- 1Department of Biology, Temple University, Philadelphia, PA, United States
- 2Department of Biology, Boston University, Boston, MA, United States
- 3Woods Hole Oceanographic Institution, Woods Hole, MA, United States
The Phoenix Islands Protected Area, in the central Pacific waters of the Republic of Kiribati, is a model for large marine protected area (MPA) development and maintenance, but baseline records of the protected biodiversity in its largest environment, the deep sea (>200 m), have not yet been determined. In general, the equatorial central Pacific lacks biogeographic perspective on deep-sea benthic communities compared to more well-studied regions of the North and South Pacific Ocean. In 2017, explorations by the NOAA ship Okeanos Explorer and R/V Falkor were among the first to document the diversity and distribution of deep-water benthic megafauna on numerous seamounts, islands, shallow coral reef banks, and atolls in the region. Here, we present baseline deep-sea coral species distribution and community assembly patterns within the Scleractinia, Octocorallia, Antipatharia, and Zoantharia with respect to different seafloor features and abiotic environmental variables across bathyal depths (200–2500 m). Remotely operated vehicle (ROV) transects were performed on 17 features throughout the Phoenix Islands and Tokelau Ridge Seamounts resulting in the observation of 12,828 deep-water corals and 167 identifiable morphospecies. Anthozoan assemblages were largely octocoral-dominated consisting of 78% of all observations with seamounts having a greater number of observed morphospecies compared to other feature types. Overlying water masses were observed to have significant effects on community assembly across bathyal depths. Revised species inventories further suggest that the protected area it is an area of biogeographic overlap for Pacific deep-water corals, containing species observed across bathyal provinces in the North Pacific, Southwest Pacific, and Western Pacific. These results underscore significant geographic and environmental complexity associated with deep-sea coral communities that remain in under-characterized in the equatorial central Pacific, but also highlight the additional efforts that need to be brought forth to effectively establish baseline ecological metrics in data deficient bathyal provinces.
Introduction
Established in 2008, and later closed to commercial fishing in 2015, the Phoenix Islands Protected Area (PIPA) is among the largest (408,250 km2) closed marine protected areas (MPA) and the deepest UNESCO world heritage site on Earth (Claudino-Sales, 2019). Despite these designations, the deep-sea benthos below 200 m in this MPA remained vastly unexplored hindering the full understanding of what habitats the protected area encompasses. In 2017, several deep-sea exploration expeditions passed through PIPA on the NOAA ship Okeanos Explorer, as a part of the CAPSTONE (Campaign to Address Pacific monument Science, Technology, and Ocean Needs) program (Demopoulos et al., 2018), and on the R/V Falkor with the goal of enhancing understanding of deep-sea ecosystems in the MPA via international partnerships (McKinnie et al., 2018; Mangubhai et al., 2019).
Deep-sea exploration has been an on-going priority for PIPA, starting with the first formal research plan (Rotjan and Obura, 2010), and continuing in the first and second PIPA management plans. The earliest deep-sea surveys consisted of baited drop cameras deployed to observed fish communities below 1000 m depth (Obura et al., 2011). Recent expeditions contributed high-definition multibeam mapping and seafloor exploration (Kennedy et al., 2019), which also helps to better define and manage the features that lie within protected boundaries. A United Nations voluntary commitment stemming from the Oceans Sustainable Development Goals (SDG14) was recently written by the PIPA Scientific Advisory Committee, with the specific goal to increase scientific knowledge and research capacity to improve ocean health in Kiribati. Deep-sea exploration in PIPA directly helps to fulfill these goals of increased knowledge and research capacity, as well as advancing the biodiversity inventory within PIPA boundaries.
The deep slopes of islands, atolls, reef-capped shallow banks (herein referred to as reefs), and seamounts comprise the majority of high-profile seafloor relief in PIPA with at least two major geographic seamount or island clusters, the Tokelau Seamount Chain and Phoenix Island cluster, occurring entirely within or passing through its boundaries (Figure 1). Globally, seamounts are among the most extensive biomes in the Pacific Ocean with only a small percentage having been explored worldwide (Etnoyer et al., 2010). While seamounts are abundant deep-sea features, particularly in the western and central Pacific Ocean, exploration of these features and characterization of seafloor communities at bathyal depths are lacking in the region (Watling et al., 2013; Bohnenstiehl et al., 2018; Cantwell et al., 2018; Demopoulos et al., 2018; Herrera et al., 2018; Kelley et al., 2018). Deep-sea corals, primarily from the Octocorallia (soft corals), Scleractinia (stony corals), and Antipatharia (black corals), represent some of the most common groups observed on seamounts studied worldwide (Rogers et al., 2007), making them a useful group of taxa for understanding how the oceanographic and physical environment influences benthic communities across a range of spatial scales. Structures produced by deep-water corals provide important vertical relief for associated species in deep-sea ecosystems (Buhl-Mortensen et al., 2010), in addition to supporting unique symbioses with invertebrate fauna (Mosher and Watling, 2009). Benthic invertebrate and demersal fish communities on seamounts have been reported to be strongly influenced by variables including local water column productivity, overlying water masses, depth, substrate type, and topographic variability (Genin et al., 1986; Auster et al., 2005; Clark et al., 2010; Shank, 2010; Quattrini et al., 2017; Rogers, 2018).
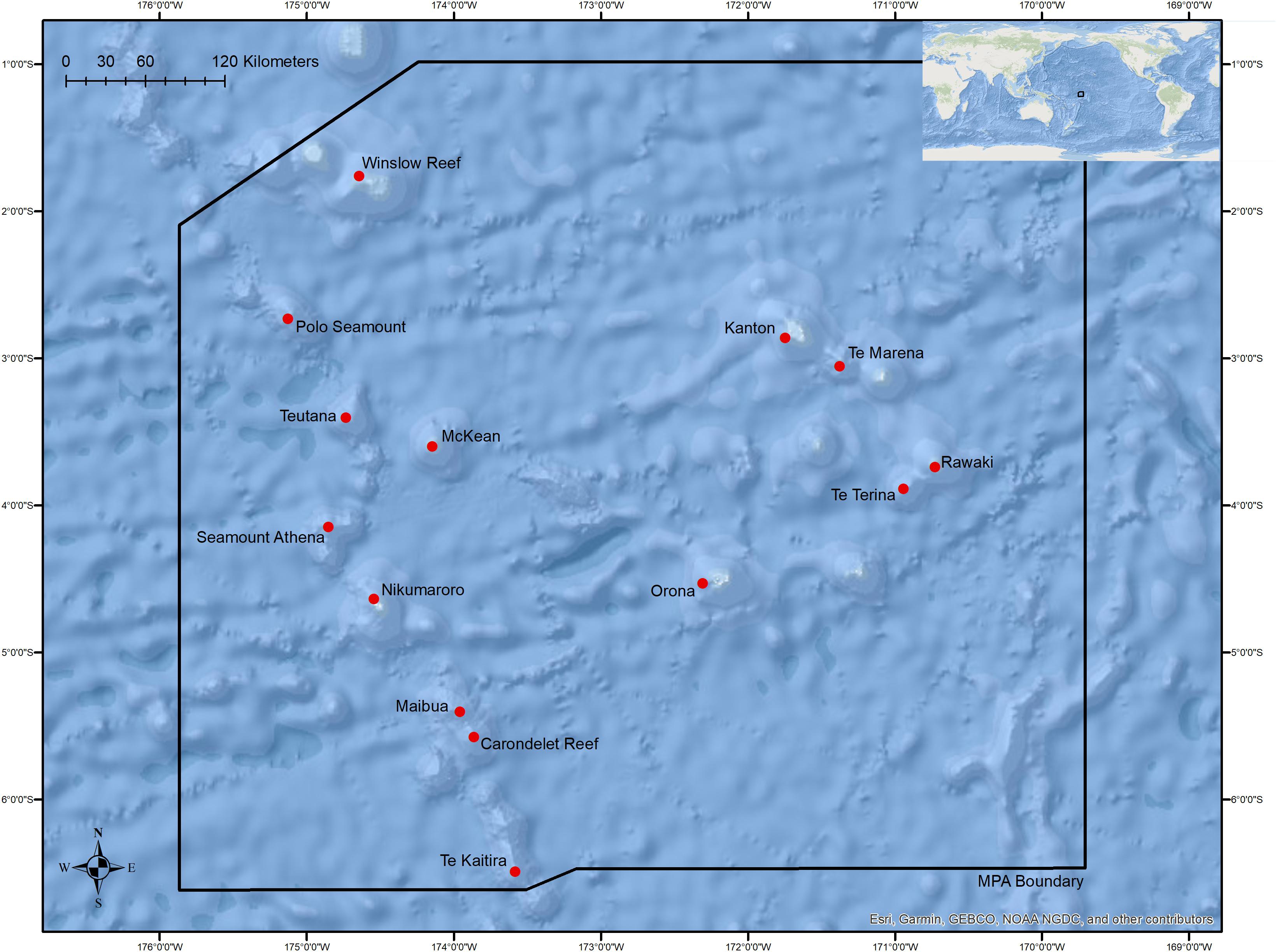
Figure 1. Overview of the boundaries of the Phoenix Islands Protected Area with locations of sites where dives were conducted in this study. Table 1 and Supplementary Figure S1 provide detailed dive site names, coordinates, and depth ranges.
The exploration and exploitation of mineral resources from seafloor crusts and the impact to benthic fauna have become an increasing regional concern given the poor state of knowledge of deep-sea biodiversity (Miller et al., 2018). World-wide, seamounts have been found to harbor benthic communities that are vulnerable to a range of disturbances and are often slow to recover to their pre-disturbed state (Watling and Norse, 1998; Williams et al., 2010; Watling and Auster, 2017). In the central Pacific, cobalt-rich mineral crusts occur on seamounts at depths that coincide with those of deep-water coral and sponge communities (Morgan et al., 2015). Such species, which are characteristically slow-growing and long-lived, stand to be disrupted should such activities occur and result in physical disturbance and increased sedimentation rates within and around affected areas (Gollner et al., 2017). Identifying and quantifying species diversity within deep-water coral communities on features throughout the Pacific that exhibit a range of geomorphological characteristics (e.g., guyots, sharp-peaked seamounts, as well as on the flanks of atolls and islands) is essential to properly manage these species and communities.
This study provides insight into the composition, diversity, and patterns among coral communities at bathyal depths in the Phoenix Islands Protected Area. Using ROV-collected high-resolution video and collections-based approaches, we provide the first deep-sea coral species inventory within PIPA boundaries. Additionally, we test two exploratory hypotheses designed to identify broader differences in community similarity between geographic features and across depth. The first being, do different feature types like islands, atolls, reefs, or seamounts harbor distinct deep-water assemblages within the protected area? Second, does vertical structure of the water column (i.e., water masses) result in differences in assemblages across depth? If so, which species might contribute most to the observed differences? And finally, which oceanographic environmental variables most strongly influence the observed variation in community structure at bathyal depths?
Due to the geographic remoteness of these features and the protected status of the area, we suggest that deep-water communities within PIPA are likely to rank among the least human-impacted environments in the deep Pacific Ocean and act as a foundation for comparison to other unprotected deep-sea features in the greater Central Pacific. The results of this work will help to set a much-needed baseline understanding of large remote marine reserves, specifically with respect to identifying abundant and diverse deep-sea communities and ensuring their future conservation.
Materials And Methods
Data for this study were collected from two expeditions to the Phoenix Islands Protected Area in March and October 2017 (Table 1). In March, the NOAA Ship Okeanos Explorer (ROV Deep Discoverer) surveyed and explored the western portion of PIPA along the Tokelau Ridge seamounts; in October, the R/V Falkor (ROV Subastian) focused on islands, atolls, and seamounts in the eastern Phoenix Islands, as well as additional dives along the Tokelau seamount chain. Both expeditions utilized remotely operated vehicle (ROV) systems to survey and characterize deep-water coral communities in the area. ROV Deep Discoverer completed 7 transects along 7 features on the Tokelau Seamount Chain and the ROV Subastian completed 17 transects across 10 features throughout the Phoenix Islands, Phoenix Seamounts, and Tokelau Seamounts (Supplementary Figure S1).
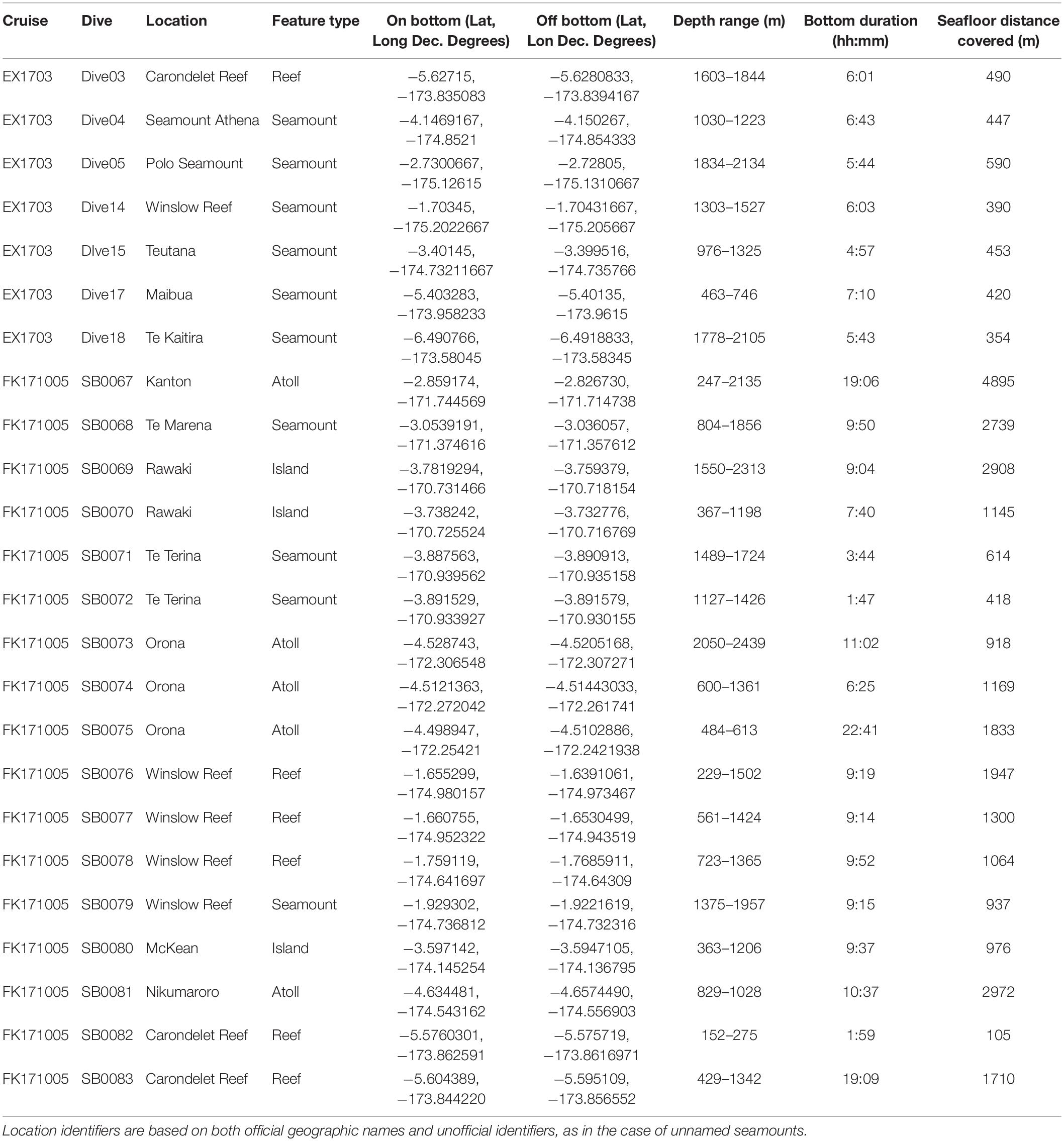
Table 1. Summary of dives conducted at seamounts, islands, atolls, and reefs during EX1703 and FK171005.
Seafloor transects were conducted upslope from deeper to shallower depths across each feature. In each case, vehicles moved upslope, perpendicular to the contours of the slope, with only occasional short zooms to confirm identifications. Motion across the seafloor occurred at an average of 0.25 kt. Video segments on the seafloor spent sampling, during long duration zooming, not in forward motion, or otherwise not in visual contact with the seabed was not included in analyses. Collection opportunities were utilized to obtain voucher specimens for further identification of coral morphospecies (Supplementary Table S1).
ROV Video Analysis
Video segments where collections were made, where the vehicle was more than 3 m off bottom, moving backwards, during sampling events, or of generally poor quality, were not assessed. Individuals or colonies were identifiable above a 5 cm height threshold, with measurements based on 10 cm wide parallel lasers in the ROV field of view. If colonies or individuals were below this size threshold, they could not be consistently identified and were omitted from analysis. Each occurrence was associated with a UTC time-stamp, and this was used to associate each observation with in situ environmental data including latitude-longitude coordinates, temperature, depth, dissolved oxygen concentration, and salinity.
Video was analyzed for coral occurrences from four major anthozoan taxonomic groups: the Scleractinia, Octocorallia, Zoantharia, and Antipatharia. Individuals or colonies were identified to the lowest taxonomic level possible or assigned morphospecies (msp.) identifiers. Only visibly live colonies were counted. If particular colonies could not be identified reliably to such a level, they were placed in family or genus-level groupings (i.e., family spp. or genus spp.). Observations with any uncertainty were flagged and underwent quality control by examining and omitting the potentially erroneous identifications. Final identification of coral species, using both photographic and voucher specimens, were made using published taxonomic keys, and assistance from taxonomic specialists.
Community Analyses
Deep-water coral patterns were assessed by dividing each of the 24 dive transects into 100 m depth bins. Each bin provided a sampling that consisted of all coral morphospecies observations occurring within that 100 m depth interval. Community-level analyses were conducted using standardized and 4th root transformed species abundance data in a resemblance matrix in PRIMER v7 with PERMANOVA add-on (Clarke and Gorley, 2006; Anderson et al., 2008). In all, 153 samples were identified across 24 dives (Supplementary Table S2). Samples were compiled into a Bray-Curtis similarity resemblance matrix for further analyses. Cluster analysis and non-metric multidimensional scaling ordinations (nMDS) were initially conducted to identify associations between groups of samples. For these analyses only, in order to reduce the effect of noise in the dataset, species abundances were averaged across samples in 100 m depth bins resulting in one data point for each depth stratum. The significance of associations between sample clusters were evaluated using the similarity profile (SIMPROF) routine (Clarke et al., 2008).
Before conducting assessments of similarity in communities, adequacy of sampling effort and species richness comparisons were evaluated using permuted sample-based species accumulation and individual-based rarefaction for feature types and water masses in Vegan 2.5-3 in R (Oksanen et al., 2015). In order to test for similarities among coral assemblages between factors, analysis of similarity (ANOSIM) tests were employed. The first comparison tested differences between feature types while controlling for the effect of depth differences using a two-way nested ANOSIM. A one-way ANOSIM test was also used to determine if differences could be identified between assemblages bathed by different water masses. All ANOSIM tests were conducted using 999 random permutations.
In order to incorporate environmental data for each sample, an average of depth, temperature, salinity, dissolved oxygen was calculated in each 100 m sample. The mean was then normalized within each variable to reduce the effects of differences in unit scales. The BEST routine (BIO-ENV) was employed to explore correlations between biological observations and environmental predictors. A distance-based linear model (distLM) was applied using the PERMANOVA package and utilized the Akaike Information Criterion (AIC) to identify environmental variables with strong explanatory power for observed variation within samples (Anderson et al., 2008). Visualizations of the resemblance matrix with predictor variables were paired with a dbRDA (distance-based redundancy analysis) ordination with distLM overlay.
Results
ROV Video Analysis Summary
Across both cruises, 212 h and 42 min of video were assessed from 24 dives, 7 dives from EX1703 and 17 dives from FK171005 (Table 1). A preliminary comparison revealed no significant difference between cruises due to methodology and thus both datasets were treated as one (two-way ANOSIM between cruises (depth within cruise), Global R = 0.018, p = 0.292). Dives spanned the depths of 152–2439 m with deep-water corals being recorded across the depths of 192–2438 m (Figure 2). In total, 12,828 observations of deep-water corals were identified with 10,995 of those being assigned a morphospecies (msp.) or species-level identifier (Supplementary Table S1). The remaining 1833 records fell into coarser identification groupings at the genus or family level. All corals were identified and categorized using consistent morphospecies identifications from the Octocorallia, Antipatharia, Scleractinia, and Zoantharia. From these groups, 167 unique morphospecies identifiers were assigned based on visual identification, morphological identification from voucher collections, or a combination of those methods (Table 2).
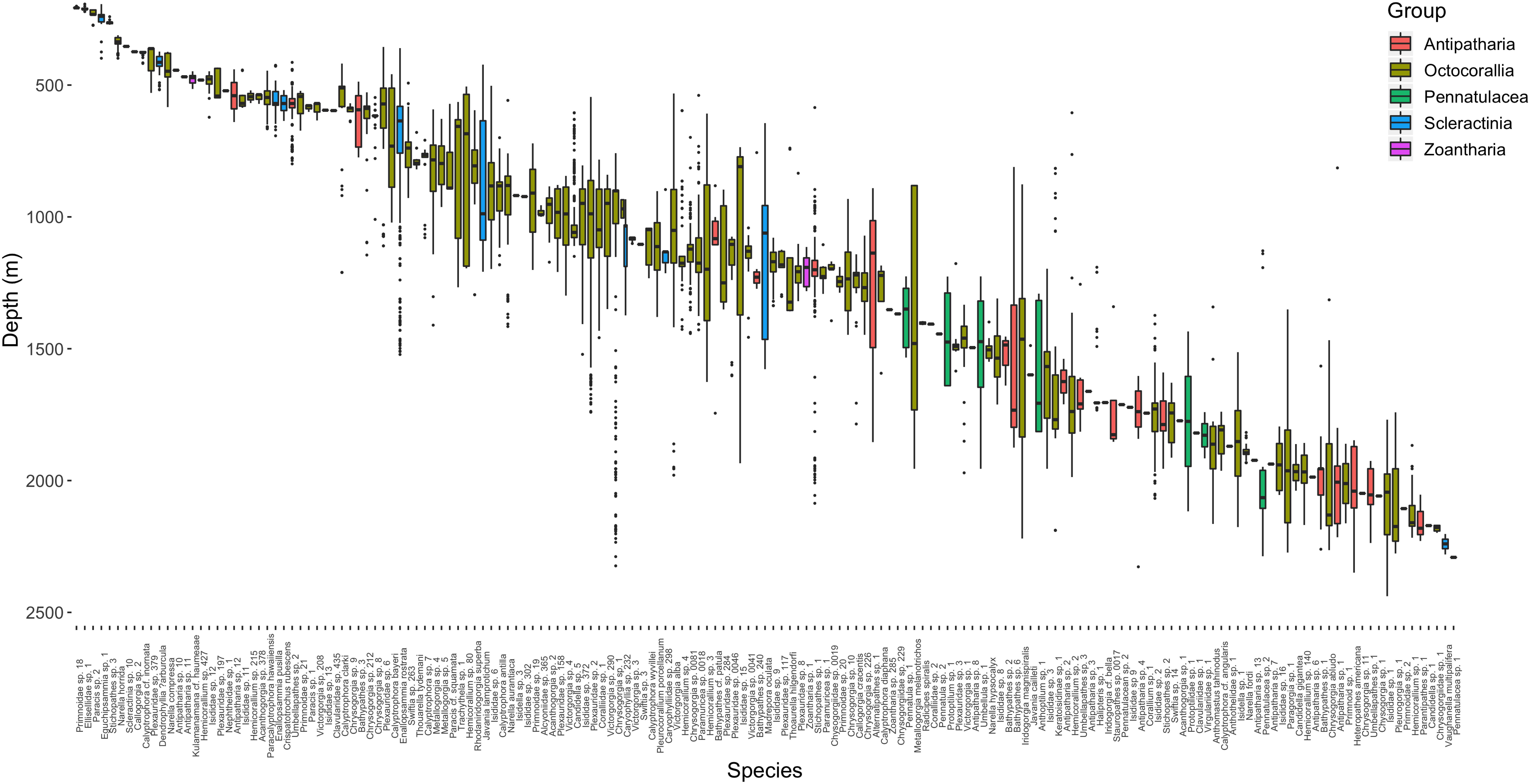
Figure 2. Depth distribution of 167 morphospecies observed from video analyses within PIPA. Boxes are colored according to taxonomic groupings and are arranged by increasing median depth of occurrence.
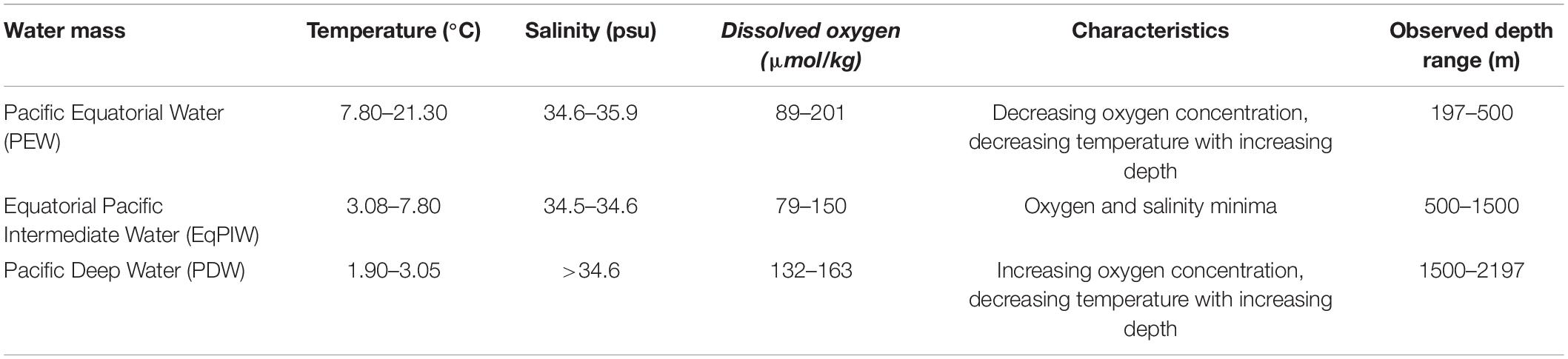
Table 2. Water masses and environmental characteristics observed in the Phoenix Islands Protected Area during cruises FK171005 and EX1703.
Oceanographic Summary
In order to determine water mass structure in the area, downcast shipboard CTD profiles of the upper 3000 m of the water column were examined for temperature and salinity changes across depth (Figure 3). Using these characteristics, major deep-ocean water masses present in this area were generally defined as Pacific Equatorial Water (PEW) between 200 and 500 m, Equatorial Pacific Intermediate Water (EqPIW) between 500 and 1500 m, and Pacific Deep Water (PDW) below 1500 m (Table 2). Temperature, salinity, and dissolved oxygen ranges for each water mass were consistent with accepted ranges from published records in the equatorial Pacific (Bostock et al., 2010).
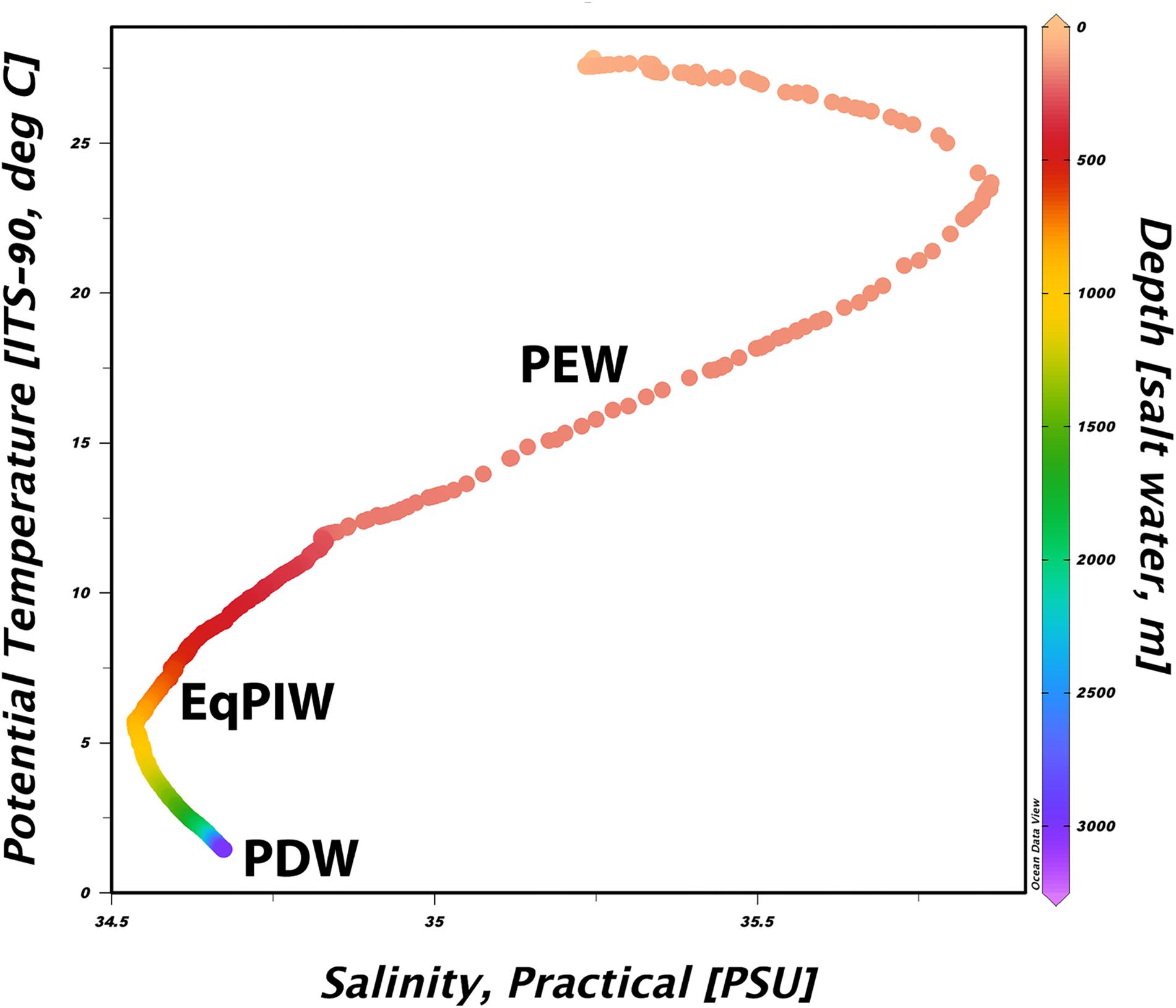
Figure 3. Temperature-salinity diagram from the sea surface to 3000 m collected via CTD rosette downcast North of Kanton Atoll. The approximate depth of each water mass identified below 200 m is overlaid in bold. Plot was constructed using Ocean Data View v5.1 (Schlitzer, 2019).
Environmental variables from ROV-mounted CTD units were in agreement with downcast CTD profiles and provided in situ records at each coral observation (Figure 4). Bottom temperatures overlying coral observations ranged from 1.91 to 21.34°C. Salinity and dissolved oxygen across those same intervals ranged from 34.52 to 35.92 psu and 79.85 to 201.70 μmol/kg, respectively. Below the thermocline at 200–300 m depth, temperature decreased to a minimum of 1.91°C at 2438 m (Figure 4A). Salinity decreased rapidly with depth to a minimum of 34.52 at 800 m, gradually increasing at deeper depths (Figure 4B). A persistent mid-water oxygen minimum of 110–120 μmol/kg was observed between 1000 and 1100 m, but oxygen values in the viscinity of Winslow Reef were reported substantially lower at shallower depths (Figure 4C). The lowest values concurrent with coral observations occurred at Winslow Reef where dissolved oxygen was measured at 79 μmol/kg at 608 m. Low oxygen values (91 μmol/kg) were also identified at Rawaki Island at between 483 and 540 m.
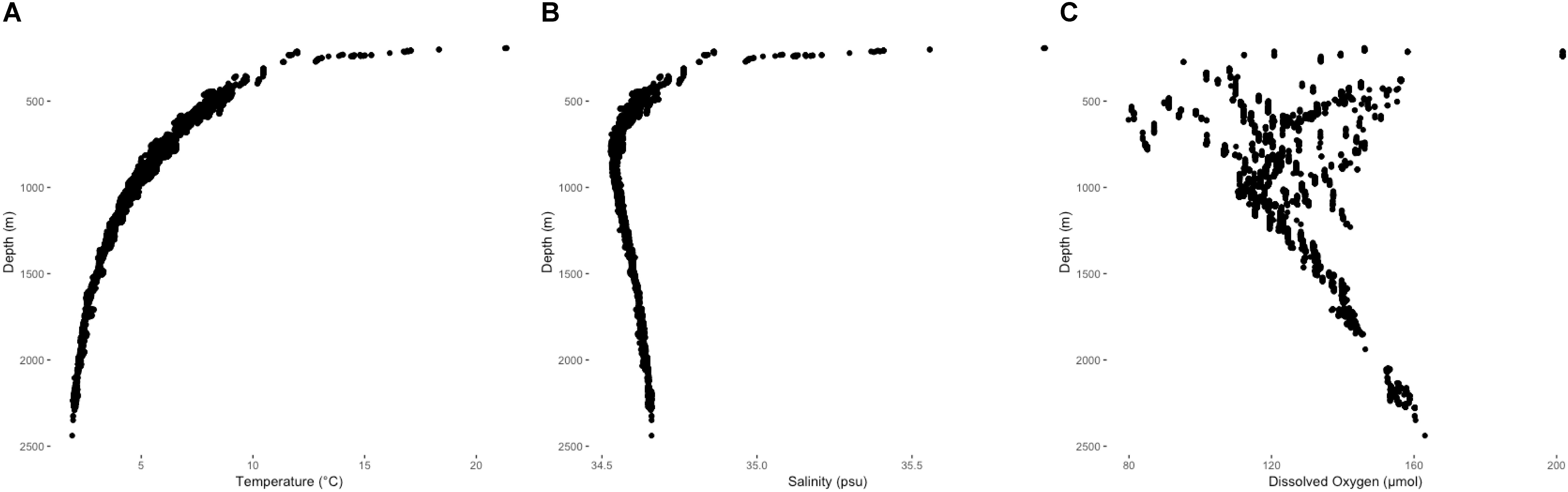
Figure 4. Temperature (A), salinity (B), and dissolved oxygen (C) profiles from ROV CTD unit records co-occurring with deep-water coral observations between 193 and 2439 m.
Coral Distribution Patterns
The dominant taxonomic group across all depths were the octocorals, followed by antipatharians, scleractinians, and finally zoantharians (Table 3). Pennatulaceans were only observed deeper than 1191 m and thus one of the only groups restricted to lower-bathyal depths (Figure 2). Rapid coral morphospecies turnover was observed in the upper 600 m of the water column with most morphospecies in this range occurring across a relatively narrow ranges of depth. The single most abundant coral morphospecies was Pleurogorgia sp. 1, with 1046 colonies observed between 1741 and 2276 m, primarily encountered at high densities on vertical walls or steep inclines.
Octocorals, inclusive of the Pennatulacea, accounted for 78% of all deep-water coral observations. Primnoids were the most speciose as well as the most abundant family in the Octocorallia. With 672 records Candidella sp. 5 (605–1150 m), and Narella aurantiaca with 738 records (558–1416 m) were the dominant primnoid taxa. Chrysogorgiids followed with the two most abundant taxa being Pleurogorgia sp. 1 and Chrysogorgia sp. 1. Plexaurids were widespread, both geographically and across depth (213–2055 m) but 38% of observations fell into coarser identifications as Plexauridae spp. due to difficulty in identifying distinguishing characters between morphospecies. Plexauridae sp. 2, Plexauridae sp. 6, and Paramuricea sp. 0018 were the most abundant taxa in this family (Supplementary Table S1). Among the 605 bamboo coral colonies observed, Isididae sp. 9 (n = 271), a branched colony, was locally abundant at Te Marena and Orona between the depths of 1079–1336 m. The broadly distributed unbranched Isididae sp. 2 (n = 91) was observed at 7 sites throughout PIPA but only deeper than 1373 m. Precious corals representation was dominated by Pleurocorallium porcellanum Pasternak, 1981, by abundance (n = 179), and Hemicorallium spp. by morphospecies richness, with 8 morphospecies present (Supplementary Table S1). Victorgorgiids were also bathymetrically widespread with 202 observations among 8 morphospecies between the depths of 532 and 1979 m.
Black corals were the second most abundant taxon by number of observed colonies with 1645 records, or 12% of all records. The most abundant morphotype was Stichopathes sp. 1 with 981 records between 585 and 2086 m followed by Umbellapathes sp. 2 with 413 colonies between 414 and 799 m. Bathypathes was the most speciose genus but individual taxa were not very abundant with most morphospecies having 30 or fewer observations (Supplementary Table S1). At least 88 black coral colonies could not be identified to the family level due to low resolution singleton observations, or lack of voucher specimens for reference (Supplementary Table S1).
Scleractinians were most commonly distributed between 600 and 1500 m depth, with only one solitary coral species (Vaughanella multipalifera) present below 2000 m depth (Figure 2). The deepest occurrences of framework-forming colonial scleractinians were Madrepora oculata (n = 45) colonies found between the depths of 644 and 1577 m. The most abundant colonial scleractinian species, Enallopsammia rostrata (n = 462) was found to have a slightly shallower distribution between 359 and 1521 m. A second species in the genus, Enallopsammia pusilla, was found to have a distribution that partially overlapped the shallower range of its congener, between 425 and 692 m. Solitary coral species were the deepest scleractinians observed but most individuals occurred shallower than 650 m. The single most abundant species, Crispatotrochus rubesecens, occurred across a depth range of 519–635 m with other solitary species, Javania cailleti and Javania lamprotichum occurring deeper up to 1599 and 1208 m, respectively (Figure 2).
Patterns in Coral Community Structure
In order to evaluate sampling effort, sample-based species accumulation analyses were conducted by feature type and water mass to evaluate the completeness of species inventories. Among feature types species accumulated at similar rates on seamounts, atolls, reefs, and islands. Overall, seamounts had the largest morphospecies inventory compared to reefs which exhibited the lowest despite similar sample sizes (Figure 5A). Among all feature types, morphospecies accumulation approached asymptotic values signifying a potential to accumulate additional species with increased effort (Figure 5A). Between water masses, morphospecies accumulation rates and numbers of morphospecies observed were highest across the depths bathed by EqPIW (Figure 5B). Lower rates of accumulation and numbers of morphospecies were observed in PDW (76 mspp.) and PEW (30 mspp.). As sample sizes were smaller in PEW and PDW compared to EqPIW, accumulation curves did not approach asymptomatic values and suggest increased morphospecies richness with additional sampling effort in those water masses (Figure 5B).
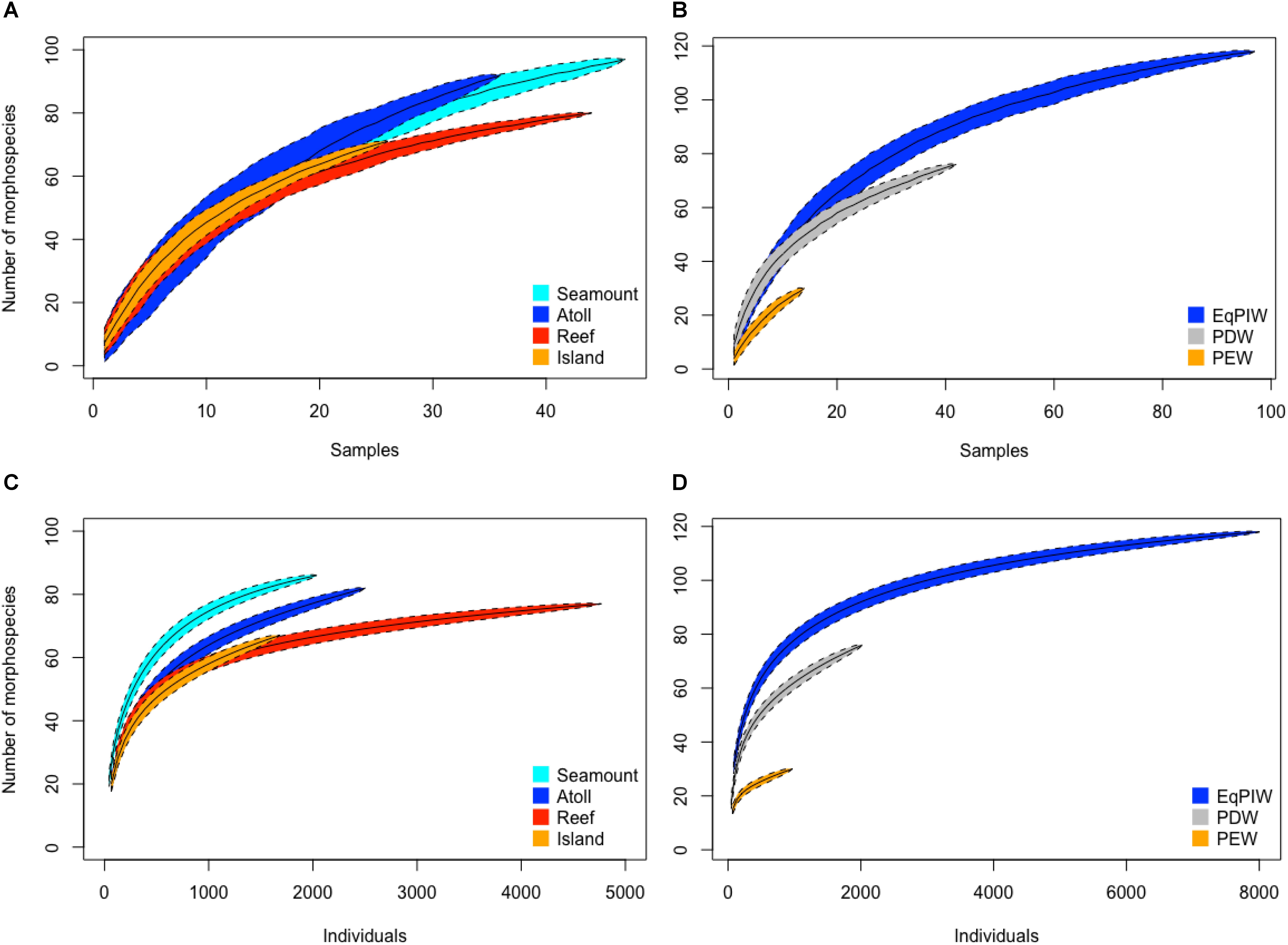
Figure 5. Species accumulation and individual-based rarefaction analyses by feature type and water mass. Sample-based species accumulation curves are presented for feature types (A) and water mass (B). All depths were included for each feature type with N = 26 samples for islands, N = 36 for atolls, N = 47 for seamounts, N = 44 for reefs. For water masses, N = 14 samples were used for the PEW (197–500 m), N = 97 for EqPIW (500–1500 m), and N = 42 for PDW (1500–2197 m). Rarefaction curves are indicated for feature types (C) and water masses (D). Curves are plotted with 95% confidence interval indicated by the colored polygons.
Individual-based rarefaction analyses indicated that the total number of species observed was higher at seamounts (86 mspp.) and atolls (82 mspp.) compared to reefs (77 mspp.) and islands (67 mspp). Greater morphospecies evenness, demonstrated by a rapid increase in morphospecies with increasing number of individuals was also higher on seamounts and atolls compared to reefs and islands (Figure 5C). Overall, shallow-water reef banks accounted for the greatest number of coral observations (Figure 5C). Between water masses, EqPIW was found to have both greater morphospecies richness (118 mspp.) and greater evenness than both PDW and PEW at comparable numbers of individuals (Figure 5D).
Cluster analysis and non-metric multidimensional scaling analyses identified patterns in assemblage structure with depth. Similarities were highest in EqPIW samples and lowest among those in PEW (Figure 6). SIMPROF analysis indicated that the significance of the 8 identified clusters were driven by a close proximity in depth between samples (Figure 6). More SIMPROF groupings were identified encompassing samples covering the depths of EqPIW versus the above or underlying water masses (Figure 7). The most dissimilar samples occurred on both the extreme upper and lower ends of the survey range. Similarities between samples were also found to occur along a clear gradient with increasing sampling depth, except those between 2300 and 2500 m (Figure 7). These two samples were the two deepest depth bins and contained only four combined coral observations (Supplementary Table S2).
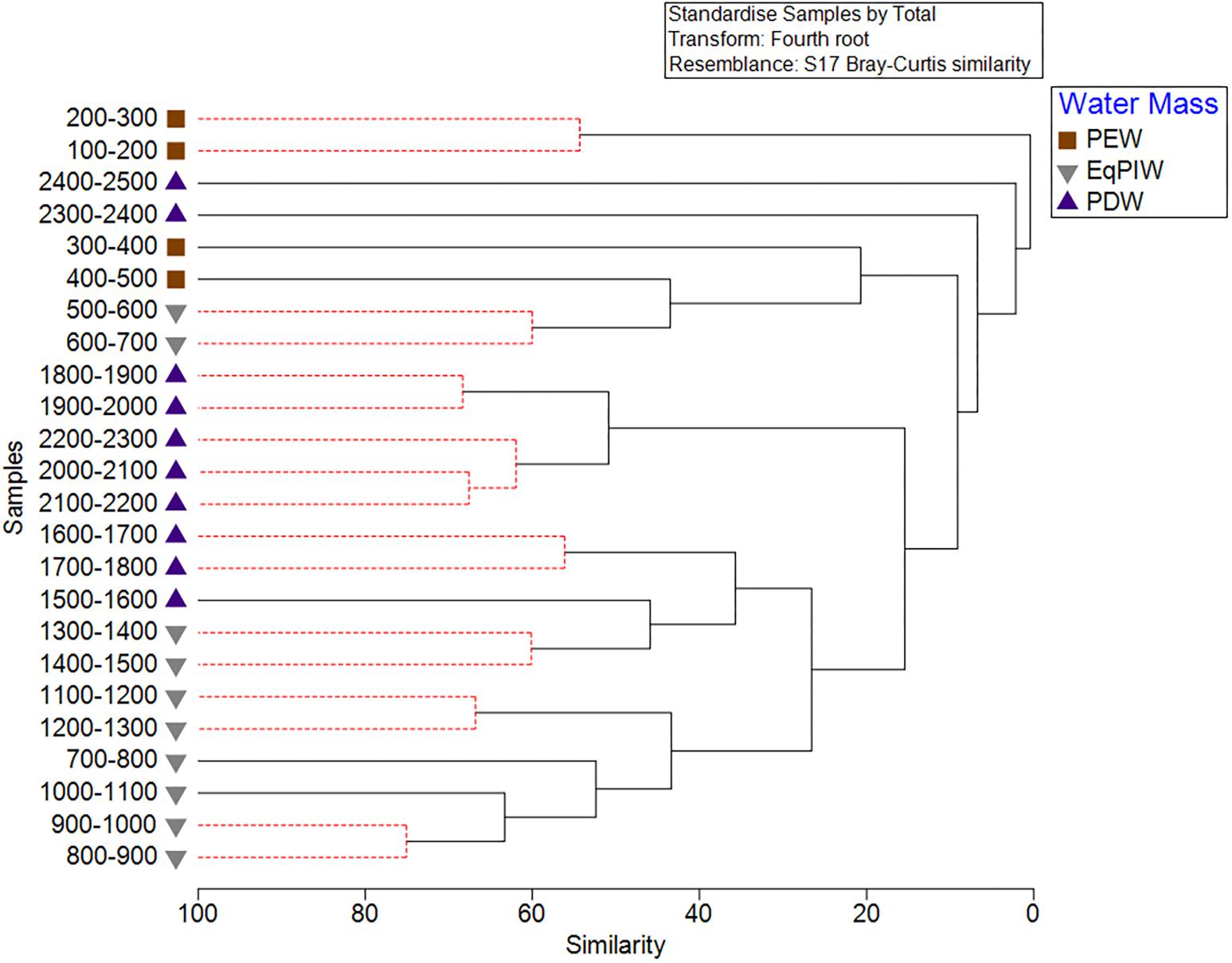
Figure 6. Cluster analysis of 100 m depth-averaged samples. Significant clusters are identified using SIMPROF analysis and are indicated by solid black lines. Symbols indicate the water masses in which those samples occurred.
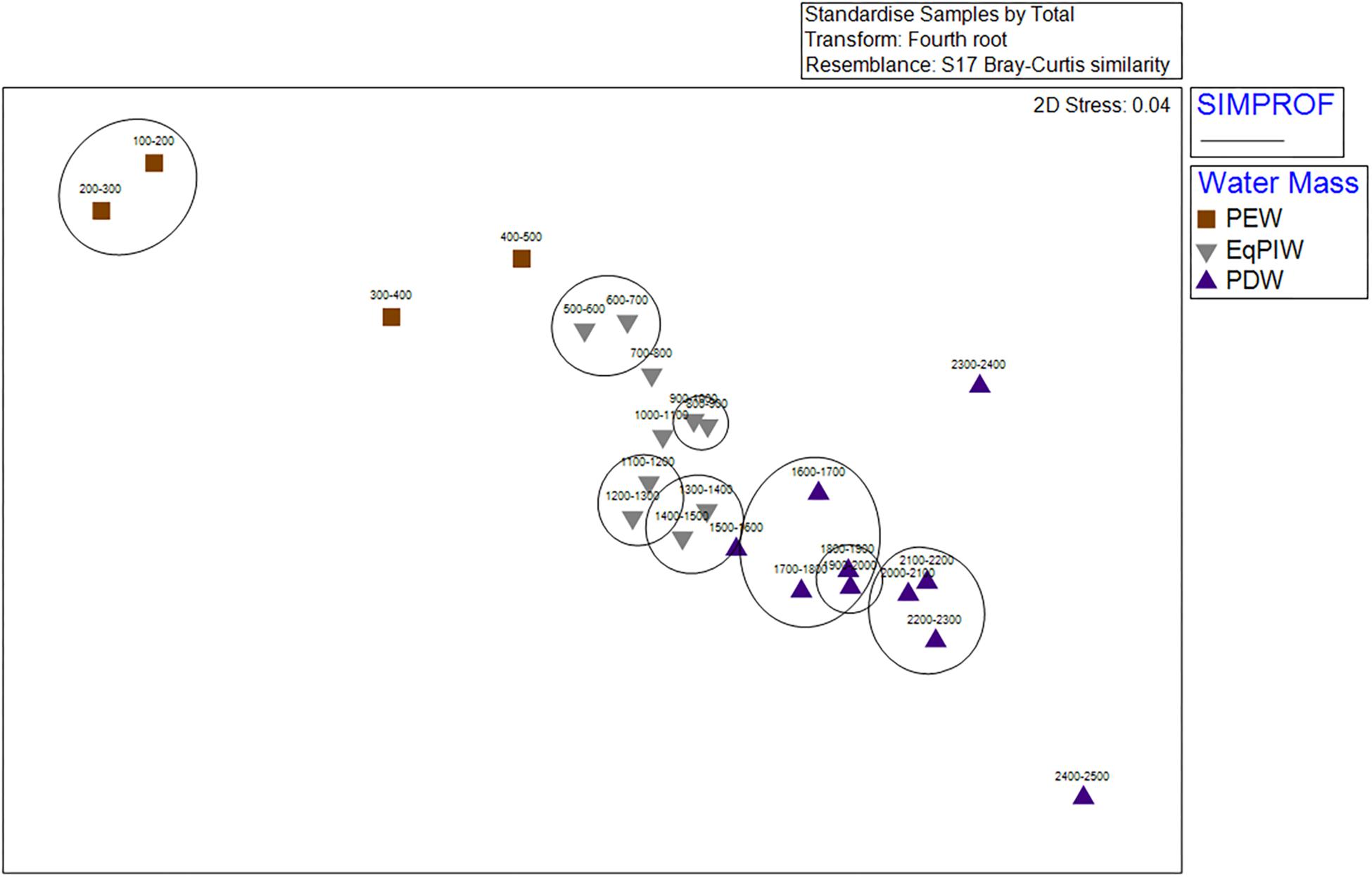
Figure 7. Non-metric multidimensional scaling ordination (nMDS) of 100 m depth-averaged samples. Abundances within samples were 4th root transformed, standardized, and analyses were conducted using a Bray-Curtis Similarity matrix. Symbols indicate the water masses in which those samples occurred. Significant SIMPROF groupings from cluster analysis are overlaid in black.
A two-way nested ANOSIM between feature types (depth within feature type) failed to identify differences in assemblages between most comparisons with the exception of reef-type features (Global R = 0.036, p = 0.096). For reefs, significant differences in assemblages were observed between each other feature, but R-statistic values remained very low indicating a high degree of similarity between samples in those comparisons (Supplementary Table S3). A one-way ANOSIM between water mass factors identified significant and highly dissimilar coral assemblages (Global R = 0.302, p = 0.001) between all 3 bathyal water masses (Supplementary Table S4). The highest dissimilarity was observed between PEW and PDW (R-statistic = 0.619, p = 0.001) and the lowest between EqPIW and PEW (R-statistic = 0.177, p = 0.006).
The similarity percentage (SIMPER) routine identified particular species that contributed a disproportionate influence among significantly different water mass assemblages (Figure 8). Average community similarity increased with increasing water mass depth from PEW (9.68%), to EqPIW (9.8%), to PDW (14.7%). Similarities between samples within the PEW assemblage (200–500 m) were driven by 3 species, Enallopsammia rostrata, Plexauridae sp. 6, and Paracalyptrophora hawaiiensis contributed 58% of the within-group similarity (Figure 8). In EqPIW (500–1500 m), Enallopsammia rostrata, Plexauridae sp. 2, and Iridogorgia magnispiralis contributed to 45% of the similarity in the group (Figure 8). Within PDW (1000–2500 m), the top 3 species Iridogorgia magnispiralis, Chrysogorgia sp. 1, and Pleurocorallium porcellanum contributed to 40% of the observed similarity (Figure 8). Between water masses, the greatest dissimilarity was found between the shallowest and deepest water masses PDW and PEW (99.4% dissimilar). Present in each water mass, differences in the abundance of Enallopsammia rostrata alone was the dominant, single-species contributor (between 4.5 and 7%) of the dissimilarity observed between water masses.
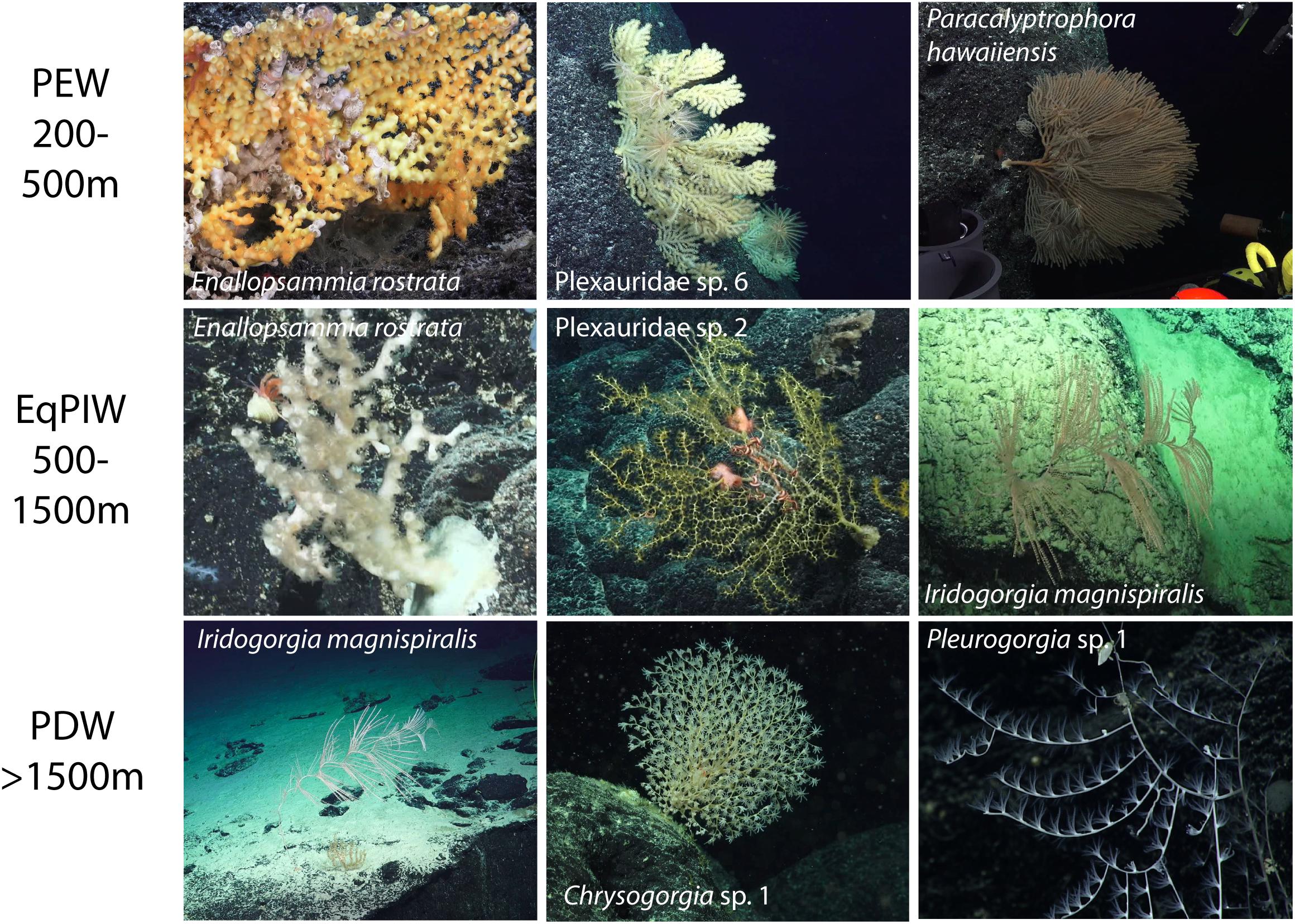
Figure 8. Top 3 morphospecies with disproportionate influence on coral assemblage similarity within each water mass as a result of the SIMPER analysis. Morphospecies on the far left are the largest contributors to within-water mass similarity with influence rank decreasing toward the right.
Results from the BEST (BIO-ENV, Spearman Rank correlation) routine examined which environmental variable or combination of variables provided the best match for the observed variation in community among all samples. Depth and salinity together were found to be the best combination of variables describing resemblances between samples (Global test, 999 permutations, Rho = 0.445, p = 0.001). Yet, together, depth, temperature, salinity, and dissolved oxygen resulted in only a slightly lower correlation (Rho = 0.415). In the distLM and dbRDA analyses, depth, temperature, salinity, and dissolved oxygen were found to explain 7.2% (dbRDA axis 1) and 3.6% (dbRDA axis 2) of the community variation for a total of 10.8% (Figure 9). From the fitted model, 49.2% of the biological variation was correlated with dbRDA axis 1 and 24.9% with axis 2. DbRDA Axis 1 was most strongly correlated with depth (r = -0.773) and dbRDA axis 2 with temperature (r = 0.800) (Supplementary Table S5).
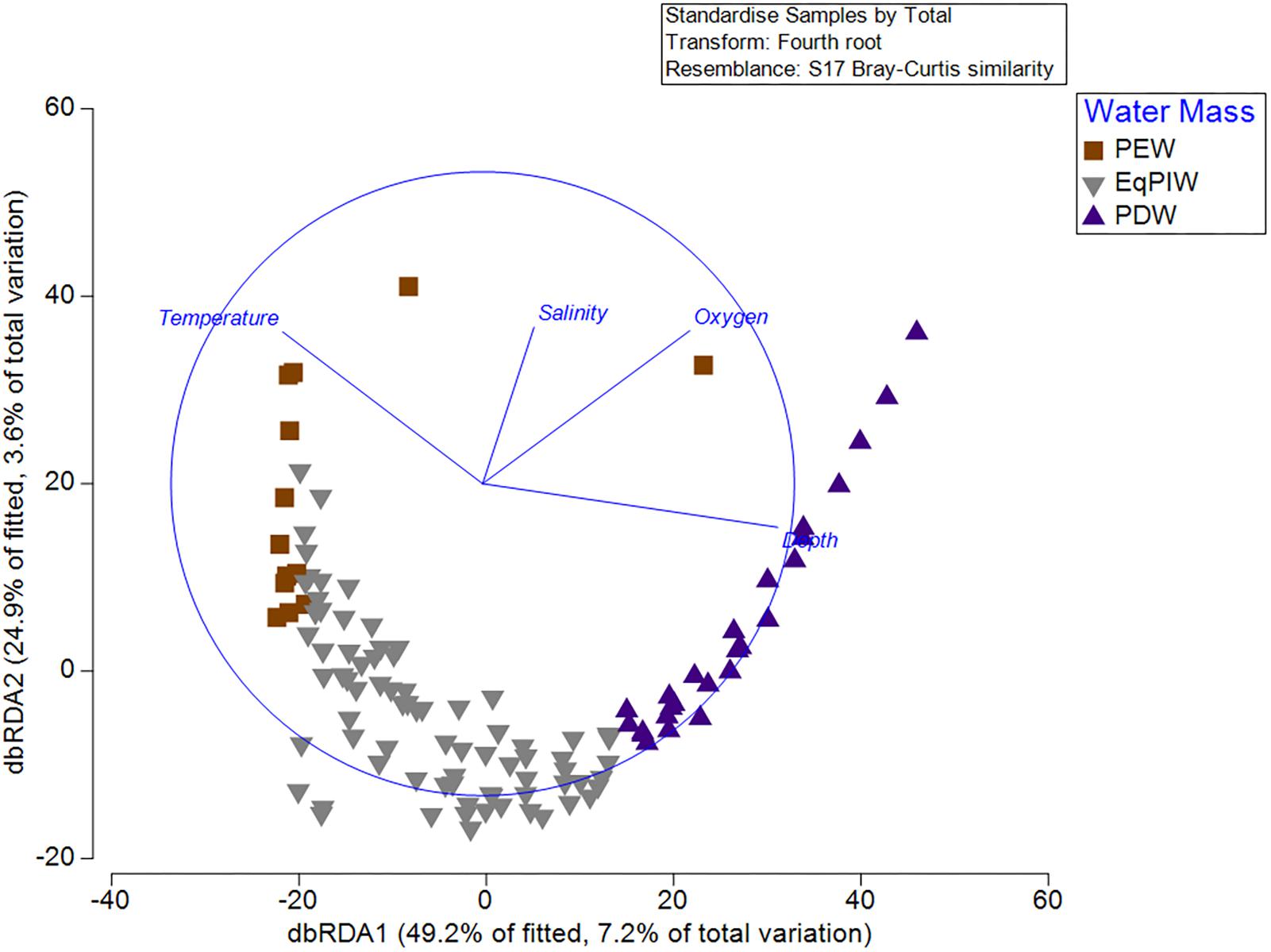
Figure 9. Distance-based redundancy analysis (dbRDA) from standardized and 4th root-transformed species abundances and normalized depth, temperature, salinity, and dissolved oxygen variables. DistLM circle is superimposed in blue with depth, temperature, salinity, and dissolved oxygen vectors. Symbol color and shape denote overlying water mass for each sample.
Discussion
This study provides a significant number of new deep-sea coral species records for a largely unexplored area of the equatorial central Pacific as well as within the boundaries of one of the largest MPAs in the world. Prior to these expeditions in 2017, no published records of any deep-water coral species were available from the Phoenix Islands below 200 m (OBIS, 2019). Over 2 cruises, this study has identified 12,828 deep-sea coral observations and 167 morphospecies at bathyal depths within the boundaries of PIPA from ROV video. From these observations we sought to test two exploratory hypotheses of community assembly in the protected area based on differences in feature type and overlying water masses.
There was no clear evidence of different assemblages across most feature types. While reefs did have some statistically significance differences among assemblages compared to each other feature type, the assemblages themselves were still identified as having high degree of similarity based on low R-statistic (R = 0.01–0.12) values (Supplementary Table S3). In comparison, the three bathyal water masses in the area, Pacific Equatorial Water, Equatorial Pacific Intermediate Water, and Pacific Deep Water were identified as having distinctly different deep-water coral assemblages (Supplementary Table S4), largely dominated by widely distributed cosmopolitan deep-water coral species (Figure 8). Furthermore, intermediate waters were found to have a greater number of morphospecies and greater evenness than shallower and deeper water masses suggesting that they are important pools of biodiversity at bathyal depths (Figure 5D).
Finally, we sought to identify which environmental variables were most important for explaining variation in coral assemblages. Community models and redundancy analyses indicated that depth and temperature were the most important oceanographic variables for explaining biological variation (Figure 9). However, dissolved oxygen and salinity also contributed significantly to the explanation of biological variation in the model (Supplementary Table S5). As a relatively low percentage (10.8%) of the total community variation was explained by oceanographic variables, it is likely that other environmental factors such as seafloor substrate and terrain variables would be useful to include in subsequent models (Supplementary Table S5). From a methodological perspective, both temperature and depth exhibited the greatest correlation between any two input variables (| r| = 0.84). However, it was not deemed necessary to remove either of these two variables due to concerns of collinearity prior to the analysis as they contained sufficiently different predictive information that would permit their inclusion to the model (Anderson et al., 2008). As temperature, salinity, and oxygen are the primary defining characteristics of major water masses (Figure 3), water masses themselves can be useful units for predicting deep-water species occurrences and community structure.
The role that water masses play in structuring biological communities in the deep-sea have been suggested as a major factor influencing species biogeography (Carney, 2005). In this study, differences were found to occur between coral assemblages bathed by PEW, EqPIW, and PDW indicating that oceanographic structure has a significant role in structuring communities. The pattern of within water mass similarities in species occurrence and community composition has been observed across multiple taxa including deep-water corals (Arantes et al., 2009; Miller et al., 2011; Radice et al., 2016), ostrocods (Ayress et al., 2004), and fishes (Quattrini et al., 2017). Furthermore, the boundaries of such water mass boundaries have been identified as important contributors to regional biodiversity in the deep ocean (Victorero et al., 2018). However, the mechanisms of why communities bear strong similarities within water masses are less clear but may include physical retention of planktotrophic larvae within water mass strata or relatively narrow physiological tolerances of species co-occurring within a water mass (Carney, 2005). The relationship between community similarities and water column structure requires further investigation to identify potential mechanisms driving such patterns.
Seamounts comprised the majority of features explored in this study and contained the greatest diversity of coral morphospecies of all feature types examined. Despite accounting for only 24% of all coral observations, seamounts hosted more than half of all morphospecies observed in this study. Diversity patterns on remote seamounts have been found to be strongly driven by species replacement along depth and temperature gradients, as well as topographic variability and along water mass interfaces (Victorero et al., 2018). From a population biogeographic perspective, seamounts may act as critical stepping stones for species distribution and population connectivity in the Pacific Ocean (Miller et al., 2011; Bors et al., 2012; Miller and Gunasekera, 2017). These baseline records of deep-sea coral abundance and diversity on seamounts in PIPA, as well as environmental factors driving community variation, will help generate new hypotheses for understanding benthic biodiversity on remote Pacific seamounts.
Insights to Bathyal Pacific Biogeography
The Phoenix Islands Protected Area lies in an area of the equatorial central Pacific that has been identified as a biodiversity and abundance hotspot for shallow-water marine organisms (Rotjan et al., 2014). At bathyal depths, benthic biodiversity is largely unknown and species inventories of deep-sea corals and sponges are lacking. One recently proposed biogeography of the bathyal regions of the Pacific suggests that the majority of seafloor features in PIPA lay in the BY14 (North Pacific Bathyal) biogeographic province (Watling et al., 2013). The PIPA boundaries also border the BY5 (Southeast Pacific Ridges), BY6 (New Zealand – Kermadec), and BY12 (West Pacific Bathyal) provinces suggesting that this area represents an area of biogeographic overlap, transition, or convergence. Still, further specimen collections and seafloor observations are necessary in refining proposed province boundaries and expanding species inventories within those provinces at bathyal depths.
Several deep-water coral species were found to have widespread geographic distributions in Pacific Ocean, but mechanisms for those observed distribution patterns remain poorly understood. Cosmopolitan deep-water coral taxa including Enallopsammia rostrata, Metallogorgia melanotrichos, Paragorgia spp., Hemicorallium spp., Pleurocorallium sp., and Iridogorgia spp., and several members of the Primnoidae were present throughout multiple sites in the Phoenix Islands at depths below 1000 m providing a biogeographic continuity for species widely reported on seamounts in both the north and south Pacific Ocean (Supplementary Table S1). Some of these same species, like Enallopsammia rostrata and Iridogorgia magnispiralis, and were also found to exhibit a disproportionate effect on assemblage differences between water masses (Figure 8). As a major source of hard substrate, seamounts and seamount chains likely provide pathways for species distribution across equatorial latitudes.
The large geographic extent of Pacific bathyal water masses may also help explain the prevalence of cosmopolitan species in PIPA. In the central and north Pacific below 1500 m, PDW dominates much of the bathyal depth range but across tropical latitudes PDW contributes to the composition of EqPIW south of 20° N (Bostock et al., 2010). Environmental homogeneity and large geographic extent of PDW may provide a mechanism for species to become widely distributed within that water mass throughout the north Pacific Ocean while also extending their range southward via EqPIW. At the same time, mid-bathyal species in the southern hemisphere occurring in Antarctic Intermediate Water (AAIW) may be encountered in equatorial intermediate waters as this water mass contributes to EqPIW north of 20°S (Bostock et al., 2010). At upper bathyal depths, PEW (200–500 m) has been identified as having a well-developed circulation along the equator (Emery, 2001) which could provide a potential distribution mechanism for species among low-latitude seafloor features.
Noteworthy Observations
During both EX1703 and FK171005 expeditions, several collected specimens have been identified as new species and many others represent significant range extensions. Voucher samples were examined alongside records of visual observations to better understand the distribution of these species in their known localities (Supplementary Table S1). Recently, octocorals in the family Primnoidae have been relatively well-studied in the Pacific compared to other octocoral taxa, thus the majority of the new species encountered occur in that group (Cairns, 2018). In the genus Narella, two new species were described with material from sites within the Phoenix Islands. The type specimen of Narella fordi Cairns (2018) was identified from Te Kaitira Seamount at 1899 m. This species was only been observed at Te Kaitira Seamount between the depths of 1817 and 1932 m. Narella aurantiaca Cairns (2018) was identified off Nikumaroro at 1112 m but also observed at McKean Island and Winslow Reef between 558 and 1105 m. This species was also encountered in the Musicians Seamounts and off Wake Island at similar depths (Cairns, 2018). Narella compressa Kinoshita, 1908, was identified off Carondelet Reef at 497 m and is likely the first record since its encounter in 1906 off Japan (Cairns, pers. comm.). N. compressa was observed and collected within PIPA at Carondelet Reef and Maibua Seamount between 373 and 583 m. One colony of Thouarella tydemani Versluys, 1906, a relatively rare species in that genus originally reported from Indonesia at 520 m, was only observed 33 times and sampled at Winslow Reef at 801 m. The sea fan, Paracalyptrophora hawaiiensis Cairns, 2009, was reported from the Phoenix Islands Protected Area between 445 and 666 m. Along with new records from Tokelau (Pao Pao Seamount), American Samoa, and Rawaki Island, this suggests P. hawaiiensis has a wider distribution throughout the southwest Pacific and north to Hawaii at upper bathyal depths (Cairns, 2018). Calyptrophora clarki Bayer, 1951 was observed 48 times and sampled 3 times between 418 and 1211 m in PIPA. Originally described from Hawaii, it has since been found in the Northern Mariana Islands and Phoenix Islands, representing a significant southward range extension. In the same genus, Calyptrophora diaphana Cairns, 2012 initially described off the North Island of New Zealand in 2012 was collected at a recorded depth of 1225 m at Orona Atoll but also was recently found around Wake Island in the western Pacific (Cairns, 2018). Only 4 colonies of C. diaphana were observed in total, all occurring at Orona Atoll.
Despite efforts to identify many deep-water coral species from physical specimens in PIPA, a significant number morphospecies as well as voucher collections from the 2017 expeditions remain unidentified to the species level. From the 167 morphospecies identified, only 39 species were identifiable to the species level from voucher collections. Nevertheless, some similarities were seen among morphotypes observed in imagery to those encountered elsewhere in the region as a part of the CAPSTONE expeditions (Kennedy et al., 2019). In this study, the abundant Pleurogorgia sp. 1 strongly resembles Pleurogorgia militaris, a widely distributed Pacific deep-water coral, but cannot yet be confirmed because specimens were not taken of this morphotype within PIPA. In addition, 8 morphospecies were identified as distinct in both the precious coral genus Hemicorallium as well as the genus Victorgorgia with none being identified to species yet. A significant number of collections were also made in the enigmatic octocoral families Plexauridae and Isididae but detailed morphological taxonomic keys for these families in the central and south Pacific are incomplete and require further attention. Recent efforts to revise the taxonomy of families and genera within the Octocorallia have provided significant contributions to our knowledge of deep-water coral diversity in the Pacific Ocean (Tu et al., 2016; Moore et al., 2017; Cairns, 2018). It is likely that as these specimens are more widely scrutinized using both molecular and morphological taxonomic tools, more species identifications can be determined.
Conservation Considerations
The Phoenix Islands Protected Area was designated as an ecologically and biologically significant area (EBSA) by the Convention on Biological Diversity1 and, upon further examination, it is becoming increasingly clear that its biological significance extends well into the deep-sea. Still, deep-sea biodiversity on remote features is still threatened by several factors including the effects of climate-driven ocean change (Tittensor et al., 2010; Sweetman et al., 2017) as well as physical disturbance from fishing gear (Clark and Koslow, 2007; Clark et al., 2016), and marine debris. Since PIPA has been closed to most fishing since 2015, with the exception of a small subsistence fishery around Kanton, it has been largely isolated from these negative impacts (Rotjan et al., 2014). Still, seamounts in the western and central Pacific have been found to be aggregators for commercially-important large pelagic fishes (Morato et al., 2011). Debris from derelict longline fishing gear, which remain prevalent in the region (McDermott et al., 2018), have the potential to have far-reaching effects deep-water benthic communities.
Other more significant risks to deep-sea coral conservation in the region include direct disturbance to the seafloor in the form of deep-sea mineral crust mining. Recently, commercial mining of ferromanganese crusts accumulating on seamounts have been proposed as a way to extract mineral resources (Hein et al., 2013; Miller et al., 2018). Detailed biological characterizations and species inventories for much of the adjacent Pacific Prime Crust Zone and south Pacific seamounts with cobalt-rich mineral crusts are critically lacking (Schlacher et al., 2014). As crust attributes are difficult to estimate from ROV video transects, it remains a question where deep-water coral communities lie with respect to areas that harbor economically viable mineral crusts in the Phoenix Islands, but crust habitats in the South Pacific have been found to be important indicators of sensitive deep-sea communities (Delavenne et al., 2019). The precautionary approach should be applied to these resources to conserve seamount benthic communities for their biodiversity, unique evolutionary histories, and ecosystem services.
Data Availability Statement
The datasets generated for this study are available on request to the corresponding author.
Author Contributions
The research was conceived and designed by EC, RR, TS, and SA. Fieldwork was conducted by SA, RR, EC, TS, and AK. SA, MD, and AK performed the video review and annotations. SA performed data quality control and statistical analyses. Figures and tables were created by SA with support from MD and AK. All authors contributed to the writing and editing of the final manuscript.
Funding
Funding for this work was provided by NOAA Office of Ocean Exploration and Research (Grant No. NA17OAR0110083) to RR, EC, TS, and David Gruber.
Conflict of Interest
The authors declare that the research was conducted in the absence of any commercial or financial relationships that could be construed as a potential conflict of interest.
Acknowledgments
We would like to thank the Schmidt Ocean Institute for ship time on the R/V Falkor during FK171005, Discovering Deep-Sea Corals of the Phoenix Islands, and to the science party and crew of this expedition for their efforts. We would also thank the expedition coordinator B. Kennedy, science co-lead A. Demopoulos, science party, as well as the crew of the NOAA ship Okeanos Explorer during the EX-17-03 Discovering the Deep: Exploring Remote Pacific Marine Protected Areas expedition. Taxonomic assistance was provided by S. Cairns. We would like to extend our deepest gratitude to the Republic of Kiribati for the opportunity to work in the Phoenix Islands under permits PFA3 No. 1/17 and 4/17 issued by the PIPA Implementation Office. We also thank Mr. Betarim Rimon and Mr. Arenteiti Tekiau, who were the official Kiribati Observers on the EX-1703 and FK171005 cruises, respectively. Finally, we would like to thank three manuscript reviewers for their input in greatly improving the quality of this manuscript.
Supplementary Material
The Supplementary Material for this article can be found online at: https://www.frontiersin.org/articles/10.3389/fmars.2020.00042/full#supplementary-material
Footnotes
References
Anderson, M., Gorley, R. N., and Clarke, R. K. (2008). Permanova+ for Primer: Guide to Software and Statisticl Methods. Plymouth: Primer-E Limited.
Arantes, R. C. M., Castro, C. B., Pires, D. O., and Seoane, J. C. S. (2009). Depth and water mass zonation and species associations of cold-water octocoral and stony coral communities in the southwestern Atlantic. Mar. Ecol. Prog. Ser. 397, 71–79. doi: 10.3354/meps08230
Auster, P. J., Moore, J., Heinonen, K. B., and Watling, L. (2005). “A habitat classification scheme for seamount landscapes: assessing the functional role of deep-water corals as fish habitat,” in Cold-Water Corals and Ecosystems, eds A. Freiwald, and J. M. Roberts, (Cham: Springer), 761–769. doi: 10.1007/3-540-27673-4_40
Ayress, M. A., De Deckker, P., and Coles, G. P. (2004). A taxonomic and distributional survey of marine benthonic Ostracoda off Kerguelen and Heard Islands, South Indian Ocean. J. Micropalaeontol. 23, 15–38. doi: 10.1144/jm.23.1.15
Bohnenstiehl, D. R., France, S. C., Cantwell, K., and White, M. (2018). Mountains in the deep: exploration of the seamounts of the Central Pacific Basin. Oceanography 31, 78–79.
Bors, E. K., Rowden, A. A., Maas, E. W., Clark, M. R., and Shank, T. M. (2012). Patterns of deep-sea genetic connectivity in the New Zealand region: implications for management of benthic ecosystems. PLoS One 7:e49474. doi: 10.1371/journal.pone.0049474
Bostock, H. C., Opdyke, B. N., and Williams, M. J. M. (2010). Characterising the intermediate depth waters of the Pacific Ocean using d 13 C and other geochemical tracers. Deep Res. Part I 57, 847–859. doi: 10.1016/j.dsr.2010.04.005
Buhl-Mortensen, L., Vanreusel, A., Gooday, A. J., Levin, L. A., Priede, I. G., Buhl-Mortensen, P., et al. (2010). Biological structures as a source of habitat heterogeneity and biodiversity on the deep ocean margins. Mar. Ecol. 31, 21–50. doi: 10.1111/j.1439-0485.2010.00359.x
Cairns, S. D. (2018). Primnoidae (Cnidaria: Octocorallia: Calcaxonia) of the okeanos explorer expeditions (CAPSTONE) to the central Pacific. Zootaxa 4532, 1–43. doi: 10.11646/zootaxa.4532.1.1
Cantwell, K., Elliott, K., and Kennedy, B. R. C. (2018). Deepwater exploration of the pacific remote islands marine national monument and Central Pacific Basin. Oceanography 31, 74–75.
Carney, R. S. (2005). Zonation of deep biota on continental margins. Oceanogr. Mar. Biol. 43, 211–278. doi: 10.1201/9781420037449.ch6
Clark, M. R., Althaus, F., Schlacher, T. A., Williams, A., Bowden, D. A., and Rowden, A. A. (2016). The impacts of deep-sea fisheries on benthic communities: a review. ICES J. Mar. Sci. 73, i51–i69. doi: 10.1093/icesjms/fsv123
Clark, M. R., and Koslow, J. A. (2007). Impacts of fisheries on seamounts. Seamounts Ecol. Fish. Conserv. 12, 413–441. doi: 10.1002/9780470691953.ch19
Clark, M. R., Rowden, A. A., Schlacher, T., Williams, A., Consalvey, M., Stocks, K. I., et al. (2010). The ecology of seamounts: structure, function, and human impacts. Ann. Rev. Mar. Sci. 2, 253–278. doi: 10.1146/annurev-marine-120308-081109
Clarke, K. R., Somerfield, P. J., and Gorley, R. N. (2008). Testing of null hypotheses in exploratory community analyses: similarity profiles and biota-environment linkage. J. Exp. Mar. Bio. Ecol. 366, 56–69. doi: 10.1016/j.jembe.2008.07.009
Delavenne, J., Keszler, L., Castelin, M., Lozouet, P., Maestrati, P., and Samadi, S. (2019). Deep-sea benthic communities in the largest oceanic desert are structured by the presence of polymetallic crust. Sci. Rep. 9, 1–11. doi: 10.1038/s41598-019-43325-0
Demopoulos, A. W. J., Auscavitch, S., Sowers, D., Pawlenko, N., and Kennedy, B. R. C. (2018). Discovering the deep: exploring remote Pacific marine protected areas. Oceanography 31, 76–77.
Emery, W. J. (2001). Water types and water masses. Encycl. Ocean Sci. 6, 3179–3187. doi: 10.1006/rwos.2001.0108
Etnoyer, P. J., Wood, J., and Shirley, T. C. (2010). BOX 12| how large is the seamount biome? Oceanography 23, 206–209. doi: 10.5670/oceanog.2010.96
Genin, A., Dayton, P. K., Lonsdale, P. F., and Spiess, F. N. (1986). Corals on seamount peaks provide evidence of current acceleration over deep-sea topography. Nature 322, 59–61. doi: 10.1038/322059a0
Gollner, S., Kaiser, S., Menzel, L., Jones, D. O. B., Brown, A., Mestre, N. C., et al. (2017). Resilience of benthic deep-sea fauna to mining activities. Mar. Environ. Res. 129, 76–101. doi: 10.1016/j.marenvres.2017.04.010
Hein, J. R., Mizell, K., Koschinsky, A., and Conrad, T. A. (2013). Deep-ocean mineral deposits as a source of critical metals for high- and green-technology applications: comparison with land-based resources. Ore Geol. Rev. 51, 1–14. doi: 10.1016/j.oregeorev.2012.12.001
Herrera, S., Jackson, M., Konter, J., Lobecker, E., and Elliott, K. (2018). 2017 American samoa expedition: suesuega o le moana Amerika Samoa. Oceanography 31, 72–73.
Kelley, C., Mah, C., Malik, M., and Elliott, K. (2018). Laulima O Ka moana: exploring deep monument waters around johnston atoll. Oceanography 31, 80–81.
Kennedy, B. R. C., Cantwell, K., Malik, M., Kelley, C., Potter, J., Elliott, K., et al. (2019). The unknown and the unexplored: insights into the pacific deep-sea following NOAA CAPSTONE expeditions. Front. Mar. Sci. 6:480. doi: 10.3389/fmars.2019.00480
Mangubhai, S., Lovell, E., Abeta, R., Donner, S., Redfern, F. M., O’Brien, M., et al. (2019). “Kiribati: atolls and marine ecosystems,” in World Seas: An Environmental Evaluation, ed. C. Sheppard, (Amsterdam: Elsevier), 807–826. doi: 10.1016/b978-0-08-100853-9.00054-3
McDermott, G. R., Meng, K. C., Mcdonald, G. G., and Costello, C. J. (2018). The blue paradox: preemptive overfishing in marine reserves. Proc. Natl. Acad. Sci. U.S.A. 116, 5319–5325. doi: 10.1073/pnas.1802862115
McKinnie, D., Rotjan, R., and Peau, A. L. (2018). Exploring the Pacific through international partnerships. Oceanography 31, 86–87.
Miller, K. A., Thompson, K. F., Johnston, P., and Santillo, D. (2018). An overview of seabed mining including the current state of development, environmental impacts, and knowledge gaps. Front. Mar. Sci. 4:418. doi: 10.3389/fmars.2017.00418
Miller, K. J., and Gunasekera, R. M. (2017). A comparison of genetic connectivity in two deep sea corals to examine whether seamounts are isolated islands or stepping stones for dispersal. Sci. Rep. 7, 1–14. doi: 10.1038/srep46103
Miller, K. J., Rowden, A. A., Williams, A., and Haussermann, V. (2011). Out of their depth? Isolated deep populations of the cosmopolitan coral Desmophyllum dianthus may be highly vulnerable to environmental change. PLoS One 6:e19004. doi: 10.1371/journal.pone.0019004
Moore, K. M., Alderslade, P., and Miller, K. J. (2017). A taxonomic revision of Anthothela (Octocorallia: Scleraxonia: Anthothelidae) and related genera, with the addition of new taxa, using morphological and molecular data. Zootaxa 4304, 1–212.
Morato, T., Hoyle, S. D., Allain, V., and Nicol, S. J. (2011). Tuna longline fishing around west and central Pacific seamounts. PLoS One 5:e14453. doi: 10.1371/journal.pone.0014453
Morgan, N. B., Cairns, S., Reiswig, H., and Baco, A. R. (2015). Benthic megafaunal community structure of cobalt-rich manganese crusts on Necker Ridge. Deep Sea Res. Part I Oceanogr. Res. Pap. 104, 92–105. doi: 10.1016/j.dsr.2015.07.003
Mosher, C. V., and Watling, L. (2009). Partners for life: a brittle star and its octocoral host. Mar. Ecol. Prog. Ser. 397, 81–88. doi: 10.3354/meps08113
Obura, D., Stone, G., Mangubhai, S., Bailey, S., Yoshinaga, H., and Barrel, R. (2011). Baseline marine biological surveys of the Phoenix Islands, July 2000. Atoll Res. Bull. 589, 1–61. doi: 10.5479/si.00775630.589.1
Oksanen, J., Blanchet, F. G., Kindt, R., Legendre, P., Minchin, P. R., O’hara, R. B., et al. (2015). Package ‘Vegan.’ Community Ecol. Packag. Version 2.
Quattrini, A. M., Demopoulos, A. W. J., Singer, R., Roa-Varon, A., and Chaytor, J. D. (2017). Demersal fish assemblages on seamounts and other rugged features in the northeastern Caribbean. Deep Sea Res. Part I Oceanogr. Res. Pap. 123, 90–104. doi: 10.1016/j.dsr.2017.03.009
Radice, V. Z., Quattrini, A. M., Wareham, V. E., Edinger, E. N., and Cordes, E. E. (2016). Vertical water mass structure in the North Atlantic influences the bathymetric distribution of species in the deep-sea coral genus Paramuricea. Deep Sea Res. Part I Oceanogr. Res. Pap. 116, 253–263. doi: 10.1016/j.dsr.2016.08.014
Rogers, A. D. (2018). The biology of seamounts: 25 Years on. Adv. Mar. Biol. 79, 137–224. doi: 10.1016/BS.AMB.2018.06.001
Rogers, A. D., Baco, A., Griffiths, H., and Hall-Spencer, J. M. (2007). “Corals on seamounts,” in Seamounts: Ecology Fisheries and Conservation, Blackwell Fisheries and Aquatic Resources Series, eds T. J. Pitcher, T. Morato, P. J. B. Hart, M. R. Clark, N. Haggan, and R. S. Santos, (Oxford: Blackwell Scientific), 3343–3352.
Rotjan, R., Jamieson, R., Carr, B., Kaufman, L., Mangubhai, S., Obura, D., et al. (2014). Establishment, management, and maintenance of the phoenix islands protected area. Adv. Mar. Biol. 69, 289–324. doi: 10.1016/B978-0-12-800214-8.00008-6
Rotjan, R., and Obura, D. (2010). Phoenix Islands Protected Area 10-Year Research Vision. Boston, MA: New England Aquarium.
Schlacher, T. A., Baco, A. R., Rowden, A. A., O’Hara, T. D., Clark, M. R., Kelley, C., et al. (2014). Seamount benthos in a cobalt-rich crust region of the central P acific: conservation challenges for future seabed mining. Divers. Distrib. 20, 491–502. doi: 10.1111/ddi.12142
Schlitzer, R. (2019). Ocean Data View. Available at: https://odv.awi.de/ (accessed May 29, 2019).
Shank, T. M. (2010). Seamounts: deep-ocean laboratories of faunal connectivity, evolution, and endemism. Oceanography 23, 108–122. doi: 10.5670/oceanog.2010.65
Sweetman, A. K., Thurber, A. R., Smith, C. R., Levin, L. A., Mora, C., Wei, C.-L., et al. (2017). Major impacts of climate change on deep-sea benthic ecosystems. Elem. Sci. Anth. 5:4. doi: 10.1525/elementa.203
Tittensor, D. P., Baco, A. R., Hall-Spencer, J. M., Orr, J. C., and Rogers, A. D. (2010). Seamounts as refugia from ocean acidification for cold-water stony corals. Mar. Ecol. 31, 212–225. doi: 10.1111/j.1439-0485.2010.00393.x
Tu, T.-H., Dai, C.-F., and Jeng, M.-S. (2016). Taxonomic revision of Coralliidae with descriptions of new species from New Caledonia and the Hawaiian Archipelago. Mar. Biol. Res. 12, 1003–1038. doi: 10.1080/17451000.2016.1241411
Victorero, L., Robert, K., Robinson, L. F., Taylor, M. L., and Huvenne, V. A. I. (2018). Species replacement dominates megabenthos beta diversity in a remote seamount setting. Sci. Rep. 8:4152. doi: 10.1038/s41598-018-22296-8
Watling, L., and Auster, P. J. (2017). Seamounts on the high seas should be managed as vulnerable marine ecosystems. Front. Mar. Sci. 4:14. doi: 10.3389/fmars.2017.00014
Watling, L., Guinotte, J., Clark, M. R., and Smith, C. R. (2013). A proposed biogeography of the deep ocean floor. Prog. Oceanogr. 111, 91–112. doi: 10.1016/j.pocean.2012.11.003
Watling, L., and Norse, E. A. (1998). Disturbance of the seabed by mobile fishing gear: a comparison to forest clearcutting. Conserv. Biol. 12, 1180–1197. doi: 10.1046/j.1523-1739.1998.0120061180.x
Keywords: deep sea coral, seamounts, marine protected area, marine biogeography, community structure, equatorial central Pacific, water masses
Citation: Auscavitch SR, Deere MC, Keller AG, Rotjan RD, Shank TM and Cordes EE (2020) Oceanographic Drivers of Deep-Sea Coral Species Distribution and Community Assembly on Seamounts, Islands, Atolls, and Reefs Within the Phoenix Islands Protected Area. Front. Mar. Sci. 7:42. doi: 10.3389/fmars.2020.00042
Received: 14 April 2019; Accepted: 22 January 2020;
Published: 13 February 2020.
Edited by:
Ricardo Serrão Santos, University of the Azores, PortugalReviewed by:
Amy Baco-Taylor, Florida State University, United StatesFrank Alan Parrish, Pacific Islands Fisheries Science Center (NOAA), United States
Marco Taviani, Italian National Research Council, Italy
Copyright © 2020 Auscavitch, Deere, Keller, Rotjan, Shank and Cordes. This is an open-access article distributed under the terms of the Creative Commons Attribution License (CC BY). The use, distribution or reproduction in other forums is permitted, provided the original author(s) and the copyright owner(s) are credited and that the original publication in this journal is cited, in accordance with accepted academic practice. No use, distribution or reproduction is permitted which does not comply with these terms.
*Correspondence: Steven R. Auscavitch, c3RldmVuLmF1c2Nhdml0Y2hAdGVtcGxlLmVkdQ==