- 1Marine Ecology Department, Faculty of Biology and Chemistry, University of Bremen, Bremen, Germany
- 2Red Sea Research Center, King Abdullah University of Science and Technology, Thuwal, Saudi Arabia
- 3Baltic Sea Centre, Stockholm University, Stockholm, Sweden
- 4Faculty of Biological and Environmental Sciences, Tvärminne Zoological Station, University of Helsinki, Helsinki, Finland
- 5Department of Biology, University of Konstanz, Konstanz, Germany
- 6Laboratory for Biological Geochemistry, School of Architecture, Civil and Environmental Engineering, École Polytechnique Fédérale de Lausanne (EPFL), Lausanne, Switzerland
- 7Analytical Chemistry Core Laboratory (CORE Labs), King Abdullah University of Science and Technology, Thuwal, Saudi Arabia
Nitrogen (N) cycling in coral reefs is of key importance for these oligotrophic ecosystems, but knowledge about its pathways is limited. While dinitrogen (N2) fixation is comparably well studied, the counteracting denitrification pathway is under-investigated, mainly because of expensive and relatively complex experimental techniques currently available. Here, we combined two established acetylene-based assays to one single setup to determine N2-fixation and denitrification performed by microbes associated with coral reef substrates/organisms simultaneously. Accumulating target gases (ethylene for N2-fixation, nitrous oxide for denitrification) were measured in gaseous headspace samples via gas chromatography. We measured N2-fixation and denitrification rates of two Red Sea coral reef substrates (filamentous turf algae, coral rubble), and demonstrated, for the first time, the co-occurrence of both N-cycling processes in both substrates. N2-fixation rates were up to eight times higher during the light compared to the dark, whereas denitrification rates during dark incubations were stimulated for turf algae and suppressed for coral rubble compared to light incubations. Our results highlight the importance of both substrates in fixing N, but their role in relieving N is potentially divergent. Absolute N2-fixation rates of the present study correspond with rates reported previously, even though likely underestimated due to an initial lag phase. Denitrification is also presumably underestimated due to incomplete nitrous oxide inhibition and/or substrate limitation. Besides these inherent limitations, we show that a relative comparison of N2-fixation and denitrification activity between functional groups is possible. Thus, our approach facilitates cost-efficient sample processing in studies interested in comparing relative rates of N2-fixation and denitrification.
Introduction
Nitrogen (N) is one of the primary nutrients critical for the survival of all living organisms. Natural N availability is limited in many ecosystems, while others suffer from eutrophication. Thus, understanding N cycling processes is of paramount interest. Two antagonistic biological processes within the N cycle are of particular importance: (a) the import of new N into the ecosystem via microbes (diazotrophs) capable of converting atmospheric dinitrogen (N2) into bioavailable forms of N, which is called biological N2-fixation (hereafter N2-fix), and (b) its counteracting process that removes N from the ecosystem via a reduction of nitrate to N2, commonly described as denitrification (hereafter DENI; Vitousek et al., 1997; Gruber and Sarmiento, 2002; Jickells and Weston, 2011). Both processes appear in terrestrial and aquatic ecosystems, where N can act as an important factor limiting productivity (Lesser et al., 2007).
In coral reefs, N cycling is of particular importance as these ecosystems flourish in the oligotrophic waters of the tropics. However, attempts to describe microbial N cycling in coral reef environments are primarily restricted to N2-fix to provide information about how coral reefs flourish in nutrient-poor waters and how their N demand is satisfied (Neil and Capone, 2008). Appropriate methods to quantify other pathways are largely missing to date (Groffman et al., 2006).
N2-fix is often quantified by one of two commonly applied methods: labeled isotope tracing techniques (Montoya et al., 1996; Grover et al., 2014) or acetylene reduction assays (ARA; Pogoreutz et al., 2017; Tilstra et al., 2017). Isotope based techniques can be highly specific in identifying the exact location of N2-fix, but they are comparatively expensive and require higher effort to set up, perform and analyze samples. Whereas isotope tracer approaches track the fate of fixed N to the cellular compartments of a target organism/substrate (Wilson et al., 2012), acetylene assays measure gross N2-fix activities on a “holobiont-wide” level of an organisms/substrate (Mulholland et al., 2004; Mohr et al., 2010; Grover et al., 2014). This property is of significance, as coral reef organisms/substrates are considered as holobionts, consisting of the host organism/substrate and all of its associated symbiotic microorganisms (Rosenberg et al., 2007). The ARA is frequently used due to its comparatively easy handling and cost-effectiveness and has received first attention in the 1960s (Hardy et al., 1968). It makes use of the fact that acetylene acts as an alternative substrate to N2, resulting in the preferential reduction of acetylene to ethylene (C2H4) instead of N2 to ammonium by the nitrogenase enzyme (Supplementary Figure S1). The C2H4 evolution is then quantified, e.g., via gas chromatography (GC), as an indirect measurement of N2-fix.
DENI can be detected using the same methods as described above (Koop et al., 2001; Groffman et al., 2006; Hoffmann et al., 2009; Myrstener et al., 2016). Acetylene assays are carried out as acetylene inhibits the nitrous oxide (N2O) reductase of denitrifying bacteria, which subsequently results in an accumulation of N2O (Supplementary Figure S1; Fedorova et al., 1973; Balderston et al., 1976; Yoshinari and Knowles, 1976). Accumulated N2O can then be quantified via GC and used as an approximation of DENI activity.
DENI received attention as a mechanism to relieve coral reefs from excessive N only recently, as coral reefs experience and suffer more frequently from anthropogenically N inputs (Halpern et al., 2008; Smith and Schindler, 2009); upcoming studies should, thus, focus on determining the capacities of coral reef environments to cope both with limited and excessive N by quantifying N2-fix and DENI activities simultaneously (Rädecker et al., 2015).
In this context, acetylene assays have the potential for detecting both N2-fix and DENI simultaneously. Indeed, both methods have been applied in terrestrial (Yoshinari et al., 1977) and aquatic (Bertics et al., 2012) systems. Capone and Montoya (2001) already hypothesized a potential simultaneous usage in coral reef environments, but applications on various coral reef organisms and substrates are still missing to date. Comparing multiple functional groups of coral reefs, however, is of key importance, as coral reefs experience regime-shifts that in return alter N cycling patterns that potentially exacerbate or alleviate anthropogenic impacts.
We acknowledge the ongoing scientific debate whether isotope-based approaches or acetylene assays are the methods of choice in order to investigate N cycling pathways. We, therefore, do not compare or discuss both approaches in the present study. Instead, we highlight the application of a combined acetylene-based assay to two common coral reef substrates. We were able to evaluate both process measurements in one single experimental setup and analysis, which we termed COBRA (= combined blockage/reduction acetylene assay). Based on the results of the application, we discuss advantages and limitations as well as its potential use in coral reef science.
Materials and Procedures
A complete list of all materials and the equipment used in this protocol as well as a full description of how the acetylene assay was performed can be found in Supplementary Material.
Collection and Maintenance
Specimens were collected randomly from a semi-exposed area of Abu Shosha reef in the Jeddah region (22°18′15″ N, 39°02′56″E) on the west coast of Saudi Arabia in the central Red Sea in March 2018. Turf algae (n = 5) and coral rubble fragments (n = 4) were selected in order to cover prevailing benthic substrates of the region. Turf algae were defined as dense and flat (<2 cm in height) assemblages of filamentous algae of different species, including small individuals of macroalgae and cyanobacteria. Coral rubble was defined as dislodged parts of framework builders or loose reef rock larger than the sand fraction with its associated microbial community according to Rasser and Riegl (2002). All fragments were ~10 cm long and were collected with hammer and chisel from 5 to 6 m water depth. They were immediately transferred to recirculation aquaria on the boat (each filled with 10 L of ambient seawater) and kept until experiments at ambient water temperature and light conditions.
Dinitrogen Fixation and Denitrification Measurements
Incubations were conducted ex situ and directly after sample collection (<3 h after collection). N2-fix and DENI incubations were conducted using COBRA according to the steps outlined in detail in Supplementary Material S1. Briefly, all COBRA incubations were conducted in gas-tight 1 L glass chambers, each filled with 800 mL of nutrient-enriched seawater (5 μM NO3–; NO3– enrichment consisted of previously prepared NaNO3 stock solution, prepared with MilliQ water and NaNO3, ≥99.0%, Sigma-Aldrich) and 200 mL headspace (Figure 1). Both incubation water and headspace were 10% acetylene enriched, as a 10% saturation has been successfully applied in acetylene-based assays for the quantification of N2-fix (Mulholland et al., 2004; Pogoreutz et al., 2017) and DENI (Yoshinari and Knowles, 1976; Shrewsbury et al., 2016) previously. Nutrient enriched seawater was used, as acetylene inhibits the production of NO3– in the nitrification pathway (Hynes and Knowles, 1978; Oremland and Capone, 1988). Thus, added NO3– served as a substrate for DENI (see section “Limitations” for detailed information). Replicates were arranged in individual incubation chambers, and an additional chamber that was filled exclusively with 5 μM NO3– enriched seawater as “seawater blank” (Figure 1). Solely one seawater blank was chosen because negligible microbial activity (diazotrophs and hypothetically denitrifyers) in seawater communities was expected according to previous studies (Foster et al., 2009; Bednarz et al., 2015; Cardini et al., 2016). All incubation chambers were placed in a tempered water bath and stirred continuously (500 rpm) to ensure stable measurement conditions for 12 h at in situ temperatures (25°C). Dark and light incubations were conducted separately; dark incubations were performed at night in complete darkness. Light incubations were performed with a photon flux of ∼200 μM quanta m–2 s–1. Gaseous samples were taken immediately after starting the incubations (t0), after 2 h (t2), 4 h (t4), 8 h (t8), and 12 h (t12) and analyzed using GC (Agilent 7890A, HP-Plot/Q column, helium pulsed discharge detector) via manual injection. N2-fix and DENI were quantified by changes in gas concentrations according to equations 2–6 outlined in Supplementary Material S1. Besides start and end concentrations, two different intervals were selected as basis for the rate calculations, particularly t4–t12 for N2-fix and t0–t4 for DENI activity. Finally, concentrations were corrected for the seawater blank signal, related to incubation volume and normalized to the surface area of the substrates (Supplementary Tables S2, S3). Surface areas for turf algae and coral rubble fragments were calculated using cloud-based 3D models of samples (Autodesk Remake v19.1.1.2; Lavy et al., 2015; Gutierrez-Heredia et al., 2016).
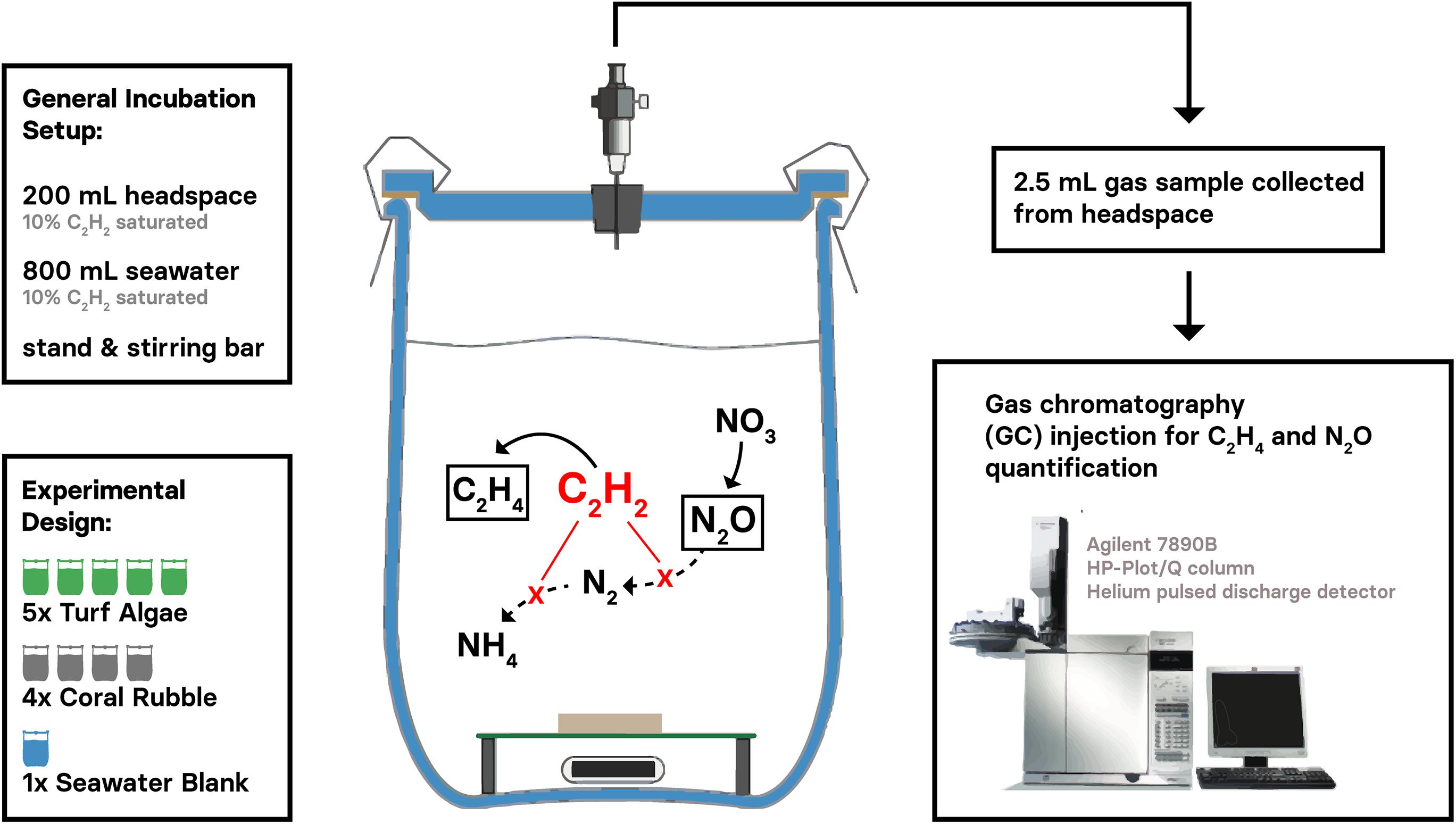
Figure 1. Experimental design and general setup of the CORBA (= Combined Reduction/Blockage Acetylene assay) to simultaneously measure dinitrogen (N2) fixation and denitrification in environmental samples. The two target gases ethylene and nitrous oxide accumulate (framed C2H4 and N2O, resp.) as provided acetylene (C2H2) is (a) preferentially reduced to C2H4 instead of N2 to ammonium (NH4), and (b) inhibiting the evolution of in the N2 gas at the N2O-stage. Gaseous samples can be analyzed using gas chromatography.
Calibration
A concentration series of C2H4 (Abdullah Hashim Industrial Gases & Equipment Co. Ltd. Specialty Gases Center, 19.6 ppm in balanced air; 210 ppm in balanced air) and N2O (Air Liquide, 199.6 ppm in balanced Helium) was prepared by diluting commercial standards to desired standard concentrations. Standards covered the expected ranges of 0–210 ppm for C2H4 and 0–2 ppm for N2O, respectively, and were injected manually into the GC. For the C2H4 calibration curve, standards with known concentrations of 210, 105, 52.5, 21, 20, 10, 5, 2, 1, and 0 ppm were measured in duplicates. The same was done for the N2O calibration curve, where standards with known concentrations of 2, 1, 0.5, 0.2 ppm, and 0 ppm were injected.
Statistical Analysis
The statistical analysis was performed using Sigmaplot 12 (Systat software, v12.0). A two-way analysis of variance (ANOVA) with factors “substrates” and “sampling time” was performed. In case of p < 0.05, a post hoc test (Tukey HSD) was performed to check for significant differences between substrates and sampling times. Significance level was set at α = 0.05.
Results
Limits of detection (LOD), obtained from duplicate measurements of prepared standard samples, were 0.3 ppm for both target gases (Supplementary Figure S2). Standard curves showed high linearity for both target gases (linear regressions; R2 > 0.99; p < 0.0001, F > 800; Supplementary Figure S2).
Concentrations for both C2H4 and N2O in seawater blank incubations over the incubation time of 12 h were stable (Supplementary Figure S3). Production of C2H4 (as a proxy for N2-fix) and N2O (as a proxy for DENI) were measured in both dark and light conditions (Figure 2, Supplementary Tables S2, S3). Statistical analysis identified “time” as a significant factor in both processes and regardless of light availability (Supplementary Table S4). C2H4 evolution was measured in both substrates and was higher in light compared to dark incubations. Overall, C2H4 increased over time, with an initial lag phase in the first 4 h of incubation. Consequently, N2-fix rates calculated discounting the initial lag phase were one third higher than rates resulting from start-end concentrations (Table 1).
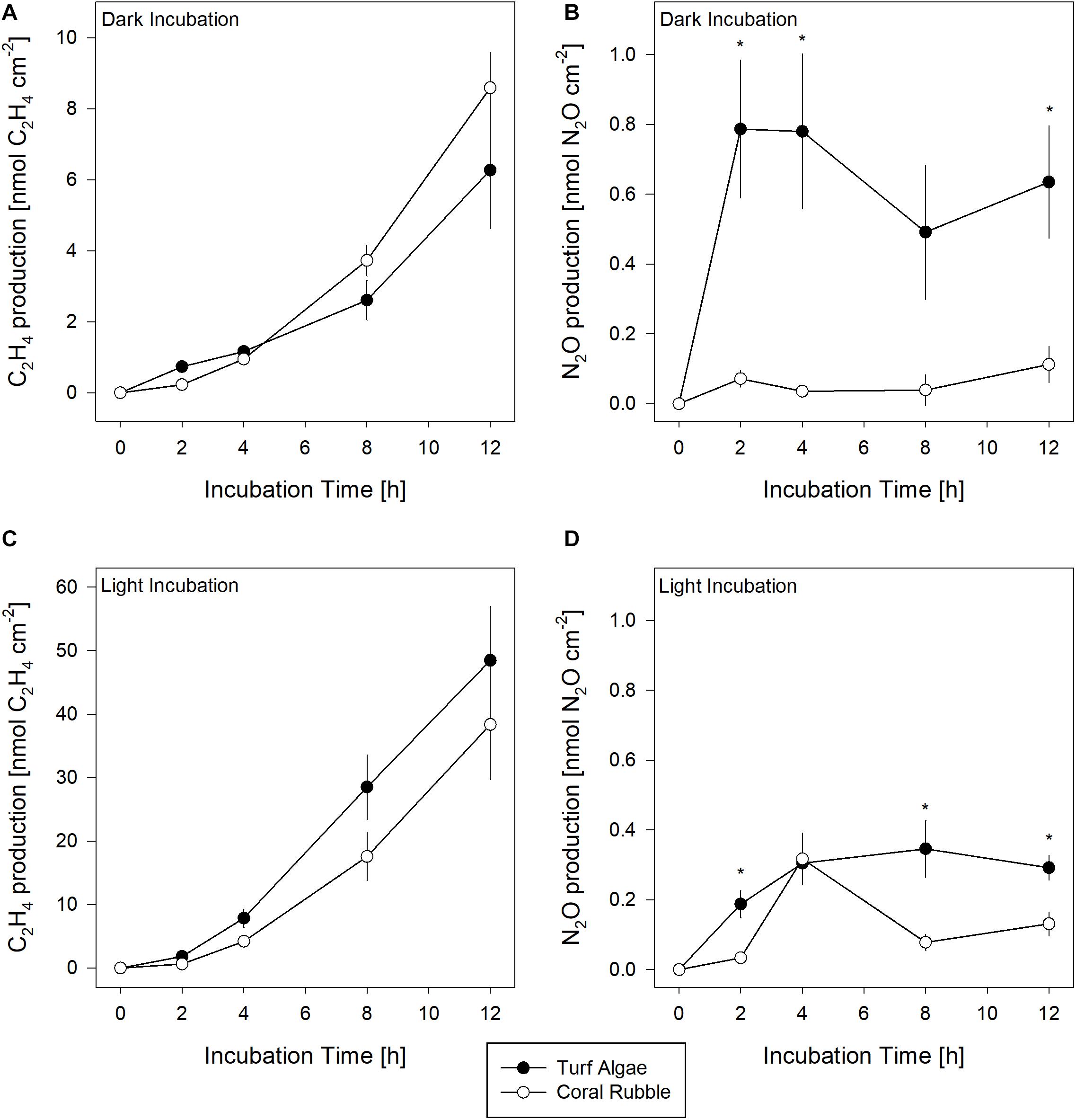
Figure 2. Ethylene (C2H4) production as a proxy for N2-fixation (A,C) and nitrous oxide (N2O) production as a proxy for denitrification (B,D) in turf algae (n = 5) and coral rubble (n = 4) in dark (top graphs) and light (bottom graphs) incubation over time. Asterisks indicate significant differences (p < 0.05) between both substrates. Note: Different scales for C2H4 concentrations.
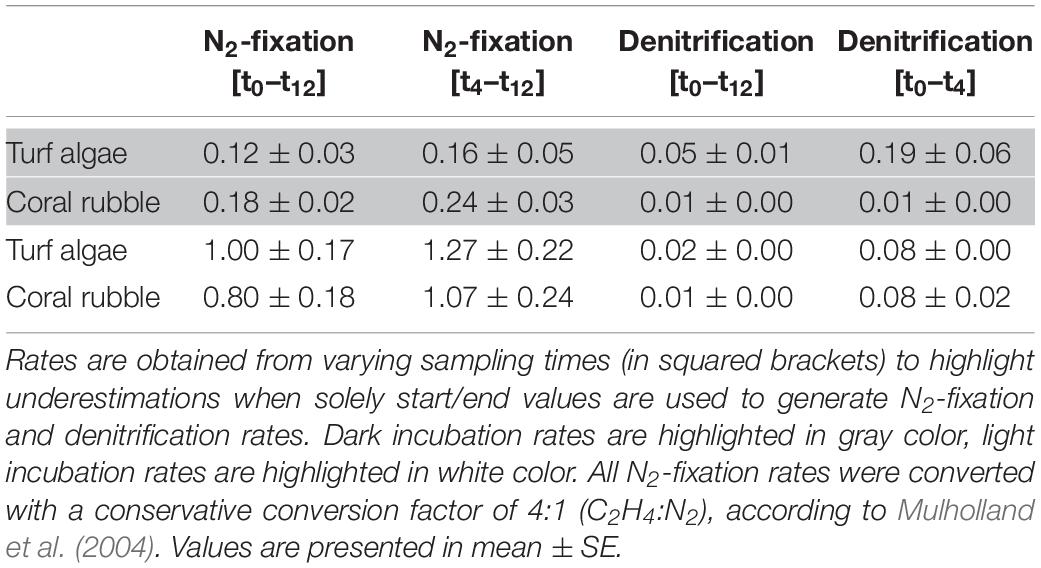
Table 1. N2-fixation (nmol N2 cm–2 h–1) and denitrification (nmol N2O cm–2 h–1) rates/potentials in turf algae and coral rubble.
N2O evolution was detected for turf algae and coral rubble, with turf algae showing significantly higher amounts of N2O after 2, 8, and 12 h incubation time regardless of light availability (Supplementary Table S5). For both substrates, the highest amounts of N2O were measured after 4 h of incubation. Unlike to C2H4 evolution, no initial lag phase was observed. In contrast, N2O only increased in the first 4 h and remained stable or decreased afterward. Turf algae DENI rates were higher in dark than those for coral rubble, but similar when incubated in light (Table 1). DENI rates obtained from the first 4 h of incubation were up to 8 times higher than those resulting from start-end concentrations (Table 1).
Discussion
Dinitrogen Fixation and Denitrification Measurements
In the present study, we were able to demonstrate the co-occurrence of N2-fix and DENI in turf algae and coral rubble for the first time. The simultaneous presence of these antinomic processes has been detected in coral reef associated sediments (Koop et al., 2001) and microbial mats (Joye and Paerl, 1993) before. Potentially, both pathways can be carried out by the same microbes (Bothe et al., 1981) and are indirectly linked by their similar environmental requirements (Tilstra et al., 2019). Absolute N2-fix rates (i.e., calculated based on sampling points t0 and t12) measured in the present study were in the same range for turf algae (Rix et al., 2015) and coral rubble (Cardini et al., 2016), who also obtained N2-fix rates via acetylene-based assays (Table 2). Potentially, the similarity of rates of both substrates was due to a similar associated microbial composition on the substrates in terms of ecological niche and thus N2-fix activity. To the best of our knowledge, this was the first study to quantify rates of DENI in coral rubble and turf algae, and, thus, no comparison to previous reports is possible.
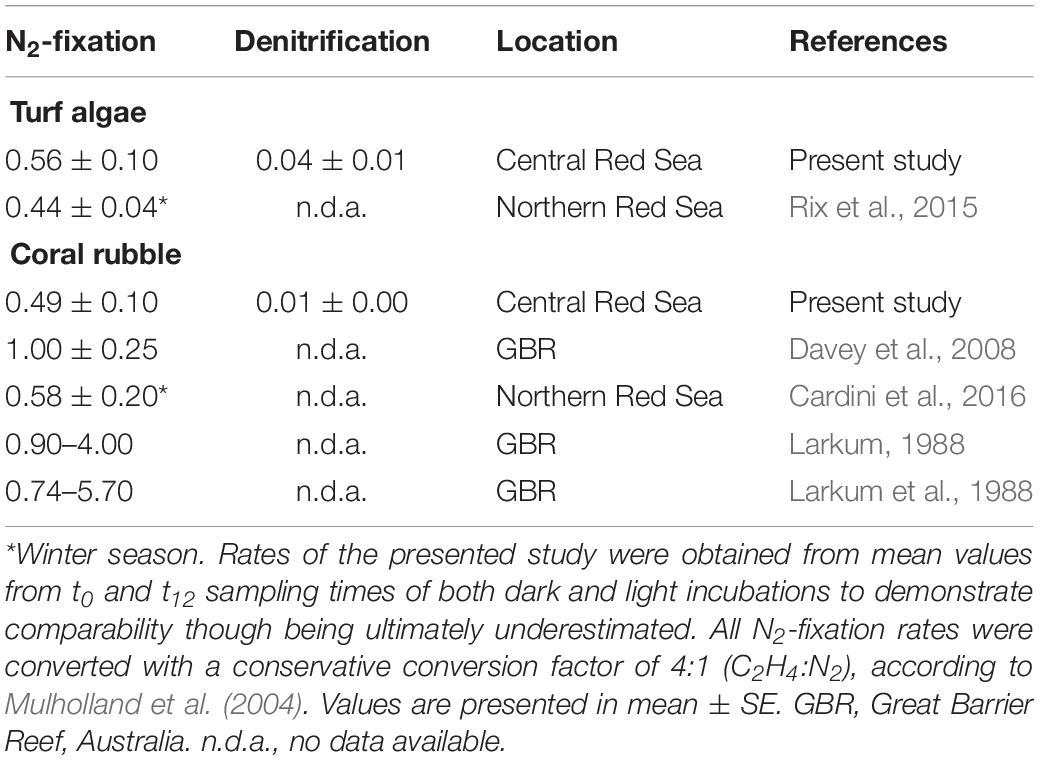
Table 2. N2-fixation (nmol N2 cm–2 h–1) and denitrification (nmol N2O cm–2 h–1) rates of turf algae and coral rubble in comparison with values reported from other coral reef areas worldwide acquired via acetylene assays.
Interestingly, both substrates showed stimulated N2-fix during light compared to dark incubations. DENI activity was similar in both substrates during light incubations, whereas dark incubations showed suppressed DENI activity in coral rubble but stimulated DENI in turf algae (Table 1). Nitrogenase, the key enzyme performing N2-fix, is extremely sensitive to oxygen (Robson and Postgate, 1980), and N2-fix is generally considered as an anaerobic process (Compaoré and Stal, 2010). Likewise, DENI is also an anaerobic process (Zumft, 1997). Both incubations (dark and light) were started with freshly collected seawater containing natural, ambient oxygen concentrations, which likely increased during light and decreased during dark incubations due to photosynthesis or respiration (Mague et al., 1974; Rix et al., 2015; Cardini et al., 2016). The occurrence of both anaerobic processes despite the presence of oxygen (i.e., during light) indicates that both N2-fix and DENI may be spatially separated from oxygen evolution in both substrates, or that the involved diazotrophs and denitrifiers are capable of performing N2-fix and DENI, resp., in the presence of oxygen (Lloyd et al., 1987; Berman-Frank et al., 2001; Silvennoinen et al., 2008; Bednarz et al., 2018). Furthermore, aerobic conditions promote nitrification (Rysgaard et al., 1994), i.e., the oxidation from ammonium to nitrite and NO3–, which serves as a substrate for DENI (Devol, 2008). However, in how far photosynthesis and respiration affected N2-fix and DENI activities in both substrates in the present study remains speculative. Synoptically, the method aids to reveal interesting N cycling patterns. This emphasizes the general applicability of the combined technique for the quantification of N2-fix rates, yet revealing interesting patterns for both N cycling pathways.
Our findings revealed increasing C2H4 concentrations over the total incubation time of 12 h with an initial lag phase in the first 4 h, which is shorter than reported before (Patriquin and McClung, 1978; Williams et al., 1987; Shieh and Lin, 1992). Considering start (t0) and end (t12) concentrations will thus result in an underestimation of N2-fix rates (Table 1). However, N2-fix rates based on start/end concentrations are comparable to previous studies that have applied standard acetylene reduction assays (Table 2), indicating that COBRA still provides sufficient information to go beyond relative comparisons between functional groups but also allowing comparative analysis with other studies. Nevertheless, the most accurate rates are derived by omitting the initial lag phase when calculating rates and considering sampling points t4 and t12 instead.
In the case of N2O rates, we revealed higher N2O rates obtained from the first 4 h incubation time (Table 1). This leads to the suggestion of a short sampling interval to identify DENI potentials (i.e., t0 and t4). The detected maximum after 4 h likely reflects an incomplete blockage of the DENI pathway (Yu et al., 2010). A depletion of accumulated N2O gas concentrations via denitrifying bacteria is likely due to reduced DENI inactivity as NO3– substrate has been consumed (see next section). However, our findings reveal a significant difference in accumulated N2O after 12 h between turf algae and coral rubble fragments, despite a decrease in N2O concentrations after 4 h. As a result, we recommend short sampling intervals but also conclude that even over 12 h incubation time, a relative comparison between different functional groups may be possible as long as methodological limitations (e.g., substrate availability) are considered.
Altogether, we recommend performing incubations for 12 h, with sampling points at t0, t4, and t12. If feasible with regard to costs and workload, we suggest a higher temporal resolution (i.e., more sampling points) to achieve rates that are potentially more precise. By all means, the temporal resolution chosen in the present study enables calculations for most reliable N2-fix (from t4 until t12) rates and DENI (from t0 until t4) potentials. In case that solely start- and end-measurements can be performed (from t0 until t12), we conclude that a) the rates measured with the COBRA still allow comparisons with other studies in case of N2-fix (Table 2); and b) COBRA provides sufficient information about the relative importance by accounting for relative changes in rates across substrates in case of DENI activity, even though both N cycling pathways may be underestimated.
Limitations
Acetylene assays have faced criticism in the last decades. Limitations of acetylene assays have been reviewed extensively in Giller (1987), Groffman et al. (2006), and Wilson et al. (2012). Reported restraints are of general nature and cannot be directly related to a combination of both acetylene reduction and inhibition as in the presented setup here. Thus, we focus on methodological limitations that are important to consider for the interpretation of relative rather than absolute rates (Groffman et al., 2006).
Firstly, Oremland and Capone (1988) observed an unspecific inhibitory effect of acetylene on other N cycling pathways, as acetylene inhibits the formation of NO3– via the nitrification pathway. This can ultimately result in an underestimation of DENI (Hynes and Knowles, 1978; Jenkins and Kemp, 1984; Seitzinger, 1993; Groffman et al., 2006), as there is a close coupling of both nitrification and DENI (i.e., the production of NO3– via nitrification serves as a substrate for DENI). This is particularly the case in oligotrophic systems, where NO3– may be a limiting factor for DENI (Miyajima et al., 2001). Hence, to preclude substrate limitation, incubations with NO addition, as used in this study, can aid maintaining DENI activity in the absence of nitrification (Haines et al., 1981; Joye and Paerl, 1993; Miyajima et al., 2001). However, the results obtained with COBRA and nutrient addition reflect, thus, a DENI potential rather than actual DENI rates, as artificially increased substrate availability drives DENI above natural occurring rates. A potential side effect of added NO3– on N2-fix activity should be addressed in future studies. Theoretically, with the addition of NO3–, an energetically more cost-efficient alternative N source (i.e., through assimilation) is provided, as compared to N2-fix (Falkowski, 1983). The preferential NO3– assimilation potentially results in lower N2-fix rates. Yet, there is both evidence for the inhibition of N2-fix by the availability of NO3– and counterproof that N2-fix occurs with substantial rates in the presence of up to 30 μM NO3– (Knapp, 2012).
A time-dependent incomplete inhibition of N2O through the presence of acetylene has been discussed before (Groffman et al., 2006; Yu et al., 2010). An incomplete inhibition and a subsequent N2O reduction in the presence of acetylene can, thus, result in an incorrect estimation of total DENI. Yu et al. (2010) have reported incomplete inhibitions after ≥ 24 h. Our results indicate that observed patterns of decreasing N2O concentrations after > 4 h incubation time potentially occur due to an incomplete blockage. Possibly, substrate depletion during the incubation time also lead to decreasing N2O concentrations after > 4 h of incubation time, as NO3– a) is potentially used as a substrate for DENI (Devol, 2008) or) may be assimilated immediately by turf algae (den Haan et al., 2016) and potentially also by coral rubble. It remains unclear if saturating the incubation chamber with >10% acetylene will result in a complete inhibition.
Advantages and Use in Research
Generally, acetylene assays are applied as indirect approaches to identify N2-fix as well as DENI. Despite their limitations (see above), they have provided N2-fix and DENI rate quantifications and estimates for many aquatic systems.
We could demonstrate that a combination of both approaches (i.e., acetylene reduction and acetylene blockage assay) can be performed simultaneously. Both assays have been performed separately over a wide range of aquatic substrates and organisms before (Olson et al., 1998; Cardini et al., 2016). As no changes in the basic setup have been carried out here, we hypothesize that the here presented approach (similar to commonly applied acetylene assays) can be used as a versatile method for organisms and substrates of various aquatic environments.
By using COBRA, the number of samples and measuring time is halved, as only one gaseous sample and consequently, only one sample run is required to detect both target gases. In addition, we were able to measure four gaseous headspace samples per hour using either manual or autosampler injection (see Supplementary Material), whereby the autosampler has the capacity to measure 71 samples within < 18 h. Overall, the high sample throughput enables the generation of profound datasets in a minimum of time, with the possibility to receive a high temporal resolution with multiple functional groups of a targeted coral environment. This opens the door to a deeper understanding of temporal and spatial patterns of N2-fix and DENI across various substrates and organisms and their associated microbial community.
Previous acetylene-based investigations mostly used flame ionization detectors for N2-fix quantification and either electron capture or thermal conductivity detectors for DENI detection (Haines et al., 1981; Capone et al., 1992; Joye and Paerl, 1993; Capone and Montoya, 2001). The latter have a high sensitivity for N2O but are also prone to interference from other compounds (Capone and Montoya, 2001; Roberge et al., 2004). In the present study, we used a helium pulsed discharge ionization detector. This detector is up to 500 times more sensitive than the thermal conductivity sensor and up to 50 times more sensitive than the flame ionization detector (Woo et al., 1996; Hunter et al., 1998). Owing to the use of only one single and very sensitive detector (Roberge et al., 2004) only one type of carrier gas was required for one sample run. This leads to a reduction in costs, time, storage, and usage of further gases.
In the future, unprecedented dramatic environmental changes will be a major challenge for life in the earths’ oceans (Harnik et al., 2012). Thus, research will undoubtedly focus on understanding and predicting the effects of climate change and other anthropogenic pressures on marine ecosystems and their organisms (Bijma et al., 2013; Pandolfi et al., 2011; Runge et al., 2016). Special emphasis will be laid on the effects of environmental change on marine microbial communities, which act as the major drivers of elemental transformations of terrestrial and marine biogeochemical cycles (Gruber and Galloway, 2008; Gruber, 2011). N cycling is one of the most important biogeochemical cycles, and, as such, changes in N availability (through eutrophication) will likely evoke physiological and metabolic responses in coral reef organisms/substrates. Their study is paramount for understanding N cycling from a species to ecosystem level with knowledge of a changing environment (Cardini et al., 2014). Eutrophication, as well as ocean warming and acidification, belong to the mainly discussed and assessed threats. These can easily be implemented in the COBRA by adjusting incubation parameters, e.g., temperature, nutrient availability and pH, or a combination of such. Hence, the described N cycling pathways can be investigated in an anthropogenically influenced system that simulates conditions comparable to common anthropogenic stressor scenarios. As investigations on the effects of climate change have become a major scientific interest, the opportunities to include them in the here presented technique underline its potential.
Data Availability Statement
The datasets generated for this study are available on request to the corresponding author.
Author Contributions
YE-K, CW, FR, BJ, and CV conceptualized and designed the research. YE-K and NK performed the research. YE-K, FR, and NR analyzed the data. YE-K, FR, NR, and CW wrote the manuscript. All authors read and approved the final version of this manuscript.
Funding
This work was funded by the German Research Association (DFG Project: Wi 2677/9-1) to CW and KAUST baseline funding to BJ and CV.
Conflict of Interest
The authors declare that the research was conducted in the absence of any commercial or financial relationships that could be construed as a potential conflict of interest.
Acknowledgments
We are grateful to Arjen Tilstra, Rodrigo Villalobos, João Cúrdia, and Denis B. Karcher for support during fieldwork. Many thanks also to Söphiä Tobler, Rainer Willhaus, and Jan Krause for their help in developing the figures. Further, we would like to thank the editor as well as the two reviewers for their constructive comments on the manuscript.
Supplementary Material
The Supplementary Material for this article can be found online at: https://www.frontiersin.org/articles/10.3389/fmars.2020.00411/full#supplementary-material
References
Balderston, W. L., Sherr, B., and Payne, W. J. (1976). Blockage by acetylene of nitrous-oxide reduction in Pseudomonas-perfectomarinus. Appl. Environ. Microbiol. 31, 504–508.
Bednarz, V. N., Cardini, U., Van Hoytema, N., Al-Rshaidat, M. M. D., and Wild, C. (2015). Seasonal variation in dinitrogen fixation and oxygen fluxes associated with two dominant zooxanthellate soft corals from the northern Red Sea. Mar. Ecol. Prog. Ser. 519, 141–152. doi: 10.3354/meps11091
Bednarz, V. N., Naumann, M. S., Cardini, U., Van Hoytema, N., Rix, L., Al-Rshaidat, M. M. D. D., et al. (2018). Contrasting seasonal responses in dinitrogen fixation between shallow and deep-water colonies of the model coral Stylophora pistillata in the northern Red Sea. PLoS One 13:e0199022. doi: 10.1371/journal.pone.0199022
Berman-Frank, I., Lundgren, P., Chen, Y. B., Küpper, H., Kolber, Z., Bergman, B., et al. (2001). Segregation of nitrogen fixation and oxygenic photosynthesis in the marine cyanobacterium Trichodesmium. Science 294, 1534–1537. doi: 10.1126/science.1064082
Bertics, V. J., Sohm, J. A., Magnabosco, C., and Ziebis, W. (2012). Denitrification and nitrogen fixation dynamics in the area surrounding an individual ghost shrimp (Neotrypaea californiensis) burrow system. Appl. Environ. Microbiol. 78, 3864–3872. doi: 10.1128/AEM.00114-12
Bijma, J., Pörtner, H. O., Yesson, C., and Rogers, A. D. (2013). Climate change and the oceans - What does the future hold? Mar. Pollut. Bull. 74, 495–505. doi: 10.1016/j.marpolbul.2013.07.022
Bothe, H., Klein, B., Stephan, M. P., and Döbereiner, J. (1981). Transformations of inorganic nitrogen by Azospirillum spp. Arch. Microbiol. 130, 96–100. doi: 10.1007/BF00411058
Capone, D. G., Dunham, S. E., Horrigan, S. G., and Duguay, L. E. (1992). Microbial nitrogen transformations in unconsolidated coral reef sediments. Mar. Ecol. Prog. Ser. 80, 75–88. doi: 10.3354/meps080075
Capone, D. G., and Montoya, J. P. (2001). Nitrogen fixation and denitrification. Methods Microbiol. 30, 501–515. doi: 10.1016/S0580-9517(01)30060-3006
Cardini, U., Bednarz, V. N., Foster, R. A., and Wild, C. (2014). Benthic N2 fixation in coral reefs and the potential effects of human-induced environmental change. Ecol. Evol. 4, 1706–1727. doi: 10.1002/ece3.1050
Cardini, U., Bednarz, V. N., van Hoytema, N., Rovere, A., Naumann, M. S., Al-Rshaidat, M. M. D., et al. (2016). Budget of primary production and dinitrogen fixation in a highly seasonal red sea coral reef. Ecosystems 19, 771–785. doi: 10.1007/s10021-016-9966-1
Compaoré, J., and Stal, L. J. (2010). Effect of temperature on the sensitivity of nitrogenase to oxygen in two heterocystous cyanobacteria. J. Phycol. 46, 1172–1179. doi: 10.1111/j.1529-8817.2010.00899.x
Davey, M., Holmes, G., and Johnstone, R. (2008). High rates of nitrogen fixation (acetylene reduction) on coral skeletons following bleaching mortality. Coral Reefs 27, 227–236. doi: 10.1007/s00338-007-0316-9
den Haan, J., Huisman, J., Brocke, H. J., Goehlich, H., Latijnhouwers, K. R. W., Van Heeringen, S., et al. (2016). Nitrogen and phosphorus uptake rates of different species from a coral reef community after a nutrient pulse. Sci. Rep. 6:28821. doi: 10.1038/srep28821
Devol, A. H. (2008). “Denitrification including Anammox,” in Nitrogen in the Marine Environment, 2nd Edn, eds D. G. Capone, D. A. Bronck, M. R. Mulholland, and E. J. Carpenter (Amsterdam: Elsevier), 263–302. doi: 10.1016/B978-0-12-372522-6.00006-2
Falkowski, P. G. (1983). “Enzymology of nitrogen assimilation,” in Nitrogen in the Marine Environment, 1st Edn, eds E. J. Carpenter, and D. G. Capone (Cambridge, MA: Academic Press, Inc), doi: 10.1016/b978-0-12-160280-2.50031-6
Fedorova, R. I., Milekhina, E. I., and Il’Yukhina, N. I. (1973). Evaluation of the method of “gas metabolism” for detecting extraterrestrial life. Identification of nitrogen-fixation microorganisms. Izv. Akad. Hauk SSSR Ser. Biol. 6, 797–806.
Foster, R. A., Paytan, A., and Zehr, J. P. (2009). Seasonality of N2 fixation and nifH gene diversity in the Gulf of Aqaba (Red Sea). Limnol. Oceanogr. 54, 219–233. doi: 10.4319/lo.2009.54.1.0219
Giller, K. E. (1987). Use and abuse of the acetylene reduction assay for measurement of “associative” nitrogen fixation. Soil Biol. Biochem. 19, 783–784. doi: 10.1016/0038-0717(87)90066-6
Groffman, P. M., Altabet, M. A., Böhlke, J. K., Butterbach-Bahl, K., David, M. B., Firestone, M. K., et al. (2006). Methods for measuring denitrification: diverse approaches to a difficult problem. Ecol. Appl. 16, 2091–2122.
Grover, R., Ferrier-Pages, C., Maguer, J.-F., Ezzat, L., and Fine, M. (2014). Nitrogen fixation in the mucus of Red Sea corals. J. Exp. Biol. 217, 3962–3963. doi: 10.1242/jeb.111591
Gruber, N. (2011). Warming up, turning sour, losing breath: ocean biogeochemistry under global change. Philos. Trans. R. Soc. A Math. Phys. Eng. Sci. 369, 1980–1996. doi: 10.1098/rsta.2011.0003
Gruber, N., and Galloway, J. N. (2008). An earth-system perspective of the global nitrogen cycle. Nature 451, 293–296. doi: 10.1038/nature06592
Gruber, N., and Sarmiento, J. L. (2002). “Large-scale biogeochemical-physical interactions in elemental cycles,” The Sea, eds A. R. Robinson, J. J. McCarthy, and B. J. Rothschild (New York, NY: John Wiley), 337–399.
Gutierrez-Heredia, L., Benzoni, F., Murphy, E., and Reynaud, E. G. (2016). End to end digitisation and analysis of three-dimensional coral models, from communities to corallites. PLoS One 11:e0149641. doi: 10.1371/journal.pone.0149641
Haines, J. R., Atlas, R. M., Griffiths, R. P., and Morita, R. Y. (1981). Denitrification and nitrogen fixation in alaskan continental shelf sediments. Appl. Environ. Microbiol. 41, 412–421.
Halpern, B. S., Walbridge, S., Selkoe, K. A., Kappel, C. V., Micheli, F., D’Agrosa, C., et al. (2008). A global map of human impact on marine ecosystems. Science 319, 948–952. doi: 10.1126/science.1149345
Hardy, R. W. F., Holsten, R. D., Jackson, E. K., and Burns, R. C. (1968). The acetylene - ethylene assay for N2 fixation: laboratory and field evaluation. Plant Physiol. 43, 1185–1207. doi: 10.1104/pp.43.8.1185
Harnik, P. G., Lotze, H. K., Anderson, S. C., Finkel, Z. V., Finnegan, S., Lindberg, D. R., et al. (2012). Extinctions in ancient and modern seas. Trends Ecol. Evol. 27, 608–617. doi: 10.1016/j.tree.2012.07.010
Hoffmann, F., Radax, R., Woebken, D., Holtappels, M., Lavik, G., Rapp, H. T., et al. (2009). Complex nitrogen cycling in the sponge Geodia barretti. Environ. Microbiol. 11, 2228–2243. doi: 10.1111/j.1462-2920.2009.01944.x
Hunter, M. C., Bartle, K. D., Lewis, A. C., McQuaid, J. B., Myers, P., Seakins, P. W., et al. (1998). The use of the helium ionization detector for gas chromatographic monitoring of trace atmospheric components. HRC J. High Resolut. Chromatogr. 21, 75–80.
Hynes, R. K., and Knowles, R. (1978). Inhibition by acetylene of ammonia oxidation in Nitrosomonas europaea. FEMS Microbiol. Lett. 4, 319–321. doi: 10.1111/j.1574-6968.1978.tb02889.x
Jenkins, M. C., and Kemp, W. M. (1984). The coupling of nitrification and denitrification in two estuarine sediments. Limnol. Oceanogr. 29, 609–619. doi: 10.4319/lo.1984.29.3.0609
Jickells, T. D., and Weston, K. (2011). “Nitrogen cycle - external cycling: losses and gains,” in Treatise on Estuarine and Coastal Science, eds E. Wolanski, and D. S. McLusky (Waltham, MA: Academic Press), 261–278. doi: 10.1016/B978-0-12-374711-2.00509-X
Joye, S. B., and Paerl, H. W. (1993). Contemporaneous nitrogen fixation and denitrification in intertidal microbial mats: rapid response to runoff events. Mar. Ecol. Prog. Ser. 94, 267–274. doi: 10.3354/meps094267
Knapp, A. N. (2012). The sensitivity of marine N2 fixation to dissolved inorganic nitrogen. Front. Microbiol. 3:374. doi: 10.3389/fmicb.2012.00374
Koop, K., Booth, D., Broadbent, A., Brodie, J., Bucher, D., Capone, D., et al. (2001). ENCORE: The effect of nutrient enrichment on coral reefs. Synthesis of results and conclusions. Mar. Pollut. Bull. 42, 91–120. doi: 10.1016/S0025-326X(00)00181-8
Larkum, A. W. D. (1988). High rates of nitrogen fixation on coral skeletons after predation by the crown of thorns starfish Acanthaster planci. Mar. Biol. 97, 503–506. doi: 10.1007/BF00391046
Larkum, A. W. D., Kennedy, I. R., and Muller, W. J. (1988). Nitrogen fixation on a coral reef. Mar. Biol. 98, 143–155. doi: 10.1007/BF00392669
Lavy, A., Eyal, G., Neal, B., Keren, R., Loya, Y., and Ilan, M. (2015). A quick, easy and non-intrusive method for underwater volume and surface area evaluation of benthic organisms by 3D computer modelling. Methods Ecol. Evol. 6, 521–531. doi: 10.1111/2041-210x.12331
Lesser, M. P., Falcón, L. I., Rodríguez-Román, A., Enríquez, S., Hoegh-Guldberg, O., and Iglesias-Prieto, R. (2007). Nitrogen fixation by symbiotic cyanobacteria provides a source of nitrogen for the scleractinian coral Montastraea cavernosa. Mar. Ecol. Prog. Ser. 346, 143–152. doi: 10.3354/meps07008
Lloyd, D., Boddy, L., and Davies, K. J. P. (1987). Persistence of bacterial denitrification capacity under aerobic conditions: the rule rather than the exception. FEMS Microbiol. Lett. 45, 185–190. doi: 10.1016/0378-1097(87)90015-2
Mague, T. H., Weare, N. M., and Holm-Hansen, O. (1974). Nitrogen fixation in the North Pacific Ocean. Mar. Biol. 24, 109–119. doi: 10.1007/BF00389344
Miyajima, T., Suzumura, M., Umezawa, Y., and Koike, I. (2001). Microbiological nitrogen transformation in carbonate sediments of a coral-reef lagoon and associated seagrass beds. Mar. Ecol. Prog. Ser. 217, 273–286. doi: 10.3354/meps217273
Mohr, W., Großkopf, T., Wallace, D. W. R., and LaRoche, J. (2010). Methodological underestimation of oceanic nitrogen fixation rates. PLoS One 5:e12583. doi: 10.1371/journal.pone.0012583
Montoya, J. P., Voss, M., Kahler, P., and Capone, D. G. (1996). A simple, high-precision, high-sensitivity tracer assay for N2 fixation. Appl. Environ. Microbiol. 62, 986–993.
Mulholland, M. R., Bronk, D. A., and Capone, D. G. (2004). Dinitrogen fixation and release of ammonium and dissolved organic nitrogen by Trichodesmium IMS101. Aquat. Microb. Ecol. 37, 85–94. doi: 10.3354/ame037085
Myrstener, M., Jonsson, A., and Bergström, A. K. (2016). The effects of temperature and resource availability on denitrification and relative N2O production in boreal lake sediments. J. Environ. Sci. 47, 82–90. doi: 10.1016/j.jes.2016.03.003
Neil, J. M. O., and Capone, D. G. (2008). “Nitrogen cycling in coral reef environments,” Nitrogen in the Marine Environment, eds D. G. Capone, D. A. Bronk, M. R. Mullholland, and E. J. Carpenter (Burlington, VT: Academic Press). doi: 10.1016/B978-0-12-372522-6.00021-9
Olson, J. B., Steppe, T. F., Litaker, R. W., and Paerl, H. W. (1998). N2-fixing microbial consortia associated with the ice cover of Lake Bonney, Antarctica. Microb. Ecol. 36, 231–238. doi: 10.1007/s002489900110
Oremland, R. S., and Capone, D. G. (1988). “Use of ‘Specific’ inhibitors in biogeochemistry and microbial ecology,” in Advances in Microbial Ecology, ed. K. C. Marshall (Boston, MA: Springer), 285–383. doi: 10.1007/978-1-4684-5409-3_8
Pandolfi, J. M., Connolly, S. R., Marshall, D. J., and Cohen, A. L. (2011). Projecting coral reef futures under global warming and ocean acidification. Science 333, 418–422. doi: 10.1126/science.1204794
Patriquin, D. G., and McClung, C. R. (1978). Nitrogen Accretion, and the Nature and Possible Significance of N2 Fixation (Acetylene Reduction) in a Nova Scotian Spartina alterniflora Stand. Mar. Biol. 47, 227–242. doi: 10.1007/BF00541001
Pogoreutz, C., Rädecker, N., Cárdenas, A., Gärdes, A., Wild, C., and Voolstra, C. R. (2017). Nitrogen fixation aligns with nifH abundance and expression in two coral trophic functional groups. Front. Microbiol. 8:1187. doi: 10.3389/fmicb.2017.01187
Rädecker, N., Pogoreutz, C., Voolstra, C. R., Wiedenmann, J., and Wild, C. (2015). Nitrogen cycling in corals: the key to understanding holobiont functioning? Trends Microbiol. 23, 490–497. doi: 10.1016/j.tim.2015.03.008
Rasser, M. W., and Riegl, B. (2002). Holocene coral reef rubble and its binding agents. Coral Reefs 21, 57–72. doi: 10.1007/s00338-001-0206-5
Rix, L., Bednarz, V. N., Cardini, U., Van Hoytema, N., Al-Horani, F. A., Wild, C., et al. (2015). Seasonality in dinitrogen fixation and primary productivity by coral reef framework substrates from the northern Red Sea. Mar. Ecol. Prog. Ser. 533, 79–92. doi: 10.3354/meps11383
Roberge, M. T., Finley, J. W., Lukaski, H. C., and Borgerding, A. J. (2004). Evaluation of the pulsed discharge helium ionization detector for the analysis of hydrogen and methane in breath. J. Chromatogr. A 1027, 19–23. doi: 10.1016/j.chroma.2003.08.083
Robson, R. L., and Postgate, J. R. (1980). Oxygen and hydrogen in biological nitrogen fixation. Annu. Rev. Microbiol. 34, 183–207. doi: 10.1146/annurev.mi.34.100180.001151
Rosenberg, E., Koren, O., Reshef, L., Efrony, R., and Zilber-Rosenberg, I. (2007). The role of microorganisms in coral health, disease and evolution. Nat. Rev. Microbiol. 5, 355–362. doi: 10.1038/nrmicro1635
Runge, J. A., Fields, D. M., Thompson, C. R. S., Shema, S. D., Bjelland, R. M., Durif, C. M. F., et al. (2016). End of the century CO2 concentrations do not have a negative effect on vital rates of Calanus finmarchicus, an ecologically critical planktonic species in North Atlantic ecosystems. ICES J. Mar. Sci. 73, 937–950. doi: 10.1093/icesjms/fsv258
Rysgaard, S., Risgaard-Petersen, N., Sloth, N. P., Jensen, K., Peter, L., and Peter, N. L. (1994). Oxygen regulation of nitrification and denitrification in sediments. Limnol. Oceanogr. 39, 1643–1652. doi: 10.4319/lo.1994.39.7.1643
Seitzinger, S. (1993). “Denitrification and nitrification rates in aquatic sediments,” in Handbook of Methods in Aquatic Microbiology Ecology, eds P. F. Kemp, B. F. Sherr, E. B. Sherr, and J. J. Cole (Boca Raton, FL: Lewis Publishers), 633–641.
Shieh, W. Y., and Lin, Y. M. (1992). Nitrogen fixation (acetylene reduction) associated with the zoanthid Palythoa tuberculosa Esper. J. Exp. Mar. Bio. Ecol. 163, 31–41. doi: 10.1016/0022-0981(92)90145-Z
Shrewsbury, L. H., Smith, J. L., Huggins, D. R., Carpenter-Boggs, L., and Reardon, C. L. (2016). Denitrifier abundance has a greater influence on denitrification rates at larger landscape scales but is a lesser driver than environmental variables. Soil Biol. Biochem. 103, 221–231. doi: 10.1016/j.soilbio.2016.08.016
Silvennoinen, H., Liikanen, A., Torssonen, J., Stange, C. F., and Martikainen, P. J. (2008). Denitrification and N2O effluxes in the Bothnian Bay (northern Baltic Sea) river sediments as affected by temperature under different oxygen concentrations. Biogeochemistry 88, 63–72. doi: 10.1007/s10533-008-9194-7
Smith, V. H., and Schindler, D. W. (2009). Eutrophication science: where do we go from here? Trends Ecol. Evol. 24, 201–207. doi: 10.1016/j.tree.2008.11.009
Tilstra, A., Bednarz, V. N., Cardini, U., Van Hoytema, N., Al-Rshaidat, M. M. D., and Wild, C. (2017). Seasonality affects dinitrogen fixation associated with two common macroalgae from a coral reef in the northern Red Sea. Mar. Ecol. Prog. Ser. 575, 69–80. doi: 10.3354/meps12206
Tilstra, A., El-Khaled, Y. C., Roth, F., Rädecker, N., Pogoreutz, C., Voolstra, C. R., et al. (2019). Denitrification aligns with N2 fixation in red sea corals. Sci. Rep. 9:19460. doi: 10.1038/s41598-019-55408-z
Vitousek, P. M., Aber, J. D., Howarth, R. W., Likens, G. E., Matson, P. A., Schindler, D. W., et al. (1997). Human alteration of the global nitrogen cycle: sources and consequences. Ecol. Appl. 7, 737–750.
Williams, W. M., Viner, A. B., and Broughton, W. J. (1987). Nitrogen fixation (acetylene reduction) associated with the living coral Acropora variabilis. Mar. Biol. 94, 531–535. doi: 10.1007/BF00431399
Wilson, S. T., Böttjer, D., Church, M. J., and Karl, D. M. (2012). Comparative assessment of nitrogen fixation methodologies, conducted in the oligotrophic north pacific ocean. Appl. Environ. Microbiol. 78, 6516–6523. doi: 10.1128/AEM.01146-12
Woo, J.-C., Moon, D.-M., and Kawaguchi, H. (1996). Design and characterization of a helium-discharge ionization detector for gas chromatography. Anal. Sci. 12, 195–200. doi: 10.2116/analsci.12.195
Yoshinari, T., Hynes, R., and Knowles, R. (1977). Acetylene inhibition of nitrous oxide reduction and measurement of denitrification and nitrogen fixation in soil. Soil Biol. Biochem. 9, 177–183. doi: 10.1016/0038-0717(77)90072-4
Yoshinari, T., and Knowles, R. (1976). Acetylene inhibition of nitrous oxide reduction by denitrifying bacteria. Biochem. Biophys. Res. Commun. 3, 705–710. doi: 10.1016/0006-291X(76)90932-3
Yu, K., Seo, D. C., and Delaune, R. D. (2010). Incomplete acetylene inhibition of nitrous oxide reduction in potential denitrification assay as revealed by using 15N-Nitrate tracer. Commun. Soil Sci. Plant Anal. 41, 2201–2210. doi: 10.1080/00103624.2010.504800
Keywords: nitrogen cycling, metabolism, gas chromatography, ethylene, nitrous oxide
Citation: El-Khaled YC, Roth F, Rädecker N, Kharbatia N, Jones BH, Voolstra CR and Wild C (2020) Simultaneous Measurements of Dinitrogen Fixation and Denitrification Associated With Coral Reef Substrates: Advantages and Limitations of a Combined Acetylene Assay. Front. Mar. Sci. 7:411. doi: 10.3389/fmars.2020.00411
Received: 18 December 2019; Accepted: 12 May 2020;
Published: 11 June 2020.
Edited by:
Linda Wegley Kelly, San Diego State University, United StatesReviewed by:
Samuel T. Wilson, University of Hawai‘i at Moa, United StatesRenaud Grover, Centre Scientifique de Monaco, Monaco
Copyright © 2020 El-Khaled, Roth, Rädecker, Kharbatia, Jones, Voolstra and Wild. This is an open-access article distributed under the terms of the Creative Commons Attribution License (CC BY). The use, distribution or reproduction in other forums is permitted, provided the original author(s) and the copyright owner(s) are credited and that the original publication in this journal is cited, in accordance with accepted academic practice. No use, distribution or reproduction is permitted which does not comply with these terms.
*Correspondence: Yusuf C. El-Khaled, eWVrMjAxMkB1bmktYnJlbWVuLmRl