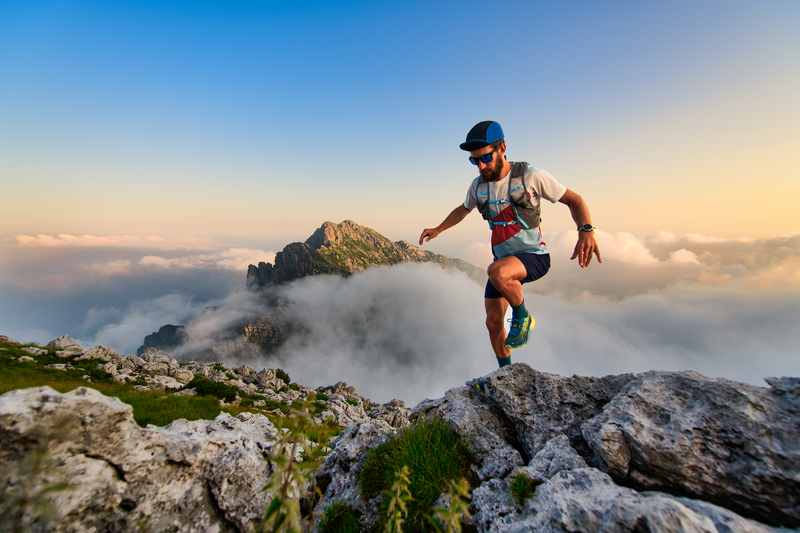
94% of researchers rate our articles as excellent or good
Learn more about the work of our research integrity team to safeguard the quality of each article we publish.
Find out more
ORIGINAL RESEARCH article
Front. Mar. Sci. , 19 May 2020
Sec. Microbial Symbioses
Volume 7 - 2020 | https://doi.org/10.3389/fmars.2020.00266
Marine sponges host highly diverse but specific bacterial communities that provide essential functions for the sponge holobiont, including antimicrobial defense. Here, we characterized the bacterial microbiome of the marine sponge Haliclona cnidata that has been in culture in an artificial marine aquarium system. We tested the hypotheses (1) that the long-term aquarium cultured sponge H. cnidata is tightly associated with a typical sponge bacterial microbiota and (2) that the symbiotic Bacteria sustain bioactivity under harmful environmental conditions to facilitate holobiont survival by preventing pathogen invasion. Microscopic and phylogenetic analyses of the bacterial microbiota revealed that H. cnidata represents a high microbial abundance (HMA) sponge with a temporally stable bacterial community that significantly shifts with changing aquarium conditions. A 4-week incubation experiment was performed in small closed aquarium systems with antibiotic and/or light exclusion treatments to reduce the total bacterial and photosynthetically active sponge-associated microbiota to a treatment-specific resilient community. While the holobiont was severely affected by the experimental treatment (i.e., bleaching of the sponge, reduced bacterial abundance, shifted bacterial community composition), the biological defense and bacterial community interactions (i.e., quorum sensing activity) remained intact. 16S rRNA gene amplicon sequencing revealed a resilient community of 105 bacterial taxa, which remained in the treated sponges. These 105 taxa accounted for a relative abundance of 72–83% of the bacterial sponge microbiota of non-treated sponge fragments that have been cultured under the same conditions. We conclude that a sponge-specific resilient community stays biologically active under harmful environmental conditions, facilitating the resilience of the holobiont. In H. cnidata, bacteria are located in bacteriocytes, which may have contributed to the observed phenomenon.
Sponges (phylum Porifera) occur in marine habitats as significant members of benthic environments (Bell and Smith, 2004; Bell, 2008). As sessile filter-feeders, sponges are permanently exposed to microorganisms from their surrounding aquatic environment, including opportunistic and pathogenic bacteria. However, sponges also host complex, sponge-specific, and evolutionary adapted microbial consortia, which form a functional unit with the host – the sponge holobiont (Rohwer et al., 2002; Rosenberg et al., 2007; Webster and Taylor, 2012; Youle et al., 2013; Bordenstein and Theis, 2015; Webster and Thomas, 2016; Thomas et al., 2016). The sponge microbiome represents a high metabolic diversity and facilitates various, highly efficient nutrient cycles of sponges (Zhang et al., 2019). For example, heterotrophic symbionts have a high impact on the dissolved organic carbon (DOC) uptake and breakdown of carbon. Phototrophic symbionts like Cyanobacteria and zooxanthellae provide further carbon sources to their host and can have beneficial effects on the sponge growth (Erwin and Thacker, 2008; Weisz et al., 2010). Moreover, associated bacteria may most likely protect the holobiont efficiently against invading, potentially pathogenic bacteria via antimicrobial defense (Taylor et al., 2007; Webster and Thomas, 2016).
Based on abundance and diversity of the sponge-associated bacterial assemblages, sponges were divided in two groups (Hentschel et al., 2003, 2006; Gloeckner et al., 2014; Webster and Thomas, 2016): high microbial abundance (HMA) sponges, which harbor abundant and diverse bacterial communities, and low microbial abundance (LMA) sponges, which feature a reduced community diversity and host a low bacterial load similar to the concentrations of the ambient water. Noteworthy, some sponges have been reported that could not be assigned to either group (e.g., Thomas et al., 2016). Distinct microbial community structures were observed for HMA and LMA sponges from the operational taxonomic unit (OTU) and genera to the phylum level (Moitinho-Silva et al., 2017b). A general host specificity of sponge microbiomes at higher taxonomic ranks was more recently studied in detail by Steinert et al. (2017). So far, representatives of more than 60 bacterial phyla, including a large proportion of candidate phyla, were recovered from a broad range of marine sponges (Moitinho-Silva et al., 2017a). This exceptionally high bacterial diversity is unmatched among invertebrate hosts and ranges from dominant to rare taxa (Reveillaud et al., 2014; Thomas et al., 2016).
Sponge-bacteria associations are predominantly stable (Thiel et al., 2007; Pita et al., 2013; Reveillaud et al., 2014), even in long-term cultures of sponges in artificial aquarium systems (Friedrich et al., 2001; Thoms et al., 2003; Gerçe et al., 2009; Bergman et al., 2011). However, it has been previously shown that changing environmental conditions can affect the composition of sponge-associated microbiota (Webster et al., 2010; Fan et al., 2013). Moreover, the stability of the sponge microbiota varies among different host species and may depend on the applied cultivation conditions (Webster and Taylor, 2012). Yet, a large proportion of sponge-associated bacteria remains unclassified from the species up to the phylum level (Moitinho-Silva et al., 2017b). Most of the tightly sponge-associated bacterial taxa represent distinct phylogenetic clusters, which were defined as sponge- (SSC) and sponge-coral-specific (SCS) (e.g., Hentschel et al., 2002; Simister et al., 2012; Taylor et al., 2013). As members of SSCs were also detected in lower abundance in ambient seawater and sediment samples, the term “sponge-enriched specific clusters” was established (Webster and Taylor, 2012; Webster and Thomas, 2016; Thomas et al., 2016). Moitinho-Silva et al. (2014) showed for both, a HMA and a LMA sponge, that typical sponge symbionts had a low transcriptional activity in seawater. This indicated that sponge symbionts are inactive outside the host. The comprehensive global microbiome study of the Porifera performed by Thomas et al. (2016) revealed that the sponge microbiome exhibited little commonality in species composition. Despite the predominance of generalists and specialists among such communities, the specialists were underrepresented in the core symbiont communities. These findings led Thomas et al. (2016) to the conclusion that symbiotic sponge microbiota developed independently within different members of the Porifera, but a convergent force resulted in analogous community organization and interaction. The close interaction of the host and associated bacteria is still not fully understood and several mechanisms may be responsible for the high stability and specificity of such interactions, e.g., the presence of eukaryotic-like proteins coded in the genomes of microbial symbionts (Reynolds and Thomas, 2016). Furthermore, it has been shown that the genomes of bacterial sponge symbionts are enriched with genes associated with bacterial defense, host colonization, and extracellular matrix utilization, as well as nutritional specialization, which can be regarded as a consequence of evolutionary adaptation of symbionts to the host environment (Slaby et al., 2017). Several studies revealed the production of a variety of bioactive compounds by symbionts including those applied for quorum sensing (QS) and antimicrobial defense, which often form the basis for pathogen defense systems (Bewley et al., 1996; Paul and Puglisi, 2004; Hentschel et al., 2006; Taylor et al., 2007; Thomas et al., 2016). The antimicrobial defense was likely one of the key elements in the evolutionary selectivity of sponge-associated microbial communities and thus their resilience against pathogens (Kelman et al., 2001; Rohde et al., 2012). In this context, QS may play an important role in shaping microbial community composition and interactions, and controlling the biological activity of the sponge microbiota. However, the linkage between the functionality of the sponge microbiota and the specificity (dominant vs. rare members), diversity, abundance, and location of symbionts within the sponge matrix remains little studied. Moreover, it is largely unknown whether the sponge contributes to the protection of the associated bacterial consortia.
Here we hypothesized (1) that the long-term aquarium cultured sponge H. cnidata is tightly associated with a typical sponge bacterial microbiota and (2) that the symbiotic Bacteria sustain bioactivity under harmful environmental conditions, facilitating holobiont survival by preventing pathogen invasion. To test these hypotheses, first the bacterial community of the healthy sponge was characterized in terms of abundance, diversity, and location in the sponge matrix. Second, detrimental conditions were simulated via antibiotic exposure and/or light exclusion in a 29 days lasting aquarium experiment to remove transient and non-resilient members of the bacterial microbiota. Finally, the bioactivity (i.e., antimicrobial defense and QS activity) of the affected holobiont was assessed with regard to potential agents among the resilient bacterial community.
Experiments were performed with the marine sponge Haliclona cnidata (Schellenberg et al., 2019), which has been in long-term culture at the Justus Liebig University Giessen (Giessen, Germany) since 2000. The model organism has been selected to assess bioactivity-based defense due to clear indication for a pronounced bioactivity (unpublished data). The natural distribution of H. cnidata is unknown (Schellenberg et al., 2019), hence material was sampled from a tropical marine aquarium (4000 L closed water circulating tank system, a constant water temperature of 26°C, 10:14 light:dark photoperiod, salinity of 32–33%). Water currents were created using a wavebox (10,000 L/h; Tunze, Penzberg, Germany) with a water flow through of 2000 L/h. Water parameters are given as Supplementary Table S1.
Sponge-associated bacterial communities were first determined microscopically from fixed and partially maturated sponge fragments using different microscopical techniques including SybrGreen I (SG-I) based cell staining, catalyzed reporter deposition fluorescence in-situ hybridization (CARD-FISH) with a mixture of universal Bacteria 16S rRNA probes, and by transmission electron microscopy (TEM) analysis. Details of the analyses are described as Supplementary Material and Methods.
To reduce the associated microbiota to a resilient community, sponge specimens were exposed to antibiotics (ampicillin, gentamicin) to remove most of the host-associated bacterial symbionts and dark incubated to reduce the phototrophic symbionts. Four treatments were performed over 29 days: control (LN), exposure to antibiotics (LA), dark incubation (DN), and dark incubation combined with antibiotic exposure (DA) (Supplementary Figure S1). Antibiotic treatments contained two antibiotics to affect Gram-positive and Gram-negative bacteria, the ß-lactam antibiotic ampicillin (ampicillin sodium salt >99%) and the aminoglycoside antibiotic gentamicin (gentamycin sulfate >590 I.E. mg−1) in a concentration of 100 mg L−1 each (both Carl Roth, Karlsruhe, Germany) (according to Friedrich et al., 2001). Three non-connected 12-liter tanks containing three sponge fragments each (independent biological replicates) were used per treatment (Supplementary Figure S1). Each tank was equipped with a submersible 10 W heater (NWO-10P, Aquarium Systems, Sarrebourg, France), and an air stone for water movement and oxygenation. Water parameters were monitored regularly (Supplementary Table S1). The water temperature was set to 26°C. For acclimation, sponge fragments were placed in the tanks 14 days prior to the start of the experiment with the tanks connected to the closed-circulating water system. For the experiment, all tanks were disconnected from the closed-circulating water system to prevent the distribution of antibiotics. Water movement and oxygenation were maintained via air stones and fresh water supply was assured through manual water exchange (8 L day−1) including a replacement of the antibiotics, respectively. Sponges were sampled right before the start of the experiment (t0, sponge 2012), after 17 (t17), and after 29 days (t29) of the experiment. Samples of 1–2 cm were cut with a sterile razor blade from the growing tip of the individual sponge fragments. Cut sponge fragments were dipped off gently on sterile paper and stored directly at −20°C for subsequent bacterial abundance and community composition, or bioactivity analyses. Further details are provided as Supplementary Information.
Sponge fragments (∼100 mg per sponge individual) were washed with autoclaved and 0.2 μm-filtered ambient water (AW) obtained from the studied marine aquaria system and stored at −20°C. In 2015, water-associated bacteria were collected before sponge sampling from 1 L surrounding aquarium water of three locations of the tank on 0.22 μm SterivexTM-GP filter units (Merck Millipore, Burlington, United States). Remaining water was fully removed from the cartridges and filter units were frozen in liquid nitrogen. Frozen plastic cartridges of the filter units were broken by mechanical treatment and filters subjected to DNA extraction. Total DNA was extracted from sponge samples and filters using the Gen Elute Plant Genomic DNA Kit (Sigma-Aldrich, St. Louis, United States). All samples were homogenized by bead-beating with 1 g of silica beads in the lysis solution of the kit using a Retsch mill (MM2; Retsch GmbH, Haan, Germany) for 1 min at maximum speed. Subsequent DNA extraction was performed according to the manufacturer’s protocol using two elution steps with 100 μL elution solution each and stored at −20°C.
The concentration of sponge-associated Bacteria was assessed by quantitative real-time PCR (qPCR). Subsequently, the number of bacterial 16S rRNA gene targets was set in relation to the number of mitochondrial cytochrome oxidase I (COI) gene targets of the sponge. Analysis was performed in a CFX96 Touch cycler (Bio-Rad, Munich, Germany) using the SsoFastTM EvaGreen Supermix (Bio-Rad). All qPCRs were performed in a total volume of 10 μL including 1x Sso Fast EVA Green reaction mixture, primer system specific primer concentrations, and 1 μL template DNA. Bacterial 16S rRNA gene targets were amplified with 0.2 μM (each) of primers Universal-F (5′-GTGSTGCAYGGYTGTCGTCA-3′) and Universal-R (5′-CCCCTCKGSAAAGCCTTCTTC-3′) (Maeda et al., 2003). PCR conditions were: 98°C for 2 min, followed by 35 cycles of 98°C for 20 s, 60°C for 20 s, and 72°C for 20 s. QPCR analysis of the COI gene was performed with 0.5 μM (each) of primers LCO1490 (5′-GGTCAACAAATCATAAAGATATTGG-3′) and HCO2198 (5′-TAAACTTCAGGGTGACCAAAAAATCA-3′) (Folmer et al., 1994). PCR conditions were: 98°C for 2 min, 37 cycles of 94°C for 40 s, 42°C for 40 s, and 72°C for 60 s. Plate reads during qPCRs were taken at the end of each cycle. Melting curve analyses by subsequent heating from 65 to 95°C with an increase of 0.5°C each 5 s confirmed the amplification of specific targets. The size of the PCR products was checked by 1.7% (w/v) agarose gel electrophoresis performed in 1x TBE buffer using the 100 bp DNA ladder plus (Thermo Fischer Scientific) to determine the product size. A serial dilution of known target numbers of 16S rRNA gene fragments amplified from genomic DNA of Citrobacter freundii ATCC 8090T was used to generate a standard curve and to calculate the efficiency of the qPCR. Bacterial 16S rRNA gene fragments for qPCR standards were amplified in a total volume of 100 μL with 1 μL template DNA and the primers 8F (5′-AGAGTTTGATCCTGCTCAG-3′; Edwards et al., 1989) and 1492R (5’-GGTTACCTTGTTACGACTT-3′; Stackebrandt and Liesack, 1993) using standard conditions (Schauss et al., 2015). PCR products were purified using the QIAquick PCR Purification Kit (Qiagen, Hilden, Germany) and DNA quantity was measured with a NanoDropTM 2000 spectrophotometer (Thermo Fisher Scientific, Waltham, United States). The concentration of 16S rRNA gene copies was calculated according to Kolb et al. (2003). Samples and corresponding standards were run in technical duplicates. Gene copy numbers and qPCR efficiency were calculated using the Bio-Rad CFX Manager software (version 3.0). Efficiencies of qPCRs were 102.7% (R2 = 0.969) for primer system Universal-F/Universal-R and 111.8% (R2 = 0.990) for LCO1490/HCO2198, respectively. Relative changes of bacterial gene copy numbers were calculated according to the 2−ΔΔCT method (Livak and Schmittgen, 2001). The COI gene of the sponge was used as an internal control. Standard deviations were calculated by the standard propagation of error method. QPCR data were subjected to Kruskal-Wallis One Way Analysis of Variance (ANOVA) on Ranks and a post hoc Tukey test in SigmaPlot (version 12.0, Systat Software Inc., San Jose, CA, United States).
Sponge- and water-associated bacterial communities were analyzed including independent biological replicates by denaturing gradient gel electrophoresis (DGGE) based bacterial community fingerprinting. Specifically, the sponge microbiota was assessed in 2012 (“Sponge 2012”) and 2015 (“Sponge 2015”). Universal bacterial 16S rRNA gene targeting primers GC339F and 907R (Muyzer et al., 1993) were used for PCR amplification of 16S rRNA gene fragments from the total DNA extracts of individual sponge fragments and water samples according to Glaeser et al. (2010). PCRs were performed in a total volume of 50 μL including 1x DreamTaq buffer, 0.1 μM of each dNTPs, 0.4 μM of each primer, 0.4 mg mL−1 BSA, 0.02 U μL−1 DreamTaq DNA polymerase (all chemicals from Thermo Fisher Scientific, formerly Fermentas), and 1 μL undiluted DNA extract. PCR cycling conditions were: 95°C for 3 min, followed by 32 cycles of 95°C for 30 s, 55°C for 30 s, and 72°C for 40 s, and finally 72°C for 30 min. DGGE analysis was performed as described in detail by Glaeser et al. (2010, 2014) using an Ingeny PhorU system model IPU-S (Ingeny International BV, GP Goes, the Netherlands) and ethidium bromide staining of DGGE gels. DGGE based community fingerprint patterns were analyzed in GelCompar II (version 4.5, Applied Maths, Sint Martens Latem, Belgium) after image pre-processing in Photoshop CS6.0 (Adobe, San Jose, United States). Presence, absence, and relative abundance of DNA bands were accounted using the Pearson similarity coefficient to generate similarity matrices. The band matching tool in GelCompar II was applied to export relative abundance values of DNA band patterns for statistical analysis in PAST (version 3.11). Relative abundance patterns of DNA bands (based on DNA band intensities) were used for community comparison using PAST, respectively. Non-metric multidimensional scaling (NMDS) ordination analyses and hierarchical clustering were performed based on distance matrices generated with the Bray-Curtis dissimilarity coefficient. Bacterial profiles of the sponge and water communities were tested for significant differences by two-sample Kolmogorov-Smirnov tests in R Studio version 1.2.1335 (R Studio Team 2018). Differences among the bacterial community profiles of the resilient community experiment (including three biological replicates per sample) were tested for significance by one-way nested analysis of similarity (ANOSIM; strata = time) applying Bray-Curtis distances calculations in R (version 3.2.0 statistical environment; R Studio Team 2018) with the “Vegan” R package version 2.3-1 (Oksanen et al., 2015).
For identification, DGGE bands were either directly sequenced or cloned before sequence analysis. Briefly, cloning was performed by re-amplification of excised DNA bands with primers 339F and 907R using the CloneJET PCR cloning kit (Thermo Fisher Scientific) according to manufacturer’s protocol and competent DH5α cells (Bioline, London, United Kingdom) for transformation. After verifying the melting behavior of re-amplified cloned DNA fragments by DGGE in comparison to the environmental samples, cloned DNA fragments were re-amplified from E. coli clones using primers pJET1.2F and pJET1.2R (Thermo Fisher Scientific, formerly Fermentas) and sequenced with the Sanger technology by LGC genomics using one of the pJET vector primers. DGGE band derived sequences are available under GenBank/EMBL/DDBJ accession numbers MH045531 – MH045540.
Pooled replicates of sponge and water DNA extracts were analyzed by paired-end 16S rRNA gene amplicon high-throughput sequencing using an Illumina MiSeq V3 system. 16S rRNA gene fragments were PCR amplified with primers 341F and 785R (cited as S-D-Bact-0341-b-S-17/S-D-Bact-0785-a-A-21 by Klindworth et al., 2013) and 300 bp paired-end read sequencing was performed by LGC Genomics (Berlin, Germany). The Illumina bcl2fastq 1.8.4 software was used for demultiplexing of all libraries and reads were sorted by amplicon inline barcodes (allowing one barcode mismatch; discarding reads with missing, one-sided, or conflicting barcodes). After clipping sequence adaptors, reads with a length <100 bp were discarded and primers (allowing 3 mismatches) were used for sequence orientation. Forward and reverse reads were combined using BBMerge (version 34.48)1. The combined read pair data set was used for further analysis. FASTQC files were converted to fasta files using the fastq.info program as implemented on the Galaxy platform2 and fasta files were submitted to the NGS analysis pipeline of the SILVA rRNA gene database project (SILVAngs 1.3) (Quast et al., 2013). The SILVA Incremental Aligner [SINA v1.2.10 for ARB SVN (revision 21008)] (Pruesse et al., 2012) was used for read alignment against the SILVA SSU rRNA SEED and quality controlled (Quast et al., 2013). Subsequent processing omitted reads that were shorter than 50 aligned nucleotides or showed either more than 2% of ambiguities, 2% of homopolymers, or putative contaminations and artifacts that were seen as reads with a low alignment quality (50 alignment identity, 40 alignment score reported by SINA), respectively. Quality control continued with dereplication and clustering of unique reads as OTUs on a per sample basis using cd-hit-est (version 3.1.2)3 (Li and Godzik, 2006). The tool was run in accurate mode, ignoring overhangs and applying identity criteria of 1.00 and 0.98, respectively. Classification of the reference read of each OTU was performed with blastn (version 2.2.28+)4 with standard settings (Camacho et al., 2009) against the non-redundant version of the SILVA SSU Ref dataset (release 128)5. Each classified OTU reference read was mapped onto all reads that were assigned to the respective OTU to yield information about the number of individual reads per taxonomic path within the limitations of both multiple rRNA operons and PCR and sequencing technique biases. Reads remained unclassified and were assigned to the meta group “no relative” if they lacked BLAST hits or showed weak BLAST hits [defined as a minimum of 93 for function (% sequence identity + % alignment coverage)/2]. This method was established by Klindworth et al. (2013) and Ionescu et al. (2012). Raw data of paired-end sequence read are available at the Sequence Read Archive (SRA) under BioSample accessions number SAMN08957770-SAMN08957776 within the BioProject PRJNA451083.
Sequences assigned as Archaea, chloroplast, mitochondria, or “no relative” were omitted from the analysis (1.3 and 0.3% of the total sequences obtained for sponge 2012 and 2015 and 7.4% for the water sample; Supplementary Table S2). Bacterial communities were compared at the level of relative abundances of bacterial phyla and phylogenetic groups (i.e., taxonomic paths to the lowest taxonomic rank not exceeding the genus rank). Diversity indices were calculated based on the absolute sequences assigned to phylogenetic groups using PAST (version 3.11). Principal component analysis (PCA) and SIMPER analysis was performed to determine phyla or individual phylogenetic groups that contributed most to the community dissimilarity between samples. Venn diagrams showing presence and absence of phylogenetic groups were generated using the online tool of Bioinformatics and Evolutionary Genomics6.
Bioactivity of holobiont-derived compounds was analyzed for individual sponge fragments of the four different experimental treatments at t29 by high-performance thin-layer chromatography (HPTLC) coupled to a bioluminescence assay according to Klöppel et al. (2008). In this assay, extracts are coated with a bioluminescent bacterial strain (Aliivibrio fischeri) and bioactivity of the separated compounds inhibit or enhance the bacterial luminescence on the plate, which facilitates detection of antimicrobial compounds and QS signals. Briefly, 1 cm of sponge fragments (of one individual colony per treatment) were frozen for 24 h at −20°C and subsequently lyophilized and grounded with a mortar and pestle. Bioactive compounds were extracted in methanol (2 mL per 20 mg sponge fragment) on a magnetic stirrer (250 min−1) for 24 h in a cooling chamber at 4 °C. Samples were centrifuged at 6,000 × g for 4 min to remove tissue and cell remnants and 14 μL of the crude extract was applied to a HPTLC silica gel 60 F254 plate (Merck, Darmstadt, Germany) as 4 mm bands using the Automatic TLC Sampler 4 (ATS 4; CAMAG, Muttenz, Switzerland) and dried in a stream of warm air for 1 min. The plates was developed in an Automated Multiple Developing System 2 (AMD2; CAMAG) with a fifteen-step gradient based on methanol, dichloromethane, and n-hexane. The plate was dipped automatically by the TLC Chromatogram Immersion Device (CAMAG) into a Aliivibrio fischeri DSM 7151 suspension. Thereafter, the plate was kept moist and covered with a glass plate to prevent dehydration. Enhancement and inhibition of bioluminescence appeared as brightened and darkened zones, respectively, by the bioluminescence measurement (Klöppel et al., 2008). Changes in the bioluminescence signal were documented every second minute over 38 min by the BioLuminizer (CAMAG). Patterns of bioactivity were analyzed in GelCompar II after image pre-processing in Photoshop CS6.0. Bioactivity zone areas were accounted by the application of the Pearson similarity coefficient to generate similarity matrices for Unweighted Pair Group Method with Arithmetic Mean (UPGMA) cluster analysis.
The bacterial consortium associated with H. cnidata was first characterized with respect to the bacterial load of the long-term cultured sponge. Counting of SG-I stained bacterial cells in maturated sponge fragments resulted in a mean concentration of 4.1 (± 1.9) × 1010 bacterial cells per g sponge wet weight (mean of three independent replicates). Epifluorescence microscopy of SG-I (Figure 1A) and CARD-FISH (Figures 1B,C) stained sponge-associated bacterial assemblages showed aggregates of morphologically diverse bacterial cells. Among those, large rod-shaped, morphologically identical autofluorescent cells occurred, indicating a high abundance of phototrophic bacteria. High amounts of filamentous cells were visualized in aggregates after SG-I staining, but went undetected in CARD-FISH analysis with the universal Bacteria 16S rRNA targeting probes. Transmission electron microscopy showed a low number of free bacterial cells in the extracellular sponge matrix (mesohyl) (Figure 2A), while morphologically diverse bacterial consortia were localized in interconnected vacuole-like structures of amoeboid host cells (Figure 2B). Those bacteria-containing sponge cells were previously reported as bacteriocytes (Vacelet and Donadey, 1977). In H. cnidata, bacteriocytes were found evenly distributed within the mesohyl. Coccoid cells with ring-like structures indicated the presence of thylakoid membrane containing phototrophic bacteria, most likely Cyanobacteria, within the vacuoles of bacteriocytes (Figure 2B) and narrow cells cut in length in bacteriocytes (Figure 2B) may represent the filamentous cells shown by SG-I staining (Figure 1A).
Figure 1. Epifluorescence microscopy of bacterial assemblages derived from maturated sponge fragments of the studied Haliclona cnidata. Composite images of SybrGreen-I (SG-I) and CARD-FISH stained sponge-associated bacterial assemblages present in maturated sponge tissue, green: SG-I or probe signal; red: autofluorescence. SG-I stained bacterial cells of washed and maturated sponge fragments diluted to 10−2 for quantification (A). Single and composite CARD-FISH images after hybridization with the universal Bacteria probe EUB338 I-III mix (B) or a non-binding probe non-EUB (C) conjugated with Alexa Fluor488. DAPI (blue) was used for counterstaining. Other colors refer to autofluorescence. Auto-fluorescence cells (red) indicate the presence of phototrophs. Those cells had a mean size of 1.8 (± 0.6) μm × 1.0 (± 0.3) μm (length x width) and were larger than the majority of the visualized green-fluorescent (heterotrophic) bacterial cells, which had a mean size of 1.5 (± 0.5) μm × 0.5 (± 0.2) μm. Scale bars 5 μm each.
Figure 2. Localization of bacteria in the studied Haliclona cnidata. Transmission electron microscopy (TEM) images of sponge fragments revealed a low number of free bacterial cells in the mesohyl of the sponge (A, scale bar 2 μm) but abundant interconnected vacuole-like structures (indicated by asterisks) of amoeboid host cells (bacteriocytes) comprising morphologically diverse bacterial cells (B, scale bar 1 μm). bc, bacterial cells; cy, potentially spiral thylakoid membranes of a coccoid cyanobacterium.
The stability, diversity, and phylogenetic composition of the sponge-associated microbiota were further characterized by 16S rRNA gene sequence-based approaches. At least three individual sponge fragments were collected in 2012 and 2015 from different parts of the same aquarium system. In 2015, the bacterial assemblage of the surrounding water was studied in parallel. Bacterial community fingerprinting by 16S rRNA gene-based PCR-DGGE analysis showed three characteristics of the sponge-associated bacterial microbiota (Figures 3A,B). First, sponge fragments sampled from different locations in the aquaria system at the same time (both in 2012 and 2015) had identical DGGE fingerprint patterns, indicating the presence of a stable, abundant bacterial community associated with the sponge. Second, the comparison of DGGE patterns obtained from 2012 and 2015 collected sponge fragments revealed no significant differences (Kolmogorov-Smirnov test, Bonferroni corrected p-value; p > 0.05; Supplementary Table S3), indicating the presence of a temporally stable microbiota of the sponge in the marine aquarium system (Figure 3). Minor dissimilarities between the samples were caused by different intensities of a few abundant DNA bands (representing the most abundant bacterial community members). Third, the sponge-associated bacterial assemblages were significantly different (Kolmogorov–Smirnov test, Bonferroni corrected p-value; p < 0.05; Supplementary Table S3) from the bacterial assemblages present in the surrounding water (only shown for 2015; Figures 3A,B). The sponge and water-associated bacterial community patterns differed regarding the presence of abundant DNA bands and the total number of DNA bands. Sponge samples were characterized by a larger number of highly abundant DNA bands, while water samples featured many faint DNA bands indicated by the smear in the DGGE gel (Figure 3B). Neither the sponge nor the water-associated bacterial assemblages showed a high variability among replicates (Figures 3A,B). For the DGGE patterns of sponge samples, most abundant DNA bands represented typical sponge symbionts as indicated by high 16S rRNA gene sequence similarities to cloned 16S rRNA gene fragments of marine sponges including Proteobacteria next related to the genera Roseovarius, Thioprofundimonas, Lutimaribacter, and Thalassobaculum, Bacteroidetes next related to Ownenweeksia and Rhodothermus, and an Acidobacterium next related to Paludibaculum as well as Cyanobacteria next related to Prochlorococcus species (Figure 3B and Table 1).
Table 1. Phylogenetic assignment of DNA bands from DGGE based community fingerprinting of the bacterial microbiota of the studied sponge Haliclona cnidata.
Figure 3. 16S rRNA gene sequence-based characterization of the specificity, stability, and diversity of the bacterial consortia associated with the studied Haliclona cnidata. Bacterial assemblages associated with the sponge and ambient water were investigated by PCR-DGGE community fingerprinting. (A) NMDS plot based on a Bray-Curtis dissimilarity matrix generated from relative abundance patterns of DNA bands in community fingerprint patterns. (B) Cluster analysis of DGGE community fingerprint patterns. Clustering was performed in GelCompar II with the UPGMA method based on a Pearson correlation matrix considering DNA band presence/absence and intensity. For each sample (Sponge 2012, Sponge 2015, Water 2015) denotation of biological replicate is given. DNA bands B01 to B10 in (B) refer to identified DNA bands representing most abundant taxa of the bacterial sponge microbiota (Table 1).
To assess the diversity and phylogenetic composition of the sponge (2012, 2105) and water (2015) associated bacterial communities on a higher taxonomic resolution, pooled replicates that showed identical DGGE patterns were subjected to 16S rRNA gene amplicon Illumina MiSeq sequencing. Total numbers of 89,577 (sponge 2012), 56,738 (sponge 2015), and 12,640 (water 2015) trimmed high quality paired-end 16S rRNA gene sequences identified as Bacteria were obtained (Supplementary Table S2). Analysis of the pooled DNA samples of biological replicates indicated that the richness of sponge-associated bacterial assemblages was much lower than that of the water-associated bacterial assemblages (lower richness and Chao1-bc index values) (Supplementary Figure S2A). A total of 134 (2015) and 275 (2012) phylogenetic groups were detected in sponge samples, while twice as much phylogenetic groups (n = 578) were detected in ambient water samples (Supplementary Table S4). Phylogenetic groups were less evenly distributed in the sponge than in water-associated bacterial assemblages (lower evenness index values in sponge samples; Supplementary Figure S2A) with few abundant phylogenetic groups in the sponge samples (higher dominance values), while many phylogenetic groups occurred with an equally low abundance in the water sample. This also confirmed the observation obtained by DGGE (see above). The higher diversity of the water-associated bacterial assemblages compared to the less diverse bacterial assemblages of the sponge samples was also illustrated by rarefaction curves (Supplementary Figure S2A), where the number of sequences was plotted against rarefied numbers of phylogenetic groups.
Sponge-associated bacteria were assigned to 27 phyla including several Candidatus phyla (Figure 4A and Supplementary Table S5). The sponge microbiome was dominated by Proteobacteria (relative abundance of 46–49%), followed by Acidobacteria (9–17%), Verrucomicrobia (10–11%), Gemmatiomonadetes (7–14%), Bacteroidetes (9%), Chloroflexi (4–5%), and Actinobacteria and Cyanobacteria (both 3%). Among Proteobacteria, Alphaproteobacteria (14–19%), Gammaproteobacteria (13–21%), the uncultured JTB23 group (2–14%), and Deltaproteobacteria (4–7%) were most abundant (Figure 4A). The high relative abundance of Acidobacteria, Verrucomicrobia, Gemmatiomonadetes, and Chloroflexi in sponge samples mainly contributed to the specificity of the sponge-associated bacterial microbiome, while Actinobacteria and Planctomycetes mainly accounted for the specificity of the water-associated bacterial community as indicated by principle component analysis (PCA) (Supplementary Figure S3).
Figure 4. Phylogenetic composition of the bacterial Haliclona cnidata microbiome analysed based on 16S rRNA gene amplicon sequence data. Phylogenetic analysis of sponge and water-associated bacterial communities: (A) Relative abundance pattern of phyla present in sponge-fragment samples in 2012 and 2015 and water samples collected in 2015. (B) Ternary plots showing the distribution of phylogenetic groups among the sponge and water samples. Only taxa with a relative abundance ≥1% were considered. Each circle represents one taxon labeled with a taxon-specific tax-ID. (C) Phylogenetic assignment and relative abundance (presented as heat map) values of respective taxa in individual samples. Corresponding DNA bands (Figure 3B) are shown if applicable.
In total, 340 different phylogenetic groups were detected in the sponge, among those 23 phylogenetic groups that occurred with a relative abundance of at least 1% in one of the samples (Supplementary Table S6). Most of those phylogenetic groups (21 out of 23) occurred in the sponge collected in 2012 and 2015 and comprised phylogenetic groups that represented the abundant DNA bands in the DGGE profiles (Figure 4C). Ternary plots based on phylogenetic groups with a relative abundance of ≥ 1%, visualized the clear differences in the presence of the phylogenetic group in the sponge vs. water samples (Figures 4B,C). The most abundant (>10% relative abundance in at least one sponge sample) phylogenetic groups in the sponge-microbiome were uncultured bacteria of the JTB23 group (T1; Proteobacteria), the BD2-11 terrestrial group (T2; Gemmatimonadetes), the OPB35 soil group (T3; Verrucomicrobia), and PAUC26f (T4; DNA band B04; Acidobacteria) (Figure 4C). Additional phylogenetic groups with a moderate relative abundance (3–7%) in the sponge samples were all sponge-specific (i.e., undetected in water samples) and comprised the genera Pseudoruegeria (T5; DNA band B08; Alphaproteobacteria), Owenweeksia (T6; DNA band B02; Bacteroidetes), Pseudospirillum (T7; Gammaproteobacteria) as well as phylogenetic groups representing uncultured taxa, such as clades KI89A (T10; Gammaproteobacteria), Sh765B-TzT-29 (T9; Deltaproteobacteria), and SAR202 (T12; Chloroflexi).
To impair transient and non-resilient members of the bacterial microbiota, sponge fragments were exposed to antibiotics (ampicillin/gentamicin) and/or grew under light exclusion for a period of 29 days (Supplementary Figures S1A,B). Three treatments with three independent replicates each were performed, light and antibiotics, LA; dark and no antibiotics, DN; dark and antibiotics, DA) and compared to untreated controls (LN). The treatments led to a color change of the sponge compared to its native dark gray to purple color shown under control conditions (normal light conditions, LN) to weak brown and pale (Figure 5A). These changes were observed in all three replicate tanks. The tips of the erected fingers of the sponge started to bleach after four days (t4). After eight days (t8), sponges of the antibiotic light treatment (LA) were completely white and first signs of fouling occurred in all antibiotic treatments (LA, DA). After 17 days of treatment (t17), the color of sponges had changed to weak brown under light exclusion (DN and DA), while the tips appeared pale and the fouling had intensified for the white fragments in antibiotic light treatments (LA). After 29 days (t29), at the end of the experiment, sponges of the dark treatments had completely lost their color and the fouling in antibiotic light treatments had proceeded. For individuals of the antibiotic dark treatment (DA), no changes could be observed in comparison to t17. Sponges of the control aquaria (LN) showed no morphological changes during the experiment.
Figure 5. Morphological changes of Haliclona cnidata fragments and bioactivity screening after exposure to antibiotics and light depletion. Stressor specific morphological changes (A) were observed for sponge fragments over time being exposed to either antibiotics in light (LA), dark incubation without antibiotics (DN) or antibiotics plus dark incubation (DA) compared to normal light conditions (LN). (B) After 29 days (t29), methanolic extracts of the sponge were comparatively screened for bioactivity by HPTLC coupled with the A. fischeri bioluminescence assay. (C,D) Bioluminescence intensity was measured after 2 min. Bar chart showing relative contribution (%) of enhancement (similarity, Sim. [E], brightened zones, white bar) and inhibition (Sim. [I], darkened zones, black bar) to overall luminescence intensity (Lum. Int.) per sample. Relative intensity patterns of A. fischeri bioactive zones were subjected to Pearson correlation-based cluster analysis. Respective analysis was performed in GelCompar II.
The biological activity of the individual sponge fragments was assessed by the A. fischeri based bioactivity screening (Klöppel et al., 2008, 2013; Kirchhoff et al., 2014). Following sponge symbiont manipulating stress conditions, methanolic extracts of sponge fragments were analyzed at t29. Bioluminescence of A. fischeri was monitored after the extracted compounds were separated by HPTLC (Figure 5B). Here, A. fischeri was used as a proxy for a pathogenic, invading bacterium as the fish-pathogen had been previously applied to determine the antimicrobial activity of sponge symbionts (Graça et al., 2013). The bioactivity profiles obtained from sponges of the antibiotic and/or light exclusion treatments (LA, DN, and DA), indicated a residual antimicrobial activity profile with only few changes (up/down regulation of bioactive substances produced) compared to the bioactivity profile obtained for the control (LN). One prominent, broad inhibiting compound zone (in the middle of each profile at hRF 45) and four minor inhibiting zones were evident. In addition, eight zones with enhanced bioluminescence were detected in all treatments. Compared to LN, slight differences were observed for sponge fragments of the stress treatments. Differences were mostly due to the relative contribution of QS enhancement to the total bioactivity, which slightly increased in the stress treatments (Figure 5C). However, the absolute values for luminescent inhibition were similar among treatments. For both, antimicrobial and QS activity patterns, bioactivity was more similar among the two antibiotic treatments (LA, DA) and the treatments without antibiotics (LN, LD; Figure 5D).
Tips of the individual sponge fragments were collected for DNA extraction from each of the tank replicates to study changes in the abundance and community structure of sponge symbionts at the beginning (t0), the middle (t17), and end (t29) of the incubation experiment.
Quantification of bacterial 16S rRNA gene copies normalized to the quantified sponge marker gene showed a significant decrease of the bacterial load in all sponge fragments including the control treatment (LN) after the disconnection of the experimental system (tank effect; ANOVA; p < 0.05). A subsequent comparison to the bacterial abundance in sponges under control conditions (LN) of the same experimental days (either t17 or t29), showed that the dark treatment (DN) alone had no significant effect on the bacterial load; in contrast to a significant decrease of the bacterial abundance in the antibiotic treatments (LA, DA) (Figure 6A; ANOVA; p < 0.05). Over time, the concentration of sponge-associated bacteria continued to decrease (Figure 6A). Only for sponges of the antibiotic light treatment (LA) a higher relative bacterial abundance was determined at the end of the experiment (t29) compared to the initial decrease measured at t17.
Figure 6. Stressors specific effect on bacterial abundance, community composition, and diversity. Triplicates of Haliclona cnidata fragments were exposed to either normal light (LN, control), light and antibiotics (LA), dark incubation and no antibiotics (DN) or antibiotics plus dark incubation (DA) and microbiota were assessed after 0 (t0), 17 (t17), and 29 (t29) days. (A) Fold changes of bacterial abundances relative to t0 shown as 16S rRNA gene copy numbers derived by qPCR normalized by 2-ΔCT method. Lowercases indicate significant differences based on one-way ANOVA on Ranks and Tukey’s HSD post hoc test. (B) Cluster analysis of 16S rRNA gene-based PCR-DGGE community fingerprints. DNA bands B01-B10 refer to identified bands (Table 1) performed according to Figure 3B. (C) NMDS plot based on a Bray Curtis dissimilarity matrix of relative band surface data of DGGE community fingerprint patterns. Biplots depict the contribution of treatments on changes of the abundant bacterial communities.
The bacterial community patterns of treated sponges were studied by bacterial 16S rRNA gene targeting PCR-DGGE. UPGMA clustering using the Pearson correlation coefficient (Figure 6B) and NMDS plots (Bray-Curtis similarity index; Figure 6C) revealed the following: First, the abundant sponge microbiota differed significantly in composition after the sponge was cultured in the experimental system (comparison of t0 to LN; tank effect; Figures 6B,C; ANOSIM; p < 0.05; Supplementary Table S7). Subsequent comparisons of the sponge microbiota of treated and control sponges at t17 and t29 showed treatment specific effects on the bacterial community composition (ANOSIM; p < 0.05; Supplementary Table S7). Both at t17 and t29, antibiotic treatments (LA, DA) had a stronger and significant effect on the composition of the sponge microbiota while light exclusion alone had no significant effect on the community patterns (DN vs. LN and DA vs. LA; ANOSIM; p > 0.05; Supplementary Table S7). Based on the comparison of DGGE profiles, several of the abundant sponge-associated bacteria (represented as abundant DNA bands in the DGGE patterns) were negatively affected by the antibiotic treatment while few other abundant bacteria remained (Figure 6B).
The phylogenetic composition of the sponge microbiome present in sponge samples collected from the main aquarium system in 2012 and 2015 and in the experimental closed aquarium systems was compared by 16S rRNA gene amplicon sequencing of pooled replicates of DNA samples which had identical DGGE profiles. Analysis was performed to identify abundant contributing bacteria and not to perform a statistical analysis of community changes. Homogeneity of sponge symbiont communities among replicates was already demonstrated by DGGE. Similarity percentage (SIMPER) analyses (Supplementary Tables S8, S9) were performed to determine phylogenetic groups that explained differences (i) among the bacterial communities of sponge fragments grown in the main water system compared to the experimental system (t0 compared to t17) and (ii) of sponge fragments grown under different experimental treatments.
The sponge microbiota was nearly identical in the main system (2012, 2015) and the connected experimental tanks at the time of sampling (t0). After the disconnection of the experimental tanks from the large water circulation system, the sponge microbiome composition changed. Changes were mostly driven by Sphingomonas (T23, SIMPER contribution 8.9%), Thermodesulforhabdus (T26, SIMPER contribution 8.5%; both Proteobacteria), and Thermoanaerobacter (T29, SIMPER contribution 6.0%; Firmicutes), which strongly increased in relative abundance in sponge fragments in the experimental system (Supplementary Tables S6, S8). Vice versa, JTB23 (T1, uncultured Proteobacteria), Pseudoruegeria (T5; Alphaproteobacteria), Sh765B-TzT-29 (uncultured Deltaproteobacteria), and clade KI89A (uncultured Gammaproteobacteria), decreased strongly in relative abundance in sponge fragments of the experimental treatment (Supplementary Figure S3B and Supplementary Table S6).
Based on our hypothesis, the sustained biological activity in the antibiotic treated and dark incubated sponges (analyzed in the small tank systems disconnected from the main water circulation system) may be linked to those phylogenetic groups that remained associated to the host. A total of 105 phylogenetic groups belonging to 20 phyla were shared among the microbiomes of the non-treated (LN) and treated (LA, DA, DN) sponges at t17 (Figure 7A). Of the 105 phylogenetic groups, 23 occurred with a relative abundance of ≥1% in at least one of the analyzed sponge samples. These 23 phylogenetic groups were defined as the resilient bacterial community of H. cnidata in the closed experimental tanks. The resilient community comprised about 7–11% of the total number of phylogenetic groups that were detected in individual sponge fragments in the experimental setup. However, the combined relative abundance of the resilient community per sponge samples (pool of treatment replicates) was high and varied between 72.1 and 79.6% (Figure 7B and Supplementary Table S10). Triangle plots were used to visualize differences in the relative abundance of the shared 105 phylogenetic groups (Figure 7C) and the resilient bacterial community (Figure 7D). Two main cluster of phylogenetic groups became obvious in the resilient community (Figure 7D). First, phylogenetic groups that were hardly impaired by dark incubation and antibiotic treatments and thus, shared in a similar manner by sponges of all treatments (Figure 7D). Second, taxa which were only present with a high relative abundance in control and dark incubated sponges (LN and DN) but negatively affected by antibiotic treatments (LA, DA) (Figure 7D). This observation reflected clearly the community shifts that were detected by the NMDS and cluster analyses of the DGGE fingerprints of the biological replicates (Figures 6B,C).
Figure 7. The resilient bacterial community of Haliclona cnidata. 16S rRNA gene amplicon Illumina MiSeq sequencing revealed a Bacteria community of 105 phylogenetic groups that were shared among sponges of all treatments. (A) Venn diagram showing specific and shared phylogenetic groups detected in the sponge-fragments after 17 days (t17) exposure to either antibiotics in light (LA), dark incubation without antibiotics (DN), antibiotics plus dark incubation (DA), or normal light (LN, control) incubation. Numbers indicate the number of phylogenetic groups (relative abundance in parentheses). (B) Taxonomic assignment and relative abundance pattern of the 105 shared phylogenetic groups. Among them, 23 phylogenetic groups occurred with a relative abundance of ≥1% in at least one of the analyzed sponge samples. These 23 phylogenetic groups were defined as the resilient bacterial community (highlighted in bold). Hierarchical clustering based on a Bray-Curtis matrix. Full taxa paths and relative abundance values are provided in Supplementary Table S10. Potential functions assigned in previous studies to respective taxa are given. (C,D) Ternary plots of phylogenetic groups indicating their relative distribution of respective taxa in sponge fragments of the different treatments, considering all 105 phylogenetic groups (C) or the 23 phylogenetic groups of the resilient bacterial community (D). Size of taxa represents presence in LN. Green labeled taxa in (D) represent the most abundant taxa. Abbreviations: AOB, ammonia-oxidizing bacteria; b. act., bioactive; cm, core microbiota; ch. CO2 fix., chemoautotrophic CO2 fixation; CP, Cophenetic Correlation Coefficient; HMA ind., HMA indicator; p.d., polysaccharide degradation; QS, quorum sensing; QS (p.m.), peptide mediated quorum sensing; QS (L.t.), LUXI/LUXR-type quorum sensing; SOB, sulfate-oxidizing bacterium; SRB, sulfate-reducing bacterium.
Dominant phylogenetic groups of the resilient community were Sphingomonas (T23; Alphaproteobacteria), Thermodesulforhabdus (T26; Deltaproteobacteria), and Thermoanaerobacter (T29; Firmicutes). Phylogenetic groups of the resilient community that occurred abundantly in LN and DN but were reduced by antibiotic exposure were the BD2-11 terrestrial group (T2; Gemmatimonadetes), Owenweeksia (T6; Bacteriodetes), PAUC26f (T4; Acidobacteria), uncultured Rhodothermaceae (T8; Bacteroidetes), and the OPB35 soil group (T3; Verrucomicrobia), and with an only minor abundance (2% in LN) Pseudoruegeria (T5; Alphaproteobacteria) and JTB23 (T3; uncultured lineage of Proteobacteria) (Figure 7D and Supplementary Table S10). The most abundant phylogenetic group of Cyanobacteria (T11; DGGE bands B05-B07, 8B) was not assigned to the resilient community due to absence in the antibiotic light treatment (LA).
Detailed characterization of the model organism enabled the designation of the long-term cultured H. cnidata as a HMA sponge. First, the bacterial load was in the range of HMA sponges (108–1010 bacteria per gram sponge wet weight) (Webster et al., 2001; Hentschel et al., 2002, 2003, 2006, 2012; Gloeckner et al., 2013). Second, the sponge harbored a diverse bacterial community typical for HMA sponges with high phylum-level diversity (Gloeckner et al., 2013). Among the phyla detected several are known as HMA-indicator phyla (Moitinho-Silva et al., 2017a) such as Chloroflexi, Acidobacteria, Actinobacteria, PAUC34f, Gemmatimonadetes, Nitrospira, and Spirochaetes. Phylogenetic groups with a high relative abundance in H. cnidata comprised typical sponge-specific taxa, i.e., PAUC26f (T4) (Hentschel et al., 2002) or JTB23 (T1) (Burgsdorf et al., 2014), and known HMA indicator-taxa such as SAR202 (T12), Acidimicrobiales (T14), and Anaeroloneaceae (T22; Chloroflexi) (Moitinho-Silva et al., 2017a,b). Members of the sponge core microbiome occurred most likely abundantly in H. cnidata as DNA bands of the DGGE profiles were sequence identical to the representative OTU001995 (Thomas et al., 2016). These DNA bands were also affiliated with phylogenetic groups assigned as T1 and T4 in the amplicon dataset. The study illustrates that the long-term maricultured model organism developed a stable, sponge-typical microbiota comprising well known sponge symbionts that occur in sponges growing in the native environment. However, the absence of the candidate phylum Poribacteria, which is normally present in HMA sponges (Gloeckner et al., 2013), has to be emphasized. Although the effect of the cultivation conditions on the HMA status remains to be elucidated, quantity, diversity, and presence of indicator species warrant the assignment of H. cnidata to the HMA group.
The low concentration of bacterial cells in the mesohyl of H. cnidata is interesting as diverse microbial communities are typically located extracellularly in HMA sponges (Hentschel et al., 2006; Webster et al., 2011; Gloeckner et al., 2013). Moreover, abundant morphological diverse bacterial cells were located in interconnected bacteriocytes, which is considered atypical for an HMA sponge despite few exceptions (Levi and Levi, 1976; Vacelet and Donadey, 1977; Webster and Thomas, 2016). The function of the bacterial consortia in the bacteriocytes remains largely unknown. In our study, bacteriocytes might have contributed to the protection of the sponge-associated bacteria against the antibiotic exposure. Interestingly, the bacteriocytes are interconnected, potentially indicating microbe-microbe interactions across these consortia. However, the function of the bacteriocytes was beyond the scope and future studies have to show whether they potentially facilitate antimicrobial defense or other key functions for the host.
We found indication for a persistent bioactivity in the holobiont despite the detrimental conditions following the experimental treatment. Specifically, the host exhibited several signs of deterioration like bleaching and fouling under light exclusion and/or in the presence of antibiotics but the bioactivity of the holobiont was apparently sustained. For sponges, morphological plasticity related to environmental conditions is a common phenomenon (Palumbi, 1984) and it is well known that their color can turn brighter under low light conditions (Maldonado and Young, 1998). Such a color change can be induced by sponge-associated microbiota, e.g., through pigments of phototrophic symbionts including Cyanobacteria (Usher et al., 2004; Burgsdorf et al., 2015) or associated zooxanthellae (Rützler, 2002). The latter type of microbiota is known to occur in H. cnidata (Schellenberg et al., 2019). Additionally, evidence for Cyanobacteria were found as bacterial cells with thylakoid membranes were detected in bacteriocytes resembling Candidatus Synechococcus spongiarum (Usher, 2008) as well as the highly abundant autofluorescent rod-shaped cells that were arranged in aggregates. Therefore, the decline of photosymbionts may have been one of the main reasons of the observed bleaching of H. cnidata in dark incubated sponges, similar to the well-known phenomenon among corals (Buddemeier and Fautin, 1993). Interestingly, the sponges in LA turned pale even sooner and faster than the dark incubated sponge fragments. Accordingly, 16S rRNA gene-based data showed that the most abundant phylogenetic group of Cyanobacteria (T11; Subsection I, Family I, uncultured) was fully depleted in the antibiotic light (LA) treatment. The applied antibiotics differ in their mechanism of action as ampicillin interferes with the bacterial cell wall synthesis, while gentamicin impairs the protein synthesis. Accordingly, several members of the microbiota were negatively impacted in the LA treatment. The effect of antibiotics on the mostly unculterd microbiota remains to be elucidated but at least for the genera Oweenweeksia (T6) and Pseudoruegeria (T5) susceptibility to either ampicillin or gentamicin is known (Lau et al., 2005; Cha et al., 2016).
Fouling of sponges by microbes, invertebrates, and algae is commonly prevented through the presence of bioactive compounds in sponges (Paul and Puglisi, 2004; Thoms and Schupp, 2007; Rohde et al., 2012). Hence, an impaired chemical defense should have led to overgrowth or colonization of H. cnidata by invading microbes. However, 16S rRNA gene amplicon data did not indicate such a high abundance of sponge unspecific bacteria, which is likely due to the sustained biological activity. Therefore, the fouling of the sponge fragments in LA might be interpreted as a severe sign of imbalanced nutrient supply in the holobiont due to symbiont depletion as observed for the potential key bacterial taxa BD2-11 and PAUC26f. This assumption seems more reasonable as holobiont function has already been linked to microbiota and their symbiotic interactions with the sponge (e.g., Fan et al., 2013).
The impaired defense of H. cnidata in the antibiotic light treatment (LA) could be due to quantitative or qualitative differences of the bioactive compounds present in the sponge extracts. If the sponge-associated bacterial community is, as expected, responsible for bioactivity (Unson and Faulkner, 1993; Faulkner et al., 1994; Piel, 2009; Hentschel et al., 2012; Webster and Taylor, 2012), either the abundance of the respective bacteria might have changed, or the nutritional requirements of specific bacterial taxa were no longer fulfilled. Alternatively, bioactivity might be facilitated by the associated dinoflagellates (Kita et al., 2010). However, for Haliclona sp. 628, which shares certain similarities with H. cnidata (Schellenberg et al., 2019), the associated dinoflagellates were not accountable for the bioactivity (Garson et al., 1998). If the sponge host itself was accountable for the bioactivity (Thompson et al., 1983; Garson et al., 1998; Salomon et al., 2001; Sipkema et al., 2005), the energetically expensive production of the bioactive secondary metabolites (Thoms and Schupp, 2007; Loh and Pawlik, 2014) may have been constrained because of an imbalanced microbial community that no longer provides full nutritional support to the host. Interestingly, the highest impairment of antimicrobial activity in LA corresponds to the degree of host health decline, which appeared to be most severe in this treatment.
Despite of the bacterial community shifts seen in impaired sponge fragments, the remaining bacterial community most likely facilitates the survival of the sponge under harmful conditions. Further, enhanced QS activity was observed in the bioassay experiments. The operational QS implies the presence of signal molecules that regulate physiological processes and such cell to cell communication may even occur between species (Miller and Bassler, 2001). Thus, we conclude that physiological processes among the associated bacterial community of H. cnidata are still coordinated and controlled. Furthermore, it is tempting to speculate that bacteriocytes and the observed dense aggregates of symbionts are the respective microhabitats of the sponge were QS activity contributes to or drives microbe-microbe interactions in the resilient community.
To assess the biological activity and the general physiological capacity of the shared community, a literature-based comparison of biological activities for the respective bacterial taxa was performed. Chemical defense might be facilitated by several phylogenetic groups of the Alphaproteobacteria such as the Rhodobacteraceae (T21, T42, T71, T96, T196) or Sphingomonadaceae (T23, T253, T712, T714) (Schmitt et al., 2011, 2012). Both families had a combined average relative abundance of 16.0% in the compromised sponge, while Sphingomonas (T23) alone accounted for 15.3%. Furthermore, several other phylogenetic groups are known for their antimicrobial activity, i.e., Bacillus (T468), Staphylococcus (T89; both Firmicutes), and Pseudomonas (T62; Gammaproteobacteria). Bioactive members of those genera were previously isolated from sponges (Graça et al., 2013). Among these taxa, the resilient bacterial community comprised Sphingomonas (T23) and Staphylococcus (T89), while the remaining taxa were shared, but occurred with a relative abundance below 1%.
Within the shared community, bacteria were detected that are well known for both peptide-mediated and LUXI/LUXR-type QS, such as Bacillus (T468) and Staphylococcus (T89), as well as Rhizobium (T639), Ralstonia (T54), and Pseudomonas (T62), respectively (Miller and Bassler, 2001). Interestingly, the two latter groups (T54, T62) were persistent members in our model sponge occurring in all analyzed samples. Staphylococcus (T89) and Ralstonia (T54) affiliated to the resilient bacterial community while the remaining shared taxa occurred with a relative abundance below 1%. Recently, Gutiérrez-Barranquero et al. (2019) shed new light on the complexity of QS in sponge holobionts by detecting dual activity in some marine sponge isolates. However, it must be acknowledged that the taxa associated functions are currently hypothetical for the microbiome of our model sponge since the total microbiome (complete genetic potential of the resilient sponge microbiota) has not yet been studied.
Bioactivity-derived defense is likely only one potential essential function provided by the resilient bacterial community in addition to other metabolic core functions (sensu Fan et al., 2013). Several taxa were detected in the shared community of H. cnidata that might be involved in other key functions of the sponge holobiont such as nitrogen or sulfur cycles of the host. Among others, these taxa comprised Nitrosomonas (T158) as a known ammonia-oxidizing bacterium, sulfate-reducing bacteria such as Thermodesulforhabdus (T26) and Desulfovibrio (T41) (Kuever et al., 2002) or sulfur-oxidizing bacteria like members of the Rhodospirillaceae (T16), and potentially by the core microbiome member JTB23 (T1) (Jensen et al., 2017). Based on their relative abundance, JTB23 (T1), Thermodesulforhabdus (T26) and Desulfovibrio (T41) are affiliated to the resilient bacterial community of H. cnidata. However, functional annotation of specific bacterial phylotypes is still largely missing for sponges due to the lack of successful isolation of undescribed taxa. It has to be acknowledged that the assignment of the here observed bioactivity to a specific sponge-associated bacterial consortium remains ambiguous, because amplicon studies targeting the 16S rRNA gene sequence alone can neither show the metabolic activity of the detected bacteria (Moitinho-Silva et al., 2014) nor resolve taxa at the species and the even more ecological relevant subspecies/ecotype level (Schleifer, 2009; Kämpfer and Glaeser, 2012; Glaeser and Kämpfer, 2015; Rosselló-Mora and Amann, 2015).
Further, functionally equivalent bacteria have already been identified in the associated communities of sponges (Ribes et al., 2012; Fan et al., 2013) and thus, the resilient community might rather be assessed at the level of provided functions than the species composition (sensu the insurance hypothesis; Yachi and Loreau, 1999). The concept of the insurance hypothesis assumes that diverse communities may better maintain functionality and compensate disturbances due to functional redundancy and presence of more resilient agents. Fan et al. (2013) identified such core functions comprising various aspects of metabolism provided by functionally equivalent Bacteria in phylogenetically distinct sponges. Functional equivalence may explain the small fraction of the 23 resilient taxa in H. cnidata. It remains to be investigated to which degree this community may be responsible for shaping the total microbiota composition of the sponge holobiont (e.g., via QS activity). Further, it cannot be excluded that the antimicrobial activity is based on the host itself and not provided by the symbiotic microbiota. However, at least the observed QS response to A. fischeri is expected to be based on bacteria derived QS signals.
Haliclona cnidata was characterized as a HMA sponge containing a temporally stable, sponge-specific bacterial microbiome under aquarium conditions. Dark incubation and exposure to antibiotics affected the abundance and to some extent the community composition of the sponge microbiota while the bioactivity (i.e., operational antimicrobial defense and QS activity) was apparently maintained. Despite the impact on the microbiota, a resilient bacterial community of 105 shared phylogenetic groups was identified, which we assume to be responsible for the sustained bioactivity and thus, the functional integrity of the compromised sponge holobiont. Only 23% of the resilient community members were temporally stable, probably being obligate bacterial symbionts of H. cnidata. However, the degree to which the resilience of the community depends on the diversity and functional redundancy of the sponge microbiome, and thus whether such a resilient community can also be identified in less diverse LMA sponges, remains to be investigated. Likewise, further studies are needed to examine the impact of mariculture on our findings, specifically on the occurrence of bacteriocytes in our model organism. In H. cnidata, the main fraction of sponge-associated Bacteria was localized in bacteriocytes, which may have protected the sponge microbiota against antimicrobial stress, or even triggered biological activity. For H. cnidata, it remains to be investigated if the majority of the sponge microbiota and/or only the resilient community are hosted in bacteriocytes. However, the role of bacteriocytes alongside the diversity, composition, and functionality of the contained Bacteria has not been studied yet in the field of sponge microbiology. To the best of our knowledge, this is the first study indicating a potential role of bacteriocytes in sponges. Taken together with the concept of a resilient community, our findings could provide a starting point for future studies to further delineate holobiont functioning.
Publicly available datasets were analyzed in this study. This data can be found here: MH045531-MH045540, SAMN08957770-SAMN08957776.
JS, JR, GM, PS, TW, and SG conceived and designed the experiments. JS and JR performed the experiments. JS, JR, and SG analyzed the data. JS and SG wrote the manuscript. JR, MH, IK, GM, PS, MB, H-PG, PK, and TW revised and approved the final manuscript version and are accountable for all aspects of the work.
JS received financial support through a scholarship from the CEMarin (Center of Excellence in Marine Sciences, Colombia) and JR through a scholarship from the Justus Liebig University Giessen.
The authors declare that the research was conducted in the absence of any commercial or financial relationships that could be construed as a potential conflict of interest.
We gratefully acknowledge Sabine Agel, Barbara Hönig, and Anna Möbus from the Imaging Unit, Justus Liebig University Giessen for their excellent technical assistance. The CEMarin (Center of Excellence in Marine Sciences, Colombia) is gratefully acknowledged for funding. We thank both reviewers for their valuable comments.
The Supplementary Material for this article can be found online at: https://www.frontiersin.org/articles/10.3389/fmars.2020.00266/full#supplementary-material
Bell, J. J. (2008). The functional roles of marine sponges. Estuar. Coast. Shelf Sci. 79, 341–353. doi: 10.1016/j.ecss.2008.05.002
Bell, J. J., and Smith, D. (2004). Ecology of sponge assemblages (Porifera) in the Wakatobi region, south-east Sulawesi, Indonesia: richness and abundance. J. Mar. Biol. Assoc. 84, 581–591. doi: 10.1017/S0025315404009580h
Bergman, O., Haber, M., Mayzel, B., Anderson, M. A., Shpigel, M., Hill, R. T., et al. (2011). Marine-based cultivation of diacarnus sponges and the bacterial community composition of wild and maricultured sponges and their larvae. Mar. Biotechnol. 13, 1169–1182. doi: 10.1007/s10126-011-9391-6
Bewley, C. A., Holland, N. D., and Faulkner, D. J. (1996). Two classes of metabolites from Theonella swinhoei are localized in distinct populations of bacterial symbionts. Experientia 52, 716–722. doi: 10.1007/bf01925581
Bordenstein, S. R., and Theis, K. R. (2015). Host biology in light of the microbiome: ten principles of holobionts and hologenomes. PLoS Biol. 13:e1002226. doi: 10.1371/journal.pbio.1002226
Buddemeier, R. W., and Fautin, D. G. (1993). Coral bleaching as an adaptive mechanism. BioScience 43, 320–326. doi: 10.2307/1312064
Burgsdorf, I., Erwin, P. M., López-Legentil, S., Cerrano, C., Haber, M., Frenk, S., et al. (2014). Biogeography rather than association with cyanobacteria structures symbiotic microbial communities in the marine sponge Petrosia ficiformis. Front. Microbiol. 5:529. doi: 10.3389/fmicb.2014.00529
Burgsdorf, I., Slaby, B. M., Handley, K. M., Haber, M., Blom, J., Marshall, C. W., et al. (2015). Lifestyle evolution in cyanobacterial symbionts of sponges. MBio 6:e391-15. doi: 10.1128/mBio.00391-15
Camacho, C., Coulouris, G., Avagyan, V., Ma, N., Papadopoulos, J., Bealer, K., et al. (2009). BLAST+: architecture and applications. BMC Bioinformatics 10:421. doi: 10.1186/1471-2105-10-421
Cha, I. T., Park, I., Lee, H. W., Lee, H., Park, J. M., Roh, S. W., et al. (2016). Pseudoruegeria aestuarii sp. nov., of the family Rhodobacteraceae, isolated from a tidal flat. Int. J. Syst. Evol. Microbiol. 66, 3125–3131. doi: 10.1099/ijsem.0.001156
Edwards, U., Rogall, T., Blöcker, H., Emde, M., and Böttger, E. C. (1989). Isolation and direct complete nucleotide determination of entire genes: characterization of a gene coding for 16S ribosomal RNA. Nucleic Acids Res. 17, 7843–7853. doi: 10.1093/nar/17.19.7843
Erwin, P. M., and Thacker, R. W. (2008). Phototrophic nutrition and symbiont diversity of two Caribbean sponge–cyanobacteria symbioses. Mar. Ecol. Prog. Ser. 362, 139–147. doi: 10.3354/meps07464
Fan, L., Liu, M., Simister, R., Webster, N. S., and Thomas, T. (2013). Marine microbial symbiosis heats up: the phylogenetic and functional response of a sponge holobiont to thermal stress. ISME J. 7, 991–1002. doi: 10.1038/ismej.2012.165
Faulkner, D. J., Unson, M. D., and Bewley, C. A. (1994). The chemistry of some sponges and their symbionts. Pure Appl. Chem. 66, 1983–1990. doi: 10.1351/pac199466101983
Folmer, O., Black, M. B., Hoeh, W., Lutz, R., and Vrijenhoek, R. C. (1994). DNA primers for amplification of mitochondrial Cytochrome C oxidase subunit I from diverse metazoan invertebrates. Mol. Mar. Biol. Biotechnol. 3, 294–299.
Friedrich, A. B., Fischer, I., Proksch, P., Hacker, J., and Hentschel, U. (2001). Temporal variation of the microbial community associated with the mediterranean sponge Aplysina aerophoba. FEMS Microbiol. Ecol. 38, 105–115. doi: 10.1016/S0168-6496(01)00185-4
Garson, M. J., Flowers, A. E., Webb, R. I., Charan, R. D., and McCaffrey, E. J. (1998). A sponge/dinoflagellate association in the haplosclerid sponge Haliclona sp.: cellular origin of cytotoxic alkaloids by Percoll density gradient fractionation. Cell Tissue Res. 293, 365–373. doi: 10.1007/s004410051128
Gerçe, B., Schwartz, T., Voigt, M., Rühle, S., Kirchen, S., Putz, A., et al. (2009). Morphological, bacterial, and secondary metabolite changes of Aplysina aerophoba upon long-term maintenance under artificial conditions. Microb. Ecol. 58, 865–878. doi: 10.1007/s00248-009-9560-6
Glaeser, S. P., Berghoff, B. A., Stratmann, V., Grossart, H.-P., Glaeser, J., Netto et al. (2014). Contrasting effects of singlet oxygen and hydrogen peroxide on bacterial community composition in a humic lake. PLoS ONE 9:e92518. doi: 10.1371/journal.pone.0092518
Glaeser, S. P., Grossart, H.-P., and Glaeser, J. (2010). Singlet oxygen, a neglected but important environmental factor: short-term and long-term effects on bacterioplankton composition in a humic lake. Environ. Microbiol. 12, 3124–3136. doi: 10.1111/j.1462-2920.2010.02285.x
Glaeser, S. P., and Kämpfer, P. (2015). Multilocus sequence analysis (MLSA) in prokaryotic taxonomy. Syst. Appl. Microbiol. 38, 237–245. doi: 10.1016/j.syapm.2015.03.007
Gloeckner, V., Hentschel, U., Ereskovsky, A. V., and Schmitt, S. (2013). Unique and species-specific microbial communities in Oscarella lobularis and other Mediterranean Oscarella species (Porifera: Homoscleromorpha). Mar. Biol. 160, 781–791. doi: 10.1007/s00227-012-2133-0
Gloeckner, V., Wehrl, M., Moitinho-Silva, L., Gernert, C., Schupp, P., Pawlik, J. R., et al. (2014). The HMA-LMA dichotomy revisited: an electron microscopical survey of 56 sponge species. Biol. Bull.-US 227, 78–88. doi: 10.1086/BBLv227n1p78
Graça, A. P., Bondoso, J., Gaspar, H., Xavier, J. R., Monteiro, M. C., La Cruz, M., et al. (2013). Antimicrobial activity of heterotrophic bacterial communities from the marine sponge Erylus discophorus (Astrophorida, Geodiidae). PLoS ONE 8:e78992. doi: 10.1371/journal.pone.0078992
Gutiérrez-Barranquero, J. A., Reen, F. J., Parages, M. L., McCarthy, R., Dobson, A. D. W., and O’Gara, F. (2019). Disruption of N-acyl-homoserine lactone-specific signalling and virulence in clinical pathogens by marine sponge bacteria. Microb. Biotechnol. 12, 1049–1063. doi: 10.1111/1751-7915.12867
Hentschel, U., Fieseler, L., Wehrl, M., Gernert, C., Steinert, M., Hacker, J., et al. (2003). Microbial diversity of marine sponges. Prog. Mol. Subcell. Biol. 37, 59–88. doi: 10.1007/978-3-642-55519-0_3
Hentschel, U., Hopke, J., Horn, M., Friedrich, A. B., Wagner, M., Hacker, J., et al. (2002). Molecular evidence for a uniform microbial community in sponges from different oceans. Appl. Environ. Microbiol. 68, 4431–4440. doi: 10.1128/AEM.68.9.4431-4440.2002
Hentschel, U., Piel, J., Degnan, S. M., and Taylor, M. W. (2012). Genomic insights into the marine sponge microbiome. Nat. Rev. Microbiol. 10, 641–654. doi: 10.1038/nrmicro2839
Hentschel, U., Usher, K. M., and Taylor, M. W. (2006). Marine sponges as microbial fermenters. FEMS Microbiol. Ecol. 55, 167–177. doi: 10.1111/j.1574-6941.2005.00046.x
Ionescu, D., Siebert, C., Polerecky, L., Munwes, Y. Y., Lott, C., Häusler, S., et al. (2012). Microbial and chemical characterization of underwater fresh water springs in the Dead Sea. PLoS One 7:e38319. doi: 10.1371/journal.pone.0038319
Jensen, S., Fortunato, S. A. V., Hoffmann, F., Hoem, S., Rapp, H. T., Øvreås, L., et al. (2017). The relative abundance and transcriptional activity of marine sponge-associated microorganisms emphasizing groups involved in sulfur cycle. Microb. Ecol. 73, 668–676. doi: 10.1007/s00248-016-0836-3
Kämpfer, P., and Glaeser, S. P. (2012). Prokaryotic taxonomy in the sequencing era–the polyphasic approach revisited. Environ. Microbiol. 14, 291–317. doi: 10.1111/j.1462-2920.2011.02615.x
Kelman, D., Kashman, J., Rosenberg, E., Ilan, M., Ilan, I., and Loya, Y. (2001). Antimicrobial activity of the reef sponge Amphimedon viridis from the Red Sea: evidence for selective toxicity. Aquat. Microb. Ecol. 24, 9–16. doi: 10.3354/ame024009
Kirchhoff, K. N., Klingelhöfer, I., Dahse, H.-M., Morlock, G., and Wilke, T. (2014). Maturity-related changes in venom toxicity of the freshwater stingray Potamotrygon leopoldi. Toxicon 92, 97–101. doi: 10.1016/j.toxicon.2014.10.011
Kita, M., Ohno, O., Han, C., and Uemura, D. (2010). Bioactive secondary metabolites from symbiotic marine dinoflagellates: symbiodinolide and durinskiols. Chem. Rec. 10, 57–69. doi: 10.1002/tcr.200900007
Klindworth, A., Pruesse, E., Schweer, T., Peplies, J., Quast, C., Horn, M., et al. (2013). Evaluation of general 16S ribosomal RNA gene PCR primers for classical and next-generation sequencing-based diversity studies. Nucleic Acids Res. 41:e1. doi: 10.1093/nar/gks808
Klöppel, A., Brümmer, F., Schwabe, D., and Morlock, G. (2013). Detection of bioactive compounds in the mucus nets of dendropoma maxima sowerby 1825 (Prosobranch Gastropod Vermetidae, Mollusca). J. Mar. Biol. 2013, 1–9.
Klöppel, A., Grasse, W., Brümmer, F., and Morlock, G. (2008). HPTLC coupled with bioluminescence and mass spectrometry for bioactivity-based analysis of secondary metabolites in marine sponges. J. Planar Chromatogr.-Mod. TLC 21, 431–436. doi: 10.1556/JPC.21.2008.6.7
Kolb, S., Knief, C., Stubner, S., and Conrad, R. (2003). Quantitative detection of methanotrophs in soil by novel pmoA-targeted real-time PCR assays. Appl. Environ. Microbiol. 69, 2423–2429. doi: 10.1128/AEM.69.5.2423-2429.2003
Kuever, J., Sievert, S. M., Stevens, H., Brinkhoff, T., and Muyzer, G. (2002). Microorganisms of the oxidative and reductive part of the sulphur cycle at a shallow-water hydrothermal vent in the Aegean Sea (Milos, Greece). Cah. Biol. Mar. 43, 413–416.
Lau, K. W., Ng, C. Y., Ren, J., Lau, S. C., Qian, P. Y., Wong, P. K., et al. (2005). Owenweeksia hongkongensis gen. nov., sp. nov., a novel marine bacterium of the phylum ‘Bacteroidetes’. Int. J. Syst. Evol. Microbiol. 55, 1051–1057. doi: 10.1099/ijs.0.63155-0
Levi, C., and Levi, P. (1976). Embryogenese de Chondrosia reniformis (Nardo), demosponge ovipare, et transmission des bacteriessymbiotiques. Ann. Sci. Nat. Zool. 367–380.
Li, W., and Godzik, A. (2006). Cd-hit: a fast program for clustering and comparing large sets of protein or nucleotide sequences. Bioinformatics 22, 1658–1659. doi: 10.1093/bioinformatics/btl158
Livak, K. J., and Schmittgen, T. D. (2001). Analysis of relative gene expression data using real-time quantitative PCR and the 2−ΔΔCT method. Methods 25, 402–408. doi: 10.1006/meth.2001
Loh, T.-L., and Pawlik, J. R. (2014). Chemical defenses and resource trade-offs structure sponge communities on Caribbean coral reefs. Proc. Natl. Acad. Sci. U.S.A. 111, 4151–4156. doi: 10.1073/pnas.1321626111
Maeda, H., Fujimoto, C., Haruki, Y., Maeda, T., Kokeguchi, S., Petelin, M., et al. (2003). Quantitative real-time PCR using TaqMan and SYBR Green for Actinobacillus actinomycetemcomitans, Porphyromonas gingivalis, Prevotella intermedia, tetQ gene and total bacteria. FEMS Immunol. Med. Microbiol. 39, 81–86. doi: 10.1016/S0928-8244(03)00224-4
Maldonado, M., and Young, C. M. (1998). Limits on the bathymetric distribution of keratose sponges: a field test in deep water. Mar. Ecol. Prog. Ser. 174, 123–139. doi: 10.3354/meps174123
Miller, M. B., and Bassler, B. L. (2001). Quorum sensing in bacteria. Annu. Rev. Microbiol. 55, 165–199. doi: 10.1146/annurev.micro.55.1.165
Moitinho-Silva, L., Bayer, K., Cannistraci, C. V., Giles, E. C., Ryu, T., Seridi, L., et al. (2014). Specificity and transcriptional activity of microbiota associated with low and high microbial abundance sponges from the Red Sea. Mol. Ecol. 23, 1348–1363. doi: 10.1111/mec.12365
Moitinho-Silva, L., Nielsen, S., Amir, A., Gonzalez, A., Ackermann, G. L., Cerrano, C., et al. (2017a). The sponge microbiome project. GigaScience 6, 1–7. doi: 10.1093/gigascience/gix077
Moitinho-Silva, L., Steinert, G., Nielsen, S., Hardoim, C. C. P., Wu, Y.-C., McCormack, G. P., et al. (2017b). Predicting the HMA-LMA status in marine sponges by machine learning. Front. Microbiol. 8:752. doi: 10.3389/fmicb.2017.00752
Muyzer, G., de Wall, E. C., and Uitterlinden, A. G. (1993). Profiling of complex microbial populations by denaturing gradient gel electrophoresis analysis of polymerase chain reaction-amplified genes coding for 16S rRNA. Appl. Environ. Microbiol. 59, 695–700.
Oksanen, J., Blanchetm, F., Guillaume, Kindt, R., Legendre, P., Minchin, P. R., et al. (2015). vegan: Community Ecology Package. R Package Version 2.3-1. Available online at: http://CRAN.R-project.org/package=vegan
Palumbi, S. R. (1984). Tactics of acclimation: morphological changes of sponges in an unpredictable environment. Science 225, 1478–1480. doi: 10.1126/science.225.4669.1478
Paul, V. J., and Puglisi, M. P. (2004). Chemical mediation of interactions among marine organisms. Nat. Prod. Rep. 35, 189–209. doi: 10.1039/b302334f
Piel, J. (2009). Metabolites from symbiotic bacteria. Nat. Prod. Rep. 26, 338–362. doi: 10.1039/b703499g
Pita, L., Erwin, P. M., Turon, X., and López-Legentil, S. (2013). Till death do us part: stable sponge-bacteria associations under thermal and food shortage stresses. PLoS ONE 8:e80307. doi: 10.1371/journal.pone.0080307
Pruesse, E., Peplies, J., and Glöckner, F. O. (2012). SINA: accurate high-throughput multiple sequence alignment of ribosomal RNA genes. Bioinformatics 28, 1823–1829. doi: 10.1093/bioinformatics/bts252
Quast, C., Pruesse, E., Yilmaz, P., Gerken, J., Schweer, T., Yarza, P., et al. (2013). The SILVA ribosomal RNA gene database project: improved data processing and web-based tools. Nucleic Acids Res. 41, D590–D596. doi: 10.1093/nar/gks1219
Reveillaud, J., Maignien, L., Murat Eren, A., Huber, J. A., Apprill, A., Sogin, M. L., et al. (2014). Host-specificity among abundant and rare taxa in the sponge microbiome. ISME J. 8, 1198–1209. doi: 10.1038/ismej.2013.227
Reynolds, D., and Thomas, T. (2016). Evolution and function of eukaryotic-like proteins from sponge symbionts. Mol. Ecol. 25, 5242–5253. doi: 10.3389/fmicb.2017.02499
Ribes, M., Jiménez, E., Yahel, G., López-Sendino, P., Diez, B., Massana, R., et al. (2012). Functional convergence of microbes associated with temperate marine sponges. Environ. Microbiol. 14, 1224–1239. doi: 10.1111/j.1462-2920.2012.02701.x
Rohde, S., Gochfeld, D. J., Ankisetty, S., Avula, B., Schupp, P. J., and Slattery, M. (2012). Spatial variability in secondary metabolites of the indo-pacific sponge Stylissa massa. J. Chem. Ecol. 38, 463–475. doi: 10.1007/s10886-012-0124-8
Rohwer, F., Seguritan, V., Azam, F., and Knowlton, N. (2002). Diversity and distribution of coral-associated bacteria. Mar. Ecol. Prog. Ser. 243, 1–10. doi: 10.3354/meps243001
Rosenberg, E., Koren, O., Reshef, L., Efrony, R., and Zilber-Rosenberg, I. (2007). The role of microorganisms in coral health, disease and evolution. Nat. Rev. Microbiol. 5, 355–362. doi: 10.1038/nrmicro1635
Rosselló-Mora, R., and Amann, R. (2015). Past and future species definitions for Bacteria and Archaea. Syst. Appl. Microbiol. 38, 209–216. doi: 10.1016/j.syapm.2015.02.001
Rützler, K. (2002). Impact of crustose clionid sponges on caribbean reef corals. Acta Geol. Hisp. 37, 61–72.
Salomon, C. E., Deerinck, T., Ellisman, M. H., and Faulkner, D. J. (2001). The cellular localization of dercitamide in the Palauan sponge Oceanapia sagittaria. Mar. Biol. 139, 313–319. doi: 10.1007/s002270100493
Schauss, T., Glaeser, S. P., Gütschow, A., Dott, W., and Kämpfer, P. (2015). Improved detection of extended spectrum beta-lactamase (ESBL)-producing Escherichia coli in input and output samples of German biogas plants by a selective pre-enrichment procedure. PLoS ONE 10:e0119791. doi: 10.1371/journal.pone.0119791
Schellenberg, J., Reichert, J., Hardt, M., Schmidtberg, H., Kämpfer, P., Glaeser, S. P., et al. (2019). The precursor hypothesis of sponge kleptocnidism: development of nematocysts in Haliclona cnidata sp. nov. (Porifera, Demospongiae, Haplosclerida). Front. Mar. Sci. 5:341. doi: 10.3389/fmars.2018.00509
Schleifer, K. H. (2009). Classification of bacteria and archaea: past, present and future. Syst. Appl. Microbiol. 32, 533–542. doi: 10.1016/j.syapm.2009.09.002
Schmitt, S., Hentschel, U., and Taylor, M. W. (2011). Deep sequencing reveals diversity and community structure of complex microbiota in five Mediterranean sponges. Hydrobiologia 687, 341–351. doi: 10.1007/s10750-011-0799-9
Schmitt, S., Tsai, P., Bell, J., Fromont, J., Ilan, M., Lindquist, N., et al. (2012). Assessing the complex sponge microbiota: core, variable and species-specific bacterial communities in marine sponges. ISME J. 6, 564–576. doi: 10.1038/ismej.2011.116
Simister, R. L., Deines, P., Botté, E. S., Webster, N. S., and Taylor, M. W. (2012). Sponge-specific clusters revisited: a comprehensive phylogeny of sponge-associated microorganisms. Environ. Microbiol. 14, 517–524. doi: 10.1111/j.1462-2920.2011.02664.x
Sipkema, D., Franssen, M. C. R., Osinga, R., Tramper, J., and Wijffels, R. H. (2005). Marine sponges as pharmacy. Mar. Biotechnol. 7, 142–162. doi: 10.1007/s10126-004-0405-5
Slaby, B. M., Hackl, T., Horn, H., Bayer, K., and Hentschel, U. (2017). Metagenomic binning of a marine sponge microbiome reveals unity in defense but metabolic specialization. ISME J. 11, 2465–2478. doi: 10.1038/ismej.2017.101
Stackebrandt, E., and Liesack, W. (1993). “Nucleic acids and classification,” in Handbook of New Bacterial Systematics, ed. M. Goodfellow (London: Academic Press), 152–189.
Steinert, G., Rohde, S., Janussen, D., Blaurock, C., and Schupp, P. J. (2017). Host-specific assembly of sponge-associated prokaryotes at high taxonomic ranks. Sci. Rep. 7:2542. doi: 10.1038/s41598-017-02656-6
Taylor, M. W., Radax, R., Steger, D., and Wagner, M. (2007). Sponge-associated microorganisms: evolution, ecology, and biotechnological potential. Microbiol. Mol. Biol. Rev. 71, 295–347. doi: 10.1128/MMBR.00040-06
Taylor, M. W., Tsai, P., Simister, R. L., Deines, P., Botte, E., Ericson, G., et al. (2013). ‘Sponge-specific’ bacteria are widespread (but rare) in diverse marine environments. ISME J. 7, 438–443. doi: 10.1038/ismej.2012.111
Thiel, V., Leininger, S., Schmaljohann, R., Brümmer, F., and Imhoff, J. F. (2007). Sponge-specific bacterial associations of the Mediterranean sponge Chondrilla nucula (Demospongiae, Tetractinomorpha). Microb. Ecol. 54, 101–111. doi: 10.1007/s00248-006-9177-y
Thomas, T., Moitinho-Silva, L., Lurgi, M., Björk, J. R., Easson, C., Astudillo-García, C., et al. (2016). Diversity, structure and convergent evolution of the global sponge microbiome. Nat. Commun. 7:11870. doi: 10.1038/ncomms11870
Thompson, J. E., Barrow, K. D., and Faulkner, D. J. (1983). Localization of two brominated metabolites, aerothionin and homoaerothionin, in spherulous cells of the marine sponge aplysina fistularis (=Verongia thiona). Acta Zool. 64, 199–210. doi: 10.1111/j.1463-6395.1983.tb00801.x
Thoms, C., Horn, M., Wagner, M., Hentschel, U., and Proksch, P. (2003). Monitoring microbial diversity and natural product profiles of the sponge Aplysina cavernicola following transplantation. Mar. Biol. 142, 685–692. doi: 10.1007/s00227-002-1000-9
Thoms, C., and Schupp, P. (2007). “Chemical defense strategies in sponges: a review,” in Porifera Research: Biodiversity, Innovation and Sustainability: Série Livros 28, eds M. R. Custódio, G. Lôbo-Hajdu, E. Hajdu, and G. Muricy (Rio de Janeiro: Museu Nacional), 627–637.
Unson, M. D., and Faulkner, D. J. (1993). Cyanobacterial symbiont biosynthesis of chlorinated metabolites from Dysidea herbacea (Porifera). Experientia 49, 349–353. doi: 10.1007/BF01923420
Usher, K. M. (2008). The ecology and phylogeny of cyanobacterial symbionts in sponges. Mar. Ecol. 29, 178–192. doi: 10.1111/j.1439-0485.2008.00245.x
Usher, K. M., Fromont, J., Sutton, D. C., and Toze, S. (2004). The biogeography and phylogeny of unicellular cyanobacterial symbionts in sponges from Australia and the Mediterranean. Microb. Ecol. 48, 167–177. doi: 10.1007/s00248-003-1062-3
Vacelet, J., and Donadey, C. (1977). Electron microscope study of the association between some sponges and bacteria. J. Exp. Mar. Biol. Ecol. 30, 301–314. doi: 10.1016/0022-0981(77)90038-7
Webster, N. S., Cobb, R. E., Soo, R., Anthony, S. L., Battershill, C. N., Whalan, S., et al. (2011). Bacterial community dynamics in the marine sponge Rhopaloeides odorabile under in situ and ex situ cultivation. Mar. Biotechnol. 13, 296–304. doi: 10.1007/s10126-010-9300-4
Webster, N. S., and Taylor, M. W. (2012). Marine sponges and their microbial symbionts: love and other relationships. Environ. Microbiol. 14, 335–346. doi: 10.1111/j.1462-2920.2011.02460.x
Webster, N. S., Taylor, M. W., Behnam, F., Lücker, S., Rattei, T., Whalan, S., et al. (2010). Deep sequencing reveals exceptional diversity and modes of transmission for bacterial sponge symbionts. Environ. Microbiol. 12, 2070–2082. doi: 10.1111/j.1462-2920.2009.02065.x
Webster, N. S., and Thomas, T. (2016). The sponge hologenome. MBio 7:e135-16. doi: 10.1128/mBio.00135-16
Webster, N. S., Webb, R. I., Ridd, M. J., Hill, R. T., and Negri, A. P. (2001). The effects of copper on the microbial community of a coral reef sponge. Environ. Microbiol. 3, 19–31. doi: 10.1046/j.1462-2920.2001.00155.x
Weisz, J. B., Massaro, A. J., Ramsby, B. D., and Hill, M. S. (2010). Zooxanthellar symbionts shape host sponge trophic status through translocation of carbon. Biol. Bull-US. 219, 189–197. doi: 10.1086/BBLv219n3p189
Yachi, S., and Loreau, M. (1999). Biodiversity and ecosystem productivity in a fluctuating environment: the insurance hypothesis. Proc. Natl. Acad. Sci. U.S.A. 96, 1463–1468. doi: 10.1073/pnas.96.4.1463
Yoon, S.-H., Ha, S.-M., Kwon, S., Lim, J., Kim, Y., Seo, H., et al. (2017). Introducing EzBioCloud: a taxonomically united database of 16S rRNA gene sequences and whole-genome assemblies. Int. J. Syst. Evol. Microbiol. 67, 1613–1617. doi: 10.1099/ijsem.0.001755
Youle, M., Knowlton, N., Rohwer, F., Gordon, J., and Relman, D. A. (2013). Superorganisms and holobionts. Microbe Wash. DC 8, 152–153. doi: 10.1007/s12064-018-0265-6
Keywords: HMA sponge, bacterial symbionts, holobiont, antimicrobial defense, quorum sensing, bacteriocytes
Citation: Schellenberg J, Reichert J, Hardt M, Klingelhöfer I, Morlock G, Schubert P, Bižić M, Grossart H-P, Kämpfer P, Wilke T and Glaeser SP (2020) The Bacterial Microbiome of the Long-Term Aquarium Cultured High-Microbial Abundance Sponge Haliclona cnidata – Sustained Bioactivity Despite Community Shifts Under Detrimental Conditions. Front. Mar. Sci. 7:266. doi: 10.3389/fmars.2020.00266
Received: 09 December 2019; Accepted: 02 April 2020;
Published: 19 May 2020.
Edited by:
Russell T. Hill, University of Maryland, United StatesReviewed by:
Jan Vicente, University of Maryland Center for Environmental Science (UMCES), United StatesCopyright © 2020 Schellenberg, Reichert, Hardt, Klingelhöfer, Morlock, Schubert, Bižić, Grossart, Kämpfer, Wilke and Glaeser. This is an open-access article distributed under the terms of the Creative Commons Attribution License (CC BY). The use, distribution or reproduction in other forums is permitted, provided the original author(s) and the copyright owner(s) are credited and that the original publication in this journal is cited, in accordance with accepted academic practice. No use, distribution or reproduction is permitted which does not comply with these terms.
*Correspondence: Stefanie P. Glaeser, U3RlZmFuaWUuR2xhZXNlckB1bXdlbHQudW5pLWdpZXNzZW4uZGU=
†These authors share first authorship
Disclaimer: All claims expressed in this article are solely those of the authors and do not necessarily represent those of their affiliated organizations, or those of the publisher, the editors and the reviewers. Any product that may be evaluated in this article or claim that may be made by its manufacturer is not guaranteed or endorsed by the publisher.
Research integrity at Frontiers
Learn more about the work of our research integrity team to safeguard the quality of each article we publish.