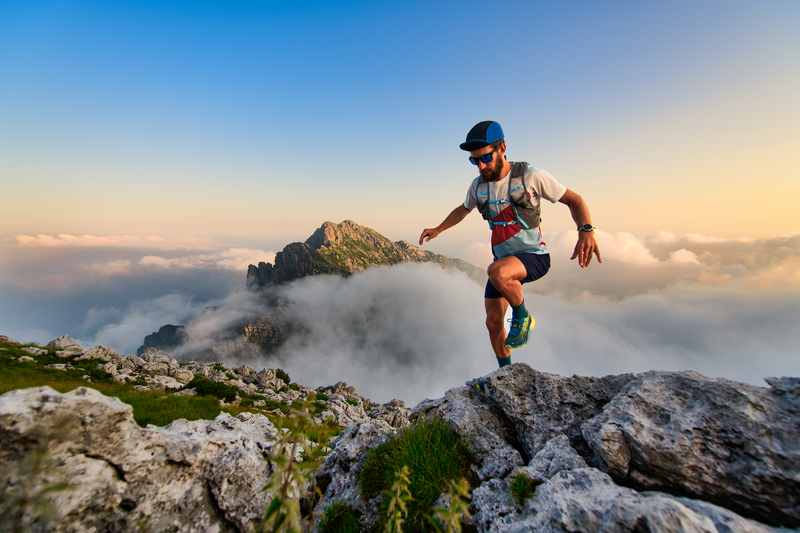
95% of researchers rate our articles as excellent or good
Learn more about the work of our research integrity team to safeguard the quality of each article we publish.
Find out more
REVIEW article
Front. Mar. Sci. , 14 August 2020
Sec. Marine Conservation and Sustainability
Volume 7 - 2020 | https://doi.org/10.3389/fmars.2020.00617
This article is part of the Research Topic Marine Ecosystem Restoration (MER) – Challenges and New Horizons View all 23 articles
Seagrasses are important marine ecosystems situated throughout the world’s coastlines. They are facing declines around the world due to global and local threats such as rising ocean temperatures, coastal development and pollution from sewage outfalls and agriculture. Efforts have been made to reduce seagrass loss through reducing local and regional stressors, and through active restoration. Seagrass restoration is a rapidly maturing discipline, but improved restoration practices are needed to enhance the success of future programs. Major gaps in knowledge remain, however, prior research efforts have provided valuable insights into factors influencing the outcomes of restoration and there are now several examples of successful large-scale restoration programs. A variety of tools and techniques have recently been developed that will improve the efficiency, cost effectiveness, and scalability of restoration programs. This review describes several restoration successes in Australia and New Zealand, with a focus on emerging techniques for restoration, key considerations for future programs, and highlights the benefits of increased collaboration, Traditional Owner (First Nation) and stakeholder engagement. Combined, these lessons and emerging approaches show that seagrass restoration is possible, and efforts should be directed at upscaling seagrass restoration into the future. This is critical for the future conservation of this important ecosystem and the ecological and coastal communities they support.
Seagrasses are marine angiosperms that grow in the coastal waters of every continent except Antarctica (Cullen-Unsworth and Unsworth, 2016), providing a wide range of ecosystem services to coastal communities (Nordlund et al., 2018a). Some of the key ecosystem services provided by seagrasses include coastal protection (Ondiviela et al., 2014; Boudouresque et al., 2016), nutrient cycling (Hemminga and Duarte, 2000; McGlathery et al., 2007), pathogen reduction (Lamb et al., 2017), storage of sedimentary carbon (Macreadie et al., 2014; Serrano et al., 2019), and the provision of nursery grounds for many species that support fisheries (de la Torre-Castro et al., 2014; Tuya et al., 2014; Nordlund et al., 2018b). Yet, despite their environmental, socio-economic and cultural value, seagrasses globally are undergoing accelerated rates of decline due to a range of threats including rising sea surface temperatures, extreme temperature events, coastal development, coastal urban and agricultural runoffs, and untreated sewage and industrial waste outfalls (Freeman et al., 2008; Grech et al., 2012; Arias-Ortiz et al., 2018). Declines to date have amounted to an estimated loss of 29% of areal extent, or 3370 km2, since records started in 1879 (Waycott et al., 2009). However, the true extent of seagrass loss remains uncertain due to estimates of seagrass areal extent globally being unknown, with many regions of Southeast Asia, the Caribbean, and the western Indian Ocean still largely understudied and/or undocumented (Gullström et al., 2002; Wabnitz et al., 2008; Fortes et al., 2018). Furthermore, seagrass losses are expected to continue, further exacerbated by climate change impacts. While increased temperatures and carbon dioxide concentrations associated with climate change could potentially increase growth rates in various species (Olsen et al., 2012; Koch et al., 2013), the increased frequency of extreme temperature and storm events is expected to increase mortality (Collier and Waycott, 2014; Rasheed et al., 2014).
The decline in seagrass habitats has clear and detrimental ecological and socio-economic consequences, and stemming this decline through facilitating recovery is urgently needed. Passive restoration efforts, or rehabilitation, have reduced anthropogenic stressors to facilitate natural regeneration, such as the improvement of water quality through removal of sewage outfalls and agricultural run-off to tackle eutrophication (Bryars and Neverauskas, 2004; Riemann et al., 2016). Despite the potential to curb the influence of anthropogenic stressors, rehabilitation efforts on a global scale have seen varying degrees of success. It is widely acknowledged that seagrass rehabilitation is a slow process, often taking years to decades for successful recolonization and meadow establishment (Leschen et al., 2010; Vaudrey et al., 2010; Greening et al., 2011). Rehabilitation failure has been attributed to a variety of factors including limited propagule supply (Orth et al., 1994; Kendrick et al., 2012), biotic and abiotic interactions e.g., predation or physical disturbance (Moksnes et al., 2008; Valdemarsen et al., 2010), shifts to unsuitable environmental conditions e.g., sediment type or sediment resuspension (Munkes, 2005; Carstensen et al., 2013), or failing to fully take into account the original cause of loss. Significant investment in seagrass restoration or the creation of new seagrass meadows where they were previously not found has been used to facilitate recovery of seagrass meadows in different parts of the world including Europe, North America, Australia, and New Zealand (e.g., Campbell, 2002; Bastyan and Cambridge, 2008; Orth and McGlathery, 2012; Matheson et al., 2017; Paulo et al., 2019). Unlike rehabilitation which ultimately relies on natural recolonization (Kirkman, 1989), restoration involves active intervention geared toward returning degraded habitats to a condition resembling their original condition (Paling et al., 2009), while habitat creation establishes new meadows in areas suitable for seagrass establishment but that were historically uninhabited by these plants (Morris et al., 2006). Habitat restoration and creation may include efforts such as the physical planting of seagrasses, distribution or planting of seagrass seeds, or coastal engineering to modify sediment and/or hydrodynamic regimes (Campbell, 2003; Weatherall et al., 2016). In this review, the term seagrass restoration is used to encompass rehabilitation, habitat restoration, and habitat creation.
Historically, marine restoration has trailed behind terrestrial and freshwater ecosystems, owing in part due to the scale of the marine environment and common ownership of resources (e.g., in international waters) which often leads to difficulties in management (Hawkins et al., 2002). Furthermore, marine environments are much more difficult to access and work in compared to terrestrial environments, and the impacts of degradation are not always clearly visible to society (Sinclair et al., 2013). Restoration of terrestrial systems (including forests, lakes, and grasslands) has a relatively long history, developing restoration techniques that are now sufficiently advanced for adequate returns on high levels of investment (Ruiz-Jaen and Aide, 2005). The successes currently experienced in terrestrial restoration have been built upon decades of knowledge and experience gained through numerous studies and experiments, many of which were not successful initially but were invaluable for understanding why early restoration attempts did not work, and allowed for improvements to restoration methods and techniques to be made (Nellemann and Corcoran, 2010). In contrast, restoration of marine coastal ecosystems (seagrasses, macroalgae, corals, saltmarshes, mangroves) is still a maturing area of science (Wood et al., 2019). Seagrass restoration is often deemed too expensive due to a multitude of reasons including but not limited to high labor costs, challenges of propagation, and the need for repeated planting efforts due to losses (Bayraktarov et al., 2016). These high costs have hindered efforts over the years. The median cost of seagrass restoration was estimated at USD 106,782 per hectare based on 64 published studies (Bayraktarov et al., 2016), and this can be 10–400 times higher than the costs documented for terrestrial ecosystem restoration (Jacob et al., 2018).
Seagrass practitioners, indeed all marine restoration practitioners, can benefit from restoration science and practice that has been developed over decades in terrestrial ecosystems and could be applied in marine environments. For instance, mine site rehabilitation practices considerably focus on the preparation, composition, form and microbial community of top soils before planting (Cooke and Johnson, 2002). These parameters are also likely to be important for seagrass colonization, and should be given equal consideration as the more well-known and studied parameters of light, depth and water quality. Yet sediment dynamics are relatively understudied (except see Campbell et al., 2018) and often neglected in seagrass site suitability assessments and preparation. Valuable lessons are still to be learned from the broader field of applied ecosystem restoration and continued exploration of methodologies will yield improved outcomes for some systems. Incorporating knowledge from the broader field of ecological restoration, and particularly seeking out ecosystems and methods that are less familiar to marine ecologists, is likely to yield many benefits and shortcuts for the young yet rapidly maturing field of seagrass restoration.
Initial seagrass restoration studies date back to 1939, with the majority of the work occurring in the United States, Europe or eastern Asia (China, Japan, and Korea). Efforts were largely focused on Zostera marina (van Katwijk et al., 2016). A successful example is the recovery of approximately 1700 ha of Z. marina in the Virginia Coast Reserve (Orth and McGlathery, 2012). These efforts resulted in epifauna invertebrate recovery in the 1990s (Lefcheck et al., 2017). Recent success has also occurred in Whangarei Harbor, New Zealand, with at least 600 ha of Zostera muelleri being rehabilitated due to management actions taken to improve water quality and subsequent restoration planting trials (Matheson et al., 2017). However, many other restoration efforts have seen lower rates of success (van Katwijk et al., 2016). Nonetheless, the knowledge and experience gained from these early studies have proved invaluable for developing the knowledge that has made large-scale seagrass restoration feasible today.
This review aims to highlight some of the recent seagrass losses in Australia and New Zealand, and emphasize the seagrass restoration successes we have experienced. We focus on some of the challenges that remain and need to be overcome to enable large-scale seagrass restoration and highlight emerging tools and techniques being developed that can help achieve restoration success. Lastly, we discuss the need for management strategies that address the threats of climate change and incorporate evolutionary potential for “climate-proofing” remnant and restored seagrass meadows. Heavier emphasis is given to Australian restoration work in this review, largely due to the fact that although there has been recent activity with regards to seagrass restoration in New Zealand, the New Zealand effort to date, lags far behind Australia and the world. With the exception of the research undertaken by Matheson, restoration efforts in New Zealand are typically focused upon shellfish (e.g., Marsden and Adkins, 2010; Hewitt and Cummings, 2013), which are important taonga for Māori (e.g., Paul-Burke et al., 2018). Seagrass research in New Zealand has focused on understanding fundamental community ecology and biology (e.g., Dos Santos et al., 2012; Kohlmeier et al., 2014; Morrison et al., 2014; Sørensen et al., 2018; Cussioli et al., 2019, 2020), macroinvertebrate and fish communities interactions (e.g., Mills and Berkenbusch, 2009; Lundquist et al., 2018) and impacts upon these communities (e.g., Bulmer et al., 2016; Cussioli et al., 2019; Li et al., 2019; Matheson et al., submitted). The fundamental research that is occurring in New Zealand is required to understand how New Zealand seagrass function and thereby formulate a comprehensive understanding of local seagrass dynamics to successfully implement site specific restoration practices (e.g., Matheson et al., 2017).
Seagrass losses in Australia follow global patterns, with a reported loss of at least 291,783 ha, representing 5.5% of estimated areal extent, since the 1930s (Statton et al., 2018). These losses include several large-scale declines in Shark Bay, West Australia, Western Port, Victoria, and metropolitan Adelaide, which lost 154,800, 17,800, and 5,200 ha of seagrass habitat, respectively (Tanner et al., 2014; Arias-Ortiz et al., 2018; Statton et al., 2018). Losses have also been documented in New Zealand (Park, 1999, 2016; Inglis, 2003; Turner and Schwarz, 2006; Matheson et al., 2011), with one of the more significant examples being the disappearance of 14,100 ha of seagrass from Tauranga Harbor since 1959 (Park, 1999, 2016). These losses, and the associated losses in ecosystem goods and services, can have major ecological, socioeconomic, and political ramifications (Smale et al., 2019). For example, the recent estimated loss of 36% of seagrass meadows in Shark Bay followed extreme temperature events and resulted in declines of various herbivorous species such as green turtles and dugongs, seagrass-associated fish populations, and closure of scallop and blue swimmer crab fisheries (Nowicki et al., 2017; Kendrick et al., 2019). Similarly, carbon and nutrient cycling was disrupted (Smale et al., 2019). Declining seagrass habitats are recognized as a significant threat to fisheries production, with estimates that seagrasses contribute AUD $31.5 million per year to Australia’s commercial fisheries (Jänes et al., 2019). In the tropics of Queensland, historically, seagrasses have shown a remarkable capacity to recover from large disturbance events without direct intervention (Rasheed et al., 2014; Coles et al., 2015). This is likely due to a combination of relatively well-connected seagrass populations (Grech et al., 2018) and life history strategies of tropical species allowing for rapid colonization and growth (Rasheed, 1999, 2004). However, in recent times this situation has changed, with the relative frequency of La Niña climate events and severe storms leading to sustained losses (McKenna et al., 2015) and cases where natural seagrass recovery is unlikely. These conditions are predicted to become more common with climate change (Rasheed and Unsworth, 2011), making knowledge of how to restore these tropical species increasingly important. Thus, seagrass losses represent a major financial cost that could escalate in the event of complete habitat destruction.
Restoration research in Australia and New Zealand has focused on small-scale experimental tests using a variety of techniques ranging from the planting of sprigs (seagrass fragments) or plugs (seagrass cores) to seed-based restoration (Supplementary Table S1; Figure 1). The majority of seagrass restoration trials to date have used shoot-based techniques, with at least 46 studies since 1986 (Supplementary Table S1). These have ranged from small-scale pilot studies (e.g., Irving et al., 2010) to large-scale transplantation trials (e.g., West et al., 1990; Bastyan and Cambridge, 2008), involving both manual and mechanical planting (e.g., Paling et al., 2001), and a wide range of anchoring methods [e.g., artificial seagrass (West et al., 1990; Campbell and Paling, 2003; Matheson et al., 2017), biodegradable pots (Kirkman, 1999), and hooks or pegs (Bastyan and Cambridge, 2008)]. Although survival of transplanted seagrass fragments or cores was low in many studies, promising results are increasingly reported, with transplant units surviving more than 2 years or showing shoot densities similar to naturally occurring meadows (e.g., Bastyan and Cambridge, 2008; Oceanica Consulting Pty Ltd., 2011, Matheson et al., 2017).
Figure 1. Infographic showing (a) all seagrass restoration trials carried out to date in Australasia, with inset map showing the concentration of studies carried out in Cockburn Sound, Western Australia; (b) length of monitoring of seagrass restoration trials based on states, (c) the proportion of different types of transplant units used in restoration trials across Australasia; and (d) the target genera in restoration trials across Australasia.
There are no published trials of restoration using seeds in Australia and New Zealand to date. However, scientists at the University of Western Australia are currently developing an approach to collect, process, and remotely deliver seeds of Posidonia australis, and have seen some early successes at the trial stages1. Scientists from Central Queensland University in the multicommodity Port of Gladstone (Great Barrier Reef World Heritage Area) have also assessed the practicality of seed collection, storage, and germination for Z. muelleri restoration (E. L. Jackson, Central Queensland University, personal communication). The use of seedlings in restoration is more well-established, especially in the use of hessian bags which act as a substrate for Amphibolis seedling recruitment. Long-term trials involving the use of hessian bags placed on the ocean floor to aid natural seedling recruitment started in 2004, with many showing long-term survival (Irving et al., 2010; Tanner, 2015). Studies on seed-based restoration for other species have been highly variable and less successful, highlighting the need for more in-depth research (Lord et al., 1999; Irving et al., 2010).
The restoration successes seen in Australia and New Zealand today largely come from studies on Posidonia and Amphibolis, as well as Z. muelleri in New Zealand (Figure 1). While these studies have contributed to the overall knowledge of restoration, more species- and habitat-specific studies are required to improve restoration success. Species-specific studies are required to establish clarity around seagrass resilience, especially local adaptive potential in the face of climate change. Successful restoration efforts will rely upon whether transplants or seeds are able to persist under future conditions. Success will require accurate forecasting, which requires rigorous species, site, habitat, and methodological data.
There are several well-established seagrass restoration frameworks published to date, including the five-step program by Campbell (2002), which consists of decision-making trees in the planning process for restoration. A review by van Katwijk et al. (2016) has highlighted considerations, such as the removal of the original cause of seagrass decline or site-specific planting techniques, that needs to occur before starting on any restoration activity. Yet, knowledge gaps still exist, which are discussed in the following sections. There are also lessons to be learned and applied from the terrestrial restoration community here, and that designing effective restoration efforts will require input from multiple disciplines (Miller et al., 2017).
It is essential to put enabling policies and legislation in place to facilitate broad-scale seagrass restoration efforts required to halt or reverse rates of seagrass decline. This includes clear delineation of roles and responsibilities between various agencies, and attachment of necessary resources to deliver meaningful programs. For example, the Catchment Management Framework in Victoria, Australia, set up under the Catchment and Land Protection Act 1994 (CaLP Act), incorporates environmental, economic, and social considerations for the coordinated management of land, water, and biodiversity resources based on catchment areas (State Government of Victoria, 2020). Each catchment area has its own management authority, which works together with other agencies and groups such as Parks Victoria, Victorian Coastal Council, and local councils and Landcare groups (State Government of Victoria, 2016). This ensures legislative accountability for all involved parties, leading to resources being utilized efficiently, and reasons for failures identified. In New Zealand, seagrass (Z. muelleri) is listed as at risk – declining by the Department of Conservation (de Lange et al., 2017). The New Zealand Government’s draft National Policy Statement for Freshwater Management (NPS-FM) issued by the Minister for the Environment in September 2019 requires councils to provide for and encourage the restoration of inland wetlands. However, there is as yet no similar requirement for coastal wetlands (which include seagrass meadows to 2 m below low water). Nevertheless, the proposed NPS-FM and proposed National Environmental Standard for Freshwater do provide increased protections for coastal wetlands from activities including disturbance of the bed and removal of indigenous vegetation. Whilst restoration policy is rapidly being embedded into international agreements (e.g., New Deal for Nature, Convention on Biological Diversity Conference of Parties 15 (COP), and climate change challenges for COP26 Glasgow), it is yet to filter down adequately into Australian and New Zealand state or federal government policies for seagrass restoration. For example, the Environment Protection and Biodiversity Conservation Act 1999 (EPBC Act) is one of the key environmental protection legislations in Australia. While the EPBC Act does provide some provisions for restoration such as through species recovery plans, these are generally only for threatened ecosystems (of which seagrass is yet to become), and these plans are slow to develop. However, there are also a range of restoration policies in the context of other ecosystems (e.g., Victorian Waterway Health Management Strategy), and others that do aim to protect seagrasses (e.g., Ramsar management framework, NZ’s NPS-FM). Australia and New Zealand could expand on these existing polices, and learn from other regions which have more proactive policies on marine restoration, such as Living Shorelines (National Oceanic and Atmospheric Administration [NOAA], 2015), or from terrestrial ecosystems [e.g., Working for Water South Africa (Department of Environmental Affairs, 2019) or the Atlantic Forest Restoration Pact (2016)].
While sound polices and legislation may provide a firm foundation for upscaling seagrass restoration efforts, investment may be quickly undermined if resources are not carefully targeted to areas where threats to seagrass persistence have been removed or reduced, successful seagrass restoration is feasible, stakeholders are willing and able to invest, and the benefits to other environmental and social values are the greatest. Accordingly, for many natural resource management agencies that operate at a regional to National scale, we emphasize the importance of adopting strategic prioritization approaches to identify high priority areas to guide conservation and/or restoration efforts. While more complex models (e.g., Leathwick et al., 2008; Moilanen et al., 2011) may be desirable in the longer term, simple decision support tools for prioritizing seagrass restoration already exist that allow management agencies to maximize returns by targeting sites where intervention would be the most cost-effective (e.g., Grech et al., 2011; Tan et al., 2018). These decision support tools are easy to use, and easily adaptable to suit different conservation priorities. Furthermore, these support tools can also be coupled or sequenced with ecosystem risk assessments, such as the IUCN Red List of Ecosystems (Rodríguez et al., 2015), allowing for a complete understanding of the conservation status of seagrass ecosystems, their current and future threats and opportunities for restoration. Identification of sites for seagrass restoration should be carried out collaboratively (i.e., between managers, scientists and the community), to not only ensure that financial resources are spent efficiently and effectively (instead of opportunistically as it is largely being done currently), but also so there is a shared understanding and ownership of restoration priorities.
Once priority areas are identified, at a more local scale, a clear understanding of environmental conditions should be incorporated into site selection before investing effort into restoration (Campbell, 2002; van Katwijk et al., 2016). This includes consideration of the original stressors that resulted in seagrass loss, whether they have been mediated or removed, resulting in a return to a favorable state for seagrass restoration; or if the environment has been too drastically altered to sustain seagrass. Accurate information on historical changes is preferred (but not always available), such as persistence, loss, gain, or recovery of seagrass meadows, and environmental drivers affecting their past, present, and future potential distribution such as light, sediment, temperature, and nutrients. This information will inform decisions when tackling stressors or threats (van Katwijk et al., 2016), and when considering which restoration approach may be most appropriate at a site (Campbell, 2002). These data can then also be incorporated into habitat suitability models to identify receptive restoration sites, which are regularly used in other marine systems like shellfish (Theuerkauf and Lipcius, 2016). Whilst many types of habitat suitability models exist, the principle of spatial planning and suitability indexes can be expanded to include development of restoration suitability models which encompass not only the environmental and physical suitability for seagrasses, but cultural, logistical, and social data as well.
There is now a growing emphasis on seed-based restoration, largely due to the lower impact it has on existing meadows (i.e., removal of large numbers of fragments or cores in shoot-based restoration), and its potential for upscaling (Orth et al., 2006). To facilitate the growing area of seed-based restoration, more information is required on how flowering and seed production varies within and across species, and the environmental factors that trigger reproduction and germination (Cumming et al., 2017). Substantial inter-annual and small-scale spatial variability in sexual reproduction has been reported from studies in Western Australia, New South Wales, Victoria, and New Zealand, with results suggesting that the timing and intensity of flowering are influenced by processes occurring across a range of spatial scales (Dos Santos and Matheson, 2016; Smith et al., 2016; Sherman et al., 2018). This is important given the differences in the geographical range of some Australian and New Zealand species. For example, some species such as Halophila ovalis, can be found from the tropical waters of Southeast Asia to the temperate waters of Western Australia and New South Wales (Short et al., 2010). In contrast, some species have a more confined range such as Posidonia sinuosa, which is endemic to western and southern Australia (Short et al., 2010).
Effective seed-based restoration will require improved techniques for the collection, handling and storage of seeds to optimize germination and survival. The efficiency of Z. marina seed collection has been improved through mechanical harvesting in North America, with an estimated maximum mechanical collection rate of 132,000 seeds/labor-hour versus 62,000 seeds/labor-hour using manual collection, with minimal damage to the donor meadow (Marion and Orth, 2010). Seed collection rates could differ based on meadow productivity, therefore, studies into whether mechanical harvesting efficiencies in the United States can be replicated on Australian and New Zealand species are worthwhile. Besides collection methods, species-specific studies on seed storage could also enhance restoration opportunities, with seeds being collected and stored for planting during a later more optimal planting season (Marion and Orth, 2010). Such studies need to take into account the different characteristics of Australian and New Zealand seagrass seeds, which have varying lengths of dormancy and sensitivity to desiccation that will affect the efficacy of seed storage (Orth et al., 2000; Statton et al., 2012). For example, the seeds of Zostera spp. which possess a hard seed coat can be viable up to 12 months in storage (Orth et al., 2000), whereas the seeds of Enhalus acoroides were only able to survive a maximum of 11 days in storage (Ambo-Rappe and Yasir, 2015). Lastly, while seed collection is considered to have less of an impact on donor meadows than collection of shoots, it is still important to understand the impact seed collection might have, especially surrounding how much can be collected without impacting donor meadows and other connected meadows.
Seagrasses have the potential to disperse over long distances via ocean currents during various life-history stages (Kendrick et al., 2012; McMahon et al., 2018). Population genetic studies in combination with hydrodynamic models have increased our understanding of the role/potential of connectivity in natural seagrass meadow recovery (e.g., Sinclair et al., 2016, 2018; Smith et al., 2018). Although there is a growing understanding of the movement ecology of seagrasses (McMahon et al., 2014; Smith et al., 2018), studies on propagule viability and survival, and establishment success are currently limited (but see Campbell, 2003; Weatherall et al., 2016). A combined understanding of the dispersal mechanisms and reproductive biology of seagrasses will add to the overall understanding of spatial and genetic connectivity.
Spatial patterns of genetic connectivity can inform decision-making and help to prioritize management actions (e.g., Sherman et al., 2016). For example, connectivity estimates can be used to identify areas that are more likely to recover naturally following decline (e.g., areas that have steady supply of propagules from non-local sources) and areas that have limited recovery potential due to recruitment limitations (e.g., isolated areas expected to receive minimal or no propagule recruitment from non-local sources). Habitat enhancement and ecological engineering to encourage settlement would become priority management actions for areas showing limited signs of recovery despite expected propagule supply. In contrast, translocations (e.g., physical planting) in combination with habitat restoration investments would be needed in areas with limited propagule supply to ensure population establishment.
The level of genetic diversity of source and recipient seagrass meadows is also an important factor to consider when augmenting remnant seagrass meadows or establishing new meadows. Seagrass meadows at the edge of their range may have lower genetic diversity and higher levels of clonality (e.g., Evans et al., 2014) or have reduced seed production as a result of pollen limitation (e.g., Sinclair et al., in press). Small isolated populations often have similar issues (Kendrick et al., 2012; Reynolds et al., 2013; McMahon et al., 2014). Overall genetic diversity is positively associated with population fitness (Connolly et al., 2018), and standing genetic variation within populations is closely tied to adaptive capacity and resilience to environmental change (Reed and Frankham, 2003; Leimu et al., 2006). Previous studies also indicate that genetic diversity is positively correlated with seagrass-related community species richness and productivity (Crutsinger et al., 2006; Whitlock, 2014). Consequently, selecting genetically diverse meadow(s) as a donor source is important for maximizing restoration success (Reynolds et al., 2012, 2013).
Declines of many seagrass species have been attributed to thermal stress associated with rising sea surface temperatures and extreme temperature events (Marbà and Duarte, 2010; Thomson et al., 2015; Carlson et al., 2018; Babcock et al., 2019). Many seagrass species persist in low energy and shallow water environments such as coastal embayments, inlets and fjords. These environments are particularly susceptible to warming and extreme temperature fluctuations, compared with open coastal environments (Harley et al., 2006). Consequently, projections of rising sea surface temperatures and more frequent heatwave events pose a significant risk to both natural and restored seagrass populations (Smale et al., 2019). Populations inhabiting trailing (or warming) range edges are often less well connected through dispersal, but can exhibit a high degree of local adaptation and thus contain unique genotypes necessary for future survival. However, they are also often most threatened under climate change (Hampe and Petit, 2005; Zardi et al., 2015). Similarly, intertidal species show niche partitioning of rhizome depth based upon substrate temperature envelopes (Campbell et al., 2018), which has further implications when selecting plants from donor for receiving environs and transplantation depth. This reinforces the need for management strategies to address the risks of climate change and maximize adaptive potential.
Traditionally, restoration policy has advocated the use of local genetic material (known as local provenancing) when undertaking restoration activities (Jordan et al., 2019). However, evidence suggests that non-local sources of restoration material may outperform those from local provenance under future climates (Sgrò et al., 2011; Aitken and Whitlock, 2013; Miller et al., 2019a). Therefore, future restoration may require looking at alternative strategies, such as mixed provenancing (mixing of seed from multiple sources; Broadhurst et al., 2008). Such approaches can help to broaden the genetic basis of restored populations to overcome risks maladaptation by providing new genetic variants for selection to act on (Prober et al., 2015; Wood et al., 2019). Such approaches can also help to overcome fitness reductions is small, inbred and genetically depauperate populations (Broadhurst et al., 2008; Weeks et al., 2011).
Overcoming risks of maladaptation may be further assisted by identifying and introducing pre-adapted genotypes (Browne et al., 2019). This might be achieved by moving genotypes from warmer adapted populations to cooler locations to increase the probability of tolerance to rising ocean temperatures (Schueler et al., 2013; Bansal et al., 2015; Wood et al., 2019). This typically applies to widespread species with wide latitudinal ranges, many of which show genetically based clines in performance across thermal gradients (Staehr and Wernberg, 2009; Mohring et al., 2014; Bennett et al., 2015). Identifying pre-adapted or “climate-ready” seed sources for restoration requires an understanding of adaptive genetic differentiation among populations spanning thermal gradients. Typically, this can be determined empirically using quantitative (e.g., glass house or common garden experiments; Byars et al., 2007; Browne et al., 2019; Miller et al., 2019a) or correlative (e.g., genomic assessments for genotype x environment associations; Jordan et al., 2017; Miller et al., 2019b) genetic approaches. When genetic data is not available, climate matching (between restoration areas and others spanning the species climatic distribution) can help identify genotypes expected to be suited to future predicted climates at the restoration area (Winder et al., 2011; Benito-Garzón et al., 2013).
While opportunities may exist to bolster the genetic basis of seagrass populations, consideration needs to be given to species’ climate niches under future climates. Species distribution models suggest that major shifts in suitable habitat will occur under climate change. In some cases, it has been suggested that there will be no (or minimal) overlap between current and future predicted climatic niches (Rehfeldt and Jaquish, 2010; Ledig et al., 2012; Wang et al., 2016). In such cases conservation of remnant populations persisting in areas more likely to support the species under climate change becomes a priority, and interventions, such as assisted range expansion, to facilitate species movement to climatically suitable areas may need to be considered (Hoegh-Guldberg et al., 2008; Cole et al., 2011; Winder et al., 2011; Wadgymar et al., 2015). Numerous cautions have been raised when considering assisted migration, particularly in aquatic systems (Ricciardi and Simberloff, 2009, but see Schlaepfer et al., 2009): potential for the species to become invasive (Hoegh-Guldberg et al., 2008; Mueller and Hellman, 2008, Aitken and Whitlock, 2013; Hancock and Gallagher, 2014) and transfer of pests and pathogens from source locations (Simler et al., 2019). Clearly no assisted migration is risk free, but appropriate precautions including rigorous risk assessment and biosecurity protocols will act to minimize some concerns (Hancock and Gallagher, 2014; Simler et al., 2019).
One of the major gaps in knowledge remains our understanding of how to restore tropical species in Australia. Most of the specific knowledge and current restoration paradigms have been developed from temperate or subtropical experience. There have been small process studies in the tropics (Rasheed, 1999, 2004) but to date no larger scale restoration attempts. What we do know from observations of natural recovery processes in tropical Australia (Rasheed et al., 2014; McKenna et al., 2015) is that the life history and reproductive strategies of many tropical species could be well-suited to restoration as they have the capacity for rapid clonal growth once established. As to whether a modified or different set of considerations are required for successful tropical restoration requires an increased research focus on understanding the basics.
Ecological engineering was first formally defined as “the design of sustainable ecosystems that integrate human society with its natural environment for the benefit of both” (Mitsch, 2012). Since its inception, ecological engineering has come to encompass approaches such as replacing traditional built infrastructure with newly created or restored coastal ecosystems such as mangroves or salt marshes (Temmerman et al., 2013), or designing new or altering old infrastructure to add structural complexity to promote settlement of marine organisms (Martins et al., 2010; Loke et al., 2017) and reduce settlement of non-indigenous species (e.g., Dafforn, 2017). These same principles could be applied to seagrass restoration, following studies to elucidate conditions where settlement and/or colonization can be promoted. The suitable conditions for seagrass growth are generally well established, including light availability (Duarte, 1991; Ralph et al., 2007), hydrodynamic environment (Fonseca and Kenworthy, 1987; Schanz and Asmus, 2003), substratum type (Erftemeijer and Middelburg, 1993; van Katwijk and Wijgergangs, 2004), or nutrient availability (Udy and Dennison, 1997; Touchette and Burkholder, 2000). This knowledge combined with site-specific studies can be used to inform future development and restoration plans in terms of ecological engineering. In this instance, ecological engineering is a form of “passive” restoration, where the conditions to encourage settlement and establishment are included in development design. Unintended recruitment has already been reported, for example, in Singapore, where a small seagrass meadow formed on a reclaimed shoreline behind a breakwater (Yaakub et al., 2014; Lai et al., 2018). In certain situations, coastal development will proceed, and loss of seagrass habitat is inevitable. However, there are opportunities through the coastal development design phases to incorporate ecological engineering, such as building breakwaters to mimic shallow embayments to enhance the settlement of seagrass seeds, propagules or fragments. To be most effective, these designs need to be combined with other studies, such as modeling of seagrass seed or fragment dispersal and meadow connectivity.
There have been many small-scale restoration trials that have shown success. However, the challenge remains to translate small-scale success into large-scale restoration programs (van Katwijk et al., 2016). To upscale restoration programs, the involvement and commitment of industry partners, local communities, non-governmental organizations (NGOs), and state and federal government agencies are required to establish multi-year to decadal funded restoration projects. Programs need to be holistic and focus on the landscapes and associated benefits, rather than just a single species. Collaborative designing of restoration programs between researchers, managers, and the various community stakeholders would likely increase restoration success, as each of these groups bring their own unique (though sometimes overlapping) skills and experiences. While grassroots restoration efforts are important for upscaling restoration efforts, there needs to be effective communication of the science underpinning restoration to regional managers and community volunteers to improve the likelihood of success. In turn, scientists can also gain valuable insights into local environmental and socio-economic conditions from regional managers who have on-the-ground experience and knowledge, which could prove to be extremely beneficial to restoration programs. For example, coordinated efforts by governmental bodies and restoration scientists to restore Amphibolis meadows in South Australia has shown promising results, with trials using hessian bags in seedling recruitment showing survival for at least 5 years and greater stem lengths compared to natural meadows (Tanner, 2015; Supplementary Table S1).
Various academic research groups and management bodies around the globe work on seagrass restoration, and while their research outputs are published in international journals and gray literature, it is well-established that a publication bias toward successful restoration outcomes exists (Zedler, 2007). There is much that can be learned from unsuccessful restoration attempts, or unexpected outcomes that largely go unpublished, and a platform for knowledge sharing would greatly aid in disseminating information and generating greater discussion between seagrass scientists, managers and practitioners. Scientists clearly provide an important role during research and development, and ongoing monitoring and providing information for adaptive management. However, as restoration projects scale up, they need to be managed like any other large infrastructure development projects, and most researchers rarely have the skill sets, experience or capacity to manage such projects. There is therefore a need to facilitate knowledge sharing not only between researchers, but also with industries that have the expertise and knowledge that allows upscaling of seagrass restoration efforts. Knowledge sharing can be facilitated through a variety of platforms including formal partnerships and agreements between industry and research institutions to collaborate on delivering restoration projects, workshops that facilitate a broad range of stakeholder engagement and through the development of network communities. One example of this is the Seagrass Restoration Network2, which was established in 2015 to link researchers, managers and practitioners worldwide and facilitate the sharing of knowledge and tools for seagrass conservation and restoration. The Network currently has more than 50 members from around the globe.
Lastly, more long-term and regular monitoring of seagrass meadows is needed to understand rates and patterns of seagrass loss, the likely drivers of these losses, identify potential restoration sites, and effectively determine the success of intervention (Duffy et al., 2019). In Australia, estimates of seagrass coverage are still incomplete, with many regions currently lacking this basic knowledge (York et al., 2017). In New Zealand, a national scale inventory of seagrass has recently been collated by the Department of Conservation’s SeaSketch project. The NZ SeaSketch project is acknowledged as incomplete, lacking data for the many unmapped locations, especially those with smaller areas of seagrass (Anderson et al., 2019). Further monitoring is required to make informed decisions on where seagrass has been lost, and where it could potentially be restored. Importantly such monitoring needs to incorporate larger spatial scale assessments and mapping to be effective. Long-term monitoring is also needed for restoration projects, with approximately 61% of Australian and New Zealand studies monitoring beyond a year, although the longest running Posidonia restoration site has been monitored in excess of 20 years (Supplementary Table S1; Figure 1). This is a problem not unique to Australian and New Zealand studies (van Katwijk et al., 2016). Among the studies with long-term monitoring, many lack regular monitoring, with a few studies only monitoring at the start and end of the restoration trial (e.g., Connolly et al., 2016). Without long-term information, the effectiveness of the restoration methods cannot be assessed accurately, and improvements to the method cannot be made. Monitoring could incorporate the use of appropriately trained volunteers, which will help drive research costs down, raise awareness and encourage local communities to have a sense of ownership in restoration programs. The use of novel and potentially more efficient monitoring techniques should also be expanded upon, such as the use of remote sensing or drones (Duffy et al., 2018; Nahirnick et al., 2019; Phinn et al., 2018).
Future restoration programs should dedicate funding for monitoring programs to ensure that appropriate monitoring durations are captured. It is important to note that seagrass restoration is at the stage where effective methods are still in development, and while we are going through this phase, many failures are to be expected. As a result, monitoring results from restoration programs may be discouraging to managers or community groups who may feel pressure for successful outcomes due to substantial investment of time and/or money. This may in turn make it harder to secure funding for future restoration works if the outcomes are uncertain, or even long-term monitoring programs which may be more expensive than the restoration itself. However, it is essential to recognize that results are not immediate, and that resources need to be invested to work toward effective restoration programs.
There are a variety of new restoration tools and techniques that have been developed and trialed internationally that show relatively high degrees of success. However, there is no “one solution fits all” approach to suit the life history traits of all species across all conditions. The emerging tools now make seagrass restoration feasible for many species and at the large spatial scales needed to restore seagrass meadows and associated ecosystem services. Emerging approaches focusing on holistic and collaborative restoration practices have also recently been highlighted, aimed at creating a “global restorative culture” in order to improve human, and ecosystem health (Aronson et al., 2020). The success of these tools, techniques, and approaches are underpinned from previous lessons learned, including many restoration “failures.”
The BuDS technique involves the collection of mature reproductive shoots which are placed in mesh nets attached to buoys, suspended above plots to be restored with the aim that negatively buoyant seeds when released, will settle over the desired restoration plot (Pickerell et al., 2005; Figure 2). The collection of reproductive shoots can be relatively easy and rapid (depending on the target species), and BuDS can be deployed over relatively large spatial scales. Recruitment based on this technique is currently low, at approximately 1 (Marion and Orth, 2010) to 6.9% of seeds deployed (Pickerell et al., 2005). The use of BuDS ensures high genetic diversity, which is positively correlated to rates of sexual reproduction, vegetative propagation, and overall shoot density (Williams, 2001; Reynolds et al., 2012). However, BuDS are unlikely to be suitable for all conditions and species, having only been tested on Z. marina thus far (Pickerell et al., 2005; Busch et al., 2010; Marion and Orth, 2010). BuDS deployed in areas with strong hydrodynamics might be less effective as seeds can be washed away at high rates. Marion and Orth (2010) also found that many of the buoys deployed were coated in drifting macroalgae, and were potentially grazed upon by large numbers of amphipods and juvenile crabs, reducing the number of seeds available. Thus, the overall effectiveness of the method might be limited.
Figure 2. Emerging tools and techniques developed within the international seagrass restoration community. First row left to right: buoy-deployed seeding (© Jannes Heusinkveld), Dispenser Injection Seeding (© Laura Govers). Second row left to right: seagrass nurseries (© Gary Kendrick and John Statton); anchoring shoots using iron nails (© Troels Lange). Third row left to right: artificial in-water structures (© Peter Macreadie), and collection and use of alternative sources of transplantation (© Harriet Spark).
One of the most recently developed techniques in seagrass restoration comes from the Dutch Wadden Sea, where seeds are mixed with local sediment to create a sediment-seed mixture that is then injected into the substrate using modified sealant guns (Figure 2). A predetermined amount of seeds is mixed with sieved fine-grained sediment (median grain size <100 μm), loaded into sealant tubes, and injected into the sediment using calibrated sealant guns up to a depth of 1–4 cm, depending on the depth that the seeds of the target species naturally recruit from. Sediment is collected close to the restoration sites so as not to introduce foreign substrate, and is fine-grained as this allows a cohesive substrate to be formed which in turn keeps the seeds together for injection into the sediment and keeps injections standardized. Inorganic clay can also be added to the natural sediment to improve cohesiveness of the seed-sediment mixture. This method was trialed in the intertidal Dutch Wadden Sea, using Z. marina seeds in 2017 and 2018 (Govers, 2018). Resulting plant densities exceeded target densities of 10 plants m–2 (Figure 2).
This method is promising, especially for sites with strong tidal currents, such as the intertidal zone, where hand-casting and BuDS have not been very successful. However, direct injecting of seeds has yet to be trialed for other seagrass species and is likely more labor intensive compared to other seeding techniques such as hand-casting. The technique is also currently suitable for seeds between 0.5 and 4 mm in size, however, the equipment needed can be adjusted accordingly for different seed sizes. Additionally, if the restoration site is predominantly coarse-grained sediment, the use of mud (fine-grained sediment) required by this technique might not be ideal. Nonetheless, it is a valuable technique and is still less labor-intensive than attempting restoration via planting vegetative fragments. An adapted version of this method is currently being trialed for underwater seeding.
The use of aquaculture systems in seagrass restoration is relatively new, and the few published studies to date have shown promising results. Under controlled conditions, Tanner and Parham (2010) were able to germinate and grow Z. marina plants to a size that was large enough for transplanting within 70–100 days. Furthermore, the nursery reared plants had a higher survival rate and better growth than plants transplanted from natural beds (Tanner et al., 2010). In Australia, seedling culture has also been carried out on Prunus angustifolia (Irving et al., 2010) and P. australis (Statton et al., 2013). While the survival of cultured P. angustifolia seedlings and subsequent outplanting survival was low (Irving et al., 2010), P. australis fared much better, with 100% cultured seedling survival after 7 months (Statton et al., 2013). Furthermore, Statton et al. (2013) were able to produce larger P. australis seedlings by modifying the sediment composition used (Figure 2). These studies demonstrate that aquaculture of seagrass seedlings is a viable source of planting units in restoration. In combination with more species-specific studies on seed germination and optimum storage conditions, the use of nurseries is an effective addition in seagrass restoration, especially for areas or species where seed production is high.
The use of shoots has been widely used in restoration. These are often planted directly into the substrate (e.g., Matheson et al., 2017), however, several anchoring techniques have been used to varying degrees of success. One of the most successful examples of the use of anchored shoots for seagrass restoration has been in Denmark (Lange et al., in review). Danish waters are typically characterized with periods of severe wave action, and it has not been possible to transplant Z. marina as unanchored shoots which tend to be uprooted within short periods. Instead, the transplanted shoots which had 5–10 cm rhizome were attached to iron nails of 8 × 0.3 cm by winding a thin iron wire of 0.5 mm thickness around the rhizome and the nail (Figure 2). The nails were uncoated pure iron (not corrosion treated) and corroded within the first year without leaving heavy metals in the sediment. During transplanting, the rhizome and nail are gently pushed about 1 cm down into the sediment, ensuring that the base of the shoots is sitting at the sediment-water interface. This technique provides sufficient weight to keep the transplanted shoots in place and has led to the successful restoration of about 1.5 ha of seagrass in three estuaries (Lange et al., in review). Altogether, more than 40,000 shoots were transplanted in the three systems. The transplantations were laid out in a chess board pattern, mixing bare bottom and transplanted areas. The shoot density increased from 19 to 900 m–2 within the first 2 years, with vegetative propagations also partly covering the bare bottom areas. The return of ecosystem services was also measurable as increased accumulation of C, N, and P in the sediment, increased infauna and epifauna biodiversity and species densities compared to the bare bottom. The success of this technique could be due to the addition of iron into the sediment when the nail corrodes. Iron addition into a well-oxidized seagrass rhizosphere increases the absorption capacity for phosphorus and reduces sulfide toxicity, in turn increasing seagrass productivity (Holmer et al., 2005; Ruiz-Halpern et al., 2008). As such, the benefits of iron addition in combination with this anchoring technique should also be considered as a mechanism for increasing restoration success.
The use of artificial in-water structures to protect restoration trials is not new, and some have been shown to improve survival of both transplanted shoots and seedlings (Campbell and Paling, 2003; Tuya et al., 2017). For example, artificial seagrass units (Figure 2) which mimic the physical properties of seagrass have been shown to enhance aspects of the habitat, such as stabilizing sediment grain size (Campbell and Paling, 2003), preventing herbivory (Tuya et al., 2017). However, artificial seagrass is often made of plastic, and given the growing awareness of marine plastic pollution (Haward, 2018), its use in restoration is generally undesirable. To counter the use of plastic, researchers are developing artificial seagrass made entirely out of fully biodegradable materials to help facilitate restoration without the plastic footprint (The SeaArt Project, 2020).
Artificial in-water structures can also be used as anchoring devices to increase the chance of transplant unit survival. These include tying seagrass shoots to metal frames which are lowered to the seafloor (e.g., Transplanting Eelgrass Remotely with Frame Systems (TERFS), Calumpong and Fonseca, 2001; Wendländer et al., 2020), or to oyster shells (Lee and Park, 2008). These methods increase restoration success through ensuring adequate anchoring and tend to be more cost-effective as they do not require the planting of individual shoots one at a time.
Biodegradable materials, such as hessian and jute, have also been trialed with great success. These materials can promote the establishment of naturally dispersing seedlings (Tanner, 2015), protect seeds from predation (Orth et al., 2006), enhance survival of restored shoots (Ferretto et al., 2019), and exclude bioturbating animals, thus increasing survival rates (Wendländer et al., 2020). The use of these biodegradable products should continue to be explored to improve seagrass restoration outcomes through the challenging establishment phase, although their utility will likely vary with location.
Seagrass propagules are often limited and highly seasonal, and the collection of transplantation material could potentially put greater risks on donor meadows. Thus, alternative sources of transplant units are required to minimize the overall negative impact of sourcing restoration material. A potentially viable source of transplant units is seagrass wrack, detached biomass transported by wind and tides and accumulated on beaches globally (Macreadie et al., 2017). Seagrass wrack has many important ecological functions (Kirkman and Kendrick, 1997; Ince et al., 2007; Del Vecchio et al., 2017), but can also pose problems for coastal managers as its over-accumulation is often viewed as a nuisance by the public and high costs are incurred in their removal (Macreadie et al., 2017). Efforts are already being made to make use of this valuable resource, such as during the aquaculture of P. australis seedlings where it was recommended as a low cost and readily available nutritional supplement in restoration (Statton et al., 2013). Beyond its use as a nutritional supplement, seagrass wrack can also be a valuable source of transplant material. Terrados et al. (2013) used Posidonia oceanica seedlings from beach-cast fruits for seagrass plantings and obtained relatively high success, with 44% survival for 3 years. The use of wrack-collected seedlings in culturing and outplanting was also trialed in Australia, albeit to lower success. Wrack-collected P. angustifolia seedlings showed survival of 6–9% after 11 months in aquaculture, and survival upon outplanting was also low (Irving et al., 2010). Storm-generated rhizome fragments of Posidonia found within the wrack have also been used successfully for restoration in the Mediterranean (Balestri et al., 2011) and are currently being used successfully to restore P. australis in Australia (Ferretto et al., 2019; Figure 2).
Harnessing positive biological interactions can increase restoration success (Halpern et al., 2007; Silliman et al., 2015; Gagnon et al., 2020). Biological interactions in seagrasses include plant-substrate, plant-microbial communities, plant-plant (both intra- and interspecific), and between seagrass and other marine organisms/species such as shellfish, mangroves, and coral reefs. Many of these interactions are currently well-understood, and should be considered and included during restoration.
Plant-bivalve interactions have been shown to be largely positive, with a review which included all marine angiosperms (i.e., seagrass, salt marshes, mangroves, and freshwater submerged aquatic vegetation) showing that 70% of studies with a restoration focus showed positive interactions compared to 5% for negative interactions (Gagnon et al., 2020). For example, oyster reefs have been shown to facilitate seagrass productivity through a variety of mechanisms. Oyster reefs enhance conditions for seagrass growth through provision of physical protection from wave action (Piazza et al., 2005), improve water clarity through filtering particulate organic matter (Plutchak et al., 2010), and increase sedimentation and nutrient inputs through addition of feces (Newell and Koch, 2004). Like seagrasses, shellfish reefs are also some of the most degraded marine ecosystems globally (Beck et al., 2011), and a cross-ecosystem restoration approach could benefit both oyster reefs and seagrasses. For example, restored oyster reefs in the Northern Gulf of Mexico resulted in an increase in seagrass cover which was not observed in nearby areas (Sharma et al., 2016). New Zealand provides an ideal location to incorporate seagrass restoration with existing shellfish restoration efforts that are a major focus of Māori coastal research (e.g., Mullard, 2018). Similarly, several shellfish restoration projects have also been initiated in Australia (Gillies et al., 2018). The linkages between healthy shellfish and seagrass are a stepping stone to increase focus on seagrass restoration in a holistic manner.
Similarly, plant-soil feedbacks are emerging as an important area in terrestrial restoration projects, where interactions between plants, soil and soil microorganisms can have positive impacts on ecosystem functions. As marine angiosperms with root and rhizome systems anchored in sediments, seagrasses exist in a unique ecological niche in the marine system as organisms that create underwater rhizospheres. The composition of the microbiome on seagrass roots or within rhizospheres is an important indicator of seagrass health, and is already being applied for seagrass monitoring purposes (Trevathan-Tackett et al., 2019; Martin et al., 2020a). As an extension, understanding seagrass-microbial interactions could allow us to manipulate the seagrass microbiome in order to increase restoration success, given the strong effect the microbiome can have on sediment biogeochemical processes. For example, the bacterium Ca. Thiodiazotropha oxidizes sulfides in sediments, which can prevent sulfide intrusion, a driver that is known to hinder seagrass recolonization (Fraser and Kendrick, 2017). These bacteria are present in lucinid clam gills (van der Heide et al., 2012) but have also been found growing directly on the roots of numerous different seagrass species and may alleviate sulfide stress (Martin et al., 2020b). Fundamental research is required to further understand the nature of similar seagrass-microbe interactions in a natural context, before potentially beneficial bacteria (like Ca. Thiodiazotropha) are identified and incorporated into restoration plans.
Lastly, the current consensus in seagrass restoration is to arrange transplanted seeds, fragments or cores spaced apart to reduce competition, allowing for increased growth and survival (Halpern et al., 2007). However, this negates the benefits of self-facilitation (van der Heide et al., 2007). Saltmarsh restoration experiments carried out in the Western and Eastern Atlantic found that planting saltmarsh propagules in close proximity increased yields by 107% on average compared to dispersed planting (Silliman et al., 2015). Facilitation can also occur between seagrass species. Compressed succession was used in a restoration project in the Florida Keys National Marine Sanctuary, whereby Halodule wrightii, a fast-growing, opportunistic species was used to facilitate the recovery of Thalassia testudinum, a slow-growing, climax species by promoting more suitable conditions and reducing additional erosion (Fonseca et al., 2000; Kenworthy et al., 2018). While long-term monitoring in this study has shown that T. testudinum recolonization was still incomplete at 7 years (Furman et al., 2019), Tanner and Theil (2019) have found both Zostera and Posidonia seagrasses naturally recruiting within patches of restored Amphibolis.
These positive interactions could be especially important for species that are not abundant seed producers, and should be carefully considered to not only increase restoration success, but also potentially reduce donor meadow impact through making the best use of the plants harvested.
A longstanding limitation in seagrass restoration is the high labor costs associated with collection of restoration material and deployment of transplant units. This cost can be greatly reduced by engaging “citizen scientists” or volunteers. Community involvement in seagrass conservation is not new, with long term community-based monitoring programs in Queensland (McKenzie et al., 2000) and Western Australia (McKenzie et al., 2017). Well-established volunteer-based programs are often associated with assigning simple, realistic, achievable and locally appropriate tasks (Danielsen et al., 2005), while keeping volunteers and the wider community up to date on the progress or results of the program (Sharpe and Conrad, 2006). These same principles can be applied to seagrass restoration.
A few restoration trials in Australia and New Zealand have utilized community volunteers, such as the collection of Posidonia shoots detached after storms for transplantation3, community planting days on Kangaroo Island (Tanner et al., 2014), engaging recreational fishers in broadcasting seagrass seeds (Seeds for Snapper)4, collection of Z. muelleri spathes for seed-based restoration in partnership with Indigenous sea rangers (Gidarjil Development Corporation) in the Port of Gladstone (Central Queensland University, 2020), and participation in seagrass transplantation trials in Whangarei and Porirua Harbors, New Zealand (F.E. Matheson, NIWA, pers. comm.). These were done in consultation with restoration ecologists to ensure appropriate methods were deployed. Involving community members in restoration and subsequent monitoring of restoration success not only raises awareness but creates a sense of ownership and encourages volunteers to return and donate more of their time (Tanner et al., 2014). Volunteers are a valuable resource for seagrass restoration, and there is an opportunity to engage with growing public awareness surrounding marine conservation and willingness to participate in citizen science to supplement current restoration efforts (Martin et al., 2016).
Indigenous cultures have been keen observers and active managers of their natural environment for thousands of years, and have long-held cultural and traditional responsibilities to protect and manage their land and sea country. They can provide valuable insights, observations, and interpretations relating to the state of the biological, physical, and spiritual environments (Ens et al., 2015). The Aboriginal and Torres Strait Islander peoples in Australia are important landowners and managers of coastal land and sea Country through native title bodies, cultural and natural resource management organizations, and other corporations (McLeod et al., 2018). In New Zealand, the Treaty of Waitangi (Te Tiriti O Waitangi) underpins the Crown-Māori relationship and Treaty settlements are enabling Māori to be increasingly involved in resource management as owners, managers or co-managers of strategic environmental assets.
Integration of western science and Traditional Ecological Knowledge (TEK) through collaborations is likely to provide improved outcomes for restoration activities, as well as improved custodianship of the environment by all stakeholders into the future (Aronson et al., 2020). In Australia, national guidelines for engagement of Traditional Owners in research exist (see AIATSIS, 2012) and workshops at the Australian Marine Science Association annual conferences have been key to establishing protocols for collaborations on sea country (e.g., Hedge and Bessen, 2019), and protocols/strategies are being developed to establish collaborative and respectful partnerships for sea country research, conservation, restoration, and monitoring. In New Zealand, it is recommended that restoration initiatives be grounded in tikanga Māori and Māori values and perspectives, and be co-designed with Māori to ensure benefit and utility to Māori (Williams et al., 2018).
TEK is useful in countering perceptions around “shifting baselines,” especially in “pristine” conservation areas (Jardine, 2019), and observations and whole system approaches to ecosystem health and climate change (e.g., Mantyka-Pringle et al., 2017; Nursey-Bray et al., 2019). However, the needs and aspirations of Traditional Owners should be kept at the forefront. It is important to consider how Traditional Owners and the local community are likely to benefit from a collaborative project. Facilitating time on Country and aligning research questions with the land and sea management aspirations of the local Indigenous community can be developed through employment and training opportunities. This requires extensive pre-planning and sufficient time to consult with Traditional Owners prior to a project commencing. An Australian example from Western Australia’s Dampier Peninsula includes a collaboration between Western Scientists and the Bardi Jawi Indigenous rangers who have joint objectives to incorporate indigenous knowledge into the management of over 250 km of Kimberley coastline (Depczynski et al., 2019).
A new program for assisting seagrass recovery in Shark Bay is also a collaborative effort between western scientists and newly trained Malgana Indigenous rangers. Such collaborations with Indigenous sea ranger programs provide a great model to facilitate restoration and assist the existing traditional custodianship of Sea Country into the future. New Zealand Māori have been important initiators, partners and/or supporters of seagrass restoration projects in Whangarei Harbor (i.e., Whangarei Harbor kaitiaki roopu, Reed et al., 2004), Bay of Islands (i.e., Ngāti Kuta-Patukeha, Matheson et al., 2010), and Porirua Harbor (i.e., Ngāti Toa, Matheson and Wadhwa, 2012).
There is a growing number of seagrass restoration success stories and an increasing number of researchers and practitioners in seagrass restoration in Australia and New Zealand. However, there are still many knowledge gaps that need to be filled, especially surrounding species-specific studies and the lack of knowledge for tropical Australian species to avoid generalization of restoration techniques. This is further exacerbated by a lack of funding, as restoration is often perceived as too expensive and wrought with failures. We have now reached a point where ecologically meaningful large-scale seagrass restoration is possible given enough scientific, community, and political support. Restoration success rates are improving globally, and while future failures cannot be ruled out, they will offer guidance for improving subsequent attempts. Seagrass meadows continue to be threatened by anthropogenic impacts, so it is imperative that we attempt to stem the decline and work toward restoring degraded habitats. A lot of the hard work has now occurred, and we should now build upon our collective knowledge, engage with emerging tools, technology, and techniques, and maintain and build our research effort into seagrass restoration in Australia and New Zealand.
All authors contributed to the writing of the manuscript and gave final approval for publication.
This manuscript was the result of two workshops, one conducted at Deakin University and a second at Department of Environment and Energy, Canberra, that was sponsored by the Australian Government’s National Environmental Science Program (NESP) Marine Biodiversity Hub. ES was supported by an Australian Research Council grant to GK (DP180100668). This work was supported by The Nature Conservancy and the Marine Biodiversity Hub, a collaborative partnership supported through funding from the Australian Government’s National Environmental Science Program (NESP) Marine Biodiversity Hub.
The authors declare that the research was conducted in the absence of any commercial or financial relationships that could be construed as a potential conflict of interest.
The reviewer RL declared a past supervisory role with one of the authors OD to the handling editor.
The original impetus for this study originated from two practitioner workshops hosted by Deakin University (2017) and Department of the Environment and Energy (2018). We thank additional workshop participants Simon Branigan, John Ford, Steffan Howe, Paul Maxwell, Erik Paling, and Brooke Sullivan for their contributions, and Harriet Davies and Dr. Erica Williams for their advice on Indigenous engagement. We would also like to thank the two reviewers for their constructive comments to improve this manuscript.
The Supplementary Material for this article can be found online at: https://www.frontiersin.org/articles/10.3389/fmars.2020.00617/full#supplementary-material
AIATSIS (2012). Guidelines for Ethical Research in Australian Indigenous Studies. Canberra, CBR: Australian Institute of Aboriginal and Torres Strait Islander Studies.
Aitken, S. N., and Whitlock, M. C. (2013). Assisted gene flow to facilitate local adaptation to climate change. Annu. Rev. Ecol. Evol. Syst. 44, 367–388. doi: 10.1146/annurev-ecolsys-110512-135747
Ambo-Rappe, R., and Yasir, I. (2015). The effect of storage condition on viability of Enhalus acoroides seedlings. Aquat. Bot. 127, 57–61. doi: 10.1016/j.aquabot.2015.07.004
Anderson, T. J., Morrison, M., MacDiarmid, A., Clark, M., D’Archino, R., Nelson, W., et al. (2019). Review of New Zealand’s Key Biogenic Habitats Report 2018139WN. Auckland: National Institute of Water and Atmospheric Research, 190.
Arias-Ortiz, A., Serrano, O., Masqué, P., Lavery, P. S., Mueller, U., Kendrick, G. A., et al. (2018). A marine heatwave drives massive losses from the world’s largest seagrass carbon stocks. Nat. Clim. Change 8, 338–344. doi: 10.1038/s41558-018-0096-y
Aronson, J., Goodwin, N., Orlando, L., Eisenberg, C., and Cross, A. T. (2020). A world of possibilities: six restoration strategies to support the United Nation’s decade on ecosystem restoration. Restor. Ecol. 28, 730–736. doi: 10.1111/rec.13170
Atlantic Forest Restoration Pact (2016). A Movement of Brazilian Society to Restore and Transform Their Most Threatened Biome. Availale at: https//www.pactomataatlantica.org.br/the-pact (accessed May 28, 2020).
Babcock, R. C., Bustamante, R. H., Fulton, E. A., Fulton, D. J., Haywood, M. D. E., Hobday, A. J., et al. (2019). Severe continental-scale impacts of climate change are happening now: extreme climate events impact marine habitat forming communities along 45% of Australia’s coast. Front. Mar. Sci. 6:411. doi: 10.3389/fmars.2019.00411
Balestri, E., Vallerini, F., and Lardicci, C. (2011). Storm-generated fragments of the seagrass Posidonia oceanica from beach wrack – a potential source of transplants for restoration. Biol. Conserv. 144, 1644–1654. doi: 10.1016/j.biocon.2011.02.020
Bansal, S., Harrington, C., Gould, P. J., and St.Clair, J. B. (2015). Climate-related genetic variation in drought-resistance of Douglas-fir (Pseudotsuga menziesii). Glob. Change Biol. 21, 947–958. doi: 10.1111/gcb.12719
Bastyan, G. R., and Cambridge, M. L. (2008). Transplantation as a method for restoring the seagrass Posidonia australis. Estuar. Coast. Shelf Sci. 79, 289–299. doi: 10.1016/j.ecss.2008.04.012
Bayraktarov, E., Saunders, M. I., Abdullah, S., Mills, M., Beher, J., Possingham, H. P., et al. (2016). The cost and feasibility of marine coastal restoration. Ecol. Appl. 26, 1055–1074. doi: 10.1890/15-1077
Beck, M. W., Brumbaugh, R. D., Airoldi, L., Carranza, A., Coen, L. D., Crawford, C., et al. (2011). Oyster reefs at risk and recommendations for conservation, restoration, and management. Bioscience 61, 107–116. doi: 10.1525/bio.2011.61.2.5
Benito-Garzón, M., Ha-Duong, M., Frascaria-Lacoste, N., and Fernández-Manjarrés, J. (2013). Habitat restoration and climate change: dealing with climate variability, incomplete data, and management decisions with tree translocations. Restor. Ecol. 21, 530–536. doi: 10.1111/rec.12032
Bennett, S., Wernberg, T., Arackal Joy, B., de Bettignies, T., and Campbell, A. H. (2015). Central and rear-edge populations can be equally vulnerable to warming. Nat. Commun. 6:10280. doi: 10.1038/ncomms10280
Boudouresque, C., Pergent, G., Pergent-Martini, C., Ruitton, S., Thibaut, T., and Verlaque, M. (2016). The necromass of the Posidonia oceanica seagrass meadow: fate, role, ecosystem services and vulnerability. Hydrobiologia 781, 25–42. doi: 10.1007/s10750-015-2333-y
Broadhurst, L. M., Lowe, A., Coates, D. J., Cunningham, S. A., McDonald, M., Vesk, P. A., et al. (2008). Seed supply for broadscale restoration: maximizing evolutionary potential. Evol. Appl. 1, 587–597. doi: 10.1111/j.1752-4571.2008.00045.x
Browne, L., Wright, J. W., Fitz-Gibbon, S., Gugger, P. F., and Sork, V. L. (2019). Adaptational lag to temperature in valley oak (Quercus lobata) can be mitigated by genome-informed assisted gene flow. Proc. Natl. Acad. Sci. U.S.A. 116, 25179–25185. doi: 10.1073/pnas.1908771116
Bryars, S., and Neverauskas, V. (2004). Natural recolonisation of seagrasses at a disused sewage sludge outfall. Aquat. Bot. 80, 283–289. doi: 10.1016/j.aquabot.2004.09.001
Bulmer, R., Kelly, S., and Jeffs, A. G. (2016). Light requirements of the seagrass, Zostera muelleri, determined by observations at the maximum depth limit in a temperate estuary, New Zealand. N. Z. J. Mar. Freshw. Res. 50, 183–194. doi: 10.1080/00288330.2015.1120759
Busch, K. E., Golden, R. R., Parham, T. A., Karrh, L. P., Lewandowski, M. J., and Naylor, M. D. (2010). Large-Scale Zostera marina (eelgrass) restoration in Chesapeake Bay, Maryland, USA. Part I: a comparison of techniques and associated costs. Restor. Ecol. 18, 490–500. doi: 10.1111/j.1526-100X.2010.00690.x
Byars, S. G., Papst, W., and Hoffmann, A. A. (2007). Local adaptation and cogradient selection in the alpine plant, Poa Hiemata, along a narrow altitudinal gradient. Evoloution 61, 2925–2941. doi: 10.1111/j.1558-5646.2007.00248.x
Calumpong, H. P., and Fonseca, M. S. (2001). “Seagrass transplantation and other seagrass restoration methods,” in Global Seagrass Research Methods, eds F. T. Short and R. G. Coles (Amsterdam: Elsevier Science), 425–443. doi: 10.1016/b978-044450891-1/50023-2
Campbell, M. L. (2002). Getting the foundation right: a scientifically based management framework to aid in the planning and implementation of seagrass transplant efforts. Bull. Mar. Sci. 71, 1405–1414.
Campbell, M. L. (2003). Recruitment and colonisation of vegetative fragments of Posidonia australis and Posidonia coriacea. Aquat. Bot. 76, 175–184. doi: 10.1016/S0304-3770(03)00016-0
Campbell, M. L., Heppenstall, L. D., Hendry, R., Martin, R., Sørensen, S., Rubenstein, A. N., et al. (2018). Niche partitioning of intertidal seagrasses: evidence of the influence of substrate temperature. New Phytol. 217, 1449–1462. doi: 10.1111/nph.14944
Campbell, M. L., and Paling, E. I. (2003). Evaluating vegetative transplant success in Posidonia australis: a field trial with habitat enhancement. Mar. Pollut. Bull. 46, 828–834. doi: 10.1016/S0025-326X(03)00093-6
Carlson, D. F., Yarbro, L. A., Scolaro, S., Poniatowski, M., McGee-Absten, V., and Carlson, P. R. (2018). Sea surface temperatures and seagrass mortality in Florida Bay: spatial and temporal patterns discerned from MODIS and AVHRR data. Remote Sens. Environ. 208, 171–188. doi: 10.1016/j.rse.2018.02.014
Carstensen, J., Krause-Jensen, D., Markager, S., Timmermann, K., and Windolf, J. (2013). Water clarity and eelgrass responses to nitrogen reductions in the eutrophic Skive Fjord. Denmark. Hydrobiologia 704, 293–309. doi: 10.1007/s10750-012-1266-y
Central Queensland University (2020). Research Projects: Restoring the “kidneys” of the Great Barrier Reef. Norman Gardens, QLD: Central Queensland University.
Cole, K. L., Ironside, K., Eischeid, J., Garfin, G., Duffy, P. B., and Toney, C. (2011). Past and ongoing shifts in Joshua tree distribution support future modeled range contraction. Ecol. Appl. 21, 137–149. doi: 10.1890/09-1800.1
Coles, R., Rasheed, M., McKenzie, L., Grech, A., York, P., Sheaves, M., et al. (2015). The great barrier reef world heritage area seagrasses: managing this iconic Australian ecosystem resource for the future. Estuar. Coast. Shelf Sci. 153, A1–A12. doi: 10.1016/j.ecss.2014.07.020
Collier, C. J., and Waycott, M. (2014). Temperature extremes reduce seagrass growth and induce mortality. Mar. Pollut. Bull. 83, 483–490. doi: 10.1016/j.marpolbul.2014.03.050
Connolly, R. M., Dunn, R. J. K., Flindt, M. R., Jackson, E. L., Kristensen, E., McKenna, S., et al. (2016). Assessment of the Effects of Foreshore Nourishment and Mitigation Projects on Seagrass Ecosystems (SRMP-004). Report to Gold Coast Waterways Authority. Gold Coast: Griffith University.
Connolly, R. M., Smith, T. M., Maxwell, P. S., Olds, A. D., Macreadie, P. I., et al. (2018). Highly disturbed habitats show increased resistance but lower genotypic diversity in a foundation marine macrophyte. Front. Plant Sci. 9:894.
Cooke, J. A., and Johnson, M. S. (2002). Ecological restoration of land with particular reference to the mining of metals and industrial minerals: a review of theory and practice. Environ. Rev. 10, 41–71. doi: 10.1139/a01-014
Crutsinger, G. M., Collins, M. D., Fordyce, J. A., Gompert, Z., Nice, C. C., and Sanders, N. J. (2006). Plant genotypic diversity predicts community structure and governs an ecosystem process. Science 313, 966–968. doi: 10.1126/science.1128326
Cullen-Unsworth, L. C., and Unsworth, R. K. F. (2016). Strategies to enhance the resilience of the world’s seagrass meadows. J. Appl. Ecol. 53, 967–972. doi: 10.1111/1365-2664.12637
Cumming, E., Jarvis, J. C., Sherman, C. D. H., York, P. H., and Smith, T. M. (2017). Seed germination in a southern Australian temperate seagrass. PeerJ 5:e3114. doi: 10.7717/peerj.3114
Cussioli, M. C., Bryan, K. R., Pilditch, C. A., de Lange, W. P., and Bischoff, K. (2019). Light penetration in a temperate meso-tidal lagoon: implications for seagrass growth and dredging in Tauranga Harbour, New Zealand. Ocean Coast. Manage. 174, 25–37. doi: 10.1016/j.ocecoaman.2019.01.014
Cussioli, M. C., Seeger, D., Pratt, D. R., Bryan, K. R., Bischoff, K., de Lange, W. P., et al. (2020). Spectral differences in the underwater light regime caused by sediment types in New Zealand estuaries: implications for seagrass photosynthesis. Geo-Mar. Lett. 40, 217–225. doi: 10.1007/s00367-020-00640-0
Dafforn, K. A. (2017). Eco-engineering and management strategies for marine infrastructure to reduce establishment and dispersal of non-indigenous species. Biol. Invasions 8, 153–161. doi: 10.3391/mbi.2017.8.2.03
Danielsen, F., Burgess, N. D., and Balmford, A. (2005). Monitoring matters: examining the potential of locally-based approaches. Biodivers. Conserv. 14, 2507–2542. doi: 10.1007/s10531-005-8375-0
de la Torre-Castro, M., Di Carlo, G., and Jiddawi, N. S. (2014). Seagrass importance for a small-scale fishery in the tropics: the need for seascape management. Mar. Pollut. Bull. 83, 398–407. doi: 10.1016/j.marpolbul.2014.03.034
de Lange, P. J., Rolfe, J. R., Barkla, J. W., Courtney, S. O., Champion, P. D., et al. (2017). Conservation Status of New Zealand Indigenous Vascular Plants, 2017. New Zealand Threat Classification Series 22. Wellinton: Department of Conservation, 82.
Del Vecchio, S., Jucker, T., Carboni, M., and Acosta, A. T. R. (2017). Linking plant communities on land and at sea: the effects of Posidonia oceanica wrack on the structure of dune vegetation. Estuar. Coast. Shelf Sci. 184, 30–36. doi: 10.1016/j.ecss.2016.10.041
Department for Environment and Water (2017). Seagrass Restoration. Adelaide: Department for Environment and Water.
Department of Environmental Affairs (2019). Working for Water (WfW) Programme. Pretoria: Department of Environmental Affairs.
Depczynski, M., Cook, K., Cure, K., Davies, H., Evans-Illidge, L., Forester, T., et al. (2019). Marine monitoring of Australia’s indigenous sea country using remote technologies. J. Ocean Technol. 14, 60–75.
Dos Santos, V. M., and Matheson, F. E. (2016). Higher seagrass cover and biomass increases sexual reproductive effort: a rare case study of Zostera muelleri in New Zealand. Aquat. Bot. 138, 29–36. doi: 10.1016/j.aquabot.2016.12.003
Dos Santos, V. M., Matheson, F. E., Pilditch, C. A., and Elger, A. (2012). Is black swan grazing a threat to seagrass: indications from an observations study in New Zealand. Aquat. Bot. 100, 41–50. doi: 10.1016/j.aquabot.2012.03.009
Duarte, C. M. (1991). Seagrass depth limits. Aquat. Bot. 40, 363–377. doi: 10.1016/0304-3770(91)90081-F
Duffy, J. E., Benedetti-Cecchi, L., Trinanaes, J., Muller-Karger, F. E., Ambo-Rappe, R., Boström, C., et al. (2019). Toward a coordinated global observing system for seagrasses and marine macroalgae. Front. Mar. Sci. 6:317. doi: 10.3389/fmars.2019.00317
Duffy, J. P., Pratt, L., Anderson, K., Land, P. E., and Shutler, J. D. (2018). Spatial assessment of intertidal seagrass meadows using optical imaging systems and a lightweight drone. Estuar. Coast. Shelf Sci. 200, 169–180. doi: 10.1016/j.ecss.2017.11.001
Ens, E. J., Pert, P., Clarke, P. A., Budden, M., Clubb, L., Doran, B., et al. (2015). Indigenous biocultural knowledge in ecosystem science and management: review and insight from Australia. Biol. Conserv. 181, 133–149. doi: 10.1016/j.biocon.2014.11.008
Erftemeijer, P. L. A., and Middelburg, J. J. (1993). Sediment-nutrient interactions in tropical seagrass beds: a comparison between a terrigenous and a carbonate sedimentary environment in South Sulawesi (Indonesia). Mar. Ecol. Prog. Ser. 102, 187–198. doi: 10.3354/meps102187
Evans, S. M., Sinclair, E. A., Poore, A. G. B., Steinberg, P. D., Kendrick, G. A., and Vergés, A. (2014). Genetic diversity in threatened Posidonia australis seagrass meadows. Conservat. Genet. 15, 717–728. doi: 10.1007/s10592-014-0573-4
Ferretto, G., Glasby, T. M., Housefield, G. P., Poore, A. G. P., Statton, J., Sinclair, E. A., et al. (2019). Threatened plant translocation case study: Posidonia australis (strapweed), Posidoniaceae. Aus. Plant Conserv. 28, 24–26.
Fonseca, M. S., Julius, B. E., and Kenworthy, W. J. (2000). Integrating biology and economics in seagrass restoration: how much is enough and why? Ecol. Eng. 15, 227–237. doi: 10.1016/S0925-8574(00)00078-1
Fonseca, M. S., and Kenworthy, W. J. (1987). Effects of current on photosynthesis and distribution of seagrasses. Aquat. Bot. 27, 59–78. doi: 10.1016/0304-3770(87)90086-6
Fortes, M. D., Ooi, J. L. S., Tan, Y. M., Prathep, A., Bujang Japar, S., and Yaakub, S. M. (2018). Seagrass in Southeast Asia: a review of status and knowledge gaps, and a road map for conservation. Bot. Mar. 61, 269–288. doi: 10.1515/bot-2018-0008
Fraser, M. W., and Kendrick, G. A. (2017). Belowground stressors and long-term seagrass declines in a historically degraded seagrass ecosystem after improved water quality. Sci. Rep. 7:14469. doi: 10.1038/s41598-017-14044-1
Freeman, A. S., Short, F. T., Isnain, I., Razak, F. A., and Coles, R. G. (2008). Seagrass on the edge: land-use practices threaten coastal seagrass communities in Sabah, Malaysia. Biol. Conserv. 141, 2993–3005. doi: 10.1016/j.biocon.2008.09.018
Furman, B. T., Merello, M., Shea, C. P., Kenworthy, W. J., and Hall, M. O. (2019). Monitoring of physically restored seagrass meadows reveals a slow rate of recovery for Thalassia testudinum. Restor. Ecol. 27, 421–430. doi: 10.1111/rec.12877
Gagnon, K., Rinde, E., Bengil, E. G., Carugati, L., Christianen, M. J., Danovaro, R., et al. (2020). Facilitating foundation species: the potential for plant–bivalve interactions to improve habitat restoration success. J. Appl. Ecol. 57, 1161–1179. doi: 10.1111/1365-2664.13605
Gillies, C. L., McLeod, I. M., Alleway, H. K., Cook, P., Crawford, C., Creighton, C., et al. (2018). Australian shellfish ecosystems: past distribution, current status and future direction. PLoS One 13:e0190914. doi: 10.1371/journal.pone.0190914
Govers, L. L. (2018). “Set-backs and successes: seagrass restoration in the Dutch Wadden Sea,” Paper Presented to World Seagrass Conference, Singapore.
Grech, A., Chartrand-Miller, K., Erftemeijer, P., Fonseca, M., McKenzie, L., Rasheed, M., et al. (2012). A comparison of threats, vulnerabilities and management approaches in global seagrass bioregions. Environ. Res. Lett. 7:024006. doi: 10.1088/1748-9326/7/2/024006
Grech, A., Coles, R., and Marsh, H. (2011). A broad-scale assessment of the risk to coastal seagrasses from cumulative threats. Mar. Policy 35, 560–567. doi: 10.1016/j.marpol.2011.03.003
Grech, A., Hanert, E., McKenzie, L., Rasheed, M., Thomas, C., Tol, S., et al. (2018). Predicting the cumulative effect of multiple disturbances on seagrass connectivity. Glob. Change Biol. 24, 3093–3104. doi: 10.1111/gcb.14127
Greening, H. S., Cross, L. M., and Sherwood, E. T. (2011). A multiscale approach to seagrass recovery in Tampa Bay, Florida. Ecol. Restor. 29, 82–93. doi: 10.3368/er.29.1-2.82
Gullström, M., de la Torre Castro, M., Bandeira, S. O., Björk, M., Dahlberg, M., Kautsky, N., et al. (2002). Seagrass ecosystems in the Western Indian Ocean. Ambio 31, 588–596. doi: 10.1579/0044-7447-31.7.588
Halpern, B. S., Silliman, B. R., Olden, J. D., Bruno, J. P., and Bertness, M. D. (2007). Incorporating positive interactions in aquatic restoration and conservation. Front. Ecol. Environ. 5:153–160. doi: 10.1890/1540-929520075[153:IPIIAR]2.0.CO;2
Hampe, A., and Petit, R. J. (2005). Conserving biodiversity under climate change: the rear edge matters. Ecol. Lett. 8, 461–467. doi: 10.1111/j.1461-0248.2005.00739.x
Hancock, N., and Gallagher, R. (2014). How ready are we to move species threatened from climate change? Insights into the assisted colonization debate from Australia. Aust. Ecol. 39, 830–838. doi: 10.1111/aec.12151
Harley, C. D. G., Hughes, A. R., Hultgren, K. M., Miner, B. G., Sorte, C. J. B., Thornber, C. S., et al. (2006). The impacts of climate change in coastal marine systems. Ecol. Lett. 9, 228–241. doi: 10.1111/j.1461-0248.2005.00871.x
Haward, M. (2018). Plastic pollution of the world’s seas and oceans as a contemporary challenge in ocean governance. Nat. Commun. 9:667. doi: 10.1038/s41467-018-03104-3
Hawkins, S. J., Allen, J. R., Ross, P. M., and Genner, M. J. (2002). “Marine and coastal ecosystems,” in Handbook of Ecological Restoration Volume 2: Restoration in Practice, eds M. R. Perrow and A. J. Davy (Cambridge: Cambridge University Press), 121–148.
Hedge, P., and Bessen, B. (eds) (2019). “Indigenous Workshop: summary report – promoting collaborative partnerships for sea country research and monitoring in Western Australia,” in Proceedings of the 4th annual Indigenous workshop convened under a partnership between the NESP Marine Biodiversity Hub and the Australian Marine Sciences Association (Fremantle: AMSA Indigenous Workshop Organising Committee).
Hewitt, J. E., and Cummings, V. (2013). Context-dependent success of restoration if a key species biodiversity and community composition. Mar. Ecol. Prog. Ser. 479, 63–73. doi: 10.3354/meps10211
Hoegh-Guldberg, O., Hughes, L., McIntyre, S., Lindenmayer, D. B., Parmesan, C., Possingham, H. P., et al. (2008). Assisted colonization and rapid climate change. Science 321, 345–346. doi: 10.1126/science.1157897
Holmer, M., Duarte, C., and Marbá, N. (2005). Iron additions reduce sulfate reduction rates and improve seagrass growth on organic-enriched carbonate sediments. Ecosystem 8, 721–730. doi: 10.1007/s10021-003-0180-6
Ince, R., Hyndes, G. A., Lavery, P. S., and Vanderklift, M. A. (2007). Marine macrophytes directly enhance abundances of sandy beach fauna through provision of food and habitat. Estuar. Coast. Shelf Sci. 74, 77–86. doi: 10.1016/j.ecss.2007.03.029
Inglis, G. (2003). “The seagrasses of New Zealand,” in World Atlas of Seagrasses, eds E. P. Green and F. T. Short (Berkeley, CA: University of California Press), 134–143.
Irving, A. D., Tanner, J. E., Seddon, S., Miller, D., Collings, G. J., Wear, R. J., et al. (2010). Testing alternate ecological approaches to seagrass rehabilitation: links to life-history traits. J. Appl. Ecol. 47, 1119–1127. doi: 10.1111/j.1365-2664.2010.01852.x
Jacob, C., Buffard, A., Pioch, S., and Thorin, S. (2018). Marine ecosystem restoration and biodiversity offset. Ecol. Eng. 120, 585–594. doi: 10.1016/j.ecoleng.2017.09.007
Jänes, H., Macreadie, P. I., Nicholson, E., Ierodioconou, D., Reeves, S., Taylor, M. D., et al. (2019). Stable isotope infer the value of Australia’s coastal vegetated ecosystems from fisheries. Fish. Fish. 20, 80–90. doi: 10.1111/faf.12416
Jardine, T. D. (2019). Indigenous knowledge as a remedy for shifting baseline syndrome. Front. Ecol. Evol. 17:13–14. doi: 10.1002/fee.1991
Jordan, R., Breed, M. F., Prober, S. M., Miller, A. D., and Hoffmann, A. A. (2019). How well do revegetation plantings capture genetic diversity? Biol. Lett. 15:20190460. doi: 10.1098/rsbl.2019.0460
Jordan, R., Hoffmann, A. A., Dillon, S. K., and Prober, S. M. (2017). Evidence of genomic adaptation to climate in Eucalyptus microcarpa: implications for adaptive potential to projected climate change. Mol. Ecol. 26, 6002–6020. doi: 10.1111/mec.14341
Kendrick, G. A., Nowicki, R. J., Olsen, Y. S., Strydom, S., Fraser, M. W., Sinclair, E. A., et al. (2019). A systematic review of how multiple stressors from an extreme event drove ecosystem-wide loss of resilience in an iconic seagrass community. Front. Mar. Sci. 6:455. doi: 10.3389/fmars.2019.00455
Kendrick, G. A., Waycott, M., Carruthers, T. J. B., Cambridge, M. L., Hovey, R. K., Krauss, S. L., et al. (2012). The central role of dispersal in the maintenance and persistence of seagrass populations. Bioscience 62, 56–65. doi: 10.1525/bio.2012.62.1.10
Kenworthy, W. J., Hall, M. O., Hammerstrom, K. K., Merello, M., and Schwartzschild, A. (2018). Restoration of tropical seagrass beds using wild bird fertilization and sediment regrading. Ecol. Eng. 112, 72–81. doi: 10.1016/j.ecoleng.2017.12.008
Kirkman, H. (1989). Restoration and Creation of Seagrass Meadows with Special Emphasis on Western Australia. Technical Series No. 30. Perth: Environmental Protection Authority.
Kirkman, H. (1999). Pilot experiments on planting seedlings and small seagrass propagules in Western Australia. Mar. Pollut. Bull. 37, 460–467. doi: 10.1016/S0025-326X(99)00146-0
Kirkman, H., and Kendrick, G. A. (1997). Ecological significance and commercial harvesting of drifting and beach-cast macro-algae and seagrasses in Australia: a review. J. Appl. Phycol. 9, 311–326. doi: 10.1023/a:1007965506873
Koch, M., Bowes, G., Ross, C., and Zhang, X. (2013). Climate change and ocean acidification effects on seagrasses and marine macroalgae. Glob. Change Biol. 19, 103–132. doi: 10.1111/j.1365-2486.2012.02791.x
Kohlmeier, D., Pilditch, C. A., Bornman, J. F., and Bischof, K. (2014). Site specific differences in morphometry and photophysiology in intertidal Zostera muelleri meadows. Aquat. Bot. 116, 104–109. doi: 10.1016/j.aquabot.2014.02.011
Lai, S., Yaakub, S. M., Poh, T. S. M., Bouma, T. J., and Todd, P. A. (2018). Unlikely nomads: settlement, establishment, and dislodgement processes of vegetative seagrass fragments. Front. Plant. Sci. 9:160. doi: 10.3389/fpls.2018.00160
Lamb, J. B., van de Water, J. A. J. M., Bourne, D. G., Altier, C., Hein, M. Y., Fiorenza, E. A., et al. (2017). Seagrass ecosystems reduce exposure to bacterial pathogens of humans, fishes, and invertebrates. Science 355, 731–733. doi: 10.1126/science.aal1956
Leathwick, J. R., Moilanen, A., Francis, M., Elith, J., and Taylor, P. (2008). Novel methods for the design and evaluation of marine protected areas in offshore waters. Cons. Lett. 1, 91–102. doi: 10.1111/j.1755-263X.2008.00012.x
Ledig, F. T., Rehfeldt, G. E., and Jaquish, B. (2012). Projections of suitable habitat under climate change scenarios: implications for trans-boundary assisted colonization. Am. J. Bot. 99, 1217–1230. doi: 10.3732/ajb.1200059
Lee, K.-S., and Park, J.-I. (2008). An effective transplanting technique using shells for restoration of Zostera marina habitats. Mar. Pollut. Bull. 56, 1015–1021. doi: 10.1016/j.marpolbul.2008.02.010
Lefcheck, J. S., Marion, S. R., and Orth, R. J. (2017). Restored Eelgrass (Zostera marina L.) as a refuge for epifaunal biodiversity in mid-western Atlantic coastal bays. Estuar. Coasts 40, 200–212. doi: 10.1007/s12237-016-0141-x
Leimu, R., Mutikainen, P., Koricheva, J., and Fischer, M. (2006). How general are positive relationships between plant population size, fitness and genetic variation? J. Ecol. 94, 942–952. doi: 10.1111/j.1365-2745.2006.01150.x
Leschen, A. S., Ford, K. H., and Evans, N. T. (2010). Successful eelgrass (Zostera marina) restoration in a formerly eutrophic estuary (Boston Harbor) supports the use of a multifaceted watershed approach to mitigating eelgrass loss. Estuar. Coasts 33, 1340–1354. doi: 10.1007/s12237-010-9272-7
Li, M., Lundquist, C. J., Pilditch, C. A., Rees, T. A. V., and Ellis, J. (2019). Implications of nutrient enrichment for the conservation and management of Zostera muelleri meadows. Aquat. Conserv. Mar. Freshw. Ecosyst. 29, 1484–1502. doi: 10.1002/aqc.3141
Loke, L. H. L., Bouma, T., and Todd, P. (2017). The effects of manipulating microhabitat size and variability on tropical seawall biodiversity: field and flume experiments. J. Exp. Mar. Biol. Ecol. 492, 113–120. doi: 10.1016/j.jembe.2017.01.024
Lord, D., Paling, E., and Gordon, D. (1999). “Review of Australian rehabilitation and restoration programs,” in Seagrass in Australia. Strategic Review and Development of an R&D plan, eds A. Butler and P. Jernakoff (Clayton: CSIRO Publishing), 65–115.
Lundquist, C. J., Jones, T. C., Parkes, S. M., and Bulmer, R. H. (2018). Changes in benthic community structure and sediment characteristics after natural recolonisation of the seagrass Zostera muelleri. Sci. Rep. 8:13250. doi: 10.1038/s41598-018-31398-2
Macreadie, P. I., Baird, M. E., Trevathan-Tackett, S. M., Larkum, A. W. D., and Ralph, P. J. (2014). Quantifying and modelling the carbon sequestration capacity of seagrass meadows – a critical assessment. Mar. Pollut. Bull. 83, 430–439. doi: 10.1016/j.marpolbul.2013.07.038
Macreadie, P. I., Trevathan-Tackett, S. M., Baldock, J. A., and Kelleway, J. J. (2017). Converting beach-cast seagrass wrack into biochar: a climate-friendly solution to a coastal problem. Sci. Total Environ. 574, 90–94. doi: 10.1016/j.scitotenv.2016.09.021
Mantyka-Pringle, C. S., Jardine, T. D., Bradford, L., Bharadwaj, L., Kythreotis, A. P., Fresque-Baxter, J., et al. (2017). Bridging science and traditional knowledge to assess cumulative impacts of stressors on ecosystem health. Environ. Int. 102, 125–137. doi: 10.1016/j.envint.2017.02.008
Marbà, N., and Duarte, C. M. (2010). Mediterranean warming triggers seagrass (Posidonia oceanica) shoot mortality. Glob. Change Biol. 16, 2366–2375. doi: 10.1111/j.1365-2486.2009.02130.x
Marion, S. R., and Orth, R. J. (2010). Innovative techniques for large-scale seagrass restoration using Zostera marina (eelgrass) seeds. Restor. Ecol. 18, 514–526. doi: 10.1111/j.1526-100X.2010.00692.x
Marsden, I. D., and Adkins, S. C. (2010). Current stock of cockle bed restoration in New Zealand. Aquacult. Int. 18, 83–97. doi: 10.1007/s10499-009-9270-6
Martin, B. C., Alarcon, M. S., Gleeson, D., Middleton, J. A., Fraser, M. W., Ryan, M. H., et al. (2020a). Root microbiomes as indicators of seagrass health. FEMS Microbiol. Ecol. 96:fiz201. doi: 10.1093/femsec/fiz201
Martin, B. C., Middleton, J. A., Fraser, M. W., Marshall, I. P., Scholz, V. V., and Schmidt, H. (2020b). Cutting out the middle clam: lucinid endosymbiotic bacteria are also associated with seagrass roots worldwide. bioRxiv doi: 10.1101/2020.05.06.080127
Martin, V., Smith, L., Bowling, A., Christidis, L., Lloyd, D., and Pecl, G. (2016). Citizens as Scientists: what influences public contributions to marine research? Sci. Commun. 38, 495–522. doi: 10.1177/1075547016656191
Martins, G. M., Thompson, R. C., Neto, A. I., Hawkins, S. J., and Jenkins, S. R. (2010). Enhancing stocks of the exploited limpet Patella candei d’Orbigny via modifications in coastal engineering. Biol. Conserv. 143, 203–211. doi: 10.1016/j.biocon.2009.10.004
Matheson, F., and Wadhwa, S. (2012). Seagrass in Porirua Harbour: Preliminary Assessment of Restoration Potential. NIWA Report no. HAM2012-037. Hamilton: NIWAR.
Matheson, F., Wadhwa, S., Taumoepeau, A., and Smith, J. (2010). Seagrass in the Eastern Bay of Islands: Past and Present Abundance, Threats and Management Options. NIWA Report no. HAM2010-023. Hamilton: NIWAR.
Matheson, F. E., Lundquist, C. J., Gemmill, C. E. C., and Pilditch, C. A. (2011). New Zealand seagrass – more threatened than IUCN indicates. Biol. Conserv. 144, 2749–2750. doi: 10.1016/j.biocon.2011.08.020
Matheson, F. E., Reed, J., Dos Santos, V. M., Mackay, G., and Cummings, V. J. (2017). Seagrass rehabilitation: successful transplants and evaluation of methods at different spatial scales. N. Z. J. Mar. Freshw. Res. 51, 96–109. doi: 10.1080/00288330.2016.1265993
Matheson, F. E., Zabarte-Maeztu, I., Mackay, G., Middleton, C., Oliver, M., and Griffiths, R., et al. (submitted). Estuary muddiness and seasonally low light constrains seagrass restoration. Mar. Pollut. Bull.
McGlathery, K. J., Sundbäck, K., and Anderson, I. C. (2007). Eutrophication in shallow coastal bays and lagoons: the role of plants in the coastal filter. Mar. Ecol. Prog. Ser. 348, 1–18. doi: 10.3354/meps07132
McKenna, S., Jarvis, J., Sankey, T., Reason, C., Coles, R., and Rasheed, M. (2015). Declines of seagrasses in a tropical harbour, North Queensland, Australia, are not the result of a single event. J. Biosci. 40, 389–398. doi: 10.1007/s12038-015-9516-6
McKenzie, L., Lee Long, W., Coles, R., and Roder, C. (2000). Seagrass-watch: community based monitoring of seagrass resources. Biol. Mar. Mediterr. 7, 393–396.
McKenzie, L. J., Yoshida, R. L., Langlois, L., Rau, J., Weatherall, K., Bishop, F., et al. (2017). Long-term Seagrass Monitoring in Roebuck Bay, Broome: Report on the First 10 Years. A Report for the Broome Community Seagrass Monitoring Project, Environs Kimberley. Centre for Tropical Water & Aquatic Ecosystem Research (TropWATER) Report 17/35. Cairns: James Cook University, 44.
McLeod, I., Schmider, J., Creighton, C., and Gillies, C. (2018). Seven pearls of wisdom: advice from traditional owners to improve engagement of local indigenous people in shellfish ecosystem restoration. Ecol. Manag. Restor. 19, 98–101. doi: 10.1111/emr.12318
McMahon, K., Sinclair, E. A., Sherman, C. D. H., van Dijk, K.-J., Hernawan, U. E., Verduin, J. J., et al. (2018). “Genetic connectivity in tropical and temperate Australian Seagrass species,” in Seagrasses of Australia: Structure, Ecology and Conservation, eds A. W. D. Larkum, G. A. Kendrick, and P. J. Ralph (Cham: Springer International Publishing), 155–194. doi: 10.1007/978-3-319-71354-0_6
McMahon, K., van Dijk, K.-J., Ruiz-Montoya, L., Kendrick, G. A., Krauss, S. L., Waycott, M., et al. (2014). The movement ecology of seagrasses. P. R. Soc. B Biol. Sci. 281:20140878. doi: 10.1098/rspb.2014.0878
Miller, A. D., Coleman, M. A., Clark, J., Cook, R., Naga, Z., Doblin, M. A., et al. (2019a). Local thermal adaptation and limited gene flow constrain future climate responses of a marine ecosystem engineer. Ecol. Appl. 13, 918–934. doi: 10.1111/eva.12909
Miller, A. D., Hoffmann, A. A., Tan, M. H., Young, M., Ahrens, C., Cocomazzo, M., et al. (2019b). Local and regional scale habitat heterogeneity contribute to genetic adaptation in a commercially important marine mollusc (Haliotis rubra) from southeastern Australia. Mol. Ecol. 28, 3053–3072. doi: 10.1111/mec.15128
Miller, B. P., Sinclair, E. A., Menz, M. H. M., Elliott, C. P., Bunn, E., Commander, L. E., et al. (2017). A framework for the practical science necessary to restore sustainable, resilient and biodiverse ecosystems. Rest. Ecol. 25, 605–617. doi: 10.1111/rec.12475
Mills, V. S., and Berkenbusch, K. (2009). Seagrass (Zostera muelleri) patch size and spatial location influence infaunal macroinvertebrate assemblages. Estuar. Coast. Shelf Sci. 81, 123–129. doi: 10.1016/j.ecss.2008.10.005
Mitsch, W. J. (2012). What is ecological engineering? Ecol. Eng. 45, 5–12. doi: 10.1016/j.ecoleng.2012.04.013
Mohring, M. B., Wernberg, T., Wright, J. T., Connell, S. D., and Russell, B. D. (2014). Biogeographic variation in temperature drives performance of kelp gametophytes during warming. Mar. Ecol. Prog. Ser. 513, 85–96. doi: 10.3354/meps10916
Moilanen, A., Anderson, B. J., Eigenbrod, F., Heinemeyer, A., Roy, D. B., et al. (2011). Balancing alternative land uses in conservation prioritization. Ecol. Appl. 21, 1419–1426. doi: 10.1890/10-1865.1
Moksnes, P.-O., Gullström, M., Tryman, K., and Baden, S. (2008). Trophic cascades in a temperate seagrass community. Oikos 117, 763–777. doi: 10.1111/j.0030-1299.2008.16521.x
Morris, R. K. A., Alonso, I., Jefferson, R. G., and Kirby, K. J. (2006). The creation of compensatory habitat—Can it secure sustainable development? J. Nat. Conserv. 14, 106–116. doi: 10.1016/j.jnc.2006.01.003
Morrison, M. A., Lowe, M. L., Grant, C. M., Smith, P. J., Carbines, G., Reed, J., et al. (2014). Seagrass Meadows as Biodiversity and Productivity Hotspots. New Zealand Aquatic Environment and Biodiversity Report No. 137. Wellington: New Zealand Aquatic Environment and Biodiversity.
Mueller, J. T., and Hellman, J. T. (2008). An assessment of invasion risk from assisted migration. Conserv. Biol. 22, 562–567. doi: 10.1111/j.1523-1739.2008.00952.x
Mullard, A. (2018). Māori Mussel Memory. Available online at: https://www.hakaimagazine.com/news/maori-mussel-memory/ (accessed May 22, 2020).
Munkes, B. (2005). Eutrophication, phase shift, the delay and the potential return in the Greifswalder Bodden. Baltic Sea. Aquat. Sci. 67, 372–381. doi: 10.1007/s00027-005-0761-x
Nahirnick, N. K., Reshitnyk, L., Campbell, M., Hessing-Lewis, M., Costa, M., Yakimishyn, J., et al. (2019). Mapping with confidence; delineating seagrass habitats using unoccupied aerial systems (UAS). Remote Sens. Ecol. Conserv. 5, 121–135. doi: 10.1002/rse2.98
National Oceanic and Atmospheric Administration [NOAA] (2015). Guidance for Considering the Use of Living Shorelines. Silver Spring: NOAA
Nellemann, C., and Corcoran, E. (2010). Dead Planet, Living Planet - Biodiversity and Ecosystem Restoration for Sustainable Development. A Rapid Response Assessment. GRID-Arendal: United Nations Environment Programme.
Newell, R. I., and Koch, E. W. (2004). Modeling seagrass density and distribution in response to changes in turbidity stemming from bivalve filtration and seagrass sediment stabilization. Estuaries 27, 793–806. doi: 10.1007/BF02912041
Nordlund, L. M., Jackson, E. L., Nakaoka, M., Samper-Villarreal, J., Beca-Carretero, P., and Creed, J. C. (2018a). Seagrass ecosystem services – What’s next? Mar. Pollut. Bull. 134, 145–151. doi: 10.1016/j.marpolbul.2017.09.014
Nordlund, L. M., Unsworth, R. K. F., Gullström, M., and Cullen-Unsworth, L. C. (2018b). Global significance of seagrass fishery activity. Fish Fish. 19, 399–412. doi: 10.1111/faf.12259
Nowicki, R. J., Thomson, J. A., Burkholder, D. A., Fourqurean, J. W., and Heithaus, M. R. (2017). Predicting seagrass recovery times and their implications following an extreme climate event. Mar. Ecol. Prog. Ser. 567, 79–93. doi: 10.3354/meps12029
Nursey-Bray, M., Palmer, R., Smith, T. F., and Rist, P. (2019). Old ways for new days: Australian Indigenous peoples and climate change. Local Environ. 24, 473–486. doi: 10.1080/13549839.2019.1590325
Oceanica Consulting Pty Ltd. (2011). Seagrass Research and Rehabilitation Plan Synthesis Report July 2003 to June 2010. Report No. 520_001/3. Leederville: Oceanica Consulting Pty Ltd.
Olsen, Y. S., Sánchez-Camacho, M., Murbà, N., and Duarte, C. M. (2012). Mediterranean seagrass growth and demography responses to experimental warming. Estuar. Coast. 35, 1205–1213. doi: 10.1007/s12237-012-9521-z
Ondiviela, B., Losada, I. J., Lara, J. L., Maza, M., Galván, C., Bouma, T. J., et al. (2014). The role of seagrasses in coastal protection in a changing climate. Coast. Eng. 87, 158–168. doi: 10.1016/j.coastaleng.2013.11.005
Orth, R. J., Bieri, J. R., Fishman, J., Harwell, M. R., Marion, S., Moore, K. A., et al. (2006). “A review of techniques using adult plants and seeds to transplant eelgrass (Zostera marina L.) in Chesapeake Bay and the Virginia Coastal Bays,” in Seagrass Restoration: Success, Failure, and the Costs of Both, eds S. F. Treat and R. R. Lewis (Sarasota, FL: Mote Marine Laboratory).
Orth, R. J., Harwell, M. C., Bailey, E. M., Bartholomew, A., Jawad, J. T., Lombana, A. V., et al. (2000). A review of issues in seagrass seed dormancy and germination: implications for conservation and restoration. Mar. Ecol. Prog. Ser. 200, 277–288. doi: 10.3354/meps200277
Orth, R. J., Luckenbach, M., and Moore, K. A. (1994). Seed dispersal in a marine macrophyte: implications for colonization and restoration. Ecology 75, 1927–1939. doi: 10.2307/1941597
Orth, R. J., and McGlathery, K. J. (2012). Eelgrass recovery in the coastal bays of the Virginia Coast Reserve, USA. Mar. Ecol. Prog. Ser. 448, 173–176. doi: 10.3354/meps09596
OzFish Unlimited (2020). Seeds for Snapper. Available online at: https://ozfish.org.au/projects/seeds-for-snapper/ (accessed March 3, 2019).
Paling, E. I., Fonseca, M., van Katwijk, M. M., and van Keulen, M. (2009). “Seagrass restoration,” in Coastal Wetlands: An Integrated Ecosystem Approach, eds G. M. E. Perillo, E. Wolanski, D. R. Cahoon, and M. M. Brinson (Amsterdam: Elsevier), 687–713.
Paling, E. T., van Keulen, M., Wheeler, K. D., Phillips, J., Dyhrberg, R., and Lord, D. A. (2001). Improving mechanical seagrass transplantation. Ecol. Eng. 18, 107–113. doi: 10.1016/s0925-8574(01)00065-9
Park, S. (2016). Extent of Seagrass in the Bay of Plenty in 2011. Environmental Publication 2016/03. Whakatane: Bay of Plenty Regional Council.
Park, S. G. (1999). Changes in Abundance of Seagrass (Zostera spp.) in Tauranga Harbour from 1959–1996. Environmental Report 99/30. Whakatane: Environment Bay of Plenty.
Paul-Burke, K., Burke, J., Te, Ūpokorehe Resource, Management Team, Bluett, C., et al. (2018). Using Maori knowledge to assist understandings and management of shellfish populations in Ohiwa harbor, Aotearoa, New Zealand. N. Z. J. Mar. Freshw. Res. 52, 542–556. doi: 10.1080/00288330.2018.1506487
Paulo, D., Cunha, A. H., Boavida, J., Serrão, E. A., Gonçalves, E. J., and Fonseca, M. (2019). Open coast seagrass restoration. can we do it? Large scale seagrass transplants. Front. Mar. Sci. 6:52. doi: 10.3389/fmars.2019.00052
Phinn, S., Roelfsema, C., Kovacs, E., Canto, R., Lyons, M., Saunders, M., et al. (2018). “Mapping, monitoring and modelling seagrass using remote sensing techniques,” in Seagrasses of Australia: Structure, Ecology and Conservation, eds A. W. D. Larkum, G. A. Kendrick, and P. J. Ralph (Cham: Springer International Publishing), 445–487. doi: 10.1007/978-3-319-71354-0_15
Piazza, B. P., Banks, P. D., and La Peyre, M. K. (2005). The potential for created oyster shell reefs as a sustainable shoreline protection strategy in Louisiana. Restor. Ecol. 13, 499–506. doi: 10.1111/j.1526-100X.2005.00062.x
Pickerell, C. H., Schott, S., and Wyllie-Echeverria, S. (2005). Buoy-deployed seeding: demonstration of a new eelgrass (Zostera marina L.) planting method. Ecol. Eng. 25, 127–136. doi: 10.1016/j.ecoleng.2005.03.005
Plutchak, R., Major, K., Cebrian, J., Foster, C. D., Miller, M. E. C., Anton, A., et al. (2010). Impacts of oyster reef restoration on primary productivity and nutrient dynamics in tidal creeks of the north central Gulf of Mexico. Estuar. Coast. 33, 1355–1364. doi: 10.1007/s12237-010-9327-9
Prober, S. M., Byrne, M., McLean, E. H., Steane, D. A., Potts, B. M., Vaillancourt, R. E., et al. (2015). Climate-adjusted provenancing: a strategy for climate-resilient ecological restoration. Front. Ecol. Evol. 3:65. doi: 10.3389/fevo.2015.00065
Ralph, P. J., Durako, M. J., Enríquez, S., Collier, C. J., and Doblin, M. A. (2007). Impact of light limitation on seagrasses. J. Exp. Mar. Biol. Ecol. 350, 176–193. doi: 10.1016/j.jembe.2007.06.017
Rasheed, M. A. (1999). Recovery of experimentally created gaps within a tropical Zostera capricorni (Aschers.) seagrass meadow, Queensland Australia. J. Exp. Mar. Biol. Ecol. 235, 183–200. doi: 10.1016/S0022-0981(98)00158-0
Rasheed, M. A. (2004). Recovery and succession in a multi-species tropical seagrass meadow following experimental disturbance: the role of sexual and asexual reproduction. J. Exp. Mar. Biol. Ecol. 310, 13–45. doi: 10.1016/j.jembe.2004.03.022
Rasheed, M. A., McKenna, S., Carter, A., and Coles, R. G. (2014). Contrasting recovery of shallow and deep water seagrass communities following climate associated losses in tropical north Queensland, Australia. Mar. Pollut. Bull. 83, 491–499. doi: 10.1016/j.marpolbul.2014.02.013
Rasheed, M. A., and Unsworth, R. K. F. (2011). Long-term climate-associated dynamics of a tropical seagrass meadow: implications for the future. Mar. Ecol. Prog. Ser. 422, 93–103. doi: 10.3354/MEPS08925
Reed, D. H., and Frankham, R. (2003). Correlation between fitness and genetic diversity. Conserv. Biol. 17, 230–237. doi: 10.1046/j.1523-1739.2003.01236.x
Reed, J., Schwarz, A., Gosai, A., and Morrison, M. (2004). Feasibility Study to Investigate the Replenishment/Reinstatement of Seagrass Beds in Whangarei Harbour – Phase 1. Auckland: Northland Regional Council.
Rehfeldt, G. E., and Jaquish, B. C. (2010). Ecological impacts and management strategies for western larch in the face of climate-change. Mitig. Adapt. Strat. Gl. 15, 283–306. doi: 10.1007/s11027-010-9217-2
Reynolds, L. K., McGlathery, K. J., and Waycott, M. (2012). Genetic diversity enhances restoration success by augmenting ecosystem services. PLoS One 7:e38397. doi: 10.1371/journal.pone.0038397
Reynolds, L. K., Waycott, M., and McGlathery, K. J. (2013). Restoration recovers population structure and landscape genetic connectivity in a dispersal-limited ecosystem. J. Ecol. 101, 1288–1297. doi: 10.1111/1365-2745.12116
Ricciardi, A., and Simberloff, D. (2009). Assisted colonization is not a viable conservation strategy. Trends Ecol. Evol. 24, 248–253. doi: 10.1016/j.tree.2008.12.006
Riemann, B., Carstensen, J., Dahl, K., Fossing, H., Hansen, J. W., Jakobsen, H. H., et al. (2016). Recovery of Danish coastal ecosystems after reductions in nutrient loading: a holistic ecosystem approach. Estuar. Coasts 39, 82–97. doi: 10.1007/s12237-015-9980-0
Rodríguez, J. P., Keith, D. A., Rodríguez-Clark, K. M., Murray, N. J., Nicholson, E., et al. (2015). A practical guide to the application of the IUCN red list of ecosystems criteria. Philos. Trans. R. Soc. London, Ser. B 370:20140003.
Ruiz-Halpern, S., Macko, S. A., and Fourqurean, J. W. (2008). The effects of manipulation of sedimentary iron and organic matter on sediment biogeochemistry and seagrasses in a subtropical carbonate environment. Biogeochem 87, 113–126. doi: 10.1007/s10533-007-9162-7
Ruiz-Jaen, M. C., and Aide, T. M. (2005). Restoration success: how is it being measured? Restor. Ecol. 13, 569–577. doi: 10.1111/j.1526-100X.2005.00072.x
Schanz, A., and Asmus, H. (2003). Impact of hydrodynamics on development and morphology of intertidal seagrasses in the Wadden Sea. Mar. Ecol. Prog. Ser. 261, 123–134. doi: 10.3354/meps261123
Schlaepfer, M. A., Helenbrook, W. D., Searing, K. B., and Shoemaker, K. T. (2009). Assisted colonization: evaluating contrasting management actions and values in the face of uncertainty. Trends. Ecol. Evol. 24, 471–472. doi: 10.1016/j.tree.2009.05.008
Schueler, S., Kapeller, S., Konrad, H., Geburek, T., Mengl, M., Bozzano, M., et al. (2013). Adaptive genetic diversity of trees for forest conservation in a future climate: a case study on Norway spruce in Austria. Biodivers. Conserv. 22, 1151–1166. doi: 10.1007/s10531-012-0313-3
Serrano, O., Lovelock, C. E., Atwood, T. B., Macreadie, P. I., Canto, R., Phinn, S., et al. (2019). Australian vegetated coastal ecosystems as global hotspots for climate change mitigation. Nat. Comm. 10:4313. doi: 10.1038/s41467-019-12176-8
Sgrò, C. M., Lowe, A. J., and Hoffmann, A. A. (2011). Building evolutionary resilience for conserving biodiversity under climate change. Evol. Appl. 4, 326–337. doi: 10.1111/j.1752-4571.2010.00157.x
Sharma, S., Goff, J., Moody, R. M., Byron, D., Heck, K. L. Jr., Powers, S. P., et al. (2016). Do restored oyster reefs benefit seagrasses? An experimental study in the Northern Gulf of Mexico. Restor. Ecol. 24, 306–313. doi: 10.1111/rec.12329
Sharpe, A., and Conrad, C. (2006). Community based ecological monitoring in Nova Scotia: challenges and opportunities. Environ. Monit. Assess. 113, 395–409. doi: 10.1007/s10661-005-9091-7
Sherman, C., Smith, T., York, P. H., Jarvis, J. C., Ruiz-Montoya, L., and Kendrick, G. A. (2018). “Reproductive, dispersal and recruitment strategies in Australian seagrasses,” in Seagrasses of Australia: Structure, Ecology and Conservation, eds A. W. D. Larkum, G. A. Kendrick, and P. J. Ralph (Cham: Springer International Publishing), 665–704.
Sherman, C. D. H., York, P., Smith, T., and Macreadie, P. I. (2016). Fine-scale patterns of genetic variation in a widespread clonal seagrass species. Mar. Biol. 163, 1–11. doi: 10.1007/s00227-016-2861-7
Short, F. T., Carruthers, T. J. R., Waycott, M., Kendrick, G. A., Fourqurean, J. W., Callabine, A., et al. (2010). Posidonia sinuosa. IUCN Red List Threat. Spec. 2010:e.T173349A6996688.
Silliman, B. R., Schrack, E., He, Q., Cope, R., Santoni, A., van der Heide, T., et al. (2015). Facilitation shifts paradigms and can amplify coastal restoration efforts. Proc. Natl. Acad. Sci. U.S.A. 112, 14295–14300. doi: 10.1073/pnas.1515297112
Simler, A. B., Williamson, M. A., Schwartz, M. W., and Rizzo, D. M. (2019). Amplifying plant disease risk through assisted migration. Conserv. Lett. 12:e12605. doi: 10.1111/conl.12605
Sinclair, E. A., Anthony, J. M., Greer, D., Ruiz-Montoya, L., Evans, S. M., Krauss, S. L., et al. (2016). Genetic signatures of Bassian glacial refugia and contemporary connectivity in a marine foundation species. J. Biogeog. 43, 2209–2222. doi: 10.1111/jbi.12822
Sinclair, E. A., Ruiz-Montoya, L., Krauss, S. L., Anthony, J. M., Hovey, R. K., Lowe, R. J., et al. (2018). Seeds in motion: genetic assignment and hydrodynamic models demonstrate concordant patterns of seagrass dispersal. Molec. Ecol. 27, 5019–5034. doi: 10.1111/mec.14939
Sinclair, E. A., Verduin, J. J., Krauss, S. L., Hardinge, J., Anthony, J. M., and Kendrick, G. A. (2013). A genetic assessment of a successful seagrass meadow (Posidonia australis) restoration trial. Ecol. Manag. Restor. 14, 68–71. doi: 10.1111/emr.12028
Sinclair, E. A., Edgeloe, J. M., Anthony, J. M., Statton, J., Breed, M. F., and Kendrick, G. A. (in press). Variation in reproductive effort, genetic diversity and mating systems across Posidonia australis seagrass meadows in Western Australia. AoB PLANTS.
Smale, D. A., Wernberg, T., Oliver, E. C. J., Thomsen, M., Harvey, B. P., Straub, S. C., et al. (2019). Marine heatwaves threaten global biodiversity and the provision of ecosystem services. Nat. Clim. Change 9, 306–312. doi: 10.1038/s41558-019-0412-1
Smith, T. M., York, P. H., Broitman, B. R., Thiel, M., Hays, G. C., et al. (2018). Rare long-distance dispersal event leads to the world’s largest marine clone. Glob. Ecol. Biogeogr. 27, 487–496. doi: 10.1111/geb.12713
Smith, T. M., York, P. H., Macreadie, P. I., Keough, M. J., Ross, D. J., and Sherman, C. D. H. (2016). Spatial variation in reproductive effort of a southern Australian seagrass. Mar. Environ. Res. 120, 214–224. doi: 10.1016/j.marenvres.2016.08.010
Sørensen, S. T., Campbell, M. L., Duke, E., and Manley-Harris, M. (2018). A standard, analytical protocol for the quantitation of non-structural carbohydrates in seagrasses that permits inter-laboratory comparison. Aquat. Bot. 151, 71–79. doi: 10.1016/j.aquabot.2018.08.006
Staehr, P. A., and Wernberg, T. (2009). Physiological responses of Ecklonia Radiata (Laminariales) to a latitudinal gradient in ocean temperature. J. Phycol. 45, 91–99. doi: 10.1111/j.1529-8817.2008.00635.x
State Government of Victoria (2016). Overview of Victoria’s Catchment Management Framework. Available online at: https://www.water.vic.gov.au/__data/assets/pdf_file/0023/52439/FINAL-Fact-sheet-1_Overview-of-Victorias-CMF_Apr-2016_1.pdf.pdf (accessed May 28, 2020).
State Government of Victoria (2020). Catchment Management Framework. Melbourne, MLB: State Government of Victoria.
Statton, J., Cambridge, M. L., Dixon, K. W., and Kendrick, G. A. (2013). Aquaculture of Posidonia australis seedlings for seagrass restoration programs: effect of sediment type and organic enrichment on growth. Restor. Ecol. 21, 250–259. doi: 10.1111/j.1526-100X.2012.00873.x
Statton, J., Dixon, K. W., Hovey, R. K., and Kendrick, G. A. (2012). A comparative assessment of approaches and outcomes for seagrass revegetation in Shark Bay and Florida Bay. Mar. Freshw. Res. 63, 984–993. doi: 10.1071/MF12032
Statton, J., Dixon, K. W., Irving, A. D., Jackson, E. L., Kendrick, G. A., Orth, R. J., et al. (2018). “Decline and restoration ecology of Australian Seagrasses,” in Seagrasses of Australia: Structure, Ecology and Conservation, eds A. W. D. Larkum, G. A. Kendrick, and P. J. Ralph (Cham: Springer International Publishing), 665–704. doi: 10.1007/978-3-319-71354-0_20
Tan, Y. M., Saunders, J. E., and Yaakub, S. M. (2018). A proposed decision support tool for prioritising conservation planning of Southeast Asian seagrass meadows: combined approaches based on ecosystem services and vulnerability analyses. Bot. Mar. 61, 305–320. doi: 10.1515/bot-2017-0117
Tanner, C. E., Hunter, S., Reel, J., Parham, T., Naylor, M., Karrh, L., et al. (2010). Evaluating a large-scale eelgrass restoration project in the Chesapeake Bay. Restor. Ecol. 18, 538–548. doi: 10.1111/j.1526-100X.2010.00694.x
Tanner, C. E., and Parham, T. (2010). Growing Zostera marina (eelgrass) from seeds in land-based culture systems for use in restoration projects. Restor. Ecol. 18, 527–537. doi: 10.1111/j.1526-100X.2010.00693.x
Tanner, J. E. (2015). Restoration of the seagrass Amphibolis antarctica-temporal variability and long-term success. Estuar. Coasts 38, 668–678. doi: 10.1007/s12237-014-9823-4
Tanner, J. E., Irving, A. D., Fernandes, M., Fotheringham, D., McArdle, A., and Murray-Jones, S. (2014). Seagrass rehabilitation off metropolitan Adelaide: a case study of loss, action, failure and success. Ecol. Manage. Restor. 15, 168–179. doi: 10.1111/emr.12133
Tanner, J. E., and Theil, M. J. (2019). Adelaide Seagrass Rehabilitation Project: 2017-2019. Final Report Prepared for the Adelaide and Mount Lofty Ranges Natural Resources Management Board. Adelaide: South Australian Research and Development Institute Aquatic Sciences.
Temmerman, S., Meire, P., Bouma, T. J., Herman, P. M. J., Ysebaert, T., and De Vriend, H. J. (2013). Ecosystem-based coastal defence in the face of global change. Nature 504, 79–83. doi: 10.1038/nature12859
Terrados, J., Marín, A., and Celdrán, D. (2013). Use of Posidonia oceanica seedlings from beach-cast fruits for seagrass planting. Bot. Mar. 56, 185–195. doi: 10.1515/bot-2012-0200
The SeaArt Project (2020). SeaArt – Long Term Establishment of SEAgrass Ecosystems Through Biodegradable ARTificial Meadows. Available at; http://www.sea-art.org/en/home.html (accessed March 3, 2019).
Theuerkauf, S. J., and Lipcius, R. N. (2016). Quantitative validation of a habitat suitability index for oyster restoration. Front. Mar. Sci. 3:64. doi: 10.3389/fmars.2016.00064
Thomson, J. A., Burkholder, D. A., Heithaus, M. R., Fourqurean, J. W., Fraser, M. W., Statton, J., et al. (2015). Extreme temperatures, foundation species, and abrupt ecosystem change: an example from an iconic seagrass ecosystem. Glob. Change Biol. 21, 1463–1474. doi: 10.1111/gcb.12694
Touchette, B. W., and Burkholder, J. M. (2000). Review of nitrogen and phosphorus metabolism in seagrasses. J. Exp. Mar. Biol. Ecol. 250, 133–167. doi: 10.1016/S0022-0981(00)00195-7
Trevathan-Tackett, S. M., Sherman, C. D., Huggett, M. J., Campbell, A. H., Laverock, B., Hurtado-McCormick, V., et al. (2019). A horizon scan of priorities for coastal marine microbiome research. Nat. Ecol. Evol. 3, 1509–1520. doi: 10.1038/s41559-019-0999-7
Turner, S. J., and Schwarz, A. M. (2006). Management and Conservation of Seagrass in New Zealand: An Introduction. Science for Conservation Report 254. Auckland: Department of Conservation, 90.
Tuya, F., Haroun, R., and Espino, F. (2014). Economic assessment of ecosystem services: monetary value of seagrass meadows for coastal fisheries. Ocean Coast. Manag. 96, 181–187. doi: 10.1016/j.ocecoaman.2014.04.032
Tuya, F., Vila, F., Bergasa, O., Zarranz, M., Espino, F., and Robaina, R. R. (2017). Artificial seagrass leaves shield transplanted seagrass seedlings and increase their survivorship. Aquat. Bot. 136, 31–34. doi: 10.1016/j.aquabot.2016.09.001
Udy, J. W., and Dennison, W. C. (1997). Growth and physiological responses of three seagrass species to elevated sediment nutrients in Moreton Bay, Australia. J. Exp. Mar. Biol. Ecol. 217, 253–277. doi: 10.1016/S0022-0981(97)00060-9
Valdemarsen, T., Canal-Vergés, P., Kristensen, E., Holmer, M., Kristiansen, M. D., and Flindt, M. R. (2010). Vulnerability of Zostera marina seedlings to physical stress. Mar. Ecol. Prog. Ser. 418, 119–130. doi: 10.3354/meps08828
van der Heide, T., Govers, L. L., de Fouw, J., Olff, H., van der Geest, M., van Katwijk, M. M., et al. (2012). A three-stage symbiosis forms the foundation of seagrass ecosystems. Science 336, 1432–1434. doi: 10.1126/science.1219973
van der Heide, T., van Nes, E. H., Geerling, G. W., Smolders, A. J. P., Bouma, T. J., and van Katwijk, M. M. (2007). Positive feedbacks in seagrass ecosystems: implications for success in conservation and restoration. Ecosystem 10, 1311–1322. doi: 10.1007/s10021-007-9099-7
van Katwijk, M. M., Thorhaug, A., Marbà, N., Orth, R. J., Duarte, C. M., Kendrick, G. A., et al. (2016). Global analysis of seagrass restoration: the importance of large-scale planting. J. Appl. Ecol. 53, 567–578. doi: 10.1111/1365-2664.12562
van Katwijk, M. M., and Wijgergangs, L. J. M. (2004). Effects of locally varying exposure, sediment type and low-tide water cover on Zostera marina recruitment from seed. Aquat. Bot. 80, 1–12. doi: 10.1016/j.aquabot.2004.04.003
Vaudrey, J. M. P., Kremer, J. N., Branco, B. F., and Short, F. T. (2010). Eelgrass recovery after nutrient enrichment reversal. Aquat. Bot. 93, 237–243. doi: 10.1016/j.aquabot.2010.08.005
Victoria Landcare and Gateway (2020). Community Leads Restoration of Seagrass at Corner Inlet. LandCare, VIC: Victoria Landcare Gateway.
Wabnitz, C. C., Andréfouët, S., Torres-Pulliza, D., Müller-Karger, F. E., and Kramer, P. A. (2008). Regional-scale seagrass habitat mapping in the Wider Caribbean region using Landsat sensors: applications to conservation and ecology. Remote Sens. Environ. 112, 3455–3467. doi: 10.1016/j.rse.2008.01.020
Wadgymar, S. M., Cumming, M. N., and Weis, A. E. (2015). The success of assisted colonization and assisted gene flow depends on phenology. Glob. Change Biol. 21, 3786–3799. doi: 10.1111/gcb.12988
Wang, T. L., Wang, G. Y., Innes, J., Nitschke, C., and Kang, H. J. (2016). Climatic niche models and their consensus projections for future climates for four major forest tree species in the Asia-Pacific region. Forest Ecol. Manag. 360, 357–366. doi: 10.1016/j.foreco.2015.08.004
Waycott, M., Duarte, C. M., Carruthers, T. J. B., Orth, R. J., Dennison, W. C., Olyarnik, S., et al. (2009). Accelerating loss of seagrasses across the globe threatens coastal ecosystems. Proc. Natl. Acad. Sci. U.S.A. 106, 12377–12381. doi: 10.1073/pnas.0905620106
Wear, R. J., Tanner, J. E., and Hoare, S. L. (2010). Facilitating recruitment of Amphibolis as a novel approach to seagrass rehabilitation in hydrodynamically active waters. Mar. Freshw. Res. 61, 1123–1133. doi: 10.1071/MF09314
Weatherall, E. J., Jackson, E. L., Hendry, R. A., and Campbell, M. L. (2016). Quantifying the dispersal potential of seagrass vegetative fragments: a comparison of multiple subtropical species. Estuar. Coast. Shelf Sci. 169, 207–215. doi: 10.1016/j.ecss.2015.11.026
Weeks, A. R., Sgro, C. M., Young, A. G., Frankham, R., Mitchell, N. J., Miller, K. A., et al. (2011). Assessing the benefits and risks of translocations in changing environments: a genetic perspective. Evol. Appl. 4, 709–725. doi: 10.1111/j.1752-4571.2011.00192.x
Wendländer, N. S., Lange, T., Connolly, R. M., Kristensen, E., Pearson, R. M., Valdemarsen, T., et al. (2020). Assessing methods for restoring seagrass (Zostera muelleri) in Australia’s subtropical waters. Mar. Freshw. Res. 71, 996–1005. doi: 10.1071/MF19237
West, R., Jacobs, N., and Roberts, D. (1990). Experimental transplanting of seagrasses in Botany Bay. Aust. Mar. Pollut. Bull. 21, 197–203. doi: 10.1016/0025-326X(90)90502-Y
Whitlock, R. (2014). Relationships between adaptive and neutral genetic diversity and ecological structure and functioning: a meta-analysis. J. Ecol. 102, 857–872. doi: 10.1111/1365-2745.12240
Williams, E. K., Watene-Rawiri, E. M., and Tipa, G. T. (2018). “Empowering indigenous community engagement and approaches in lake restoration: an Āotearoa-New Zealand perspective,” in Lake Restoration Handbook. A New Zealand Perspective, eds D. P. Hamilton, K. J. Collier, J. M. Quinn, and C. Howard-Williams (Switzerland: Springer International Publishing), 495–532. doi: 10.1007/978-3-319-93043-5_15
Williams, S. L. (2001). Reduced genetic diversity in eelgrass transplantation affects both population growth and individual fitness. Ecol. Appl. 11, 1472–1488. doi: 10.1890/1051-0761(2001)011[1472:rgdiet]2.0.co;2
Winder, R. S., Nelson, E., and Beardmore, T. (2011). Ecological implications for assisted migration in Canadian forests. For. Chron. 87, 731–744. doi: 10.5558/tfc2011-090
Wood, G., Marzinelli, E. M., Coleman, M. A., Campbell, A. H., Santini, N. S., Kajlich, L., et al. (2019). Restoring subtidal marine macrophytes in the Anthropocene: trajectories and future-proofing. Mar. Freshw. Res. 70, 936–951. doi: 10.1071/MF18226
Yaakub, S. M., McKenzie, L. J., Erftemeijer, P. L. A., Bouma, T., and Todd, P. A. (2014). Courage under fire: seagrass persistence adjacent to a highly urbanised city–state. Mar. Pollut. Bull. 83, 417–424. doi: 10.1016/j.marpolbul.2014.01.012
York, P. H., Smith, T. M., Coles, R. G., McKenna, S. A., Connolly, R. M., Irving, A. D., et al. (2017). Identifying knowledge gaps in seagrass research and management: an Australian perspective. Mar. Environ. Res. 127, 163–172. doi: 10.1016/j.marenvres.2016.06.006
Zardi, G. I., Nicastro, K. R., Serrao, E. A., Jacinto, R., Monteiro, C. A., and Pearson, G. A. (2015). Closer to the rear edge: ecology and genetic diversity down the core-edge gradient of a marine macroalga. Ecosphere 6:23. doi: 10.1890/ES14-00460.1
Keywords: seagrass ecosystems, coastal, climate change, marine plants, restoration
Citation: Tan YM, Dalby O, Kendrick GA, Statton J, Sinclair EA, Fraser MW, Macreadie PI, Gillies CL, Coleman RA, Waycott M, van Dijk K, Vergés A, Ross JD, Campbell ML, Matheson FE, Jackson EL, Irving AD, Govers LL, Connolly RM, McLeod IM, Rasheed MA, Kirkman H, Flindt MR, Lange T, Miller AD and Sherman CDH (2020) Seagrass Restoration Is Possible: Insights and Lessons From Australia and New Zealand. Front. Mar. Sci. 7:617. doi: 10.3389/fmars.2020.00617
Received: 14 February 2020; Accepted: 06 July 2020;
Published: 14 August 2020.
Edited by:
Christine Angelini, University of Florida, United StatesReviewed by:
Ken L. Heck, Dauphin Island Sea Lab, United StatesCopyright © 2020 Tan, Dalby, Kendrick, Statton, Sinclair, Fraser, Macreadie, Gillies, Coleman, Waycott, van Dijk, Vergés, Ross, Campbell, Matheson, Jackson, Irving, Govers, Connolly, McLeod, Rasheed, Kirkman, Flindt, Lange, Miller and Sherman. This is an open-access article distributed under the terms of the Creative Commons Attribution License (CC BY). The use, distribution or reproduction in other forums is permitted, provided the original author(s) and the copyright owner(s) are credited and that the original publication in this journal is cited, in accordance with accepted academic practice. No use, distribution or reproduction is permitted which does not comply with these terms.
*Correspondence: Craig D. H. Sherman, Y3JhaWcuc2hlcm1hbkBkZWFraW4uZWR1LmF1
Disclaimer: All claims expressed in this article are solely those of the authors and do not necessarily represent those of their affiliated organizations, or those of the publisher, the editors and the reviewers. Any product that may be evaluated in this article or claim that may be made by its manufacturer is not guaranteed or endorsed by the publisher.
Research integrity at Frontiers
Learn more about the work of our research integrity team to safeguard the quality of each article we publish.