Corrigendum: Thermally Variable, Macrotidal Reef Habitats Promote Rapid Recovery From Mass Coral Bleaching
- 1Department of Freshwater and Marine Ecology, Institute for Biodiversity and Ecosystem Dynamics, University of Amsterdam, Amsterdam, Netherlands
- 2Oceans Graduate School and ARC Centre of Excellence for Coral Reef Studies, The University of Western Australia, Perth, WA, Australia
- 3The UWA Oceans Institute, Indian Ocean Marine Research Centre, The University of Western Australia, Perth, WA, Australia
- 4Department of Biology and Chemistry, University of Bremen, Bremen, Germany
- 5Trace and Environmental DNA (TrEnD) Laboratory, School of Molecular and Life Sciences, Curtin University, Perth, WA, Australia
- 6School of Environmental and Life Sciences, University of Newcastle, Callaghan, NSW, Australia
- 7Australian Institute of Marine Science, Indian Ocean Marine Research Centre, Perth, WA, Australia
Coral reefs are severely threatened by climate change and recurrent mass bleaching events, highlighting the need for a better understanding of the factors driving recovery and resilience both at the community and species level. While temperature variability has been shown to promote coral heat tolerance, it remains poorly understood whether this also influences coral recovery capacity. Similarly, few studies have investigated how the presence of cryptic species influences bleaching and recovery responses. Using an integrated ecological, physiological, and genetic approach (i.e., reef-wide coral health surveys as well as chlorophyll a concentration and cryptic species diversity of Acropora aspera), we examined the recovery of both coral communities and their dominant species from the 2016 mass bleaching event in the macrotidal Kimberley region, NW Australia. We show that recovery of coral communities inhabiting adjacent but environmentally contrasting reef habitats differed dramatically following unprecedented bleaching in 2016. Both intertidal (thermally extreme) and subtidal (thermally moderate) habitats experienced extensive bleaching (72–81%), but subtidal coral communities had a greater percentage of severely bleached corals than the intertidal community (76 versus 53%). Similarly, subtidal A. aspera corals suffered much greater losses of chlorophyll a than intertidal conspecifics (96 versus 46%). The intertidal coral community fully recovered to its prebleaching configuration within 6 months, whereas the adjacent subtidal suffered extensive mortality (68% loss of live coral cover). Despite the presence of three cryptic genetic lineages in the dominant coral species, the physiological response of A. aspera was independent of host cryptic genetic diversity. Furthermore, both intertidal and subtidal A. aspera harbored symbionts in the genus Cladocopium (previously clade C). Our findings therefore highlight the important role of tidally controlled temperature variability in promoting coral recovery capacity. While the underlying physiological and molecular mechanisms require further investigation, we propose that shallow reef environments characterized by strong environmental gradients may generally promote coral resilience to extreme climatic events. Thermally variable reef environments may therefore provide important spatial refugia for coral reefs under rapid climate change.
Introduction
Tropical coral reefs are biodiversity hotspots that provide income and resources to millions of people worldwide (Moberg and Folke, 1999); however, they are in serious decline globally due to climate change and a wide range of other stressors (Hughes et al., 2017, 2018, 2019). As recurrent mass bleaching events progressively reduce the recovery time available to coral reefs (Hughes et al., 2018), there is an urgent need to better understand the mechanisms and drivers that promote rapid recovery from extreme climatic events (Graham et al., 2011; Gouezo et al., 2019). While recovery dynamics of coral communities are commonly studied, this is rarely combined with more detailed physiological and genetic investigations of the coral taxa dominating these communities.
Reef-building corals often exist over strong environmental gradients and are characterized by wide variation in thermal tolerance (Bay and Palumbi, 2014; Palumbi et al., 2014; Dixon et al., 2015; Kenkel et al., 2015b), although their bleaching thresholds are typically only 1–2°C above their local maximum summer temperatures. Thermal tolerance can vary across latitudes and regional scales (Coles et al., 1976; Berkelmans and van Oppen, 2006; Riegl et al., 2011; Howells et al., 2013) but also over much smaller spatial gradients (<10 km), including thermally distinct habitats within a single reef (Palumbi et al., 2014; Kenkel et al., 2015a; Schoepf et al., 2015b; Barshis et al., 2018). While much attention has recently focused on how these different thermal environments shape the heat tolerance and bleaching resistance of corals (McClanahan et al., 2005; Castillo et al., 2012; Palumbi et al., 2014; Kenkel et al., 2015a; Schoepf et al., 2015b; Louis et al., 2016; Barshis et al., 2018; Safaie et al., 2018), it is poorly understood if and how high-frequency environmental variability influences coral recovery capacity. This is despite the fact that coral community studies have shown that recovery can be highly heterogeneous (Hoogenboom et al., 2017) and habitat specific (McClanahan and Maina, 2003; Golbuu et al., 2007; Le Nohaïc et al., 2017), for example due to differences in local environmental conditions and community composition. In addition, specific biological traits, such as the type of algal symbionts, often play an important role in influencing bleaching resistance and recovery (Stat and Gates, 2011; Putnam et al., 2012; Grottoli et al., 2014; Silverstein et al., 2015). Finally, unrecognized species diversity can mask differences in functional ecology, including microhabitat distributions and bleaching resistance (Boulay et al., 2014; Rose et al., 2017), and although it is increasingly being recognized that many coral species may in fact consist of several cryptic species (Knowlton, 1993; Souter, 2010; Ladner and Palumbi, 2012; Warner et al., 2015), this is rarely considered in studies investigating coral responses to and recovery from bleaching.
Thermally variable and extreme reef environments, such as back-reef environments and tide-dominated reefs (Brown et al., 2000; Palumbi et al., 2014; Schoepf et al., 2015b; Camp et al., 2018), have provided important insights into the mechanisms underlying coral heat tolerance. Therefore, these systems also have the potential to advance our understanding of how corals living in such environments recover from heat stress events. Here, we examined the divergent recovery responses of coral communities in adjacent reef habitats following an unprecedented mass bleaching event in the macrotidal Kimberley region in NW Australia in 2016 (Le Nohaïc et al., 2017; Gilmour et al., 2019). Shallow coral reefs in this region are subject to tidally induced (up to 12-m tidal range), extreme environmental gradients (e.g., temperature, light, and aerial exposure) that fluctuate strongly across multiple temporal and spatial scales (Dandan et al., 2015; Schoepf et al., 2015b; Gruber et al., 2017; Cornwall et al., 2018). Using an integrated ecological, physiological, and genetic approach, we compared the recovery capacity of two reef habitats with distinct environmental conditions at low tide (Figure 1): (i) an environmentally extreme and thermally variable intertidal pool where corals regularly get exposed to air and have a naturally elevated heat tolerance and (ii) a thermally moderate subtidal reef with less heat-tolerant corals (Dandan et al., 2015; Schoepf et al., 2015b). We combined reef-wide ecological surveys with physiological and genetic tissue analyses of the dominant coral species, Acropora aspera, to explore drivers of recovery capacity. We hypothesized that (1) recovery capacity differs between habitats due to differences in environmental conditions (especially temperature variability); (2) intertidal corals recover better from bleaching than subtidal corals, as demonstrated by lower levels of mortality (reef-wide surveys) and higher chlorophyll a levels (A. aspera); (3) cryptic species diversity is present in A. aspera and linked to differential bleaching resilience across habitats; and (4) Symbiodiniaceae communities do not differ between intertidal versus subtidal, as reported in previous work (Schoepf et al., 2015b).
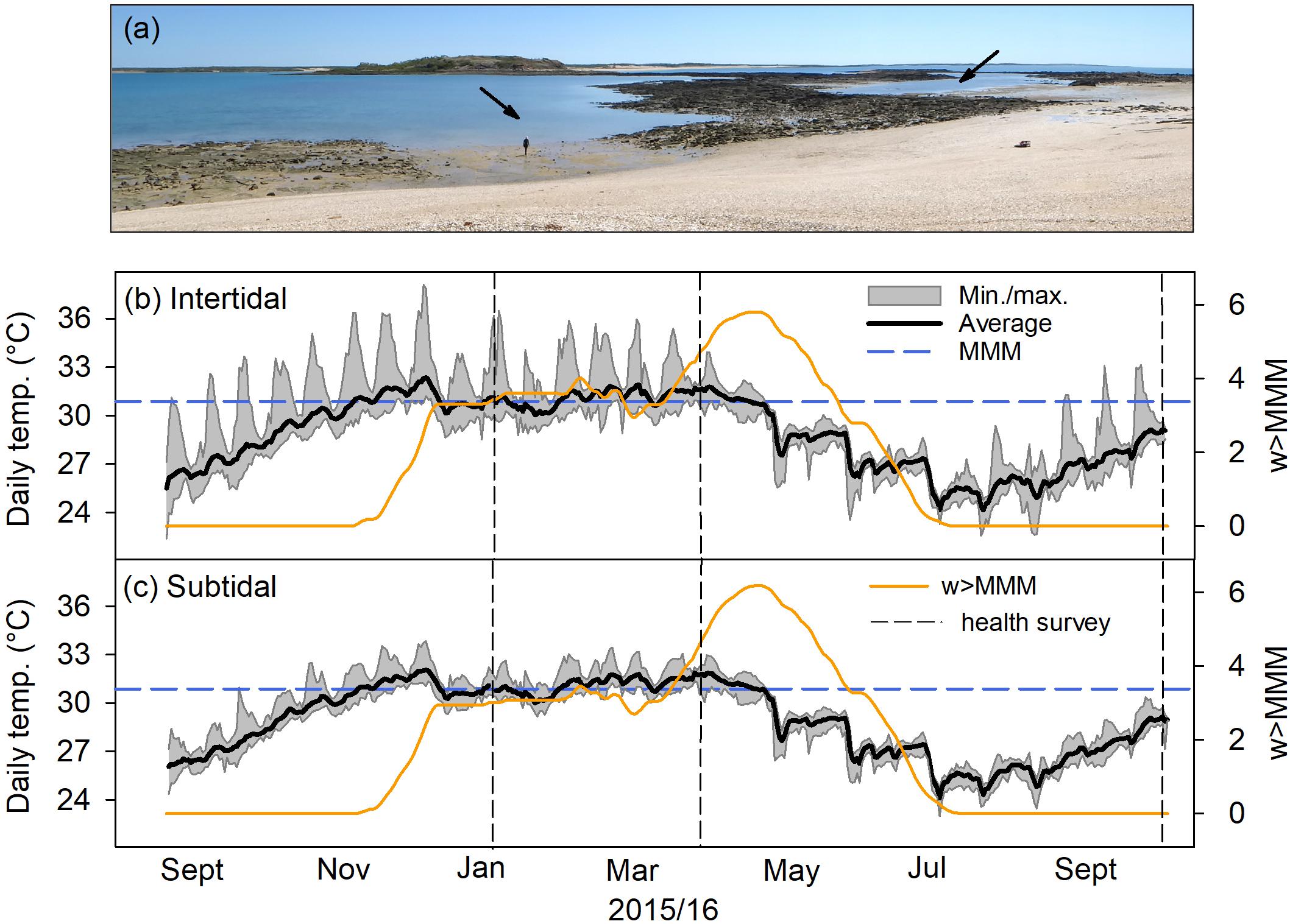
Figure 1. Temperature and heat stress exposure at intertidal and subtidal environments. (a) The subtidal (arrow center left) and intertidal (arrow upper right) at Shell Island, Kimberley region at mid- to low tide. Arrow indicated approximate area where surveys and coral collection was conducted. (b) Daily average, minimum, and maximum temperature in the intertidal from September 1, 2015 to October 18, 2016. The blue dashed lines show the local maximum monthly mean (MMM) temperature. Orange solid lines indicate weekly cumulative heat stress above the local MMM (w > MMM; see section “Materials and Methods”). Vertical dashed lines indicate when coral health was assessed in reef-wide surveys. (c) Same as in (b) but for the subtidal.
Materials and Methods
Study Site
Our study site was located at Shell Island (Shenton Bluff), Cygnet Bay, in the macrotidal Kimberley region of NW Australia (Figure 1a). Shell Island has a tidal range of ∼8 m, which creates extreme environmental gradients across small spatial scales resulting in a mosaic of environmentally different habitats depending on tidal exposure (Dandan et al., 2015; Schoepf et al., 2015b). The intertidal environment (16°28′ 45.8″ S, 123°2′ 41.3″ E) is a small shallow pool (ca. 200 m × 100 m, Figure 1a) that becomes isolated from the surrounding waters of King Sound during low tide (minimum depth, ∼20–30 cm; average depth, ∼3 m; maximum depth, ∼7 m). Although the pool retains at least 20–30 cm of water during spring low tides, the upper parts of coral colonies growing there regularly get exposed to air for up to several hours. The associated slack water period lasts for up to 4 h, with corals experiencing a combination of stagnant flow conditions, high light levels (up to 2,400 μmol m–2 s–1) and short-term maximum temperatures of up to 37°C (Dandan et al., 2015; Schoepf et al., 2015b). In contrast, the nearby subtidal environment (16°28′ 46.8″ S, 123°2′ 36.6″ E; within 200–300 m of the intertidal; minimum depth, 0 cm; average depth, ∼4 m; maximum depth, ∼8 m) represents a less extreme environment (Figure 1a) where corals experience maximum light levels of up to 1,800 μmol m–2 s–1 and more moderate temperatures, although average temperatures in the subtidal are the same as in the intertidal. Corals in the subtidal environment are typically not exposed to air during low tides, except during the most extreme spring low tides (i.e., only a few days per year). Short-term maximum temperatures (Figure 1) as well as daily temperature variability differ strongly between intertidal and subtidal, with intertidal corals being exposed to up to 7°C daily temperature variability, whereas subtidal corals only experience up to 3°C daily variability (Schoepf et al., 2015b). Both intertidal and subtidal environments feature diverse coral communities dominated by branching Acropora spp. (Le Nohaïc et al., 2017); however, intertidal corals have a higher heat tolerance than subtidal corals (Schoepf et al., 2015b; Le Nohaïc et al., 2017).
Reef-Wide Coral Health Surveys and Environmental Monitoring
In the austral summer of 2016, a marine heatwave associated with strong El Niño conditions caused unprecedented mass bleaching in NW Australia, including the Kimberley region (Le Nohaïc et al., 2017; Gilmour et al., 2019; but see Richards et al., 2019). To quantify coral recovery and mortality following this bleaching event, reef-wide coral health surveys were conducted at Shell Island 6 months after peak bleaching from 18 to 21 October 2016 using the same methods that were used by Le Nohaïc et al. (2017) to assess coral health prior to and during peak bleaching (January 13–17 and April 6–9, 2016, respectively). Surveys were conducted along six randomly positioned, 15 m transects in each of the intertidal and subtidal environments. High-resolution photos of a 50-cm × 50-cm quadrat were taken every 0.5–1 m along the transect line. Photo-quadrats were analyzed using the software (Trygonis and Sini, 2012). All species of hard corals encountered in the photo-quadrats were scored using the following four health categories as a categorical bleaching score (McClanahan et al., 2004): unbleached/healthy (H), moderately bleached (M: <50% of the colony bleached or colony pale), severely bleached (S: >50% bleached), and dead (D).
From September 2015 until October 2016, water temperature, water level, and photosynthetically active irradiance (PAR) were recorded in both intertidal and subtidal environments. Water temperature was recorded every 15 min by HOBO U22 v2 temperature loggers (±0.2°C) in both intertidal and subtidal environments. To assess cumulative heat stress, we calculated the days when daily average temperature exceeded the local maximum monthly mean (MMM) temperature over the previous 12 weeks from September 1, 2015 until October 18, 2016 and then accumulated the positive temperature anomalies from these days. This value was then divided by seven to calculate the metric “w > MMM,” which can easily be compared to the National Oceanic and Atmospheric Administration’s (NOAA’s) degree heating week (DHW) product, except that our metric calculates the sum of all positive temperature anomalies exceeding the local MMM, whereas DHW only represents the sum of the positive temperature anomalies exceeding the local MMM by more than 1°C. This new metric “w > MMM” was developed because it provides more realistic estimates of heat stress in the complex macrotidal environment at our study site than NOAA’s DHW methodology (Le Nohaïc et al., 2017); however, in contrast to Le Nohaïc et al. (2017), we here chose to rename the metric to avoid confusion with the widely used DHW terminology. Bleaching thresholds for both intertidal and subtidal corals were previously experimentally established to be ∼32°C (Schoepf et al., 2015b), ∼1°C above the local MMM of 30.827°C from NOAA’s 5-km virtual station North Western Australia (version 2).
Water level was monitored continuously over the same time period at both sites using HOBO U20-001-02-Ti water level loggers (±0.05%) and RBR virtuoso water level loggers (±0.05%). Downwelling planar PAR was measured at each site for a few days over a spring tide at three time periods in 2016 (12–17 January, 6–8 and 10–12 April, 17–20 October) using Odyssey light loggers. No light data are available from January 2016 due to the logger malfunctioning. Each of the Odyssey loggers was calibrated under water against a factory-calibrated LiCor PAR sensor. All loggers were deployed on tripods ∼20 cm above the benthos.
Physiological Analyses
In addition to the community surveys, we also tagged visibly healthy and pale/bleached colonies of the dominant coral species at our study site, A. aspera, which is widespread on shallow reef habitats in both the Kimberley (Richards et al., 2015) and Indo-Pacific. Five to 10 visibly healthy and 5–10 pale/bleached colonies were tagged in both intertidal and subtidal environments during peak bleaching (April 2016) using cattle tags epoxied to the coral (Z-Spar). The health status of all tagged colonies was assessed in April 2016 and after 7 months of recovery in November 2016 using the Coral Watch® Coral Health Chart where a change of two units in brightness indicates a significant change in symbiont density and chlorophyll a content (Siebeck et al., 2006). Colonies were considered either “healthy” (brightness scale, 3.6–6) or “bleached” (brightness, 1–3.5). Replicate branch tips (∼3 cm) were collected from the upper part of all tagged colonies in April and November 2016 for physiological and genetic analyses (see below). However, by November 2016, several of the (mostly bleached) tagged colonies had died or could not be relocated, which led to reduced sample sizes for this time point (Supplementary Table S1). Corals were collected using exemption #2549 from the Western Australia Department of Fisheries.
Corals were stored at −80°C prior to processing. To quantify bleaching, chlorophyll a concentration was determined spectrophotometrically (Jeffrey and Humphrey, 1975) and used as a proxy for bleaching susceptibility. Tissue was removed from a branch tip using an airbrush and separated into animal and symbiont fraction via centrifugation (2 × 10 min at 3,000 g). Chlorophyll a from the symbiont fraction was extracted in 100% acetone in the dark at 4°C for 24 h and the concentration determined spectrophotometrically (Jeffrey and Humphrey, 1975) and then standardized to surface area. Surface area was calculated using the relationship between skeletal mass (x, in g) and the respective computer tomography (CT)-determined surface area (y, in cm2) of A. aspera skeletons from our study site (y = 9.4871x0.7729, n = 6, R2 = 0.99).
Genetic Analyses
Acropora aspera forms a cryptic species complex in northwest Australia, with at least four distinct genetic lineages occurring in sympatry on reefs in the Kimberley (Underwood et al., 2017). To determine if the presence of morphologically cryptic genetic lineages within our dataset influenced the bleaching and recovery responses across the two reef habitats, we used a shallow shotgun sequencing approach and generated ∼1 M paired end reads per sample and aligned them using bwa mem to the complete mitochondrion of A. aspera (GenBank accession NC_022827). This shallow sequencing approach does not provide genome-wide coverage and instead makes use of the higher copy number of mitochondrial DNA and requires only a fraction of the sequencing depth. DNA was extracted from 32 samples (16 per environment) for the April 2016 timepoint using a Qiagen DNeasy blood and tissue kit, and Illumina compatible libraries were generated using Nextera DNA Library Prep Kits at 1/20 of the manufacturers’ recommended volumes as in Therkildsen and Palumbi (2017). We used samtools to sort and index and picard tools (Li et al., 2009) to remove duplicate reads. We estimated allele frequencies in each population based on genotype likelihoods in Angsd (Korneliussen et al., 2014). We masked sites with minQ < 10, minMapQ < 10, maf < 0.05, and a snp_value >e-6. The resulting covariance matrix was used to carry out a principle components analysis (PCA) in R using the eigen functions (R base package). We also explored clustering of the data using ngsadmix (Skotte et al., 2013) to identify admixture proportions among samples assigned to predefined clusters, restricting our analyses to loci scored in >95% of individuals. Mapped mitochondrial reads were also used to generate a consensus sequence for each sample using bcftools, and popart was used to build a TCS haplotype network. Since the DNA was extracted from whole coral samples (coral host + dinoflagellate symbiont), we were also able to opportunistically explore differences in Symbiodiniaceae communities among samples for the April 2016 time point. We followed a similar approach as outlined above, but aligned our shotgun sequence data to a reference file containing a representative ITS2 haplotype for different genera (clades A–D) in the family Symbiodiniaceae. We calculated the proportion of reads that uniquely mapped to each reference sequence as a measure for symbiont composition.
Statistical Analyses
Coral Health Surveys
Coral health and community composition was analyzed using permutational multivariate analysis of variance (PERMANOVAs). Prior to multivariate statistical analysis, count data were converted to percent abundance and square root transformed. The four health categories (UB, M, S, and D) across all coral genera were statistically tested for differences between environments (intertidal, subtidal) and time points (January, April, and October 2016) using two-way PERMANOVAs, the Bray–Curtis similarity index, and 9999 permutations. Transects served as replicates. Six replicate transects were used for all environments and time points, except in the intertidal in January 2016 where n = 7 transects were conducted (Le Nohaïc et al., 2017). Since PERMANOVA is largely unaffected by heterogeneity of multivariate dispersions and differences in correlation structure in balanced designs (Anderson and Walsh, 2013), we did not specifically test for this when the design was balanced (i.e. all analyses not involving the intertidal January 2016 time point). For analyses involving the intertidal January 2016 time point, we conducted a permutation test for homogeneity of multivariate dispersions using the betadisper function of the vegan package in R (Oksanen et al., 2016) with 999 permutations. The tests revealed that the assumption of homogeneity of multivariate dispersions was indeed fulfilled for both PERMANOVAs that involved the “intertidal January 2016” group (Table 1).
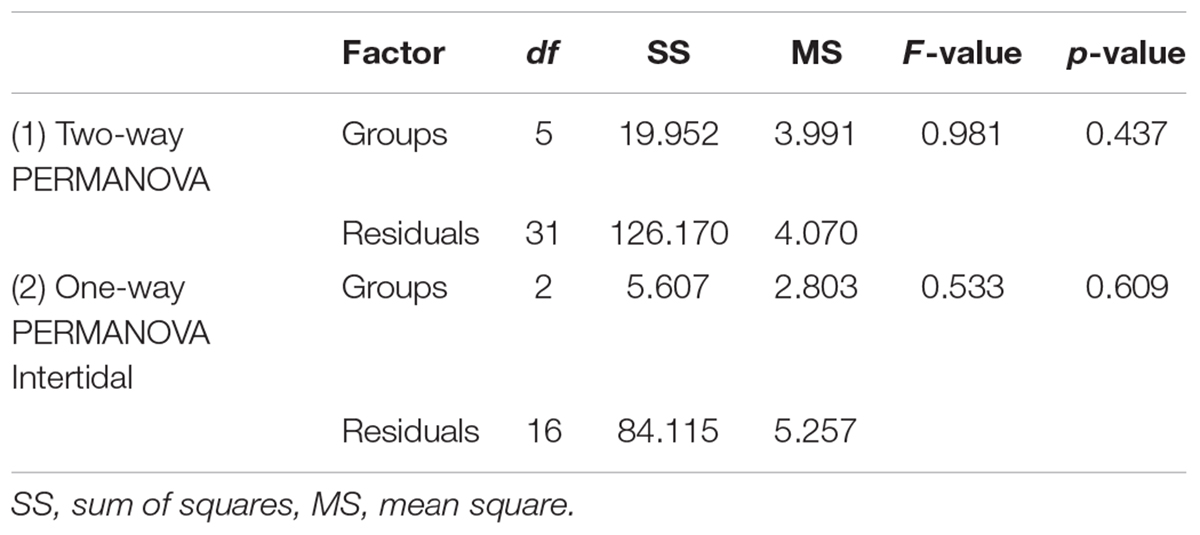
Table 1. Results from tests for homogeneity of multivariate dispersions for PERMANOVAs assessing (1) the effect of environment and time on coral health across all surveyed coral genera and (2) the effect of time on coral health across all surveyed coral genera in the intertidal only.
Additional one-way PERMANOVAs were conducted to (1) to test for the effect of time on coral health across all genera in the intertidal and subtidal, respectively and (2) to compare intertidal and subtidal at the recovery time point (October). Post hoc pairwise comparisons were calculated, with p values adjusted using the sequential Bonferroni correction. Principal component analysis was used to visualize the data. The software PAST was used for the PERMANOVA and PCA analyses (Hammer et al., 2001).
Physiological Analyses
Since three cryptic genetic lineages were identified (see section “Results”), the effect of genetic lineage on coral chlorophyll a concentration was assessed using generalized linear mixed model (GLMM) analysis. Generalized linear mixed model analyses were conducted for (1) corals from all three lineages and (2) corals from the two main lineages only, to see if the small sample size of the third lineage (n = 3) affected the robustness of this analyses. As there was no significant effect of genetic lineage on chla concentration in either case (Supplementary Table S2), further statistical analysis to test for the effect of environment, health, and time on chla concentration was conducted for corals pooled from all three lineages (see below). However, chla data for the dominant lineage only are also presented in the Supplementary Material to facilitate comparison (Supplementary Figure S2). Prior to GLMM analysis of the chla a data, the distribution of the residuals was visually assessed, and the data were square root transformed to meet assumptions associated with GLMM analysis.
Generalized linear mixed model analysis was then also used to test for the effect of health (healthy and bleached as determined in April 2016), environment (intertidal, subtidal), and time (April, November 2016) on chla concentration. Tukey adjusted p values were used for post hoc tests when main effects were significant. When a significant interaction was observed, multiple pair-wise comparisons were conducted using Tukey adjusted p values. Differences between healthy and bleached corals in their respective environments were tested a priori. Generalized linear mixed model analyses were performed using SAS. p values ≤0.05 were considered significant.
Results
Recovery Responses of Intertidal Versus Subtidal Coral Communities
Coral community health surveys conducted before, during, and 6 months after the 2016 bleaching event revealed strong differences in survival and recovery across small spatial scales (hundreds of meters) (Figure 1 and Table 1). The intertidal coral community suffered 72% bleaching (±5 SE; moderately and severely bleached corals combined) but mostly recovered within 6 months with little mortality (9% ± 5 SE) (Figure 2). In stark contrast, the large majority of the bleached subtidal coral community ultimately died (71% ± 11 SE) (Figure 2) although being separated by only 200–300 m and experiencing a similar though slightly higher degree of bleaching (81% ± 4 SE versus 72% ± 5 SE for intertidal). Thus, live coral cover in the intertidal was maintained at essentially prebleaching levels (6% proportional decrease in coral cover), whereas in the subtidal, it dropped by 68%, resulting in a degraded reef dominated by dead Acropora spp. corals that differed significantly from its prebleaching state (Figure 2A and Table 1).
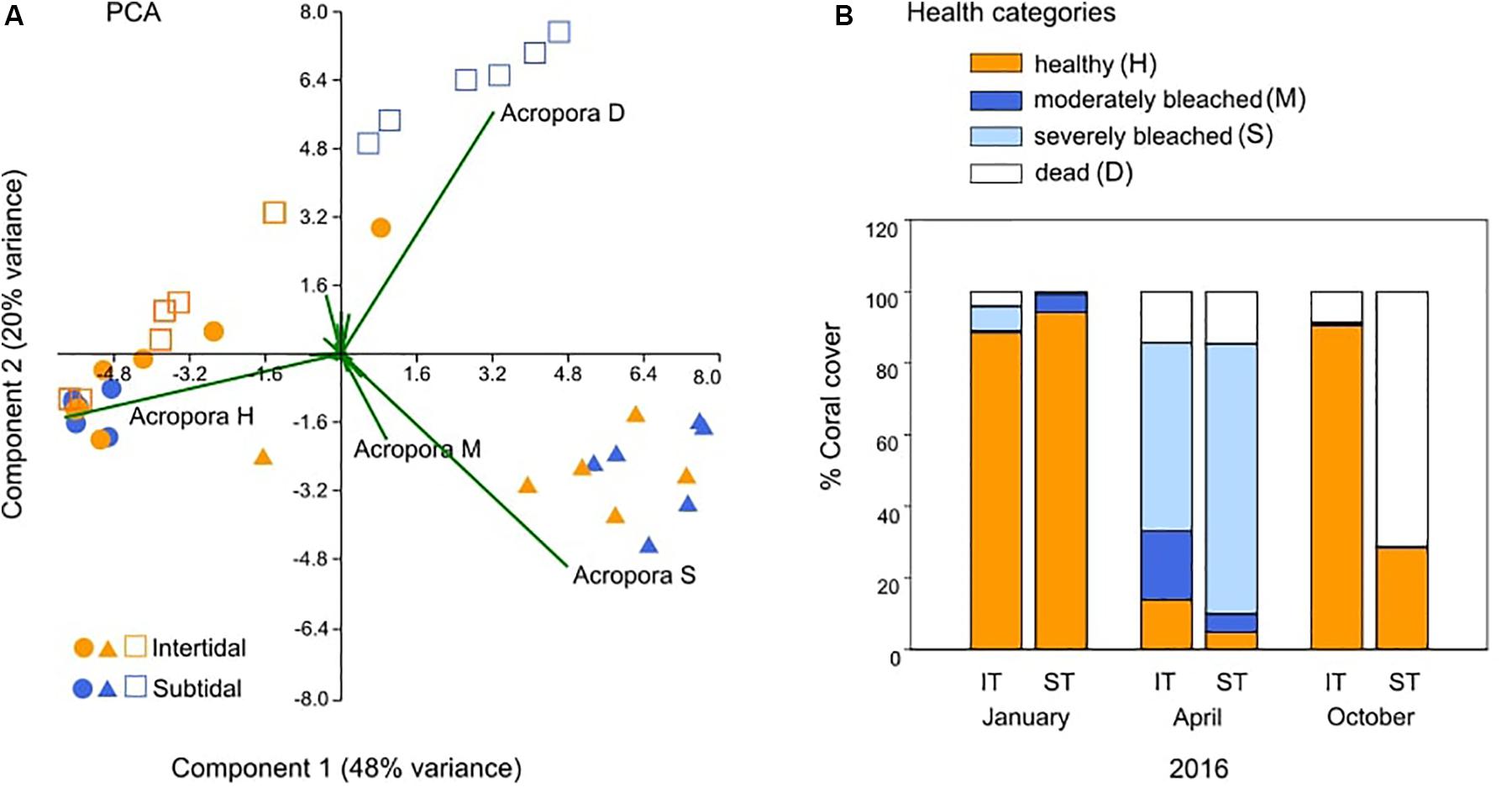
Figure 2. Changes in reef-wide coral health over time based on coral health surveys. (A) Principal components analysis (PCA) of coral health across all hard coral genera in the intertidal (IT) and subtidal (ST) before (January, •), during (April, ▲), and 6 months after (October, □) a bleaching event in 2016. Vectors represent the dominant coral genus Acropora and its associated health status (see below) because it had the greatest influence on overall coral health. (B) Percent coral cover that was healthy (H), moderately bleached (M), severely bleached (S), or dead (D) at the same time points. Data for January and April 2016 are also included in Le Nohaïc et al. (2017).
Despite the different recovery responses, intertidal and subtidal coral communities had a similar composition prior to bleaching and experienced similar levels of heat stress exposure. Both reef environments were strongly dominated by healthy Acropora spp. corals prior to bleaching (Figure 2A); thus, the bleaching and recovery response of this genus largely determined the overall recovery trajectory within each environment. Similarly, heat stress in both reef environments accumulated along a similar trajectory, reaching 4.5 and 4.3 w > MMM in the intertidal and subtidal during peak bleaching, respectively (April 2016; Figures 1b,c). w > MMM values ultimately peaked in early May 2016 with 5.8 and 6.2 w > MMM in the intertidal and subtidal, respectively, and declined in a similar manner in both environments as temperatures seasonally decreased throughout autumn and winter (Figures 1b,c). However, daily temperature variability was generally much greater in the intertidal compared to the subtidal, with temperatures reaching short-term maxima of up to 38.1°C in the intertidal, yet only 33.8°C in the subtidal (Figures 1b,c).
Light levels across spring tides were not consistently higher or more variable in the intertidal, as this depended on season (Supplementary Figure S1), even though average water depth is generally ∼1 m lower in the intertidal compared to the subtidal (IT, 3.07 m ± 1.82 SD; max = 6.77 m; ST, 4.07 m ± 1.89, max = 7.89 m). In April 2016, both intertidal and subtidal corals experienced average daily light intensities of ∼500 μmol m–2 s–1 (IT, 514 ± 519; SD, 541 ± 571), and maximum light intensities of 1,990 and 2,115 μmol m–2 s–1, respectively (Supplementary Figure S1a). In October 2016, intertidal and subtidal corals experienced similar but slightly lower average light intensities of ∼400 μmol m–2 s–1 than in April (IT, 442 ± 426; SD, 406 ± 388), and maximum light intensities were also lower with 1,573 and 1,357 μmol m–2 s–1, respectively (Supplementary Figure S1b).
Cryptic Genetic Diversity and Symbiont Communities of Tagged A. aspera Corals
Using a shallow sequencing approach, we mapped 36,717,172 reads from 32 A. aspera colonies to the complete A. aspera mitochondrion (18,479 bp). Mapped mitochondrial reads for each sample ranged from 6 to 110x coverage (mean, 35x ± 4.5 SE). We calculated genotype likelihoods at 78 variable sites spread across the mitogenome. The majority of these variant sites occurred in cytochrome c oxidase I (CO1, n = 17) and control region (CR, n = 22) (Figure 3A). Using various clustering methods, we identified at least three distinct genetic lineages in our dataset (Figure 3). While one lineage was clearly more abundant than the others (group 3), all three lineages co-occurred in intertidal and subtidal environments and were comprised of both bleached and healthy coral colonies (Figure 3B). Furthermore, the chlorophyll a concentration of the A. aspera complex was not significantly influenced by host genetic lineage (Supplementary Table S2). We therefore analyzed the effects of environment, health, and time on chlorophyll a concentration pooled across all lineages (see below). Mapping reads to a list of dominant symbiont ITS2 haplotypes identified that all A. aspera colonies in both habitats were dominated by Cladocopium (previously clade C; LaJeunesse et al., 2018). These symbiont results need to be interpreted cautiously as we recovered few reads per sample (24 ± 3.5 SE) that mapped to our symbiont reference file.
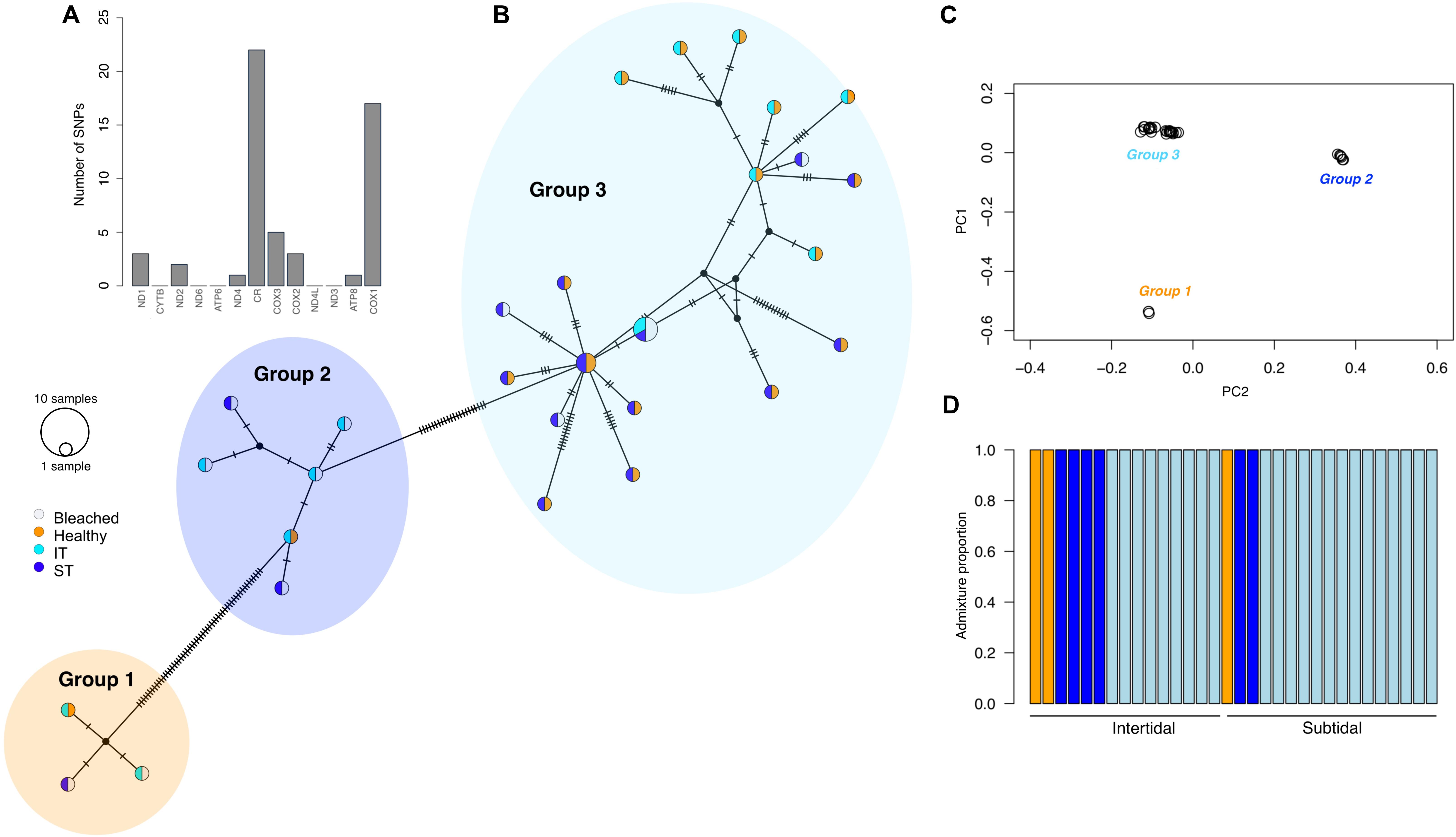
Figure 3. Cryptic genetic diversity in the Acropora aspera species complex on Shell Island, Kimberley. (A) Barplot of number of variant sites found in each gene of the A. aspera mitochondrion; (B) TCS network of the complete mitochondrial genome consensus sequences for each sample; (C) principal components analysis and (D) admixture plot showing the presence of three clear genetic lineages in the colonies (vertical bars) that were tagged during peak bleaching (April 2016).
Chlorophyll a Concentrations and Survival of Tagged A. aspera Corals
Tissue samples collected during and 7 months after bleaching revealed that the physiological bleaching and recovery response of A. aspera was characterized by significant interactive effects of environment, health status, and time (Supplementary Table S3). Although coral community health surveys showed a relatively similar overall extent of bleaching for both reef habitats, chlorophyll a analyses revealed that intertidal A. aspera bleached less severely than subtidal A. aspera, losing only 46% of their area-normalized chlorophyll a concentration compared to 96% in subtidal corals (Figure 4A). This relatively higher bleaching resistance was also observed in the community-wide surveys as reflected in the lower percentage of severely bleached corals (53%) in the intertidal coral community compared to the subtidal community (76%) (Figure 2B).
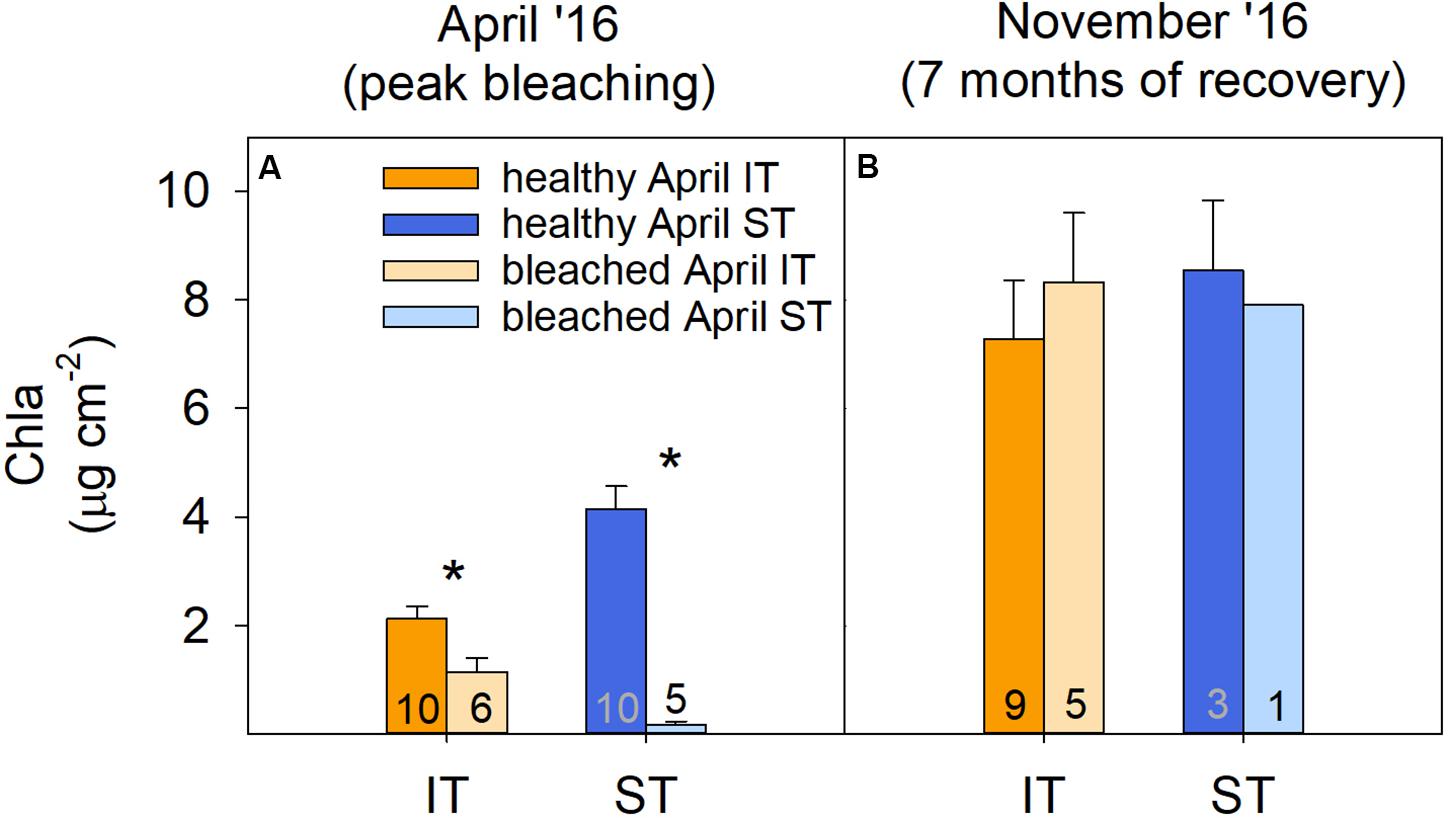
Figure 4. Coral physiology. Chlorophyll a concentration of intertidal (IT) and subtidal (ST) Acropora aspera (all genetic lineages) in (A) April and (B) November 2016. Asterisks indicate significant differences between healthy and bleached/recovering corals within a specific environment and time point. Numbers indicate sample size per treatment. Note that only one of the tagged bleached subtidal corals survived.
Survival of tagged A. aspera differed strongly between environments, with 83% (n = 5) of bleached colonies surviving in the intertidal, yet only one bleached colony (20%, n = 1) surviving in the subtidal, thus strongly mirroring the trends observed in the coral community surveys (Figure 2). However, among the surviving colonies, chlorophyll a concentrations of previously bleached corals were no longer significantly lower compared to the healthy coral and had, thus, fully recovered 7 months after peak bleaching in both intertidal and subtidal environments (Figure 4B).
Discussion
As coral reefs face more frequent mass bleaching events and reduced recovery times (Hughes et al., 2017, 2018), attention has increasingly focused on how different thermal environments shape the bleaching resistance of corals (McClanahan et al., 2005; Castillo et al., 2012; Palumbi et al., 2014; Kenkel et al., 2015a; Schoepf et al., 2015b; Louis et al., 2016; Barshis et al., 2018; Safaie et al., 2018). However, if and how recovery from heat stress events is influenced by environmental variability has received much less attention. We show here that strong environmental variability associated with a naturally extreme, macrotidal reef site not only increases coral heat tolerance as has been shown previously for other thermally variable reefs (McClanahan and Maina, 2003; Oliver and Palumbi, 2011; Palumbi et al., 2014; Schoepf et al., 2015b; Safaie et al., 2018) but also promoted rapid recovery of coral communities from mass bleaching.
Thermally Variable Reef Habitats Promote Rapid Recovery From Mass Bleaching
Bleaching and recovery responses of coral communities in adjacent reef habitats differed strongly across fine-scale but extreme environmental gradients following unprecedented mass bleaching in the macrotidal Kimberley region of NW Australia in 2016. Both intertidal and subtidal reef habitats experienced extensive, severe bleaching during peak heat stress (Le Nohaïc et al., 2017), with 72 and 81% of corals bleached, respectively (Figure 2B). However, the subtidal coral community had a greater percentage of severely bleached corals than the intertidal community (76 versus 53%), indicative of lower heat tolerance as observed in previous experimental work (Schoepf et al., 2015b). These differences in community-wide heat tolerance were further corroborated by the physiological analyses of tagged A. aspera corals, the dominant coral species at our study site: bleached subtidal corals lost 96% of their area-normalized chlorophyll a concentration compared to their healthy-looking counterparts, whereas intertidal bleached corals lost only 46% of their chlorophyll a concentration (Figure 4).
Surveys conducted 6 months after peak bleaching further revealed dramatic differences in survival and recovery. The intertidal coral community was able to fully recover to its prebleaching health configuration within 6 months and only lost 6% of live coral cover (Figure 2 and Table 1). In contrast, the subtidal community only 200–300 m away suffered extensive mortality (71%), loss of live coral cover (-68%), and reduced framework complexity driven by death and overgrowth of branching Acropora corals, resulting in a significantly different community configuration compared to prebleaching (Figure 2 and Table 1). The markedly distinct recovery responses contradict results from a meta-analysis, finding no evidence for reef zone impacting recovery rates (Graham et al., 2011) but are consistent with other studies showing habitat-dependent reef recovery. For example, thermally variable Kenyan reef habitats suffered less mortality and changes in community composition than thermally less variable habitats after the 1998 bleaching event (McClanahan and Maina, 2003). Similarly, sheltered bays in Palau suffered less mortality and recovered better from the 1998 and 2010 bleaching events than other habitats due to naturally higher temperatures and lower light levels (Golbuu et al., 2007; van Woesik et al., 2012). Our findings also agree with recent experimental work showing that two genera of intertidal Kimberley corals (Acropora and Dipsastraea) had a higher bleaching resistance and survival rate than their subtidal counterparts (Schoepf et al., 2015b). Given that the two habitats experienced similar heat stress exposure and were both dominated by heat-sensitive Acropora corals (Le Nohaïc et al., 2017), this points to the more extreme environmental conditions, particularly greater daily temperature fluctuations (Oliver and Palumbi, 2011; Schoepf et al., 2015b; Safaie et al., 2018) and/or high light levels (Brown et al., 2000, 2002) in the intertidal, playing a key role in promoting coral heat tolerance (Schoepf et al., 2015b) and recovery capacity (this study). Since our findings are consistent with those from other reefs characterized by environmental variability (McClanahan and Maina, 2003; Golbuu et al., 2007; van Woesik et al., 2012), we propose that strong, fine-scale environmental gradients may be significant drivers of coral recovery from mass bleaching.
The 2016 bleaching event is the first documented mass bleaching event in the inshore Kimberley region (Le Nohaïc et al., 2017; Gilmour et al., 2019), highlighting that global warming is increasingly also impacting remote coral populations with naturally high stress tolerance (Dandan et al., 2015; Schoepf et al., 2015b), although some areas seem to have escaped bleaching (Richards et al., 2019). This bleaching event coincided with an extremely unusual and dry wet season in the Kimberley (Le Nohaïc et al., 2017), high local night-time temperatures (Richards et al., 2019), and the most extreme tides of the year; thus, it is likely that the presumably increased light and UV stress as well as aerial exposure contributed to the extensive mortality observed in the more heat-sensitive subtidal corals. However, the high survival and rapid recovery of the intertidal community is expected to enhance the longer-term recovery of the subtidal coral community via recruitment, even though we note that bleaching can have negative impacts on coral reproduction (e.g., Szmant and Gassman, 1990).
Limited Influence of Host Cryptic Genetic Diversity and Symbiont Genus on Recovery Responses
Our study showed that the divergent recovery responses of intertidal and subtidal coral communities were not significantly influenced by the presence of cryptic genetic diversity in the dominant coral species, A. aspera. Using a low-pass sequencing approach and mapping reads to the A. aspera mitochondrion, we identified three cryptic genetic lineages in our dataset that cannot be distinguished based on colony morphology; however, neither lineage was associated with a specific habitat nor displayed particular susceptibility/resistance during bleaching. This is in contrast to other cryptic coral species found to have different environmental niches and/or stress tolerance (Boulay et al., 2014; Rose et al., 2017). We caution, however, that two of the lineages were represented by only a small number of samples. Environmentally extreme habitats may favor cryptic species diversity because evolving under extreme conditions can constrain morphological change, thus resulting in morphological stasis (Bickford et al., 2007). Therefore, our study adds macrotidal reef environments to a growing list of extreme environments, such as underwater karst (Lefebure et al., 2006) or deep-sea environments (Vrijenhoek et al., 1994), which support significant cryptic species diversity.
Corals from the A. aspera complex from both intertidal and subtidal habitats harbored symbionts from the broadly distributed genus Cladocopium, which is consistent with other work at our study site (Schoepf et al., 2015b) and in the north Kimberley region (Thomas et al., 2014). However, these findings differ from other thermally extreme reef habitats, such as the back-reef pools in American Samoa, where higher proportions of Durusdinium symbionts (LaJeunesse et al., 2018) were found in the pool with higher and more variable temperatures (Palumbi et al., 2014). The genus Cladocopium comprises many physiologically diverse species, which are often locally adapted to a range of environmental conditions (Fisher et al., 2012; LaJeunesse et al., 2018), including high temperatures (Howells et al., 2011). Because our shallow sequencing approach could only be used to characterize genus level differences in symbiont communities, it is possible that intertidal and subtidal corals in our study hosted different species of Cladocopium and/or that shifts in dominant symbiont genus or species occurred during recovery (Grottoli et al., 2014; Silverstein et al., 2015). Alternatively, the same species of Cladocopium could be locally adapted to the different environmental regimes (Howells et al., 2011). However, a growing body of literature suggests that resistance to high temperatures (though not light) can be strongly mediated by the coral host (Baird et al., 2008) and is, thus, often (but not always) independent of the symbiont (Barshis et al., 2010, 2018; Bellantuono et al., 2012; Palumbi et al., 2014). Analysis of finer-scale symbiont dynamics at greater taxonomic and temporal resolution was beyond the scope of this study, but our findings provide a framework for future research investigating this topic.
Conclusion
In summary, we show here that strong daily temperature fluctuations promoted rapid recovery of an intertidal coral community from mass bleaching and return to prebleaching configurations in a macrotidal, shallow reef system. Our integrated ecological, physiological, and genetic approach revealed that the divergent responses of intertidal and subtidal reef habitats to the 2016 bleaching event were largely independent of host cryptic genetic diversity and association with certain symbiont genera in the dominant coral species. This suggests that the presence of tidally induced strong environmental gradients at our study site led to local adaptation and/or acclimatization of the coral holobiont to the different environmental conditions in the intertidal and subtidal reef habitat. We caution, however, that we did not resolve symbiont type to species level, and two of the three cryptic lineages were only represented in a small number of samples. Furthermore, we were not able to investigate any signals of local adaptation in the nuclear genome since our study focused on the mitogenome. Future research is needed to investigate other traits associated with increased recovery capacity, such as high levels of energy reserves (Grottoli et al., 2014; Schoepf et al., 2015a) or heterotrophic plasticity (Grottoli et al., 2006; Connolly et al., 2012), as well as the evolutionary and possible genetic mechanisms underlying the higher bleaching resilience of intertidal corals.
While the macrotidal reef site investigated here represents a naturally extreme environment, such thermally extreme reef habitats have significantly advanced our understanding of the mechanisms underlying climate change resilience (e.g., Palumbi et al., 2014). Furthermore, improved recovery from bleaching has also been observed in other reefs with higher or more variable temperatures (McClanahan and Maina, 2003; Golbuu et al., 2007; van Woesik et al., 2012), although temperatures on those reefs are much less extreme than at our study site. Our findings therefore highlight the important role that tidally controlled temperature variability can play in promoting coral heat tolerance (Schoepf et al., 2015b; Safaie et al., 2018). While the underlying physiological and molecular mechanisms require further research, we propose that shallow reef environments characterized by strong environmental gradients may generally promote the resilience of local coral populations to extreme climatic events. They may therefore provide critical refugia and spatial resilience to recurrent mass bleaching events while also providing stocks of stress-resilient coral populations that could be targeted for new management approaches (Morikawa and Palumbi, 2019; Schoepf et al., 2019).
Data Availability Statement
The datasets presented in this study can be found in online repositories. The names of the repository/repositories and accession number(s) can be found below: (1) Open Science Framework (doi: 10.17605/OSF.IO/T6CSG); (2) Batch scripts are available at https://github.com/molecular-ecology-WestOz/kimberley_intertidals; (3) All other raw data will be submitted to www.pangea.de after publication.
Author Contributions
VS designed the experiments and conducted the field work. MJ analyzed the coral health surveys and conducted the physiological analyses. LT and NW conducted the genetic analyses. VS, MJ, and LT conducted the statistical analyses. All authors provided feedback and contributed to the final manuscript.
Funding
Funding was provided by the PADI Foundation (VS; Research Grant #21737), the University of Western Australia (VS, LT and MS; Research Collaboration Award), the ARC Centre of Excellence for Coral Reef Studies (VS and MM; CE140100020), the Western Australian Marine Science Institution (MM and VS), and an ARC Laureate Fellowship awarded to MM (LF120100049), and ARC Linkage grant (MS, LT, and NW; LP160101508).
Conflict of Interest
The authors declare that the research was conducted in the absence of any commercial or financial relationships that could be construed as a potential conflict of interest.
Acknowledgments
We thank J. Brown, G. Firman, M. Le Nohaïc, X. Chen, D. Thomson, S. Comeau, C. Cornwall, and Cygnet Bay Pearl Farm for assistance in the field. Special thanks to the Bardi Jawi people who enabled this research through their advice and consent to access their traditional lands.
Supplementary Material
The Supplementary Material for this article can be found online at: https://www.frontiersin.org/articles/10.3389/fmars.2020.00245/full#supplementary-material
References
Anderson, M. J., and Walsh, D. C. I. (2013). PERMANOVA, ANOSIM, and the mantel test in the face of heterogeneous dispersions: what null hypothesis are you testing? Ecol. Monogr. 83, 557–574. doi: 10.1890/12-2010.1
Baird, A. H., Bhagooli, R., Ralph, P. J., and Takahashi, S. (2008). Coral bleaching: the role of the host. Trends Ecol. Evol. 24, 16–20. doi: 10.1016/j.tree.2008.09.005
Barshis, D. J., Birkeland, C., Toonen, R. J., Gates, R. D., and Stillman, J. H. (2018). High-frequency temperature variability mirrors fixed differences in thermal limits of the massive coral Porites lobata. J. Exp. Biol. 221:jeb188581. doi: 10.1242/jeb.188581
Barshis, D. J., Stillman, J. H., Gates, R. D., Toonen, R. J., Smith, L. W., and Birkeland, C. (2010). Protein expression and genetic structure of the coral Porites lobata in an environmentally extreme Samoan back reef: does host genotype limit phenotypic plasticity? Mol. Ecol. 19, 1705–1720. doi: 10.1111/j.1365-294X.2010.04574.x
Bay, R. A., and Palumbi, S. R. (2014). Multilocus adaptation associated with heat resistance in reef-building corals. Curr. Biol. 24, 2952–2956. doi: 10.1016/j.cub.2014.10.044
Bellantuono, A. J., Hoegh-Guldberg, O., and Rodriguez-Lanetty, M. (2012). Resistance to thermal stress in corals without changes in symbiont composition. Proc. R. Soc. B Biol. Sci. 279, 1100–1107. doi: 10.1098/rspb.2011.1780
Berkelmans, R., and van Oppen, M. J. H. (2006). The role of zooxanthellae in the thermal tolerance of corals: a “nugget of hope” for coral reefs in an era of climate change. Proc. R. Soc. B Biol. Sci. 273, 2305–2312. doi: 10.1098/rspb.2006.3567
Bickford, D., Lohman, D. J., Sodhi, N. S., Ng, P. K. L., Meier, R., Winker, K., et al. (2007). Cryptic species as a window on diversity and conservation. Trends Ecol. Evol. 22, 148–155. doi: 10.1016/j.tree.2006.11.004
Boulay, J. N., Hellberg, M. E., Cortés, J., and Baums, I. B. (2014). Unrecognized coral species diversity masks differences in functional ecology. Proc. R. Soc. B Biol. Sci. 281:1580. doi: 10.1098/rspb.2013.1580
Brown, B., Downs, C., Dunne, R., and Gibb, S. (2002). Exploring the basis of thermotolerance in the reef coral Goniastrea aspera. Mar. Ecol. Prog. Ser. 242, 119–129. doi: 10.3354/meps242119
Brown, B. E., Dunne, R. P., Goodson, M. S., and Douglas, A. E. (2000). Bleaching patterns in reef corals. Nature 404, 142–143. doi: 10.1038/35004657
Camp, E. F., Schoepf, V., Mumby, P. J., Hardtke, L. A., Rodolfo-Metalpa, R., Smith, D. J., et al. (2018). The future of coral reefs subject to rapid climate change: lessons from natural extreme environments. Front. Mar. Sci. 5:4. doi: 10.3389/fmars.2018.00004
Castillo, K. D., Ries, J. B., Weiss, J. M., and Lima, F. P. (2012). Decline of forereef corals in response to recent warming linked to history of thermal exposure. Nat. Clim. Chang. 2, 756–760. doi: 10.1038/nclimate1577
Coles, S. L., Jokiel, P. L., and Lewis, C. R. (1976). Thermal tolerance in tropical versus subtropical Pacific reef corals. Pacific Sci. 30, 159–166.
Connolly, S. R., Lopez-Yglesias, M. A., and Anthony, K. R. N. (2012). Food availability promotes rapid recovery from thermal stress in a scleractinian coral. Coral Reefs 31, 951–960. doi: 10.1007/s00338-012-0925-929
Cornwall, C. E., Comeau, S., DeCarlo, T. M., Moore, B., D’Alexis, Q., and McCulloch, M. T. (2018). Resistance of corals and coralline algae to ocean acidification: physiological control of calcification under natural pH variability. Proc. Biol. Sci. 285: 20181168. doi: 10.1098/rspb.2018.1168
Dandan, S. S., Falter, J. L., Lowe, R. J., and McCulloch, M. T. (2015). Resilience of coral calcification to extreme temperature variations in the Kimberley region, northwest Australia. Coral Reefs 34, 1151–1163. doi: 10.1007/s00338-015-1335-1336
Dixon, G. B., Davies, S. W., Aglyamova, G. A., Meyer, E., Bay, L. K., and Matz, M. V. (2015). Genomic determinants of coral heat tolerance across latitudes. Science 348, 1460–1462. doi: 10.1126/science.1261224
Fisher, P. L., Malme, M. K., and Dove, S. (2012). The effect of temperature stress on coral–Symbiodinium associations containing distinct symbiont types. Coral Reefs 31, 473–485. doi: 10.1007/s00338-011-0853-850
Gilmour, J. P., Cook, K. L., Ryan, N. M., Puotinen, M. L., Green, R. H., Shedrawi, G., et al. (2019). The state of Western Australia’s coral reefs. Coral Reefs 38, 651–667. doi: 10.1007/s00338-019-01795-1798
Golbuu, Y., Victor, S., Penland, L., Idip, D., Emaurois, C., Okaji, K., et al. (2007). Palau’s coral reefs show differential habitat recovery following the 1998-bleaching event. Coral Reefs 26, 319–332. doi: 10.1007/s00338-007-0200-207
Gouezo, M., Golbuu, Y., Fabricius, K., Olsudong, D., Mereb, G., Nestor, V., et al. (2019). Drivers of recovery and reassembly of coral reef communities. Proc. R. Soc. B Biol. Sci. 286:20182908. doi: 10.1098/rspb.2018.2908
Graham, N. A. J., Nash, K. L., and Kool, J. T. (2011). Coral reef recovery dynamics in a changing world. Coral Reefs 30, 283–294. doi: 10.1007/s00338-010-0717-z
Grottoli, A. G., Rodrigues, L. J., and Palardy, J. E. (2006). Heterotrophic plasticity and resilience in bleached corals. Nature 440, 1186–1189. doi: 10.1038/nature04565
Grottoli, A. G., Warner, M. E., Levas, S. J., Aschaffenburg, M. D., Schoepf, V., McGinley, M., et al. (2014). The cumulative impact of annual coral bleaching can turn some coral species winners into losers. Glob. Chang. Biol. 20, 3823–3833. doi: 10.1111/gcb.12658
Gruber, R. K., Lowe, R. J., and Falter, J. (2017). Metabolism of a tide-dominated reef platform subject to extreme diel temperature and oxygen variations. Limnol. Ocean 62, 1701–1717.
Hammer, Ø, Harper, D. A. T., and Ryan, P. D. (2001). Paleontological statistics software package for education and data analysis. Palaeontol. Electron. 41:1.
Hoogenboom, M. O., Frank, G. E., Chase, T. J., Jurriaans, S., Álvarez-Noriega, M., Peterson, K., et al. (2017). Environmental drivers of variation in bleaching severity of Acropora species during an extreme thermal anomaly. Front. Mar. Sci. 4:376. doi: 10.3389/fmars.2017.00376
Howells, E. J., Berkelmans, R., Oppen, M. J. H., van Willis, B. L., and Bay, L. K. (2013). Historical thermal regimes define limits to coral acclimatization. Ecology 94, 1078–1088. doi: 10.1890/12-1257.1
Howells, J. E., Beltran, V. H., Larsen, N. W., Bay, L. K., Willis, B. L., and van Oppen, M. J. H. (2011). Coral thermal tolerance shaped by local adaptation of photosymbionts. Nat. Clim. Chang. 2, 116–120. doi: 10.1038/nclimate1330
Hughes, T. P., Anderson, K. D., Connolly, S. R., Heron, S. F., Kerry, J. T., Lough, J. M., et al. (2018). Spatial and temporal patterns of mass bleaching of corals in the Anthropocene. Science 359, 80–83. doi: 10.1126/science.aan8048
Hughes, T. P., Kerry, J. T., Álvarez-Noriega, M., Álvarez-Romero, J. G., Anderson, K. D., Baird, A. H., et al. (2017). Global warming and recurrent mass bleaching of corals. Nature 543, 373–377. doi: 10.1038/nature21707
Hughes, T. P., Kerry, J. T., Baird, A. H., Connolly, S. R., Chase, T. J., Dietzel, A., et al. (2019). Global warming impairs stock–recruitment dynamics of corals. Nature 568, 387–390. doi: 10.1038/s41586-019-1081-y
Jeffrey, S. W., and Humphrey, G. F. (1975). New spectrophotometric equations for determining chlorophylls a, b, c1 and c2 in higher plants, algae and natural phytoplankton. Biochem. Physiol. Pflanz. BPP 167, 191–194.
Kenkel, C. D., Almanza, A. T., and Matz, M. V. (2015a). Fine-scale environmental specialization of reef-building corals might be limiting reef recovery in the Florida Keys. Ecology 96, 3197–3212. doi: 10.1890/14-2297.1
Kenkel, C. D., Setta, S. P., and Matz, M. V. (2015b). Heritable differences in fitness-related traits among populations of the mustard hill coral, Porites astreoides. Heredity 115:509. doi: 10.1038/hdy.2015.52
Knowlton, N. (1993). Sibling species in the sea. Annu. Rev. Ecol. Syst. 24, 189–216. doi: 10.1146/annurev.es.24.110193.001201
Korneliussen, T. S., Albrechtsen, A., and Nielsen, R. (2014). ANGSD: analysis of next generation sequencing data. BMC Bioinform. 15:356. doi: 10.1186/s12859-014-0356-354
Ladner, J. T., and Palumbi, S. R. (2012). Extensive sympatry, cryptic diversity and introgression throughout the geographic distribution of two coral species complexes. Mol. Ecol. 21, 2224–2238. doi: 10.1111/j.1365-294X.2012.05528.x
LaJeunesse, T. C., Parkinson, J. E., Gabrielson, P. W., Jeong, H. J., Reimer, J. D., Voolstra, C. R., et al. (2018). Systematic revision of Symbiodiniaceae highlights the antiquity and diversity of coral endosymbionts. Curr. Biol. 28, 2570–2580. doi: 10.1016/j.cub.2018.07.008
Le Nohaïc, M., Ross, C. L., Cornwall, C. E., Comeau, S., Lowe, R., McCulloch, M. T., et al. (2017). Marine heatwave causes unprecedented regional mass bleaching of thermally resistant corals in northwestern Australia. Sci. Rep. 7, 1–11. doi: 10.1038/s41598-017-14794-y
Lefebure, T., Douady, C. J., Gouy, M., Trontelj, P., Briolay, J., and Gibert, J. (2006). Phylogeography of a subterranean amphipod reveals cryptic diversity and dynamic evolution in extreme environments. Mol. Ecol. 15, 1797–1806. doi: 10.1111/j.1365-294X.2006.02888.x
Li, H., Handsaker, B., Wysoker, A., Fennell, T., Ruan, J., Homer, N., et al. (2009). The sequence alignment/Map format and SAMtools. Bioinformatics 25, 2078–2079. doi: 10.1093/bioinformatics/btp352
Louis, Y. D., Kaullysing, D., Gopeechund, A., Mattan-Moorgawa, S., Bahorun, T., Dyall, S. D., et al. (2016). In hospite Symbiodinium photophysiology and antioxidant responses in Acropora muricata on a coast-reef scale: implications for variable bleaching patterns. Symbiosis 68, 61–72. doi: 10.1007/s13199-016-0380-4
McClanahan, T., Maina, J., Moothien-Pillay, R., and Baker, A. (2005). Effects of geography, taxa, water flow, and temperature variation on coral bleaching intensity in Mauritius. Mar. Ecol. Prog. Ser. 298, 131–142. doi: 10.3354/meps298131
McClanahan, T. R., Baird, A. H., Marshall, P. A., and Toscano, M. A. (2004). Comparing bleaching and mortality responses of hard corals between southern kenya and the great barrier Reef. Australia. Mar. Pollut. Bull. 48, 327–335. doi: 10.1016/j.marpolbul.2003.08.024
McClanahan, T. R., and Maina, J. (2003). Response of coral assemblages to the interaction between natural temperature variation and rare warm-water events. Ecosystems 6, 551–563. doi: 10.1007/s10021-002-0104-x
Moberg, F., and Folke, C. (1999). Ecological goods and services of coral reef ecosystems. Ecol. Econ. 29, 215–233. doi: 10.1016/S0921-8009(99)00009-9
Morikawa, M. K., and Palumbi, S. R. (2019). Using naturally occurring climate resilient corals to construct bleaching-resistant nurseries. Proc. Natl. Acad. Sci. U.S.A. 116, 10586–10591. doi: 10.1073/pnas.1721415116
Oksanen, J., Blanchet, F. G., Friendly, M., Kindt, R., Legendre, P., McGlinn, D., et al. (2016). Vegan: Community Ecology Package.
Oliver, T. A., and Palumbi, S. R. (2011). Do fluctuating temperature environments elevate coral thermal tolerance? Coral Reefs 30, 429–440. doi: 10.1007/s00338-011-0721-y
Palumbi, S. R., Barshis, D. J., Traylor-Knowles, N., and Bay, R. A. (2014). Mechanisms of reef coral resistance to future climate change. Science 344, 895–898. doi: 10.1126/science.1251336
Putnam, H. M., Stat, M., Pochon, X., and Gates, R. D. (2012). Endosymbiotic flexibility associates with environmental sensitivity in scleractinian corals. Proc. R. Soc. B Biol. Sci. 279, 4352–4361. doi: 10.1098/rspb.2012.1454
Richards, Z. T., Garcia, R., Moore, G., Fromont, J., Kirkendale, L., Bryce, M., et al. (2019). A tropical Australian refuge for photosymbiotic benthic fauna. Coral Reefs 38, 669–676. doi: 10.1007/s00338-019-01809-1805
Richards, Z. T., Garcia, R. A., Wallace, C. C., Rosser, N. L., and Muir, P. R. (2015). A diverse assemblage of reef corals thriving in a dynamic intertidal reef setting (Bonaparte Archipelago, Kimberley, Australia). Public Libr. Sci. One 10:e0117791. doi: 10.1371/journal.pone.0117791
Riegl, B. M., Purkis, S. J., Al-Cibahy, A. S., Abdel-Moati, M. A., and Hoegh-Guldberg, O. (2011). Present limits to heat-adaptability in corals and population-level responses to climate extremes. Public Libr. Sci. One 6:e24802. doi: 10.1371/journal.pone.0024802
Rose, N. H., Bay, R. A., Morikawa, M. K., and Palumbi, S. R. (2017). Polygenic evolution drives species divergence and climate adaptation in corals. Evolution 72, 82–94. doi: 10.1111/evo.13385
Safaie, A., Silbiger, N. J., McClanahan, T. R., Pawlak, G., Barshis, D. J., Hench, J. L., et al. (2018). High frequency temperature variability reduces the risk of coral bleaching. Nat. Commun. 9:1671. doi: 10.1038/s41467-018-04074-4072
Schoepf, V., Carrion, S. A., Pfeifer, S. M., Naugle, M., Dugal, L., Bruyn, J., et al. (2019). Stress-resistant corals may not acclimatize to ocean warming but maintain heat tolerance under cooler temperatures. Nat. Commun. 10:4031. doi: 10.1038/s41467-019-12065-12060
Schoepf, V., Grottoli, A. G., Levas, S. J., Aschaffenburg, M. D., Baumann, J. H., Matsui, Y., et al. (2015a). Annual coral bleaching and the long-term recovery capacity of coral. Proc. R. Soc. B 282:20151887. doi: 10.1098/rspb.2015.1887
Schoepf, V., Stat, M., Falter, J. L., and McCulloch, M. T. (2015b). Limits to the thermal tolerance of corals adapted to a highly fluctuating, naturally extreme temperature environment. Sci. Rep. 5:17639. doi: 10.1038/srep17639
Siebeck, U. E., Marshall, N. J., Klüter, A., and Hoegh-Guldberg, O. (2006). Monitoring coral bleaching using a colour reference card. Coral Reefs 25, 453–460. doi: 10.1007/s00338-006-0123-128
Silverstein, R. N., Cunning, R., and Baker, A. C. (2015). Change in algal symbiont communities after bleaching, not prior heat exposure, increases heat tolerance of reef corals. Glob Chang. Biol. 21, 236–249. doi: 10.1111/gcb.12706
Skotte, L., Korneliussen, T. S., and Albrechtsen, A. (2013). Estimating individual admixture proportions from next generation sequencing data. Genetics 195, 693–702. doi: 10.1534/genetics.113.154138
Souter, P. (2010). Hidden genetic diversity in a key model species of coral. Mar. Biol. 157, 875–885. doi: 10.1007/s00227-009-1370-1373
Stat, M., and Gates, R. D. (2011). Clade D Symbiodinium in scleractinian corals: a “nugget” of hope, a selfish opportunist, an ominous sign, or all of the above? J. Mar. Biol. 2011:9. doi: 10.1155/2011/730715
Szmant, A. M., and Gassman, N. J. (1990). The effects of prolonged “bleaching” on the tissue biomass and reproduction of the reef coral Montastrea annularis. Coral Reefs 8, 217–224.
Therkildsen, N. O., and Palumbi, S. R. (2017). Practical low-coverage genomewide sequencing of hundreds of individually barcoded samples for population and evolutionary genomics in nonmodel species. Mol. Ecol. Resour. 17, 194–208. doi: 10.1111/1755-0998.12593
Thomas, L., Kendrick, G. A., Kennington, W. J., Richards, Z. T., and Stat, M. (2014). Exploring Symbiodinium diversity and host specificity in Acropora corals from geographical extremes of Western Australia with 454 amplicon pyrosequencing. Mol. Ecol. 23, 3113–3126. doi: 10.1111/mec.12801
Trygonis, V., and Sini, M. (2012). photoQuad: a dedicated seabed image processing software, and a comparative error analysis of four photoquadrat methods. J. Exp. Mar. Bio. Ecol. 42, 99–108. doi: 10.1016/j.jembe.2012.04.018
Underwood, J., Richards, Z. T., Berry, O., and Gilmour, J. P. (2017). Population Connectivity And Genetic Diversity In Brooding And Broadcast Spawning Corals In The Kimberley. WAMSI Kimberley Marine Research Program, Executive Summary, Subproject 1.1.3.1. Available at: https://www.wamsi.org.au/sites/wamsi.org.au/files/files/Connectivity_Coral%20Report%20WAMSI%20KMRP%20Project%201_1_3_1_Underwood%20et%20al%202017_EXECUTIVE%20SUMMARY(1).pdf (accessed 10 January, 2020).
van Woesik, R., Houk, P., Isechal, A. L., Idechong, J. W., Victor, S., and Golbuu, Y. (2012). Climate-change refugia in the sheltered bays of Palau: analogs of future reefs. Ecol. Evol. 2, 2474–2484. doi: 10.1002/ece3.363
Vrijenhoek, R. C., Schutz, S. J., Gustafson, R. G., and Lutz, R. A. (1994). Cryptic species of deep-sea clams (Mollusca: Bivalvia: Vesicomyidae) from hydrothermal vent and cold-water seep environments. Deep Sea Res. Part I Oceanogr. Res. Pap. 41, 1171–1189. doi: 10.1016/0967-0637(94)90039-90036
Keywords: coral bleaching, recovery capacity, temperature variability, Kimberley region, Acropora aspera, cryptic species
Citation: Schoepf V, Jung MU, McCulloch MT, White NE, Stat M and Thomas L (2020) Thermally Variable, Macrotidal Reef Habitats Promote Rapid Recovery From Mass Coral Bleaching. Front. Mar. Sci. 7:245. doi: 10.3389/fmars.2020.00245
Received: 18 January 2020; Accepted: 27 March 2020;
Published: 19 May 2020.
Edited by:
Peng Jin, University of Guangzhou, ChinaReviewed by:
Kefu Yu, Guangxi University, ChinaBenjamin Charles Clayton Hume, King Abdullah University of Science and Technology, Saudi Arabia
Copyright © 2020 Schoepf, Jung, McCulloch, White, Stat and Thomas. This is an open-access article distributed under the terms of the Creative Commons Attribution License (CC BY). The use, distribution or reproduction in other forums is permitted, provided the original author(s) and the copyright owner(s) are credited and that the original publication in this journal is cited, in accordance with accepted academic practice. No use, distribution or reproduction is permitted which does not comply with these terms.
*Correspondence: Verena Schoepf, di5zY2hvZXBmQHV2YS5ubA==
†These authors have contributed equally to this work