- 1School of GeoSciences, University of Edinburgh, Edinburgh, United Kingdom
- 2Department of Life Sciences, Imperial College London, Silwood Park Campus, Berkshire, United Kingdom
- 3Department of Oceanography, University of Cape Town, Cape Town, South Africa
- 4Laboratório de Estudos dos Oceanos e Clima, Instituto de Oceanografia, Universidade Federal do Rio Grande - FURG, Rio Grande, Brazil
- 5Departments of Marine and Coastal Sciences and Earth and Planetary Sciences, Rutgers University, New Brunswick, NJ, United States
- 6Institute for Marine and Antarctic Studies, University of Tasmania, Battery Point, TAS, Australia
- 7British Antarctic Survey, Cambridge, United Kingdom
- 8Instituto Antártico Argentino, Buenos Aires, Argentina
- 9Centro Austral de Investigaciones Científicas, Consejo Nacional de Investigaciones Científicas y Técnicas, Ushuaia, Argentina
- 10Universidad Nacional de Tierra del Fuego, Ushuaia, Argentina
The Southern Ocean plays a critical role in regulating global climate as a major sink for atmospheric carbon dioxide (CO2), and in global ocean biogeochemistry by supplying nutrients to the global thermocline, thereby influencing global primary production and carbon export. Biogeochemical processes within the Southern Ocean regulate regional primary production and biological carbon uptake, primarily through iron supply, and support ecosystem functioning over a range of spatial and temporal scales. Here, we assimilate existing knowledge and present new data to examine the biogeochemical cycles of iron, carbon and major nutrients, their key drivers and their responses to, and roles in, contemporary climate and environmental change. Projected increases in iron supply, coupled with increases in light availability to phytoplankton through increased near-surface stratification and longer ice-free periods, are very likely to increase primary production and carbon export around Antarctica. Biological carbon uptake is likely to increase for the Southern Ocean as a whole, whilst there is greater uncertainty around projections of primary production in the Sub-Antarctic and basin-wide changes in phytoplankton species composition, as well as their biogeochemical consequences. Phytoplankton, zooplankton, higher trophic level organisms and microbial communities are strongly influenced by Southern Ocean biogeochemistry, in particular through nutrient supply and ocean acidification. In turn, these organisms exert important controls on biogeochemistry through carbon storage and export, nutrient recycling and redistribution, and benthic-pelagic coupling. The key processes described in this paper are summarised in the Graphical Abstract. Climate-mediated changes in Southern Ocean biogeochemistry over the coming decades are very likely to impact primary production, sea-air CO2 exchange and ecosystem functioning within and beyond this vast and critically important ocean region.
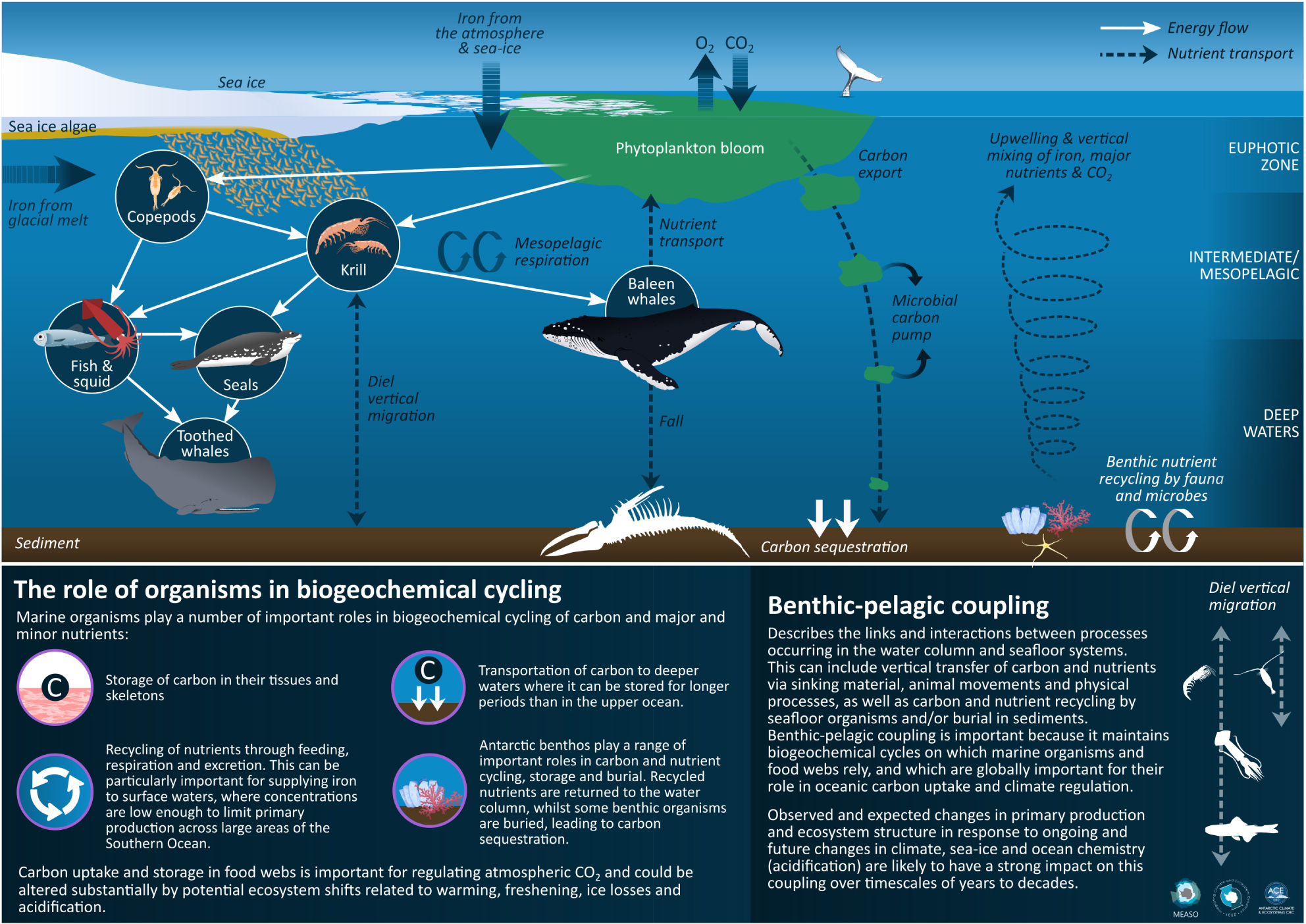
Graphical Abstract. Infographic summarising the key processes described in this paper. Drawn by Dr. Stacey McCormack, University of Tasmania.
Introduction
Biogeochemistry refers to the cycling of chemical elements through living systems and their environments by physical, chemical, biological and geological processes, and is a fundamental component of the functioning of Planet Earth. Biogeochemical cycling of carbon, micronutrients and macronutrients in the Southern Ocean has strong implications for regional ecosystem functioning and sea-air gas exchange. The Southern Ocean plays a major role in modulating Earth’s climate over seasonal-to-millennial timescales by taking up atmospheric carbon dioxide (CO2) via biological and solubility pump processes and by releasing CO2 from the deep ocean (e.g., Sarmiento and LeQuere, 1996; Gruber et al., 2009; Takahashi et al., 2009; Sigman et al., 2010). Ocean biogeochemistry exerts a critical control on primary production and phytoplankton species composition (Pinkerton et al., to be published in this research topic), which in turn have a strong impact on ocean biogeochemistry. Storage, transfer and transformation of carbon and nutrients in benthic (seafloor) and pelagic (water column) food webs regulate the degree to which these constituents are exported, sequestered or recycled and redistributed throughout the Southern Ocean system. Mode and intermediate water masses formed in the Sub-Antarctic influence primary production and carbon export throughout the world’s oceans by setting the biogeochemistry of the global thermocline (subsurface layer characterised by a strong temperature gradient), from which nutrients are supplied to surface waters (Sarmiento et al., 2004; Marinov et al., 2006; Moore et al., 2018).
Anthropogenic climate change is affecting Southern Ocean biogeochemical cycling, directly through oceanic uptake of CO2 and the resultant ocean acidification, and indirectly via its effect on sea ice dynamics, glacial meltwater inputs, winds and ocean physics (e.g., Le Quere et al., 2007; Midorikawa et al., 2012; Henley et al., 2017; Kerr et al., 2018; Gruber et al., 2019a; St-Laurent et al., 2019). Documented and projected climate-driven changes in biogeochemistry and primary production will impact ecosystem functioning and the transfer of carbon, energy and nutrients through benthic and pelagic food webs, with complex feedbacks on ocean biogeochemistry and climate. Biogeochemical and ecosystem responses to ongoing climate and environmental change may differ substantially in time and space, and in particular between shelf regions and the open Southern Ocean as a result of fundamental differences in phytoplankton dynamics, nutrient requirements and supply mechanisms, and carbon export (Arrigo et al., 2008a, b, 2015; Tagliabue et al., 2009a; De Jong et al., 2015).
Within the framework of the Marine Ecosystem Assessment for the Southern Ocean (MEASO), we examine the status of and changes in Southern Ocean biogeochemistry in relation to its biological and physical drivers and its consequences for ecosystem functioning and sea-air CO2 exchange. We consider the entire Southern Ocean south of 30°S, and focus on specific sectors, zones, regions and areas where appropriate. Zones are defined by the major circumpolar fronts, with the Antarctic Zone to the south of the Polar Front, the Polar Frontal Zone between the Polar Front and the Sub-Antarctic Front, the Sub-Antarctic Zone between the Sub-Antarctic Front and the Sub-Tropical Front, and the Northern Zone to the north of the Sub-Tropical Front (Deacon, 1982; Orsi et al., 1995).
We present new data and analyses to address outstanding questions regarding macronutrient cycling and carbon sink dynamics in the context of contemporary climate change. These analyses deepen our regional understanding of Southern Ocean biogeochemistry and reinforce its global-scale importance through modulation of atmospheric CO2 concentrations and control of nutrient transport to the major ocean basins to the north. Finally, we highlight a number of current and future research priorities that will improve our understanding further, as well as a range of developments that are ongoing and anticipated in support of achieving these ambitions (Box 1). The biogeochemical phenomena and changes we describe for the Southern Ocean have strong implications for the marine organisms, food webs and ecosystem processes described throughout this research topic.
Changes in Primary Production and Phytoplankton Species Composition
Phytoplankton Dynamics and Biogeochemistry
Southern Ocean biogeochemical cycles are influenced by phytoplankton through community-level processes (such as net primary production, NPP) and species composition. For example, carbon, nitrogen, phosphorus and iron are required to fuel primary production in euphotic (well-lit) surface waters, and in turn primary producers influence the cycling of these elements. In particular, the vertical export of organic matter produced by phytoplankton to the deep ocean (Section “Changes in the Biological Carbon Pump”) is a key driver of temporal and spatial variability in Southern Ocean biogeochemistry, and this biological carbon pump exerts a strong control on oceanic CO2 uptake and global climate (Section “Changes in the Southern Ocean Carbon Sink”). Different phytoplankton groups play multifaceted roles across a range of biogeochemical cycles (Boyd, 2019), with diatoms being major drivers of the biological carbon pump (Tréguer et al., 2018), as well as remineralisation and ecological stoichiometry (silicon and nitrogen cycles in particular). In addition, the small haptophyte Phaeocystis antarctica is important in the sulphur cycle (Kettle et al., 1999; Goto-Azuma et al., 2019) and coccolithophores are major modifiers of carbonate chemistry (Balch et al., 2011).
Current Status of Primary Production and Phytoplankton Species Composition
The Southern Ocean comprises the largest high nutrient low chlorophyll (HNLC) region globally, with primary production limited by iron, as well as silicon (as silicic acid) in summer north of the Polar Front and light during winter (e.g., de Baar et al., 1995; Boyd et al., 1999; Franck et al., 2000). Phytoplankton biomass and NPP are highest north of the Polar Front in the Atlantic sector, around the Sub-Tropical Front in the west Pacific sector, and over the Antarctic shelves in Prydz Bay and the Ross, Amundsen and Bellingshausen Seas (Pinkerton et al., to be published in this research topic). Biomass and NPP are lowest between the Polar Front and Southern Boundary of the Antarctic Circumpolar Current (ACC), particularly in the Indian sector within and just north of the open ocean sea ice zone (Pinkerton et al., to be published in this research topic). Despite sparse coverage of species composition data, HNLC waters in the Antarctic and Sub-Antarctic Zones where phytoplankton growth is limited by iron (Section “Changes in Micronutrient Biogeochemistry”) tend to have mixed and seasonally changing assemblages of pico-, nano- and micro-phytoplankton (Gall et al., 2001; Eriksen et al., 2018). High-chlorophyll regions, such as island wakes and marginal ice zones (Boyd et al., 2012), tend to be dominated by blooms of diatoms, Phaeocystis or nanoplankton (Arrigo et al., 1999; Moreau et al., 2012; Quéguiner, 2013; Rembauville et al., 2015; Mangoni et al., 2017). One such region in the northern part of the west Antarctic Peninsula (WAP) experienced an intense diatom-dominated bloom with chlorophyll a concentrations >45 mg m–3 under favourable water column conditions (Costa et al., 2020). Diatoms also tend to dominate in silicic acid-rich waters south of the Polar Front (Wright et al., 2010; Petrou et al., 2016; Rembauville et al., 2017), whilst seasonally silicic acid-limited waters north of the Polar Front favour smaller phytoplankton (Freeman et al., 2018; Nissen et al., 2018; Trull et al., 2018). The Great Calcite Belt provides strong evidence for high coccolithophore abundance in the Sub-Antarctic (Balch et al., 2011).
Projected Changes in NPP and Drivers
Projected changes in primary production and phytoplankton species composition have been explored using model simulations (e.g., Bopp et al., 2013; Leung et al., 2015; Moore et al., 2018), manipulation experiments in polar and subpolar waters (e.g., Boyd et al., 2000, 2016; Hernando et al., 2015, 2018; Zhu et al., 2016; Petrou et al., 2019), and insights from paleoproxy records (Goto-Azuma et al., 2019) and contemporary natural variability such as the Southern Annular Mode (SAM) and El Niño Southern Oscillation (ENSO) (e.g., Saba et al., 2014; Schine et al., 2016). Projections of changes in NPP for the entire Southern Ocean based on model simulations within the coupled model intercomparison project 5 (CMIP5) show a good degree of agreement across models (Bopp et al., 2013; Leung et al., 2015; Moore et al., 2018). Simulations point to increases in NPP of 50% or more above present-day rates across much of the Southern Ocean (Leung et al., 2015; Fu et al., 2016), driven by changes in environmental forcings including iron supply, surface mixed layer depth and its effect on underwater light climate, declining sea ice, and poleward shifts and increasing strength of westerly wind belts. Increases in temperature are expected, with medium confidence, to increase phytoplankton growth rates overall (Eppley, 1972; Steinacher et al., 2010; Sherman et al., 2016; Moore et al., 2018; Boyd, 2019), whilst changes in light attenuation by clouds may complicate the response (Meskhidze and Nenes, 2006). Increases in the partial pressure of CO2 (pCO2) are expected to have contrasting effects on different phytoplankton species, with the overall effect on primary production being uncertain (Section “Ocean Acidification and Its Effects on the Ecosystem”).
Increasing NPP in the Southern Ocean is in contrast to the global trend of declining NPP (Moore et al., 2018). The models show that the dominant forcing of Southern Ocean NPP changes across latitudinal bands, corresponding to different circumpolar water masses separated by fronts (Leung et al., 2015). Increased iron supply and shallowing mixed layers play a key role in increasing NPP in the Northern Zone and sub-Antarctic waters north of 50°S, whilst factors controlling light availability are more influential in reducing NPP between 50 and 65°S. Increasing iron supply and a reduction in seasonal sea ice cover drive increases in NPP south of 65°S. Moore et al. (2018) report that the increase in wind-driven upwelling of nutrient-rich waters is the key driver of projected increases in NPP until 2150, after which increased surface stratification driven by ice melt becomes the dominant forcing. A more productive Southern Ocean would lead to greater nutrient uptake and potentially vertical export fluxes, thus retention of nutrients in this region, which would exacerbate declining NPP rates in low latitude waters to the north (Moore et al., 2018).
Projected Changes in Phytoplankton Species Composition and Drivers
Fewer model studies have provided projections of changes in phytoplankton species distributions (Bopp et al., 2005). Nevertheless, assessments of how species composition will be altered by ocean global change are possible by combining modelling, experimental and observational approaches. There is evidence that phytoplankton distributions are shifting southward as surface water temperatures increase, with sub-tropical waters and their warmer-water species incurring into the Sub-Antarctic Zone, and the poleward contraction of winter sea ice coverage extending southward the range of open ocean communities at the expense of sea ice zone communities (McLeod et al., 2012; Constable et al., 2014; Deppeler and Davidson, 2017). These southward shifts may increase the contribution of non-diatom phytoplankton overall, and this could be compounded in the Sub-Antarctic by the proposed favouring of small flagellates by projected increases in stratification (e.g., Petrou et al., 2016). Species shifts observed in response to sea ice changes at the WAP also suggest that phytoplankton communities in the seasonal ice zone may become less diatom-dominated as climate change proceeds (Montes-Hugo et al., 2008, 2009; Mendes et al., 2012, 2018; Hernando et al., 2015; Schofield et al., 2017). In contrast, it is well established from a range of in situ and laboratory studies that increased iron supply and ocean temperature have a beneficial effect on diatoms and other bloom-forming species, such that the projected increases in NPP may also lead to species shifts to diatoms, and in some regions P. antarctica (Gall et al., 2001; Hutchins and Boyd, 2016; Zhu et al., 2016; Boyd, 2019). Within diatom communities, species-specific responses to ocean acidification and other environmental factors are likely to alter species composition, primary productivity and biogeochemical cycles (Section “Ocean Acidification and Its Effects on the Ecosystem”). As such, the biogeographic provinces of phytoplankton assemblages in the Southern Ocean are likely to shift spatially or change fundamentally in the coming decades (Deppeler and Davidson, 2017). On longer timescales, a persistent new set of environmental conditions may drive evolutionary adaptation of existing species (Denman, 2017). Changes in NPP and phytoplankton species composition influence many biogeochemical processes, such as the magnitude and stoichiometry of nutrient uptake and recycling (Arrigo et al., 1999; Weber and Deutsch, 2010; Section “Changes in Macronutrient Biogeochemistry”) and carbon transfer to higher trophic levels (Section “Carbon Transfer and Storage in Pelagic and Benthic Food Webs”). Changes in carbon export to the deep ocean will reflect changes in NPP to a varying extent, with important differences caused by phytoplankton species composition and other factors (e.g., Fu et al., 2016; Rembauville et al., 2017; Section “Changes in the Biological Carbon Pump”).
Changes in Micronutrient Biogeochemistry
Changes to Iron Supply and Availability in the Southern Ocean
The delivery and cycling of important trace elements that underpin all Southern Ocean productivity are being altered by shifts in physics, chemistry and biology in response to ongoing changes in Earth’s climate (high confidence). Iron is required by phytoplankton for photosynthesis and nitrate assimilation, and is the primary limiting micronutrient in the HNLC region across large parts of the Southern Ocean (e.g., de Baar et al., 1990; Moore et al., 2013). Changes in the magnitude and pathways of iron supply to surface waters are being imposed from both the north and the south (Figure 1 and Table 1). For example, the frequency and scale of iron-rich aerosol emissions from dust and wildfires are predicted to increase with trends in regional climate, such as drought and increased winds (IPCC, 2019). Strengthening western boundary currents are very likely to lead to greater eddy transport and thus advective inputs of iron-rich waters from the sub-tropics to the Sub-Antarctic (Bowie et al., 2009), whilst a weakening of the overturning circulation and altered winds may result in changes in stratification patterns impacting delivery of nutrients from below (Tagliabue et al., 2009b; Rintoul, 2018). Warming waters surrounding Antarctica are virtually certain to drive glacial melt and increased calving of icebergs, releasing iron-rich terrigenous material (Lin et al., 2011; Sherrell et al., 2015; Herraiz-Borreguero et al., 2016; Raiswell et al., 2016; Hopwood et al., 2017; Laufkötter et al., 2018; van der Merwe et al., 2019). Increasing iceberg scour in shallow coastal areas in response to ongoing glacial retreat and receding ice shelves is also likely to enhance the important sedimentary iron supply mechanisms to shelf surface waters (Marsay et al., 2014; McGillicuddy et al., 2015), and dust input from Antarctica itself may increase as ice loss exposes more glacial sediments to wind-driven transport (Winton et al., 2016; Duprat et al., 2019; Gao et al., 2020). Warming is very likely to reduce the extent and thickness of Antarctic sea ice (Lannuzel et al., 2016), which accumulates iron and other bioactive metals for subsequent release to surface waters in spring and early summer (Noble et al., 2013). Ocean acidification will alter the speciation (chemical form) and solubility of essential trace elements and nutrients in seawater, but it is unclear whether lowered pH will increase or decrease iron availability to phytoplankton (Shi et al., 2010; Hutchins and Boyd, 2016).
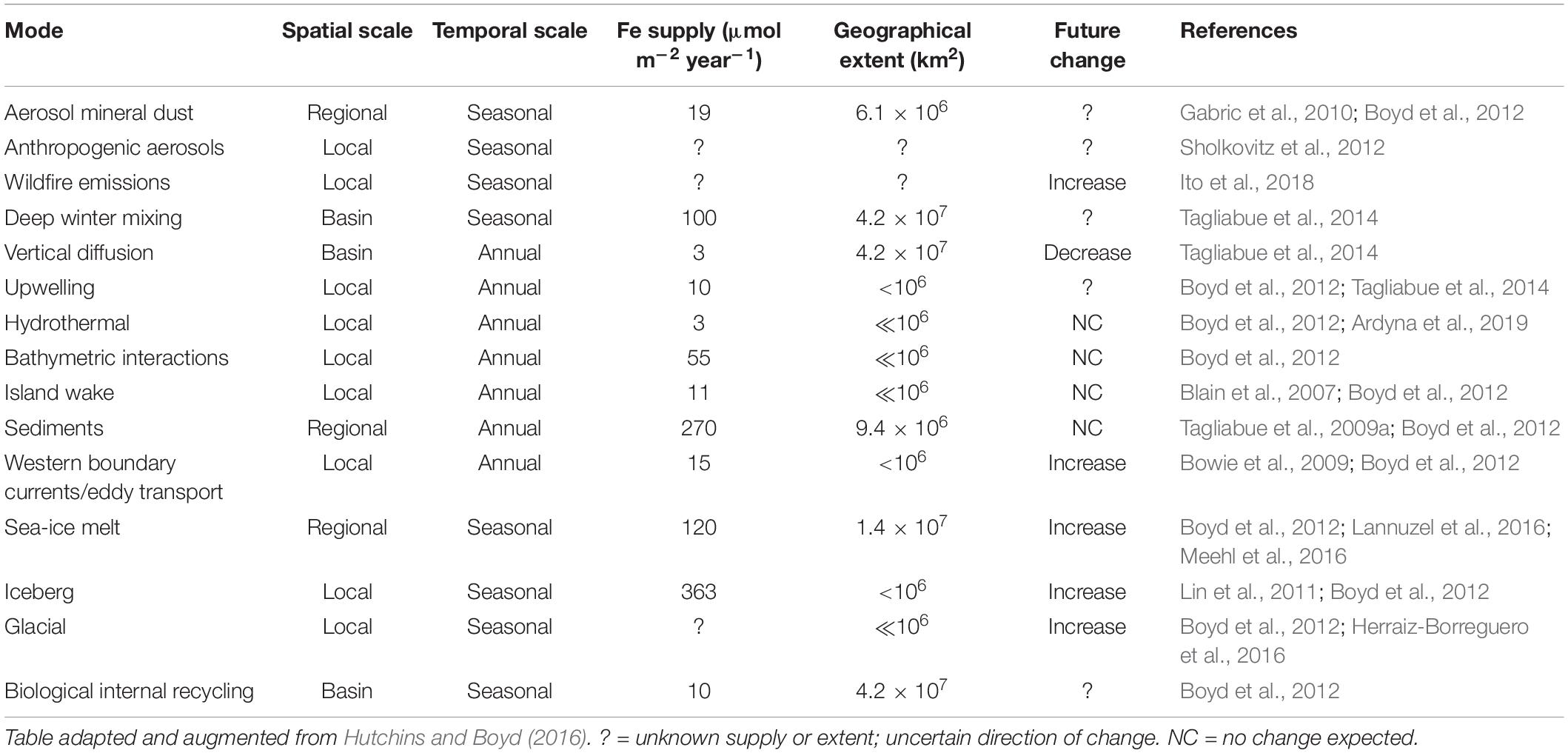
Table 1. Mechanisms of iron supply to the Southern Ocean, with estimates of geographical extent and projected trends under anthropogenic climate change.
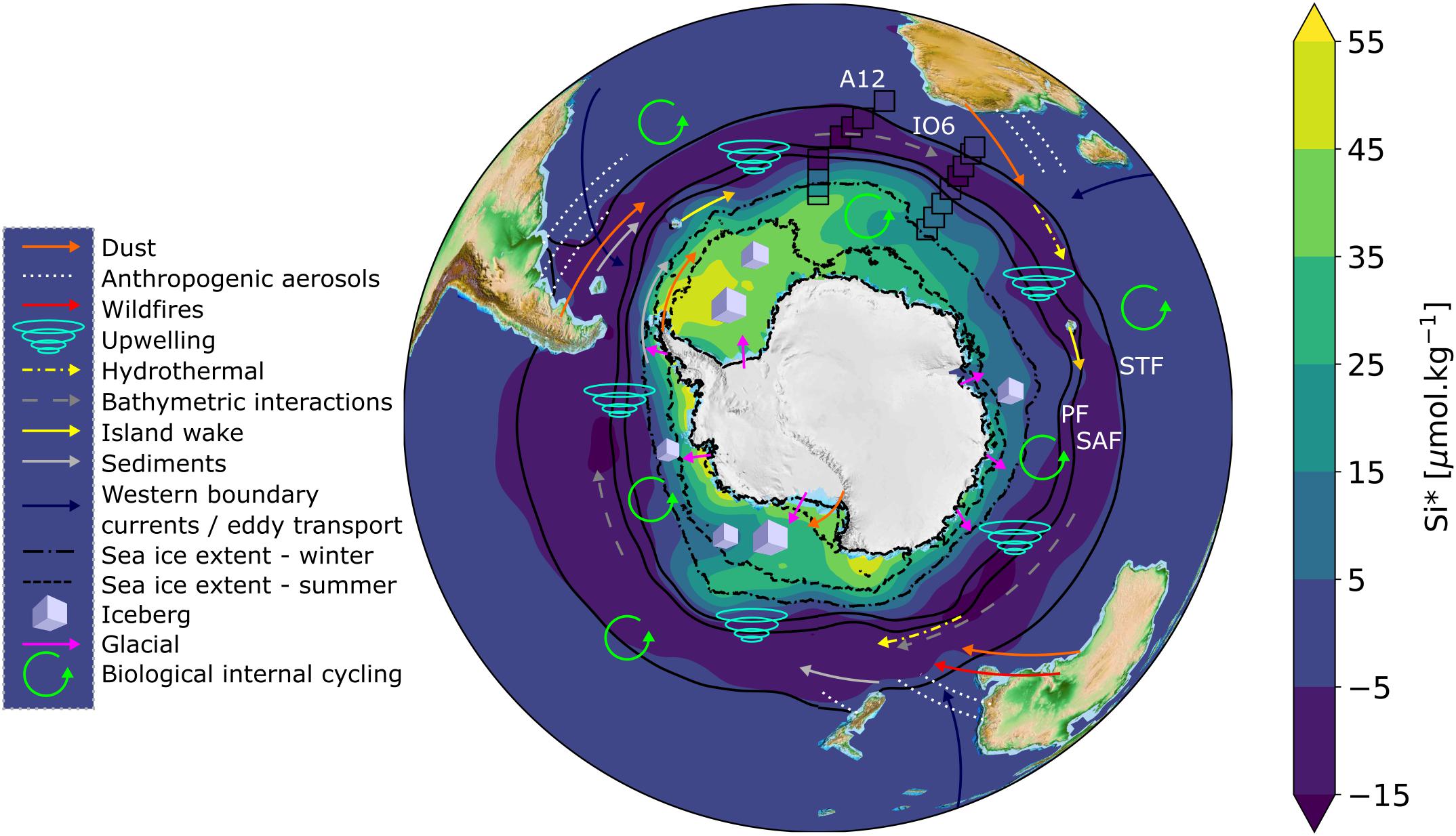
Figure 1. Map of Si∗ with schematic representations of iron sources overlain. Annual climatological Si∗ concentrations (=[Si(OH)4] – [NO3–]) for Southern Ocean surface waters (data source: WOA18, 1 degree grid resolution) are overlain by the measured Si∗ concentrations from A12 2019 and IO6 2017 winter cruises (open black boxes; this study). Solid black contours represent climatological frontal positions based on sea surface temperature; STF = Sub-Tropical Front at 11.5°C, SAF = Sub-Antarctic Front at 4°C, PF = Polar Front at 2°C (Deacon, 1982; Orsi et al., 1995) (data source: WOA18, 1/4 degree resolution). Dashed and dot-dashed black lines represent the mean climatological sea ice extent for summer (Dec/Jan/Feb) and winter (July/Aug/Sep), respectively, defined as 15% sea ice concentration (data source: NSIDC, 12.5 km resolution, Stroeve and Meier, 2018). Iron sources are represented according to the legend, and summarised in Table 1. The exact locations of some iron sources (e.g., hydrothermal vents, bathymetric interactions) are not well-defined, but are depicted schematically. The important processes of deep winter mixing and vertical diffusion occur throughout the Southern Ocean, but are not depicted.
Predicting Ecosystem Responses to Changes in Iron Sources and Their Magnitude
Changes in environmental conditions can have complex and sometimes counterintuitive effects on iron sources and cycling. For instance, whilst increased dust input from Australasia, Patagonia and South Africa would be expected to increase surface iron concentrations and enhance productivity, a large proportion of the dust may remain undissolved and act as additional ballast for sinking particulate organic carbon (POC). This would deepen the mean iron remineralisation depth, and possibly supply new particle surfaces for adsorptive scavenging (i.e., removal) of dissolved iron, reinforcing a possible reduction in the subsurface iron pool (Ellwood et al., 2014; Bressac et al., 2019). The vertical iron gradient and upward iron flux would then be reduced, even in Southern Ocean regions that experience reduced stratification associated with declining sea ice and/or intensification of westerly winds (Rintoul, 2018). With reduced stratification, autotrophic cells are mixed over a greater depth range, thus spending more time under light limitation, which can increase iron demand unless physiological adaptation occurs (Strzepek et al., 2019). In contrast, closer to the Antarctic continent, stratification may be enhanced by increased atmospheric heat flux and freshwater delivery from icebergs, glaciers and ice shelves (e.g., Morley et al., to be published in this research topic). Shifts in phytoplankton species composition could alter cellular iron uptake and remineralisation, whilst changes in the chemical speciation of iron in the upper ocean, in response to altered iron sources, as well as changes in ocean pH, temperature and oxygen content, further complicate an accurate prediction of ecosystem response (Boyd, 2019).
Speciation, Internal Cycling and Remineralisation of Iron in the Southern Ocean
The relative availability of the myriad forms of dissolved (Blain and Tagliabue, 2016) and particulate (Planquette et al., 2013) iron in Southern Ocean surface waters is a key determinant of primary productivity, but remains poorly understood. Major uncertainties include the relative availability of iron bound to organic ligands (Buck et al., 2018; Bundy et al., 2018; Zhang et al., 2019), the interconversion of soluble and colloidal iron (Fitzsimmons et al., 2015; Santschi, 2018), the recycling rate of organic matter-bound iron in surface and subsurface waters (Bressac et al., 2019), and the adsorptive scavenging of regenerated iron in intermediate water masses (Tagliabue et al., 2019). There is a near-consensus that the largest flux of bioavailable iron to surface waters of the open Southern Ocean is currently delivered by wintertime deep vertical mixing from iron-enriched layers below (Tagliabue et al., 2012, 2014; Schallenberg et al., 2018). Except in regions proximal to, or downstream of, lateral sub-surface iron inputs from continental shelves and slopes (De Jong et al., 2012; Hatta et al., 2013; Annett et al., 2017; Sherrell et al., 2018), submerged plateaus (Blain et al., 2007; Bowie et al., 2015) or hydrothermal vents (Ardyna et al., 2019), the increase in iron below the surface mixed layer is ascribed to remineralisation from sinking particulate organic carbon, modulated by iron scavenging with depth (Sedwick et al., 2008; Middag et al., 2011; Abadie et al., 2017).
Effect of Multiple Stressors on Iron Availability and Phytoplankton Physiological Responses
Changes in iron availability in the future Southern Ocean will occur concurrent with, and as a function of, an array of other physical and chemical changes forced by climate change, that will impact the primary producers directly and indirectly by influencing iron dynamics (e.g., Boyd et al., 2014; Section “Changes in Primary Production and Phytoplankton Species Composition”). Although there is reasonable consensus among current models that Southern Ocean primary productivity will increase, largely as a function of increased iron supply, light availability and warming (Bopp et al., 2013; Leung et al., 2015; Moore et al., 2018; Boyd, 2019), no models capture mechanistically the myriad interactions among changing environmental variables and the physiological effects, adaptation strategies and evolutionary responses of the phytoplankton (Hutchins and Boyd, 2016; Strzepek et al., 2019). The magnitude and in most cases even the sense of these interactions remain insufficiently understood. Changes in phytoplankton species composition may also alter iron availability in surface waters by modifying the balance among biological uptake, chemical speciation, adsorptive scavenging, vertical export and organic matter recycling as controlling mechanisms. In addition, many cell types are surprisingly plastic in their iron requirements, and some have the ability, even in the short term, to adjust their iron assimilation mechanisms and maintain similar growth rates despite changes in iron availability (Andrew et al., 2019). Phytoplankton in a cold-core eddy with very low surface iron concentrations have been shown recently to upregulate iron uptake and utilise iron from enhanced microbially mediated recycling (Ellwood et al., 2020). Given the widespread distribution of eddies in the iron-limited Southern Ocean (Frenger et al., 2015), these mechanisms may have large-scale importance in influencing phytoplankton dynamics under climate change.
Micronutrient-Limitation by Other Trace Elements
In addition to iron, phytoplankton require manganese and can be co-limited by manganese and iron in Southern Ocean regions where supplies of both metals to surface waters are restricted (Middag et al., 2011, 2013). Iron-manganese co-limitation of phytoplankton growth has been shown for P. antarctica in the Ross Sea in late summer (Wu et al., 2019) and in the Antarctic diatom Chaetoceros debilis (Pausch et al., 2019). The future status of iron-manganese co-limitation may depend in part on the poorly understood effects of ocean acidification on the availability of these metals (Koch et al., 2019). Cobalt in the form of cobalamin (vitamin B12) can also co-limit, with iron, some Southern Ocean phytoplankton species (Moore et al., 2013; Bertrand et al., 2015). Because cobalamin can only be produced by bacteria and archaea, as is the case for some strong iron ligands, this points to a critical role for complex phytoplankton−bacteria interactions in regulating Southern Ocean primary productivity. Laboratory experiments investigating the interactions among all of these factors and their combined physiological and biogeochemical implications suggest that a complex and seasonally varying mosaic of limitation scenarios may apply in various subregions of the Southern Ocean and Antarctic shelf waters (Koch and Trimborn, 2019).
Differences Between Open Southern Ocean and Antarctic Shelf Regions
The open Southern Ocean and Antarctic shelves differ substantially in micronutrient dynamics due to large differences in circulation, bottom depth, productivity and biogeochemistry, and interactions with the atmosphere and cryosphere. Whilst vertical mixing and atmospheric dust inputs from more northerly continents are the main iron sources for much of the Southern Ocean, surface waters of the shelf regions are proximal to both continental and sedimentary iron sources. In regions bordered by ice shelves, ice-shelf melting by warmer oceanic waters at the ice-ocean interface can provide a glacial iron source to the adjacent surface ocean from iron-rich particulates within the ice shelf and from liquid water at the base of land-grounded ice (Gerringa et al., 2012; Sherrell et al., 2015; Raiswell et al., 2018). The buoyant plume transporting ice-derived iron also entrains iron from shelf sediments via the ice-shelf meltwater pump (St-Laurent et al., 2017, St-Laurent et al., 2019). Most evidence for these phenomena is from West Antarctica, where glacial meltwater input has been increasing for decades, adding iron that is potentially bioavailable for phytoplankton growth (Monien et al., 2017), but new evidence shows increased melting in East Antarctica (Rignot et al., 2019) that may be driving similar iron inputs. These glacial and sedimentary iron sources, in addition to oceanic sources, mean that surface waters are iron-replete in most shelf regions in spring (Marsay et al., 2014; Arrigo et al., 2017) and in coastal inner shelf regions in summer (Carvalho et al., 2019), although summertime iron limitation has been indicated off the WAP (Annett et al., 2017) and in the Amundsen and Ross Seas (Alderkamp et al., 2015, 2019). Whilst there is evidence for off-shelf export of shelf sediment-derived iron from the WAP and western Weddell Sea and transport to downstream open ocean regions (De Jong et al., 2012), much of the continental iron is retained within shelf systems by along-shelf circulation patterns and fronts (Rintoul, 2018), and by uptake in intense phytoplankton blooms that export iron to depth over the shelves (Annett et al., 2017). Contrasting physical and biogeochemical conditions between the shelf and open ocean regions dictate that future changes in iron dynamics and impacts on primary production are also likely to differ between these provinces. Given the expected increases in shelf-proximal glacial meltwater inputs and sedimentary iron delivery by the ice-shelf meltwater pump, and the effects on shelf sediment redox processes of enhanced carbon export from sea-ice-free surface waters, it is reasonable to speculate that larger increases in iron supply to primary producers will occur over the shelves than in the open Southern Ocean as climate change proceeds (Tagliabue et al., 2009a; Marsay et al., 2014; De Jong et al., 2015; St-Laurent et al., 2019; Dinniman et al., 2020).
Changes in Macronutrient Biogeochemistry
Macronutrient Supply and Limitation of Phytoplankton Growth
Whilst iron is the primary limiting nutrient for phytoplankton growth in the Southern Ocean, macronutrients (nitrate, phosphate, silicic acid) sourced mainly from the Circumpolar Deep Water (CDW) mass in the ACC play an important role in regulating primary production and carbon export within and far beyond the Southern Ocean (Moore et al., 2013, 2018). Here, we present three new datasets to explore the seasonal changes in macronutrient concentrations and ratios in the Atlantic and Indian sectors of the open Southern Ocean south of South Africa (Figure 2) and in Marguerite Bay in the coastal zone of the central WAP region (Figure 3). Nitrogen and phosphorus do not limit Southern Ocean primary production in general, although short periods of nitrate limitation have been observed during peak-bloom conditions in high-productivity coastal and shelf areas (Henley et al., 2017, 2018; Figures 2, 3). Additionally, diatoms under varying degrees of iron limitation deplete silicic acid in the Antarctic and Polar Frontal Zones to a far greater extent than nitrate (Pondaven et al., 2000; Smith et al., 2000), leading to summertime instances of silicic acid-limitation (Pollard et al., 2002). The preferential removal of silicic acid south of the Sub-Antarctic Front also yields northward-flowing surface waters that are silicic acid-deplete and nitrate- and phosphate-rich (Figure 2; Sarmiento et al., 2004). This condition, which favours the growth of non-siliceous phytoplankton (Balch et al., 2011; Quéguiner, 2013; Deppeler and Davidson, 2017 and references therein), could reduce carbon export since the dense siliceous shells of diatoms cause them to sink more rapidly than other phytoplankton (Buesseler, 1998; Ducklow et al., 2001; Armstrong et al., 2009).
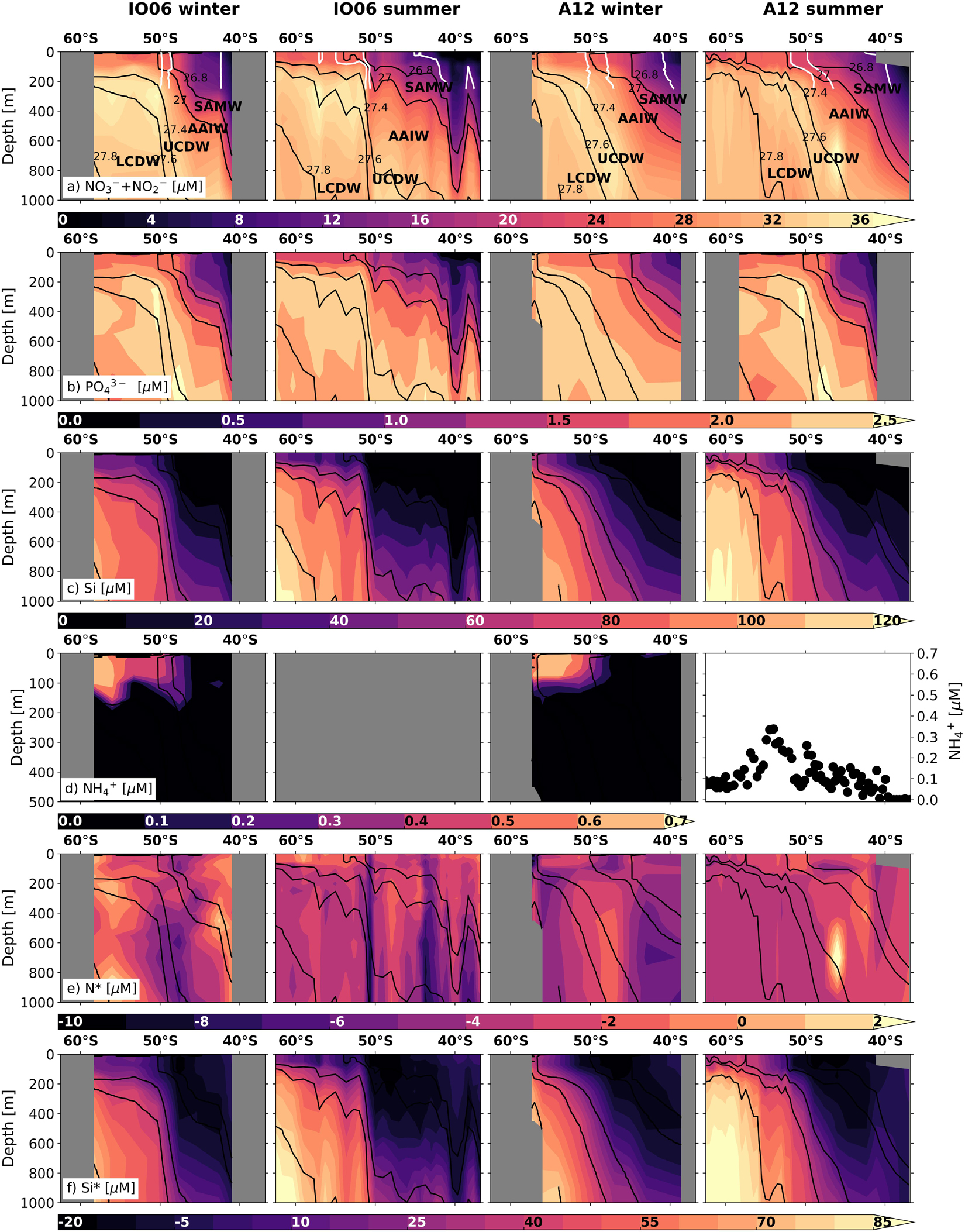
Figure 2. Nutrient concentration and stoichiometry data across the Indian (WOCE IO6 line; left-hand columns) and Atlantic (WOCE A12 line; right-hand columns) sectors of the Southern Ocean in summer and winter (see Figure 1 and Supplementary Figure S1 for transect locations). Shown are the concentrations (0–1000 m, except for ammonium) of (a) nitrate + nitrite, (b) phosphate, (c) silicic acid, (d) ammonium (0–500 m), (e) N* (=[NO3– + NO2–] – 16 × [PO43–]), and (f) Si* (=[Si(OH)4] – [NO3–]). Winter measurements, which extend to the edge of the marginal ice zone, are for samples collected in 2017 (IO6) and 2019 (A12) (see Supplementary Information 1 for details). Summer samples were collected in 1996 (IO6; WOCE 2018) and 2008 (A12; Schlitzer et al., 2018). No summer ammonium concentration data are available for IO6, whilst the summertime values shown for A12 are for samples collected at the surface along A12 in 2019. Where ammonium data are available, N* is calculated as N*DIN = [NO3– + NO2– + NH4+] – 16 × [PO43–]. The major water masses defined according to Whitworth and Nowlin (1987), Park et al. (1993), Orsi et al. (1995), and Belkin and Gordon (1996) are labelled on panel a: SAMW, AAIW, Upper and Lower CDW (UCDW, LCDW). The positions of the major fronts at the time of sampling (after Deacon, 1982 and Orsi et al., 1995) are shown in white to 400 m on panel (a): (from south to north) the Polar Front, Sub-Antarctic Front and Sub-Tropical Front. The new winter data presented here are available at https://doi.org/10.5281/zenodo.3883618.
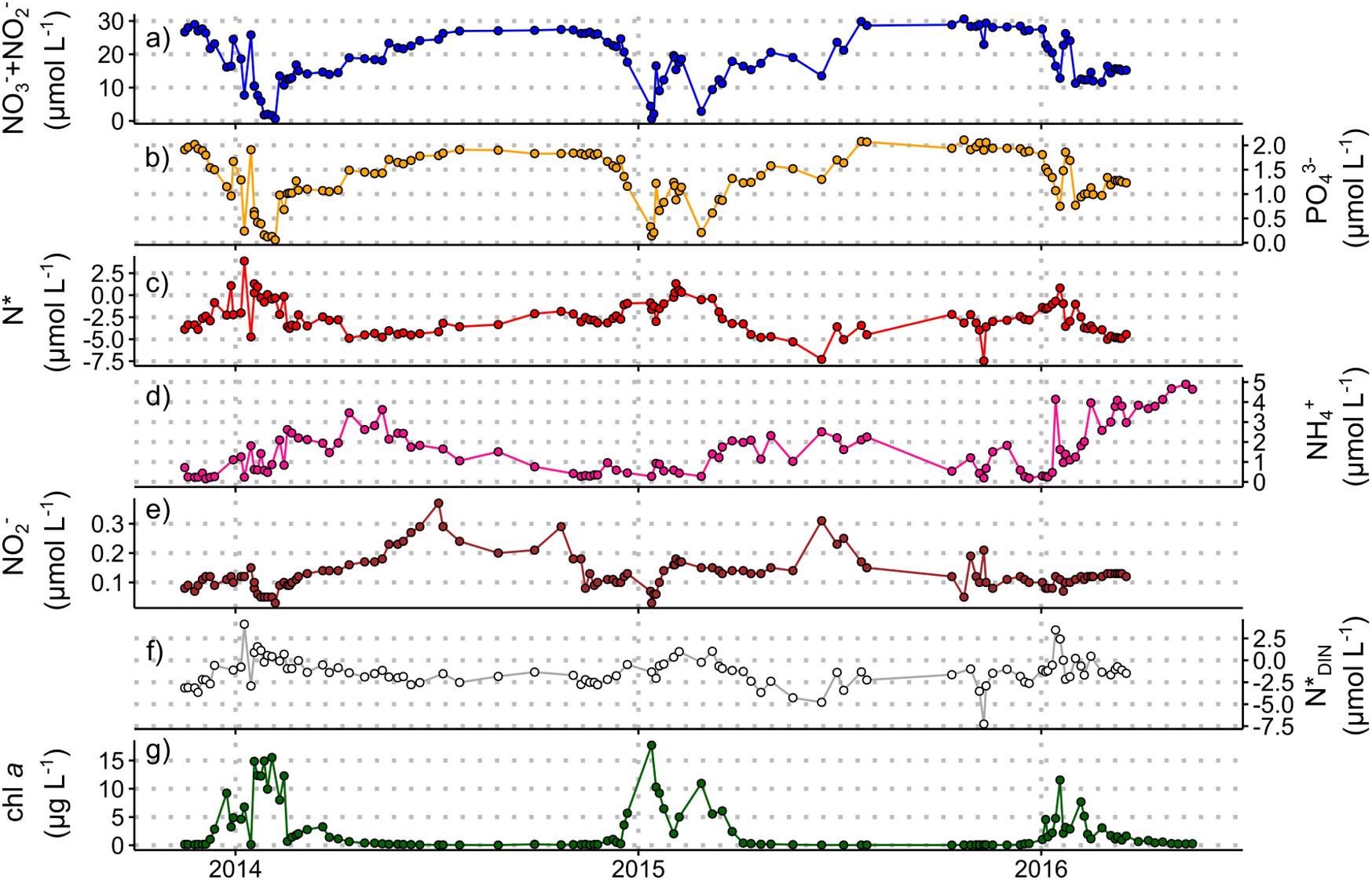
Figure 3. Nutrient concentration and stoichiometry data from the Rothera Time Series, November 2013 to March 2016 (see Supplementary Information 2 for details). Measured concentrations for samples taken at 15 m water depth of (a) nitrate + nitrite, (b) phosphate, (c) N*, (d) ammonium, (e) nitrite, and (f) N*DIN. Chlorophyll a concentrations (g) are included to indicate the timing and magnitude of summer phytoplankton blooms. Chlorophyll data courtesy of the British Antarctic Survey. These data are available through the British Oceanographic Data Centre (BODC) at https://www.bodc.ac.uk/data/published_data_library/catalogue/10.5285/98cc0722-e337-029c-e053-6c86abc02029/ (Henley and Venables, 2019).
Seasonal Nutrient Dynamics in the Open Southern Ocean
The balance between wintertime nutrient supply and summertime nutrient drawdown is central to the Southern Ocean’s role in setting atmospheric CO2 (Sarmiento and Toggweiler, 1984; Section “Changes in the Southern Ocean Carbon Sink”). In general, Southern Ocean nutrient measurements are biased towards the spring and summer due in part to the challenges of sampling in winter. For the open Southern Ocean, two new winter datasets from the IO6 and A12 meridional transects (Figures 2a–c and Supplementary Information 1) show that surface nutrient concentrations as far south as the marginal ice zone, whilst higher in winter than summer, are still lower than in the underlying source waters despite vigorous mixing. This occurs because the seasonal incorporation of the relatively low-nutrient summer surface mixed layer into the winter mixed layer dilutes the nutrient concentrations of the latter (Smart et al., 2015). Biological uptake in summer thus affects mixed-layer nutrient concentrations year-round. In addition, nitrification (the chemoautotrophic oxidation of ammonium to nitrate via nitrite) occurs at high rates in the winter mixed layer across the Southern Ocean (Smart et al., 2015; Mdutyana et al., 2020), producing recycled nitrate subsequently available for consumption in spring. Nitrification is favoured in winter due to low light and reduced competition for ammonium (Olson, 1981; Ward, 2005; Smith et al., 2014). This effect may be augmented by enhanced iron availability (Shafiee et al., 2019) and, south of the Polar Front, by mixed-layer ammonium accumulation (Figure 2d) resulting from an enhanced microbial loop in late summer/autumn (Becquevort et al., 2000; Dennett et al., 2001; Lourey et al., 2003). Currently, the implications of nutrient (re)cycling within the seasonally varying mixed layer for Southern Ocean carbon production and export remain poorly understood.
Seasonal and Spatial Variability in Nutrient N:P Ratios
The ratios in which phytoplankton communities take up macronutrients exert important controls on carbon export, and are influenced by taxonomy, growth rate, resource allocation, equilibrium cellular nutrient ratios relative to supply ratios, and micronutrient limitation (Weber and Deutsch, 2010; Martiny et al., 2013). The nitrogen-to-phosphorus (N:P) uptake ratio varies broadly with latitude across the Southern Ocean, ranging from ∼20:1 in the Sub-Antarctic to 11−16:1 in the Antarctic Zone, corresponding with latitudinal gradients in phytoplankton species composition, light and temperature (Arrigo et al., 1999; Finkel et al., 2006; Green and Sambrotto, 2006; Weber and Deutsch, 2010; Martiny et al., 2013). This produces the opposite trend in seawater nitrate-to-phosphate concentration ratios, as described by the stoichiometric parameter N∗ ([NO3– + NO2–]–16 × [PO43–]; Gruber and Sarmiento, 1997). For the two new winter datasets (Figure 2 and Supplementary Information 1), the previously observed summertime trend persists, with lower mixed-layer N∗ in the Sub-Antarctic and higher N∗ in the Antarctic Zone (Figure 2e). The meridional gradients are weaker in winter (average N∗ of −3.7 ± 0.7 μmol L–1 in the Sub-Antarctic and −2.3 ± 0.7 μmol L–1 in the Antarctic Zone), probably because they largely reflect the imprint of summertime uptake partially eroded by lateral and vertical mixing. Similarly, the wintertime surface-subsurface N∗ gradients are weaker because the rate of upward nutrient supply outpaces that of surface uptake and subsurface regeneration. Nonetheless, the data confirm that summertime phytoplankton N:P uptake ratios set surface and subsurface N∗ year-round in this open ocean region.
New year-round data from Marguerite Bay, in the coastal WAP, show distinct seasonal variations in upper ocean N∗ (Figure 3; Supplementary Information 2). Maximum N∗ in summer is driven by diatom-dominated phytoplankton blooms characterised by low N:P uptake ratios. Diatom-dominated ice algal production may also contribute to increasing N∗ in spring before sea ice retreats. Minimum N∗ in early winter (April−June), similar to deep-water values (−4.8 ± 0.6 μmol L–1 below 100 m), indicates a near-complete reset of surface nutrient stoichiometry due to vertical mixing of nutrient-rich modified CDW. This resupply, augmented by a minor contribution from remineralisation, drives a steady increase in surface nitrate and phosphate in a ratio close to the Redfield ratio (15.4 ± 0.9 in winter 2014; 16.2 ± 0.6 in winter 2015), although surface concentrations do not reach high CDW values. In mid-late winter, increases in N∗ coincident with decreasing ammonium concentrations, high and variable nitrite concentrations, and stable N∗DIN ([NO3– + NO2–] + [NH4+]–16 × [PO43–]; Gruber and Sarmiento, 1997) indicate in situ nitrification. Nitrification within the sea-ice matrix and ice-ocean nutrient exchange may also contribute to the observed signals. By contrast, phytoplankton nutrient uptake cannot account for the changes over winter when light is absent at these latitudes.
Taken together, the three new datasets presented here show that vertical mixing does not completely reset the surface macronutrient inventory over the Antarctic shelves or in the open Southern Ocean, at least in the regions examined here, such that summertime uptake influences surface and subsurface nutrient concentrations and stoichiometry year-round. We also highlight the importance of wintertime nitrification in shelf and open ocean settings.
Southern Ocean Nutrient Budgets and Silicic Acid-to-Nitrate Ratios in Global Biogeochemical Cycles
Southern Ocean macronutrient budgets are important for global nutrient distributions and carbon export in addition to regional processes and ecosystems (Sarmiento et al., 2004; Moore et al., 2018). In particular, surface nutrient concentrations and ratios in the formation regions of Sub-Antarctic Mode Water (SAMW) and Antarctic Intermediate Water (AAIW) are transported northward by these water masses and mixed through the thermocline into macronutrient-limited surface waters north of 30°S (Marinov et al., 2006). This is the case for low preformed silicic acid-to-nitrate ratios, quantified as Si∗ ([Si(OH)4]–[NO3–]; Sarmiento et al., 2004). Although the Si∗ of upwelling CDW is high (∼10−55 μmol L–1; Figure 2f), diatoms experiencing iron stress consume silicic acid and nitrate in a ratio higher than the 1:1 ratio expected for iron-replete diatoms (Hutchins and Bruland, 1998; Takeda, 1998). This decreases Si∗ south of the Polar Front and in northward-flowing surface waters, such that SAMW and AAIW form in the near-absence of silicic acid (i.e., Si∗ < −10 μmol L–1) (Figures 1, 2). The low Si∗ signal is transferred to the low-latitude thermocline, favouring non-silicifying phytoplankton species in the overlying surface waters.
Future Changes to Southern Ocean Nutrient Budgets and Global Consequences
Observed and anticipated changes in primary production and phytoplankton species composition across the Southern Ocean (Section “Changes in Primary Production and Phytoplankton Species Composition”) influence the quantity and stoichiometry of nutrients transported in northward-flowing water masses. Increases in primary production would reduce total northward nutrient transport, and therefore primary production and carbon export throughout the lower latitudes (Moore et al., 2018). Southward shifts of phytoplankton communities as warming proceeds, and the resultant shift towards smaller species with higher N:P uptake ratios in each latitudinal band (Arrigo et al., 1999; Martiny et al., 2013), would increase the mean N:P uptake ratio at the basin scale (Weber and Deutsch, 2010), altering carbon export, which is more tightly coupled to nitrogen than phosphorus. The subsequent decrease of N∗ in mode and intermediate waters would lead to more intense nitrogen limitation relative to phosphorus in the low-latitudes, reducing carbon export and potentially influencing competition between nitrogen fixers and other autotrophs (Weber and Deutsch, 2010; Martiny et al., 2013). In contrast, a species shift to diatoms in the Sub-Antarctic could increase N∗ supply to the low latitudes, which could partially offset the reduction in low-latitude carbon export. Whilst such a shift could further reduce Si∗ in northward-transported water masses, the well-established silicic acid leakage hypothesis (Nozaki and Yamamoto, 2001; Brzezinski et al., 2002; Matsumoto et al., 2002) suggests that the projected increase in iron supply (Sections “Changes in Primary Production and Phytoplankton Species Composition” and “Changes in Micronutrient Biogeochemistry”) would reduce the diatom silicon-to-nitrogen (Si:N) uptake ratio and thus increase Si∗ supply to the north. This increase in Si∗ could also be exacerbated by a reduction in diatom silica production under ocean acidification, which would further reduce the Si:N uptake ratio (Petrou et al., 2019; Section “Ocean Acidification and Its Effects on the Ecosystem”). The extent to which changes in the stoichiometry of northward-transported nutrients compound or alleviate the impact of increased Southern Ocean nutrient retention on global-scale primary production and carbon export (Moore et al., 2018) requires further investigation.
Changes in the Southern Ocean Carbon Sink
The Solubility Pump, Biological Pump and Upwelling
The Southern Ocean is a globally important region for ocean ventilation and the sea-air exchange of CO2 and other climate-active gases, due to a complex interaction (sometimes counterintuitive) between physical-chemical and biological processes (Marinov et al., 2006). The solubility pump, whereby atmospheric CO2 is taken up by dissolution into surface waters and subsequently subducted into the subsurface, exporting CO2 into the ocean interior, is particularly strong in the high southern latitudes due to cold surface waters and deep and intermediate water mass formation (e.g., Sabine et al., 2004; Van Heuven et al., 2014; Gruber et al., 2019b). The Southern Ocean also has regions of strong upwelling linked to oceanographic fronts, which brings CO2-rich deep waters to the surface, increasing pCO2 in surface waters, altering the carbonate system equilibrium and driving CO2 release to the atmosphere (e.g., Pardo et al., 2017; Chapman et al., 2020). The biological pump is also important in the Southern Ocean, particularly during spring and summer (e.g., Ducklow et al., 2001; DeVries et al., 2012; Cavan et al., 2019a). CO2 is converted into organic carbon during photosynthesis by phytoplankton and other primary producers (Section “Changes in Primary Production and Phytoplankton Species Composition”), stored in plant and animal tissues (Section “Carbon Transfer and Storage in Pelagic and Benthic Food Webs”) and subsequently exported to the deep ocean and seafloor when microalgae and other organisms die. The balance between solubility and biological pump processes and upwelling processes, and their combined effect on the difference between seawater and atmospheric pCO2 (ΔpCO2), determine whether the surface ocean behaves as a CO2 sink or source. The magnitude of CO2 fluxes depends mostly on wind speed, which strongly affects the sea–air gas transfer velocity (e.g., Fay et al., 2014; Wanninkhof, 2014).
Net CO2 Sink Behaviour of the Southern Ocean
The Southern Ocean between 30°S and 50°S is currently a major net annual sink for atmospheric CO2 since biological uptake during summer and solubility pump processes exceed CO2 outgassing driven by upwelling and vertical mixing predominantly during winter (e.g., Takahashi et al., 2012; Roobaert et al., 2019). The Southern Ocean CO2 sink has taken up approximately 40% of the total oceanic uptake of anthropogenic CO2 (Orr et al., 2001; Fletcher et al., 2006; DeVries, 2014), increasing surface water pCO2 and causing ocean acidification (Section “Ocean Acidification and Its Effects on the Ecosystem”). Export of CO2 to the deep Southern Ocean occurs in specific locations and depends on the interactions between physical properties, such as mixed layer depth, ocean currents, fronts, eddies and winds, all of which are potentially sensitive to climate variability and change, and with bathymetric features (Sallee et al., 2012; Chapman et al., 2020). In addition to intense seasonality in sea–air CO2 fluxes driven by the solubility and biological pumps, a high degree of interannual variability has been reported for particular regions, such as the WAP (Ito et al., 2018; Brown et al., 2019) and the Ross Sea (Dejong and Dunbar, 2017). Across the Southern Ocean, summertime conditions have been shown to drive sub-decadal variability in ΔpCO2 whilst winter variability contributes to longer-term changes associated with the SAM (Lenton and Matear, 2007; Gregor et al., 2018).
Trends and Changes in Carbon Sink Strength
The strength of the Southern Ocean carbon sink declined between the 1980s and early 2000s, largely due to an increase in natural CO2 release associated with stronger winds, which enhanced the advection and vertical mixing of dissolved inorganic carbon (DIC) into surface waters (Le Quere et al., 2007; Lovenduski et al., 2008; Lenton et al., 2013). Model simulations have shown that this increase in CO2 outgassing was sustained from the late 1950s (Lovenduski et al., 2013). However, this trend reversed during the 2000s as the overturning circulation weakened (DeVries et al., 2017). The strength of the sink has increased again since around 2002, and by 2012 had recovered to its expected strength based on the increase in atmospheric CO2 concentrations (Landschutzer et al., 2015). The Southern Ocean sea–air CO2 flux was recently estimated to be −0.75 ± 0.22 Pg C year–1 by combining estimates of seawater pCO2 from biogeochemical floats with shipboard measurements (Bushinsky et al., 2019). This estimate of CO2 sink strength is reduced compared to that based on shipboard measurements alone (–1.14 ± 0.19 Pg C year–1) because the use of floats increases substantially the data availability during winter when CO2 outgassing is maximal.
Spatial and Temporal Variability in ΔpCO2 and Its Physical and Biological Drivers
Here we use the Surface Ocean CO2 Atlas (SOCAT) version 2019 (Bakker et al., 2016; Figure 4) to elucidate how carbon sink strength in the different sectors of the Southern Ocean south of 30°S has changed in recent decades (Figure 5). We also attempt to compare the proportional contributions of the solubility and biological pumps to the carbon sink (Figure 6). Analysis of ΔpCO2 by season for 2002−2007 and 2012−2017 confirms the expected net CO2 sink behaviour for both coastal and open-ocean regions where data are available (Figure 5). Net CO2 uptake (negative ΔpCO2) is observed overall in all seasons, with lower spatially-averaged ΔpCO2 indicating higher CO2 uptake during the summer seasons and particularly in the coastal regions (Figures 5a,e). However, variability is high within and between sectors across seasons and some sectors show net CO2 release (positive ΔpCO2) during winter (Table 2). For example, net CO2 release is identified along the WAP during the austral winters of both time periods, and for distinct regions of the Western Pacific sector in all seasons for 2012−2017 (Figure 5). In addition, the ΔpCO2 distribution appears to show a zone with values close to zero at approximately 50−60°S. Although this zone has been described as circumpolar (Takahashi et al., 2012), it is not clear in the Weddell Sea sector, especially considering the increased data coverage for 2012−2017. Our analysis reveals no clear trend in ΔpCO2 overall between 2002−2007 and 2012−2017, consistent with equivalent increases in atmospheric and surface water pCO2, and thus an increase in CO2 sink strength, since the early 2000s (Landschutzer et al., 2015), as well as substantial variation in ΔpCO2 changes between sectors and seasons (Table 2).
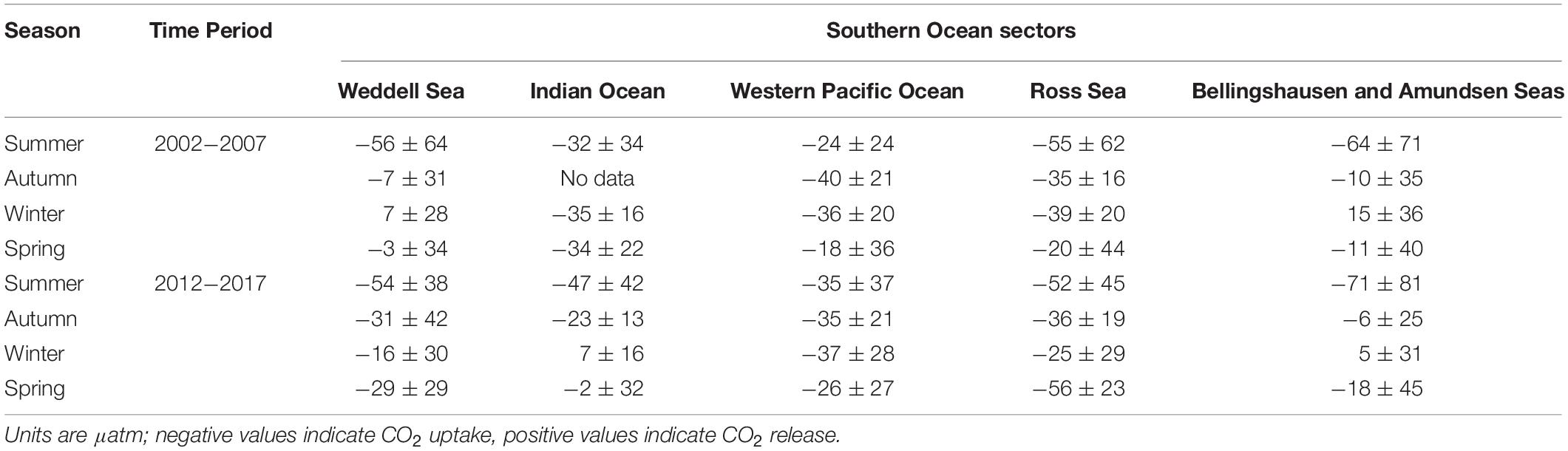
Table 2. Regional average ± standard deviation of the pCO2 difference between the surface ocean and atmosphere (ΔpCO2), by season for two composited time periods: 2002−2007 and 2012−2017.
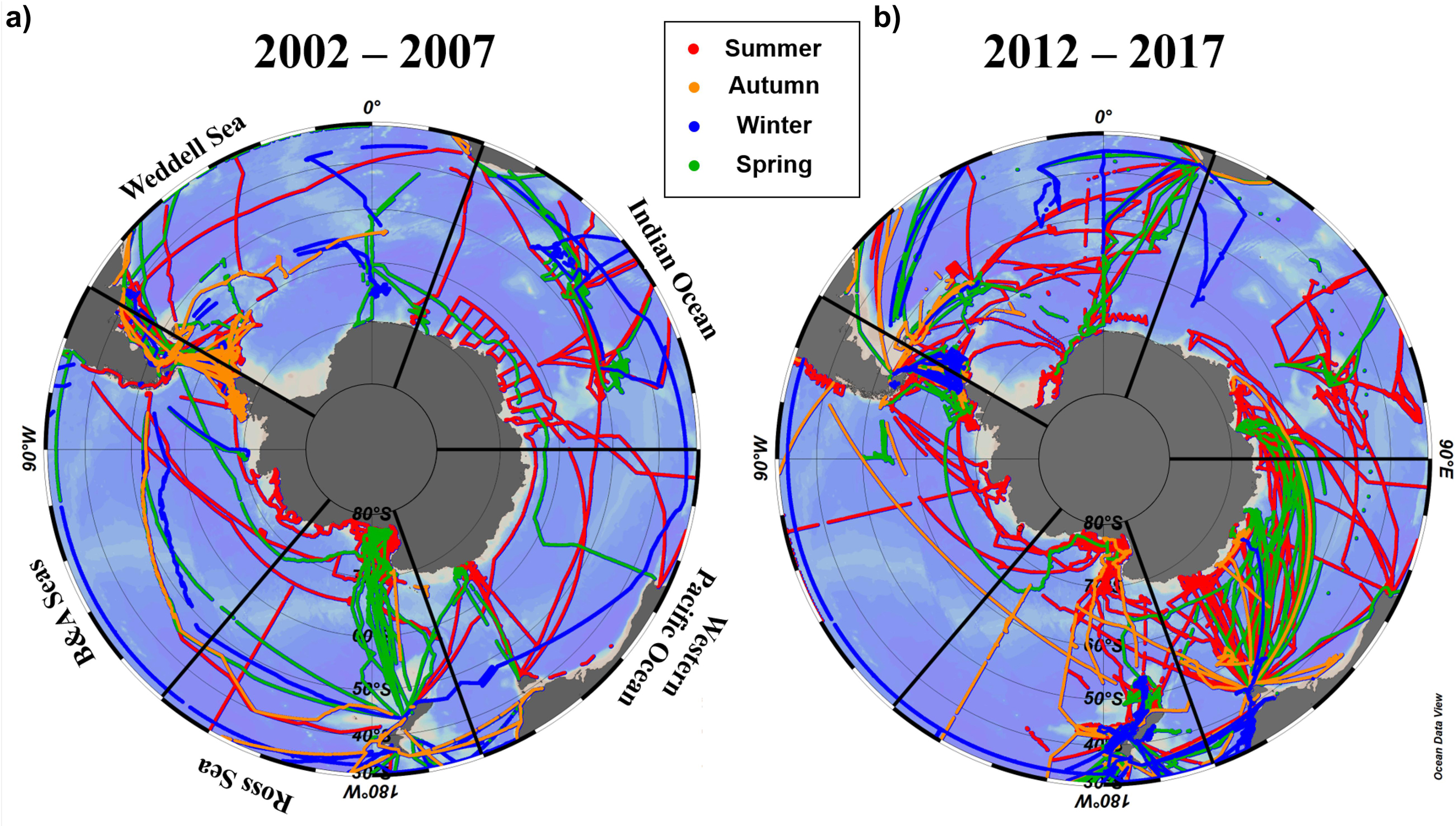
Figure 4. Distribution of seasonal pCO2 observational data used here from SOCAT version 2019 (Bakker et al., 2016) for (a) 2002–2007 and (b) 2012–2017. The seasonal periods computed were austral summer (JFM; red trajectories), autumn (AMJ; orange trajectories), winter (JAS; blue trajectories) and spring (OND; green trajectories).
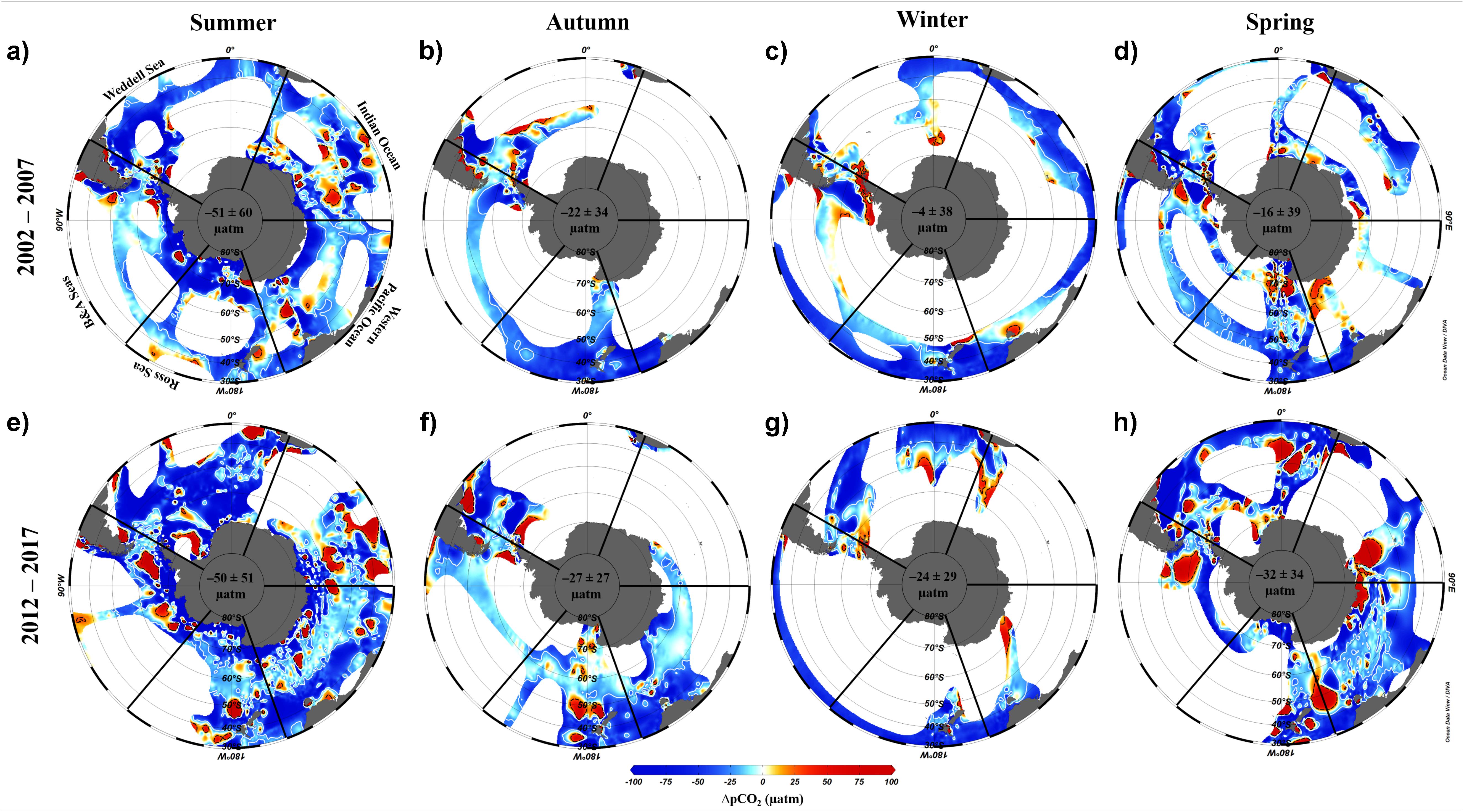
Figure 5. Difference between the pCO2 of the ocean and atmosphere (ΔpCO2, μatm) by season for two composited periods: (a–d) 2002–2007 and (e–h) 2012–2017. The maps were produced using Data-Interpolating Variational Analysis (DIVA; 10 × 10 per mille) in the Ocean Data View software (Schlitzer, 2018). Seasonal ΔpCO2 was computed using publicly available data from SOCAT version 2019 (Bakker et al., 2016). Atmospheric pCO2 was determined using monthly averaged values obtained by Palmer, Halley, Syowa and South Pole meteorological stations on Antarctica (https://www.esrl.noaa.gov/gmd/dv/site/?stacode= none). The white and black isolines depict the ΔpCO2 values of –25 and +25 μatm, respectively. The mean and standard deviation of ΔpCO2 for the entire area for each season is shown in the centre of each map. The Southern Ocean sectors are named as: Weddell Sea, Indian Ocean, Western Pacific Ocean, Ross Sea, and Bellingshausen and Amundsen Seas. The seasonal periods computed were austral summer (JFM), autumn (AMJ), winter (JAS), and spring (OND).
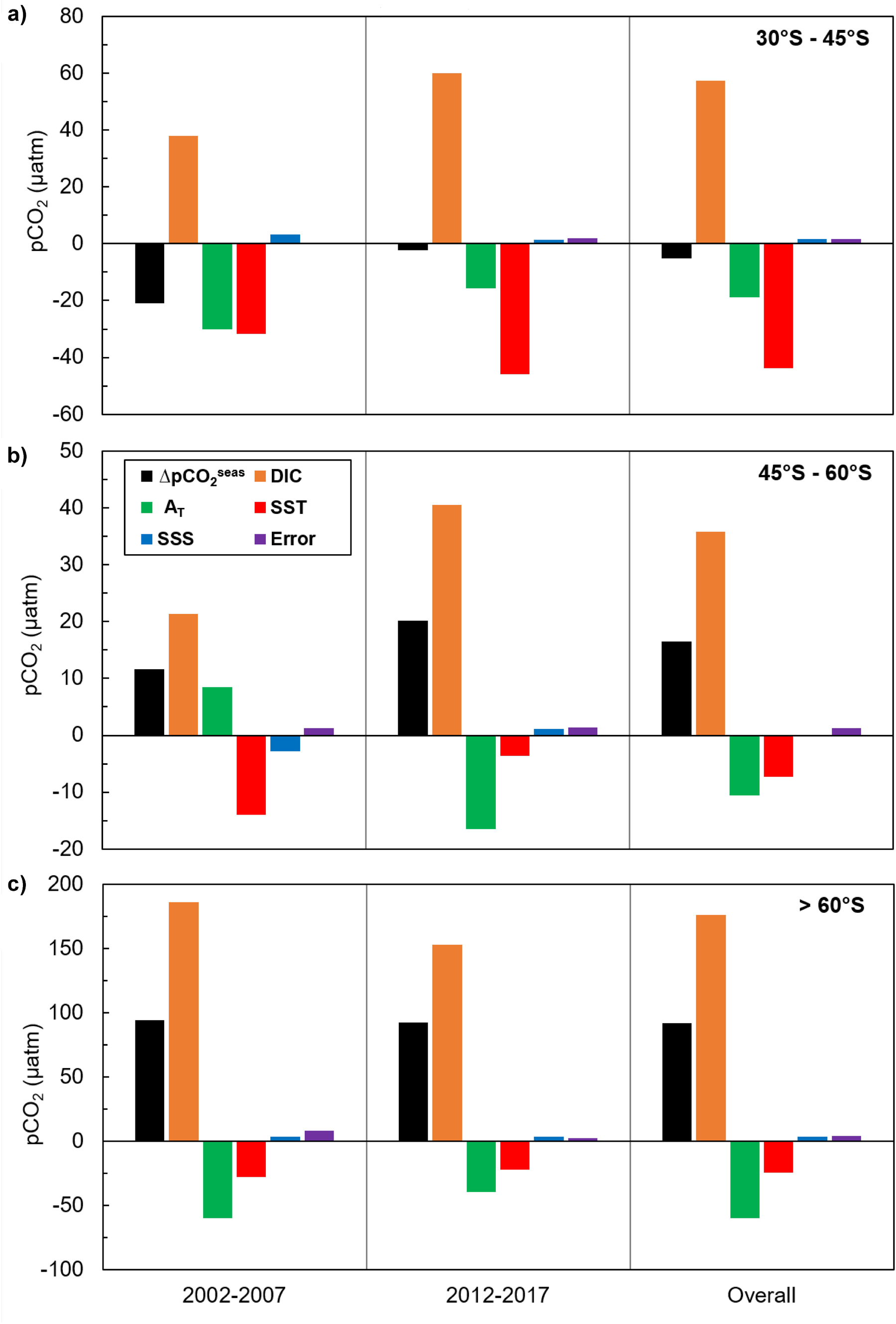
Figure 6. Effects of seasonal variation in DIC, total alkalinity (AT), temperature (SST) and salinity (SSS) on the seasonal variation in seawater pCO2 for each latitudinal zone of the Southern Ocean for 2002–2007 and 2012–2017: (a) 30–45°S, (b) 45–60°S, and (c) >60°S. The seasonal variation in each parameter is calculated as the difference between the winter (JAS) mean value and the summer (JFM) mean value. The unit of all drivers is the same as the unit of pCO2 (μatm), and their magnitudes represent their influence on seasonal pCO2 changes (ΔpCO2seas), from summer to winter. Positive values indicate that an increase in the parameter led to an increase in pCO2; negative values indicate that a decrease in the parameter led to a decrease in pCO2. The only exception to this is alkalinity because an increase in alkalinity leads to a decrease in pCO2 and vice versa. The error bars (purple) show the difference in seasonal variation between the sum of all drivers and pCO2, indicating the extent to which the decomposition of pCO2 into its drivers differs from the actual seasonal variation in pCO2 (ΔpCO2seas).
Changes in surface water pCO2, and therefore ΔpCO2 and sea-air CO2 fluxes, are driven by biological and physical processes acting simultaneously. Whilst it is difficult to separate the biological and thermal effects, examining the seasonal changes in DIC, total alkalinity and sea surface temperature and salinity can inform our understanding of the biological and solubility pump contributions to Southern Ocean CO2 uptake. Figure 6 shows the influence of seasonal changes in DIC, alkalinity, temperature and salinity on seasonal changes in seawater pCO2 in three latitudinal bands in 2002−2007 and 2012−2017 (after Takahashi et al., 2014; see Supplementary Information 3 for details). Between 30 and 45°S, DIC and temperature exert a similar influence on pCO2, indicating that seasonal changes in DIC driven by biological uptake in the summer and upwelling in winter are approximately balanced by seasonal changes in temperature and their control on the solubility pump. South of 45°S, and particularly in the Antarctic Zone south of 60°S, DIC and alkalinity exert a much stronger influence on pCO2 than temperature. As the influence of DIC is almost three times that of alkalinity, this is most likely to reflect the role of biological activity in spring and summer. Upwelling increases DIC around the Sub-Antarctic Front especially in winter, but the effect of this on pCO2 is partially compensated by the reduction in pCO2 driven by wintertime cooling and the increase in alkalinity. These results suggest that the contributions of biological and solubility pump processes to seasonal changes in pCO2 across the Southern Ocean are similar in magnitude for the time periods examined. Whilst it remains challenging to separate fully the effects of biological and solubility pump processes on DIC, alkalinity, temperature and salinity, and therefore pCO2, our findings are in agreement with previous studies assessing the magnitude of the two sinks. Estimates of net community production (NCP) over a large area of the Southern Ocean (38−55°S, 60°W−60°E) based on observations and modelling suggest that biological carbon uptake accounts for a sink of 0.2 Pg C year–1 (Merlivat et al., 2015). Compared to a total Southern Ocean (south of 35°S) sea-air CO2 flux of –0.75 ± 0.22 Pg C year–1 (Bushinsky et al., 2019), this suggests that the biological and solubility pumps account for a similar proportion of circumpolar CO2 uptake. A modelling study comparing outputs from different marine ecosystem and general circulation models also shows that biological and physical forcings of pCO2 are of the same order of magnitude for the Southern Ocean south of 44°S (Hauck et al., 2015).
Changes in the Biological Carbon Pump
Key Controls on Biological Carbon Uptake and Export
The important contribution of biological carbon uptake, export and storage in organisms to the Southern Ocean carbon sink is strongly influenced by primary and secondary production. Although primary production is limited by iron availability over much of the Southern Ocean (Sections “Changes in Primary Production and Phytoplankton Species Composition” and “Changes in Micronutrient Biogeochemistry”), hotspots of productivity around and downstream of sub-Antarctic islands and submerged plateaus (e.g., South Georgia, Kerguelen, Crozet), and in upwelling and mixing zones, coastal/shelf areas and the sea ice zone where iron is not limiting can lead to substantial export of organic carbon to deep waters and/or sediments. Primary production and carbon export are particularly high in the Atlantic sector (Figure 7a) due to enhanced iron supply from upstream land masses (Patagonia, Falkland/Malvinas Islands, South Georgia and the WAP).
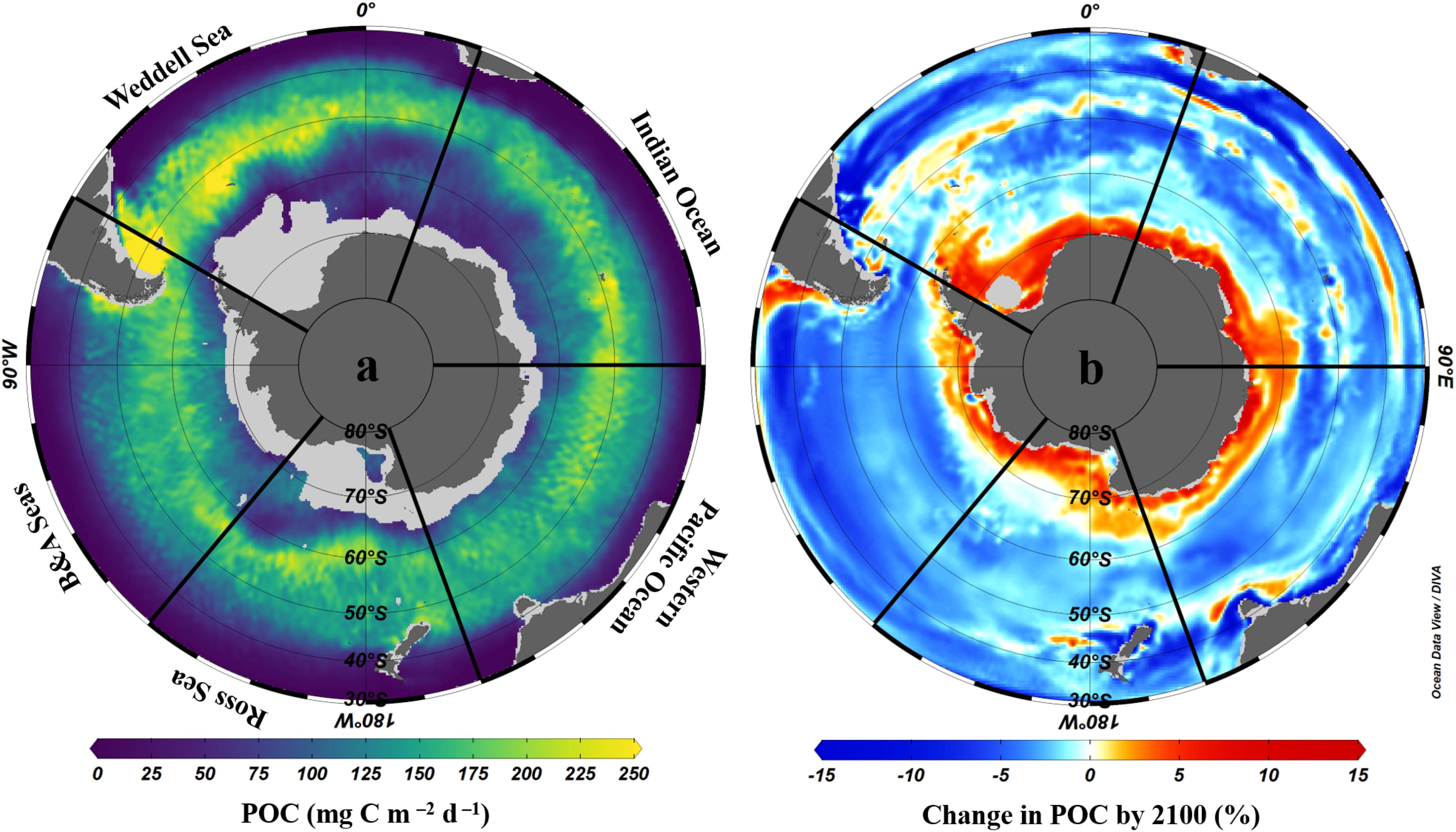
Figure 7. Mean annual POC export at 100 m across the Southern Ocean; (a) present day (2003–2016) from LIDAR data using the Britten et al. (2017) algorithm as in Arteaga et al. (2018) and (b) mean change by the year 2100 according to eight CMIP5 models following methods used in Cavan et al. (2019b). Data are interpolated using the DIVA method in Ocean Data View software (Schlitzer, 2018), with scale-length for Y and X of 2 per mille. The Southern Ocean sectors are named as: Weddell Sea, Indian Ocean, Western Pacific Ocean, Ross Sea, and Bellingshausen and Amundsen Seas.
Phytoplankton species composition exerts an important control on the biological pump, with diatoms being exported quickly and promoting export compared to smaller non-diatom phytoplankton, due to their large size and ballasting by biogenic silica (Buesseler, 1998; Ducklow et al., 2001; Armstrong et al., 2009). As such, sinking diatoms are important vectors transporting organic carbon to the deep Southern Ocean (e.g., Cavan et al., 2015), with burial of whole diatoms in sediments emphasising their importance (Armand et al., 2008) and showing that they were exported directly rather than being consumed. Diatoms can escape predation after a spring bloom by transforming into resting spores that sink rapidly to the deep before returning to the surface as viable cells after winter, but many do not return and instead remain at depth contributing to longer-term carbon storage (Rembauville et al., 2018).
Zooplankton faecal pellets also often dominate the biological carbon sink in the Southern Ocean (Cavan et al., 2015). Euphausia superba (Antarctic krill) are particularly prevalent in the Atlantic sector, where their swarms can release huge numbers of faecal pellets of up to 0.04 Gt C year–1 (Belcher et al., 2019). Whilst a large contribution of faecal pellets to sinking material can result in a large carbon flux to depth, flux efficiency varies substantially because pellets can be broken up easily before they reach the deep sea (Iversen and Poulsen, 2007). For instance, at one site near the Kerguelen Islands, in the Indian sector, only 17% of the exported material sank below 400 m even though copepod faecal pellets dominated the flux at this depth (72%) (Laurenceau-Cornec et al., 2015). Conversely at a nearby site, 58% of export reached 400 m with a more even distribution between phytoplankton and faecal pellet components (48% pellets), because primary production was dominated by large fast-sinking diatoms, which escaped grazing by copepods (Cavan et al., 2019b). This emphasises the importance of both phytoplankton and zooplankton species composition in Southern Ocean carbon export and its complex variability in time and space.
Organic matter recycling via the microbial loop reduces substantially the amount of carbon available for export and consumption by higher trophic levels (Azam et al., 1991; Azam, 1998; Sailley et al., 2013). Interactions between bacteria and viruses have been shown to contribute to these recycling processes and the resultant regeneration of nutrients including iron (Evans et al., 2009; Evans and Brussaard, 2012), as well as producing refractory dissolved organic matter, which is largely inaccessible to biological uptake by organisms (Weinbauer et al., 2011; Weitz and Wilhelm, 2012). Whilst these interactions reduce the export of particulate organic carbon, as well as carbon storage within the food web (Section “Carbon Transfer and Storage in Pelagic and Benthic Food Webs”), the large pool of refractory dissolved organic matter in the deep ocean derived from this microbial carbon pump constitutes an important carbon sink (Jiao et al., 2010; Jiao et al., 2011) that is poorly quantified and warrants further investigation.
Future Changes in Biological Carbon Uptake and Export
Changes in primary production have a direct and significant impact on biological carbon uptake. As such, changes in carbon export across the Southern Ocean are likely to follow trends in primary production, with an overall increase over the 21st century, in contrast to reductions at the global scale (Cabré et al., 2015; Moore et al., 2018). Consistent with projections of primary production (Section “Changes in Primary Production and Phytoplankton Species Composition;” Pinkerton et al., to be published in this research topic), CMIP5 model simulations project, on average, increases in carbon export in the Sub-Antarctic north of 50°S, particularly in the Atlantic sector, and south of ∼60°S in the Antarctic Zone, particularly in the Indian sector, whilst decreases are projected between 50 and ∼60°S in the open Southern Ocean (Figure 7b). Documented and projected increases in primary production and export for the Southern Ocean as a whole, and particularly in regions where mode, intermediate and deep waters form and where net CO2 uptake is already observed, suggest an increase in its capacity for biological CO2 uptake (Del Castillo et al., 2019). This would be compounded by a projected increase in the Revelle factor over the 21st century, which increases CO2 uptake for a given amount of export production, strongly increasing the importance of the biological carbon pump across the Southern Ocean (Hauck and Völker, 2015; Hauck et al., 2015). Increases in export are driven primarily by phytoplankton responses to increased iron inputs, shallowing mixed layers and ocean warming, both in terms of community growth rates and thus total primary production, and species composition (Cabré et al., 2015; Leung et al., 2015; Fu et al., 2016; Boyd, 2019). Some models predict that diatoms will be favoured by increased nutrient availability in Southern Ocean surface waters driven by increasing wind stress, further enhancing export, whilst others suggest equivalent increases across phytoplankton groups because warming-induced growth rate increases become the dominant effect (e.g., Laufkötter et al., 2013, 2015).
In the coastal and shelf areas, which are known to be strong local/regional CO2 sinks (Arrigo et al., 2008b; Mu et al., 2014; Brown et al., 2019; Monteiro et al., 2020), carbon uptake may be further enhanced by increasing glacial meltwater inputs (Cook et al., 2016), because surface water cooling and freshening can augment the solubility pump, in addition to increased iron supply and primary production (Gerringa et al., 2012; Sherrell et al., 2015; Annett et al., 2017; Monteiro et al., 2020). Changes in sea ice dynamics are also likely to influence carbon uptake, because longer ice-free growing seasons and/or more stratified upper ocean conditions promote the development of phytoplankton blooms that drive the biological pump (Montes-Hugo et al., 2009; Venables et al., 2013; Moreau et al., 2015; Costa et al., 2020).
Changes in phytoplankton species composition and size distribution may reduce the efficiency of the biological pump, and thus complicate our understanding of future changes to the Southern Ocean CO2 sink (e.g., Passow and Carlson, 2012; Laufkötter et al., 2013). At the WAP, some local increases in primary production and changes in phytoplankton development are linked to sea ice declines, increasing glacial meltwater inputs, ocean warming and freshening (Schloss et al., 2014; Hernando et al., 2015; Moreau et al., 2015; Rogers et al., 2020), and have led to a fivefold increase in summertime CO2 uptake along the shelf in recent decades (Brown et al., 2019). However, interannual variability is pronounced, and ongoing and projected species shifts towards smaller non-diatom phytoplankton could reduce export substantially by lowering the export efficiency as well as primary production (Montes-Hugo et al., 2008, 2009; Mendes et al., 2012, 2018; Rozema et al., 2017; Schofield et al., 2017). An overall decline in biological carbon uptake has thus been hypothesised for the coming decades, in response to the continued warming and sea ice declines expected throughout the WAP region (e.g., Laufkötter et al., 2013; Brown et al., 2019). Even in regions where diatom communities thrive, reductions in diatom silica production and shifts toward smaller species with thinner shells in response to ocean acidification could reduce diatom sinking rates and diminish carbon export efficiency in the coming decades (Petrou et al., 2019). In the ACC, projected increases in iron supply could increase the amount of carbon exported with the sinking diatom flux (Assmy et al., 2013), partially offsetting the reduction in export efficiency associated with acidification, although the relative strength of these effects is unknown.
Amongst CMIP5 models, there is an order of magnitude difference in projected phytoplankton growth rates as well as spatial differences in carbon export, with some models projecting increases across the entire Southern Ocean and others suggesting increases in the marginal and seasonal ice zones but declines in the HNLC Sub-Antarctic (Bopp et al., 2013; Hauck et al., 2015; Laufkötter et al., 2016). Major uncertainties arise from future changes in the efficiency of the microbial loop, in particular the role of bacteria−virus interactions, and the impact of environmental changes on the coupling of phytoplankton and zooplankton dynamics and consequences for export. Although there is greater disparity among climate models in biological projections than in projections of nutrient distributions globally, increases in Southern Ocean primary production and carbon export are expected overall, with the potential to increase the relative importance of the biological carbon pump (Cabré et al., 2015; Hauck et al., 2015; Laufkötter et al., 2016).
Carbon Transfer and Storage in Pelagic and Benthic Food Webs
Carbon Fixation by Primary Producers
Carbon uptake and storage by organisms in the Southern Ocean are dominated by coastal and shelf ecosystems, the marginal ice zone and downstream of Sub-Antarctic islands (e.g., Bakker et al., 2007; Blain et al., 2007; Arrigo et al., 2008a, b; Jones et al., 2012; Hoppe et al., 2017). Most carbon uptake is by phytoplankton through primary production when light and nutrients are sufficient, and carbon is stored by animal components of the food web. The major contribution of large diatoms to primary production and phytoplankton biomass, and the regional, seasonal and interannual variability in phytoplankton species composition (Section “Changes in Primary Production and Phytoplankton Species Composition”), influence the degree and duration of carbon uptake and storage within the ecosystem. Macroalgae are also important primary producers in coastal areas, and new evidence shows that macroalgal organic matter reaches the open ocean and the deep sea in several ocean basins, including the Southern Ocean (Ortega et al., 2019). Whilst carbon uptake by primary production is similar in magnitude to the direct uptake of CO2 by solubility pump processes across the Southern Ocean (Section “Changes in the Southern Ocean Carbon Sink”), transfer of carbon fixed during primary production into heterotrophic organisms can lead to rapid sequestration, adding to the complete removal of carbon from cycling, through burial in sediments or storage as refractory carbon in the deep ocean. The immediate fate of primary production is an important food web step in determining the ultimate fate of carbon (sequestration or recycling), transfer efficiency and pathway, yet the relative proportions of these fate paths are poorly constrained.
Fate Pathways of Primary Production
Phytoplankton carbon can either be sequestered directly, broken down by microbes or eaten by pelagic animals, whose faecal pellets may eventually reach the seafloor, or by benthic consumers. Estimates of the proportion of primary production following each of these fate paths vary considerably between studies, depending on region, timescale and the number of these paths examined. Figure 8 presents a broad overview of the approximate mean percentages across large spatial and temporal scales. Approximately 1% is sequestered directly by sinking to and burial in the seabed, avoiding complete microbial breakdown in the water column and oxygenated seafloor (Ducklow et al., 2007). This is hugely variable; for example, there is more than an order of magnitude variation between years in estimates of how much primary production reaches the WAP shelf floor (from <0.2 to 4 g C m–2 year–1; Ducklow et al., 2007). Approximately half of global primary production is broken down by microbial processes in the water column or on the seabed (Azam, 1998). In the cold Southern Ocean, microbial cycling and bacterial production may be slower than elsewhere, but can account for a large proportion of primary production and contribute significantly to food web dynamics by cycling organic matter and regenerating nutrients (Azam et al., 1991; Sailley et al., 2013). Enhancement of the microbial loop by bacteria-virus interactions has also been observed in the Southern Ocean (Evans et al., 2009; Evans and Brussaard, 2012).
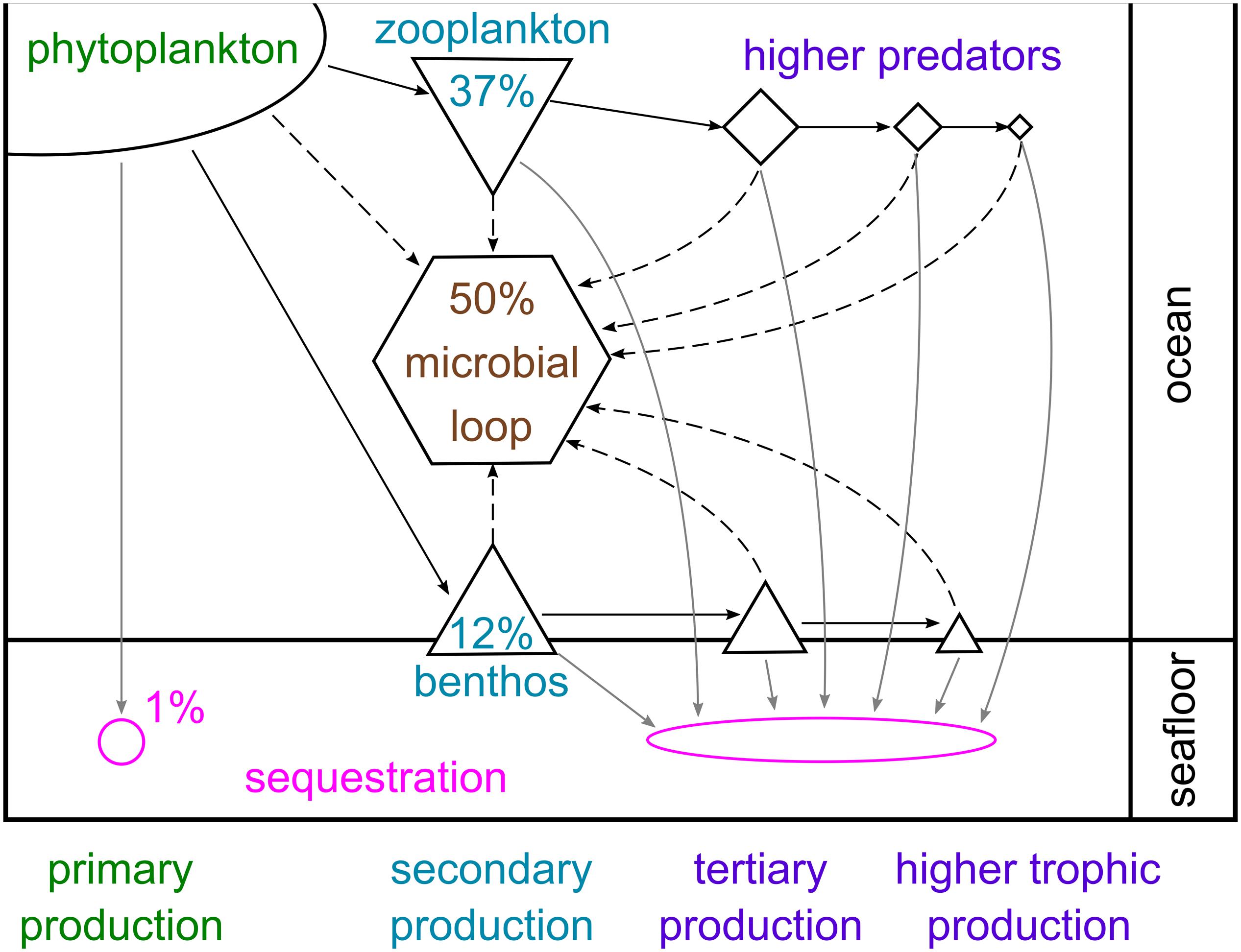
Figure 8. Schematic representation of carbon flows through pelagic and benthic food webs in the Southern Ocean. The percentages given represent approximate mean proportions of total primary production sequestered in the sea floor, broken down via the microbial loop and consumed by zooplankton and benthic consumers, based on several studies from high-productivity shelf environments and the wider literature (Azam, 1998; Ducklow et al., 2007; Hill et al., 2012; Garzio et al., 2013; Sailley et al., 2013; Ballerini et al., 2014; Murphy et al., 2016; Barnes, 2017).
The second largest carbon fate is grazing by zooplankton (micro-, meso- and macrozooplankton), of which annual production can account for up to 80% of primary production (Hill et al., 2012; Garzio et al., 2013; Sailley et al., 2013; Murphy et al., 2016; Moreau et al., 2020). Consumption by benthic animals is the carbon fate characterised by the highest diversity (many animal phyla) and richness (tens of thousands of species), and has been estimated to account for up to 18% of primary production (Barnes, 2017). The nature of animal production in the water column and seabed, and therefore carbon pathways, is strongly influenced by the timing, duration and composition of the phytoplankton bloom (as well as by other factors such as sea ice). For example, E. superba typically prefer larger diatoms over small phytoplankton (Quetin and Ross, 1985; Haberman et al., 2003; Bernard et al., 2012), and have also been shown to graze on copepods, microzooplankton and even salps (Price et al., 1988; Atkinson and Snyder, 1997; Schmidt et al., 2006; Dubischar et al., 2012). Microzooplankton can consume almost the full range of phytoplankton size classes (Klaas, 1997; Calbet and Landry, 2004), whilst salps are non-selective filter feeders on phytoplankton as well as microzooplankton and small copepods (Bernard et al., 2012). In contrast, nanophytoplankton are preferred by benthic suspension feeders (Barnes and Clarke, 1995).
Strong regional and onshore-offshore differences in grazing and retention of organic carbon in euphotic surface waters show that the location of consumption also affects carbon fate (Gleiber, 2015). The type of animal consuming phytoplankton has important consequences for faecal and carcass sinking rates, and hence likelihood of reaching the seabed (carbon sequestration), support for higher trophic levels (food web carbon storage), growth rates and longevity (storage and sequestration). Larval krill are abundant at the WAP and near sea ice because sea ice provides protection from predators and an important overwintering food source of ice-derived organic material (Meyer et al., 2017). Faecal pellets of larval krill are smaller and contain less carbon than those of adults, but deeper diel vertical migrations by larvae (∼400 m) than adults (∼200 m) (Tarling et al., 2018) mean their faeces has a higher chance of reaching the sea floor (Cavan et al., 2019a). On the sea floor, the quantity and quality of the food supply from overlying waters varies considerably between the shallow coastal areas and the deep shelf, with annual accumulation of carbon by benthos at 500 m being only 10% of that at 25 m along the WAP (Barnes, 2017). Animals at slope, abyssal and deep-shelf depths are not in direct contact with the phytoplankton bloom, so are more likely to be dominated by deposit feeders and their predators.
Carbon Storage in Pelagic and Benthic Consumers
The animal pathway beyond primary consumption is typically short, but still supports considerable abundances of up to four trophic levels (Gillies et al., 2013), much of which hinges around E. superba as a key consumer. The number of trophic levels has a direct effect on the amount of carbon (or energy) stored in a food chain (Dickman et al., 2008), whilst the duration of carbon storage depends on the life spans of consumers. In the Atlantic sector, a highly efficient pelagic food chain consists of only three trophic levels: diatoms, krill and baleen whales (Tranter, 1982; Smetacek, 2008). Baleen whales found in the Southern Ocean are slow-growing and can have long life spans of up to 90 years. In the Atlantic sector, blue and fin whales feed predominantly on krill, and with mean weights of ∼90 and ∼50 tonnes, respectively, store a huge amount of carbon as organic mass for almost a century (Laws, 1977). After death, most whales’ carcasses sink to the sea floor, providing a rich food source for many benthic and deep-sea organisms. Microbes also decompose organic material from the whale fall, releasing large amounts of nutrients, and respired CO2 and potentially refractory dissolved organic carbon are locked in the deep ocean for decades or even centuries (Jiao et al., 2010; Cavan et al., 2019a). Elsewhere in the Southern Ocean, such as in the central Indian sector, E. superba are important but do not dominate the ecosystem as they do in the Atlantic (Everson, 2000; McCormack et al., to be published in this research topic). Near Prydz Bay, cephalopods dominate the food web, with most carbon cycling through them to sperm whales and leopard seals (McCormack et al., 2019). This constitutes a longer food chain of at least five trophic levels: phytoplankton, copepods, krill, cephalopods and sperm whales or seals. Less carbon is stored in this pathway due to multiple trophic transfers and because the top predators are smaller (sperm whales weigh ∼30 tonnes and seals weigh ∼0.3 tonnes) and die younger than blue or fin whales, such that carbon may only be stored for a few decades (Laws, 1977). Smaller mammals may also sink more slowly and be less likely to reach the deep ocean, although more research into this topic is required.
Climate Change Impacts on Food Webs and Carbon Uptake and Storage
There is emerging evidence of climate forcing on Southern Ocean food webs, with complex effects on species and trophic interactions (e.g., Henley et al., 2019). Projected increases in primary production (Section “Changes in Primary Production and Phytoplankton Species Composition”) are likely to be beneficial for pelagic and benthic consumers, with shifts in phytoplankton species composition favouring certain consumers and disadvantaging others. At the WAP, pronounced interannual variability in primary production, with some local increases, and changes in phytoplankton species composition (e.g., Schloss et al., 2014; Moreau et al., 2015; Kim et al., 2018) have strong implications for krill and other zooplankton (e.g., Steinberg et al., 2015). For example, summers characterised by large diatom-dominated phytoplankton blooms, associated with long-lived winter sea ice cover and stratified surface waters in spring and summer, lead to strong krill recruitment the following summer (Saba et al., 2014). Long-term sea ice declines and ongoing shifts toward smaller phytoplankton could thus drive reductions in krill populations (Saba et al., 2014). At the larger scale, there is significant debate in the scientific literature regarding decadal trends in the distribution and density of krill populations and their drivers (Johnston et al., to be published in this research topic, and references therein). There is evidence for krill populations in the southwest Atlantic sector declining and/or shifting their distribution southward, related to wintertime sea ice cover particularly in the important spawning and nursery areas around the WAP and Southern Scotia Arc, with potential linked increases in salp abundance (Atkinson et al., 2004, 2019; Hill et al., 2019). However, other studies suggest important spatial differences in observed patterns of krill variability and change, and potentially a more stable trajectory overall (Cox et al., 2018; Johnston et al., to be published in this research topic, and references therein). If krill declines are real and sustained, there could be significant disruption to higher predators as well as ecosystem functions such as ocean fertilisation and carbon cycling and storage (Dubischar et al., 2012; Alcaraz et al., 2014; Atkinson et al., 2019; Cavan et al., 2019a). Amongst benthos, both sea ice losses and ice shelf disintegration have had pronounced effects on production and carbon storage over the last two decades. New and longer phytoplankton blooms (Schloss et al., 2014; Moreau et al., 2015; Kim et al., 2018) have increased benthos growth overall despite decreased growth in the shallows due to increased iceberg scour (Barnes et al., 2018). More than a doubling of carbon storage with recent sea ice losses suggests a rare and powerful short-term negative feedback on climate by Southern Ocean biota (Peck et al., 2010; Barnes et al., 2018), although the persistence of this trend is in doubt (Brown et al., 2019).
Exchange and Redistribution of Carbon and Nutrients by Animals
Benthic-Pelagic Coupling and Water Column Transports
Coupling of benthic and pelagic ecosystems through a range of biological and physical processes plays a key role in modulating biogeochemistry and ecological function in the Southern Ocean. Nutrient recycling within and release from sediments, particularly of iron, and subsequent delivery to euphotic surface waters via lateral and vertical transport, is a key benthic-pelagic coupling mechanism sustaining food webs. Benthic iron fluxes are largest in continental shelf and slope regions, but can also be important in the deep Southern Ocean, and are regulated by organic carbon oxidation rates in sediments and bottom water oxygen concentrations (Tagliabue et al., 2009a; De Jong et al., 2012; Dale et al., 2015). Nutrient transport within the pelagic realm between the deeper waters, enriched with macro- and micronutrients (i.e., iron) as a result of water column remineralisation of organic matter, and the surface waters is also critical for ocean productivity and ecosystem function (Sections “Changes in Primary Production and Phytoplankton Species Composition,” “Changes in Micronutrient Biogeochemistry,” and “Changes in Macronutrient Biogeochemistry”). There is a growing body of evidence for krill, whales and other higher organisms enhancing the transport of these nutrients regenerated in the subsurface, deep waters and sediments into surface waters (e.g., Schmidt et al., 2011; Ratnarajah et al., 2014).
Nutrient Recycling and Redistribution by Animals
Krill have been shown to release dissolved iron, ammonium and phosphate through grazing and excretion processes (Tovar-Sanchez et al., 2007; Ratnarajah et al., 2014; Schmidt et al., 2016), each providing a nutrient source to phytoplankton blooms (Atkinson and Whitehouse, 2000; Ariśtegui et al., 2014). Copepods also play an important role in regenerating and retaining iron in the surface ocean through grazing and rapid recycling of faecal pellets (Sarthou et al., 2008; Laglera et al., 2017). Whales produce iron-rich faeces, which constitute a highly concentrated, albeit highly localised, iron source (Nicol et al., 2010; Lavery et al., 2014). Recycling of iron is particularly important in the iron-limited regions of the Southern Ocean, and release by krill, other zooplankton, marine mammals and seabirds in the upper layers could stimulate phytoplankton productivity (Nicol et al., 2010; Ratnarajah et al., 2018; Cavan et al., 2019a). For instance, sperm whales have been shown to increase carbon export in the Southern Ocean, as primary production and carbon export stimulated by their faecal iron supply to surface waters exceeds their respiration by an estimated 200,000 t C year–1 (Lavery et al., 2010).
Southern Ocean organisms are also important as transporters and redistributors of the nutrients that they recycle, depending on their migration and feeding behaviours (Ratnarajah et al., 2018). Krill and other zooplankton species that undergo daily (diel) vertical migrations between surface waters where they feed and deeper waters where they avoid predation (Hernández-León et al., 2001; Tarling et al., 2018) can transfer significant quantities of carbon and nutrients to the deep ocean, via defecation of organic matter as faecal pellets and release of CO2 at their deep resting depths (Cavan et al., 2015; Belcher et al., 2017). Similarly, zooplankton species such as copepods that migrate seasonally between food-rich surface waters in spring/summer and deeper waters below the permanent thermocline in winter also transport carbon and nutrients to depth via net consumption in surface waters and net respiration at depth (Lee and Hagen, 2006; Jónasdóttir et al., 2015). Conversely, krill that live and feed at the seafloor may transfer nutrients, particularly iron, to the surface from benthic environments (Schmidt et al., 2011). Marine mammals, seabirds and squid that feed at depth can transport iron and other nutrients into the euphotic zone, where their release can stimulate phytoplankton growth (Ratnarajah et al., 2018 and references therein). Nutrient recycling through krill and other organisms in water masses that are transported north from the Southern Ocean may also impact organisms in the lower latitudes (Cavan et al., 2019a).
Land-Ocean Nutrient Transfer
Seals, penguins and other seabirds play an important role in transporting carbon, nitrogen and phosphorus from the ocean into terrestrial environments of the sub-Antarctic islands and Antarctica (Moss, 2017 and references therein). There is also evidence for nutrient supply, including iron, to coastal marine environments from seals and seabirds and their colonies in the Sub-Antarctic via faecal material and run-off from guano deposits (Wing et al., 2014; Treasure et al., 2015). Whilst these nutrient fluxes are small in the context of Southern Ocean biogeochemistry, they can be important locally for fertilising coastal phytoplankton blooms and macroalgal production, thus supporting nearshore ecosystems and benthic food webs in particular.
Turbulent Mixing by Animals
In addition to biological recycling and redistribution of nutrients by animals, large planktonic migrations consisting of many thousands of individuals have the potential to bring nutrient-rich waters from depth towards the surface by stimulating physical mixing of the water column. This has been shown in jellyfish swarms (Katija and Dabiri, 2009) but is yet to be studied in krill or other zooplankton, although mixing between density layers has been shown by brine shrimp in laboratory experiments (Houghton et al., 2018). The high abundance of E. superba, their relatively large size (up to 6 cm) and large migrations suggest that they could promote physical mixing and vertical nutrient transport in the Southern Ocean (Tarling and Thorpe, 2017; Cavan et al., 2019a). The contribution of these migratory behaviours to total nutrient transfer is not well constrained, and should be prioritised for future research in order to assess its role now and in the future under climate change.
Ocean Acidification and Its Effects on the Ecosystem
Ocean Carbonate Chemistry and Acidification
Uptake of CO2 into the Southern Ocean by biological and solubility pump processes is modifying ocean chemistry and driving ocean acidification. Ocean acidification is a climate change issue impacting marine biota, marine ecosystem functioning (Fabry et al., 2008; Guinotte and Fabry, 2008), and potentially marine ecosystem services at the global scale (Cooley et al., 2009). Increasing oceanic CO2 concentrations in response to increasing atmospheric CO2 are altering the equilibrium between the carbonate system parameters (pCO2, DIC, total alkalinity and pH), leading to reductions in the pH and carbonate ion concentration of seawater (Doney et al., 2009; Feely et al., 2009). Carbonate ion availability is essential for marine organisms that build their shells and skeletons out of aragonite, a metastable form of calcium carbonate, such that lowering the seawater carbonate ion concentration has adverse effects on vital processes and survival of a large number of organisms. The aragonite saturation state (Ω) is a measure of the seawater carbonate ion concentration relative to aragonite at equilibrium, with a critical threshold of Ω = 1 below which seawater is undersaturated with respect to aragonite and can become corrosive to the shells and skeletons of marine organisms. The Southern Ocean is expected to experience aragonite undersaturation earlier than most other oceans (except the Arctic), particularly during winter, due to its large uptake of atmospheric CO2 and because its carbonate ion concentration is already low due to low temperatures and upwelling of CO2-rich deep waters (Orr et al., 2005; McNeil and Matear, 2008; Feely et al., 2009).
Observed and Expected Southern Ocean Acidification
Decadal trends of increasing DIC, decreasing pH and/or decreasing Ω have been documented in surface and deeper waters in a number of Southern Ocean regions, including Drake Passage (Takahashi et al., 2014; Munro et al., 2015), the coastal northern WAP (Lencina-Avila et al., 2018), the Weddell Sea (Hauck et al., 2010; Van Heuven et al., 2014), and the Pacific sector (Midorikawa et al., 2012; Williams et al., 2015). Aragonite undersaturation has been observed in the Southern Ocean, and is caused by upwelling of deeper waters, advection of waters enriched with anthropogenic carbon and/or in situ uptake of atmospheric CO2 (Bednarsek et al., 2012; Jones et al., 2017; Kerr et al., 2018). Model simulations project a rapid spread and increased duration of aragonite undersaturation events around 2030, affecting ∼30% of Southern Ocean surface waters by 2060 (Hauri et al., 2016). However, the combined effects of multiple natural and anthropogenic drivers (e.g., warming, freshening, meltwater inputs, oceanic water intrusions, sea-air CO2 exchange) on the carbonate equilibrium may partially offset the trends in acidification and aragonite undersaturation and their effects in Antarctic coastal and oceanic regimes (McNeil et al., 2010; Lencina-Avila et al., 2018).
Effects of Ocean Acidification on Organisms and Ecosystem Functioning
Direct and indirect effects of ocean acidification on the Southern Ocean food web are increasingly well-documented, with benefits for some organisms and disadvantages for others. Ross Sea phytoplankton communities have been observed to shift their species composition from P. antarctica or pennate diatoms at low CO2 to large centric chain-forming Chaetoceros diatoms at higher CO2 (Tortell et al., 2008; Feng et al., 2010). This is consistent with increased growth rates of C. debilis in experiments with high pCO2 (Trimborn et al., 2013). In contrast, high CO2 in coastal East Antarctica has been shown to reduce primary production and favour small diatoms (≤20 μm) over large diatoms and P. antarctica, indicating regional variation in the phytoplankton response to acidification (Hancock et al., 2018; Westwood et al., 2018). Primary production, biomass accumulation and nutrient uptake rates declined significantly above a threshold of pCO2 three times higher than ambient levels, although photosynthetic performance showed evidence of acclimation to high CO2 over time (Deppeler et al., 2018). Ocean acidification has also been shown to reduce diatom silica production directly at a lower threshold than diatom growth, by reducing both the cell-specific rates of silica precipitation and the relative abundance of larger species with thicker denser shells (Petrou et al., 2019). Further, iron uptake by diatoms has shown sensitivity to carbonate ion availability, such that reductions in carbonate ion concentration may also have deleterious indirect consequences for diatom growth (McQuaid et al., 2018). These studies highlight the importance of species-specific CO2 sensitivity, species interactions and physiological processes in modifying the phytoplankton response to increasing CO2, with a high degree of complexity over a range of spatial and temporal scales leading to changes in primary production and species composition that may act synergistically or antagonistically with the effects of changes in iron, light and temperature (Section “Changes in Primary Production and Phytoplankton Species Composition”).
Changes in phytoplankton dynamics associated with ocean acidification will have indirect impacts on zooplankton communities, in addition to the direct effects of acidification on a number of zooplankton species. Limacina helicina pteropods from the Scotia Sea have shown shell dissolution under aragonite-undersaturated in situ conditions (Bednarsek et al., 2012) and reduced larval survival due to decreased shell growth and increased fragility in aragonite-undersaturated experiments (Gardner et al., 2018). In contrast, E. superba have shown resilience to ocean acidification in laboratory simulations by maintaining the acid-base balance of their body fluids in near-future pCO2 (Ericson et al., 2018). However, E. superba in the WAP region are known to increase feeding and excretion rates under high CO2 conditions, especially pregnant females, indicating metabolic shifts consistent with increased physiological costs of maintaining their acid-base balance (Saba et al., 2012). Combined experimental and modelling approaches suggest that important E. superba habitats in the Weddell Sea are likely to suffer from reduced recruitment within a century, due to the sensitivity of egg hatch rates to increased CO2 (Kawaguchi et al., 2013).
BOX 1. Research gaps, progress and opportunities.
Scientific understanding of the key biogeochemical processes at work across the Southern Ocean throughout the seasonal cycle is limited by inconsistent data coverage between regions and over different timescales. Coverage is particularly poor in autumn and winter, biasing our understanding towards spring/summer processes. We lack year-round quantification of CO2 sink dynamics in both coastal and open-ocean regions, as well as an adequate understanding of the micro- and macronutrient cycling – and particularly the supply and bioavailability of iron – that regulates biological carbon uptake. Strong spatial and temporal variability in carbon and nutrient uptake and cycling, as well as the range of atmospheric, oceanic and cryospheric forcings and ongoing changes, complicate our estimates of the strength of the Southern Ocean CO2 sink (Lovenduski et al., 2015; Ritter et al., 2017; Woolf et al., 2019) and our projections of future changes in productivity and biogeochemical cycles. Moreover, the relative biogeochemical influence of changes in phytoplankton community rates versus species shifts remains poorly understood. Current model parameterisations cannot capture the complexity associated with the phytoplankton community response to concurrent shifts in at least five influential ocean properties, including light, iron, CO2, nutrients and temperature (Boyd et al., 2016). As such, model outcomes may change in the coming decade as mechanistic understanding and parameterisations improve.
In order to address these challenges, innovative measurement approaches must be integrated with traditional ship-based methods and satellite-based measurements to provide efficient long-term observational programmes. For example, autonomous biogeochemical floats are driving a substantial increase in spatial and temporal coverage of pCO2, nitrate and dissolved oxygen data (Johnson et al., 2017; Bushinsky et al., 2019). A range of autonomous platforms (e.g., gliders, powered autonomous underwater vehicles, moorings) and instrumented marine mammals equipped with a growing suite of biological and biogeochemical sensors (e.g., pH, iron, nutrients, fluorescence, bio-optics, acoustics) are increasingly supporting observational programmes across Southern Ocean regions (e.g., Henley et al., 2019). Focused experiments, process studies and increased use of novel tracers (e.g., isotopes of nitrate, silicic acid and iron), alongside and complementary to long-term observations, are augmenting our mechanistic understanding of the key processes, feedbacks and changes underway. Further investigations into species and physiological shifts in phytoplankton, zooplankton, bacterial and archaeal communities, and the importance of viruses, including molecular and other omics techniques will be critical to reducing uncertainties around projected changes in productivity, ecosystem function and biogeochemical cycling. Financial and logistical challenges associated with long-term monitoring in the Southern Ocean necessitate enhanced coordination of projects and national programmes, integration, intercalibration and, where possible, standardisation of analytical techniques, and improved access to biogeochemical data (Newman et al., 2019); for example through the Southern Ocean Observing System (SOOS) data portal SOOSmap (http://www.soosmap.aq/). Assimilation of expanding observational datasets into increasingly sophisticated models will lead to substantial improvements in our understanding of decadal changes and trends. In particular, models of iron biogeochemical cycling are enhancing our ability to project change in primary production, nutrient cycling and the Southern Ocean carbon sink (Tagliabue et al., 2016; St-Laurent et al., 2019). Only by taking an end-to-end approach to examining the ecosystem and accounting for the multiple stressors acting across a range of climate change scenarios will we achieve an integrated analysis of the entire Southern Ocean, and its central role in modulating changes in global biogeochemical cycles now and into the future (Murphy and Hofmann, 2012; Constable et al., 2014).
For Antarctic benthos, there is evidence for higher tolerance to ocean acidification than expected (Catarino et al., 2012; Kapsenberg and Hofmann, 2014), with increasing temperature being a more important factor (Byrne et al., 2013). Most organisms that have been studied in shallow coastal areas can acclimate to lowered pH to at least end-century conditions given sufficient acclimation periods (Cross et al., 2015; Suckling et al., 2015; Morley et al., 2016). However, shoaling of the aragonite saturation horizon (the depth above which Ω is >1 and below which waters are aragonite-undersaturated) from its current depth of ∼700 m towards the surface over the coming decades is likely to drive threshold responses in deep-shelf benthic communities, as the waters in which they reside shift from aragonite-saturated to aragonite-undersaturated (Orr et al., 2005; McNeil and Matear, 2008; Gutt et al., 2015).
The pelagic microbial community has shown sensitivity to ocean acidification, with increases in bacterial production and/or abundance observed in high-CO2 experiments in coastal East Antarctica (Deppeler et al., 2018; Westwood et al., 2018). In Ross Sea communities, lower seawater pH reduced bacterial diversity, but increased bacterial activity and rates of carbohydrate and lipid hydrolysis and nutrient regeneration (Maas et al., 2013). These increased rates of carbon and nutrient recycling could accelerate the microbial loop and reduce the amount of organic matter available for export or consumption. Combined with the acidification-induced reduction in diatom silica production and the likely reduction in sinking rates as a result (Petrou et al., 2019), a more efficient microbial loop has the potential to partially offset the projected increases in carbon export under climate change (Cabré et al., 2015; Laufkötter et al., 2016). There remains a significant degree of uncertainty regarding the impacts of ocean acidification on Southern Ocean food webs and biogeochemical cycling at present. Nevertheless, aragonite undersaturation is expected, with medium confidence, to play an influential role in the Southern Ocean under multiple stressors associated with anthropogenic climate change in the near future (Gutt et al., 2015).
Summary and Conclusions
This assessment of Southern Ocean biogeochemistry has assimilated existing knowledge and presented new data that show the integral role of biogeochemical cycling – of iron, carbon and major nutrients in particular – in supporting marine ecosystem functioning and regulating sea-air CO2 fluxes at regional and global scales. Strong variability in the key processes, controls and responses exists spatially, especially between shelf and open ocean regions, and over seasonal-to-decadal timescales.
Iron supply is extremely likely to increase in polar waters proximal to the Antarctic continent and also north of 50°S. Coupled with increasing light availability, this is very likely to increase primary production and carbon export in polar waters. Production, export and total biological carbon uptake are likely to increase for the Southern Ocean as a whole, whilst there is only medium confidence in projected increases in the Sub-Antarctic due to disparity among CMIP5 models. Changes in phytoplankton species composition are associated with higher uncertainty than changes in primary production. Three new datasets show that macronutrient uptake during summer influences nutrient availability and stoichiometry year-round in the coastal Antarctic and open Southern Ocean, despite vertical mixing and nutrient regeneration. Nutrient dynamics in the Sub-Antarctic are virtually certain to regulate the nutrient supply to the global thermocline and nutrient-limited surface waters to the north, thus modulating the global biological carbon pump. New analyses of ΔpCO2 changes show, on average, net CO2 sink behaviour for the entire Southern Ocean across all seasons, with similar contributions from biological and solubility pump processes and stronger CO2 uptake during summer. Ocean acidification has already been observed in some regions, with impacts on phytoplankton, microbial communities, zooplankton and other calcifying organisms, and is likely to become an important driver of Southern Ocean ecosystems in the coming decades.
Climate-driven changes in the productivity, biomass and distribution of phytoplankton, zooplankton, higher trophic level organisms and microbial communities are virtually certain to impact Southern Ocean biogeochemistry by modifying food web carbon transfer and storage, carbon export, nutrient recycling and redistribution, and benthic-pelagic coupling. In turn, changes in Southern Ocean biogeochemistry – in particular iron supply and ocean acidification – are very likely to alter productivity and function across marine ecosystem components, with important feedbacks on carbon and nutrient cycling at regional and global scales.
Data Availability Statement
The datasets presented in this study can be found in online repositories. The new winter nutrient data from the WOCE IO6 (2017) and A12 (2019) lines presented in Section “Changes in Macronutrient Biogeochemistry” are available at https://doi.org/10.5281/zenodo.3883618. The biogeochemical data from the Rothera Time Series programme in northern Marguerite Bay (2013−2016) presented in Section “Changes in Macronutrient Biogeochemistry” are available through the British Oceanographic Data Centre (BODC) at https://www.bodc.ac.uk/data/published_data_library/catalogue/10.5285/98cc0722-e337-029c-e053-6c86abc02029/ (Henley and Venables, 2019).
Author Contributions
SH led the study and writing of the manuscript. EC, SF, RK, and TMo provided data and contributed substantially to writing the manuscript and producing the figures. RS, AB, PB, DB, and IS contributed substantially to writing the manuscript. TMa provided data and contributed to figure production and writing the manuscript. RF and SS provided data and contributed to writing the manuscript.
Funding
SH was supported by the United Kingdom Natural Environment Research Council through grant NE/K010034/1. EC was supported by the United Kingdom Natural Environment Research Council research programme grant NE/S000348/1. SF was supported by the South African National Research Foundation’s Antarctic Programme (SANAP) through grants 105539 and 110735, the UCT Vice-Chancellor’s Future Leaders 2030 award, and the DSI’s Biogeochemistry Research Infrastructure Platform (BIOGRIP). RK and TMo received financial support through researcher grant No. 304937/2018-5 from CNPq and Ph.D. grant No. 88887.360799/2019-00 from CAPES, respectively, the latter available through the PROVOCCAR project grant No. 442628/2018-8 from CNPq/PROANTAR. AB would like to acknowledge the Australian Research Council (Grants FT130100037, DP150100345, and DP190103504) and the Antarctic Climate and Ecosystems Cooperative Research Centre for funding this research. PB was funded by a Laureate Fellowship (FL160100131) from the Australian Research Council. TMa, RF, and SS were funded by UCT Vice Chancellor Doctoral Research Scholarships and UCT Postgraduate Funding Merit Awards. TMa and SS were also funded by the South African National Research Foundation through postgraduate grants and a Ph.D. Innovation Scholarship (114590) to SS. RF was also supported by a UCT Science Faculty Fellowship.
Conflict of Interest
The authors declare that the research was conducted in the absence of any commercial or financial relationships that could be construed as a potential conflict of interest.
Acknowledgments
This work is a core contribution to the first Marine Ecosystem Assessment for the Southern Ocean (MEASO). We thank the MEASO Support Group and Steering Committee for assisting with figures, coordination, and editing of the text. We also thank the British Antarctic Survey for scientific and logistical support during all fieldwork campaigns at the Rothera Research Station, and for chlorophyll a data from the Rothera Time Series programme presented in Figure 3. We also thank Malcolm Woodward for macronutrient analysis of Marguerite Bay samples. The macronutrient data presented in Figure 2 were generated for samples collected during South African Department of Science and Innovation (DSI) supported research cruises. We thank Captain Knowledge Bengu and the crew of the R/V SA Agulhas II, the Department of Environment, Forestry and Fisheries, Chief Scientist Marcello Vichi (University of Cape Town; UCT), Raymond Roman and Kurt Spence (UCT) for assistance with nutrient and ammonium concentration analysis. We appreciate the availability of high-quality data from the Surface Ocean CO2 Atlas (SOCAT) and Palmer Station datasets. We also thank Lionel Arteaga for the LIDAR primary production and carbon export data. Finally, we thank Katherina Petrou and Dieter Wolf-Gladrow for thorough and constructive reviews of our work.
Supplementary Material
The Supplementary Material for this article can be found online at: https://www.frontiersin.org/articles/10.3389/fmars.2020.00581/full#supplementary-material
References
Abadie, C., Lacan, F., Radic, A., Pradoux, C., and Poitrasson, F. (2017). Iron isotopes reveal distinct dissolved iron sources and pathways in the intermediate versus deep Southern Ocean. Proc. Natl. Acad. Sci. U.S.A. 114, 858–863. doi: 10.1073/pnas.1603107114
Alcaraz, M., Almeda, R., Duarte, C. M., Horstkotte, B., Lasternas, S., and Agustí, S. (2014). Changes in the C, N, and P cycles by the predicted salps-krill shift in the southern ocean. Front. Mar. Sci. 1:13. doi: 10.3389/fmars.2014.00045
Alderkamp, A. C., Van Dijken, G. L., Lowry, K. E., Connelly, T. L., Lagerstrom, M., Sherrell, R. M., et al. (2015). Fe availability drives phytoplankton photosynthesis rates during spring bloom in the Amundsen Sea Polynya, Antarctica. Elem. Sci. Anth. 3:000043.
Alderkamp, A. C., Van Dijken, G. L., Lowry, K. E., Lewis, K. M., Joy-Warren, H. L., De Poll, W., et al. (2019). Effects of iron and light availability on phytoplankton photosynthetic properties in the Ross Sea. Mar. Ecol. Prog. Ser. 621, 33–50. doi: 10.3354/meps13000
Andrew, S. M., Morell, H. T., Strzepek, R. F., Boyd, P. W., and Ellwood, M. J. (2019). Iron availability influences the tolerance of southern ocean phytoplankton to warming and elevated irradiance. Front. Mar. Sci. 6:681. doi: 10.3389/fmars.2019.00681
Annett, A. L., Fitzsimmons, J. N., Séguret, M. J. M., Lagerström, M., Meredith, M. P., Schofield, O., et al. (2017). Controls on dissolved and particulate iron distributions in surface waters of the Western Antarctic Peninsula shelf. Mar. Chem. 196, 81–97. doi: 10.1016/j.marchem.2017.06.004
Ardyna, M., Lacour, L., Sergi, S., D’ovidio, F., Sallee, J. B., Rembauville, M., et al. (2019). Hydrothermal vents trigger massive phytoplankton blooms in the Southern Ocean. Nat. Commun. 10:2451.
Ariśtegui, J., Duarte, C. M., Reche, I., and Gómez-Pinchetti, J. L. (2014). Krill excretion boosts microbial activity in the Southern Ocean. PLoS One 9:e89391. doi: 10.1371/journal.pone.0089391
Armand, L. K., Crosta, X., Queguiner, B., Mosseri, J., and Garcia, N. (2008). Diatoms preserved in surface sediments of the northeastern Kerguelen Plateau. Deep Sea Res. Part II Top. Stud. Oceanogr. 55, 677–692. doi: 10.1016/j.dsr2.2007.12.032
Armstrong, R. A., Peterson, M. L., Lee, C., and Wakeham, S. G. (2009). Settling velocity spectra and the ballast ratio hypothesis. Deep Sea Res. Part II Top. Stud. Oceanogr. 56, 1470–1478. doi: 10.1016/j.dsr2.2008.11.032
Arrigo, K. R., Robinson, D. H., Worthen, D. L., Dunbar, R. B., Ditullio, G. R., Vanwoert, M., et al. (1999). Phytoplankton community structure and the drawdown of nutrients and CO2 in the Southern Ocean. Science 283, 365–367. doi: 10.1126/science.283.5400.365
Arrigo, K. R., Van Dijken, G. L., Alderkamp, A. C., Erickson, Z. K., Lewis, K. M., Lowry, K. E., et al. (2017). Early spring phytoplankton dynamics in the Western Antarctic Peninsula. J. Geophys. Res. Oceans 122, 9350–9369. doi: 10.1002/2017jc013281
Arrigo, K. R., Van Dijken, G. L., and Bushinsky, S. (2008a). Primary production in the southern ocean, 1997-2006. J. Geophys. Res. Oceans 113:C08004.
Arrigo, K. R., Van Dijken, G. L., and Long, M. (2008b). Coastal southern ocean: a strong anthropogenic CO2 sink. Geophys. Res. Lett. 35:L21602.
Arrigo, K. R., Van Dijken, G. L., and Strong, A. L. (2015). Environmental controls of marine productivity hot spots around Antarctica. J. Geophys. Res. Oceans 120, 5545–5565. doi: 10.1002/2015jc010888
Arteaga, L., Haëntjens, N., Boss, E., Johnson, K. S., and Sarmiento, J. L. (2018). Assessment of export efficiency equations in the southern ocean applied to satellite-based net primary production. J. Geophys. Res. Oceans 123, 2945–2964. doi: 10.1002/2018jc013787
Assmy, P., Smetacek, V., Montresor, M., Klaas, C., Henjes, J., Strass, V. H., et al. (2013). Thick-shelled, grazer-protected diatoms decouple ocean carbon and silicon cycles in the iron-limited Antarctic circumpolar current. Proc. Natl. Acad. Sci. U.S.A. 110, 20633–20638. doi: 10.1073/pnas.1309345110
Atkinson, A., Hill, S. L., Pakhomov, E. A., Siegel, V., Reiss, C. S., Loeb, V. J., et al. (2019). Krill (Euphausia superba) distribution contracts southward during rapid regional warming. Nat. Clim. Change 9, 142–147. doi: 10.1038/s41558-018-0370-z
Atkinson, A., Siegel, V., Pakhomov, E., and Rothery, P. (2004). Long-term decline in krill stock and increase in salps within the Southern Ocean. Nature 432, 100–103. doi: 10.1038/nature02996
Atkinson, A., and Snyder, R. (1997). Krill-copepod interactions at South Georgia, Antarctica, I. Omnivory by Euphausia superba. Mar. Ecol. Prog. Ser. 160, 63–76. doi: 10.3354/meps160063
Atkinson, A., and Whitehouse, M. J. (2000). Ammonium excretion by Antarctic krill Euphausia superba at South Georgia. Limnol. Oceanogr. 45, 55–63. doi: 10.4319/lo.2000.45.1.0055
Azam, F. (1998). Microbial control of oceanic carbon flux: the plot thickens. Science 280, 694–696. doi: 10.1126/science.280.5364.694
Azam, F., Smith, D. C., and Hollibaugh, J. T. (1991). The role of the microbial loop in Antarctic Pelagic ecosystems. Polar Res. 10, 239–243.
Bakker, D. C. E., Nielsdóttir, M. C., Morris, P. J., Venables, H. J., and Watson, A. J. (2007). The island mass effect and biological carbon uptake for the subantarctic Crozet Archipelago. Deep Sea Res. Part II Top. Stud. Oceanogr. 54, 2174–2190. doi: 10.1016/j.dsr2.2007.06.009
Bakker, D. C. E., Pfeil, B., Landa, C. S., Metzl, N., O’brien, K. M., Olsen, A., et al. (2016). A multi-decade record of high-quality fCO2 data in version 3 of the Surface Ocean CO2 Atlas (SOCAT). Earth Syst. Sci. Data 8, 383–413.
Balch, W. M., Drapeau, D. T., Bowler, B. C., Lyczskowski, E., Booth, E. S., and Alley, D. (2011). The contribution of coccolithophores to the optical and inorganic carbon budgets during the Southern ocean gas exchange experiment: new evidence in support of the “Great Calcite Belt” hypothesis. J. Geophys. Res. Oceans 116:C00F06.
Ballerini, T., Hofmann, E. E., Ainley, D. G., Daly, K., Marrari, M., Ribic, C. A., et al. (2014). Productivity and linkages of the food web of the southern region of the western Antarctic Peninsula continental shelf. Prog. Oceanogr. 122, 10–29. doi: 10.1016/j.pocean.2013.11.007
Barnes, D. K. A. (2017). Polar zoobenthos blue carbon storage increases with sea ice losses, because across-shelf growth gains from longer algal blooms outweigh ice scour mortality in the shallows. Glob. Change Biol. 23, 5083–5091. doi: 10.1111/gcb.13772
Barnes, D. K. A., and Clarke, A. (1995). Seasonality of feeding activity in Antarctic suspension feeders. Polar Biol. 15, 335–340.
Barnes, D. K. A., Fleming, A., Sands, C. J., Quartino, M. L., and Dereqibus, D. (2018). Icebergs, Sea ice, blue carbon and Antarctic climate feedbacks. Philos. Trans. R. Soc. Math. Phys. Eng. Sci. 376:20170176. doi: 10.1098/rsta.2017.0176
Becquevort, S., Menon, P., and Lancelot, C. (2000). Differences of the protozoan biomass and grazing during spring and summer in the Indian sector of the Southern Ocean. Polar Biol. 23, 309–320. doi: 10.1007/s003000050450
Bednarsek, N., Tarling, G. A., Bakker, D. C. E., Fielding, S., Jones, E. M., Venables, H. J., et al. (2012). Extensive dissolution of live pteropods in the Southern Ocean. Nat. Geosci. 5, 881–885. doi: 10.1038/ngeo1635
Belcher, A., Henson, S. A., Manno, C., Hill, S. L., Atkinson, A., Thorpe, S. E., et al. (2019). Krill faecal pellets drive hidden pulses of particulate organic carbon in the marginal ice zone. Nat. Commun. 10:889.
Belcher, A., Tarling, G. A., Manno, C., Atkinson, A., Ward, P., Skaret, G., et al. (2017). The potential role of Antarctic krill faecal pellets in efficient carbon export at the marginal ice zone of the South Orkney Islands in spring. Polar Biol. 40, 2001–2013. doi: 10.1007/s00300-017-2118-z
Belkin, I. M., and Gordon, A. L. (1996). Southern Ocean fronts from the Greenwich meridian to Tasmania. J. Geophys. Res. C Oceans 101, 3675–3696. doi: 10.1029/95jc02750
Bernard, K. S., Steinberg, D. K., and Schofield, O. M. E. (2012). Summertime grazing impact of the dominant macrozooplankton off the Western Antarctic Peninsula. Deep Sea Res. Part I Oceanogr. Res. Papers 62, 111–122. doi: 10.1016/j.dsr.2011.12.015
Bertrand, E. M., Mccrow, J. P., Moustafa, A., Zheng, H., Mcquaid, J. B., Delmont, T. O., et al. (2015). Phytoplankton-bacterial interactions mediate micronutrient colimitation at the coastal Antarctic sea ice edge. Proc. Natl. Acad. Sci. U.S.A. 112, 9938–9943. doi: 10.1073/pnas.1501615112
Blain, S., Queguiner, B., Armand, L., Belviso, S., Bombled, B., Bopp, L., et al. (2007). Effect of natural iron fertilization on carbon sequestration in the Southern Ocean. Nature 446, 1070–1074.
Bopp, L., Aumont, O., Cadule, P., Alvain, S., and Gehlen, M. (2005). Response of diatoms distribution to global warming and potential implications: a global model study. Geophys. Res. Lett. 32:L19606.
Bopp, L., Resplandy, L., Orr, J. C., Doney, S. C., Dunne, J. P., Gehlen, M., et al. (2013). Multiple stressors of ocean ecosystems in the 21st century: projections with CMIP5 models. Biogeosciences 10, 6225–6245. doi: 10.5194/bg-10-6225-2013
Bowie, A. R., Lannuzel, D., Remenyi, T. A., Wagener, T., Lam, P. J., Boyd, P. W., et al. (2009). Biogeochemical iron budgets of the Southern Ocean south of Australia: decoupling of iron and nutrient cycles in the subantarctic zone by the summertime supply. Glob. Biogeochem. Cycles 23:GB4034.
Bowie, A. R., Van Der Merwe, P., Quéroué, F., Trull, T., Fourquez, M., Planchon, F., et al. (2015). Iron budgets for three distinct biogeochemical sites around the Kerguelen Archipelago (Southern Ocean) during the natural fertilisation study, KEOPS-2. Biogeosciences 12, 4421–4445. doi: 10.5194/bg-12-4421-2015
Boyd, P., Laroche, J., Gall, M., Frew, R., and Mckay, R. M. L. (1999). Role of iron, light, and silicate in controlling algal biomass in subantarctic waters SE of New Zealand. J. Geophys. Res. Oceans 104, 13395–13408. doi: 10.1029/1999jc900009
Boyd, P. W. (2019). Physiology and iron modulate diverse responses of diatoms to a warming Southern Ocean. Nat. Clim. Change 9, 148–152. doi: 10.1038/s41558-018-0389-1
Boyd, P. W., Arrigo, K. R., Strzepek, R., and Van Dijken, G. L. (2012). Mapping phytoplankton iron utilization: insights into Southern Ocean supply mechanisms. J. Geophys. Res. Oceans 117:C06009.
Boyd, P. W., Dillingham, P. W., Mcgraw, C. M., Armstrong, E. A., Cornwall, C. E., Feng, Y. Y., et al. (2016). Physiological responses of a Southern Ocean diatom to complex future ocean conditions. Nat. Clim. Change 6, 207–213. doi: 10.1038/nclimate2811
Boyd, P. W., Lennartz, S. T., Glover, D. M., and Doney, S. C. (2014). Biological ramifications of climate-change-mediated oceanic multi-stressors. Nat. Clim. Change 5, 71–79. doi: 10.1038/nclimate2441
Boyd, P. W., Watson, A. J., Law, C. S., Abraham, E. R., Trull, T., Murdoch, R., et al. (2000). A mesoscale phytoplankton bloom in the polar Southern Ocean stimulated by iron fertilization. Nature 407, 695–702. doi: 10.1038/35037500
Bressac, M., Guieu, C., Ellwood, M. J., Tagliabue, A., Wagener, T., Laurenceau-Cornec, E. C., et al. (2019). Resupply of mesopelagic dissolved iron controlled by particulate iron composition. Nat. Geosci. 12, 995–1000. doi: 10.1038/s41561-019-0476-6
Britten, G. L., Wakamatsu, L., and Primeau, F. W. (2017). The temperature-ballast hypothesis explains carbon export efficiency observations in the Southern Ocean. Geophys. Res. Lett. 44, 1831–1838. doi: 10.1002/2016gl072378
Brown, M. S., Munro, D. R., Feehan, C. J., Sweeney, C., Ducklow, H. W., and Schofield, O. M. (2019). Enhanced oceanic CO2 uptake along the rapidly changing West Antarctic Peninsula. Nat. Clim. Change 9, 678–683. doi: 10.1038/s41558-019-0552-3
Brzezinski, M. A., Pride, C. J., Franck, V. M., Sigman, D. M., Sarmiento, J. L., Matsumoto, K., et al. (2002). A switch from Si(OH)(4) to NO3- depletion in the glacial Southern Ocean. Geophys. Res. Lett. 29:1564
Buck, K. N., Sedwick, P. N., Sohst, B., and Carlson, C. A. (2018). Organic complexation of iron in the eastern tropical South Pacific: results from US GEOTRACES Eastern Pacific Zonal Transect (GEOTRACES cruise GP16). Mar. Chem. 201, 229–241. doi: 10.1016/j.marchem.2017.11.007
Buesseler, K. O. (1998). The decoupling of production and particulate export in the surface ocean. Glob. Biogeochem. Cycles 12, 297–310. doi: 10.1029/97gb03366
Bundy, R. M., Boiteau, R. M., Mclean, C., Turk-Kubo, K. A., Mcilvin, M. R., Saito, M. A., et al. (2018). Distinct siderophores contribute to iron cycling in the mesopelagic at Station ALOHA. Front. Mar. Sci. 5:61. doi: 10.3389/fmars.2018.00061
Bushinsky, S. M., Landschützer, P., Rödenbeck, C., Gray, A. R., Baker, D., Mazloff, M. R., et al. (2019). Reassessing Southern ocean air−sea CO2 flux estimates with the addition of biogeochemical float observations. Glob. Biogeochem. Cycles 33, 1370–1388. doi: 10.1029/2019gb006176
Byrne, M., Ho, M. A., Koleits, L., Price, C., King, C. K., Virtue, P., et al. (2013). Vulnerability of the calcifying larval stage of the Antarctic sea urchin Sterechinus neumayeri to near-future ocean acidification and warming. Glob. Change Biol. 19, 2264–2275. doi: 10.1111/gcb.12190
Cabré, A., Marinov, I., and Leung, S. (2015). Consistent global responses of marine ecosystems to future climate change across the IPCC AR5 earth system models. Clim. Dyn. 45, 1253–1280. doi: 10.1007/s00382-014-2374-3
Calbet, A., and Landry, M. R. (2004). Phytoplankton growth, microzooplankton grazing, and carbon cycling in marine systems. Limnol. Oceanogr. 49, 51–57. doi: 10.4319/lo.2004.49.1.0051
Carvalho, F., Fitzsimmons, J. N., Couto, N., Waite, N., Gorbunov, M., Kohut, J., et al. (2019). Testing the canyon hypothesis: evaluating light and nutrient controls of phytoplankton growth in penguin foraging hotspots along the West Antarctic Peninsula. Limnol. Oceanogr. 65, 455–470. doi: 10.1002/lno.11313
Catarino, A. I., De Ridder, C., Gonzalez, M., Gallardo, P., and Dubois, P. (2012). Sea urchin Arbacia dufresnei (Blainville 1825) larvae response to ocean acidification. Polar Biol. 35, 455–461. doi: 10.1007/s00300-011-1074-2
Cavan, E. L., Belcher, A., Atkinson, A., Hill, S. L., Kawaguchi, S., Mccormack, S., et al. (2019a). The importance of Antarctic krill in biogeochemical cycles. Nat. Commun. 10:4742.
Cavan, E. L., Laurenceau-Cornec, E. C., Bressac, M., and Boyd, P. W. (2019b). Exploring the ecology of the mesopelagic biological pump. Prog. Oceanogr. 176, 102125–102125.
Cavan, E. L., Le Moigne, F. A. C., Poulton, A. J., Tarling, G. A., Ward, P., Daniels, C. J., et al. (2015). Attenuation of particulate organic carbon flux in the Scotia Sea, Southern Ocean, is controlled by zooplankton fecal pellets. Geophys. Res. Lett. 42, 821–830. doi: 10.1002/2014gl062744
Chapman, C. C., Lea, M.-A., Meyer, A., Sallée, J.-B., and Hindell, M. (2020). Defining Southern Ocean fronts and their influence on biological and physical processes in a changing climate. Nat. Clim. Change 10, 209–219. doi: 10.1038/s41558-020-0705-4
Constable, A. J., Melbourne-Thomas, J., Corney, S. P., Arrigo, K. R., Barbraud, C., Barnes, D. K., et al. (2014). Climate change and Southern Ocean ecosystems I: how changes in physical habitats directly affect marine biota. Glob. Change Biol. 20, 3004–3025.
Cook, A. J., Holland, P. R., Meredith, M. P., Murray, T., Luckman, A., and Vaughan, D. G. (2016). Ocean forcing of glacier retreat in the western Antarctic Peninsula. Science 353, 283–286. doi: 10.1126/science.aae0017
Cooley, S. R., Kite-Powell, H. L., and Doney, S. C. (2009). Ocean acidification’s potential to alter global marine ecosystem services. Oceanography 22, 172–181. doi: 10.5670/oceanog.2009.106
Costa, R. R., Rafael, C., Mendes, C. R. B., Tavano, V. M., Dotto, T. D., Kerr, R., et al. (2020). Dynamics of an intense diatom bloom in the Northern Antarctic Peninsula, February 2016. Limnol. Oceanogr. 66, 1–20.
Cox, M. J., Candy, S., De La Mare, W. K., Nicol, S., Kawaguchi, S., and Gales, N. (2018). No evidence for a decline in the density of Antarctic krill Euphausia superba Dana, 1850, in the Southwest Atlantic sector between 1976 and 2016. J. Crustacean Biol. 38, 656–661.
Cross, E. L., Peck, L. S., and Harper, E. M. (2015). Ocean acidification does not impact shell growth or repair of the Antarctic brachiopod Liothyrella uva (Broderip, 1833). J. Exp. Mar. Biol. Ecol. 462, 29–35. doi: 10.1016/j.jembe.2014.10.013
Dale, A. W., Nickelsen, L., Scholz, F., Hensen, C., Oschlies, A., and Wallmann, K. (2015). A revised global estimate of dissolved iron fluxes from marine sediments. Glob. Biogeochem. Cycles 29, 691–707. doi: 10.1002/2014gb005017
de Baar, H. J. W., Buma, A. G. J., Nolting, R. F., Cadée, G. C., Jacques, G., and Tréguer, P. J. (1990). On iron limitation of the Southern Ocean: experimental observations in the Weddell and Scotia Seas. Mar. Ecol. Prog. Ser. 65, 105–122. doi: 10.3354/meps065105
de Baar, H. J. W., De Jong, J. T. M., Bakker, D. C. E., Löscher, B. M., Veth, C., Bathmann, U., et al. (1995). Importance of iron for plankton blooms and carbon dioxide drawdown in the Southern Ocean. Nature 373, 412–415. doi: 10.1038/373412a0
De Jong, J., Schoemann, V., Lannuzel, D., Croot, P., De Baar, H., and Tison, J.-L. (2012). Natural iron fertilization of the Atlantic sector of the Southern Ocean by continental shelf sources of the Antarctic Peninsula. J. Geophys. Res. Biogeosci. 117:G01029.
De Jong, J. T. M., Stammerjohn, S. E., Ackley, S. F., Tison, J. L., Mattielli, N., and Schoemann, V. (2015). Sources and fluxes of dissolved iron in the Bellingshausen Sea (West Antarctica): the importance of sea ice, icebergs and the continental margin. Mar. Chem. 177, 518–535. doi: 10.1016/j.marchem.2015.08.004
Deacon, G. E. R. (1982). Physical and biological zonation in the Southern Ocean. Deep Sea Res. Part A Oceanogr. Res. Papers 29, 1–15. doi: 10.1016/0198-0149(82)90058-9
Dejong, H. B., and Dunbar, R. B. (2017). Air-Sea CO2 Exchange in the Ross Sea, Antarctica. J. Geophys. Res. Oceans 122, 8167–8181. doi: 10.1002/2017jc012853
Del Castillo, C. E., Signorini, S. R., Karaköylü, E. M., and Rivero−Calle, S. (2019). Is the Southern ocean getting greener? Geophys. Res. Lett. 46, 6034–6040. doi: 10.1029/2019gl083163
Denman, K. L. (2017). A model simulation of the adaptive evolution through mutation of the coccolithophore Emiliania huxleyi based on a published laboratory study. Front. Mar. Sci. 3:286. doi: 10.3389/fmars.2016.00286
Dennett, M. R., Mathot, S., Caron, D. A., Smith, W. O., and Lonsdale, D. J. (2001). Abundance and distribution of phototrophic and heterotrophic nano- and microplankton in the southern Ross Sea. Deep Sea Res. Part II Top. Stud. Oceanogr. 48, 4019–4037. doi: 10.1016/s0967-0645(01)00079-0
Deppeler, S., Petrou, K., Schulz, K. G., Westwood, K., Pearce, I., Mckinlay, J., et al. (2018). Ocean acidification of a coastal Antarctic marine microbial community reveals a critical threshold for CO2 tolerance in phytoplankton productivity. Biogeosciences 15, 209–231. doi: 10.5194/bg-15-209-2018
Deppeler, S. L., and Davidson, A. T. (2017). Southern ocean phytoplankton in a changing climate. Front. Mar. Sci. 4:40. doi: 10.3389/fmars.2017.00040
DeVries, T. (2014). The oceanic anthropogenic CO2 sink: storage, air-sea fluxes, and transports over the industrial era. Glob. Biogeochem. Cycles 28, 631–647. doi: 10.1002/2013gb004739
DeVries, T., Holzer, M., and Primeau, F. (2017). Recent increase in oceanic carbon uptake driven by weaker upper-ocean overturning. Nature 542, 215–218. doi: 10.1038/nature21068
DeVries, T., Primeau, F., and Deutsch, C. (2012). The sequestration efficiency of the biological pump. Geophys. Res. Lett. 39:L13601
Dickman, E. M., Newell, J. M., González, M. J., and Vanni, M. J. (2008). Light, nutrients, and food-chain length constrain planktonic energy transfer efficiency across multiple trophic levels. Proc. Natl. Acad. Sci. U.S.A. 105, 18408–18412. doi: 10.1073/pnas.0805566105
Dinniman, M. S., St-Laurent, P., Arrigo, K. R., Hofmann, E. E., and Van Dijken, G. L. (2020). Analysis of iron sources in Antarctic continental shelf waters. J. Geophys. Res. Oceans 125:e2019JC015736.
Doney, S. C., Fabry, V. J., Feely, R. A., and Kleypas, J. A. (2009). Ocean acidification: the other CO2 problem. Annu. Rev. Mar. Sci. 1, 169–192.
Dubischar, C. D., Pakhomov, E. A., Von Harbou, L., Hunt, B. P. V., and Bathmann, U. V. (2012). Salps in the Lazarev Sea, Southern ocean: II. Biochemical composition and potential prey value. Mar. Biol. 159, 15–24. doi: 10.1007/s00227-011-1785-5
Ducklow, H. W., Baker, K., Martinson, D. G., Quetin, L. B., Ross, R. M., Smith, R. C., et al. (2007). Marine pelagic ecosystems: the West Antarctic Peninsula. Philos. Trans. R. Soc. B Biol. Sci. 362, 67–94. doi: 10.1098/rstb.2006.1955
Ducklow, H. W., Steinberg, D. K., and Buesseler, K. O. (2001). Upper ocean carbon export and the biological pump. Oceanography 14, 50–58. doi: 10.5670/oceanog.2001.06
Duprat, L., Kanna, N., Janssens, J., Roukaerts, A., Deman, F., Townsend, A. T., et al. (2019). Enhanced iron flux to antarctic sea ice via dust deposition from ice−free coastal areas. J. Geophys. Res. Oceans 124, 8538–8557. doi: 10.1029/2019jc015221
Ellwood, M. J., Nodder, S. D., King, A. L., Hutchins, D. A., Wilhelm, S. W., and Boyd, P. W. (2014). Pelagic iron cycling during the subtropical spring bloom, east of New Zealand. Mar. Chem. 160, 18–33.
Ellwood, M. J., Strzepek, R. F., Strutton, P. G., Trull, T. W., Fourquez, M., and Boyd, P. W. (2020). Distinct iron cycling in a Southern Ocean eddy. Nat. Commun. 11:825.
Ericson, J. A., Hellessey, N., Kawaguchi, S., Nicol, S., Nichols, P. D., Hoem, N., et al. (2018). Adult Antarctic krill proves resilient in a simulated high CO2 ocean. Commun. Biol. 1:190.
Eriksen, R., Trull, T. W., Davies, D., Jansen, P., Davidson, A. T., Westwood, K., et al. (2018). Seasonal succession of phytoplankton community structure from autonomous sampling at the Australian Southern ocean time series (SOTS) observatory. Mar. Ecol. Prog. Ser. 589, 13–31. doi: 10.3354/meps12420
Evans, C., and Brussaard, C. P. D. (2012). Regional variation in lytic and lysogenic viral infection in the Southern Ocean and its contribution to biogeochemical cycling. Appl. Environ. Microbiol. 78, 6741–6748. doi: 10.1128/aem.01388-12
Evans, C., Pearce, I., and Brussaard, C. P. D. (2009). Viral-mediated lysis of microbes and carbon release in the sub-Antarctic and Polar Frontal zones of the Australian Southern Ocean. Environ. Microbiol. 11, 2924–2934. doi: 10.1111/j.1462-2920.2009.02050.x
Everson, I. (2000). “Roles of krill in marine food webs: the Southern Ocean,” in Krill: Biology, Ecology and Fisheries, ed. I. Everson, (Oxford, UK: Blackwell Science), 194–201.
Fabry, V. J., Seibel, B. A., Feely, R. A., and Orr, J. C. (2008). Impacts of ocean acidification on marine fauna and ecosystem processes. ICES J. Mar. Sci. 65, 414–432. doi: 10.1093/icesjms/fsn048
Fay, A. R., Mckinley, G. A., and Lovenduski, N. S. (2014). Southern ocean carbon trends: sensitivity to methods. Geophys. Res. Lett. 41, 6833–6840. doi: 10.1002/2014gl061324
Feely, R. A., Doney, S. C., and Cooley, S. R. (2009). Ocean acidification: present conditions and future changes in a high-CO2 world. Oceanography 22, 36–47. doi: 10.5670/oceanog.2009.95
Feng, Y., Hare, C. E., Rose, J. M., Handy, S. M., Ditullio, G. R., Lee, P. A., et al. (2010). Interactive effects of iron, irradiance and CO2 on Ross Sea phytoplankton. Deep Sea Res. Part I Oceanogr. Res. Papers 57, 368–383. doi: 10.1016/j.dsr.2009.10.013
Finkel, Z. V., Quigg, A., Raven, J. A., Reinfelder, J. R., Schofield, O. E., and Falkowski, P. G. (2006). Irradiance and the elemental stoichiometry of marine phytoplankton. Limnol. Oceanogr. 51, 2690–2701. doi: 10.4319/lo.2006.51.6.2690
Fitzsimmons, J. N., Carrasco, G. G., Wu, J., Roshan, S., Hatta, M., Measures, C. I., et al. (2015). Partitioning of dissolved iron and iron isotopes into soluble and colloidal phases along the GA03 GEOTRACES North Atlantic Transect. Deep Sea Res. Part II Top. Stud. Oceanogr. 116, 130–151. doi: 10.1016/j.dsr2.2014.11.014
Fletcher, S. E. M., Gruber, N., Jacobson, A. R., Doney, S. C., Dutkiewicz, S., Gerber, M., et al. (2006). Inverse estimates of anthropogenic CO2 uptake, transport, and storage by the ocean. Glob. Biogeochem. Cycles 20:GB2002.
Franck, V. M., Brzezinski, M. A., Coale, K. H., and Nelson, D. M. (2000). Iron and silicic acid concentrations regulate Si uptake north and south of the polar frontal zone in the pacific sector of the Southern ocean. Deep Sea Res. Part II Top. Stud. Oceanogr. 47, 3315–3338. doi: 10.1016/s0967-0645(00)00070-9
Freeman, N. M., Lovenduski, N. S., Munro, D. R., Krumhardt, K. M., Lindsay, K., Long, M. C., et al. (2018). The variable and changing southern ocean silicate front: insights from the CESM large ensemble. Glob. Biogeochem. Cycles 32, 752–768.
Frenger, I., Münnich, M., Gruber, N., and Knutti, R. (2015). Southern ocean eddy phenomenology. J. Geophys. Res. Oceans 120, 7413–7449.
Fu, W. W., Randerson, J. T., and Moore, J. K. (2016). Climate change impacts on net primary production (NPP) and export production (EP) regulated by increasing stratification and phytoplankton community structure in the CMIP5 models. Biogeosciences 13, 5151–5170. doi: 10.5194/bg-13-5151-2016
Gabric, A. J., Cropp, R. A., Mctainsh, G. H., Johnston, B. M., Butler, H., Tilbrook, B., et al. (2010). Australian dust storms in 2002–2003 and their impact on Southern Ocean biogeochemistry. Global Biogeochem. Cy. 24. doi: 10.1029/2009GB003541
Gall, M. P., Boyd, P. W., Hall, J., Safi, K. A., and Chang, H. (2001). Phytoplankton processes. Part 1: community structure during the Southern Ocean Iron RElease Experiment (SOIREE). Deep Sea Res. Part II Top. Stud. Oceanogr. 48, 2551–2570. doi: 10.1016/s0967-0645(01)00008-x
Gao, Y., Yu, S., Sherrell, R. M., Fan, S., Bu, K., and Anderson, J. R. (2020). Particle-size distributions and solubility of aerosol iron over the Antarctic Peninsula during austral summer. J. Geophys. Res. Atmospheres 125:e2019JD032082.
Gardner, J., Manno, C., Bakker, D. C. E., Peck, V. L., and Tarling, G. A. (2018). Southern Ocean pteropods at risk from ocean warming and acidification. Mar. Biol. 165:8.
Garzio, L. M., Steinberg, D. K., Erickson, M., and Ducklow, H. W. (2013). Microzooplankton grazing along the Western Antarctic Peninsula. Aqua. Microb. Ecol. 70, 215–232. doi: 10.3354/ame01655
Gerringa, L. J. A., Alderkamp, A. C., Laan, P., Thuroczy, C. E., De Baar, H. J. W., Mills, M. M., et al. (2012). Iron from melting glaciers fuels the phytoplankton blooms in Amundsen Sea (Southern Ocean): iron biogeochemistry. Deep Sea Res. Part II Top. Stud. Oceanogr. 7, 16–31. doi: 10.1016/j.dsr2.2012.03.007
Gillies, C. L., Stark, J. S., Johnstone, G. J., and Smith, S. D. A. (2013). Establishing a food web model for coastal Antarctic benthic communities: a case study from the Vestfold Hills. Mar. Ecol. Prog. Ser. 478, 27–41. doi: 10.3354/meps10214
Gleiber, M. R. (2015). Long-term Change in Copepod Community Structure in the Western Antarctic Peninsula: Linkage to Climate and Implications for Carbon Cycling. Ph.D. thesis, Virginia Institute of Marine Science, Gloucester Point, VA.
Goto-Azuma, K., Hirabayashi, M., Motoyama, H., Miyake, T., Kuramoto, T., Uemura, R., et al. (2019). Reduced marine phytoplankton sulphur emissions in the Southern Ocean during the past seven glacials. Nat. Commun. 10:3247.
Green, S. E., and Sambrotto, R. N. (2006). Plankton community structure and export of C, N, P and Si in the Antarctic circumpolar current. Deep Sea Res. Part II Top. Stud. Oceanogr. 53, 620–643. doi: 10.1016/j.dsr2.2006.01.022
Gregor, L., Kok, S., and Monteiro, P. M. S. (2018). Interannual drivers of the seasonal cycle of CO2 in the Southern ocean. Biogeosciences 15, 2361–2378. doi: 10.5194/bg-15-2361-2018
Gruber, N., Clement, D., Carter, B. R., Feely, R. A., Van Heuven, S., Hoppema, M., et al. (2019a). The oceanic sink for anthropogenic CO2 from 1994 to 2007. Science 363, 1193–1199.
Gruber, N., Gloor, M., Fletcher, S. E. M., Doney, S. C., Dutkiewicz, S., Follows, M. J., et al. (2009). Oceanic sources, sinks, and transport of atmospheric CO2. Glob. Biogeochem. Cycles 23:GB1005.
Gruber, N., Landschützer, P., and Lovenduski, N. S. (2019b). The variable Southern ocean carbon sink. Annu. Rev. Mar. Sci. 11, 159–186. doi: 10.1146/annurev-marine-121916-063407
Gruber, N., and Sarmiento, J. L. (1997). Global patterns of marine nitrogen fixation and denitrification. Glob. Biogeochem. Cycles 11, 235–266. doi: 10.1029/97gb00077
Guinotte, J. M., and Fabry, V. J. (2008). Ocean acidification and its potential effects on marine ecosystems. Ann. N. Y. Acad. Sci. 1134, 320–342. doi: 10.1196/annals.1439.013
Gutt, J., Bertler, N., Bracegirdle, T. J., Buschmann, A., Comiso, J., Hosie, G., et al. (2015). The Southern ocean ecosystem under multiple climate change stresses – an integrated circumpolar assessment. Glob. Change Biol. 21, 1434–1453. doi: 10.1111/gcb.12794
Haberman, K. L., Quetin, L. B., and Ross, R. M. (2003). Diet of the Antarctic krill (Euphausia superba Dana): I. comparisons of grazing on Phaeocystis antarctica (Karsten) and Thalassiosira antarctica (Comber). J. Exp. Mar. Biol. Ecol. 283, 79–95.
Hancock, A. M., Davidson, A. T., Mckinlay, J., Mcminn, A., Schulz, K. G., and Van Den Enden, R. L. (2018). Ocean acidification changes the structure of an Antarctic coastal protistan community. Biogeosciences 15, 2393–2410. doi: 10.5194/bg-15-2393-2018
Hatta, M., Measures, C. I., Selph, K. E., Zhou, M., and Hiscock, W. T. (2013). Iron fluxes from the shelf regions near the South Shetland islands in the drake passage during the austral-winter 2006. Deep Sea Res. Part II Top. Stud. Oceanogr. 90, 89–101. doi: 10.1016/j.dsr2.2012.11.003
Hauck, J., Hoppema, M., Bellerby, R. G. J., Volker, C., and Wolf-Gladrow, D. (2010). Data-based estimation of anthropogenic carbon and acidification in the Weddell Sea on a decadal timescale. J. Geophys. Res. Oceans 115:C03004
Hauck, J., and Völker, C. (2015). Rising atmospheric CO2 leads to large impact of biology on Southern ocean CO2 uptake via changes of the Revelle factor. Geophys. Res. Lett. 42, 1459–1464. doi: 10.1002/2015gl063070
Hauck, J., Völker, C., Wolf-Gladrow, D. A., Laufkötter, C., Vogt, M., Aumont, O., et al. (2015). On the Southern Ocean CO2 uptake and the role of the biological carbon pump in the 21st century. Glob. Biogeochem. Cycles 29, 1451–1470. doi: 10.1002/2015gb005140
Hauri, C., Friedrich, T., and Timmermann, A. (2016). Abrupt onset and prolongation of aragonite undersaturation events in the Southern ocean. Nat. Clim. Change 6, 172–176. doi: 10.1038/nclimate2844
Henley, S. F., Jones, E. M., Venables, H. J., Meredith, M. P., Firing, Y. L., Dittrich, R., et al. (2018). Macronutrient and carbon supply, uptake and cycling across the Antarctic Peninsula shelf during summer. Philos. Trans. R. Soc. A Math. Phys. Eng. Sci. 376:20170168. doi: 10.1098/rsta.2017.0168
Henley, S. F., Schofield, O. M., Hendry, K. R., Schloss, I. R., Steinberg, D. K., Moffat, C., et al. (2019). Variability and change in the west Antarctic Peninsula marine system: research priorities and opportunities. Prog. Oceanogr. 173, 208–237.
Henley, S. F., Tuerena, R. E., Annett, A. L., Fallick, A. E., Meredith, M. P., Venables, H. J., et al. (2017). Macronutrient supply, uptake and recycling in the coastal ocean of the west Antarctic Peninsula. Deep Sea Res. Part II Top. Stud. Oceanogr. 139, 58–76. doi: 10.1016/j.dsr2.2016.10.003
Henley, S. F., and Venables, H. J. (2019). Water Column Nutrient, Particulate Organic Matter and Isotopic Data for Ryder Bay, West Antarctic Peninsula 2013-2016. Liverpool: British Oceanographic Data Centre, National Oceanography Centre. doi: 10.5285/98cc0722-e337-029c-e053-6c86abc02029
Hernández-León, S., Portillo-Hahnefeld, A., Almeida, C., Bécognée, P., and Moreno, I. (2001). Diel feeding behaviour of krill in the Gerlache Strait, Antarctica. Mar. Ecol. Prog. Ser. 223, 235–242. doi: 10.3354/meps223235
Hernando, M., Schloss, I. R., Malanga, G., Almandoz, G. O., Ferreyra, G. A., Aguiar, M. B., et al. (2015). Effects of salinity changes on coastal Antarctic phytoplankton physiology and assemblage composition. J. Exp. Mar. Biol. Ecol. 466, 110–119. doi: 10.1016/j.jembe.2015.02.012
Hernando, M. P., Schloss, I. R., Almandoz, G. O., Malanga, G., Varela, D., and De Troch, M. (2018). Combined effects of temperature and salinity on fatty acid content and lipid damage in Antarctic phytoplankton. J. Exp. Mar. Biol. Ecol. 503, 120–128. doi: 10.1016/j.jembe.2018.03.004
Herraiz-Borreguero, L., Lannuzel, D., Van Der Merwe, P., Treverrow, A., and Pedro, J. B. (2016). Large flux of iron from the Amery Ice Shelf marine ice to Prydz Bay, East Antarctica. J. Geophys. Res. Oceans 121, 6009–6020. doi: 10.1002/2016jc011687
Hill, S. L., Atkinson, A., Pakhomov, E. A., and Siegel, V. (2019). Evidence for a decline in the population density of Antarctic krill Euphausia superba Dana, 1850 still stands. A comment on Cox et al. J. Crustacean Biol. 39, 316–322. doi: 10.1093/jcbiol/ruz004
Hill, S. L., Keeble, K., Atkinson, A., and Murphy, E. J. (2012). A foodweb model to explore uncertainties in the South Georgia shelf pelagic ecosystem. Deep Sea Res. Part II Top. Stud. Oceanogr. 59, 237–252. doi: 10.1016/j.dsr2.2011.09.001
Hoppe, C. J. M., Klaas, C., Ossebaar, S., Soppa, M. A., Cheah, W., Laglera, L. M., et al. (2017). Controls of primary production in two phytoplankton blooms in the Antarctic Circumpolar current. Deep Sea Res. Part II Top. Stud. Oceanogr. 138, 63–73. doi: 10.1016/j.dsr2.2015.10.005
Hopwood, M. J., Cantoni, C., Clarke, J. S., Cozzi, S., and Achterberg, E. P. (2017). The heterogeneous nature of Fe delivery from melting icebergs. Geochem. Perspect. Lett. 200–209. doi: 10.7185/geochemlet.1723
Houghton, I. A., Koseff, J. R., Monismith, S. G., and Dabiri, J. O. (2018). Vertically migrating swimmers generate aggregation-scale eddies in a stratified column. Nature 556, 497–500. doi: 10.1038/s41586-018-0044-z
Hutchins, D. A., and Boyd, P. W. (2016). Marine phytoplankton and the changing ocean iron cycle. Nat. Clim. Change 6, 1072–1079. doi: 10.1038/nclimate3147
Hutchins, D. A., and Bruland, K. W. (1998). Iron-limited diatom growth and Si : N uptake ratios in a coastal upwelling regime. Nature 393, 561–564. doi: 10.1038/31203
Ito, R. G., Tavano, V. M., Mendes, C. R. B., and Garcia, C. A. E. (2018). Sea-air CO2 fluxes and pCO2 variability in the Northern Antarctic Peninsula during three summer periods (2008–2010). Deep Sea Res. Part II Top. Stud. Oceanogr. 149, 84–98. doi: 10.1016/j.dsr2.2017.09.004
Iversen, M. H., and Poulsen, L. K. (2007). Coprorhexy, coprophagy, and coprochaly in the copepods Calanus helgolandicus, Pseudocalanus elongatus, and Oithona similis. Mar. Ecol. Prog. Ser. 350, 79–89. doi: 10.3354/meps07095
Jiao, N., Herndl, G. J., Hansell, D. A., Benner, R., Kattner, G., Wilhelm, S. W., et al. (2010). Microbial production of recalcitrant dissolved organic matter: long-term carbon storage in the global ocean. Nat. Rev. Microbiol. 8, 593–599. doi: 10.1038/nrmicro2386
Jiao, N., Herndl, G. J., Hansell, D. A., Benner, R., Kattner, G., Wilhelm, S. W., et al. (2011). The microbial carbon pump and the oceanic recalcitrant dissolved organic matter pool. Nat. Rev. Microbiol. 9:555. doi: 10.1038/nrmicro2386-c5
Johnson, K. S., Plant, J. N., Dunne, J. P., Talley, L. D., and Sarmiento, J. L. (2017). Annual nitrate drawdown observed by SOCCOM profiling floats and the relationship to annual net community production. J. Geophys. Res. Oceans 122, 6668–6683. doi: 10.1002/2017jc012839
Jónasdóttir, S. H., Visser, A. W., Richardson, K., and Heath, M. R. (2015). Seasonal copepod lipid pump promotes carbon sequestration in the deep North Atlantic. Proc. Natl. Acad. Sci. U.S.A. 112, 12122–12126. doi: 10.1073/pnas.1512110112
Jones, E. M., Bakker, D. C. E., Venables, H. J., and Watson, A. J. (2012). Dynamic seasonal cycling of inorganic carbon downstream of South Georgia, Southern Ocean. Deep Sea Res. Part II Top. Stud. Oceanogr. 5, 25–35. doi: 10.1016/j.dsr2.2011.08.001
Jones, E. M., Fenton, M., Meredith, M. P., Clargo, N. M., Ossebaar, S., Ducklow, H. W., et al. (2017). Ocean acidification and calcium carbonate saturation states in the coastal zone of the West Antarctic Peninsula. Deep Sea Res. Part II Top. Stud. Oceanogr. 139, 181–194. doi: 10.1016/j.dsr2.2017.01.007
Kapsenberg, L., and Hofmann, G. E. (2014). Signals of resilience to ocean change: high thermal tolerance of early stage Antarctic sea urchins (Sterechinus neumayeri) reared under present-day and future pCO(2) and temperature. Polar Biol. 37, 967–980. doi: 10.1007/s00300-014-1494-x
Katija, K., and Dabiri, J. O. (2009). A viscosity-enhanced mechanism for biogenic ocean mixing. Nature 460, 624–626. doi: 10.1038/nature08207
Kawaguchi, S., Ishida, A., King, R., Raymond, B., Waller, N., Constable, A., et al. (2013). Risk maps for Antarctic krill under projected Southern Ocean acidification. Nat. Clim. Change 3, 843–847. doi: 10.1038/nclimate1937
Kerr, R., Goyet, C., Da Cunha, L. C., Orselli, I. B. M., Lencina-Avila, J. M., Mendes, C. R. B., et al. (2018). Carbonate system properties in the Gerlache Strait, Northern Antarctic Peninsula (February 2015): II. Anthropogenic CO2 and seawater acidification. Deep Sea Res. Part II Top. Stud. Oceanogr. 149, 182–192. doi: 10.1016/j.dsr2.2017.07.007
Kettle, A. J., Andreae, M. O., Amouroux, D., Andreae, T. W., Bates, T. S., Berresheim, H., et al. (1999). A global database of sea surface dimethylsulfide (DMS) measurements and a procedure to predict sea surface DMS as a function of latitude, longitude, and month. Glob. Biogeochem. Cycles 13, 399–444. doi: 10.1029/1999gb900004
Kim, H., Ducklow, H. W., Abele, D., Barlett, E. M. R., Buma, A. G. J., Meredith, M. P., et al. (2018). Inter-decadal variability of phytoplankton biomass along the coastal West Antarctic Peninsula. Philos. Trans. R. Soc. A Math. Phys. Eng. Sci. 376:20170174. doi: 10.1098/rsta.2017.0174
Klaas, C. (1997). Microprotozooplankton distribution and their potential grazing impact in the antarctic circumpolar current. Deep Sea Res. Part II Top. Stud. Oceanogr. 44, 375–393. doi: 10.1016/s0967-0645(96)00080-x
Koch, F., Beszteri, S., Harms, L., and Trimborn, S. (2019). The impacts of iron limitation and ocean acidification on the cellular stoichiometry, photophysiology, and transcriptome of Phaeocystis antarctica. Limnol. Oceanogr. 64, 357–375. doi: 10.1002/lno.11045
Koch, F., and Trimborn, S. (2019). Limitation by Fe, Zn, Co, and B12 results in similar physiological responses in two antarctic phytoplankton species. Front. Mar. Sci. 6:514. doi: 10.3389/fmars.2019.00514
Laglera, L. M., Tovar-Sánchez, A., Iversen, M. H., González, H. E., Naik, H., Mangesh, G., et al. (2017). Iron partitioning during LOHAFEX: copepod grazing as a major driver for iron recycling in the southern ocean. Mar. Chem. 196, 148–161. doi: 10.1016/j.marchem.2017.08.011
Landschutzer, P., Gruber, N., Haumann, A., Rodenbeck, C., Bakker, D. C. E., Van Heuven, S., et al. (2015). The reinvigoration of the southern ocean carbon sink. Science 349, 1221–1224. doi: 10.1126/science.aab2620
Lannuzel, D., Vancoppenolle, M., Van Der Merwe, P., De Jong, J., Meiners, K. M., Grotti, M., et al. (2016). Iron in sea ice: review and new insights. Elemen. Sci. Anthropocene 4:130.
Laufkötter, C., Stern, A. A., John, J. G., Stock, C. A., and Dunne, J. P. (2018). Glacial iron sources stimulate the southern ocean carbon cycle. Geophys. Res. Lett. 13:385.
Laufkötter, C., Vogt, M., and Gruber, N. (2013). Long-term trends in ocean plankton production and particle export between 1960–2006. Biogeosciences 10, 7373–7393. doi: 10.5194/bg-10-7373-2013
Laufkötter, C., Vogt, M., Gruber, N., Aita-Noguchi, M., Aumont, O., Bopp, L., et al. (2015). Drivers and uncertainties of future global marine primary production in marine ecosystem models. Biogeosciences 12, 6955–6984. doi: 10.5194/bg-12-6955-2015
Laufkötter, C., Vogt, M., Gruber, N., Aumont, O., Bopp, L., Doney, S. C., et al. (2016). Projected decreases in future marine export production: the role of the carbon flux through the upper ocean ecosystem. Biogeosciences 13, 4023–4047. doi: 10.5194/bg-13-4023-2016
Laurenceau-Cornec, E. C., Trull, T. W., Davies, D. M., Bray, S. G., Doran, J., Planchon, F., et al. (2015). The relative importance of phytoplankton aggregates and zooplankton fecal pellets to carbon export: insights from free-drifting sediment trap deployments in naturally iron-fertilised waters near the Kerguelen Plateau. Biogeosciences 12, 1007–1027. doi: 10.5194/bg-12-1007-2015
Lavery, T. J., Roudnew, B., Gill, P., Seymour, J., Seuront, L., Johnson, G., et al. (2010). Iron defecation by sperm whales stimulates carbon export in the southern ocean. Proc. R. Soc. B Biol. Sci. 277, 3527–3531. doi: 10.1098/rspb.2010.0863
Lavery, T. J., Roudnew, B., Seymour, J., Mitchell, J. G., Smetacek, V., and Nicol, S. (2014). Whales sustain fisheries: blue whales stimulate primary production in the southern ocean. Mar. Mammal Sci. 30, 888–904. doi: 10.1111/mms.12108
Laws, R. M. (1977). Seals and whales of the southern ocean. Philos. Trans. R. Soc. B 279, 81–96. doi: 10.1098/rstb.1977.0073
Le Quere, C., Rodenbeck, C., Buitenhuis, E. T., Conway, T. J., Langenfelds, R., Gomez, A., et al. (2007). Saturation of the southern ocean CO2 sink due to recent climate change. Science 316, 1735–1738. doi: 10.1126/science.1136188
Lee, R. F., and Hagen, W. (2006). Lipid storage in marine zooplankton. Mar. Ecol. Prog. Ser. 307, 273–306. doi: 10.3354/meps307273
Lencina-Avila, J. M., Goyet, C., Kerr, R., Orselli, I. B. M., Mata, M. M., and Touratier, F. (2018). Past and future evolution of the marine carbonate system in a coastal zone of the Northern Antarctic Peninsula. Deep Sea Res. Part II Top. Stud. Oceanogr. 149, 193–205. doi: 10.1016/j.dsr2.2017.10.018
Lenton, A., and Matear, R. J. (2007). Role of the southern annular mode (SAM) in southern ocean CO2 uptake. Glob. Biogeochem. Cycles 21, 1–17.
Lenton, A., Tilbrook, B., Law, R. M., Bakker, D., Doney, S. C., Gruber, N., et al. (2013). Sea-air CO2 fluxes in the Southern Ocean for the period 1990-2009. Biogeosciences 10, 4037–4054.
Leung, S., Cabre, A., and Marinov, I. (2015). A latitudinally banded phytoplankton response to 21st century climate change in the southern ocean across the CMIP5 model suite. Biogeosciences 12, 5715–5734. doi: 10.5194/bg-12-5715-2015
Lin, H., Rauschenberg, S., Hexel, C. R., Shaw, T. J., and Twining, B. S. (2011). Free-drifting icebergs as sources of iron to the Weddell Sea. Deep Sea Res. Part II Top. Stud. Oceanogr. 58, 1392–1406. doi: 10.1016/j.dsr2.2010.11.020
Lourey, M. J., Trull, T. W., and Sigman, D. M. (2003). Sensitivity of delta N-15 of nitrate, surface suspended and deep sinking particulate nitrogen to seasonal nitrate depletion in the southern ocean. Glob. Biogeochem. Cycles 17:GB1081.
Lovenduski, N. S., Fay, A. R., and Mckinley, G. A. (2015). Observing multidecadal trends in southern ocean CO2 uptake: what can we learn from an ocean model? Glob. Geochem. Cycles 29, 416–426. doi: 10.1002/2014gb004933
Lovenduski, N. S., Gruber, N., and Doney, S. C. (2008). Toward a mechanistic understanding of the decadal trends in the southern ocean carbon sink. Glob. Biogeochem. Cycles 22:GB3016.
Lovenduski, N. S., Long, M. C., Gent, P. R., and Lindsay, K. (2013). Multi-decadal trends in the advection and mixing of natural carbon in the southern ocean. Geophys. Res. Lett. 40, 139–142. doi: 10.1029/2012gl054483
Maas, E. W., Law, C. S., Hall, J. A., Pickmere, S., Currie, K. I., Chang, F. H., et al. (2013). Effect of ocean acidification on bacterial abundance, activity and diversity in the Ross Sea, Antarctica. Aqua. Microb. Ecol. 70, 1–15. doi: 10.3354/ame01633
Mangoni, O., Saggiomo, V., Bolinesi, F., Margiotta, F., Budillon, G., Cotroneo, Y., et al. (2017). Phytoplankton blooms during austral summer in the Ross Sea, Antarctica: Driving factors and trophic implications. PLoS One 12:e0176033. doi: 10.1371/journal.pone.0176033
Marinov, I., Gnanadesikan, A., Toggweiler, J. R., and Sarmiento, J. L. (2006). The southern ocean biogeochemical divide. Nature 441, 964–967. doi: 10.1038/nature04883
Marsay, C. M., Sedwick, P. N., Dinniman, M. S., Barrett, P. M., Mack, S. L., and Mcgillicuddy, D. J. Jr. (2014). Estimating the benthic efflux of dissolved iron on the Ross Sea continental shelf. Geophys. Res. Lett. 41, 7576–7583. doi: 10.1002/2014gl061684
Martiny, A. C., Pham, C. T. A., Primeau, F. W., Vrugt, J. A., Moore, J. K., Levin, S. A., et al. (2013). Strong latitudinal patterns in the elemental ratios of marine plankton and organic matter. Nat. Geosci. 6, 279–283. doi: 10.1038/ngeo1757
Matsumoto, K., Sarmiento, J. L., and Brzezinski, M. A. (2002). Silicic acid leakage from the southern ocean: a possible explanation for glacial atmospheric pCO(2). Glob. Biogeochem. Cycles 16:GB001442.
McCormack, S. A., Melbourne-Thomas, J., Trebilco, R., Blanchard, J. L., and Constable, A. (2019). Alternative energy pathways in southern ocean food webs: Insights from a balanced model of Prydz Bay, Antarctica. Deep Sea Res. Part II Top. Stud. Oceanogr. 174:104613. doi: 10.1016/j.dsr2.2019.07.001
McGillicuddy, D. J., Sedwick, P. N., Dinniman, M. S., Arrigo, K. R., Bibby, T. S., Greenan, B. J. W., et al. (2015). Iron supply and demand in an Antarctic shelf ecosystem. Geophys. Res. Lett. 42, 8088–8097. doi: 10.1002/2015gl065727
McLeod, D. J., Hallegraeff, G. M., Hosie, G. W., and Richardson, A. J. (2012). Climate-driven range expansion of the red-tide dinoflagellate Noctiluca scintillans into the southern ocean. J. Plankton Res. 34, 332–337. doi: 10.1093/plankt/fbr112
McNeil, B. I., and Matear, R. J. (2008). Southern ocean acidification: a tipping point at 450-ppm atmospheric CO(2). Proc. Natl. Acad. Sci. U.S.A. 105, 18860–18864. doi: 10.1073/pnas.0806318105
McNeil, B. I., Tagliabue, A., and Sweeney, C. (2010). A multi-decadal delay in the onset of corrosive ‘acidified’ waters in the Ross Sea of Antarctica due to strong air-sea CO2 disequilibrium. Geophys. Res. Lett. 37:L19607.
McQuaid, J. B., Kustka, A. B., Oborník, M., Horák, A., Mccrow, J. P., Karas, B. J., et al. (2018). Carbonate-sensitive phytotransferrin controls high-affinity iron uptake in diatoms. Nature 555, 534–537. doi: 10.1038/nature25982
Mdutyana, M., Thomalla, S. J., Philibert, R., Ward, B. B., and Fawcett, S. E. (2020). The seasonal cycle of nitrogen uptake and nitrification in the Atlantic sector of the Southern Ocean. Glob. Biogeochem. Cycles
Meehl, G. A., Arblaster, J. M., Bitz, C. M., Chung, C. T. Y., and Teng, H. (2016). Antarctic sea-ice expansion between 2000 and 2014 driven by tropical Pacific decadal climate variability. Nat. Geosci. 9, 590–595. doi: 10.1038/ngeo2751
Mendes, C. R. B., Souza, M. S., Garcia, V. M. T., Leal, M. C., Brotas, V., and Garcia, C. A. E. (2012). Dynamics of phytoplankton communities during late summer around the tip of the Antarctic Peninsula. Deep Sea Res. Part I Oceanogr. Res. Papers 65, 1–14. doi: 10.1016/j.dsr.2012.03.002
Mendes, C. R. B., Tavano, V. M., Dotto, T. D., Kerr, R., Souza, M. S., Garcia, C. A. E., et al. (2018). New insights on the dominance of cryptophytes in Antarctic coastal waters : a case study in Gerlache Strait. Deep Sea Res. Part II Top. Stud. Oceanogr. 149, 161–170. doi: 10.1016/j.dsr2.2017.02.010
Merlivat, L., Boutin, J., and Antoine, D. (2015). Roles of biological and physical processes in driving seasonal air–sea CO2 flux in the Southern Ocean: New insights from CARIOCA pCO2. J. Mar. Syst. 147, 9–20. doi: 10.1016/j.jmarsys.2014.04.015
Meskhidze, N., and Nenes, A. (2006). Phytoplankton and cloudiness in the southern ocean. Science 314:1419. doi: 10.1126/science.1131779
Meyer, B., Freier, U., Grimm, V., Groeneveld, J., Hunt, B. P. V., Kerwath, S., et al. (2017). The winter pack-ice zone provides a sheltered but food-poor habitat for larval Antarctic krill. Nat. Ecol. Evol. 1, 1853–1861. doi: 10.1038/s41559-017-0368-3
Middag, R., De Baar, H. J. W., Klunder, M. B., and Laan, P. (2013). Fluxes of dissolved aluminum and manganese to the Weddell Sea and indications for manganese co-limitation. Limnol. Oceanogr. 58, 287–300. doi: 10.4319/lo.2013.58.1.0287
Middag, R., De Baar, H. J. W., Laan, P., Cai, P. H., and Van Ooijen, J. C. (2011). Dissolved manganese in the Atlantic sector of the Southern Ocean. Deep Sea Res. Part II Top. Stud. Oceanogr. 58, 2661–2677. doi: 10.1016/j.dsr2.2010.10.043
Midorikawa, T., Inoue, H. Y., Ishii, M., Sasano, D., Kosugi, N., Hashida, G., et al. (2012). Decreasing pH trend estimated from 35-year time series of carbonate parameters in the Pacific sector of the southern ocean in summer. Deep Sea Res. Part I Oceanogr. Res. Papers 61, 131–139. doi: 10.1016/j.dsr.2011.12.003
Monien, D., Monien, P., Brunjes, R., Widmer, T., Kappenberg, A., Busso, A. A. S., et al. (2017). Meltwater as a source of potentially bioavailable iron to Antarctica waters. Antarctic Sci. 29, 277–291. doi: 10.1017/s095410201600064x
Monteiro, T., Kerr, R., Orselli, I. B. M., and Lencina-Avila, J. M. (2020). Towards an intensified summer CO2 sink behaviour in the Southern Ocean coastal regions. Prog. Oceanogr. 183:102267. doi: 10.1016/j.pocean.2020.102267
Montes-Hugo, M., Doney, S. C., Ducklow, H. W., Fraser, W., Martinson, D., Stammerjohn, S. E., et al. (2009). Recent changes in phytoplankton communities associated with rapid regional climate change along the western Antarctic Peninsula. Science 323, 1470–1473. doi: 10.1126/science.1164533
Montes-Hugo, M. A., Vernet, M., Martinson, D., Smith, R., and Iannuzzi, R. (2008). Variability on phytoplankton size structure in the western Antarctic Peninsula (1997-2006). Deep Sea Res. Part II Top. Stud. Oceanogr. 55, 2106–2117. doi: 10.1016/j.dsr2.2008.04.036
Moore, C. M., Mills, M. M., Arrigo, K. R., Berman-Frank, I., Bopp, L., Boyd, P. W., et al. (2013). Processes and patterns of oceanic nutrient limitation. Nat. Geosci. 6, 701–710.
Moore, J. K., Fu, W. W., Primeau, F., Britten, G. L., Lindsay, K., Long, M., et al. (2018). Sustained climate warming drives declining marine biological productivity. Science 359, 1139–1142.
Moreau, S., Boyd, P. W., and Strutton, P. G. (2020). Remote assessment of the fate of phytoplankton in the Southern Ocean sea-ice zone. Nat. Commun. 11:1234567890.
Moreau, S., Mostajir, B., Belanger, S., Schloss, I. R., Vancoppenolle, M., Demers, S., et al. (2015). Climate change enhances primary production in the western Antarctic Peninsula. Glob. Change Biol. 21, 2191–2205. doi: 10.1111/gcb.12878
Moreau, S., Schloss, I. R., Mostajir, B., Demers, S., Almandoz, G. O., Ferrario, M. E., et al. (2012). Influence of microbial community composition and metabolism on air-sea Delta pCO(2) variation off the western Antarctic Peninsula. Mar. Ecol. Prog. Ser. 446, 45–59. doi: 10.3354/meps09466
Morley, S. A., Bates, A. E., Lamare, M., Richard, J., Nguyen, K. D., Brown, J., et al. (2016). Rates of warming and the global sensitivity of shallow water marine invertebrates to elevated temperature. J. Mar. Biol. Assoc. U. K. 96, 159–165. doi: 10.1017/s0025315414000307
Moss, B. (2017). Marine reptiles, birds and mammals and nutrient transfers among the seas and the land: an appraisal of current knowledge. J. Exp. Mar. Biol. Ecol. 492, 63–80. doi: 10.1016/j.jembe.2017.01.018
Mu, L., Stammerjohn, S. E., Lowry, K. E., and Yager, P. L. (2014). Spatial variability of surface pCO2 and air-sea CO2 flux in the Amundsen Sea Polynya, Antarctica. Element. Sci. Anthropocene 3:36.
Munro, D. R., Lovenduski, N. S., Takahashi, T., Stephens, B. B., Newberger, T., and Sweeney, C. (2015). Recent evidence for a strengthening CO2 sink in the Southern Ocean from carbonate system measurements in the Drake Passage (2002-2015). Geophys. Res. Lett. 42, 7623–7630. doi: 10.1002/2015gl065194
Murphy, E. J., Cavanagh, R. D., Drinkwater, K. F., Grant, S. M., Heymans, J. J., Hofmann, E. E., et al. (2016). Understanding the structure and functioning of polar pelagic ecosystems to predict the impacts of change. Proc. R. Soc. B Biol. Sci. 283:20161646. doi: 10.1098/rspb.2016.1646
Murphy, E. J., and Hofmann, E. E. (2012). End-to-end in Southern Ocean ecosystems. Curr. Opin. Environ. Sustain. 4, 264–271. doi: 10.1016/j.cosust.2012.05.005
Newman, L., Heil, P., Trebilco, R., Katsumata, K., Constable, A., Van Wijk, E., et al. (2019). Delivering sustained, coordinated, and integrated observations of the southern ocean for global impact. Front. Mar. Sci. 6:433. doi: 10.3389/fmars.2019.00433
Nicol, S., Bowie, A., Jarman, S., Lannuzel, D., Meiners, K. M., and Van Der Merwe, P. (2010). Southern Ocean iron fertilization by baleen whales and Antarctic krill. Fish Fish. 11, 203–209. doi: 10.1111/j.1467-2979.2010.00356.x
Nissen, C., Vogt, M., Münnich, M., Gruber, N., and Haumann, F. A. (2018). Factors controlling coccolithophore biogeography in the Southern Ocean. Biogeosciences 15, 6997–7024. doi: 10.5194/bg-15-6997-2018
Noble, A. E., Moran, D. M., Allen, A. E., and Saito, M. A. (2013). Dissolved and particulate trace metal micronutrients under the McMurdo sound seasonal sea ice: basal sea ice communities as a capacitor for iron. Front. Chem. 1:25. doi: 10.3389/fchem.2013.00025
Nozaki, Y., and Yamamoto, Y. (2001). Radium 228 based nitrate fluxes in the eastern Indian Ocean and the South China Sea and a silicon-induced “alkalinity pump” hypothesis. Glob. Biogeochem. Cycles 15, 555–567. doi: 10.1029/2000gb001309
Olson, R. J. (1981). Differential photoinhibition of marine nitrifying bacteria: a possible mechanism for the formation of the primary nitrite maximum. J. Mar. Res. 39, 227–238.
Orr, J. C., Fabry, V. J., Aumont, O., Bopp, L., Doney, S. C., Feely, R. A., et al. (2005). Anthropogenic ocean acidification over the twenty-first century and its impact on calcifying organisms. Nature 437, 681–686. doi: 10.1038/nature04095
Orr, J. C., Maier-Reimer, E., Mikolajewicz, U., Monfray, P., Sarmiento, J. L., Toggweiler, J. R., et al. (2001). Estimates of anthropogenic carbon uptake from four three-dimensional global ocean models. Glob. Biogeochem. Cycles 15, 43–60. doi: 10.1029/2000gb001273
Orsi, A. H., Whitworth, T., and Nowlin, W. D. (1995). On the meridional extent and fronts of the Antarctic circumpolar current. Deep Sea Res. Part I Oceanogr. Res. Papers 42, 641–673. doi: 10.1016/0967-0637(95)00021-w
Ortega, A., Geraldi, N. R., Alam, I., Kamau, A. A., Acinas, S. G., Logares, R., et al. (2019). Important contribution of macroalgae to oceanic carbon sequestration. Nat. Geosci. 12, 748–754. doi: 10.1038/s41561-019-0421-8
Pardo, P. C., Tilbrook, B., Langlais, C., Trull, T. W., and Rintoul, S. R. (2017). Carbon uptake and biogeochemical change in the Southern Ocean, south of Tasmania. Biogeosciences 14, 5217–5237. doi: 10.5194/bg-14-5217-2017
Park, Y.-H., Gamberoni, L., and Charriaud, E. (1993). Frontal structure, water masses, and circulation in the Crozet Basin. J. Geophys. Res. 98, 12361–12385.
Passow, U., and Carlson, C. A. (2012). The biological pump in a high CO2 world. Mar. Ecol. Prog. Ser. 470, 249–271.
Pausch, F., Bischof, K., and Trimborn, S. (2019). Iron and manganese co-limit growth of the Southern Ocean diatom Chaetoceros debilis. PLoS One 14:e0221959. doi: 10.1371/journal.pone.0221959
Peck, L. S., Barnes, D. K. A., Cook, A. J., Fleming, A. H., and Clarke, A. (2010). Negative feedback in the cold: ice retreat produces new carbon sinks in Antarctica. Glob. Change Biol. 16, 2614–2623. doi: 10.1111/j.1365-2486.2009.02071.x
Petrou, K., Baker, K. G., Nielsen, D. A., Hancock, A. M., Schulz, K. G., and Davidson, A. T. (2019). Acidification diminishes diatom silica production in the Southern Ocean. Nat. Clim. Change 9, 781–786. doi: 10.1038/s41558-019-0557-y
Petrou, K., Kranz, S. A., Trimborn, S., Hassler, C. S., Ameijeiras, S. B., Sackett, O., et al. (2016). Southern Ocean phytoplankton physiology in a changing climate. J. Plant Physiol. 203, 135–150. doi: 10.1016/j.jplph.2016.05.004
Planquette, H., Sherrell, R. M., Stammerjohn, S., and Field, M. P. (2013). Particulate iron delivery to the water column of the Amundsen Sea, Antarctica. Mar. Chem. 153, 15–30. doi: 10.1016/j.marchem.2013.04.006
Pollard, R. T., Lucas, M. I., and Read, J. F. (2002). Physical controls on biogeochemical zonation in the Southern Ocean. Deep Sea Res. Part II Top. Stud. Oceanogr. 49, 3289–3305. doi: 10.1016/s0967-0645(02)00084-x
Pondaven, P., Ragueneau, O., Treguer, P., Hauvespre, A., Dezileau, L., and Reyss, J. L. (2000). Resolving the ‘opal paradox’ in the Southern Ocean. Nature 405, 168–172. doi: 10.1038/35012046
Price, H. J., Boyd, K. R., and Boyd, C. M. (1988). Omnivorous feeding behavior of the Antarctic krill Euphausia superba. Mar. Biol. 97, 67–77. doi: 10.1007/bf00391246
Quéguiner, B. (2013). Iron fertilization and the structure of planktonic communities in high nutrient regions of the Southern Ocean. Deep Sea Res. Part II Top. Stud. Oceanogr. 90, 43–54. doi: 10.1016/j.dsr2.2012.07.024
Quetin, L. B., and Ross, R. M. (1985). “Feeding by Antarctic Krill, Euphausia superba: does size matter?,” in Antarctic Nutrient Cycles and Food Webs, eds W. R. Siegfried, P. R. Condy, and R. M. Laws, (Berlin: Springer).
Raiswell, R., Hawkings, J., Elsenousy, A., Death, R., Tranter, M., and Wadham, J. (2018). Iron in glacial systems: speciation, reactivity, freezing behavior, and alteration during transport. Front. Earth Sci. 6:222. doi: 10.3389/feart.2018.00222
Raiswell, R., Hawkings, J. R., Benning, L. G., Baker, A. R., Death, R., Albani, S., et al. (2016). Potentially bioavailable iron delivery by iceberg-hosted sediments and atmospheric dust to the polar oceans. Biogeosciences 13, 3887–3900. doi: 10.5194/bg-13-3887-2016
Ratnarajah, L., Bowie, A. R., Lannuzel, D., Meiners, K. M., and Nicol, S. (2014). The biogeochemical role of baleen whales and krill in southern ocean nutrient cycling. PLoS One 9:e114067. doi: 10.1371/journal.pone.0114067
Ratnarajah, L., Nicol, S., and Bowie, A. R. (2018). Pelagic iron recycling in the southern ocean: exploring the contribution of marine animals. Front. Mar. Sci. 5:109. doi: 10.3389/fmars.2018.00109
Rembauville, M., Blain, S., Armand, L., Quéguiner, B., and Salter, I. (2015). Export fluxes in a naturally iron-fertilized area of the southern ocean – Part 2: importance of diatom resting spores and faecal pellets for export. Biogeosciences 12, 3171–3195. doi: 10.5194/bg-12-3171-2015
Rembauville, M., Blain, S., Manno, C., Tarling, G., Thompson, A., Wolff, G., et al. (2018). The role of diatom resting spores in pelagic–benthic coupling in the Southern Ocean. Biogeosciences 15, 3071–3084. doi: 10.5194/bg-15-3071-2018
Rembauville, M., Briggs, N., Ardyna, M., Uitz, J., Catala, P., Penkerc’h, C., et al. (2017). Plankton assemblage estimated with BGC-argo floats in the southern ocean: implications for seasonal successions and particle export. Journal of Geophysical Research-Oceans 122, 8278–8292. doi: 10.1002/2017jc013067
Rignot, E., Mouginot, J., Scheuchl, B., Van Den Broeke, M., Van Wessem, M. J., and Morlighem, M. (2019). Four decades of Antarctic Ice sheet mass balance from 1979-2017. Proc. Natl. Acad. Sci. U.S.A. 116, 1095–1103. doi: 10.1073/pnas.1812883116
Rintoul, S. R. (2018). The global influence of localized dynamics in the Southern Ocean. Nature 558, 209–218. doi: 10.1038/s41586-018-0182-3
Ritter, R., Landschützer, P., Gruber, N., Fay, A. R., Iida, Y., Jones, S., et al. (2017). Observation-based trends of the southern ocean carbon sink. Geophys. Res. Lett. 12:348.
Rogers, A. D., Frinault, B. A. V., Barnes, D. K. A., Bindoff, N. L., Downie, R., Ducklow, H. W., et al. (2020). Antarctic futures: an assessment of climate-driven changes in ecosystem structure, function, and service provisioning in the southern ocean. Annu. Rev. Mar. Sci. 12, 87–120. doi: 10.1146/annurev-marine-010419-011028
Roobaert, A., Laruelle, G. G., Landschützer, P., Gruber, N., Chou, L., and Regnier, P. (2019). The spatiotemporal dynamics of the sources and sinks of CO2 in the global coastal ocean. Glob. Biogeochem. Cycles 33, 1–22.
Rozema, P. D., Venables, H. J., Poll, W. H., Clarke, A., Meredith, M. P., and Buma, A. G. J. (2017). Interannual variability in phytoplankton biomass and species composition in northern Marguerite Bay (West Antarctic Peninsula) is governed by both winter sea ice cover and summer stratification. Limnol. Oceanogr. 62, 235–252. doi: 10.1002/lno.10391
Saba, G. K., Fraser, W. R., Saba, V. S., Iannuzzi, R. A., Coleman, K. E., Doney, S. C., et al. (2014). Winter and spring controls on the summer food web of the coastal West Antarctic Peninsula. Nat. Commun. 5:4318.
Saba, G. K., Schofield, O., Torres, J. J., Ombres, E. H., and Steinberg, D. K. (2012). Increased feeding and nutrient excretion of adult antarctic krill, Euphausia superba, exposed to enhanced carbon dioxide (CO2). PLoS One 7:e52224. doi: 10.1371/journal.pone.0052224
Sabine, C. L., Feely, R. A., Gruber, N., Key, R. M., Lee, K., Bullister, J. L., et al. (2004). The oceanic sink for anthropogenic CO2. Science 305, 367–371. doi: 10.1126/science.1097403
Sailley, S. F., Ducklow, H. W., Moeller, H. V., Fraser, W. R., Schofield, O. M., Steinberg, D. K., et al. (2013). Carbon fluxes and pelagic ecosystem dynamics near two western Antarctic Peninsula Adelie penguin colonies: an inverse model approach. Mar. Ecol. Prog. Ser. 492, 253–272. doi: 10.3354/meps10534
Sallee, J. B., Matear, R. J., Rintoul, S. R., and Lenton, A. (2012). Localized subduction of anthropogenic carbon dioxide in the Southern Hemisphere oceans. Nat. Geosci. 5, 579–584. doi: 10.1038/ngeo1523
Santschi, P. H. (2018). Marine colloids, agents of the self-cleansing capacity of aquatic systems: historical perspective and new discoveries. Mar. Chem. 207, 124–135. doi: 10.1016/j.marchem.2018.11.003
Sarmiento, J. L., Gruber, N., Brzezinski, M. A., and Dunne, J. P. (2004). High-latitude controls of thermocline nutrients and low latitude biological productivity. Nature 427, 56–60. doi: 10.1038/nature02127
Sarmiento, J. L., and LeQuere, C. (1996). Oceanic carbon dioxide uptake in a model of century-scale global warming. Science 274, 1346–1350. doi: 10.1126/science.274.5291.1346
Sarmiento, J. L., and Toggweiler, J. R. (1984). A new model for the role of the oceans in determining atmospheric P CO2. Nature 308, 621–624. doi: 10.1038/308621a0
Sarthou, G., Vincent, D., Christaki, U., Obernosterer, I., Timmermans, K. R., and Brussaard, C. P. D. (2008). The fate of biogenic iron during a phytoplankton bloom induced by natural fertilisation: impact of copepod grazing. Deep Sea Res. Part II Top. Stud. Oceanogr. 55, 734–751. doi: 10.1016/j.dsr2.2007.12.033
Schallenberg, C., Bestley, S., Klocker, A., Trull, T. W., Davies, D. M., Gault−Ringold, M., et al. (2018). Sustained upwelling of subsurface iron supplies seasonally persistent phytoplankton blooms around the Southern Kerguelen plateau, Southern ocean. J. Geophys. Res. Oceans 123, 5986–6003. doi: 10.1029/2018jc013932
Schine, C. M. S., Van Dijken, G., and Arrigo, K. R. (2016). Spatial analysis of trends in primary production and relationship with large-scale climate variability in the Ross Sea, Antarctica (1997-2013). J. Geophys. Res. Oceans 121, 368–386. doi: 10.1002/2015jc011014
Schlitzer, R. (2018). Ocean Data View. Available online at: https://odv.awi.de (accessed June 3, 2020).
Schlitzer, R., Anderson, R. F., Dodas, E. M., Lohan, M., Geibere, W., Tagliabue, A., et al. (2018). The GEOTRACES intermediate data product 2017. Chem. Geol. 493, 210–223.
Schloss, I. R., Wasilowska, A., Dumont, D., Almandoz, G. O., Hernando, M. P., Michaud-Tremblay, C. A., et al. (2014). On the phytoplankton bloom in coastal waters of southern King George Island (Antarctica) in January 2010: An exceptional feature? Limnol. Oceanogr. 59, 195–210. doi: 10.4319/lo.2014.59.1.0195
Schmidt, K., Atkinson, A., Petzke, K.-J., Voss, M., and Pond, D. W. (2006). Protozoans as a food source for Antarctic krill, Euphausia superba: complementary insights from stomach content, fatty acids, and stable isotopes. Limnol. Oceanogr. 51, 2409–2427. doi: 10.4319/lo.2006.51.5.2409
Schmidt, K., Atkinson, A., Steigenberger, S., Fielding, S., Lindsay, M. C. M., Pond, D. W., et al. (2011). Seabed foraging by Antarctic krill: implications for stock assessment, bentho-pelagic coupling, and the vertical transfer of iron. Limnol. Oceanogr. 56, 1411–1428.
Schmidt, K., Schlosser, C., Atkinson, A., Fielding, S., Venables, H. J., Waluda, C. M., et al. (2016). Zooplankton gut passage mobilizes lithogenic iron for ocean productivity. Curr. Biol. 26, 2667–2673. doi: 10.1016/j.cub.2016.07.058
Schofield, O., Saba, G., Coleman, K., Carvalho, F., Couto, N., Ducklow, H., et al. (2017). Decadal variability in coastal phytoplankton community composition in a changing West Antarctic Peninsula. Deep Sea Res. Part I Oceanogr. Res. Papers 124, 42–54. doi: 10.1016/j.dsr.2017.04.014
Sedwick, P. N., Bowie, A. R., and Trull, T. W. (2008). Dissolved iron in the Australian sector of the Southern Ocean (CLIVAR SR3 section): Meridional and seasonal trends. Deep Sea Res. Part I Oceanogr. Res. Papers 55, 911–925. doi: 10.1016/j.dsr.2008.03.011
Shafiee, R. T., Snow, J. T., Zhang, Q., and Rickaby, R. E. M. (2019). Iron requirements and uptake strategies of the globally abundant marine ammonia-oxidising archaeon, Nitrosopumilus maritimus SCM1. ISME J. 13, 2295–2305. doi: 10.1038/s41396-019-0434-8
Sherman, E., Moore, J. K., Primeau, F., and Tanouye, D. (2016). Temperature influence on phytoplankton community growth rates. Glob. Biogeochem. Cycles 30, 550–559. doi: 10.1002/2015gb005272
Sherrell, R. M., Annett, A. L., Fitzsimmons, J. N., Roccanova, V. J., and Meredith, M. P. (2018). A ‘shallow bathtub ring’ of local sedimentary iron input maintains the Palmer Deep biological hotspot on the West Antarctic Peninsula shelf. Philos. Trans. R. Soc. A Math. Phys. Eng. Sci. 376:20170171. doi: 10.1098/rsta.2017.0171
Sherrell, R. M., Lagerstrom, M. E., Forsch, K. O., Stammerjohn, S. E., and Yager, P. L. (2015). Dynamics of dissolved iron and other bioactive trace metals (Mn. Ni, Cu, Zn) in the Amundsen Sea Polynya, Antarctica. Elementa Sci. Anthropocene 3, 1–27.
Shi, D., Xu, Y., Hopkinson, B. M., and Morel, F. M. M. (2010). Effect of ocean acidification on iron availability to marine phytoplankton. Science 327, 676–679. doi: 10.1126/science.1183517
Sholkovitz, E. R., Sedwick, P. N., Church, T. M., Baker, A. R., and Powell, C. F. (2012). Fractional solubility of aerosol iron: synthesis of a global-scale data set. Geochim. Cosmochim. Acta 89, 173–189. doi: 10.1016/j.gca.2012.04.022
Sigman, D. M., Hain, M. P., and Haug, G. H. (2010). The polar ocean and glacial cycles in atmospheric CO2 concentration. Nature 466, 47–55. doi: 10.1038/nature09149
Smart, S. M., Fawcett, S. E., Thomalla, S. J., Weigand, M. A., Reason, C. J. C., and Sigman, D. M. (2015). Isotopic evidence for nitrification in the Antarctic winter mixed layer. Glob. Biogeochem. Cycles 29, 427–445. doi: 10.1002/2014gb005013
Smetacek, V. (2008). “Are declining Antarctic krill stocks a result of global warming or of the decimation of the whales?,” in Impacts of Global Warming on Polar Ecosystems, ed. C. M. Duarte, (Madrid: Fundación BBVA).
Smith, J. M., Chavez, F. P., and Francis, C. A. (2014). Ammonium uptake by phytoplankton regulates nitrification in the sunlit ocean. PLoS One 9:e108173. doi: 10.1371/journal.pone.0108173
Smith, W. O., Anderson, R. F., Keith Moore, J., Codispoti, L. A., and Morrison, J. M. (2000). The US southern ocean joint global ocean flux study: an introduction to AESOPS. Deep Sea Res. Part II Top. Stud. Oceanogr. 47, 3073–3093. doi: 10.1016/s0967-0645(00)00059-x
Steinacher, M., Joos, F., Frölicher, T. L., Bopp, L., Cadule, P., Cocco, V., et al. (2010). Projected 21st century decrease in marine productivity: a multi-model analysis. Biogeosciences 7, 979–1005. doi: 10.5194/bg-7-979-2010
Steinberg, D. K., Ruck, K. E., Gleiber, M. R., Garzio, L. M., Cope, J. S., Bernard, K. S., et al. (2015). Long-term (1993-2013) changes in macrozooplankton off the Western Antarctic Peninsula. Deep Sea Res. Part I Oceanogr. Res. Papers 101, 54–70. doi: 10.1016/j.dsr.2015.02.009
St-Laurent, P., Yager, P. L., Sherrell, R. M., Stammerjohn, S. E., and Dinniman, M. S. (2017). Pathways and supply of dissolved iron in the Amundsen Sea (Antarctica). J. Geophys. Res. Oceans 122, 7135–7162. doi: 10.1002/2017jc013162
Stroeve, J., and Meier, W. N. (2018). Sea Ice Trends and Climatologies from SMMR and SSM/I-SSMIS, Version 3. Boulder, CO: NASA National Snow and Ice Data Center Distributed Active Archive Center. doi: 10.5067/IJ0T7HFHB9Y6
Strzepek, R. F., Boyd, P. W., and Sunda, W. G. (2019). Photosynthetic adaptation to low iron, light, and temperature in Southern Ocean phytoplankton. Proc. Natl. Acad. Sci. U.S.A. 116, 4388–4393. doi: 10.1073/pnas.1810886116
St-Laurent, P., Yager, P. L., Sherrell, R. M., Oliver, H., Dinniman, M. S., and Stammerjohn, S. E. (2019). Modeling the seasonal cycle of iron and carbon fluxes in the amundsen sea Polynya, Antarctica. J. Geophys. Res. Oceans 124, 1544–1565. doi: 10.1029/2018jc014773
Suckling, C. C., Clark, M. S., Richard, J., Morley, S. A., Thorne, M. A. S., Harper, E. M., et al. (2015). Adult acclimation to combined temperature and pH stressors significantly enhances reproductive outcomes compared to short-term exposures. J. Anim. Ecol. 84, 773–784. doi: 10.1111/1365-2656.12316
Tagliabue, A., Aumont, O., Death, R., Dunne, J. P., Dutkiewicz, S., Galbraith, E., et al. (2016). How well do global ocean biogeochemistry models simulate dissolved iron distributions? Glob. Biogeochem. Cycles 30, 149–174. doi: 10.1002/2015gb005289
Tagliabue, A., Bopp, L., and Aumont, O. (2009a). Evaluating the importance of atmospheric and sedimentary iron sources to Southern Ocean biogeochemistry. Geophys. Res. Lett. 36:L13601.
Tagliabue, A., Bopp, L., Aumont, O., and Arrigo, K. R. (2009b). Influence of light and temperature on the marine iron cycle: from theoretical to global modeling. Glob. Biogeochem. Cycles 23:GB2017.
Tagliabue, A., Bowie, A. R., Devries, T., Ellwood, M. J., Landing, W. M., Milne, A., et al. (2019). The interplay between regeneration and scavenging fluxes drives ocean iron cycling. Nat. Commun. 10:4960.
Tagliabue, A., Mtshali, T., Aumont, O., Bowie, A. R., Klunder, M. B., Roychoudhury, A. N., et al. (2012). A global compilation of dissolved iron measurements: focus on distributions and processes in the Southern Ocean. Biogeosciences 9, 2333–2349. doi: 10.5194/bg-9-2333-2012
Tagliabue, A., Sallée, J.-B., Bowie, A. R., Lévy, M., Swart, S., and Boyd, P. W. (2014). Surface-water iron supplies in the Southern Ocean sustained by deep winter mixing. Nat. Geosci. 7, 314–320. doi: 10.1038/ngeo2101
Takahashi, T., Sutherland, S. C., Chipman, D. W., Goddard, J. G., Ho, C., Newberger, T., et al. (2014). Climatological distributions of pH, pCO(2), total CO2, alkalinity, and CaCO3 saturation in the global surface ocean, and temporal changes at selected locations. Mar. Chem. 164, 95–125. doi: 10.1016/j.marchem.2014.06.004
Takahashi, T., Sutherland, S. C., Wanninkhof, R., Sweeney, C., Feely, R. A., Chipman, D. W., et al. (2009). Climatological mean and decadal change in surface ocean pCO2, and net sea-air CO2 flux over the global oceans. Deep Sea Res. Part II Top. Stud. Oceanogr. 56, 554–577. doi: 10.1016/j.dsr2.2008.12.009
Takahashi, T., Sweeney, C., Hales, B., Chipman, D. W., Newberger, T., Goddard, J. G., et al. (2012). The changing carbon cycle in the southern ocean. Oceanography 25, 26–37. doi: 10.5670/oceanog.2012.71
Takeda, S. (1998). Influence of iron availability on nutrient consumption ratio of diatoms in oceanic waters. Nature 393, 774–777. doi: 10.1038/31674
Tarling, G. A., and Thorpe, S. E. (2017). Oceanic swarms of Antarctic krill perform satiation sinking. Proc. R. Soc. B Biol. Sci. 284:20172015. doi: 10.1098/rspb.2017.2015
Tarling, G. A., Thorpe, S. E., Fielding, S., Klevjer, T., Ryabov, A., and Somerfield, P. J. (2018). Varying depth and swarm dimensions of open-ocean Antarctic krill Euphausia superba Dana, 1850 (Euphausiacea) over diel cycles. J. Crustacean Biol. 38, 716–727.
Tortell, P. D., Payne, C. D., Li, Y. Y., Trimborn, S., Rost, B., Smith, W. O., et al. (2008). CO2 sensitivity of Southern Ocean phytoplankton. Geophys. Res. Lett. 35:L04605.
Tovar-Sanchez, A., Duarte, C. M., Hernández-León, S., and Sañudo-Wilhelmy, S. A. (2007). Krill as a central node for iron cycling in the Southern Ocean. Geophys. Res. Lett. 34:L11601.
Tranter, D. J. (1982). Interlinking of physical and biological processes in the Antarctic Ocean. Oceanogr. Mar. Biol. Annu. Rev. 20, 11–35.
Treasure, A. M., Ruzicka, J. J., Moloney, C. L., Gurney, L. J., and Ansorge, I. J. (2015). Land–sea interactions and consequences for sub-antarctic marine food webs. Ecosystems 18, 752–768. doi: 10.1007/s10021-015-9860-2
Tréguer, P., Bowler, C., Moriceau, B., Dutkiewicz, S., Gehlen, M., Aumont, O., et al. (2018). Influence of diatom diversity on the ocean biological carbon pump. Nat. Geosci. 11, 27–37. doi: 10.1038/s41561-017-0028-x
Trimborn, S., Brenneis, T., Sweet, E., and Rost, B. (2013). Sensitivity of Antarctic phytoplankton species to ocean acidification: Growth, carbon acquisition, and species interaction. Limnol. Oceanogr. 58, 997–1007. doi: 10.4319/lo.2013.58.3.0997
Trull, T. W., Passmore, A., Davies, D. M., Smit, T., Berry, K., and Tilbrook, B. (2018). Distribution of planktonic biogenic carbonate organisms in the Southern Ocean south of Australia: a baseline for ocean acidification impact assessment. Biogeosciences 15, 31–49. doi: 10.5194/bg-15-31-2018
van der Merwe, P., Wuttig, K., Holmes, T., Trull, T. W., Chase, Z., Townsend, A. T., et al. (2019). High Lability Fe particles sourced from glacial erosion can meet previously unaccounted biological demand: heard island, southern ocean. Front. Mar. Sci. 6:332. doi: 10.3389/fmars.2019.00332
Van Heuven, S. M. A. C., Hoppema, M., Jones, E. M., and De Baar, H. J. W. (2014). Rapid invasion of anthropogenic CO2 into the deep circulation of the Weddell Gyre. Philos. Trans. R. Soc. A Math. Phys. Eng. Sci. 372:20130056. doi: 10.1098/rsta.2013.0056
Venables, H. J., Clarke, A., and Meredith, M. P. (2013). Wintertime controls on summer stratification and productivity at the western Antarctic Peninsula. Limnol. Oceanogr. 58, 1035–1047. doi: 10.4319/lo.2013.58.3.1035
Wanninkhof, R. (2014). Relationship between wind speed and gas exchange over the ocean revisited. Limnol. Oceanogr. Methods 12, 351–362. doi: 10.4319/lom.2014.12.351
Ward, B. B. (2005). Molecular approaches to marine microbial ecology and the marine nitrogen cycle. Annu. Rev. Earth Planet. Sci. 33, 301–333. doi: 10.1146/annurev.earth.33.092203.122514
Weber, T. S., and Deutsch, C. (2010). Ocean nutrient ratios governed by plankton biogeography. Nature 467, 550–554. doi: 10.1038/nature09403
Weinbauer, M., Chen, F., and Wilhelm, S. (2011). “Virus-mediated redistribution and partitioning of carbon in the global oceans,” in Microbial Carbon Pump in the Ocean, eds N. Jiao, F. Azam, and S. Sanders, (Washington, DC: Science/AAAS), 54–56.
Weitz, J. S., and Wilhelm, S. W. (2012). Ocean viruses and their effects on microbial communities and biogeochemical cycles. F1000 Biol. Rep. 4:17.
Westwood, K. J., Thomson, P. G., Van Den Enden, R. L., Maher, L. E., Wright, S. W., and Davidson, A. T. (2018). Ocean acidification impacts primary and bacterial production in Antarctic coastal waters during austral summer. J. Exp. Mar. Biol. Ecol. 498, 46–60. doi: 10.1016/j.jembe.2017.11.003
Whitworth, T., and Nowlin, W. D. (1987). Water masses and currents of the Southern Ocean at the Greenwich Meridian. J. Geophys. Res. 92, 6462–6476.
Williams, N. L., Feely, R. A., Sabine, C. L., Dickson, A. G., Swift, J. H., Talley, L. D., et al. (2015). Quantifying anthropogenic carbon inventory changes in the Pacific sector of the Southern Ocean. Mar. Chem. 174, 147–160. doi: 10.1016/j.marchem.2015.06.015
Wing, S. R., Jack, L., Shatova, O., Leichter, J. J., Barr, D., Frew, R. D., et al. (2014). Seabirds and marine mammals redistribute bioavailable iron in the Southern Ocean. Mar. Ecol. Prog. Ser. 510, 1–13. doi: 10.3354/meps10923
Winton, V. H. L., Dunbar, G. B., Atkins, C. B., Bertler, N. A. N., Delmonte, B., Andersson, P. S., et al. (2016). The origin of lithogenic sediment in the south-western Ross Sea and implications for iron fertilization. Antarctic Sci. 28, 250–260. doi: 10.1017/s095410201600002x
Woolf, D. K., Shutler, J. D., Goddijn−Murphy, L., Watson, A. J., Chapron, B., Nightingale, P. D., et al. (2019). Key uncertainties in the recent air−sea flux of CO2. Glob. Biogeochem. Cycles 33, 1548–1563.
Wright, S. W., Van Den Enden, R. L., Pearce, I., Davidson, A. T., Scott, F. J., and Westwood, K. J. (2010). Phytoplankton community structure and stocks in the Southern Ocean (30–80°E) determined by CHEMTAX analysis of HPLC pigment signatures. Deep Sea Res. Part II Top. Stud. Oceanogr. 57, 758–778. doi: 10.1016/j.dsr2.2009.06.015
Wu, M., Mccain, J. S. P., Rowland, E., Middag, R., Sandgren, M., Allen, A. E., et al. (2019). Manganese and iron deficiency in Southern Ocean Phaeocystis antarctica populations revealed through taxon-specific protein indicators. Nat. Commun. 10:3582.
Zhang, J., Kattner, G., and Koch, B. P. (2019). Interactions of trace elements and organic ligands in seawater and implications for quantifying biogeochemical dynamics: a review. Earth Sci. Rev. 192, 631–649. doi: 10.1016/j.earscirev.2019.03.007
Keywords: Southern Ocean, biogeochemistry, primary production, iron, nutrients, carbon, ecosystem, ocean acidification
Citation: Henley SF, Cavan EL, Fawcett SE, Kerr R, Monteiro T, Sherrell RM, Bowie AR, Boyd PW, Barnes DKA, Schloss IR, Marshall T, Flynn R and Smith S (2020) Changing Biogeochemistry of the Southern Ocean and Its Ecosystem Implications. Front. Mar. Sci. 7:581. doi: 10.3389/fmars.2020.00581
Received: 20 April 2020; Accepted: 23 June 2020;
Published: 31 July 2020.
Edited by:
Jess Melbourne-Thomas, CSIRO Oceans and Atmosphere (O&A), AustraliaReviewed by:
Dieter Alfred Wolf-Gladrow, Alfred Wegener Institute Helmholtz Centre for Polar and Marine Research (AWI), GermanyKatherina Petrou, University of Technology Sydney, Australia
Copyright © 2020 Henley, Cavan, Fawcett, Kerr, Monteiro, Sherrell, Bowie, Boyd, Barnes, Schloss, Marshall, Flynn and Smith. This is an open-access article distributed under the terms of the Creative Commons Attribution License (CC BY). The use, distribution or reproduction in other forums is permitted, provided the original author(s) and the copyright owner(s) are credited and that the original publication in this journal is cited, in accordance with accepted academic practice. No use, distribution or reproduction is permitted which does not comply with these terms.
*Correspondence: Sian F. Henley, cy5mLmhlbmxleUBlZC5hYy51aw==
†ORCID: Sian F. Henley, orcid.org/0000-0003-1221-1983; Emma L. Cavan, orcid.org/0000-0003-1099-6705; Sarah E. Fawcett, orcid.org/0000-0002-0878-6496; Rodrigo Kerr, orcid.org/0000-0002-2632-3137; Thiago Monteiro, orcid.org/0000-0001-7643-0646; Andrew R. Bowie, orcid.org/0000-0002-5144-7799; Philip W. Boyd, orcid.org/0000-0001-7850-1911; Irene R. Schloss, orcid.org/0000-0002-5917-8925; Tanya Marshall, orcid.org/0000-0002-5982-5926; Raquel Flynn, orcid.org/0000-0003-3118-4474; Shantelle Smith, orcid.org/0000-0003-3917-4545