- 1Centre for Marine Living Resources and Ecology, Ministry of Earth Sciences, Kochi, India
- 2National Institute of Oceanography, Panaji, India
Repeat observations over the Kochi and Mangalore shelves of the southeastern Arabian Sea (SEAS) during April to December 2012 revealed substantial accumulation of methane (CH4) in the nearshore waters (48.6 ± 34.4 nM) compared to the outer shelf (2.9 ± 0.7 nM). Sediment methanogenesis and estuarine discharge appear to be the major sources of CH4 in the nearshore regions during non-upwelling period. But under oxygen deficient conditions that prevail during the upwelling period, extremely low concentrations of CH4 in the nearshore anoxic region of Mangalore (14 ± 2 nM) compared to similar region of hypoxic Kochi shelf (35.5 ± 15.4 nM) have been observed. We propose that this is mainly due to its greater loss through anaerobic oxidation and in part by the reduced sedimentary inputs by weak bioturbation over Mangalore relative to Kochi. On an annual basis, SEAS is found to be a net source of CH4 to the atmosphere with its efflux ranging from 0.03 to 170 μmol m–2 d–1 (21.9 ± 36.7 μmol m–2 d–1). Following a zonal extrapolation approach, the estimated CH4 efflux from the SEAS (7–14°N; 3.2 Gg y–1) accounts for up to ∼16% of the total CH4 emission from the Arabian Sea.
Introduction
Coastal seas, important sites of methane (CH4) production, are estimated to contribute up to ∼20% emission of all greenhouse gases (IPCC, 2013). Despite their relatively small geographical coverage (16%), the shelf region accounts for 75% of the oceanic CH4 emission (Bange et al., 1994). Tropical oceans are found to be a net source of CH4 although their effective contribution to the global CH4 budget is relatively small (<4%; Reeburgh, 2007; Kirschke et al., 2013). Marine CH4 is produced under anaerobic conditions in sediments, interior of suspended particles, and in the guts of zooplankton (de Angelis and Lee, 1994; Karl and Tilbrook, 1994; Boetius et al., 2000). CH4 production in the coastal zones is linked to high phytoplankton biomass (Tilbrook and Karl, 1995; Oudot et al., 2002; Damm et al., 2008), its seepage from sea bed reserves (Borges et al., 2016) and sediment-water exchange is augmented by upwelling (Kock et al., 2008; Brown et al., 2014). CH4 is also likely to be produced by aerobic decomposition of methylphosphonate releasing phosphorus in phosphate poor environments (Karl et al., 2008). Inland waters and estuaries also contribute to high coastal CH4 concentrations (Jayakumar et al., 2001; Rao and Sarma, 2016, 2017; Upstill-Goddard and Barnes, 2016).
CH4 emission from coastal zones exhibit large variability due to variations in primary production, decomposition of organic matter, upwelling intensity, etc. (Rehder et al., 2002; Kock et al., 2008). A good example for this is the eastern Arabian Sea (EAS) coastal (alias west coast of India) upwelling system, one of the most intense oxygen deficient shelf systems of world’s ocean, where the magnitude of CH4 emissions has not been well quantified. The previous studies on CH4 from the EAS reported its high surface supersaturation (89–2521%) and several fold higher effluxes compared to oceanic averages (Owens et al., 1991; Patra et al., 1998; Jayakumar et al., 2001; Shirodkar et al., 2018). A significant part of its supersaturation is apparently contributed by the release from sediments along the Indian continental shelf (Karisiddaiah and Veerayya, 1994, 1996). Bange et al. (1998) reported near saturation of CH4 in the central Arabian Sea (103–107%) and supersaturation in the coastal upwelling off Oman (up to 156%) and in an upwelling filament (up to 145%). On the other hand, highly productive regions of west coast of India, with one of the largest low-oxygen zone in the world (Naqvi et al., 2006, 2009), favor in situ production of CH4 in the water column (Owens et al., 1991; Jayakumar et al., 2001).
Most of the CH4 data from the Arabian Sea (AS) correspond to offshore regions and very few datasets are available from the coastal regions. Patra et al. (1998) and Jayakumar et al. (2001) have reported considerable spatial and temporal variation in CH4 distribution and fluxes along the EAS, with highest concentration of ∼24 nM during the late summer monsoon. Naqvi et al. (2005, 2010) have reviewed the importance and cycling of CH4 mostly based on the data generated during the Arabian Sea Process Study (1992–1997). They have reported that the coastal wetlands along the west coast of India potentially contribute CH4 to the nearby coastal regions. Recently, Shirodkar et al. (2018) reported moderate accumulation of CH4 under oxygen deficient conditions over the EAS shelf during the late summer monsoon. Most of the studies on CH4 cycling along the EAS shelf were based on the observations from its central region where intense oxygen deficiency (anoxic/suphidic) prevailed seasonally. Although strong upwelling also occurs over the southeastern Arabian Sea (SEAS), the existence of both anoxic/sulphidic and hypoxic conditions (Gupta et al., 2016; Sudheesh et al., 2016) makes this region different. The present study examined the CH4 distribution and its emissions from the water column to evaluate the influence of upwelling and estuarine contribution over the SEAS shelf. Accordingly, the study focused on (i) factors influencing the distribution and variability of CH4 concentrations, (ii) role of deoxygenation and macrofauna on CH4 concentrations, and (ii) its fluxes from the SEAS shelf.
Materials and Methods
Study Area
The present study focuses on the SEAS, between ∼7° and 14°N (Figure 1). The biogeochemistry of the SEAS is closely linked to the seasonally changing coastal currents by northward and southward flowing West India Coastal Current (WICC) during winter monsoon (WM) and summer monsoon (SM), respectively (Shankar et al., 2002; Amol et al., 2018). The transition seasons viz. spring inter-monsoon (SIM) and fall inter-monsoon (FIM) exhibit mixed signals between SM and WM (Shankar et al., 2002). The SEAS is largely impacted by monsoonal forcing on the mixed layer variations and nutrient cycling. The seasonal reversal of surface circulation enables the southward transport of high salinity waters from the northern Arabian Sea during SM, and northward transport of low salinity waters during WM. The oxygen minimum zone (OMZ; DO < 20 μM) in the AS is among the largest in the world and its advection onto the continental shelf during SM results in severe coastal hypoxia causing drastic changes in the biogeochemical properties including the emission of greenhouse gases (Naqvi et al., 2000; Naqvi and Jayakumar, 2000; Gupta et al., 2016; Sudheesh et al., 2016, 2017). Many small rivers like Mandovi and Zuari in Goa, Netravati in Mangalore flow across the coastal plain and drain into the northern part of SEAS. Cochin (Kochi) estuary (CE), the largest estuarine system of the southwest coast of India, debouch freshwater into the southern part of SEAS, and its annual mean discharge is much higher (12.33 km3) compared to other major estuaries like Zuari (3.25 km3), Mandovi (3.31 km3) and Netravati (11.06 km3) of this region (Krishna et al., 2016). The CE, one of the largest estuarine systems along the southwest coast of India, is a highly heterotrophic system impacted with increased anthropogenic activities (Thottathil et al., 2008; Gupta et al., 2009) and opens directly at the present study site. Recent studies have, however, shown that the CE is acting as heavy sink zone due to high turnover rates of nitrogen, hence its export impact on coastal biogeochemistry is limited mostly to the nearshore region (Bhavya et al., 2016, 2017, 2018; Gupta et al., 2016). During the SM upwelling, shelf waters of southern SEAS experience hypoxia but anoxic conditions prevail both in the water column and sediments of the northern SEAS, triggering varying degree of denitrification over these shelves (Sudheesh et al., 2016).
Sample Collection and Analysis
Repeat observations in the shelf waters off Kochi (∼10°N), SEAS were conducted from April to December 2012 (7 times) onboard FORV Sagar Sampada covering four seasons viz. spring inter-monsoon (SIM: April), summer monsoon (SM: June-September), fall inter-monsoon (FIM: October), and winter monsoon (WM: November-December). Samples were also collected from a nearby transect off Mangalore (12.8°N) during SIM (April), SM (September) and WM (December) to compare CH4 dynamics in the two shelf regions. Along both transects, six stations each were occupied at water depths of 13, 20, 30, 40, 50, and 100 m (Figure 1). Both transects can be classified into four zones viz. nearshore (station 1), inner shelf (stations 2 and 3), mid-shelf (stations 4 and 5), and outer shelf (station 6).
Temperature and salinity profiles were recorded using Sea-Bird CTD profiler (911 plus). Water samples were collected from different depths using Niskin samplers (mounted to CTD rosette for coastal stations). Samples for dissolved oxygen (DO) were collected in 60 ml glass bottles. 0.5 ml each of Winkler reagent was added to the sample to fix DO. After acidification the liberated iodine was titrated against 0.001 N sodium thiosulphate using starch-based visual end point detection (Grasshoff et al., 1999). The small amount of DO carried by the reagents was not subtracted and sodium azide was not used to prevent nitrite interference in the Winkler method. The detection limit is ∼2 μM. Samples for nitrate (NO3–) and nitrite (NO2–) were analyzed spectrophotometrically (Thermo Evolution 201) within few hours of collection following standard procedures (Grasshoff et al., 1999). The analytical precision, expressed as standard deviation based on replicate analyses, was ± 0.01 and ± 0.05 μM for NO2– and NO3–, respectively. Samples (2 L) for chlorophyll (Chla) estimation were filtered onboard through Whatman 47 mm GF/F filter (0.7 μm) under gentle vacuum (<50 mm Hg) and stored at −20°C until analysis. Chla on the filters were extracted with 90% acetone at 4°C in the dark for 12 h and measured fluorometrically (Joint Global Ocean Flux Study, 1994). Samples for particulate organic carbon (POC) were filtered through pre-combusted (4 h at 450°C) 47 mm Whatman GF/F filters and stored at −20°C until analysis. The samples were dried at 40°C overnight, fumigated with HCl in a desiccator for 6 h to remove the inorganic carbon, again dried overnight and analyzed using CHN analyser (Flash EA 2000, Thermo, United States).
Water samples for CH4 and nitrous oxide (N2O) were collected in 60 ml glass bottles immediately following the sampling of DO. Samples were poisoned using saturated mercuric chloride (0.5% v/v) to arrest the microbial activity. The neck of the bottles was sealed using parafilm and samples were kept in dark until analysis. Dissolved CH4 and N2O were analyzed by multiple phase equilibration technique (McAuliffe, 1971). The details of N2O analysis were already described by Sudheesh et al. (2016). Briefly, 25 ml of sample was equilibrated with an equal volume of ultrapure helium in a gas-tight syringe by vigorous shaking at room temperature for 5 min. The headspace gas was then injected through a 5 ml sampling loop into a gas chromatograph (Shimadzu) equipped with a Flame Ionization Detector for CH4 and 63Ni Electron Capture Detector for N2O. The separation was achieved over a stainless steel column (1.8 m length and 2 mm ID) packed with molecular sieve 5A (80/100 mesh, Toshvin) maintained at a temperature of 60°C (40°C for N2O). The instrument was calibrated by several dilutions of CH4 standard (Scotty II Analyzed Gases) procured from Supelco Inc., United States. All the carrier gases and combustion gases used in the analysis were of ultra-pure grade. Air samples were frequently run to monitor instrument response, which was quite linear within the range of concentrations encountered during the course of this study.
Equilibrium solubility of CH4 in the surface waters at the observed temperature and salinity was calculated according to Wiesenburg and Guinasso (1979) using an average atmospheric CH4 mole fraction of 2.03 ppm as reported recently by Rao and Sarma (2017) for the east coast of India.
The percentage saturation of CH4 was calculated as,
where, Cw is the measured concentration of dissolved CH4 and Ca is the equilibrium concentration in seawater.
The sea-air exchange flux density was computed from
where, k (cm h–1) is the gas exchange velocity derived as a function of wind speed (U, m s–1) according to Wanninkhof (2014):
Sc, the Schmidt number for CH4, was calculated from temperature (t) according to the polynomial fit given by Wanninkhof (2014). Continuous wind speed recorded along the ship’s cruise track using automated weather station installed onboard (10 m above sea surface) was used to obtain daily average wind speed and was used for sea-air flux calculations.
Results and Discussion
Spatio-Temporal Variation in Shelf Hydrography, Dissolved Oxygen and Nutrients
The spatio-temporal variations in hydrographical properties, DO and nutrients observed during this study were presented earlier in detail by Gupta et al. (2016) and Sudheesh et al. (2016). Briefly, upwelling off Kochi was found to initiate at the shelf break during January-March, progressed steadily to mid shelf by April and to inner shelf by May. It intensified over the entire shelf from June to August, began to retreat rapidly in September and completely disappeared by October. Under intense solar radiation, sea surface temperature (SST) showed a maximum (>30°C) in April. By May, SST over the inner shelf dropped by 2.8–3.4°C (Table 1) due to upwelling and the cooling trend continued till the SM peaked in August. Thereafter, with the withdrawal of upwelling, SST again increased till December (Figure 2A). The mixed layer depth (MLD, defined as 0.2 kg m–3 density difference from surface) was thin (∼20 m) during SM and thick (∼50 m) over the outer shelf during WM. During SM, the influence of fresh water could be noticed from the sea surface salinity (SSS) which dropped by about 1.5–2.0 during April-June (Table 1), and varied between 33.8 (July) and 31.65 (September).
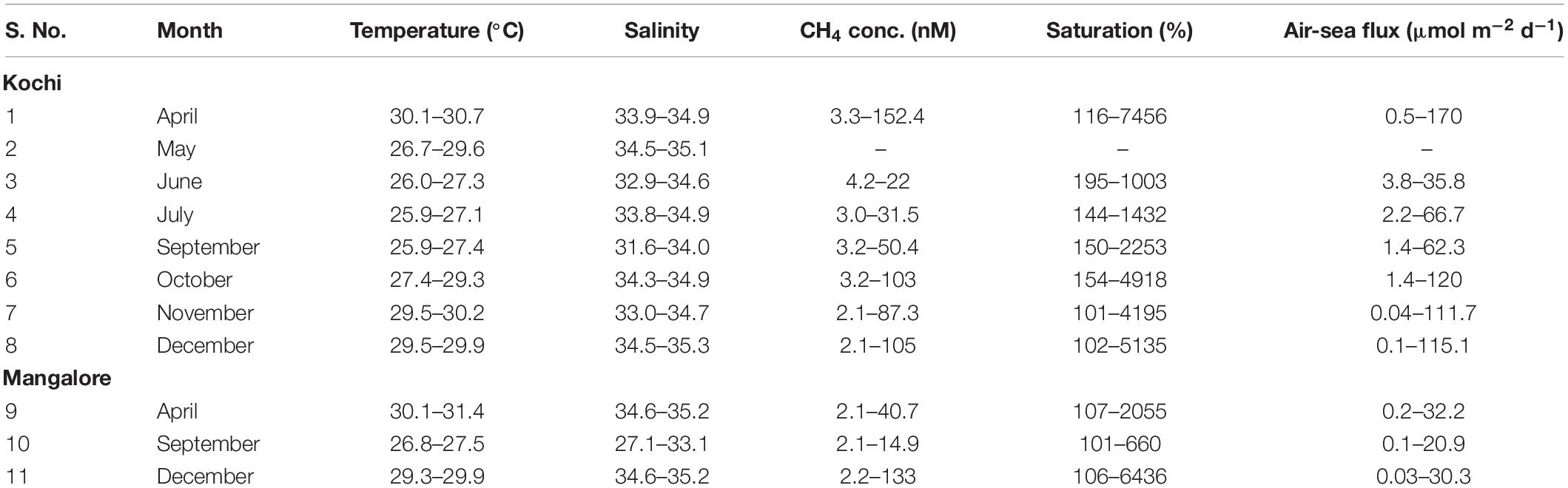
Table 1. Monthly variation in temperature, salinity, and CH4 saturation and fluxes in the surface waters of SEAS.
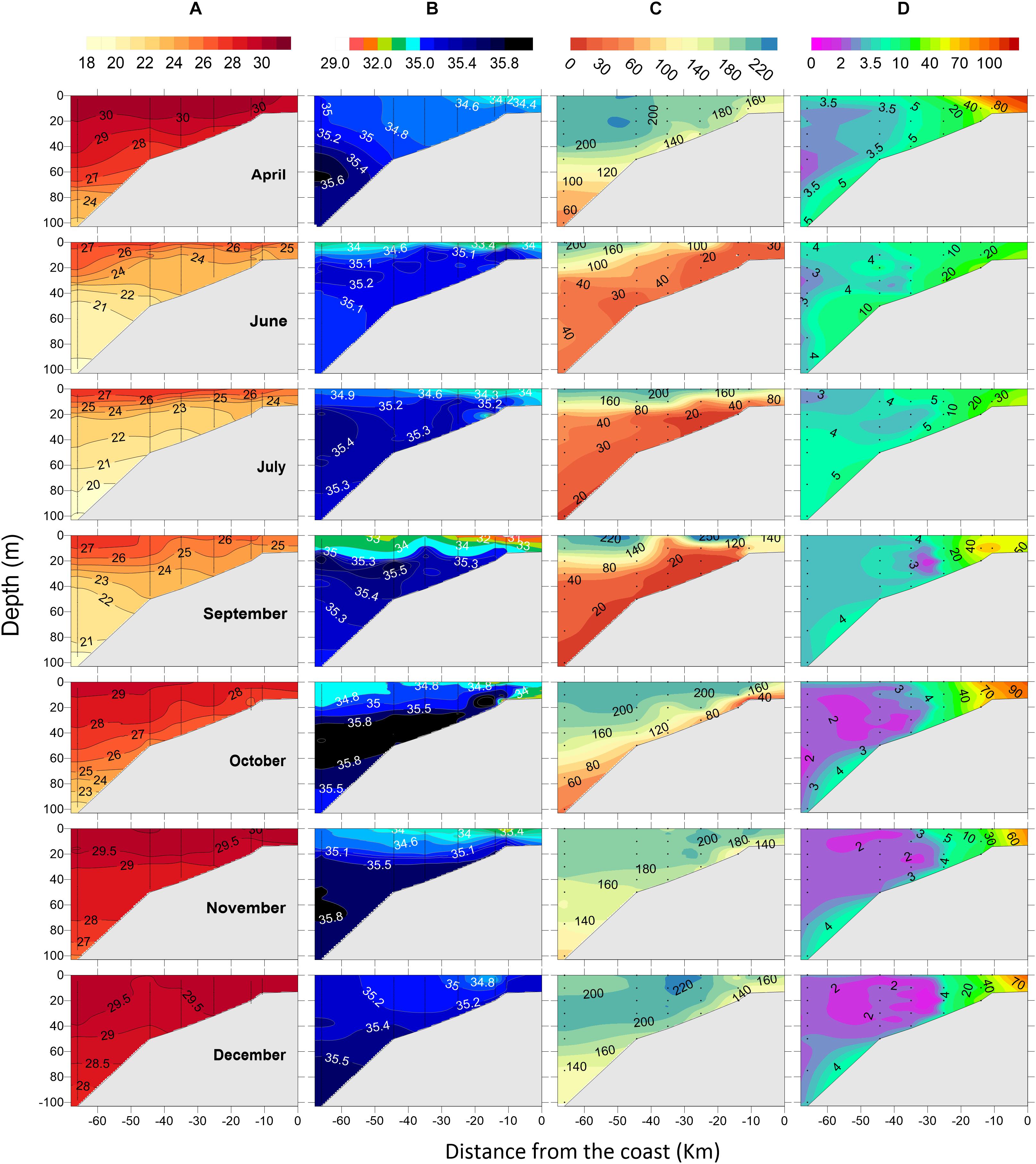
Figure 2. Monthly distribution of (A) temperature (°C), (B) salinity, (C) dissolved oxygen (μM), and (D) methane (nM) off Kochi.
Like Kochi, coastal waters off Mangalore also exhibited clear seasonality in hydrographical properties. Surface waters were warm in April (>30°C) and significantly cooler in September (<27°C, Figure 3A) due to strong upwelling. Thereafter, SST again increased by December (>29°C). The depth of 26°C isotherm (D26), which is used to track the progression of upwelling, shoaled from ∼70 m to <10 m between April and September off Mangalore whereas the same shoaled only up to 20 m off Kochi. This indicates a more vigorous upwelling over the Mangalore shelf during September (late SM) when this process was already waning off Kochi. Strong stratification prevailed in September with low salinity waters (29–33) occupying the upper 5 m of the water column up to mid-shelf off Mangalore due to the SM runoff (Figure 3B). Such an intense upwelling and runoff during the SM led to much stronger thermohaline stratification off Mangalore relative to off Kochi.
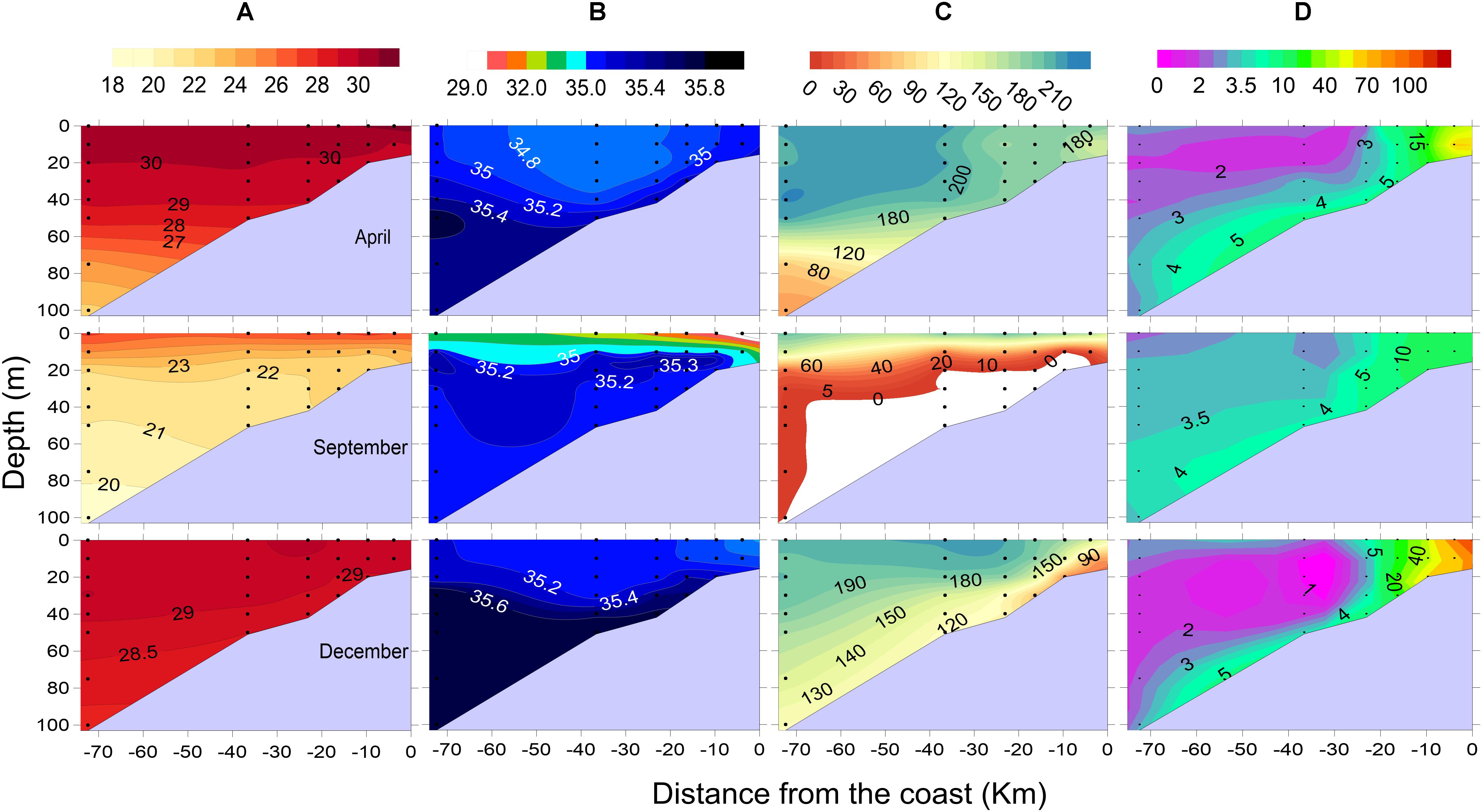
Figure 3. Seasonal distribution of (A) temperature (°C), (B) salinity, (C) dissolved oxygen (μM), and (D) methane (nM) off Mangalore.
With the advent of upwelling, the Kochi shelf experienced oxygen depletion by June and the intense hypoxic conditions in subsurface waters persisted in July (Figures 2, 4; Sudheesh et al., 2016). Between April and June, the water column below 30 m turned intensely hypoxic (DO < 50 μM) and the euphotic zone was enriched with nutrients (NO3–: 8 ± 7 μM; NO2–: 0.7 ± 0.9 μM) (see Supplementary Figures S1–S5 of Gupta et al., 2016). The concentration of DO decreased up to 7 μM in bottom waters and NO2– concentration reached a maximum of 3.2 μM in June due to possible sedimentary denitrification off Kochi. Relative to Kochi, the more sustained and intense upwelling of suboxic/anoxic waters over the Mangalore shelf resulted in bottom water anoxia during September (Figures 2, 3). The resultant intense reducing conditions in the nearshore bottom waters and sediments were manifested by the occurrence of denitrification and sulfate reduction (Sudheesh et al., 2016). Intense H2S smell was noticed from the bottom waters and surface sediments up to its inner shelf during this period, though its concentration was not measured. But Naqvi et al. (2009) have reported build-up of H2S as high as 15.4 μM in these anoxic shallow regions during September. The near-absence of transient reduced forms like NO2– and N2O in the nearshore waters off Mangalore indicated complete denitrification to molecular N2 (Sudheesh et al., 2016).
CH4 Distribution
Dissolved CH4 concentrations over the Kochi (Figure 2D) and Mangalore (Figure 3D) shelves were generally in excess of saturation values with a gradual decrease from the inner shelf toward the outer shelf. Over the annual cycle, CH4 concentrations off Kochi ranged from 10.6 to 152 nM (52.5 ± 34.3 nM) in the nearshore, from 2.3 to 23.6 nM (7.6 ± 5.0 nM) over the inner shelf, from 2.1 to 13.4 nM (3.5 ± 1.8 nM) over the mid-shelf and from 2.1 to 5.0 nM (3.0 ± 0.7 nM) over the outer shelf. Similarly, CH4 concentration off Mangalore varied from 8.9 to 133 nM (39.5 ± 34.2 nM), from 5 to 17 nM (10.0 ± 3.4 nM), from 1.8 to 6.2 nM (3.0 ± 1.1 nM), and from 1.8 to 4.0 nM (2.6 ± 0.6 nM) for the nearshore, inner shelf, mid-shelf and outer shelf regions, respectively. Relatively high CH4 concentrations were recorded in the mid-shelf and outer shelf regions during the peak SM upwelling (June-July), but the nearshore regions showed high concentrations during non-upwelling periods except in April off Mangalore. High tide conditions during the sampling time in April could have resulted in lower contribution of CH4 from the adjoining Netravati estuary to the nearshore region off Mangalore.
Between April and September, when upwelling occurred over both the shelves, the cooler bottom waters (24–26°C) of the outer shelf characterized by somewhat elevated CH4 concentrations (>3 nM) shoaled up from ∼80 to 20 m (Figures 2D, 3D), but returned back following the retrieval of upwelling. This upliftment of subsurface waters led to relatively high CH4 supersaturation in the upper water column of the outer shelf during the summer monsoon (148 ± 25%) than non-monsoon periods (120 ± 22%). This is consistent with the results of earlier studies that reported high CH4 supersaturation (124–286%) during the summer monsoon due to offshore upliftment of subsurface CH4 maxima (Owens et al., 1991; Bange et al., 1998; Patra et al., 1998; Upstill-Goddard et al., 1999; Shirodkar et al., 2018).
The CH4 distribution generally showed a sharp decrease away from the coast (Figures 2D, 3D). Our parallel studies (unpublished) from the Cochin estuary (CE, Figure 1), a wetland eutrophic ecosystem, showed that CH4 levels in its lower estuary were consistently high in April (SIM): 300 ± 246 nM, September (SM): 334 ± 277 nM and December (WM): 217 ± 93 nM (Supplementary Figure S1). Sediments of CE in these regions have been found to contain organic carbon in high concentrations (3–5%) associated with high clay fraction (60–90%) (Martin et al., 2011) and its high decomposition rate (Gupta et al., 2009; Rao and Sarma, 2016) results in substantially high benthic CH4 fluxes to overlying water column (2.54–210 mg m–2 h–1, Verma et al., 2002). CH4 exported out of the CE through tidal exchange and runoff can influence its adjoining nearshore and inner shelf concentrations. This effect is significantly seen during dry non-upwelling periods, for example, in April and December when Kochi coastal waters are warm and saline (Figure 2 and Table 1), its nearshore waters are observed with higher surface concentrations of CH4 (152 and 105 nM) compared to bottom (110 and 70 nM) (Figure 4). In contrast, such estuarine effect is not significantly pronounced during monsoon as upwelling brings about 100 times lower CH4 concentrations (3.5 ± 0.5 nM; Figure 2) compared to estuarine concentrations (334 ± 277 nM; Supplementary Figure S1), thereby the nearshore CH4 concentrations (35 ± 21 nM; Figure 4) are significantly diluted by these two source waters. It must be noted that the significant thermohaline stratification prevailed during monsoon (Figure 2) will prevent the surfacing of upwelling waters. But there used to be spells of weak stratification when wind induced vertical mixing brings low CH4 subsurface waters to surface.
Significant correlations of CH4 with Chla (p< 0.001) and POC (p< 0.005) in nearshore waters during non-upwelling periods (Figure 5) indicate that apart from the estuarine inputs high phytoplankton production can also lead to water column methanogenesis (Owens et al., 1991; Karl et al., 2008). It can also be argued that tidal export flux of nutrients, apart from CH4, from the CE promotes phytoplankton production in the nearshore region; and this unrelated phenomenon is being “misrepresented” as a correlation between CH4 and Chla. The oligotrophic environments, as observed at mid and outer shelves during the non-upwelling period, may also favor CH4 production as reported elsewhere. For example, Karl et al. (2008) have argued that CH4 is produced aerobically through decomposition of methylphosphonate that might serve as a source of phosphorus in phosphate poor environments. However, Damm et al. (2010) reported CH4 production in nitrate-depleted systems that contained sufficient phosphate.
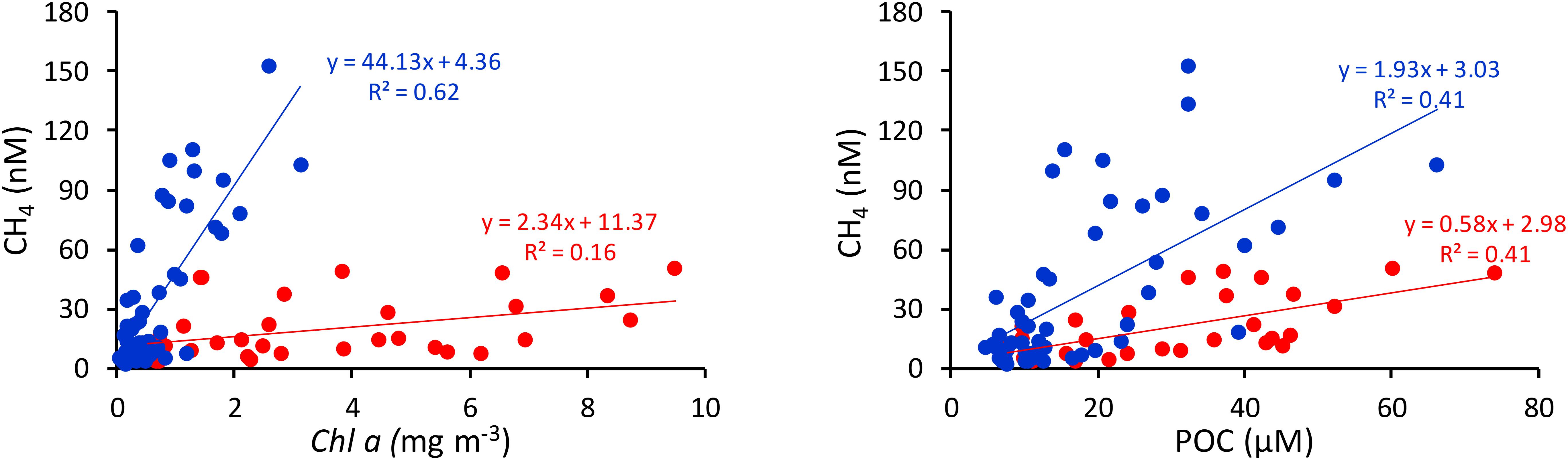
Figure 5. Relationship of CH4 with POC and Chla in the nearshore waters of SEAS during summer monsoon (red) and rest of the year (blue).
Consistently high concentrations of CH4 in bottom waters over both the shelves irrespective of season (Figures 2D, 3D) clearly pointed to its benthic origin. Karisiddaiah and Veerayya (1994, 1996) reported large reservoir of CH4 few meters beneath the sediments of western continental shelf of India. During dry periods, the seepage of this CH4 probably maintains high levels in the overlying waters due to stable and less turbulent conditions. Sediment methanogenesis and CH4 diffusion to the overlying waters, augmented through sedimentary disturbances by benthic organisms, is expected to be the main source of CH4 in bottom waters (Reeburgh, 2007; Naqvi et al., 2010; Borges et al., 2016). This is in agreement with high CH4 production rates (0.2–3.1 μg CH4 g–1 d–1) measured in the upper 25 cm of nearshore sediments of the central west coast of India (Gonsalves et al., 2011). Significantly high CH4 concentrations over the inner shelf observed in the present study are supported by the results of Gireeshkumar et al. (2017) who reported up to 400 nM of CH4 in the nearshore bottom waters of Alapuzha region (∼9.2°N), south of present study area.
Methane Cycling Under Hypoxia/Anoxia
One of the most important factors that control biogeochemical cycling of CH4 is the redox state of the environment, which is primarily determined by the ambient oxygen concentration. Hypoxic/anoxic shelf waters, in general, serve as significant source for CH4 to the atmosphere (Naqvi et al., 2010). The shift in the redox state of the waters from oxic to anoxic (reducing) has been found to have a variable effect on CH4 accumulation (Naqvi et al., 2010, and references therein). This is more important in case of highly productive coastal upwelling systems, including SEAS, where the hypoxic/anoxic conditions prevailed seasonally. High CH4 accumulation up to 5.2 μM in the nearshore bottom waters has been reported in the upwelling waters off Namibia during intense anoxia (Monteiro et al., 2006; Brüchert et al., 2009; Table 2). Though the biological production in these upwelling waters (1.8 g C m–2 day–1) is comparable with that in the SEAS (up to 2.2 g C m–2 day–1; Shirodkar et al., 2018, and reference therein), the massive outbreaks of gases – mostly H2S and CH4 – from its anoxic seafloor have long been known to occur (Emeis et al., 2004), making this region different from the SEAS. The other upwelling systems like off Peru and Chile are also well known for acute oxygen deficiency including sulphidic conditions (Dugdale et al., 1977; Schunck et al., 2013) due to extremely high sediment organic carbon, especially off Peru (20–40%: Fossing, 1990) compared to the SEAS (<4%; Shirodkar et al., 2018, and references therein). This can fuel sedimentary CH4 production and elevate its concentrations in the overlying water column, though to our knowledge CH4 data is not available from these nearshore waters. But from a deeper Chilean shelf site (∼92 m) high CH4 concentrations of ∼80 nM were recorded (Farias et al., 2009). However, contrary to expectation, the present study showed significant reduction in CH4 concentrations in the nearshore waters of both the shelves, when hypoxic/anoxic conditions associated with upwelling prevailed (Figure 4). Upwelling enhances biological production and the reworking/remineralisation of this fresh organic matter may significantly elevate the sedimentary CH4 production (Gonsalves et al., 2011); this is expected to be more at Mangalore than Kochi shelf due to relatively intense upwelling and elevated phytoplankton production in the former region (Sudheesh et al., 2016). Yet, in September, lowest CH4 concentrations from entire study were recorded in the sub-pycnocline anoxic waters off Mangalore up to the inner shelf (13.9 ± 2.7 nM) compared to those over the hypoxic shelf off Kochi (39.0 ± 14.8 nM) (Figures 2, 3, 6). Such extreme low concentrations of CH4 are in consistent with those reported earlier from the same region/season (Naqvi et al., 2009).
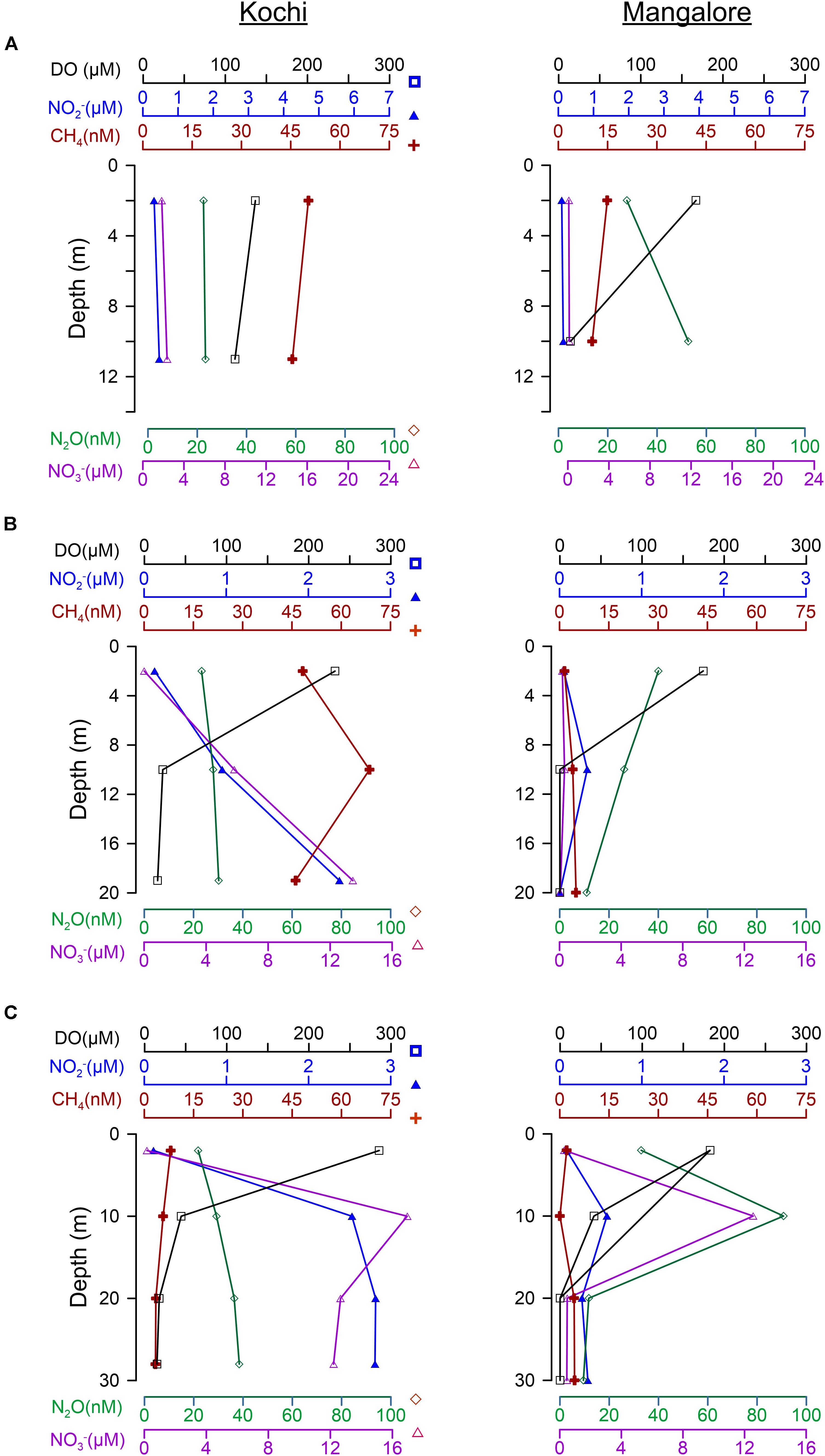
Figure 6. Comparison of vertical profiles for DO, NO3–, NO2–, N2O, and CH4 between Kochi and Mangalore for 13, 20, and 30 m stations (A–C, respectively) during September 2012.
The lower than expected CH4 levels in bottom waters over both the shelves during SM is possible due to mixing with relatively low CH4 concentrations in upwelling waters (Figures 2D, 3D). The upwelling intensity and associated dilution effect over the Kochi is at peak during peak SM (June–July), resulting in low concentrations of CH4 in its nearshore waters (Figure 2). But by late SM (September), the upwelling is in its recession thereby the weak dilution effect has facilitated accumulation of CH4 in the shallow stations near to the coast. However, unlike at Kochi, the upwelling over the Mangalore shelf during September is still sustained thereby the continued dilution effect has reduced the CH4 concentrations (Figure 3). Despite this intra-seasonal variability, the degree of dilution effect over both the shelves is expected to be similar, if so, the mixing alone cannot explain the observed lowest concentrations of CH4 off Mangalore during September.
Earlier studies by Naqvi et al. (2009) and our parallel studies by Sudheesh et al. (2016) reported that during upwelling the Mangalore shelf witnessed shifting from suboxic conditions in peak SM to anoxic conditions by late SM (Figure 3), which led to significant N loss through heterotrophic denitrification during peak phase but switched to autotrophic denitrification during late phase (Pratihary et al., 2014) when sediments of nearshore and inner shelf turned to sulphidic (Naqvi et al., 2009). Such autotrophic denitrification under sulphidic conditions has reduced NO3– to molecular N2 leading to complete loss of N with near absence of intermittent reduced forms like NO2– and N2O in the subpycnocline waters up to inner shelf. Similar absence of NO3– and NO2– were also reported recently off Goa (just north of present study) due to complete denitrification supported by labile sediment organic matter and intense sulfate reduction rates (Naik et al., 2017). Coincidentally, CH4 concentrations in these sulphidic waters are also distinctly low (Figures 3, 6), inferring either a lower sedimentary efflux or greater loss through oxidation under intense reducing conditions.
It has been shown recently that high rates of CH4 oxidation are sustained in the intensely oxygen depleted coastal environments (Steinle et al., 2017) and the CH4 removal through anaerobic oxidation is supported by complete denitrification (Ettwig et al., 2010; Haroon et al., 2013). The sedimentary CH4 has also been stated to undergo microbially mediated anaerobic oxidation by NO2–, Fe3+, Mn4+, and sulfate (Boetius et al., 2000; Beal et al., 2009; Haroon et al., 2013). The marked low concentrations of CH4 coinciding with extremely low concentrations of NO2– and N2O (Figures 6, 7) under excessive presence of H2S (Naqvi et al., 2009) over the Mangalore shelf are therefore possibly infer that greater loss of CH4 is augmented by complete denitrification and/or intense sulfate reduction. Gas charged sediments with pockets of CH4 were observed in the inner shelf regions of SEAS (Mazumdar et al., 2009) and the accumulation of H2S has been attributed to the oxidation of CH4 by sulfate in these shallow sediments (Naik et al., 2017), as well as from coastal sediments off Namibia and elsewhere (Fossing et al., 2000; Brüchert et al., 2003). The recent finding on significant influence of anaerobic oxidation of CH4 driven sulfate reduction across the sulfate-methane transition zone in the sediment cores of SEAS (Fernandes et al., 2020) has further strengthened above arguments. Indeed, a careful examination of annual climatology developed based on monthly/fortnightly averaged records over the Goa inner shelf (see Plate 2 of Naqvi et al., 2009) clearly depicted intra-seasonal shift during SM: NO2– (∼4 μM) and N2O (∼200 μM) were enriched under no sulphidic conditions during peak SM (August) but by late SM (September–October) they were down by an order of magnitude when the waters turned to sulphidic; many times CH4 enrichment was not experienced under such intense sulphidic and denitrified conditions (Shirodkar et al., 2018). Similarly, Mangalore shallow waters were also observed with substantial low concentrations of NO2– and N2O (<2 μM and ∼40 nM, respectively) under intense sulphidic conditions (up to ∼15 μM of H2S) during September 2001 (Naqvi et al., 2009), which is also the case from the present study (Figures 6, 7). There were also occasions at off Goa and Mangalore when maximum CH4 enrichment occurred under strong reducing conditions without being sulphidic (Shirodkar et al., 2018). Though the role of anoxia in CH4 accumulation/removal is not straightforward (Shirodkar et al., 2018) as was also seen from our data (figure not shown), the co-occurrence of poor CH4, and NO2– and N2O (Figure 7) along with high H2S concentrations (Naqvi et al., 2009) can infer that CH4 removal is succeeded by anaerobic oxidation. Recent studies suggest that nitrite-dependent anaerobic CH4 oxidation (n-damo) is an important pathway for its loss in aquatic systems (Ettwig et al., 2010; Hu et al., 2014). However, Shirodkar et al. (2018) based on their incubation experiments of anoxic waters with 15N-labeled NO2– in the presence/absence of CH4 have found that n-damo is not significant off Goa. If this is true, we expect very high accumulation of N2O as n-damo is known to bypass its formation. But the present as well as earlier studies (Naqvi et al., 2009) have clearly showed no substantial build-up of N2O (Figures 6, 7) during sulphidic periods in September, implying that the role of n-damo in CH4 sink cannot be ruled out completely.
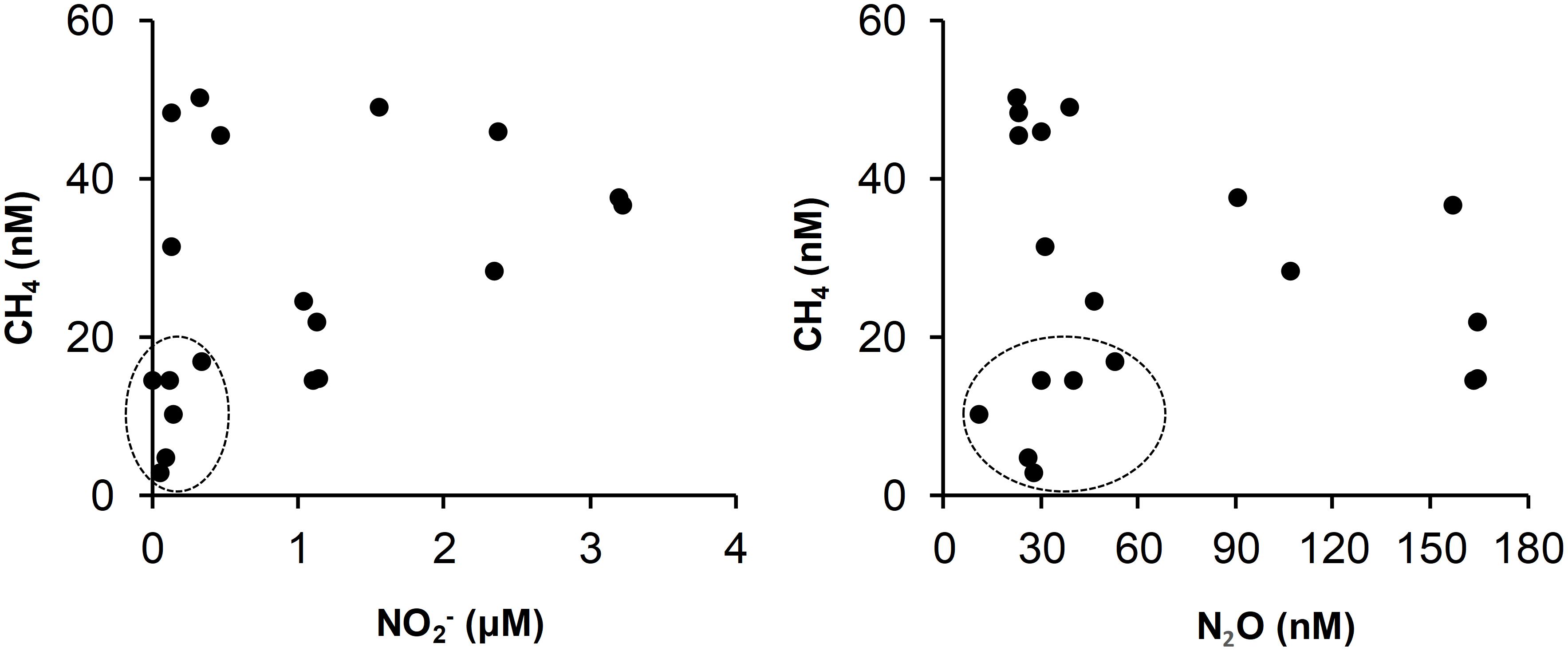
Figure 7. Relationship between CH4 concentrations and transient denitrified products in the nearshore regions during SM. Circled points depicting lowest concentrations correspond to Mangalore during September.
On the other hand, Kochi shelf being remained at hypoxic conditions has prevailed with anoxic sediments only at ∼20 cm below the surface at nearshore (unpublished data) when such sediments exist even close to sediment-water interface at Mangalore (Pratihary et al., 2014; Naik et al., 2017). This restricts to the occurrence of non-sulphidic and weak sediment denitrified/reduced conditions at Kochi (Sudheesh et al., 2016), as evidenced by relatively higher concentrations of NO2– and N2O, which does not support significant loss of CH4 relative to Mangalore (Figures 6, 7).
Apart from anaerobic oxidative loss of CH4, its low concentrations during SM are also possible to some extent due to less sedimentary diffusive efflux by the benthic fauna. In the SEAS shelf, macro infaunal communities are overwhelmingly dominated by deposit feeding opportunistic polychaetes (Abdul Jaleel et al., 2015). During the SM, the density and diversity of larger benthic fauna (prawns, crabs, molluscs, etc.) become insignificant and groups which are sensitive to hypoxia, like echinoderms, are either absent or least abundant (Parameswaran et al., 2018). Summer monsoon being the recruitment period for many marine organisms, the benthic fauna from the SEAS shelf is characterized by proliferation of juveniles but their abundance depends on the intensity of hypoxia (Abdul Jaleel et al., 2015). As stated earlier, unlike at Mangalore, Kochi surface sediments are just short of being anoxic in nature. Accordingly, benthic fauna in less stressful (hypoxic) environment off Kochi are able to survive and function, albeit at lower abundance and diversity, thereby contributing to sediment-water exchanges through bioturbation (Sivadas et al., unpublished). In contrast, the more stressful anoxic (sulphidic) conditions off Mangalore are expected to cause exclusion of the macro and megafauna, impacting ecosystem functions such as bioturbation and bio-irrigation (Levin et al., 2009). Our recent studies (unpublished) showed that even meiofaunal density in these anoxic regions have suffered maximum with almost an order of less magnitude compared to those in hypoxic regions. A significant reduction in sediment reworking (∼75%) by the less abundant burrowing organisms in such intense reducing environment (Sturdivant et al., 2012) is expected to cause lower CH4 diffusion rate (Bonaglia et al., 2017) at Mangalore relative to Kochi.
It has been shown that CH4 accumulation in the oxygen depleted waters over the eastern Arabian Sea shelf during late SM is gradually decreasing southwards from ∼22 to 104 nM off Goa, 14–70 nM off Karwar (between Goa and Mangalore) and 10–18 nM off Mangalore but increased to 80–84 nM off Kochi (Shirodkar et al., 2018). Though this trend is matching with the present results, it is unclear of what controls such trend. Our study, therefore, offers a plausible explanation that the reduction in CH4 concentrations in the nearshore anoxic/hypoxic waters of Mangalore/Kochi during SM can largely be defined by the degree of CH4 loss through its anaerobic oxidation and to some extent by its differential sedimentary diffusion rates controlled by the benthic bioturbation potential. Though the oxygen depleted coastal upwelling regions are the largest source of greenhouse gasses to the atmosphere amongst the world oceans, intense anoxic/sulphidic periods at times are beneficial in naturally reducing the potential impact of this greenhouse effect by converting CH4 to CO2 and/or bypassing N2O production.
Quantification of CH4 Emissions
The CH4 saturation levels off Kochi ranged from 101% to 7456% (Table 1) with maximum values in the nearshore region (2839 ± 1965%) followed by inner shelf (464 ± 183%), mid-shelf (148 ± 32%), and outer shelf (145 ± 27%). Similar saturation levels (101–6, 435%) were recorded off Mangalore where they also decreased drastically from nearshore (2011 ± 2132%) to inner shelf (546 ± 285%) and then to mid-shelf (156 ± 38%) and outer shelf (115 ± 16%). Relatively high nearshore saturation levels of CH4 off Kochi compared to those off Mangalore are attributed to greater CH4 contribution from the adjoining highly eutrophic CE relative to the Netravati estuary. The saturation levels observed over the mid and outer shelves were within the range reported earlier from the continental shelf of India by Jayakumar et al. (2001) and Shirodkar et al. (2018).
The air-sea CH4 emissions from SEAS (7–14°N) ranged from 3 to 170 μmol m–2 d–1 in the nearshore, 2.3–23.5 μmol m–2 d–1 in the inner shelf, 0.04–3.9 μmol m–2 d–1 in the mid-shelf and 0.03–3.8 μmol m–2 d–1 in the offshore regions. The computed fluxes from the mid and outer shelves are comparable with earlier studies from the Arabian Sea (Owens et al., 1991; Patra et al., 1998; Jayakumar et al., 2001; Shirodkar et al., 2018) and other coastal upwelling systems (Table 2). However, significantly higher fluxes from the nearshore region underscore the importance of these regions in contributing to the global CH4 flux as reported from elsewhere (Borges et al., 2016). On an annual basis, the air-sea CH4 emissions from the near-shore region of the SEAS (57.7 ± 45.4 μmol m–2 d–1) are 2–3 times higher than the global average for the continental shelves (22–37 μmol m–2 d–1 by Bange et al., 1994; 30 μmol m–2 d–1 by Borges et al., 2016) and ∼200 times higher than the global average of open oceanic waters (0.2–0.5 μmol m–2 d–1 by Rhee et al., 2009).
The annual sea-air flux of CH4 from the SEAS shelf (7–14°N) was estimated following a zonal and gridded extrapolation method (Sudheesh et al., 2016). Accordingly, the SEAS shelf was divided into two zones viz. 7–11°N and 11–14°N latitudes, which represent the Kochi and Mangalore shelves respectively. In addition, each of these shelf zones were subdivided into six grid boxes according to the water depth (viz. 0–15, 15–25, 25–35, 35–45, 45–75, 75–200 m), which correspond to six monitoring stations respectively from each transect. The fluxes from each grid box were computed by multiplying the surface area of each grid box with the corresponding station CH4 flux density. The sum of these grid box fluxes yielded CH4 flux of 2.6 and 0.6 Gg y–1 to the zones 7–11°N and 11–14°N, respectively. Combining these zones, CH4 efflux from the SEAS shelf (7–14°N) is estimated at 3.2 Gg y–1, which is equivalent to ∼16% of total emission from the Arabian Sea (∼20 Gg y–1; Bange et al., 1998 – flux computed according to Wanninkhof, 1992). The CH4 emissions from the offshore regions of Arabian Sea have been reported to account for <1% of its global oceanic emissions (Naqvi et al., 2005), however, the inclusion of emissions from the coastal Arabian Sea would elevate the contribution to global oceanic fluxes. It is suggested that the zonal integration approach followed in this study should be undertaken all along the western continental shelf of India to reduce uncertainties in estimation of CH4 emissions from the Arabian Sea.
Conclusion
The present study shows that the SEAS is a significant source of CH4 to the atmosphere. Higher CH4 concentrations were recorded in the nearshore region which gradually decreased toward offshore. Significantly high CH4 concentrations in the nearshore waters during non-upwelling period are presumably due to its combined inputs from sediments, in-situ production and estuarine export flux through tidal flushing. Contrary to expectation, increase in CH4 concentrations during seasonal hypoxic/anoxic periods was not observed. Instead, a decrease in CH4 concentration occurred in nearshore region of Mangalore during bottom water anoxic events in late summer is largely attributed to its greater sedimentary loss through anaerobic oxidation and in part by its reduced supply from sediment due to weak bioturbation. The estimated CH4 efflux varied between 0.03 and 170 μmol m–2 d–1 with an annual emission of 3.2 Gg from the SEAS (7–14°N), which is ∼16% of the previously reported total CH4 emission from the Arabian Sea. However, further studies are needed to understand the changes in CH4 emissions from the Indian continental shelves arising from the ongoing regional (e.g., eutrophication) and global (e.g., warming) environmental changes.
Data Availability Statement
All datasets generated for this study are included in the article/Supplementary Material.
Author Contributions
GG conceived the study. VS and GG conducted field studies and processed the data. All authors prepared the manuscript.
Conflict of Interest
The authors declare that the research was conducted in the absence of any commercial or financial relationships that could be construed as a potential conflict of interest.
Acknowledgments
We thank Secretary, Ministry of Earth Sciences (MoES), Government of India and Director, Centre for Marine Living Resources and Ecology (CMLRE) for the support of this study. Drs. Damodar Shenoy and Hema Naik, National Institute of Oceanography, Goa helped in extending the lab facilities for CH4 analysis. Thanks to Dr. Sanjeev Kumar, Physical Research Laboratory for analyzing POC samples. Thanks also to the FORV Sagar Sampada vessel management group for allotting the cruises and to the fishing hands and crew members for their onboard support during the cruises. VS thanks CMLRE for financial support. The data presented are archived at www.incois.gov.in. This work was undertaken as part of the SIBER-India program of MoES and executed under the Marine Living Resources program of CMLRE. This is CMLRE contribution number 113.
Supplementary Material
The Supplementary Material for this article can be found online at: https://www.frontiersin.org/articles/10.3389/fmars.2020.00324/full#supplementary-material
FIGURE S1 | Surface CH4 concentrations in the Cochin estuary during 2012.
References
Abdul Jaleel, K. U., Parameswaran, U. V., Gopal, A., Khader, C., Ganesh, T., Sanjeevan, V. N., et al. (2015). Evaluation of changes in macrobenthic standing stock and polychaete community structure along the south eastern Arabian Sea shelf during the monsoon trawl-ban. Cont. Shelf Res. 102, 9–18. doi: 10.1016/j.csr.2015.04.011
Amol, P., Vijith, V., Fernando, V., Pednakar, P., and Singh, J. P. (2018). Impact of local and remote winds on the shelf circulation off the central west coast of India. Ocean Dyn. 68, 1607–1623.
Bange, H. W., Bartell, U. H., Rapsomanikis, S., and Andreae, M. O. (1994). Methane in the Baltic and North Seas and a reassessment of the marine emissions of methane. Glob. Biogeochem. Cycles 8:465. doi: 10.1029/94GB02181
Bange, H. W., Ramesh, R., Rapsomanikis, S., and Andreae, M. O. (1998). Methane in surface waters of the Arabian Sea. Geophys. Res. Lett. 25, 3547–3550.
Beal, E. J., House, C. H., and Orphan, V. J. (2009). Manganese- and iron-dependent marine methane oxidation. Science 325, 184–187. doi: 10.1126/science.1169984
Berner, U., Poggenburg, J., Faber, E., Quadfasel, D., and Frische, A. (2003). Methane in ocean waters of the Bay of Bengal: its sources and exchange with the atmosphere. Deep. Res. II Top. Stud. Oceanogr. 50, 925–950. doi: 10.1016/S0967-0645(02)00613-6
Bhavya, P. S., Kumar, S., Gupta, G. V. M., Sudharma, K. V., and Sudheesh, V. (2018). Spatio-temporal variation in δ13CDIC of a tropical eutrophic estuary (Cochin estuary, India) and adjacent Arabian Sea. Cont. Shelf Res. 153, 75–85. doi: 10.1016/j.csr.2017.12.006
Bhavya, P. S., Kumar, S., Gupta, G. V. M., and Sudheesh, V. (2017). Carbon uptake rates in the cochin estuary and adjoining coastal Arabian Sea. Estuar. Coast. 40, 447–456. doi: 10.1007/s12237-016-0147-4
Bhavya, P. S., Kumar, S., Gupta, G. V. M., Sudheesh, V., Sudharma, K. V., Varrier, D. S., et al. (2016). Nitrogen uptake dynamics in a tropical eutrophic estuary (Cochin, India) and adjacent coastal waters. Estuar. Coast. 39, 54–67. doi: 10.1007/s12237-015-9982-y
Boetius, A., Ravenschlag, K., Schubert, C. J., Rickert, D., Widdel, F., Gieseke, A., et al. (2000). A marine microbial consortium apparently mediating anaerobic oxidation of methane. Nature 407, 623–626. doi: 10.1038/nrmicro2944
Bonaglia, S., Brüchert, V., Callac, N., Vicenzi, A., Chi Fru, E., and Nascimento, F. J. A. (2017). Methane fluxes from coastal sediments are enhanced by macrofauna. Sci. Rep. 7:13145. doi: 10.1038/s41598-017-13263-w
Borges, A. V., Champenois, W., Gypens, N., Delille, B., and Harlay, J. (2016). Massive marine methane emissions from near-shore shallow coastal areas. Sci. Rep. 6:27908. doi: 10.1038/srep27908
Brown, I. J., Torres, R., and Rees, A. P. (2014). The origin of sub-surface source waters define the sea-air flux of methane in the Mauritanian Upwelling, NW Africa. Dyn. Atmos. Ocean. 67, 39–46. doi: 10.1016/j.dynatmoce.2014.06.001
Brüchert, V., Jørgensen, B. B., Neumann, K., Riechmann, D., Schlösser, M., and Schulz, H. (2003). Regulation of bacterial Sulphate reduction and hydrogen sulfide fluxes in the central Namibian coastal upwelling zone. Geochim. Cosmochim. Acta 67, 4505–4518.
Brüchert, V., Currie, B., and Peard, K. R. (2009). Hydrogen sulphide and methane emissions on the central Namibian shelf. Prog. Oceanog. 83, 169–179.
Damm, E., Helmke, E., Thoms, S., Schauer, U., Nöthig, E., Bakker, K., et al. (2010). Methane production in aerobic oligotrophic surface water in the central Arctic Ocean. Biogeosciences 7, 1099–1108. doi: 10.5194/bgd-6-10355-2009
Damm, E., Kiene, R. P., Schwarz, J., Falck, E., and Dieckmann, G. (2008). Methane cycling in Arctic shelf water and its relationship with phytoplankton biomass and DMSP. Mar. Chem. 109, 45–59. doi: 10.1016/j.marchem.2007.12.003
de Angelis, M. A., and Lee, C. (1994). Methane production during zooplankton grazing on marine phytoplankton. Limnol. Ocean. 39, 1298–1308. doi: 10.1126/science.233.4770.1314
Dugdale, R. C., Goering, J. J., Barber, R. T., Smith, R. L., and Packard, T. T. (1977). Denitrification and hydrogen sulfide in the Peru upwelling region during 1976. Deep Sea Res. 24, 601–608. doi: 10.1016/0146-6291(77)90530-6
Emeis, K. C., Brüchert, V., Currie, B., Endler, R., Ferdelman, T., Kiessling, A., et al. (2004). Shallow gas in shelf sediments of the Namibian coastal upwelling ecosystem. Cont. Shelf Res. 24, 627–642. doi: 10.1016/j.csr.2004.01.007
Ettwig, K. F., Butler, M. K., Le Paslier, D., Pelletier, E., Mangenot, S., Kuypers, M. M. M., et al. (2010). Nitrite-driven anaerobic methane oxidation by oxygenic bacteria. Nature 464, 543–548. doi: 10.1038/nature08883
Farías, L., Fernández, C., Faúndez, J., Cornejo, M., and Alcaman, M. E. (2009). Chemolithoautotrophic production mediating the cycling of the greenhouses gases N2O and CH4 in an upwelling ecosystem. Biogeosciences 6, 3053–3069. doi: 10.5194/bg-6-3053-2009
Fernandes, S., Mazumdar, A., Peketi, A., Anand, S. S., Rengarajan, R., Jose, A., et al. (2020). Sulfidization processes in seasonally hypoxic shelf sediments: a study off the West coast of India. Mar. Petrol. Geol. 117:104353. doi: 10.1016/j.marpetgeo.2020.104353
Fossing, H. (1990). Sulfate reduction in shelf sediments in the upwelling region off Central Peru. Cont. Shelf Res. 10, 355–367. doi: 10.1016/0278-4343(90)90056-R
Fossing, H., Ferdelman, T. G., and Berg, P. (2000). Sulfate reduction and methane oxidation in continental margin sediments influenced by irrigation (South-East Atlantic off Namibia). Geochim. Cosmochim. Acta 64, 897–910.
Gireeshkumar, T. R., Mathew, D., Pratihary, A. K., Naik, H., Narvekar, K. U., Araujo, J., et al. (2017). Influence of upwelling induced near shore hypoxia on the Alappuzha mud banks, South West Coast of India. Cont. Shelf Res. 139, 1–8. doi: 10.1016/j.csr.2017.03.009
Gonsalves, M.-J., Fernandes, C. E. G., Fernandes, S. O., Kirchman, D. L., and Bharathi, P. A. L. (2011). Effects of composition of labile organic matter on biogenic production ofmethane in the coastal sediments of the Arabian Sea. Environ. Monit. Assess. 182, 385–395. doi: 10.1007/s10661-011-1883-3
Grasshoff, K., Kremling, K., and Ehrhardt, M. (1999). Methods of Seawater Analysis. Hoboken, NJ: Wiley.
Gupta, G. V. M., Sudheesh, V., Sudharma, K. V., Saravanane, N., Dhanya, V., Dhanya, K. R., et al. (2016). Evolution to decay of upwelling and associated biogeochemistry over the southeastern Arabian Sea shelf. J. Geophys. Res. Biogeosci. 121, 159–175. doi: 10.1002/2015JG003163
Gupta, G. V. M., Thottathil, S. D., Balachandran, K. K., Madhu, N. V., Madeswaran, P., and Nair, S. (2009). CO2 supersaturation and net heterotrophy in a tropical estuary (Cochin, India): influence of anthropogenic effect: carbon dynamics in tropical estuary. Ecosystems 12, 1145–1157. doi: 10.1007/s10021-009-9280-2
Haroon, M. F., Hu, S., Shi, Y., Imelfort, M., Keller, J., Hugenholtz, P., et al. (2013). Anaerobic oxidation of methane coupled to nitrate reduction in a novel archaeal lineage. Nature 500, 567–570. doi: 10.1038/nature12375
Hu, B.-L., Shen, L.-D., Lian, X., Zhu, Q., Liu, S., Huang, Q., et al. (2014). Evidence for nitrite-dependent anaerobic methane oxidation as a previously overlooked microbial methane sink in wetlands. Proc. Natl. Acad. Sci. U.S.A. 111, 4495–4500. doi: 10.1073/pnas.1318393111
IPCC (2013). Climate Change 2013: The Physical Science Basis. Contribution of Working Group I to the Fifth Assessment Report of the Intergovernmental Panel on Climate Change. Cambridge: Cambridge University Press.
Jayakumar, D. A. (1999). Biogeochemical Cycling of Methane and Nitrous Oxide in the Northern Indian Ocean. Ph. D. Thesis, Goa University, Goa.
Jayakumar, D. A., Naqvi, S. W. A., Narvekar, P. V., and George, M. D. (2001). Methane in coastl and offshore waters of the Arabian Sea. Mar. Chem. 74, 1–13.
Joint Global Ocean Flux Study, (1994). Protocols for the Joint Global Ocean Flux Study (JGOFS) Core Measurements, IOC Manual and Guides, 29, 119–122, 155–162. Paris: UNESCO.
Karisiddaiah, S. M., and Veerayya, M. (1994). Methane-bearing shallow gas-charged sediments in the eastern Arabian Sea: a probable source for greenhouse gas. Cont. Shelf Res. 14, 1361–1370. doi: 10.1016/0278-4343(94)90053-1
Karisiddaiah, S. M., and Veerayya, M. (1996). Potential distribution of subsurface methane in the sediments of the eastern Arabian Sea and its possible implications. J. Geophys. Res. 101, 25887–25895.
Karl, D. M., Beversdorf, L., Björkman, K. M., Church, M. J., Martinez, A., and Delong, E. F. (2008). Aerobic production of methane in the sea. Nat. Geosci. 1, 473–478. doi: 10.1038/ngeo234
Karl, D. M., and Tilbrook, B. D. (1994). Production and transport of methane in oceanic particulate organic matter. Nature 368, 732–734.
Kirschke, S., Bousquet, P., Ciais, P., Saunois, M., Canadell, J. G., Dlugokencky, E. J., et al. (2013). Three decades of global methane sources and sinks. Nat. Geosci. 6, 813–823. doi: 10.1038/ngeo1955
Kock, A., Gebhardt, S., and Bange, H. W. (2008). Methane emissions from the upwelling area off Mauritania (NW Africa). Biogeosciences 5, 1119–1125.
Krishna, M. S., Prasad, M. H. K., Rao, D. B., Viswanadham, R., Sarma, V. V. S. S., and Reddy, N. P. C. (2016). Export of dissolved inorganic nutrients to the northern Indian Ocean from the Indian monsoonal rivers during discharge period. Geochim. Cosmochim. Acta 172, 430–443. doi: 10.1016/j.gca.2015.10.013
Levin, L. A., Ekau, W., Gooday, A. J., Jorissen, F., Middelburg, J. J., Naqvi, S. W. A., et al. (2009). Effects of natural and human-induced hypoxia on coastal benthos. Biogeosciences 6, 2063–2098. doi: 10.5194/bgd-6-3563-2009
Martin, G. D., Nisha, P. A., Balachandran, K. K., Madhu, N. V., Nair, M., Shaiju, P., et al. (2011). Eutrophication induced changes in benthic community structure of a flow-restricted tropical estuary (Cochin backwaters), India. Environ. Monit. Assess. 176, 427–438. doi: 10.1007/s10661-010-1594-1
Mazumdar, A., Peketi, A., Dewangan, P., Badesab, F., Ramprasad, T., Ramana, M. V., et al. (2009). Shallow gas charged sediments off the Indian west coast: genesis and distribution. Mar. Geol. 267, 71–85.
McAuliffe, C. (1971). GC determination of solutes by multiple phase equilibrations. Chem. Tech. 1, 46–50.
Monteiro, P. M. S., Van Der Plas, A., Mohrholz, V., Mabille, E., Pascall, A., and Joubert, W. (2006). Variability of natural hypoxia and methane in a coastal upwelling system: oceanic physics or shelf biology? Geophys. Res. Lett. 33:L16614. doi: 10.1029/2006GL026234
Naik, R., Naqvi, S. W. A., and Araujo, J. (2017). Anaerobic carbon mineralisation through Sulphate reduction in the inner shelf sediments of Eastern Arabian Sea. Estuar. Coast. 40, 134–144.
Naqvi, S., Naik, H., Jayakumar, A., Pratihary, A. K., Narvenkar, G., Kurian, S., et al. (2009). Seasonal anoxia over the western Indian continental shelf. Geophys. Monogr. 185, 333–345.
Naqvi, S. W. A., and Jayakumar, D. A. (2000). Ocean biogeochemistry and atmospheric composition: significance of the Arabian Sea. Curr. Sci. 78, 289–299.
Naqvi, S. W. A., Bange, H. W., Farías, L., Monteiro, P. M. S., Scranton, M. I., and Zhang, J. (2010). Marine hypoxia/anoxia as a source of CH4 and N2O. Biogeosciences 7, 2159–2190. doi: 10.5194/bg-7-2159-2010
Naqvi, S. W. A., Bange, H. W., Gibb, S. W., Goyet, C., Hatton, A. D., and Upstill-Goddard, R. C. (2005). Biogeochemical ocean-atmosphere transfers in the Arabian Sea. Prog. Oceanogr. 65, 116–144. doi: 10.1016/j.pocean.2005.03.005
Naqvi, S. W. A., Jayakumar, D. A., Narvekar, P. V., Naik, H., Sarma, V. V. S. S., D’Souza, W., et al. (2000). Increased marine production of N2O due to intensifying anoxia on the Indian continental shelf. Nature 408, 346–349. doi: 10.1038/35042551
Naqvi, S. W. A., Naik, H., Jayakumar, D. A., Shailaja, M. S., and Narvekar, P. A. (2006). “Seasonal Oxygen Deficiency over the western continental shelf of India,” in Past Present Water Column Anoxia, ed. L. Neretin (Dordrecht: Springer), 195–224.
Oudot, C., Jean-Baptiste, P., Fourré, E., Mormiche, C., Guevel, M., Ternon, J. F., et al. (2002). Transatlantic equatorial distribution of nitrous oxide and methane. Deep Res. I Oceanogr. Res. Pap. 49, 1175–1193. doi: 10.1016/S0967-0637(02)00019-5
Owens, N. P. J., Law, C. S., Mantoura, R. F. C., Burkill, P. H., and Llewellyn, C. A. (1991). Methane flux to the atmosphere from the Arabian Sea. Nature 354, 293–295.
Parameswaran, U. V., Abdul Jaleel, K. U., Sanjeevan, V. N., Gopal, A., Vijayan, A. K., Gupta, G. V. M., et al. (2018). Diversity and distribution of echinoderms in the South Eastern Arabian Sea shelf under the influence of seasonal hypoxia. Prog. Oceanogr. 165, 189–204. doi: 10.1016/j.pocean.2018.06.005
Patra, P. K., Lal, S., and Venkataramani, S. (1998). Seasonal variability in distribution and fluxes of methane in the Arabian Sea. J. Geophys. Res. 103, 1167–1176.
Pratihary, A. K., Naqvi, S. W. A., Narvenkar, G., Kurian, S., Naik, H., Naik, R., et al. (2014). Benthic mineralization and nutrient exchange over the inner continental shelf of western India. Biogeosciences 11, 2771–2791.
Rao, G. D., and Sarma, V. V. S. S. (2016). Variability in concentrations and fluxes of methane in the Indian Estuaries. Estuar. Coast. 39, 1639–1650. doi: 10.1007/s12237-016-0112-2
Rao, G. D., and Sarma, V. V. S. S. (2017). Influence of river discharge on the distribution and flux of methane in the coastal Bay of Bengal. Mar. Chem. 197, 1–10. doi: 10.1016/j.marchem.2017.11.002
Reeburgh, W. (2007). Oceanic methane biogeochemistry. Chem. Rev. 107, 486–513. doi: 10.1021/cr050362v
Rehder, G., Collier, R. W., Heeschen, K., Kosro, P. M., Barth, J., and Suess, E. (2002). Enhanced marine CH4 emissions to the atmosphere off Oregon caused by coastal upwelling. Glob. Biogeochem. Cycles 16, 2-1–2-11. doi: 10.1029/2000gb001391
Rhee, T. S., Kettle, A. J., and Andreae, M. O. (2009). Methane and nitrous oxide emissions from the ocean: a reassessment using basin-wide observations in the Atlantic. J. Geophys. Res. Atmos. 114:D12304. doi: 10.1029/2008JD011662
Sansone, F. J., Graham, A. W., and Berelson, W. M. (2004). Methane along the western Mexican margin. Limnol. Oceanogr. 49, 2242–2255. doi: 10.4319/lo.2004.49.6.2242
Schunck, H., Lavik, G., Desai, D. K., Großkopf, T., Kalvelage, T., Löscher, C. R., et al. (2013). Giant hydrogen sulfide plume in the oxygen minimum zone off Peru supports Chemolithoautotrophy. PLoS One. 8:e68661. doi: 10.1371/journal.pone.0068661
Shankar, D., Vinayachandran, P., and Unnikrishnan, A. S. (2002). The monsoon currents in the north Indian Ocean. Prog. Oceanog. 52, 63–120. doi: 10.1007/s10661-015-4701-5
Shirodkar, G., Naqvi, S. W. A., Naik, H., Pratihary, A. K., Kurian, S., and Shenoy, D. M. (2018). Methane dynamics in the shelf waters of the West coast of India during seasonal anoxia. Mar. Chem. 203, 55–63. doi: 10.1016/j.marchem.2018.05.001
Steinle, L., Maltby, J., Treude, T., Kock, A., Bange, H. W., Engbersen, N., et al. (2017). Effects of low oxygen concentrations on aerobic methane oxidation in seasonally hypoxic coastal waters. Biogeosciences 14, 1631–1645. doi: 10.5194/bg-14-1631-2017
Sturdivant, S. K., Díaz, R. J., and Cutter, G. R. (2012). Bioturbation in a declining oxygen environment, in situ observations from wormcam. PLoS One 7:e34539. doi: 10.1371/journal.pone.0034539
Sudheesh, V., Gupta, G. V. M., Sudharma, K. V., Naik, H., Shenoy, D. M., Sudhakar, M., et al. (2016). Upwelling intensity modulates N2O concentrations over the western Indian shelf. J. Geophys. Res. Ocean. 121, 8551–8565. doi: 10.1002/2016JC012166
Sudheesh, V., Movitha, M., Hatha, A. A. M., Renjith, K. R., Resmi, P., Rahiman, M., et al. (2017). Effects of seasonal anoxia on the distribution of phosphorus fractions in the surface sediments of southeastern Arabian Sea shelf. Cont. Shelf Res. 150, 57–64. doi: 10.1016/j.csr.2017.09.011
Thottathil, S. D., Balachandran, K. K., Gupta, G. V. M., Madhu, N. V., and Nair, S. (2008). Influence of allochthonous input on autotrophic-heterotrophic switch-over in shallow waters of a tropical estuary (Cochin Estuary). India. Estuar. Coast. Shelf Sci. 78, 551–562. doi: 10.1016/j.ecss.2008.01.018
Tilbrook, B. D., and Karl, D. M. (1995). Methane sources, distributions and sinks from California coastal waters to the oligotrophic North Pacific gyre. Mar. Chem. 49, 51–64. doi: 10.1016/0304-4203(94)00058-L
Upstill-Goddard, R. C., and Barnes, J. (2016). Methane emissions from UK estuaries: re-evaluating the estuarine source of tropospheric methane from Europe. Mar. Chem. 180, 14–23. doi: 10.1016/j.marchem.2016.01.010
Upstill-Goddard, R. C., Barnes, J., and Owens, N. J. P. (1999). Nitrous oxide and methane during the 1994 SW monsoon in the Arabian Sea/northwestern Indian Ocean. J. Geophys. Res. Ocean. 104, 30067–30084. doi: 10.1029/1999jc900232
Verma, A., Subramanian, V., and Ramesh, R. (2002). Methane emissions from a coastal lagoon: Vembanad Lake. West Coast, India. Chemosphere 47, 883–889. doi: 10.1016/s0045-6535(01)00288-0
Wanninkhof, R. (1992). Relationship between wind speed and gas exchange overn the ocean. J. Geophys. Res. 97, 7373–7382. doi: 10.1029/92JC00188
Wanninkhof, R. (2014). Relationship between wind speed and gas exchange over the ocean revisited. Limnol. Oceanogr. 12, 351–362. doi: 10.4319/lom.2014.12.351
Keywords: methane, upwelling, coastal anoxia/hypoxia, anaerobic oxidation, bioturbation, methane flux
Citation: Sudheesh V, Gupta GVM and Naqvi SWA (2020) Massive Methane Loss During Seasonal Hypoxia/Anoxia in the Nearshore Waters of Southeastern Arabian Sea. Front. Mar. Sci. 7:324. doi: 10.3389/fmars.2020.00324
Received: 07 January 2020; Accepted: 21 April 2020;
Published: 15 May 2020.
Edited by:
Xianghui Guo, Xiamen University, ChinaReviewed by:
Guiling Zhang, Ocean University of China, ChinaKunpeng Zang, National Marine Environmental Monitoring Center, China
Copyright © 2020 Sudheesh, Gupta and Naqvi. This is an open-access article distributed under the terms of the Creative Commons Attribution License (CC BY). The use, distribution or reproduction in other forums is permitted, provided the original author(s) and the copyright owner(s) are credited and that the original publication in this journal is cited, in accordance with accepted academic practice. No use, distribution or reproduction is permitted which does not comply with these terms.
*Correspondence: G. V. M. Gupta, Z3ZtZ3VwdGFAZ21haWwuY29t; Z3ZtZ3VwdGFAY21scmUuZ292Lmlu
†Present address: S. W. A. Naqvi, Council of Scientific and Industrial Research, New Delhi, India