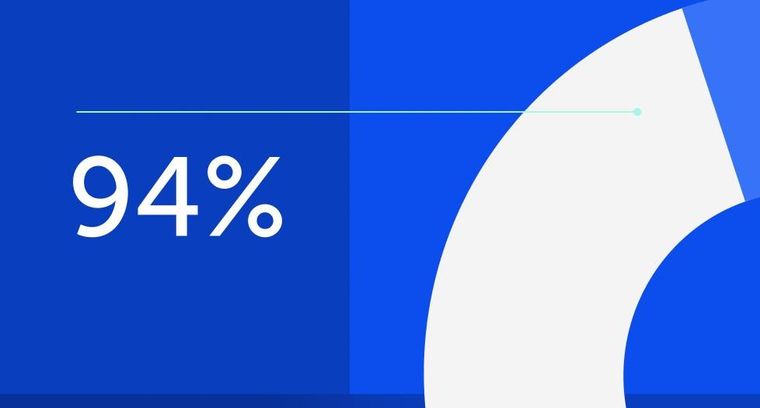
94% of researchers rate our articles as excellent or good
Learn more about the work of our research integrity team to safeguard the quality of each article we publish.
Find out more
ORIGINAL RESEARCH article
Front. Mar. Sci., 17 June 2020
Sec. Marine Biogeochemistry
Volume 7 - 2020 | https://doi.org/10.3389/fmars.2020.00428
This article is part of the Research TopicCurrent Topics in Marine Organic Biogeochemical ResearchView all 21 articles
Over the last decades, the Arctic Ocean has suffered a substantial decline in sea ice cover due to global warming. The impacts of these variations on primary productivity, fluxes of dissolved and particulate organic matter (OM) and turnover at the seafloor are still poorly understood. Here we focus on the characteristics and dynamics of the pool of marine dissolved OM (DOM) in surface sediments of the Arctic Ocean. To investigate spatial and temporal variations of DOM in relation to particulate OM input and benthic microbial community parameters, sediment porewater and overlying bottom water were collected from the long-term observatory HAUSGARTEN in June 2013 and 2014. The study area in the Fram Strait, which is partially covered by sea ice, was sampled along a bathymetric transect (1050–5500 m water depth), from east to west (7°0.2′ E to 5°17′ W), and from south to north (78°37’ to 79°43’ N). Molecular data on solid phase extracted DOM obtained via Fourier Transform Ion Cyclotron Resonance Mass Spectrometric analysis and a suite of bulk chemical parameters were related to benthic biogeochemical data. Our results demonstrate a close coupling between the production and input of OM from the surface ocean to the seafloor, and the concentration and composition of DOC/DOM in the deep sea. Surface porewaters collected in 2013 from shallower stations (≤1500 m water depth) in the eastern Fram Strait, had a signal of a larger and more recent input of OM (higher concentrations of phytodetritus). This was associated with higher numbers of molecular formulas, abundances of unsaturated aliphatic and N-containing formulas, in concert with higher enzymatic activity, phospholipids, total organic carbon and protein content. In contrast, porewaters collected in 2014 from deeper stations and from the West, were associated with lower OM input, and showed higher abundances of aromatic and oxygen-poor compounds. Higher OM input was also reflected in higher DOC concentrations and fluxes from the sediment into the water column. Our study demonstrates that regional and temporal variations in OM input can quickly translate into changes in the quantity and quality of surface porewater DOM, the latter substantially altered by deep-sea sediment bacteria.
Dissolved organic matter (DOM) is a complex mixture of organic molecules produced as an intermediate during the mineralization of sedimentary OM (Burdige and Komada, 2015). In the Arctic Ocean, which is surrounded by massive shelf zones and margins, a terrestrial signature is found in the DOM from surface sediments of the deep basins (Rossel et al., 2016). DOM plays a crucial role in the global carbon cycle, although its molecular composition, which is expected to influence its susceptibility to biotic and abiotic alteration, is largely unknown (Dittmar and Stubbins, 2014; Zhang et al., 2018 and references therein). At the seafloor, DOM may become a component of sediment porewater or absorbed to the sediment matrix (Burdige and Komada, 2015). Porewater DOM is further reworked during a degradation continuum that begins in surface sediments, with the uptake of monomeric molecules (e.g., amino acids, sugars and fatty acids) by benthic organisms dominated in biomass by bacteria, and continues in deeper sediment layers, with the utilization of more complex substrates by subsurface microbial communities (Burdige and Komada, 2015). Compared to the amount remineralized in the seabed, a significant fraction of this pool escapes diagenesis via diffusion to the water column (Burdige et al., 1992, 1999; Burdige and Komada, 2015; Rossel et al., 2016; Loginova et al., 2020), sorption onto minerals (Arnarson and Keil, 2001; Aufdenkampe et al., 2001), formation of complexes with metals (Seidel et al., 2014, 2015a; Linkhorst et al., 2017) or due to sulfurization, which favors preservation of OM under anoxic conditions (Sinninghe Damste and de Leeuw, 1990; Schmidt et al., 2014; Jessen et al., 2017; Pohlabeln et al., 2017). Although previous studies have evaluated the composition and reactivity of porewater DOM (Burdige and Komada, 2015 and references therein), the complexity of this pool limits our understanding of its role in the preservation and degradation of OM. Also, its influence on the DOM composition of the water column and as a potential carbon source is poorly understood, because the reactivity of the material released into the water column is not well known. Knowledge of these processes is even more restricted in areas of limited access such as the deep-sea floor, which represents 60% of the Earth’s surface (Smith et al., 2009).
In the Arctic Ocean, particulate OM (POM) export from the mixed surface layer strongly varies temporally as a result of the pronounced seasonality, and regionally and interannually depending on ocean conditions, such as sea ice cover and extent, and phytoplankton community composition (e.g., Wassmann and Reigstad, 2011; Boetius et al., 2013; Lalande et al., 2013). Sea ice cover and the input of primary produced and terrigenous OM have been shown to modulate the molecular composition and distribution of porewater DOM in central Arctic sediments (Rossel et al., 2016). In addition, the sedimentation of POM is one of the major factors influencing the activity and structure of benthic communities (Boetius and Damm, 1998; Klages et al., 2004; Bienhold et al., 2012; Jacob et al., 2013; Kêdra et al., 2015). Thus, variations in the quality and quantity of OM exported to the seafloor are expected to severely impact benthic communities, as previously reported for other environments (Wohlers et al., 2009; Kortsch et al., 2012; Jones et al., 2014). However, our understanding of the interrelations between DOM composition and benthic communities in deep-sea sediments is still limited. Furthermore, the Arctic Ocean is rapidly changing as a result of ocean warming and the decline and thinning of the sea ice cover (Kwok and Rothrock, 2009; Notz and Stroeve, 2016; Peng and Meier, 2018). The amplified warming trend in the Arctic (Dobricic et al., 2016; Sun et al., 2016) could shift the system from a cold and ice-covered to a warmer and ice-free ocean by the end of the century (Overland and Wang, 2013; Polyakov et al., 2017). This is expected to affect ecosystem structure and function, and OM cycling from the surface to the deep sea (Grebmeier et al., 2006; Leu et al., 2011; Wassmann and Reigstad, 2011; Boetius et al., 2013).
To better understand links between spatial (regional) and temporal (interannual) variations in surface ocean conditions and OM input with benthic communities and the molecular composition of DOM at the Arctic seafloor, we collected porewater and overlying bottom water samples from the well-studied area of the Long-Term Ecological Research (LTER) observatory HAUSGARTEN in Fram Strait (Soltwedel et al., 2016). Surface sediments (0–1 cm) represent the horizon that receives most of the fresh organic material from surface waters, while subsurface sediments, here defined as 1–10 cm due to the low sediment accumulation rates in the Arctic system (Stein, 2008), obtain partly reworked organic matter.
The Fram Strait can be divided into two hydrographic regimes (Bauerfeind et al., 2009). The eastern part is characterized by the inflow of warm and nutrient-rich Atlantic Water to the central Arctic, and the western part is characterized by the outflow of cooler and less saline Polar Water that exits the central Arctic and carries a large part of the Arctic sea ice toward the North Atlantic (Paquette et al., 1985; de Steur et al., 2009; Beszczynska-Moller et al., 2012). The two regions are also characterized by different sea ice conditions. The eastern part is only seasonally ice- covered or permanently ice- free, while the western part is predominantly ice- covered throughout the year (Soltwedel et al., 2016). Previously reported POC fluxes at HAUSGARTEN are around 1.3–1.5 mol C m–2 yr–1 (45 mg Cm–2 d–1, Bauerfeind et al., 2009; ca. 50 mg C m–2 d–1, Lalande et al., 2014), which are in a similar range as fluxes reported for the ice margin (1–3.5 mol C m–2 yr–1) of the central Arctic Ocean, and three- to ten-fold higher than those of its permanently ice covered basins (0.1–0.4 mol C m–2 yr–1) (Lalande et al., 2014). Observations at HAUSGARTEN have revealed a tight coupling between variations in sea ice cover and hydrography with the development of the seasonal phytoplankton bloom and shifts in species composition on temporal (Nöthig et al., 2015; Soltwedel et al., 2016) and spatial (Fadeev et al., 2018) scales. These changes in the water column also lead to differences in the quantity and quality of POM exported to the deep sea (Lalande et al., 2013; Soltwedel et al., 2016).
Here, we combined DOM molecular characterization using Fourier Transform Ion Cyclotron Resonance Mass Spectrometry (FT-ICR-MS) with the analysis of environmental parameters, including sea ice cover and a range of benthic parameters obtained in the framework of the LTER HAUSGARTEN. We test the hypothesis that the molecular composition of DOM as assessed by FT-ICR-MS, as well as specific derived indicators for OM freshness can resolve spatial and temporal variations across local and ocean-wide scales. Specifically, we tested (i) that regional and interannual differences in the oceanographic regimes of Fram Strait are reflected in the molecular composition of DOM in deep-sea surface sediments, (ii) that these differences are also linked with variations in other benthic parameters, such as OM input, and the abundance, biomass and activity of microbial communities, and (iii) that POM degradation in surface sediments is an important source to DOM in bottom water and subsurface sediments, but that benthic bacteria alter the DOM spectra substantially.
Porewater (0–1, 1–5, and 5–10 cm; n = 124) from oxygenated sediments (Donis et al., 2016; Hoffmann et al., 2018) and overlying bottom water samples (n = 52) were collected at the deep-sea LTER HAUSGARTEN. The LTER HAUSGARTEN is located in the Fram Strait between northern Greenland and the Svalbard archipelago (Figure 1), and is the only deep water connection for the exchange of intermediate and deep water masses between the Arctic Ocean and the North Atlantic (Fahrbach et al., 2001). Stations cover a latitudinal and a longitudinal transect, including a bathymetric gradient from around 250 to 5500 m water depth (Soltwedel et al., 2016). Ice conditions vary throughout seasons and years, but there are some general characteristics that define different regimes (Soltwedel et al., 2016): Stations in the eastern Fram Strait (from this study: S1-S3, KH, HGI-HGIX and N4) vary from permanently ice-free in the south to seasonally ice-covered in the center and north. Stations in the west (EGI-EGIV, part of HAUSGARTEN observations since 2014; Soltwedel et al., 2016) are predominantly ice-covered. In summer months, the northern and central stations of the eastern Fram Strait are close to the marginal ice zone, which is rich in nutrients and can cause intense phytoplankton blooms and regionally enhanced fluxes of POM to the seafloor (Schewe and Soltwedel, 2003; Bauerfeind et al., 2009; Soltwedel et al., 2016).
Figure 1. Bathymetric map of the Arctic Ocean indicating sampling stations at the LTER observatory HAUSGARTEN (Fram Strait). The color of the dots indicates concentrations of chloroplastic pigment equivalents (CPE) in surface sediments (0–1 cm, in μg/cm3 sediment indicated on the color legend on the left). The colors of the lines indicate the location of the sea ice margin during each sampling year, defined as >15% ice coverage (Supplementary Table S1).
Sampling was performed during two cruises, with RV Maria S. Merian MSM 29 in summer 2013 (23 June-12 July) and with RV Polarstern PS85 in summer 2014 (6 June-3 July). In 2013, sampling mainly took place along the latitudinal gradient in the eastern part of the Strait (between 1270 to 2700 m water depths, Supplementary Table S1). During the 2014 expedition, samples were mainly retrieved from the longitudinal East-West transect across Fram Strait (7°0.2′ E to 5°17’ W), including bathymetric transects between 1050 and 5500 m water depth (Supplementary Table S1). Three stations were sampled in both years (HGI, HGII, N4). Thus, the combination of both cruises provides a first perception of the spatial (latitudinal and longitudinal trends assessed based on 2013 and 2014 sampling, respectively), and interannual variability of DOM in deep-sea sediments of the Fram Strait.
Sediment cores and bottom water (defined as overlying water collected above the sediment) were collected with a TV-guided multicorer at stations in the eastern and western Fram Strait, between 78–79.5°N and 5°W–7°E (Figure 1 and Supplementary Table S1). After retrieval, the sediment cores were stored in a 0°C cold room, and from these undisturbed cores the bottom water was cautiously collected. Sediment porewater was collected from three sediment depth horizons, i.e., 0–1, 1–5, and 5–10 cm using rhizons (pore size 0.15 μm CSS, Rhizosphere Research Products) connected to 10 ml syringes without rubber on the piston. Porewater was then transferred to 50 ml Sarstedt vials. Two or more pseudoreplicates, prepared with the same porewater mixture collected from the cores at each depth, and replicates from parallel cores were analyzed separately in order to evaluate sampling variability and potential contamination during sample handling (Supplementary Table S1). All material had been previously rinsed at least three times with Milli-Q water. Prior to analysis, all samples were stored at −20°C.
The extraction of DOM from acidified (pH 2) porewater and bottom water samples was performed with 100 mg styrene divinyl benzene polymer columns (Varian PPL, Dittmar et al., 2008), formerly rinsed with MeOH (HPLC grade; Sigma-Aldrich, United States). Before DOM elution, the columns were rinsed several times with ultrapure water acidified at pH 2 with HCl to remove the salt from the cartridges (a prerequisite for MS analysis), and then dried under a stream of ultrapure N2. Elution of the solid phase extracted (SPE)-DOM from the PPL columns was performed with 1 ml of MeOH. The extracts were stored at −20°C until MS analysis. Analysis of DOC and TDN were performed by hand injection via catalytic oxidation at high temperature with a TOC-VCPH Shimadzu instrument (Stubbins and Dittmar, 2012) using 1 ml aliquots of each sample, after acidification with HCl (25% Carl Roth, Germany) to pH 2 and purging with synthetic air. The precision of the analysis was better than 6% and was assessed by the analysis of deep-sea water from the Consensus Reference Material Project (University of Miami, United States). DOC and TDN data (Supplementary Table S1) were stored in the earth system database PANGAEA (Rossel and Dittmar, 2019a).
Dissolved organic matter efflux from the sediment (Jdiff) was determined according to Fick’s first law of diffusion:
where Jdiff: diffusive flux (mmol/m2/d), Φ: porosity, Dsed: the diffusion coefficient in the sediment (in m2/s and corrected for temperature) (Iversen and Jorgensen, 1993) and dC/dZ: DOC concentration gradient between bottom water (defined at 0 mm) and 5 mm in the sediment core (Supplementary Table S1). Following Burdige et al. (1992), the diffusion coefficient in seawater (D)° was adjusted to the molecular weight of DOM molecules according to the following relationship: Log D° = 1.72–0.39 × log MWwa, where MWwa is the molecular weight intensity-weighted average obtained from the analysis of compounds amenable by electrospray ionization via FT-ICR-MS of each SPE-DOM sample (see section “Molecular Analysis of DOM via FT-ICR-MS”). The diffusion coefficient in the sediment was calculated as Dsed = D°/1-ln(Φ2) (Boudreau, 1997; Schulz, 2000). To constrain the time (t) for an avg. DOM compound detected by our analysis to diffuse into the sediment, the equation t = L2/2D was used (Jorgensen, 2006). In this equation, L represents the distance (cm) and D the diffusion coefficient (avg. from all stations). In this calculation, we assume the DOC fluxes in the sediment rather than in the diffusive boundary layer (Rossel et al., 2016), which is a more conservative approach for the resolution of our measurements.
Molecular analysis of SPE-DOM from bottom water and porewater samples was performed with a 15 Tesla FT-ICR-MS instrument (Bruker Solarix), equipped with an electrospray ionization source (Bruker Apollo II) in negative ion mode. Ultrahigh resolution mass spectrometry techniques via FT-ICR-MS have been successfully applied for the analysis of complex DOM molecular mixtures in sediment porewaters from a variety of marine environments (Schmidt et al., 2009, 2014; Seidel et al., 2014, 2015a; Rossel et al., 2016). Prior to analysis, SPE-DOM aliquots were diluted in 1:1 MeOH: ultrapure water to a final DOC concentration of 15–20 mg C/l, assuming extraction efficiency of ca. 50% (due to restricted SPE volumes, SPE-DOM samples were not analyzed for DOC concentration; Schmidt et al., 2009; Schmidt et al., 2017). Samples were infused at 120 μl/h using an ion accumulation of 0.2 s and a capillary voltage of 4 kV. Sample spectrum was obtained after 500 individual scans. External and internal calibrations were performed based on the arginine cluster and based on a list of >30 mass peaks of known formulas in the samples, respectively. Calculation of molecular formulas for all samples was performed using an in-house MATLAB (2010) routine that searches, with an error <0.5 ppm, for all potential combinations of C∞, O∞, H∞, N ≤ 4; S ≤ 2 and P ≤ 1. Formulas were calculated for intensities above the method detection limit (MDL; Riedel and Dittmar, 2014). Molecular masses present in the Milli-Q water blanks were only retained in the dataset if their signal/MDL was <20, since higher intensities in the blanks could indicate these masses are likely present in the samples due to contamination. Furthermore, molecular masses that appeared only once in the whole dataset were eliminated. In order to remove double assignments of formulas to the same mass, CH2 homologous series extended to lower mass range were considered correct and the combination of >3 N, S or P atoms (NSP, N2S, N3S, N4S, N2P, N3P, N4P, NS2, N2S2, N3S2, N4S2, S2P) was not allowed (Rossel et al., 2013, 2017). With this procedure, the whole dataset is represented by 7400 formulas, not considering isotopologues (Rossel and Dittmar, 2019b). To obtain structural information, the aromaticity index (AImod; Koch and Dittmar, 2006; Koch and Dittmar, 2016) and the double bond equivalent (DBE, McLafferty and Turecek, 1994) were used. In addition to the intensity weighted-average values for AImod and DBE, H/C and O/C elemental ratios, as well as the molecular weight, were also calculated (DBEwa, AImodwa, H/Cwa, O/Cwa, MWwa). Additional indices reported to evaluate natural transformation processes in the water column DOM were also calculated for bottom and porewater samples: The SPE-DOM degradation state and lability were evaluated by the IDeg (Flerus et al., 2012) and molecular lability boundary (MLBwl, D’Andrilli et al., 2015), respectively. Furthermore, the recently reported index Ibioprod, was used to assess the impact of biological production in shaping the DOM present in the samples (Seibt, 2017). The Ibioprod index was calculated using the intensity of 10 molecular formulas in each sample according to the following equation:
The formulas in the dividend have been selected as “markers” for bioproduction based on mesocosm experiments (Osterholz et al., 2015), while those in the divisor were not influenced by bioproduction (Seibt, 2017).
In order to provide an overview, molecular formulas were additionally organized into previously defined molecular categories (Seidel et al., 2014; Rossel et al., 2016), even though this categorization is not unambiguous, due to the occurrence of isomers (Zark et al., 2017; Hawkes et al., 2018). However, this categorization has been widely used to represent the regions in the van Krevelen diagram where known molecular groups are located according to their elemental compositions (Supplementary Table S2 and Supplementary Figure S1). Furthermore, carboxyl-rich alicyclic molecules (CRAM-like, Supplementary Figure S1), which are considered an important refractory component of DOM, were also calculated based on the ratios DBE/C (0,30–0,68), DBE/H (0,20–0,95), and DBE/O (0,77–1,78) (Hertkorn et al., 2006).
Daily sea ice concentration data on a 12.5 × 12.5 km grid were retrieved from the PANGAEA database (Krumpen, 2017). Average sea ice concentrations for June 2013 and June 2014 were calculated for each station to reflect sea ice conditions at the time of sampling. In addition, we calculated average sea ice concentrations over the past 5 years for each station, i.e., from 15 June 2008–15 June 2013 for stations sampled in 2013 and from 15 June 2009–15 June 2014 for stations sampled in 2014, to reflect longer-term trends in sea ice cover at the different stations (Supplementary Table S1).
To estimate biological productivity regimes in surface waters at the time of sampling in 2013 and 2014, we used satellite-based chlorophyll estimates, which were obtained from http://www.globcolour.info (Supplementary Figure S2). Particulate organic carbon (POC) flux estimates were obtained from sediment trap moorings (e.g., Lalande et al., 2013, 2016 for description of the moorings) at stations HGIV and N4 in the central and northern parts of the sampling area, respectively (Supplementary Table S3).
Contextual data obtained in the framework of the LTER program at HAUSGARTEN were used to evaluate the DOM molecular composition of porewater and bottom water samples collected in the Fram Strait. Data were retrieved from ICSU World Data Center PANGAEA (see doi links in Supplementary Table S1). Briefly, the methods used to generate the reported data were as follows:
Samples from replicate multicorer cores collected at the same stations were used for chlorophyll pigment analyses to evaluate the phytodetritus contribution to the seafloor (Schewe, 2018, Schewe, 2019a-g, 2019a-m in Supplementary Table S1). Briefly, pigments were extracted in acetone (90%), using glass beads and grinding of each sample in a cell mill. Concentrations of chlorophyll a (chl a) and phaeopigments were determined fluorometrically with a Turner fluorometer (Shuman and Lorenzen, 1975), and the sum of chl a and phaeopigments is expressed as chloroplast pigment equivalents (CPE; Thiel, 1982). The ratio of chl a to CPE (expressed as a proportion) was used as an indicator of phytodetrital freshness. Benthic community data included phospholipid content (Schewe, 2018, Schewe, 2019a-g; 2019a-m in Supplementary Table S1), which were obtained from chloroform-methanol extracts, after lipid-bound phosphates were liberated by persulfate oxidation technique (Findlay et al., 1989; Boetius and Lochte, 1994). Total phospholipids were used as an indicator of total microbial biomass (including bacteria, microbial eukaryotes and meiofauna) (Soltwedel et al., 2016). Particulate proteins (operationally defined as γ-globulin equivalents and from here on referred to as proteins) were analyzed photometrically according to Greiser and Faubel (1989) and represent the bulk of “living” (small organisms) and “dead” biomass (detrital matter) (Schewe, 2018, Schewe, 2019a-g, 2019a-m in Supplementary Table S1). The potential activity of hydrolytic exo-enzymes acting as esterases was evaluated according to Köster et al. (1991) using the fluorogenic substrate fluorescein-di-acetate (FDA, from here on referred to as esterase activity) (Schewe, 2018, Schewe, 2019a-m in Supplementary Table S1). Bacterial cell counts were performed using acridine orange direct counts (Bienhold, 2019), following the procedure described in Hoffmann et al. (2017). For each sample two replicate filters were counted. Cell volumes were determined using a stage micrometer and converted to biomass using a conversion factor of 3 × 10–13 g C μm–3 (Børsheim et al., 1990). Sediment porosity for the stations sampled in 2013 and 2014 (except for N4, for which an avg. value of all stations sampled in 2014 was used) was calculated according to Burdige (2006) and Hoffmann et al. (2018). Additionally, total organic carbon content (TOC) was determined by measuring ash-free dry weight of sediments after combustion at 500°C for 2 h (Schewe, 2018, Schewe, 2019a-g, a-m in Supplementary Table S1).
The DOM dataset consisted of 7400 molecular formulas, each one represented by a mass peak whose intensity was normalized to the sum of intensities in the sample. For pseudoreplicates (subsamples from porewater collected from the same sediment core but analyzed separately on the FT-ICR-MS and indicated by sample # in Supplementary Table S1), formulas were considered present when they occurred in all pseudoreplicates. For repeated analysis of samples, formulas were considered present when they occurred in all of their respective pseudoreplicates and their average intensity was used for further statistical analysis. This is a conservative approach proposed when no real replicates (i.e., samples from parallel sediment cores) are available (Buttigieg and Ramette, 2014). Thus, each sediment depth in the multivariate statistical analysis is represented by several FT-ICR-MS analysis. In order to give less weight to rare formulas in the multivariate statistical analysis, data were Hellinger transformed (Ramette, 2007).
Contextual data of environmental parameters (ice cover, phytodetrital proxies, benthic community data and sediment properties) were standardized prior to analyses (Ramette, 2007). Geographic distances between stations were calculated using their geographic coordinates. Distances were then represented in a two-dimensional space using a principal coordinate analysis, yielding X (longitude) and Y (latitude) coordinates to be used in further statistical analyses. All of the statistical analyses above were performed in R (v3.1.0; R: A Language and Environment for Statistical Computing, R Core Team R Foundation for Statistical Computing, Vienna, Austria, 20141) using RStudio (v0.99.903; RStudio Team, 2015) and the packages vegan (v2.4-1; Oksanen, 2017) and gmt (v2.0-1; Magnusson, 2010).
Multivariate statistical analysis was performed by Partial Least Square analysis (PLS; Unscrumbler X v10.5 from Camo Software), which considered the contextual data and the formulas along with their relative abundances in each sample, thereby allowing an identification of formulas that were more strongly associated with the environmental data. PLS has been successfully applied for the statistical analysis of FT-ICR-MS data (Rossel et al., 2016, 2017). The outcome of the PLS analysis is displayed by scores and loadings, the latter for both the environmental (X loadings) and molecular data (Y loadings), which offers a representation of a linear relationship between variables (loadings) and samples (scores). In order to better visualize the results from the PLS analysis, molecular categories, calculated indices and intensity-weighted ratios were also passively displayed in the PLS model, thus they do not influence the model but their location in the PLS indicates their correlation with other variables. Molecular loadings from PLS analysis were also represented in van Krevelen diagrams to better visualize the trends in the relative abundance of the defined molecular categories (see section “Molecular Analysis of DOM via FT-ICR-MS,” Supplementary Figure S1) in relation to the environmental parameters.
To test our overarching question whether spatial and temporal variations in oceanographic regimes are reflected in the molecular composition of DOM in sediment porewaters, we evaluated data from summer expeditions to Fram Strait in two different years, 2013 and 2014. The sampling campaigns covered longitudinal variations (East-West) between stations of similar water depths (EGI-EGIV vs. HGI-HGIV) sampled during 2014, as well as latitudinal trends (North-South, N4 vs. S1-S3) in 2013. Furthermore, temporal variations (2013 vs. 2014) were determined for eastern stations sampled during both years (HGI-HGII and N4) (for sampling details see section “Sampling Sites and Sample Description”). All data were evaluated with two multivariate statistical analyses (partial least squares, PLS). The first PLS was performed to constrain the role of environmental parameters (longitudinal, latitudinal and temporal variations) for the porewater DOM composition of all sampled sediment depths. In a second approach, only variations in surface (0–1 cm) porewaters and sediments were assessed. This is where we expect strongest variations due to the input and remineralization of fresh organic material. Benthic environmental parameters were available at a different resolution (i.e. 0–5 cm in 1 cm intervals), but overlapped with DOM measurements for the uppermost horizon of 0–1 cm. Thus, the relationship between DOM and sediment parameters could not be tested for deeper sediment layers.
The ice margin (defined as ≥15% ice cover) in the HAUSGARTEN area was located closest to the southern (S1, S2, and S3) and the shallower eastern stations (KH and HGI), where the latter were sampled in both years (Figure 1). Ice cover ranged between 0 and 31% for stations in the eastern part of the Strait, and a maximum ice cover of 57% at the northernmost station in June 2013. In June 2014, ice cover ranged between 10–38% for eastern stations, and 70–91% for western and northern stations (Supplementary Table S1). Average sea ice concentrations over the past 5 years reflected similar conditions, with average ice coverage of 0–7% for stations in the eastern and southern part of the Strait, 17–20% at the northern station and 69–84% at western stations. Hydrographical features varied between the three stations that were sampled in both years (i.e., HGI, HGII in the eastern and N4 in the northern part of the sampling area). While at the time of sampling in both years most stations were characterized by Atlantic water and a 30–50 m thick layer of Polar water at the surface, HGI and N4 showed some differences between the years (MSM29, 2013: Wenzhöfer et al., 2013; PS85, 2014: Rabe et al., 2014). During sampling in 2013, HGI was characterized by Atlantic instead of Polar water at the surface, the former of which is usually more nutrient-rich (Randelhoff et al., 2018). At the same time also N4 was characterized by warmer temperatures at the surface in 2013 (≥1°C), suggesting the local absence of ice. In contrast, during sampling in 2014, N4 was characterized by cold surface waters close to the freezing point and with low salinity, indicating the presence of melting ice. Thus, although sampling was performed in the same summer months, ice and hydrographic conditions varied between years and stations.
Satellite-derived chlorophyll concentrations in surface waters of the eastern HAUSGARTEN area, as an indicator of phytoplankton biomass, showed highest values in June 2013 and June 2014, i.e., shortly before or during the time of sampling (Supplementary Figure S2). However, the bloom was much stronger (i.e., higher chlorophyll concentrations) in 2013 compared to 2014 (Figure 2 in Engel et al., 2019). Satellite-derived chlorophyll concentrations integrated over the HAUSGARTEN area from April to June were 1.5x higher in 2013 compared to 2014 (4 vs. 2.5 mg Chl a m–3) (Engel et al., 2019, Dinter pers. comm.). In 2013 sampling took place about 2–3 weeks after the bloom (6–7 weeks after the first appearance of the bloom), while in 2014 sampling took place in the middle of the bloom, i.e., about 3 weeks after the first chlorophyll peak in the area (see Engel et al., 2019 for indication of blooms based on satellite chlorophyll data for the region).
POC fluxes were measured at the central (HGIV) and northern (N4) HAUSGARTEN stations. Peak POC fluxes from the upper water column (∼200 m water depth) occurred in April and March/April of 2013 and 2014, respectively, and were much higher in 2013 (21 mg POC m–2 d–1 in 2013; 5 and 8 mg POC m–2 d–1 in 2014 Supplementary Table S3).
Variations in phytodetritus input as indicated by phytodetritus pigment concentrations in the sediments were related to water depth and were more pronounced in the eastern (HGI-HGIV) than at similar water depths in the western (EGI-IV) Fram Strait (Figures 1, 2). During both sampling years, highest CPE concentrations (see methods for definitions of phytodetritus pigments) were detected in surface sediments (0–1 cm) and decreased in deeper sediment layers (Supplementary Table S1). Surface CPE concentrations generally decreased with increasing water depth in the eastern Fram Strait, while in the western part only a slight decrease was observed (Figure 2 and Supplementary Table S1). An exception to the decreasing CPE trend with water depth in the eastern Fram Strait was the deepest station (HGIX), at which also higher values of TOC, phospholipids and protein content were detected in sediments (Supplementary Table S1). Surface sediment CPE concentrations in the western Fram Strait were on average much lower than at similar water depths in the East (Supplementary Table S1). Nevertheless, based on the proportion of chl a, the phytodetrital material at stations in the western and eastern Fram Strait was similarly fresh. In agreement with the differences in surface CPE concentrations between eastern and western Fram Strait, sediment TOC, phospholipid and protein content exhibited generally lower concentrations at western than at eastern stations (Supplementary Table S1).
Figure 2. Pigment concentrations. Phaeopigments and Chlorophyll content for surface sediments in 2013 and 2014 (A,B, respectively). The discontinuity in the black arrow in panel (A), indicates that HGII, HGI, KH are at a similar latitude. Stations written in gray font were sampled in both years.
Temporal variations in CPE concentrations and % chl a for stations sampled during both cruises (HGI, HGII, N4, Figure 1) indicate a larger and more recent (based on the avg. CPE and % chl a, respectively), phytodetritus deposition in 2013 compared to 2014, especially at shallower stations (HGI-II; Figure 2, Supplementary Table S1). Latitudinal variations in CPE in the eastern Fram strait were not as pronounced as the longitudinal trends. Surface CPE concentrations were slightly lower at the southern (S1-S3) than at the northern (N4) stations, although similarly fresh (Supplementary Table S1). Furthermore, based on the 2013 data, sediment TOC, enzymatic activity and phospholipid content increased from north to south (Supplementary Table S1). Chlorophyll pigment concentrations in the top 5 cm of sediment were significantly correlated (p ≤ 0.05; Table 1) with benthic community activity and biomass (esterase activity, phospholipid and protein concentrations) and TOC content in sediments (Supplementary Figure S3). Bacterial abundance and biomass (Supplementary Table S1) in surface sediments (0–1 cm), were not significantly correlated with phytodetrital pigments, but followed similar patterns (e.g., higher avg. numbers at eastern compared to western stations, Supplementary Table S1).
Table 1. Pearson correlation matrix between DOC and TDN concentrations, and phytodetrital proxies and benthic community data from the LTER HAUSGARTEN program for the top 5 cm of sediment.
Dissolved organic matter concentrations in porewater were generally higher in surface sediments and also significantly lower (p < 0.05 for paired sample t-test) in western Fram Strait compared to similar water depths in the eastern Strait (Supplementary Table S1 and Figure 3). Additionally, on the eastern side (KH, HGI-HGIX), DOC concentrations decreased with increasing water depth (Supplementary Table S1 and Figure 3). Comparing eastern stations sampled in both years, generally higher DOC concentrations were observed in 2013 (HGI and HGII, Supplementary Table S1 and Figure 3). Latitudinal trends indicated that DOC concentrations in the eastern Fram Strait increased from north to south (N4 to S1-S3, Supplementary Table S1 and Figure 3), following the trend of sediment TOC, enzymatic activity and phospholipid content (Supplementary Table S1).
Figure 3. Concentrations of dissolved organic carbon (DOC) and total dissolved nitrogen (TDN) in all analyzed porewaters for the years 2013 (A,C) and 2014 (B,D). Note that sampling in 2013 displays latitudinal variations and 2014 longitudinal trends. The discontinuity in the black arrow in (C), indicates that HGII, HGI, KH are at a similar latitude. Stations written in gray font were sampled in both years.
Contrary to surface porewater DOC concentrations, TDN concentrations did not decrease with increasing water depth. However, they were lower in the western (EGI-EGIV) than at similar water depths in the eastern Fram Strait (HGI-HGIV) and higher in 2013 compared to 2014 (HGI and HGII, Supplementary Table S1 and Figure 3). Contrary to DOC, latitudinal trends in the eastern Fram Strait showed a decrease in TDN concentrations from north to south (Supplementary Table S1 and Figure 3).
Both DOC and TDN concentrations in porewater were significantly correlated with each other and also with phytodetrital data, enzymatic activity and phospholipid content in the sediments (p ≤ 0.05; Table 1). Additionally, porewater DOC was also significantly correlated with sediment TOC, both also negatively correlated with sea ice cover and distance from the ice margin. The observed differences between eastern and western Fram Strait were also evidenced by a significant correlation between longitude and porewater DOC and TDN, and phytodetrital pigments, phospholipid, protein and TOC content in sediments (Table 1), all of which were significantly different between east and west (p < 0.05 for paired sample t-test), especially at the stations shallower than 2000 m (EGI-III and HGI-III).
Dissolved organic matter and TDN concentrations in bottom waters (defined as the overlying water collected above the freshly sampled sediment) were two- to four-fold and one- to two-fold lower than in surface sediment porewaters, respectively. Furthermore, DOC and TDN concentrations did not follow variations in water depth, contrary to surface porewater DOC (Supplementary Table S1 and Supplementary Figure S4).
Bottom water DOC concentrations varied spatially and were generally lower and less variable at western stations (EGI-EGIV) compared to similar water depths in the East (HGI-HGIV; Supplementary Table S1 and Supplementary Figure S4). Contrary to DOC, TDN concentrations did not display big differences between stations in the western and eastern Fram Strait.
Comparing both sampling years, DOC and TDN concentrations in bottom waters were higher in 2013 than in 2014 (HGI-HGII, Supplementary Table S1 and Supplementary Figure S4) and also higher at shallower stations located ≤1500 m water depth (KH, HGI and HGII in 2013, Supplementary Table S1 and Supplementary Figure S4).
As a result of the DOC gradients between porewater and bottom waters, which were used to calculate DOC flux, an export of DOC from surface sediments to the overlying bottom water (DOC efflux) was observed for all sampling stations (Supplementary Table S1). Along with the general trend in DOC efflux, spatial and temporal variations were also observed. The average DOC efflux was two-fold lower on average for the western stations compared to similar water depths in the eastern Fram Strait (Supplementary Table S1). Furthermore, southern stations had higher DOC efflux than further north (Supplementary Table S1). Also, eastern stations displayed higher DOC efflux in 2013 than in 2014 (Supplementary Table S1).
Comparing bottom water, surface porewater (0–1 cm) and deeper porewater (1–5 and 5–10 cm) samples on a molecular level, bottom water DOM had a lower number of formulas compared to surface and deeper porewaters (Figure 4). Most of these formulas were shared and only 2, 3, and 22% of each pool were represented by unique formulas (Figure 4). On a compositional level, shared formulas among the three DOM pools were represented by more degraded DOM, with more than half of them associated with highly unsaturated formulas (Figure 4). Nevertheless, fresh molecular indicators (molecular categories previously associated with phytodetritus input; Rossel et al., 2016) such as unsaturated aliphatics and unsaturated-N also contributed to the shared fraction with 27% (Figure 4).
Figure 4. Venn diagram with molecular formulas identified in the whole dataset. Number of formulas detected in bottom water, surface (0–1 cm) and deeper porewater (1–5, 5–10 cm) pools are indicated in bold inside the parentheses. Number of unique formulas in each pool and those shared between two or all pools are color coded and displayed in parentheses. Number of formulas contributing to specific molecular categories are indicated in italics also in parentheses. In the gray circle (with formulas shared between the three pools) “others” represent the sum of aromatics, polyphenols and sugars.
Comparing porewater with bottom water, it was found that less formulas in surface porewater were absent in bottom water compared to those in deeper porewater. Consequently, bottom water was more similar to surface porewater than to deeper porewater (see shared formulas between two pools, Figure 4). The main difference between bottom water, surface and deeper porewaters was the increase of unique formulas associated with highly unsaturated formulas toward the deeper porewater (Figure 4).
Dissolved organic matter in surface porewater was represented by 58% of highly unsaturated, 14% of unsaturated aliphatics and 13% of unsaturated-N formulas, of which 10, 11, and 23% were not detected in bottom water, respectively. In deeper porewaters a smaller fraction was absent (2, 8, and 10%, respectively). Consequently, more formulas in surface porewater (associated with both degraded and fresh OM) were absent from bottom water than from deeper porewater.
The higher number of unsaturated-N formulas in surface porewaters compared to bottom waters was also reflected in a higher relative abundance of these formulas as indicated by higher freshness indices, such as MLBwl and Ibioprod, observed in surface porewaters compared to bottom waters (Supplementary Table S5). Despite the higher number of highly unsaturated formulas in surface porewater compared to bottom water, which could indicate more degraded DOM in the former pool, only minor differences were detected for the calculated degradation index, IDeg (Supplementary Table S5). This could be related to the fact that the IDeg index was established for water column samples (see section “Discussion”). Also, DOM in bottom waters was generally characterized as more unsaturated (DBEwa), had higher molecular weight (MWwa), but lower relative abundances of aromatics (especially polyphenols) compared to surface porewater (Supplementary Tables S4, S5).
Similar to bulk data, the DOM composition also displayed longitudinal differences. Although bottom water from the western and eastern Fram Strait shared similar number of formulas with their surface porewaters, more unique formulas were observed in bottom waters of the western compared to the eastern Fram Strait (Supplementary Figures S5a,b, respectively). These unique formulas in the western Fram Strait, which were in the central area of the van Krevelen diagram (Supplementary Figure S5a), were predominantly CRAM-like (Supplementary Figure S1). Additionally, the lower relative abundance of aromatics detected in bottom water compared to surface porewater was more pronounced, especially for polyphenols, at eastern stations (HGI-HGIV) compared to similar water depths in the western Fram Strait (EGI-EGIV, Supplementary Table S5). Other molecular categories that behaved differently along the longitudinal transect (Supplementary Table S5) were unsaturated aliphatic O-rich formulas. These formulas were only significantly higher (p < 0.05 for paired sample t-test) in surface porewaters compared to bottom waters at the eastern stations (HGI-HGIV), but not at the western stations (EGI-EGIV; Supplementary Table S5).
Temporal variations indicated higher numbers of shared formulas between surface porewater and respective bottom waters in 2013 than in 2014 (HGI-II comparison, Supplementary Figures S5c,d). Of the shared formulas in 2013, 25% were not detected in bottom water in 2014 with more than half of them in the higher H/C region of the van Krevelen diagram (Supplementary Figure S5c), commonly associated with fresh molecular indicators such as unsaturated aliphatics and unsaturated-N (Supplementary Figure S1). This is consistent with a higher relative abundance of unsaturated aliphatic O-rich and unsaturated-N formulas in bottom waters in 2013 compared to 2014 (Supplementary Table S5).
In sediment porewaters (0–10 cm) from HAUSGARTEN, variations in the relative abundance of molecular categories were related to differences between eastern and western regions of the Strait, evaluated based on 2014 data, and with interannual variability for stations in the eastern part that were sampled in both years. At stations with similar water depths, mainly polyphenols displayed significant differences (p < 0.05 for paired sample t-test) between western (EGI-IV) and eastern (HGI-IV) Fram Strait, with higher contribution in the latter location (Supplementary Table S5).
At stations with similar water depths in the western and eastern Fram Strait, fresh molecular indicators such as unsaturated aliphatics O-rich and O-poor displayed different trends. Unsaturated aliphatics O-poor were significantly higher (p < 0.05) in the western Fram Strait, while O-rich aliphatics were similar at both locations (p = 0.3). However, comparing both sampling years, unsaturated aliphatics O-rich were two-fold higher in 2013 than in 2014 (HGI-II, Supplementary Table S5). Also, the contribution of unsaturated-N formulas was significantly higher (p < 0.05) in the western Fram Strait. However, the contribution of unsaturated-N formulas was two-fold higher during 2013 than in 2014 (HGI-HGII). The contribution of unsaturated-N formulas to the total N-containing compounds was on avg. 14 ± 6% and 10 ± 4% for western and eastern stations of similar water depths, respectively, and 19 ± 4% for HGI-HGII in 2013.
To assess the role of the environment on the molecular DOM composition of HAUSGARTEN porewaters, two partial least squares (PLS) analyses were performed: The first PLS included all porewater samples (i.e., 0–1, 1–5, 5–10 cm) and the second only evaluated surface porewaters (0–1 cm, Supplementary Table S1) to assess the role of benthic parameters in shaping the composition of surface porewater DOM (see first paragraph of section “Results”).
Based on the first PLS analysis (Supplementary Tables S6, S7), DOM composition was explained by two axes (Figure 5). The first axis, factor 1, explained 48% of the environmental (X loadings) and 11% of the DOM molecular data (Y loadings) variability, while factor 2 explained 18% and 5%, respectively (Figure 5). Factor 1 was scaled with DOC and TDN concentrations, which negatively correlated with sea ice cover and the distance to the ice margin. DOC and sea ice variables significantly influenced DOM composition (uncertainty test <2 Std. based on cross validation; Figure 5D). Samples with higher DOC concentrations were mainly collected in 2013 and from eastern Fram Strait (KH, HGI-HGII ≤ 1500 m water depth, Figures 5B,C). These stations were characterized by higher abundance of fresher DOM (higher relative abundance of unsaturated-N and unsaturated aliphatic O-rich formulas and higher indexes of lability MLBwl, and lower degradation, Ideg, Figure 5D). Furthermore, longitude also significantly influenced the DOM composition of porewaters, separating eastern (mainly KH, HGI-HGII) and western Fram Strait (EGI-EGIII) samples along both axes (factor 1 and 2; Figure 5B). Along the first axis, eastern (HGI, HGII, and KH) and western Fram Strait (EGI-EGIII) were separated, due to the higher abundance of oxygen-rich compounds (higher O/Cwa), polyphenols and higher number of formulas in eastern compared to western porewaters (Figure 5D). The second axis was mainly associated with sediment and water depth, which did not significantly influence the DOM composition of the porewaters beyond the surface layer, i.e., 1–5 and 5–10 cm porewater overlapped, and its DOM was overall more degraded, more aromatic and had higher molecular weight (higher Ideg, AImodwa, MWwa) and abundance of CRAM-like formulas (Figures 5A,D).
Figure 5. Partial Least Square (PLS) analysis of all porewater samples with factor 1 explaining 48 and 11% of the environmental and molecular variability, respectively, and factor 2 explaining 18 and 5%. (A) Scores plot (samples indicated by the name of the station, Supplementary Table S1) color coded with sediment depth, (B) longitude and (C) DOC concentration, and (D) correlation of environmental (X loadings) and molecular loadings (Y loadings), passively displaying the evaluated molecular categories, ratios and indices (see molecular indicators in Supplementary Figure S1 and Supplementary Table S2). Underlined environmental variables significantly influence porewater DOM composition. Sea ice variables: Sea Ice_montly, Sea Ice_5yrs and NearDist_sampl_yr, stand for sea ice monthly average, 5 years average and nearest distance to the ice margin (see section “Sea Ice Conditions,” Supplementary Table S1).
Based on the second PLS analysis including only DOM from surface porewaters in relation to benthic environmental parameters (Supplementary Tables S8, S9), DOM composition was explained by two axes (Figure 6). The first axis, factor 1, explained 49% of the environmental (X loadings) and 18% of the DOM molecular data (Y loadings) variability, while factor 2 explained 17 and 8%, respectively (Figure 6). Similar to the first PLS (Figure 5), the first axis was influenced by DOC and TDN concentrations, which also correlated with phytodetritus concentrations (CPE, Chl a and phaeopigments) (TDN and phytodetritus proxies significantly influenced surface porewater composition; uncertainty test <2 Std. based on cross validation; Figure 6D). Surface porewater DOM composition was mainly separated between those samples collected in 2013 at shallower stations of the eastern Fram Strait (KH, HI-HGII) and those collected in 2014 at deeper stations of the bathymetric transect (HGV, VI and HGIX) and in the western Fram Strait (especially EGI-EGIII) (Figures 6B,D). This separation was due to the higher concentrations of DOC, TDN and phytodetritus at the eastern stations in 2013. Higher phytodetritus concentrations were accompanied by higher protein, TOC and phospholipid content and enzymatic activity in the sediments, as well as higher numbers of formulas, and higher relative abundances of CHON and unsaturated aliphatic O-rich formulas in porewater DOM (Figures 6D, 7A). The second axis was influenced by water depth, and prokaryotic abundance and biomass. Abundance and biomass were higher at the eastern (HGI collected in 2014) and southern stations (S2-S3, collected in 2013; Supplementary Table S1). These stations were characterized by porewater DOM that had higher molecular weight (MWwa) compared to the DOM from shallower but permanently ice-covered stations (EGI-III, Figure 6D). Porewater DOM from deeper stations in the eastern Fram Strait (HGV, HGVI, and HGIX) was more aromatic than at shallower stations in the same region (higher AImodwa; Figure 7B).
Figure 6. Partial Least Square (PLS) analysis of surface porewater (0–1 cm) samples with factor 1 explaining 49 and 18% of the environmental and molecular variability, respectively, and factor 2 explaining 17 and 8%. (A) Scores plot (samples indicated by the name of the station, Supplementary Table S1) color coded with CPE, (B) longitude and (C) protein content, and (D) correlation of environmental (X loadings) and molecular loadings (Y loadings), passively displaying the evaluated molecular categories, ratios and indices (see molecular indicators in Supplementary Figure S1 and Supplementary Table S2). Underlined environmental variables are significantly influencing surface porewater DOM composition. Sea ice variables: Sea Ice_montly, Sea Ice_5yrs and NearDist_sampl_yr, stand for sea ice monthly average, 5 years average and nearest distance to the ice margin (Supplementary Table S1).
Figure 7. van Krevelen diagrams (VKD) of H/C and O/C ratios using the PLS molecular loadings from Figure 6D to summarize the main trends in the molecular composition of surface porewater. (A) VKD with factor 1 loadings and (B) factor 2 loadings in the z axis indicated in the color bar to the right, where the relationship to sea ice cover and water depth are indicated (see Figure 6). For definition and location on the VKD of discussed molecular categories see section “Molecular Analysis of DOM via FT-ICR-MS,” Supplementary Figure S1 and Supplementary Table S2.
Environmental conditions at the LTER HAUSGARTEN in Fram Strait differed between regions and years. While the eastern Strait off Svalbard is mostly ice free during the year, the western part off Greenland is characterized by sea ice coverage of over 50%. This influences the input of POM to the seafloor, which was also reflected in CPE concentrations in surface sediments, with much higher values in the East compared to the West (Figure 1). This trend was also reflected in other benthic parameters, e.g., sediment TOC, phospholipids and protein content. An exception to the trends observed in the eastern Fram Strait was the deepest station (HGIX), consistent with the funnel-like topography of this station that facilitates accumulation of phytodetrital matter (Cathalot et al., 2015). In 2014 both regions were sampled, and while the eastern Fram Strait exhibited post-bloom conditions at the time of sampling, the western Fram Strait was likely still in a pre-bloom stage; the estimated productivity since winter was twice as high in the East when compared to the West (Fadeev et al., 2018). Regionally, the two parts of the Strait thus clearly differ in productivity and the amount of POM that is received by the seafloor, in agreement with previous reports (Fadeev et al., 2018; Hoffmann et al., 2018; Käss et al., 2019). In addition, there were also temporal differences between 2013 and 2014. The higher productivity indicated by satellite-derived chlorophyll concentrations in 2013 may have been supported by different hydrographical features, namely by the presence of nutrient-rich Atlantic waters at the surface of HGI and less ice and thus higher light availability at N4. Higher productivity in 2013 was followed by about three times higher POC export from the upper water column. The larger export event in 2013 may have also resulted in a faster input of fresher phytodetritus to the seafloor, which is supported by higher surface sediment CPE concentrations and chlorophyll a proportions in 2013 compared to 2014. All of these variations were well reflected in porewater DOC concentrations, which were higher in the eastern compared to the western Fram Strait and higher in 2013 compared to 2014, in agreement with the higher OM availability (assessed with chlorophyll pigment content) in those regions and year.
Also, DOM composition in porewaters from sediments collected at the LTER HAUSGARTEN differed regionally (East vs. West), as well as interannually (2013 vs. 2014). Porewaters from stations sampled in 2013 from shallower water depth (≤1500 m) and seasonally ice-covered regions (KH, HGI-II, located in the eastern Fram Strait), were associated with fresher DOM signals (higher number of formulas, and abundance of aliphatics formulas and N-containing compounds). Higher contribution of this “fresh” DOM concurs with higher benthic biomass (phospholipids and protein content), enzymatic activity, TOC and phytodetritus proxies in surface sediments of the eastern Fram Strait. On the contrary, the DOM composition of porewaters collected in 2014 at deeper water depths in the eastern Strait were associated with higher abundances of more degraded compound classes (CRAM-like, highly unsaturated oxygen-poor molecular formulas and more aromatic compounds), in agreement with the influence of water depth on benthic remineralization mainly in the eastern Fram Strait (Hoffmann et al., 2018). In the western Fram Strait (EGI-EGIII) porewater DOM was dominated by oxygen-poor compounds (lower O/Cwa) in agreement with their association with more degraded material and a lower contribution of fresh material at these permanently ice-covered locations. The observed differences in the sediment DOM pool are in agreement with surface water conditions at the time of sampling during 2014. Because the eastern Fram Strait was characterized by post-bloom conditions (Fadeev et al., 2018) and peak fluxes of POC had already occurred in April, there was likely already an input of fresh organic material to stations in the East, which resulted in higher DOC concentrations and fresh DOM signals due to the breakdown of fresh POM by benthic communities. In contrast, western stations had likely not received (as much) fresh organic material and receive lower inputs of organic material in general, due to the higher ice cover, resulting in a more degraded DOM pool. Differences in DOM composition between north and south were minor compared to those between western and eastern Fram Strait.
Compared to the central Arctic basins which are permanently ice-covered and strongly nutrient limited, the Fram Strait is much more productive. Overall, surface sediment CPE concentrations at HAUSGARTEN were between 10 to 400-fold higher compared to the Arctic continental slope and the central Arctic (Boetius and Damm, 1998; Rossel et al., 2016), indicating a much larger input of OM in this region. Proportions of chlorophyll a (% chl a relative to all pigments) were similar between the eastern and western regions, indicating similarly “fresh” material, although overall pigment concentrations differed considerably. Chlorophyll a proportions at HAUSGARTEN sediments were higher compared to similar water depths at the Arctic continental slope (Boetius and Damm, 1998), but in a similar range or lower than those previously reported from multiyear ice-covered stations and ice margin stations in the central Arctic (12 and 20%, respectively; Rossel et al., 2016). These values, however, significantly depend on the type of OM that is exported (e.g., quality, fast sinking vs. slow sinking), as well as the timing between deposition at the seafloor and subsequent sampling, and are thus difficult to compare directly.
Bottom water DOC and TDN concentrations in both years were in the range of those reported for the central Arctic (Rossel et al., 2016). Concentrations of DOC were higher in surface sediments than in bottom waters and were in the higher range of those reported for ice margin stations in the central Arctic (Rossel et al., 2016), reflecting the higher OM input especially in the eastern Fram Strait in concert with higher CPE and TOC concentrations in the sediments. Compared to the central Arctic, porewater DOC concentrations in the western Fram Strait were similar to those reported at multiyear ice-covered stations, while those at the eastern side were similar or higher than those at the ice margin (avg. ± SD of 134 ± 34 and 212 ± 78 μmol/L, respectively; Rossel et al., 2016). Compared to other marine systems, DOC concentrations in Arctic porewaters of Fram Strait were in the lower range, and those of the Central Arctic substantially lower than that of other oceans (Burdige and Komada, 2015; Schmidt et al., 2017; Fox et al., 2018; Loginova et al., 2020). Nonetheless, the gradients between bottom water and porewater are in line with previous reports indicating at least one magnitude higher DOC concentration in porewater compared to bottom water, supporting the role of POM diagenesis in surface sediments for the production of DOC and flux to overlying bottom water (Schmidt et al., 2011; Burdige and Komada, 2015; Loginova et al., 2020).
DOC fluxes from the sediment followed the trends observed for surface porewater DOC concentrations, with higher fluxes in the eastern Strait and in 2013, in agreement with previously reported higher bacterial benthic remineralization in the East, especially at shallower stations (Hoffmann et al., 2018). The calculated DOC flux values from eastern Fram Strait were two to six-fold higher (except for station N4 in the north) than the values reported for ice margin stations in the central Arctic (Rossel et al., 2016). Please note that we re-calculated efflux values for the Central Arctic from this earlier paper to match the model of DOC flux applied for HAUSGARTEN sediments, i.e., assuming fluxes in the sediment rather than across the diffusive boundary layer (see section “DOC Efflux From Porewater Gradients” and Supplementary Table S10). Compared to other deep-sea sediments from the Atlantic (ca. 4500 m water depth) and Antarctic Ocean (<2500 m water depth), DOC fluxes in the western Fram Strait were in the lower range, while DOC fluxes in the eastern Fram Strait (HG, S1-3 and N4 stations) were up to 9 fold higher (37–259 and 66 mmol/m2/yr for Weddell Sea and for Atlantic, respectively; Hulth et al., 1997; Hall et al., 2007). DOC fluxes from Arctic shelves located far from our sampling area, and from productive systems were similar or higher than those from the eastern shallower stations in our study (Hulthe et al., 1997; Loginova et al., 2020), supporting the hypothesis of strong links to productivity regimes and the availability of OM at the seafloor.
HAUSGARTEN porewaters (0–10 cm) displayed similar contributions of highly unsaturated formulas compared to those reported for ice margin stations in the central Arctic (Rossel et al., 2016). Of these formulas, 73% were CRAM-like compounds, slightly lower than the values reported for central Arctic porewaters (79%; Rossel et al., 2016). However, much higher contributions of polyphenols were detected at HAUSGARTEN compared to the central Arctic (polyphenols ranged between 1.9–3.6%; Rossel et al., 2016). According to previously reported relationships between polyphenols and terrigenous OM (Seidel et al., 2015b), our results suggest a higher contribution of terrigenous material in the Fram Strait (increasing from the western to the eastern side) compared to the central Arctic (Rossel et al., 2016), in agreement with the location of HAUSGARTEN and with the increase of terrestrial biomarkers nearby the Svalbard Archipelago (Lalande et al., 2016).
Molecular formulas of unsaturated aliphatics O-rich and unsaturated-N, previously associated with fresh material, displayed similar to lower contributions compared to ice margin stations in the central Arctic (unsaturated aliphatics O-rich and unsaturated-N avg. 1.6 ± 0.7 and 7.6 ± 8.7, respectively; Rossel et al., 2016). Nevertheless, the contribution of unsaturated-N formulas to the total N-containing compounds was similar or lower at HAUSGARTEN than in the central Arctic (unsaturated-N formulas at the ice margin and multiyear ice stations avg. 40 ± 19% and 17 ± 6%, Rossel et al., 2016). This difference may indicate that at HAUSGARTEN some organic N is stored in compounds that may be less accessible for microorganisms or that less fresh material reaches the benthos at these locations. Indeed, proportions of chlorophyll a were slightly higher at ice margin stations in the central Arctic, probably due to the input of fast-sinking algae aggregates at the time of sampling (Boetius et al., 2013). Although the abundance of unsaturated-N formulas was lower at HAUSGARTEN than in the central Arctic, higher proportions were observed in 2013 than in 2014, favoring the idea that an increase in phytodetritus would increase the contribution of unsaturated-N formulas in porewater.
Contrary to previous observations in the central Arctic (Rossel et al., 2016), sediment depth (top 10 cm) was not a main driving force of porewater DOM composition, but rather spatial (east and west differences) and temporal variability in OM input. The lack of clear gradients in DOM molecular patterns with sediment depth is likely due to more active bioturbation at HAUSGARTEN sediments compared to the central Arctic (Soltwedel et al., 2019).
Overall, the associations between porewater DOM composition and food availability (i.e., phytodetritus proxies) were similar to ones previously reported for central Arctic sediments (Rossel et al., 2016). Porewater DOM composition in surface sediments at HAUSGARTEN was, based on our multivariate statistical analysis, correlated to enzymatic activity, and phospholipid and protein content, but not with bacterial abundance and biomass, which did not show large differences between eastern and western Fram Strait. Furthermore, porewater DOM composition in the Arctic Ocean may rather be related to the composition of bacterial groups than to their total abundance, in agreement with previous observations in coastal seafloor (Oni et al., 2015) and deep-sea sediments (Jessen et al., 2017; Pop Ristova et al., 2017).
The molecular composition of bottom water DOM was characterized as more unsaturated and with higher molecular weight (DBEwa and MWwa) compared to surface porewater, in agreement with previous observations in central Arctic sediments (Rossel et al., 2016). However, in the Black Sea generally larger and more unsaturated formulas are accumulated in the porewater compared to bottom water (Schmidt et al., 2011), which may result from a different composition of the OM reaching the seafloor in oxic and anoxic ocean basins. Furthermore, bottom water is reported to display a lower richness of molecular formulas and N-containing compounds compared to porewater (Schmidt et al., 2011, 2017), in agreement with our observations in the Arctic Ocean (Rossel et al., 2016; and this study). For this study, we found that only 2% of the formulas present in bottom water were unique to this pool. However, more formulas in surface (16% of the formulas) and deep porewater (32% of the formulas) were absent or below detection in bottom water. Because 84% of the DOM formulas in surface porewater were also found in the bottom water, overlying bottom water reflects surface porewater composition due to an exchange across the sediment-water interface. This observation aligns with the DOC efflux and a more similar composition between bottom water and surface porewater DOM than between bottom water and subsurface porewater (Schmidt et al., 2017; and this study). However, even a higher number of formulas in surface porewater DOM (96% of its formulas) were shared with the subsurface (here defined as 1–10 cm). Therefore, surface sediment DOM is a source for bottom water and subsurface sediments, but the bacterial communities appear to filter specific components of DOM in surface sediments, and allowed less molecular formulas to escape into the bottom water (16% of it) than to subsurface sediments (4%). In the subsurface, overall DOM composition was most diverse (the number of unique formulas increased from bottom water to subsurface porewater), especially due to the presence of more degraded DOM (highly unsaturated formulas). Thus, the bacterial communities in surface and subsurface sediments reworked DOM, affecting its composition substantially. The calculated average diffusion time for a DOM molecule in the sediment is 0.01 yr per 1 cm. The DOM composition in the upper 10 cm of sediments suggests that the signal of recent phytodetritus was restricted to surface sediment porewaters, indicating that the signal is rapidly lost after deposition, due to porewater DOM alteration by the bacterial communities in the sediment. Our observations are in agreement with the increase of more refractory DOM with increasing sediment depth detected by nuclear magnetic resonance (Fox et al., 2018) and optical properties (Loginova et al., 2020) of porewater DOM in other marine sediments.
Unsaturated-N formulas, which have previously been associated with fresh OM input, were higher in surface porewaters than bottom water (also referred to as peptide formulas; Rossel et al., 2016; Schmidt et al., 2011, 2017). Unsaturated-N contributions were in agreement with other molecular indicators in surface porewater (higher MLBwl and Ibioprod), which suggests a higher contribution of fresh and bioproduced material in surface porewaters compared to bottom waters. This observation is in line with the deposition and accumulation of OM at the seafloor, where the bulk degradation of OM is mediated by microbial communities in surface sediments by a wider spectrum of active enzymes (Arnosti, 2011; Middelburg, 2018). However, the calculated degradation index, IDeg, did not reflect differences in composition between bottom water and surface porewaters. This may indicate that the application of this index, which was defined based on the correlation of molecular formulas to radiocarbon age for water column DOM (Flerus et al., 2012), cannot be used to compare overlying bottom water and surface porewater due to the exchange of DOM across their interface.
Unsaturated aliphatic O-rich formulas, which have also been reported to be higher in central Arctic sediments than in their overlying bottom waters (Rossel et al., 2016), only followed this trend in the eastern Fram Strait in agreement with their relation to higher phytodetritus input at this location. Although higher contribution of these compounds was observed in surface porewater, molecular indicators associated with fresh material (unsaturated aliphatics O-rich and unsaturated-N) were also higher in bottom water in 2013 compared to 2014, in agreement with higher OM input and DOC efflux in the former year. Nevertheless, a comparison of bottom waters from eastern and western Fram Strait indicated that the latter had higher abundances of unique CRAM-like compounds (absent in their surface porewaters) compared to the East, consistent with less input of fresh material at these permanently ice-covered stations. These data suggest that Arctic sediments are a source of CRAM and fresh DOM to the overlying bottom water, whose contributions vary depending on the quality and quantity of the OM deposited, as previously suggested for other environments (Fox et al., 2018; Loginova et al., 2020).
In this study, we show that regional and interannual differences in the production and deposition of OM are reflected in the DOM pool (DOC concentration and DOM composition) of deep-sea surface sediment porewaters, both regionally as well as across ocean basins. The permanent ice cover in the western Fram Strait limits productivity and OM input to the sediment, resulting in a porewater DOM composition that is characterized as more degraded. In contrast, porewater DOM in the eastern Fram Strait has a stronger signal of fresh phytodetrital matter, which decreased with increasing water depth. In this regard, the FT-ICR-MS method and the DOM freshness indicators used were generally able to resolve local and regional differences in surface productivity, export fluxes and OM availability which varied by at least 40, 70, and 30%, respectively, between years and regions. By comparing our results of Fram Strait to data from the Central Arctic basins, the Atlantic and Black Sea, the study also shows that differences between ocean basin productivity and export regimes are captured in the molecular composition of DOM in pore- and bottom waters. Furthermore, we find evidence for the production of a considerable fraction of DOM formulas related to degradation of OM in subsurface sediments, and a differential export from surface sediments to the bottom water and subsurface layers, indicating the substantial role of surface-sediment bacteria for the transformation of DOM. Although this study only provides a snapshot, our results stress the potential impact of changes in primary productivity and OM export on OM composition and benthic fluxes. In the future, more studies should aim to investigate the temporal scales at which these processes are relevant. A better understanding of seasonal variations will help to contrast these against changes resulting from longer-term environmental shifts. Furthermore, surface porewater DOM composition (which was related to enzymatic activity and the content of protein and phospholipids) was not associated with bacterial abundance and biomass, rather suggesting a coupling at the level of bacterial community composition/function. Therefore, studies exploring the interplay between microbial community composition/function and DOM molecular diversity are necessary to identify key microorganisms involved in the first steps of OM decomposition and production of DOM in porewater.
The data were stored in the Data Publisher for Earth and Environmental Science PANGAEA (www.pangaea.de). Details for 2013 and 2014 data from the LTER observatory HAUSGARTEN and from this study (Bacterial cell counts and cell volume, DOC and TDN, DOM composition, etc.) are reported in the reference list.
PR, CB, and AB designed the study. CB performed the sampling. PR analyzed the data and wrote the manuscript with contributions from CB, AB, LH, and TD.
This work has received funding from the European Research Council (ERC) under the European Union’s Seventh Framework Program (FP7/2007-2013) research project ABYSS (Grant No. 294757) to AB. This publication is Eprint ID 50429 of the Alfred Wegener Institute Helmholtz Center for Polar and Marine research, Bremerhaven, Germany. We are grateful for the funding by the Hanse Institute for Advanced Studies (HWK), the Deutsche Forschungsgemeinschaft (DFG, German Research Foundation No. 422798570) and the Geochemical Society, which made the workshop “Marine Organic Biogeochemistry” at HWK possible.
The authors declare that the research was conducted in the absence of any commercial or financial relationships that could be construed as a potential conflict of interest.
We thank the captain and crew and chief scientists of the RV Maria S Merian expedition MSM29 and RV Polarstern expedition PS85, as well as S. Becker, J. Rapp, and K. Hoffmann for support with sampling on board. We thank M. Friebe, I. Ulber, and K. Klaproth for support during sample analysis. V. Schourup-Kristensen provided chlorophyll maps, and C. Lorenzen and E.-M. Nöthig POC flux data. We also thank F. Wenzhöfer for support with carbon flux calculations, and Wilken-Jon von Appen for advice on interpreting hydrographical data. The work was carried out in the framework of the HGF Infrastructure Program FRAM of the Alfred-Wegener-Institute Helmholtz Center for Polar and Marine Research.
The Supplementary Material for this article can be found online at: https://www.frontiersin.org/articles/10.3389/fmars.2020.00428/full#supplementary-material
Arnarson, T. S., and Keil, R. G. (2001). Organic–mineral interactions in marine sediments studied using density fractionation and X-ray photoelectron spectroscopy. Org. Geochem. 32, 1401–1415. doi: 10.1016/s0146-6380(01)00114-0
Arnosti, C. (2011). Microbial extracellular enzymes and the marine carbon cycle. Annu. Rev. Mar. Sci. 3, 401–425. doi: 10.1146/annurev-marine-120709-142731
Aufdenkampe, A. K., Hedges, J. I., Richey, J. E., Krusche, A. V., and Llerena, C. A. (2001). Sorptive fractionation of dissolved organic nitrogen and amino acids onto fine sediments within the Amazon Basin. Limnol. Oceanogr. 46, 1921–1935. doi: 10.4319/lo.2001.46.8.1921
Bauerfeind, E., Nöthig, E.-M. M., Beszczynska, A., Fahl, K., Kaleschke, L., Kreker, K., et al. (2009). Particle sedimentation patterns in the eastern Fram Strait during 2000–2005: results from the Arctic long-term observatory HAUSGARTEN. Deep Sea Res. Part I Oceanogr. Res. Pap. 56, 1471–1487. doi: 10.1016/j.dsr.2009.04.011
Beszczynska-Moller, A., Fahrbach, E., Schauer, U., and Hansen, E. (2012). Variability in Atlantic water temperature and transport at the entrance to the Arctic Ocean, 1997-2010. ICES J. Mar. Sci. 69, 852–863. doi: 10.1093/icesjms/fss056
Bienhold, C. (2019). Prokaryotic Cell Abundance and Biomass Measured During Maria S Merian Cruise MSM29 and Polarstern Cruise PS85 to the Fram Strait. Bremerhaven: PANGAEA. doi: 10.1594/PANGAEA.908130
Bienhold, C., Boetius, A., and Ramette, A. (2012). The energy–diversity relationship of complex bacterial communities in Arctic deep-sea sediments. ISME J. 6, 724–732. doi: 10.1038/ismej.2011.140
Boetius, A., Albrecht, S., Bakker, K., Bienhold, C., Felden, J., Fernandez-Mendez, M., et al. (2013). Export of algal biomass from the melting Arctic sea ice. Science 339, 1430–1432. doi: 10.1126/science.1231346
Boetius, A., and Damm, E. (1998). Benthic oxygen uptake, hydrolytic potentials and microbial biomass at the Arctic continental slope. Deep Sea Res. Part I Oceanogr. Res. Pap. 45, 239–275. doi: 10.1016/s0967-0637(97)00052-6
Boetius, A., and Lochte, K. (1994). Regulation of microbial enzymatic degradation of organic matter in deep-sea sediments. Mar. Ecol. Prog. Ser. 104, 299–307. doi: 10.3354/meps104299
Børsheim, K. Y., Bratbak, G., and Heldal, M. (1990). Enumeration and biomass estimation of planktonic bacteria and viruses by transmission electron microscopy. Appl. Environ. Microbiol. 56, 352–356. doi: 10.1128/aem.56.2.352-356.1990
Boudreau, B. B. (1997). Diagenetic Models and their Implementation: Modelling Transport and Reactions in Aquatic Sediments. Heidelberg: Springer.
Burdige, D., Berelson, W., and Coale, K. H. (1999). Fluxes of dissolved organic carbon from California continental margin sediments. Geochim. Cosmochim. Acta 63, 1507–1515. doi: 10.1016/s0016-7037(99)00066-6
Burdige, D. J. (2006). Geochemistry of Marine Sediments. Xviii. Princeton, NJ: Princeton University Press.
Burdige, D. J., Alperin, M. J., Homstead, J., and Martens, C. S. (1992). The role of benthic fluxes of dissolved organic carbon in oceanic and sedimentary carbon cycling. Geophys. Res. Lett. 19, 1851–1854. doi: 10.1029/92GL02159
Burdige, D. J., and Komada, T. (2015). “Sediment pore waters,” in Biogeochemistry of Marine Dissolved Organic Matter, 2nd Edn, eds D. A. Hansell and C. A. Carlson (Boston: Academic Press), 535–577. doi: 10.1016/B978-0-12-405940-5.00012-1
Buttigieg, P. L., and Ramette, A. (2014). A guide to statistical analysis in microbial ecology: a community-focused, living review of multivariate data analyses. FEMS Microbiol. Ecol. 90, 543–550. doi: 10.1111/1574-6941.12437
Cathalot, C., Rabouille, C., Sauter, E., Schewe, I., and Soltwedel, T. (2015). Benthic oxygen uptake in the Arctic Ocean margins - A case study at the deep-sea observatory HAUSGARTEN (Fram Strait). PLoS One 10:e0138339. doi: 10.1371/journal.pone.0138339
D’Andrilli, J., Cooper, W. T., Foreman, C. M., and Marshall, A. G. (2015). An ultrahigh-resolution mass spectrometry index to estimate natural organic matter lability. Rapid Commun. Mass Spectrom. 29, 2385–2401. doi: 10.1002/rcm.7400
de Steur, L., Hansen, E., Gerdes, R., Karcher, M., Fahrbach, E., and Holfort, J. (2009). Freshwater fluxes in the East Greenland Current: a decade of observations. Geophys. Res. Lett. 36, 2–6. doi: 10.1029/2009gl041278
Dittmar, T., Koch, B., Hertkorn, N., and Kattner, G. (2008). A simple and efficient method for the solid-phase extraction of dissolved organic matter (SPE-DOM) from seawater. Limnol. Oceanogr. Methods 6, 230–235. doi: 10.4319/lom.2008.6.230
Dittmar, T., and Stubbins, A. (2014). “Dissolved organic matter in aquatic systems,” in Treatise on Geochemistry, eds H. D. Holland and K. K. Turekian (Amsterdam: Elsevier), 125–156. doi: 10.1016/B978-0-08-095975-7.01010-X
Dobricic, S., Vignati, E., and Russo, S. (2016). Large-scale atmospheric warming in winter and the arctic sea ice retreat. J. Clim. 29, 2869–2888. doi: 10.1175/JCLI-D-15-0417.1
Donis, D., McGinnis, D. F., Holtappels, M., Felden, J., and Wenzhoefer, F. (2016). Assessing benthic oxygen fluxes in oligotrophic deep sea sediments (HAUSGARTEN observatory). Deep Sea Res. Part I Oceanogr. Res. Pap. 111, 1–10. doi: 10.1016/j.dsr.2015.11.007
Engel, A., Bracher, A., Dinter, T., Endres, S., Grosse, J., Metfies, K., et al. (2019). Inter-annual variability of organic carbon concentration in the eastern Fram strait during summer (2009–2017). Front. Mar. Sci. 6:187. doi: 10.3389/fmars.2019.00187
Fadeev, E., Salter, I., Schourup-Kristensen, V., Nöthig, E.-M., Metfies, K., Engel, A., et al. (2018). Microbial communities in the East and West Fram strait during sea ice melting season. Front. Mar. Sci. 5:429. doi: 10.3389/fmars.2018.00429
Fahrbach, E., Rohardt, G., Shauer, U., Meincke, J., Osterhus, S., and Verduin, J. (2001). Direct measurements of heat and mass transport through Fram Strait. Polar Res. 20, 217–224. doi: 10.3402/polar.v20i2.6520
Findlay, R. H., King, G. M., and Watling, L. (1989). Efficacy of phospholipid analysis in determining microbial biomass in sediments. Appl. Environ. Microbiol. 55, 2888–2893. doi: 10.1128/aem.55.11.2888-2893.1989
Flerus, R., Lechtenfeld, O. J., Koch, B. P., McCallister, S. L., Schmitt-Kopplin, P., Benner, R., et al. (2012). A molecular perspective on the ageing of marine dissolved organic matter. Biogeosciences 9, 1935–1955. doi: 10.5194/bg-9-1935-2012
Fox, C. A., Abdulla, H. A., Burdige, D. J., Lewicki, J. P., and Komada, T. (2018). Composition of dissolved organic matter in pore waters of anoxic marine sediments analyzed by 1H nuclear magnetic resonance spectroscopy. Front. Mar. Sci. 5:172. doi: 10.3389/fmars.2018.00172
Grebmeier, J. M., Overland, J. E., Moore, S. E., Farley, E. V., Carmack, E. C., Cooper, L. W., et al. (2006). A major ecosystem shift in the northern Bering Sea. Science 311, 1461–1464. doi: 10.1126/science.1121365
Greiser, N., and Faubel, A. (1989). “Biotic factors,” in Introduction to the Study of Meiofauna, eds R. P. Higgens and H. Thiel (London: Smithsonian Institution Press), 79–114.
Hall, P. O. J., Brunnega, J., Hulthe, G., Martin, W. R., Stahl, H., and Tengberg, A. (2007). Dissolved organic matter in abyssal sediments?: core recovery artifacts. Limnol. Oceanogr. 52, 19–31. doi: 10.4319/lo.2007.52.1.0019
Hawkes, J. A., Patriarca, C., Sjöberg, P. J. R., Tranvik, L. J., and Bergquist, J. (2018). Extreme isomeric complexity of dissolved organic matter found across aquatic environments. Limnol. Oceanogr. Lett. 3, 21–30. doi: 10.1002/lol2.10064
Hertkorn, N., Benner, R., Frommberger, M., Schmitt-kopplin, P., Witt, M., Kaiser, K., et al. (2006). Characterization of a major refractory component of marine dissolved organic matter. Geochim. Cosmochim. Acta 70, 2990–3010. doi: 10.1016/j.gca.2006.03.021
Hoffmann, K., Hassenrück, C., Salman-Carvalho, V., Holtappels, M., and Bienhold, C. (2017). Response of bacterial communities to different detritus compositions in arctic deep-sea sediments. Front. Microbiol. 8:266. doi: 10.3389/fmicb.2017.00266
Hoffmann, R., Braeckman, U., Hasemann, C., and Wenzhöfer, F. (2018). Deep-sea benthic communities and oxygen fluxes in the Arctic Fram Strait controlled by sea-ice cover and water depth. Biogeosciences 15, 4849–4869. doi: 10.5194/bg-15-4849-2018
Hulth, S., Tengberg, A., Landén, A., and Hall, P. O. J. (1997). Mineralization and burial of organic carbon in sediments of the southern Weddell Sea (Antarctica). Deep Sea Res. Part I Oceanogr. Res. Pap. 44, 955–981. doi: 10.1016/s0967-0637(96)00114-8
Hulthe, G., Hall, P., and Damm, E. (1997). Benthic carbon fluxes – DOC versus ?CO2 in shelf, slope and deep-sea environments, and relation to oxygen fluxes. Rep. Polar Res. 226, 115–116.
Iversen, N., and Jorgensen, B. B. (1993). Diffusion coefficients of sulfate and methane in marine sediments: influence of porosity. Geochim. Cosmochim. Acta 57, 571–578. doi: 10.1016/0016-7037(93)90368-7
Jacob, M., Soltwedel, T., Boetius, A., and Ramette, A. (2013). Biogeography of deep-sea benthic bacteria at regional scale (LTER HAUSGARTEN, Fram Strait, Arctic). PLoS One 8:e72779. doi: 10.1371/journal.pone.0072779
Jessen, G. L., Lichtschlag, A., Ramette, A., Pantoja, S., Rossel, P. E., Schubert, C. J., et al. (2017). Hypoxia causes preservation of labile organic matter and changes seafloor microbial community composition (Black Sea). Sci. Adv. 3:e1601897. doi: 10.1126/sciadv.1601897
Jones, D. O. B., Yool, A., Wei, C. L., Henson, S. A., Ruhl, H. A., Watson, R. A., et al. (2014). Global reductions in seafloor biomass in response to climate change. Glob. Change Biol. 20, 1861–1872. doi: 10.1111/gcb.12480
Jorgensen, B. B. (2006). “Bacteria and marine biogeochemistry,” in Marine Geochemistry, eds H. D. Schulz and M. Zabel (Berlin: Springer), 169–206. doi: 10.1007/3-540-32144-6_5
Käss, M., Vedenin, A., Hasemann, C., Brandt, A., and Soltwedel, T. (2019). Community structure of macrofauna in the deep Fram Strait: a comparison between two bathymetric gradients in ice-covered and ice-free areas. Deep Sea Res. Part I Oceanogr. Res. Pap. 152:103102. doi: 10.1016/j.dsr.2019.103102
Kêdra, M., Moritz, C., Choy, E. S., David, C., Degen, R., Duerksen, S., et al. (2015). Status and trends in the structure of Arctic benthic food webs. Polar Res. 34:23775. doi: 10.3402/polar.v34.23775
Klages, M., Boetius, A., Christensen, J. P., Deubel, H., Piepenburg, D., Schewe, I., et al. (2004). “The benthos of Arctic seas and its role for the organic carbon cycle at the seafloor,” in The Organic Carbon Cycle in the Arctic Ocean, eds R. W. Stein and R. Macdonald (Berlin: Springer), 139–167. doi: 10.1007/978-3-642-18912-8_6
Koch, B. P., and Dittmar, T. (2006). From mass to structure?: an Aromaticity index for high-resolution mass data of natural organic matter. Rapid Commun. Mass Spectrom. 20, 926–932. doi: 10.1002/rcm.2386
Koch, B. P., and Dittmar, T. (2016). Erratum of: from mass to structure: an Aromaticity index for high-resolution mass data of natural organic matter. Rapid Commun. Mass Spectrom. 30:250. doi: 10.1002/rcm.7433
Kortsch, S., Primicerio, R., Beuchel, F., Renaud, P. E., Rodrigues, J., Lonne, O. J., et al. (2012). Climate-driven regime shifts in Arctic marine benthos. Proc. Natl. Acad. Sci. U.S.A. 109, 14052–14057. doi: 10.1073/pnas.1207509109
Köster, M., Jensen, P., and Meyer-Reil, L.-A. (1991). “Hydrolytic activities of organisms and biogenic structures in deep-sea sediments,” in Microbial Enzymes in Aquatic Environments, ed. R. J. Chróst (New York, NY: Springer), 298–310. doi: 10.1007/978-1-4612-3090-8_19
Krumpen, T. (2017). Sea Ice and Amtospheric Conditions at HAUSGARTEN Between 2000-2016 (Daily Resolution), Link to Model Results. Bremerhaven: PANGAEA. doi: 10.1594/PANGAEA.878244
Kwok, R., and Rothrock, D. A. (2009). Decline in Arctic sea ice thickness from submarine and ICESat records: 1958-2008. Geophys. Res. Lett. 36, 1–5. doi: 10.1029/2009GL039035
Lalande, C., Bauerfeind, E., Nöthig, E.-M., and Beszczynska-Möller, A. (2013). Impact of a warm anomaly on export fluxes of biogenic matter in the eastern Fram Strait. Prog. Oceanogr. 109, 70–77. doi: 10.1016/j.pocean.2012.09.006
Lalande, C., Nöthig, E.-M., Bauerfeind, E., Hardge, K., Beszczynska-Möller, A., and Fahl, K. (2016). Lateral supply and downward export of particulate matter from upper waters to the seafloor in the deep eastern Fram Strait. Deep Sea Res. Part I Oceanogr. Res. Pap. 114, 78–89. doi: 10.1016/j.dsr.2016.04.014
Lalande, C., Nöthig, E.-M., Somavilla, R., Bauerfeind, E., Shevchenko, V., and Okolodkov, Y. (2014). Variability in under-ice export fluxes of biogenic matter in the Arctic Ocean. Glob. Biogeochem. Cycles 28, 571–583. doi: 10.1002/2013GB004735
Leu, E., Søreide, J. E., Hessen, D. O., Falk-Petersen, S., and Berge, J. (2011). Consequences of changing sea-ice cover for primary and secondary producers in the European Arctic shelf seas: timing, quantity, and quality. Prog. Oceanogr. 90, 18–32. doi: 10.1016/j.pocean.2011.02.004
Linkhorst, A., Dittmar, T., and Waska, H. (2017). Molecular fractionation of dissolved organic matter in a shallow subterranean estuary: the role of the iron curtain. Environ. Sci. Technol. 51, 1312–1320. doi: 10.1021/acs.est.6b03608
Loginova, A. N., Dale, A. W., LeMoigne, F. A. C., Thomsen, S., Sommer, S., Wallmann, K., et al. (2020). Sediment release of dissolved organic matter to the oxygen minimum zone off Peru. Biogeosci. Discuss. 2020, 1–30.
Magnusson, A. (2010). gmt: Interface Between GMT Map-Making Software and R. Available online at: https://CRAN.R-project.org/package=gmt
McLafferty, F. W., and Turecek, F. (1994). Interpretation of Mass Spectra, 4th Edn, ed. W. Vetter (Hoboken, NJ: John Wiley & Sons, Ltd). doi: 10.1002/bms.1200230614
Middelburg, J. J. (2018). Reviews and syntheses: to the bottom of carbon processing at the seafloor. Biogeosciences 15, 413–427. doi: 10.5194/bg-15-413-2018
Nöthig, E. M., Bracher, A., Engel, A., Metfies, K., Niehoff, B., Peeken, I., et al. (2015). Summertime plankton ecology in Fram strait-a compilation of long-and short-term observations. Polar Res. 34:23349. doi: 10.3402/polar.v34.23349
Notz, D., and Stroeve, J. (2016). Observed Arctic sea-ice loss directly follows anthropogenic CO2 emission. Science 354, 747–750. doi: 10.1126/science.aag2345
Oni, O. E., Schmidt, F., Miyatake, T., Kasten, S., Witt, M., Hinrichs, K.-U., et al. (2015). Microbial communities and organic matter composition in surface and subsurface sediments of the Helgoland mud area, North Sea. Front. Microbiol. 6:1290. doi: 10.3389/fmicb.2015.01290
Osterholz, H., Niggemann, J., Giebel, H.-A., Simon, M., and Dittmar, T. (2015). Inefficient microbial production of refractory dissolved organic matter in the ocean. Nat. Commun. 6:7422. doi: 10.1038/ncomms8422
Overland, J. E., and Wang, M. (2013). When will the summer Arctic be nearly sea ice free? Geophys. Res. Lett. 40, 2097–2101. doi: 10.1002/grl.50316
Paquette, R. G., Bourke, R. H., Newton, J. F., and Perdue, W. F. (1985). The East Greenland polar front in autumn. J. Geophys. Res. Oceans 90, 4866–4882. doi: 10.1029/JC090iC03p04866
Peng, G., and Meier, W. N. (2018). Temporal and regional variability of Arctic sea-ice coverage from satellite data. Ann. Glaciol. 59, 191–200. doi: 10.1017/aog.2017.32
Pohlabeln, A. M., Gomez-Saez, G. V., Noriega-Ortega, B. E., and Dittmar, T. (2017). Experimental evidence for abiotic sulfurization of marine dissolved organic matter. Front. Mar. Sci. 4:364. doi: 10.3389/fmars.2017.00364
Polyakov, I. V., Pnyushkov, A. V., Alkire, M. B., Ashik, I. M., Baumann, T. M., Carmack, E. C., et al. (2017). Greater role for Atlantic inflows on sea-ice loss in the Eurasian Basin of the Arctic Ocean. Science 356, 285–291. doi: 10.1126/science.aai8204
Pop Ristova, P., Bienhold, C., Wenzhöfer, F., Rossel, P. E., and Boetius, A. (2017). Temporal and spatial variations of bacterial and faunal communities associated with deep-sea wood falls. PLoS One 12:e0169906. doi: 10.1371/journal.pone.0169906
Rabe, B., von Appen, W.-J., Latarius, K., and Wisotzki, A. (2014). Physical Oceanography During POLARSTERN Cruise PS85 (ARK-XXVIII/2). Bremerhaven: PANGAEA. doi: 10.1594/PANGAEA.837425
Ramette, A. (2007). Multivariate analyses in microbial ecology. FEMS Microbiol. Ecol. 62, 142–160. doi: 10.1111/j.1574-6941.2007.00375.x
Randelhoff, A., Reigstad, M., Chierici, M., Sundfjord, A., Ivanov, V., Cape, M., et al. (2018). Seasonality of the physical and biogeochemical hydrography in the inflow to the Arctic Ocean through Fram Strait. Front. Mar. Sci. 5:224. doi: 10.3389/fmars.2018.00224
Riedel, T., and Dittmar, T. (2014). A method detection limit for the analysis of natural organic matter via Fourier Transform Ion Cyclotron Resonance Mass Spectrometry. Anal. Chem. 86, 8376–8382. doi: 10.1021/ac501946m
Rossel, P. E., Bienhold, C., Boetius, A., and Dittmar, T. (2016). Dissolved organic matter in pore water of Arctic Ocean sediments: environmental influence on molecular composition. Organ. Geochem. 97, 41–52. doi: 10.1016/j.orggeochem.2016.04.003
Rossel, P. E., and Dittmar, T. (2019a). Dissolved Organic Carbon and Total Dissolved Organic Nitrogen in Sediment Porewater and Overlying Bottom Water Collected During Maria S Merian Cruise MSM29 and Polarstern Cruise P85 to the Fram Strait. Bremerhaven: PANGAEA. doi: 10.1594/PANGAEA.908702
Rossel, P. E., and Dittmar, T. (2019b). Normalized Peak Intensities from Solid Phase Extracted Dissolved Organic Matter from Porewater and Overlying Bottom Water Collected During Maria S Merian Cruise MSM29 and Polarstern Cruise PS85 to the Fram Strait. Bremerhaven: PANGAEA. doi: 10.1594/PANGAEA.909107
Rossel, P. E., Stubbins, A., Rebling, T., Koschinsky, A., Hawkes, J. A., and Dittmar, T. (2017). Thermally altered marine dissolved organic matter in hydrothermal fluids. Organ. Geochem. 110, 73–86. doi: 10.1016/j.orggeochem.2017.05.003
Rossel, P. E., Vähätalo, A. V., Witt, M., and Dittmar, T. (2013). Molecular composition of dissolved organic matter from a wetland plant (Juncus effusus) after photochemical and microbial decomposition (1.25 yr): common features with deep sea dissolved organic matter. Organ. Geochem. 60, 62–71. doi: 10.1016/j.orggeochem.2013.04.013
RStudio Team (2015). RStudio: Integrated Development Environment for R. Available online at: http://www.rstudio.com/
Schewe, I. (2018). Biochemical Investigation of Multicorer Sediment Profile MSM29_432-2. Bremerhaven: PANGAEA. doi: 10.1594/PANGAEA.885304
Schewe, I. (2019). Biochemical Investigation of Multicorer Sediment Profile PS85/436-1. Bremerhaven: PANGAEA. doi: 10.1594/PANGAEA.903935
Schewe, I., and Soltwedel, T. (2003). Benthic response to ice-edge-induced particle flux in the Arctic Ocean. Polar Biol. 26, 610–620. doi: 10.1007/s00300-003-0526-8
Schmidt, F., Elvert, M., Koch, B. P., Witt, M., and Hinrichs, K.-U. (2009). Molecular characterization of dissolved organic matter in pore water of continental shelf sediments. Geochim. Cosmochim. Acta 73, 3337–3358. doi: 10.1016/j.gca.2009.03.008
Schmidt, F., Koch, B. P., Elvert, M., Schmidt, G., Witt, M., and Hinrichs, K. (2011). Diagenetic transformation of dissolved organic nitrogen compounds under contrasting sedimentary redox conditions in the Black Sea. Environ. Sci. Technol. 45, 5223–5229. doi: 10.1021/es2003414
Schmidt, F., Koch, B. P., Goldhammer, T., Elvert, M., Witt, M., Lin, Y. S., et al. (2017). Unraveling signatures of biogeochemical processes and the depositional setting in the molecular composition of pore water DOM across different marine environments. Geochim. Cosmochim. Acta 207, 57–80. doi: 10.1016/j.gca.2017.03.005
Schmidt, F., Koch, B. P., Witt, M., and Hinrichs, K. (2014). Extending the analytical window for water-soluble organic matter in sediments by aqueous Soxhlet extraction. Geochim. Cosmochim. Acta 141, 83–96. doi: 10.1016/j.gca.2014.06.009
Schulz, H. D. (2000). “Quantification of early diagenesis: dissolved constituents in marine pore water,” in Marine Geochemistry, eds H. D. Schulz and M. Zabel (Berlin: Springer), 85–128. doi: 10.1007/978-3-662-04242-7_3
Seibt, M. (2017). The Molecular Geography of Dissolved Organic Matter in the Atlantic and Southern Ocean. Ph.D. thesis, University of Oldenburg, Oldenburg.
Seidel, M., Beck, M., Greskowiak, J., Riedel, T., Waska, H., Suryaputra, I. N. A., et al. (2015a). Benthic-pelagic coupling of nutrients and dissolved organic matter composition in an intertidal sandy beach. Mar. Chem. 176, 150–163. doi: 10.1016/j.marchem.2015.08.011
Seidel, M., Yager, P. L., Ward, N. D., Carpenter, E. J., Gomes, H. R., Krusche, A. V., et al. (2015b). Molecular-level changes of dissolved organic matter along the Amazon River-to-ocean continuum. Mar. Chem. 177, 218–231. doi: 10.1016/j.marchem.2015.06.019
Seidel, M., Beck, M., Riedel, T., Waska, H., Suryaputra, I. G. N. A., Schnetger, B., et al. (2014). Biogeochemistry of dissolved organic matter in an anoxic intertidal creek bank. Geochim. Cosmochim. Acta 140, 418–434. doi: 10.1016/j.gca.2014.05.038
Shuman, F. R., and Lorenzen, C. J. (1975). Quantitative degradation of chlorophyll by a marine herbivore. Limnol. Oceanogr. 20, 580–586. doi: 10.4319/lo.1975.20.4.0580
Sinninghe Damste, J. S., and de Leeuw, J. W. (1990). Analysis, structure and geochemical significance of organically-bound sulphur in the geosphere: state of the art and future research. Organ. Geochem. 16, 1077–1101. doi: 10.1016/0146-6380(90)90145-P
Smith, K. L., Ruhl, H. A., Bett, B. J., Billett, D. S. M., Lampitt, R. S., and Kaufmann, R. S. (2009). Climate, carbon cycling, and deep-ocean ecosystems. Proc. Natl. Acad. Sci. U.S.A. 106, 19211–19218. doi: 10.1073/pnas.0908322106
Soltwedel, T., Bauerfeind, E., Bergmann, M., Bracher, A., Budaeva, N., Busch, K., et al. (2016). Natural variability or anthropogenically-induced variation? Insights from 15 years of multidisciplinary observations at the arctic marine LTER site HAUSGARTEN. Ecol. Indic. 65, 89–102. doi: 10.1016/j.ecolind.2015.10.001
Soltwedel, T., Hasemann, C., Vedenin, A., Bergmann, M., Taylor, J., and Krauß, F. (2019). Bioturbation rates in the deep Fram strait: results from in situ experiments at the arctic LTER observatory HAUSGARTEN. J. Exp. Mar. Biol. Ecol. 511, 1–9. doi: 10.1016/j.jembe.2018.11.001
Stein, R. (2008). Arctic Ocean Sediments: Processes, Proxies, and Paleoenvironment: Processes, Proxies, and Paleoenvironment. Amsterdam: Elsevier.
Stubbins, A., and Dittmar, T. (2012). Low volume quantification of dissolved organic carbon and dissolved nitrogen. Limnol. Oceanogr. Methods 10, 347–352. doi: 10.4319/lom.2012.10.347
Sun, L., Perlwitz, J., and Hoerling, M. (2016). What caused the recent “Warm Arctic, Cold Continents” trend pattern in winter temperatures? Geophys. Res. Lett. 43, 5345–5352. doi: 10.1002/2016GL069024.Received
Thiel, H. (1982). Zoobenthos of the CINECA area and other upwelling regions. Rapp. Proc. Verb. Réun. Cons. Int. Explor. Mer 180, 323–334.
Wassmann, P., and Reigstad, M. (2011). Future Arctic Ocean seasonal ice zones and implications for pelagic-benthic coupling. Oceanography 24, 220–231. doi: 10.5670/oceanog.2011.74
Wenzhöfer, F., Bauerfeind, E., and Rohardt, G. (2013). Physical Oceanography During Maria S. Merian Cruise MSM29. Bremerhaven: PANGAEA. doi: 10.1594/PANGAEA.819391
Wohlers, J., Engel, A., Zollner, E., Breithaupt, P., Jurgens, K., Hoppe, H.-G., et al. (2009). Changes in biogenic carbon flow in response to sea surface warming. Proc. Natl. Acad. Sci. U.S.A. 106, 7067–7072. doi: 10.1073/pnas.0812743106
Zark, M., Christoffers, J., and Dittmar, T. (2017). Molecular properties of deep-sea dissolved organic matter are predictable by the central limit theorem: evidence from tandem FT-ICR-MS. Mar. Chem. 191, 9–15. doi: 10.1016/j.marchem.2017.02.005
Keywords: dissolved organic matter, porewater, Fourier-transform ion cyclotron mass spectrometry, Arctic Ocean, benthic communities
Citation: Rossel PE, Bienhold C, Hehemann L, Dittmar T and Boetius A (2020) Molecular Composition of Dissolved Organic Matter in Sediment Porewater of the Arctic Deep-Sea Observatory HAUSGARTEN (Fram Strait). Front. Mar. Sci. 7:428. doi: 10.3389/fmars.2020.00428
Received: 19 February 2020; Accepted: 15 May 2020;
Published: 17 June 2020.
Edited by:
Christian Lønborg, Aarhus University, DenmarkReviewed by:
Krista Longnecker, Woods Hole Oceanographic Institution, United StatesCopyright © 2020 Rossel, Bienhold, Hehemann, Dittmar and Boetius. This is an open-access article distributed under the terms of the Creative Commons Attribution License (CC BY). The use, distribution or reproduction in other forums is permitted, provided the original author(s) and the copyright owner(s) are credited and that the original publication in this journal is cited, in accordance with accepted academic practice. No use, distribution or reproduction is permitted which does not comply with these terms.
*Correspondence: Pamela E. Rossel, UGFtZWxhLnJvc3NlbC5jYXJ0ZXNAdW5pLW9sZGVuYnVyZy5kZQ==
Disclaimer: All claims expressed in this article are solely those of the authors and do not necessarily represent those of their affiliated organizations, or those of the publisher, the editors and the reviewers. Any product that may be evaluated in this article or claim that may be made by its manufacturer is not guaranteed or endorsed by the publisher.
Research integrity at Frontiers
Learn more about the work of our research integrity team to safeguard the quality of each article we publish.