- Institute of Oceanography, University of Gdańsk, Gdynia, Poland
Photoacclimation to constant and changing light conditions was studied in the benthic diatom Nitzschia cf. aurariae isolated from the littoral zone of the Baltic Sea. The diatom was grown under a wide range of irradiances, i.e., 15–350 μmol photons m–2 s–1 with the photoperiod of 16 h of light and 8 h of darkness. In the first experiment, three levels of a constant light were applied, i.e., 30, 115, and 350 μmol photons m–2 s–1. In the second experiment, the diatom was exposed to two ranges of changing light conditions, i.e., the lower-range of variable light, i.e., 15–30–150 μmol photons m–2 s–1, and the higher-range of variable light, i.e., 30–60–350 μmol photons m–2 s–1. The cellular content of photosynthetic (chlorophyll a and fucoxanthin), as well as photoprotective pigments (diadinoxanthin and diatoxanthin), was determined by the total daily light doses. The de-epoxidation state of the xanthophyll cycle reached higher values in cultures maintained under variable light regimes. The analysis of photosynthesis-irradiance curves suggested that N. cf. aurariae acclimated primarily through the changes in the number of photosynthetic units (PSU). Higher photosynthetic rates observed under variable irradiance indicated the maximization of photosynthesis at lower light intensities. In constant high light, the diatom accumulated more photoprotective pigments, however, the activity of the xanthophyll cycle was limited. Under variable light regimes wide changes in the de-epoxidation state allowed for efficient photoprotection, depending on the light intensities applied. Photoprotection appeared to represent an interplay between long-term photoacclimation and rapid adjustment to ambient light conditions within the constraints set by the former. Prolonged exposure to high light caused a decrease in photosynthetic rates. However, the stable growth of the diatom across the applied light intensities showed that it can survive periods of potentially stressful light conditions. Acclimation mechanisms observed in the studied diatom were consistent with those observed in microalgae present in habitats characterized by high irradiance and rapid changes in light conditions.
Introduction
Microphytobenthos is a collection of photosynthetic organisms inhabiting various substrata in shallow aquatic environments, with diatoms being the most prominent algal group (Round, 1981). It is a key player in marine ecosystems as it may be responsible for a considerable proportion of the primary production (Blanchard and Cariou-Le Gall, 1994). Microphytobenthos facilitates the biogeochemical cycling of major nutrients and mediates their fluxes at the water-sediment surface (Sundbäck et al., 2006). It also stabilizes sediments, and due to resuspension is an important food source not only to benthic but also pelagic organisms (Sutherland et al., 1998; Lucas et al., 2001; Saint-Béat et al., 2014).
Microphytobenthos is subjected to a wide and often, rapid variations in ecological variables with light being a crucial factor. Light drives and dictates its photosynthetic processes and thus governs its species composition, biomass distribution, and productivity (e.g., MacIntyre et al., 1996; Underwood, 2005). The underwater light field strongly differs from that of terrestrial habitats, as the light intensity can be a few orders of magnitude lower, and its spectral composition can be altered by the water quality (Depauw et al., 2012). In marine environments, light depends on incident solar radiation, seasons, the time of day and atmospheric conditions, such as cloud cover, mist, and pollution, etc. Light passing through water is attenuated due to the absorptive and scattering water properties caused by water itself, the presence of dissolved organic matter and suspended particles (Dring, 1998). The movement of water due to tides and wind-induced waves, also influences light conditions. Tides reduce light intensity through sediment resuspension, while waves cause rapid focusing and defocusing of light (caustic light), resulting in flashes of extremely high light. Sediment granulometry also influences light conditions; in muddy sediments, light is fully attenuated at a depth of a fraction of a millimeter, and in sandy sediments it can penetrate deeper, up to several millimeters (Kühl et al., 1994; Cartaxana et al., 2011).
Being exposed to such extreme irradiance changes, microphytobenthos require complex adaptive mechanisms maximizing the rate of photosynthesis and CO2 fixation under limiting light conditions, whilest minimizing the damage induced by high light intensities. The balance between these processes is achieved through numerous photoacclimatory and photoprotective mechanisms, spanning various time scales (e.g., Depauw et al., 2012). Short-term regulation of photosynthesis is a reversible process, which enables immediate tracking of changes in the light intensity (Raven and Geider, 2003). For example, the activity of Rubisco accounts for the variations in maximum photosynthetic rates (MacIntyre et al., 2000). Whereas the xanthophyll cycle, which is based on covalent changes in the chromatophores, alters the capacity to quench the excitation energy by down-regulating PSII activity, and thus limits possible photo-damage (Goss et al., 2006). Long-term acclimation, on the other hand, requires altering the gene expression, manifested in the changes of the photosynthetic apparatus (Eberhard et al., 2008). Falkowski and Owens (1980) divided long-term acclimation responses into two categories, namely: a photoacclimation strategy, involving a change in the number of photosynthetic units (PSU) and a strategy, in which light acclimation is accomplished by altering the size of PSU.
The shallow and essentially non-tidal coastal areas of the Baltic Sea provide favorable conditions for the development of microphytobenthos (usually below <1 m depth). Sandy sediments promote the growth of epipsammic species, which tend to be small, with no or strongly limited ability to move (unlike epipelic species, which conversely, are large and motile). As a result epipsammon photo-regulates relying on its physiology (e.g., Cartaxana et al., 2011). In the Baltic Sea, diatoms may constitute up to 60% of the epipsammon biomass, therefore, to understand their ecological dominance, this study investigated the photosynthetic activity of the benthic diatom Nitzschia cf. aurariae Cholnoky 1966, a typical representative of the Baltic epipsammic communities (Pniewski et al., 2015). The main goal was to assess the effect of variable light conditions on the N. cf. aurariae long-term photoacclimation and photoprotection. In order to do so the diatom was grown under different light regimes, i.e., in constant and in changing light, the photo-regulation mechanisms and the activity of the xanthophyll cycle were examined through the analyses of changes in (1) pigment composition, (2) the shape of photosynthesis vs. light (P-E) curves and (3) variable fluorescence (the maximum quantum yield of photosystem II and the non-photochemical quenching).
Materials and Methods
Diatom Culture and Experimental Conditions
The study was carried out on the benthic diatom N. cf. aurariae isolated from the sediment samples collected in Puck Bay located in the southern part of the Baltic Sea, in the proximity of Władysławowo (54°43′N, 18°34′E). The strain (BA 156) is maintained as a unialgal culture in the Culture Collection of the Baltic Algae (CCBA) at the Gdańsk University, and it is grown in the f/2 medium (Guillard, 1975) prepared from Baltic seawater (with salinity of ca. 6.7), at 50 μmol photons m–2 s–1 and 17°C (Figure 1). N. cf. aurariae belongs to rather small-size diatoms [10.8-(11.6)-12.6 μm long, and 2.9-(3.4)-4 μm broad, n = 30, in brackets the average value is provided]. It is a widespread cosmopolitan species, commonly occurring in the Baltic Sea (Witkowski et al., 2000).
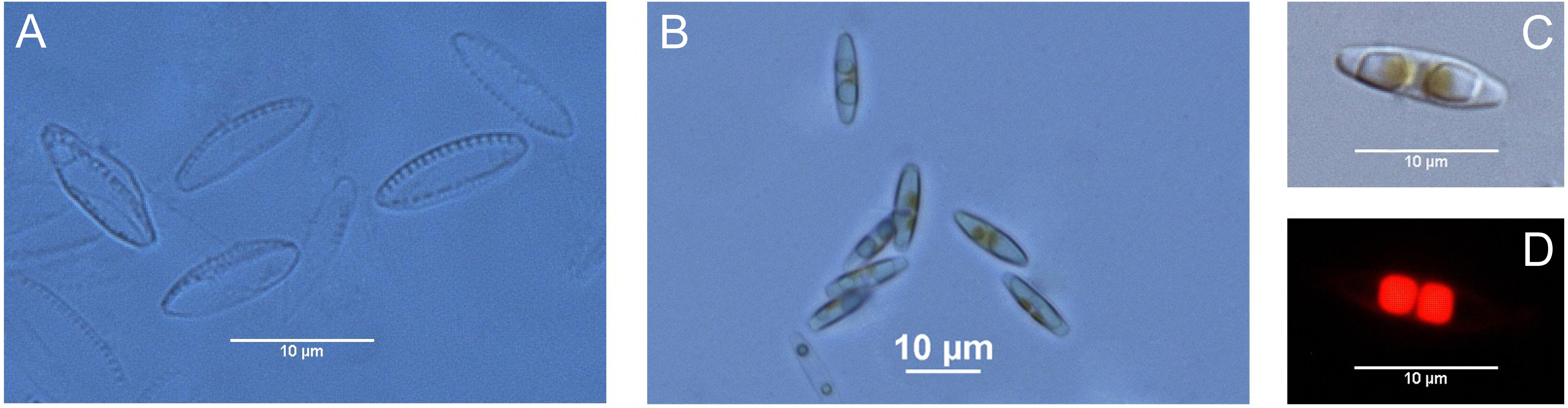
Figure 1. Morphological characteristics of N. cf. aurariae, frustuls mounted in Naphrax (A), living cells observed under 40x (B) and 100x oil immersion objective (C), epifluorescence microscopy of chloroplasts (D).
Experiments were conducted with cultures grown in 100 ml Erlenmeyer flask filled with 50 ml of the f/2 medium (Guillard, 1975) prepared from artificial seawater with salinity of 7, which were kept in a growth chamber at the constant temperature of 17°C. The cultures were grown for 7 days until they reached the exponential growth phase under three different light regimes, i.e., constant light (CL) and two variable light regimes (VL). Under constant light conditions, three different levels of light were applied, i.e., 30 (low light, LL), 115 (medium light, ML), and 350 (high light, HL) μmol photons m–2 s–1. Under variable light, the irradiance was increased automatically from the lowest to the highest level of light at fixed time intervals; after a period of darkness, the samples were illuminated with the lowest level of light for 3 h, after which the light was increased to reach the medium light intensity for another 3 h, and then the highest light was applied for 4 h. Subsequently, the light intensity was decreased in the reversed order at the same time intervals. In the first variant of variable light regimes, i.e., the lower range (LRVL – lower-range variable light), the following set of light intensities was applied, 15 (low light), 30 (medium light), and 150 (high light) μmol photons m–2 s–1, whereas in the second one, the higher range (HRVL – higher-range variable light), these were 30 (low light), 60 (medium light), and 350 (high light) μmol photons m–2 s–1 (Supplementary Figure S1). For each light regime, a photoperiod of 16 h of light and 8 h of darkness was used. Illumination was provided with a set of fluorescent lamps Philips TLD 36 W emitting cool white light. At each applied light level, measurements were performed after an hour of illumination. The oxygen evolution measurements were repeated at 1-h intervals. All measurements were performed on independent biological samples.
Growth Rates
The growth of the diatoms was monitored by cell count using a hemocytometer with the Bürker grid. Specific growth rates (μ, d–1) were calculated according to the equation provided by Fogg and Thake (1987).
Photosynthetic Pigments Analysis
Five millimeter aliquots of cell suspension were taken and filtered through GF/C Whatman glass filters (25 mm diameter) under low vacuum and then frozen and stored at −20°C until further analysis. Pigments were extracted using 4 ml of cold 90% acetone (HPLC grade) at −20°C for 4 h (Strickland and Parsons, 1972). Subsequently, extracts were centrifuged (7000 rpm, 10 min) and filtered through PTFE filters. Pigments were separated with the Waters liquid chromatograph comprising a double pump (Waters 515) system and equipped with the Diode Array Detector 440 (set at 440 nm). Pigment separation was performed using reverse phase chromatography (RP-HPLC) (250 × 4.6 mm LiChrospher 100, octadecylsilica bonded phase with the particle size of 5 μm) following optimized analytical gradient protocol provided by Pniewski (2020). The HPLC system was calibrated using high purity pigment standards purchased from The International Agency for 14C Determination DHI Institute for Water and Environment in Denmark. Pigments were identified from their absorbance spectra and retention times and quantified according to the procedure described by Mantoura and Repeta (1997).
Photosynthesis vs. Irradiance (P-E) Curves
After a 7-day cultivation period, the final concentration of chlorophyll a (Chla) reached 2.00 ± 0.3, 0.91 ± 0.07, 1.0 ± 0.17, 2.21 ± 0.15, 2.12 ± 0.04 mg l–1 at LL, ML, HL, LRVL, and HRVL, respectively. Net oxygen evolution was performed using the Chlorolab 2 system equipped with the DW2/2 liquid-phase chamber unit with an integral oxygen electrode (Hansatech, Norfolk, United Kingdom) (Hansatech, Norfolk, United Kingdom). The system was kept at a constant temperature of 17°C by connecting it to a circulating water bath (Julabo Labortechnik, Seelbach, Germany). Light was provided by LH11/2R high-intensity red LED probe with the maximum light value of 1700 μmol photons m–2 s–1, which was measured with a Quantitherm Light meter (Hansatech, Norfolk, United Kingdom) placed inside the DW2/2 chamber. Polarographic measurements were performed using 2 ml cell suspension aliquots. P-E curves were constructed by measuring oxygen production rates at 10 stepwise increments of light each of which was applied for 5 min (11, 40, 85, 145, 225, 450, 650, 990, 1385, and 1700 μmol photons m–2 s–1). Dark respiration was measured for 10 min prior to the constructing of the light curve. Oxygen evolution rates were expressed per cell and Chla unit. Subsequently, the model provided by Platt et al. (1980) was mathematically fitted to the obtained data in order to estimate photosynthetic parameters, i.e., the maximum photosynthetic capacity (GPmax), the maximum photosynthetic efficiency (α) and the light saturation index (Ek = GPmax/α).
Fluorescence Measurements
Chla variable fluorescence was measured using a computer-operated FMS1 system (Hansatech, Norfolk, United Kingdom) equipped with an amber light (594 nm) emitting diode to excite fluorescence and a PIN-photodiode with l > 700 nm filter to detect it. An internal halogen lamp (8 V/20 W) was the source of actinic and saturating light. All measurements were performed with a 5.5-mm-diameter Fiberoptic kept perpendicular to the sample placed in the temperature-controlled DW2/2 chamber at a constant distance of 4 mm.
The cell suspension subsamples were harvested at an exponential growth phase and filtered onto Whatman GF/C filters under low vacuum (Jesus et al., 2006). Next, small subsamples were cut out from the filter, placed onto a small plastic base, and put into a DW2/2 chamber (Hansatech, Norfolk, United Kingdom) and filled with the medium in which the cells were grown. Prior to each measurement, samples were dark-adapted for 15 min. Next, a pulse of saturating light (SP > 3500 μmol photons m–2 s–1 for 400 ms) was applied to measure minimum (Fo) and maximum fluorescence (Fm) in the dark-adapted state, then the maximum quantum yield of photosystem II (PSII) [Fv/Fm = (Fm–Fo)/Fm] was calculated (Schreiber, 2004). Subsequently, actinic light (AL) of an intensity matching growth irradiance was applied. Once the steady-state was achieved, usually within 8 min of illumination, a saturating pulse was applied to measure a steady-state fluorescence level (F) and maximum fluorescence (Fm’) in the light-adapted state. These allowed calculating the non-photochemical quenching [NPQ = (Fm-Fm’)/Fm’] (Genty et al., 1989; Serôdio et al., 2005).
Statistics
The mean values of all analyzed parameters were compared separately for each applied light regime using the one-way analysis of variance (ANOVA). Post hoc comparisons were performed with Tukey HSD test. To describe linear relationships between analyzed variables, if present, the Pearson correlation coefficient was applied. All statistical analyses were performed using Statistica 10 (StatSoft Inc., United States). The results of statistical tests are provided in Supplementary Information.
Results
Growth Rates
The cell count showed that the diatom N. cf. aurariae grew under all applied light regimes. Considering the fact that there were no statistically significant differences between applied light intensities within each variable light regime, i.e., the lower- (LRVL) and higher-range (HRVL) variable light, all of the obtained growth rate (m) values were pooled together. Comparing constant light levels, a significant difference between low (LL) and high (HL) light (30 and 350 μmol photons m–2 s–1, respectively; Supplementary Table S1) (Tukey HSD test, p < 0.05) was observed. The m value obtained for the lower-range variable light (LRVL) did not vary from the value obtained for the cells from the constant high light (Tukey HSD test, p > 0.05). The highest m was found in cells grown at the higher-range variable light (HRVL) (Figure 2).
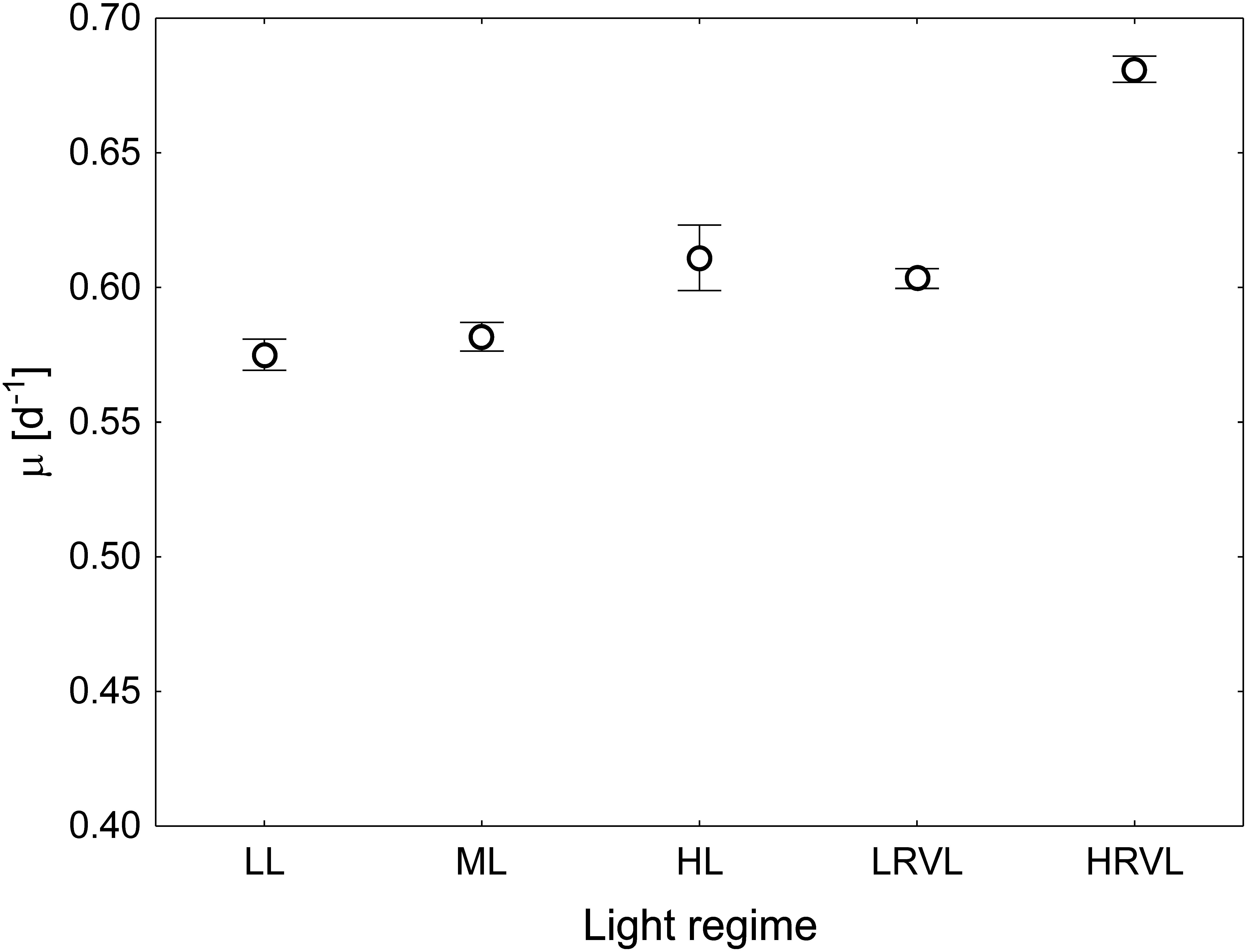
Figure 2. Specific growth rates of N. cf. aurariae grown under various light regimes, i.e., constant light: low light (LL) – 30, medium light (ML) – 115, high light (HL) – 350 μmol photons m–2 s–1, lower range variable light (LRVL), i.e., irradiance varying within the range of 15–150 μmol photons m–2 s–1, and higher range variable light (HRVL), i.e., irradiance varying within the range 30–350 μmol photons m–2 s–1. Each data point is the mean of 3 independent cultures, error bars represent standard error.
Pigments
Cellular pigment content changed significantly under applied light conditions (Supplementary Table S2). Under constant light, photosynthetic pigments, including chlorophyll a (Chla) (Figure 3A) and fucoxanthin (Fuco) decreased, while the content of the xanthophyll cycle pigments, namely: diadinoxanthin and diatoxanthin (Dd + Dt), increased (Tukey HSD test, p < 0.05). No significant changes were observed with respect to chlorophyll c (Chlc) and β-carotene (β-car). Under the lower-range variable light, the content of all pigments did not vary significantly. Conversely, under the higher-range variable light, only Chlc and β-car remained unchanged. Under constant light conditions, the xanthophylls pool [(Dd + Dt)/Chla] increased ca. Ten-fold, reaching the highest value at the highest applied irradiance (350 μmol photons m–2 s–1, corresponding to the TDLD of 20.2 μmol photons m–2; Figure 3B) (Tukey HSD test, p < 0.05). Whereas cells grown at both variable light regimes showed no change in (Dd + Dt)/Chla (ANOVA, p > 0.05) comparing the applied light levels. When the content of Chla was analyzed as a function of total daily light dose (TDLD). It was shown that the values calculated for cells grown under variable light conditions matched the decreasing pattern set by values obtained for cells from the constant light (Figure 3A). Fuco followed the same variation pattern (data not shown). The xanthophylls pool [(Dd + Dt)/Chla] values from LRVL and HRVL, likewise, fitted the constant light pattern (Figure 3B). Furthermore, direct comparison of values obtained for cultures from ML and HRVL, as they received almost the same TDLDs, showed no statistically significant differences considering Chla and (Dd + Dt)/Chla as well (Tukey HSD test, p < 0.05). Under each light regime, the de-epoxidation state [Dt/(Dd + Dt)] differed considerably between the applied light levels (ANOVA, p < 0.05; Figure 4). In constant light, Dt/(Dd + Dt) was two-fold higher at the highest applied irradiance (350 μmol photons m–2 s–1) comparing it to the lowest one (30 μmol photons m–2 s–1) (Tukey HSD test, p < 0.05), whereas in variable light, the Dt/(Dd + Dt) values increased ca. three and four times in LRVL and HRVL, respectively (Tukey HSD test, p < 0.001).
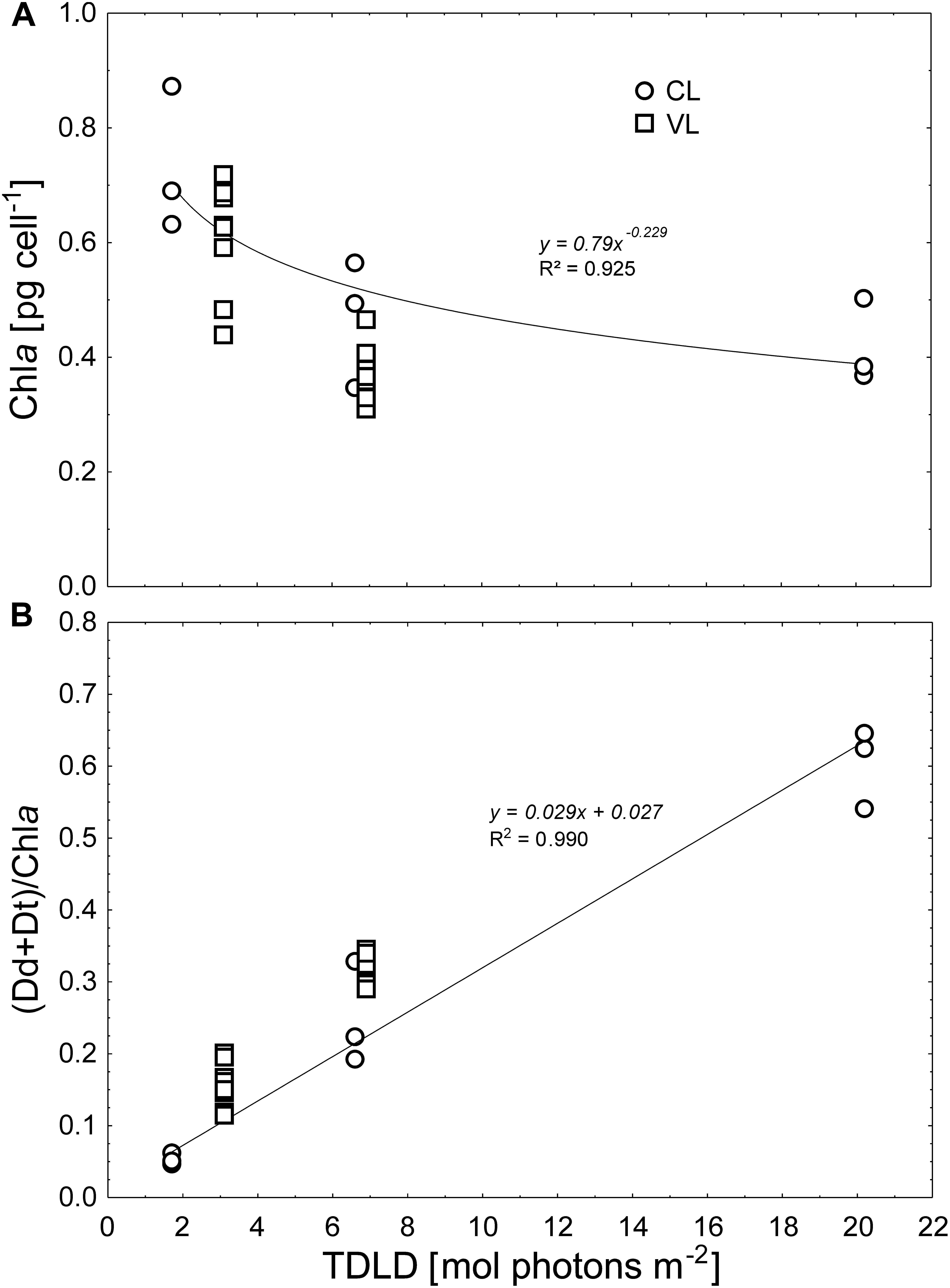
Figure 3. Chlorophyll a content (A) and xanthophylls pool (Dd + Dt)/Chla (B) as a function of total daily light doses (TDLD) in N. cf. aurariae grown under different light regimes: constant light (CL) (∘), and variable light (□).
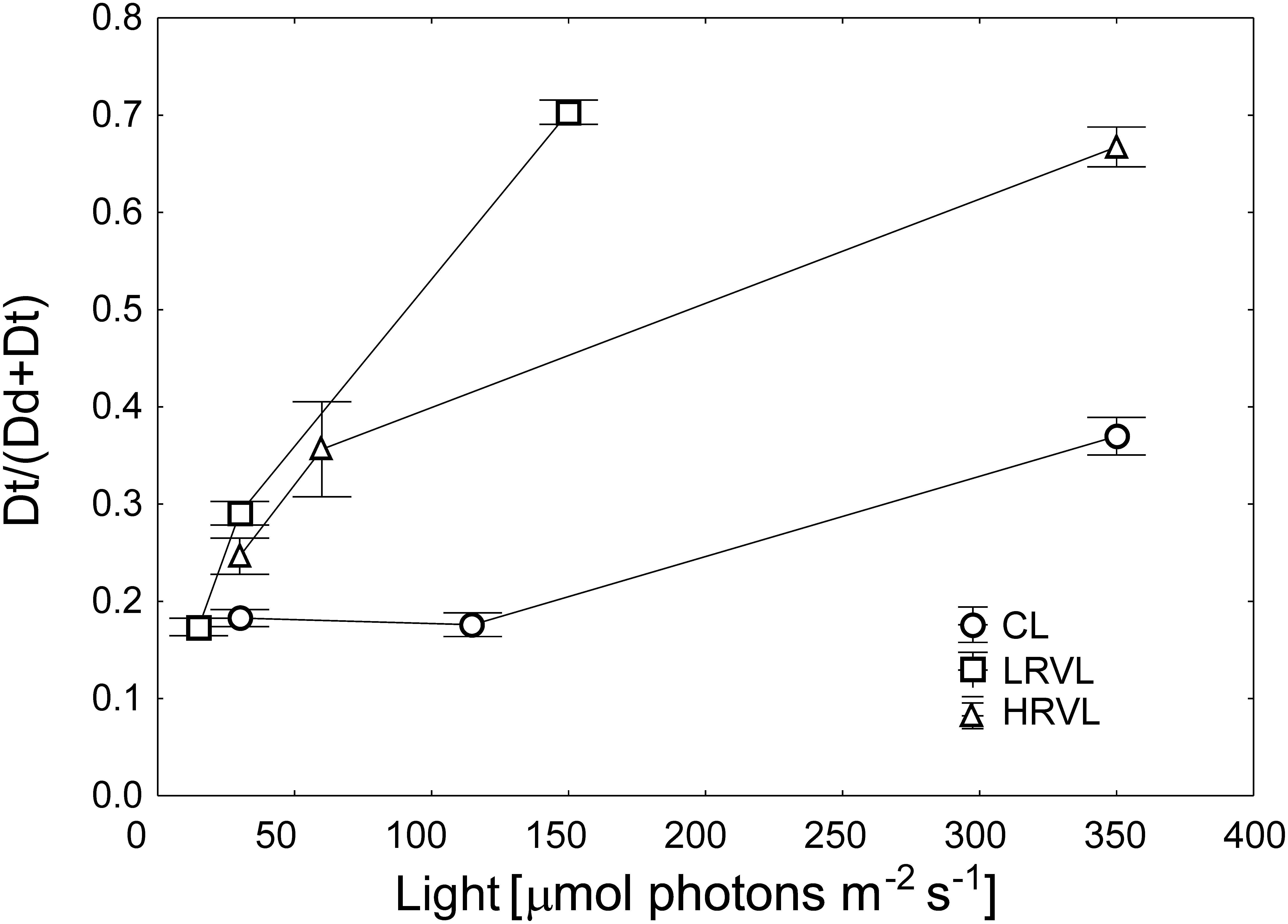
Figure 4. De-epoxidation state [Dt/(Dt + Dd)] in N. cf. aurariae grown under different light regimes: constant light (CL) (∘), i.e., 30, 115, and 350 μmol photons m–2 s–1, lower range variable light (LRVL) (□), i.e., 15, 30, and 150 μmol photons m–2 s–1, and higher range variable light (HRVL) (△), i.e., 30, 60, and 350 μmol photons m–2 s–1. Each data point is the mean of 3 independent cultures, error bars represent standard error.
P-E Curves and Photosynthetic Parameters
The fitting of the mathematical model (Platt et al., 1980) to the data was always good, with R2 exceeding the value of 0.9. The photosynthetic response of the diatom varied significantly between applied light conditions (ANOVA, p < 0.05, Figure 5 and Supplementary Figure S2). In constant light, GPmax expressed per cell basis (GPmax/cell) decreased at higher light intensities, ca. two and four times at ML and HL, respectively (Tukey HSD test, p < 0.05; Figure 5A and Supplementary Table S3). When expressed per Chla basis, no change was observed between LL and ML, whereas at HL GPmax/Chla was two-fold lower (Tukey HSD test, p < 0.05; Figure 5B). The a values for cells grown at LL and ML did not differ (Tukey HSD test, p > 0.05; Supplementary Table S3), while at HL α/cell significantly decreased (Tukey HSD test, p > 0.05; Figure 5C). When expressed per Chla unit, statistical tests showed no difference in α/Chla (Tukey HSD test, p > 0.05; Figure 5D). Although some variations in the shape of the P-E curves were observed in cultures maintained under variable light (LRVL and HRVL as well), differences between the values of these parameters were not statistically significant when comparing the applied light levels (ANOVA, p > 0.05). The means of both parameters calculated for cells from the variable light regimes were systematically higher with respect to those of the constant light regimes (Figure 5). Particular pairs of means were compared, i.e., LL and LRVL, ML and HRVL, as they had similar Chla content. GPmax/cell obtained for cells from LRVL differed significantly from LL (Tukey HSD test, p < 0.05). On the other hand, no significant differences were found between ML and HRVL (Tukey HSD test, p > 0.05; Figure 6). When comparing the same pairs of α/cell means, it was found that they showed significant differences (Tukey HSD test, p < 0.05). Generally, similar patterns were also observed in GPmax/Chla and α/Chla (data not shown). Despite some variations in the light saturation index (Ek), its values estimated for cultures acclimated to LL and ML did not differ significantly (ANOVA, p < 0.5; Supplementary Table S3), while at the highest light, Ek markedly decreased (Tukey HSD test, p < 0.05). The same variation pattern was also exhibited by cells maintained in variable light (Supplementary Table S3).
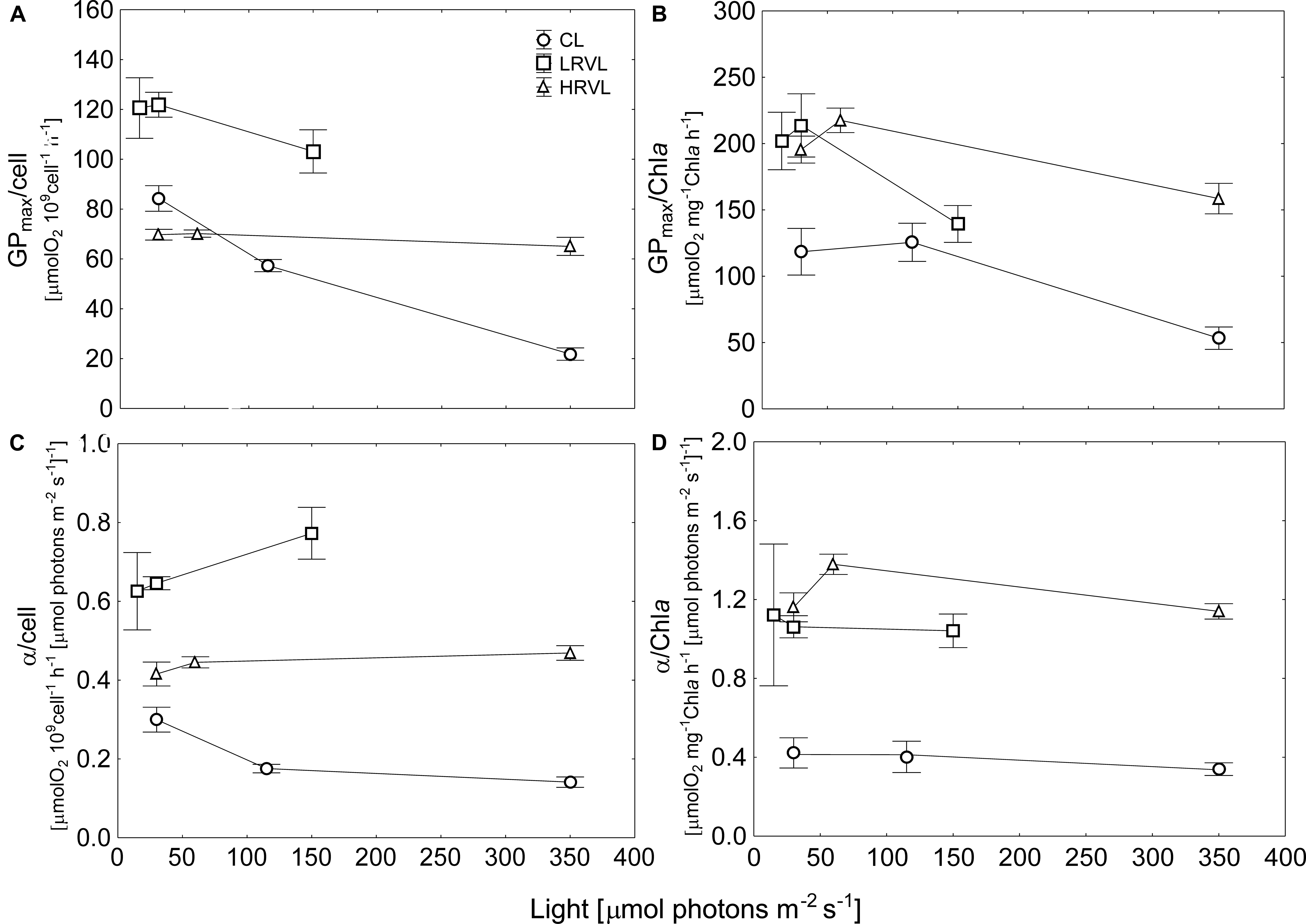
Figure 5. Photosynthetic parameters, the gross maximum photosynthetic capacity expressed per cell (GPmax/cell) (A), the gross maximum photosynthetic capacity expressed per Chla unit (GPmax/Chla) (B), the maximum photosynthetic efficiency expressed per cell (α/cell) (C), the maximum photosynthetic efficiency expressed per Chla unit (α/Chla) (D), in N. cf. aurariae grown under different light regimes: constant light (CL) (∘), i.e., 30, 115, 350 μmol photons m−2 s−1, lower range variable light (LRVL) (□), i.e., 15, 30, 150 μmol photons m−2 s−1, and higher range variable light (HRVL) (△), i.e., 30, 60, 350 μmol photons m−2 s−1. Each data point is the mean of 3 independent cultures, error bars represent standard error.
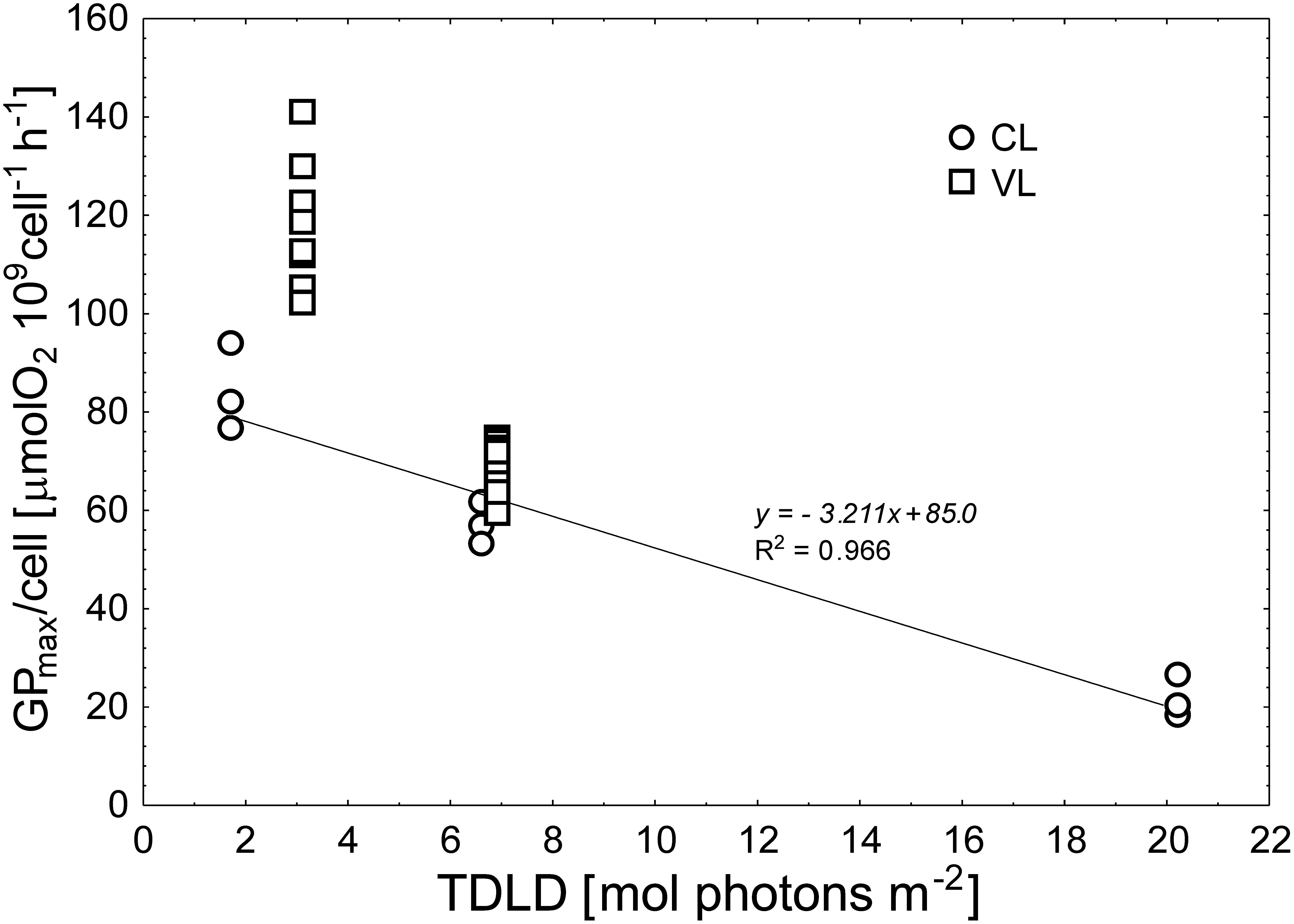
Figure 6. Maximum photosynthetic capacity expressed per cell (GPmax/cell) as a function of total daily light doses (TDLD) in N. cf. aurariae grown under different light regimes: constant light (CL) (∘), and variable light (□).
PAM Fluorescence and Dt Molecules Concentration
Under each light regime, the fluorescence parameters varied significantly (ANOVA, p < 0.5, Supplementary Table S4). Under constant light, Fv/Fm decreased significantly at high light (ANOVA, p > 0.5). In variable light, the trend was more pronounced; the wider the range of the applied light intensities, the larger the decrease in Fv/Fm was observed. The dynamics of Fv/Fm showed a negative correlation with the de-epoxidation state (Figure 7A). NPQ rose with the increase in the light intensity. At the constant light regimes, the sharp NPQ increase was recorded at ML compared to LL, followed by its significant build-up at HL. This pattern was further reflected in the relationship between NPQ and Dt, which was broken down into two linear correlations formed by values obtained for cells from LL and ML; ML and HL. The slope of the former was 0.332, whereas for the latter it decreased to 0.068 (Figure 7B). Under both variable light regimes, at the lowest light levels NPQ was rarely measured (due to the fact that Fm values were lower than Fm’) despite Dt molecules being present (3.6 ± 0.47 at 15 μmol photons m–2 s–1 and 12.5 ± 1.22 at 30 μmol photons m–2 s–1 under LRVL and HRVL, respectively, data not shown). The NPQ increased linearly with the increase in Dt content, reaching values similar to those exhibited by cultures from ML and HL (Figure 7B).
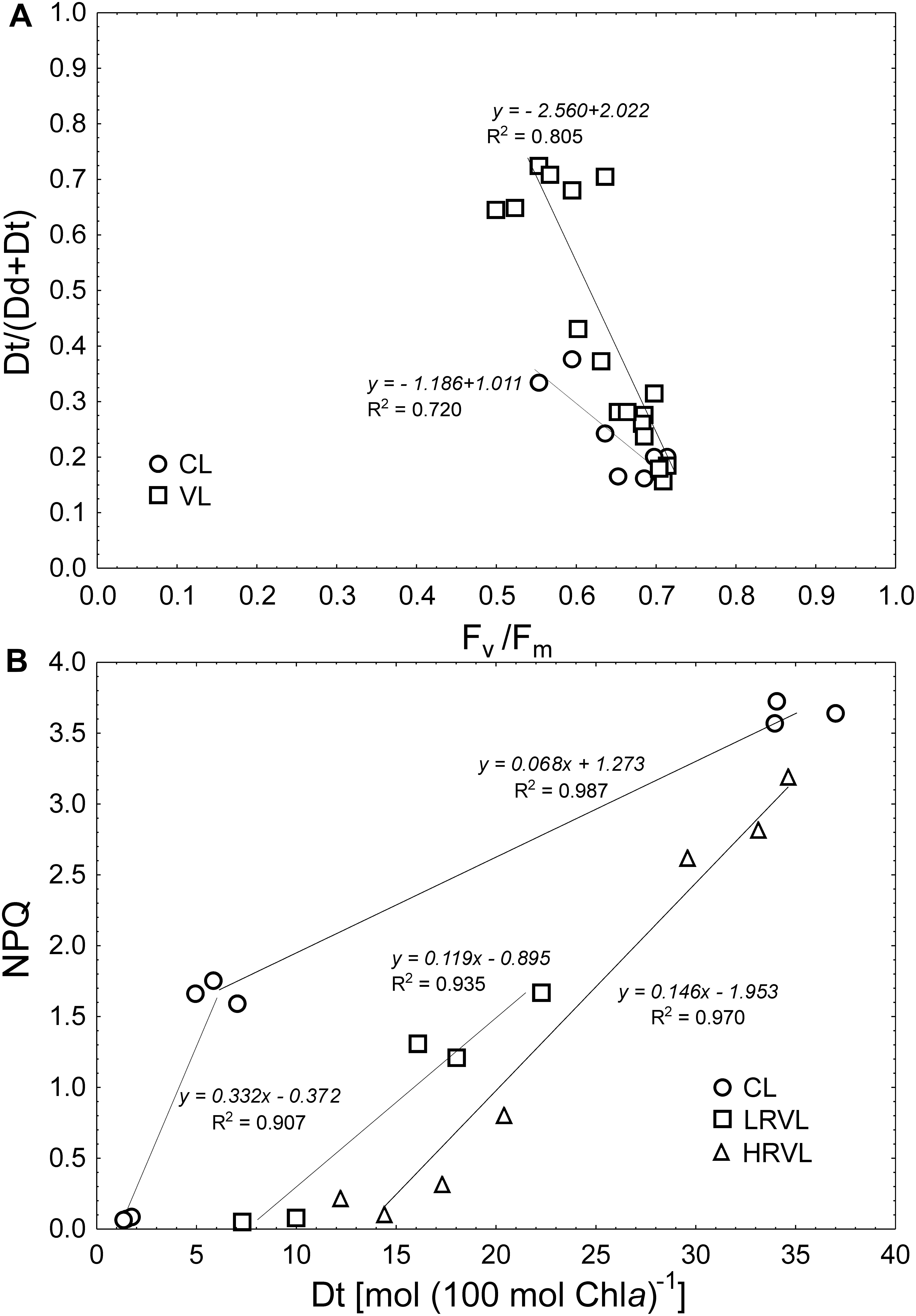
Figure 7. Relationship between de-epoxidation state [Dt/(Dd + Dt)] and the maximum quantum yield of photosystem II (Fv/Fm) (A) in N. cf. aurariae grown under different light regimes: (1) constant light (CL) (∘), and variable light (□), and non-photochemical quenching (NPQ) and diatoxanthin (Dt) concentration (B) in N. cf. aurariae grown under different light regimes: constant light (CL) (∘), lower range variable light (LRVL) (□), and higher range variable light (HRVL) (△).
Discussion
Photoacclimation allows an adjustment of a photosynthetic apparatus for varying light conditions. It also serves to minimize growth variations under changing environmental conditions (as it may also be affected by other variables such as temperature and the availability of nutrients) (e.g., Dubinsky and Stambler, 2009; Lacour et al., 2018; Liefer et al., 2018). This process is reflected in photosynthetic pigment content and, by extension, in the variations of P-E curves, which enable identification of main photoacclimation strategies (Richardson et al., 1983).
The applied variations of light intensities changed pigment composition. The primary acclimation mechanism involved a decrease of the photosynthetic pigments, which is a typical response in microalgae to increasing irradiance (e.g., Ruivo et al., 2011). Pigment composition was determined by the applied TDLD. Similar results were observed in the green alga Dunaliella tertiolecta when the sinusoidal light regime was applied (Havelková-Doušová et al., 2004). Previous studies showed that microalgae do not acclimate to the average, but somewhat lower irradiance when grown under highly fluctuating light conditions thus substantiating the importance of the light regime (e.g., van de Poll et al., 2007). In this study, N. cf. aurariae was incubated for several days under changing light conditions emulating, in a simplified manner, a diurnal irradiance variation (which partially explains differences between results obtained here and in other studies; e.g., Flameling and Kromkamp, 1997) and therefore it can be assumed that the changes in pigment content reflected long-term photoacclimation.
Changes in the content of Chla can be associated with the change in the size and/or number of photosynthetic units (defined as the functional photosynthetic structure comprising the reaction centers together with their accessory pigments and electron carriers (Falkowski and Owens, 1980). Newly synthesized Chla molecules can be added to the already existing PSU or by building up a new one, determining a response of microalgae to changing light conditions (Dring, 1998). These changes can be identified by analyzing variations in the shape of P-E curves produced for microalgae acclimated to diverse light conditions (i.e., low and high light), and several predictive models were provided (Richardson et al., 1983; Sakshaug et al., 1997). Their interpretation, however, strongly depends on the unit to which photosynthetic rates are expressed as they may give different images of photoacclimation (MacIntyre et al., 2002).
In the diatoms grown under constant light conditions, the initial slope (α) as well as the maximum photosynthetic capacity (GPmax), expressed on a per cell basis decreased when grown at higher light intensities. The observed phenotypic responses of P-E curves correspond with models depicting changes in the PSU number (Prézelin, 1981; Richardson et al., 1983). To the best of our knowledge, such variations in the shape of P-E curves have not been previously reported for diatoms. However, it is interesting to note that the same variation pattern was also found in another Baltic diatom, i.e., Opephora sp., isolated from the same location (unpublished data). Previous studies showed that the light-shade photoacclimation, and by extension, the underlying photoacclimation mechanism might be related to the ecological niches occupied by phototrophs (e.g., Falkowski and Owens, 1980). The mechanism involving changes in the number of PSU was reported in green algae and dinoflagellates (e.g., Prézelin and Sweeney, 1978; Falkowski and Owens, 1980). It was also observed in Skeletonema costatum, although it was accompanied by the changes in the size of PSU (Kromkamp and Limbeek, 1993). A photoacclimation strategy based on the change in the PSU number has been considered to be more favorable in habitats with highly fluctuating light (Falkowski and Owens, 1980; Behrenfeld et al., 1998). In the coastal shallows of the Baltic Sea, microalgae experience highly variable light conditions not only due to the seasonal and daily changes in light intensity. They can easily be transported deeper into the sediment due to bioturbation and sediment movement (Josefson et al., 2012), where they can undergo prolonged periods of darkness. They can also be moved to the water column, experiencing light conditions typical of phytoplankton (Pliński, 1993), where, due to the turbidity, the average irradiance decreases while light fluctuations increase. Thus, photoacclimation in N. cf. aurariae through the hypothesized changes in the PSU number coincides with the light climate characteristics typical of its habitat.
Comparing cultures from the constant and variable light regimes, i.e., LL vs. LRVL and ML vs. HRVL, it was shown that despite comparable Chla contents, and in the latter similar TDLDs, LRVL and HRVL cultures had different photosynthetic traits. This confirmed the substantial influence of the light regime and demonstrated that the diatom optimized photosynthesis accordingly. The LRVL and HRVL cultures were also characterized by lower Ek values (ca. two-fold on average), showing that photosynthesis was saturated at lower irradiances. Changes in photosynthetic parameters observed in cultures grown under variable light regimes might have resulted from further alternations in the number of PSUs (as it was indicated to be the primary acclimatory mechanism). With regard to the fact that no significant differences in the Chla content values were observed, it can be presumed that changes in the size of PSUs also occurred. This can be supported by the fact that changes in the PSU size primarily affect the photosynthetic efficiency (a) (Dubinsky and Stambler, 2009); in both compared cultures pairs (i.e., LL vs. LRVL and ML vs. HRVL) a expressed on a per cell and Chla, decreased 2.5 times on average. A change in the number, as well as the size of PSUs induced by variable light regimes, was previously reported in diatoms (Kromkamp and Limbeek, 1993). The authors showed that in fluctuating light, S. costatum acclimated by increasing the number and decreasing the size of PSUs. Moreover, it was also shown that in cultures receiving higher TDLD, α/Chla and GPmax/Chla increased in cells from fluctuating light as compared to those maintained under the sinusoidal light regime. Similar variations in the aforementioned parameters were also observed when the cultures receiving an approximate TDLDs, i.e., ML and HRVL, were compared. Thus, it could be cautiously speculated that under variable light regimes, N. cf. aurariae also increased the number of PSUs and decreased their size. A higher number of PSUs with relatively small size seems to be particularly beneficial to algae inhabiting environments of variable light conditions. They facilitate effective light harvesting at low light and enhance the capacity for compensatory changes in PSII at higher irradiance, decreasing the risk of overexcitation of PSII (Behrenfeld et al., 1998).
In HL cells, maximum photosynthetic rates were strongly reduced. Previous studies argued that such photosynthesis impairment could be caused by high light-induced damage of PSII (e.g., Samuelsson and Richardson, 1982). Photodamage lowers the number of PSU and thus decreases both a and GPmax; a usually decreases first until a critical percentage of PSII centers is deactivated. After that, any additional photodamage causes a decrease in GPmax (Vassiliev et al., 1994; Behrenfeld et al., 1998). Regarding the fact that photoinhibition is not only a light- but also a time-dependent process (Henley, 1993; Blanchard et al., 2004; Serôdio et al., 2012), long-term exposure to elevated irradiance (350 μmol photons m–2 s–1) indicated that N. cf. aurariae experienced high light stress. Under variable light conditions, on the other hand, the photoinhibitory effect of high light was diminished, possibly due to the shorter exposure time (4 vs. 16 h) as well as a putative decrease in the PSU size. Also a high light period was followed by low light, which stimulates D1 turnover and PSII repair (Behrenfeld et al., 1998; Tyystjärvi, 2013). Generally, the capacity for repair of PSII in epipelic and epispammic species was shown to be greater than suffered photodamage. Although epipsammon species seem to rely more on the reduction of photoinactivation due to physiological photoprotection rather than costly repair mechanisms (Frankenbach et al., 2018). Thus, the limited (mostly statistically insignificant) decrease in photosynthetic rates obtained when the diatom was exposed to the highest light levels under the variable light regimes could possibly be an effect of the up-regulation of photoprotection (Henley, 1993; Zhu and Green, 2010). Moreover, photoinhibition may also be considered as a photoprotective mechanism. Damaged PSII itself can act as non-photochemical quenchers (Matsubara and Chow, 2004; Zavafer et al., 2019), preventing over-reduction of the photosynthetic electron transport chain (Rumeau et al., 2007). As a result, N. cf. aurariae was able to achieve a certain balance between photoinhibition and photoprotection, resulting in sufficient photosynthesis supporting its growth. And consequently, enabling the diatom to survive prolonged periods of high light.
The cultures grown under constant light conditions exhibited a similar growth rate independent of light intensity. There was no considerably lower growth rate at LL, as it was reported for other diatoms (e.g., Mouget et al., 1999). Alternatively, it could be stated that the growth of N. cf. aurariae at HL, although still higher than at LL and ML, was somewhat restricted due to the inability of the diatom to acclimate to high irradiance. In variable light, when the diatom was mainly exposed to low light intensities (15–30 and 30–60 μmol photons m–2 s–1 at LR and HRVL, respectively, for 12 out of 16 h of illumination period), growth rates were even higher. Such results partially explain environmental observations showing that N. cf. aurariae was present in epispammic communities throughout the year, and it was most frequently found in autumn (Pniewski, 2010), when lower irradiance predominates (monthly means of light intensity at the sea surface are below ca. 345 μmol photons m–2 s–1; Kaczmarek and Dera, 1998). Nonetheless, increased photosynthetic rates under variable light conditions suggested that the diatom, due to its specific photoacclimation (i.e., an increased number and decreased size of PSU), could also benefit from the shorter high light illumination periods.
In constant light, the xanthophylls pool [(Dd + Dt)/Chla] was determined by the applied TDLD. As with the Chla content, (Dd + Dt)/Chla for LR and HLVL fitted the pattern set by the values obtained from the constant light cultures. Both xanthophylls, i.e., Dd and Dt, form the one-step reaction xanthophyll cycle, allowing diatoms to instantaneously balance excitation energy and/or ensure photoprotection (e.g., Lhor and Wilhelm, 1999; Goss and Jakob, 2010; Lavaud and Lepetit, 2013). The main difference between cells grown under constant and variable light conditions was the activity of the xanthophyll cycle; Dt/(Dd + Dt) was almost twice as high in variable light as at constant high light. A high amount of Dt molecules is crucial for efficient photoprotection, enabling NPQ build-up responsible for the thermal dissipation of excess energy (Lavaud et al., 2004; Zhu and Green, 2010). Recent studies showed that NPQ, as well as the xanthophyll cycle, are species/strain-specific traits regulating a functional response of microalgae to varying light conditions (e.g., Lavaud et al., 2007; Lavaud and Lepetit, 2013). In benthic diatoms, it was proven that photoprotective mechanisms are also strongly dependent on their growth form. The data obtained here coincided with features characteristic for non-motile epispammic diatoms (Barnett et al., 2015; Blommaert et al., 2017). N. cf. aurariae was able to reach very high NPQ (which agrees with NPQ values previously observed in Baltic microphytobenthos; Pniewski et al., 2017), regardless of the light regime. In ML cultures of N. cf. aurariae, the xanthophylls pool increased (as compared to LL), while no change in the de-epoxidation state was observed. This indicated that the NPQ was developed due to higher Dt accumulation, originating from a larger xanthophylls pool and not from an increased de-epoxidiaiton state (Blommaert et al., 2018). However, in HL cells, together with the increased (Dd + Dt)/Chla values, Dt/(Dd + Dt) also increased. The prominence of the de-epoxidation state was even greater in variable light, and it was dependent on the light intensities applied. Such observations are also consistent with previous reports showing a stronger de-epoxidation state in diatom-dominated epipsammic communities (e.g., Jesus et al., 2009; Cartaxana et al., 2011; Pniewski et al., 2015). A high NPQ capacity in epipsammic diatoms was shown to be their main photoprotection mechanism as they have no or strongly limited behavioral control over their light environment (Barnett et al., 2015; Frankenbach et al., 2018).
In constant light, NPQ increased with the content of Dt molecules. However, this relationship was not linear. At HL, despite a sharp increase in Dt, the expected build-up of NPQ was not observed. Furthermore, despite the fact that in cells from the variable light regimes, at low light intensities (i.e., 15 and 30 μmol photons m–2 s–1) a substantial amount of Dt molecules was already present, NPQ could not be measured, or it was very low. This suggests that not all Dt molecules participated to the NPQ development. The possible explanation could be the presence of Dt molecules in the lipid matrix of thylakoids and protein-bound Dt in PSI, which may help to avoid lipid peroxidation, and to scavenge ROS reducing the possibility of oxidative damage (Lepetit et al., 2010; Lavaud and Lepetit, 2013). These additional Dt pools (i.e., not involved in NPQ development) could play an essential role in photoprotection, especially under constant high light conditions, preventing further PSII inactivation and preserving its functionality as turnover of D1 is inhibited at saturating light intensities (Behrenfeld et al., 1998). Growing the diatom under various light conditions suggested that its photoprotection was based on a certain balance between (Dd + Dt) content and the de-epoxidation state (Lacour et al., 2018). Long-term photoacclimation regulated the xanthophylls pool according to the received TDLD, and it seemed that it also determined the distribution of the xanthophyll cycle pigments in the cell (at least with respect to their involvement, or lack of it, in the NPQ build-up), while the de-epoxidation state acted as a fine-tuning process allowing precise regulation of the dissipation of excess energy, as required by specific light intensities. This further shows that epipsammic diatoms use various mechanisms to cope with changing light (Blommaert et al., 2017).
The Fv/Fm values decreased at higher light intensities; before measurements, samples were dark-adapted for 15 min, retaining some fraction of NPQ (“dark” NPQ) and thus lowering Fv/Fm. Such observations were reported in various diatom species as well as microphytobenthos communities (e.g., Serôdio et al., 2005; Lacour et al., 2018). “Dark” NPQ is often observed after prolonged exposure to high light resulting from the restricted epoxidation of Dt to Dd as Dt epoxidase functions more effectively in low light than in darkness (Goss et al., 2006; Lavaud and Lepetit, 2013). It can also be developed in the dark due to the chlororespiratory proton gradient (Jakob et al., 2001; Serôdio et al., 2005). The presence of “dark” NPQ is considered as an advantageous feature allowing diatoms to maintain their antenna system in a dissipative state promptly providing photoprotection upon rapid light exposure (Lavaud and Goss, 2014). Such an adaptation seems to be particularly relevant in species occupying habitats with highly fluctuating light, including sediments of coastal shallows (Perkins et al., 2011).
Conclusion
Previous studies carried out on the Baltic epipsammic assemblages dominated by diatoms showed that they are characterized by high primary production, similar to epipelic communities and that they are resistant to high light. They also quickly respond to changing light conditions (Pniewski et al., 2015, 2017, 2018). The photophysiological properties of microalgal communities seem to be the resultant characteristics typical of their members. The data obtained provided evidence for the considerable photoacclimatory potential of N. cf. aurariae that can help to ensure the resilience of microphytobenthos communities, especially under lower light conditions. The cultures grown in constant light showed general long-term photoacclimation patterns of the species. Whereas, variable light regimes depicted specific modifications in their photosynthetic characteristics. The photosynthesis of N. cf. auraraie was more efficient at lower light intensities, which may be explained by the fact that in its natural environment, the diatom can experience subsaturating irradiance (or even darkness) for an extended time. In contrast, photoprotection represented an interplay between long-term photoacclimation and rapid adjustment to ambient light conditions within the constraints set by the former. Diminished photosynthetic rates under constant high light indicated that the diatom can suffer from prolonged exposure to excess irradiance, most likely resulting from lower light acclimation. Overall, the adaptive plasticity of the studied diatom seems to be developed as a response to variable light conditions characteristic of its natural habitat, i.e., coastal shallows, and shaped by the lifestyle and growth form of the diatom. It can also be tentatively assumed that the physiological versatility of N. cf. aurariae facilitated its ecological success as one of the most common diatoms in the Baltic epipsammon.
Data Availability Statement
The datasets generated for this study are available on request to the corresponding author.
Author Contributions
FP developed the idea and performed HPLC pigment analyses. IP-J prepared and carried out the experiments as well as performed growth, photosynthesis, and fluorescence measurements. FP and IP-J performed statistical analyses and wrote the manuscript.
Funding
This study was funded within the statutory funds of the Department of Marine Ecosystems Functioning at the Institute of Oceanography University of Gdańsk.
Conflict of Interest
The authors declare that the research was conducted in the absence of any commercial or financial relationships that could be construed as a potential conflict of interest.
Acknowledgments
We thank John Breslin and Daniel Naude for language consultations, and two reviewers for critical comments on the manuscript.
Supplementary Material
The Supplementary Material for this article can be found online at: https://www.frontiersin.org/articles/10.3389/fmars.2020.00381/full#supplementary-material
References
Barnett, A., Méléder, V., Blommaert, L., Lepetit, B., Gaudin, P., Vyverman, W., et al. (2015). Growth form defines physiological photoprotective capacity in intertidal benthic diatoms. ISME J. 9, 32–42. doi: 10.1038/ismej.2014.105
Behrenfeld, M. J., Prasil, O., Kolber, Z. S., Babin, M., and Falkowski, P. G. (1998). Compensatory changes in Photosystem II electron turnover rates protect photosynthesis from photoinhibition. Photosynth. Res. 58, 259–268. doi: 10.1023/A:1019894720789
Blanchard, G. F., and Cariou-Le Gall, V. (1994). Photosynthetic characteristic of microphytobenthos in Marennes-Oléron Bay, France: preliminary results. J. Exp. Mar. Biol. Ecol. 182, 1–14.
Blanchard, G. F., Guarini, J.-M., Dang, C., and Richard, P. (2004). Characterizing and quantifying photoinhibition in intertidal microphytobenthos. J. Phycol. 40, 692–696.
Blommaert, L., Huysman, M. J. J., Vyverman, W., Lavaud, J., and Sabbe, K. (2017). Contrasting NPQ dynamics and xanthophyll cycling in a motile and a non-motile intertidal benthic diatom. Limnol. Oceanogr. 62, 1466–1479.
Blommaert, L., Lavaud, J., Vyverman, W., and Sabbe, K. (2018). Behavioural versus physiological photoprotection in epipelic and epipsammic benthic diatoms. Eur. J. Phycol. 53, 146–155.
Cartaxana, P., Ruivo, M., Hubas, C., Davidson, I., Serôdio, J., and Jesus, B. (2011). Physiological versus behavioral photoprotection in intertidal epipelic and epipsammic benthic diatom communities. J. Exp. Mar. Biol. Ecol. 405, 120–127.
Depauw, F. A., Rogato, A., d’Alcalá, M. R., and Falciatore, A. (2012). Exploring the molecular basis of responses to light in marine diatoms. J. Exp. Bot. 63, 1575–1591. doi: 10.1093/jxb/ers005
Dubinsky, Z., and Stambler, N. (2009). Photoacclimation processes in phytoplankton: mechanisms, consequences, and applications. Aquat. Microb. Ecol. 56, 163–176.
Eberhard, S., Finazzi, G., and Wollman, F.-A. (2008). The dynamics of photosynthesis. Annu. Rev. Genet. 42, 462–515.
Flameling, I. A., and Kromkamp, J. (1997). Photoacclimation of Scenedesmus protuberans (Chlorophyceae) to fluctuating irradiances simulating vertical mixing. J. Plankton Res. 19, 1011–1024.
Fogg, G. E., and Thake, B. (1987). Algal Culture and Phytoplankton Ecology. Madison, WI: The University of. Wisconsin press.
Frankenbach, S., Schmidt, W., Frommlet, J. C., and Serôdio, J. (2018). Photoinactivation, repair and the motility-physiology trade-off in microphytobenthos. Mar. Ecol. Prog. Ser. 601, 41–57.
Genty, B., Briantais, J.-M., and Baker, N. R. (1989). The relationship between the quantum yield of photosynthetic electron transport and quenching of chlorophyll fluorescence. Biochim. Biophys. Acta 990, 87–92.
Goss, R., and Jakob, T. (2010). Regulation and function of xanthophyll cycle-dependent photoprotection in algae. Photosynth. Res. 106, 103–122. doi: 10.1007/s11120-010-9536-x
Goss, R., Pinto, E. A., Wilhelm, C., and Richter, M. (2006). The importance of a highly active and ΔpH-regulated diatoxanthin epoxidase for the regulation of the PS II antenna function in diadinoxanthin cycle containing algae. J. Plant Physiol. 163, 1008–1021. doi: 10.1016/j.jplph.2005.09.008
Guillard, R. L. (1975). “Culture of phytoplankton for feeding marine invertebrates,” in Culture of Marine Invertebrate Animals, eds W. L. Smith and M. N. Chanle (New York, NY: Plenum Press), 29–60.
Havelková-Doušová, H., Prášil, O., and Behrenfeld, M. J. (2004). Photoacclimation of Dunaliella tertiolecta (Chlorophyceae) under fluctuating irradiance. Photosynthetica 42, 273–281.
Henley, W. J. (1993). Measurement and interpretation of photosynthetic light-response curves in algae in the context of photoinhibition and diel changes. J. Phycol. 29, 729–739.
Jakob, T., Goss, R., and Wilhelm, C. (2001). Unusual pH-dependence of diadinoxanthin de-epoxidase activation causes chlororespiration induced accumulation of diatoxanthin in the diatom Phaeodactylum tricornutum. J. Plant Physiol. 158, 383–390.
Jesus, B., Brotas, V., Ribeiro, L., Mendes, C. R., Cartaxana, P., and Paterson, D. M. (2009). Adaptations of microphytobenthos assemblages to sediment type and tidal position. Cont. Shelf Res. 29, 1624–1634.
Jesus, B., Perkins, R. G., Mendes, C. R., Brotas, V., and Paterson, D. M. (2006). Chlorophyll fluorescence as a proxy for microphytobenthic biomass: alternatives to the current methodology. Mar. Biol. 150, 17–28.
Josefson, A. B., Norkko, J., and Norkko, A. (2012). Burial and ecomposition of plant pigments in surface sediments of the Baltic Sea: role of oxygen and benthic fauna. Mar. Ecol. Prog. Ser. 455, 33–49.
Kaczmarek, S., and Dera, J. (1998). Radiation flux balance of the sea-atmosphere system over the southern Baltic Sea. Oceanologia 40, 277–306.
Kromkamp, J., and Limbeek, M. (1993). Effect of short-term variaition in irradiance on light harvesting and photosynthesis of the marine diatom Skeletonema costatum: a laboratory study simulating vertical mixing. J. Gen. Microbiol. 1993, 2277–2284.
Kühl, M., Lassen, C., and Jørgensen, B. B. (1994). Light penetration and light intensity in sand marine sediments measured with irradiance and scalar irradiance fiber-optic microprobes. Mar. Ecol. Prog. Ser. 105, 139–148.
Lacour, T., Larivière, J., Ferland, J., Bruyant, F., Lavaud, J., and Babin, M. (2018). The role of sustained photoprotective non-photochemical quenching in low temperature and high light acclimation in the bloom-forming arctic diatom Thalassiosira gravida. Front. Mar. Sci. 5:354. doi: 10.3389/fmars.2018.00354
Lavaud, J., and Goss, R. (2014). “The peculiar features of non-photochemical fluorescence quenching in diatoms and brown algae,” in Non-Photochemical Quenching and Energy Dissipation in Plants, Algae and Cyanobacteria, eds B. Demmig-Adams, G. Garab, and W. Adams Govindjee (Dordrecht: Springer Netherlands), 421–443.
Lavaud, J., and Lepetit, B. (2013). An explanation for the inter-species variability of the photoprotective non-photochemical chlorophyll fluorescence quenching in diatoms. Biochim. Biophys. Acta 1827, 294–302. doi: 10.1016/j.bbabio.2012.11.012
Lavaud, J., Rousseau, B., and Etienne, A.-L. (2004). General features of photoprotection by energy dissipation in planktonic diatoms (Bacillariophyceae). J. Phycol. 40, 130–137.
Lavaud, J., Strzepek, R. F., and Kroth, P. G. (2007). Photoprotection capacity differs among diatoms: possible consequences on the spatial distribution of diatoms related to fluctuations in the underwater light climate. Limnol. Oceanogr. 52, 1188–1194.
Lepetit, B., Volke, D., Gilbert, M., Wilhelm, C., and Goss, R. (2010). Evidence for the existence of one antenna-associated, lipid-dissolved and two protein-bound pools of diadinoxanthin cycle pigments in diatoms. Plant Physiol. 154, 1905–1920. doi: 10.1104/pp.110.166454
Lhor, M., and Wilhelm, C. (1999). Algae displaying the diadinoxanthin cycle also possess the violaxanthin cycle. Proc. Natl. Acad. Sci. U.S.A. 96, 8784–8789. doi: 10.1073/pnas.96.15.8784
Liefer, J. D., Garg, A., Campbell, D. A., Irwin, A. J., and Finkel, Z. V. (2018). Nitrogen starvation induces distinct photosynthetic responses and recovery dynamics in diatoms and prasinophytes. PLoS One 13:e0195705. doi: 10.1371/journal.pone.0195705
Lucas, C. H., Banham, C., and Holligan, P. M. (2001). Benthic-pelagic exchange of microalgae at a tidal flat. 2. Taxonomic analysis. Mar. Ecol. Prog. Ser. 212, 39–52.
MacIntyre, H. L., Geider, R. J., and Miller, D. C. (1996). Microphytobenthos: the ecological role of the “secret garden” of unvegetated, shallow-water marine habitats. I. Distribution, abundance and primary production. Estuaries 19, 186–201.
MacIntyre, H. L., Kana, T. M., Anning, T., and Geider, R. J. (2002). Photoacclimation of photosynthesis irradiance response curves and photosynthetic pigments in microalgae and cyanobacteria. J. Phycol. 38, 17–38.
MacIntyre, H. L., Kana, T. M., and Geider, R. J. (2000). The effect of water motion on short-term rates of photosynthesis by marine phytoplankton. Trends Plant Sci. 5, 12–17. doi: 10.1016/s1360-1385(99)01504-6
Mantoura, R. F. C., and Repeta, D. J. (1997). “Calibration methods for HPLC,” in Phytoplankton Pigments in Oceanography: Guidelines to Modern Methods, eds S. W. Jeffrey, R. F. C. Mantoura, and S. W. Wright (Paris: UNESCO Publishing), 407–428.
Matsubara, S., and Chow, W. S. (2004). Populations of photoinactivated photosystem II reaction centers characterized by chlorophyll a fluorescence lifetime in vivo. Proc. Natl. Acad. Sci. U.S.A. 101, 18234–18239. doi: 10.1073/pnas.0403857102
Mouget, J.-L., Tremblin, G., Morant-Manceau, A., Morançais, M., and Robert, J.-M. (1999). Long-term photoacclimation of Haslea ostrearia (Bacillariophyta): effect of irradiance on growth rates, pigment content and photosynthesis. Eur. J. Phycol. 34, 109–115.
Perkins, R. G., Kromkamp, J. C., Serôdio, J., Lavaud, J., Jesus, B., Mouget, J. L., et al. (2011). “The application of variable chlorophyll fluorescence to microphytobenthic biofilms,” in Chlorophyll a Fluorescence in Aquatic Sciences: Methods and Applications, eds J. D. Suggett, O. Prášil, and A. M. Borowitzka (Dordrecht: Springer Netherlands), 237–275.
Platt, T., Gallegos, C. L., and Harrison, W. G. (1980). Photoinhibition of photosynthesis in natural assemblages of marine phytoplankton. J. Mar. Res. 38, 687–701.
Pliński, M. (1993). “Fitoplankton,” in Zatoka Pucka, ed. K. Korzeniewski (Gdańsk: Fundacja Rozwoju Uniwersytetu Gdańskiego), 378–387.
Pniewski, F. (2010). Photosynthetic Performance of Microphytobenthos from Intertidal Mudflats in Aiguillon Bay (Atlantic coast, France) and Non-tidal Coastal Shallows of Puck Bay (Baltic Sea, Poland). Ph.D. thesis, University of Gdańsk, Gdynia.
Pniewski, F. (2020). HPLC separation of cyanobacterial and algal photosynthetic pigments. Biologia 75, 223–233. doi: 10.2478/s11756-019-00407-8
Pniewski, F. F., Biskup, P., Bubak, I., Richard, P., Latała, A., and Blanchard, G. (2015). Photoregulation in microphytobenthos from intertidal mudflats and non-tidal coastal shallows. Estuar. Coast. Shelf Sci. 152, 153–161.
Pniewski, F. F., Richard, P., Latała, A., and Blanchard, G. (2017). Non–photochemical quenching in epipsammic and epipelic microalgal assemblages from two marine ecosystems. Cont. Shelf Res. 136, 74–82.
Pniewski, F. F., Richard, P., Latała, A., and Blanchard, G. (2018). Long- and short-term photoacclimation in epipsammon from non-tidal coastal shallows compared to epipelon from intertidal mudflat. J. Sea Res. 136, 1–9.
Prézelin, B. B. (1981). “Light reactions in photosynthesis,” in Physiological Bases of Phytoplankton Ecology, ed. T. Platt (Ottawa, ON: Fisheries Research Board of Canada), 1–46.
Prézelin, B. B., and Sweeney, B. M. (1978). Photoadaptation of photosynthesis in Gonyaulax polyedra. Mer. Biol. 48, 27–35.
Raven, J. A., and Geider, R. J. (2003). “Adaptation, acclimation and regulation in algal photosynthesis,” in Photosynthesis in Algae, eds A. W. D. Larkum, S. E. Douglas, and J. A. Raven (Dordrecht: Academic Publisher), 385–412. doi: 10.1038/nature08587
Richardson, K., Beardall, L., and Raven, J. A. (1983). Adaptation of unicellular algae to irradiance: an analysis of strategies. New Phytol. 93, 157–191.
Ruivo, M., Amorin, A., and Cartaxana, P. (2011). Effects of growth phase and irradiance on phytoplankton pigment ratios: implications for chemotaxonomy in coastal waters. J. Plankton Res. 33, 1012–1022.
Rumeau, D., Peltier, G., and Cournac, L. (2007). Chlororespiration and cyclic electron flow around PSI during photosynthesis and plant stress response. Plant Cell Environ. 30, 1041–1051. doi: 10.1111/j.1365-3040.2007.01675.x
Saint-Béat, B., Dupuy, C., Agogué, H., Carpentier, A., Chalumeau, J., Como, S., et al. (2014). How does the resuspension of the biofilm alter the functioning of the benthos–pelagos coupled food web of a bare mudflat in Marennes-Oléron Bay (NE Atlantic)? J. Sea Res. 92, 144–157.
Sakshaug, E., Bricaud, A., Dandonneau, Y., Falkowski, P. G., Kiefer, D. A., Legendre, L. L., et al. (1997). Parameters of photosynthesis: definitions, theory and interpretation of results. J. Plankton Res. 19, 1637–1670.
Samuelsson, G., and Richardson, K. (1982). Photoinhibition at low quantum flux densities in marine dinoflagellate (Amphidinium carterae). Mar. Biol. 70, 21–26.
Schreiber, U. (2004). “Pulse-amplitude-modulation (PAM) fluorometry and saturation pulse method: an overview,” in Chlorophyll a Fluorescence. A Signature of Photosynthesis, eds G. C. Papageorgiou Govindjee (Dordrecht: Springer), 279–319.
Serôdio, J., Cruz, S., Vieira, S., and Brotas, V. (2005). Non-photochemical quenching of chlorophyll fluorescence and operation of the xanthophyll cycle in estuarine microphytobenthos. J. Exp. Mar. Biol. Ecol. 326, 157–169.
Serôdio, J., Ezequiel, J., Barnett, A., Mouget, J.-L., Méléder, V., Laviale, M., et al. (2012). Efficiency of photoprotection in microphytobenthos: role of vertical migration and the xanthophyll cycle against photoinhibition. Aquat. Microb. Ecol. 67, 161–175.
Strickland, I. D. H., and Parsons, T. R. (1972). A Practical Handbook of Seawater Analysis. Ottawa, ON: Fisheries Research Board of Canada, 310.
Sundbäck, K., Miles, A., and Linares, F. (2006). Nitrogen in nontidal littoral sediments: role of microphytobenthos and denitrification. Estuaries Coasts 29, 1196–1211.
Sutherland, T. F., Grant, J., and Amos, C. L. (1998). The effect of carbohydrate production by the diatom Nitzschia curvilineata on the erodibility of sediment. Limnol. Oceanogr. 43, 65–72.
Underwood, G. J. C. (2005). Microalgal (microphytobenthic) biofilms in shallow coastal waters: how important are species? Proc. Calif. Acad. Sci. 56, 162–169.
van de Poll, W. H., Visser, R. J. W., and Buma, A. G. J. (2007). Acclimation to a dynamic irradiance regime changes excessive irradiance sensitivity of Emiliania huxleyi and Thalassiosira weissflogii. Limnol. Oceanogr. 52, 1430–1438.
Vassiliev, I. R., Prasil, O., Wyman, K. D., and Kolber, Z. (1994). Inhibition of PSII photochemistry by PAR and UV radiation in natural phytoplankton communities. Photosynth. Res. 42, 51–64. doi: 10.1007/BF00019058
Witkowski, A., Lange-Bertalot, H., and Metzeltin, A. (2000). Diatom Flora of Marine Coast I. Koönigstein: A.R.G. Ganter, 369–370.
Zavafer, A., Iermak, I., Cheah, M. H., and Chow, W. S. (2019). Two quenchers formed during photodamage of phostosystem II and the role of one quencher in preemptive photoprotection. Sci. Rep. 9:17275. doi: 10.1038/s41598-019-53030-7
Keywords: photoacclimation, photosynthetic units, photosynthesis-irradiance curves, photosynthetic pigments, diatoms, Baltic
Citation: Pniewski F and Piasecka-Jędrzejak I (2020) Photoacclimation to Constant and Changing Light Conditions in a Benthic Diatom. Front. Mar. Sci. 7:381. doi: 10.3389/fmars.2020.00381
Received: 15 October 2019; Accepted: 04 May 2020;
Published: 29 May 2020.
Edited by:
João Serôdio, University of Aveiro, PortugalReviewed by:
Johann Lavaud, Centre National de la Recherche Scientifique (CNRS), FranceTorsten Jakob, Leipzig University, Germany
Copyright © 2020 Pniewski and Piasecka-Jędrzejak. This is an open-access article distributed under the terms of the Creative Commons Attribution License (CC BY). The use, distribution or reproduction in other forums is permitted, provided the original author(s) and the copyright owner(s) are credited and that the original publication in this journal is cited, in accordance with accepted academic practice. No use, distribution or reproduction is permitted which does not comply with these terms.
*Correspondence: Filip Pniewski, ZmlsaXAucG5pZXdza2lAdWcuZWR1LnBs