- 1Marine Program, South African National Biodiversity Institute, Cape Town, South Africa
- 2Institute for Coastal and Marine Research, Nelson Mandela University, Port Elizabeth, South Africa
- 3Egagasini Node, South African Environmental Observation Network, Cape Town, South Africa
- 4Department of Biological Sciences and Marine Research Institute, University of Cape Town, Cape Town, South Africa
Without baseline data from near pristine assemblages, measures of ecosystem change may be significantly underestimated. A unique historical dataset provided an opportunity to investigate long-term change in demersal fish assemblages of South Africa’s inshore trawl grounds. Three sites surveyed over a period from 1903 to 1904 were re-surveyed in 2015 using replicated historical gear and methods. Catch composition was contrasted between historical and modern periods using unconstrained ordination, permutational multivariate analysis of variance, permutational tests of the homogeneity of multivariate group dispersions and similarity percentage analyses. After 111 years, the re-survey revealed a drastically transformed demersal assemblage, with the period effect explaining nearly half of the variance among samples. Historical catches were dominated by kob (Argyrosomus spp.), panga (Pterogymnus laniarius) and east coast sole (Austroglossus pectoralis), jointly contributing 70–84% of the catch. The same taxa made up a minor component (1.5–5.5%) of modern assemblages. Instead, the re-survey catches consisted predominantly of gurnards (Chelidonichthys spp.), Cape horse mackerel (Trachurus capensis), spiny dogfish (Squalus spp.), shallow-water hake (Merluccius capensis), and white sea catfish (Galeichthys feliceps), with their summed contribution rising to 85% from the historical 3%. These results suggest that a century of trawling may have altered benthic habitats, indirectly contributing to changes in the fish community. Historical assemblages included a substantial proportion of taxa that associate with reef habitats, whereas the re-survey assemblages were characterized by species that inhabit unconsolidated sediments or both reef and non-reef habitats. The unique historical context and data, comparable gear and methods and long temporal period revealed striking baseline changes that may be overlooked in most fisheries. Reconstructing this important historical context improves our ability to assess, interpret and manage changing marine ecosystems.
Introduction
Effective policy advice on the management of biodiversity, fisheries and the ecosystems that support them requires information about the scale and drivers of past changes in the ocean (Schwerdtner Máñez et al., 2014; Engelhard et al., 2016). Without knowledge of previous change and baseline reference points, recent variability within a fishery or ecosystem may provide little context for interpretation (Thurstan et al., 2010; Moloney et al., 2013), which hinders informed decision making.
Anthropogenic impacts such as fishing and climate change have altered marine ecosystems (Jackson et al., 2001; Lotze et al., 2006; Brander, 2010; Cheung et al., 2013; Moloney et al., 2013). Most of our knowledge of these systems is based on research and monitoring initiated long after such impacts started altering them (Lotze and Worm, 2009). For example, some of the most intense trawl fishing pressures in South Africa occurred between the late 1950s and 1977, when South Africa declared its exclusive fishing zone (Griffiths et al., 2004; Sink et al., 2012b). Yet investigations into periods prior to modern time-series of research trawl surveys (1983 to present), commercial catches (1978 to present) and fishery observer data (1995 to present), are few and typically hampered by the availability of comparable data. As a result, knowledge of near pristine ecosystems and the reference points that describe them are broadly lacking, both in South Africa and across most of the globe.
Valuable insight has been gained from research into long-term assemblage changes in demersal catches. By comparing trawl surveys from 1909 and 1910 to the 1980s, Novaglio et al. (2020) showed that substantial population declines and change in fish community structure occurred during the early decades of commercial trawling in south-east Australia. Periods of warmer water have been associated with greater abundances of certain taxa, specifically smaller, faster-growing species (Genner et al., 2004, 2010; ter Hofstede and Rijnsdorp, 2011). Investigating demersal assemblage changes in the North Sea over three decades, Engelhard et al. (2011) concluded that both climatic changes and fishing impacts explained observed patterns, which included higher abundances of warm-water, smaller, lower-trophic-level taxa and declines of mostly larger, cooler-water, higher-trophic-level species. Fishing impacts are commonly blamed for decreased abundances observed in demersal populations or assemblages (Silvestre et al., 1986; Rijnsdorp et al., 1996; Jackson et al., 2001; Kongprom et al., 2003; Ferretti et al., 2013; Novaglio et al., 2020), as well as declines in larger species or larger sizes within a population (Rijnsdorp et al., 1996; Greenstreet et al., 1999; Rogers and Ellis, 2000; Genner et al., 2010). Decreased diversity and greater species dominance in trawl-caught assemblages have also been attributed to the effects of fishing (Greenstreet and Hall, 1996; Rijnsdorp et al., 1996; Greenstreet et al., 1999). Heath and Speirs (2012) investigated fishing impacts on the demersal community following the resumption of trawling after it had been banned for 73 years from the Firth of Clyde in Scotland. They documented substantial change in the demersal assemblage in the decades that followed, including reduced evenness, marked reductions in fish sizes and an increase in small-sized fish, notably whiting (Merlangius merlangus).
Development and Change in South African Demersal Communities
Pre-colonial exploitation of marine resources in South Africa has a long and important history (Parkington, 2001), but it was focused on intertidal reaches (Griffiths et al., 2004). Relative to most temperate shelf regions around Europe and North America, industrialized fishing was late to reach South African ecosystems, similar to the developments in south-east Australia (Novaglio et al., 2018). Commercial fishing of finfish began near Cape Town in the late 1700s and spread with the growth of colonial settlements along the coasts. Fish were netted from the shore, but greater volumes were taken by handline fishers from small boats that caught most of their fish close to ports (Lees, 1969). By 1895 the line fishery had spread east to Port Elizabeth, and reported impressive catches across a broad spectrum of coastal fishes (Department of Agriculture, 1897).
Following substantial catches made by the government research vessel, SS Pieter Faure, industrial fishing arrived, with the first steam trawler registered at Simon’s Town (Cape Town) in 1898 (Department of Agriculture, 1899). The inshore Agulhas Bank sole grounds were initially the most important trawl fishing grounds (Scott, 1949). The industry expanded with developing transport networks and markets. In 1922 there were 17 steam trawlers registered in the Cape Province (Lees, 1969). At this time, large hake resources (Merluccius capensis and M. paradoxus) were discovered in deeper waters on the west coast (Scott, 1949; Payne and Punt, 1995), after which their catches dominated regional trawl landings. Catches of hake have remained the most valuable and largest part of South Africa’s trawl catches to the present.
Growth of the trawl industry accelerated after the Second World War, with hake landings growing from 50,000 tons in 1950 to 160,000 tons by 1960 (Payne and Punt, 1995). Foreign fleets arrived in the region in the 1960s, rapidly escalating fishing effort (Payne and Punt, 1995; Durholtz et al., 2015). Hake landings reached a peak in the early 1970s (∼300,000 tons), after which they fell due to over-exploitation (Payne and Punt, 1995). In 1972 the International Commission for the Southeast Atlantic Fisheries was formed to regulate regional fishing pressures and in 1977 the South African government proclaimed its exclusive fishing zone that progressively removed international effort (Durholtz et al., 2015). Hake populations started to recover in the 1980s and their catches have subsequently remained relatively stable at ∼150,000 tons per year (Griffiths et al., 2004; DAFF, 2015). An overview of the development, gear and vessel technology, management strategies and fleet structure of South Africa’s trawl fishery are provided in Sink et al. (2012b) and Durholtz et al. (2015).
The line fishery also grew substantially during the 20th century and its efficiency greatly increased with technological progress. Although the inshore trawlers are confined to soft-sediments and line fishers exploit mostly reef habitat, conflict arose early on and there remains substantial overlap in fishing grounds and in catch composition. Line fishers first experienced catch restrictions as late as 1985, in the way of bag and size limits and a cap on commercial effort. These provisions were insufficient and likely too late. Griffiths (2000) showed substantial changes in the composition of line catches from similar reef sites sampled on the Agulhas Bank in 1931–1933 and 1987–1993. Other investigations of line catches during the 20th century similarly documented concerning depletion of species (Penney et al., 1989; Van der Elst, 1989; Attwood and Farquhar, 1999; Griffiths, 2000). Such evidence contributed toward the government’s decision to legislate emergency provisions in the management of line-fish resources in 2000, leading to 70% curtailment of commercial line fishing effort (Blamey et al., 2015). Confounding the effects of fishing regulations was the creation of several coastal marine protected areas (MPAs) between 1970 and 2008, some of which improved the status of line-caught species (Bennett and Attwood, 1991; Kerwath et al., 2013).
Investigations of trawl species composition changes in South Africa have focused predominantly on recent decades. On the west coast, Atkinson et al. (2011b) assessed spatial and temporal changes in the demersal fish composition from trawl surveys conducted between 1986 and 2009, while Kirkman et al. (2015) looked for regime shifts on the west coast of South Africa, Namibia, and Angola over a similar period. The Agulhas Bank demersal community appears to have increased in diversity and evenness over the period of 1986 to 2003 (Yemane et al., 2010), even though fish size indices decreased (Yemane et al., 2008) and abundance-biomass-comparison curves pointed to greater disturbance during the same period (Yemane et al., 2005).
Retrospective analysis of historical trawl survey data (prior to the 1980s) has received little attention in South Africa. Mussgnug (2013) investigated long-term changes in the Agulhas Bank trawl assemblage, by comparing a variety of datasets from historical (1898–1904; 1922–1948) and recent (1985–2010) trawl surveys and a recent scientific observer program (2003–2006). Despite the uncertainty of comparing among different gears and methods, his results reveal notable assemblage changes, showing that several taxonomic groups decreased markedly while others had increased. Mussgnug (2013) concluded that small, fast-growing generalists withstood expanding fishing pressure better than large, slow-growing, low-fecundity taxa.
Standardization of Fishing Gear and Repeat Surveys
The materials, construction and methods used in trawling operations influence the fishing performance, specifically the relative selectivity of fish that are caught due to their size, shape and behavior (Engås and Godø, 1986; Dealteris et al., 1989; Johnson et al., 2008). Researchers typically try to minimize fishing performance effects by standardizing survey gear and methods throughout a survey series. If that is not possible, estimation of selectivity factors, usually for each species, is a way of addressing the problem when comparing among gear types (Sparre and Venema, 1998). Studies contrasting trawl records from different periods generally have to grapple with this potential bias of varying (and unquantified) selectivity among gear technologies (Rijnsdorp et al., 1996; Greenstreet et al., 1999; Rogers and Ellis, 2000; Mussgnug, 2013; Novaglio et al., 2020), which limits certainty in those results. A few examples exist where researchers set out to repeat historical trawl surveys and thus had the opportunity to imitate trawl gear used previously.
On the south-east coast of England, McHugh et al. (2011) repeated trawl surveys in 2008–2009 at two sites that had been surveyed during 1913–1922. They used an otter trawl net that was the same size and had similar mesh to the historical gear. Their comparison documented notable changes in the assemblage, predominantly in elasmobranch species, which included dramatic declines and an apparent extirpation of angel shark (Squatina squatina).
Employing data from 1964 trawl surveys conducted before initiation of a prawn trawl fishery in the Gulf of Carpentaria in Australia, Harris and Poiner (1991) compared the fish assemblage with 1985/1986 repeat surveys that used the same fishing gear. They documented substantial changes in the fish assemblage. Of the 82 taxa analyzed, 18 had decreased in abundance and 12 had increased significantly. Trawling pressure was considered the likely cause of these changes although environmental variability due to river input and sediment changes also might have had an influence (Harris and Poiner, 1991).
In New South Wales, Australia, Andrew et al. (1997) and Graham et al. (2001) compare upper continental slope trawl survey catches from 1976/1977, a time when commercial trawling was expanding into those areas, with a repeat survey using the same vessel and similar gear 20 years later. Although they did not investigate the assemblage structure, their results showed that both target and non-target species decreased substantially between survey periods and the authors concluded that fishing pressure was likely responsible for such declines.
Unique Pre-exploitation Data From South Africa
The development of a South African trawl industry was prompted by impressive catch records from the government-led exploration of trawl grounds between 1897 and 1906 (Scott, 1949). Recent digitization of detailed survey records from those early research efforts provide an unprecedented view of the demersal ecosystem at a time when human pressures on those grounds are considered to have been negligible (Currie, 2017). As trawl catch records collected prior to significant exploitation pressures are rare globally (though see Silvestre et al., 1986; Harris and Poiner, 1991; Andrew et al., 1997; Klaer, 2001; Kongprom et al., 2003; Novaglio et al., 2020), our knowledge of demersal change stems predominantly from previously impacted systems. The early South African trawl survey data provided a valuable opportunity to investigate baselines and subsequent changes from a minimally disturbed temperate demersal ecosystem.
Despite their obvious value, quantitative contrasts between the historical records and more recent survey data are challenging due to the substantial changes in trawling technology over time (Rijnsdorp et al., 1996; Rogers and Ellis, 2000; Mussgnug, 2013). We addressed this challenge by replicating the trawl surveys conducted by the Pieter Faure survey vessel more than a century previously. The aim was to assess long-term changes in the assemblage composition of trawl-caught fish on the inshore Agulhas Bank. Survey sites were revisited in 2015 with reconstructed trawl gear and methods that imitated the fishing power of 1903/1904 surveys. Changes in the fish assemblage were investigated and key taxa driving such changes were considered. Besides detection and description of long-term change, this experiment provided a measure of current demersal fish assemblages relative to their near-pristine state. Results reveal the scale of change, reiterate the value of pre-disturbed baselines and provide informative context for management of demersal ecosystems.
Materials and Methods
Selection of Data and Re-survey Sites
Three sites were identified as suitable for repeat surveys, named here as Cape Infanta, Mossel Bay and Bird Island (west to east). They were chosen due to spatial aggregations of historical trawl samples within the modern commercial trawl footprint. Once sites were identified, trawl samples were selected for inclusion in analyses based on the following criteria: (1) they included a start and end time or trawl duration, of which the latter was limited to <200 min; (2) they commenced at least 30 min after sunrise and ended before sunset; (3) their start locations were positioned outside current MPAs or trawl exclusion zones; (4) they were not associated with comments suggesting problems or deviation from normal trawling practices; and (5) their survey dates were restricted within a 3-month period at each site. The location, selection grids and trawl samples are illustrated in Figure 1.
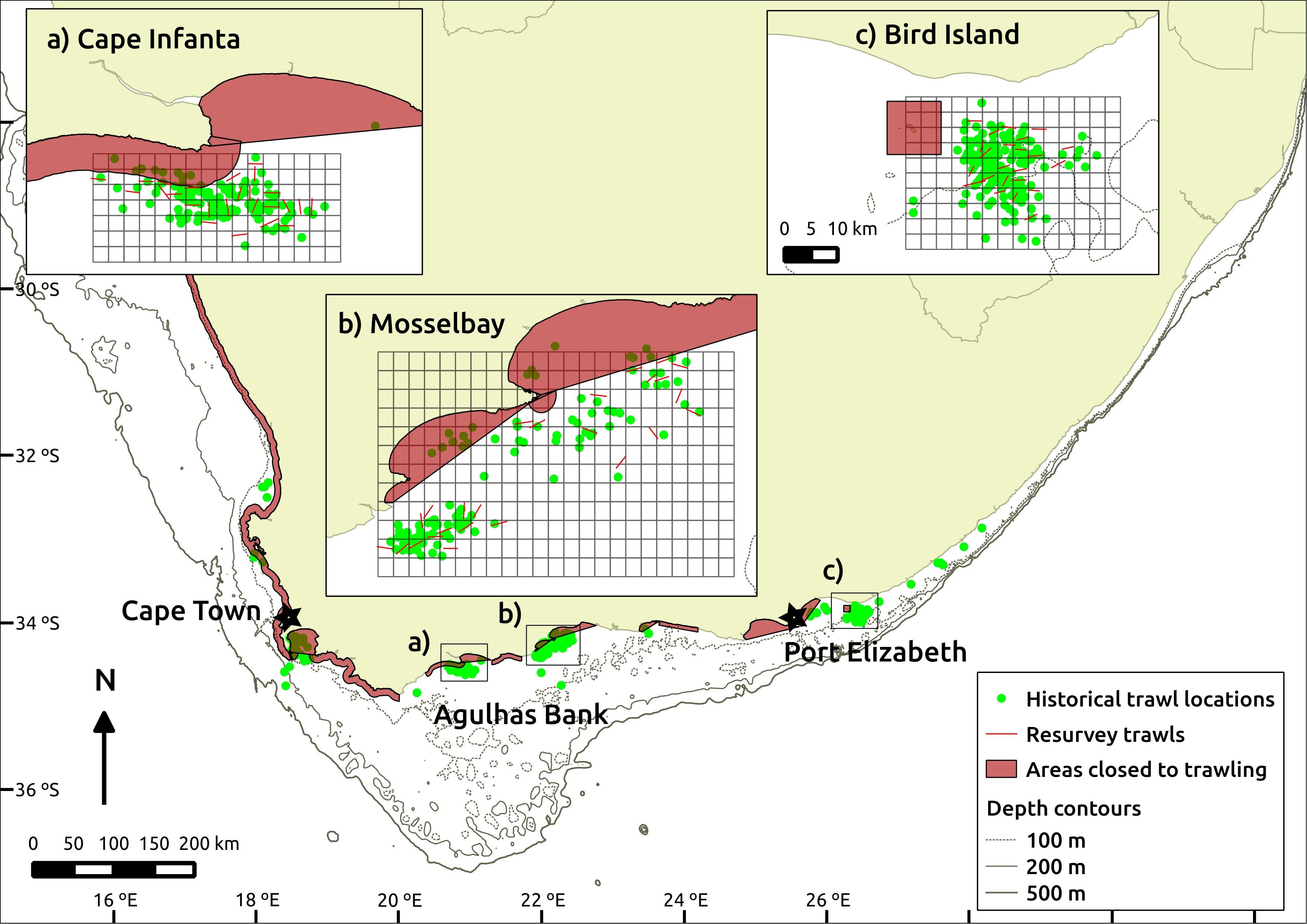
Figure 1. Map showing the location of historical trawl samples, together with the sampling grids and re-survey trawl locations at the three chosen re-survey sites (a–c). Scale used across the three inserts is consistent. Areas closed to trawling include designated trawl closures and legislated marine protected areas at the time of the fieldwork. Historical trawls represented by start position of trawl, whereas re-survey trawls are shown as a straight line connecting start- and end-positions.
Qualifying trawl samples were plotted in geographic information software (QGIS Development Team, 2015). A sampling grid was created, the width and height of each cell equal to the approximate length (1.3 nautical miles, ∼2.4 km) of a standard 30-min research trawl at a towing speed of 2.6 knots. The survey grid thus defined sampling units from which re-survey cells could be selected. Re-survey targets were chosen by random selection, without replacement, from those grid cells that contained the start location of at least one historical trawl.
Prior to fieldwork, the target sample sizes for repeat surveys were considered using univariate and multivariate approaches (Currie, 2017). To assess the impact of increasing sample sizes on multivariate precision, the pseudo multivariate dissimilarity-based standard error (MultSE) was calculated (Anderson and Santana-Garcon, 2015). Specifically, the ‘MSEgroup.d’ function (Anderson and Santana-Garcon, 2015) was applied to the historical survey data with site as a grouping factor and using a Bray–Curtis dissimilarity matrix based on square-root transformed catch data that had been standardized by their trawl distance (see below). Profiles of decreasing MultSE with increasing sample size were visually examined and indicated minimal precision increases beyond 20 samples per site (Supplementary Figure S1). A target sample size of 25 trawls per site was set. Unless specified differently, data preparation and analyses were conducted in the R programming environment (R Core Team, 2016).
Although recent decades of government-led research trawl surveys were not contrasted directly with historical catches (due to differences in gear technology), they were employed to assess the magnitude of seasonal or interannual variability. These recent (1986–2015) demersal trawl survey data were obtained from the Department of Agriculture, Forestry and Fisheries and are referred to as DAFF data hereafter. They were collected during annual or bi-annual stratified random surveys of the south coast continental shelf between 1986 and 2015. The sampling protocol for the DAFF data is detailed by Yemane et al. (2010) and is similar to that described below for the repeat surveys. The trawl gear used to collect these data changed in the mid-2000s, as detailed by Atkinson et al. (2011b). Both sets of gear consisted of a 55 m (180 ft) German otter trawl and are similar in many aspects, yet the fishing performance (and selectivity among taxa) may vary between the two. Therefore, analyses in this study treated the DAFF ‘old gear’ (1986–2003; 2006) and ‘new gear’ (2004–2005; 2007–2015) separately. DAFF survey samples were selected if they fell within the spatial grids described above, if they had a duration between 20 and 35 min, a towing speed of 3–3.5 knots and did not suffer net damage or other disruptions that may have influenced the catch.
Trawl Gear
A historical Granton trawl net and flat wooden trawl doors were constructed specifically to imitate those used on the Pieter Faure between 1897 and 1906. Details of the gear are provided in Currie et al. (2019a) and only a salient summary is provided here.
The trawl net was based on the plan and descriptions provided by Kyle (1903) and was made from Manila hemp and a mixed polyester-polyethylene alternative with similar properties. The headline consisted of a 28-mm Manila rope, 27.4 m long. The ground-rope consisted of a 20-mm fiber-core cable served with Manila rope to make up a total diameter of 96 mm. Mesh sizes varied from 150 mm stretched mesh in the forward parts of the net, to 100 mm in the trailing parts of the net. The trawl doors were 395-kg, flat, wooden doors with a heavy steel frame and shoe, which were shackled directly onto the net headline and foot-rope.
Repeat Fieldwork
In February, March and May 2015, repeat trawl surveys were conducted at Mossel Bay, Cape Infanta and Bird Island, accomplishing 25, 25, and 23 trawl samples respectively (Figure 1). These were compared with 42, 60, and 54 historical trawls. The repeat surveys were conducted from a commercial trawler, the MFV Leeukop, owned by the inshore division of Viking Fishing Company (Pty) Ltd. One of the last remaining side-trawlers in the local industry, the vessel was built in 1963, is 20.6 m in length, has a gross tonnage of 96.26 tons and an indicated engine shaft power of 189.41 kW.
Survey trawls commenced half an hour after sunrise and were completed prior to sunset. If conditions were overcast, those time limits were shifted 30 min later and/or earlier, respectively. The tow period, measured between settling of the net on the sea-floor (as observed from vessel speed) and the start of hauling, was approximately 30 min. The targeted towing speed was 2.5 knots. The length of trawl warps was set at ∼5.5 times the depth of water after initial attempts with shorter ratios failed to provide good ground contact of the doors and net.
After each trawl, the content of the net was sorted to species level where possible. Reference samples of unidentified fauna and voucher specimens were stored for examination ashore. The catch weight of each species was measured using calibrated marine scales (Marel M1100). A representative sub-sample was selected for enumeration when large catches were encountered. In such cases the number of fish caught was calculated as the mean unit weight from the sub-sample multiplied by the weight of that species’ catch.
A field identification error of Polysteganus coeruleopunctatus (blueskin) meant that this taxon was recorded with Pterogymnus laniarius (panga) at Bird Island. P. coeruleopunctatus normally occurs further east than Bird Island (Smith and Heemstra, 1986) and was not expected in the trawl catches. While their catches are not differentiated in analyses, the great majority are expected to have consisted of P. laniarius and are referred to as such hereafter.
To monitor depth and temperature, a compact CTD probe was attached to the headline of the trawl net, about 1.5 m behind the starboard trawl door. During the first survey (Mossel Bay) a RBR TDR-2050 device was employed and during the following surveys a RBR Concerto unit was used. In both cases, the device was set to record the pressure (depth) and temperature every second.
Data Preparation
This study was restricted to classes Actinopteri, Elasmobranchii, and Holocephali, which are broadly included in the term ‘fish’ hereafter. Historical records resolved 27 taxonomic groups that could inform analyses (Table 1), the majority of which were at species-level but included five genera, one family, three orders and one mixed group of remaining ‘other’ fish. Grouped taxa were assigned the lowest taxonomic classification that could be assumed with confidence. In comparison with subsequent datasets, catches of taxa were summed within the same groups and contrasts were restricted to taxa that were recorded throughout each of the periods.
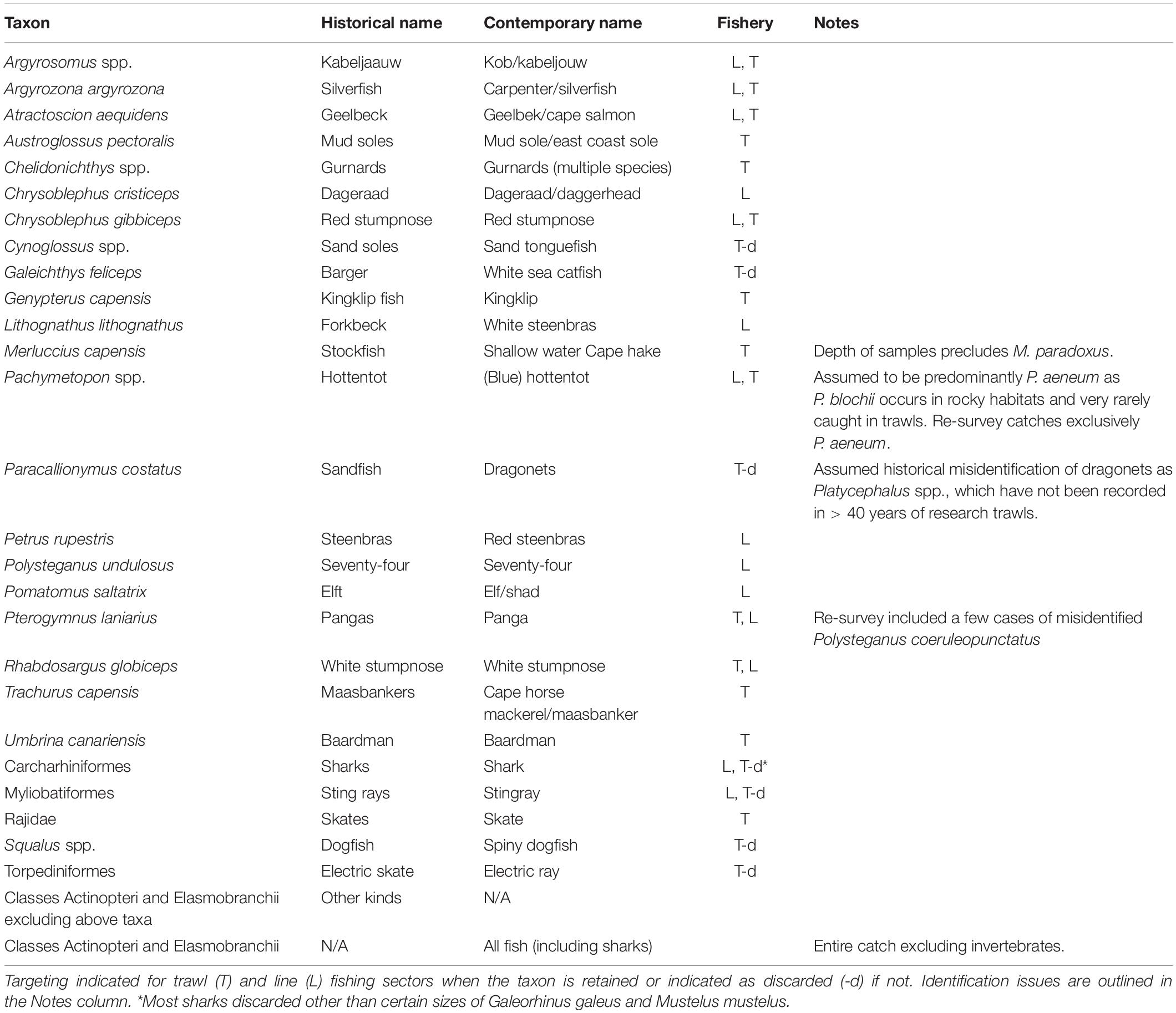
Table 1. The resolution of taxa used in analyses, including their historical and contemporary common names.
Data from the RBR CTD sensors were processed using RBR Ruskin software (version 1.8.14). Profiles of measured variables were exported as text files for further analysis.
The primary unit of measurement in the DAFF trawl survey data is mass (kg). The associated length-frequency records were employed to convert catches into abundances of fish. If a sub-sample had been taken for length measurements, a mean weight per fish was calculated from the sub-sample and scaled up to the catch weight. A few cases existed where no length-frequencies were recorded for a particular species’ catch. In such cases, the mean weight per fish was calculated for the relevant site, using remaining trawl samples that did contain weight and length data.
Comparison of Historical and Re-survey Catches
Analyses used catches standardized by the distance of the trawl tow, assuming a constant net width across the study:
where Cst is the standardized catch (count⋅nautical mile–1); C is the count for the specified taxon; d is the trawl distance; r is the duration of the haul (hours); and is the average trawl speed (knots).
The Pieter Faure trawl speed was estimated to be 1.35 m⋅s–1 (2.62 knots; Currie et al., 2019a). The re-survey trawl speed was calculated as the GPS-logged distance over the trawl duration. Trawl distance (duration∗speed) was considered a marginally more accurate measure of trawl effort than duration (at least for the re-surveys) and was therefore used in analyses.
Due to logistical constraints, the re-survey trawl durations (∼30 min) had to be shorter than those of the historical surveys (60–200 min). The trawl duration was recorded historically as ‘time trawl down,’ which was the difference between the ‘trawl hauled’ time and the ‘trawl shot’ time. For the re-survey trawls, ‘fishing duration’ (period that the net is settled on the sea-floor) was estimated using profiles from the temperature-depth sensors attached to the net. The latter method is a more accurate measure of the fishing effort, as it excludes the sinking time of the gear (mean = 3.5 min ± 0.3 SE across re-survey records). Inclusion of sinking duration would have minimal effect on the longer-duration historical samples but proportionally greater influence on the catch rates of shorter re-survey trawls (Battaglia et al., 2006), hence the more accurate ‘fishing duration’ was used for standardization of the re-survey samples.
Because the DAFF survey net is a different size, analyses including those survey data standardized catches by swept area (rather than distance):
where Csw is the swept-area standardized catch (count⋅nautical mile–2); a is the area of sea-floor swept by the trawl (nautical mile2); w is the width of the mouth of the trawl net (m).
Further analyses were based on a dissimilarity matrix of the community catch data. To down-weight the dominant influence of abundant taxa, dissimilarity matrices were based on square-root transformed standardized catches. Ordination of trawl samples was achieved by non-metric multidimensional scaling (NMDS) of a Bray–Curtis dissimilarity matrix. This was applied using the ‘metaMDS’ function from package ‘vegan’ (Oksanen et al., 2016).
The null hypothesis that there was no difference in the multivariate community composition between periods was assessed using a permutational multivariate analysis of variance using distance matrices (Anderson, 2001; hereafter PERMANOVA). A Bray–Curtis dissimilarity matrix was regressed against covariate depth and categorical variables site, period and a site-period interaction to account for potential depth and between-site influences while testing the period effect:
where D is the dissimilarity matrix, β1 represents the intercept, β2 the estimated coefficient for depth, β3 the estimated coefficient for the site effect, β4 the estimated coefficient for the period effect, β5 the estimated coefficient for the site-period interaction, and ∈ the residual error term.
The ‘adonis’ function in ‘vegan’ (Oksanen et al., 2016) was used to run the PERMANOVA models, with 9,999 permutations restricted to within-site levels. Because the period effect was of main interest, after having accounted for depth and site effects, we used sequential sums of squares and added the period effect last in the model structure. Maximum variability is attributed to the remaining main effects in this way, thereby providing a conservative estimate of the period effect.
To assess multivariate differences among pairs of surveys, a pairwise PERMANOVA analysis was also conducted among the levels of a combined period-site factor, including depth as a covariate. The Holm adjustment for multiple comparisons (Holm, 1979) was applied to avoid an inflated likelihood of obtaining a significant result.
PERMANOVA models are robust in the face of multivariate heterogeneity, but not if the experimental design is unbalanced (Anderson and Walsh, 2013). Therefore, the results from the unbalanced design were augmented with parameter ranges obtained from 1,000 randomly re-sampled (without replacement), balanced datasets containing 23 trawl samples per site per period.
To ascertain whether there may have been a change in multivariate heterogeneity between periods, a permutation test of the homogeneity of multivariate group dispersions was employed (PERMDISP2; Anderson, 2006). The same dissimilarity matrix was used as in the multivariate model and ordination above. Pairwise tests among period-site level combinations were assessed using 9,999 permutations and employing the Holm adjustment of p-values for multiple comparisons (Holm, 1979). The multivariate group dispersions were implemented using the ‘betadisper’ function in package ‘vegan’ (Oksanen et al., 2016).
A two-way (period∗site) similarity percentage analysis (SIMPER; Clarke, 1993) was used to investigate taxa that discriminate between periods across sites. Similarly, a pairwise SIMPER was used to identify the taxa discriminating periods for each site. These analyses were based on the same Bray–Curtis matrix described above and conducted in Primer-E (Clarke and Gorley, 2006).
To contrast the magnitude of variability between historical and re-survey samples with that among modern DAFF research trawl surveys, a multivariate measure of pseudo variance (MultV) was employed as per Anderson and Santana-Garcon (2015). Consistent with other analyses, MultV was computed from Bray–Curtis dissimilarity matrices derived from square-root transformed, swept-area standardized catches (count⋅nm–2). At each site, 500 pairs of historical and re-survey samples were randomly selected with replacement. DAFF samples were stratified by their temporal proximity to each other, so that the difference between pairs of seasonal (≥2 months and <12 months), interannual (≥12 months and <18 months) and multi-year (≥18 months but ≤60 months) samples could be calculated. As the sample sizes were relatively small for the DAFF pairs, all possible pairwise contrasts were included. The magnitude of MultV values were visually contrasted on scatterplots using their mean and bootstrapped non-parametric 95% confidence intervals.
As some uncertainties remained in the size of the historical net and the speed at which it was towed (Currie et al., 2019a), their potential effect on results was examined. This was accomplished by repeating the full set of analyses on swept-area standardized catches, using the extreme combinations of plausible bounds for headline length and trawl speed. This resulted in two additional sets of results, the one based on the assumption of a 26.8 m (88 ft) headline length and trawl speed of 1.10 m⋅s–1 (2.15 knots); the other on a 36.6 m (120 ft) headline and a speed of 1.61 m⋅s–1 (3.13 knots; Currie et al., 2019a).
In a similar manner, the impact on results of potential biases of depth were examined. Again the entire set of results was re-generated, excluding re-survey Bird Island samples with a depth ≥ 100 m. Differences between these additional results and the main set presented below were assessed.
Results
The targeted sites were successfully re-surveyed in 2015, revealing catches similar in abundance but substantially different in taxonomic composition to those of the historical surveys. The seasonality, time-of-day and trawl depths of samples were similar between periods, but trawl durations (and therefore distances) were greater in the historical surveys (Supplementary Figure S2) and their potential effect on results is discussed below.
Ordination of the standardized catches showed a clear separation of historical from re-survey trawl samples (Figure 2), predominantly along the primary x-axis. Site groupings were also discernible in the ordination, partially separating along the secondary y-axis. The large period effect was confirmed by the PERMANOVA results, accounting for 41–47% of the variance explained by the model (Table 2). The highly significant site effect explained 10–13% of the model variance. Together with their interaction, these two variables jointly explained 64–69% of the model variance. The depth of trawls also affected multivariate distances among trawls, but to a lesser extent (6–10% variance). The R2 estimates from the full (unbalanced) dataset fell within ranges of those estimated by the under-sampled (balanced) models, except for the depth effect, which was underestimated in the full design (Table 2). All terms combined, accounted for 72–76% of total model variance. Results of a pairwise PERMANOVA suggested that all period-site levels were significantly different from each other (p ≤ 0.01), except for the re-survey samples from Cape Infanta and Mossel Bay.
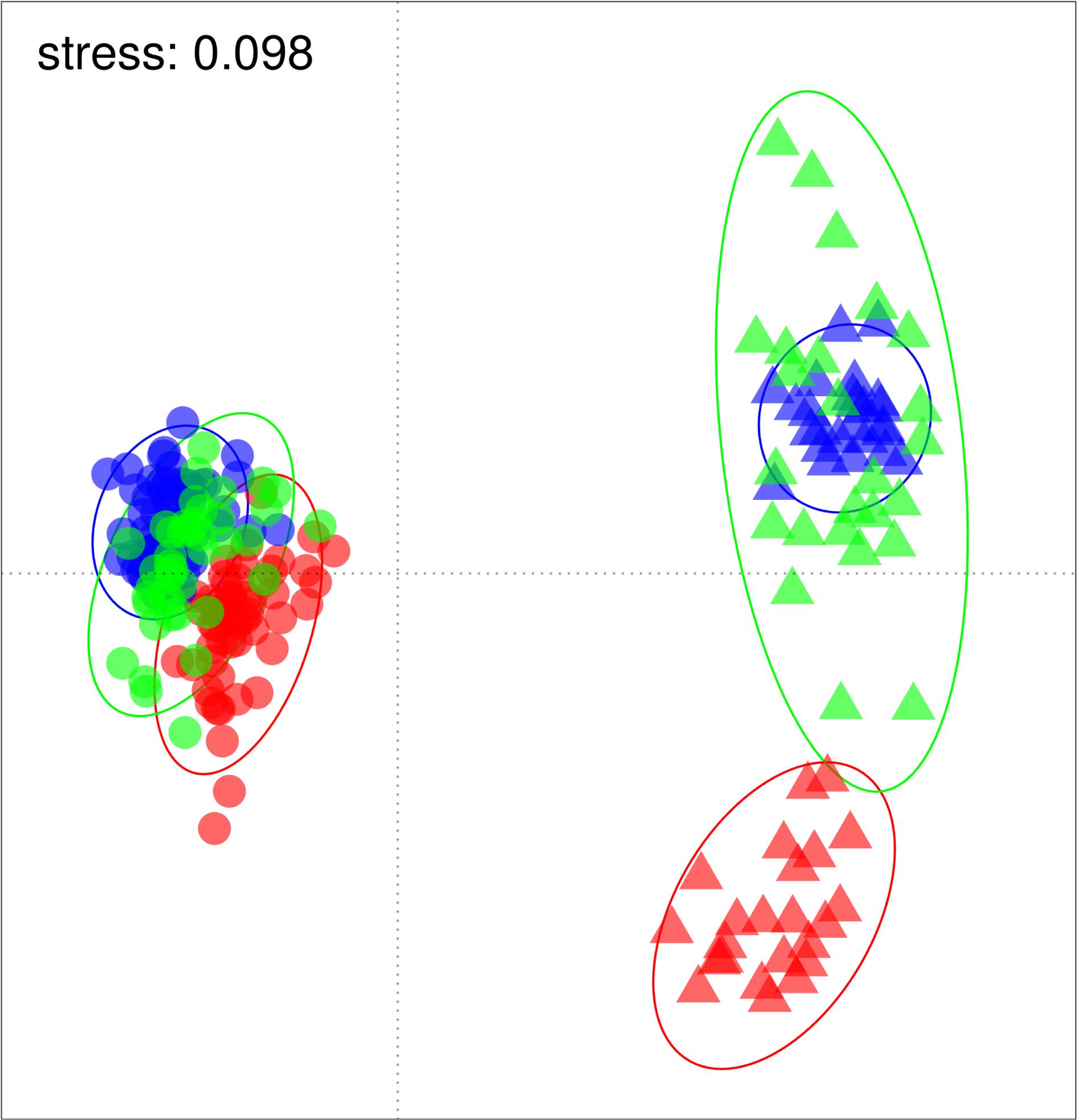
Figure 2. Non-metric multidimensional scaling (NMDS) plot based on Bray-Curtis dissimilarities of square-root transformed standardized catch. Historical and re-survey samples are indicated by circles and triangles respectively, while sites are separated by color (Cape Infanta blue, Mossel Bay green, Bird Island red). Bounding ellipses represent 95% probability contours estimated for each period-site level.
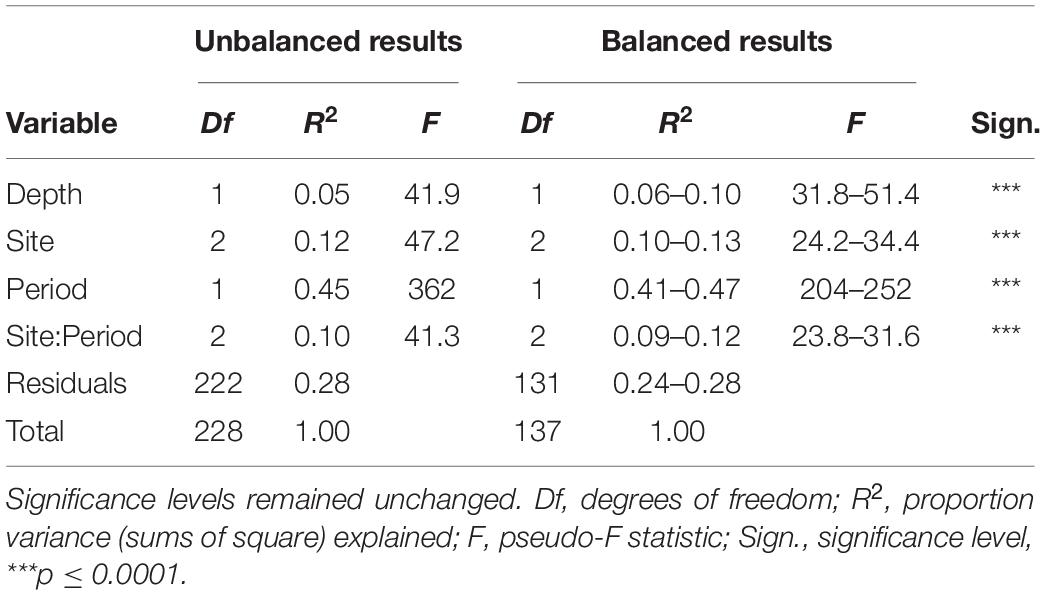
Table 2. PERMANOVA results shown from the full (unbalanced) dataset as well as ranges from 1,000 under-sampled, balanced datasets.
Shifting focus to the dispersion among samples, historical trawls tended to cluster closer together than the repeat surveys (Figure 2). The PERMDISP results confirmed that dispersion was different between periods (F = 101.3, p ≤ 0.0001). This difference appears to be caused mainly by divergence between the modern Bird Island samples and those of the other two sites, and greater variance among Mossel Bay re-survey samples (Figure 2). Dispersion was significantly different among period-site levels (F = 14.7, p ≤ 0.0001), but a pairwise test revealed that only Mossel Bay differed between historical and re-survey periods (Table 3). The modern survey at Mossel Bay was significantly more heterogeneous than all other period-site levels (Figure 2 and Table 3).
A large dissimilarity (81.7%) of historical and modern assemblages was supported by SIMPER analysis. Focusing on the distinguishing taxa that contributed 90% to between-period dissimilarity, six teleost taxa and Torpediniformes (electric rays) were more abundant historically, whereas four teleost taxa and Squalus spp. (spiny dogfish) were more numerous in the re-surveys (Figure 3). Argyrosomus spp. (kob), Pterogymnus laniarius (panga), Austroglossus pectoralis (east coast sole) and Chelidonichthys spp. (gurnards) contributed over 50% of the dissimilarity between periods and were relatively consistent in their distinguishing contribution (dissimilarity/SD > 1; Supplementary Table S1). The first three of those taxa dominated the composition of historical catches at each of the three sites, jointly contributing 82, 70, and 84% of Cape Infanta, Mossel Bay and Bird Island catches respectively. Contrasting the repeat surveys, Argyrosomus spp. were absent and the summed contribution of the further two species had dropped to between 1.5 and 5.5%. Other species that were nearly absent in repeat surveys included Argyrozona argyrozona (carpenter seabream; three individuals) and Rhabdosargus globiceps (white stumpnose; one individual), whereas they made up 8 and 4% of historical catches, respectively.
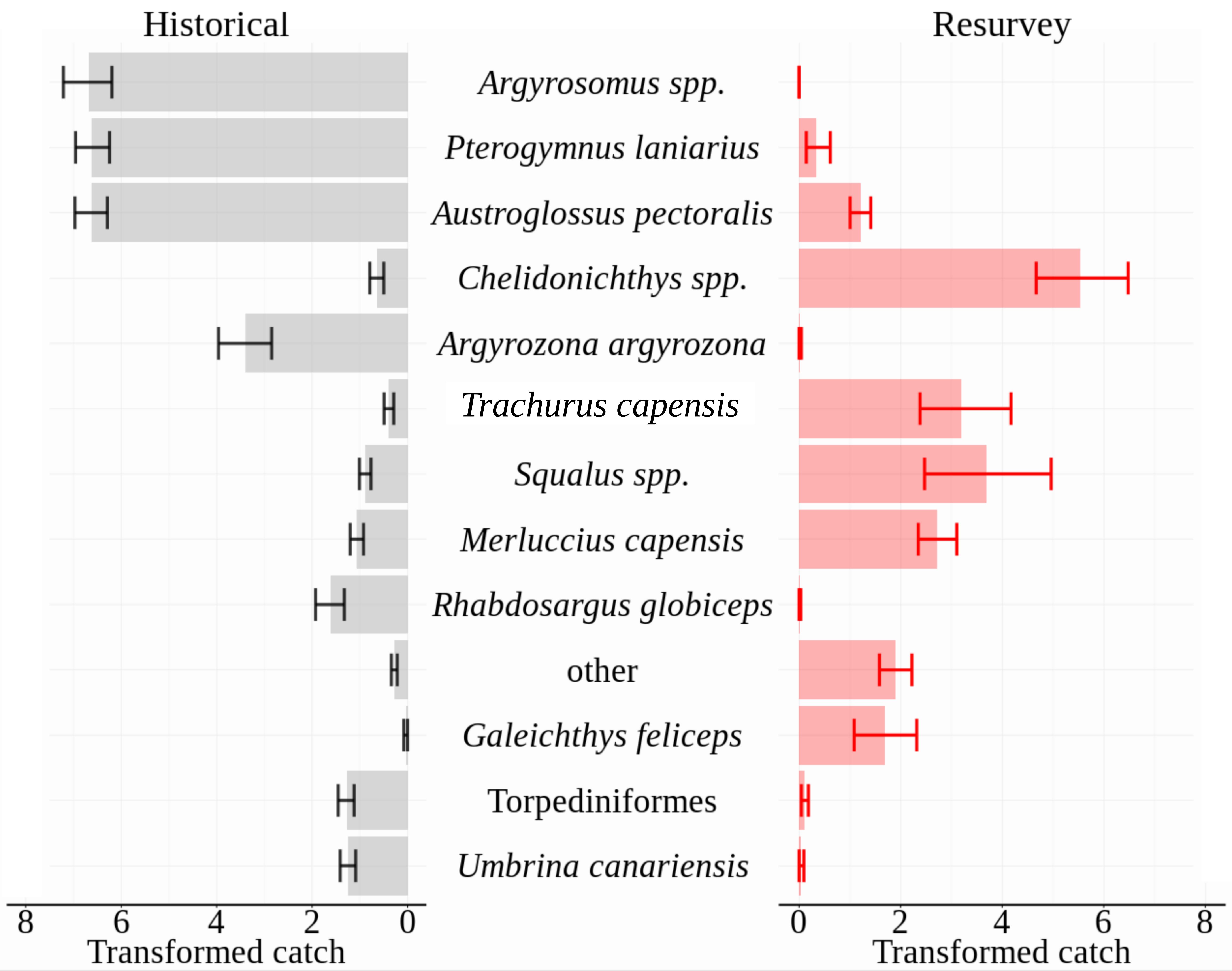
Figure 3. Mean abundances of distinguishing taxa that cumulatively contributed 90% of dissimilarity between periods, as identified by SIMPER analysis. Taxa are plotted in order of contribution to dissimilarity. Standardized abundances (count⋅nautical mile–1) were square-root transformed prior to analysis and plotting. SIMPER results for all taxa are tabled in the Supplementary Material. Error bars are non-parametric 95% confidence intervals.
Standardized abundances of Chelidonichthys spp., Trachurus capensis (Cape horse mackerel), Squalus spp., Merluccius capensis (shallow-water hake) and Galeichthys feliceps (white sea catfish) increased between periods, their joint contribution rising from 3% of historical catches to 85% of re-survey catches. Inspection of DAFF trawl survey data showed that by the late 1980s (1986–1990), the proportional contribution of the same suite of taxa matched more closely the re-survey than the historical assemblage structure: Argyrosomus spp., P. laniarius, and A. pectoralis contributed a cumulative average of 11% (by weight), whereas the five taxa that were most numerous in re-survey catches contributed 75% of the DAFF catch biomass. The long-term differences were substantially greater than shorter-term (seasonal, interannual and 5-year) variability characterized in the DAFF dataset (Supplementary Figure S3).
Due to the survey design, depths were expected to be similar between periods. However, at Bird Island, a bias toward deeper sampling depths was noted in the re-survey trawls (mean = 90 m) relative to historical surveys (mean = 78 m; Supplementary Figure S2). Repetition of analyses on a dataset that excluded re-survey Bird Island samples with a depth ≥ 100 m showed no material impact on results other than reducing statistical power.
Discussion
This research revealed dramatic change in demersal fish communities on South Africa’s Agulhas Bank over a 111-year period. The contrast between historical and re-survey records convincingly rejected a null hypothesis of no difference in catch composition between survey periods. The substantial temporal separation of assemblage composition was evident from an unconstrained ordination plot and supported by the period effect explaining almost half of the variance among trawl samples.
The striking transformation of the community assemblage was driven by previously dominant species that were absent or rare in the re-survey, as well as a suite of taxa that were numerically sparse in historical surveys but increased to make up the majority of 2015 assemblages (Figure 3). The most severe catch declines were on average detected in Argyrosomus spp., P. laniarius, A. pectoralis, A. argyrozona and, specifically at Mossel Bay, R. globiceps. The relatively consistent proportions across sites (roughly a quarter each) of Argyrosomus spp., P. laniarius, and A. pectoralis, suggests that these taxa may have historically dominated similar habitats in this region and are expected to have played a substantial role in the trophic ecosystem. Their large size and varied diet makes this especially relevant for Argyrosomus spp. (Smale and Bruton, 1985), which we presume exerted substantial predation pressure on the surrounding community.
The drastic decreases of the above taxa were accompanied by large increases of Chelidonichthys spp., Squalus spp., M. capensis, and T. capensis caught in repeat surveys. Whereas the joint historical contribution of these taxa was 5% at Bird Island and 2% at Cape Infanta and Mossel Bay, their numbers dominated the modern assemblages (73–87% of catches). Abundances of these taxa were less consistent across re-survey sites, with M. capensis more numerous at the two western sites and Squalus spp. exceptionally abundant at the eastern site of Bird Island. Inspection of the earliest years (1986–1990) from current government trawl survey records indicated that the above transition of dominant taxa had taken place before the start of the modern-era trawl surveys. Without the historical context, this substantial shift would be overlooked, highlighting the value of re-establishing these baselines.
Factors Affecting Assemblage Changes Between Periods
During the 20th century, fishing impacts are likely to have been a dominant driver of ecological change in marine communities in South Africa (Moloney et al., 2013) and many other regions (Jackson et al., 2001). Several of the taxa that declined in our study are fishery targets and we assume much of the change observed is associated with fishing pressures. However, some targeted taxa increased or remained similar in abundance, signaling complexity in responses. Additional pressures no doubt played a role, which could including climate change, changes in riverine inputs, pollution and hydrocarbon exploration and extraction (Moloney et al., 2013). Besides evolving fishery regulations, the closure of bays to trawling (which spanned 1928–1986) and proclamation of new MPAs (1970–2019) may have mitigated some of the impacts. Unraveling past effects of the various pressures on demersal fish assemblages will require substantial additional data, much of it not currently available. As they may indicate potential drivers of change, we instead considered factors that might explain why certain taxa had declined and others had increased or maintained numbers, following 111 years of mostly increasing pressures.
Life-history characteristics are frequently cited as determinants of the sensitivity of populations to fishing pressures, including fecundity, age at maturity, spawning strategies, growth rate, longevity and body size (Rogers and Ellis, 2000; Stevens et al., 2000; Graham et al., 2001; Genner et al., 2010; McHugh et al., 2011). Declines of elasmobranch taxa, for example, are often linked to their low fecundity, slow growth and/or old age at maturity (Stevens et al., 2000; Graham et al., 2001; Ferretti et al., 2010; McHugh et al., 2011). Yet in the current study, some elasmobranch catches appeared to increase between periods. While abundances of Torpediniformes declined across sites, the numbers of Squalus spp., Myliobatiformes and Carcharhiniformes increased overall (Supplementary Table S1). It is worth noting that those increases were predominantly due to large catches at only one site (Bird Island; Supplementary Table S2) and therefore may not be representative of the region. Rajidae appeared to decline at the two western sites but were also caught in far greater abundances in the Bird Island re-survey. The reasons for these high elasmobranch catches at Bird Island in this study are not clear.
Habitat preferences may provide insight into the potential drivers of observed assemblage changes. Multiple taxa that declined heavily are associated with reef habitats to varying degrees, including Argyrosomus spp. (Griffiths, 1997), P. laniarius (Booth and Buxton, 1997), A. argyrozona (Brouwer and Griffiths, 2005), and R. globiceps (Mann, 2013). On the contrary, the majority of species that increased between surveys tend to be those associated with soft substrates (e.g., Chelidonichthys spp. (Booth, 1997), Squalus spp. (Stehlik, 2007), G. feliceps (Tilney and Hecht, 1990) and Rajidae (Serra-Pereira et al., 2014; De Vos et al., 2015), or inhabit both soft and hard benthic substrates (M. capensis; Fairweather et al., 2006; Mann, 2013). Trawling has continued on these grounds for over a century and reef-like habitat or structure-forming benthic communities that may have been present historically are likely to have been degraded or removed (Engel and Kvitek, 1998; Auster and Langton, 1999; McConnaughey et al., 2000; Moran and Stephenson, 2000). Trawling can reduce habitat complexity in a number of ways (Auster and Langton, 1999), including the removal and damage of emergent and high-biomass fauna, which may become replaced by smaller-bodied and infaunal animals (Kaiser et al., 2000). Initial trawl passes may cause the greatest impact in sensitive epifaunal communities that include three-dimensional structure (Collie et al., 2000; Hiddink et al., 2006).
Sainsbury et al. (1993) document a case in which trawl-related habitat changes appeared to impact the fish assemblage: Two decades of escalating trawl pressure led to marked changes in the fish catch composition in north-western Australia. Examination of the composition changes showed that previously dominant taxa (Lutjanus spp. and Lethrinus spp.), which associated with dense epibenthic cover, had been replaced by two commercially less-valuable fish genera (Nemipterus spp. and Saurida spp.), which preferred areas of open sandy substrate (Sainsbury et al., 1993). The catches of epibenthic fauna (mainly sponges, alcyonarians and gorgonians) had declined over the same period.
It is conceivable that habitat-related assemblage changes similar to those documented in north-west Australia (Sainsbury et al., 1993) played out during the early years of trawling, both in South Africa and elsewhere. Trawl intensity has been linked to changes in epifaunal and infaunal communities on South Africa’s west coast (Atkinson et al., 2011a), but those changes were from an environment that had been extensively fished prior to its study. Knowledge of benthic invertebrate baselines and their subsequent changes are difficult to reconstruct (Callaway et al., 2007), as fishers and scientists tended to pay little attention to documenting catches of non-target invertebrate fauna until relatively recently. Our investigation lacked data on the epifaunal biogenic habitat that occurred historically and we cannot make predictions about how long its recovery might take if fishing impacts were to cease (though see e.g., Collie et al., 2000; Kaiser et al., 2006; Hiddink et al., 2019). Further work is needed to evaluate the impact of trawling on Agulhas Bank habitats, their recovery potential and link with observed fish assemblage changes.
Geographic and depth ranges are additional factors that might contribute toward the sensitivity of species to exploitation. Argyrosomus spp., P. laniarius, A. pectoralis, and R. globiceps are restricted to relatively shallow depths (≤120 m), generally close to the coastline. Sought-after fishing targets limited to shallower coastal areas and in reach of the majority of fishers and fishing gears will inherently suffer greater exposure to fishing pressures. Most taxa that increased (Chelidonichthys spp., Squalus spp., T. capensis, M. capensis) have a substantially wider range in terms of distance from the coastline and depth. Their greater distribution range may enable larger populations, but such populations are also more likely to have maintained refuges that are less accessible or uneconomical for fishers to exploit.
Increased Variability and Spatial Differences in Catch
The variability (dispersion) of Mossel Bay samples was inflated compared to all other period-site combinations (Figure 2). One explanation might be an impacted demersal community due to persistent anthropogenic pressures: Caswell and Cohen (1991) predicted that disturbance would increase heterogeneity within a community and Warwick and Clarke (1993) demonstrated that increased variability was associated with stress or degradation across four examples of widely varying marine communities. Of the three survey sites, Mossel Bay appears to be exposed to the highest level of anthropogenic pressures (Sink et al., 2012a), which include fishing effort and its proximity to an industrialized town and harbor servicing offshore hydrocarbon extraction.
Summed across taxa, catches were historically lowest at Bird Island and increased to highest levels at Cape Infanta. This contrasts with the re-survey data, which showed Bird Island catches to be larger than at the two western sites, due to considerable abundances of Chelidonichthys spp., G. feliceps and a host of elasmobranchs (Squalus spp., Rajidae, Myliobatiformes and Carcharhiniformes). Inspection of the last decade (2006–2015) of government trawl survey data suggested that some of these catch contributions were unusual (Currie, 2017), but that catches of Chelidonichthys spp., Squalus spp., Myliobatiformes and all taxa combined have recently been higher at Bird Island than at the other two sites.
A measure of multivariate variability between historical and re-survey samples suggests that demersal communities may have transformed less at Bird Island than at Cape Infanta and Mossel Bay. Multiple reasons might contribute to a less impacted Bird Island site. Relative to the other sites, trawl intensity has been lower at Bird Island in recent decades (Currie et al., 2019b). In addition, the site is adjacent to the Bird Island MPA and in relative proximity to the eastern-most boundary of commercial trawling activities during the last four decades (Booth and Hecht, 1998). Its demersal communities are, therefore, likely under less direct fishing pressure and may benefit from spillover from untrawled areas nearby. The Cape Infanta site is also adjacent to an MPA, which has benefited surf-zone fish species (Bennett and Attwood, 1991). MPAs can offer protection against multiple pressures and safeguard resilient assemblages (Bennett and Attwood, 1991; Dayton et al., 2000; Micheli et al., 2012; Mellin et al., 2016), together with their critical habitats (Lindholm et al., 2001; Kritzer et al., 2016). The benefits of such MPAs to demersal trawl communities have not been tested in South Africa. Neither has the effect of closing bays to trawling, some of which were implemented as early as 1928 (Scott, 1949). Contrasting habitats and demersal communities inside and outside these protected areas could provide valuable insight on trawling-induced habitat impacts, their recovery and the efficacy of protection.
Pre-disturbance Baselines
Few other studies have had access to demersal survey records prior to, or early in the development of a trawl fishery. Of those that did (Silvestre et al., 1986; Harris and Poiner, 1991; Andrew et al., 1997; Graham et al., 2001; Klaer, 2001; Kongprom et al., 2003; Mussgnug, 2013; Novaglio et al., 2020), most did not assess assemblage composition changes in the same way that we did. Nonetheless, some commonalities with their results were identified. Examining commercial catch rates between 1918 and 1957, Klaer (2001) documented strong declines in the south-east Australia trawl fishery, despite substantial technological improvements during that time. Marked changes in the composition of catches included the virtual disappearance of two species that had been abundant during early years of the fishery. Before the same fishery expanded into deeper waters, trawl surveys were conducted on the upper continental slope in 1976/1977. Twenty years later, when a repeat survey was conducted, the previously dominant dogsharks (Centrophorus spp.) and redfish (Centroberyx affinis) had declined to <1% and <5% of initial catch rates, respectively (Andrew et al., 1997). Comparing south-east Australian trawl surveys from the 1980s with those conducted prior to commercial trawling in 1909–1910, Novaglio et al. (2020) documented substantial (83–99%) declines for several demersal fish families and notable shifts in catch composition, which they attributed predominantly to fishing. Unlike our study, they found a marked catch rate decline of 77% for the entire assemblage. On the northern coast of Australia, Harris and Poiner (1991) showed that two decades after the development of prawn trawling in the Gulf of Carpentaria, the combined catch rate of all fish had declined to less than half of initial values. Their results revealed substantial changes in the fish assemblage within 21 years of the fishery, including collapse of a previously dominant taxon (Paramonacanthus spp.).
Mussgnug (2013) included the same historical data and investigated comparable geographic areas to those of the current study, but with a focus on chondrichthyan taxa. He showed similar notable differences between historical (1898–1933) and recent (1985–2010) assemblages, including decreased catch contributions of P. laniarius, A. inodorus, A. pectoralis, A. argyrozona, Umbrina canariensis and R. globiceps. He also reported increases in catch contributions for T. capensis, Chelidonichthys spp. and M. capensis. The main discordance between the two studies pertains to certain elasmobranch taxa: Whereas Mussgnug (2013) emphasized decreases of all elasmobranch taxa, multiple elasmobranch groups increased between periods in our results (Supplementary Table S1). This may partly be explained by the unusually high catches of elasmobranch taxa encountered at Bird Island. A benefit of current results over those of Mussgnug (2013), is that differences in trawl gear and trawl speeds were explicitly examined and accounted for, reducing uncertainty and strengthening confidence in results.
In the North Sea, where commercial fishing (including trawling) was extensive prior to early trawl surveys, demersal assemblage changes in the 20th century appear to have been less severe. McHugh et al. (2011) investigated long-term changes in the demersal fish community of the western English Channel. Although multivariate differences between 1913–1922 and 2008–2009 did separate statistically, their ordination did not show strikingly disparate periods (as seen in Figure 2). Differences were driven predominantly by alterations in the elasmobranch assemblage, which was interpreted as evidence of the loss of larger, slow-growing taxa due to fishing pressures (McHugh et al., 2011). Other studies showed relatively subtle abundance changes of mostly less common demersal fish species in the North Sea (Greenstreet and Hall, 1996; Greenstreet et al., 1999; Rogers and Ellis, 2000). To illustrate, Greenstreet et al. (1999) noted that the same five dominant species contributed over 50% of similarity across all four periods they examined between 1925 and 1996.
It appears that the few studies with access to records from the earliest stages of a trawl fishery demonstrate more drastic assemblage changes than those based on baseline data collected many years after the development of fishing impacts. This implies that the magnitude of change measured in long-term comparisons depends largely on the state of communities first sampled. If so, it illustrates the erosive effect of shifting baselines (Pauly, 1995) and the importance of documenting pre-disturbed reference points to counter them. The majority of research into long-term demersal assemblage changes has been based in Northern Hemisphere regions, where decades, if not centuries of trawling preceded the earliest survey records (e.g., Greenstreet et al., 1999; Genner et al., 2010; e.g., Greenstreet and Hall, 1996; Rijnsdorp et al., 1996; ter Hofstede and Rijnsdorp, 2011; McHugh et al., 2011). Such studies provide useful insight on change and previous ecological states, yet the historical systems they document had suffered substantial impacts and do not represent near-pristine ecosystems (Jackson et al., 2001; Thurstan et al., 2010; McHugh et al., 2011). This study, however, had access to trawl survey data from almost pristine demersal communities. It contributes valuable knowledge of the baseline fish assemblages on the inshore Agulhas Bank and how these changed during the intervening century. Such information is pertinent to species and ecosystem assessments, could help estimate the productivity potential of undisturbed ecosystems and provide benchmarks against which change or the efficacy of management strategies can be evaluated. Estimates of unfished species abundances provide useful diagnostics and informative priors to assess or constrain fishery stock assessment models (Hilborn and Walters, 2001) and inform the choice of baseline conditions used in ecological models (e.g., Mackinson, 2001; Watermeyer et al., 2008).
Examination of Assumptions
Factors that might bias comparisons between historical and re-survey trawl catches were accounted for as far as possible. Season, time of day, geographical locations and replication of gear were standardized. Nonetheless, contrasts of the historical and re-survey catches required certain assumptions, which evoked their examination. These included that (1) the comparisons were not biased by remaining uncertainty in the historical gear dimensions and trawling speeds; (2) differences in trawl durations did not skew catch rates; (3) a depth bias at Bird Island did not impact results; (4) shorter-term interannual variability could not explain the changes observed; and (5) the taxonomic resolution imposed by historical records did not confound results or inferences drawn. These assumptions are unpacked in detail in the Supplementary Material. In summary, none of these factors appeared likely to have caused or substantially biased the observed differences between periods: (1) The effect on standardized catches that might result from uncertainties of gear size and trawling speed had negligible effect on results and interpretations reported; (2) examination of the literature suggested that a trawl duration impact would likely be marginal; (3) omitting historical Bird Island stations that caused a slight depth bias did not cause material differences in results; (4) seasonal, interannual and multi-year variability in trawl surveys from recent decades (expected to include the effects of environmental variability at these time scales) could not account for the magnitude of differences measured between periods; (5) the reduced taxonomic resolution recorded in the historical surveys would likely act to reduce (hide) rather than magnify differences observed between periods.
Conclusion
This quantitative comparison of historical and repeat trawl survey data provided evidence of dramatic long-term change in the demersal fish assemblages of South Africa’s inshore Agulhas Bank. Although historical data have previously indicated severe declines of line-caught species (Griffiths, 2000), our results highlight the scale of ecosystem change and the remarkable historic abundance of three species that previously dominated these demersal habitats. With this new information, scientists and managers are better equipped to understand change and consider historical reference points in developing strategies to manage and rebuild stocks.
Although we did not anticipate it, the observed fish assemblage changes suggest altered benthic habitats, most likely caused by the cumulative demersal trawling impacts at the studied sites. If substantial trawling pre-dates baseline data collection, such potential habitat impacts are likely to be missed in similar research globally. If trawling has altered the sediment structure (Auster et al., 1996), the benthic habitats, together with invertebrate and fish communities dependent on them, may fail to recover to their previous states even if trawling and other pressures were to be removed.
As noted, additional human impacts, including other fisheries, climate change, pollution and river flow reduction may have contributed to the state of demersal assemblages investigated here. Improved management of these communities may require addressing impacts from multiple sources. The recently declared Agulhas Bank, Agulhas Muds and Addo Elephant National Park MPAs are expected to contribute to the protection and recovery of demersal communities in the studied region. Monitoring and dedicated research efforts are required to track current and future states of those demersal ecosystems.
The severity of the assemblage reorganization, relative to similar investigations elsewhere, is interpreted to be due to the near-pristine state of the historical community surveyed. The majority of previous studies may miss the initial, and perhaps most dramatic, shifts in assemblage composition if they lack baseline data collected prior to substantial exploitation. Researchers and managers need to be cognizant of such missing baselines when interpreting change, estimating reference points in fished ecosystems, and in advancing plans for resource management and protection of seabed habitats.
Data Availability Statement
The historical data are available from SAEON (https://doi.org/10.15493/HCDB-8A05). The repeat survey data are available upon request from JC. The DAFF survey data can be requested from the director of Resources Research in the Fisheries Branch of the Department of Fisheries, Forestry and Environment in South Africa.
Ethics Statement
The animal study was reviewed and approved by Faculty of Science Animal Ethics Committee (University of Cape Town).
Author Contributions
This manuscript was based on the Ph.D. work of JC. CA, KS, and LA co-supervized the work. JC conducted analyses and wrote the draft. LA, KS, and CA contributed text, comments and editorial input. All authors made a substantial, direct and intellectual contribution to the work, and approved it for publication.
Funding
Funding support is acknowledged from the Pew Charitable Trusts, Claude Leon Foundation, Mia J Tegner Historical Marine Ecology Grant, National Research Foundation, including its Professional Development Programme, SAEON (South African Environmental Observation Network), University of Cape Town, African Coelacanth Ecosystem Programme (ACEP) Deep Secrets Project, British Ecological Society Ecologists in Africa Grant (no. 5139/6181), Explorer’s Club Exploration Fund Grant, and the Climate Change Division at SANBI (South African National Biodiversity Institute).
Conflict of Interest
The authors declare that the research was conducted in the absence of any commercial or financial relationships that could be construed as a potential conflict of interest.
Acknowledgments
Fieldwork assistance from Stewart Norman, Stephen Lamberth, and Denham Parker is gratefully acknowledged, as well as the guiding expertise from Noel Less. The Fisheries Management branch at the Department of Fisheries, Forestry and Environment are acknowledged for allowing the use of their data. This work benefited from JC’s involvement in the ICES Working Group on the History of Fish and Fisheries.
Supplementary Material
The Supplementary Material for this article can be found online at: https://www.frontiersin.org/articles/10.3389/fmars.2020.00355/full#supplementary-material
References
Anderson, M. J. (2001). A new method for non-parametric multivariate analysis of variance. Aust. Ecol. 26, 32–46. doi: 10.1111/j.1442-9993.2001.01070.pp.x
Anderson, M. J. (2006). Distance-based tests for homogeneity of multivariate dispersions. Biometrics 62, 245–253. doi: 10.1111/j.1541-0420.2005.00440.x
Anderson, M. J., and Santana-Garcon, J. (2015). Measures of precision for dissimilarity-based multivariate analysis of ecological communities. Ecol. Lett. 18, 66–73. doi: 10.1111/ele.12385
Anderson, M. J., and Walsh, D. C. I. (2013). PERMANOVA, ANOSIM, and the Mantel test in the face of heterogeneous dispersions: what null hypothesis are you testing? Ecol. Monogr. 83, 557–574. doi: 10.1890/12-2010.1
Andrew, N. L., Graham, K. J., Hodgson, K. E., and Gordon, G. N. G. (1997). Changes After Twenty Years in Relative Abundance and Size Composition of Commercial Fishes Caught During Fishery Independent Surveys on SEF Trawl Grounds. Cronulla, NSW: Fisheries Research and Development Corporation, NSW Fisheries.
Atkinson, L. J., Field, J. G., and Hutchings, L. (2011a). Effects of demersal trawling along the west coast of southern Africa: multivariate analysis of benthic assemblages. Mar. Ecol. Prog. Ser. 430, 241–255. doi: 10.3354/meps08956
Atkinson, L. J., Leslie, R. W., Field, J. G., and Jarre, A. (2011b). Changes in demersal fish assemblages on the west coast of South Africa, 1986–2009. Afr. J. Mar. Sci. 33, 157–170.
Attwood, C. G., and Farquhar, M. (1999). Collapse of linefish stocks between Cape Hangklip and Walker Bay. South Africa. South Afr. J. Mar. Sci. 21, 415–432. doi: 10.2989/025776199784126150
Auster, P. J., and Langton, R. W. (1999). “The effects of fishing on fish habitat,” in Fish Habitat: Essential Fish Habitat and Restoration, ed. L. Benaka, (Silver Spring, MA: NOAA), 150–187.
Auster, P. J., Malatesta, R. J., Langton, R. W., Watting, L., Valentine, P. C., Donaldson, C. L. S., et al. (1996). The impacts of mobile fishing gear on seafloor habitats in the gulf of Maine (Northwest Atlantic): implications for conservation of fish populations. Rev. Fish. Sci. 4, 185–202. doi: 10.1080/10641269609388584
Battaglia, A., Trenkel, V. M., and Rochet, M.-J. (2006). Estimating end effects in trawl catches. ICES J. Mar. Sci. 63, 956–959. doi: 10.1016/j.icesjms.2006.03.002
Bennett, B. A., and Attwood, C. G. (1991). Evidence for recovery of a surf-zone fish assemblage following the establishment of a marine reserve on the southern coast of South Africa. Mar. Ecol. Prog. Ser. 75, 173–181.
Blamey, L. K., Shannon, L. J., Bolton, J. J., Crawford, R. J. M., Dufois, F., Evers-King, H., et al. (2015). Ecosystem change in the southern Benguela and the underlying processes. J. Mar. Syst. 144, 9–29. doi: 10.1016/j.jmarsys.2014.11.006
Booth, A. J. (1997). On the life history of the lesser gurnard (Scorpaeniformes: Triglidae) inhabiting the Agulhas Bank. South Africa. J. Fish Biol. 51, 1155–1173. doi: 10.1111/j.1095-8649.1997.tb01133.x
Booth, A. J., and Buxton, C. D. (1997). The biology of the panga, Pterogymnus laniarius (Teleostei: Sparidae), on the Agulhas Bank, South Africa. Environ. Biol. Fishes 49, 207–226. doi: 10.1023/A:1007362700687
Booth, A. J., and Hecht, T. (1998). Changes in the Eastern Cape demersal inshore trawl fishery between 1967 and 1995. South Afr. J. Mar. Sci. 19, 341–353. doi: 10.2989/025776198784126971
Brander, K. M. (2010). Impacts of climate change on fisheries. J. Mar. Syst. 79, 389–402. doi: 10.1016/j.jmarsys.2008.12.015
Brouwer, S. L., and Griffiths, M. H. (2005). Stock separation and life history of Argyrozona argyrozona (Pisces: Sparidae) on the South African east coast. Afr. J. Mar. Sci. 27, 585–595. doi: 10.2989/18142320509504119
Callaway, R., Engelhard, G. H., Dann, J., Cotter, J., and Rumohr, H. (2007). A century of North Sea epibenthos and trawling: comparison between 1902–1912, 1982–1985 and 2000. Mar. Ecol. Prog. Ser. 346, 27–43.
Caswell, H., and Cohen, J. E. (1991). “Communities in patchy environments: a model of disturbance, competition, and heterogeneity,” in Ecological Heterogeneity Ecological Studies, eds J. Kolasa, and S. T. A. Pickett, (New York, NY: Springer), 97–122. doi: 10.1007/978-1-4612-3062-5_6
Cheung, W. W. L., Watson, R., and Pauly, D. (2013). Signature of ocean warming in global fisheries catch. Nature 497, 365–368. doi: 10.1038/nature12156
Clarke, K. R. (1993). Non-parametric multivariate analyses of changes in community structure. Aust. J. Ecol. 18, 117–143. doi: 10.1111/j.1442-9993.1993.tb00438.x
Collie, J. S., Hall, S. J., Kaiser, M. J., and Poiner, I. R. (2000). A quantitative analysis of fishing impacts on shelf-sea benthos. J. Anim. Ecol. 69, 785–798. doi: 10.1046/j.1365-2656.2000.00434.x
Currie, J. C. (2017). Historical Baselines and A Century of Change in the Demersal Fish Assemblages on South Africa’s Agulhas Bank. Available at: https://open.uct.ac.za/handle/11427/27385 (accessed May 29, 2017).
Currie, J. C., Sink, K. J., Attwood, C. G., Atkinson, L. J., and Engelhard, G. H. (2019a). Reconstructing the past: design and function of Granton otter trawl gear at the turn of the twentieth century, as used in South Africa’s first trawl surveys (1897–1904). Marit. Stud. 18, 1–16. doi: 10.1007/s40152-018-0095-97
Currie, J. C., Thorson, J. T., Sink, K. J., Atkinson, L. J., Fairweather, T. P., and Winker, H. (2019b). A novel approach to assess distribution trends from fisheries survey data. Fish. Res. 214, 98–109. doi: 10.1016/j.fishres.2019.02.004
DAFF (2015). Status of the South African Marine Fishery Resources 2014. Cape Town: Department of Agriculture, Forestry and Fisheries.
Dayton, P. K., Sala, E., Tegner, M. J., and Thrush, S. (2000). Marine reserves: parks, baselines, and fishery enhancement. Bull. Mar. Sci. 66, 617–634.
De Vos, L., Watson, R. G. A., Götz, A., and Attwood, C. G. (2015). Baited remote underwater video system (BRUVs) survey of chondrichthyan diversity in False Bay. South Africa. Afr. J. Mar. Sci. 37, 209–218.
Dealteris, J. T., Recksiek, C. W., Fahfouhi, A., and Liuxiong, X. (1989). Comparison of the performance of two bottom-sampling trawls. Trans. Am. Fish. Soc. 118, 119–130. doi: 10.1577/1548-86591989118(0119:COTPOT(2.3.CO;2
Department of Agriculture (1897). Report of the Marine Biologist for the Year 1896. Cape Town: Cape of Good Hope.
Department of Agriculture (1899). Report of the Marine Biologist for the Year 1898. Cape Town: Cape of Good Hope.
Durholtz, M. D., Singh, L., Fairweather, T. P., Leslie, R. W., Lingen, C. D., Bross, C. A. R., et al. (2015). “Fisheries, ecology and markets of South African hake,” in Hakes: Biology and Exploitation, ed. H. Arancibia, (Chichester: John Wiley & Sons, Ltd), 38–69. doi: 10.1002/9781118568262.ch2
Engås, A., and Godø, O. R. (1986). Influence of trawl geometry and vertical distribution of fish on sampling with bottom trawl. J. Northwest Atl. Fish. Sci. 7, 35–42.
Engel, J., and Kvitek, R. (1998). Effects of otter trawling on a benthic community in monterey bay national marine sanctuary. Conserv. Biol. 12, 1204–1214. doi: 10.1046/j.1523-1739.1998.0120061204.x
Engelhard, G. H., Ellis, J. R., Payne, M. R., ter Hofstede, R., and Pinnegar, J. K. (2011). Ecotypes as a concept for exploring responses to climate change in fish assemblages. ICES J. Mar. Sci. 68, 580–591. doi: 10.1093/icesjms/fsq183
Engelhard, G. H., Thurstan, R. H., MacKenzie, B. R., Alleway, H. K., Bannister, R. C. A., Cardinale, M., et al. (2016). ICES meets marine historical ecology: placing the history of fish and fisheries in current policy context. ICES J. Mar. Sci. 73, doi: 10.1093/icesjms/fsv219
Fairweather, T., Booth, A., Sauer, W., and Leslie, R. (2006). Spatial description of hake-directed fishing activity off the west coast of South Africa. Afr. J. Mar. Sci. 28, 13–24. doi: 10.2989/18142320609504129
Ferretti, F., Osio, G. C., Jenkins, C. J., Rosenberg, A. A., and Lotze, H. K. (2013). Long-term change in a meso-predator community in response to prolonged and heterogeneous human impact. Sci. Rep. 3:1057. doi: 10.1038/srep01057
Ferretti, F., Worm, B., Britten, G. L., Heithaus, M. R., and Lotze, H. K. (2010). Patterns and ecosystem consequences of shark declines in the ocean. Ecol. Lett. 13, 1055–1071. doi: 10.1111/j.1461-0248.2010.01489.x
Genner, M. J., Sims, D. W., Southward, A. J., Budd, G. C., Masterson, P., Mchugh, M., et al. (2010). Body size-dependent responses of a marine fish assemblage to climate change and fishing over a century-long scale. Glob. Change Biol. 16, 517–527. doi: 10.1111/j.1365-2486.2009.02027.x
Genner, M. J., Sims, D. W., Wearmouth, V. J., Southall, E. J., Southward, A. J., Henderson, P. A., et al. (2004). Regional climatic warming drives long-term community changes of british marine fish. Proc. Biol. Sci. 271, 655–661. doi: 10.1098/rspb.2003.2651
Graham, K. J., Andrew, N. L., and Hodgson, K. E. (2001). Changes in relative abundance of sharks and rays on Australian South East Fishery trawl grounds after twenty years of fishing. Mar. Freshw. Res. 52, 549–561.
Greenstreet, S. P., Spence, F. E., and McMillan, J. A. (1999). Fishing effects in northeast Atlantic shelf seas: patterns in fishing effort, diversity and community structure. V. Changes in structure of the North Sea groundfish species assemblage between 1925 and 1996. Fish. Res. 40, 153–183. doi: 10.1016/S0165-7836(98)00210-210
Greenstreet, S. P. R., and Hall, S. J. (1996). Fishing and the ground-fish assemblage structure in the North-Western North sea: an analysis of long-term and spatial trends. J. Anim. Ecol. 65, 577–598. doi: 10.2307/5738
Griffiths, C., van Sittert, L., Best, P., Brown, A., Clark, B., Cook, P., et al. (2004). Impacts of human activities on marine animal life in the Benguela. Oceanogr. Mar. Biol. Annu. Rev. 42, 303–392. doi: 10.1111/cobi.12923
Griffiths, M. H. (1997). The life history and stock separation of silver kob, Argyrosomus inodorus, in South African waters. Fish. Bull. 95, 47–67.
Griffiths, M. H. (2000). Long-term trends in catch and effort of commercial linefish off South Africa’s Cape Province: snapshots of the 20th century. South Afr. J. Mar. Sci. 22, 81–110.
Harris, A. N., and Poiner, I. R. (1991). Changes in species composition of demersal fish fauna of Southeast Gulf of Carpentaria, Australia, after 20 years of fishing. Mar. Biol. 111, 503–519. doi: 10.1007/BF01319424
Heath, M. R., and Speirs, D. C. (2012). Changes in species diversity and size composition in the Firth of Clyde demersal fish community (1927–2009). Proc. R. Soc. B Biol. Sci. 279, 543–552. doi: 10.1098/rspb.2011.1015
Hiddink, J. G., Jennings, S., Kaiser, M. J., Queirós, A. M., Duplisea, D. E., and Piet, G. J. (2006). Cumulative impacts of seabed trawl disturbance on benthic biomass, production, and species richness in different habitats. Can. J. Fish. Aquat. Sci. 63, 721–736.
Hiddink, J. G., Jennings, S., Sciberras, M., Bolam, S. G., Cambiè, G., McConnaughey, R. A., et al. (2019). Assessing bottom trawling impacts based on the longevity of benthic invertebrates. J. Appl. Ecol. 56, 1075–1084. doi: 10.1111/1365-2664.13278
Hilborn, R., and Walters, C. J. (2001). Quantitative Fisheries Stock Assessment: Choice, Dynamics and Uncertainty. London: Kluwer Academic Publishers.
Jackson, J. B. C., Kirby, M. X., Berger, W. H., Bjorndal, K. A., Botsford, L. W., Bourque, B. J., et al. (2001). Historical overfishing and the recent collapse of coastal ecosystems. Science 293, 629–637. doi: 10.1126/science.1059199
Johnson, D. D., Rotherham, D., and Gray, C. A. (2008). Sampling estuarine fish and invertebrates using demersal otter trawls: effects of net height, tow duration and diel period. Fish. Res. 93, 315–323. doi: 10.1016/j.fishres.2008.05.012
Kaiser, M. J., Clarke, K. R., Hinz, H., Austen, M. C. V., Somerfield, P. J., Karakakkis, I., et al. (2006). Global analysis of response and recovery of benthic biota to fishing. Mar. Ecol. Prog. Ser. 311, 1–14.
Kaiser, M. J., Ramsay, K., Richardson, C. A., Spence, F. E., and Brand, A. R. (2000). Chronic fishing disturbance has changed shelf sea benthic community structure. J. Anim. Ecol. 69, 494–503. doi: 10.1046/j.1365-2656.2000.00412.x
Kerwath, S. E., Winker, H., Götz, A., and Attwood, C. G. (2013). Marine protected area improves yield without disadvantaging fishers. Nat. Commun. 4:3347. doi: 10.1038/ncomms3347
Kirkman, S. P., Yemane, D., Atkinson, L. J., Kathena, J., Nsiangango, S. E., Singh, L., et al. (2015). Regime shifts in demersal assemblages of the Benguela Current Large Marine Ecosystem: a comparative assessment. Fish. Oceanogr. 24, 15–30. doi: 10.1111/fog.12053
Klaer, N. L. (2001). Steam trawl catches from south-eastern Australia from 1918 to 1957: trends in catch rates and species composition. Mar. Freshw. Res. 52, 399–410.
Kongprom, A., Khaemakorn, P., Eiamsa-ard, M., and Supongpan, M. (2003). “Status of demersal fishery resources in the Gulf of Thailand,” in Assessment, Management and Future Directions for Coastal Fisheries in Asian Countries, eds G. Silvestre, L. Garces, I. Stobutzki, M. Ahmed, R. A. Valmonte-Santos, C. Luna, et al. (Penang: WorldFish Center), 137–152.
Kritzer, J. P., DeLucia, M.-B., Greene, E., Shumway, C., Topolski, M. F., Thomas-Blate, J., et al. (2016). The importance of benthic habitats for coastal fisheries. Bioscience 66, 274–284. doi: 10.1093/biosci/biw014
Kyle, H. M. (1903). Fishing nets, with special reference to the otter-trawl. J. Mar. Biol. Assoc. U. K. New Ser. 6, 562–586. doi: 10.1017/S0025315400072684
Lees, R. (1969). Fishing for Fortunes: The Story of the Fishing Industry in Southern Africa and the Men Who Made It. Cape Town: Purnell & Sons.
Lindholm, J. B., Auster, P. J., Ruth, M., and Kaufman, L. (2001). Modeling the effects of fishing and implications for the design of marine protected areas: juvenile fish responses to variations in seafloor habitat. Conserv. Biol. 15, 424–437. doi: 10.1046/j.1523-1739.2001.015002424.x
Lotze, H. K., Lenihan, H. S., Bourque, B. J., Bradbury, R. H., Cooke, R. G., Kay, M. C., et al. (2006). Depletion, degradation, and recovery potential of estuaries and coastal seas. Science 312:1806. doi: 10.1126/science.1128035
Lotze, H. K., and Worm, B. (2009). Historical baselines for large marine animals. Trends Ecol. Evol. 24, 254–262. doi: 10.1016/j.tree.2008.12.004
Mackinson, S. (2001). “Representing trophic interactions in the North Sea in the 1880 s, using the Ecopath mass-balance approach,” in Fisheries Impacts on North Atlantic Ecosystems: Models and Analyses Fisheries Centre Research Reports 9(4), eds S. Guenette, V. Christensen, and D. Pauly, 35–98.
Mann, B. (ed.) (2013). Southern African marine linefish species profiles. Durban: South African Association for Marine Biological Research.
McConnaughey, R. A., Mier, K. L., and Dew, C. B. (2000). An examination of chronic trawling effects on soft-bottom benthos of the eastern Bering Sea. ICES J. Mar. Sci. 57, 1377–1388. doi: 10.1006/jmsc.2000.0906
McHugh, M., Sims, D. W., Partridge, J. C., and Genner, M. J. (2011). A century later: long-term change of an inshore temperate marine fish assemblage. J. Sea Res. 65, 187–194. doi: 10.1016/j.seares.2010.09.006
Mellin, C., Aaron MacNeil, M., Cheal, A. J., Emslie, M. J., and Julian Caley, M. (2016). Marine protected areas increase resilience among coral reef communities. Ecol. Lett. 19, 629–637. doi: 10.1111/ele.12598
Micheli, F., Saenz-Arroyo, A., Greenley, A., Vazquez, L., Espinoza Montes, J. A., Rossetto, M., et al. (2012). Evidence that marine reserves enhance resilience to climatic impacts. PLoS One 7:e40832. doi: 10.1371/journal.pone.0040832
Moloney, C. L., Fennessy, S. T., Gibbons, M. J., Roychoudhury, A., Shillington, F. A., von der Heyden, B. P., et al. (2013). Reviewing evidence of marine ecosystem change off South Africa. Afr. J. Marine Sci. 35, 427–448. doi: 10.2989/1814232X.2013.836135
Moran, M. J., and Stephenson, P. C. (2000). Effects of otter trawling on macrobenthos and management of demersal scalefish fisheries on the continental shelf of north-western Australia. ICES J. Mar. Sci. 57, 510.
Mussgnug, R. S. (2013). An Assessment of the Long-Term Changes in Chondrichthyan Abundance on the Inshore Trawl Grounds of the Agulhas Bank, South Africa. Available at: https://open.uct.ac.za/handle/11427/14017 (Accessed January 31, 2017).
Novaglio, C., Smith, A. D. M., Frusher, S., and Ferretti, F. (2020). Identifying historical baseline at the onset of exploitation to improve understanding of fishing impacts. Aquatic Conserv. 30, 475–485. doi: 10.1002/aqc.3264
Novaglio, C., Smith, A. D. M., Frusher, S., Ferretti, F., Klaer, N., and Fulton, E. A. (2018). Fishery development and exploitation in South East Australia. Front. Mar. Sci. 5:145. doi: 10.3389/fmars.2018.00145
Oksanen, J., Blanchet, F. G., Friendly, M., Kindt, R., Legendre, P., McGlinn, D., et al. (2016). vegan: Community Ecology Package. Available at: https://CRAN.R-project.org/package=vegan (Accessed July 14, 2016).
Parkington, J. (2001). “Milestones: the impact of the systematic exploitation of marine foods on human evolution,” in Humanity from African Naissance to Coming Millennia, eds P. V. Tobias, M. A. Raath, J. Moggi-Cecchi, and G. A. Doyle, (Florence: Firenze University Press), 327–336.
Pauly, D. (1995). Anecdotes and the shifting baseline syndrome of fisheries. Trends Ecol. Evol. 10:430. doi: 10.1016/s0169-5347(00)89171-5
Payne, A. I. L., and Punt, A. E. (1995). “Biology and fisheries of South African Cape hakes (M. capensis and M. paradoxus),” in Hake Chapman & Hall Fish and Fisheries Series, eds J. Alheit, and T. J. Pitcher, (Netherlands: Springer), 15–47. doi: 10.1007/978-94-011-1300-7_2
Penney, A. J., Buxton, C. D., Garratt, P. A., and Smale, M. J. (1989). “The commercial marine linefishery,” in Oceans of Life off Southern Africa, eds A. I. L. Payne, and R. J. M. Crawford, (Vlaeberg: Vlaeberg Publishers), 214–229.
R Core Team (2016). R: A Language and Environment for Statistical Computing. Vienna: R Foundation for Statistical Computing.
Rijnsdorp, A. D., Leeuwen, P. I., van, Daan, N., and Heessen, H. J. L. (1996). Changes in abundance of demersal fish species in the North Sea between 1906–1909 and 1990–1995. ICES J. Mar. Sci. 53, 1054–1062. doi: 10.1006/jmsc.1996.0132
Rogers, S. I., and Ellis, J. R. (2000). Changes in the demersal fish assemblages of British coastal waters during the 20th century. ICES J. Mar. Sci. 57, 866–881. doi: 10.1006/jmsc.2000.0574
Sainsbury, K. J., Campbell, R. A., and Whitelaw, A. W. (1993). “Effects of trawling on the marine habitat on the north west shelf of Australia and implications for sustainable fisheries management,” in Sustainable Fisheries Through Sustaining Fish Habitat, Australian Society for Fish Biology Workshop, ed. A. Hancock, (Canberra: Bureau of Resource Sciences Proceedings), 137–145.
Schwerdtner Máñez, K., Holm, P., Blight, L., Coll, M., MacDiarmid, A., Ojaveer, H., et al. (2014). The future of the oceans past: towards a global marine historical research initiative. PLoS One 9:e101466. doi: 10.1371/journal.pone.0101466
Scott, P. (1949). Otter-trawl fisheries of South Africa. Geogr. Rev. 39, 529–551. doi: 10.2307/210671
Serra-Pereira, B., Erzini, K., Maia, C., and Figueiredo, I. (2014). Identification of potential essential fish habitats for skates based on fishers’ knowledge. Environ. Manage 53, 985–998. doi: 10.1007/s00267-014-0257-253
Silvestre, G. T., Regalado, R. B., and Pauly, D. (1986). “Status of Philippine demersal stocks: inferences from underutilized catch rate data,” in Resources management and socioeconomics of Philippine marine fisheries Technical Reports of the Department of Marine Fisheries, eds D. Pauly, J. Saeger, and G. T. Silvestre, (Philippines: University of the Philippines in the Visayas), 47–96.
Sink, K. J., Holness, S., Harris, L., Majiedt, P., Atkinson, L. J., Robinson, T., et al. (2012a). National Biodiversity Assessment 2011: Technical Report. Volume 4: Marine and Coastal Component. Pretoria: South African National Biodiversity Institute.
Sink, K. J., Wilkinson, S., Atkinson, L. J., Sims, P. F., Leslie, R. W., and Attwood, C. G. (2012b). The Potential Impacts of South Africa’s Demersal Hake Trawl Fishery on Benthic Habitats: Historical Perspectives, Spatial Analyses, Current Review and Potential Management Actions. Cape Town: South African National Biodiversity Institute.
Smale, M. J., and Bruton, M. N. (1985). Predation and prey selectivity by Argyrosomus hololepidotus (Osteichthyes: Sciaenidae) in south-eastern Cape waters of South Africa. South Afr. J. Zool. 20, 97–108. doi: 10.1080/02541858.1985.11447921
Sparre, P., and Venema, S. C. (1998). Introduction to Tropical Fish Stock Assessment: Part 1. Paris: Food and Agriculture Organization of the United Nations.
Stehlik, L. L. (2007). Essential Fish Habitat Source Document. Spiny dogfish, Squalus Acanthias, Life History and Habitat Characteristics. Silver Spring, MA: NOAA.
Stevens, J. D., Bonfil, R., Dulvy, N. K., and Walker, P. A. (2000). The effects of fishing on sharks, rays, and chimaeras (chondrichthyans), and the implications for marine ecosystems. ICES J. Mar. Sci. 57, 476–494. doi: 10.1006/jmsc.2000.0724
QGIS Development Team (2015). QGIS Geographic Information System. Beaverton: Open Source Geospatial Foundation.
ter Hofstede, R., and Rijnsdorp, A. D. (2011). Comparing demersal fish assemblages between periods of contrasting climate and fishing pressure. ICES J. Mar. Sci. 68, 1189–1198. doi: 10.1093/icesjms/fsr053
Thurstan, R. H., Brockington, S., and Roberts, C. M. (2010). The effects of 118 years of industrial fishing on UK bottom trawl fisheries. Nat. Commun. 1:15. doi: 10.1038/ncomms1013
Tilney, R. L., and Hecht, T. (1990). The food and feeding habits of two co-occurring marine catfish Galeichthys feliceps and G. ater (Osteichthyes: Ariidae) along the south-east coast of South Africa. J. Zool. 221, 171–193. doi: 10.1111/j.1469-7998.1990.tb03990.x
Van der Elst, R. P. (1989). “Marine recreational angling in South Africa,” in Oceans of Life off Southern Africa, eds A. I. L. Payne, and R. J. M. Crawford, (Vlaeberg: Vlaeberg Publishers), 164–176.
Warwick, R. M., and Clarke, K. R. (1993). Increased variability as a symptom of stress in marine communities. J. Exp. Mar. Biol. Ecol. 172, 215–226. doi: 10.1016/0022-0981(93)90098-90099
Watermeyer, K. E., Shannon, L. J., and Griffiths, C. L. (2008). Changes in the trophic structure of the southern Benguela before and after the onset of industrial fishing. Afr. J. Marine Sci. 30, 351–382.
Yemane, D., Field, J. G., and Leslie, R. W. (2005). Exploring the effects of fishing on fish assemblages using abundance biomass comparison (ABC) curves. ICES J. Mar. Sci. 62, 374–379. doi: 10.1016/j.icesjms.2005.01.009
Yemane, D., Field, J. G., and Leslie, R. W. (2008). Indicators of change in the size structure of fish communities: a case study from the south coast of South Africa. Fish. Res. 93, 163–172. doi: 10.1016/j.fishres.2008.03.005
Keywords: historical baselines, trawl surveys, demersal communities, Agulhas Bank, long-term change
Citation: Currie JC, Atkinson LJ, Sink KJ and Attwood CG (2020) Long-Term Change of Demersal Fish Assemblages on the Inshore Agulhas Bank Between 1904 and 2015. Front. Mar. Sci. 7:355. doi: 10.3389/fmars.2020.00355
Received: 06 January 2020; Accepted: 27 April 2020;
Published: 26 May 2020.
Edited by:
John A. Cigliano, Cedar Crest College, United StatesReviewed by:
Camilla Novaglio, CSIRO Oceans and Atmosphere (O&A), AustraliaMontserrat Demestre, Institute of Marine Sciences, CSIC, Spain
Copyright © 2020 Currie, Atkinson, Sink and Attwood. This is an open-access article distributed under the terms of the Creative Commons Attribution License (CC BY). The use, distribution or reproduction in other forums is permitted, provided the original author(s) and the copyright owner(s) are credited and that the original publication in this journal is cited, in accordance with accepted academic practice. No use, distribution or reproduction is permitted which does not comply with these terms.
*Correspondence: Jock C. Currie, am9ja2N1cnJpZUBnbWFpbC5jb20=