- 1Department of Environmental and Earth Sciences (DISAT), University of Milano-Bicocca, Milan, Italy
- 2Computational Bioscience Research Center and Red Sea Research Center, King Abdullah University of Science and Technology, Thuwal, Saudi Arabia
- 3Red Sea Research Center, King Abdullah University of Science and Technology, Thuwal, Saudi Arabia
Although millions of tons of plastics end up in oceans each year, floating plastics account for only about 1% of all plastic inputs in the ocean. Particularly, microplastics below 1 mm in length, are missing in surface waters due to removal processes like ingestion by marine animals, biofouling, and sinking. Here, we studied how a species of mushroom corals (Danafungia scruposa), common in the Maldives, contributed to the removal of microplastics from the water suspension through active (ingestion) and passive (adhesion to the surface) mechanisms. We evaluated if removal rates were affected by the presence of the coral natural prey (i.e., Artemia salina) and by biofouling on the surface of the microplastic. We found that the coral quickly interacts both actively and passively with microplastics and that the probability for the coral to ingest and retain microplastics was higher when the surface of the microplastic was biofouled. We also found that passive adhesion of microplastics was the primary mechanism through which corals sequester microplastics from the water column.
Introduction
In the ocean, plastic debris accounts for more than 90% of marine litter (Galgani et al., 2015) and fragmentation of this debris into smaller particles forms microplastics (<5 mm in length, Moore, 2008; Barnes et al., 2009). Microplastics can also directly enter the marine environment in the form of microbeads or pre-production pellets (Van Cauwenberghe et al., 2013). Although millions of tons of plastic are estimated to enter the ocean every year, only about 1% of that amount remains floating in the surface waters (Jambeck et al., 2015; Law, 2017). Especially, microplastics below 1 mm are found in much lower concentrations than expected in near-surface waters (Cozar et al., 2014; Eriksen et al., 2014). Fragmentation of plastics, ingestion by marine biota, deposition on the shore, biofouling, and consequent sinking are possible mechanisms leading to the removal of plastics from surface waters (Cozar et al., 2014). Among these mechanisms, ingestion of microplastics, which has been observed in a wide range of organisms (Murray and Cowie, 2011; Baulch and Perry, 2014; Hämer et al., 2014; Baalkhuyur et al., 2018; Arossa et al., 2019) is of particular concern as it introduces risks into the marine food web, including for humans (Carbery et al., 2018). Ingestion of microplastics has been shown to have negative effects on organisms. For example, the presence of microplastics in cultures reduced the algal feeding rates of copepods, with a consequent reduction in ingested carbon biomass over time (Cole et al., 2013). Furthermore, plastics can act as both sinks and sources for chemical pollutants (Teuten et al., 2009) and may provide substrata for the development of non-indigenous and complex microbial communities (Harrison et al., 2011).
Corals support biodiversity in the oceans as a source of both food and shelter for a wide range of marine life. Given the importance of corals in the marine system, their exposure to microplastic pollution threatens the health of the whole coral reef ecosystem. Indeed, corals have been reported to be able to capture and ingest microplastics (Hall et al., 2015). When corals contact alien particles, such as microplastics, they have been found to respond in a variety of ways, depending on the species. Such responses include deploying cleaning mechanisms, retaining particles through overgrowth, and egesting ingested plastic particles (Stafford-Smith and Ormond, 1992; Reichert et al., 2018). Previous studies on the ingestion of microplastics by corals have, altogether, considered only a few species of corals (Hall et al., 2015; Allen et al., 2017; Reichert et al., 2018; Martin et al., 2019; Rotjan et al., 2019) and have employed experimental conditions that depart from the natural conditions of the marine environment. For instance, the corals previously tested in microplastics studies were fed industrially produced virgin microplastics rather than environmental microplastics and no alternative food sources were provided during the experiments (Hall et al., 2015; Allen et al., 2017; Reichert et al., 2018). Moreover, although corals were often observed to trap microplastics on their surfaces during microplastic feeding experiments in the laboratory (Allen et al., 2017; Reichert et al., 2018), adhesion has only just recently been identified as an effective microplastic sink in three coral species in the Red Sea (Acropora hemprichii, Goniastrea retiformis, and Pocillopora verrucosa (Martin et al., 2019). That study showed that adhesion of microplastics to coral is 40 times more effective in removing microplastics from the water column than is ingestion of microplastics.
The coral reefs of the Maldives constitute one of the largest reef systems on the planet (8920 km2) (Spalding et al., 2001). These reefs are affected by a number of natural and anthropogenic threats, such as bleaching, algal overgrowth, and coral and invertebrate disease outbreaks (Montano et al., 2012; Saponari et al., 2018), along with pollution and physical impacts derived from human activities, such as coral mining, pollution, land reclamation, tourism and fishing (Jaleel, 2013; Fallati et al., 2017). The amount of microplastics polluting these reefs is staggering: 4.2 ± 1.2 microplastic L–1 were measured in the surface waters of the Indian Ocean (Barrows et al., 2018), whereas 0.32 ± 0.15 particles m–3 and 22.8 ± 10.5 particles m–2 were, respectively, counted in the surface waters and in the beach sediments at Magoodhoo island in the Faafu Atoll in the Maldives (Saliu et al., 2018).
Mushroom corals (Scleractinia, Fungiidae) are attached to a hard substratum as juveniles but occur mainly as free-living organisms as adults. After reaching maturity, these corals may migrate onto the soft substratum or down the reef slope (Hoeksema, 1989; Hoeksema and Moka, 1989; Hoeksema and Yeemin, 2011). Mushroom corals are common on reef flats and shallow slopes but are rare in deeper waters (Goffredo and Chadwick-Furman, 2000). Some species of fungiids can also move actively toward light (Yamashiro and Nishira, 1995) and are able to colonize, as adults, the sandy substratum, such as that in reef lagoons (Goffredo and Chadwick-Furman, 2000), unlike other coral species. Among mushroom corals, Danafungia scruposa is a solitary species with a large polyp (3–38 cm in diameter) commonly distributed across inner reefs and lagoons (Hoeksema, 1989; Hoeksema and Moka, 1989). It is one of the few coral species still thriving near Magoodhoo Island, Maldives, where corals were heavily impacted by the 2016 global bleaching event (Perry and Morgan, 2017). Its free-living nature, its distribution and its size distinguish D. scruposa from the other coral species used in studies of marine microplastics. In this study, we measured both active (ingestion) and passive (adhesion) removal of microplastics by D. scruposa. To go beyond the limitations of the laboratory conditions of earlier studies, we then evaluated if this species’ microplastic removal rates were affected by the presence of the coral’s natural prey (e.g., Artemia salina) and by biofouling on the surface of the microplastic.
Materials and Methods
Specimen Sampling and Acclimation
We sampled and exposed to microplastics one species of mushroom coral, D. scruposa (Scleractinia, Fungiidae), easily identifiable due to its serrated septa (Figure 1). Individuals of D. scruposa (>9 cm in diameter) were collected from a reef close to the inhabited island of Magoodhoo, Faafu Atoll, Republic of Maldives (N 3° 05′ 24.3′′ E 72° 58′ 04.5′′) (Supplementary Figure S1), between 5 m and 15 m below the surface. The sampling site, called “Coral Garden,” is located in the inner part of the southeast portion of the atoll, about 140 km south of Malé, the capital city of the Maldives. The site exhibits the features of a typical low-energy reef with a gentle slope.
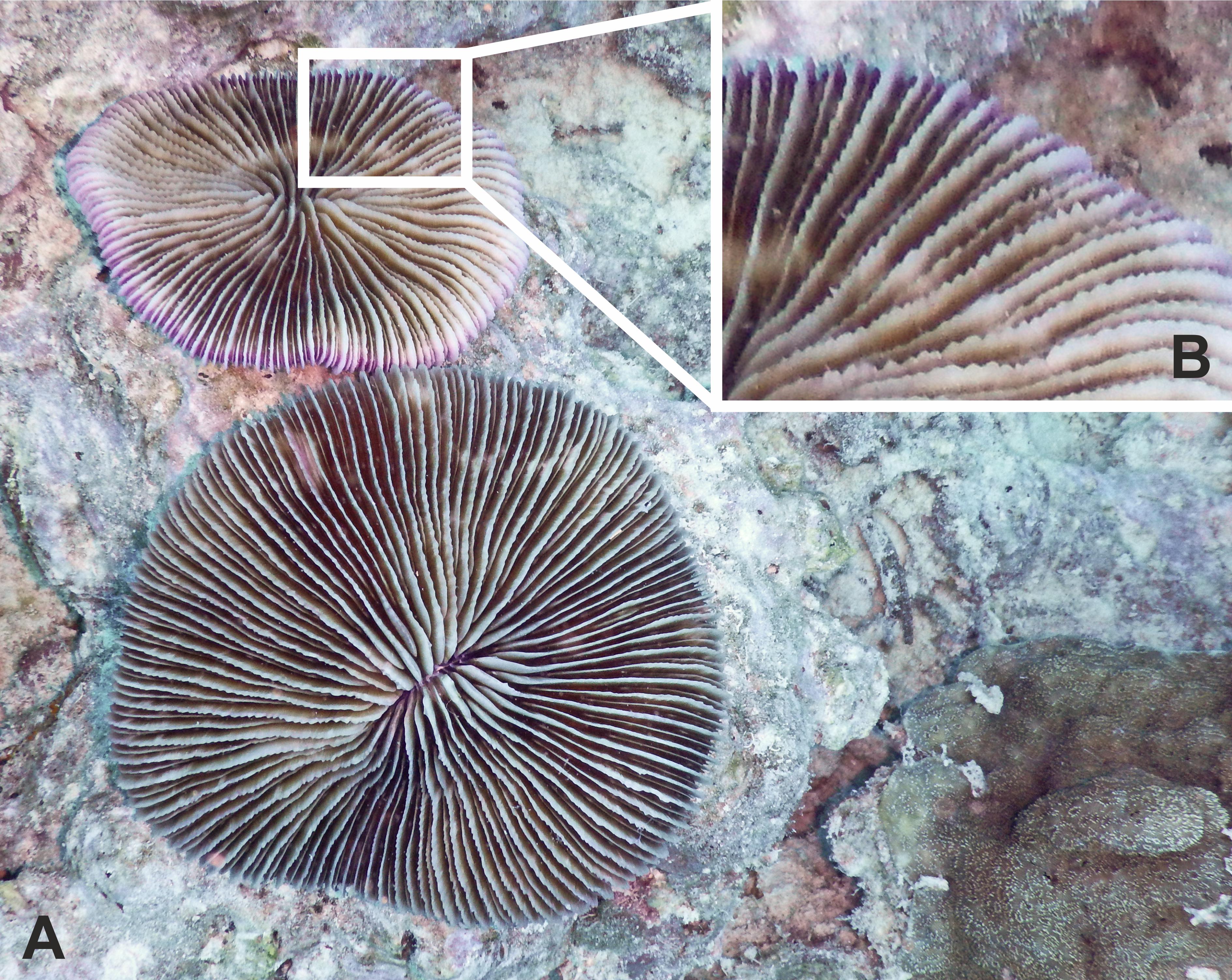
Figure 1. (A) Two samples of Danafungia scruposa (Scleractinia, Fungiidae), each a solitary coral composed of a single polyp. (B) Recognizable serrated septa.
Collected corals were transported to the laboratory inside tubs filled with seawater and oxygenated with air pumps.
Subsequently, the corals were placed inside crates, covered with a mesh to reduce sunlight exposure, and left for 24 h in the lagoon close to the laboratory for acclimation to the experimental conditions (∼31°C).
Experimental Set-Up
After acclimation, 28 healthy individuals were individually transferred to transparent and reinforced plastic bags filled with 1.5 L of filtered seawater (200-μm filter). We conducted two experiments, each involving 14 corals, run from 3 pm to 11 pm on two consecutive days (4 and 5 April 2018), during which time the corals were exposed to 4 h of light and 4 h of darkness.
In the first experiment, the corals were subjected to two treatments. In the first treatment, seven corals were exposed to fluorescent virgin microplastic beads (“Plastic treatment”) and in the second treatment, seven individuals were exposed to a mix of the same microplastics and Artemia salina, representing the coral natural prey (“Plastic + Artemia salina treatment”), to verify if the rate of plastic ingestion was affected during natural feeding on A. salina. Details on the size and number of microplastics are reported below (“Microplastic preparation”) and in Supplementary Table S1.
In the second experiment, the corals were subjected to three treatments in absence of the natural prey. In the first treatment, four individuals were exposed to fluorescent virgin microplastic beads (“Virgin treatment”); in the second treatment, four other individuals were exposed to biofouled microplastics (“Biofouled treatment”) and in the third, six other individuals were exposed to a mix of virgin and biofouled microplastics in equal number (“Mixed treatment,” Supplementary Table S2). Details on size and number of microplastics used for this experiment are reported below (“Microplastic preparation”) and in Supplementary Table S2. The aim of this second experiment was to evaluate if the presence of biofouling on microplastic particles enhances their ingestion.
In both experiments, all replicates were put back inside the crates and moved from the lagoon to the nearby reef (N 03° 04′ 55.17′′ E 72° 57′ 54.88′′), where we observed that the stronger current favored the mixing of plastic particles in the suspension inside the plastic bags. In fact, each crate contained only seven plastic bags, not fixed inside the crate, allowing freedom of movement. The crates were covered with a mesh to reduce sunlight and placed at 2.5 m depth, where the temperature reached 31°C and the salinity was 34.9 ppm.
Microplastic Preparation
In the first experiment, we used fluorescent polyethylene microbeads from Cospheric LLC (virgin microplastics). To each of the 14 plastic bags, we added microplastic beads from three size classes (212–355 μm, 600–710 μm, and 850–1000 μm), 989 ± 2 beads per size class for a total of 2996 ± 5 beads (0.57 ± 0.0001 g per plastic bag; Supplementary Table S1).
In the treatment in which virgin microplastics were mixed with A. salina (7 replicates), we cultured A. salina cysts 1 day prior to the start of the experiment. To estimate the density of A. salina in the culture batch, we counted A. salina in three aliquots of 1 ml drawn from the previously homogenized batch, under a stereoscope using a Sedgewick rafter counting chamber. Then, we added to each plastic bag a volume of A. salina culture of about 10.5 ml, corresponding to 2989 ± 155 A. salina, similar to the microplastic concentration in each bag.
In the second experiment, we used virgin microplastics as described above and biofouled ones. The biofouled plastic came from the Great Pacific Garbage Patch; it was composed of hard fragments made mainly of polyethylene and polypropylene exhibiting an accumulation of microorganisms, algae, and small animals on their surfaces (Supplementary Figure S2). We further shredded the plastic fragments using a mortar and pestle and then divided them in three size classes of microplastics (200–500 μm, 500–800 μm, and 800–1000 μm) by using sieves with different mesh sizes. Stereomicroscopic pictures of the microplastics obtained after the fragmentation step and acquired with a Leica S8AP0 indicated the continued presence of biological material and aging on the surfaces of the microplastic fragments (Supplementary Figure S3). To ensure that the material on the microplastics was biological in nature, we extracted and quantified DNA from the three size classes. We did the same on the virgin plastic beads to confirm that no biological contamination had occurred on them. Total DNA extractions were performed using the MOBIO Powersoil commercial extraction kit (MOBIO Laboratories, INC., Carlsbad, United States). From each size class and type of plastic (biofouled or virgin), the DNA was extracted starting from a total of 0.25 ± 0.01 g of dry microplastic (stored at room temperature) following the manufacturers’ instructions. Extracted DNA was resuspended in a final volume of 100 μL and stored at −20°C. The amount of DNA obtained was quantified with a QubitTM dsDNA HS Assay Kit (Thermo Fisher Scientific) following the manufacturer’s instructions. The DNA concentration of each sample was determined and expressed as ng per gram of microplastics (Supplementary Table S2). The extraction quality was also assessed by measuring absorbance at 260/280 nm and 260/230 nm wavelengths using a NanoDrop spectrometer (Supplementary Table S3). The DNA from the virgin microplastic was under the detection limit of the high sensitivity kit (<0.01 ng/μl).
The second experiment consisted of three treatments: the “Virgin treatment,” the “Biofouled treatment,” and the “Mixed treatment” in which the corals were exposed to microplastics in absence of their natural prey. To each of four bags assigned to the first treatment (Virgin treatment), we added 2997 ± 6 fluorescent beads (0.57 ± 0.0001 g) from the three size classes (212–355 μm, 600–710 μm, and 850–1000 μm). To four other bags assigned to the second treatment (Biofouled treatment), we added 3005 ± 35 fragments (0.55 ± 0.0001 g) of biofouled plastic from the three previously separated size classes. To the last six bags, we added 1480 ± 11 (0.29 ± 0.0001 g) of virgin plastic and 1506 ± 14 (0.29 ± 0.0001 g) of biofouled plastic (Supplementary Table S2).
Measurements
At the end of both experiments, all D. scruposa individuals were carefully collected from the plastic bags. While extracting the coral from the water, we masked it with a hand to avoid contact between the coral and the microplastic floating on the surface of the water. The microplastics that attached to the hand were then rinsed back into the bag such that they could be counted in the further step as plastic that was not retained during ingestion nor that adhered to the coral surface. The corals were then rinsed thoroughly to remove the microplastics that had adhered to their surfaces, which were saved for further counting. The corals treated with fluorescent beads were further checked under UV light to verify that the whole surface had been cleared of plastic. The corals treated with biofouled plastic were further checked under a stereoscope to ensure the same. The water from each plastic bag was also collected and the plastic bag was washed thoroughly to ensure that all microplastics were saved for further counting. A. salina left in seven of the bags in the first experiment and attached to the corresponding coral were also counted.
Subsequently, plastic beads from the coral surfaces and the plastic bags were counted and measured under a Discovery V.20 Carl Zeiss stereoscope, using UV light for fluorescent beads and halogen light for biofouled fragments, and characterized by size using the Axion Vision 4.8.2 software (Supplementary Data S1). Considering the high abundance of microplastics retrieved from the plastic bag, washes from the bags were first concentrated to a 200-mL solution and then microplastics contained in a 5 mL subsample of the previously homogenized solution were counted.
Finally, to count the microplastic particles ingested by the corals, each individual was decalcified in 5% formic acid overnight until saturation of the solution (adapted from Hall et al., 2015) and then tissue digestion was obtained by soaking the decalcified coral in 8.25% sodium hypochlorite for 24 h (Allen et al., 2017). After the digestion ended, we washed the coral skeletons to remove any tissue still attached. This and the digestion solution were filtered using a 25-μm nylon mesh and the microplastic particles on the filter were counted and measured with the stereoscope, under UV light for fluorescent beads and halogen light for biofouled fragments (Supplementary Data S1). Before digestion, each coral was placed on a rotating platform to record 360° videos, later used to calculate their surface areas by 3D modeling using the Autodesk ReCap 5.0 software.
To determine the recovery rates of plastics, we repeated the previously described procedure on three mushroom corals after spiking them with a known number of microplastic particles. Specifically, each coral was spiked with 30 pieces of plastic: five pieces of each type (virgin and biofouled) and each size (as those used during the experiment). We then decalcified each coral in formic acid and digested its tissue in sodium hypochlorite. After the digestion, we filtered the solution and counted the number of plastics particles we could recover. Recovery rates were 100% for all sizes of virgin plastic and for the larger biofouled plastics (800–1000 μm), while they were, respectively, 86.6 ± 6.6% and 53.3 ± 6.6% for medium sized (500–800 μm) and smaller (200–500 μm) biofouled plastics. To account for possible underestimation, we corrected the values of ingested biofouled plastics accordingly by multiplying by a factor of 1.15 the number of medium-sized plastic particles and by 1.87 the number of smaller-sized plastic particles. We ran the following statistical analyses with both uncorrected and corrected values for comparison.
Calculation and Statistical Analyses
For both experiments, we calculated the removal rate of microplastics by ingestion or adhesion as the number of microplastic particles ingested and then retained or the number of microplastic particles adhered per individual per hour. Differences in ingestion or adhesion between treatments were tested with a Wilcoxon rank sum test. We characterized the contribution of each plastic removal mechanism by calculating the relative proportion (mean ± SE) of plastic removed by ingestion and removed by adhesion.
Plastic retrieved after washing the bag was considered as plastic left in the suspension and therefore not removed from the water by the coral. Indeed, given the presence of strong currents at the field station where the experiment was conducted, plastic particles were well mixed inside the bag and available to the coral during the experiment, except for some plastic pieces that became permanently stuck in the closing strip of the plastic bag. To account for, and later exclude from calculations, the plastic stuck in the closing strips of the bags, we conducted an experiment in the laboratory that also allowed us to verify that the mixing of the plastics occurred during the 8 h of the experiment. We filled three plastic bags with 1.5 L seawater and 2891 ± 8 plastic pieces (similar to the plastic concentration used in the experiments), placed them in a box and gently agitated them on an orbital shaker for 8 h. Finally, we stopped the mixing and we cut the strip out of the bag, opened it and counted the plastic pieces. We determined that 82 ± 3 beads were stuck in the strip, corresponding to 2.8 ± 0.1% of the plastic initially added. We excluded this portion of the plastic to ultimately compile a budget of plastic distribution in the presence of D. scruposa by calculating the relative proportion of plastic left in the plastic bag and removed for either ingestion and retention or adhesion.
To determine the number of A. salina ingested during the experiment, we subtracted the number of A. salina left in each plastic bag and adhered to the coral surfaces to the number of A. salina added at the beginning of the first experiment. Differently from the plastic, A. salina was not observed to stick to the closing strips of the plastic bags.
To determine if there were a preference for the size of microplastic either ingested or adhered, we plotted the ingestion or adhesion removal rates against the mid-value of each size class bin and built a linear model. The preference was considered significant if the p-value of the linear model was less than 0.05. Differences in the preference for size classes between treatments were tested using Analysis of Covariance (ANCOVA).
All values are reported as mean ± SE and the results were considered statistically significant if the p-value was less than 0.05. All statistical analyses were performed by using the JMP Software from SAS.
Results
Plastic Ingestion
During the first experiment, D. scruposa ingested its natural prey (A. salina) at high rates (311.8 ± 13.9 number of A. salina individual–1 hour–1, 83.1 ± 3.7% of the A. salina added at the beginning of the experiment, N = 7). However, the corals ingested and retained only 0.12 ± 0.04 plastic beads individual–1 hour–1 (0.05 ± 0.02% of plastic initially added, N = 14) independently if they were concurrently exposed to A. salina or if they were fed only microplastics (Figure 2A and Supplementary Table S4). Plastic ingestion by D. scruposa was dependent on size, with the corals preferring small-sized virgin particles (215–355 μm, Figure 2C and Supplementary Table S5).
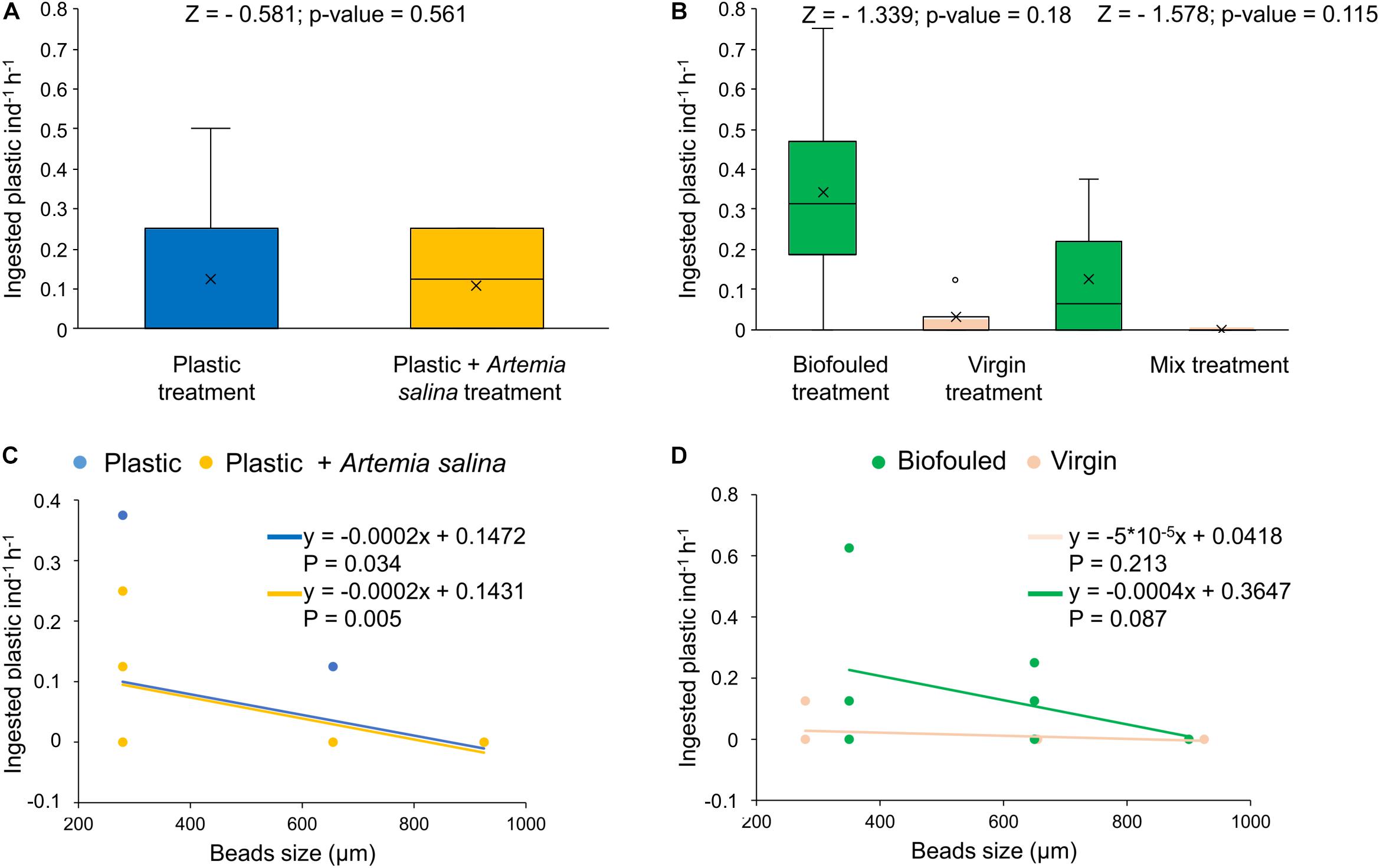
Figure 2. Plastic removal by ingestion and distribution of ingested plastic in size classes (uncorrected values). (A) Ingested plastic (number of microplastic beads individual– 1hour– 1) in corals exposed to two treatments: corals treated with plastic (“Plastic,” in blue, N = 7) and treated with plastic mixed with Artemia salina (“Plastic + A. salina,” in yellow, N = 7). (B) Ingested plastic (number of microplastics individual– 1hour– 1) in corals exposed to three treatments: corals treated with biofouled plastic only (“Biofouled,” in green, N = 4), with virgin plastic only (“Virgin,” in pink, N = 4) and biofouled plastic (in green), and virgin plastic (in pink) mixed (“Mixed,” N = 6). All estimates have been compared with Wilcoxon tests for which the p-value and Z value are reported in the figures. (C) Distribution of ingested plastic in size classes in the Plastic treatment (blue) and in the Plastic + A. salina treatment (yellow). (D) Distribution of ingested plastic in size classes in the Virgin treatment (pink) and in the Biofouled treatment (green). In plots (C,D), size classes are reported as the mean value of the size class bin. Lines represent the linear fit of each set of data of the corresponding color. Equations and p-values of each linear model are reported in figure.
During the second experiment, D. scruposa ingested and retained plastic at rates ranging from 0.16 ± 0.06 (N = 14) to 0.25 ± 0.1 (N = 14) plastic beads individual–1 hour–1, using uncorrected (Figure 2B and Supplementary Table S4) and corrected (Supplementary Figure S4 and Supplementary Table S4) values for the ingested biofouled plastic (see recovery test in Materials and Methods), respectively. The ingested plastic was only a 0.06 ± 0.02% (N = 14) of the plastic initially added. Ingestion rates were independent of the type of plastic (virgin or biofouled) both if corals were exposed to virgin and biofouled plastics separately or to a mixture of these two types (Figure 2B, Supplementary Figure S4A, and Supplementary Table S4). However, the higher interquartile range (IQR) for biofouled plastic compared to the IQR for virgin plastic (when the two types of plastics were given separately or mixed; Figure 2B, Supplementary Figure S4A, and Supplementary Table S4) indicates that the likelihood of ingesting and retaining more plastic pieces was higher when D. scruposa were exposed to biofouled fragments compared to when they were exposed to virgin plastic. Plastic ingestion was not dependent on size when the corals were exposed to biofouled microplastics (Figure 2D, Supplementary Figure S4B, and Supplementary Table S5).
Plastic Adhesion
In the first experiment, plastic beads were removed from the suspension by adhesion to mushroom coral surface at rates of 4.8 ± 0.7 of plastic beads individual–1 hour–1 (1.8 ± 0.3% of plastic added, N = 14, Supplementary Data S1), independently of whether they were exposed to plastic alone or concurrently with A. salina (Figure 3A). After the first experiment, only 0.2 ± 0.09% (N = 7) of A. salina were passively removed by adhesion to the coral surfaces.
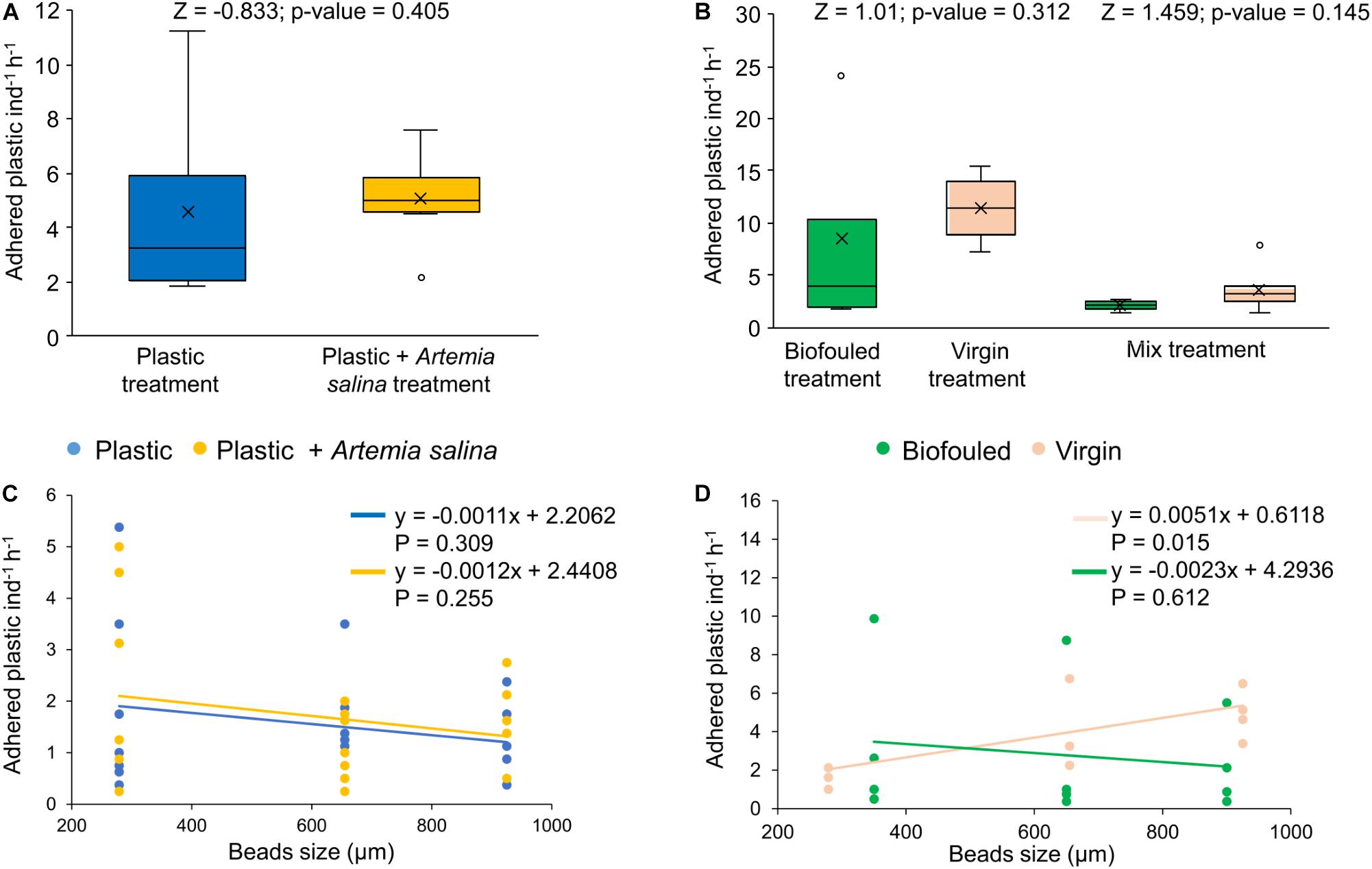
Figure 3. Plastic removal by adhesion and distribution of adhered plastic in size classes. (A) Adhered plastic (number of microplastic beads individual– 1hour– 1) in corals exposed to two treatments: corals treated with plastic (“Plastic,” in blue, N = 7) and treated with plastic mixed with Artemia salina (“Plastic + Artemia salina,” in yellow, N = 7). (B) Adhered plastic (number of microplastics individual– 1hour– 1) in corals exposed to three treatments: corals treated with biofouled plastic only (“Biofouled,” in green, N = 4), with virgin plastic only (“Virgin,” in pink, N = 4) and biofouled plastic (in green), and virgin plastic (in pink) mixed (“Mixed,” N = 6). All estimates have been compared with Wilcoxon tests for which the p-value and Z value are reported in the figures. (C) Distribution of adhered plastic in size classes in the Plastic treatment (blue) and in the Plastic + A. salina treatment (yellow). (D) Distribution of adhered plastic in size classes in the Virgin treatment (pink) and in the Biofouled treatment (green). In plots (C,D), size classes are reported as the mean value of the size class bin. Lines represent the linear fit of each set of data of the corresponding color. Equations and p-values of each linear model are reported in figure.
In the second experiment, 8.1 ± 1.6 of plastic beads individual–1 hour–1 (N = 14) were removed by adhesion, independently of whether the corals were treated with virgin or biofouled plastic or a mix of the two (Figure 3B). Adhered plastic accounted for 3.0 ± 0.6% of the plastic added at the beginning of the experiment (Supplementary Data S1).
In both experiments, adhesion of plastic to the coral surface was not dependent on size (Figures 3C,D and Supplementary Table S5).
Discussion
Our results show that D. scruposa removes microplastics from the water suspension through both passive (adhesion to the coral surface) and active (ingestion) mechanisms. Adhesion was the dominant mechanism responsible for removing microplastics from the water column. Indeed, of all the plastic removed by the coral, 97.7 ± 0.7% of plastic pieces individial–1 hour–1 was removed by adhesion, whereas ingestion by the corals removed 40 times less plastic, at a rate of only 2.3 ± 0.7% plastic beads removed individual–1 hour–1 (N = 28). The higher efficiency of the passive mechanism in removing microplastics from the water suspension compared to ingestion supports the finding that adhesion is an efficient sink for microplastics in the marine environment, as previously reported for three species of corals from the Red Sea (Martin et al., 2019). The confirmation of this finding despite the difference in growth form, polyp size and distribution of the studied species, further strengthens its universality.
Adhesion, the dominant process responsible for the loss of microplastics from the water column, is a consequence of the rugose skeleton structure of corals that entraps microplastics transported by water currents. Long-term exposure to microplastics, and possibly its adhesion, may have very negative consequences on corals, such as necrosis and bleaching (Reichert et al., 2018) and may promote pathogen transmission (Zettler et al., 2013). Recently, sea anemones were found to incorporate microplastics into their host cells, consequently suppressing the uptake of Symbiodinium and seriously impacting symbiosis (Biquand et al., 2017). Overall, adhesion of microplastics to the coral surfaces could partly help explain the loads of plastic missing from the ocean surface, where only 1% of plastic discarded annually into sea can be quantified (Jambeck et al., 2015; Law, 2017), particularly in tropical regions supporting expansive coral reefs. As a consequence, adhered microplastics can enter the food web of coral reef ecosystems (Wilson et al., 2003).
Our results show that ingestion of plastic was independent of the presence of natural prey. Indeed, the presence of a natural food source did not affect the coral feeding on microplastics, neither negatively, e.g., for preference of the natural prey over plastic, neither positively, e.g., for accidental ingestion during predation. This result confirms that corals are generalist feeders who unselectively capture and ingest particles from the water column, as previously indicated by their non-selective feeding on zooplankton (Palardy et al., 2008). Indiscriminate capture of appropriately sized particles by corals results in the ingestion of plastic, as shown by Hall et al. (2015), who reported microplastic ingestion rates by Dipsastrea pallida corals of up to ∼ 50 μg plastic cm–2h–1. However, some scleractinian corals can egest previously ingested microplastics by directly rejecting the piece or excreting it with fecal pellets, although this activity is energetically expensive (Hankins et al., 2018). Indeed, we observed that ingestion rates of microplastics were three orders of magnitude lower than the ingestion rates of A. salina, suggesting that either the mushroom corals tested here can discriminate between plastic and living prey or, more likely, they can egest part of the plastic ingested.
Average loads of plastic retained after ingestion were independent of the presence of biofouling on the plastic surface. However, the likelihood of ingesting more plastic pieces was higher when corals were exposed to biofouled plastics, suggesting that the capacity of the coral to discriminate plastic as an alien particle is reduced if this is covered by biological material. In fact, biofouling on plastic, or generally particles, constitutes a highly nutritional source of food for corals that are able to digest and assimilate it, thus increasing the processing and retention time of biofouled plastic, while readily discarding virgin plastic lacking any coating of biological material (Anthony, 1999).
Scleractinian corals were found to preferentially retain smaller virgin plastic beads, both in these experiments involving D. scruposa and in previous experiments (Martin et al., 2019). Indeed, the smaller plastic beads matched the dimensions of a typical natural prey (10–100 μm, Anthony and Fabricius, 2000; Mills et al., 2004). In contrast, retention of biofouled plastic in D. scruposa was independent of size and larger sizes were equally retained, which is expected given the large polyp-size dimensions of D. scruposa. Indeed, mushroom corals have been shown to feed on prey with a broad range of dimensions, reaching a few centimeters (Hoeksema and Waheed, 2012). This suggests that unfouled plastic (virgin) is more likely recognized as an alien particle and consequently egested if larger than the natural prey, while smaller pieces are not easily distinguished and therefore not egested. Instead, biofouling inhibits plastic egestion, allowing retention of all sizes indiscriminately. Indeed, corals often rely on chemoreception to capture their prey, initiating feeding responses stimulated by compounds found in prey items (Lindstedt, 1971; Lenhoff and Heagy, 1977).
Ingestion of microplastics potentially affects coral energetics, by reducing feeding on organic matter and spending energy in egesting plastic (Rotjan et al., 2019), enhances the toxicity of pollutants absorbed by the plastic that resides undigested for long periods in the coral gut (Rotjan et al., 2019) and impacts trophic transfer affecting organisms that feed on corals and entering the food web (Reichert et al., 2018). The same egested plastic might have an impact along different components of the food web, and it could also transfer components of the coral microbiome, including pathogens to the organism ingesting it next (Oberbeckmann et al., 2015).
Danafungia scruposa was one of the few thriving species present in the study area that had been affected by a strong bleaching event during the 2016 global event (Hughes et al., 2018). The negative effects resulting from interactions of this species with microplastics could further impact the already severely affected coral reef ecosystem. Whereas our goal was to test the role played by mushroom corals as sinks for microplastics, the evidence reported here suggests that D. scruposa may be impacted by plastic ingestion and adhesion. However, long-term exposure experiments investigating the effects of plastic ingestion and adhesion on D. scruposa are needed to demonstrate the impact of plastic pollution on the reef, followed by confirmation in the field of the actual loads of plastic to which the species could be exposed. Indeed, since mushroom corals inhabit the inner reef, which may be an accumulation zone for plastic litter (Saliu et al., 2018), they are potentially subjected to high loads of microplastics. In addition, mushroom corals are mobile and tend to move toward shallower waters, where floating plastic abounds. Moreover, in these shallow waters, they are also exposed both to plastics in the surface waters and plastics sequestered in sediments, which are highly abundant at Magodhoo Island (Saliu et al., 2018). Plastics in surface sediments may be trapped by the polyp tissue, generally used for locomotion (Hoeksema and Bongaerts, 2016).
In conclusion, our experiments showed that D. scruposa removes microplastics from the water column mainly through a passive mechanism of plastic adhesion to the coral surface. Given that plastics ingestion by corals is already recognized as a sink for plastics in the oceans, the much higher removal rate through adhesion of plastics to the coral surfaces indicates that the role played by coral reefs in removing plastic is more important than previously realized. Mushroom corals also ingest plastic particles, with a higher probability of retaining biofouled plastics, possibly because plastic that has been in the marine environment long enough to be colonized by micro- and macro-organisms becomes more palatable to corals. Further experiments on other species conducted directly in the field would be useful to confirm our results and better understand the role played by coral reefs as sink for microplastics.
Data Availability Statement
All data needed to evaluate the conclusion in the article are present in the article and/or the Supplementary Material. Additional data related to this article may be requested from the authors.
Author Contributions
CD conceived the work. EC and CM executed the experiment, and acquired and analyzed the data. RM characterized biofouling on plastics. EC, CM, and CD interpreted the data. EC drafted the manuscript. All authors contributed to improve the manuscript and approved the submission.
Funding
This work was supported and funded by the King Abdullah University of Science and Technology (KAUST) through the baseline funding of CD.
Conflict of Interest
The authors declare that the research was conducted in the absence of any commercial or financial relationships that could be construed as a potential conflict of interest.
Acknowledgments
We thank the MaRHE Center of the University of Milano-Bicocca, especially Davide Maggioni for support during field-work. We also thank Julia Reisser, with the Ocean Clean-up Project for providing the biofouled microplastic from the N. Pacific Gyre used in the experiment.
Supplementary Material
The Supplementary Material for this article can be found online at: https://www.frontiersin.org/articles/10.3389/fmars.2020.00128/full#supplementary-material
References
Allen, A. S., Seymour, A. C., and Rittschof, D. (2017). Chemoreception drives plastic consumption in a hard coral. Mar. Pollut. Bull. 124, 198–205. doi: 10.1016/j.marpolbul.2017.07.030
Anthony, K. R. (1999). Coral suspension feeding on fine particulate matter. J. Exp. Mar. Biolo. Ecol. 232, 85–106. doi: 10.1016/s0022-0981(98)00099-9
Anthony, K. R., and Fabricius, K. E. (2000). Shifting roles of heterotrophy and autotrophy in coral energetics under varying turbidity. J. Exp. Mar. Biol. Ecol. 252, 221–253. doi: 10.1016/s0022-0981(00)00237-9
Arossa, S., Martin, C., Rossbach, S., and Duarte, C. M. (2019). Microplastic removal by red sea giant clam (Tridacna maxima). Environ. Pollut. 252, 1257–1266. doi: 10.1016/j.envpol.2019.05.149
Baalkhuyur, F. M., Dohaish, E. J. A. B., Elhalwagy, M. E., Alikunhi, N. M., Al Suwailem, A. M., Røstad, A., et al. (2018). Microplastic in the gastrointestinal tract of fishes along the Saudi Arabian Red Sea coast. Mar. Pollut. Bull. 131, 407–415. doi: 10.1016/j.marpolbul.2018.04.040
Barnes, D. K., Galgani, F., Thompson, R. C., and Barlaz, M. (2009). Accumulation and fragmentation of plastic debris in global environments. Philos. T. R. Soc. B. 364, 1985–1998. doi: 10.1098/rstb.2008.0205
Barrows, A. P. W., Cathey, S. E., and Petersen, C. W. (2018). Marine environment microfiber contamination: global patterns and the diversity of microparticle origins. Environ. Pollut. 237, 275–284. doi: 10.1016/j.envpol.2018.02.062
Baulch, S., and Perry, C. (2014). Evaluating the impacts of marine debris on cetaceans. Mar. Pollut. Bull. 80, 210–221. doi: 10.1016/j.marpolbul.2013.12.050
Biquand, E., Okubo, N., Aihara, Y., Rolland, V., Hayward, D. C., Hatta, M., et al. (2017). Acceptable symbiont cell size differs among cnidarian species and may limit symbiont diversity. ISME J. 11, 1702. doi: 10.1038/ismej.2017.17
Carbery, M., O’Connor, W., and Palanisami, T. (2018). Trophic transfer of microplastics and mixed contaminants in the marine food web and implications for human health. Environ. Int. 115, 400–409. doi: 10.1016/j.envint.2018.03.007
Cole, M., Lindeque, P., Fileman, E., Halsband, C., Goodhead, R., Moger, J., et al. (2013). Microplastic ingestion by zooplankton. Environ. Sci. Technol. 47, 6646–6655. doi: 10.1021/es400663f
Cozar, A., Echevarría, F., González-Gordillo, J. I., Irigoien, X., Úbeda, B., Hernández-León, S., et al. (2014). Plastic debris in the open ocean. Proc. Natl. Acad. Sci. U.S.A. 111, 10239–10244. doi: 10.1073/pnas.1314705111
Eriksen, M., Lebreton, L. C., Carson, H. S., Thiel, M., Moore, C. J., Borerro, J. C., et al. (2014). Plastic pollution in the world’s oceans: more than 5 trillion plastic pieces weighing over 250,000 tons afloat at sea. PLoS One 9:e111913. doi: 10.1371/journal.pone.0111913
Fallati, L., Savini, A., Sterlacchini, S., and Galli, P. (2017). Land use and land cover (LULC) of the Republic of the Maldives: first national map and LULC change analysis using remote-sensing data. Environ. Monit. Assess. 189:417. doi: 10.1007/s10661-017-6120-2
Galgani, F., Hanke, G., and Maes, T. (2015). “Global distribution, composition and abundance of marine litter,”in Marine Anthropogenic Litter, eds M. Bergmann, L. Gutow, and M. Klages, (Cham: Springer), 29–56. doi: 10.1007/978-3-319-16510-3_2
Goffredo, S., and Chadwick-Furman, N. E. (2000). Abundance and distribution of mushroom corals (Scleractinia: Fungiidae) on a coral reef at Eilat, northern Red Sea. B. Mar. Sci. 66, 241–245.
Hall, N. M., Berry, K. L. E., Rintoul, L., and Hoogenboom, M. O. (2015). Microplastic ingestion by scleractinian corals. Mar. Biol. 162, 725–732. doi: 10.1007/s00227-015-2619-7
Hämer, J., Gutow, L., Köhler, A., and Saborowski, R. (2014). Fate of microplastics in the marine isopod idotea emarginata. Environ. Sci. Technol. 48, 13451–13458. doi: 10.1021/es501385y
Hankins, C., Duffy, A., and Drisco, K. (2018). Scleractinian coral microplastic ingestion: potential calcification effects, size limits, and retention. Mar. Pollut. Bull. 135, 587–593. doi: 10.1016/j.marpolbul.2018.07.067
Harrison, J. P., Sapp, M., Schratzberger, M., and Osborn, A. M. (2011). Interactions between microorganisms and marine microplastics: a call for research. Mar. Technol. Soc. J. 45, 12–20. doi: 10.4031/mtsj.45.2.2
Hoeksema, B. W. (1989). Taxonomy, phylogeny and biogeography of mushroom corals (Scleractinia: Fungiidae). Zool. Verhandelingen 254, 1–295.
Hoeksema, B. W., and Bongaerts, P. (2016). Mobility and self-righting by a free-living mushroom coral through pulsed inflation. Mar. Biodiv. 46, 521–524. doi: 10.1007/s12526-015-0384-y
Hoeksema, B. W., and Moka, W. (1989). Species assemblages and eco-morph variation of mushroom corals (Scleractinia: Fungiidae) related to reef habitats in the Flores Sea. Neth. J. Sea Res. 23, 149–160. doi: 10.1016/0077-7579(89)90009-4
Hoeksema, B. W., and Waheed, Z. (2012). It pays to have a big mouth: mushroom corals ingesting salps at northwest Borneo. Mar. Biodivers. 42, 297–302. doi: 10.1007/s12526-012-0110-y
Hoeksema, B. W., and Yeemin, T. (2011). Late detachment conceals serial budding by the free-living coral Fungia fungites in the Inner Gulf of Thailand. Coral Reefs. 30:975. doi: 10.1007/s00338-011-0784-9
Hughes, T. P., Anderson, K. D., Connolly, S. R., Heron, S. F., Kerry, J. T., Lough, J. M., et al. (2018). Spatial and temporal patterns of mass bleaching of corals in the Anthropocene. Science 359, 80–83. doi: 10.1126/science.aan8048
Jaleel, A. (2013). The status of coral reefs and the management approaches: the case of the Maldives. Ocean Coast. Manag. 82, 104–118. doi: 10.1016/j.ocecoaman.2013.05.009
Jambeck, J. R., Geyer, R., Wilcox, C., Siegler, T. R., Perryman, M., Andrady, A., et al. (2015). Plastic waste inputs from land into the ocean. Science 347, 768–771. doi: 10.1126/science.1260352
Law, K. L. (2017). Plastics in the marine environment. Ann. Rev. Mar. Sci. 9, 205–229. doi: 10.1146/annurev-marine-010816-060409
Lenhoff, H. M., and Heagy, W. (1977). Aquatic invertebrates: model systems for study of receptor activation and evolution of receptor proteins. Annu. Rev. Pharmacol. Toxicol. 17, 243–258. doi: 10.1146/annurev.pa.17.040177.001331
Martin, C., Corona, E., Mahadik, G. A., and Duarte, C. M. (2019). Adhesion to coral surface as a potential sink for marine microplastics. Environ. Poll. 255:113281. doi: 10.1016/j.envpol.2019.113281
Mills, M. M., Lipschultz, F., and Sebens, K. P. (2004). Particulate matter ingestion and associated nitrogen uptake by four species of scleractinian corals. Coral Reefs 23, 311–323. doi: 10.1007/s00338-004-0380-3
Montano, S., Strona, G., Seveso, D., and Galli, P. (2012). First report of coral diseases in the Republic of Maldives. Dis. Aquat. Org. 101, 159–165. doi: 10.3354/dao02515
Moore, C. J. (2008). Synthetic polymers in the marine environment: a rapidly increasing, long-term threat. Environ. Res. 108, 131–139. doi: 10.1016/j.envres.2008.07.025
Murray, F., and Cowie, P. R. (2011). Plastic contamination in the decapod crustacean Nephrops norvegicus (Linnaeus, 1758). Mar. Pollut. Bull. 62, 1207–1217. doi: 10.1016/j.marpolbul.2011.03.032
Oberbeckmann, S., Löder, M. G., and Labrenz, M. (2015). Marine microplastic-associated biofilms–a review. Environ. Chem. 12, 551–562.
Palardy, J. E., Rodrigues, L. J., and Grottoli, A. G. (2008). The importance of zooplankton to the daily metabolic carbon requirements of healthy and bleached corals at two depths. Exp. Mar. Biol. Ecol. 367, 180–188. doi: 10.1016/j.jembe.2008.09.015
Perry, C. T., and Morgan, K. M. (2017). Bleaching drives collapse in reef carbonate budgets and reef growth potential on southern Maldives reefs. Sci. Rep. 7:40581. doi: 10.1038/srep40581
Reichert, J., Schellenberg, J., Schubert, P., and Wilke, T. (2018). Responses of reef building corals to microplastic exposure. Environ. Pollut. 237, 955–960. doi: 10.1016/j.envpol.2017.11.006
Rotjan, R. D., Sharp, K. H., Gauthier, A. E., Yelton, R., Lopez, E. M. B., Carilli, J., et al. (2019). Patterns, dynamics and consequences of microplastic ingestion by the temperate coral. Astrangia poculata. P. Roy. Soc. B Biol. Sci. 286:20190726. doi: 10.1098/rspb.2019.0726
Saliu, F., Montano, S., Garavaglia, M. G., Lasagni, M., Seveso, D., and Galli, P. (2018). Microplastic and charred microplastic in the Faafu Atoll. Maldives. Mar. Pollut. Bull. 136, 464–471. doi: 10.1016/j.marpolbul.2018.09.023
Saponari, L., Montalbetti, E., Galli, P., Strona, G., Seveso, D., Dehenet, I., et al. (2018). Monitoring and assessing a 2-year outbreak of the corallivorous sea star Acanthaster planci in ari atoll. republic of maldives. Environ. Monit. Assess. 190:344. doi: 10.1007/s10661-018-6661-z
Spalding, M. D., Ravilious, C., and Green, E. P. (2001). World Atlas of Coral Reefs. Berkeley, CA: University of California Press.
Stafford-Smith, M. G., and Ormond, R. F. G. (1992). Sediment-rejection mechanisms of 42 species of Australian scleractinian corals. Mar. Freshwater Res. 43, 683–705.
Teuten, E. L., Saquing, J. M., Knappe, D. R., Barlaz, M. A., Jonsson, S., Björn, A., et al. (2009). Transport and release of chemicals from plastics to the environment and to wildlife. P. Roy. Soc. B Biol. Sci. 364, 2027–2045. doi: 10.1098/rstb.2008.0284
Van Cauwenberghe, L., Vanreusel, A., Mees, J., and Janssen, C. R. (2013). Microplastic pollution in deep-sea sediments. Environ. Pollut. 182, 495–499. doi: 10.1016/j.envpol.2013.08.013
Wilson, S. K., Bellwood, D. R., Choat, J. H., and Furnas, M. J. (2003). Detritus in the epilithic algal matrix and its use by coral reef fishes. Oceanogr. Mar. Biol. 41, 279–310.
Yamashiro, H., and Nishira, M. (1995). Phototaxis in Fungiidae corals (Scleractinia). Mar. Biol. 124, 461–465. doi: 10.1007/BF00363920
Keywords: microplastic ingestion, microplastic adhesion, Maldives, biofouled microplastics, Fungiidae, coral reef
Citation: Corona E, Martin C, Marasco R and Duarte CM (2020) Passive and Active Removal of Marine Microplastics by a Mushroom Coral (Danafungia scruposa). Front. Mar. Sci. 7:128. doi: 10.3389/fmars.2020.00128
Received: 18 September 2019; Accepted: 17 February 2020;
Published: 05 March 2020.
Edited by:
Julia Reisser, The University of Western Australia, AustraliaReviewed by:
Ilaria Corsi, University of Siena, ItalyMaira Proietti, Federal University of Rio Grande Foundation, Brazil
Copyright © 2020 Corona, Martin, Marasco and Duarte. This is an open-access article distributed under the terms of the Creative Commons Attribution License (CC BY). The use, distribution or reproduction in other forums is permitted, provided the original author(s) and the copyright owner(s) are credited and that the original publication in this journal is cited, in accordance with accepted academic practice. No use, distribution or reproduction is permitted which does not comply with these terms.
*Correspondence: Elena Corona, ZS5jb3JvbmEyQGNhbXB1cy51bmltaWIuaXQ=