Efficiency Equations in the Southern Ocean Applied to Satellite-Based Net Primary Production. J. Geophys. Res. Oceans 123, 2945–2964. doi: 10.1002/2018jc013787
Arteaga, L., Pahlow, M., and Oschlies, A. (2014). Global patterns of phytoplankton nutrient and light colimitation inferred from an optimality-based model. Glob. Biogeochem. Cycles 28, 648–661. doi: 10.1002/2013GB004668
Arteaga, L. A., Pahlow, M., Bushinsky, S. M., and Sarmiento, J. L. (2019). Nutrient Controls on Export Production in the Southern Ocean. Glob. Biogeochem. Cycles 33, 942–956. doi: 10.1029/2019gb006236
Assmy, P., Smetacek, V., Montresor, M., Klaas, C., Henjes, J., Strass, V. H., et al. (2013). Thick-shelled, grazer-protected diatoms decouple ocean carbon and silicon cycles in the iron-limited Antarctic Circumpolar Current. Proc. Natl. Acad. Sci. U.S.A. 110, 20633–20638. doi: 10.1073/pnas.1309345110
Atkinson, A., Ward, P., Williams, R., and Poulet, S. A. (1992). Feeding rates and diel vertical migration of copepods near South Georgia: comparison of shelf and oceanic sites. Mar. Biol. 114, 49–56. doi: 10.1007/bf00350855
Aumont, O., Ethé, C., Tagliabue, A., Bopp, L., and Gehlen, M. (2015). PISCES-v2: an ocean biogeochemical model for carbon and ecosystem studies. Geosci. Model Dev. 8, 2465–2513. doi: 10.5194/gmd-8-2465-2015
Aumont, O., Maier-Reimer, E., Blain, S., and Monfray, P. (2003). An ecosystem model of the global ocean including Fe, Si, P colimitations. Glob. Biogeochem. Cycles 17:1060. doi: 10.1029/2001GB001745
Barbieux, M., Uitz, J., Gentili, B., Pasqueron de Fommervault, O., Mignot, A., Poteau, A., et al. (2019). Bio-optical characterization of subsurface chlorophyll maxima in the Mediterranean Sea from a Biogeochemical-Argo float database. Biogeosciences 16, 1321–1342. doi: 10.5194/bg-16-1321-2019
Bathmann, U. V., Scharek, R., Klaas, C., Dubischar, C. D., and Smetacek, V. (1997). Spring development of phytoplankton biomass and composition in major water masses of the Atlantic sector of the Southern Ocean. Deep-Sea Res. Part II Top. Stud. Oceanogr. 44, 51–67. doi: 10.1016/S0967-0645(96)00063-X
Behrenfeld, M. J., Boss, E., Siegel, D. A., and Shea, D. M. (2005). Carbon-based ocean productivity and phytoplankton physiology from space. Glob. Biogeochem. Cycles 19:GB1006. doi: 10.1029/2004gb002299
Behrenfeld, M. J., Westberry, T. K., Boss, E. S., O’Malley, R. T., Siegel, D. A., Wiggert, J. D., et al. (2009). Satellite-detected fluorescence reveals global physiology of ocean phytoplankton. Biogeosciences 6, 779–794. doi: 10.5194/bg-6-779-2009
Bergquist, B. A., and Boyle, E. A. (2006). Dissolved iron in the tropical and subtropical Atlantic Ocean. Glob. Biogeochem. Cycles 20:GB1015. doi: 10.1029/2005GB002505
Bertrand, E. M., Saito, M. A., Rose, J. M., Riesselman, C. R., Lohan, M. C., Noble, A. E., et al. (2007). Vitamin B12and iron colimitation of phytoplankton growth in the Ross Sea. Limnol. Oceanogr. 52, 1079–1093. doi: 10.4319/lo.2007.52.3.1079
Biermann, L., Guinet, C., Bester, M., Brierley, A., and Boehme, L. (2015). An alternative method for correcting fluorescence quenching. Ocean Sci. 11, 83–91. doi: 10.5194/os-11-83-2015
Blain, S., Quéguiner, B., Armand, L., Belviso, S., Bombled, B., Bopp, L., et al. (2007). Effect of natural iron fertilization on carbon sequestration in the Southern Ocean. Nature 446, 1070–1074. doi: 10.1038/nature05700
Blain, S., Sarthou, G., and Laan, P. (2008). Distribution of dissolved iron during the natural iron-fertilization experiment KEOPS (Kerguelen Plateau, Southern Ocean). Deep Sea Res. Part II Top. Stud. Oceanogr. 55, 594–605. doi: 10.1016/j.dsr2.2007.12.028
Blain, S., Tréguer, P., Belviso, S., Bucciarelli, E., Denis, M., Desabre, S., et al. (2001). A biogeochemical study of the island mass effect in the context of the iron hypothesis: kerguelen Islands, Southern Ocean. Deep Sea Res. Part I Top. Stud. Oceanogr. 48, 163–187. doi: 10.1016/S0967-0637(00)00047-9
Boetius, A., Albrecht, S., Bakker, K., Bienhold, C., Felden, J., Fernández-Méndez, M., et al. (2013). Export of Algal Biomass from the Melting Arctic Sea Ice. Science 339, 1430–1432. doi: 10.1126/science.1231346
Boss, E., Waite, A., Muller-Karger, F., Yamazaki, H., Wanninkhof, R., Uitz, J., et al. (2018). Beyond Chlorophyll Fluorescence: the Time is Right to Expand Biological Measurements in Ocean Observing Programs. Limnol. Oceanogr. Bull. 27, 89–90. doi: 10.1002/lob.10243
Bouvet, M., Hoepffner, N., and Dowell, M. D. (2002). Parameterization of a spectral solar irradiance model for the global ocean using multiple satellite sensors. J. Geophys. Res. Oceans 107:3215. doi: 10.1029/2001JC001126
Bowie, A. R., Van Der Merwe, P., Quéroué, F., Trull, T., Fourquez, M., Planchon, F., et al. (2015). Iron budgets for three distinct biogeochemical sites around the Kerguelen Archipelago (Southern Ocean) during the natural fertilisation study. KEOPS-2. Biogeosciences 12, 4421–4445. doi: 10.5194/bg-12-4421-2015
Boyd, P. W., Arrigo, K. R., Strzepek, R., and Van Dijken, G. (2012). Mapping phytoplankton iron utilization: insights into Southern Ocean supply mechanisms. J. Geophys. Res. Oceans 117:C06009. doi: 10.1029/2011JC007726
Boyd, P. W., Crossley, A. C., DiTullio, G. R., Griffiths, F. B., Hutchins, D. A., Queguiner, B., et al. (2001). Control of phytoplankton growth by iron supply and irradiance in the subantarctic Southern Ocean: experimental results from the SAZ Project. J. Geophys. Res. Oceans 106, 31573–31583. doi: 10.1029/2000jc000348
Boyd, P. W., Doney, S. C., Strzepek, R., Dusenberry, J., Lindsay, K., and Fung, I. (2008). Climate-mediated changes to mixed-layer properties in the Southern Ocean: assessing the phytoplankton response. Biogeosciences 5, 847–864. doi: 10.5194/bg-5-847-2008
Boyd, P. W., and Ellwood, M. J. (2010). The biogeochemical cycle of iron in the ocean. Nat. Geosci. 3, 675–682. doi: 10.1038/ngeo964
Boyd, P. W., Strzepek, R., Takeda, S., Jackson, G., Wong, C., McKay, R. M., et al. (2005). The evolution and termination of an iron-induced mesoscale bloom in the northeast subarctic Pacific. Limnol. Oceanogr. 50, 1872–1886. doi: 10.4319/lo.2005.50.6.1872
Brett, A., Leape, J., Abbott, M., Sakaguchi, H., Cao, L., Chand, K., et al. (2020). Ocean data need a sea change to help navigate the warming world. Nature 582, 181–183. doi: 10.1038/d41586-020-01668-z
Bryden, H. L., and Heath, R. A. (1985). Energetic eddies at the Northern edge of the Antarctic circumpolar current in the Southwest Pacific. Progr. Oceanogr. 14, 65–87. doi: 10.1016/0079-6611(85)90006-0
Buongiorno Nardelli, B., Guinehut, S., Verbrugge, N., Cotroneo, Y., Zambianchi, E., and Iudicone, D. (2017). Southern Ocean Mixed-Layer Seasonal and Interannual Variations From Combined Satellite and In Situ Data. J. Geophys. Res. Oceans 122, 10042–10060. doi: 10.1002/2017JC013314
Cailliau, C., Belviso, S., Goutx, M., Bedo, A., Park, Y., and Charriaud, E. (1999). Sedimentation pathways in the Indian sector of the Southern Ocean during a production regime dominated by regeneration. Mar. Ecol. Progr. Ser. 190, 53–67. doi: 10.3354/meps190053
Cailliau, C., Claustre, H., and Giannino, S. (1997). Chemotaxonomic analysis of phytoplankton distribution in the Indian sector of the Southern Ocean during late austral summer. Oceanol. Acta 20, 721–732.
Carranza, M. M., Gille, S. T., Franks, P. J. S., Johnson, K. S., Pinkel, R., and Girton, J. B. (2018). When Mixed Layers Are Not Mixed. Storm-Driven Mixing and Bio-optical Vertical Gradients in Mixed Layers of the Southern Ocean. J. Geophys. Res. Oceans 123, 7264–7289. doi: 10.1029/2018JC014416
Cermeño, P., and Falkowski, P. G. (2009). Controls on Diatom Biogeography in the Ocean. Science 325, 1539–1541. doi: 10.1126/science.1174159
Cheah, W., McMinn, A., Griffiths, F. B., Westwood, K. J., Wright, S. W., and Clementson, L. A. (2013). Response of Phytoplankton Photophysiology to Varying Environmental Conditions in the Sub-Antarctic and Polar Frontal Zone. PLoS One 8:e72165. doi: 10.1371/journal.pone.0072165
Chekalyuk, A., and Hafez, M. (2011). Photo-physiological variability in phytoplankton chlorophyll fluorescence and assessment of chlorophyll concentration. Optics Express 19, 22643–22658. doi: 10.1364/OE.19.022643
Chever, F., Sarthou, G., Bucciarelli, E., Blain, S., and Bowie, A. R. (2010). An iron budget during the natural iron fertilisation experiment KEOPS (Kerguelen Islands, Southern Ocean). Biogeosciences 7, 455–468. doi: 10.5194/bg-7-455-2010
Christaki, U., Obernosterer, I., Van Wambeke, F., Veldhuis, M., Garcia, N., and Catala, P. (2008). Microbial food web structure in a naturally iron-fertilized area in the Southern Ocean (Kerguelen Plateau). Deep Sea Res. Part II Top. Stud. Oceanogr. 55, 706–719. doi: 10.1016/j.dsr2.2007.12.009
Claustre, H., Johnson, K. S., and Takeshita, Y. (2020). Observing the Global Ocean with Biogeochemical-Argo. Ann. Rev. Mar. Sci. 12, 23–48. doi: 10.1146/annurev-marine-010419-010956
Clementson, L. A., Parslow, J. S., Griffiths, F. B., Lyne, V. D., Mackey, D. J., Harris, G. P., et al. (1998). Controls on phytoplankton production in the Australasian sector of the subtropical convergence. Deep Sea Res. Part I Oceanogr. Res. Pap. 45, 1627–1661. doi: 10.1016/S0967-0637(98)00035-1
Closset, I., Lasbleiz, M., Leblanc, K., Quéguiner, B., Cavagna, A. J., Elskens, M., et al. (2014). Seasonal evolution of net and regenerated silica production around a natural Fe-fertilized area in the Southern Ocean estimated with Si isotopic approaches. Biogeosciences 11, 5827–5846. doi: 10.5194/bg-11-5827-2014
Coale, K. H., Wang, X., Tanner, S. J., and Johnson, K. S. (2003). Phytoplankton growth and biological response to iron and zinc addition in the Ross Sea and Antarctic Circumpolar Current along 170°W. Deep Sea Res. Part II Top. Stud. Oceanogr. 50, 635–653. doi: 10.1016/s0967-0645(02)00588-x
Constable, A. J., Costa, D. P., Schofield, O., Newman, L., Urban, E. R., Fulton, E. A., et al. (2016). Developing priority variables (“ecosystem Essential Ocean Variables” — eEOVs) for observing dynamics and change in Southern Ocean ecosystems. J. Mar. Syst. 161, 26–41. doi: 10.1016/j.jmarsys.2016.05.003
Cullen, J. J. (1982). The deep chlorophyll maximum: comparing vertical profiles of chlorophyll a. Can. J. Fish. Aquat. Sci. 39, 791–803. doi: 10.1139/f82-108
Cullen, J. J. (2015). Subsurface Chlorophyll Maximum Layers: enduring Enigma or Mystery Solved? Ann. Rev. Mar. Sci. 7, 207–239. doi: 10.1146/annurev-marine-010213-135111
Daemen, E. (1986). Comparison of methods for the determination of chlorophyll in estuarine sediments. Netherlands J. Sea Res. 20, 21–28. doi: 10.1016/0077-7579(86)90057-8
Daly, K. L., Smith, W. O. Jr., Johnson, G. C., DiTullio, G. R., Jones, D. R., Mordy, C. W., et al. (2001). Hydrography, nutrients, and carbon pools in the Pacific sector of the Southern Ocean: implications for carbon flux. J. Geophys. Res. Oceans 106, 7107–7124. doi: 10.1029/1999jc000090
Davies, C. H., Ajani, P., Armbrecht, L., Atkins, N., Baird, M. E., Beard, J., et al. (2018). A database of chlorophyll a in Australian waters. Sci. Data 5:180018. doi: 10.1038/sdata.2018.18
Dawson, H. R. S., Strutton, P. G., and Gaube, P. (2018). The Unusual Surface Chlorophyll Signatures of Southern Ocean Eddies. J. Geophys. Res. Oceans 123, 6053–6069. doi: 10.1029/2017jc013628
de Boyer Montégut, C., Madec, G., Fischer, A. S., Lazar, A., and Iudicone, D. (2004). Mixed layer depth over the global ocean: an examination of profile data and a profile-based climatology. J. Geophys. Res. Oceans 109:C12003. doi: 10.1029/2004JC002378
de Villiers, S., Siswana, K., and Vena, K. (2015). In situ measurement of the biogeochemical properties of Southern Ocean mesoscale eddies in the Southwest Indian Ocean, April 2014. Earth Syst. Sci. Data 7, 415–422. doi: 10.5194/essd-7-415-2015
Death, R., Wadham, J. L., Monteiro, F., Le Brocq, A. M., Tranter, M., Ridgwell, A., et al. (2014). Antarctic ice sheet fertilises the Southern Ocean. Biogeosciences 11, 2635–2643. doi: 10.5194/bg-11-2635-2014
Demidov, A. B., Mosharov, S. A., Gagarin, V. I., and Mosharova, I. V. (2013). Vertical variability of primary production and chlorophyll a in the Drake Passage during the austral spring period (October–November). Moscow Univ. Biol. Sci. Bull. 68, 19–24. doi: 10.3103/S0096392513010021
Demidov, A. B., Vedernikov, V. I., and Sheberstov, S. V. (2007). Spatiotemporal variability of chlorophyll a in the Altantic and Indian sectors of the Southern Ocean during February-April of 2000 according to satellite and expeditionary data. Oceanology 47, 507–518. doi: 10.1134/s000143700704008x
Deppeler, S. L., and Davidson, A. T. (2017). Southern ocean phytoplankton in a changing climate. Front. Mar. Sci. 4:40. doi: 10.3389/fmars.2017.00040
Dierssen, H. M. (2010). Perspectives on empirical approaches for ocean color remote sensing of chlorophyll in a changing climate. Proc. Natl. Acad. Sci. U.S.A. 107, 17073–17078. doi: 10.1073/pnas.0913800107
Dinniman, M. S., Klinck, J. M., and Smith, W. O. (2011). A model study of Circumpolar Deep Water on the West Antarctic Peninsula and Ross Sea continental shelves. Deep Sea Res. Part II Top. Stud. Oceanogr. 58, 1508–1523. doi: 10.1016/j.dsr2.2010.11.013
Dinniman, M. S., St-Laurent, P., Arrigo, K. R., Hofmann, E. E., and van Dijken, G. L. (2020). Analysis of Iron Sources in Antarctic Continental Shelf Waters. J. Geophys. Res. Oceans 125:e2019JC015736. doi: 10.1029/2019jc015736
Doblin, M. A., Petrou, K. L., Shelly, K., Westwood, K., van den Enden, R., Wright, S., et al. (2011). Diel variation of chlorophyll-a fluorescence, phytoplankton pigments and productivity in the Sub-Antarctic and Polar Front Zones south of Tasmania. Australia. Deep Sea Res. Part II Top. Stud. Oceanogr. 58, 2189–2199. doi: 10.1016/j.dsr2.2011.05.021
Dong, S., Sprintall, J., Gille, S. T., and Talley, L. (2008). Southern Ocean mixed-layer depth from Argo float profiles. J. Geophys. Res. Oceans 113:C06013. doi: 10.1029/2006JC004051
Dragon, A.-C., Monestiez, P., Bar-Hen, A., and Guinet, C. (2010). Linking foraging behaviour to physical oceanographic structures: southern elephant seals and mesoscale eddies east of Kerguelen Islands. Progr. in Oceanogr. 87, 61–71. doi: 10.1016/j.pocean.2010.09.025
Duprat, L. P., Bigg, G. R., and Wilton, D. J. (2016). Enhanced Southern Ocean marine productivity due to fertilization by giant icebergs. Nat. Geosci. 9, 219–221. doi: 10.1038/ngeo2633
Dusenberry, J. A., Olson, R. J., and Chisholm, S. W. (2000). Field observations of oceanic mixed layer dynamics and picophytoplankton photoacclimation. J. Mar. Syst. 24, 221–232. doi: 10.1016/S0924-7963(99)00087-1
Edwards, K. F. (2019). Mixotrophy in nanoflagellates across environmental gradients in the ocean. Proc. Natl. Acad. Sci. U.S.A. 116, 6211–6220. doi: 10.1073/pnas.1814860116
Erickson, Z. K., Thompson, A. F., Cassar, N., Sprintall, J., and Mazloff, M. R. (2016). An advective mechanism for deep chlorophyll maxima formation in southern Drake Passage. Geophys. Res. Lett. 43, 10846–10855. doi: 10.1002/2016gl070565
Estrada, M., Delgado, M., Blasco, D., Latasa, M., Cabello, A. M., Benitez-Barrios, V., et al. (2016). Phytoplankton across Tropical and Subtropical Regions of the Atlantic, Indian and Pacific Oceans. PLoS One 11:e0151699. doi: 10.1371/journal.pone.0151699
Estrada, M., Marrasé, C., Latasa, M., Berdalet, E., Delgado, M., and Riera, T. (1993). Variability of deep chlorophyll maximum characteristics in the Northwestern Mediterranean. Mar. Ecol. Progr. Ser. 289–300. doi: 10.3354/meps092289
Fahrbach, E., Rohardt, G., and Krause, G. (1992). The Antarctic coastal current in the southeastern Weddell Sea. Polar Biol. 12, 171–182. doi: 10.1007/978-3-642-77595-6_19
Falkowski, P., and Kolber, Z. (1995). Variations in chlorophyll fluorescence yields in phytoplankton in the world oceans. Funct. Plant Biol. 22, 341–355. doi: 10.1071/PP9950341
Ferrario, M. E., Sar, E. A., and Vernet, M. (1998). Chaetoceros resting spores in the Gerlache Strait. Antarctic Peninsula. Polar Biol. 19, 286–288. doi: 10.1007/s003000050247
Fiala, M., Semeneh, M., and Oriol, L. (1998). Size-fractionated phytoplankton biomass and species composition in the Indian sector of the Southern Ocean during austral summer. J. Mar. Syst. 17, 179–194. doi: 10.1016/s0924-7963(98)00037-2
Fisher, A. E., and Harrison, P. J. (1996). Does carbohydrate content affect the sinking rates of marine diatoms?1. J. Phycol. 32, 360–365. doi: 10.1111/j.0022-3646.1996.00360.x
Fitch, D. T., and Moore, J. K. (2007). Wind speed influence on phytoplankton bloom dynamics in the Southern Ocean Marginal Ice Zone. J. Geophys. Res. Oceans 112:C08006. doi: 10.1029/2006jc004061
Fitzsimmons, J. N., Zhang, R., and Boyle, E. A. (2013). Dissolved iron in the tropical North Atlantic Ocean. Mar. Chem. 154, 87–99. doi: 10.1016/j.marchem.2013.05.009
Froneman, P., and Perissinotto, R. (1996). Microzooplankton grazing and protozooplankton community structure in the South Atlantic and in the Atlantic sector of the Southern Ocean. Deep Sea Res. Part I Oceanogr. Res. Pap. 43, 703–721. doi: 10.1016/0967-0637(96)00010-6
Furuya, K. (1990). Subsurface chlorophyll maximum in the tropical and subtropical Western Pacific-Ocean - vertical profiles of phytoplankton biomass and its relationship with chlorophyll-a and particulate organic-carbon. Mar. Biol. 107, 529–539. doi: 10.1007/bf01313438
Galbraith, E. D., Gnanadesikan, A., Dunne, J. P., and Hiscock, M. R. (2010). Regional impacts of iron-light colimitation in a global biogeochemical model. Biogeosciences 7, 1043–1064. doi: 10.5194/bg-7-1043-2010
Garibotti, I. A., Vernet, M., Ferrario, M. E., Smith, R. C., Ross, R. M., and Quetin, L. B. (2003). Phytoplankton spatial distribution patterns along the western Antarctic Peninsula (Southern Ocean). Mar. Ecol. Progr. Ser. 261, 21–39. doi: 10.3354/meps261021
Gemmell, B. J., Oh, G., Buskey, E. J., and Villareal, T. A. (2016). Dynamic sinking behaviour in marine phytoplankton: rapid changes in buoyancy may aid in nutrient uptake. Proc. R. Soc. B Biol. Sci. 283:20161126. doi: 10.1098/rspb.2016.1126
Goessling, J. W., Wardley, W. P., and Lopez-Garcia, M. (2020). Highly Reproducible. Bio-Based Slab Photonic Crystals Grown by Diatoms. Adv. Sci. 7:1903726. doi: 10.1002/advs.201903726
Gomi, Y., Fukuchi, M., and Taniguchi, A. (2010). Diatom assemblages at subsurface chlorophyll maximum layer in the eastern Indian sector of the Southern Ocean in summer. J. Plankton Res. 32, 1039–1050. doi: 10.1093/plankt/fbq031
Gomi, Y., Taniguchi, A., and Fukuchi, M. (2007). Temporal and spatial variation of the phytoplankton assemblage in the eastern Indian sector of the Southern Ocean in summer 2001/2002. Polar Biol. 30, 817–827. doi: 10.1007/s00300-006-0242-2
Gottschalk, J., Skinner, L. C., Lippold, J., Vogel, H., Frank, N., Jaccard, S. L., et al. (2016). Biological and physical controls in the Southern Ocean on past millennial-scale atmospheric CO2 changes. Nat. Commun. 7:11539. doi: 10.1038/ncomms11539
Graham, R. M., De Boer, A. M., van Sebille, E., Kohfeld, K. E., and Schlosser, C. (2015). Inferring source regions and supply mechanisms of iron in the Southern Ocean from satellite chlorophyll data. Deep Sea Res. Part I Oceanogr. Res. Pap. 104, 9–25. doi: 10.1016/j.dsr.2015.05.007
Grant, S., Constable, A., Raymond, B., and Doust, S. (2006). Bioregionalisation of the Southern Ocean:Report of Experts Workshop. Hobart: WWF-Australia Head Office.
Grenier, M., Della Penna, A., and Trull, T. W. (2015). Autonomous profiling float observations of the high-biomass plume downstream of the Kerguelen Plateau in the Southern Ocean. Biogeosciences 12, 2707–2735. doi: 10.5194/bg-12-2707-2015
Grossi, S. M., Kottmeier, S. T., Moe, R. L., Taylor, G. T., and Sullivan, C. W. (1987). Sea ice microbial communities. VI. Growth and primary production in bottom ice under graded snow cover. Mar. Ecol. Progr. Ser. 35, 153–164. doi: 10.3354/meps035153
Guinet, C., Xing, X., Walker, E., Monestiez, P., Marchand, S., Picard, B., et al. (2013). Calibration procedures and first dataset of Southern Ocean chlorophyll a profiles collected by elephant seals equipped with a newly developed CTD-fluorescence tags. Earth Syst. Sci. Data 5, 15–29. doi: 10.5194/essd-5-15-2013
Haëntjens, N., Boss, E., and Talley, L. D. (2017). Revisiting Ocean Color algorithms for chlorophyll a and particulate organic carbon in the Southern Ocean using biogeochemical floats. J. Geophys. Res. Oceans 122, 6583–6593. doi: 10.1002/2017jc012844
Hansen, A. N., and Visser, A. W. (2019). The seasonal succession of optimal diatom traits. Limnol. Oceanogr. 64, 1442–1457. doi: 10.1002/lno.11126
Herbland, A., and Voituriez, B. (1979). Hydrological structure analysis for estimating the primary production in the tropical Atlantic Ocean. J. Mar. Res. 37, 87–101.
Holligan, P. M., Pingree, R. D., and Mardell, G. T. (1985). Oceanic solitons, nutrient pulses and phytoplankton growth. Nature 314, 348–350. doi: 10.1038/314348a0
Holm-Hansen, O., and Hewes, C. D. (2004). Deep chlorophyll-a maxima (DCMs) in Antarctic waters - I. Relationships between DCMs and the physical, chemical, and optical conditions in the upper water column. Polar Biol. 27, 699–710. doi: 10.1007/s00300-004-0641-1
Holm-Hansen, O., Kahru, M., and Hewes, C. D. (2005). Deep chlorophyll a maxima (DCMs) in pelagic Antarctic waters. II. Relation to bathymetric features and dissolved iron concentrations. Mar. Ecol. Progr. Ser. 297, 71–81. doi: 10.3354/meps297071
Holm-Hansen, O., Lorenzen, C. J., Holmes, R. W., and Strickland, J. D. (1965). Fluorometric determination of chlorophyll. ICES J. Mar. Sci. 30, 3–15. doi: 10.1093/icesjms/30.1.3
Hopkinson, B. M., Mitchell, B., Greg, R., Rick, A., Wang, H., Selph, K. E., et al. (2007). Iron limitation across chlorophyll gradients in the southern Drake Passage: phytoplankton responses to iron addition and photosynthetic indicators of iron stress. Limnol. Oceanogr. 52, 2540–2554. doi: 10.4319/lo.2007.52.6.2540
Johnson, R., Strutton, P. G., Wright, S. W., McMinn, A., and Meiners, K. M. (2013). Three improved satellite chlorophyll algorithms for the Southern Ocean. J. Geophys. Res. Oceans 118, 3694–3703. doi: 10.1002/jgrc.20270
Kahl, L. A., Schofield, O., and Fraser, W. R. (2010). Autonomous Gliders Reveal Features of the Water Column Associated with Foraging by Adélie Penguins. Integr. Compar. Biol. 50, 1041–1050. doi: 10.1093/icb/icq098
Kara, A. B., Rochford, P. A., and Hurlburt, H. E. (2003). Mixed layer depth variability over the global ocean. J. Geophys. Res. Oceans 108:3079. doi: 10.1029/2000JC000736
Kemp, A. E. S., Pearce, R. B., Grigorov, I., Rance, J., Lange, C. B., Quilty, P., et al. (2006). Production of giant marine diatoms and their export at oceanic frontal zones: implications for Si and C flux from stratified oceans. Glob. Biogeochem. Cycles 20:GB4S04. doi: 10.1029/2006gb002698
Kemp, A. E. S., Pike, J., Pearce, R. B., and Lange, C. B. (2000). The “Fall dump” — a new perspective on the role of a “shade flora” in the annual cycle of diatom production and export flux. Deep Sea Res. Part II Top. Stud. Oceanogr. 47, 2129–2154. doi: 10.1016/S0967-0645(00)00019-9
Kemp, A. E. S., and Villareal, T. A. (2013). High diatom production and export in stratified waters – A potential negative feedback to global warming. Progr. Oceanogr. 119, 4–23. doi: 10.1016/j.pocean.2013.06.004
Kemp, A. E. S., and Villareal, T. A. (2018). The case of the diatoms and the muddled mandalas: time to recognize diatom adaptations to stratified waters. Progr. Oceanogr. 167, 138–149. doi: 10.1016/j.pocean.2018.08.002
Klunder, M. B., Laan, P., De Baar, H. J. W., Middag, R., Neven, I., and Van Ooijen, J. (2014). Dissolved Fe across the Weddell Sea and Drake Passage: impact of DFe on nutrient uptake. Biogeosciences 11, 651–669. doi: 10.5194/bg-11-651-2014
Kopczynska, E. E., Dehairs, F., Elskens, M., and Wright, S. (2001). Phytoplankton and microzooplankton variability between the Subtropical and Polar Fronts south of Australia: thriving under regenerative and new production in late summer. J. Geophys. Res. Oceans 106, 31597–31609. doi: 10.1029/2000jc000278
Lancelot, C., Mathot, S., Veth, C., and de Baar, H. (1993). Factors controlling phytoplankton ice-edge blooms in the marginal ice-zone of the northwestern Weddell Sea during sea ice retreat 1988: field observations and mathematical modelling. Polar Biol. 13, 377–387. doi: 10.1007/bf01681979
Lannuzel, D., Vancoppenolle, M., Merwe, P., van der Jong, J., de Meiners, K. M., et al. (2016). Iron in sea ice: review and new insights. Elemental Sci. Anthropol. 4:000130. doi: 10.12952/journal.elementa.000130
Latasa, M., Cabello, A. M., Morán, X. A. G., Massana, R., and Scharek, R. (2017). Distribution of phytoplankton groups within the deep chlorophyll maximum. Limnol. Oceanogr. 62, 665–685. doi: 10.1002/lno.10452
Latasa, M., Gutiérrez-Rodríguez, A., Cabello, A. M., and Scharek, R. (2016). Influence of light and nutrients on the vertical distribution of marine phytoplankton groups in the deep chlorophyll maximum. Sci. Mar. 80, 57–62. doi: 10.3989/scimar.04316.01A
Lavigne, H., D’Ortenzio, F., Ribera, D., Alcalà, M., Claustre, H., Sauzède, R., et al. (2015). On the vertical distribution of the chlorophyll a concentration in the Mediterranean Sea: a basin-scale and seasonal approach. Biogeosciences 12, 5021–5039. doi: 10.5194/bg-12-5021-2015
Lee, Z., Marra, J., Perry, M. J., and Kahru, M. (2015). Estimating oceanic primary productivity from ocean color remote sensing: a strategic assessment. J. Mar. Syst. 149, 50–59. doi: 10.1016/j.jmarsys.2014.11.015
Litchman, E., Klausmeier, C. A., Miller, J. R., Schofield, O. M., and Falkowski, P. G. (2006). Multi-nutrient, multi-group model of present and future oceanic phytoplankton communities. Biogeosciences 3, 585–606. doi: 10.5194/bg-3-585-2006
Lizotte, M. P. (2015). The Contributions of Sea Ice Algae to Antarctic Marine Primary Production1. Am. Zool. 41, 57–73. doi: 10.1093/icb/41.1.57
Llort, J., Langlais, C., Matear, R., Moreau, S., Lenton, A., and Strutton, P. G. (2018). Evaluating Southern Ocean Carbon Eddy-Pump From Biogeochemical-Argo Floats. J. Geophys. Res. Oceans 123, 971–984. doi: 10.1002/2017jc012861
Lomas, M. W., and Glibert, P. M. (2000). Comparisons Of Nitrate Uptake, Storage, And Reduction In Marine Diatoms And Flagellates. J. Phycol. 36, 903–913. doi: 10.1046/j.1529-8817.2000.99029.x
Louanchi, F., and Najjar, R. G. (2000). A global monthly climatology of phosphate, nitrate, and silicate in the upper ocean: spring-summer export production and shallow remineralization. Glob. Biogeochem. Cycles 14, 957–977. doi: 10.1029/1999gb001215
Ma, S., Tao, Z., Yang, X., Yu, Y., Zhou, X., Ma, W., et al. (2014). Estimation of Marine Primary Productivity From Satellite-Derived Phytoplankton Absorption Data. IEEE J. Select. Top. Appl. Earth Observ. Remote Sens. 7, 3084–3092. doi: 10.1109/JSTARS.2014.2298863
Mangoni, O., Saggiomo, M., Modigh, M., Catalano, G., Zingone, A., and Saggiomo, V. (2009). The role of platelet ice microalgae in seeding phytoplankton blooms in Terra Nova Bay (Ross Sea, Antarctica): a mesocosm experiment. Polar Biol. 32, 311–323. doi: 10.1007/s00300-008-0507-z
Martin, J. H., Fitzwater, S. E., and Gordon, R. M. (1990). Iron deficiency limits phytoplankton growth in Antarctic waters. Glob. Biogeochem. Cycles 4, 5–12. doi: 10.1029/gb004i001p00005
McClain, C. R. (2009). A decade of satellite ocean color observations. Ann. Rev. Mar. Sci. 1, 19–42. doi: 10.1146/annurev.marine.010908.163650
McKie-Krisberg, Z. M., Gast, R. J., and Sanders, R. W. (2015). Physiological Responses of Three Species of Antarctic Mixotrophic Phytoflagellates to Changes in Light and Dissolved Nutrients. Microb. Ecol. 70, 21–29. doi: 10.1007/s00248-014-0543-x
Michel, C., Legendre, L., Ingram, R. G., Gosselin, M., and Levasseur, M. (1996). Carbon budget of sea-ice algae in spring: evidence of a significant transfer to zooplankton grazers. J. Geophys. Res. Oceans 101, 18345–18360. doi: 10.1029/96jc00045
Mikaelyan, A. S., and Belyaeva, G. A. (1995). Chlorophyll “a” content in cells of Antarctic phytoplankton. Polar Biol. 15, 437–445. doi: 10.1007/BF00239721
Mitchell, B. G., and Holm-Hansen, O. (1991). Bio-optical properties of Antarctic Peninsula waters: differentiation from temperate ocean models. Deep Sea Res. Part A Oceanogr. Res. Pap. 38, 1009–1028. doi: 10.1016/0198-0149(91)90094-V
Moeller, H. V., Laufkötter, C., Sweeney, E. M., and Johnson, M. D. (2019). Light-dependent grazing can drive formation and deepening of deep chlorophyll maxima. Nat. Commun. 10:1978. doi: 10.1038/s41467-019-09591-2
Mongin, M., Nelson, D. M., Pondaven, P., and Tréguer, P. (2007). Potential phytoplankton responses to iron and stratification changes in the Southern Ocean based on a flexible-composition phytoplankton model. Glob. Biogeochem. Cycles 21:GB4020. doi: 10.1029/2007gb002972
Moore, C. M., Mills, M. M., Arrigo, K. R., Berman-Frank, I., Bopp, L., Boyd, P. W., et al. (2013). Processes and patterns of oceanic nutrient limitation. Nat. Geosci. 6, 701–710. doi: 10.1038/ngeo1765
Moore, J. K., Doney, S. C., Glover, D. M., and Fung, I. Y. (2001). Iron cycling and nutrient-limitation patterns in surface waters of the World Ocean. Deep Sea Res. Part II Top. Stud. Oceanogr. 49, 463–507. doi: 10.1016/S0967-0645(01)00109-6
Moore, J. K., and Villareal, T. A. (1996). Buoyancy and growth characteristics of three positively buoyant marine diatoms. Mar. Ecol. Progr. Ser. 132, 203–213. doi: 10.3354/meps132203
Moorthi, S., Caron, D. A., Gast, R. J., and Sanders, R. W. (2009). Mixotrophy: a widespread and important ecological strategy for planktonic and sea-ice nanoflagellates in the Ross Sea. Antarctica. Aquat. Microb. Ecol. 54, 269–277. doi: 10.3354/ame01276
Mtshali, T. N., van Horsten, N. R., Thomalla, S. J., Ryan-Keogh, T. J., Nicholson, S. A., Roychoudhury, A. N., et al. (2019). Seasonal Depletion of the Dissolved Iron Reservoirs in the Sub-Antarctic Zone of the Southern Atlantic Ocean. Geophys. Res. Lett. 46, 4386–4395. doi: 10.1029/2018GL081355
Murray, A., Gibbs, C., Longmore, A., and Flett, D. (1986). Determination of chlorophyll in marine waters: intercomparison of a rapid HPLC method with full HPLC, spectrophotometric and fluorometric methods. Mar. Chem. 19, 211–227. doi: 10.1016/0304-4203(86)90024-1
Norbury, J., Moroz, I. M., and Cropp, R. (2019). The Role of Mixotrophy in Southern Ocean Ecosystems. Environ. Model. Assess. 24, 421–435. doi: 10.1007/s10666-019-09670-0
Ostrowska, M., Woźniak, B., and Dera, J. (2012). Modelled quantum yields and energy efficiency of fluorescence, photosynthesis and heat production by phytoplankton in the World Ocean. Oceanologia 54, 565–610. doi: 10.5697/oc.54-4.565
Parekh, P., Follows, M. J., and Boyle, E. (2004). Modeling the global ocean iron cycle. Glob. Biogeochem. Cycles 18, GB1002. doi: 10.1029/2003GB002061
Park, Y.-H., Durand, I., Kestenare, E., Rougier, G., Zhou, M., d’Ovidio, F., et al. (2014). Polar Front around the Kerguelen Islands: an up-to-date determination and associated circulation of surface/subsurface waters. J. Geophys. Res. Oceans 119, 6575–6592. doi: 10.1002/2014jc010061
Parslow, J. S., Boyd, P. W., Rintoul, S. R., and Griffiths, F. B. (2001). A persistent subsurface chlorophyll maximum in the Interpolar Frontal Zone south of Australia: seasonal progression and implications for phytoplankton-light-nutrient interactions. J. Geophys. Res. Oceans 106, 31543–31557. doi: 10.1029/2000jc000322
Pellichero, V., Boutin, J., Claustre, H., Merlivat, L., Sallée, J.-B., and Blain, S. (2020). Relaxation of wind stress drives the abrupt onset of biological carbon uptake in the kerguelen bloom: a multisensor approach. Geophys. Res. Lett. 47:e2019GL085992. doi: 10.1029/2019gl085992
Pinckney, J., Papa, R., and Zingmark, R. (1994). Comparison of high-performance liquid chromatographic, spectrophotometric, and fluorometric methods for determining chlorophyll a concentrations in estaurine sediments. J. Microbiol. Methods 19, 59–66. doi: 10.1016/0167-7012(94)90026-4
Planquette, H., Statham, P. J., Fones, G., Charette, M. A., Moore, C. M., Salter, I., et al. (2007). Dissolved iron in the vicinity of the Crozet Islands, Southern Ocean. Deep Sea Res. Part II Top. Stud. Oceanogr. 54, 1999–2019. doi: 10.1016/j.dsr2.2007.06.019
Quéguiner, B. (2001). Biogenic silica production in the Australian sector of the Subantarctic Zone of the Southern Ocean in late summer 1998. J. Geophys. Res. Oceans 106, 31627–31636. doi: 10.1029/2000JC000249
Quilty, P., Kerry, K., and Marchant, H. (1985). A seasonally recurrent patch of Antarctic planktonic diatoms. Search 16, 48–51.
Raiswell, R., Benning, L. G., Tranter, M., and Tulaczyk, S. (2008). Bioavailable iron in the Southern Ocean: the significance of the iceberg conveyor belt. Geochem. Trans. 9:7. doi: 10.1186/1467-4866-9-7
Rembauville, M., Blain, S., Caparros, J., and Salter, I. (2016a). Particulate matter stoichiometry driven by microplankton community structure in summer in the Indian sector of the Southern Ocean. Limnol. Oceanogr. 61, 1301–1321. doi: 10.1002/lno.10291
Rembauville, M., Manno, C., Tarling, G., Blain, S., and Salter, I. (2016b). Strong contribution of diatom resting spores to deep-sea carbon transfer in naturally iron-fertilized waters downstream of South Georgia. Deep Sea Res. Part I Oceanogr. Res. Pap. 115, 22–35. doi: 10.1016/j.dsr.2016.05.002
Rigual-Hernández, A. S., Trull, T. W., Bray, S. G., Cortina, A., and Armand, L. K. (2015a). Latitudinal and temporal distributions of diatom populations in the pelagic waters of the Subantarctic and Polar Frontal zones of the Southern Ocean and their role in the biological pump. Biogeosciences 12, 5309–5337. doi: 10.5194/bg-12-5309-2015
Rigual-Hernández, A. S., Trull, T. W., Bray, S. G., Closset, I., and Armand, L. K. (2015b). Seasonal dynamics in diatom and particulate export fluxes to the deep sea in the Australian sector of the southern Antarctic Zone. J. Mar. Syst. 142, 62–74. doi: 10.1016/j.jmarsys.2014.10.002
Rivière, P., Jaud, T., Siegelman, L., Klein, P., Cotté, C., Le Sommer, J., et al. (2019). Sub-mesoscale fronts modify elephant seals foraging behavior. Limnol. Oceanogr. Lett. 4, 193–204. doi: 10.1002/lol2.10121
Robinson, J., Popova, E. E., Srokosz, M. A., and Yool, A. (2016). A tale of three islands: downstream natural iron fertilization in the Southern Ocean. J. Geophys. Res. Oceans 121, 3350–3371. doi: 10.1002/2015jc011319
Roesler, C., Uitz, J., Claustre, H., Boss, E., Xing, X., Organelli, E., et al. (2017). Recommendations for obtaining unbiased chlorophyll estimates from in situ chlorophyll fluorometers: a global analysis of WET Labs ECO sensors. Limnol. Oceanogr. 15, 572–585. doi: 10.1002/lom3.10185
Saijo, D., Mitani, Y., Abe, T., Sasaki, H., Goetsch, C., Costa, D. P., et al. (2017). Linking mesopelagic prey abundance and distribution to the foraging behavior of a deep-diving predator, the northern elephant seal. Deep Sea Res. Part II Top. Stud. Oceanogr. 140, 163–170. doi: 10.1016/j.dsr2.2016.11.007
Salter, I., Kemp, A. E. S., Moore, C. M., Lampitt, R. S., Wolff, G. A., and Holtvoeth, J. (2012). Diatom resting spore ecology drives enhanced carbon export from a naturally iron-fertilized bloom in the Southern Ocean. Glob. Biogeochem. Cycles 26:GB1014. doi: 10.1029/2010GB003977
Schallenberg, C., Harley, J. W., Jansen, P., Davies, D. M., and Trull, T. W. (2019). Multi-Year Observations of Fluorescence and Backscatter at the Southern Ocean Time Series (SOTS) shed light on two distinct seasonal bio-optical regimes. Front. Mar. Sci. 6:595. doi: 10.3389/fmars.2019.00595
Schallenberg, C., Strzepek, R. F., Schuback, N., Clementson, L. A., Boyd, P. W., and Trull, T. W. (2020). Diel quenching of Southern Ocean phytoplankton fluorescence is related to iron limitation. Biogeosciences 17, 793–812. doi: 10.5194/bg-17-793-2020
Scharek, R., Smetacek, V., Fahrbach, E., Gordon, L. I., Rohardt, G., and Moore, S. (1994). The transition from winter to early spring in the eastern Weddell Sea, Antarctica: plankton biomass and composition in relation to hydrography and nutrients. Deep Sea Res. Part I Oceanogr. Res. Pap. 41, 1231–1250. doi: 10.1016/0967-0637(94)90042-6
Schlosser, C., Schmidt, K., Aquilina, A., Homoky, W. B., Castrillejo, M., Mills, R. A., et al. (2018). Mechanisms of dissolved and labile particulate iron supply to shelf waters and phytoplankton blooms off South Georgia. Southern Ocean. Biogeosciences 15, 4973–4993. doi: 10.5194/bg-15-4973-2018
Shoaf, W. T. (1978). Rapid method for the separation of chlorophylls a and b by high-pressure liquid chromatography. J. Chromatogr. A 152, 247–249. doi: 10.1016/S0021-9673(00)85360-1
Siegel, D. A., Buesseler, K. O., Doney, S. C., Sailley, S. F., Behrenfeld, M. J., and Boyd, P. W. (2014). Global assessment of ocean carbon export by combining satellite observations and food-web models. Glob. Biogeochem. Cycles 28, 181–196. doi: 10.1002/2013gb004743
Siegelman, L., O’Toole, M., Flexas, M., Rivière, P., and Klein, P. (2019). Submesoscale ocean fronts act as biological hotspot for southern elephant seal. Sci. Rep. 9:5588. doi: 10.1038/s41598-019-42117-w
Smetacek, V., Assmy, P., and Henjes, J. (2004). The role of grazing in structuring Southern Ocean pelagic ecosystems and biogeochemical cycles. Antarctic Sci. 16, 541–558. doi: 10.1017/S0954102004002317
Smetacek, V., Scharek, R., Gordon, L. I., Eicken, H., Fahrbach, E., Rohardt, G., et al. (1992). Early spring phytoplankton blooms in ice platelet layers of the southern Weddell Sea, Antarctica. Deep Sea Res. Part A Oceanogr. Res. Pap. 39, 153–168. doi: 10.1016/0198-0149(92)90102-Y
Smetacek, V., Scharek, R., and Nöthig, E. M. (1990). “Seasonal and regional variation in the pelagial and its relationship to the life history cycle of krill,” in Antarctic Ecosystems, eds K. R. Kerry and G. Hempel (Berlin: Springer), doi: 10.1007/978-3-642-84074-6_10
Smetacek, V. S. (1985). Role of sinking in diatom life-history cycles: ecological, evolutionary and geological significance. Mar. Biol. 84, 239–251. doi: 10.1007/BF00392493
Sohrin, Y., Iwamoto, S., Matsui, M., Obata, H., Nakayama, E., Suzuki, K., et al. (2000). The distribution of Fe in the Australian sector of the Southern Ocean. Deep Sea Res. Part I Oceanogr. Res. Pap. 47, 55–84. doi: 10.1016/S0967-0637(99)00049-7
Soppa, M. A., Hirata, T., Silva, B., Dinter, T., Peeken, I., Wiegmann, S., et al. (2014). Global Retrieval of Diatom Abundance Based on Phytoplankton Pigments and Satellite Data. Remote Sens. 6, 10089–10106. doi: 10.3390/rs61010089
Stewart, A. L., and Thompson, A. F. (2013). Connecting Antarctic Cross-Slope Exchange with Southern Ocean Overturning. J. Phys. Oceanogr. 43, 1453–1471. doi: 10.1175/jpo-d-12-0205.1
Stretch, J. J., Hamner, P. P., Hamner, W. M., Michel, W. C., Cook, J., and Sullivan, C. W. (1988). Foraging behavior of Antarctic krill Euphausia superba on sea ice microalgae. Mar. Ecol. Progr. Ser. 44, 131–139. doi: 10.3354/meps044131
Strickland, J. D., and Parsons, T. R. (1972). A Practical Handbook of Seawater Analysis. Ottawa: Fisheries Research Board of Canada.
Strom, S. L. (2001). Light-aided digestion, grazing and growth in herbivorous protists. Aquat. Microb. Ecol. 23, 253–261. doi: 10.3354/ame023253
Strzepek, R. F., Boyd, P. W., and Sunda, W. G. (2019). Photosynthetic adaptation to low iron, light, and temperature in Southern Ocean phytoplankton. Proc. Natl. Acad. Sci. U.S.A. 116, 4388–4393. doi: 10.1073/pnas.1810886116
Sullivan, C., Arrigo, K., McClain, C., Comiso, J., and Firestone, J. (1993). Distributions of phytoplankton blooms in the Southern Ocean. Science 262, 1832–1837. doi: 10.1126/science.262.5141.1832
Suzuki, H., Sasaki, H., and Fukuchi, M. (2001). Short-term variability in the flux of rapidly sinking particles in the Antarctic marginal ice zone. Polar Biol. 24, 697–705. doi: 10.1007/s003000100271
Tagliabue, A., Mtshali, T., Aumont, O., Bowie, A. R., Klunder, M. B., Roychoudhury, A. N., et al. (2012). A global compilation of dissolved iron measurements: focus on distributions and processes in the Southern Ocean. Biogeosciences 9, 2333–2349. doi: 10.5194/bg-9-2333-2012
Tagliabue, A., Sallée, J.-B., Bowie, A. R., Lévy, M., Swart, S., and Boyd, P. W. (2014). Surface-water iron supplies in the Southern Ocean sustained by deep winter mixing. Nat. Geosci. 7:314. doi: 10.1038/ngeo2101
Taylor, M. H., Losch, M., and Bracher, A. (2013). On the drivers of phytoplankton blooms in the Antarctic marginal ice zone: a modeling approach. J. Geophys. Res. Oceans 118, 63–75. doi: 10.1029/2012jc008418
Thomalla, S. J., Moutier, W., Ryan-Keogh, T. J., Gregor, L., and Schütt, J. (2018). An optimized method for correcting fluorescence quenching using optical backscattering on autonomous platforms. Limnol. Oceanogr. 16, 132–144. doi: 10.1002/lom3.10234
Thomalla, S. J., Ogunkoya, A. G., Vichi, M., and Swart, S. (2017). Using Optical Sensors on Gliders to Estimate Phytoplankton Carbon Concentrations and Chlorophyll-to-Carbon Ratios in the Southern Ocean. Front. Mar. Sci. 4:34. doi: 10.3389/fmars.2017.00034
Tréguer, P., Bowler, C., Moriceau, B., Dutkiewicz, S., Gehlen, M., Aumont, O., et al. (2018). Influence of diatom diversity on the ocean biological carbon pump. Nat. Geosci. 11, 27–37. doi: 10.1038/s41561-017-0028-x
Tripathy, S., Pavithran, S., Sabu, P., Naik, R., Noronha, S., Bhaskar, P., et al. (2014). Is primary productivity in the Indian Ocean sector of Southern Ocean affected by pigment packaging effect? Curr. Sci. 107, 1019–1026.
Tripathy, S. C., Pavithran, S., Sabu, P., Pillai, H. U. K., Dessai, D. R. G., and Anilkumar, N. (2015). Deep chlorophyll maximum and primary productivity in Indian Ocean sector of the Southern Ocean: case study in the Subtropical and Polar Front during austral summer 2011. Deep-Sea Res. Part II Top. Stud. Oceanogr. 118, 240–249. doi: 10.1016/j.dsr2.2015.01.004
Tyrrell, T. (1999). The relative influences of nitrogen and phosphorus on oceanic primary production. Nature 400, 525–531. doi: 10.1038/22941
Uitz, J., Claustre, H., Morel, A., and Hooker, S. B. (2006). Vertical distribution of phytoplankton communities in open ocean: an assessment based on surface chlorophyll. J. Geophys. Res. Oceans 111:C08005. doi: 10.1029/2005JC003207
Valente, A., Sathyendranath, S., Brotas, V., Groom, S., Grant, M., Taberner, M., et al. (2016). A compilation of global bio-optical in situ data for ocean-colour satellite applications. Earth Syst. Sci. Data 8, 235–252. doi: 10.5194/essd-8-235-2016
Venables, H., and Moore, C. M. (2010). Phytoplankton and light limitation in the Southern Ocean: learning from high-nutrient, high-chlorophyll areas. J. Geophys. Res. Oceans 115:C02015. doi: 10.1029/2009JC005361
Verdy, A., and Mazloff, M. R. (2017). A data assimilating model for estimating Southern Ocean biogeochemistry. J. Geophys. Res. Oceans 122, 6968–6988. doi: 10.1002/2016jc012650
Villareal, T. A. (1988). Positive buoyancy in the oceanic diatom Rhizosolenia debyana H. Peragallo. Deep Sea Res. Part A Oceanogr. Res. Papers 35, 1037–1045. doi: 10.1016/0198-0149(88)90075-1
Waite, A. M., and Nodder, S. D. (2001). The effect of in situ iron addition on the sinking rates and export flux of Southern Ocean diatoms. Deep Sea Res. Part II Top. Stud. Oceanogr. 48, 2635–2654. doi: 10.1016/S0967-0645(01)00012-1
Waite, A. M., Thompson, P. A., and Harrison, P. J. (1992). Does energy control the sinking rates of marine diatoms? Limnol. Oceanogr. 37, 468–477. doi: 10.4319/lo.1992.37.3.0468
Westwood, K. J., Griffiths, F. B., Webb, J. P., and Wright, S. W. (2011). Primary production in the Sub-Antarctic and Polar Frontal Zones south of Tasmania. Australia; SAZ-Sense survey, 2007. Deep-Sea Res. Part II Top. Stud. Oceanogr. 58, 2162–2178. doi: 10.1016/j.dsr2.2011.05.017
Whitehouse, M. J., Korb, R. E., Atkinson, A., Thorpe, S. E., and Gordon, M. (2008). Formation, transport and decay of an intense phytoplankton bloom within the High-Nutrient Low-Chlorophyll belt of the Southern Ocean. J. Mar. Syst. 70, 150–167. doi: 10.1016/j.jmarsys.2007.05.003
Williams, G. D., Hindell, M., Houssais, M. N., Tamura, T., and Field, I. C. (2011). Upper ocean stratification and sea ice growth rates during the summer-fall transition, as revealed by Elephant seal foraging in the Adélie Depression, East Antarctica. Ocean Sci. 7, 185–202. doi: 10.5194/os-7-185-2011
Wright, S. W., and van den Enden, R. L. (2000). Phytoplankton community structure and stocks in the East Antarctic marginal ice zone (BROKE survey, January-March 1996) determined by CHEMTAX analysis of HPLC pigment signatures. Deep Sea Res. Part II Top. Stud. Oceanogr. 47, 2363–2400. doi: 10.1016/s0967-0645(00)00029-1
Wright, S. W., van den Enden, R. L., Pearce, I., Davidson, A. T., Scott, F. J., and Westwood, K. J. (2010). Phytoplankton community structure and stocks in the Southern Ocean (30–80°E) determined by CHEMTAX analysis of HPLC pigment signatures. Deep Sea Res. Part II Topic. Stud. Oceanogr. 57, 758–778. doi: 10.1016/j.dsr2.2009.06.015
Xing, X., Briggs, N., Boss, E., and Claustre, H. (2018). Improved correction for non-photochemical quenching of in situ chlorophyll fluorescence based on a synchronous irradiance profile. Optics Express 26, 24734–24751. doi: 10.1364/OE.26.024734
Yentsch, C. S., and Menzel, D. W. (1963). A method for the determination of phytoplankton chlorophyll and phaeophytin by fluorescence. Deep Sea Res. Oceanogr. Abstracts 10, 221–231. doi: 10.1016/0011-7471(63)90358-9
Yoder, J. A., McClain, C. R., Feldman, G. C., and Esaias, W. E. (1993). Annual cycles of phytoplankton chlorophyll concentrations in the global ocean: a satellite view. Glob. Biogeochem. Cycles 7, 181–193. doi: 10.1029/93gb02358
Keywords: Southern Ocean, phytoplankton, chlorophyll-a, chlorophyll fluorescence, subsurface chlorophyll maxima
Citation: Baldry K, Strutton PG, Hill NA and Boyd PW (2020) Subsurface Chlorophyll-a Maxima in the Southern Ocean. Front. Mar. Sci. 7:671. doi: 10.3389/fmars.2020.00671
Received: 30 March 2020; Accepted: 22 July 2020;
Published: 14 August 2020.
Edited by:
Anne Babcock Hollowed, National Oceanic and Atmospheric Administration (NOAA), United StatesReviewed by:
Lisa Eisner, Alaska Fisheries Science Center (NOAA), United StatesCopyright © 2020 Baldry, Strutton, Hill and Boyd. This is an open-access article distributed under the terms of the Creative Commons Attribution License (CC BY). The use, distribution or reproduction in other forums is permitted, provided the original author(s) and the copyright owner(s) are credited and that the original publication in this journal is cited, in accordance with accepted academic practice. No use, distribution or reproduction is permitted which does not comply with these terms.
*Correspondence: Kimberlee Baldry, a2ltYmVybGVlLmJhbGRyeUB1dGFzLmVkdS5hdQ==; YmFsZHJ5LmtpbWJlcmxlZUBnbWFpbC5jb20=
Disclaimer: All claims expressed in this article are solely those of the authors and do not necessarily represent those of their affiliated organizations, or those of the publisher, the editors and the reviewers. Any product that may be evaluated in this article or claim that may be made by its manufacturer is not guaranteed or endorsed by the publisher.
Research integrity at Frontiers
Learn more about the work of our research integrity team to safeguard the quality of each article we publish.