- 1College of Life and Environmental Sciences, Wenzhou University, Wenzhou, China
- 2College of Education, Wenzhou University, Wenzhou, China
Cadmium (Cd), one of the most toxic metals found in inshore sediments of China, is a persistent environmental contaminant capable of exerting irreversible toxic effects on aquatic organisms and their associated ecosystems. Although Cd is known to be toxic to marine animals, the underlying mechanism of this toxicity is not clear. In this study, Meretrix meretrix, a commercially and ecologically important species of clam, was exposed to different concentrations of cadmium chloride (0, 1.5, 3, 6, and 12 mg L–1) for 5 days, and the levels of Cd accumulation, antioxidant enzyme activity, and expression of metallothionein (MT) in the hepatopancreas, gill, foot, and mantle were evaluated. The results revealed a sharp increase in Cd accumulation in the tissues in response to increased Cd2+ concentrations in the water, and significant differences in Cd accumulation were observed among the different tissues. Increased Cd2+ level in the tissues also led to a significant increase in malondialdehyde content, caused by increased lipid peroxidation. The activities of superoxide dismutase and catalase also increased, peaking at different Cd2+ concentrations, depending on the tissue. Glutathione peroxidase (GPx) activity in the gill and mantle initially increased but then decreased with increasing external Cd2+ concentration. In the hepatopancreas and foot, GPx activity was inhibited by Cd2+, even at low concentrations. Furthermore, Cd2+ also stimulated the expression of MT in all four tissues. However, the levels of reduced glutathione (GSH) and oxidized glutathione (GSSG) in the gill and mantle, as well as the GSH/GSSG ratios in all four tissues, decreased with increasing external Cd2+ concentrations. Taken together, the results suggested that in M. meretrix, response to the toxic effect of Cd2+ might occur through a combination of mechanisms, which involve both enhanced antioxidant enzyme activities and the ability to bind and sequester Cd2+ via cysteine-rich molecules such as GSH and MT, both of which would eventually lead to the reduction of heavy metal-induced oxidative stress.
Highlights
- Cd2+ accumulation in different tissue of M. meretrix is concentration dependent.
- Cd2+ induces a significant increase in MDA level in different M. meretrix tissues.
- MT response significantly correlates with Cd2+ bioaccumulation.
- CAT and SOD activities vary according to external Cd2+ concentrations.
- Antioxidant enzymes, GSH and MT, might act cooperatively against oxidative stress.
Introduction
Toxic metal pollution, which can seriously threaten the biological sustainability of coastal ecosystems, has now become a major problem for the aquatic environment (Liu et al., 2018). Among the toxic metals, cadmium (Cd) is considered to be one of the most dangerous, and it is usually found in marine environments (Liu et al., 2015; Gu et al., 2019). In normal water, the level of dissolved Cd generally ranges from 10 to 500 ng L–1, but levels that exceed 10 μg L–1 have also been recorded in some industrialized areas in China (Hu et al., 2011; Meng et al., 2013; Chan and Wang, 2018). Cadmium has a long half-life and can be bioaccumulated in organisms through the food chain. The accumulation of Cd within living aquatic animals would exert a wide range of toxicological effects on the various tissues of the animals and their human consumers (Liu et al., 2015; García-Navarro et al., 2017). Cadmium can induce the body to produce a large number of reactive oxygen species (ROS) (Sandbichler and Höckner, 2016; Jing et al., 2019). The ROS in turn can cause oxidative damage, which is often linked to lipid peroxidation, protein denaturation, and inactivation of enzymes as well as DNA replication errors (Chandurvelan et al., 2015; Xia et al., 2016; Lin et al., 2017). Malondialdehyde (MDA), a final product of the decomposition of lipid peroxidation, has recently been considered as a sensitive biomarker of oxidative damage. It can be used to determine the extent of lipid peroxidation and the accumulation of ROS in an organism (Liu and Wang, 2016; Lin et al., 2017; Haque et al., 2018).
In general, aquatic organisms have independently evolved an antioxidant defense mechanism to protect biomolecules from oxidative stress caused by ROS, in which both enzymatic antioxidants and non-enzymatic antioxidants are functionally involved (Lavradas et al., 2014; Xia et al., 2016; Cui et al., 2020). A clear understanding of the link between antioxidants’ defense systems and metal bioconcentrations may help to identify markers that can reflect the status of aquatic ecosystems (Haque et al., 2018). Superoxide dismutase (SOD) is considered to be the first line of defense against oxidative stress, since O2–⋅ is converted to O2 and H2O2, and H2O2 is subsequently converted to O2 and H2O by catalase (CAT) present in the peroxisomes or by glutathione peroxidase (GPx) present in the mitochondria and cytosol (Chen et al., 2019; Gu et al., 2019). Glutathione is an important sulfhydryl non-protein compound found in the tissue, where it is involved in the protection of cell membrane from lipid peroxidation by scavenging oxygen radicals (O2–⋅, H2O2, and OH). This function plays a key role in the complexation and detoxification of heavy metal ions (Lavradas et al., 2014; Bouzahouane et al., 2018; Chan and Wang, 2018). Glutathione can exist in a thiol-reduced state (GSH) or in an oxidized state (GSSG), which consists of two GSH molecules that are linked together by a disulfide bond (Fraternale et al., 2017). The content of GSH and GSH/GSSG ratio can act as an important indicator of the body’s antioxidant capacity (Nair et al., 2015). In the cytosol, hydroxyl (⋅OH) and superoxide (O2–⋅) radicals are preferably scavenged by metallothioneins (MTs), which are low-molecular-weight (6–7 kDa) cysteine-rich metal-binding proteins involved in multiple physiological activities, such as metal homeostasis and detoxification (Amiard et al., 2006; Min et al., 2016). Moreover, MT can protect cells from metal toxicity not only by acting as an oxyradical scavenger but also through metal-binding/release dynamics (Gu et al., 2019; Ohta et al., 2019). Metallothioneins can participate directly in antioxidative defenses, and the synthesis of MT mRNA is correlated with the extent of metal stress (Zhu et al., 2018; Chen et al., 2019; Gu et al., 2019). In addition, MT can be excreted from the cells and absorbed by the cells via a receptor-mediated mechanism, in which MT remains in an endocytotic compartment while the metal is transported to the cytosol (Amiard et al., 2006; Ohta et al., 2019).
Meretrix meretrix is a clam that is widely found along the coastal areas of China, and it is an economically and ecologically important bivalve mollusk. It has low-metabolism and high filter-feeding activity, and it lives mainly in sandy or muddy substrates in estuarine lower intertidal and shallow subtidal areas, and therefore it is vulnerable to environmental pollutants (Zhang et al., 2011; Xia et al., 2016). M. meretrix is known to have a strong capacity to accumulate metals (Zhang et al., 2011; Wang et al., 2013) and is therefore widely used as a biological tool for monitoring toxic metal pollution in the marine environment (Hamad et al., 2011; Meng et al., 2013; Liu et al., 2018). While research has focused on the effect of Cd2+ toxicity on mollusks, there is a lack of data with regard to the effect of Cd2+ exposure on MT and other antioxidants in the different tissues of the clam. Our objectives were to determine the toxicological and biochemical responses of M. meretrix to acute Cd exposure and to investigate the bioaccumulation of Cd in different tissues as a function of exposure concentration. This could allow us to better understand how the antioxidant defense system of these bivalves might respond to Cd2+ stress. Furthermore, the relationships between Cd accumulation in the tissues and MT as well as the antioxidants examined were also investigated. Our findings highlight the potential application of Cd toxicity-associated biomarkers in clam in the future selection of biomarkers and bioindicator species for aquatic environmental monitoring.
Materials and Methods
Animals and Treatments
Meretrix meretrix clams (shell length, 64.36 ± 2.85 mm; shell width, 31.69 ± 1.42 mm; shell height, 53.57 ± 2.45 mm; weight, 71.30 ± 8.28 g) were purchased from Linkun aquafarm, Wenzhou, Zhejiang, China. The clams were acclimated in 15‰ artificial seawater without food for 2 days in an auto-temperature-controlled aquarium at 22 ± 1°C and pH 8.0. After acclimation, the clams were randomly assigned to five groups: a control group and four CdCl2-treated groups. Each group consisted of 120 clams, and these were kept in 3 separate aquariums, with 40 clams per aquarium. For Cd2+ treatment, the clams were placed in an aquarium containing a different Cd2+ concentration (1.5, 3, 6, or 12 mg L–1) for 5 days. The Cd2+ solutions were prepared in 15‰ artificial before being added to the aquariums. These concentrations of Cd2+ corresponded to 1/10, 1/5, 1/2.5, and 1/1.25 of the previously determined LC50 (15.01 mg L–1) (Xia et al., 2016). To maintain the quality of the water and the concentration of Cd2+ in the water, the water in the tank was replaced daily with 15‰ fresh artificial seawater containing the same Cd2+ concentration and pH as the original water. All other conditions were kept the same as during acclimation. After 5 days of exposure, all clams were collected and the mantle, gill, foot, and hepatopancreas of each animal were quickly removed and immediately frozen in liquid nitrogen before being stored at a −80°C freezer for further analysis.
Determination of Cadmium Content in Different Tissues of the Clam
Cadmium concentrations in the various tissues (mantle, gill, foot, and hepatopancreas) of the clams were measured according to Lin et al. (2017) and expressed as mg kg–1 wet weight tissue.
MT mRNA Expression Analysis
Total RNA was extracted from 20–40 mg of gill, mantle, foot, or hepatopancreas using a UNIQ-10 column TRIzol Total RNA Extraction Kit (Shanghai Shenggong, China) according to the manufacturer’s protocol. Briefly, the tissue sample was ground in TRIzol Reagent and then extracted with chloroform followed by centrifugation. After centrifugation, the upper aqueous layer was withdrawn and the RNA in it was precipitated with ethanol. The precipitated RNA was washed with PRE solution to remove the impurities and then resuspended in DPEC-distilled water and stored at −80°C for subsequent experiments. The RNA quality was evaluated by electrophoresis in 1.5% agarose gel (28S/18S RNA), and RNA concentration, as well as purity, was determined by spectrophotometry (NanoDrop 2000, Thermo Fisher Scientific, United States). cDNA was synthesized using a commercial cDNA synthesis kit (PrimeScriptTM RT Reagent, TaKaRa Bio. Inc., Dalian) according to the manufacturer’s instructions. Briefly, for reverse transcription, the reaction mixture contained 1 μg total RNA, 1 μL oligo dT Primer, 4 μL of 5 × PrimeScript Buffer (for Real Time), 1 μL PrimeScript RT enzyme mix, and RNase-free water in a final volume of 20 μL. The mixture was incubated at 37°C for 15 min and then heated at 85°C for 5 s to inactivate the reverse transcriptase. The cDNA product was stored at −80°C until use. The following gene-specific primers were used in the amplification: MT RT1f (forward) CGAGGACTGTTCATCAACCACTG, MT RT1r (reverse) GCAAACAACTTTACACCCTGGAC, β-actin1f (forward) TTGTCTGGTGGTTCAACTATG, and β-actin1r (reverse) TCCACATCTGCTGGAAGGTG (Wang et al., 2010). The expression of MT mRNA in different tissues was determined by quantitative real-time PCR (qRT-PCR) using a Roche LC480 fluorescent quantitative PCR (Switzerland) and AccuPower 2 × GreenStarTM qPCR Master Mix Kit (Bioneer Co., Shanghai, China) according to the manufacturer’s instructions. The qRT-PCR reaction mixture consisted of 2 μL of cDNA template, 10 μL of 2 × Green Master Mix, 0.4 μM of each forward and reverse primers, and 0.4 μL of 50 × ROX dye and DEPC-distilled water in a final volume of 20 μL. The PCR conditions were as follows: 5 min at 95°C, and 40 cycles of 15 s at 95°C, 15 s at 58°C, and 20 s at 72°C. The relative expression level of MT mRNA was determined by the 2–△△Ct method (Livak and Schmittgen, 2001).
Assays of SOD, CAT, and GPx Activities and MDA, GSH, and GSSG Contents
Frozen samples of different tissues were macerated on ice in 9 vols of 0.9% physiological saline using a SCIENTZ DY89-II type motor-driven homogenizer and then centrifuged at 1200 × g and 4°C for 15 min. Each supernatant was collected, and the activities of SOD, GPx, and CAT and the levels of MDA, GSH, and GSSG were then determined using a commercial kit according to the manufacturer’s instructions (Nanjing Jiancheng Biological Engineering Institute). Catalase activity was assayed spectrophotometrically by monitoring the decrease in absorbance at 240 nm due to H2O2 consumption (Saint-Denis et al., 1998). Superoxide dismutase activity was determined from the extent of inhibition of cytochrome C reduction at 550 nm (Beauchamp and Fridovich, 1971). Glutathione peroxidase activity was determined by detecting the rate of NADPH oxidation at 412 nm (Rotruck et al., 1973). All enzyme activities were defined as units of activity per mg of protein (U mg–1 protein). Thiobarbituric acid-reactive substance (TBARS) was expressed as MDA equivalents, and the absorbance of samples was read at 532 nm. Malondialdehyde level was expressed in nmol mg–1 protein (Ohkawa et al., 1979). Total glutathione (GSH plus GSSG) content was detected by a colorimetric method (5,5’-dithio-bis-2-nitrobenzoic acid, DTNB). The absorbance of the sample was measured at 405 nm using a microplate reader (Epoch, BioTek Co., United States). Oxidized glutathione was determined by the same method in the presence of 2-vinylpyridine, and GSH content was calculated from the difference between total glutathione and GSSG (Baker et al., 1990). Reduced glutathione and GSSG levels were expressed in mg g–1 protein. Soluble protein concentration was assayed by the method of Bradford (1976) using bovine serum albumin (BSA) as standard.
Statistical Analysis
Statistical analysis of the data was performed with the SPSS Statistical Package (Version 16.0, Chicago, IL, United States), and the regression analysis between GSH and MDA or GPx was performed using Statistica 6.0. Tukey’s multiple comparison test with one-way analysis of variance (ANOVA) was used to evaluate the differences among the treatment and control groups, and differences were considered statistically significant at the P < 0.05 level. The least significant difference test (LSD) was used to perform multiple comparisons among different Cd-treated groups.
Results
Cd2+ Accumulation in Different Tissues of Meretrix meretrix
Meretrix meretrix individuals that were exposed to Cd2+ all had significantly (P < 0.05) higher Cd2+ levels in their tissues than their non-exposed counterparts (controls) (Table 1). In the control group, there were significant differences among the different tissues except for the mantle and hepatopancreas. The gill was found to contain the highest Cd2+ concentration, followed by the foot, mantle, and hepatopancreas. As for all Cd2+-treated groups, the order was gill > mantle > foot > hepatopancreas, with significant differences among the different tissues from the same group, except for the hepatopancreas and foot in the group exposed to 12 mg L–1 Cd2+. The highest increase in tissue Cd2+ concentration between the control and Cd2+-exposed groups was found in the mantle (18,510%) and the lowest increase in the foot (7240%). Thus, the accumulation of Cd2+ in M. meretrix appeared to be tissue-specific.

Table 1. Accumulation of Cd2+ in different tissues of M. meretrix exposed to various Cd2+ concentrations in water.
Effects of Cd2+ on MDA Content
Meretrix meretrix individuals exposed to Cd2+ exhibited significant (P < 0.05) increase in MDA concentration compared with the non-exposed individuals, with the maximum concentration found in the group exposed to 12 mg L–1 Cd2+ (Figure 1). For the gill and mantle, significant differences were observed among groups exposed to different Cd2+ concentrations. The order of MDA content was gill > foot > mantle > hepatopancreas for the control group and gill > mantle > foot > hepatopancreas for the Cd2+-exposed groups. Thus, the levels of MDA in the various tissues of M. meretrix were consistent with the levels of Cd2+ in these tissues.
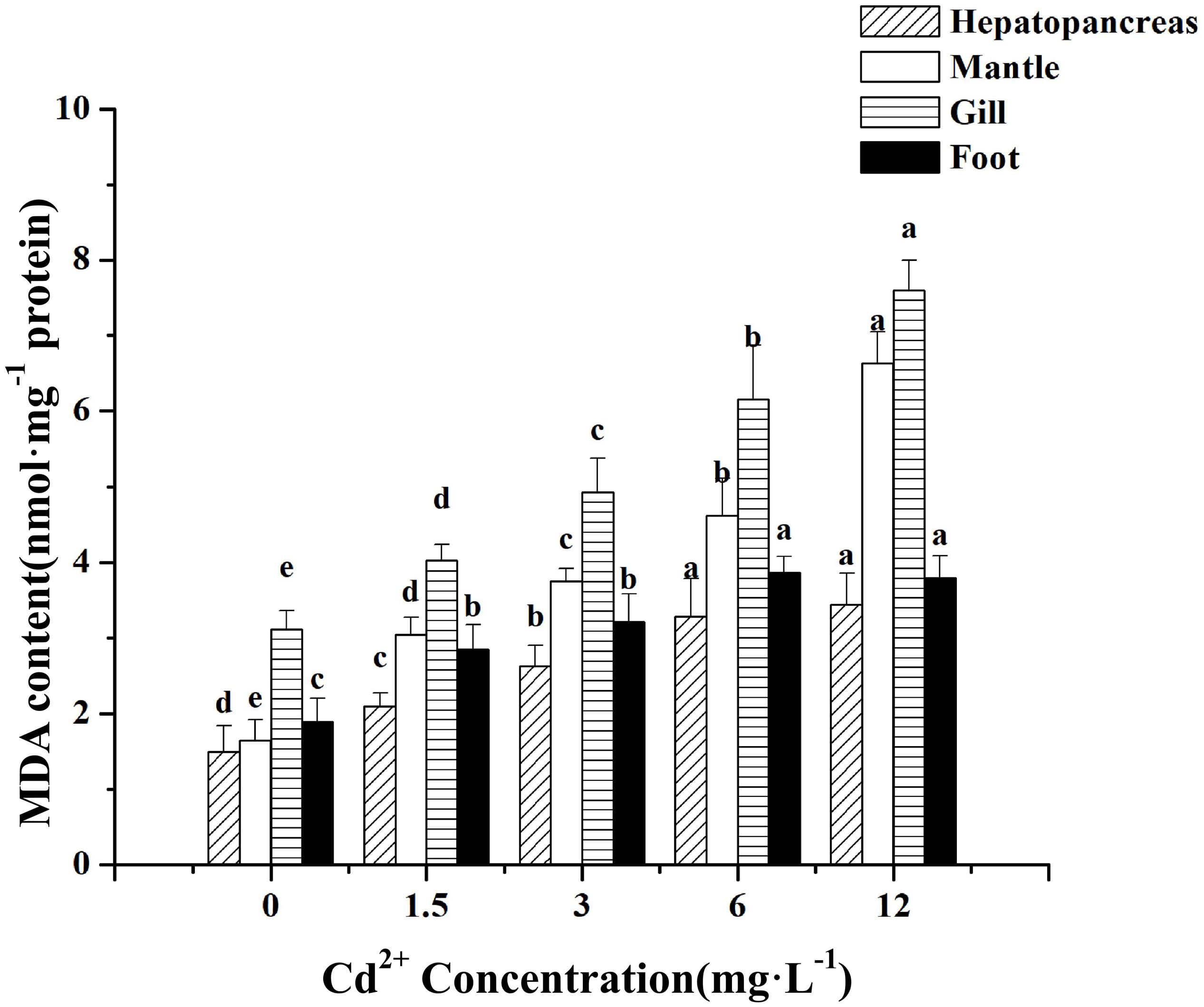
Figure 1. Effect of Cd 2+ on the levels of MDA in different tissues of M. meretrix following Cd2+ exposure. Hepatopancreas (twill pillars), mantle (white pillars), gill (horizontal stripes pillars), and foot (black pillars). Data are the means ± standard errors (SEs) (n = 6). Different letters above the pillars indicate significant (P < 0.05) differences among the different Cd2+-treated groups (0, 1.5, 3, 6, or 12 mg L–1) for the same tissue as revealed by Tukey’s post hoc multiple comparison tests.
Effect of Cd2+ on MT mRNA Expression Level
In general, the expression of MT mRNA in the various tissues of Cd2+-treated M. meretrix increased significantly compared with the control (P < 0.05) (Figure 2), but variations in the levels of increase among the tissues were obvious for the different groups. For example, at the lowest Cd2+ concentration, maximal MT mRNA level occurred in the gill and declined to the level of the control group at the highest Cd2+ concentration. However, at the two intermediate concentrations, the mantle exhibited the highest level of MT mRNA, whereas, at the highest Cd2+ concentration, the hepatopancreas exhibited the highest level of MT mRNA. The result appeared to suggest that lower concentrations of Cd2+ in these tissues could stimulate the expression of MT to enhance the binding of Cd2+ in the cell, but the stimulation seemed to be less profound at higher concentrations of Cd2+.
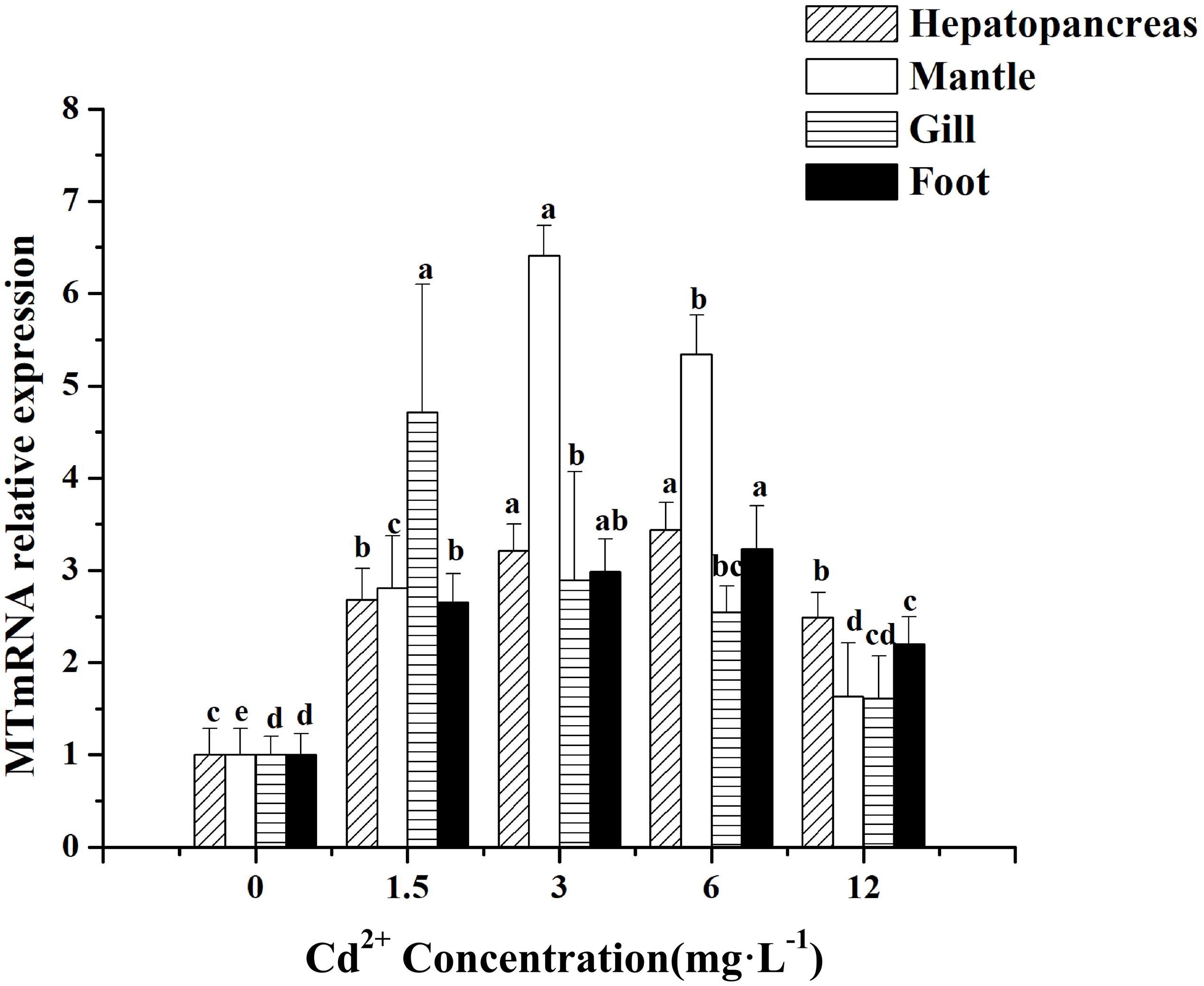
Figure 2. Effect of Cd2+ on MT expression in different tissues of M. meretrix following Cd2+ exposure. Hepatopancreas (twill pillars), mantle (white pillars), gill (horizontal stripes pillars), and foot (black pillars). Data are the means ± standard errors (SEs) (n = 6). Different letters above the pillars indicate significant (P < 0.05) differences among the different Cd2+-treated groups (0, 1.5, 3, 6, or 12 mg L–1) for the same tissue as revealed by Tukey’s post hoc multiple comparison tests.
Effects of Cd2+ on CAT, SOD, and GPx Activities
Among the four tissues tested, the hepatopancreas was found to have the highest level of CAT activity, followed by the gill, mantle, and foot, both for the control and Cd2+-exposed groups (Figure 3A). Significant increases in CAT activity were observed in the hepatopancreas and foot of the groups exposed to 1.5 and 3 mg L–1 Cd2+ compared with the control group (P < 0.05), but at higher Cd2+ concentrations (6 and 12 mg L–1), no significant increase in CAT activity was observed. No significant difference in CAT activity was detected in the mantle between the control group and any of the four Cd2+-exposed groups (P > 0.05) (Figure 3A). CAT activity in the gills of the group exposed to 1.5 mg L–1 Cd2+ increased significantly compared with the control group, whereas at higher Cd2+ concentration, no significant increase in CAT activity relative to the control group was observed.
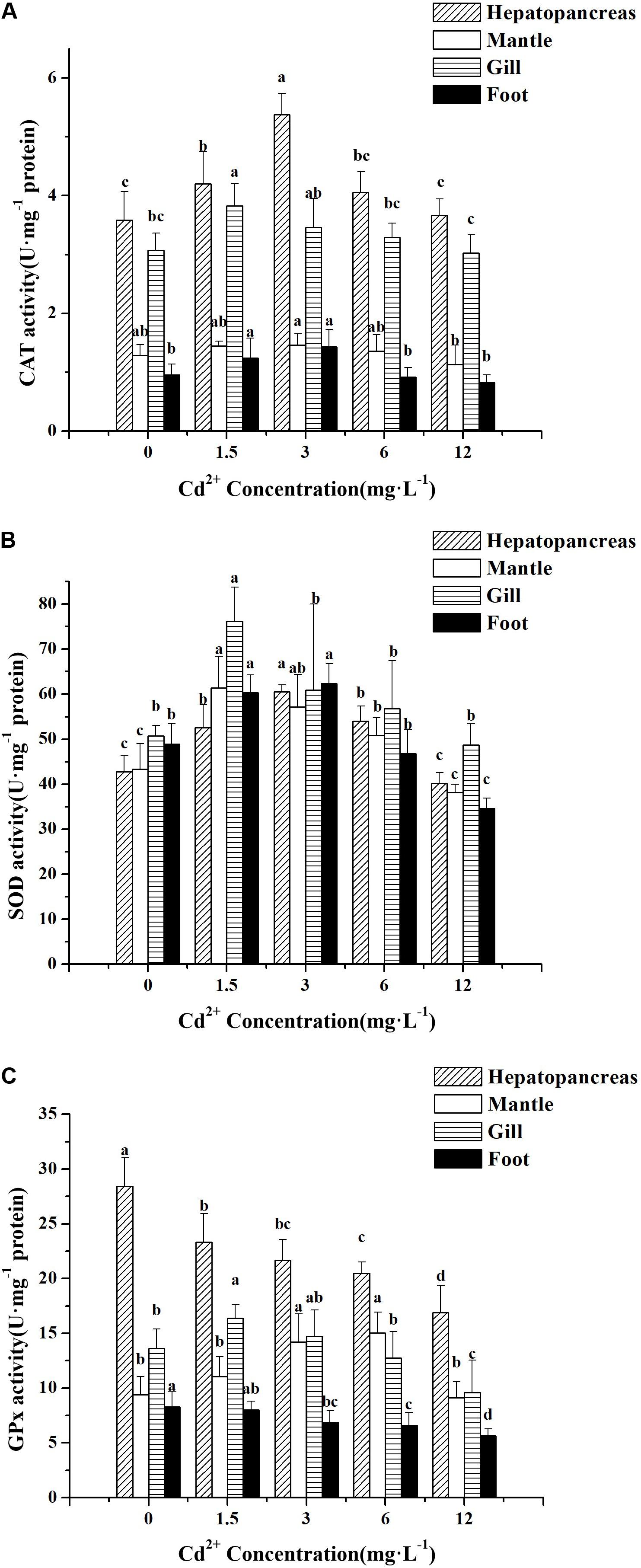
Figure 3. Effect of Cd2+ on the activities of antioxidant enzymes in different tissues of M. meretrix following Cd2+ exposure. Hepatopancreas (twill pillars), mantle (white pillars), gill (horizontal stripes pillars), and foot (black pillars). (A) CAT, (B) SOD, and (C) GPx. Data are the means ± standard errors (SEs) (n = 6). Different letters above the pillars indicate significant (P < 0.05) differences among the different Cd2+-treated groups (0, 1.5, 3, 6, or 12 mg L–1) for the same tissue as revealed by Tukey’s post hoc multiple comparison tests.
Increases in SOD activity over the control group were significant for all four tissues in the groups exposed to 1.5 and 3 mg L–1 Cd2+, with the gill displaying the highest increase (Figure 3B). Lower SOD activity was found in all the four tissues of the group exposed to 12 mg L–1 Cd2+ compared with all the other Cd2+ groups. Furthermore, no significant difference between the SOD activity of this group and that of the control group was observed except for the activity in the foot (Figure 3B).
As for GPx activity, Cd2+ had both enhancing and inhibitory effects, depending on the tissue. In the case of the hepatopancreas and foot, Cd2+ treatment led to a drop in GPx activity compared with the control, whereas in the gill and mantle, GPx activity tended to increase at lower Cd2+ concentrations, which then decreased at higher Cd2+ concentrations (Figure 3C). In the hepatopancreas, significant decreases in GPx activity relative to the control were also observed for all the Cd2+-exposed groups. As for the other three tissues, not all the differences in GPx activity between the control and Cd2+-exposed groups were significant.
Taken together, the results suggested that exposure of M. meretrix to Cd2+ could significantly alter the levels of antioxidant activity, but the effect on individual tissues might vary depending on the exact tissue.
Effects of Cd2+ on GSH and GSSG Content
The levels of GSH in the hepatopancreas and foot were increased at lower Cd2+ concentrations but decreased at higher Cd2+ concentrations compared with the control group. A maximum level of GSH was found in the group exposed to 1.5 mg L–1 Cd2+, which was significantly different (P < 0.05) from the control group and other Cd2+-exposed groups (Figure 4A). As for the mantle and gill, the GSH level decreased significantly with increasing Cd2+ concentrations, and the highest GSH level was found in the control group, which differed significantly (P < 0.05) from all Cd2+-exposed groups. Similar to GSH level, changes in GSSG level in response to Cd2+ exposure followed the same trend, but the decreases observed at higher Cd2+ concentrations (3–12 mg L–1) were much smaller than those shown by GSH level (Figure 4B).
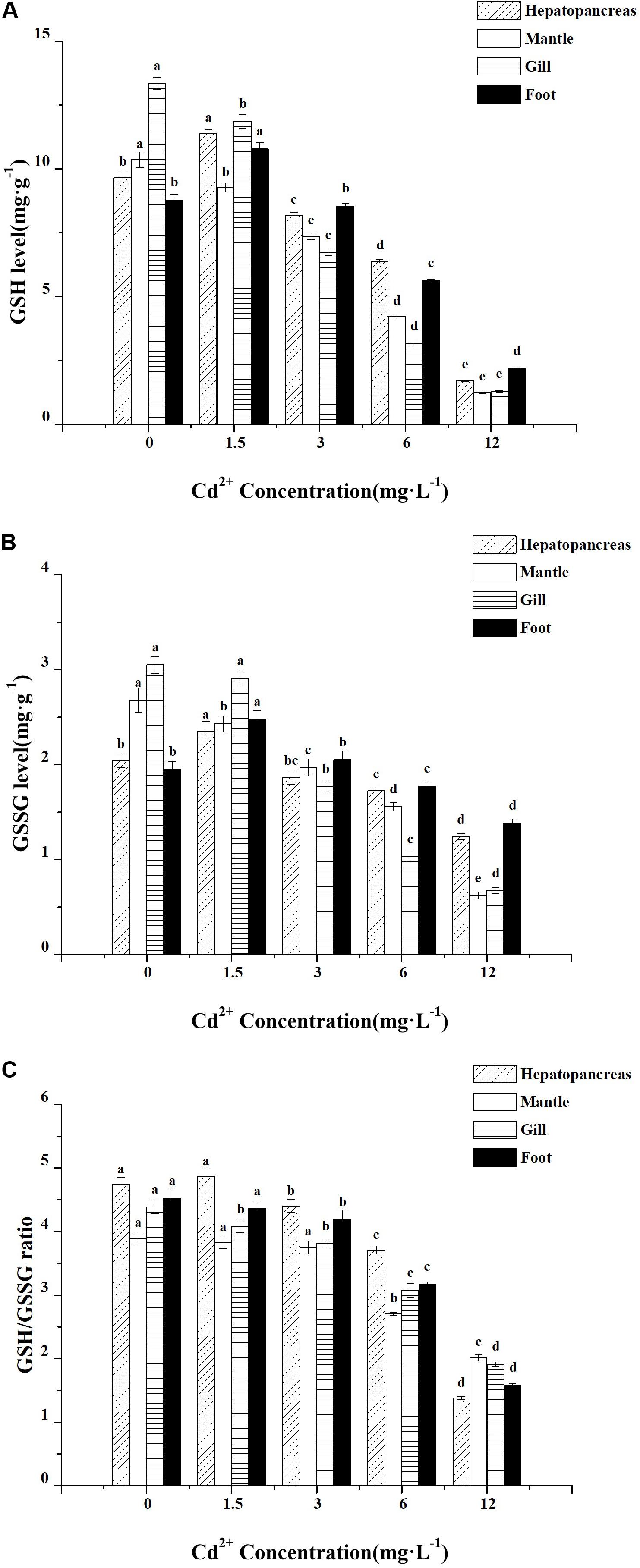
Figure 4. Effect of Cd2+ on the levels of glutathione in different tissues of M. meretrix following Cd2+ exposure. Hepatopancreas (twill pillars), mantle (white pillars), gill (horizontal stripes pillars), and foot (black pillars). (A) GSH, (B) GSSG, and (C) GSH/GSSG ratio. Data are the means ± standard errors (SEs) (n = 6). Different letters above the pillars indicate significant (P < 0.05) differences among the different Cd2+-treated groups (0, 1.5, 3, 6, or 12 mg L–1) for the same tissue as revealed by Tukey’s post hoc multiple comparison tests.
In the hepatopancreas and foot, there were no significant changes in GSH/GSSG ratio compared with the control group when the clams were exposed to low (1.5 mg L–1) Cd2+ concentration, but a dose-dependent decrease in GSH/GSSG ratio was observed in clams exposed to higher Cd2+ concentrations (3–12 mg L–1) (Figure 4C). The ratio of GSH/GSSG in the gill decreased continuously and reached the lowest level in the group exposed to the highest Cd2+ concentration (12 mg L–1). However, in the mantle, exposure to lower Cd2+ concentrations (1.5–3 mg L–1) did not result in significant changes in GSH/GSSG ratio, although exposure to higher Cd2+ concentrations (6 and 12 mg L–1) resulted in a marked reduction in GSH/GSSG ratio.
Thus, cadmium could lead to a general decrease in GSH and GSSG levels as well as GSH/GSSG ratio. However, the GSH level was most affected by higher Cd2+ concentrations (6–12 mg L–1).
Regression Analysis Between GSH and GPx or MDA
Regression analysis showed a significant negative correlation between the mean values of GSH and MDA found in the gill (Table 2, P = 0.005; Figure 5A, R2 = 0.947) and mantle (Table 2, P = 0.004; Figure 5B, R2 = 0.857). A significant positive correlation between GSH and GPx in the foot was observed (Table 2, P = 0.018; Figure 5C, R2 = 0.881). There was no significant correlation between GSH and the other oxidative stress indexes.
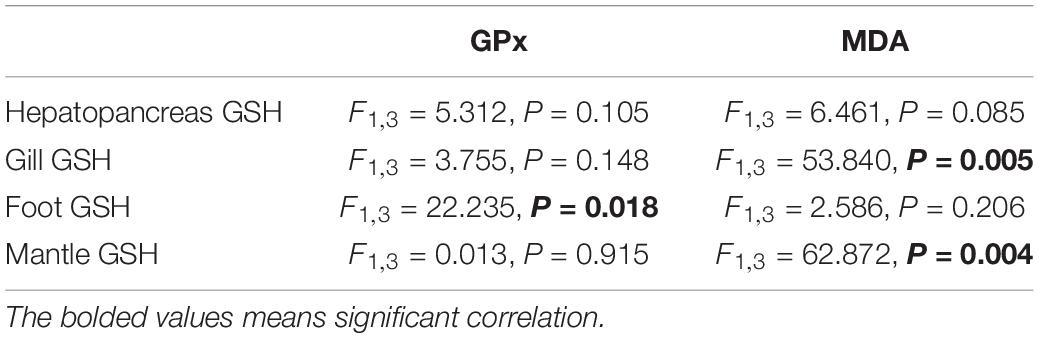
Table 2. Regression analysis of the mean data between GSH and GPx or MDA in different tissues of M. meretrix.
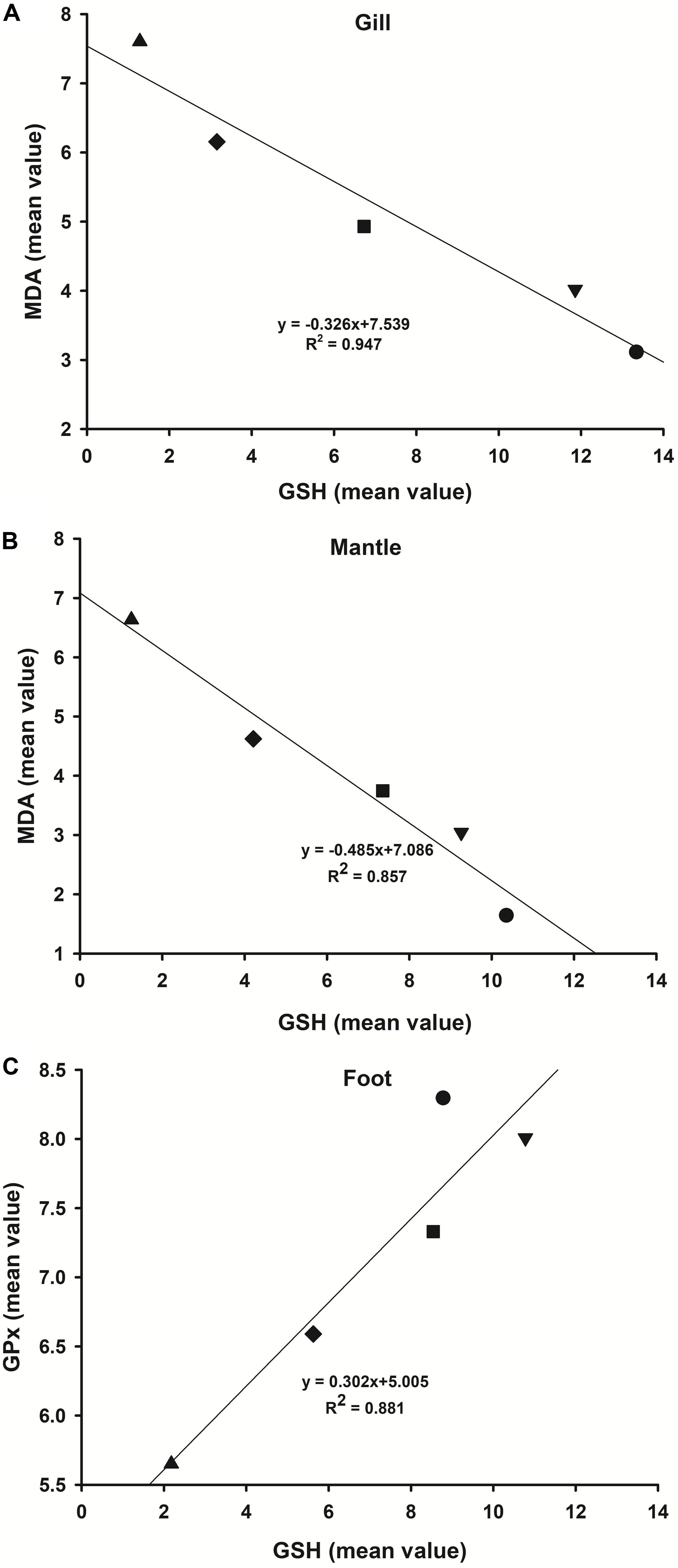
Figure 5. Regression analysis of the mean data of GSH and MDA or GPx in the various tissues of Cd2+-exposed M. meretrix. GSH and MDA in the gill (A) and mantle (B). GSH and GPx in the foot (C). Symbols in the plot denote control or one of the Cd2+-treated groups.
Discussion
Cd Bioaccumulation in Different Tissues
Among the benthic species, bivalves are characterized by their ability to accumulate very high levels of metals, which can reflect the bioavailability of metal contaminants in the water environments (Huo et al., 2012; Chan and Wang, 2018; Chen et al., 2020). Depending on whether the uptake is directly from the water or via the ingested food, the properties of the biological barriers such as the gills, mantle, and gut wall that separate the animals from their surrounding medium can lead to varying degrees of specific metal distribution in the different tissues (Huo et al., 2012; Marasinghe Wadige et al., 2017; Jing et al., 2019). The levels of Cd2+ accumulated in the four tissues of M. meretrix examined differed markedly, both within and among groups (Table 1), demonstrating that the accumulation of Cd2+ in M. meretrix was tissue-dependent. Similar to many aquatic animals, such as Tegillarca granosa (Huo et al., 2012), Hyridella australis (Marasinghe Wadige et al., 2017), and Eriocheir sinensis (Cheng et al., 2018), the gill was the major target for Cd2+ accumulation. As mentioned before, the gills are the primary target organ of metal contaminants and both as a site of metal uptake and as an important reservoir for metal storage, which could be one of the main causes of higher Cd bioaccumulation in the gill (Cheng et al., 2018; Zhen et al., 2018). Zhu et al. (2018) found that Cd can alter the gill morphology of Scylla paramamosain, which can result in osmoregulation dysfunction, lower oxygen uptake, and even hypoxic stress. Domouhtsidou and Dimitriadis (2000) found that toxic metals can cause the gill filaments of Mytilus gallolprovincialis Lamarck to rupture, thus affecting the transport of toxic metal to the other tissues. Zhen et al. (2018) found that Cd2+ can destroy the structure of gill epithelial cells in M. meretrix and deprive these cells of their normal physiological functions. The higher level of Cd2+ found in the gill of M. meretrix (Table 1) suggested that Cd might influence the functions of the gill, such as gas exchange, ion transport, waste excretion, osmoregulation, and transport of Cd2+ to other tissues. On the other hand, our data revealed significantly (P < 0.05) lower Cd2+ level in the hepatopancreas compared with the other tissues examined (Table 1). However, other investigators have reported different results for bivalves. For example, Wang et al. (2009) found the order of Cd2+ level in Patinopecten yessoensis raised in a typical mariculture area in northern China to be hepatopancreas > gill > sexual gland > mantle > muscle and suggested that it could be related to the induction of MT since the hepatopancreas is the main tissue for the synthesis of MT. In a different study, García-Navarro et al. (2017) demonstrated that Mytilus galloprovincialis exposed to Cd2+ for seven days exhibited a significantly higher Cd2+ concentration in the digestive gland than in the gill. However, Jing et al. (2019) found that Cd concentrations in the different tissues of Anodonta woodiana can display different orders when exposed to 0.168 mg L–1 Cd2+ (digestive gland > gills > mantle > visceral mass > foot) and to 0.675 mg L–1 Cd2+ (gills > digestive gland > mantle > visceral mass > foot) for 28 days. Additionally, Cd concentrations in the gills and digestive gland of the mussel Perna canaliculus were found to exhibit different orders as a result of different exposure methods (Chandurvelan et al., 2015). Metal accumulation in the tissues of aquatic organisms is dependent upon the exposure time, exposure method, and exposure dose as well as other factors, such as temperature, age, interaction with other metals, water chemistry, and the metabolic activity of the organism (Wang et al., 2009; Huo et al., 2012; Min et al., 2016; Jing et al., 2019). Thus, differences in Cd2+ accumulation level in the hepatopancreases, gill, mantle, and foot of M. meretrix may be due to the different physiological functions and responses of these tissues to acute toxicity. The higher levels of Cd2+ found in the gill, mantle, and foot compared with the hepatopancreases could be attributed to the location of these tissues, which is in direct contact with the external water. For example, the mantle has a relatively large contact area with water, allowing easier adsorption or passive diffusion of Cd from the water. This could have led to the highest increase of 18,510% in the mantle of the 12 mg L–1 Cd2+-exposed groups compared with the control group. Similar results have also been demonstrated for A. woodiana (Jing et al., 2019) and Mytilus edulis (Zhang et al., 2015). It has been demonstrated that shellfish can absorb both dissolved and particulate heavy metals. Dissolved metals are absorbed mainly by direct adsorption occurring on the animal surface (gill, mantle, and foot), while particulate metals tend to be absorbed more by the digestive organs along with the food (Chandurvelan et al., 2015; García-Navarro et al., 2017; Jing et al., 2019). Previous studies have shown that in invertebrates, Cd2+ concentration in the gill is higher than in the hepatopancreas following acute exposure (Huo et al., 2012; Zhang et al., 2015; Marasinghe Wadige et al., 2017), but in chronic exposure, the final concentration of Cd2+ in the gill was found to be lower than that in the digestive gland after its absorption, excretion, and transfer to other tissues (Chandurvelan et al., 2012; Jing et al., 2019). Jing et al. (2019) also suggested that Cd bioaccumulation in the gills could reflect short-term Cd pollution, and Cd bioaccumulation in the digestive glands might indicate longer Cd exposure. The higher concentrations of Cd2+ in the digestive gland or gill could be explained by the redistribution of cadmium among the tissues before its excretion (Zhang et al., 2015). Ghosh et al. (2020) found that the bioaccumulation process of metal in a specific tissue or organ of Lamellidens marginalis is temporary (gills, alimentary canal, and foot) and may be transported to the next tissue or organ in a series that may be the site of depuration or sequestration or excretion (anterior and posterior adductor muscles, hepatopancreas, and kidney). García-Navarro et al. (2017) suggested that Cd can quickly saturate the gill surface as a result of the direct contact and holding of Pb, Cd, and Cu, which would accelerate the distribution of Cd to the digestive gland.
Responses of Metallothionein and Other Biomarkers to Cd Exposure
The biomarker approach has been extensively employed as an indicator of early biological changes caused by toxic metal pollution (Chan and Wang, 2018). Several studies have shown that formation of oxygen free radicals or ROS would increase in response to toxic metal exposure, and these can act on membrane lipids, leading to lipid peroxidation (Sandbichler and Höckner, 2016; Zheng et al., 2016; Lin et al., 2017). The level of MDA, a secondary product of lipid peroxidation, is commonly used as an indicator of oxidative stress (Chan and Wang, 2018). The MDA levels in the different tissues of M. meretrix increased significantly with rising Cd2+ concentrations (Figure 1), indicating Cd2+-induced lipid peroxidation in the clam by raising the levels of ROS. Increased MDA level has been shown to result in the saturation of antioxidant systems, leading to oxidative damage (Lin et al., 2017; Haque et al., 2018; Cui et al., 2020). This is also consistent with the work reported by Xia et al. (2016), which shows that Cd2+ can induce significant apoptosis and oxidative stress in the hepatopancreas of M. meretrix, even at concentrations far below the LC50. An early event to prevent free Cd2+ from interfering with cellular metabolism is by chelation of Cd2+ via cysteine-rich molecules, such as MTs or GSH (Nair et al., 2015; Chan and Wang, 2018; Gu et al., 2019). Besides, the induction of antioxidant enzymes might also form an important protective mechanism for minimizing oxidative damage linked to pollution in the environments. These enzymes might also serve as important biomarkers for detecting toxic metal contaminants (Wang et al., 2010; Min et al., 2016; Chen et al., 2020).
Metallothioneins are non-enzymatic proteins that bind metals with their cysteine thiol groups (–SH), and this binding can detoxify toxic metals and regulate the metabolism of trace elements (Amiard et al., 2006; Zhu et al., 2018). Binding of Cd2+ to MT can lower the availability of free in vivo metal ions, thus controlling the intracellular levels of Cd2+ and reducing its toxicity (Lavradas et al., 2014; Chan and Wang, 2018). Cadmium is a known MT inducer, but sensitivity to Cd appears to vary depending on the particular tissue and the duration of exposure (Gu et al., 2019). In M. meretrix, the level of MT mRNA in different tissues could be induced by Cd2+ (Figure 2), suggesting that the increase in MT mRNA level could have led to more Cd2+ being sequestered by MT. The upregulation of MT genes by a low dose of Cd2+ appears to be mediated by a zinc-sensitive inhibitor that interacts with a constitutively active transcription factor (Amiard et al., 2006). Similar results have been obtained in previous studies, in which increase in MT mRNA level was found to occur in a dose- and duration-dependent manner in Charybdis japonica (Pan and Zhang, 2006), Mactra veneriformis (Fang et al., 2010), and E. sinensis (Chen et al., 2019). Gu et al. (2019) found that different durations of Cd2+ exposure led to differences in MT expression in the various tissues (ganglion, dorsal tissue, hepatopancreas, and abdominal tissue) of Onchidium reevesii and suggested that MT expression level can reveal a clear effect of heavy metal stress that is time- and Cd concentration-dependent. The expression of MT was significantly correlated with Cd2+ bioaccumulation in the tissues (Table 1, Figure 2), consistent with a role of Cd2+ detoxification for MT. However, such a positive correlation between MT expression and tissue Cd2+ accumulation only occurred up to a certain external Cd2+ concentration. This might be due to the physiological disturbance caused by the strong toxicity exerted by the high concentration of Cd2+ within the body, or that the concentration of Cd2+ in the body exceeded the capability of the MT-mediated detoxification pathway and started to affect the biosynthesis of MT mRNA. Many studies have reported that the synthesis of MT mRNA or MT protein is stimulated to deal with the increasing amount of ROS generated by the cadmium stress, suggesting that the MT gene could serve as a sensitive biomarker for Cd exposure and potentially other heavy metals (Cenov et al., 2018; Zhu et al., 2018). Previous studies looking at the induction of MT by toxic metals in O. reevesii (Gu et al., 2019) and E. sinensis (Chen et al., 2019) have also suggested that MT possibly plays a role in the scavenging of ROS generated in response to the oxidative damage induced by Cd, a phenomenon that is supported by our data. However, the regulatory mechanism of MT gene expression requires more in-depth study to better understand the roles of the MTs in Cd detoxification.
GSH, a major intracellular antioxidant in living organisms, can be used as an indicator to evaluate the free radical-scavenging capacity of an organism. It can serve as a potential biomarker for trace metals when coupled with lipid peroxidation (Chan and Wang, 2018). Reduced glutathione is involved in cellular protection by relieving the effect of oxidative stress (caused by heavy metals) through forming oxidized glutathione (GSSG) and metal complexes (Lavradas et al., 2014). Our data suggested that GSH might play a role in the initial response to Cd2+ stress by chelating with Cd2+ ions, as demonstrated by its decreased level at higher Cd2+ concentrations (Figure 4A). This is in agreement with previously published data, in which the accumulation of Cd2+ can form a GSH–metal complex, resulting in the depletion of cellular GSH (Nair et al., 2015; Xia et al., 2016; Yao et al., 2020). At higher Cd2+ concentrations (3–12 mg L–1), the significantly lower levels of GSH in the gill and mantle compared with the foot and hepatopancreas might indicate partial toxicity caused by Cd2+. Regression analysis also showed a significant negative correlation between the mean values of MDA and GSH in the gill and mantle (Figures 5A,B). This could mean that the antioxidant capacity might not be adequate to rid the system of the oxidative stress induced by Cd2+, consequently leading to redox imbalance in the cells (Dabas et al., 2014), as a concomitant increase in MDA amount was observed in both tissues (Figure 1). The lack of change observed for the GSH/GSSG ratio upon exposure to low Cd2+ concentration (1.5 mg L–1) (Figure 4C) suggested that adaptive processes might have been activated to maintain a state of redox balance. However, under high Cd2+ concentrations, a significant decrease in GSH/GSSG ratio occurred, and this could be the cause of high lipid peroxidation occurring in M. meretrix exposed to high Cd2+ concentration (Figure 1).
In mollusks, antioxidant enzymes also respond to excessive toxic metal stress by eliminating excessive ROS (Cong et al., 2012; Chan and Wang, 2018), and hence, antioxidant enzymes are also recognized as important biomarkers which can reveal the early effects of toxic metal contamination (Liu and Wang, 2016; Cui et al., 2020). Endogenous antioxidants such as SOD, CAT, and GPx are key components of the antioxidant defense system that protects organisms against oxidative stress (Xia et al., 2016; Lin et al., 2017; Chen et al., 2020). Our results showed that lower Cd2+ levels in the tissues of M. meretrix could activate the antioxidant protective mechanisms to cope with the potential oxidative stress inflicted by the increasing levels of O2–⋅ and H2O2, observations that have been reported by studies conducted with other mollusks (Chandurvelan et al., 2015; Liu and Wang, 2016; Yao et al., 2020). However, higher Cd2+ levels in the tissues might suppress SOD and CAT activities through the binding of Cd2+ to the active sites of these enzymes or the extra ROS generated could have reduced the scavenging capability of both SOD and CAT, ultimately leading to cytotoxicity (Xia et al., 2016; Cui et al., 2020). For GPx activity, a significant upregulation was observed in the gill and mantle at lower Cd2+ concentrations (Figure 3C), and this could mean that GPx probably participated in the active detoxification of Cd via various biochemical mechanisms, such as the decomposition of H2O2 to O2 and H2O or the conversion of lipid hydroperoxides into less toxic hydroxyl products. However, a significant downregulation of GPx activity was observed in the gill and mantle at higher Cd2+ concentrations and in the hepatopancreas and foot of all Cd2+-exposed groups. Glutathione peroxidase activity is dependent on the level of selenium, which might be different in the different tissues of M. meretrix. By binding to Cd2+, the active site (Se–Cys) of GPx is altered, and this might inactivate the enzyme (Loro et al., 2012; Xia et al., 2016). Regression analysis between GSH and GPx in the foot (Figure 5C) suggested a role for both GSH and GPx in the elimination of peroxides and ROS, thereby providing another line of defense against oxidative damage. The antioxidant function of GSH is also due to its participation as an abundant redox buffer in the cells, where it could serve as a substrate for GSH-related enzymes (Cui et al., 2020).
Conclusion
Overall, the bioaccumulation of Cd2+ in M. meretrix was found to display a concentration-dependent trend with significant differences among different tissues exposed to the same Cd2+ concentration. This observation would support speculation that people who continue to consume cadmium-loaded clams in their diets could risk exposing themselves to Cd toxicity in the long term. Change in the level of MDA of the clams might provide a useful biomarker for Cd2+ contamination due to their constant increase with increasing Cd2+ concentrations in the water, and the gill might be a good tissue to detect the early biological responses in a Cd2+-contaminated environment. In the detoxification aspect, at low concentrations of Cd2+, the combined action of MT and GSH could lead to more sequestration of free Cd2+ inside the organism. At the same time, enhanced levels of CAT, SOD, and GPx activities would help to neutralize the toxic effect of Cd2+ and to protect the membrane lipids against oxidative stress. The continuous increase in Cd2+ concentration in the environment would lead to the depletion of GSH, triggering the MT-based mechanism, which could further sequester the Cd2+, presumably through the formation of MT-Cd2+ complexes. However, the precise mechanism associated with the detoxification of Cd2+ toxicity by MT is a subject of further study.
Data Availability Statement
All datasets generated for this study are included in the article/supplementary material.
Author Contributions
YH and HT were responsible for the experimental design, carrying out the experiments, data processing, and manuscript writing. JJ and MF performed the sample collection, sample processing, and extraction. AC and XY provided experimental guidance and supervision. All authors contributed to the article and approved the submitted version.
Funding
This study was supported by the Nature Science Foundation of Zhejiang Province (CN) under Grant number LY13C040003, LY18C030005 (to XY), the Nature Science Foundation of Wenzhou under Grant number N20190010 (to JJ), the National Student Innovation Training Project under Grant number JW201910351039 (to HT) and JWSC2019079 (to YH), and the Zhejiang Xinmiao Talents Program under grant number 2019R (to MF).
Conflict of Interest
The authors declare that the research was conducted in the absence of any commercial or financial relationships that could be construed as a potential conflict of interest.
References
Amiard, J. C., Amiard-Triquet, C., Barka, S., Pellerin, J., and Rainbowd, P. S. (2006). Metallothioneins in aquatic invertebrates: their role in metal detoxification and their use as biomarkers. Aquat. Toxicol. 76, 160–202. doi: 10.1016/j.aquatox.2005.08.015
Baker, M. A., Cerniglia, G. J., and Zaman, A. (1990). Microtiter plate assay for the measurement of glutathionein and glutathionein dissulfifide in large numbers of biological samples. Anal. Biochem. 190, 360–365. doi: 10.1016/0003-2697(90)90208-q
Beauchamp, C., and Fridovich, I. (1971). Superoxide dismutase: improved assays and an assay applicable to acrylamide gels. Anal. Biochem. 44, 276–287. doi: 10.1016/0003-2697(71)90370-8
Bouzahouane, H., Barour, C., Sleimi, N., and Ouali, K. (2018). Multi-biomarkers approach to the assessment of the southeastern Mediterranean Sea health status: preliminary study on Stramonita haemastoma used as a bioindicator for metal contamination. Chemosphere 207, 725–741. doi: 10.1016/j.chemosphere.2018.05.118
Bradford, M. M. (1976). A rapid and sensitive method for the quantification of microgram quantities of protein utilizing the principle of protein-dye binding. Anal. Biochem. 72, 248–254. doi: 10.1016/0003-2697(76)90527-3
Cenov, A., Perić, L., Glad, M., Žurga, P., Lušić, D. V., Traven, L., et al. (2018). A baseline study of the metallothioneins content in digestive gland of the Norway lobster Nephrops norvegicus from Northern Adriatic Sea: body size, season, gender and metal specifific variability. Mar. Pollut. Bull. 131, 95–105. doi: 10.1016/j.marpolbul.2018.03.002
Chan, C. Y., and Wang, W. X. (2018). Seasonal and spatial variations of biomarker responses of rock oysters in a coastal environment influenced by large estuary input. Environ. Pollut. 242, 1253–1265. doi: 10.1016/j.envpol.2018.08.013
Chandurvelan, R., Marsden, I. D., Gaw, S., and Glover, C. N. (2012). Impairment of green lipped mussel (Perna canaliculus) physiology by waterborne cadmium: relationship to tissue bioaccumulation and effect of exposure duration. Aquat. Toxicol. 12, 114–124. doi: 10.1016/j.aquatox.2012.07.013
Chandurvelan, R., Marsden, I. D., Glover, C. N., and Gaw, S. (2015). Assessment of a mussel as a metal bioindicator of coastal contamination: relationships between metal bioaccumulation and multiple biomarker responses. Sci. Total Environ. 511, 663–675.
Chen, M. X., Zhou, J. Y., Lin, J. H., Tang, H. C., Shan, Y. F., Chang, A. K., et al. (2020). Changes in oxidative stress biomarkers in Sinonovacula constricta in response to toxic metal accumulation during growth in an aquaculture farm. Chemosphere 248:125974.
Chen, R. Q., Ye, F. Y., Lin, J. H., Zhou, J. Y., Cai, J. J., and Ying, X. P. (2019). Effects of cadmium and mercury on oxidative stress indexes in the ovary of Eriocheir sinensis. Acta Hydrobiol. Sin. 43, 554–562.
Cheng, L., Zhou, J. L., and Cheng, J. P. (2018). Bioaccumulation, tissue distribution and joint toxicity of erythromycin and cadmium in Chinese mitten crab (Eriocheir sinensis). Chemosphere 210, 267–278. doi: 10.1016/j.chemosphere.2018.07.005
Cong, M., Wu, H. F., Liu, X. L., Zhao, J. M., Wang, X., Lv, J. S., et al. (2012). Effects of heavy metals on the expression of a zinc-inducible metallothionein-III gene and antioxidant enzyme activities in Crassostrea gigas. Ecotoxicology 21, 1928–1936. doi: 10.1007/s10646-012-0926-z
Cui, W. T., Cao, L., Liu, J. H., Ren, Z. H., Zhao, B., and Dou, S. Z. (2020). Effects of seawater acidifification and cadmium on the antioxidant defense of flounder Paralichthys olivaceus larvae. Sci. Total Environ. 718:137234. doi: 10.1016/j.scitotenv.2020.137234
Dabas, A., Nagpure, N. S., Mishra, R. M., Kumar, R., Kushwaha, B., Srivastava, R., et al. (2014). Cadmium induced lipid peroxidation and effects on glutathione dependent enzymes in tissues of Labeo rohita. Proc. Natl. Acad. Sci. India Sect. B Biol. Sci. 84, 981–988. doi: 10.1007/s40011-013-0280-9
Domouhtsidou, G. P., and Dimitriadis, V. K. (2000). Ultrastructural localization of heavy metals (Hg, Ag, Pb, and Cu) in gills and digestive gland of mussels, Mytilus galloprovincialis (L.). Arch. Environ. Con. Tox. 38, 472–478. doi: 10.1007/s002449910062
Fang, Y., Yang, H. S., Wang, T. M., Liu, B. Z., Zhao, H. L., and Chen, M. Y. (2010). Metallothionein and superoxide dismutase responses to sublethal cadmium exposure in the clam Mactra veneriformis. Comp. Biochem. Physiol. Part C 151, 325–333. doi: 10.1016/j.cbpc.2009.12.005
Fraternale, A., Brundu, S., and Magnani, M. (2017). Glutathione and glutathione derivatives in immunotherapy. Biol. Chem. 398, 261–275. doi: 10.1515/hsz-2016-0202
García-Navarro, J. A., Franco, L., and Romero, D. (2017). Differences in the accumulation and tissue distribution of Pb, Cd, and Cu in Mediterranean mussels (Mytilus galloprovincialis) exposed to single, binary, and ternary metal mixtures. Environ. Sci. Pollut. Res. 24, 6599–6610. doi: 10.1007/s11356-016-8349-7
Ghosh, S., Mal, M., and Mandal, S. (2020). A dynamic model of cadmium bioaccumulation in Lamellidens marginalis, an edible shellfish in India. Ecol. Model. 419:108957. doi: 10.1016/j.ecolmodel.2020.108957
Gu, B. G., Liang, W., Yang, T. Z., Hu, Z. J., and Shen, H. D. (2019). Metallothionein, hemocyte status and superoxide dismutase/aspartate aminotransferase activity are sensitive biomarkers of cadmium stress in Onchidium reevesii. Aquat. Toxicol. 215:105284. doi: 10.1016/j.aquatox.2019.105284
Hamad, A., Amel, E. G., Saleh, F., and Magdy, E. H. (2011). Evaluation of heavy metal pollution in the Arabian Gulf using the clam Meretrix meretrix Linnaeus, 1758. Water Air Soil Pollut. 214, 499–507. doi: 10.1007/s11270-010-0441-x
Haque, N., Lee, D. H., Kim, B. M., Nam, S. E., and Rhee, J. S. (2018). Dose- and age-specifific antioxidant responses of the mysid crustacean Neomysis awatschensis to metal exposure. Aquat. Toxicol. 201, 21–30. doi: 10.1016/j.aquatox.2018.05.023
Hu, X. Y., Lai, Z. Y., Zhao, Y. F., Wang, C., Wei, T. L., Jiang, W. X., et al. (2011). Seasonal characteristics of cadmium content and distribution in Pearl River estuary. J. Fish. Sci. China 18, 629–635. doi: 10.3724/sp.j.1118.2011.00629
Huo, L. H., Lin, Z. H., You, Z. J., Liu, G. X., Zhu, D. L., and Wang, J. P. (2012). Accumulation mechanism of heavy metals (Cu, Pb, Cd) in different tissues of Tegillarca granosa. Mar. Environ. Sci. 31, 395–399.
Jing, W. X., Lang, L., Lin, Z. G., Liu, N., and Wang, L. (2019). Cadmium bioaccumulation and elimination in tissues of the freshwater mussel Anodonta woodiana. Chemosphere 219, 321–327. doi: 10.1016/j.chemosphere.2018.12.033
Lavradas, R. T., Hauser-Davis, R. A., Lavandier, R. C., Rocha, R. C. C., Saint’ Pierre, T. D., Seixas, T., et al. (2014). Metal, metallothionein and glutathione levels in blue crab (Callinectes sp.) specimens from southeastern Brazil. Ecotox. Environ. Saf. 107, 55–60. doi: 10.1016/j.ecoenv.2014.04.013
Lin, Y., Huang, J. J., Dahms, H. U., Zhen, J. J., and Ying, X. P. (2017). Cell damage and apoptosis in the hepatopancreas of Eriocheir sinensis induced by cadmium. Aquat. Toxicol. 190, 190–198. doi: 10.1016/j.aquatox.2017.07.008
Liu, J. L., Xu, X. R., Ding, Z. H., Peng, J. X., Jin, M. H., Wang, Y. S., et al. (2015). Heavy metals in wild marine fish from South China Sea: levels, tissue- and species-specific accumulation and potential risk to humans. Ecotoxicology 24, 1583–1592. doi: 10.1007/s10646-015-1451-7
Liu, Q. Q., Wang, F. F., Meng, F. P., Jiang, L., Li, G. J., and Zhou, R. G. (2018). Assessment of metal contamination in estuarine surface sediments from Dongying City, China: use of a modified ecological risk index. Mar. Pollut. Bull. 126, 293–303. doi: 10.1016/j.marpolbul.2017.11.017
Liu, X., and Wang, W. X. (2016). Time changes in biomarker responses in two species of oyster transplanted into a metal contaminated estuary. Sci. Total Environ. 544, 281–290. doi: 10.1016/j.scitotenv.2015.11.120
Livak, K. J., and Schmittgen, T. D. (2001). Analysis of relative gene expression data using real-time quantitative PCR and the 2(-Delta Delta C(T)) method. Methods 25, 402–408. doi: 10.1006/meth.2001.1262
Loro, V. L., Jorge, M. B., Silva, K. R., and Wood, C. M. (2012). Oxidative stress parameters and antioxidant response to sublethal water borne zinc in a euryhaline teleost Fundulus heteroclitus: protective effects of salinity. Aquat. Toxicol. 110–111, 187–193. doi: 10.1016/j.aquatox.2012.01.012
Marasinghe Wadige, C. P. M., Taylor, A. M., Krikowa, F., Lintermans, M., and Maher, W. A. (2017). Exposure of the freshwater bivalve Hyridella australis to metal contaminated sediments in the field and laboratory microcosms: metal uptake and effects. Ecotoxicology 26, 415–434. doi: 10.1007/s10646-017-1774-7
Meng, F. P., Wang, Z. F., Cheng, F. L., Du, X. P., Fu, W. C., Wang, Q., et al. (2013). The assessment of environmental pollution along the coast of Beibu Gulf, northern South China Sea: an integrated biomarker approach in the clam Meretrix meretrix. Mar. Environ. Res. 85, 64–75. doi: 10.1016/j.marenvres.2013.01.003
Min, E. Y., Ahn, T. Y., and Kang, J. C. (2016). Bioaccumulation, alterations of metallothionein, and antioxidant enzymes in the mullet Mugil cephalus exposed to hexavalent chromium. Fish. Aquat. Sci. 19:19. doi: 10.1186/s41240-016-0020-21
Nair, A. R., Lee, W. K., Smeets, K., Swennen, Q., Sanchez, A., Thévenod, F., et al. (2015). Glutathione and mitochondria determine acute defense responses and adaptive processes in cadmium-induced oxidative stress and toxicity of the kidney. Arch. Toxicol. 89, 2273–2289. doi: 10.1007/s00204-014-1401-9
Ohkawa, H., Ohishi, N., and Yagi, K. (1979). Assay for lipid peroxides in animal tissues by thiobarbituric acid reaction. Anal. Biochem. 95, 351–358. doi: 10.1016/0003-2697(79)90738-3
Ohta, H., Qi, Y. G., Ohba, K., Toyooka, T., and Wang, R. S. (2019). Role of metallothionein-like cadmium-binding protein (MTLCdBP) in the protective mechanism against cadmium toxicity in the testis. Ind. Health 57, 570–579. doi: 10.2486/indhealth.2018-0177
Pan, L. Q., and Zhang, H. X. (2006). Metallothionein, antioxidant enzymes and DNA strand breaks as biomarkers of Cd exposure in a marine crab, Charybdis japonica. Comp. Biochem. Physiol. Part C 144, 67–75. doi: 10.1016/j.cbpc.2006.06.001
Rotruck, J. T., Pope, A. L., Ganther, H. E., Swanson, A. B., Hafeman, D. G., and Hoekstra, W. (1973). Selenium: biochemical role as a component of glutathione peroxidase. Science 179, 588–590. doi: 10.1126/science.179.4073.588
Saint-Denis, M., Labrot, F., Narbonne, J. F., and Ribera, D. (1998). Glutathione, glutathione-related enzymes and catalase activities in the earthworm Eisenia fetida andrei. Arch. Environ. Contam. Toxicol. 35, 602–614. doi: 10.1007/s002449900422
Sandbichler, A. M., and Höckner, M. (2016). Cadmium protection strategies-a hidden trade off? Int. J. Mol. Sci. 17:3390.
Wang, J., Zhai, Y. X., Ning, J. S., Jiang, Z. J., Tan, Z. J., Shang, D. R., et al. (2009). Heavy metal distribution in different tissues of Patinopecten yesoensis. Mar. Sci. 33, 44–47.
Wang, Q., Meng, F. P., Li, Y. F., Wang, Z. F., Fu, W. C., Du, X. P., et al. (2013). Study on the responses of oxidative stress biomarkers to the heavy metals accumulation in the clam Meretrix meretrix along the Beibu Bay. Sci. Sin. 43, 1189–1199.
Wang, Q., Wang, X. M., Wang, X. Y., Yang, H. S., and Liu, B. H. (2010). Analysis of metallotionein expression and antioxidant enzyme activities in Meretrix meretrix larvae under sublethal cadmium exposure. Aquat. Toxicol. 100, 321–328. doi: 10.1016/j.aquatox.2010.08.012
Xia, L. P., Chen, S. H., Dahms, H. U., Ying, X. P., and Peng, X. (2016). Cadmium induced oxidative damage and apoptosis in the hepatopancreas of Meretrix meretrix. Ecotoxicology 25, 959–969. doi: 10.1007/s10646-016-1653-7
Yao, J., Yang, Z. G., Li, H. P., Qu, Y. B. X., and Qiu, B. (2020). Effffects of waterborne exposure to cadmium on biochemical responses in the freshwater gastropod, Bellamya aeruginosa. Ecotox. Environ. Saf. 193:110365. doi: 10.1016/j.ecoenv.2020.110365
Zhang, B., Shi, Z., Wang, X., Deng, S., and Lin, H. (2015). Depuration of cadmium from blue mussel (Mytilus edulis) by hydrolysis peptides and chelating metal elements. Food Res. Int. 73, 162–168. doi: 10.1016/j.foodres.2014.12.043
Zhang, Y. K., Song, X. K., Liu, A. Y., Liu, L. J., Jiang, X. Y., and Ma, J. X. (2011). Acute toxicity effects of Cd2+ and Cu2+ on Meretrix meretrix linnaeus. Trans. Oceanol. Limnol. 3, 51–56.
Zhen, J. J., Ye, F. Y., Wang, D., Ni, X. Y., and Ying, X. Y. (2018). Effect of cadmium on ultrastructure of gill epithelial cells in clam Meretrix meretrix. Fish. Sci. 37, 469–474.
Zheng, J. L., Yuan, S. S., Wu, C. W., and Lv, Z. M. (2016). Acute exposure to waterborne cadmium induced oxidative stress and immunotoxicity in the brain, ovary and liver of zebrafish (Danio rerio). Aquat. Toxicol. 180, 36–44. doi: 10.1016/j.aquatox.2016.09.012
Zhu, Q. H., Zhou, Z. K., Tu, D. D., Zhou, Y. L., Wang, C., Liu, Z. P., et al. (2018). Effect of cadmium exposure on hepatopancreas and gills of the estuary mud crab (Scylla paramamosain): histopathological changes and expression characterization of stress response genes. Aquat. Toxicol. 195, 1–7. doi: 10.1016/j.aquatox.2017.11.020
Keywords: cadmium, Meretrix meretrix, oxidative damage, metallothionein, glutathione, antioxidant enzyme
Citation: Huang Y, Tang H, Jin J, Fan M, Chang AK and Ying X (2020) Effects of Waterborne Cadmium Exposure on Its Internal Distribution in Meretrix meretrix and Detoxification by Metallothionein and Antioxidant Enzymes. Front. Mar. Sci. 7:502. doi: 10.3389/fmars.2020.00502
Received: 06 March 2020; Accepted: 03 June 2020;
Published: 09 July 2020.
Edited by:
Andrew Stanley Mount, Clemson University, United StatesReviewed by:
Mirza Hasanuzzaman, Sher-e-Bangla Agricultural University, BangladeshKamrun Nahar, Sher-e-Bangla Agricultural University, Bangladesh
Copyright © 2020 Huang, Tang, Jin, Fan, Chang and Ying. This is an open-access article distributed under the terms of the Creative Commons Attribution License (CC BY). The use, distribution or reproduction in other forums is permitted, provided the original author(s) and the copyright owner(s) are credited and that the original publication in this journal is cited, in accordance with accepted academic practice. No use, distribution or reproduction is permitted which does not comply with these terms.
*Correspondence: Xueping Ying, eHB5aW5nMjAwOEB3enUuZWR1LmNu; MDAxMjEwMThAd3p1LmVkdS5jbg==; NjI0MjEwMTEwQHFxLmNvbQ==
†These authors have contributed equally to this work