- 1Department of Arctic and Marine Biology, Faculty of Biosciences, Fisheries, and Economics, UiT The Arctic University of Norway, Tromsø, Norway
- 2Shirshov Institute of Oceanology, Russian Academy of Sciences, Moscow, Russia
- 3Alfred-Wegener- Institute, Helmholtz Center for Polar and Marine Research, Bremerhaven, Germany
- 4MARUM, University of Bremen, Bremen, Germany
Half of the Arctic Ocean is deep sea (>1000 m), and this area is currently transitioning from being permanently ice-covered to being seasonally ice-free. Despite these drastic changes, it remains unclear how organisms are distributed in the deep Arctic basins, and particularly what feeds them. Here, we summarize data on auto- and heterotrophic organisms in the benthic, pelagic, and sympagic realm of the Arctic Ocean basins from the past three decades and put together an organic carbon budget for this region. Based on the budget, we investigate whether our current understanding of primary and secondary production and vertical carbon flux are balanced by the current estimates of the carbon demand by deep-sea benthos. At first glance, our budget identifies a mismatch between the carbon supply by primary production (3–46 g C m−2 yr−1), the carbon demand of organisms living in the pelagic (7–17 g C m−2) and the benthic realm (< 5 g C m−2 yr−1) versus the low vertical carbon export (at 200 m: 0.1–1.5 g C m−2 yr−1, at 3000–4000 m: 0.01–0.73 g C m−2 yr−1). To close the budget, we suggest that episodic events of large, fast sinking ice algae aggregates, export of dead zooplankton, as well as large food falls need to be quantified and included. This work emphasizes the clear need for a better understanding of the quantity, phenology, and the regionality of carbon supply and demand in the deep Arctic basins, which will allow us to evaluate how the ecosystem may change in the future.
Introduction: the Arctic Ocean Basins From a Seafloor Perspective
More than half of the world’s surface is covered by oceans deeper than 2000 m (Smith et al., 2009), which means that most of the sea floor experiences deep-sea conditions. In this permanently cold, dark, soft-bottom dominated, high-pressure environment, food supply is sparse and mostly limited to the vertical flux of particles from the sunlit surface waters. In the deep basins of the central Arctic Ocean (AO), with a mean depth of 2748 m (Jakobsson et al., 2004), the food limitation for benthic organisms is even more extreme than on the global average. During one to six months each year, dependent on latitude, the polar night precludes primary production in the AO’s surface, and even once the sun returns, sea ice, often covered by snow, tends to prevent irradiance to penetrate deep into the water column (Nicolaus and Katlein, 2013). In addition, nutrient concentrations in the euphotic zone are often low, largely because of the strong pycnocline in the Arctic basins (Gosselin et al., 1997; Gradinger, 2009; Bluhm et al., 2015). Dependent on the match or mismatch between the primary production and sympagic and pelagic grazers (Søreide et al., 2013), either high quality algal biomass or pelagic “leftovers,” such as fecal pellets or heavily reworked marine snow, will sink to the sea floor as food supply for the benthos (Iken et al., 2005; Wassmann and Reigstad, 2011; Boetius et al., 2013; Lalande et al., 2019).
As the sea ice cover in the AO declines, discussions about the potential exploitation of fish stocks and mineral resources in that area increase (Coakley et al., 2016; Shephard et al., 2016). A major challenge in these debates are, however, the relatively poor records on the diversity and quantity of life present in the AO basins and at its seafloor. One particularly large knowledge gap is the question what feeds the benthos in the AO basins. We approach this question here by investigating the quantity of carbon that was necessary to sustain benthic organisms in the basins during the past decades. By putting together a carbon budget (detailed information on how data were compiled in the Supplementary Material), which compiles the current understanding of surface carbon production, carbon requirements of ice-associated and pelagic grazers, as well as the carbon export, we evaluate whether the budget is balanced with the estimated carbon demand of deep-sea benthos.
A Carbon Budget for the Arctic Ocean Basins
Deep-Sea Benthos and Its Carbon Demand
The benthic carbon demand is often determined via benthic oxygen consumption, which is tightly linked to the carbon supply, the standing stock of benthic life, and benthic metabolic activity levels. In the following section, we first synthesize current knowledge about the benthic biomass in the AO basins and then give an estimate of the benthic carbon demand. In our assessment, we include microbes (specifically bacteria), meiofauna, macrofauna, as well as megafauna, and present their biomass in grams carbon (g C) per m2 from the AO basins ≥1000 m (further details in Supplementary Material). Compiled estimates for carbon demand (g C m−2 yr−1) from the literature were mostly assessed in sediment community incubations of sediment cores ex situ, although this often underrepresents megafauna (Piepenburg et al., 1995).
With the exception of bacteria, biomass of benthos inhabiting the deep AO basins tends to be lower than benthic biomass on adjacent shelves and margins. As the water depth increases over the slopes, the benthic biomass decreases by a factor of ∼5–20 (Figure 1) due to decreasing abundance of organisms (Levin et al., 2001; Bluhm et al., 2011; Degen et al., 2018; Vedenin et al., 2018), as well as by declining body sizes (Wei et al., 2010). Even though the position of the marginal ice zone determines the primary production, which in turn influences the benthic biomass distributions (Degen et al., 2018; Rybakova et al., 2019), benthic organisms of all size fractions populate the AO basins (MacDonald et al., 2010; Bluhm et al., 2011; Degen et al., 2018; Vedenin et al., 2018; Rybakova et al., 2019). This clearly reflects that there must be sufficient carbon input to sustain their present densities.
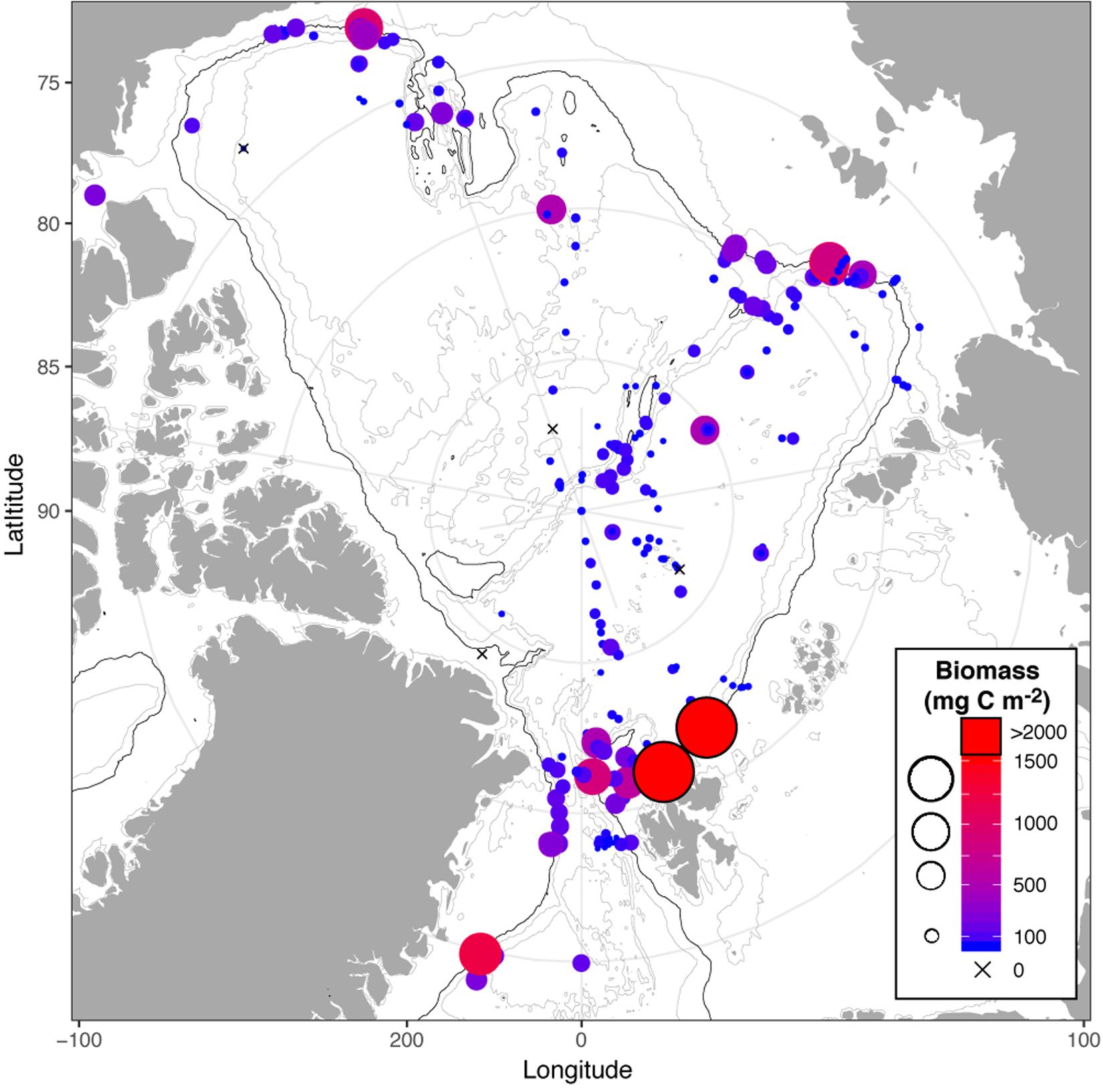
Figure 1. Biomass of benthic macrofauna in the Arctic Ocean basins and adjacent areas (data compiled from Bluhm et al., 2015; Degen et al., 2018; Vedenin et al., 2018). Note biomass values for depths ≥500 m are included in the figure to show the decline from the upper slope to the central basins. The 1000 m isobaths is depicted as a black line.
As in other deep-sea areas, bacterial communities tend to dominate the benthic biomass in high latitude systems (Deming and Yager, 1992), with Gammaproteobacteria and Flavobacteria being most abundant (Bienhold et al., 2016; Rapp et al., 2018). Standing stocks of benthic bacteria in the top 10 cm of sediments in the Arctic deep-sea are within the range of 0.5–2 g C m−2 (Boetius and Damm, 1998). These bacteria respond to input of organic matter (e.g., algal aggregates or chitin) within days to weeks, by rapidly increasing their activity and oxygen demand as well as by changing the bacterial community composition depending on the organic matter source (Hoffmann et al., 2018). Benthic bacteria may, thereby, consume more phytoplankton-based detrital carbon than benthic macrofauna in some deep-sea areas (Glud, 2008; Sweetman et al., 2019). In turn, bacteria are a relevant food source to the benthos and their standing stock is relatively invariant because it is controlled by grazing. Even though still largely overlooked, marine fungi are additional contributors to the benthic microbial food web (Morales et al., 2019), and there is increasing evidence that fungi also play a role in carbon cycling in Arctic food webs (Hassett et al., 2019). While little is known about fungal biomass in the Arctic deep-sea, sediments from 5000 m in the Indian Ocean had fungal mycelia of an estimated biomass of 7–54 μg C g−1 sediment dry weight (Damare and Raghukumar, 2008).
As in all soft-bottom systems, benthic meiofauna in the AO basins mainly consist of unicellular foraminifera (Wollenburg and Mackensen, 1998) and multi-cellular nematodes (Soltwedel, 2000; Vanreusel et al., 2000; Schewe, 2001; Hoste et al., 2007). Observations suggest that meiofaunal densities range mostly from <100,000–4,000,000 individuals (ind.) m−2 (≥1000 m) with a mean density around 500,000 ind. m−2 below 3000 m (Bluhm et al., 2011 and literature cited therein; Soltwedel, 2000; Vanreusel et al., 2000; Schewe, 2001; Soltwedel et al., 2009a, b; Hoffmann et al., 2018). The few meiofauna biomass estimates available report <0.5–22 g C m−2 (nematodes only, Vanreusel et al., 2000) and ∼0.2–0.4 g C m−2 (Pfannkuche and Thiel, 1987). Macrofaunal communities in the AO basins are dominated, as soft-bottom sediments worldwide, by polychaetes, crustaceans, and bivalves, although the proportions of these taxa vary regionally with regard to species numbers and biomass (Clough et al., 1997; Wlodarska-Kowalczuk et al., 2004; Bluhm et al., 2005, 2011; Degen et al., 2018; Vedenin et al., 2019). Our compiled data set suggests that macrofauna abundance ranges from <10 to ∼3000 ind. m−2 (>1000 m: mean ∼ 640 ind. m−2; >3000 m: mean ∼220 ind. m−2). Macrofaunal biomass ranges from 0 to 4.4 g C m−2 with an average of 0.5 g C m−2 below 1000 m (Figure 1). The epifaunal megabenthos is often dominated by brittle stars, sea cucumbers, and zoarcid, liparid, and rajid fishes in soft sediments of the AO basins (Bergmann et al., 2011; Taylor et al., 2016; Rybakova et al., 2019; Zhulay et al., 2019). Abundances are mostly ≤1–5 ind. m−2 (Rybakova et al., 2019; Zhulay et al., 2019), but epifaunal densities may be enhanced at hot spots on glacial drop stones (mainly by cnidarians and sponges, Meyer et al., 2016; Zhulay et al., 2019). Given that Arctic deep-sea epifauna is primarily assessed photographically, their biomass estimates are even sparser than abundance estimates, but new estimates yield <0.001–0.2 g C m2 (≥1000 m; Rybakova et al., 2019; Zhulay et al., 2019). Hyperbenthic taxa such as benthic jellyfish, ctenophores, highly mobile polychaetes, and mysids have been documented in the AO basins (MacDonald et al., 2010; Zhulay et al., 2019), but estimates of their abundance, biomass, or carbon demand are lacking. Fish densities are also low and sparse, with estimates of <1 per 60 min ROV transect (Stein et al., 2005), or 260 fishes km−2 in the 500–1000 m range (Majewski et al., 2017).
Food limitation in the AO basins is not only reflected in low abundance and biomass levels but also in the food web structure. The degraded nature of most organic matter at the deep-sea floor and the generally low current velocities result in high proportions of deposit-feeding organisms, even though suspension-feeders, predators, and other feeding types are also represented (Iken et al., 2001, 2005; Bergmann et al., 2009). That refractory nature of the food is also reflected in enriched stable nitrogen isotope values of deposit feeders and predators in particular (Iken et al., 2005; Bell et al., 2016). Trophic markers and observational evidence, however, also suggest that some taxa graze on freshly produced food such as ice algal matter that must have rapidly fallen down to the deep sea floor, and observations of surface-deposit feeding sea cucumbers support this notion (MacDonald et al., 2010; Boetius et al., 2013).
To sustain the above presented biomass levels, the benthos in the AO basins requires matching carbon inputs. In the compiled studies, benthic carbon demand in depths 1000–5590 m ranged from 0.2–23 g C m−2 yr−1 (Table 1). The median of lowest and highest values across studies was 1 g C m−2 yr−1 and 10 g C m−2 yr−1, respectively, and highest values were generally found near Atlantic and Pacific inflow areas. In addition, benthic carbon demand tends to decrease with water depth, and increase with increasing surface production (reviewed in Bourgeois et al., 2017; Hoffmann et al., 2018). We presume that food supply variation must be assumed to be the major driver of the variability in the carbon demand, even though methodological differences cannot be excluded either. Additionally, it is a challenge that the available carbon demand estimates are primarily based on measurements from the summer months. Applying those to the entire year, as we did here, may be overestimating the actual values. In summary, we suggest, however, that a range of 1–10 g C m−2 yr−1 is representative of the benthic AO carbon demand, with values away from the slope mostly estimated below 5 g C m−2 yr−1.
Primary Production
Autotrophic primary producers in the AO basins are found in and attached under the sea ice (ice algae) as well as in the water column (phytoplankton). Diatoms have traditionally been considered to be important primary producers in both realms, and two ice algae species in particular, Melosira arctica and Nitzschia frigida are found in high abundances (Booth and Horner, 1997; Melnikov et al., 2002; Olsen et al., 2017; Hop et al., this issue). Recently, however, the contribution of pico- or nano-autotrophs to the algal biomass and the production in the AO is increasingly acknowledged (Vader et al., 2014; Metfies et al., 2016; Wang et al., 2019). As in other oceans, autotrophic primary production in the AO basins is largely driven by the availability of light and nutrients. In terms of light, the period suitable for pronounced primary production becomes shorter with increasing latitude, because the light climate is not only modulated by the presence of the sun, but also the ice cover (Zenkevitch, 1963; Leu et al., 2011). The first accumulation of biomass in the AO basins occurs most often in form of a sea ice bloom, while the pelagic bloom tends to follow some weeks later when ice and snow melt around mid-July (Ardyna et al., 2013; Leu et al., 2015). In addition, the primary production in the AO is regulated by the nutrient availability. Surface concentrations of nitrate, the commonly limiting nutrient, tend to be low, but also a pronounced variability in surface concentrations can be found in the AO (Figure 2). As nutrient concentrations strongly depend on advection and vertical mixing processes (Codispoti et al., 2013), higher surface concentrations can be found in the regions affected by advection of Pacific or Atlantic derived waters. In contrast, in most of the Beaufort Gyre, where a strong halocline prevents the upward nutrient flux (Randelhoff et al., 2017), algal productivity is more limited (Gradinger, 2009; Slagstad et al., 2015).
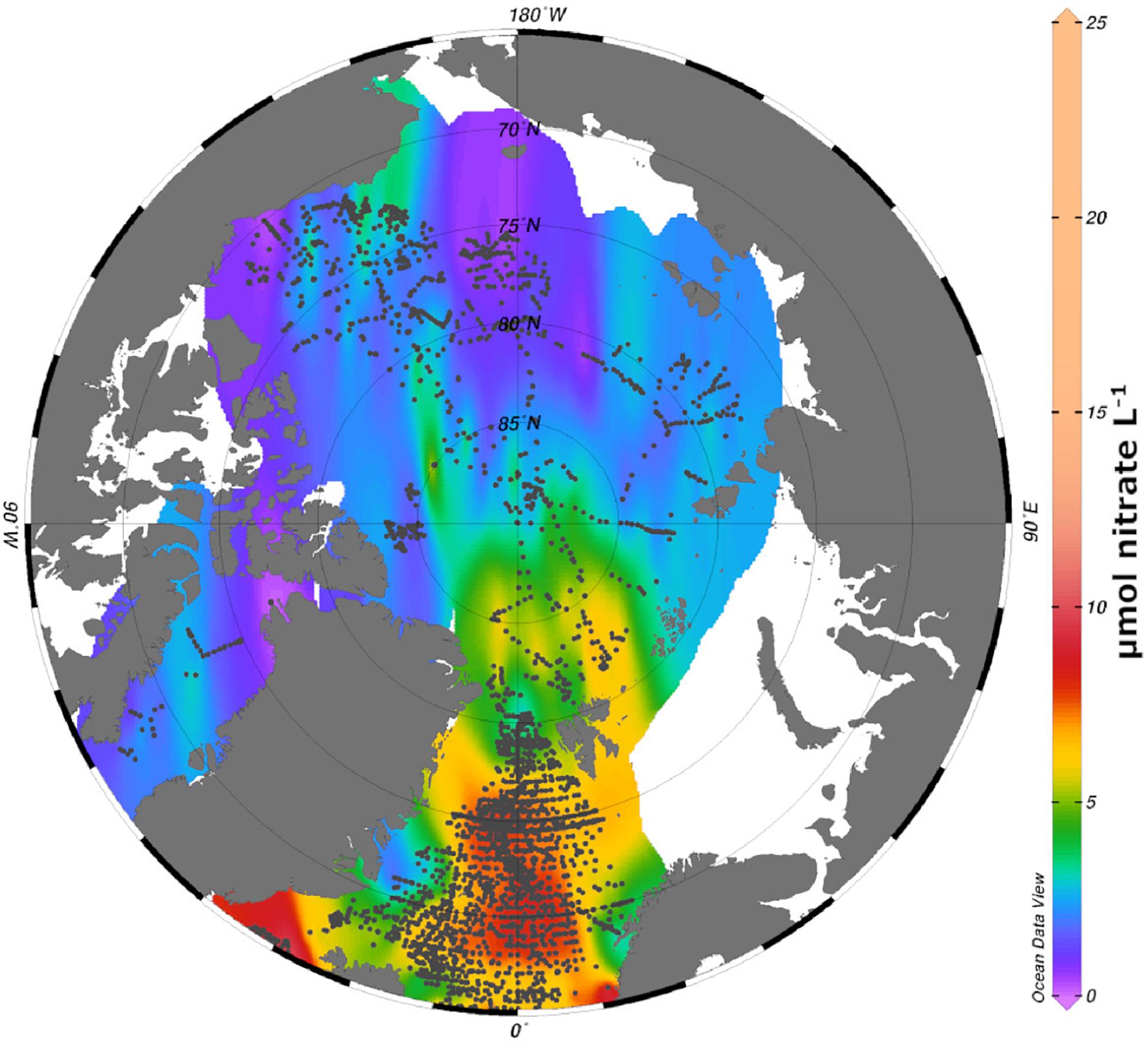
Figure 2. Compilation of nitrate concentrations (μmol nitrate L1, gray filled circles) in the Arctic basins and adjacent oceans (at stations with a bottom depth >1000 m). Data extracted from the World Ocean Database 2018. Extrapolated nitrate concentrations includes also shallower areas.
Primary production can be estimated in various ways. Tracer incubation and oxygen consumption studies of melted sea ice or water samples are still the only methods to estimate direct carbon production (Kirchman et al., 2009; Campbell et al., 2017; Kromkamp et al., 2017). These snapshot measurements are very small-scale, but they are necessary to calibrate and validate model outputs (Popova et al., 2010; Slagstad et al., 2015) and satellite estimates (Pabi et al., 2008; Ardyna et al., 2013). Satellite remote sensing has been used to estimate primary production on a large scale by using ocean color data coupled to an ecosystem model (Arrigo and van Dijken, 2011; Babin et al., 2015), although satellite coverage, clouds, and sea ice restrict primary production estimates to the ice-free regions of the AO basins. Alternatively, inorganic nutrient budgets (most often based on nitrogen) or nutrient uptake rates can also be used to assess the annual algal production (Luchetta et al., 2000), because new primary production cannot exceed a certain maximum amount defined by the available nutrients (Bluhm and Gradinger, 2008; Mills et al., 2018).
Both ice-associated and pelagic production takes place in the AO basins. The overall sea ice cover, ice thickness, and snow thickness determine the amount of light available for both types of primary production, and thus the relative contribution of ice-associated and pelagic algae to the total primary production. In the AO basins, primary production by sea ice algae has only been found to be higher than pelagic production (integrated over the euphotic zone) in very dense ice cover (>90%) (Fernández-Méndez et al., 2015: ice algal primary production (PP): 0.5–1.5 mg C m−2 d−1, pelagic PP: 0.1–0.5 mg C m−2 d−1; Gosselin et al., 1997: ice algal PP: 57 mg C m−2 d−1, pelagic PP: 30 mg C m−2 d−1). Otherwise, pelagic production (integrated down to euphotic depth of 22–85 m) tends to exceed ice algal production (Fernández-Méndez et al., 2015: ice algal PP: 0.1–13 mg C m−2 d−1, pelagic PP: 0.1–60 mg C m−2 d−1; Gosselin et al., 1997: ice algal PP: 2–69 mg C m−2 d−1, pelagic PP: 52–272 mg C m−2 d−1). So far little is understood about the role of under-ice blooms (Gradinger, 1996; Arrigo et al., 2012; Assmy et al., 2017). As the sea ice thins, conditions allowing under-ice blooms may, however, become more frequent in the central AO (Horvat et al., 2017), and recent field measurements with a remotely operated vehicles suggested that these blooms may contribute up to 939 m g C m−2 d−1 to the overall primary production (Massicotte et al., 2019).
Overall, approximating the annual primary production from point measurements in the field or from budgets of spatially variable nutrient concentrations is challenging because estimates from different regions and seasons need to be pooled and integrated over the whole growth season. Nevertheless, the complied estimates of the primary production in the AO basins reveal a very similar range of 3–46 g C m2 yr−1 (mean 13 g C m−2 yr−1), irrespective of the method used (Table 2). Based on the daily production rates presented above, we presume that pelagic production substantially contributes to this total production. Compared to primary production levels in the productive Arctic inflow shelf regions (annual primary production >70 g C m−2 yr−1), the production in the AO basins is, however, at least half to one order of magnitude lower (Codispoti et al., 2013; Varela et al., 2013).
Abundance and Carbon Demand of Under-Ice Fauna and Zooplankton
A wide range of nano- to macro-sized ice-associated and pelagic consumers are found in the AO basins, but micro- (20–200 μm, Sieburth et al., 1978) and mesozooplankton (200–2000 μm, Sieburth et al., 1978) play a central role (Thibault et al., 1999). As grazing preferences of these organisms determine the proportion and quality of carbon that will eventually reach the seabed in the AO basins, we present here an overview of the important consumers.
Seasonal and multiyear sea ice in the Arctic provides not only a platform for ice-associated primary production, but also to various ice-associated consumers. Some small taxa reside in the ice matrix (e.g., Nematoda, Acoela, Harpacticoida, Rotifera, Bluhm et al., 2018), while others, such as amphipods, use the bottom of the sea ice as a hard substrate and are important consumers of ice algae (abundances: few individuals to >500 ind. m−2) (Hop et al., 2000; Werner, 2000; Gradinger and Bluhm, 2004; David et al., 2017). In addition, analyses of lipids and isotopes showed that pelagic grazers, such as Calanus glacialis and C. hyperboreus also graze to a considerable extent on sea ice algae (Kohlbach et al., 2016).
Microzooplankton in the deep Arctic basins consist of unicellular and multicellular eukaryotic taxa, including heterotrophic flagellates, ciliates, dinoflagellates, rotifers, and small copepods (<1 mm). Their biomass is on average 0.48 g C m−2 (0–50 m) (Sherr et al., 1997; Olli et al., 2007; Kilias et al., 2014). Ciliates and dinoflagellates can consume a substantial fraction of primary production in the upper water column, but their grazing impact varies greatly (0–120% of daily phytoplankton growth, Sherr et al., 2009). They can also be mainly bacterivorous or omnivorous (Sherr et al., 2009).
The diversity and abundance of mesozooplankton including their seasonal and regional variation has been extensively studied in the AO during recent years (Mumm et al., 1998; Kosobokova and Hirche, 2000, 2009; Auel and Hagen, 2002; Ashjian et al., 2003; Hopcroft et al., 2005; Olli et al., 2007; Lane et al., 2008; Kosobokova and Hopcroft, 2010; Kosobokova et al., 2011; Kosobokova, 2012; Smoot and Hopcroft, 2017). The deep AO sustains during summer a mean mesozooplankton biomass of ∼6 g dry weight (DW) m−2 (range: 1–24 g DW m−2), which corresponds to 2.4 g C m−2 (Kiørboe, 2013), and a mean abundance of 150,000 ind. m−2 (range: <10,000–300,000 ind. m−2) (Kosobokova and Hirche, 2009; Kosobokova et al., 2011). Winter data are largely lacking, and therefore not included here. The predominant mesozooplankton group in terms of biomass and abundance are copepods (Kosobokova, 2012) with the key species in terms of biomass being C. hyperboreus, C. glacialis, C. finmarchicus, and Metridia longa. While C. hyperboreus dominates the biomass, with up to 97% in the basins, C. glacialis, M. longa, as well as the advected expatriate C. finmarchicus are important contributors to the biomass closer to the slopes (Figure 3) (Kosobokova and Hirche, 2009; Kosobokova, 2012).
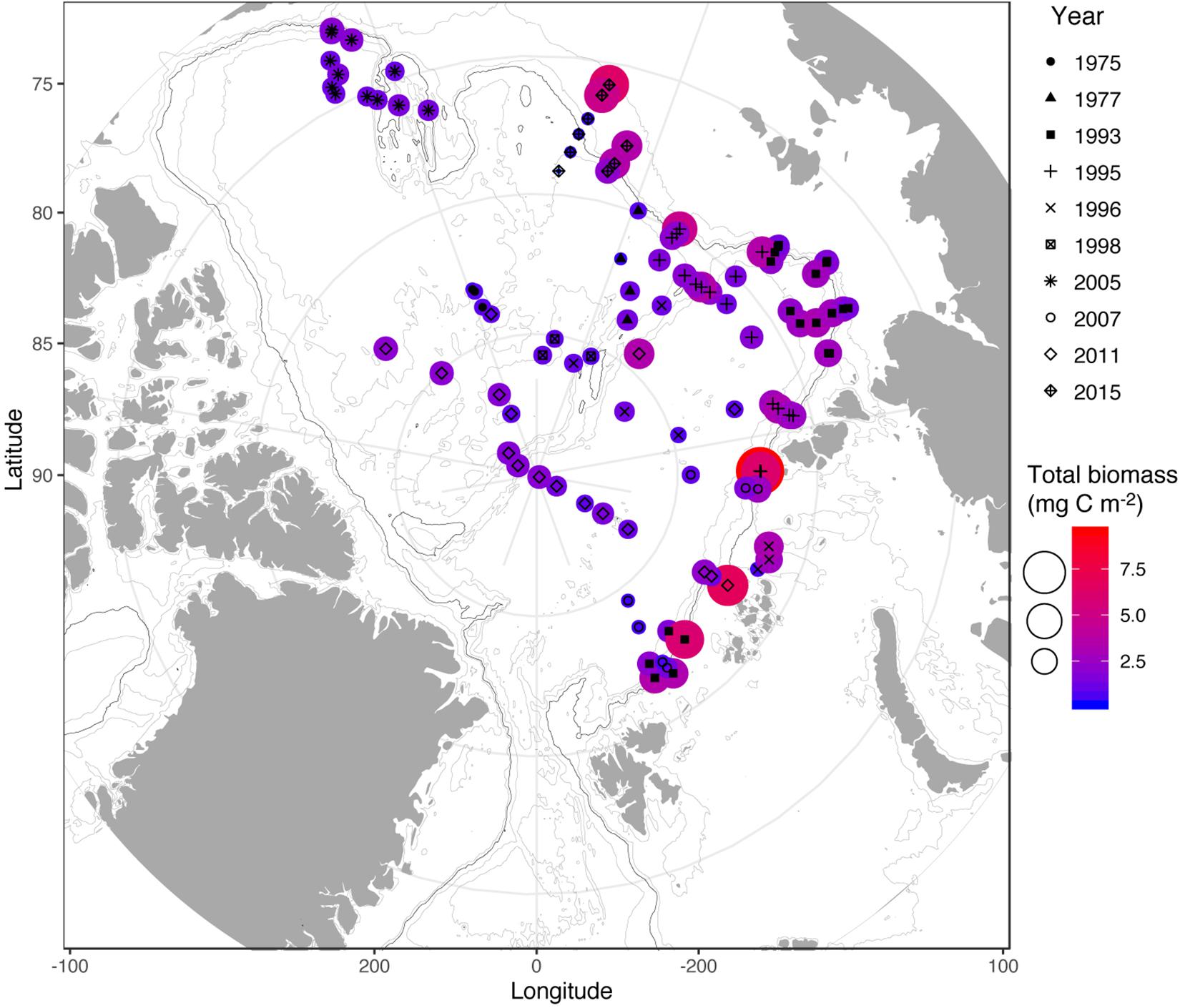
Figure 3. Compilation of depth-integrated zooplankton biomass in the Arctic Ocean basins. Data were collected in the summer-autumn periods of 1975–2015 (data compiled from Kosobokova and Hirche, 2009; Bluhm et al., 2015; Ershova and Kosobokova, 2019) and converted from biomass (g dry weight m−2) to carbon (g C m−2) using the conversion of g C = 0.4 * g dry weight (Kiørboe, 2013). The 1000 m isobaths are depicted as a black line.
Only few zooplankton species permanently inhabit surface waters where primary production takes place (e.g., Oithona similis). Others solely reside in the mesopelagic zone (Raskoff et al., 2010; Kosobokova et al., 2011), and a third group utilizes the surface and deep parts of the water column by performing daily and/or seasonal vertical migrations. The latter behavior represents a mechanism of active carbon transport between the surface and the deep ocean, with amplitude and time of ascent and descent being species- and stage-specific (Geynrikh et al., 1983; Conover, 1988; Ashjian et al., 2003; Kosobokova and Pertsova, 2005; Kosobokova, 2012; Darnis and Fortier, 2014; Kvile et al., 2019). The well known Calanus genus is for example often considered “epipelagic”, but it performs seasonal migration and resides dormant at depth for 8–9 months each year (Kosobokova, 1999; Ashjian et al., 2003; Hirche, 2013; Darnis and Fortier, 2014). In areas with large overwintering populations, C. hyperboreus transfers annually 3.5–6 g C m−2 to the deep ocean, a value comparable with the flux of detrital carbon (the “lipid pump,” Visser et al., 2017). These overwintering C. hyperboreus aggregations right above the seafloor (≤2000 m) (Auel et al., 2003; Hirche et al., 2006) may serve as a direct food source for mobile benthic animals, such as amphipods or demersal fish.
Interlinked with the zooplankton life cycles and their depth distribution is also their dietary preference. Traditionally, the Calanus species in the Arctic have been considered to be “herbivores”; however, likely only C. hyperboreus is a true herbivore (Forest et al., 2011; Campbell et al., 2016). The species C. finmarchicus and C. glacialis consume a wide spectrum of prey, and are more accurately categorized as omnivores (Hansen et al., 1993; Ohman and Runge, 1994; Hirche and Kwasniewski, 1997; Iken et al., 2005; Campbell et al., 2009). Other pelagic copepod key species (e.g., Metridia longa) are active year-round and adjust their feeding modes depending on food availability, switching to a non-algal diet during parts of the year (Haq, 1967).
The complex feeding modes and life history strategies of ice-associated grazers and zooplankton in the AO basins and the rare field observations make it challenging to estimate their annual carbon demand, and give an estimate on how much carbon remains to be potentially exported to the benthos. In Table 3, we compiled carbon demand and ingestion estimates for different ice-associated and pelagic consumers. However, the total carbon demand cannot be approximated by adding up these individual values because various consumers also prey on each other. As an alternative we estimate here the sympagic and pelagic carbon demand by quantifying the grazing on new primary production. This assumption is of course limited, because (1) we cannot in all instances be certain to which extent new production is grazed, and (2) grazing does not result in a true loss of carbon from the system. Only respired carbon is truly “lost” from the system, while the remaining fraction is used in secondary production feeding higher trophic levels. For reasons of simplification, we assume a highly efficient food web in the deep AO basins, which respires consumed carbon entirely. In addition, we presume that the fraction of new production that is not grazed in the pelagic system sinks to the benthos.
Microzooplankton grazing impact in the AO has been estimated to range from 22% (Sherr et al., 2009) to 70–76% (Verity et al., 2002; Yang et al., 2015) of the primary production during spring and summer, while mesozooplankton preys 26–40% (average of stations >1000 m, Campbell et al., 2009). By assuming algal consumption by micro- and mesozooplankton of 6–13 g C m−2 yr−1 (48–100% of mean primary production of 13 g C m−2 yr−1, range: 3–46 g C m−2 yr−1, Table 2) and a meiofauna and bacteria carbon demand of 1.3–3.6 g C m−2 yr−1 (Table 3), the total ice-associated and pelagic carbon demand would be 7–17 g C m−2 yr−1. The bacterial carbon demand has been estimated to be 15–135 g C m−2 yr−1 in the AO basins (Sherr and Sherr, 2003), which corresponds well to findings in a fjord on Svalbard (40 g C m−2 yr−1, Iversen and Seuthe, 2011). However, as bacteria covered their carbon demand through DOC, and are not directly fueled by new production, this is not taken into account here. Our calculation does not include potentially lower carbon demands during winter or alternative carbon transport mechanisms such as mortality or vertical migration (the “lipid pump,” Visser et al., 2017), because of the paucity of data. The range of the estimated ice-associated and pelagic carbon demand of 7–17 g C m−2 yr−1 illustrates a clear need for a better regional and inter-annual resolution, as well as a better understanding of the life cycles, food preferences, and carbon utilization of these grazers. Nevertheless, combining our estimate of the primary production (mean 13 g C m−2 yr−1) and the pelagic carbon demand suggests (7–17 g C m−2 yr−1) would allow an annual carbon export of up to 6 g C m−2 yr−1 to the benthos. This actually matches our estimate of the benthic carbon demand (section “Deep-Sea Benthos and Its Carbon Demand”).
Vertical Carbon Flux
Sparse field data on vertical carbon export are available from short-term sediment trap deployments from a ship or from the ice, from estimates of the carbon flux from radionuclides in seawater, or from bottom moored long-term sediment traps. Most often, surface-tethered sediment traps and radionuclide pairs (mainly 234Th/238U, 210Po/210Pb) are used to determine the vertical carbon flux in the uppermost 200–300 m over the course of one day to few weeks (Cai et al., 2010; Honjo et al., 2010; Lalande et al., 2014; Roca-Martí et al., 2016). Moored long-term sediment traps are usually deployed to estimate the flux at greater depth (few 100 to >1000 meter) during many months or a year (Fahl and Nöthig, 2007; Lalande et al., 2009b; Hwang et al., 2015), and allow investigating seasonal trends rather than short-term events.
Overall, the estimated carbon flux in the AO basins is low (120–285 m: <1.5 g C m−2 yr−1, 1550–4090 m: <1.0 g C m−2 yr−1, Table 4), and its magnitude depends on various factors, such as ice thickness, melting period, snow cover, latitude dependent light climate, surface nutrient concentration, and the sediment trap deployment depth. The general sedimentation pattern estimated from long-term sediment traps deployed in Arctic shelf regions and the southern Lomonosov Ridge suggests a daily vertical flux of <5 m g C m−2 d−1 (∼200 m) during most of the year. Only during the ice break-up, sedimentation rates tend to abruptly increase to up to 55 m g C m−2 d−1 (Fahl and Nöthig, 2007; Lalande et al., 2009b). We expect a similar pattern of seasonality in the AO basins, but presume that the quantity of the flux would be even lower.
In addition to the seasonal variation in the quantity of the carbon flux, the composition and quality of sinking matter can also vary. For example, sinking material in the Eurasian Basin consisted of primary producer-derived carbon during the ice melt (Zernova et al., 2000; Lalande et al., 2019), but carbon must also sink out in other forms, because the carbon flux was not always synchronous with the flux of microalgae (Lalande et al., 2019). Ice algae sink during the ice melt in the form of small colonies but also as large ice algal aggregates, which are assumed to sink out very fast (Syvertsen, 1991). This high sinking velocity is bolstered by two observations: algal aggregates at the >3000 m AO deep-sea floor had still high chlorophyll a to pigment ratios, suggesting that they were recently produced (Boetius et al., 2013); moreover, these aggregates at the sea floor had a very similar bacterial community as found in sea-ice (Rapp et al., 2018), which indicates that there was not sufficient time for bacteria in the water column to colonize the aggregate while sinking.
Besides large aggregates, fecal pellets are a vehicle of vertical carbon export. During the algal growth season, consumer abundance increases (Zenkevitch, 1963; Leu et al., 2011) and the ice-associated and pelagic system becomes more heterotrophic (Wassmann, 2011; Wassmann and Reigstad, 2011). With the succession of zooplankton species throughout the growth season the contribution of different fecal pellet types changes seasonally (Matsuno et al., 2016). Fecal pellets, however, only contribute to the carbon export when their sinking velocity is high enough to prevent remineralization in the surface layer. Fecal pellets of larger animals, such as Calanus spp. or larvaceans, tend in general to sink faster than those of microzooplankton. Furthermore, the carbon content and sinking rates depend on feeding rates, food assimilation, and the diet of consumers (Turner, 2015). Pellets produced from a diatom-dominated diet have been found to sink faster because of the ballasting with heavy silicate shells than pellets produced from soft-bodied phytoplankton (Leah and Hans, 1998). Undamaged fecal pellets of Calanus sink at rates of about 5–220 m d−1 (Turner, 2002), and would, in theory, reach the sea floor within several weeks. However, a large proportion of pellets is also consumed or broken up while sinking. It has been previously believed that some copepods species are coprophagous (Auel and Hagen, 2002) and can act as a “coprophagous filter” (Gonzalez and Smetacek, 1994), counteracting the export of carbon in form of fecal pellets. Recent studies, however, conclude that filtering copepods actively reject fecal pellets. During this rejection process, they break them into smaller pieces, reducing the sinking velocity of the fecal pellet carbon, and exposing the fragments also to degradation by bacteria and dinoflagellates (Poulsen and Kiørboe, 2005; Iversen and Poulsen, 2007; Poulsen et al., 2011; Svensen et al., 2014; Turner, 2015). Thus, copepods likely play the role of coprorhexy, rather than coprophagy. Minipellets produced by dinoflagellates may also contribute to the vertical carbon flux (Nöthig and Bodungen, 1989; Wassmann et al., 1991), but it is still unclear to which degree minipellets are consumed and remineralized in the pelagic realm. Overall, microzooplankton and small mesozooplankton in the deep Arctic basins appear to play an important role in fragmenting and degrading sinking material, helping retain carbon in the pelagic zone, rather than contributing substantially to vertical carbon export. In support of this, a study conducted in the Makarov Basin during August, found that the majority of sinking material in the short-term traps (∼80%) was of detrital origin, which could not be classified any further (Olli et al., 2007). It must be assumed that this matter was degraded through various zooplankton activities.
When combining the estimates of the vertical carbon flux in AO basins based on field observations (sediment traps), a vertical export of 0.08–1.50 g C m−2 yr−1 can be assumed at 120–200 m depth (Table 4). This estimate is slightly lower than the suggested downward carbon flux of 1.5–3 g C m−2 yr−1 based on the upward nitrate flux (Randelhoff and Guthrie, 2016). The discrepancy between the two estimates can be explained by the design of sediment traps, which underestimate the flux of very large, rare particles. Furthermore, the estimation of Randelhoff and Guthrie (2016) refers to the vertical carbon flux at the nitracline (located shallower than 100 m), while the data compiled in Table 4 refer (in most instances) to the vertical carbon flux at 200 m. As zooplankton reduce the carbon flux with depth, a somewhat higher carbon flux at shallower depth than at greater depth matches our current understanding of the vertical carbon flux. In addition, at approximately 3000 m and below, the vertical carbon flux was even lower, amounting to 0.01–0.73 g C m−2 yr−1 (Table 4).
A Carbon Budget for the Arctic Ocean Basins
According to the classical concept by Eppley and Peterson (1979), new production equals approximately the export production (export out of the euphotic zone), and thus the amount of carbon that is annually available for pelagic and benthic grazers below the euphotic zone. When we contrast here the benthic carbon demand in the deep AO basins with the estimates of the primary production, the zooplankton carbon demand, and the vertical carbon flux, there seems to be an imbalance between the carbon supply and demand versus the current understanding of the vertical carbon flux (Figure 4). Due to the wide ranges of all estimates, and the various unknown abundances and metabolic rates of organisms from bacteria to mammals, it is challenging to quantify the extent of this gap. However, similar conclusions of estimated vertical carbon flux being insufficient to meet benthic carbon demand have been drawn for the European continental slopes in the Atlantic (Lampitt et al., 1995), the northwest Atlantic (Smith, 1978), the California continental margin (Jahnke et al., 1990), the Canadian Arctic shelf (Amundsen Gulf, Forest et al., 2011; Beaufort Sea, Renaud et al., 2007), and also the Arctic basins (Klages et al., 2004). The mismatch between the carbon flux and the benthic carbon demand may result from either an overestimation of the benthic demand, or from an underestimation of the true carbon flux by the currently available observations. We discuss both alternatives in the following section.
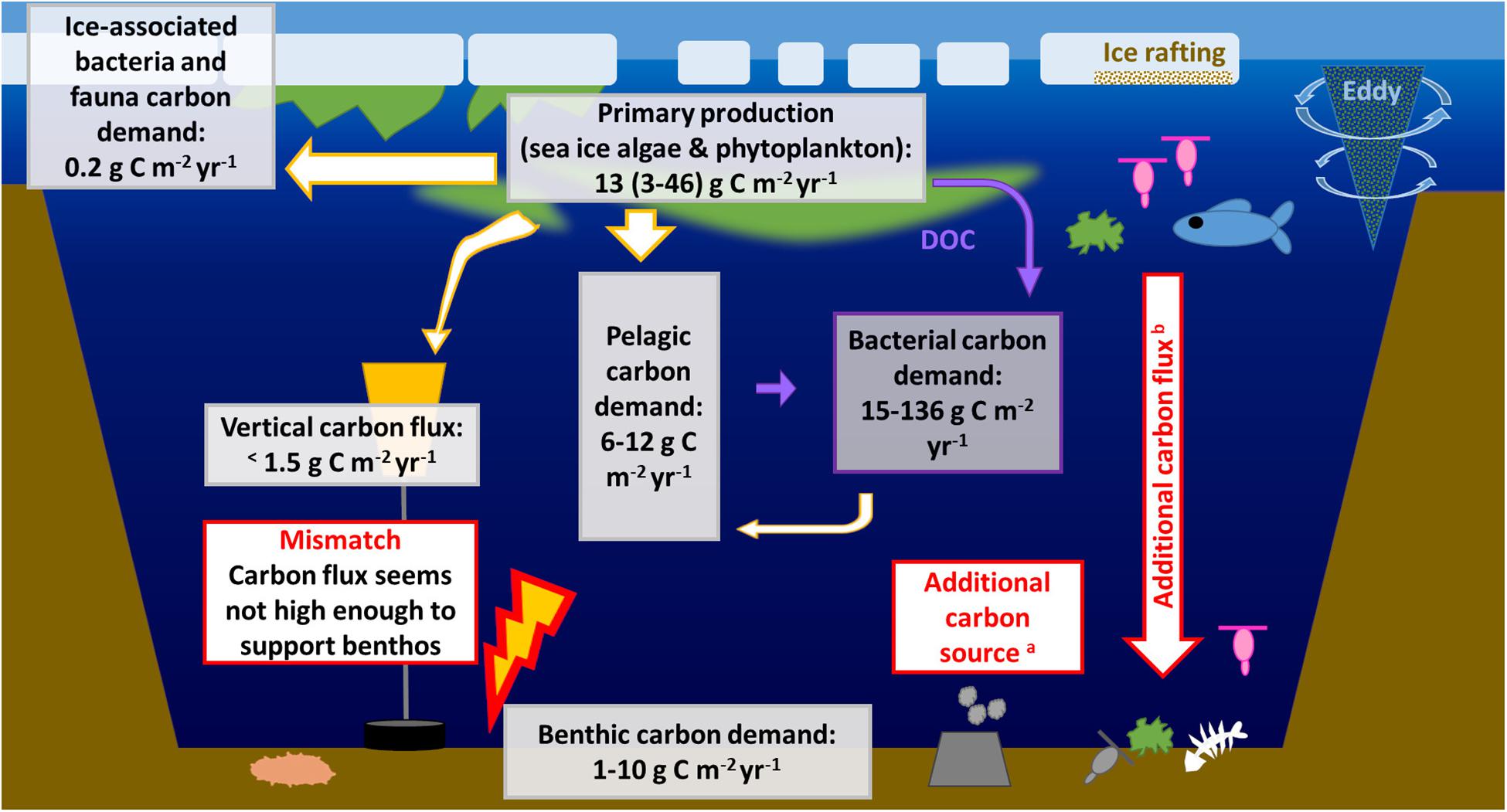
Figure 4. Carbon budget of the central Arctic Ocean basin including the annual mean production, annual ice-associated, pelagic, and benthic carbon demand as well as the vertical carbon flux. The white arrows with orange edge represent particulate organic carbon flow between producers and consumers. The microbial loop and bacterial DOC demand (purple arrows) are not discussed in detail in this work, but are included here to show a complete picture. aChemosynthesis may be a local, benthic carbon source. bAdditional, and so far largely not quantified carbon sources are advection, export of large, fast-sinking algal aggregates, migrating or dead copepods, and large food falls.
What Feeds the Benthos in the Arctic Ocean Basins? Toward Balancing the Carbon Budget
Uncertainties in Estimates of Benthic Carbon Supply and Demand
The AO basin is an open system, and the carbon for the benthos in this area may be partially provided by advection from the shelves and sub-Arctic regions. The large Arctic rivers entrain a substantial amount of dissolved and particulate material derived from terrestrial and freshwater systems into the AO (McClelland et al., 2016). Various studies have found carbon of terrestrial origin in sediment traps at the shelf break, the edge of the AO basins, as well as in the center of the Canadian Basin, the Nansen Basin, and the Amundsen Basin (O’Brien et al., 2006; Fahl and Nöthig, 2007; Forest et al., 2007; Watanabe et al., 2014; Hwang et al., 2015; Nöthig et al., unpublished). In addition, advected marine carbon may arrive from the Atlantic and Pacific Oceans (Wassmann et al., 2015) as well as from the shallow Arctic shelf seas (<200 m), which make up approximately half of the area of the AO (Bluhm et al., 2015). Irrespective of the source, it is still discussed how allochthonous carbon can reach the middle of the AO basins. One possibility of lateral transport is incorporation of organic material into sea ice, which drifts across the AO basins (Wegner et al., 2017). As the distances that these ice “rafts” travel before melting become shorter in a warmer Arctic, the role of this kind of carbon transport may diminish in the future (Krumpen et al., 2019). Moreover, neritic zooplankton and re-suspended carbon from the shelves may be laterally transported into the AO basins by eddies (Mathis et al., 2007; O’Brien et al., 2011; Watanabe et al., 2014). Hwang et al. (2015) estimated that 45–69% of the sinking carbon in the central Canadian Basin may be laterally advected, but as these observations are lacking from other regions of the AO basins, it is challenging to definitely quantify to which extent laterally advected carbon supports the benthos. Finally, the quality of the transported material must also be taken into account: mostly detritus was found in sediment traps deployed at the southern end of the Lomonosov Ridge (Fahl and Nöthig, 2007). This poses the question whether the carbon that is entrained by rivers (McClelland et al., 2016) and/or resuspended on the shelves and slopes and laterally advected to the AO basins, has a sufficient nutritious value for the benthos. Most likely, the C: N ratio of the material is high, and it provides a poor nitrogen source.
Chemosynthetic processes at the deep-sea floor may act as an alternative, local carbon source for the benthos. The extent of chemosynthesis has not been quantified in the AO basins to date, but hydrothermal vent activity may occur along the Gakkel Ridge (Edmonds et al., 2003). As shown in cold-seeps south of Svalbard, chemosynthesis-based carbon can provide a substantial carbon source for dominant macrobenthos (Åström et al., 2019), and this could locally be the case for the Gakkel Ridge as well.
In addition to the gaps in the quantification of carbon supply to the AO benthic ecosystem, the understanding of life cycles and longevity of deep-sea benthos – and thus their true annual carbon demand – is very limited (Degen et al., 2015). The benthic carbon demand estimated in this work must therefore be taken as a very rough estimate. Arctic benthos rely far less on lipid reserves than zooplankton (Graeve et al., 1997), and seem to use different strategies to survive periods of poor food supply. Studies in low- and mid-latitude deep-sea areas found that the low water temperatures at the sea floor lead to low, energy saving metabolic rates in benthos (Childress et al., 1990). In addition, mobile deep-sea organisms are very efficient at finding the sparse food available (Iken et al., 2001; Blankenship and Levin, 2007), and highly flexible in their choice of food (Blankenship and Levin, 2007). When they find food, these organisms can consume a great quantity very fast, and utilize the consumed food efficiently in their long intestine systems (Smith and Baldwin, 1982). Despite such adaptations, benthos seems to experience temporary starvation as indicated by the high δ15N signatures observed at the long-term observatory HAUSGARTEN in the Fram Strait (Bergmann et al., 2009). Thus, the imbalance in our carbon budget may originate from both an underestimation of the carbon supply by lateral advection or chemosynthesis, as well as by an overestimation of the benthic carbon demand in artificial, experimental conditions.
Uncertainties Related to the Vertical Carbon Export
Despite the potential uncertainties related to the benthos itself, we suggest that the major imbalance in our carbon budget is caused by an underestimation of the vertical carbon flux. The few available long-term sediment trap studies from the AO basins represent the vertical flux in approximately 10 m2 (=0.00000024%) of the basins (Jakobsson et al., 2004). These data reveal that annual carbon flux is characterized by long periods with a weak “drizzle,” alternating with short periods of higher sedimentation (Fahl and Nöthig, 2007; Lalande et al., 2019; Nöthig et al., unpublished). The duration of these short-term sedimentation events is still not clearly determined. A long-term sediment trap study north of the Laptev Sea showed that during two-weeks in the period of the ice break-up, 50–85% of the annual carbon flux sank out at 850 m (Lalande et al., 2009a). The lack of a finer temporal resolution leaves the question unanswered if an even heavier “rain” of carbon flux took place during an even shorter period. From the currently available data, we may therefore underestimate the carbon flux, because the really big sedimentation events so far have not been captured. Furthermore, it has been suggested that sediment traps may underestimate the vertical carbon flux when it takes place in form of large particles (Lampitt et al., 2001), because sediment traps are not designed to collect these types of particles. These particles, however, occur frequently in the AO. Scuba divers have observed aggregates of M. arctica under the sea ice already in the 1970s (Melnikov, 1997), and such aggregates were also observed in the deep AO in 2012 (Boetius et al., 2013), although their complex 3D structures make the quantification of their biomass still challenging. Due to their large size, these aggregates have a high sinking velocity, and result in an effective sympagic-benthic coupling, because they bring a substantial amount of high quality (nitrogen-rich) biomass (up to 156 g C m−2) to the sea floor. Presuming the annual benthic carbon demand of 1-10 g C m−2 (section “Deep-Sea Benthos and Its Carbon Demand”), one annual ice algae sinking event, probably taking place within few days, would be enough to feed the local benthos for a whole year. In the light of the currently thinning multiyear ice cover (Kwok, 2018), it is challenging to forecast whether sinking ice algae aggregates will also provide a carbon source to the benthos in the future. Under-ice phytoplankton blooms seem to become more abundant (Horvat et al., 2017), but they seem to be only exported into the deep sea when ballasted with cryogenic gypsum (Wollenburg et al., 2018). Despite potential microbial gardening (Mayor et al., 2014), it is therefore unlikely that slower sinking algal aggregates and marine snow particles provide as much carbon to the benthos as the thick and fast sinking aggregates of M. arctica, which mainly grow under multiyear ice (Syvertsen, 1991; Boetius et al., 2013).
As stated before, the life cycles and abundances of various pelagic organisms are defectively accounted for and so is their impact on the vertical carbon flux. Among the pelagic organisms especially poorly studied are appendicularians (Larvacea), jellyfish, and ctenophores. Studies conducted in temperate and sub-polar regions suggest that appendicularians can considerably contribute to the carbon cycling, because they have (1) high filtration rates, (2) graze upon small particles (<20 μm, Herwig et al., 2004), (3) produce fast sinking fecal pellets, and (4) their houses contribute to the formation of marine snow (Deibel, 1988; Bochdansky and Deibel, 1999; Acuña and Kiefer, 2000; López-Urrutia et al., 2003; Winder et al., 2017). Massive abundances of the appendicularian Oikopleura vanhoeffeni and their fecal pellets during late winter have contributed considerably to the vertical carbon flux in the ice-covered Beaufort Sea (Sampei et al., 2009). It is conceivable that appendicularians play a similar role in the deep Arctic basins, because they have also been observed in the Amundsen Basin (86°N, fall 2016, K. Kosobokova, pers. comm.) and in the Canada Basin (Raskoff et al., 2005, 2010). Comparably, jellyfish and ctenophores can at times contribute substantially to the zooplankton community in the upper 100 m of the AO basins during summer (Raskoff et al., 2005, 2010; Purcell et al., 2010; Wang et al., 2014; David et al., 2015), and prey on various copepod species (Purcell et al., 2010), but their impact on the carbon export is practically unknown.
Abundance and biomass data of Arctic mesozooplankton, and especially copepods in the AO have been extensively studied, but even their role in the biological carbon pump is not entirely understood. One distinct example is the sub-Arctic copepod C. finmarchicus, which is advected to the AO (Hirche and Kosobokova, 2007; Kosobokova, 2012; Wassmann et al., 2015, 2019). Peaks of biomass northeast of Svalbard and north-west of Severnaja Zemlja demarcate the gateways where C. finmarchicus populations enter the Arctic. Individuals of this species represent an allochthones carbon source to the AO, which declines from 5–7 g dry weight m−2 (ca. 2–2.8 g C m−2) at the slopes of the northern Barents Sea to < 0.01 g C m−2 in the Eurasian Basin south of 82°N and < 0.008 g C m−2 north of 86°N (Kosobokova and Hirche, 2009). Even though C. finmarchicus can currently not reproduce and complete its life cycle in the central Arctic, it can contribute up to 30% to the total zooplankton biomass over the continental slope (Bluhm et al., unpublished; Hirche and Kosobokova, 2007) and may be an important consumer of microalgae in the surface (Kosobokova and Hirche, 2009; Kosobokova, 2012; Wassmann et al., 2015). When dying off, the accumulated lipid reserves can sink to depth, and most likely represent another important carbon source. Though less prominent than C. finmarchicus, other expatriate holoplanktonic and meroplanktonic taxa follow the same inflow pathway and experience a similar fate (Sherr et al., 1997; Matsuno et al., 2014; Onda et al., 2017; Ershova et al., 2019). Advection of carbon, often in form of micro-, mero-, and mesoplankton also plays an important role on the Pacific side of the Arctic, though the lower volume transport from the Pacific into the Amerasian Basin causes less biomass advection than on the Atlantic side (Kosobokova et al., 2011; Nelson et al., 2014; Wassmann et al., 2015; Basedow et al., 2018; Moore et al., 2018; Ershova et al., 2019). As described before, lateral transport by shelfbreak eddies may then advect the planktonic expatriates further into the AO basins (Mathis et al., 2007; O’Brien et al., 2011; Watanabe et al., 2014).
Non-consumptive mortality and sinking of zooplankton is rarely measured in sediment traps and accounted for in carbon flux estimates, yet it may comprise up to 30% of total zooplankton mortality (Tang and Elliott, 2014). Measurements of zooplankton north of Svalbard in January showed that up to 95% of Calanus between 300 and 2000 m (Calanus spp. abundance: 12,750 ind. m−2) were dead, many of them still containing substantial lipid sacs (Daase et al., 2014). The causes of these mortality events are poorly known, but possible causes are life cycle features (i.e., depleted lipid stores due to reproduction), diseases, parasites, food depletion or environmental stress (i.e., due to advection from warmer regions, Tang and Elliott, 2014). Regardless of the cause, these mass mortality events may be a considerable source of biomass sinking to the seafloor in the AO basins.
In addition, fresh carbon may also reach the deep-sea benthos in form of dead, large-bodied fauna of all kinds, a phenomenon termed food falls (Stockton and DeLaca, 1982). In the Fram Strait, food falls of large crustaceans and a fish have been observed (Klages et al., 2001; Soltwedel et al., 2003), and they may also occur in the AO basins. Polar cod otoliths have for example also been found at the sea floor of the Amerasian Basin (B.A. Bluhm, pers. observation). This may suggest a frequent export of dying or dead Polar cod, the most abundant Arctic fish (densities in some parts of the Eurasian Basin: 5000 ind. km2) (David et al., 2016). Similarly, top predators, such as belugas (Suydam et al., 2001), bowhead whales (Reeves et al., 2014), and ringed seals (Hamilton et al., 2015) occur at times in the AO basins and may provide, when dying, a very occasional, but immense carbon inputs to the benthos. Detailed succession studies of large food falls from the Fram Strait show that large food falls tend to attract amphipods within minutes (Klages et al., 2001; Premke et al., 2006; Soltwedel et al., 2018), but also enhance the meiofauna biomass (mainly nematodes) in the weeks after the food fall (Soltwedel et al., 2018). As abundance estimates, however, lack for most populations of marine mammals in the AO basins, it is impossible to assess the impact of large food falls for the vertical carbon export (Marz, 2010).
Neither large food falls nor massive sedimentation events of microalgal aggregates nor zooplankton carcasses are accounted for in estimates of vertical carbon flux with sediment traps. Including these additional fluxes may allow closing the gap in the carbon budget presented in this work. In addition, it is equally important to take into consideration the quality of the exported biomass. High quality food may only be provided by short-term, local massive sedimentation events while the constant drizzle brings down highly degraded, low quality material sinking out most of the year.
Overall, we conclude that further quantitative work is needed to fully assess carbon fluxes across the ecosystem in the AO basins, an ecosystem which is characterized by pronounced seasonality and spatial variability. In our opinion, studies on the abundances, the life cycles, and the metabolic rates of a wide range of sympagic, pelagic, and especially benthic organisms are crucially needed to get a better understanding for the interwoven ecosystem structures in the AO basins. Moreover, quantifying the intensity of carbon advection, benthic carbon production, as well as the downward carbon flux in the form of ice algae aggregates may help to close the knowledge gap unraveled in the present work. Autonomous underwater vehicles with cameras as well as autonomous platforms including acoustic sensors may help study the ice underside and the vertical flux of large algal aggregates, which are undersampled in sediment traps. We think these steps forward are necessary to understand the current ecosystem in the AO basins, and to predict how changes in the sea ice, the primary production, and the grazer community may change the carbon supply to the benthos in the AO basins – a system, which is today already affected by anthropogenic waste (e.g., microplastic, Kanhai et al., 2019), and may be exposed to deep-sea mining in the future (Ludvigsen et al., 2017).
Author Contributions
IW, AB, and KK conceived the idea for this article and presented it at the fourth Pan-Arctic Symposium, Motovun, Croatia. IW led the manuscript development. All co-authors contributed to the compilation of literature data, the development, and the writing of this manuscript.
Funding
IW’s contribution was funded by ARCEx, the Research Centre for Arctic Petroleum Exploration (Norwegian Research Council #228107 and industry partners). RG, BB, and EE were supported by Arctic SIZE, a project co-funded by UiT The Arctic University of Norway and the Tromsø Research Foundation (project number 01vm/h15). The research of KK and EE was performed in the framework of the state assignment of IO RAS (theme No. 0149-2019-0008). KK was funded by RFBR grant No. 19- 04-00955 and partially by RSF grant No. 19-17-00058. AB was funded by the European Research Council Advanced Investigator grant ABYSS 294757.
Conflict of Interest
The authors declare that the research was conducted in the absence of any commercial or financial relationships that could be construed as a potential conflict of interest.
Acknowledgments
We would like to thank Paul Wassmann for organizing the fourth Pan-Arctic Symposium in Motovun, Croatia, that initiated this work and all other participants for discussions. In addition, we greatly appreciated the constructive comments by two reviewers.
Supplementary Material
The Supplementary Material for this article can be found online at: https://www.frontiersin.org/articles/10.3389/fmars.2020.00224/full#supplementary-material
References
Acuña, J. L., and Kiefer, M. (2000). Functional response of the appendicularian Oikopleura dioica. Limnol. Oceanogr. 45, 608–618. doi: 10.4319/lo.2000.45.3.0608
Ardyna, M., Babin, M., Gosselin, M., Devred, E., Bélanger, S., Matsuoka, A., et al. (2013). Parameterization of vertical chlorophyll a in the Arctic Ocean: impact of the subsurface chlorophyll maximum on regional, seasonal, and annual primary production estimates. Biogeosciences 10, 4383–4404. doi: 10.5194/bg-10-4383-2013
Arrigo, K. R., Perovich, D. K., Pickart, R. S., Brown, Z. W., van Dijken, G. L., Lowry, K. E., et al. (2012). Massive phytoplankton blooms under Arctic Sea ice. Science 336, 1408–1408. doi: 10.1126/science.1215065
Arrigo, K. R., and van Dijken, G. L. (2011). Secular trends in Arctic Ocean net primary production. J. Geophys. Res. Oceans 116:C09011. doi: 10.1029/2011jc007151
Ashjian, C. J., Campbell, R. G., Welch, H. E., Butler, M., and Van Keuren, D. (2003). Annual cycle in abundance, distribution, and size in relation to hydrography of important copepod species in the western Arctic Ocean. Deep Sea Res. PT I 50, 1235–1261. doi: 10.1016/S0967-0637(03)00129-8
Assmy, P., Fernández-Méndez, M., Duarte, P., Meyer, A., Randelhoff, A., Mundy, C. J., et al. (2017). Leads in Arctic pack ice enable early phytoplankton blooms below snow-covered sea ice. Sci. Rep. 7:40850. doi: 10.1038/srep40850
Åström, E. K. L., Carroll, M. L., Sen, A., Niemann, H., Ambrose, W. G. Jr., Lehmann, M. F., et al. (2019). Chemosynthesis influences food web and community structure in high-Arctic benthos. Mar. Ecol. Prog. Ser. 629, 19–42. doi: 10.3354/meps13101
Auel, H., and Hagen, W. (2002). Mesozooplankton community structure, abundance and biomass in the central Arctic Ocean. Mar. Biol. 140, 1013–1021. doi: 10.1007/s00227-001-0775-4
Auel, H., Klages, M., and Werner, I. (2003). Respiration and lipid content of the Arctic copepod Calanus hyperboreus overwintering 1 m above the seafloor at 2,300 m water depth in the Fram Strait. Mar. Biol. 143, 275–282. doi: 10.1007/s00227-003-1061-4
Babin, M., Bélanger, S., Ellingsen, I., Forest, A., Le Fouest, V., Lacour, T., et al. (2015). Estimation of primary production in the Arctic Ocean using ocean colour remote sensing and coupled physical–biological models: strengths, limitations and how they compare. Prog. Oceanogr. 139, 197–220. doi: 10.1016/j.pocean.2015.08.008
Basedow, S. L., Sundfjord, A., von Appen, W.-J., Halvorsen, E., Kwasniewski, S., and Reigstad, M. (2018). Seasonal variation in transport of zooplankton into the Arctic basin through the Atlantic gateway, Fram. Strait. Front. Mar. Sci. 5:194. doi: 10.3389/fmars.2018.00194
Bell, L. E., Bluhm, B. A., and Iken, K. (2016). Influence of terrestrial organic matter in marine food webs of the Beaufort Sea shelf and slope. Mar. Ecol. Prog. Ser. 550, 1–24. doi: 10.3354/meps11725
Berge, J., Varpe, Ø, Moline, M. A., Wold, A., Renaud, P. E., Daase, M., et al. (2012). Retention of ice-associated amphipods: possible consequences for an ice-free Arctic Ocean. Biol. Lett. 8, 1012–1015. doi: 10.1098/rsbl.2012.0517
Bergmann, M., Dannheim, J., Bauerfeind, E., and Klages, M. (2009). Trophic relationships along a bathymetric gradient at the deep-sea observatory HAUSGARTEN. Deep Sea Res. PT I 56, 408–424. doi: 10.1016/j.dsr.2008.10.004
Bergmann, M., Soltwedel, T., and Klages, M. (2011). The interannual variability of megafaunal assemblages in the Arctic deep sea: preliminary results from the HAUSGARTEN observatory (79°N). Deep Sea Res. PT I 58, 711–723. doi: 10.1016/j.dsr.2011.03.007
Bienhold, C., Zinger, L., Boetius, A., and Ramette, A. (2016). Diversity and biogeography of bathyal and abyssal seafloor bacteria. PLoS One 11:e0148016. doi: 10.1371/journal.pone.0148016
Blankenship, L. E., and Levin, L. A. (2007). Extreme food webs: foraging strategies and diets of scavenging amphipods from the ocean’s deepest 5 kilometers. Limnol. Oceanogr. 52, 1685–1697. doi: 10.4319/lo.2007.52.4.1685
Bluhm, B. A., Ambrose, W. G., Bergmann, M., Clough, L. M., Gebruk, A. V., Hasemann, C., et al. (2011). Diversity of the arctic deep-sea benthos. Mar. Biodivers. 41, 87–107. doi: 10.1007/s12526-010-0078-4
Bluhm, B. A., and Gradinger, R. (2008). Regional variability in food availability for Arctic marine mammals. Ecol. Appl. 18, S77–S96. doi: 10.1890/06-0562.1
Bluhm, B. A., Hop, H., Vihtakari, M., Gradinger, R., Iken, K., Melnikov, I. A., et al. (2018). Sea ice meiofauna distribution on local to pan-Arctic scales. Ecol. Evol. 8, 2350–2364. doi: 10.1002/ece3.3797
Bluhm, B. A., Kosobokova, K. N., and Carmack, E. C. (2015). A tale of two basins: an integrated physical and biological perspective of the deep Arctic Ocean. Prog. Oceanogr. 139, 89–121. doi: 10.1016/j.pocean.2015.07.011
Bluhm, B. A., MacDonald, I. R., Debenham, C., and Iken, K. (2005). Macro- and megabenthic communities in the high Arctic Canada basin: initial findings. Polar Biol. 28, 218–231. doi: 10.1007/s00300-004-0675-4
Bochdansky, A. B., and Deibel, D. (1999). Functional feeding response and behavioral ecology of Oikopleura vanhoeffeni (Appendicularia, Tunicata). J. Exp. Mar. Biol. Ecol. 233, 181–211. doi: 10.1016/S0022-0981(98)00109-9
Boetius, A., Albrecht, S., Bakker, K., Bienhold, C., Felden, J., Fernández-Méndez, M., et al. (2013). Export of algal biomass from the melting Arctic sea ice. Science 339, 1430–1432. doi: 10.1126/science.1231346
Boetius, A., and Damm, E. (1998). Benthic oxygen uptake, hydrolytic potentials and microbial biomass at the Arctic continental slope. Deep Sea Res. PT I 45, 239–275. doi: 10.1016/S0967-0637(97)00052-6
Booth, B. C., and Horner, R. A. (1997). Microalgae on the arctic ocean section, 1994: species abundance and biomass. Deep Sea Res. PT II 44, 1607–1622. doi: 10.1016/S0967-0645(97)00057-X
Bourgeois, S., Archambault, P., and Witte, U. (2017). Organic matter remineralization in marine sediments: a pan-arctic synthesis. Global Biogeochem. Cy. 31, 190–213. doi: 10.1002/2016gb005378
Cai, P., Rutgers van der Loeff, M., Stimac, I., Nöthig, E.-M., Lepore, K., and Moran, S. B. (2010). Low export flux of particulate organic carbon in the central Arctic Ocean as revealed by 234Th:238U disequilibrium. J. Geophys. Res. Oceans 115:C10037. doi: 10.1029/2009jc005595
Campbell, K., Mundy, C., Gosselin, M., Landy, J., Delaforge, A., and Rysgaard, S. (2017). Net community production in the bottom of first-year sea ice over the Arctic spring bloom. Geophys. Res. Lett. 44, 8971–8978. doi: 10.1002/2017GL074602
Campbell, R. G., Ashjian, C. J., Sherr, E. B., Sherr, B. F., Lomas, M. W., Ross, C., et al. (2016). Mesozooplankton grazing during spring sea-ice conditions in the eastern Bering Sea. Deep Sea Res. Part II 134, 157–172. doi: 10.1016/j.dsr2.2015.11.003
Campbell, R. G., Sherr, E. B., Ashjian, C. J., Plourde, S., Sherr, B. F., Hill, V., et al. (2009). Mesozooplankton prey preference and grazing impact in the western Arctic Ocean. Deep Sea Res. PT II 56, 1274–1289. doi: 10.1016/j.dsr2.2008.10.027
Cathalot, C., Rabouille, C., Sauter, E., Schewe, I., and Soltwedel, T. (2015). Benthic oxygen uptake in the Arctic ocean margins – a case study at the deep-sea observatory HAUSGARTEN (Fram Strait). PLoS One 10:e0138339. doi: 10.1371/journal.pone.0138339
Childress, J. J., Cowles, D. L., Favuzzi, J. A., and Mickel, T. J. (1990). Metabolic rates of benthic deep-sea decapod crustaceans decline with increasing depth primarily due to the decline in temperature. Deep Sea Res. 37, 929–949. doi: 10.1016/0198-0149(90)90104-4
Clough, L. M., Ambrose, W. G., Kirk Cochran, J., Barnes, C., Renaud, P. E., and Aller, R. C. (1997). Infaunal density, biomass and bioturbation in the sediments of the Arctic Ocean. Deep Sea Res. PT II 44, 1683–1704. doi: 10.1016/S0967-0645(97)00052-0
Coakley, B., Brumley, K., Lebedeva-Ivanova, N., and Mosher, D. (2016). Exploring the geology of the central Arctic Ocean; understanding the basin features in place and time. J. Geol. Soc. London 173, 967–987. doi: 10.1144/jgs2016-082
Codispoti, L. A., Kelly, V., Thessen, A., Matrai, P., Suttles, S., Hill, V., et al. (2013). Synthesis of primary production in the Arctic Ocean: III. Nitrate and phosphate based estimates of net community production. Prog. Oceanogr. 110, 126–150. doi: 10.1016/j.pocean.2012.11.006
Conover, R. J. (1988). Comparative life histories in the genera Calanus and Neocalanus in high latitudes of the northern hemisphere. Hydrobiologia 167, 127–142. doi: 10.1007/bf00026299
Daase, M., Varpe, Ø, and Falk-Petersen, S. (2014). Non-consumptive mortality in copepods: occurrence of Calanus spp. carcasses in the Arctic Ocean during winter. J. Plankton Res. 36, 129–144. doi: 10.1093/plankt/fbt079
Damare, S., and Raghukumar, C. (2008). Fungi and macroaggregation in deep-sea sediments. Microbial Ecol. 56, 168–177. doi: 10.1007/s00248-007-9334-y
Darnis, G., and Fortier, L. (2014). Temperature, food and the seasonal vertical migration of key arctic copepods in the thermally stratified Amundsen Gulf (Beaufort Sea, Arctic Ocean). J. Plankton Res. 36, 1092–1108. doi: 10.1093/plankt/fbu035
David, C., Lange, B., Krumpen, T., Schaafsma, F., van Franeker, J. A., and Flores, H. (2016). Under-ice distribution of polar cod Boreogadus saida in the central Arctic Ocean and their association with sea-ice habitat properties. Polar Biol. 39, 981–994. doi: 10.1007/s00300-015-1774-0
David, C., Lange, B., Rabe, B., and Flores, H. (2015). Community structure of under-ice fauna in the Eurasian central Arctic Ocean in relation to environmental properties of sea-ice habitats. Mar. Ecol. Prog. Ser. 522, 15–32. doi: 10.3354/meps11156
David, C., Schaafsma, F. L., van Franeker, J. A., Lange, B., Brandt, A., and Flores, H. (2017). Community structure of under-ice fauna in relation to winter sea-ice habitat properties from the Weddell Sea. Polar Biol. 40, 247–261. doi: 10.1007/s00300-016-1948-4
Degen, R., Aune, M., Bluhm, B. A., Cassidy, C., Kêdra, M., Kraan, C., et al. (2018). Trait-based approaches in rapidly changing ecosystems: a roadmap to the future polar oceans. Ecol. Indic. 91, 722–736. doi: 10.1016/j.ecolind.2018.04.050
Degen, R., Vedenin, A., Gusky, M., Boetius, A., and Brey, T. (2015). Patterns and trends of macrobenthic abundance, biomass and production in the deep Arctic Ocean. Polar Res. 34:24008. doi: 10.3402/polar.v34.24008
Deibel, D. (1988). Filter feeding by Oikopleura vanhoeffeni: grazing impact on suspended particles in cold ocean waters. Mar. Biol. 99, 177–186. doi: 10.1007/bf00391979
Deming, J. W., and Yager, P. L. (1992). “Natural bacterial assemblages in deep-sea sediments: towards a global view,” in Deep-Sea Food Chains and the Global Carbon Cycle, eds G. T. Rowe and V. Pariente (Dordrecht: Springer), 11–27. doi: 10.1007/978-94-011-2452-2_2
Edmonds, H. N., Michael, P. J., Baker, E. T., Connelly, D. P., Snow, J. E., Langmuir, C. H., et al. (2003). Discovery of abundant hydrothermal venting on the ultraslow-spreading Gakkel ridge in the Arctic Ocean. Nature 421, 252–256. doi: 10.1038/nature01351
Eppley, R. W., and Peterson, B. J. (1979). Particulate organic matter flux and planktonic new production in the deep ocean. Nature 282, 677–680. doi: 10.1038/282677a0
Ershova, E. A., Descoteaux, R., Wangensteen, O. S., Iken, K., Hopcroft, R. R., Smoot, C., et al. (2019). Diversity and distribution of meroplanktonic larvae in the Pacific Arctic and connectivity with adult benthic invertebrate communities. Front. Mar. Sci. 6:490. doi: 10.3389/fmars.2019.00490
Ershova, E. A., and Kosobokova, K. (2019). Cross-shelf structure and distribution of mesozooplankton communities in the East-Siberian Sea and the adjacent Arctic Ocean. Pol. Biol. 42, 1353–1367. doi: 10.1007/s00300-019-02523-2
Fahl, K., and Nöthig, E.-M. (2007). Lithogenic and biogenic particle fluxes on the Lomonosov Ridge (central Arctic Ocean) and their relevance for sediment accumulation: vertical vs. lateral transport. Deep Sea Res. PT I 54, 1256–1272. doi: 10.1016/j.dsr.2007.04.014
Fernández-Méndez, M., Katlein, C., Rabe, B., Nicolaus, M., Peeken, I., Bakker, K., et al. (2015). Photosynthetic production in the central Arctic Ocean during the record sea-ice minimum in 2012. Biogeosciences 12, 3525–3549. doi: 10.5194/bg-12-3525-2015
Forest, A., Sampei, M., Hattori, H., Makabe, R., Sasaki, H., Fukuchi, M., et al. (2007). Particulate organic carbon fluxes on the slope of the Mackenzie Shelf (Beaufort Sea): physical and biological forcing of shelf-basin exchanges. J. Mar. Syst. 68, 39–54. doi: 10.1016/j.jmarsys.2006.10.008
Forest, A., Tremblay, -É, Gratton, Y., Martin, J., Gagnon, J., Darnis, G., et al. (2011). Biogenic carbon flows through the planktonic food web of the Amundsen Gulf (Arctic Ocean): a synthesis of field measurements and inverse modeling analyses. Prog. Oceanogr. 91, 410–436. doi: 10.1016/j.pocean.2011.05.002
Geynrikh, A. K., Kosobokova, K., and Rudyakov, Y. A. (1983). Seasonal variations in the vertical distribution of some prolific copepods of the Arctic basin. Biol. Tsentral. Arkticheskogo Basseina 4925, 1–22.
Glud, R. N. (2008). Oxygen dynamics of marine sediments. Mar. Biol. Res. 4, 243–289. doi: 10.1080/17451000801888726
Gonzalez, H. E., and Smetacek, V. (1994). The possible role of the cyclopoid copepod Oithona in retarding vertical flux of zooplankton faecal material. Mar. Ecol. Prog. Ser. 113, 233–246. doi: 10.3354/meps113233
Gosselin, M., Levasseur, M., Wheeler, P. A., Horner, R. A., and Booth, B. C. (1997). New measurements of phytoplankton and ice algal production in the Arctic Ocean. Deep Sea Res. PT II 44, 1623–1644. doi: 10.1016/S0967-0645(97)00054-4
Gradinger, R. (1996). Occurrence of an algal bloom under Arctic pack ice. Mar. Ecol. Prog. Ser. 131, 301–305. doi: 10.3354/meps131301
Gradinger, R. (1999). Integrated abundance and biomass of sympagic meiofauna in Arctic and Antarctic pack ice. Polar Biol. 22, 169–177. doi: 10.1007/s003000050407
Gradinger, R. (2009). Sea-ice algae: major contributors to primary production and algal biomass in the Chukchi and Beaufort seas during may/june 2002. Deep Sea Res. PT II 56, 1201–1212. doi: 10.1016/j.dsr2.2008.10.016
Gradinger, R. R., and Bluhm, B. A. (2004). In-situ observations on the distribution and behavior of amphipods and Arctic cod (Boreogadus saida) under the sea ice of the High Arctic Canada Basin. Polar Biol. 27, 595–603. doi: 10.1007/s00300-004-0630-4
Graeve, M., Kattner, G., and Piepenburg, D. (1997). Lipids in Arctic benthos: does the fatty acid and alcohol composition reflect feeding and trophic interactions? Polar Biol. 18, 53–61. doi: 10.1007/s003000050158
Grebmeier, J. M., and Cooper, L. (2014). PacMARS Sediment Community Oxygen Uptake. Version 1.0. UCAR/NCAR, Earth Observing Laboratory. Available online at: http://pacmars.eol.ucar.edu (accessed October 19, 2019).
Hamilton, C. D., Lydersen, C., Ims, R. A., and Kovacs, K. M. (2015). Predictions replaced by facts: a keystone species’ behavioural responses to declining arctic sea-ice. Biol. Lett. 11:20150803. doi: 10.1098/rsbl.2015.0803
Hansen, F. C., Reckermann, M., Breteler, W. C. M., and Riegman, R. (1993). Phaeocystis blooming enhanced by copepod predation on protozoa – evidence from incubation experiments. Mar. Ecol. Prog. Ser. 102, 51–57. doi: 10.3354/meps102051
Haq, S. M. (1967). Nutritional physiology of Metridia lucens and M. longa from the Gulf of Maine. Limnol. Oceanogr. 12, 40–51. doi: 10.4319/lo.1967.12.1.0040
Hassett, B. T., Borrego, E. J., Vonnahme, T. R., Rämä, T., Kolomiets, M. V., and Gradinger, R. (2019). Arctic marine fungi: biomass, functional genes, and putative ecological roles. ISME J. 13, 1484–1496. doi: 10.1038/s41396-019-0368-1
Herwig, S., Olav, V., Bettina, L., Wendy, R., and Yngvar, O. (2004). Calanoid copepods and nutrient enrichment determine population dynamics of the appendicularian Oikopleura dioica: a mesocosm experiment. Mar. Ecol. Prog. Ser. 270, 209–215. doi: 10.3354/meps270209
Hill, V. J., Matrai, P. A., Olson, E., Suttles, S., Steele, M., Codispoti, L. A., et al. (2013). Synthesis of integrated primary production in the Arctic Ocean: II. In situ and remotely sensed estimates. Prog. Oceanogr. 110, 107–125. doi: 10.1016/j.pocean.2012.11.005
Hirche, H.-J. (2013). Long-term experiments on lifespan, reproductive activity and timing of reproduction in the Arctic copepod Calanus hyperboreus. Mar. Biol. 160, 2469–2481. doi: 10.1007/s00227-013-2242-4
Hirche, H.-J., and Kosobokova, K. (2007). Distribution of Calanus finmarchicus in the northern North Atlantic and Arctic Ocean—expatriation and potential colonization. Deep Sea Res. PT II 54, 2729–2747. doi: 10.1016/j.dsr2.2007.08.006
Hirche, H.-J., and Kwasniewski, S. (1997). Distribution, reproduction and development of Calanus species in the Northeast water in relation to environmental conditions. J. Mar. Syst. 10, 299–317. doi: 10.1016/S0924-7963(96)00057-7
Hirche, H.-J., Muyakshin, S., Klages, M., and Auel, H. (2006). Aggregation of the Arctic copepod Calanus hyperboreus over the ocean floor of the Greenland Sea. Deep Sea Res. PT I 53, 310–320. doi: 10.1016/j.dsr.2005.08.005
Hoffmann, R., Braeckman, U., Hasemann, C., and Wenzhöfer, F. (2018). Deep-sea benthic communities and oxygen fluxes in the Arctic Fram Strait controlled by sea-ice cover and water depth. Biogeosciences 15, 4849–4869. doi: 10.5194/bg-15-4849-2018
Honjo, S., Krishfield, R. A., Eglinton, T. I., Manganini, S. J., Kemp, J. N., Doherty, K., et al. (2010). Biological pump processes in the cryopelagic and hemipelagic Arctic Ocean: Canada basin and chukchi rise. Prog. Oceanogr. 85, 137–170. doi: 10.1016/j.pocean.2010.02.009
Hop, H., Poltermann, M., Lønne, O. J., Falk-Petersen, S., Korsnes, R., and Budgell, W. P. (2000). Ice amphipod distribution relative to ice density and under-ice topography in the northern Barents Sea. Polar Biol. 23, 357–367. doi: 10.1007/s003000050456
Hop, H., Vihtakari, M., Bluhm, B. A., Assmy, P., Poulin, M., Gradinger, R., et al. (this issue). Changes in sea-ice protist diversity with declining sea ice in the Arctic Ocean from the 1980s to 2010s. Front.Mar. Sci. doi: 10.3389/fmars.2020.00243
Hopcroft, R., Clarke, C., Nelson, R., and Raskoff, K. (2005). Zooplankton communities of the Arctic’s Canada basin: the contribution by smaller taxa. Polar Biol. 28, 198–206. doi: 10.1007/s00300-004-0680-7
Horvat, C., Jones, D., Iams, S., Schroeder, D., Flocco, D., and Feltham, D. (2017). The frequency and extent of sub-ice phytoplankton blooms in the Arctic Ocean. Sci. Adv. 3:e1601191. doi: 10.1126/sciadv.1601191
Hoste, E., Vanhove, S., Schewe, I., Soltwedel, T., and Vanreusel, A. (2007). Spatial and temporal variations in deep-sea meiofauna assemblages in the Marginal Ice Zone of the Arctic Ocean. Deep Sea Res. PT I 54, 109–129. doi: 10.1016/j.dsr.2006.09.007
Hulth, S., Blackburn, T. H., and Hall, P. O. J. (1994). Arctic sediments (Svalbard): consumption and microdistribution of oxygen. Mar. Chem. 46, 293–316. doi: 10.1016/0304-4203(94)90084-1
Hwang, J., Kim, M., Manganini, S. J., McIntyre, C. P., Haghipour, N., Park, J., et al. (2015). Temporal and spatial variability of particle transport in the deep Arctic Canada Basin. J. Geophys. Res. Oceans 120, 2784–2799. doi: 10.1002/2014jc010643
Iken, K., Bluhm, B., and Gradinger, R. (2005). Food web structure in the high Arctic Canada basin: evidence from δ13C and δ15N analysis. Polar Biol. 28, 238–249. doi: 10.1007/s00300-004-0669-2
Iken, K., Brey, T., Wand, U., Voigt, J., and Junghans, P. (2001). Food web structure of the benthic community at the porcupine abyssal plain (NE Atlantic): a stable isotope analysis. Prog. Oceanogr. 50, 383–405. doi: 10.1016/S0079-6611(01)00062-3
Iversen, K. R., and Seuthe, L. (2011). Seasonal microbial processes in a high-latitude fjord (Kongsfjorden, Svalbard): I. Heterotrophic bacteria, picoplankton and nanoflagellates. Polar Biol. 34, 731–749. doi: 10.1007/s00300-010-0929-2
Iversen, M. H., and Poulsen, L. K. (2007). Coprorhexy, coprophagy, and coprochaly in the copepods Calanus helgolandicus, Pseudocalanus elongatus, and Oithona similis. Mar. Ecol. Prog. Ser. 350, 79–89. doi: 10.3354/meps07095
Jahnke, R. A., Reimers, C. E., and Craven, D. B. (1990). Intensification of recycling of organic matter at the sea floor near ocean margins. Nature 348, 50–54. doi: 10.1038/348050a0
Jakobsson, M., Grantz, A., Kristoffersen, Y., and Macnab, R. (2004). “The Arctic ocean: boundary conditions and background information,” in The Organic Carbon Cycle in the Arctic Ocean, eds R. Stein and R. W. Macdonald (Berlin: Springer), 1–32. doi: 10.1007/978-3-642-18912-8_1
Kanhai, L. D. K., Johansson, C., Frias, J. P. G. L., Gardfeldt, K., Thompson, R. C., and O’Connor, I. (2019). Deep sea sediments of the Arctic central basin: a potential sink for microplastics. Deep Sea Res. I 145, 137–142. doi: 10.1016/j.dsr.2019.03.003
Kilias, E., Kattner, G., Wolf, C., Frickenhaus, S., and Metfies, K. (2014). A molecular survey of protist diversity through the central Arctic Ocean. Polar Biol. 37, 1271–1287. doi: 10.1007/s00300-014-1519-5
Kiørboe, T. (2013). Zooplankton body composition. Limnol. Oceanogr. 58, 1843–1850. doi: 10.4319/lo.2013.58.5.1843
Kirchman, D. L., Hill, V., Cottrell, M. T., Gradinger, R., Malmstrom, R. R., and Parker, A. (2009). Standing stocks, production, and respiration of phytoplankton and heterotrophic bacteria in the western Arctic Ocean. Deep Sea Res. PT II 56, 1237–1248. doi: 10.1016/j.dsr2.2008.10.018
Klages, M., Boetius, A., Christensen, J. P., Deubel, H., Piepenburg, D., Schewe, I., et al. (2004). “The benthos of Arctic seas and its role for the organic carbon cycle at the seafloor,” in The Organic Carbon Cycle in the Arctic Ocean, eds R. Stein and R. W. Macdonald (Berlin: Springer), 139–167. doi: 10.1007/978-3-642-18912-8_6
Klages, M., Vopel, K., Bluhm, H., Brey, T., Soltwedel, T., and Arntz, W. E. (2001). Deep-sea food falls: first observation of a natural event in the Arctic ocean. Polar Biol. 24, 292–295. doi: 10.1007/s003000000199
Kohlbach, D., Graeve, M., Lange, B., David, C., Peeken, I., and Flores, H. (2016). The importance of ice algae-produced carbon in the central Arctic ocean ecosystem: food web relationships revealed by lipid and stable isotope analyses. Limnol. Oceanogr. 61, 2027–2044. doi: 10.1002/lno.10351
Kosobokova, K. (1999). The reproductive cycle and life history of the Arctic copepod Calanus glacialis in the White Sea. Polar Biol. 22, 254–263. doi: 10.1007/s003000050418
Kosobokova, K., and Hirche, H.-J. (2000). Zooplankton distribution across the Lomonosov ridge, Arctic ocean: species inventory, biomass and vertical structure. Deep Sea Res. PT I 47, 2029–2060. doi: 10.1016/S0967-0637(00)00015-7
Kosobokova, K., and Hirche, H.-J. (2009). Biomass of zooplankton in the eastern Arctic ocean – a base line study. Prog. Oceanogr. 82, 265–280. doi: 10.1016/j.pocean.2009.07.006
Kosobokova, K., and Hopcroft, R. R. (2010). Diversity and vertical distribution of mesozooplankton in the Arctic’s Canada basin. Deep Sea Res. PT II 57, 96–110. doi: 10.1016/j.dsr2.2009.08.009
Kosobokova, K., Hopcroft, R. R., and Hirche, H.-J. (2011). Patterns of zooplankton diversity through the depths of the Arctic’s central basins. Mar. Biodivers. 41, 29–50. doi: 10.1007/s12526-010-0057-9
Kosobokova, K., and Pertsova, N. M. (2005). Zooplankton of the deep-water part of the white sea at the end of the hydrological winter. Oceanology 45, 819–831.
Kosobokova, K. N. (2012). Zooplankton of the Arctic Ocean: Community Structure, Ecology, Spatial Distribution. Moskow: GEOS.
Kromkamp, J., Capuzzo, E., and Philippart, C. J. M. (2017). Measuring Phytoplankton Primary Production: Review of Existing Methodologies and Suggestions for a Common Approach, EcApRHA Deliverable WP 3.2. Vol 28. Available online at: http://www.vliz.be/imisdocs/publications/89/304289.pdf (accessed October 24, 2019).
Krumpen, T., Belter, H. J., Boetius, A., Damm, E., Haas, C., Hendricks, S., et al. (2019). Arctic warming interrupts the transpolar drift and affects long-range transport of sea ice and ice-rafted matter. Sci. Rep. 9:5459. doi: 10.1038/s41598-019-41456-y
Kvile, K. Ø, Ashjian, C., and Ji, R. (2019). Pan-Arctic depth distribution of diapausing Calanus Copepods. Biol. Bull. 237, 76–89. doi: 10.1086/704694
Kwok, R. (2018). Arctic sea ice thickness, volume, and multiyear ice coverage: losses and coupled variability (1958–2018). Environ. Res. Lett. 13:105005. doi: 10.1088/1748-9326/aae3ec
Lalande, C., Bélanger, S., and Fortier, L. (2009a). Impact of a decreasing sea ice cover on the vertical export of particulate organic carbon in the northern Laptev Sea, Siberian Arctic Ocean. Geophys. Res. Lett. 36:L21604. doi: 10.1029/2009GL040570
Lalande, C., Forest, A., Barber, D. G., Gratton, Y., and Fortier, L. (2009b). Variability in the annual cycle of vertical particulate organic carbon export on Arctic shelves: contrasting the Laptev Sea, Northern Baffin Bay and the Beaufort Sea. Cont. Shelf Res. 29, 2157–2165. doi: 10.1016/j.csr.2009.08.009
Lalande, C., Nöthig, E.-M., and Fortier, L. (2019). Algal export in the Arctic ocean in times of global warming. Geophys. Res. Lett. 46, 5959–5967. doi: 10.1029/2019gl083167
Lalande, C., Nöthig, E.-M., Somavilla, R., Bauerfeind, E., Shevchenko, V., and Okolodkov, Y. (2014). Variability in under-ice export fluxes of biogenic matter in the Arctic Ocean. Global Biogeochem. Cy. 28, 571–583. doi: 10.1002/2013GB004735
Lampitt, R. S., Bett, B. J., Kiriakoulakis, K., Popova, E. E., Ragueneau, O., Vangriesheim, A., et al. (2001). Material supply to the abyssal seafloor in the Northeast Atlantic. Prog. Oceanogr. 50, 27–63. doi: 10.1016/S0079-6611(01)00047-7
Lampitt, R. S., Raine, R. C. T., Billett, D. S. M., and Rice, A. L. (1995). Material supply to the European continental slope: a budget based on benthic oxygen demand and organic supply. Deep Sea Res. PT I 42, 1865–1880. doi: 10.1016/0967-0637(95)00084-4
Lane, P. V. Z., Llinás, L., Smith, S. L., and Pilz, D. (2008). Zooplankton distribution in the western Arctic during summer 2002: hydrographic habitats and implications for food chain dynamics. J. Mar. Syst. 70, 97–133. doi: 10.1016/j.jmarsys.2007.04.001
Leah, R. F., and Hans, G. D. (1998). Effects of diet on dimensions, density and sinking rates of fecal pellets of the copepod Acartia tonsa. Mar. Ecol. Prog. Ser. 175, 87–96. doi: 10.3354/meps175087
Leu, E., Mundy, C. J., Assmy, P., Campbell, K., Gabrielsen, T. M., Gosselin, M., et al. (2015). Arctic spring awakening – steering principles behind the phenology of vernal ice algal blooms. Prog. Oceanogr. 139, 151–170. doi: 10.1016/j.pocean.2015.07.012
Leu, E., Søreide, J. E., Hessen, D. O., Falk-Petersen, S., and Berge, J. (2011). Consequences of changing sea-ice cover for primary and secondary producers in the European Arctic shelf seas: Timing, quantity, and quality. Progr. Oceanogr. 90, 18–32. doi: 10.1016/j.pocean.2011.02.004
Levin, L. A., Etter, R. J., Rex, M. A., Gooday, A. J., Smith, C. R., Pineda, J., et al. (2001). Environmental influences on regional deep-sea species diversity. Annu. Rev. Ecol. Syst. 32, 51–93. doi: 10.1146/annurev.ecolsys.32.081501.114002
López-Urrutia, Á, Irigoien, X., Acuña, J. L., and Harris, R. (2003). In situ feeding physiology and grazing impact of the appendicularian community in temperate waters. Mar. Ecol. Prog. Ser. 252, 125–141. doi: 10.3354/meps252125
Luchetta, A., Lipizer, M., and Socal, G. (2000). Temporal evolution of primary production in the central Barents Sea. J. Mar. Syst. 27, 177–193. doi: 10.1016/S0924-7963(00)00066-X
Ludvigsen, M., Søreide, F., Aasly, K., Ellefmo, S., Zylstra, M., and Pardey, M. (2017). “ROV based drilling for deep sea mining exploration,” in Proceedings of the OCEANS 2017 – Aberdeen, 19-22 June 2017, (Aberdeen: IEEE), 1–6.
MacDonald, I. R., Bluhm, B. A., Iken, K., Gagaev, S., and Strong, S. (2010). Benthic macrofauna and megafauna assemblages in the Arctic deep-sea Canada Basin. Deep Sea Res. PT II 57, 136–152. doi: 10.1016/j.dsr2.2009.08.012
Majewski, A. R., Atchison, S., MacPhee, S., Eert, J., Niemi, A., Michel, C., et al. (2017). Marine fish community structure and habitat associations on the Canadian Beaufort shelf and slope. Deep Sea Res. PT I 121, 169–182. doi: 10.1016/j.dsr.2017.01.009
Marz, S. (2010). “Arctic sea-ice ecosystems,” in Arctic Biodiversity Trends 2010 Selected Indicators of Change, eds T. Kurvits, B. Alfthan, and E. Mork (Akureyri: CAFF International Secretariat), 58–61.
Massicotte, P., Peeken, I., Katlein, C., Flores, H., Huot, Y., Castellani, G., et al. (2019). Sensitivity of phytoplankton primary production estimates to available irradiance under heterogeneous sea ice conditions. J. Geophys. Res. Oceans 124, 5436–5450. doi: 10.1029/2019jc015007
Mathis, J. T., Pickart, R. S., Hansell, D. A., Kadko, D., and Bates, N. R. (2007). Eddy transport of organic carbon and nutrients from the Chukchi Shelf: impact on the upper halocline of the western Arctic Ocean. J. Geophys. Res. Oceans 112:C05011. doi: 10.1029/2006jc003899
Matsuno, K., Ichinomiya, M., Yamaguchi, A., Imai, I., and Kikuchi, T. (2014). Horizontal distribution of microprotist community structure in the western Arctic Ocean during late summer and early fall of 2010. Polar Res. 37, 1185–1195. doi: 10.1007/s00300-014-1512-z
Matsuno, K., Yamaguchi, A., Fujiwara, A., Onodera, J., Watanabe, E., Harada, N., et al. (2016). Seasonal changes in mesozooplankton swimmer community and fecal pellets collected by sediment trap moored at the Northwind Abyssal Plain in the western Arctic Ocean. Bull. Fish. Sci. Hokkaido Univ. 66, 77–85. doi: 10.14943/bull.fish.66.2.77
Mayor, D. J., Sanders, R., Giering, S. L. C., and Anderson, T. R. (2014). Microbial gardening in the ocean’s twilight zone: detritivorous metazoans benefit from fragmenting, rather than ingesting, sinking detritus. BioEssays 36, 1132–1137. doi: 10.1002/bies.201400100
McClelland, J. W., Holmes, R. M., Peterson, B. J., Raymond, P. A., Striegl, R. G., Zhulidov, A. V., et al. (2016). Particulate organic carbon and nitrogen export from major Arctic rivers. Global Biogeochem. Cy. 30, 629–643. doi: 10.1002/2015gb005351
Melnikov, I. A., Kolosova, E. G., Welch, H. E., and Zhitina, L. S. (2002). Sea ice biological communities and nutrient dynamics in the Canada Basin of the Arctic Ocean. Deep Sea Res. PT I 49, 1623–1649. doi: 10.1016/S0967-0637(02)00042-0
Metfies, K., von Appen, W.-J., Kilias, E., Nicolaus, A., and Nöthig, E.-M. (2016). Biogeography and photosynthetic biomass of Arctic marine pico-eukaroytes during summer of the record sea ice minimum 2012. PLoS One 11:e0148512. doi: 10.1371/journal.pone.0148512
Meyer, K. S., Young, C. M., Sweetman, A. K., Taylor, J., Soltwedel, T., and Bergmann, M. (2016). Rocky islands in a sea of mud: biotic and abiotic factors structuring deep-sea dropstone communities. Mar. Ecol. Prog. Ser. 556, 45–57. doi: 10.3354/meps11822
Michel, C., Legendre, L., Ingram, R. G., Gosselin, M., and Levasseur, M. (1996). Carbon budget of sea-ice algae in spring: evidence of a significant transfer to zooplankton grazers. J. Geophys. Res. Oceans 101, 18345–18360. doi: 10.1029/96jc00045
Mills, M. M., Brown, Z. W., Laney, S. R., Ortega-Retuerta, E., Lowry, K. E., van Dijken, G. L., et al. (2018). Nitrogen limitation of the summer phytoplankton and heterotrophic prokaryote communities in the Chukchi sea. Front. Mar. Sci. 5:362. doi: 10.3389/fmars.2018.00362
Moore, S. E., Stabeno, P. J., Grebmeier, J. M., and Okkonen, S. R. (2018). The Arctic Marine Pulses Model: linking annual oceanographic processes to contiguous ecological domains in the Pacific Arctic. Deep Sea Res. PT II 152, 8–21. doi: 10.1016/j.dsr2.2016.10.011
Morales, S. E., Biswas, A., Herndl, G. J., and Baltar, F. (2019). Global structuring of phylogenetic and functional diversity of pelagic fungi by depth and temperature. Front. Mar. Sci. 6:131. doi: 10.3389/fmars.2019.00131
Mumm, N., Auel, H., Hanssen, H., Hagen, W., Richter, C., and Hirche, H.-J. (1998). Breaking the ice: large-scale distribution of mesozooplankton after a decade of Arctic and transpolar cruises. Polar Biol. 20, 189–197. doi: 10.1007/s003000050295
Nelson, R. J., Ashjian, C. J., Bluhm, B. A., Conlan, K. E., Gradinger, R. R., Grebmeier, J. M., et al. (2014). “Biodiversity and biogeography of the lower trophic taxa of the Pacific Arctic region: sensitivities to climate change,” in The Pacific Arctic Region: Ecosystem Status and Trends in a Rapidly Changing Environment, eds J. M. Grebmeier and W. Maslowski (Dordrecht: Springer), 269–336. doi: 10.1007/978-94-017-8863-2_10
Nicolaus, M., and Katlein, C. (2013). Mapping radiation transfer through sea ice using a remotely operated vehicle (ROV). Cryosphere 7, 763–777. doi: 10.5194/tc-7-763-2013
Nöthig, E. M., and Bodungen, B. V. (1989). Occurrence and vertical flux of faecal pellets of probably protozoan origin in the southeastern Weddell Sea (Antarctica). Mar. Ecol. Prog. Ser. 56, 281–289. doi: 10.3354/meps056281
O’Brien, M. C., Macdonald, R. W., Melling, H., and Iseki, K. (2006). Particle fluxes and geochemistry on the Canadian Beaufort shelf: implications for sediment transport and deposition. Cont. Shelf Res. 26, 41–81. doi: 10.1016/j.csr.2005.09.007
O’Brien, M. C., Melling, H., Pedersen, T. F., and Macdonald, R. W. (2011). The role of eddies and energetic ocean phenomena in the transport of sediment from shelf to basin in the Arctic. J. Geophys. Res. Oceans 116:C08001. doi: 10.1029/2010jc006890
Ohman, M. D., and Runge, J. A. (1994). Sustained fecundity when phytoplankton resources are in short supply: omnivory by Calanus finmarchicus in the Gulf of St. Lawrence. Limnol. Oceanogr. 39, 21–36. doi: 10.4319/lo.1994.39.1.0021
Olli, K., Wassmann, P., Reigstad, M., Ratkova, T. N., Arashkevich, E., Pasternak, A., et al. (2007). The fate of production in the central Arctic Ocean – top–down regulation by zooplankton expatriates? Prog. Oceanogr. 72, 84–113. doi: 10.1016/j.pocean.2006.08.002
Olsen, L. M., Laney, S. R., Duarte, P., Kauko, H. M., Fernández-Méndez, M., Mundy, C. J., et al. (2017). The seeding of ice algal blooms in Arctic pack ice: the multiyear ice seed repository hypothesis. J. Geophys. Res. Biogeo. 122, 1529–1548. doi: 10.1002/2016jg003668
Onda, D. F., Medrinal, E., Thaler, M., Babin, M., Lovejoy, C., and Comeau, A. (2017). Seasonal and interannual changes in ciliate and Dinoflagellate species assemblages in the Arctic Ocean (Amundsen Gulf, Beaufort Sea, Canada). Front. Mar. Sci. 4:16. doi: 10.3389/fmars.2017.00016
Pabi, S., van Dijken, G. L., and Arrigo, K. R. (2008). Primary production in the Arctic Ocean, 1998–2006. J. Geophys. Res. Oceans 113:C08005. doi: 10.1029/2007jc004578
Pfannkuche, O., and Thiel, H. (1987). Meiobenthic stocks and benthic activity on the NE-Svalbard shelf and in the Nansen Basin. Polar Biol. 7, 253–266. doi: 10.1007/bf00443943
Piepenburg, D., Blackburn, T. H., von, D. C. F., Gutt, J., Hall, P. O. J., Hulth, S., et al. (1995). Partitioning of benthic community respiration in the Arctic (northwestern Barents Sea). Mar. Ecol. Prog. Ser. 118, 199–213. doi: 10.3354/meps118199
Pomeroy, L. R. (1997). Primary production in the Arctic Ocean estimated from dissolved oxygen. J. Mar. Sys. 10, 1–8. doi: 10.1016/S0924-7963(96)00059-0
Popova, E. E., Yool, A., Coward, A. C., Aksenov, Y. K., Alderson, S. G., de Cuevas, B. A., et al. (2010). Control of primary production in the Arctic by nutrients and light: insights from a high resolution ocean general circulation model. Biogeosciences 7, 3569–3591. doi: 10.5194/bg-7-3569-2010
Poulsen, L., and Kiørboe, T. (2005). Coprophagy and coprorhexy in the copepods Acartia tonsa and Temora longicornis: clearance rates and feeding behaviour. Mar. Ecol. Prog. Ser. 299, 217–227. doi: 10.3354/meps299217
Poulsen, L. K., Moldrup, M., Berge, T., and Hansen, P. J. (2011). Feeding on copepod fecal pellets: a new trophic role of dinoflagellates as detritivores. Mar. Ecol. Prog. Ser. 441, 65–78. doi: 10.3354/meps09357
Premke, K., Klages, M., and Arntz, W. E. (2006). Aggregations of Arctic deep-sea scavengers at large food falls: temporal distribution, consumption rates and population structure. Mar. Ecol. Prog. Ser. 325, 121–135. doi: 10.3354/meps325121
Purcell, J. E., Hopcroft, R. R., Kosobokova, K. N., and Whitledge, T. E. (2010). Distribution, abundance, and predation effects of epipelagic ctenophores and jellyfish in the western Arctic Ocean. Deep Sea Res. PT II 57, 127–135. doi: 10.1016/j.dsr2.2009.08.011
Randelhoff, A., Fer, I., and Sundfjord, A. (2017). Turbulent upper-ocean mixing affected by meltwater layers during Arctic summer. J. Phys. Oceanogr. 47, 835–853. doi: 10.1175/jpo-d-16-0200.1
Randelhoff, A., and Guthrie, J. D. (2016). Regional patterns in current and future export production in the central Arctic Ocean quantified from nitrate fluxes. Geophys. Res. Lett. 43, 8600–8608. doi: 10.1002/2016gl070252
Rapp, J., Fernández-Méndez, M., Bienhold, C., and Boetius, A. (2018). Effects of ice-algal aggregate export on the connectivity of bacterial communities in the central Arctic Ocean. Front. Microbiol. 9:1035. doi: 10.3389/fmicb.2018.01035
Raskoff, K. A., Hopcroft, R. R., Kosobokova, K. N., Purcell, J. E., and Youngbluth, M. (2010). Jellies under ice: ROV observations from the Arctic 2005 hidden ocean expedition. Deep Sea Res. PT II 57, 111–126. doi: 10.1016/j.dsr2.2009.08.010
Raskoff, K. A., Purcell, J. E., and Hopcroft, R. R. (2005). Gelatinous zooplankton of the Arctic Ocean: in situ observations under the ice. Polar Biol. 28, 207–217. doi: 10.1007/s00300-004-0677-2
Reeves, R. R., Ewins, P. J., Agbayani, S., Heide-Jørgensen, M. P., Kovacs, K. M., Lydersen, C., et al. (2014). Distribution of endemic cetaceans in relation to hydrocarbon development and commercial shipping in a warming Arctic. Mar. Policy 44, 375–389. doi: 10.1016/j.marpol.2013.10.005
Renaud, P. E., Riedel, A., Michel, C., Morata, N., Gosselin, M., Juul-Pedersen, T., et al. (2007). Seasonal variation in benthic community oxygen demand: a response to an ice algal bloom in the Beaufort Sea, Canadian Arctic? J. Mar. Syst. 67, 1–12. doi: 10.1016/j.jmarsys.2006.07.006
Ritzrau, W., Graf, G., Scheltz, A., and Queisser, W. (2001). “Bentho-pelagic coupling and carbon dynamics in the northern north Atlantic,” in The Northern North Atlantic: A Changing Environment, eds P. Schäfer, W. Ritzrau, M. Schlüter, and J. Thiede (Berlin: Springer), 207–224. doi: 10.1007/978-3-642-56876-3_13
Roca-Martí, M., Puigcorbé, V., Rutgers van der Loeff, M. M., Katlein, C., Fernández-Méndez, M., Peeken, I., et al. (2016). Carbon export fluxes and export efficiency in the central Arctic during the record sea-ice minimum in 2012: a joint 234Th/238U and 210Po/210Pb study. J. Geophys. Res. Oceans 121, 5030–5049. doi: 10.1002/2016jc011816
Rybakova, E., Kremenetskaia, A., Vedenin, A., Boetius, A., and Gebruk, A. (2019). Deep-sea megabenthos communities of the Eurasian Central Arctic are influenced by ice-cover and sea-ice algal falls. PLoS One 14:e0211009. doi: 10.1371/journal.pone.0211009
Sakshaug, E. (2004). “Primary and secondary production in the Arctic seas,” in The Organic Carbon Cycle in the Arctic Ocean, eds R. Stein and R. W. Macdonald (Berlin: Springer), 57–81. doi: 10.1007/978-3-642-18912-8_3
Sampei, M., Forest, A., Sasaki, H., Hattori, H., Makabe, R., Fukuchi, M., et al. (2009). Attenuation of the vertical flux of copepod fecal pellets under Arctic sea ice: evidence for an active detrital food web in winter. Polar Biol. 32, 225–232. doi: 10.1007/s00300-008-0523-z
Schewe, I. (2001). Small-sized benthic organisms of the alpha ridge, central Arctic Ocean. Int. Rev. Hydrobiol. 86, 317–335. doi: 10.1002/1522-2632(200106)86:33.3.CO;2-M
Shephard, G. E., Dalen, K., Peldszus, R., Aparício, S., Beumer, L., Birkeland, R., et al. (2016). Assessing the added value of the recent declaration on unregulated fishing for sustainable governance of the central Arctic Ocean. Mar. Policy 66, 50–57. doi: 10.1016/j.marpol.2016.01.013
Sherr, B. F., and Sherr, E. B. (2003). Community respiration/production and bacterial activity in the upper water column of the central Arctic Ocean. Deep Sea Res. PT I 50, 529–542. doi: 10.1016/S0967-0637(03)00030-X
Sherr, E. B., Sherr, B. F., and Fessenden, L. (1997). Heterotrophic protists in the central Arctic Ocean. Deep Sea Res. PT II 44, 1665–1682. doi: 10.1016/S0967-0645(97)00050-7
Sherr, E. B., Sherr, B. F., and Hartz, A. J. (2009). Microzooplankton grazing impact in the Western Arctic Ocean. Deep Sea Res. PT II 56, 1264–1273. doi: 10.1016/j.dsr2.2008.10.036
Sieburth, J. M., Smetacek, V., and Lenz, J. (1978). Pelagic ecosystem structure: heterotrophic compartments of the plankton and their relationship to plankton size fractions. Limnol. Oceanogr. 23, 1256–1263. doi: 10.4319/lo.1978.23.6.1256
Slagstad, D., Wassmann, P. F. J., and Ellingsen, I. (2015). Physical constrains and productivity in the future Arctic Ocean. Front. Mar. Sci. 2:85. doi: 10.3389/fmars.2015.00085
Smith, K. L. (1978). Benthic community respiration in the N.W. Atlantic Ocean: in situ measurements from 40 to 5200 m. Mar. Biol. 47, 337–347. doi: 10.1007/bf00388925
Smith, K. L., and Baldwin, R. J. (1982). Scavenging deep-sea amphipods: effects of food odor on oxygen consumption and a proposed metabolic strategy. Mar. Biol. 68, 287–298. doi: 10.1007/bf00409595
Smith, K. L., Ruhl, H. A., Bett, B. J., Billett, D. S. M., Lampitt, R. S., and Kaufmann, R. S. (2009). Climate, carbon cycling, and deep-ocean ecosystems. Proc. Natl. Acad. Sci. U.S.A. 106, 19211–19218. doi: 10.1073/pnas.0908322106
Smoot, C., and Hopcroft, R. (2017). Depth-stratified community structure of Beaufort Sea slope zooplankton and its relations to water masses. J. Plankton Res. 39, 79–91. doi: 10.1093/plankt/fbw087
Soltwedel, T. (2000). Metazoan meiobenthos along continental margins: a review. Prog. Oceanogr. 46, 59–84. doi: 10.1016/S0079-6611(00)00030-6
Soltwedel, T., Guilini, K., Sauter, E., Schewe, I., and Hasemann, C. (2018). Local effects of large food-falls on nematode diversity at an arctic deep-sea site: results from an in situ experiment at the deep-sea observatory HAUSGARTEN. J. Exp. Mar. Biol. Ecol. 502, 129–141. doi: 10.1016/j.jembe.2017.03.002
Soltwedel, T., Jaeckisch, N., Ritter, N., Hasemann, C., Bergmann, M., and Klages, M. (2009a). Bathymetric patterns of megafaunal assemblages from the arctic deep-sea observatory HAUSGARTEN. Deep Sea Res. PT I 56, 1856–1872. doi: 10.1016/j.dsr.2009.05.012
Soltwedel, T., Mokievsky, V., Schewe, I., and Hasemann, C. (2009b). Yermak Plateau revisited: spatial and temporal patterns of meiofaunal assemblages under permanent ice-coverage. Polar Biol. 32, 1159–1176. doi: 10.1007/s00300-009-0612-7
Soltwedel, T., von Juterzenka, K., Premke, K., and Klages, M. (2003). What a lucky shot! Photographic evidence for a medium-sized natural food-fall at the deep seafloor. Oceanol. Acta 26, 623–628. doi: 10.1016/S0399-1784(03)00060-4
Søreide, J. E., Carroll, M. L., Hop, H., Ambrose, W. G., Hegseth, E. N., and Falk-Petersen, S. (2013). Sympagic-pelagic-benthic coupling in Arctic and Atlantic waters around Svalbard revealed by stable isotopic and fatty acid tracers. Mar. Biol. Res. 9, 831–850. doi: 10.1080/17451000.2013.775457
Stein, D. L., Felley, J. D., and Vecchione, M. (2005). ROV observations of benthic fishes in the Northwind and Canada Basins. Arctic Ocean. Polar Biol. 28, 232–237. doi: 10.1007/s00300-004-0696-z
Stockton, W. L., and DeLaca, T. E. (1982). Food falls in the deep sea: occurrence, quality, and significance. Deep Sea Res. 29, 157–169. doi: 10.1016/0198-0149(82)90106-6
Suydam, R. S., Lowry, L. F., Frost, K. J., O’Corry-Crowe, G. M., and Pikok, D. (2001). Satellite tracking of Eastern Chukchi Sea Beluga whales into the Arctic Ocean. Arctic 54, 237–243.
Svensen, C., Morata, N., and Reigstad, M. (2014). Increased degradation of copepod faecal pellets by co-acting dinoflagellates and Centropages hamatus. Mar. Ecol. Prog. Ser. 516, 61–70. doi: 10.3354/meps10976
Sweetman, A. K., Smith, C. R., Shulse, C. N., Maillot, B., Lindh, M., Church, M. J., et al. (2019). Key role of bacteria in the short-term cycling of carbon at the abyssal seafloor in a low particulate organic carbon flux region of the eastern Pacific Ocean. Limnol. Oceanogr. 64, 694–713. doi: 10.1002/lno.11069
Syvertsen, E. E. (1991). Ice algae in the Barents Sea: types of assemblages, origin, fate and role in the ice-edge phytoplankton bloom. Polar Biol. 10, 277–288. doi: 10.1111/j.1751-8369.1991.tb00653.x
Tang, K. W., and Elliott, D. T. (2014). “Copepod carcasses: occurrence, fate and ecological importance,” in Copepods: Diversity, Habitat and Behavior, ed. L. Seuront (Hauppauge, NY: Nova Science Publishers), 255–278.
Taylor, J., Krumpen, T., Soltwedel, T., Gutt, J., and Bergmann, M. (2016). Regional- and local-scale variations in benthic megafaunal composition at the Arctic deep-sea observatory HAUSGARTEN. Deep Sea Res. PT I 108, 58–72. doi: 10.1016/j.dsr.2015.12.009
Thibault, D., Head, E. J. H., and Wheeler, P. A. (1999). Mesozooplankton in the Arctic Ocean in summer. Deep Sea Res. PT I 46, 1391–1415. doi: 10.1016/S0967-0637(99)00009-6
Tremblay, J. -É, Anderson, L. G., Matrai, P., Coupel, P., Bélanger, S., Michel, C., et al. (2015). Global and regional drivers of nutrient supply, primary production and CO2 drawdown in the changing Arctic Ocean. Prog. Oceanogr. 139, 171–196. doi: 10.1016/j.pocean.2015.08.009
Turner, J. T. (2002). Zooplankton fecal pellets, marine snow and sinking phytoplankton blooms. Aquat. Microb. Ecol. 27, 57–102. doi: 10.3354/ame027057
Turner, J. T. (2015). Zooplankton fecal pellets, marine snow, phytodetritus and the ocean’s biological pump. Prog. Oceanogr. 130, 205–248. doi: 10.1016/j.pocean.2014.08.005
Vader, A., Marquardt, M., Meshram, A. R., and Gabrielsen, T. M. (2014). Key Arctic phototrophs are widespread in the polar night. Polar Biol. 38, 13–21. doi: 10.1007/s00300-014-1570-2
Vanreusel, A., Clough, L., Jacobsen, K., Ambrose, W., Jutamas, J., Ryheul, V., et al. (2000). Meiobenthos of the central Arctic Ocean with special emphasis on the nematode community structure. Deep Sea Res. PT I 47, 1855–1879. doi: 10.1016/S0967-0637(00)00007-8
Varela, D. E., Crawford, D. W., Wrohan, I. A., Wyatt, S. N., and Carmack, E. C. (2013). Pelagic primary productivity and upper ocean nutrient dynamics across Subarctic and Arctic Seas. J. Geophys. Res. Oceans 118, 7132–7152. doi: 10.1002/2013jc009211
Vedenin, A., Gusky, M., Gebruk, A., Kremenetskaia, A., Rybakova, E., and Boetius, A. (2018). Spatial distribution of benthic macrofauna in the Central Arctic Ocean. PLoS One 13:e0200121. doi: 10.1371/journal.pone.0200121
Vedenin, A., Mokievsky, V., Soltwedel, T., and Budaeva, N. (2019). The temporal variability of the macrofauna at the deep-sea observatory HAUSGARTEN (Fram Strait, Arctic Ocean). Polar Biol. 42, 527–540. doi: 10.1007/s00300-018-02442-8
Verity, P. G., Wassmann, P., Frischer, M., Howard-Jones, M., and Allen, A. (2002). Grazing of phytoplankton by microzooplankton in the Barents Sea during early summer. J. Mar. Syst. 38, 109–123. doi: 10.1016/s0924-7963(02)00172-0
Visser, A. W., Grønning, J., and Jónasdóttir, S. H. (2017). Calanus hyperboreus and the lipid pump. Limnol. Oceanogr. 62, 1155–1165. doi: 10.1002/lno.10492
Wang, C., Huang, J., Xiang, P., Wang, Y., Xu, Z., Guo, D., et al. (2014). Hydromedusae from the Arctic in 2010 during the 4th Chinese National Arctic Research Expedition (CHINARE 4). Acta Oceanol. Sin. 33, 95–102. doi: 10.1007/s13131-014-0494-6
Wang, Y., Kang, J.-H., Xiang, P., Ye, Y.-Y., Lin, H.-S., and Lin, M. (2019). Phytoplankton communities and size-fractioned chlorophyll a in newly opened summer waters of the central Arctic Ocean. Mar. Ecol. Prog. Ser. 622, 67–82. doi: 10.3354/meps13001
Wassmann, P. (2011). Arctic marine ecosystems in an era of rapid climate change. Prog. Oceanogr. 90, 1–17. doi: 10.1016/j.pocean.2011.02.002
Wassmann, P., Kosobokova, K., Slagstad, D., Drinkwater, K., Hopcroft, R., Moore, S., et al. (2015). The contiguous domains of Arctic Ocean advection: trails of life and death. Prog. Oceanogr. 139, 42–65. doi: 10.1016/j.pocean.2015.06.011
Wassmann, P., Peinert, R., and Smetacek, V. (1991). Patterns of production and sedimentation in the boreal and polar Northeast Atlantic. Polar Res. 10, 209–228. doi: 10.3402/polar.v10i1.6740
Wassmann, P., and Reigstad, M. (2011). Future Arctic Ocean seasonal ice zones and implications for pelagic-benthic coupling. Oceanography 24, 220–231. doi: 10.6570/oceanog.2011.74
Wassmann, P., Slagstad, D., and Ellingsen, I. (2019). Advection of Mesozooplankton into the Northern svalbard shelf region. Front. Mar. Sci. 6:458. doi: 10.3389/fmars.2019.00458
Watanabe, E., Onodera, J., Harada, N., Honda, M. C., Kimoto, K., Kikuchi, T., et al. (2014). Enhanced role of eddies in the Arctic marine biological pump. Nat. Commun. 5:3950. doi: 10.1038/ncomms4950
Wegner, C., Wittbrodt, K., Hölemann, J. A., Janout, M. A., Krumpen, T., Selyuzhenok, V., et al. (2017). Sediment entrainment into sea ice and transport in the transpolar drift: a case study from the Laptev Sea in winter 2011/2012. Cont. Shelf Res. 141, 1–10. doi: 10.1016/j.csr.2017.04.010
Wei, C.-L., Rowe, G. T., Escobar-Briones, E., Boetius, A., Soltwedel, T., Caley, M. J., et al. (2010). Global patterns and predictions of seafloor biomass using random forests. PLoS One 5:e15323. doi: 10.1371/journal.pone.0015323
Werner, I. (1997). Grazing of Arctic under-ice amphipods on sea-ice algae. Mar. Ecol. Prog. Ser. 160, 93–99. doi: 10.3354/meps160093
Werner, I. (2000). Faecal pellet production by Arctic under-ice amphipods – transfer of organic matter through the ice/water interface. Hydrobiologia 426, 89–96. doi: 10.1023/a:1003984327103
Wheeler, P. A., Gosselin, M., Sherr, E., Thibaultc, D., Kirchman, D. L., Benner, R., et al. (1996). Active cycling of organic carbon in the central Arctic Ocean. Nature 380:697. doi: 10.1038/380697a0
Winder, M., Bouquet, J.-M., Rafael Bermúdez, J., Berger, S. A., Hansen, T., Brandes, J., et al. (2017). Increased appendicularian zooplankton alter carbon cycling under warmer more acidified ocean conditions. Limnol. Oceanogr. 62, 1541–1551. doi: 10.1002/lno.10516
Wlodarska-Kowalczuk, M., Kendall, M. A., Marcin Weslawski, J., Klages, M., and Soltwedel, T. (2004). Depth gradients of benthic standing stock and diversity on the continental margin at a high-latitude ice-free site (off Spitsbergen, 79°N). Deep Sea Res. PT I 51, 1903–1914. doi: 10.1016/j.dsr.2004.07.013
Wollenburg, J. E., Katlein, C., Nehrke, G., Nöthig, E. M., Matthiessen, J., Wolf- Gladrow, D. A., et al. (2018). Ballasting by cryogenic gypsum enhances carbon export in a Phaeocystis under-ice bloom. Sci. Rep. 8:7703. doi: 10.1038/s41598-018-26016-0
Wollenburg, J. E., and Mackensen, A. (1998). Living benthic foraminifers from the central Arctic Ocean: faunal composition, standing stock and diversity. Mar. Micropaleontol. 34, 153–185. doi: 10.1016/S0377-8398(98)00007-3
Yang, E. J., Ha, H. K., and Kang, S.-H. (2015). Microzooplankton community structure and grazing impact on major phytoplankton in the Chukchi sea and the western Canada basin, Arctic ocean. Deep Sea Res. PT II 120, 91–102. doi: 10.1016/j.dsr2.2014.05.020
Zernova, V., Nöthig, E.-M., and Shevchenko, V. (2000). Vertical microalga flux in the northern Laptev Sea (From the data collected by the yearlong sediment trap). Oceanology 40, 801–808.
Keywords: Arctic Ocean basins, carbon budget, carbon demand, deep-sea benthos, mismatch, primary production, pelagic consumption, vertical carbon export
Citation: Wiedmann I, Ershova E, Bluhm BA, Nöthig E-M, Gradinger RR, Kosobokova K and Boetius A (2020) What Feeds the Benthos in the Arctic Basins? Assembling a Carbon Budget for the Deep Arctic Ocean. Front. Mar. Sci. 7:224. doi: 10.3389/fmars.2020.00224
Received: 13 December 2019; Accepted: 23 March 2020;
Published: 16 April 2020.
Edited by:
Jacob Carstensen, Aarhus University, DenmarkReviewed by:
David L. Kirchman, University of Delaware, United StatesNathan McTigue, University of Texas at Austin, United States
Copyright © 2020 Wiedmann, Ershova, Bluhm, Nöthig, Gradinger, Kosobokova and Boetius. This is an open-access article distributed under the terms of the Creative Commons Attribution License (CC BY). The use, distribution or reproduction in other forums is permitted, provided the original author(s) and the copyright owner(s) are credited and that the original publication in this journal is cited, in accordance with accepted academic practice. No use, distribution or reproduction is permitted which does not comply with these terms.
*Correspondence: Ingrid Wiedmann, aW5ncmlkLndpZWRtYW5uQHVpdC5ubw==