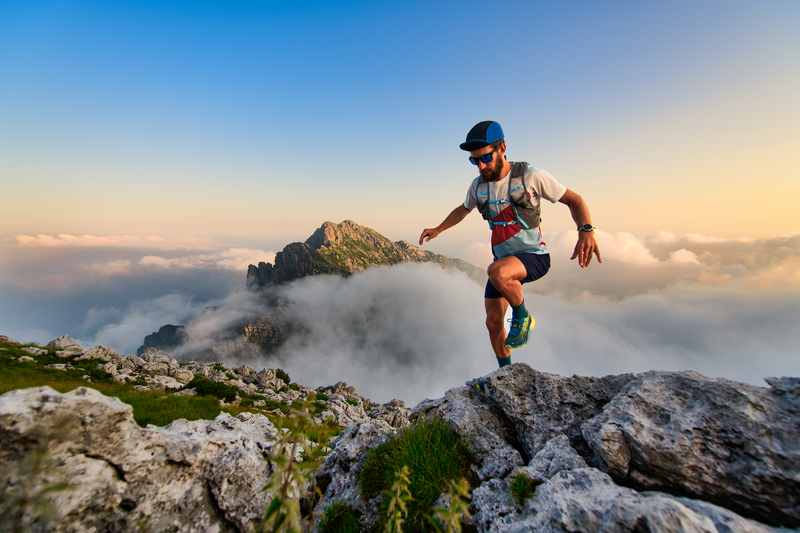
94% of researchers rate our articles as excellent or good
Learn more about the work of our research integrity team to safeguard the quality of each article we publish.
Find out more
ORIGINAL RESEARCH article
Front. Mar. Sci. , 22 July 2020
Sec. Marine Biogeochemistry
Volume 7 - 2020 | https://doi.org/10.3389/fmars.2020.00533
This article is part of the Research Topic Current Topics in Marine Organic Biogeochemical Research View all 21 articles
We studied the dynamics of fermentation and anaerobic degradation of organic matter at a fixed station in the Oxygen Minimum Zone (OMZ) within the Humboldt Current System off Concepción, central Chile. Products of the main anaerobic microbial reactions [fermentation, denitrification, and reduction of Fe(OH)3 and SO42–] were analyzed during laboratory incubations of OMZ waters. Fermentation of glucose and amino acids resulted in the production of volatile fatty acids, mainly acetate; these compounds were detected year-round in in situ water samples and were associated with high primary production rates and presence of O2-deficient waters at the sampling site. In contrast, whilst ethanol was produced from glucose fermentation by OMZ water microorganisms under laboratory conditions, it was not detected in the water column during the annual cycle. Evidence of acetate oxidation (which is thermodynamically feasible), with Fe(OH)3 as an electron acceptor, suggests that microbial activity could reduce solid-phase Fe carried by rivers using fermented metabolites in oxygen-depleted water, thus releasing dissolved bioavailable Fe. Here we present evidence for productivity-driven seasonality of biogeochemical cycles in the Humboldt system, supported by fermentation and anaerobic consumption of fermentation products oxidized by a variety of electron acceptors including NO3–, Fe(OH)3, and SO42–. Our results suggest that products of fermentation in the OMZ may provide a source of labile organics for advection to oxygenated waters of subantarctic origin during austral winter. Fermentation, anaerobic oxidation and associated advection of fermentation products are likely to be enhanced during the twenty-first century due both to temperature increase and decrease in dissolved O2 in the water column.
Hypoxic zones within the marine water column appear to be expanding due to warming and eutrophication (Diaz and Rosenberg, 2008; Rabalais et al., 2014; Schmidtko et al., 2017). These processes will enhance fermentative production of semi-reduced metabolites that act as substrates for anaerobic microbial oxidation of organic matter (Oremland and Polcin, 1982; Megonigal et al., 2004) through, for example, NO3– (denitrification) and SO42– reduction, and methanogenesis (Sansone and Martens, 1981; Jørgensen, 1982). Presently, O2-depleted marine environments are typically found in sediments, enclosed or semi-enclosed water bodies, coastal ocean dead zones, and areas referred to as Oxygen Minimum Zones or OMZs (Helly and Levin, 2004; Diaz and Rosenberg, 2008). These OMZs can be hundreds of meters in depth, and are characterized by suboxic and anoxic levels of O2 typically in the productive, weakly ventilated waters of the eastern Pacific and southeastern Atlantic Oceans, and the Arabian Sea (Wyrtki, 1962; Kamykowski and Zentara, 1990; Helly and Levin, 2004; Ulloa et al., 2012; Löscher et al., 2016; Pizarro-Koch et al., 2019). On a global scale, OMZs are significant sinks (via N2O and N2) for oceanic N (Codispoti et al., 2001), and are zones hosting active S cycling (Canfield et al., 2010) through diverse communities of anaerobic microbes (Fossing et al., 1995; Ward et al., 2009; Ulloa et al., 2012; Wright et al., 2012; Srain et al., 2015).
In low-oxygen environments, acetate is a major energy substrate for sulfate-reducing bacteria in lake (e.g., Skyring, 1988), estuarine (e.g., Suzuki et al., 2007) and marine sediments (e.g., Sørensen et al., 1981; Skyring et al., 1983; Parkes et al., 1989), and in the marine water column (Albert et al., 1995; Ho et al., 2002). Fermentation (incomplete oxidation of organics in the absence of external electron acceptors) is required to generate intermediate organic products for anaerobic oxidizers, whilst producing soluble molecules and gases that can circulate back into more oxygenated regions (Schmitz et al., 2006). The biological mechanisms, kinetics, and ecological role of fermentation have been extensively studied in rumen, sewage, soils and sediments (e.g., Sabine and Johnson, 1964; Hungate, 1965; Sansone and Martens, 1982; Shaw et al., 1984; Oremland, 1988; Conrad et al., 1989; Wagner et al., 1997; Moser-Engeler et al., 1998; Finke and Jørgensen, 2008; Bourke et al., 2017), but the occurrence and significance of this metabolic pathway has received less attention in suboxic and anoxic zones within the marine water column (e.g., Albert et al., 1995; Wu et al., 1997; Ho et al., 2002; González and Quiñones, 2009; Zhuang et al., 2019). Interest in fermentative reactions is becoming more relevant in light of expansion of OMZs (Breitburg et al., 2018), and the increasing concentrations of N (Howarth, 2008), P (Bennett et al., 2001), and organic carbon (Berner, 1982) associated with eutrophication and deoxygenation of the coastal ocean (Rabalais et al., 2014).
In the present study, we examined chemical dynamics of the water column within the OMZ off Concepción, Chile (∼36oS). This is a coastal area where intense seasonal upwelling (Sobarzo et al., 2001, 2007) of high-nutrient subsurface waters – depleted in dissolved O2 –supports high rates of primary production of up to 20 g C m–2 d–1 in near surface waters (Montero et al., 2007; Testa et al., 2018). The area off Concepción constitutes the most southerly extent of the OMZ in the eastern South Pacific (Fuenzalida et al., 2009), the fourth largest (by volume) of the six permanent hypoxic regions in the world oceans (Schneider et al., 2006). At this latitude on the continental shelf, the OMZ is located beneath surface waters of subantarctic origin (eastern South Pacific Transition Water, ESPTW) and is fed by poorly oxygenated subsurface waters (Equatorial Subsurface Water, ESSW) which upwell toward the coast driven by favorable winds during austral spring and summer (i.e., from September–October to March–April). During austral winter, northerly converging winds generate coastal downwelling and offshore bottom Ekman transport, which removes ESSW from the shelf and replaces it with ESPTW throughout most of the water column (Sobarzo et al., 2007).
The goal of the present study was to reveal relevant microbial pathways within OMZ waters [fermentation, and reduction of NO3–, Fe(OH)3 and SO42–]. A series of controlled incubations of OMZ microbial assemblages, supplemented with high concentrations of substrates, was interpreted in the context of anaerobic processes within the water column during an annual cycle. The study aimed to describe annual patterns within the water column of vertical distribution of volatile fatty acids (VFA), mainly acetate, and other indicators of anaerobic metabolism of OMZ. A further aim was to evaluate potential export of these indicators to oxygenated waters of subantarctic origin.
Cruises were carried out within the framework of the project “Microbial Initiative in Low Oxygen off Concepción and Oregon” (MILOCO), and as a part of the Time Series at Oceanographic Station 18 (33 km from the coast, 90 m depth, 36°29.94′S, 73°07.8′W) conducted by the COPAS Center for Oceanographic Research in the eastern South Pacific (FONDAP CONICYT Chile) in the upwelling ecosystem off Concepción in central Chile (Figure 1). Water samples were collected monthly aboard L/C Kay-Kay II between November 2009 and January 2011, encompassing two austral springs, one austral fall, one austral winter, and two austral summers. Ancillary water column measurements of dissolved O2, fluorescence, temperature, salinity, and nutrients were provided by the database of the COPAS Center and the MILOCO Project. Data for hourly coastal winds were collected by the meteorological station at Carriel Sur Airport (36°47′S; 73°04′W), located less than 10 km from the coast of Concepcion.
Figure 1. The geographical location of the study site at Station 18, off Concepción in central Chile. Color scale represents the average chlorophyll-a concentration observed between November 2009 and January 2011. The black solid line shows the 200 m isobath and the magenta dashed square represents the area analyzed for Net Primary Production estimates. The map was generated using Ocean Data View (Schlitzer, 2010).
Water samples for chemical measurements and incubations were collected with Niskin bottles (10 L) at six depths (0, 10, 30, 50, 65, 80 m) stored in acid-washed carboys in the dark at ca. 10°C. For VFA, ethanol, and CO2 measurements, 50 mL aliquots of seawater (in triplicate) were removed on board and dispensed into glass bottles in a N2 saturated chamber using glove bags (Aldrich® AtmosBag) and immediately poisoned with HgCl2 (0.001%). Gas-tight bottles were sealed with butyl rubber stoppers, crimped, and then stored in the dark at 10°C. In the laboratory (within 12 h), 1 L seawater per depth was filtered through pre-combusted 0.7 μm glass fiber filters (Whatman GF/F); filtrates and filters were kept at −20°C prior to analyses of dissolved amino acids, and elemental analysis of C and N and natural abundance of 13C and 15N in particulate material.
On November 29, 2010, inocula of seawater for laboratory incubations were taken from 65 m depth (10.2°C, 34.6 PSU, 8.4 μM O2), and transferred to 10 mL serum vacuum-tubes BD®. Tubes were stored at 10°C in darkness until arrival at laboratory.
Dissolved inorganic N anomalies – defined as a linear combination of nitrate and phosphate – were used in order to determine the role and distribution of nitrogen fixation and denitrification in the water column. The parameter N∗ was estimated following Hansell et al. (2004, N∗ = [NO3– + NO2– + NH4+] – 16 [PO4+3] + 2.9). N∗values lower than −3 μmol kg–1 are indicative of denitrification while values higher than 2 μmol kg–1 denotes nitrogen fixation (Gruber and Sarmiento, 1997).
Artificial seawater for incubations was prepared according to Lovley (2006). To remove O2, water was autoclaved, capped, and gently bubbled with N2 for 15 min in a laminar flow hood LABCONCO Class II Type IIA. In addition to those measures, O2-sensitive methylene blue (Resazurin 0.0001%, Wolfe, 2011) was added to incubation vessels to detect unwanted traces of O2 (higher than 700 nM).
Incubations were conducted in darkness in a Shell-Lab incubator (Sheldon Manufacturing, United States) at 10°C for 7 days (glucose fermentation), 8 days (amino acids fermentation), and 35 days (acetate oxidation). Subsamples of 2 mL were removed and filtered through 0.22 μm filters (MILLEX GVTM filter unit) every ca. 24 h for analyses of amino acids, and pH (pH–indicator paper Neutralit, pH 5.5–9.0, Merck Millipore). Measurements of Fe2+aq were carried out by removing 500 μL of water at the beginning and the end of the incubations, and amending with Fe(OH)3 as electron acceptor. For CO2 and HS– measurements, 500 μL of gas was removed from the headspace at each subsampling time. Control treatments were prepared without inocula. Filtration of solutions (0.22 μm MILLEX GVTM), bubbling with N2, and the addition of substrates and inocula were conducted under a N2 saturated chamber.
Aliquots of 30 mL artificial seawater were transferred into gas-tight bottles (60 mL, in triplicate) containing 3 mL inocula of seawater, and then amended with glucose or the amino acids alanine, leucine, threonine, phenylalanine, glutamic acid, and ornithine to final concentrations of 40 mM glucose and 10 mM of each amino acid. Such high substrate concentrations are four orders of magnitude higher than natural DOC concentrations and were intended to provide a culture medium not limited by substrate in order to achieve quick-start of microbial growth and short incubation times. Consequently, no inferences were made regarding reaction rates from these incubations because of the transient nature of zero-order reactions; however, the experiments do reveal potential reactions within OMZ waters. Incubation vessels were treated with 200 μM molybdate to prevent consumption of fermentative products by SO42– reduction (Oremland and Capone, 1988), and with 200 μM N-guanyl-1,7-diaminoheptane (GC7) to stop acetotrophic methanogenesis (Jansson et al., 2000). Bottles were capped with pre-sterilized butyl-caps and aluminum seals, and then incubated as described.
Anoxic incubations (35 days) and inoculations were conducted as described above in the glucose fermentation section. NO3– (NaNO3, 60 mM), 250 mM of poorly crystalline ferrihydrite-iron oxyhydroxide (Schwertmann and Cornell, 1991), Fe(OH)3, and SO42– (Na2SO4, 50 mM) were added to incubations as electron acceptors, and acetate (NaCH3COO, 40 mM) supplemented as C source. Artificial seawater with 5% v/v of reducing solution (Na2O2S × 5H2O and cysteine) was used in the experiments for acetotrophic SO42– reduction. Fe2+aq concentrations were measured with the 1,10 Phenanthroline method, using kit HACH (Hach Lange GmbH) and a spectrophotometer HACH DR-4000 (Method 8146, DOC316.53.01049). The detection limit of the assay was 3.5 ± 0.3 μM.
Acetate, propionate, isobutyrate, butyrate isovalerate, valerate, and ethanol were extracted from headspaces of both ambient samples and incubation vessels using solid-phase micro-extraction. Equilibrium partition between the aqueous phase and headspace was estimated by sonicating standard solutions of VFA (0.1, 1, 5 μM) in gas-tight bottles containing synthetic seawater at 30°C for 15 min, followed by adsorption of analytes from the gaseous phase on 85 μm CarboxenTM/PDMS Stable Flex micro-extraction fibers (SUPELCO). An adsorption fiber was inserted through the septum in the headspace and maintained for 5 min under continuous stirring, followed by desorption for 5 min at 250°C in the injection port of the gas chromatograph Agilent 6890N series coupled to a mass spectrometer Agilent 5973 Network. Compounds were separated with an HP-Plot/Q column 30 m (0.32 mm diameter, 0.20 μm film thickness), using He as the gas carrier.
The mass spectrometer was operated in electron impact mode (70 eV) and spectra of standards and incubations were acquired in full scan mode (m/z 40-600, 2.6 s–1). Spectra of environmental samples were acquired by selective ion monitoring (SIM): acetate (m/z 43-45-60), propionate (m/z 28-45-74), isobutyrate (m/z 43-73-88), butyrate (m/z 41-60-73), isovalerate (m/z 43-60-87), valerate (m/z 41-60-73), and ethanol (m/z 31-45). An example of a peak ID is shown in Supplementary Figure 1.
Concentrations of the above compounds were determined using calibration curves (R2 > 0.998), with a VFA mixture (Supelco 46975-U), and ethanol (HPLC grade, Fisher) added to artificial seawater in the range 5 nmol L–1 to 1 μmol L–1 of acetate, isobutyrate and ethanol (Fisher HPLC grade). Concentrations of VFA and ethanol in the gas phase were calculated as CHS = Cap/[K + (VHS/VS)] with the partition coefficient K = CAP/CHS (CHS is the concentration in the headspace, CAP is the concentration in the aqueous phase, and VHS and VS are headspace and sample volume, Slack et al., 2003). This resulted in partition coefficients K of 1.2 for acetate, 1 for isobutyrate, and 1.4 for ethanol.
HS– and CO2 were measured in incubation bottles by removing 500 μL of gas from the headspace of either incubation or ambient sample with a Hamilton gas-tight syringe. The gas sample was injected into the GC-MS and detected in SIM mode (m/z 32-33 and 34 for HS– and m/z 28 and 44 for CO2). CO2 was quantified by comparing sample chromatographic areas with those of a CH4 reference internal standard co-injected (1 ppm) as a surrogate (1750, NOAA). Henry’s Law was used to calculate aqueous concentrations from partial pressure. HS– is reported as percent of maximum abundance since we lacked an appropriate standard.
Detection limits were calculated from slopes of calibration curves, and residual standard deviations were derived from linear regressions of calibration curves (three times residual error times slope, Shrivastava and Gupta, 2011). Detection limits were 40 nmol L–1 for acetate, 10 nmol L–1 for isobutyrate, 50 nmol L–1 for ethanol, and 10 μmol L–1 for CO2.
Concentrations of DFAA from incubations, and from Station 18, were quantified as OPA-derivatized adducts (Lindroth and Mopper, 1979) with a Shimadzu LC-10AT HPLC coupled to a Shimadzu RF-10Axl fluorescence detector (set at excitation/emission of 340/450 nm), column oven CTO 10As and autosampler (Shimadzu SIL 10 ADvp). Aliquots of 600 μL sample, mixed with 400 μL methanol, were derivatized in the autosampler with 60 μL ortho-phthalaldehyde/2-mercaptoethanol reagent (OPT, Lindroth and Mopper, 1979) and 100 μL sodium acetate buffer 0.1 N, pH 5, and injected (50 μL) into the HPLC. Fifteen amino acids (asp, glu, ser, his, gly, thr, arg, ala, tyr, val, met, phe, ile, leu, lys) were separated using an Alltima C18 (5 μm, 250 × 4.6 mm) column kept at 40°C, with a mobile phase of 5% tetrahydrofuran in 25 mM sodium acetate and methanol, and at a flow rate of 1 mL min–1. A gradient of 25–30% methanol in 35 min, 30–50% in 7 min, 50–60% in 18 min, 60–100% in 12 min was used. Initial conditions were restored in 7 min, and the column was equilibrated for 10 min between injections. Amino acids were identified and quantified by comparison with chromatograms of a standard amino acid mix (Pierce 20088) run under the same conditions every 10 injections. The coefficient of variation for quantification of duplicate samples was 9.4%.
Particulate samples were acid-fumed to remove carbonate (Nieuwenhuize et al., 1994), dried at 60°C for 24 h, wrapped in tin capsules, and analyzed using continuous-flow isotope ratio mass spectrometry (IRMS, Finnigan Delta Plus) interfaced with an elemental analyzer Carlo Erba NC2500. Reproducibility of standard acetanilide was greater than 0.11% for 13C, and 0.005‰ for 15N. Isotope ratios were expressed as per mil (‰) deviations of isotopic values relative to PDB (13C) or atmospheric N2 (15N).
We examined chlorophyll-a estimates (monthly averages at 4 km resolution) from the Aqua Moderate-Resolution Imaging Spectroradiometer (MODIS) mission between November 2009 and January 2011, extracting data from the CoastWatch project1. Net primary production (NPP) estimates were made using the standard Vertically Generalized Production Model (Behrenfeld and Falkowski, 1997, Ocean Productivity Home Page2), previously validated for the upwelling ecosystem off central Chile (Testa et al., 2018). We analyzed satellite estimates within boundaries of 72°48′-73°30′ W, 36°6′-36°45′ S over the continental shelf surrounding Station 18 (Figure 1). Data were averaged every 8 days between 2009 and 2011 (at least 33% of pixels) at a spatial resolution of ca. 9 km and integrating to the depth of euphotic zone (11–51 m).
We calculated export flux of carbon through the photic zone by deriving an empirical relation (Supplementary Figure 2) based on simultaneous in situ determinations of gross primary production (GPP), and of the fraction of GPP sinking through 50 m depth at station 18 between April 2004 and May 2005. GPP experiments and drifting sediment trap deployments were conducted quasi-monthly from April 2004 to May 2005 (González et al., 2009).
Both homogeneity of variances (Levene test) and normality of variables (Shapiro–Wilk test) were not fulfilled; therefore, we also tested for significant differences among environmental data using the non-parametric Kruskal–Wallis ANOVA test, and differences among experimental results using the paired sample Wilcoxon Signed Rank test. Correlations were examined using Spearman R coefficients.
Estimates of Gibbs Energy (ΔG) values for proposed metabolic reactions in the suboxic water column were calculated using substrate and product activities, temperature, and pH resembling suboxic water column in spring at the sampling site. The following data and sources were used: temperature (10.2°C), O2 (8.4 μM), NO3– (25 μM) from the COPAS Center Oceanographic Time Series database (W Schneider, curator), acetate (430 nM, November 2010, 65 m, this study), dissolved free amino acids (100 nM, this study), glucose (90 nM, Sempéré et al., 2008), Fe2+aq (400 nM, Achterberg et al., 2001); Iron hydroxide Fe(III)s (50 μM, Schoemann et al., 1998), SO42– and HCO3– (28 and 2 mM, Pilson, 2012), N2 (389 μM, Craig et al., 1967), HS– (1 μM, Lavik et al., 2009; Schunck et al., 2013), and H2 (0.06 mM, Seiler and Schmidt, 1974). Thermodynamic calculations were carried out using Thermodyn© software (Damgaard and Hanselmann, 1991).
The microbial degradations of glucose, amino acids, and acetate were detected during laboratory incubations under excess concentration of substrates (millimolar range) in the absence of O2 (Figure 2). Production of acetate (from 0 to 1 mM), ethanol (from 0 to 2 mM), CO2 (from 0 to 4 mM), and H+ (pH from 8 to 5) indicated fermentation of glucose during the 7 days incubation period (Figure 2A). Fermentation of the amino acid mix containing alanine, leucine, threonine, phenylalanine, glutamic acid, and ornithine (decrease from ca. 60 mM to ca. 6 mM) resulted in the production of VFA, acetate, propionate, isobutyrate, butyrate, isovalerate, and valerate (from 0 to ca.7.5 mM) during 8 days of incubation (Figure 2B and Supplementary Figure 3).
Figure 2. Fermentation of glucose and amino acids during incubations. (A) Changes in concentration of acetate, ethanol, CO2, and H+ (measured as pH) during fermentation of glucose, (B) Decay of amino acid mixed substrate (alanine, leucine, threonine, phenylalanine, ornithine, glutamic acid), and changes in concentration of VFA (sum of acetate, propionate, isobutyrate, butyrate, isovalerate, and valerate), CO2 and H+ during amino acid fermentation. Anaerobic terminal oxidation of acetate. The decay of acetate and increase in concentration of CO2, Fe2+aq and HS– under induced conditions of (C) denitrification (excess NO3–, 60 mM), (D) dissimilative iron reduction [excess Fe(OH)3, 250 mM], and (E) dissimilative sulfate reduction (excess SO42–, 50 mM). Values shown represent the average ± standard deviation. Relative abundance of HS– is shown as percent of maximum production.
No decay of fermentation products (acetate, ethanol) was detected in 7 days of incubation likely due either to the absence of external electron acceptors for anaerobic oxidation in the culture media, the thermodynamically unfavorable conditions caused by buildup of fermentation products, or the slow growth rates of acetate consumers suggested by comparing decay of amino acids (90% in near 2 days, Figure 2B) with decay of acetate (90% in near 30 days, Figures 2C–E).
Microbial oxidation of acetate with excess NO3– (Figure 2C), Fe(OH)3 (Figure 2D), and SO42– as electron acceptors was detected in the inocula treatments of OMZ water incubated under anaerobic conditions (Figure 2E). In control incubations, decrease in acetate concentration was not detected (Supplementary Figure 3d) and CO2 was always below the detection limit. Estimated Gibbs Energy (ΔG) for fermentation of glucose and amino acids, and anaerobic oxidation of acetate with NO3–, Fe(OH)3, and SO42– were in the range of −73 to −3777 kJ mol–1 suggesting that these reactions were exergonic (Table 1).
Table 1. Summary of metabolic reactions and chemical equations in incubations of suboxic waters collected in November 2010 at Station 18 off Concepción.
Dissolved inorganic N anomalies (NO3– + NO2– + NH4+; N∗) showed the year-round extent of NO3– deficit in the area. Ranges of −40 to 5 ESSW, −5 to 25 ESPTW (summer) and −20 to −10 ESPTW (winter) (Supplementary Table 1 and Figure 3) were observed, with δ15N of particulate organic nitrogen (PON) enriched to values of 20–35‰ (ESSW); 10–15‰ (ESPTW summer); and 5–10‰ (ESPTW winter) (Supplementary Table 1 and Figure 3).
Figure 3. Potential temperature/salinity diagram from depth profiles taken at Station 18, off Concepción; data points are color coded (see color bar) for the following measurements (A) oxygen, (B) N∗, (C) NO3–, (D) acetate, (E) δ15N-PN. N∗ = [NO3– + NO2– + NH4+] – 16 [PO4+3] + 2.9 (Hansell et al., 2004), PN, particulate nitrogen. Rectangles indicate temperature and salinity ranges for Equatorial Subsurface Water (ESSW), and Eastern South Pacific Transition Water (ESPTW) in austral winter and summer. Dotted lines represent isopycnals as sigma-t and red line corresponds to sigma-t of 26.5 in the core of ESSW.
During the spring-summer, upwelling-favorable winds (southerlies) predominated in the study area (Figure 4A, positive values are indicative of upwelling favorable winds) and contributed to lift the 11°C isotherm, the oxycline, and more saline waters (34.4–34.6) to depths shallower than 30 m (Figures 4B–D). This near-surface layer above 30 m tends to gain heat at this time of year. During austral autumn-winter, downwelling favorable winds (northerlies) prevailed and were associated with the deepening of the 11–11.5°C isotherm, the 34.4–34.6 isohalines, and the oxycline (Figures 4A–D). In these seasons, the upper 30 m was diluted by the continental contribution from rivers (Figure 4C), as previously shown by Sobarzo et al. (2007). In particular, O2 concentrations in surface waters exceeded 150 μM throughout the year, with a hypoxic boundary layer of 22.5 μM O2 located at ca. 30 m depth in austral spring and summer, but below 70 m depth during winter (Figure 4D, white line). Anomalous bottom cooling (10–11°C) was observed during July 2010 in the absence of upwelling favorable winds (Figures 4A,B). Temporal and spatial distributions of hydrographic properties confirm the presence of two distinct water masses during the study period, with mid and bottom layers on the continental shelf occupied by ESSW with low preformed O2 concentration (<45 μM, ca. 1 mL L–1), high salinity (34.5) and low temperature <11.5°C. During austral spring-summer, the core of this water mass (indicated by isopycnal 26.5) rose to near 30 m depth (Figures 4B–D, white line) while during winter it was confined to 50–70 m depth.
Figure 4. Time series data from November 2009 through January 2011. (A) wind stress at Carriel Sur airport, Concepción (positive values represent upwelling-favorable winds); (B) temperature, (C) salinity, (D) concentration of O2 in the water column at Station 18 off Concepción. The white lines in (B,C) represent isopycnal of 26.5 in the core of ESSW and dotted line in (D) is the isoline of 22 μM O2 (ca. 0.5 mL O2 L–1, often considered the limit of suboxia).
The surface layer was dominated by ESPTW originating from Subantarctic Water (SAW) from the subantarctic front (Schneider et al., 2003), with cold and low salinity waters during austral winter and higher temperature and moderate salinity during austral summer (Figures 4B,C).
Chlorophyll-a concentration increased from ∼2 to >10 mg m–3 during spring and throughout the productive summer season (Figure 5A). Concentrations of POC and PN averaged 27 ± 46 mmol C m–3, and 4 ± 8 mmol N m–3, corresponding to an average C/N ratio of ∼ 7, with maximum values of C and N occurring during the productive season (Figures 5B,C). PO43– concentrations were higher below 30-m depth than in surface waters throughout the year, and a pronounced depletion of surface PO43– occurred in surface water during austral summer (Figure 5D). The annual cycle of NO3– generally followed expected patterns, with concentrations typically lower in near-surface waters, and higher in deeper waters. Concentrations were substantially higher throughout the water column (>10 μM) during austral winter and summer 2011, with a conspicuous depletion below 50-m depth, suggesting microbial consumption (Figure 5E). Satellite-based estimates of primary production varied from 30 mmol C m–2 d–1 (0.4 g C m–2 d–1) during winter to over 600 mmol C m–2 d–1 (7 g C m–2 d–1) during spring-summer (Figure 5F).
Figure 5. Time series data from November 2009 through January 2011 in the water column at Station 18 off Concepción; (A) chlorophyll-a, (B) particulate nitrogen, (C) particulate organic carbon, (D) phosphate concentration, (E) nitrate concentration, (F) rate of depth integrated net primary production (NPP).
Our sampling coverage for NH4+ concentrations were rather limited but showed accumulation of up to 2.5 μM NH4+ in both the deeper and subsurface layers during austral spring and summer (Figure 6A). A surface maximum of ca. 1 μM NH4+ was detected in the surface oxygenated ESPTW water mass, but otherwise, concentrations were below 0.5 μM (Figure 6A). NO2– concentrations were generally less than 1.5 μM NO2– throughout the water column, except for measurements of up to 7 μM during the productive season in austral summer in suboxic ESSW below 50 m depth (Figure 6B).
Figure 6. Time series data from November 2009 through January 2011 in the water column at Station 18 off Concepción; (A) ammonium, (B) nitrite, (C) Volatile Fatty Acids, VFA (D) Dissolved Free Amino Acids, DFAA. The white line represents isopycnal of 26.5 in the core of ESSW.
The concentrations of VFA (ca. 66% acetate, ca. 33% isobutyrate, and <1% isovalerate) ranged from 0.05 to 4 μM throughout the year, with higher concentrations observed under low O2 conditions below 50 m depth (Kruskal–Wallis Test, p < 0.05, N = 52, Figure 6C). VFA concentrations of over 2 μM were observed in the water column during suboxic conditions, peaking in austral fall 2009, austral spring 2010, and austral summer 2011. VFA correlated positively with NH4+ concentrations (Spearman r = 0.2, p < 0.05, N = 78) and negatively with dissolved O2 (Spearman r = −0.3, p < 0.05, N = 78) and temperature (Spearman r = −0.3, p < 0.05, N = 78). Concentrations of dissolved free amino acids (DFAA) reached up to ∼0.5 μM and generally decreased with depth during upwelling months (austral spring and summer). In contrast, concentrations of DFAA lower than 0.1 μM were observed throughout the water column in winter months (Figure 6D). Concentrations of DFAA and VFA were higher in ESSW during the upwelling season than in ESPTW during winter (Figures 6C,D).
In our experiments with excess concentrations of substrates (millimolar range), the fermentation of glucose produced acetate and ethanol, and the fermentation of DFAA, alanine, leucine, threonine, phenylalanine glutamic acid, and ornithine produced acetate, propionate, isobutyrate, butyrate, isovalerate and valerate. Along with sugars, DFAA are considered to be suitable substrates for microbial heterotrophic metabolism; this would explain why their concentration was maintained lower than 100 nM in the water column, since their removal rates are coupled to their production (Webb and Johannes, 1967; Crawford et al., 1974; Fuhrman, 1987). In the absence of O2, amino acids are known to undergo anaerobic degradation (Barker, 1981) as observed in our incubations of microbial assemblages from OMZ waters. Our observations are also consistent with the Stickland catabolic pathway (Stickland, 1934; Nisman, 1954; Barker, 1981), a process that couples anaerobic reduction and oxidation of amino acids and produces VFA, CO2, and NH4+. In Stickland fermentation, an electron-donating amino acid is oxidized to a VFA that is shorter by one carbon (Stickland, 1934; Nisman, 1954; Barker, 1981); thus, acetate can be produced by alanine oxidation, propionate by threonine, butyrate by glutamic acids, and valerate by leucine. An electron-accepting amino acid is reduced to a VFA of the same number of carbons (Stickland, 1934; Nisman, 1954; Barker, 1981) as in the reduction of threonine to butyrate, and of leucine to caproic acid. In the present study, incubation media remained mildly alkaline (Figure 2B), likely because of NH4+ formation during fermentation of amino acids (Prüss et al., 1994; Wolfe, 2005).
In our experiments under induced conditions of denitrification and dissimilative Fe(OH)3 and SO42– reductions, anaerobic terminal oxidation of acetate was detected together with CO2 production (Figures 2C–E and Table 1, reaction 4–6). This experimental evidence of thermodynamically feasible acetate oxidation – with Fe(OH)3 as an electron acceptor –reveals the potential ability of microbes to reduce solid-phase iron which is carried by rivers in the region, using the fermented metabolites produced in oxygen-depleted water. This process would release dissolved bioavailable Fe for utilization by phytoplankton and chemolithotrophic microbes (Segovia-Zavala et al., 2013), and metalloenzymes required for reduction of nitrate and nitrite (Milligan and Harrison, 2000). In support of this working hypothesis, bacterial reduction of solid-phase metals associated with oxidation of acetate has in fact been detected in the Baltic Sea (Berg et al., 2013). We suggest that our data provides evidence that acetate, and likely other VFA such as butyric acid, represents a principal intermediate of anaerobic metabolism in OMZ waters on the Concepción shelf.
Twice as much ethanol than acetate was produced during incubations of inocula from OMZ waters (Figure 2A, Wilcoxon Signed Rank test p < 0.05, n = 8), but ethanol was not detected in any sample from the water column. The observed production of ethanol could be an experimental artifact resulting in selection for ethanologenic copiotroph microorganisms, or because of acidification in enclosed vessels (which would normally be buffered to some extent by alkalinity in natural seawater) promoting microbial excretion of neutral molecules such as ethanol (Michels et al., 1979; Wolfe, 2005). These processes may explain the observed onset of ethanol production when pH begins to drop in the experimental vessels, preventing further decrease (Figure 2A). Alternatively, the absence of detectable ethanol could be a result of microbial consumption and fast turnover times in the water column, which would preclude its accumulation. In fact, the activity of the enzyme ethanol dehydrogenase has been previously detected in OMZ waters on the Concepción shelf (González and Quiñones, 2009), thus providing evidence the potential for ethanologenic fermentation triggered by the high concentration of organic carbon and reduced pH in these waters.
General hydrographic patterns detected during this study were consistent with previous research in this area (Schneider et al., 2003, 2017; Escribano and Schneider, 2007; Sobarzo et al., 2007; Letelier et al., 2009; Escribano and Morales, 2012). The surface layer was dominated by ESPTW which originates from Subantarctic Water (SAW) from the subantarctic front (Schneider et al., 2003), and which is characterized by cold and low salinity waters during austral winter and higher temperature and moderate salinity during austral summer. While ESPTW is transported northward along the rim of the Subtropical Gyre, ESSW is transported southward by the Peru-Chile Undercurrent off central Chile, constituting the water source for coastal upwelling (Sobarzo et al., 2007; Silva et al., 2009). The fundamental mechanisms proposed to explain seasonal variations in predominance of these two water masses on the continental shelf have been coastal upwelling, and downwelling induced by alongshore winds (Sobarzo et al., 2007; Schneider et al., 2017). Other processes, such as wind curl, coastally trapped waves, or topographic upwelling, have been less studied at these latitudes. These processes could explain a seemingly anomalous bottom cooling detected in July 2010 that could not be attributable to upwelling favorable wind.
As expected, upwelling favorable southerly winds resulted in a shallowing of the nutricline and upwelling of subsurface nutrient rich waters (up to ∼15 μM NO3– and ∼1.5 μM PO42–) closer to the surface, leading to the fertilization of the photic zone, as has been previously documented (Sobarzo et al., 2007; Farías et al., 2015). Satellite-based estimates of primary production during spring-summer 2009 and 2010 showed a three-fold increase in rates of primary production and significant increases in the concentrations of chlorophyll-a, POC and PN. The seasonal pattern of phytoplankton activity in surface waters, generally followed previously described annual patterns of primary production (e.g., Montero et al., 2007; Testa et al., 2018) during spring-summer 2009 and 2010. Sinking POC fluxes however did not follow the seasonal pattern of phytoplankton activity. Fluxes of POC sinking to 50-m depth averaged 23 ± 4 mmol C m–2 d–1 throughout the year, varying between 25 ± 3 mmol C m–2 d–1 during the upwelling seasons and 19 ± 1 mmol C m–2 d–1 in austral winter. Variation in sinking fluxes of POC at 50-m depth did not closely reflect the threefold increase in primary production observed in both water masses between winter and upwelling conditions. These sinking fluxes were therefore rather similar throughout the year (Psink upwelling ESSW/Psink winter ESPTW = 1.3), in good agreement with the low seasonal variability in export production previously detected (3–10% of surface waters PP production) for this area (González et al., 2009).
In a modeling study, Pizarro-Koch et al. (2019) concluded that the southern extension and seasonality of the OMZ at Station 18 is driven more by changes in the undercurrent transport and mesoscale eddy fluxes than by mixing and local input. Similar year-round sinking carbon fluxes reported here appear to support this conclusion.
At Station 18 off Concepción, elevated concentrations of VFA and NH4+ were concurrently observed (Figures 6A,C), suggesting fermentation of amino acids and production of NH4+ in the water column through the Stickland fermentation reaction. In the OMZ off Peru, the stoichiometric production of NH4+ from fermentation of amino acids could explain previously unaccounted NH4+ requirements of up to 17 mmol NH4+ m–2 d–1 for anammox bacteria (Lam et al., 2009). Our monthly field determinations in the water column off Concepción show that both VFA and NH4+ concurrently increased during November–March 2010 (austral spring-summer) whereas decay of VFA occurred during intrusion of oxygenated ESPTW in March–July (austral winter). In contrast, NH4+ was depleted much later in July–November (austral autumn), a likely consequence of a slow build-up of anaerobic NH4+ oxidizers (anammox) bacteria with relatively low growth rates (Kuenen, 2008; Kartal et al., 2013). Anammox reaction rates (ca. 0.4 nmol L–1 h–1, Canfield et al., 2010) are about 20 times lower than suboxic rates of degradation of amino acids (ca. 10 nmol L–1 h, Pantoja et al., 2009) in the OMZ off the northern Chilean coast, implicating that Stickland fermentation could be a source of NH4+ for anammox in OMZ waters.
Changes in water column biogeochemistry in OMZ waters are driven by the relative contribution of diverse metabolic processes, including reduction of NO3– (e.g., Naqvi et al., 2000; Bange et al., 2001; Farías et al., 2009; Dalsgaard et al., 2014), Fe(III) (e.g., Bruland, 2006; Moffett et al., 2007), and SO42– (e.g., Dugdale et al., 1977; Canfield et al., 2010), production of CH4 (e.g., Farías et al., 2009; Naqvi et al., 2010; Chronopoulou et al., 2017), and N2O (e.g., Cohen and Gordon, 1978; Farías et al., 2009; Naqvi et al., 2010; Kock et al., 2016), and anaerobic cycling of NO2– and NH4+ (e.g., Lipschultz et al., 1990; Molina et al., 2005; Lam et al., 2009; Fernandez and Farías, 2012). For reactions to occur, enzymatic hydrolysis of high molecular weight DOC (e.g., Hoppe et al., 1988; Chróst, 1990; Pantoja et al., 2009) by extracellular enzymes of marine bacteria (e.g., Arnosti, 2011; Arnosti et al., 2012) and fungi (Gutiérrez et al., 2011) produces low molecular weight substrates – such as amino acids and sugars – that are transported across cell-membranes (Weiss et al., 1991) and subsequently degraded. In the absence of metazoans in hypoxic waters (e.g., Vaquer-Sunyer and Duarte, 2008; Cavan et al., 2017), anaerobic microbes rely on low molecular weight organic substrates (acetate and other VFA) for dissimilative reduction of NO3–, Fe(III) and SO42– and for methanogenesis (Megonigal et al., 2004).
Dissolved inorganic N anomalies (NO3– + NO2– + NH4+; N∗) are probably associated with water column nitrogen cycle microaerophilic and anaerobic metabolism. N∗ showed a year-round NO3– deficit in the study area, with similar negative N∗ values that have been previously recorded in the area from 21 to 33oS (De Pol-Holz et al., 2009; Galán et al., 2014; Galán et al., 2017), and have been attributed to denitrification and anammox. Under conditions of NO3– depletion, 15N enrichment of remaining NO3– would be expected in these low O2 waters. Upwelled water with isotopically heavy NO3– may result in the enrichment of δ15N of particulate organic nitrogen (PON). This mechanism is consistent with δ15N-PON values of up to 35‰ found during this study. The similarity of the isotopic range of PON found in both OMZ and subantarctic water masses during the winter, can be explained by enhanced mixing of both water masses at that time of the year. Similar conclusions were reported by De Pol-Holz et al. (2009) analyzing δ15N-NO3– for this study area, and by Cline and Kaplan (1975), Liu and Kaplan (1989), Castro et al. (2001), and Sigman et al. (2003) for the California Current System.
Since OMZ waters (ESSW) are pushed offshore by SAW during austral winter, waters enriched in δ15N-PON and acetate, and with negative N∗, mix with the more oxygenated waters of subantarctic origin, thus imprinting a signal of anaerobic activity on ESPTW. Calculations using the mixing triangle shows that, even during austral winter, 50% of ESSW is located at depths as shallow as 50 m (the ESSW can be present at 25-m and above during austral summer). Concentrations of acetate (>1.5 μM) in ESSW were higher than in surface ESPTW, both during summer (0.01–0.8 μM) and winter (<0.2 μM). Mixing of water masses allows the byproducts of anaerobic metabolism to spread beyond the boundaries of OMZ source waters, thus providing labile and low molecular weight dissolved organics to surrounding oxygenated ESPTW.
Acetate, isobutyrate, and isovalerate were present at significant levels within the OMZ and surrounding waters as biomarkers for fermentative activity. Twice as much acetate (range 0.1–2.5 μM) was present in the water column during upwelling seasons than observed in winter (Kruskal–Wallis Test, p < 0.05, N = 52), and showed a similar trend to isobutyrate which ranged from 0.9–1.5 μM. Elevated concentrations of acetate (up to 10–60 μM) have been detected in the anoxic Black Sea (Mopper and Kieber, 1991; Albert et al., 1995), the Pettaquamscutt River Estuary (ca. 7 μM, Wu and Scranton, 1994), and at ca. 10 μM in anoxic waters of the Cariaco Basin (Ho et al., 2002). In more oxic waters, acetate concentrations have been detected in the range 0.01–2.4 μM at the Chemotaxis Dock in Woods Hole (Lee, 1992), surface waters of the Cariaco Basin (Ho et al., 2002), in Long Island Sound (Wu et al., 1997), and the Gulf of Mexico (Zhuang et al., 2019). Acetate concentrations in suboxic waters off Concepción were generally within the ranges in the oxic and anoxic waters described above, and were likely enhanced by higher rates of primary production (Figure 5F) than observed in the Cariaco Basin, Long Island Sound and the Gulf of Mexico. Higher concentration of isobutyrate observed off Concepción (up to 1.5 μM) – associated with anaerobic degradation of iso-leucine (Mueller-Harvey and John Parkes, 1987) – cannot be readily compared with those of other coastal environments because of lack of measurements in the water column. As a reference, Sansone and Martens (1982) measured iso-butyrate in the eutrophic sediments of Cape Lookout Bight within the range < 0.5–6 μmole per liter sediment, and concentrations of up to 8 μM have been determined in pore waters at several coastal sites (Finke et al., 2006).
Enhanced water column concentrations of VFA up to 4 μM were detected during austral spring, summer, and fall throughout the water column (dominated by ESSW, Supplementary Figures 4A,B and Figures 3, 6C). Intruding oxygenated ESPTW of subantarctic origin during winter contains up to 0.5 μM VFA that can be attributed to the fermentation of organic matter by ambient microbial assemblages within OMZ waters.
We identified several products of the main anaerobic microbial reactions (fermentation, denitrification, and reduction of FeOH3 and SO42–) during laboratory incubations of OMZ waters, and we related these products to the annual cycle in water column chemistry within the upwelling ecosystem on the continental margin off Concepción, central Chile. Degradation of organic matter in anoxic systems relies on fermentation products and these can then be transported to neighboring oxygenated waters as labile substrates for microbial respiration. Within OMZ waters, acetate produced by fermentation is a major substrate for SO42– reducers (Megonigal et al., 2004; Jørgensen and Kasten, 2006), and was shown to be active even in the presence of NO2– and NO3– (Canfield et al., 2010). The reduction of SO42–, with acetate as electron donor, is thermodynamically feasible in OMZ waters (Table 1) when the inhibitor HS– remains in low concentrations (Arndt et al., 2013). A group of Gammaproteobacteria affiliated to HS– oxidizers have been identified in OMZ waters off the Chilean coast (Canfield et al., 2010), and a SO42–-reducing microorganism has been isolated from the OMZ in Peruvian coastal waters (Finster and Kjeldsen, 2010); these observations provide support for the findings shown here.
Implications of HS–, SO42–, amino acids, and acetate cycling are evident in cycling of N and C in OMZ waters. Fermentation of amino acids could conceivably fuel the NH4+ requirements of anammox bacteria because degradation of organic matter by denitrification is insufficient to account for observed anammox rates in OMZ waters (Thamdrup et al., 2006; Lam et al., 2009). The potential production of NH4+ through Stickland fermentation of amino acids in the OMZ could have implications for our understanding of the biogeochemistry of C and N, and could elucidate sources of NH4+ for the microaerophilic NH4+ oxidizers Thaumarchaeota (Venter et al., 2004; Könneke et al., 2005; Francis et al., 2005) and anammox bacteria (Kuypers et al., 2005; Dalsgaard et al., 2005; Thamdrup et al., 2006); these microbes have been unexplored to date.
The datasets generated for this study are available on request to the corresponding author.
BS and SP-G initiated and planned the study. BS conducted the field campaigns. BS, SP-G, GD, HG, LF, AS, and NP provided the chemical and isotopic analyses. GT and MS provided the satellite and physical analyses. All the authors contributed to the data analysis and writing of the manuscript based on an initial version written by BS and SP-G.
This research was funded by the Center for Oceanographic Research COPAS Sur-Austral CONICYT PIA PFB31 and APOYO CCTE AFB170006, and the Gordon and Betty Moore Foundation (MI_LOCO Oregon_Concepción, grant # 1661). We thank the Hanse-Wissenschaftskolleg Delmenhorst, Germany, for sponsoring the “Marine Organic Biogeochemistry” workshop in April 2019. The workshop was funded by the Deutsche Forschungsgemeinschaft (DFG, German Research Foundation), grant # 422798570.
The authors declare that the research was conducted in the absence of any commercial or financial relationships that could be construed as a potential conflict of interest.
We acknowledge the support provided by the COPAS Oceanographic Time-Series Station 18 off Concepción maintained by the Center for Oceanographic Research in the eastern South Pacific (FONDAP COPAS Center). We would particularly thank Prof. Dr. Wolfgang Schneider for validating data used in this study. Prof. Dr. Donald Canfield is acknowledged for providing insightful comments to an earlier version of this manuscript, and Dr. David Crawford for valuable comments. We are also grateful to UdeC personnel Lilian Núñez, Víctor Acuña, and Eduardo Tejos of the Marine Organic Geochemistry Laboratory, as well as Captain Sergio Marileo and the crew of L/C Kay-Kay II for valuable help during sampling and laboratory work. BS acknowledges the support provided by a student fellowship from the Gordon and Betty Moore Foundation, and valuable insights on thermodynamics and microbial metabolism from Prof. Dr. Kurt Hanselmann and international graduate courses ECODIM (Concepción, Chile) and Geobiology (Santa Catalina Island, United States). GT acknowledges the supports provided by a student fellowship (CONICYT-PFCHA/Doctorado Nacional/2017-21170561). Figure 1 was produced using Ocean Data View software developed by Reiner Schlitzer (http://www.awi-bremerhaven.de/GEO/ODV).
The Supplementary Material for this article can be found online at: https://www.frontiersin.org/articles/10.3389/fmars.2020.00533/full#supplementary-material
Achterberg, E. P., Holland, T. W., Bowie, A. R., Mantoura, R. F. C., and Worsfold, P. J. (2001). Determination of iron in seawater. Anal. Chim. Acta 442, 1–14. doi: 10.1016/S0003-2670(01)01091-1
Albert, D. B., Taylor, C., and Martens, C. S. (1995). Sulfate reduction rates and low molecular weight fatty acid concentrations in the water column and surficial sediments of the Black Sea. Deep Sea Res. Part I 42, 1239–1260. doi: 10.1016/0967-0637(95)00042-5
Arndt, S., Jørgensen, B. B., LaRowe, D. E., Middelburg, J. J., Pancost, R. D., and Regnier, P. (2013). Quantifying the degradation of organic matter in marine sediments: a review and synthesis. Earth Sci. Rev. 123, 53–86. doi: 10.1016/j.earscirev.2013.02.008
Arnosti, C. (2011). Microbial extracellular enzymes and the marine carbon cycle. Annu. Rev. Mar. Sci. 3, 401–425. doi: 10.1146/annurev-marine-120709-142731
Arnosti, C., Fuchs, B. M., Amann, R., and Passow, U. (2012). Contrasting extracellular enzyme activities of particle-associated bacteria from distinct provinces of the north Atlantic Ocean. Front. Microbiol. 3:425. doi: 10.3389/fmicb.2012.00425
Bange, H. W., Rapsomanikis, S., and Andreae, M. O. (2001). Nitrous oxide cycling in the Arabian Sea. J. Geophys. Res. Oceans 106, 1053–1065. doi: 10.1029/1999JC000284
Barker, H. A. (1981). Amino acid degradation by anaerobic bacteria. Annu. Rev. Biochem. 50, 23–40. doi: 10.1146/annurev.bi.50.070181.000323
Behrenfeld, M. J., and Falkowski, P. G. (1997). Photosynthetic rates derived from satellite-based chlorophyll concentration. Limnol. Oceanogr. 42, 1–20. doi: 10.4319/lo.1997.42.1.0001
Bennett, E. M., Carpenter, S. R., and Caraco, N. F. (2001). Human impact on erodable phosphorus and eutrophication: a global perspective. BioScience 51, 227–234. doi: 10.1641/0006-35682001051[0227:hioepa]2.0.co;2
Berg, C., Beckmann, S., Jost, G., Labrenz, M., and Jürgens, K. (2013). Acetate-utilizing bacteria at an oxic-anoxic interface in the Baltic Sea. FEMS Microbiol. Ecol. 85, 251–261. doi: 10.1111/1574-6941.12114
Berner, R. A. (1982). Burial of organic carbon and pyrite sulfur in the modern ocean; its geochemical and environmental significance. Am. J. Sci. 282, 451–473. doi: 10.2475/ajs.282.4.451
Bourke, M. F., Marriott, P. J., Glud, R. N., Hasler-Sheetal, H., Kamalanathan, M., Beardall, J., et al. (2017). Metabolism in anoxic permeable sediments is dominated by eukaryotic dark fermentation. Nat. Geosci. 10, 30–35. doi: 10.1038/ngeo2843
Breitburg, D., Levin, L. A., Oschlies, A., Grégoire, M., Chavez, F. P., Conley, D. J., et al. (2018). Declining oxygen in the global ocean and coastal waters. Science 359:eaam7240. doi: 10.1126/science.aam7240
Bruland, K. W. (2006). A review of the chemistries of redox sensitive elements within suboxic zones of oxygen minimun regions. Gayana 70, 6–13. doi: 10.4067/s0717-65382006000300003
Canfield, D. E., Stewart, F. J., Thamdrup, B., De Brabandere, L., Dalsgaard, T., Delong, E. F., et al. (2010). A cryptic sulfur cycle in oxygen-minimum-zone waters off the Chilean coast. Science 30, 1375–1378. doi: 10.1126/science.1196889
Castro, C. G., Chavez, F. P., and Collins, C. A. (2001). Role of the California undercurrent in the export of denitrified waters from the eastern tropical North Pacific. Glob. Biogeochem. Cycles 15, 819–830. doi: 10.1029/2000GB001324
Cavan, E. L., Trimmer, M., Shelley, F., and Sanders, R. (2017). Remineralization of particulate organic carbon in an ocean oxygen minimum zone. Nat. Commun. 8:14847. doi: 10.1038/ncomms14847
Chronopoulou, P. M., Shelley, F., Pritchard, W. J., Maanoja, S. T., and Trimmer, M. (2017). Origin and fate of methane in the Eastern Tropical North Pacific oxygen minimum zone. ISME J. 11, 1386–1399. doi: 10.1038/ismej.2017.6
Chróst, R. J. (1990). “Microbial ectoenzymes in aquatic environments,” in Aquatic Microbial Ecology. Brock/Springer Series in Contemporary Bioscience, eds J. Overbeck and R. J. Chróst (New York, NY: Springer).
Cline, J. D., and Kaplan, I. R. (1975). Isotopic fractionation of dissolved nitrate during denitrification in the eastern tropical north pacific ocean. Mar. Chem. 3, 271–299. doi: 10.1016/0304-4203(75)90009-2
Codispoti, L. A., Brandes, J. A., Christensen, J. P., Devol, A. H., Naqvi, S. W. A., Paerl, H. W., et al. (2001). The oceanic fixed nitrogen and nitrous oxide budgets: moving targets as we enter the anthropocene? Sci. Mar. 65, 85–105. doi: 10.3989/scimar.2001.65s285
Cohen, Y., and Gordon, L. I. (1978). Nitrous oxide in the oxygen minimum of the eastern tropical North Pacific: evidence for its consumption during denitrification and possible mechanisms for its production. Deep Sea Res. 25, 509–524. doi: 10.1016/0146-6291(78)90640-9
Conrad, R., Bak, F., Seitz, H. J., Thebrath, B., Mayer, H. P., and Schütz, H. (1989). Hydrogen turnover by psychrotrophic homoacetogenic and mesophilic methanogenic bacteria in anoxic paddy soil and lake sediment. FEMS Microbiol. Lett. 62, 285–293. doi: 10.1016/0378-1097(89)90010-4
Craig, H., Weiss, R. F., and Clarke, W. B. (1967). Dissolved gases in the equatorial and south Pacific Ocean. J. Geophys. Res. 72, 6165–6181. doi: 10.1029/jz072i024p06165
Crawford, C. C., Hobbie, J. E., and Webb, K. L. (1974). The utilization of dissolved free amino acids by estuarine microorganisms. Ecology 55, 551–563. doi: 10.2307/1935146
Dalsgaard, T., Stewart, F. J., Thamdrup, B., De Brabandere, L., Revsbech, N. P., Ulloa, O., et al. (2014). Oxygen at nanomolar levels reversibly suppresses process rates and gene expression in anammox and denitrification in the oxygen minimum zone off Northern Chile. mBio 5:e01966-14. doi: 10.1128/mBio.01966-14
Dalsgaard, T., Thamdrup, B., and Canfield, D. E. (2005). Anaerobic ammonium oxidation (anammox) in the marine environment. Res. Microbiol. 156, 457–464. doi: 10.1016/j.resmic.2005.01.011
Damgaard, L. R., and Hanselmann, K. (1991). Thermodyn© -A spread sheet for the calculation of free reaction energies under actual conditions. Based on K.W. Hanselmann. Microbial energetics applied to waste repositories. Experientia 47, 645–687.
De Pol-Holz, R., Robinson, R. S., Hebbeln, D., Sigman, D. M., and Ulloa, O. (2009). Controls on sedimentary nitrogen isotopes along the Chile margin. Deep Sea Res. II Top. Stud. Oceanogr. 56, 1042–1054. doi: 10.1016/j.dsr2.2008.09.014
Diaz, R. J., and Rosenberg, R. (2008). Spreading dead zones and consequences for marine ecosystems. Science 321, 926–929. doi: 10.1126/science.1156401
Dugdale, R. C., Goering, J. J., Barber, R. T., Smith, R. L., and Packard, T. T. (1977). Denitrification and hydrogen sulfide in the Peru upwelling region during 1976. Deep Sea Res. 24, 601–608. doi: 10.1016/0146-6291(77)90530-6
Escribano, R., and Morales, C. E. (2012). Spatial and temporal scales of variability in the coastal upwelling and coastal transition zones off central-southern Chile (35-40°S). Prog. Oceanogr. 92, 1–7. doi: 10.1016/j.pocean.2011.07.019
Escribano, R., and Schneider, W. (2007). The structure and functioning of the coastal upwelling system off central/southern Chile. Prog. Oceanogr. 75, 343–347. doi: 10.1016/j.pocean.2007.08.020
Farías, L., Besoain, V., and García-Loyola, S. (2015). Presence of nitrous oxide hotspots in the coastal upwelling area off central Chile: an analysis of temporal variability based on ten years of a biogeochemical time series. Environ. Res. Lett. 10:044017. doi: 10.1088/1748-9326/10/4/044017
Farías, L., Fernández, C., Faúndez, J., Cornejo, M., and Alcaman, M. E. (2009). Chemolithoautotrophic production mediating the cycling of the greenhouse gases N2O and CH4 in an upwelling ecosystem. Biogeosciences 6, 3053–3069. doi: 10.5194/bg-6-3053-2009
Fernandez, C., and Farías, L. (2012). Assimilation and regeneration of inorganic nitrogen in a coastal upwelling system: ammonium and nitrate utilization. Mar. Ecol. Prog. Ser. 451, 1–14. doi: 10.3354/meps09683
Finke, N., and Jørgensen, B. B. (2008). Response of fermentation and sulfate reduction to experimental temperature changes in temperate and Arctic marine sediments. ISME J. 2, 815–829. doi: 10.1038/ismej.2008.20
Finke, N., Vandieken, V., and Jørgensen, B. B. (2006). Acetate, lactate, propionate, and isobutyrate as electron donors for iron and sulfate reduction in Arctic marine sediments, Svalbard. FEMS Microbiol. Ecol. 59, 10–22. doi: 10.1111/j.1574-6941.2006.00214.x
Finster, K. W., and Kjeldsen, K. U. (2010). Desulfovibrio oceani subsp. oceani sp. nov., subsp. nov. and Desulfovibrio oceani subsp. galateae subsp. nov., novel sulfate-reducing bacteria isolated from the oxygen minimum zone off the coast of Peru. Antonie van Leeuwenhoek 97, 221–229. doi: 10.1007/s10482-009-9403-y
Fossing, H., Gallardo, V. A., Jørgensen, B. B., Hüttel, M., Nielsen, L. P., Schulz, H., et al. (1995). Concentration and transport of nitrate by the mat-forming sulphur bacterium Thioploca. Nature 374, 713–715. doi: 10.1038/374713a0
Francis, C. A., Roberts, K. J., Beman, J. M., Santoro, A. E., and Oakley, B. B. (2005). Ubiquity and diversity of ammonia-oxidizing archaea in water columns and sediments of the ocean. Proc. Natl. Acad. Sci. U.S.A. 102, 14683–14688. doi: 10.1073/pnas.0506625102
Froelich, P. N., Klinkhammer, G. P., Bender, M. L., Luedtke, N. A., Heath, G. R., Cullen, D., et al. (1979). Early oxidation of organic matter in pelagic sediments of the eastern equatorial Atlantic: suboxic diagenesis. Geochim. Cosmochim. Acta 43, 1075–1090. doi: 10.1016/0016-7037(79)90095-4
Fuenzalida, R., Schneider, W., Garcés-Vargas, J., Bravo, L., and Lange, C. (2009). Vertical and horizontal extension of the oxygen minimum zone in the eastern South Pacific Ocean. Deep Sea Res. II Top. Stud. Oceanogr. 56, 992–1003. doi: 10.1016/j.dsr2.2008.11.001
Fuhrman, J. (1987). Close coupling between release and uptake of dissolved free amino acids in seawater studied by an isotope dilution approach. Mar. Ecol. Prog. Ser. 37, 45–52. doi: 10.3354/meps037045
Galán, A., Fauñdez, J., Thamdrup, B., Santibáñez, J. F., and Farías, L. (2014). Temporal dynamics of nitrogen loss in the coastal upwelling ecosystem off central chile: evidence of autotrophic denitrification through sulfide oxidation. Limnol. Oceanogr. 59, 1865–1878. doi: 10.4319/lo.2014.59.6.1865
Galán, A., Thamdrup, B., Saldías, G. S., and Farías, L. (2017). Vertical segregation among pathways mediating nitrogen loss (N2 and N2O production) across the oxygen gradient in a coastal upwelling ecosystem. Biogeosciences 14, 4795–4813. doi: 10.5194/bg-14-4795-2017
González, H. E., Daneri, G., Iriarte, J. L., Yannicelli, B., Menschel, E., Barría, C., et al. (2009). Carbon fluxes within the epipelagic zone of the Humboldt Current System off Chile: the significance of euphausiids and diatoms as key functional groups for the biological pump. Prog. Oceanogr. 83, 217–227. doi: 10.1016/j.pocean.2009.07.036
González, R. R., and Quiñones, R. A. (2009). Common catabolic enzyme patterns in a microplankton community of the Humboldt Current System off northern and central-south Chile: malate dehydrogenase activity as an index of water-column metabolism in an oxygen minimum zone. Deep Sea Res. II Top. Stud. Oceanogr. 56, 1095–1104. doi: 10.1016/j.dsr2.2008.09.012
Gruber, N., and Sarmiento, J. L. (1997). Global patterns of marine nitrogen fixation and denitrification. Glob. Biogeochem. Cycles 11, 235–266. doi: 10.1029/97GB00077
Gutiérrez, M. H., Pantoja, S., Tejos, E., and Quiñones, R. A. (2011). The role of fungi in processing marine organic matter in the upwelling ecosystem off Chile. Mar. Biol. 158, 205–219. doi: 10.1007/s00227-010-1552-z
Hansell, D. A., Bates, N. R., and Olson, D. B. (2004). Excess nitrate and nitrogen fixation in the North Atlantic Ocean. Mar. Chem. 84, 243–265. doi: 10.1016/j.marchem.2003.08.004
Helly, J. J., and Levin, L. A. (2004). Global distribution of naturally occurring marine hypoxia on continental margins. Deep Sea Res. 51, 1159–1168. doi: 10.1016/j.dsr.2004.03.009
Ho, T. Y., Scranton, M. I., Taylor, G. T., Varela, R., Thunell, R. C., and Muller-Karger, F. (2002). Acetate cycling in the water column of the Cariaco Basin: seasonal and vertical variability and implication for carbon cycling. Limnol. Oceanogr. 47, 1119–1128. doi: 10.4319/lo.2002.47.4.1119
Hoppe, H. G., Kim, S. J., and Gocke, K. (1988). Microbial decomposition in aquatic environments: combined process of extracellular enzyme activity and substrate uptake. Appl. Environ. Microbiol. 54, 784–790.
Howarth, R. W. (2008). Coastal nitrogen pollution: a review of sources and trends globally and regionally. Harmful Algae 8, 14–20. doi: 10.1016/j.hal.2008.08.015
Hungate, R. E. (1965). “Quantitative aspects of the rumen fermentations,” in Physiology of Digestion in the Ruminant, ed. R. W. Dougherty (Washington, D.C: Butterworths), 311–321.
Jansson, B. P. M., Malandrin, L., and Johansson, H. E. (2000). Cell cycle arrest in archaea by the hypusination inhibitor N1-guanyl- 1,7-diaminoheptane. J. Bacteriol. 182, 1158–1161. doi: 10.1128/JB.182.4.1158-1161.2000
Jørgensen, B. B. (1982). Mineralization of organic matter in the sea bed–the role of sulphate reduction. Nature 296, 643–645. doi: 10.1038/296643a0
Jørgensen, B. B., and Kasten, S. (2006). “Sulfur cycling and methane oxidation,” in Marine Geochemistry, eds H. D. Schulz and M. Zabel (Berlin: Springer), 271–309.
Kamykowski, D., and Zentara, S. J. (1990). Hypoxia in the world ocean as recorded in the historical data set. Deep Sea Res. A Oceanogr. Res. Pap. 37, 1861–1874. doi: 10.1016/0198-0149(90)90082-7
Kartal, B., De Almeida, N. M., Maalcke, W. J., Op den Camp, H. J. M., Jetten, M. S. M., and Keltjens, J. T. (2013). How to make a living from anaerobic ammonium oxidation. FEMS Microbiol. Rev. 37, 428–461. doi: 10.1111/1574-6976.12014
Kock, A., Arevalo-Martinez, D. L., Loscher, C. R., and Bange, H. W. (2016). Extreme N2O accumulation in the coastal oxygen minimum zone off Peru. Biogeosciences 13, 827–840. doi: 10.5194/bg-13-827-2016
Könneke, M., Bernhard, A. E., De La Torre, J. R., Walker, C. B., Waterbury, J. B., and Stahl, D. A. (2005). Isolation of an autotrophic ammonia-oxidizing marine archaeon. Nature 437, 543–546. doi: 10.1038/nature03911
Kuenen, J. G. (2008). Anammox bacteria: from discovery to application. Nat. Rev. Microbiol. 6, 320–326. doi: 10.1038/nrmicro1857
Kuypers, M. M. M., Lavik, G., Woebken, D., Schmid, M., Fuchs, B. M., Amann, R., et al. (2005). Massive nitrogen loss from the Benguela upwelling system through anaerobic ammonium oxidation. Proc. Natl. Acad. Sci. 102, 6478–6483. doi: 10.1073/pnas.0502088102
Lam, P., Lavik, G., Jensen, M. M., van de Vossenberg, J., Schmid, M., Woebken, D., et al. (2009). Revising the nitrogen cycle in the Peruvian oxygen minimum zone. Proc. Natl. Acad. Sci. 106, 4752–4757. doi: 10.1073/pnas.0812444106
Lavik, G., Stührmann, T., Brüchert, V., Van Der Plas, A., Mohrholz, V., Lam, P., et al. (2009). Detoxification of sulphidic African shelf waters by blooming chemolithotrophs. Nature 457, 581–584. doi: 10.1038/nature07588
Lee, C. (1992). Controls on organic carbon preservation: the use of stratified water bodies to compare intrinsic rates of decomposition in oxic and anoxic systems. Geochim. Cosmochim. Acta 56, 3323–3335. doi: 10.1016/0016-7037(92)90308-6
Letelier, J., Pizarro, O., and Nuñez, S. (2009). Seasonal variability of coastal upwelling and the upwelling front off central Chile. J. Geophys. Res. Oceans 114:C12009. doi: 10.1029/2008JC005171
Lindroth, P., and Mopper, K. (1979). High performance liquid chromatographic determination of subpicomole amounts of amino acids by precolumn fluorescence derivatization with o-phthaldialdehyde. Anal. Chem. 51, 1667–1674. doi: 10.1021/ac50047a019
Lipschultz, F., Wofsy, S. C., Ward, B. B., Codispoti, L. A., Friedrich, G., and Elkins, J. W. (1990). Bacterial transformations of inorganic nitrogen in the oxygen-deficient waters of the Eastern Tropical South Pacific Ocean. Deep Sea Res. A 37, 1513–1541. doi: 10.1016/0198-0149(90)90060-9
Liu, K. K., and Kaplan, I. R. (1989). The eastern tropical Pacific as a source of 15N-enriched nitrate in seawater off southern California. Limnol. Oceanogr. 34, 820–830. doi: 10.4319/lo.1989.34.5.0820
Löscher, C. R., Bange, H. W., Schmitz, R. A., Callbeck, C. M., Engel, A., Hauss, H., et al. (2016). Water column biogeochemistry of oxygen minimum zones in the eastern tropical North Atlantic and eastern tropical South Pacific oceans. Biogeosciences 13, 3585–3606. doi: 10.5194/bg-13-3585-2016
Lovley, D. (2006). “Dissimilatory Fe(III)- and Mn(IV)-Reducing prokaryotesin,” in The Prokaryotes, eds M. Dworkin, S. Falkow, E. Rosenberg, K.-H. Schleifer, and E. Stackebrandt (Berlin: Springer-Verlag), 287–308.
Megonigal, J. P., Mines, M. E., and Visscher, P. T. (2004). “Linkages to trace gases and aerobic processes,” in Biogeochemistry, ed. W. H. Schlesinger (Oxford, UK: Elsevier-Pergamon), 317–424.
Michels, P. A. M., Michels, J. P. J., Boonstra, J., and Konings, W. N. (1979). Generation of an electrochemical proton gradient in bacteria by the excretion of metabolic end products. FEMS Microbiol. Lett. 5, 357–364. doi: 10.1111/j.1574-6968.1979.tb03339.x
Milligan, A. J., and Harrison, P. J. (2000). Effects of non-steady-state iron limitation on nitrogen assimilatory enzymes in the marine diatom Thalassiosira weissflogii (Bacillariophyceae). J. Phycol. 36, 78–86. doi: 10.1046/j.1529-8817.2000.99013.x
Moffett, J. W., Goepfert, T. J., and Naqvi, S. W. A. (2007). Reduced iron associated with secondary nitrite maxima in the Arabian Sea. Deep Sea Res. I 54, 1341–1349. doi: 10.1016/j.dsr.2007.04.004
Molina, V., Farías, L., Eissler, Y., Cuevas, L. A., Morales, C. E., and Escribano, R. (2005). Ammonium cycling under a strong oxygen gradient associated with the Oxygen Minimum Zone off northern Chile (23°S). Mar. Ecol. Prog. Ser. 288, 35–43. doi: 10.3354/meps288035
Montero, P., Daneri, G., Cuevas, L. A., González, H. E., Jacob, B., Lizárraga, L., et al. (2007). Productivity cycles in the coastal upwelling area off Concepción: the importance of diatoms and bacterioplankton in the organic carbon flux. Prog. Oceanogr. 75, 518–530. doi: 10.1016/j.pocean.2007.08.013
Mopper, K., and Kieber, D. J. (1991). Distribution and biological turnover of dissolved organic compounds in the water column of the Black Sea. Deep Sea Res. A 38, 1021–1047. doi: 10.1016/s0198-0149(10)80022-6
Moser-Engeler, R., Udert, K. M., Wild, D., and Siegrist, H. (1998). Products from primary sludge fermentation and their suitability for nutrient removal. Water Sci. Technol. 38, 265–273. doi: 10.1016/S0273-1223(98)00411-9
Mueller-Harvey, I., and John Parkes, R. (1987). Measurement of volatile fatty acids in pore water from marine sediments by HPLC. Estua. Coast. Shelf Sci. 25, 567–579. doi: 10.1016/0272-7714(87)90115-6
Naqvi, S. W. A., Bange, H. W., Farías, L., Monteiro, P. M. S., Scranton, M. I., and Zhang, J. (2010). Marine hypoxia/anoxia as a source of CH 4 and N 2O. Biogeosciences 7, 2159–2190. doi: 10.5194/bg-7-2159-2010
Naqvi, S. W. A., Jayakumar, D. A., Narvekar, P. V., Naik, H., Sarma, V. V. S. S., D’Souza, W., et al. (2000). Increased marine production of N2O due to intensifying anoxia on the Indian continental shelf. Nature 408, 346–349. doi: 10.1038/35042551
Nieuwenhuize, J., Maas, Y. E. M., and Middelburg, J. J. (1994). Rapid analysis of organic carbon and nitrogen in particulate materials. Mar. Chem. 45, 217–224. doi: 10.1016/0304-4203(94)90005-1
Oremland, R. S. (1988). “Biogeochemistry of methanogenic bacteria,” in Biology of Anaerobic Microorganisms, ed. A. J. B. Zehnder (Hoboken, NJ: John Wiley & Sons), 641–705.
Oremland, R. S., and Capone, D. G. (1988). “Use of ‘Specific’ inhibitors in biogeochemistry and microbial ecology,” in Advances in Microbial Ecology, ed. K. C. Marshall (Boston, MA: Springer).
Oremland, R. S., and Polcin, S. (1982). Methanogenesis and sulfate reduction: competitive and noncompetitive substrates in estuarine sediments. Appl. Environ. Microbiol. 44, 1270–1276. doi: 10.1128/aem.44.6.1270-1276.1982
Pantoja, S., Rossel, P., Castro, R., Cuevas, L. A., Daneri, G., and Córdova, C. (2009). Microbial degradation rates of small peptides and amino acids in the oxygen minimum zone of Chilean coastal waters. Deep Sea Res. II Top. Stud. Oceanogr. 56, 1055–1062. doi: 10.1016/j.dsr2.2008.09.007
Parkes, R. J., Gibson, G. R., Mueller-Harvey, I., Buckingham, W. J., and Herbert, R. A. (1989). Determination of the substrates for sulphate-reducing bacteria within marine and esturaine sediments with different rates of sulphate reduction. Microbiology 135, 175–187. doi: 10.1099/00221287-135-1-175
Pilson, M. E. Q. (2012). An Introduction to the Chemistry of the Sea. Cambridge, MA: Cambridge University Press.
Pizarro-Koch, M., Pizarro, O., Dewitte, B., Montes, I., Ramos, M., Paulmier, A., et al. (2019). Seasonal variability of the southern tip of the oxygen minimum zone in the Eastern South Pacific (30°-38°S): a modeling study. J. Geophys. Res. Oceans 124, 8574–8604. doi: 10.1029/2019JC015201
Prüss, B. M., Nelms, J. M., Park, C., and Wolfe, A. J. (1994). Mutations in NADH: ubiquinone oxidoreductase of Escherichia coli affect growth on mixed amino acids. J. Bacteriol. 176, 2143–2150.
Rabalais, N., Cai, W.-J., Carstensen, J., Conley, D., Fry, B., Hu, X., et al. (2014). Eutrophication-driven deoxygenation in the Coastal Ocean. Oceanography 27, 172–183. doi: 10.5670/oceanog.2014.21
Sabine, J. R., and Johnson, B. C. (1964). Acetate metabolism in the ruminant. J. Biol. Chem. 239, 89–93.
Sansone, F. J., and Martens, C. S. (1981). Determination of volatile fatty acid turnover rates in organic-rich marine sediments. Mar. Chem. 10, 233–247. doi: 10.1016/0304-4203(81)90044-X
Sansone, F. J., and Martens, C. S. (1982). Volatile fatty acid cycling in organic-rich marine sediments. Geochim. Cosmochim. Acta 46, 1575–1589. doi: 10.1016/0016-7037(82)90315-5
Schlitzer, R. (2010). Ocean Data View Version 4.3.6. Bremerhaven: Alfred Wegener Institute. Available online at: http://odv.awi.de/
Schmidtko, S., Stramma, L., and Visbeck, M. (2017). Decline in global oceanic oxygen content during the past five decades. Nature 542, 335–339. doi: 10.1038/nature21399
Schmitz, R. A., Daniel, R., Deppenmeier, U., and Gottschalk, G. (2006). “The anaerobic way of life,” in The Prokaryotes, eds E. Rosenberg, E. F. DeLong, S. Lory, E. Stackebrandt, and F. Thompson (Berlin: Springer).
Schneider, W., Donoso, D., Garcés-Vargas, J., and Escribano, R. (2017). Water-column cooling and sea surface salinity increase in the upwelling region off central-south Chile driven by a poleward displacement of the South Pacific High. Prog. Oceanogr. 151, 38–48. doi: 10.1016/j.pocean.2016.11.004
Schneider, W., Fuenzalida, R., Garcés-Vargas, J., Bravo, L., and Lange, C. (2006). Vertical and horizontal extension of the oxygen minimum zone in the easterns south Pactific Ocean. Gayana 70(Suppl.1), 79–82. doi: 10.4067/s0717-65382006000300016
Schneider, W., Fuenzalida, R., Rodríguez-Rubio, E., Garcés-Vargas, J., and Bravo, L. (2003). Characteristics and formation of Eastern South Pacific intermediate water. Geophys. Res. Lett. 30:1581. doi: 10.1029/2003GL017086
Schoemann, V., De Baar, H. J. W., De Jong, J. T. M., and Lancelot, C. (1998). Effects of phytoplankton blooms on the cycling of manganese and iron in coastal waters. Limnol. Oceanogr. 43, 1427–1441. doi: 10.4319/lo.1998.43.7.1427
Schunck, H., Lavik, G., Desai, D. K., Großkopf, T., Kalvelage, T., Löscher, C. R., et al. (2013). Giant hydrogen sulfide plume in the oxygen minimum zone off peru supports chemolithoautotrophy. PLoS One 8:e68661. doi: 10.1371/journal.pone.0068661
Schwertmann, U., and Cornell, R. M. (1991). Iron Oxides in the Laboratory: Preparation and characterization. Weinheim: VCH.
Segovia-Zavala, J. A., Delgadillo-Hinojosa, F., Huerta-Díaz, M. Á, Muñoz-Barbosa, A., Galindo-Bect, M. S., Hernández-Ayón, J. M., et al. (2013). Concentración de hierro disuelto en la zona del mínimo de oxígeno frente al umbral de San Esteban, golfo de California. Ciencias Mar. 39, 231–237. doi: 10.7773/cm.v39i2.2199
Seiler, W., and Schmidt, U. (1974). “Dissolved nonconservative gases in seawater,” in The Sea, ed. E. D. Goldberg (New York, NY: Wiley lnterscience), 219–244.
Sempéré, R., Tedetti, M., Panagiotopoulos, C., Charrière, B., and Van Wambeke, F. (2008). Distribution and bacterial availability of dissolved neutral sugars in the South East Pacific. Biogeosciences 5, 1165–1173. doi: 10.5194/bg-5-1165-2008
Shaw, D. G., Alperin, M. J., Reeburgh, W. S., and McIntosh, D. J. (1984). Biogeochemistry of acetate in anoxic sediments of Skan Bay, Alaska. Geochim. Cosmochim. Acta 48, 1819–1825. doi: 10.1016/0016-7037(84)90035-8
Shrivastava, A., and Gupta, V. (2011). Methods for the determination of limit of detection and limit of quantitation of the analytical methods. Chron. Young Sci. 2, 15–21. doi: 10.4103/2229-5186.79345
Sigman, D. M., Robinson, R., Knapp, A. N., Van Geen, A., McCorkle, D. C., Brandes, J. A., et al. (2003). Distinguishing between water column and sedimentary denitrification in the Santa Barbara Basin using the stable isotopes of nitrate. Geochem. Geophys. Geosyst. 4:1040. doi: 10.1029/2002GC000384
Silva, N., Rojas, N., and Fedele, A. (2009). Water masses in the Humboldt Current system: properties, distribution, and the nitrate deficit as a chemical water mass tracer for Equatorial Subsurface Water off Chile. Deep Sea Res. II Top. Stud. Oceanogr. 56, 1004–1020.
Skyring, G. W. (1988). Acetate as the main energy substrate for the sulfate-reducing bacteria in Lake Eliza (South Australia) hypersaline sediments. FEMS Microbiol. Lett. 4, 87–93. doi: 10.1016/0378-1097(88)90016-X
Skyring, G. W., Chambers, L. A., and Bauld, J. (1983). Sulfate reduction in sediments colonized by cyanobacteria, spencer gulf, south australia. Mar. Freshw. Res. 34, 359–374. doi: 10.1071/MF9830359
Slack, G. C., Snow, N. H., and Kou, D. (2003). “Extraction of volatile organic compounds from solids and liquids,” in Sample Preparation Techniques in Analytical Chemistry, ed. S. Mitra (Canada: John Wiley & Sons).
Sobarzo, M., Bravo, L., Donoso, D., Garcés-Vargas, J., and Schneider, W. (2007). Coastal upwelling and seasonal cycles that influence the water column over the continental shelf off central Chile. Prog. Oceanogr. 75, 363–382. doi: 10.1016/j.pocean.2007.08.022
Sobarzo, M., Figueroa, M., and Djurfeldt, L. (2001). Upwelling of subsurface water into the rim of the Biobío submarine canyon as a response to surface winds. Continent. Shelf Res. 21, 279–299. doi: 10.1016/S0278-4343(00)00082-0
Sørensen, J., Christensen, D., and Jørgensen, B. B. (1981). Volatile fatty acids and hydrogen as substrates for sulfate-reducing bacteria in anaerobic marine sediment. Appl. Environ. Microbiol. 42, 5–11. doi: 10.1128/aem.42.1.5-11.1981
Srain, B., Sepúlveda, J., Pantoja, S., Summons, R. E., Quiñones, R. A., and Levipan, H. A. (2015). Archaeal and bacterial assemblages in the Oxygen Minimum Zone of the upwelling ecosystem off Central Chile as determined by organic biomarkers. Gayana 79, 26–44. doi: 10.4067/s0717-65382015000100005
Stickland, L. H. (1934). The chemical reactions by which C. sporogenes obtains its energy. Biochem. J. 28, 1746–1759.
Suzuki, D., Ueki, A., Amaishi, A., and Ueki, K. (2007). Desulfobulbus japonicus sp. nov., a novel Gram-negative propionate-oxidizing, sulfate-reducing bacterium isolated from an estuarine sediment in Japan. Int. J. Syst. Evol. Microbiol. 57(Pt 4), 849–855. doi: 10.1099/ijs.0.64855-0
Testa, G., Masotti, I., and Farías, L. (2018). Temporal variability in net primary production in an upwelling area off Central Chile (36°S). Front. Mar. Sci. 5:179. doi: 10.3389/fmars.2018.00179
Thamdrup, B., Dalsgaard, T., Jensen, M. M., Ulloa, O., Farías, L., and Escribano, R. (2006). Anaerobic ammonium oxidation in the oxygen-deficient waters off northern Chile. Limnol. Oceanogr. 51, 2145–2156. doi: 10.4319/lo.2006.51.5.2145
Ulloa, O., Canfield, D. E., DeLong, E. F., Letelier, R. M., and Stewart, F. J. (2012). Microbial oceanography of anoxic oxygen minimum zones. Proc. Natl. Acad. Sci.U.S.A. 109, 15996–16003. doi: 10.1073/pnas.1205009109
Vaquer-Sunyer, R., and Duarte, C. M. (2008). Thresholds of hypoxia for marine biodiversity. Proc. Natl. Acad. Sci. U.S.A. 105, 15452–15457. doi: 10.1073/pnas.0803833105
Venter, J. C., Remington, K., Heidelberg, J. F., Halpern, A. L., Rusch, D., Eisen, J. A., et al. (2004). Environmental genome shotgun sequencing of the sargasso Sea. Science 304, 66–74. doi: 10.1126/science.1093857
Wagner, R. D., Warner, T., Roberts, L., Farmer, J., and Balish, E. (1997). Colonization of congenitally immunodeficient mice with probiotic bacteria. Infect. Immun. 65, 3345–3351. doi: 10.1128/iai.65.8.3345-3351.1997
Ward, B. B., Devol, A. H., Rich, J. J., Chang, B. X., Bulow, S. E., Naik, H., et al. (2009). Denitrification as the dominant nitrogen loss process in the Arabian Sea. Nature 461, 78–81. doi: 10.1038/nature08276
Webb, K. L., and Johannes, R. E. (1967). Studies of the release of dissolved free amino acids by marine zooplankton. Limnol. Oceanogr. 12, 376–382. doi: 10.4319/lo.1967.12.3.0376
Weiss, M. S., Abele, U., Weckesser, J., Welte, W., Schiltz, E., and Schulz, G. E. (1991). Molecular architecture and electrostatic properties of a bacterial porin. Science 254, 1627–1630. doi: 10.1126/science.1721242
Wolfe, A. J. (2005). The acetate switch. Microbiol. Mol. Biol. Rev. 69, 12–50. doi: 10.1128/mmbr.69.1.12-50.2005
Wolfe, R. S. (2011). Techniques for cultivating methanogens. Methods Enzymol. 494, 1–22. doi: 10.1016/B978-0-12-385112-3.00001-9
Wright, J. J., Konwar, K. M., and Hallam, S. J. (2012). Microbial ecology of expanding oxygen minimum zones. Nat. Rev. Microbiol. 10, 381–394. doi: 10.1038/nrmicro2778
Wu, H., Green, M., and Scranton, M. I. (1997). Acetate cycling in the water column and surface sediment of Long Island Sound following a bloom. Limnol. Oceanogr. 42, 705–713. doi: 10.4319/lo.1997.42.4.0705
Wu, H., and Scranton, M. I. (1994). Cycling of some low molecular weight volatile fatty acids in a permanently anoxic estuarine basin. Mar. Chem. 47, 97–113. doi: 10.1016/0304-4203(94)90102-3
Wyrtki, K. (1962). The oxygen minima in relation to ocean circulation. Deep Sea Res. Oceanogr. Abstr. 9, 11–23. doi: 10.1016/0011-7471(62)90243-7
Zhuang, G. C., Peña-Montenegro, T. D., Montgomery, A., Montoya, J. P., and Joye, S. B. (2019). Significance of acetate as a microbial carbon and energy source in the water column of gulf of Mexico: implications for marine carbon cycling. Glob. Biogeochem. Cycles 33, 223–235. doi: 10.1029/2018GB006129
Keywords: fermentation, oxygen minimum zone, anaerobic respiration, volatile fatty acids, acetate, Chile, South East Pacific
Citation: Srain BM, Sobarzo M, Daneri G, González HE, Testa G, Farías L, Schwarz A, Pérez N and Pantoja-Gutiérrez S (2020) Fermentation and Anaerobic Oxidation of Organic Carbon in the Oxygen Minimum Zone of the Upwelling Ecosystem Off Concepción, in Central Chile. Front. Mar. Sci. 7:533. doi: 10.3389/fmars.2020.00533
Received: 05 August 2019; Accepted: 11 June 2020;
Published: 22 July 2020.
Edited by:
Stuart Wakeham, University of Georgia, United StatesReviewed by:
Doug LaRowe, University of Southern California, United StatesCopyright © 2020 Srain, Sobarzo, Daneri, González, Testa, Farías, Schwarz, Pérez and Pantoja-Gutiérrez. This is an open-access article distributed under the terms of the Creative Commons Attribution License (CC BY). The use, distribution or reproduction in other forums is permitted, provided the original author(s) and the copyright owner(s) are credited and that the original publication in this journal is cited, in accordance with accepted academic practice. No use, distribution or reproduction is permitted which does not comply with these terms.
*Correspondence: Silvio Pantoja-Gutiérrez, c3BhbnRvamFAdWRlYy5jbA==
†Present address: Norma Pérez, Departamento de Acuicultura, Universidad Católica del Norte, Coquimbo, Chile
Disclaimer: All claims expressed in this article are solely those of the authors and do not necessarily represent those of their affiliated organizations, or those of the publisher, the editors and the reviewers. Any product that may be evaluated in this article or claim that may be made by its manufacturer is not guaranteed or endorsed by the publisher.
Research integrity at Frontiers
Learn more about the work of our research integrity team to safeguard the quality of each article we publish.