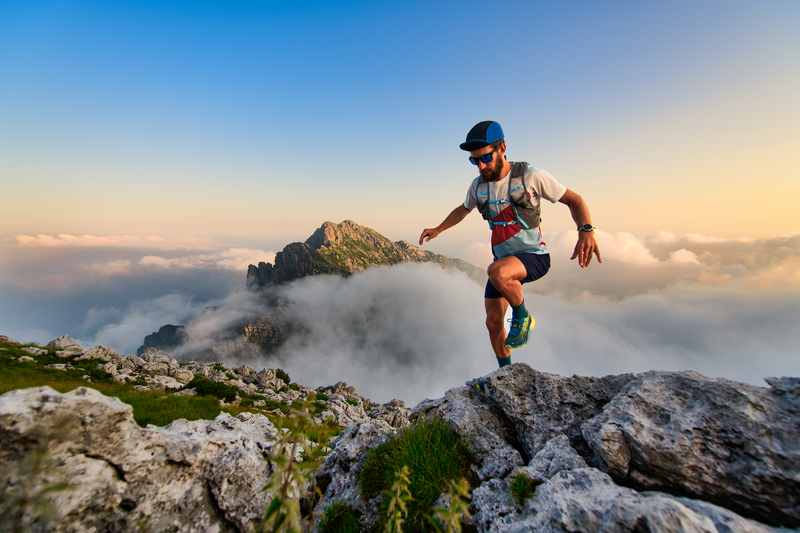
94% of researchers rate our articles as excellent or good
Learn more about the work of our research integrity team to safeguard the quality of each article we publish.
Find out more
BRIEF RESEARCH REPORT article
Front. Mar. Sci. , 20 December 2019
Sec. Marine Biogeochemistry
Volume 6 - 2019 | https://doi.org/10.3389/fmars.2019.00780
This article is part of the Research Topic Current Topics in Marine Organic Biogeochemical Research View all 21 articles
Authentic biomarker standards were obtained from algal cultures in an attempt to accurately determine blank C added during sample processing for compound-specific radiocarbon analysis. Emiliania huxleyi and Thalassiosira pseudonana were grown under manipulated Δ14C dissolved inorganic carbon (DIC) levels and chlorophyll a and either alkenones (E. huxleyi) or low molecular weight (LMW) alkanoic acids (T. pseudonana) were isolated from the respective biomass using preparative liquid chromatography (LC), wet chemical techniques or preparative gas chromatography, respectively. DI14C in the seawater medium was determined pre- and post-growth. Biomarker Δ14C values mostly agree within 1σ or 2σ analytical uncertainties. In those cases where biomarker Δ14C values differ significantly, chlorophyll a is up to 104‰ more 14C-depleted than alkenones or LMW alkanoic acids, consistent with a larger LC blank compared to the other purification methods. However, in the majority of experimental setups pre- and post-growth DIC Δ14C values seem to be compromised by an unknown and variable blank C contribution. DIC Δ14C values deviate strongly from the anticipated Δ14C values (by up to ca. 560‰), pre- and post-growth Δ14C values differ significantly (by up to ca. 460‰), and changes are not unidirectional. Accordingly, since the substrate Δ14C value cannot unequivocally be constrained, blank C contributions for the different biomarker purification methods cannot be accurately calculated. This study illustrates the challenges and problems of producing authentic standards that are not readily commercially available and exemplifies how a laborious and time-consuming culturing approach may enter a vicious cycle of blank C contamination hampering accurate blank C determination.
Compound-specific radiocarbon analysis (CSRA) has revolutionized our understanding of carbon cycling in the ocean including both sedimentary and metabolic processes in the water column and sediments (e.g., Pearson et al., 2005; Ingalls et al., 2006; Mollenhauer and Eglinton, 2007; Mollenhauer et al., 2007), as well as land to ocean carbon transfer (e.g., Drenzek et al., 2009; Kusch et al., 2010b; Feng et al., 2013). Accordingly, there are efforts to extend CSRA to an ever-increasing number of compounds and compound classes. Nonetheless, CSRA is quite laborious and compound isolation requires various wet-chemical techniques as well as analytically more advanced steps such as preparative gas chromatography (GC) or preparative liquid chromatography (LC). In order to achieve meaningful results, it is a pivotal necessity to accurately determine the blank carbon contribution associated with compound isolation (sample processing), i.e., both the amount as well as 14C isotopic composition of this blank C (e.g., Shah and Pearson, 2007). Two approaches can be taken for this purpose (i) obtaining “true” blank runs void of any sample, e.g., collection of the LC effluent volume, or (ii) processing authentic standards with known Δ14C values. “True” blank runs require the pooling of many consecutive runs in order to retrieve sufficient C amounts for 14C analysis, which may not always be successful (Shah and Pearson, 2007), and yield the combined blank C isotopic composition. In contrast, processing of authentic standards is likely quicker and allows the characterization of blank C contributions from both fossil (“14C-dead”) and modern (atmospheric 14C) sources if these standards have modern and fossil Δ14C values, respectively.
However, for a range of compounds or even compound classes typically found in marine particulate organic matter, authentic standards are not readily available. Either, they are not produced commercially or are manufactured solely from either plant biomass or petroleum sources limiting our ability to characterize either the fossil or modern blank sources, respectively. Under these circumstances, scientists are required to use surrogate standards (e.g., Shah and Pearson, 2007; Birkholz et al., 2013) or to obtain their own authentic standards for example via culturing approaches (Mollenhauer et al., 2005). Surrogate standards only provide the best approximation of the actual blank C, since they do not have chemical properties identical to the target compounds, e.g., they will show different chromatographic behavior and, thus, entrain slightly different column “bleed” from stationary phases during chromatography. Authentic standards can be obtained when culturing marine algae in the laboratory under controlled dissolved inorganic carbon (DIC) Δ14C levels (Mollenhauer et al., 2005). While this approach is laborious and time-consuming, it allows for the determination of blank C contribution associated with the isolation of target compounds from different compound classes and with various Δ14C endmembers. However, it is crucial that the Δ14C isotopic composition of C sources during culturing remains constrained and the culturing experiments themselves are not associated with significant blank C contributions.
Here, we illustrate the challenging nature of natural level 14C manipulation in batch cultures of Emiliania huxleyi and Thalassiosira pseudonana grown under three different Δ14C DIC levels (40‰, –480‰, and –1000‰). We show that blank C of unknown origin contributed significantly to the DIC Δ14C isotopic composition during the experiments, impairing a valuable assessment of blank C associated with the purification of chlorophyll a as well as alkenones (E. huxleyi) and low molecular weight (LMW) alkanoic acids (T. pseudonana).
North Sea seawater was enriched with nutrients including nitrate, phosphate, silicate (for T. pseudonana), metals, and vitamins at concentrations equaling f/2 medium (Guillard and Ryther, 1962). The seawater was filtered through sterile 0.2 μm PTFE filters and collected in sterile 2.4 l borosilicate glass bottles. The seawater was percolated with CO2-free air for 36 h at 25°C to expel DIC species (Figure 1). A similar setup is routinely used to achieve DIC-free medium, which has been verified by direct measurements using Membrane-Inlet MS (Rost et al., 2007). Subsequently, CO2 of known 14C isotopic composition was percolated through the seawater at 380 ppm for 48 h at 25°C each. CO2 endmembers represent “Modern” (40‰; ambient air in AD2010/AD2011; Levin et al., 2013) and “Fossil” (–1000‰; Air Liquide N45) Δ14C values as well as a 1:1 “Intermediate” mixture (–480‰; gas volume v:v using a custom-made gas flow controller). The seawater was cooled to 15°C and the E. huxleyi strain RCC 1238 (Roscoff Culture Collection) or the T. pseudonana strain CCMP 1335 (Provasoli-Guillard National Center for Culture of Marine Phytoplankton) were inoculated at a cell density of approximately 200 cells ml–1. Both strains were checked for their vitality under a light microscope prior to inoculation. Culture bottles were topped with seawater to reduce headspace volume and closed with sterile PTFE-lined caps (to limit diffusion of atmospheric 14C). Bottles were stored in a RUMED 1200 light-thermostat at 15°C with a photon flux density of 100 μmol photons m–2 s–1 to stimulate pigment production (Nielsen, 1997). Starter cultures were grown in 16 h: 8 h light: dark cycles until they reached stationary phase (based on cell densities determined from 1 ml aliquots). Cell densities were determined using a Beckman Multisizer 3 Coulter Counter (E. huxleyi) or visual counting under a microscope (T. pseudonana). Afterward an aliquot of the starter cultures was taken and approximately 200 cells ml–1 of the isotopically equilibrated starter culture were then inoculated into triplicates of new medium (sterile 2.4 l borosilicate glass bottles) and main cultures were grown in 16 h: 8 h light: dark cycles. After reaching stationary phase (9–13 days), an aliquot of the main culture was taken for final cell density determination and cultures were harvested (mid-day) by filtration onto pre-combusted GF/F filters (–200 m bar; triplicates of each batch were combined) and stored frozen at –20°C in the dark until analysis.
Figure 1. Schematic of the experimental setup during (A) DIC 14C manipulation and (B) batch culture growth of the E. huxleyi and T. pseudonana cultures.
For pigment extraction, GF/F filters were transferred into pre-combusted glass vials and 20 ml dehydrated acetone was added, samples were extracted for 15 min in an ultrasonic ice bath, centrifuged at 1200 rpm for 3 min, and the acetone was recovered. This extraction step was repeated twice. Subsequently, the combined acetone extracts were concentrated under N2 and transferred into a 1:3 hexane: Seralpure® mixture for liquid-liquid extraction. Liquid-liquid extraction was performed using hexane (pigments) and dichloromethane (residual lipids), each extraction was repeated until the solvent layer was colorless. The hexane fraction was concentrated under N2, rinsed through pre-combusted sodium sulfate to remove Seralpure® residues, dehydrated dimethylformamide was added, the sample homogenized, and stored at –20°C overnight. Chlorophyll a was purified with an Agilent 1200 Series HPLC/DAD system using the method described in Kusch et al. (2010a). After initial ultrasonic extraction of pigments, the GF/F filters were additionally extracted with 9:1 dichloromethane: methanol using Soxhlet (48 h; cellulose thimbles pre-extracted 24 h) in order to ensure maximum lipid recovery. The total lipid extract (TLE) was combined with the dichloromethane fraction recovered during liquid-liquid extraction of the acetone-Seralpure® mixture. The TLE was saponified in 0.5 M potassium hydroxide in methanol for 3 h at 85°C to separate alkanoic acids and neutral lipids. Alkenones were purified wet-chemically according to Ohkouchi et al. (2005). LMW alkanoic acids were methylated and purified using an Agilent HP6890N GC connected to a Gerstel preparative fraction collector following Kusch et al. (2010b).
For radiocarbon measurements, chlorophyll a, alkenones, and LMW alkanoic acids methyl esters (FAMEs) were converted into CO2. The samples were transferred into pre-combusted quartz tubes (900°C, 4 h), 150 μg pre-combusted copper oxide were added as oxygen source, and samples were evacuated and flame-sealed in vacuo. Afterward, the samples were combusted to CO2 at 900°C for 8 h. The resulting CO2 gas was stripped of water in vacuo and quantified manometrically. AMS measurements of the Δ14C of chlorophyll a, alkenones, and FAME isolates containing >1 μmol CO2 were performed at the National Ocean Sciences Accelerator Mass Spectrometry Facility (NOSAMS) at Woods Hole Oceanographic Institution, United States following the protocol for small samples (Pearson et al., 1998). Aliquots of the CO2 were measured on a VG Optima to obtain δ13C values. 14C analyses of biomarker isolates <1 μmol CO2 were performed at the Ion Beam Physics Department at ETH, Switzerland using the MICADAS gas ion source system (Ruff et al., 2007). Seawater sampled before and after batch culturing was transferred into 500 ml pre-combusted borosilicate glass bottles, 100 μl saturated mercuric chloride solution was added, and bottles were filled without headspace. Seawater DIC was analyzed at NOSAMS following standard protocols (McNichol et al., 1994).
Radiocarbon data are reported as Δ14C in ‰ according to Stuiver and Polach (1977), δ13C data are reported in ‰ relative to Vienna Pee Dee Belemnite (VPDB). FAME Δ14C and δ13C values were corrected for the addition of one methyl group during derivatization.
In the “Modern” E. huxleyi culture, pre- and post-growth DIC Δ14C values are 60.0 ± 3.4‰ and 83.0 ± 3.5‰, respectively (Figure 2 and Supplementary Table S1). The stable carbon isotopic composition (δ13C) of the seawater DIC was –1.0 ± 0.1‰ prior to culture growth and 4.0 ± 0.1‰ after E. huxleyi growth. The alkenone Δ14C value of 77.0 ± 3.9‰ falls within the pre- and post-growth DIC Δ14C values, while the chlorophyll a Δ14C value (24.5 ± 10.6‰) is significantly (>2σ analytical uncertainty) more depleted. Corresponding δ13C values are –20.2 ± 0.1‰ for alkenones and –15.4 ± 0.1‰ for chlorophyll a.
Figure 2. Radiocarbon isotopic composition (Δ14C in ‰) of the Emiliania huxleyi and Thalassiosira pseudonana cultures. Errors bars (shaded area for DIC) represent 1σ analytical uncertainty.
Pre- and post-growth DIC Δ14C values for the “Modern” T. pseudonana culture show the highest deviation of the entire data set (∼460‰) with a pre-growth Δ14C value of –43.9 ± 2.9‰ and a post-growth Δ14C value of –505.2 ± 2.5‰. Analogous to the “Modern” E. huxleyi culture, seawater DIC became 13C-enriched during the course of the experiment increasing from a pre-growth δ13C value of –14.8 ± 0.1‰ to a δ13C value of –11.0 ± 0.1‰ post-growth. Both, T. pseudonana derived C14:0 alkanoic acid (–348.8 ± 3.1‰) and chlorophyll a (−452.3 ± 1.9‰) Δ14C values fall within the range of the pre- and post-growth DIC Δ14C values but deviate significantly (>2σ analytical uncertainty) from one another. The δ13C values of the C14:0 alkanoic acid and chlorophyll a are –30.2 ± 0.1‰ and –27.8 ± 0.1‰, respectively.
The seawater DIC Δ14C value in the “Intermediate” E. huxleyi culture was –380.1 ± 2.4‰ before culture growth and −437.1 ± 2.0‰ after growth (Figure 2 and Supplementary Table S1) with corresponding pre- and post-growth δ13C values of –14.5 ± 1.0‰ and –12.1 ± 0.1‰, respectively. Both, alkenones and chlorophyll a from E. huxleyi are more 14C-depleted than DIC with Δ14C values of –451.3 ± 2.1‰ and −463.9 ± 2.0‰, respectively, and δ13C values of –35.0 ± 0.1‰ and –27.6 ± 0.1‰, respectively.
In the “Intermediate” T. pseudonana culture both the pre-growth DIC (–135.7 ± 3.0‰) and the post-growth DIC (–172.8 ± 2.6‰) Δ14C values are considerably 14C-enriched in comparison to the anticipated Δ14C value (ca. –480‰) that should have resulted from a 1:1 CO2 mixture of ambient air and Air Liquide N45. Pre- and post-growth seawater δ13C values are –8.8 ± 0.1‰ and –2.4 ± 0.1‰, respectively. The biomarker Δ14C values were measured on ultra-small samples sizes (<1 μmol) and are, thus, associated with large errors. While C14:0 alkanoic acid (–490.5 ± 37.2‰) and C16:0 alkanoic acid (–332.9 ± 25.5‰) deviate significantly, C14:0 alkanoic acid and chlorophyll a (–357.0 ± 42.8‰) agree within 2σ analytical uncertainty and C16:0 alkanoic acid and chlorophyll a agree within 1σ analytical uncertainty. Biomarker δ13C values could not be measured independently on splits of the sample CO2 due to size restrictions.
In the “Fossil” E. huxleyi culture, pre- and post-growth seawater DIC Δ14C values (–711.1 ± 1.6‰ and –519.4 ± 2.1‰, respectively) differ significantly with a ∼200‰ enrichment in 14C during culture growth (Figure 2 and Supplementary Table S1). A concurrent strong isotopic enrichment is also evident for the seawater DIC δ13C. The pre-growth value was –22.1 ± 0.1‰ whereas the post-growth value increased to –13.1 ± 0.1‰. Both alkenones (–280.3 ± 2.5‰) and chlorophyll a (–277.6 ± 2.5‰) are even more enriched in 14C than the post-growth DIC but agree within 1σ analytical uncertainty with each other. Alkenones have a δ13C value of –30.2 ± 0.1‰. Chlorophyll a has a δ13C value of –22.5 ± 0.1‰.
The pre-growth seawater DIC Δ14C value (–439.8 ± 2.2‰) in the “Fossil” T. pseudonana culture is significantly 14C-enriched in comparison to the post-growth Δ14C value (−843.1 ± 1.3‰). The δ13C values of the pre- and post-growth DIC show the exceptional pattern of a 13C-depletion during the culture growth. While the DIC δ13C value equaled –16.5 ± 0.1‰ before growth of T. pseudonana, it decreased to –23.6 ± 0.1‰ after culture growth representing the most depleted DIC δ13C value of the entire data set. All biomarker Δ14C values are bracketed by the DIC Δ14C values. The C16:0 alkanoic acid (−653.3 ± 2.0‰) and the C16:1 alkanoic acid (–689.6 ± 23.7‰) agree within 2σ analytical uncertainty. Chlorophyll a is more 14C-depleted (–706.5 ± 2.3‰) than both concurrent alkanoic acids but agrees within 1σ analytical uncertainty with the C16:1 alkanoic acid. The δ13C value of the C16:0 alkanoic acid (–42.6 ± 1.0‰) is the most 13C-depleted value of the entire data set. Likewise, chlorophyll a is substantially more 13C-depleted (–34.7 ± 1.0‰) than chlorophyll a in any of the other cultures.
All E. huxleyi and T. pseudonana main cultures reached stationary phase after 9–13 days. During this time interval, DIC utilization by algae ranged from ∼0.8–1.2 mmol/kg in the E. huxleyi cultures (in good agreement with final biomass; r2 = 0.99) and ∼0.4–0.8 mmol/kg in the T. pseudonana cultures (Supplementary Table S2). While pre-growth DIC concentrations of 1.8–2.2 mmol/kg were in the range typically observed in the global ocean (Feely et al., 2001), it is obvious that the pre-growth DIC Δ14C values differ from the theoretical values of the CO2 used to manipulate the original seawater DIC, i.e., 40‰ (ambient air in AD2010/AD2011; Levin et al., 2013), –480‰, and –1000‰ for the “Modern,” “Intermediate,” and “Fossil” cultures, respectively. Only the pre-growth DIC Δ14C value in the “Modern” E. huxleyi culture (60.0 ± 3.4‰) is close to the anticipated Δ14C value. With offsets of approximately –85‰ and –100‰, the pre-growth DIC Δ14C values in the “Modern” T. pseudonana culture and the “Intermediate” E. huxleyi cultures (Figure 2 and Supplementary Table S1), respectively, are moderately close to the anticipated DIC Δ14C values. In contrast, the pre-growth DIC Δ14C values in the “Intermediate” T. pseudonana culture and both “Fossil” cultures are significantly more Δ14C-enriched (∼290–560‰). This 14C-enrichment is significantly higher than the 14C-enrichment observed by Mollenhauer et al. (2005) in their “dead air” Isochrysis sp. culture (∼120–170‰). These authors attributed the 14C-enrichment to exchange with the ambient air during the experiment (equaling ca. 10% of the total DIC species). Assuming diffusion of ambient air (Δ14C = 40‰) was the cause in our experiments as well, it would account for 66.2, 27.8, and 53.9% of the DIC species in the “Intermediate” T. pseudonana culture, the “Fossil” E. huxleyi culture, and the ‘Fossil” T. pseudonana culture, respectively. However, our post-growth DIC Δ14C values challenge this explanation for our experiments. Relative to pre-growth DIC, the post-growth DIC Δ14C values are only 14C-enriched in the “Modern” and “Fossil” E. huxleyi cultures and are in fact more 14C-depleted in the “Intermediate” E. huxleyi and all T. pseudonana cultures (Figure 2), which cannot be explained by diffusion of atmospheric 14C. This observation requires the introduction of a 14C-depleted carbon source to the DIC pool, which, however, is not observed in the post-growth DIC pool of the “Modern” and “Fossil” E. huxleyi cultures. The 14C-depletion cannot be explained by kinetic or equilibrium isotope fractionation either. Equilibrium fractionation of carbon isotopes occurs during exchange of gaseous CO2 and the different seawater DIC species, but equilibrium effects should result in Δ14C-enrichment of bicarbonate and carbonate (the primary DIC species at pH ∼8) relative to CO2(aq)/carbonic acid analogous to δ13C values (Mook, 1986; Zhang et al., 1995). For example, an equilibrium effect of ∼8–9‰ in δ13C for total DIC at pH ∼8.2 and 15°C (Mook, 1986; Zhang et al., 1995) would result in an approximately 16–18‰ enrichment in Δ14C. Likewise, algal DIC utilization causes 13C and 14C enrichment of the substrate, as evident for the δ13C values in our data set, which show enrichment ranging from 2.4 to 9.0‰ (Figure 3 and Supplementary Table S1). Accordingly, kinetic effects would account for roughly 5 to 18‰Δ14C-enrichment irrespective of which DIC species was utilized. However, Δ14C values are corrected for such fractionation effects by normalizing to a δ13C value of –25‰ (Stuiver and Polach, 1977). Accordingly, the 14C-depletion in the “Intermediate” E. huxleyi and all T. pseudonana cultures have to be explained by other factors.
Figure 3. Stable carbon isotopic composition (δ13C in ‰) of the E. huxleyi and T. pseudonana cultures. Errors bars are ±0.1‰, not shown due to scale.
The mismatch between the anticipated DIC Δ14C values and the measured Δ14C values may indicate problems during initial DIC manipulation. In comparison to Mollenhauer et al. (2005), we did not acidify the seawater to expel DIC, but rather expelled DIC by sparging with CO2-free air directly. This is a common approach in phytoplankton physiology to achieve DIC-free media, e.g., for testing the DIC-dependence of photosynthesis (Badger et al., 1994; Rost et al., 2003). Even though acidification accelerates the procedure, our setup has been routinely used to achieve DIC-free medium without acidification (typically within 24 h), which has been confirmed by direct measurements using Membrane-Inlet MS (Rost et al., 2007). In general, the measured DIC δ13C and Δ14C values attest to isotopic exchange of the DIC species since they would otherwise mirror natural North Sea surface seawater values, i.e., δ13C values between 0‰ and 1‰ (Burt et al., 2016) and Δ14C values close to atmospheric values in AD2010. We did not obtain Δ14C values for untreated North Sea seawater DIC and (to our knowledge) no direct observational data are available for the most recent decades, but post-bomb surface water Δ14C values until AD1989 inferred from A. islandica indicate that Δ14C values should be slightly more enriched than atmospheric Δ14C values (Scourse et al., 2012). Mass balance using pre-growth DIC Δ14C values for the “Fossil” E. huxleyi and T. pseudonana cultures reveals that 72 and 46% of the total DIC was exchanged, respectively (endmembers –1000‰ and 40‰). We cannot exclude problems during DIC manipulation such as potential contamination with other CO2 sources in the custom-made gas flow control unit or deviations from 1:1 v:v gas mixtures, which may explain the deviation of the pre-growth DIC Δ14C values from the anticipated values. However, such problems cannot explain the observed isotopic shift to more depleted post-growth DIC Δ14C values in the “Intermediate” E. huxleyi and all T. pseudonana cultures (Figure 2). In the absence of other processes explaining this 14C-depletion, we conclude that contribution of blank carbon is the most likely explanation for the observed isotopic shift between pre- and post-growth DIC Δ14C values.
Final DIC-derived C amounts ranged from 66.7 to 140.6 μmol, i.e., 0.8–1.7 mg C (Table 1). Accordingly, we consider combustion and graphitization blanks, combined typically in the range of ∼1 μg C (e.g., Pearson et al., 1998; Shah and Pearson, 2007; Santos et al., 2010), negligible. Hence, it is more likely that the shift in both pre- and post-growth DIC Δ14C values has occurred due to the contribution of some unknown blank source during the experiment or during sample storage until 14C analysis. A significant sample storage effect has been observed for DIC δ13C values due to gas exchange through the septum even in the absence of an atmospheric headspace (Olack et al., 2018). As discussed above, an atmospheric blank C source cannot explain 14C-depletion of DIC during the “Intermediate” E. huxleyi and all T. pseudonana experiments or subsequent sample storage, but likely has to derive from material produced from petroleum sources. However, the only such material used during the experiments were the septa, which are made of chemically highly resistant PTFE. It is also puzzling that the observed shifts are not unidirectional in each of the E. huxleyi and T. pseudonana cultures grown under the same manipulated DIC 14C levels, e.g., 14C-enrichment in the “Fossil” cultures and 14C-depletion in the “Modern” cultures if the actual blank C had some intermediate Δ14C value. The non-unidirectional nature of the shift between pre- and post-growth DIC Δ14C makes it virtually impossible to determine a common blank source in the samples. Moreover, although the pre- and post-growth DIC Δ14C value in the “Modern” E. huxleyi culture is close to the anticipated value of 40‰, it is obvious that a 14C-enriched blank C source was added during this particular experiment, resulting in pre- and post-growth DIC Δ14C values of 60.0 ± 3.4‰ and 83.0 ± 3.5‰, respectively. In this particular DIC sample, the δ13C values are 13C-enriched (–1.0 ± 0.1‰ and 4.2 ± 0.1‰, Figure 3) in comparison to atmospheric δ13C values, which globally were around –8.3‰ in AD2010/AD2011 (Graven et al., 2017), but in agreement with equilibrium fractionation effects (∼9.0‰ for total DIC at pH ∼8.2 and 15°C; Mook, 1986) in the pre-growth DIC sample. With the exception of the “Fossil” T. pseudonana culture, δ13C values in the post-growth DIC are enriched compared to pre-growth DIC as can be expected due to kinetic isotope fractionation during DIC assimilation in algal biomass (Degens et al., 1968). Irrespective of a kinetic effect, however, it is evident that the pre-growth DIC δ13C values differ significantly (5.6–13.8‰) between the E. huxleyi and T. pseudonana cultures of each 14C manipulation experiment further emphasizing significant blank C contribution of unknown origin during the experiments (based on the 14C-depleted DIC signature of the “Modern” T. pseudonana culture). Overall, the large scatter of DIC Δ14C (and δ13C) values and the non-unidirectionality of the pre- and post-growth DIC Δ14C shifts (Figure 2) does not allow us to constrain a common blank C source.
The somewhat random pattern of pre- and post-growth DIC Δ14C values also impairs our ability to accurately quantify the blank C added during biomarker purification. If the DIC Δ14C values were properly constrained (or at least the pre-growth DIC Δ14C value), deviations of biomarker Δ14C values from the DIC Δ14C values could be used to calculate the amount and isotopic composition of the blank C using propagated isotope mass balance (e.g., Shah and Pearson, 2007). In the absence of uncompromised DIC Δ14C values, biomarker Δ14C values can only be compared relative to one another, which may allow blank C calculations. The comparison shows that LC-purified chlorophyll a is significantly more 14C-depleted in the “Modern” and “Intermediate” E. huxleyi and the “Modern” T. pseudonana cultures compared to wet-chemically purified alkenones and the GC-purified C14:0 alkanoic acid (Figure 2). In contrast, chlorophyll a and alkenones as well as chlorophyll a and LMW alkanoic acids agree within 2σ analytical uncertainty in the “Fossil” E. huxleyi culture and the “Intermediate” and “Fossil” T. pseudonana cultures. So far, the wet-chemical alkenone isolation procedure and preparative GC methods have primarily been shown not to add significant blank C to samples, i.e., Δ14C values of pure and processed standards agree within 2σ analytical uncertainty (Mollenhauer et al., 2005; Zencak et al., 2007; Mollenhauer and Rethemeyer, 2009; Wakeham and McNichol, 2014). Theoretically, alkenone and LMW n-alkanoic acid Δ14C values should, thus, mirror DIC Δ14C values. In comparison, LC isolation methods have been shown to add blank C with an Δ14C isotopic composition of approximately –400‰ to –600‰, the amount of which depends on the effluent volume (Shah and Pearson, 2007; Birkholz et al., 2013). This would be consistent with a 14C-depletion of chlorophyll a in comparison to alkenones and LMW alkanoic acids in the “Modern” cultures. However, closer inspection of the actual Δ14C values of compound pairs in our cultures shows that this is not a consistent pattern. For example, the LMW alkanoic acids in the “Modern” and “Intermediate” T. pseudonana cultures agree within 2σ analytical uncertainty, but chlorophyll a is significantly more 14C-depleted in the “Modern” culture while it agrees with LMW alkanoic acids in the “Intermediate” culture (Figure 2). Accordingly, if we use the alkenone or alkanoic acid Δ14C values to calculate the blank for the LC-based chlorophyll a isolation method (Figure 4), a non-unidirectionality of the blank C contribution to the different chlorophyll a samples becomes obvious as well as the resulting large range of possible blank amount and isotopic composition. Assuming alkenones or alkanoic acids were unaffected by extraneous carbon (Supplementary Figures S1–S11) and represent the Δ14C (DIC) value of each culture, extrapolation of the Δ14C and 1/mass values of the respective compound pairs in each culture reveals that the blank C contribution (based on the intercepts) falls somewhere between 0.23 and 0.65 μmol (2.7 to 7.8 μg) with a Δ14C value of –870.9‰ to –251.1‰, respectively, for the E. huxleyi culture and 2.93 μmol (35.3 μg) with a Δ14C value of –651.9‰ in the T. pseudonana culture. Further, if 1σ analytical uncertainties are included, the chlorophyll a isolation blank in the E. huxleyi culture for example ranges from 0.15 μmol with a Δ14C value of –1000.0‰ to 0.89 μmol with a Δ14C value of –223.4‰. Such large ranges are obviously of little help for blank corrections. Whether this simply results from the relative blank C amount in the respective chlorophyll a sample or whether alkanoic acids may in fact also be affected by blank C cannot unequivocally be determined in the absence of reliable DIC Δ14C constraints. The respective kinetic 13C fractionation factors also do not aid in distinguishing these effects since they are similar for the alkenones (–19.2‰ and –20.5‰) or chlorophyll a (–14.4‰ and –13.1‰) in both the “Modern” and “Intermediate” cultures, respectively (Figure 3). Furthermore, in case of the “Fossil” E. huxleyi culture, the good agreement of chlorophyll a and alkenone Δ14C values and their positive offset to pre-growth DIC Δ14C values would suggest that both purification methods are affected by similar 14C-enriched blank C contributions. However, the δ13C value of chlorophyll a in this sample basically mirrors the pre-growth DIC δ13C value, which would imply the absence of any kinetic isotope fractionation during pigment synthesis in this batch. This could result from 13C-enrichment of DIC during batch growth, but DIC uptake was even higher in the “Modern” E. huxleyi culture (Supplementary Table S2) and chlorophyll a is 13C-depleted relative to pre-growth DIC in that culture (and all other cultures). This strongly suggests that the chlorophyll a Δ14C value is affected by some modern blank C. Mass balance calculations to obtain blank C amounts and isotopic compositions based on relative biomarker Δ14C differences, thus, need to be corroborated by reliable DIC Δ14C values. Alternatively, bulk biomass Δ14C values could aid at determining blank C contributions, unfortunately, however, sample size restrictions did not permit bulk analyses in our study.
Figure 4. Blank C characterization for the chlorophyll a isolation procedure assuming alkenones (E. huxleyi) or alkanoic acids (T. pseudonana) represent the “true” Δ14C value for the different 14C manipulation experiments of each algal culture. The intercepts of the respective regression lines represent the Δ14C value and mass (1/μmol) of the respective blank C. Ctotal is defined as the C concentration of the sample plus blank.
Our study illustrates the difficulties associated with natural level 14C manipulation of algal cultures in an attempt to obtain authentic “isotopically labeled” biomarker standards, which are not commercially available. Our data show that we entered a vicious cycle of blank C contamination hampering our initial goal to accurately determine the blank C associated with different compound purification methods for CSRA. Considering that culturing is a laborious and time-consuming approach to obtain authentic standards, researchers should be certain that natural level 14C manipulation of DIC has yielded the anticipated Δ14C values prior to inoculation of algae and that these Δ14C values can be maintained during the course of the experiment (e.g., via continuous sparging with CO2). This, however, is only possible if access to AMS facilities and very quick turnaround is assured to avoid blank C addition during sample storage until inoculation. This possibly restricts the 14C-manipulation culturing approach to laboratories housing both culturing and AMS facilities. In any case, potential blank C addition during sample storage should be tested in future studies and we would also recommend obtaining total biomass Δ14C values if samples sizes permit these analyses.
All datasets generated for this study are included in the article/Supplementary Material.
SK, GM, and BR designed the study. SK, AB, and K-UR performed the analyses. SK wrote the manuscript with input from all authors.
The authors declare that the research was conducted in the absence of any commercial or financial relationships that could be construed as a potential conflict of interest.
This study was funded by the Helmholtz Young Investigator Group “Applications of molecular 14C analysis for the study of sedimentation processes and carbon cycling in marine sediments”. The workshop was funded by the Deutsche Forschungsgemeinschaft (DFG, German Research Foundation) – project number: 422798570.
The authors thank Ralph Kreutz for help with sample extraction and biomarker purification, and the Hanse-Wissenschaftskolleg Delmenhorst, Germany, for sponsoring the “Marine Organic Biogeochemistry” workshop in April 2019. The authors also thank all reviewers for their constructive feedback which helped to improve this manuscript. This is a contribution to the Frontiers in Marine Science Marine Biogeochemistry Research Topic emanating from this workshop.
The Supplementary Material for this article can be found online at: https://www.frontiersin.org/articles/10.3389/fmars.2019.00780/full#supplementary-material
Badger, M. R., Palmqvist, K., and Yu, J.-W. (1994). Measurement of CO2 and HCO3– fluxes in cyanobacteria and microalgae during steady-state photosynthesis. Physiol. Plant. 90, 529–536. doi: 10.1034/j.1399-3054.1994.900314.x
Birkholz, A., Smittenberg, R. H., Hajdas, I., Wacker, L., and Bernasconi, S. M. (2013). Isolation and compound specific radiocarbon dating of terrigenous branched glycerol dialkyl glycerol tetraethers (brGDGTs). Organ. Geochem. 60, 9–19. doi: 10.1016/j.orggeochem.2013.04.008
Burt, W. J., Thomas, H., Hagens, M., Pätsch, J., Clargo, N. M., Salt, L. A., et al. (2016). Carbon sources in the North Sea evaluated by means of radium and stable carbon isotope tracers. Limnol. Oceangr. 61, 666–683. doi: 10.1002/lno.10243
Degens, E. T., Guillard, R. R. L., Sackett, W. M., and Hellebust, J. A. (1968). Metabolic fractionation of carbon isotopes in marine plankton—I. Temperature and respiration experiments. Deep Sea Res. Oceanogr. Abstr. 15, 1–9. doi: 10.1016/0011-7471(68)90024-7
Drenzek, N. J., Hughen, K. A., Montlucon, D. B., Southon, J. R., Santos dos, G. M., Druffel, E. R. M., et al. (2009). A new look at old carbon in active margin sediments. Geology 37, 239–242. doi: 10.1130/g25351a.1
Feely, R. A., Sabine, C. L., Takahashi, T., and Wanninkhof, R. (2001). Uptake and storage of carbon dioxide in the ocean: the global CO2 survey. Oceanography 14, 18–32. doi: 10.5670/oceanog.2001.03
Feng, X., Vonk, J., van Dongen, B., Gustafsson, Ö, Semiletov, I. P., Dudarev, O. V., et al. (2013). Differential mobilization of terrestrial carbon pools in Eurasian Arctic river basins. Proc. Natl. Acad. Sci. U.S.A. 110, 14168–14173. doi: 10.1073/pnas.1307031110
Graven, H., Allison, C. E., Etheridge, D. M., Hammer, S., Keeling, R. F., Levin, I., et al. (2017). Compiled records of carbon isotopes in atmospheric CO2 for historical simulations in CMIP6. Geosci. Model Dev. 10, 4405–4417. doi: 10.5194/gmd-10-4405-2017
Guillard, R. R. L., and Ryther, J. H. (1962). Studies of marine planktonic diatoms: I. Cyctotella nana Hustedt, and Detonula confervacea (Cleve) gran. Can. J. Microbiol. 8, 229–239. doi: 10.1139/m62-029
Ingalls, A. E., Shah, S. R., Hansman, R. L., Aluwihare, L. I., Santos, G., Druffel, E. R. M., et al. (2006). Quantifying archaeal community autotrophy in the mesopelagic ocean using natural radiocarbon. Proc. Natl. Acad. Sci. U.S.A. 103, 6442–6447. doi: 10.1073/pnas.0510157103
Kusch, S., Kashiyama, Y., Ogawa, N. O., Altabet, M., Butzin, M., Friedrich, J., et al. (2010a). Implications for chloro-and pheopigment synthesis and preservation from combined compound-specific δ13C, δ15N, and Δ14C analysis. Biogeosciences 7, 4105–4118. doi: 10.5194/bg-7-4105-2010
Kusch, S., Rethemeyer, J., Schefuß, E., and Mollenhauer, G. (2010b). Controls on the age of vascular plant biomarkers in Black Sea sediments. Geochim. Cosmochim. Acta 74, 7031–7047. doi: 10.1016/j.gca.2010.09.005
Levin, I., Kromer, B., and Hammer, S. (2013). Atmospheric Δ14CO2 trend in Western European background air from 2000 to 2012. Tellus B 65, 57–57.
McNichol, A. P., Osborne, E. A., Gagnon, A. R., Fry, B., and Jones, G. A. (1994). TIC, TOC, DIC, DOC, PIC, POC—unique aspects in the preparation of oceanographic samples for 14C-AMS. Nuclear Instr. Methods Phys. Res. B 92, 162–165. doi: 10.1016/0168-583x(94)95998-6
Mollenhauer, G., and Eglinton, T. I. (2007). Diagenetic and sedimentological controls on the composition of organic matter preserved in California Borderland Basin sediments. Limnol. Oceangr. 52, 558–576. doi: 10.4319/lo.2007.52.2.0558
Mollenhauer, G., Inthorn, M., Vogt, T., Zabel, M., Sinninghe Damsté, J. S., and Eglinton, T. I. (2007). Aging of marine organic matter during cross-shelf lateral transport in the Benguela upwelling system revealed by compound-specific radiocarbon dating. Geochem. Geophys. Geosyst. 8:Q09004. doi: 10.1029/2007GC001603
Mollenhauer, G., Montlucon, D. B., and Eglinton, T. I. (2005). Radiocarbon dating of alkenones from marine sediments: II. Assessment of carbon process blanks. Radiocarbon 47, 413–424. doi: 10.1017/s0033822200035190
Mollenhauer, G., and Rethemeyer, J. (2009). Compound-specific radiocarbon analysis – Analytical challenges and applications. IOP Confer. Ser. Earth Environ. Sci. 5, 1–9.
Mook, W. G. (1986). 13C in atmospheric CO2. Netherlands J. Sea Res. 20, 211–223. doi: 10.1016/0077-7579(86)90043-8
Nielsen, M. V. (1997). Growth, dark respiration and photosynthetic parameters of the coccolithophorid Emiliania huxleyi (prymnesiophyceae) acclimated to different day length-irradiance combinations. J. Phycol. 33, 818–822. doi: 10.1111/j.0022-3646.1997.00818.x
Ohkouchi, N., Xu, L., Reddy, C. M., Montlucon, D., and Eglinton, T. I. (2005). Radiocarbon dating of alkenones from marine sediments: I. Isolation protocol. Radiocarbon 47, 401–412. doi: 10.1017/s0033822200035189
Olack, G. A., Colman, A. S., Pfister, C. A., and Wootton, J. T. (2018). Seawater DIC analysis: the effects of blanks and long-term storage on measurements of concentration and stable isotope composition. Limnol. Oceanogr. Methods 16, 160–179. doi: 10.1002/lom3.10235
Pearson, A., McNichol, A. P., Schneider, R. J., von, R. F., and Zheng, Y. (1998). Microscale AMS 14C measurement at NOSAMS. Radiocarbon 40, 61–75. doi: 10.1017/s0033822200017902
Pearson, A., Seewald, J. S., and Eglinton, T. I. (2005). Bacterial incorporation of relict carbon in the hydrothermal environment of Guaymas Basin. Geochim. Cosmochim. Acta 69, 5477–5486. doi: 10.1016/j.gca.2005.07.007
Rost, B., Kranz, S. A., Richter, K.-U., and Tortell, P. D. (2007). Isotope disequilibrium and mass spectrometric studies of inorganic carbon acquisition by phytoplankton. Limnol. Oceanogr. Meth. 5, 328–337. doi: 10.4319/lom.2007.5.328
Rost, B., Riebesell, U., Burkhardt, S., and Sültemeyer, D. (2003). Carbon acquisition of bloom-forming marine phytoplankton. Limnol. Oceanogr. 48, 55–67. doi: 10.4319/lo.2003.48.1.0055
Ruff, M., Wacker, L., Gäggeler, H. W., Suter, M., Synal, H.-A., and Szidat, S. (2007). A gas ion source for radiocarbon measurements at 200kV. Radiocarbon 49, 307–314. doi: 10.1017/s0033822200042235
Santos, G. M., Southon, J. R., Drenzek, N. J., Ziolkowski, L. A., Druffel, E., Xu, X., et al. (2010). Blank assessment for ultra-small radiocarbon samples: chemical extraction and separation versus AMS. Radiocarbon 52, 1322–1335. doi: 10.1017/s0033822200046415
Scourse, J. D., Wanamaker, A. D., Weidman, C., Heinemeier, J., Reimer, P. J., Butler, P. G., et al. (2012). The marine radiocarbon bomb pulse across the temperate North Atlantic: a compilation of Δ14C time histories from Arctica islandica growth increments. Radiocarbon 54, 165–186. doi: 10.2458/azu_js_rc.v54i2.16026
Shah, S. R., and Pearson, A. (2007). Ultra-microscale (5–25 μg C) analysis of individual lipids by 14C AMS: assessment and correction for sample processing blanks. Radiocarbon 49, 69–82. doi: 10.1017/s0033822200041904
Stuiver, M., and Polach, H. A. (1977). Discussion: reporting of 14C Data. Radiocarbon 19, 355–363. doi: 10.1017/s0033822200003672
Wakeham, S. G., and McNichol, A. P. (2014). Transfer of organic carbon through marine water columns to sediments - insights from stable and radiocarbon isotopes of lipid biomarkers. Biogeosciences 11, 6895–6914. doi: 10.5194/bg-11-6895-2014
Zencak, Z., Reddy, C. M., Teuten, E. L., Xu, L., McNichol, A. P., and Gustafsson, Ö (2007). Evaluation of gas chromatographic isotope fractionation and process contamination by carbon in compound-specific radiocarbon analysis. Anal. Chem. 79, 2042–2049. doi: 10.1021/ac061821a
Keywords: compound-specific radiocarbon analysis, authentic standards, blank, chlorophyll a, alkenones, alkanoic acids, algal cultures, natural level 14C manipulation
Citation: Kusch S, Benthien A, Richter K-U, Rost B and Mollenhauer G (2019) Dead in the Water: The Vicious Cycle of Blanks During Natural Level 14 C Manipulation of Marine Algal Cultures. Front. Mar. Sci. 6:780. doi: 10.3389/fmars.2019.00780
Received: 11 September 2019; Accepted: 03 December 2019;
Published: 20 December 2019.
Edited by:
Cindy Lee, Stony Brook University, United StatesReviewed by:
Tiantian Tang, Harvard University, United StatesCopyright © 2019 Kusch, Benthien, Richter, Rost and Mollenhauer. This is an open-access article distributed under the terms of the Creative Commons Attribution License (CC BY). The use, distribution or reproduction in other forums is permitted, provided the original author(s) and the copyright owner(s) are credited and that the original publication in this journal is cited, in accordance with accepted academic practice. No use, distribution or reproduction is permitted which does not comply with these terms.
*Correspondence: Stephanie Kusch, c3RlcGhhbmllLmt1c2NoQHVuaS1rb2Vsbi5kZQ==
†Present address: Stephanie Kusch, CologneAMS, University of Cologne, Cologne, Germany
Disclaimer: All claims expressed in this article are solely those of the authors and do not necessarily represent those of their affiliated organizations, or those of the publisher, the editors and the reviewers. Any product that may be evaluated in this article or claim that may be made by its manufacturer is not guaranteed or endorsed by the publisher.
Research integrity at Frontiers
Learn more about the work of our research integrity team to safeguard the quality of each article we publish.