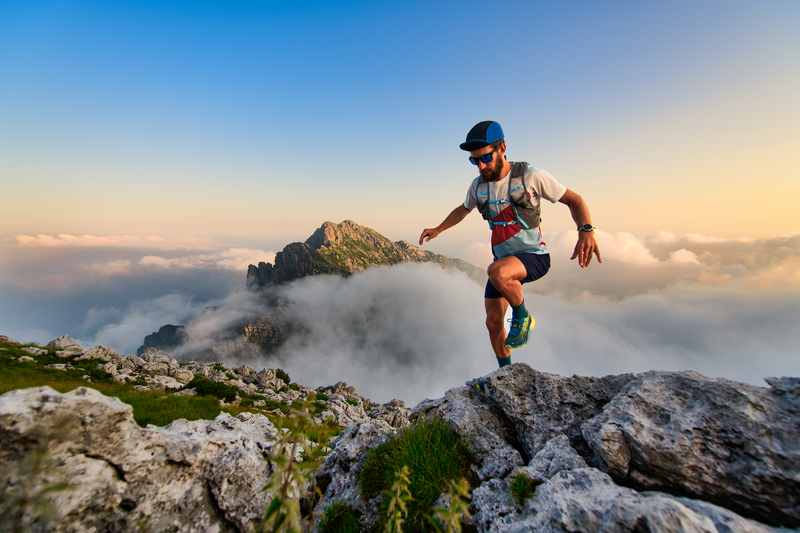
95% of researchers rate our articles as excellent or good
Learn more about the work of our research integrity team to safeguard the quality of each article we publish.
Find out more
REVIEW article
Front. Mar. Sci. , 20 February 2020
Sec. Marine Conservation and Sustainability
Volume 7 - 2020 | https://doi.org/10.3389/fmars.2020.00091
This article is part of the Research Topic Marine Ecosystem Restoration (MER) – Challenges and New Horizons View all 23 articles
Seagrasses provide multiple ecosystem services including nursery habitat, improved water quality, coastal protection, and carbon sequestration. However, seagrasses are in crisis as global coverage is declining at an accelerating rate. With increased focus on ecological restoration as a conservation strategy, methods that enhance restoration success need to be explored. Decades of work in coastal plant ecosystems, including seagrasses, has shown that positive species relationships and feedbacks are critical for ecosystem stability, expansion, and recovery from disturbance. We reviewed the restoration literature on seagrasses and found few studies have tested for the beneficial effects of including positive species interactions in seagrass restoration designs. Here we review the full suite of positive species interactions that have been documented in seagrass ecosystems, where they occur, and how they might be integrated into seagrass restoration. The few studies in marine plant communities that have explicitly incorporated positive species interactions and feedbacks have found an increase in plant growth with little additional resource investment. As oceans continue to change and stressors become more prevalent, harnessing positive interactions between species through innovative approaches will likely become key to successful seagrass restoration.
Seagrasses are present on the coasts of all continents except Antarctica and are among the most productive ecosystems on Earth (Hemminga and Duarte, 2000; Green and Short, 2003). They provide habitat for multiple life stages of many commercially- and recreationally-important fishes, shellfish, and crustaceans, improve water quality, sequester carbon, stabilize sediment, and reduce coastal erosion (Nagelkerken et al., 2000; Jackson et al., 2001; Heck et al., 2003; Orth et al., 2006; Fourqurean et al., 2012; Duarte et al., 2013; James et al., 2019; Lefcheck et al., 2019). However, the total area covered by seagrass is estimated to have declined by 30–60%, including total loss in some places (Evans et al., 2018). Losses of seagrasses have been caused by anthropogenic influences including direct removal during coastal development (e.g., harbors, marinas, and channels), destructive fishing methods (such as trawling), run-off of nutrients and other pollutants from land-based sources, and climate change (Short and Wyllie-Echeverria, 1996; Orth et al., 2006; Hughes et al., 2013; He and Silliman, 2019). The causal mechanisms typically involve increased frequency and intensity of stressors such as light reduction, extreme weather events (e.g., heat waves or cold snaps), high nutrient concentrations, and poor sediment conditions (e.g., high sulfide concentrations).
With recognition that seagrass habitats — together with the many ecosystem services they offer — are in decline globally (but see Santos et al., 2019), conservation and restoration of seagrass has renewed urgency. Historically, seagrass conservation has focused on decreasing environmental stressors (e.g., nutrients and sediment that affect water quality, Lefcheck et al., 2018). In addition, many restoration efforts have typically been conducted across small spatial extents limited to a few hectares. This is partly because of a perception that efforts would yield limited success (∼30%), and partly because of the time and money required for the methods used (Fonseca et al., 1998; Orth et al., 2006), which have included planting of shoots sourced from elsewhere (Cambridge et al., 2002) to broadcast of seeds (Orth et al., 2012), and deployment of substrata to enhance settlement of propagules (Tanner, 2015). Recent successes have demonstrated that active restoration of seagrass beds can be an important tool to facilitate recovery of seagrass meadows (Orth et al., 2012; Statton et al., 2014; van Katwijk et al., 2016; Statton et al., 2018).
Often, seagrass restoration is focused on reducing physical stressors (Bastyan and Cambridge, 2008) and or avoiding negative intraspecific interactions to enhance outplant growth (i.e., dispersed planting methods, Williams, 1987; Rose and Dawes, 1999; Worm and Reusch, 2000). However, positive interactions such as mutualism and facilitation are common in seagrass ecosystems (Peterson and Heck, 2001; Bruno et al., 2003; Van der Heide et al., 2007; Zhang et al., 2018). In tidal marshes, inclusion of positive interactions in restoration has shown recent success (Silliman et al., 2015). Whether such positive interactions might help improve seagrass restoration has rarely been explored. Given they are widespread, it is plausible that judicious inclusion of positive intra- and inter-species interactions into the design of restoration programs might also enhance seagrass restoration.
Amid growing international recognition of the importance of ecological restoration to return ecosystem services (e.g., the United Nations Decade of Ecological Restoration 2021–2030), restoration practices need to innovate to achieve increasingly ambitious goals. Positive interactions are worth examining to see if they can contribute to this innovation. In this paper, we review positive species interactions in seagrass ecosystems (Figure 1), and provide suggestions for research and restoration that, if implemented, has the potential to improve the outcomes of seagrass restoration.
Figure 1. Conceptual drawing of many positive interactions impacting seagrass. This includes but is not limited to long distance facilitation with corals, oysters, mangroves, seabirds, and salt marshes, mutualisms between seagrass and lucinid clams, and facilitation cascades of bivalves in seagrasses as examples.
Positive density dependence occurs when an increase in the density of conspecifics improves survival and reproductive success of an individual or population (Allee, 1931). Classic examples of positive density dependence include prey avoidance behavior in areas of high predation (i.e., nesting seabirds or schooling fish, Neill and Cullen, 1974; Oro et al., 2006). Alternatively, positive density dependence might be prevalent in areas where environmental stress is high and could be a mechanism to support ecosystem resilience (Bertness and Callaway, 1994; Callaway et al., 2002; Gross et al., 2010; Silliman et al., 2011, 2015; He et al., 2013). Restoration is potentially a high-stress scenario, as some sites are less than ideal for growth and survival. Overcoming restrictions to growth and survival (biotic or abiotic), can be challenging (Hobbs and Harris, 2001), but the limited evidence available suggests that positive density dependence might help.
For seagrasses, positive density dependence has been shown to be important for successful reproduction. Several seagrass species are pollen-limited (van Tussenbroek et al., 2016), leading to a prediction that restoration success might be improved if we are able to increase density of seeds or shoots, because this could eventually led to increased density of flowering shoots, and thus more seeds to facilitate natural recovery. Furthermore, positive density dependence has been observed in seagrass colonization and patch survival. In both Zostera marina and Posidonia oceanica beds, higher number of shoots in a patch increased survival and patch expansion (Olesen and Sand-Jensen, 1994; Almela et al., 2008). Indeed, van Katwijk et al. (2016) found, from a global meta-analysis, that seagrass restoration success increased by 20% when large enough numbers (<100000) of shoots or seeds are used. They hypothesized that this was because it spreads risk over time and space as well as allows for net positive feedbacks that promote growth and reproduction — mechanisms intrinsic to positive density dependence. Furthermore, recent restoration efforts support the idea of positive density dependence. Paulo et al. (2019) found larger transplant areas with more shoots had greater long-term survival and expansion than smaller plots. This is likely due to a breached threshold that confers protection from winter storms.
Although planting large numbers of seagrass shoots or seeds might initially be beneficial, the spatial arrangement need to be considered with caution as some work has shown negative effects of self-shading in meadows that are too dense (Ralph et al., 2007). Similar to salt marshes and mangroves, seagrass establishment likely also benefits from aggregated rather than dispersed planting arrangements under stressful conditions (Figure 2, Gedan and Silliman, 2009; Silliman et al., 2015).
Figure 2. Illustration of experimental design and hypothesis of planting design for seagrass along stress gradients. Hypothesized beneficial planting method is denoted by the red box. In a low stress environment, dispersed planting method may work well but in the face of environmental stress, clumped planting is likely to alleviate some stress via neighbor facilitation (Silliman et al., 2015). Figure inspired by a figure in Renzi et al. (2019).
Indeed, a growing body of literature suggests that positive density dependence is important to seagrass ecosystems as seagrasses can facilitate their own growth via multiple feedbacks. Moreover, theory suggests that such positive feedbacks can cause alternative stable states (Van der Heide et al., 2007; Maxwell et al., 2017). However, the ability to breach thresholds and achieve beneficial stable states is complex. In seagrass, there is the potential for several feedbacks that dictate ecosystem states and limiting factors are potentially nested within such feedbacks (Maxwell et al., 2017). Maxwell et al. (2017) suggests that identifying feedbacks such as positive density dependence in limited reproduction or stressful environmental conditions may aid seagrass recovery. The little work that has been done on positive density dependence in seagrass restoration illustrates the need for further exploration into how intraspecific facilitation may be harnessed to improve success and change stable states.
Interspecific mutualism is an interaction between two species that benefits both. Interspecific mutualisms have been well-documented in marine systems. For example, cleaner shrimp interactions with fish and the sea anemone interactions with clownfish (Mariscal, 1970; Bshary and Grutter, 2006). Likewise, in salt marshes, aggregates of mussels deposit nutrients into the soil, enhancing growth of smooth cordgrass (Spartina alterniflora) — in return the mussels receive a refuge from heat stress and predation (Angelini et al., 2015; Bilkovic et al., 2017).
Similarly, mutualism has been shown between seagrass and mussels. Peterson and Heck (2001) found that in the presence of filter-feeding mussels (Modiolus americanus) that likely transfer particulates and nutrients in the water column to the sediment, seagrass (Thalassia testudinum) growth rates and blade width increased, while epiphyte load decreased. Moreover, mussel survival rates increased in the presence of seagrass. Another mutualism occurs between seagrasses and the lucinid clams which harbor sulfide-oxidizing bacteria in their gills that limit toxic sulfide compounds (Van der Heide et al., 2012). While the lucinid clam and its associated bacteria benefit from nutrients sequestered by plant roots (Van der Heide et al., 2012). Seagrass shoot biomass increased 1.4–1.9 fold and root biomass increased 1.3–1.5 fold in treatments where lucinid bivalves were present. In treatments with sulfide addition and no lucinids, seagrass shoot biomass was half that of controls.
While recent work has documented the recovery of mutualisms in seagrass (e.g., recovery of seagrass and associated epifauna) after large-scale restoration in mid-Atlantic coastal bays (Lefcheck et al., 2017). There are no published examples of mutualisms incorporated into seagrass restoration. Restoration in salt marshes with mussel addition (Derksen-Hooijberg et al., 2018) and coral reef with sponge addition (Biggs, 2013) illustrated the creative ways in which mutualisms can be incorporated to enhance restoration. Given the common occurrence of mutualisms in seagrass, we believe there is a strong possibility that restoration outcomes would improve with their inclusion.
Microorganisms, which live within (endophytic) and on the surface of (epiphytic) plants, can profoundly influence plant health and productivity by inducing physiological or biochemical changes within their host (Bacon and White, 2016). They increase nutrient availability, by nitrogen fixation and mineralization of organic compounds, producing phytohormones that promote root and shoot development, and alleviate plant stress (Baligar et al., 2001; Vessey, 2003; Mantelin and Touraine, 2004). Some bacteria facilitate plants by actively detoxifying heavy metals (Lloyd and Lovely, 2001; De et al., 2008; Rajkumar et al., 2012) while others can assist and promote plant growth under high metal stress by directly providing nutrients, phytohormones, and enzymes (Burd et al., 2000; Sheng et al., 2008).
Yet, our knowledge of plant-microbial interactions in the marine environment is limited. However, recent work in salt marshes suggests they could be important. Daleo et al. (2007) showed that mycorrhizal fungi facilitates nutrient uptake in dense-flowered cordgrass (Spartina densiflora). Seagrasses also form symbiotic relationships with a variety of microorganisms (bacteria, archaea, and fungi) both above- and belowground (Venkatachalam et al., 2015; Garcias-Bonet et al., 2016; Tarquinio et al., 2019). For example, seagrass association with sulfide-oxidizing bacteria alleviates toxic sulfate accumulation (Cúcio et al., 2016; Martin et al., 2019). Sulfide-oxidizing bacteria associated with the seagrass rhizosphere have not only been linked with reduction of toxic soil conditions, but also with higher biomass and more complex rhizome structures (Welsh, 2000). Some bacteria (such as Actinobacteria and Cyanobacteria) present on leaves and roots of seagrasses synthesize a wide range of antimicrobial molecules. These bacteria may protect plants by releasing bioactive compounds that selectively target pathogens and biofouling organisms, as has been found in kelp (Egan et al., 2013). On the other hand, some species of sulfide-oxidizing bacteria could indicate poor environmental conditions for seagrass. Mat forming Beggiatoa have been associated with decline of seagrass (Elliott et al., 2006).
Given the limited, but potentially beneficial microbial interactions in seagrass, future research needs to be conducted before use in restoration. Research should test the types of interactions occurring between seagrass and microbes, the benefits or consequences seagrass accrues, and the methods for implementation into a restoration framework.
Trophic interactions can facilitate survival and growth indirectly. For instance, herbivores can promote specific plant species by selectively feeding on their competitors. Plants can also be facilitated indirectly by consumers in simple three-level food chains via a trophic cascade. In these interactions, plants benefit from higher trophic levels that suppress the abundance or behavior of herbivores that would otherwise eat the plants (Figures 1, 3). A classic example of a trophic cascade is sea otters’ maintenance of kelp forests through the removal of kelp-eating sea urchins (Estes and Palmisano, 1974).
Figure 3. Illustration depicting trophic facilitations where: (A) indirect consumption by top predators (sea otters) creates a cascade effect reducing mesopredators (crabs), increasing mesograzers (herbivorous invertebrates) that remove harmful epiphytes on seagrass that result in increased seagrass or (B) direct consumption or removal of seagrass eating herbivores (turtles) by top predators (sharks) maintains seagrass.
Prolific epiphytic macroalgae on seagrass blades can sometimes form aggregations that drift over meadows, negatively affecting seagrass (Silberstein et al., 1986; Drake et al., 2003; Heck and Valentine, 2006). Associated invertebrates often keep epiphyte abundance low (Heck and Valentine, 2006; Cook et al., 2011), which has shown to improve seagrass growth, production, and increase secondary production (Montfrans et al., 1984; Neckles et al., 1993; Duffy et al., 2003). Vertebrates such as great blue herons (Ardea herodias) and sea otters (Enhydra lutris) have also been shown to regulate biomass of seagrass epiphytes through trophic cascades, in which the consumption of fish and crustaceans increases the abundance of grazing invertebrates (Hughes et al., 2013; Huang et al., 2015). Alternatively, seagrass can be directly grazed upon. Seagrass herbivory by macrograzers (i.e., fish) and megagrazers (i.e., turtles and dugongs) are important to maintaining ecosystem function and reproduction in balanced ecosystems (i.e., systems with predators, grazers, and seagrasses) (Tol et al., 2017; Scott et al., 2018). With the loss of predators and conservation of large herbivores without habitat consideration, it is suggested that seagrass ecosystem functions could be lost from uncontrolled rates of grazing (Burkholder et al., 2013; Christianen et al., 2014; Heithaus et al., 2014; Scott et al., 2018).
Top predator introduction and herbivore management as methods for conservation is not a new idea. However, the generality of these chains of interactions, and how we might use them to benefit seagrass restoration, is not well-known. It is possible that predator introduction or recolonization might help stabilize or reverse seagrass decline in some places (Silliman et al., 2018). Predator addition is unlikely to be effective in areas where the cause of predator loss is unknown or seagrass has been completely lost, unless viable sources of propagules are nearby. Joint reintroduction of predators and seagrass restoration or consideration of sites with established predator populations might mitigate stressors in some conditions, especially where epiphyte consumers are uncommon, seagrass consumers are common, or nutrient concentrations are high.
Interspecies, positive interactions are not limited to close proximity. Long distance facilitation occurs when a species is benefited by another that is not in direct physical contact (van de Koppel et al., 2015). This interaction is unique from the symbiotic mutualisms described above as physical contact between the interacting species does not occur and the positive effect is one- way, not requiring a feedback.
A recent review has shown long distance facilitations are important for maintaining stability and resilience in many marine ecosystems, including seagrasses. In this review, van de Koppel et al. (2015) describes how long-distance facilitations may mitigate light limitation, nutrient stress, and physical stress on seagrasses — which are all challenges in restoration. Light limitation can be reduced by non-sympatric bivalve reefs and mangroves that remove particulates from the water (Newell and Koch, 2004; Gillis et al., 2014; van de Koppel et al., 2015). Nutrient limitation can be improved in several ways by long distance facilitation as nutrient input from nearby but not overlapping mussel reefs (Reusch et al., 1994; van de Koppel et al., 2015), mangroves (Mohammed et al., 2001), bird colonies (Powell et al., 1991), and kelp forests (Wernberg et al., 2006; Hyndes et al., 2012) can improve plant growth. Alternatively, the negative effects of eutrophication on seagrass are diminished by the interception and burial of nutrients by salt marshes and mangroves (Valiela and Cole, 2002). Finally, physical stress on seagrass such as wave action can be reduced by coral and bivalve reefs (Moberg and Folke, 1999; Ferrario et al., 2014; van de Koppel et al., 2015). Incorporating long-distance facilitations into site selection for seagrass restoration is likely to benefit planting successes, but much more research is needed to understand context dependency of this type of interaction and the physical conditions under which it is likely to be most beneficial.
Facilitation cascades are indirect positive effects that emerge from direct facilitations. A non-trophic example is when a primary foundation species facilitates a secondary one which, in turn, enhance biodiversity (Altieri et al., 2007). This particular facilitation cascade is called a habitat cascade. A recent review, Thomsen et al. (2018) shows that these facilitation cascades occur across all ecosystems and their impacts on biodiversity is measurable.
The role of seagrasses as primary foundation species that facilitate other organisms suggests facilitation cascades might be widespread. For example, seagrasses can be epiphytized by a range of taxa including algae, bryozoans, and sponges (Borowitzka and Lethbridge, 1989), which in turn have been shown to provide food and shelter for a range of small invertebrates (Jernakoff et al., 1996). Interactions in Zostera muelleri meadows found that razor clams, Pinna sp. formed a complex array of positive and negative interactions culminating in a net increase in overall diversity when live clams were present (Gribben et al., 2017). Another study found that density of pen clams (Atrina rigida and Atrina serrata) were positively associated with eelgrass (Z. marina) and that in the presence of pen clams, diversity of animals in the meadow increased (Zhang and Silliman, 2019). Although these studies did not observe a change in seagrass growth and production during the study period, others have found that an increase in overall biodiversity can lead to more stable and productive seagrass habitat as well as buffer ecosystems from changing conditions (Duffy, 2006).
As facilitation cascades are a research frontier, their importance and occurrence in seagrasses is mostly unexplored. However, given the effect size they have in other systems, their importance could be high. Future research should test generality and impacts of facilitation cascades in seagrass systems, and, if found, systematically test how addition of secondary foundation species impacts seagrass restoration success, both in terms of plant growth and increased overall system functioning (e.g., provisioning of biodiversity).
Biodiversity can refer to genetic, species, and functional diversity of an ecosystem. Increased biodiversity can facilitate healthier, more productive ecosystems that are resilient to disturbance and stable due to a repetition in functional groups (Chapin et al., 2000; Reed and Frankham, 2003; Hooper et al., 2005; Hughes et al., 2008; Hensel and Silliman, 2013). Given these findings, ecosystem restoration is likely to benefit from inclusion of similar intra- and interspecies diversity facilitations.
Globally, there are 72 seagrass species, ranging from temperate to tropical climates with many of them co-occurring (Orth et al., 2006). Traditional ecological theory suggests that the presence of co-existing species would create competitive interactions (McGilchrist, 1965; Hassell and Comins, 1976). However, some studies suggest that the co-existence of seagrass species is beneficial, especially in areas of frequent disturbance where interspecies diversity increases ecosystem resilience (Williams, 1990). Williams et al. (2017) found that increased species richness of seagrasses also increased transplant success. They hypothesized that the mechanism underlaying this effect was niche partitioning of resources and a diversity of growth patterns. Restoring multiple species, if originally present, could restore function and self-reliance in the system (Duffy, 2006). To make our knowledge about seagrass diversity more impactful in restoration more research in multispecies conditions is needed.
Species diversity is not the only means of diversity to consider for seagrass restoration. Genetically diverse populations are often more productive in stressful environments (Hughes and Stachowicz, 2009). Unlike previous topics in this paper, genetic diversity has been studied and even considered in seagrass restoration. The studies that implemented genetic diversity into their restoration scheme observed increased restoration success (Williams, 2001; van Katwijk et al., 2009; Reynolds et al., 2012). It is thought that donor populations from already stressful environments will be better adapted to restoration site conditions (Franssen et al., 2014; Marín-Guirao et al., 2016; Tutar et al., 2017). As well, diverse populations spread the risk of complete collapse by a single stressor (van Katwijk et al., 2009). Considering genetic variation in donor populations that have similar habitats to restoration sites and account for a variety of stressors could be valuable in restoration planning.
Restoration is increasingly becoming an important component in conservation. Restoration of seagrass has mostly been limited in extent with generally low success (Statton et al., 2018). As seagrass restoration continues to mature, practices will improve. We suggest that incorporation of knowledge gained through ecology will help accelerate improvement. Incorporating positive interactions into restoration methods appears to be a promising avenue for restoration research and practice. However, positive interactions are likely not a shortcut to restoration success but rather a complimentary method to traditional methods.
Traditionally, marine restoration has focused on systematically reducing stressors. Here, we propose to expand the perspective on seagrass restoration to also include systematic reduction of physical stressors, but to also methodically incorporate positive species interactions (Figure 4). This means that efforts to improve water and sediment conditions should be continued and that positive interactions should be additionally included in such efforts. Of course, the expression of positive and negative interactions in a system vary depending on the organisms available, environmental conditions, and site characteristics (He et al., 2013), and this will be reflected in restoration approaches that are sensitive to these contexts. However, our review shows that seagrasses participate in many potential positive interactions that could be usefully harnessed to enhance restoration success. Many studies have considered the balance between positive and negative interactions and the layering of human and biological interventions, but none have discussed the potential to harness these in seagrass restoration (Bertness et al., 1999; Maestre et al., 2003; Cheong et al., 2013). We suggest that researchers and restoration practitioners should consider positive interactions as an untapped resource with potential to enhance seagrass restoration goals (Figure 5). Progress will be facilitated if researchers and practitioners work in tandem to test the potential of a range of positive interactions to improve restoration.
Figure 4. Tree diagram of interactions discussed in this paper, organismal level at which we think they are prevalent, and examples of organisms that have been observed having these interactions with seagrasses. Organisms are color coded by the alleviation they likely have on seagrasses. In some cases, organisms can demonstrate many beneficial roles denoted by an asterisk color coded by another effect. The primary and secondary coloration of the word and asterisk are arbitrary and do not denote strength of the effect.
Figure 5. Conceptual map of some common restoration goals (Yap, 2000) and the positive interactions that could be used to accomplish such goals, and brief recommendations of how to explore the positive interactions as a starting point for researchers and practitioners.
Many positive interactions are not fully understood — for example the role of microbes and facilitation cascades — so we encourage researchers to develop an understanding of such interactions so that they can be effectively applied in restoration. This is not without challenges in these complex, sometimes distant interactions, especially where ecosystems develop slowly. The inclusion of positive interactions into restoration will not occur simultaneously but should be considered as research progresses. In this paper, we have outlined a broad suite of positive interactions, shown how they are expressed in seagrass ecosystems, and offered some ideas about how they might be used to enhance restoration. Below, we outline a restoration and research framework that deserve further consideration to enhance future seagrass restoration:
(1) State clearly restoration goals — this will help understand whether positive interactions are applicable and which ones.
(2) Test whether planting arrangements (e.g., dispersed versus clumped) and number of units (plants, shoots, seeds, or other units) improve survival, growth and reproduction in a variety of contexts.
(3) Include bivalves (such as lucinid clams or mussels) when planting seagrass to help improve survival and growth, and test different arrangements and methods of including them.
(4) Determine sites near established mangroves, coral reefs, or oyster that generate potential long-distance facilitations.
(5) Identify sites with intact assemblages of seagrass facilitating herbivores and predators.
(6) Design restoration projects with initial cohorts that are genetically diverse, selecting transplant units (such as whole plants or seeds) from several distinct parent populations to increase resiliency.
(7) Test whether restoration using multiple species of seagrasses, in different arrangements, improves restoration success in places where seagrass diversity is naturally high.
(8) Consider restoring ecosystems rather than single, target species.
BS conceived the idea for the manuscript. SV wrote the manuscript with additions from FT on microbial interactions. SV created all figures. BS, TH, YZ, FT, MV, and RO provided comments and improvements.
This work was supported in part by the National Science Foundation (GRFP DGE – 1644868 to SV), the Duke University Wetland Center Student Research Grant Program to SV, Sigma Xi Grant in Aid of Graduate Research (G201903158855877 to SV), the Lenfest Ocean Foundation to BS, and by NWO-Vidi career grant 16588 to TH. This is contribution no. 3871 from the Virginia Institute of Marine Science.
The authors declare that the research was conducted in the absence of any commercial or financial relationships that could be construed as a potential conflict of interest.
Almela, E. D., Marbà, N., Álvarez, E., Santiago, R., Martínez, R., and Duarte, C. M. (2008). Patch dynamics of the mediterranean seagrass Posidonia oceanica: implications for recolonisation process. Aquat. Bot. 89, 397–403. doi: 10.1016/j.aquabot.2008.04.012
Altieri, A. H., Silliman, B. R., and Bertness, M. D. (2007). Hierarchical organization via a facilitation cascade in intertidal cordgrass bed communities. Am. Nat. 169, 195–206. doi: 10.1086/510603
Angelini, C., van der Heide, T., Griffin, J. N., Morton, J. P., Derksen-Hooijberg, M., Lamers, L. P. M., et al. (2015). Foundation species’ overlap enhances biodiversity and multifunctionality from the patch to landscape scale in Southeastern United States Salt Marshes. Proc. R. Soc. B Biol. Sci. 282:20150421. doi: 10.1098/rspb.2015.0421
Bacon, C. W., and White, J. F. (2016). Functions, mechanisms and regulation of endophytic and epiphytic microbial communities of plants. Symbiosis 68, 87–98. doi: 10.1007/s13199-015-0350-2
Baligar, V. C., Fageria, N. K., and He, Z. L. (2001). Nutrient use efficiency in plants. Commun. Soil Sci. Plant Anal. 32, 921–950. doi: 10.1081/CSS-100104098
Bastyan, G. R., and Cambridge, M. L. (2008). Transplantation as a method for restoring the seagrass Posidonia australis. Estuar. Coast. Shelf Sci. 79, 289–299. doi: 10.1016/j.ecss.2008.04.012
Bertness, M. D., and Callaway, R. (1994). Positive interactions in communities. Trends Ecol. Evol. 9, 191–193. doi: 10.1016/0169-5347(94)90088-4
Bertness, M. D., Leonard, G. H., Levine, J. M., Schmidt, P. R., and Ingraham, A. O. (1999). Testing the relative contribution of positive and negative interactions in rocky intertidal communities. Ecology 80, 2711–2726. doi: 10.1890/0012-9658(1999)080
Biggs, B. C. (2013). Harnessing natural recovery processes to improve restoration outcomes: an experimental assessment of sponge-mediated coral reef restoration. PLoS One 8:e64945. doi: 10.1371/journal.pone.0064945
Bilkovic, D. M., Mitchell, M. M., Isdell, R. E., Schliep, M., and Smyth, A. R. (2017). Mutualism between ribbed mussels and Cordgrass enhances salt marsh nitrogen removal. Ecosphere 8:e01795. doi: 10.1002/ecs2.1795
Borowitzka, M. A., and Lethbridge, R. C. (1989). “Seagrass epiphytes,” in Biology of Seagrasses: A Treatise on the Biology of Seagrasses with Special Reference to the Australian Region, eds A. W. D. Larkum, A. J. McComb, and S. A. Shephard, (Amsterdam: Elsevier), 458–499.
Bruno, J. F., Stachowicz, J. J., and Bertness, M. D. (2003). Inclusion of facilitation into ecological theory. Trends Ecol. Evol. 18, 119–125. doi: 10.1016/S0169-5347(02)00045-9
Bshary, R., and Grutter, A. S. (2006). Image scoring and cooperation in a cleaner fish mutualism. Nature 441, 975–978. doi: 10.1038/nature04755
Burd, G. I., Dixon, D. G., and Glick, B. R. (2000). Plant growth-promoting bacteria that decrease heavy metal toxicity in plants. Can. J. Microbiol. 46, 237–245. doi: 10.1139/w99-143
Burkholder, D. A., Heithaus, M. R., Fourqurean, J. W., Wirsing, A., and Dill, L. M. (2013). Patterns of top-down control in a seagrass ecosystem: could a roving apex predator induce a behaviour-mediated trophic cascade?” J. Anim. Ecol. 82, 1192–1202. doi: 10.1111/1365-2656.12097
Callaway, R. M., Brooker, R. W., Choler, P., Kikvidze, Z., Lortie, C. J., Michalet, R., et al. (2002). Positive interactions among alpine plants increase with stress. Nature 417, 844–848. doi: 10.1038/nature00812
Cambridge, M. L., Bastyan, G. R., and Walker, D. I. (2002). Recovery of Posidonia Meadows in Oyster Harbour, Southwestern Australia Text. Available at: http://www.ingentaconnect.com/content/umrsmas/bullmar/2002/00000071/00000003/art00014 (accessed November 01, 2002).
Chapin, III F. S., Zavaleta, E. S., Eviner, V. T., Naylor, R. L., Vitousek, P. M., Reynolds, H. L., et al. (2000). Consequences of changing biodiversity. Nature 405, 234–242. doi: 10.1038/35012241
Cheong, S., Silliman, B. R., Wong, P. P., van Wesenbeeck, B., Kim, C., and Guannel, G. (2013). Coastal adaptation with ecological engineering. Nat. Clim. Change 3, 787–791. doi: 10.1038/nclimate1854
Christianen, M. J. A., Herman, P. M. J., Bouma, T. J., Lamers, L. P. M., van Katwijk, M., van der, H. T., et al. (2014). Habitat collapse due to overgrazing threatens turtle conservation in marine protected areas. Proc. R. Soc. B Biol. Sci. 281:20132890. doi: 10.1098/rspb.2013.2890
Cook, K., Vanderklift, M. A., and Poore, A. G. B. (2011). Strong effects of herbivorous amphipods on epiphyte biomass in a temperate seagrass meadow. Mar. Ecol. Prog. Ser. 442, 263–269. doi: 10.3354/meps09446
Cúcio, C., Engelen, A. H., Costa, R., and Muyzer, G. (2016). Rhizosphere microbiomes of European + seagrasses are selected by the plant, but are not species specific. Front. Microbiol. 7:440. doi: 10.3389/fmicb.2016.00440
Daleo, P., Fanjul, E., Casariego, A. M., Silliman, B. R., Bertness, M. D., and Iribarne, O. (2007). Ecosystem engineers activate mycorrhizal mutualism in salt marshes. Ecol. Lett. 10, 902–908. doi: 10.1111/j.1461-0248.2007.01082.x
De, J., Ramaiah, N., and Vardanyan, L. (2008). Detoxification of toxic heavy metals by marine bacteria highly resistant to mercury. Mar. Biotechnol. 10, 471–477. doi: 10.1007/s10126-008-9083-z
Derksen-Hooijberg, M., Angelini, C., Lamers, L. P. M., Borst, A., Smolders, A., Hoogveld, J. R. H., et al. (2018). Mutualistic interactions amplify saltmarsh restoration success. J. Appl. Ecol. 55, 405–414. doi: 10.1111/1365-2664.12960
Drake, L. A., Dobbs, F. C., and Zimmerman, R. C. (2003). Effects of epiphyte load on optical properties and photosynthetic potential of the seagrasses Thalassia testudinum banks Ex König and Zostera marina L. Limnol. Oceanogr. 48, 456–463. doi: 10.4319/lo.2003.48.1_part_2.0456
Duarte, C. M., Kennedy, H., Marbà, N., and Hendriks, I. (2013). Assessing the capacity of seagrass meadows for carbon burial: current limitations and future strategies. Ocean Coast. Manag. 83, 32–38. doi: 10.1016/j.ocecoaman.2011.09.001
Duffy, J. E. (2006). Biodiversity and the functioning of seagrass ecosystems. Mar. Ecol. Prog. Ser. 311, 233–250. doi: 10.3354/meps311233
Duffy, J. E., Richardson, J. P., and Canuel, E. A. (2003). Grazer diversity effects on ecosystem functioning in seagrass beds. Ecol. Lett. 6, 637–645. doi: 10.1046/j.1461-0248.2003.00474.x
Egan, S., Harder, T., Burke, C., Steinberg, P., Kjelleberg, S., and Thomas, T. (2013). The seaweed holobiont: understanding seaweed–bacteria interactions. FEMS Microbiol. Rev. 37, 462–476. doi: 10.1111/1574-6976.12011
Elliott, J. K., Spear, E., and Wyllie-Echeverria, S. (2006). Mats of Beggiatoa bacteria reveal that organic pollution from lumber mills inhibits growth of Zostera marina. Mar. Ecol. 27, 372–380. doi: 10.1111/j.1439-0485.2006.00100.x
Estes, J. A., and Palmisano, J. F. (1974). Sea otters: their role in structuring nearshore communities. Science 185, 1058–1060. doi: 10.1126/science.185.4156.1058
Evans, S. M., Griffin, K. J., Blick, R. A. J., Poore, A. G. B., and Vergés, A. (2018). Seagrass on the brink: decline of threatened seagrass Posidonia australis continues following protection. PLoS One 13:e0190370. doi: 10.1371/journal.pone.0190370
Ferrario, F., Beck, M. W., Storlazzi, C. D., Micheli, F., Shepard, C. C., and Airoldi, L. (2014). The effectiveness of coral reefs for coastal hazard risk reduction and adaptation. Nat. Commun. 5:3794. doi: 10.1038/ncomms4794
Fonseca, M., Kenworthy, W. J., and Thayer, G. (1998). Guidelines for the Conservation and Restoration of Seagrasses in the United States and Adjacent Waters. Silver Spring, MD: NOAA Coastal Ocean Office, 222.
Fourqurean, J. W., Duarte, C. D., Kennedy, H., Marbà, N., Holmer, M., Mateo, M. A., et al. (2012). Seagrass ecosystems as a globally significant carbon stock. Nat. Geosci. 5, 505–509. doi: 10.1038/ngeo1477
Franssen, S. U., Gu, J., Winters, G., Huylmans, A., Wienpahl, I., Sparwel, M., et al. (2014). Genome-wide transcriptomic responses of the Seagrasses Zostera marina and Nanozostera noltii under a simulated heatwave confirm functional types. Mar. Genomics 15, 65–73. doi: 10.1016/j.margen.2014.03.004
Garcias-Bonet, N., Arrieta, J. M., Duarte, C. M., and Marbà, N. (2016). Nitrogen-fixing bacteria in mediterranean seagrass (Posidonia oceanica) roots. Aquat. Bot. 131, 57–60. doi: 10.1016/j.aquabot.2016.03.002
Gedan, K. B., and Silliman, B. R. (2009). Using facilitation theory to enhance mangrove restoration. Ambio 38:109. doi: 10.1579/0044-7447-38.2.109
Gillis, L. G., Bouma, T. J., Jones, C. G., van Katwijk, M. M., Nagelkerken, I., Jeuken, C. J. L., et al. (2014). Potential for landscape-scale positive interactions among tropical marine ecosystems. Mar. Ecol. Prog. Ser. 503, 289–303. doi: 10.3354/meps10716
Green, E. P., and Short, F. T. (2003). World Atlas of Seagrasses. Berkeley, CA: University of California Press.
Gribben, P. E., Kimbro, D. L., Vergés, A., Gouhier, T. C., Burrell, S., Garthwin, R. G., et al. (2017). Positive and negative interactions control a facilitation cascade. Ecosphere 8:e02065. doi: 10.1002/ecs2.2065
Gross, N., Liancourt, P., Choler, P., Suding, K. N., and Lavorel, S. (2010). Strain and vegetation effects on local limiting resources explain the outcomes of biotic interactions. Perspect. Plant Ecol. Evol. Syst. 12, 9–19. doi: 10.1016/j.ppees.2009.09.001
Hassell, M. P., and Comins, H. N. (1976). Discrete time models for two-species competition. Theor. Popul. Biol. 9, 202–221. doi: 10.1016/0040-5809(76)90045-9
He, Q., Bertness, M. D., and Altieri, A. H. (2013). Global shifts towards positive species interactions with increasing environmental stress. Ecol. Lett. 16, 695–706. doi: 10.1111/ele.12080
He, Q., and Silliman, B. R. (2019). Climate change, human impacts, and coastal ecosystems in the anthropocene. Curr. Biol. 29, R1021–R1035. doi: 10.1016/j.cub.2019.08.042
Heck, K. L., Hays, G., and Orth, R. J. (2003). Critical evaluation of the nursery role hypothesis for seagrass meadows. Mar. Ecol. Prog. Ser. 253, 123–136. doi: 10.3354/meps253123
Heck, K. L., and Valentine, J. F. (2006). Plant–herbivore interactions in seagrass meadows. J. Exp. Mar. Biol. Ecol. 330, 420–436. doi: 10.1016/j.jembe.2005.12.044
Heithaus, M. R., Alcoverro, T., Arthur, R., Burkholder, D. A., Coates, K. A., Christianen, M. J. A., et al. (2014). Seagrasses in the age of sea turtle conservation and shark overfishing. Front. Mar. Sci. 1:28. doi: 10.3389/fmars.2014.00028
Hensel, M. J., and Silliman, B. R. (2013). Consumer diversity across kingdoms supports multiple functions in a coastal ecosystem. Proc. Natl. Acad. Sci. U.S.A. 110, 20621–20626. doi: 10.1073/pnas.1312317110
Hobbs, R. J., and Harris, J. A. (2001). Restoration ecology: repairing the earth’s ecosystems in the new millennium. Restor. Ecol. 9, 239–246. doi: 10.1046/j.1526-100x.2001.009002239.x
Hooper, D. U., Chapin, F. S., Ewel, J. J., Hector, A., Inchausti, P., Lavorel, S., et al. (2005). Effects of biodiversity on ecosystem functioning: a consensus of current knowledge. Ecol. Monogr. 75, 3–35. doi: 10.1890/04-0922
Huang, A. C., Essak, M., and O’Connor, M. I. (2015). Top–down control by great blue herons Ardea herodias regulates seagrass-Associated epifauna. Oikos 124, 1492–1501. doi: 10.1111/oik.01988
Hughes, A. R., Inouye, B. D., Johnson, M. T. J., Underwood, N., and Vellend, M. (2008). Ecological consequences of genetic diversity. Ecol. Lett. 11, 609–623. doi: 10.1111/j.1461-0248.2008.01179.x
Hughes, A. R., and Stachowicz, J. J. (2009). Ecological impacts of genotypic diversity in the clonal seagrass Zostera marina. Ecology 90, 1412–1419. doi: 10.1890/07-2030.1
Hughes, B. B., Eby, R., Van Dyke, E., Tinker, M. T., Marks, C. I., Johnson, K. S., et al. (2013). Recovery of a top predator mediates negative eutrophic effects on seagrass. Proc. Natl. Acad. Sci. U.S.A. 110, 15313–15318. doi: 10.1073/pnas.1302805110
Hyndes, G., Lavery, P., and Doropoulos, C. (2012). Dual processes for cross-boundary subsidies: incorporation of nutrients from reef-derived kelp into a seagrass ecosystem. Mar. Ecol. Prog. Ser. 445, 97–107. doi: 10.3354/meps09367
Jackson, E. L., Rowden, A. A., Attrill, M. J., Bossey, S. J., and Jones, M. B. (2001). The importance of seagrass beds as a habitat for fishery species. Oceanogr. Mar. Biol. 39, 269–303. doi: 10.1201/b12588-3
James, R. K., Silva, R., van Tussenbroek, B. I., Escudero-Castillo, M., Mariño-Tapia, I., Dijkstra, H. A., et al. (2019). Maintaining tropical beaches with seagrass and algae: a promising alternative to engineering solutions. Bioscience 69, 136–142. doi: 10.1093/biosci/biy154
Jernakoff, P., Brearley, A., and Nielsen, J. (1996). Factors affecting grazer-epiphyte interactions in temperate seagrass meadows. Oceanogr. Mar. Biol. 34, 109–162.
Lefcheck, J. S., Hughes, B. B., Johnson, A. J., Pfirrmann, B. W., Rasher, D. B., Smyth, A. R., et al. (2019). Are coastal habitats important nurseries? A meta-analysis. Conserv. Lett. 12, e12645. doi: 10.1111/conl.12645
Lefcheck, J. S., Marion, S. R., and Orth, R. J. (2017). Restored Eelgrass (Zostera Marina L.) as a refuge for epifaunal biodiversity in mid-western Atlantic coastal bays. Estuaries Coast. 40, 200–212. doi: 10.1007/s12237-016-0141-x
Lefcheck, J. S., Orth, R. J., Dennison, W. C., Wilcox, D. J., Murphy, R. R., Keisman, J., et al. (2018). Long-term nutrient reductions lead to the unprecedented recovery of a temperate coastal region. Proc. Natl. Acad. Sci. U.S.A. 115, 3658–3662. doi: 10.1073/pnas.1715798115
Lloyd, J. R., and Lovely, D. R. (2001). Microbial detoxification of metals and radionuclides. Curr. Opin. Biotechnol. 12, 248–253. doi: 10.1016/S0958-1669(00)00207-X
Maestre, F. T., Bautista, S., and Cortina, J. (2003). Positive, negative, and net effects in grass–shrub interactions in mediterranean semiarid grasslands. Ecology 84, 3186–3197. doi: 10.1890/02-0635
Mantelin, S., and Touraine, B. (2004). Plant growth-promoting bacteria and nitrate availability: impacts on root development and nitrate uptake. J. Exp. Bot. 55, 27–34. doi: 10.1093/jxb/erh010
Marín-Guirao, L., Ruiz, J. M., Dattolo, E., Garcia-Munoz, R., and Procaccini, G. (2016). Physiological and molecular evidence of differential short-term heat tolerance in mediterranean seagrasses. Sci. Rep. 6:28615. doi: 10.1038/srep28615
Mariscal, R. N. (1970). The nature of the symbiosis between indo-pacific anemone fishes and sea anemones. Mar. Biol. 6, 58–65. doi: 10.1007/BF00352608
Martin, B. C., Bougoure, J., Ryan, M. H., Bennett, W. W., Colmer, T. D., Joyce, N. K., et al. (2019). Oxygen loss from seagrass roots coincides with colonisation of Sulphide-Oxidising cable bacteria and reduces Sulphide stress. ISME J. 13, 707–719. doi: 10.1038/s41396-018-0308-5
Maxwell, P. S., Eklöf, J. S., van Van Katwijk, M. M., O’Brien, K. O., de la Torre-Castro, M., Boström, C., et al. (2017). The fundamental role of ecological feedback mechanisms for the adaptive management of seagrass ecosystems – a review. Biol. Rev. 92, 1521–1538. doi: 10.1111/brv.12294
McGilchrist, C. A. (1965). Analysis of competition experiments. Biometrics 21, 975–985. doi: 10.2307/2528258
Moberg, F., and Folke, C. (1999). Ecological goods and services of coral reef ecosystems. Ecol. Econ. 29, 215–233. doi: 10.1016/S0921-8009(99)00009-9
Mohammed, S., Johnstone, R. W., Widen, B., and Jordelius, E. (2001). “The role of mangroves in nutrient cycling and productivity of adjacent seagrass communities Chawka Bay, Zanzibar,” in Proceedings of the 20th Annual Conference on Advances in Marine Science in Tanzaa (Zanzibar: University of Dar es Salaam ana).
Montfrans, J., van Wetzel, R. L., and Orth, R. J. (1984). Epiphyte-grazer relationships in seagrass meadows: consequences for seagrass growth and production. Estuaries 7, 289–309. doi: 10.2307/1351615
Nagelkerken, I., van der Velde, G., Gorissen, M. W., Meijer, G. J., Van’t Hof, T., and den Hartog, C. (2000). Importance of mangroves, seagrass beds and the shallow coral reef as a nursery for important coral reef fishes, using a visual census technique. Estuar. Coast. Shelf Sci. 51, 31–44. doi: 10.1006/ecss.2000.0617
Neckles, H. A., Wetzel, R. L., and Orth, R. J. (1993). Relative effects of nutrient enrichment and grazing on epiphyte-macrophyte (Zostera marina L.) dynamics. Oecologia 93, 285–295. doi: 10.1007/BF00317683
Neill, S. R. J., and Cullen, J. M. (1974). Experiments on whether schooling by their prey affects the hunting behaviour of cephalopods and fish predators. J. Zool. 172, 549–569. doi: 10.1111/j.1469-7998.1974.tb04385.x
Newell, R. I. E., and Koch, E. W. (2004). Modeling seagrass density and distribution in response to changes in turbidity stemming from bivalve filtration and seagrass sediment stabilization. Estuaries 27, 793–806. doi: 10.1007/BF02912041
Olesen, B., and Sand-Jensen, K. (1994). Patch dynamics of eelgrass Zostera marina. Mar. Ecol. Prog. Ser. 106, 147–156. doi: 10.3354/meps106147
Oro, D., Martínez-Abraín, A., Paracuellos, M., Nevado, J. C., and Genovart, M. (2006). Influence of density dependence on predator–prey seabird interactions at large spatio-temporal scales. Proc. R. Soc. Lond. B Biol. Sci. 273, 379–383. doi: 10.1098/rspb.2005.3287
Orth, R. J., Carruthers, T. J. B., Dennison, W. C., Duarte, C. M., Fourqurean, J. W., Heck, K. L., et al. (2006). A global crisis for seagrass ecosystems. Bioscience 56, 987–996. doi: 10.1641/0006-3568200656
Orth, R. J., Moore, K. A., Marion, S. R., Wilcox, D. J., and Parrish, D. P. (2012). Seed addition facilitates eelgrass recovery in a coastal bay system. Mar. Ecol. Prog. Ser. 448, 177–195. doi: 10.3354/meps09522
Paulo, D., Cunha, A. H., Boavida, J., Serrão, E. A., Gonçalves, E. J., and Fonseca, M. (2019). Open coast seagrass restoration. Can we do it? Large scale seagrass transplants. Front. Mar. Sci. 6:52. doi: 10.3389/fmars.2019.00052
Peterson, B. J., and Heck, K. L. (2001). Positive interactions between suspension-feeding bivalves and seagrass a facultative mutualism. Mar. Ecol. Prog. Ser. 213, 143–155. doi: 10.3354/meps213143
Powell, G. V., Fourqurean, J. W., Kenworthy, W. J., and Zieman, J. C. (1991). Bird colonies cause seagrass enrichment in a subtropical estuary: observational and experimental evidence. Estuar. Coast. Shelf Sci. 32, 567–579. doi: 10.1016/0272-7714(91)90075-M
Rajkumar, M., Sandhya, S., Prasad, M. N. V., and Freitas, H. (2012). Perspectives of plant-associated microbes in heavy metal phytoremediation. Biotechnol. Adv. 30, 1562–1574. doi: 10.1016/j.biotechadv.2012.04.011
Ralph, P. J., Durako, M. J., Enríquez, S., Collier, C. J., and Doblin, M. A. (2007). Impact of light limitation on seagrasses. J. Exp. Mar. Biol. Ecol. 350, 176–193. doi: 10.1016/j.jembe.2007.06.017
Reed, D. H., and Frankham, R. (2003). Correlation between fitness and genetic diversity. Conserv. Biol. 17, 230–237. doi: 10.1046/j.1523-1739.2003.01236.x
Renzi, J. J., Qiang, H., and Silliman, B. R. (2019). Harnessing positive species interactions to enhance coastal wetland restoration. Front. Ecol. Evol. 7:131. doi: 10.3389/fevo.2019.00131
Reusch, T. B. H., Chapman, A. R. O., and Groger, J. P. (1994). Blue mussels Mytilus edulis do not interfere with eelgrass Zostera marina but fertilize shoot growth through biodeposition. Mar. Ecol. Prog. Ser. 108, 265–282. doi: 10.3354/meps108265
Reynolds, L. K., McGlathery, K. J., and Waycott, M. (2012). Genetic diversity enhances restoration success by augmenting ecosystem services. PLoS One 7:e38397. doi: 10.1371/journal.pone.0038397
Rose, C. D., and Dawes, C. J. (1999). Effects of community structure on the seagrass Thalassia testudinum. Mar. Ecol. Prog. Ser. 184, 83–95. doi: 10.3354/meps184083
Santos, C. B., de los, Krause-Jensen, D., Alcoverro, T., Marbà, N., Duarte, C. M., Van Katwijk, M. M., et al. (2019). Recent trend reversal for declining European seagrass meadows. Nat. Commun. 10:3356. doi: 10.1038/s41467-019-11340-4
Scott, A. L., York, P. H., Duncan, C., Macreadie, P. I., Connolly, R. M., and Ellis, M. T. (2018). The Role of Herbivory in structuring tropical seagrass ecosystem service delivery. Front. Plant Sci. 9:127. doi: 10.3389/fpls.2018.00127
Sheng, X. F., Xia, J. J., Jiang, C. Y., He, L. Y., and Qian, M. (2008). Characterization of heavy metal-resistant endophytic bacteria from rape (Brassica napus) roots and their potential in promoting the growth and lead accumulation of rape. Environ. Pollut. 156, 1164–1170. doi: 10.1016/j.envpol.2008.04.007
Short, F. T., and Wyllie-Echeverria, S. (1996). Natural and human-induced disturbance of seagrasses. Environ. Conserv. 23, 17–27. doi: 10.1017/S0376892900038212
Silberstein, K., Chiffings, A. W., and McComb, A. J. (1986). The loss of seagrass in cockburn sound, Western Australia. III. The effect of epiphytes on productivity of Posidonia australis Hook. F. Aquat. Bot. 24, 355–371. doi: 10.1016/0304-3770(86)90102-6
Silliman, B. R., Bertness, M. D., Altieri, A. H., Griffin, J. N., Bazterrica, M. C., Hidalgo, F. J., et al. (2011). Whole-community facilitation regulates biodiversity on Patagonian rocky shores. PLoS One 6:e24502. doi: 10.1371/journal.pone.0024502
Silliman, B. R., Hughes, B. B., Gaskins, L. C., He, Q., Tinker, M. T., Read, A., et al. (2018). Are the Ghosts of Nature’s Past Haunting Ecology Today?” Curr. Biol. 28, R532–R537. doi: 10.1016/j.cub.2018.04.002
Silliman, B. R., Schrack, E., He, Q., Cope, R., Santoni, R., van der Heide, T., et al. (2015). Facilitation shifts paradigms and can amplify coastal restoration efforts. Proc. Natl. Acad. Sci. U.S.A. 112, 14295–14300. doi: 10.1073/pnas.1515297112
Statton, J., Dixon, K. W., Irving, A. D., Jackson, E. L., Kendrick, G. A., Orth, R. J., et al. (2018). “Decline and restoration ecology of Australian seagrasses,” in Seagrasses of Australia, eds A. W. D. Larkum, G. A. Kendrick, and P. J. Ralph, (Cham: Springer), 665–704. doi: 10.1007/978-3-319-71354-0_20
Statton, J., Kendrick, G. A., Dixon, K. W., and Cambridge, M. L. (2014). Inorganic nutrient supplements constrain restoration potential of seedlings of the seagrass, Posidonia australis. Restor. Ecol. 22, 196–203. doi: 10.1111/rec.12072
Tanner, J. E. (2015). Restoration of the seagrass Amphibolis antarctica—temporal variability and long-term success. Estuaries Coast. 38, 668–678. doi: 10.1007/s12237-014-9823-4
Tarquinio, F., Hyndes, G. A., Laverock, B., Koenders, A., and Säwström, C. (2019). The seagrass holobiont: understanding seagrass-bacteria interactions and their role in seagrass ecosystem functioning. FEMS Microbiol. Lett. 366:fnz057. doi: 10.1093/femsle/fnz057
Thomsen, M. S., Altieri, A. H., Angelini, C., Bishop, M. J., Gribben, P. E., Lear, G., et al. (2018). Secondary foundation species enhance biodiversity. Nat. Ecol. Evol. 2, 634–639. doi: 10.1038/s41559-018-0487-5
Tol, S. J., Jarvis, J. C., York, P. H., Grech, A., Congdon, B. C., and Coles, R. G. (2017). Long distance biotic dispersal of tropical seagrass seeds by marine mega-herbivores. Sci. Rep. 7, 1–8. doi: 10.1038/s41598-017-04421-4421
Tutar, O., Marín-Guirao, L., Ruiz, J. M., and Procaccini, G. (2017). Antioxidant response to heat stress in seagrasses. A gene expression study. Mar. Environ. Res. 132, 94–102. doi: 10.1016/j.marenvres.2017.10.011
Valiela, I., and Cole, M. L. (2002). Comparative evidence that salt marshes and mangroves may protect seagrass meadows from land-derived nitrogen loads. Ecosystems 5, 92–102. doi: 10.1007/s10021-001-0058-4
van de Koppel, J., van der Heide, T., Altieri, A. H., Eriksson, B. K., Bouma, T. J., Olff, H., et al. (2015). Long-distance interactions regulate the structure and resilience of coastal ecosystems. Ann. Rev. Mar. Sci. 7, 139–158. doi: 10.1146/annurev-marine-010814-015805
Van der Heide, T., van Govers, L. L., de Fouw, J., Olff, H., van der Geest, M., Van Katwijk, M. M., et al. (2012). A three-stage symbiosis forms the foundation of seagrass ecosystems. Science 336, 1432–1434. doi: 10.1126/science.1219973
Van der Heide, T., van Nes, E. H., van Geerling, G. W., Smolders, A. J. P., Bouma, T. J., and Van Katwijk, M. M. (2007). Positive feedbacks in seagrass ecosystems: implications for success in conservation and restoration. Ecosystems 10, 1311–1322. doi: 10.1007/s10021-007-9099-7
van Katwijk, M. M., Bos, A. R., de Jonge, V. N., Hanssen, L. S. A. M., Hermus, D. C. R., and de Jong, D. J. (2009). Guidelines for seagrass restoration: importance of habitat selection and donor population, spreading of risks, and ecosystem engineering effects. Mar. Pollut. Bull. 58, 179–188. doi: 10.1016/j.marpolbul.2008.09.028
van Katwijk, M. M., van Thorhaug, A., Marbà, N., Orth, R. J., Duarte, C. M., Kendrick, G. A., et al. (2016). Global analysis of seagrass restoration: the importance of large-scale planting. J. Appl. Ecol. 53, 567–578. doi: 10.1111/1365-2664.12562
van Tussenbroek, B. I., Soissons, L. M., Bouma, T. J., Asmus, R., Auby, I., Brun, F. G., et al. (2016). Pollen limitation may be a common Allee effect in marine hydrophilous plants: implications for decline and recovery in seagrasses. Oecologia 182, 595–609. doi: 10.1007/s00442-016-3665-7
Venkatachalam, A., Thirunavukkarasu, N., and Suryanarayanan, T. S. (2015). Distribution and diversity of endophytes in seagrasses. Fungal Ecol. 13, 60–65. doi: 10.1016/j.funeco.2014.07.003
Vessey, J. K. (2003). Plant growth promoting rhizobacteria as biofertilizers. Plant Soil 255, 571–586. doi: 10.1023/A:1026037216893
Welsh, D. T. (2000). Nitrogen fixation in seagrass meadows: regulation, plant-bacteria interactions and significance to primary productivity. Ecol. Lett. 3, 58–71. doi: 10.1046/j.1461-0248.2000.00111.x
Wernberg, T., Vanderklift, M. A., How, J., and Lavery, P. S. (2006). Export of detached macroalgae from reefs to adjacent seagrass beds. Oecologia 147, 692–701. doi: 10.1007/s00442-005-0318-7
Williams, S. L. (1987). Competition between the seagrasses Thalassia testudinum and Syringodium filiforme in a Caribbean lagoon. Mar. Ecol. Prog. Ser. 35, 91–98. doi: 10.3354/meps035091
Williams, S. L. (1990). Experimental studies of caribbean seagrass bed development. Ecol. Monogr. 60, 449–469. doi: 10.2307/1943015
Williams, S. L. (2001). Reduced genetic diversity in eelgrass transplantations affects both population growth and individual fitness. Ecol. Appl. 11, 1472–1488. doi: 10.1890/1051-0761(2001)011
Williams, S. L., Ambo-Rappe, R., Sur, C., Abbott, J. M., and Limbong, S. R. (2017). Species richness accelerates marine ecosystem restoration in the coral triangle. Proc. Natl. Acad. Sci. U.S.A. 114, 11986–11991. doi: 10.1073/pnas.1707962114
Worm, B., and Reusch, T. B. H. (2000). Do nutrient availability and plant density limit seagrass colonization in the Baltic sea?” Mar. Ecol. Prog. Ser. 200, 159–166. doi: 10.3354/meps200159
Yap, H. T. (2000). The case for restoration of tropical coastal ecosystems. Ocean Coast. Manag. 43, 841–851. doi: 10.1016/S0964-5691(00)00061-2
Zhang, Y. S., Cioffi, W., Cope, R., Daleo, P., Heywood, E., Hoyt, C., et al. (2018). A global synthesis reveals gaps in coastal habitat restoration research. Sustainability 10:1040. doi: 10.3390/su10041040
Keywords: coastal management, facilitation, positive species interactions, seagrass restoration, seagrass
Citation: Valdez SR, Zhang YS, van der Heide T, Vanderklift MA, Tarquinio F, Orth RJ and Silliman BR (2020) Positive Ecological Interactions and the Success of Seagrass Restoration. Front. Mar. Sci. 7:91. doi: 10.3389/fmars.2020.00091
Received: 13 September 2019; Accepted: 04 February 2020;
Published: 20 February 2020.
Edited by:
Trevor John Willis, Stazione Zoologica Anton Dohrn, ItalyReviewed by:
Ken L. Heck, Dauphin Island Sea Lab, United StatesCopyright © 2020 Valdez, Zhang, van der Heide, Vanderklift, Tarquinio, Orth and Silliman. This is an open-access article distributed under the terms of the Creative Commons Attribution License (CC BY). The use, distribution or reproduction in other forums is permitted, provided the original author(s) and the copyright owner(s) are credited and that the original publication in this journal is cited, in accordance with accepted academic practice. No use, distribution or reproduction is permitted which does not comply with these terms.
*Correspondence: Stephanie R. Valdez, c3RlcGhhbmllLnZhbGRlekBkdWtlLmVkdQ==
Disclaimer: All claims expressed in this article are solely those of the authors and do not necessarily represent those of their affiliated organizations, or those of the publisher, the editors and the reviewers. Any product that may be evaluated in this article or claim that may be made by its manufacturer is not guaranteed or endorsed by the publisher.
Research integrity at Frontiers
Learn more about the work of our research integrity team to safeguard the quality of each article we publish.