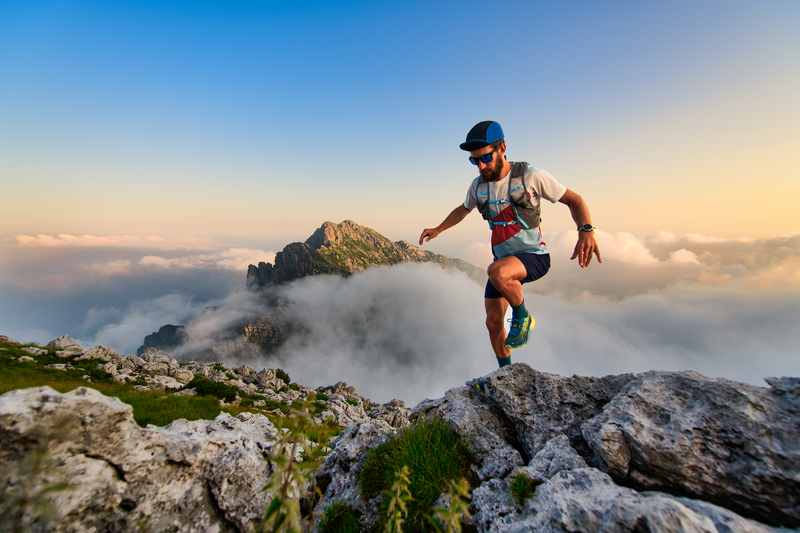
94% of researchers rate our articles as excellent or good
Learn more about the work of our research integrity team to safeguard the quality of each article we publish.
Find out more
ORIGINAL RESEARCH article
Front. Mar. Sci. , 11 October 2019
Sec. Marine Pollution
Volume 6 - 2019 | https://doi.org/10.3389/fmars.2019.00611
This article is part of the Research Topic Aquatic Physiology, Environmental Pollution, Nanotoxicology and Phytoremediation View all 13 articles
Heavy metal (HM) inputs into marine environments and their effect on marine organisms are of major concern. Here, we examined the potential use of two invasive ascidian species, Phallusia nigra and Microcosmus exasperatus, as bio-indicators of 11 HMs in the Mediterranean and Red Sea coasts of Israel. Individuals were collected on a seasonal basis from three sites over 1 year, and analysis was carried out separately for the tunic and the body. Both species accumulated high levels of HMs, which varied seasonally and spatially. In M. exasperatus the majority of HMs were found in the tunic, and in P. nigra in the body, suggesting the need to analyze total individuals in future studies. Hepato-Somatic Index values for M. exasperatus were significantly lower at the polluted site. Investigation of a popular public beach revealed high levels of certain dissolved HMs in both the water and in the ascidians. The wide geographic distribution and high filtration capacity of invasive ascidians offer great potential for their use in monitoring metal pollution in marine environments.
Biological indicators (bio-indicators) are used to measure and assess the health and quality of the environment, by examining the response of these organisms to exposure to external contaminants and the changes in response to this exposure over time (Holt and Miller, 2010; Chiarelli and Roccheri, 2015). In most cases, the risk to human health constitutes the main motivation for the development and research of bio-indicators to assess environmental health. Biological monitoring of marine pollutants presents an advantage compared to chemical analysis of environmental matrices such as seawater samples, as pollutant concentrations in water are often extremely low and their determination presents significant methodological and analytical challenges (Richir and Gobert, 2014; Bellante et al., 2016). Furthermore, live organisms used as indicators can accumulate different substances over a long period, hence providing a time-integrated evaluation of the past environmental status and its biological effects (Schettino et al., 2012). Consequently, the use of bio-indicators has greatly developed in the last decade as a promising alternative approach for the monitoring of environmental pollution (Holt and Miller, 2010; Abdul Jaffar Ali et al., 2015). For example, in order to monitor the aquatic ecosystems of the Doñana National Park, a UNESCO Biosphere Reserve in Southern Spain, the freshwater crayfish, Procambarus clarkia, was used as a bio-indicator (Vioque-Fernández et al., 2009), and bivalves have been most widely used as bio-indicators of the marine environment (Beliaeff et al., 1997; Rodríguez-Ortega et al., 2003; Hedge et al., 2009).
Anthropogenic activities are responsible for a significant increase above natural background values in metal abundances in the environment. Anthropogenic heavy-metals (HMs), introduced into the oceans mainly by river runoff, atmospheric transport, and groundwater seeps, pose a serious threat to human health, living organisms, and natural habitats. Due to their toxicity, persistence, and bioaccumulation, they lead to a reduction in species diversity and abundance, while also accumulating in living organisms and thus entering the food chain (Abreo et al., 2015).
All aquatic invertebrates accumulate metals in their tissues, whether these metals are essential to the animal’s metabolism or not (Rainbow, 2002). Essential trace elements, such as iron (Fe), manganese (Mn), copper (Cu), and zinc (Zn), are vital components of enzymes and play an important role in electron transport reactions. In contrast, aluminum (Al), nickel (Ni), vanadium (V), cadmium (Cd), lead (Pb), and arsenic (As), lack any known biological role in marine invertebrates and exhibit high toxicity if allowed to accumulate in metabolically active sites (Tchounwou et al., 2012; Horwitz et al., 2014). Different invertebrates accumulate trace metals at different concentrations in their tissues, organs, and bodies. Invertebrates dwelling in the same habitat can thus have very different body concentrations of metals, even within closely related taxa (Rainbow, 2002).
There are many factors that influence HMs accumulation in living organisms, with each factor functioning differently, depending on the species and type of metal (Jitar et al., 2014). Aquatic invertebrates take up trace metals either from their surrounding water or from food intake. The bio-concentration of contaminants such as HMs in the bio-indicator marine species can reach up to several orders of magnitude higher than their concentration in the ambient waters (Wang, 2016). The destination of the metal within the organism depends on the physiology of the invertebrate, and whether the metal is used for an essential metabolic purpose. Most metals possess a great affinity to sulfur and nitrogen, which are abundant in every living cell. Due to this affinity, all trace metals are potentially toxic, binding to proteins or other molecules and preventing them from functioning in their intended role (Rainbow, 2002). Studies on the bioaccumulation of HMs in different marine organisms are thus essential in order to expand our knowledge regarding the relative metal bioavailability from different sources (Rainbow, 2002; Aydın-Önen, 2016). Determining the metal concentrations in organisms in the coastal region should constitute an essential part of any assessment and monitoring program of contaminants in the marine ecosystem. Such assessment plays an important contributory role in decision-making regarding an ecosystem’s assessment and management (Usero et al., 2005; Jitar et al., 2014). The choice of an appropriate bio-indicator species thus constitutes an integral part of any successful bio-monitoring program (Chiarelli and Roccheri, 2015).
Here, we took advantage of the wide geographic distribution of invasive ascidians (Chordata, Ascidiacea), which are able to thrive in both contaminated and pristine environments (Lambert and Lambert, 1998), as biological indicators of HMs in marine environments. Ascidians are highly efficient filter-feeding organisms, able to accumulate and concentrate harmful toxicants when present even at low concentrations in their surroundings (Jiang et al., 2010; Treberg et al., 2012). Ascidians actively accumulate HMs, including manganese, iron, cadmium, magnesium, molybdenum, niobium, tantalum, chromium, titanium, and vanadium (Odate and Pawlik, 2007; Gallo and Tosti, 2015; Aydın-Önen, 2016). There have been a number of studies in recent years on ascidians as bio-indicators, using different measurements and indices (physiological, molecular, reproductive) the results of which demonstrate the potential of this group to function as a useful bio-indicator of contamination in the marine environment (Bellas et al., 2004; Radhalakshmi et al., 2014; Aydın-Önen, 2016; Bellante et al., 2016).
Here, we report on the first time-series study conducted along both the Red Sea and the Mediterranean coasts of Israel concomitantly to assess the use of the invasive ascidians Microcosmus exasperatus Heller, 1878 and Phallusia nigra Savigny, 1816 as bio-indicators of HMs. We also sought to determine the distribution of the studied metals in M. exasperatus body tissues: tunic, body wall, gonads, and digestive gland, and to examine any potential physiological changes caused by metal pollution, such as the hepato-somatic and gonad indices. We further determined the heavy metal abundances in seawater from each of the study sites in order to quantify their relation to heavy metal content in the corresponding ascidians.
Invasive ascidians such as M. exasperatus and P. nigra present an ideal combination of both widespread (Shenkar and Loya, 2009) and highly efficient benthic filter-feeders (Jacobi et al., 2018), closely related to vertebrates (Delsuc et al., 2006), thereby enabling us to employ the same organism and methodology on a wide geographic scale, and thus contribute to the development of an ubiquitous method for assessing environmental health.
Sampling was performed by snorkeling at three main research sites in the Mediterranean and Red Sea coasts of Israel, located in Akko (32°55′15″N 35°04′22″E, Mediterranean coast), Mikhmoret (32°22′52″N 34°51′41″E, Mediterranean coast), and Eilat (29°32′51″N 34°57′13.47″E, Red Sea coast), between November 2015 and June 2017. Additional sampling was conducted in April 2017 at “Ha’Sela” beach in Bat-Yam (32°01′27″N 34°44′20″E, Mediterranean coast), a popular recreational beach close to several potential anthropogenic contamination sources, and which served as a site for comparison (Figure 1). According to Herut et al. (2017), the Akko site is frequently polluted, mainly by industrial discharge outlets, and is in proximity to many anthropogenic contamination sources; the Mikhmoret site is considered less disturbed, and the Red Sea site in Eilat is considered moderately polluted, being located at the North Beach area and highly influenced by anthropogenic activity, yet not subjected to industrial discharges as the Akko site (Israel National Monitoring Program at the Gulf of Eilat)1.
Figure 1. Study area and location of sampling sites along the Mediterranean and Red Sea coasts of Israel (marked with a star).
Temperature data for the Akko site was recorded from November 2015 to April 2016, and from June to September 2016 with a data logger device (HOBO pendant® data logger, Onset, MA, United States) attached to a shipwreck in the shallow water area of the beach of “Akko Nautical School.” We encountered difficulties in collecting consistent data due to the reoccurring disappearance of our devices, therefore, the rest of the data were obtained from measurements recorded on the “Sea Temperature” website2. Temperature data for Mikhmoret were obtained from the National Marine Monitoring Program in the Mediterranean Sea operated by Israel’s Ministry of Environmental Protection and by Israel Oceanographic & Limnological Research Ltd. Temperature data for Eilat were obtained from the National Marine Monitoring Program at the Gulf of Eilat operated by Israel’s Ministry of Environmental Protection3 (Figure 2). Taxonomic identification of both species was carried out following Shenkar and Loya (2009).
Seawater samples were collected in acid cleaned bottles (Tarsons, HDPE 250 ml, 584230) by SCUBA or snorkeling from each study site. Sample bottles were pre-cleaned with 10% HNO3 followed by concentrated double-distilled HNO3 and a rinse with MQ water (18.2 MΩ⋅cm–1). After the samples were collected, they were brought to pH < 2 in the laboratory using concentrated (15.5 N) double distilled HNO3 (J.T.BAKER), and then kept at −20°C until further analysis. For final analysis samples were filtered through a 0.22 μm membrane (Pall AcroPak) and 125 ml of seawater were pre-concentrated using the NOBIAS PA-1 chelate resin (Hitachi High Technologies) in a method modified after Biller and Bruland (2012) and Sohrin et al. (2008). The full pre-concentration procedure is described in Appendix 1. Procedural blanks, detection limits and resin recovery are summarized in Supplementary Table S1. Procedural blanks comprise 4.2, 10.9, 0.4, 1.9, 1.5, 6.2, 6.4, 5.1, 0.3, and 1.3% of average sample signal for Al, V, Mn, Fe, Co, Ni, Cu, Cd, Ce, and Pb, respectively. Results of metal concentrations in water are reported in μg/g units in order to allow the calculation of the Bio-accumulation factor (BAF). All pre-concentration steps were performed in a trace-metal clean laboratory (class 1000 laboratory with a class 100 workbench), and all acids and reagents used were either purchased ultra-pure solutions or double distilled in the lab.
For the seasonal monitoring of heavy metal levels in the tunic and body of the investigated species, specimens were collected by snorkeling every 2–3 months, for a period of 20 months (November 2015–June 2017). In each sampling session at the Mediterranean study sites, five individuals of P. nigra and five of M. exasperatus were sampled. In Eilat, Red Sea, only five individuals of P. nigra were sampled each session, as M. exasperatus do not occur at this location. The specimens were placed in Ziploc bags, together with water from their surroundings, and transferred to the lab within 2–3 h of collection. Menthol tablets (Merck, EMPROVE® exp Ph Eur, 105995) were added to relax the animal’s muscles prior to dissection. In the laboratory, the specimens were dissected separating the tunic from the body using ceramic scissors and tweezers to avoid heavy metal contamination, preserved in 50 ml Falcon tubes (SARSTEDT, PP, 62.547.004), and kept at −20°C until further analysis.
For the purpose of determining metal accumulation in the different tissues of M. exasperatus, an additional 5–9 specimens of M. exasperatus (in accordance with their availability in the field on the date of sampling) were collected in September 2016 (Mikhmoret and Akko), February 2017 (Akko), and June 2017 (Mikhmoret and Akko). Upon arrival at the laboratory, samples were dissected and separated into the different tissues: tunic, gonads, liver, and rest of body, and each was put in a separate Falcon tube and stored at −20°C until further preparation. Epibionts were carefully removed from the tunic before weighing, in order to avoid error. Due to the small size of the dissected organs, two to three organs from different individual samples (but from the same sampling session) were combined into a single sample to obtain sufficient weight and element concentration for later analysis. All samples intended for trace metal analysis (−20°C) were frozen at −80°C for at least 24 h and then freeze-dried for 18–24 h (VirTis benchtop K, Bieleved, Germany) prior to the digestion process.
Samples for heavy metal analysis using ICP-MS were treated as follows: approximately 0.1 g of dry tissue sample was taken from each specimen. When a specimen weighed below 0.1 g, the entire specimen was used for digestion, and the weights were documented for further calibration. The dry-weighed tissue of each sample was added to 2 ml 30% H2O2 (J.T.BAKER, ACS, 2186) and 8 ml concentrated HNO3, as follows: dry tissue sample numbers 1–117 were digested using concentrated 65–69% HNO3 (J.T.BAKER, CMOS, 9606) double distilled; and dry tissue sample number 117-395 were digested using concentrated 67–70% HNO3 (Trace Metal Analysis, Fisher Chemical, 7697-37-2). This protocol follows EPA method 3052 (microwave-assisted acid digestion for siliceous and organically based materials). Elemental analysis of seawater and ascidian samples for metals and metalloids was performed by inductively coupled plasma mass spectrometry (ICP-MS, Agilent 7500cx). ICP-MS analyses was conducted at the Freddy & Nadine Herrmann Institute of Earth Science at the Hebrew University, Jerusalem. ICP-MS operating conditions were set at 15, 0.92, and 0.9 L/min for plasma gas flow, carrier gas flow and auxiliary gas flow, respectively.
The BAF represents an estimate of the potential uptake of the metal from both water and food sources (hence, from the dissolved and particulate phases). The bio-accumulation factor for each metal was calculated based on the metal concentrations determined from the collected samples (μg/g) and then divided by that metal’s concentration in the seawater (μg/g) from the same sampling session:
Separate BAFs were calculated for the tunic and for the body of each species.
Following 18 months of study of the seasonal study sites, “Ha’Sela” beach in Bat-Yam, a popular recreational beach in the central region of Israel, was chosen to test the applicability of using P. nigra and M. exasperatus as bio-indicators of metal contamination in their environment. Five specimens of M. exasperatus and five specimens of P. nigra were obtained for HMs and trace elements analysis on 18/05/2017 (spring), including a seawater sample (250 ml). Water and specimens were sampled, prepared, and analyzed as described in the previous sections.
Individuals of M. exasperatus (n = 12–13) were collected by SCUBA or snorkeling, from the Mediterranean study sites during September 2016, February 2017, and April–May 2017. The samples were collected as detailed above, relaxed using menthol tablets, and preserved in 4% formalin solution for several days prior to measurements. For each individual, total wet weight was obtained prior to dissection of the gonads and digestive gland (referred to here as “liver”). The gonadosomatic Index (GI) and Hepatosomatic Index (HSI) were calculated as follows:
Gonadosomatic Index:
Hepatosomatic Index:
Statistical analysis was performed using R software (version 3.4.1, 2017, R Core Team, 2017). For all data, the appropriate test assumptions were determined before selecting the suitable statistical test. When the data of the heavy metal concentration were not normally distributed, log transformation was performed. For the data on metal concentrations in ascidians and for body size, HIS, and GI data, a two-way ANOVA was performed. A t-test was also used to examine differences between the months in the HSI and GI analysis. One-way-ANOVA on transformed data was used to determine differences between the sites (Akko and Mikhmoret) in metal concentrations measured from M. exasperatus different tissues (tunic, body, gonads, and liver). One-way ANOVA was also used to determine significant differences among the sites (Bat-Yam, Akko, Mikhmoret, and Eilat) in the analysis of metals in the body and tunic of the research species, and in the analysis of HSI and GI. Data are presented as average and standard deviation unless denoted otherwise.
All the studied metals from summer 2016 and winter 2017 showed higher concentrations in Akko (Figure 3 and Supplementary Table S2). In Eilat and Mikhmoret, no steady trend could be observed. In summer 2016, winter 2017, and spring 2017, most of the metals demonstrated lower concentrations in Mikhmoret, except for Mn (in summer 2016 a lower concentration was measured in Eilat); Ni (in summer 2016 the concentration was similar to Eilat and in winter and spring 2017 Ni was lower in Eilat) and Cd (in summer 2016 and winter 2017 Cd was lower in Eilat). In fall 2016, all the metals showed higher concentrations in Akko, except for V (concentrations were almost identical at all the sites), and Cd (higher in Eilat). The lowest concentrations during fall 2016 were in the samples from Eilat, except for V (lower in Akko, but very similar concentrations at all sites); Cd and Pb (lower concentrations in Mikhmoret). In spring 2017, “Ha’Sela” beach in Bat-Yam was also examined for comparison, and revealed higher concentrations of all the studied metals compared to the other sites, except for Cu (higher in Akko), and Pb (higher in Eilat). The lowest metal concentrations in this season (spring 2017) were in Mikhmoret, except for Co, Ni and Cd, which were lower in Eilat (Figure 3 and Supplementary Table S2).
Figure 3. Metal concentrations in seawater samples from Mikhmoret, Akko, Eilat, and Bat-Yam, during the months of the study: summer 2016 (A), fall 2016 (B), winter 2017 (C), and spring 2017 (D). Metal concentrations are presented on a log 10 scale.
The concentrations of HMs (Al, V, Mn, Fe, Co, Ni, Cu, Zn, Cd, Ce, and Pb) accumulated in the body and tunic of M. exasperatus and P. nigra, collected from two study sites in the Mediterranean (Akko and Mikhmoret) and from one study site in the Red Sea (Eilat), between fall 2016 and winter 2017 are presented in Figures 4, 5 (full data set is provided in the Supplementary Excel File).
Figure 4. Metal concentrations (μg/g) in the bodies (A–K) and tunics (A’–K’) of M. exasperatus, measured in the specimens sampled from Akko and Mikhmoret, during the different seasons of the study (Spring 2016–Spring 2017). Mean (±SD) is presented for all the metals, except for Co and Pb, for which ±SE is presented. Note that the V concentrations are presented on a logarithmic scale (n = 3–7).
Figure 5. Metal concentrations (μg/g) in the bodies (A–K) and tunics (A’–K’) of P. nigra, measured in the specimens sampled from Akko, Mikhmoret, and Eilat, during the different seasons of the study (Spring 2016–Spring 2017). Mean ± SD is presented for all the metals, except for Al, Fe, and Ce, for which ±SE is presented (n = 3–7).
The mean metal levels in the body and tunic of P. nigra and M. exasperatus based on all the measurements conducted throughout the study are presented in Table 1, arranged in order of decreasing abundance. For an inclusive assessment of the effects of season and study site on variation in the accumulation of metals in the research species, p-values of a two-way ANOVA test for these variables are summarized in the Supplementary Table S3. A significant difference in spatial variation of all the studied metals, apart from Ni, was detected in both the body and tunic of P. nigra (two-way ANOVA, p < 0.05, Supplementary Table S3); while spatial variation in the body and tunic of M. exasperatus did not reveal significant differences for all the studied metals, with different trends in the variation being found. The effect of the seasons was significantly more prominent in the bodies of the two species than in the tunics, and was significant for most of the studied metals (p < 0.05). The season:site interaction was found to be significant in some of the metals in both species’ tunics (Al, Fe, and Ce in M. exasperatus and Co, Cu, Zn, and Cd in P. nigra).
Table 1. The mean metal levels (μg/g), and range in the body and tunic of M. exasperatus (ME body and ME tunic, n = 50) and of P. nigra (PN body, PN tunic, n = 58) measured throughout the study arranged in order of decreasing abundance (from left to right).
In many of the metals, variations existed between body and tunic. The concentrations of all the studied metals in the tunic of M. exasperatus were higher than those in the body at both research sites, except for Zn, which demonstrated an opposite trend (Figure 4).
In P. nigra, in contrast, the concentrations of all the metals in the body were higher than in the tunic, with the exception again of Zn, in which concentrations in the tunic were higher. Cadmium results were inconclusive: some of the concentrations were higher in the tunic than in the body but varied inconsistently among sites and seasons (Figure 5). Seasonal changes in metal accumulation in the tissues of M. exasperatus followed temperature changes in some cases: in the body and tunic of M. exasperatus (Figures 2, 4), most of the highest concentrations of metals were recorded in the cold seasons – fall 2016 and winter 2017, and most of the lowest concentrations in spring 2016/2017.
Seasonal changes in metal concentrations in the body and tunic of P. nigra demonstrated less of a pattern than in M. exasperatus. P. nigra was sampled from Eilat, Red Sea, in addition to Akko and Mikhmoret. Temperatures in Eilat are characterized by a smaller range of change (21–28°C, Figure 2). Some of the metals measured in P. nigra tissues did not seem to follow a seasonal trend (V, Cd, and Zn), while others did, albeit demonstrating different patterns of change for body and for tunic. The highest concentrations of metals in the body of P. nigra were measured in fall 2016 (similar to M. exasperatus), for Al, Mn, Fe, Co, and Ce, for which their lowest concentrations in the tunic were generally in fall and/or winter. For Al, Fe, Co, and Ni, the lowest concentrations were in spring (2016/2017) and summer; and for Al, Mn, Fe, Co, Ni, Cu, Ce, and Pb the highest concentrations were in spring (2016/2017) and summer.
The distribution of the metals in the tunic, body, gonads, and liver of M. exasperatus presented a steady trend (Figure 6 and Supplementary Table S4). For all of them except Cu, Zn, and Cd, the tunic accumulated higher concentrations than the other tissues (60–78%); for all of them except Zn the gonads accumulated the lowest concentrations (4–14%); the liver accumulated higher concentrations of Cu and Cd compared to the other tissues; and the body and gonads accumulated higher concentrations of Zn than the other tissues (30%). There were no significant differences in metal distribution between specimens collected in Akko and in Mikhmoret (one-way ANOVA, p > 0.05).
Figure 6. Metal distribution in the different tissues of M. exasperatus (tunic, body, gonads, and liver), from samples collected from Akko (A) and Mikhmoret (M), in summer 2016, winter 2017, and spring 2017. The data are presented as% of mean concentration (n = 28).
Accumulation of the studied metals in the tunic of the research species differed among the metals: the BAF of Al, Mn, Fe, Co, Ni, and Ce was significantly higher in M. exasperatus tunic than in P. nigra tunic (t-test, p < 0.05), while the BAF for V, and Cd was significantly higher in the tunic of P. nigra than in that of M. exasperatus. BAF of the two species’ bodies for the studied metals, was significantly higher in P. nigra (t-test, p < 0.05) except for Cd and Pb in which no significant differences were found.
The BAF values for the tunic and body of both species are presented in Figures 7A,B and Table 2, arranged in order of decreasing abundance.
Figure 7. Bio-accumulation factor values of M. exasperatus (mean + SE) and P. nigra (ME, PN), for the different metals as calculated for the tunic (A) and for the body (B) based on samples collected in Akko, Mikhmoret, and Eilat, n = 29–43.
Table 2. The mean BAF of M. exasperatus (ME) and P. nigra (PN) body and tunic, arranged in decreasing order, based on samples collected in Akko, Mikhmoret, and Eilat.
Hepato-Somatic Index (HSI) values revealed a significant difference between Akko and Mikhmoret (p < 0.05): mean HSI value in Mikhmoret was significantly higher than in Akko.
A two-way ANOVA analysis also revealed a significant difference in HSI values between average June and September measurements (p < 0.05), and a significant site:season interaction (p < 0.01). The highest mean HSI measurement was from the June sampling from Mikhmoret (1.94, ± 0.43, n = 12, Figure 8A), and the September sampling from Akko (1.26, ± 0.43, n = 12), although T-test analysis revealed a significant difference between mean HSI values of June and September only from Mikhmoret (p < 0.01).
Figure 8. Microcosmus exasperatus’ hepato-somatic index (A) (Mean ± SD) and gonad-index (B) from June and September 2016 and 2017 samples, from Akko and Mikhmoret (n = 11–12).
A two-way ANOVA test for GI revealed similar trends to those found for the HSI analysis (Figure 8B). Although there was no significant difference between site and month in GI values, the highest mean GI was measured in the samples collected from Mikhmoret in June (0.09, ± 0.02); and the lowest mean GI in the samples from Akko in June (0.04, ± 0.02). There was a strong and significant site:season interaction (two-way ANOVA, p < 0.0001), emphasizing the importance of both geographic and seasonal effect in M. exasperatus. For Mikhmoret a t-test analysis revealed a significant difference between Mean GI values in June (n = 12) and September (n = 11, t-test, p < 0.01); but no significant difference for the same period in Akko.
Metal concentrations in seawater samples during spring 2017 (Figure 3) were higher in Bat-Yam in comparison to the other sites for all metals except Cu, Zn, and Cd. Concentrations of HMs accumulated in M. exasperatus and P. nigra samples from spring 2017, collected from Bat-Yam and compared to Akko, Mikhmoret, and Eilat, are presented in Figures 9, 10. Similar to the findings presented in the previous section, metal concentrations in the tunic of M. exasperatus from Bat-Yam revealed concentrations 1.5–27-fold higher than in the body, for all metals except Cu, which was found in almost equal concentrations in tunic and body; and Zn, which was 4-fold more concentrated in the body than in the tunic of M. exasperatus. A similar contrast was found in P. nigra samples from spring 2017: metal concentrations were 1.6–18-fold higher in the body than in the tunic, except for Cd and Zn, which were 3.8 and 1.5-fold higher, respectively, in the tunic than in the body.
Figure 9. Metal concentrations (μg/g) in the bodies (A–K) and tunics (A’–K’) of M. exasperatus, measured in samples from Bat-Yam, Akko, and Mikhmoret, in spring 2017 (Mean ±SD) (n = 3–7).
Figure 10. Metal concentrations (μg/g) in the bodies (A–K) and tunics (A’–K’) of P. nigra, measured in samples from Bat-Yam, Mikhmoret, and Eilat in spring 2017 (Mean ± SD) (n = 3–7).
In M. exasperatus, significant differences among the study sites were found in concentrations of V, Co, and Cu in the body and of Co, Cu, Zn, and Ce in the tunic (one-way ANOVA, p < 0.05, Figure 9). In P. nigra significant differences were found in concentrations of V, Cu, and Zn in the body samples, and concentrations of V, Mn, Co, Cd, and Pb in the tunic samples (Figure 10, One-Way ANOVA, p < 0.05).
Mean metal bio-accumulation factors (BAF) of the studied metals, for the samples collected in spring 2017 from Bat-Yam, were found to be lower than the calculated BAF from the other sites, for the majority of metals (Supplementary Table S5). GI values in Bat-Yam were significantly lower (0.031 ± 0.009, n = 12, one-way ANOVA p < 0.05), in comparison to Mikhmoret (0.089 ± 0.023), while HSI values did not significantly differ between Akko (1.17 ± 0.44, n = 12) and Bat-Yam (1.22 ± 0.23, n = 12, one-way ANOVA p > 0.05).
Both M. exasperatus and P. nigra have demonstrated an ability to accumulate large quantities of the studied metals in their tissues (between ∼5,000 and 2.5 × 106 times higher compared to ambient seawater concentrations). The concentrations of the metals varied between the research species and among the different tissues examined. The findings from the study at a popular public beach (Ha’Sela Beach, Bat-Yam) further illustrate the feasibility of using M. exasperatus and P. nigra as biological indicators of metal contamination in a variety of habitats.
By conducting separate analyses for the tunic and the body of our two ascidian study species we were able to detect the differences in the mechanism of metal accumulation between the two. The metal content in the tunic of M. exasperatus was higher than in its body, while the opposite was the case for P. nigra. A study by Abdul Jaffar Ali et al. (2015) demonstrated a similar trend of accumulation in P. nigra. Since all the vital life functions such as absorption, circulation, storage, breeding, etc., take place in the body, the accumulation of metals is expected to be higher in the body than in the tunic (Abdul Jaffar Ali et al., 2015). A similar phenomenon of variation in accumulation pattern in organisms belonging to the same taxon was also documented in the past (Rainbow, 1998). P. nigra is characterized by a very smooth tunic, while M. exasperatus is densely covered with epibionts (Gewing et al., 2016). Such differences may influence the primary site of metal accumulation and should be taken into consideration according to the particular ascidian tissue used for metal analysis. Radhalakshmi et al. (2014) studied the accumulation of V, Cu, Pb, and Cd in the whole tissue of five species of ascidians, including M. exasperatus, and found that Cu accumulated at the highest concentrations in M. exasperatus (body and tunic), followed by V, Pb, and Cd in that order, as also reflected in the BAF values. Their results are similar to those of the current research, in which these four metals accumulated in M. exasperatus in the following order of concentration V > Cu > Pb > Cd.
In the present study, metal accumulation in the body and tunic of M. exasperatus and P. nigra was measured at different sites and seasons, revealing significant effects of both parameters. Significant spatial differences in the tunic and body of P. nigra were detected for all the studied metals, except for Ni, which showed no seasonal or spatial differences. Chiffoleau et al. (2004) examined the differences in Ni levels over a period of 1 year in mussels (Mytilus edulis) and oysters (Crassostrea gigas) and did not detect any significant variations in this metal’s levels, whereas a significant difference in the levels of V was detected. Ni is a common element and known for its toxicity, persistence, and affinity for bio-accumulation (Eisler, 1998). However, it is considered to be essential for various biological processes, mostly at very low concentrations (Muyssen et al., 2004). It has been shown to accumulate in the tissues of crayfish, primarily in the exoskeleton, which might indicate that this tissue is involved in the excretion of this metal (Kouba et al., 2010). In the current study, Ni was found to preferentially accumulate (64%) in the tunic of M. exasperatus, suggesting that the tunic may participate in the excretion of this metal in this species.
Seasonal differences in metal levels were significant only for some of the metals: in the tunic of P. nigra – Al, Mn, Fe, Cu, and Ce; and in the body of P. nigra – all the metals except Ni, Cu, and Zn. The majority of the metals in the tunic and body of M. exasperatus varied significantly in quantity between seasons: in the tunic Al, V, Co, and Zn; in the body? all the metals except Zn and Cd. In a recent study conducted by Aydın-Önen (2016) on heavy metal accumulation by the solitary ascidian Styela plicata, only Cu showed seasonal changes. However, in the present study seasonal differences in heavy metal accumulation were evident for many of the metals. In the body and tunic of M. exasperates and in the body of P. nigra, the highest concentrations of metals were measured in the fall and winter, when water temperatures were relatively low. As established in previous studies, food supply and environmental temperature vary over an annual cycle and, in response to these variations, metal uptake and accumulation by marine organisms is affected (Stacey and Driedzic, 2010; Wang, 2016). Temperature affects the speciation of metals in the water, thus affecting their bio-availability (Tan et al., 2013). Additionally, food availability and temperature influence the organism’s water pumping rate and metabolic rate as well as growth rate, all of which affect the metal accumulation process (Stacey and Driedzic, 2010; Wang, 2016).
In the above-noted study by Aydın-Önen (2016), an analysis of Cd, Cu, Pb, V, and Zn was carried out on the tissues of S. plicata, an ascidian belonging to the same order as M. exasperatus (Stolidobranchia). The accumulated concentrations of these metals were very similar to those of the current study, and of the same order of magnitude.
The bio-accumulation factor (BAF) calculated here should be cautiously interpreted, being based on a single measurement of seawater at each site. It nonetheless provides an estimation of the ability of a particular organism to accumulate metals from its surroundings. BAF values for the body of P. nigra were greater than those of M. exasperatus for all the metals. The BAF values for the tunics of both species varied among the metals, and were not consistently higher in one species. In general, for the BAF values of the tunic and body together, P. nigra showed higher values for most of the metals, revealing its promising potential as a pollutant indicator. However, because P. nigra lacks a digestive gland (a major point of interest for the present study), ideally, a combination of P. nigra and M. exasperatus would enable a broader assessment of metal contamination in the environment.
In the present study accumulation of metals was in the following order: Zn > Cu > V > Pb > Cd, for the liver and the body (which was separated from the gonads and liver and contained the branchial basket). Bellante et al. (2016) examined metal accumulation in the liver and branchial sac of S. plicata and found their accumulation to be in the following order: Cu > Zn > V > Pb > Cd for the branchial sac and V > Cu > Pb > Zn > Cd for the liver (only metals relevant for the current study are shown here). Similar findings for body tissue to those provided by Bellante et al. (2016) were also found here. However, the accumulation of metals in the liver differ here: Zn > Cu > V > Pb > Cd. Bellante et al. (2016) also found the accumulation of metals in the digestive gland of the mussel Mytilus galloprovincialis to be: Zn > Cu > V > Pb > Cd, similar to that found in the current study for the liver and body of M. exasperatus.
There were no significant differences in the distribution of metals in the different tissues between Akko and Mikhmoret. This suggests that the distribution of the studied metals in the tissues of M. exasperatus does not depend on the site or the level of contamination at the site, but on the identity of the metal and its regulation and metabolism in the examined tissues in this species.
In the present study, a high accumulation of Cu in the liver of M. exasperatus was also apparent. Cu is an essential element for normal growth and metabolism of all organisms, and was found to be a key element in the respiratory protein hemocyanin, present in certain mollusks and arthropods. In crustaceans, for example, relatively high concentrations of Cu have been found in the liver (Rainbow, 2002; Kouba et al., 2010). Cd is considered a non-essential element, and thus has no required minimum concentration in the body of living organisms, and needs to be swiftly detoxified or excreted (Rainbow, 2002). Because the liver has a major role in the detoxification of metals, it is sometimes the major tissue in which non-essential HMs accumulate (Muyssen et al., 2004; Kouba et al., 2010; El-Moselhy et al., 2014). The highest levels of Cd accumulation in the present study were measured in P. nigra tunic, suggesting that this tissue also plays a role in the excretion of this metal.
Canli and Atl (2003) examined the distribution of Fe, Cu, Pb, Cd, and Zn in certain tissues of six species of fish. The mean results showed that for most of the species the accumulation in the liver was in the following order: Fe > Zn > Cu > Pb > Cd; and the accumulation in the gills: Fe > Zn > Pb ≈ Cu > Cd. Their results for fish liver are identical to those found in the liver of M. exasperatus in the present study. Their order of accumulation in the gills (which, similar to the branchial sac of ascidians, function as the organ of oxygenation and encounter large amounts of seawater), is also very similar to that found here for M. exasperatus. The differential distribution of each metal in the different tissues of M. exasperatus may be due to the different biological requirements of the tissues and to their possessing different metal-binding proteins. Metal concentrations found in the tunic may also have originated in part from the epifauna on the tunic, despite our attempt to thoroughly remove them. The high metal accumulation capacity of the tunic of M. exasperatus should be further examined in order to determine the role of these metals in this tissue, and the reason for their high presence there. It is crucial therefore to consider the differential organ accumulation capabilities when choosing a particular tissue as a biological marker of metal accumulation, or use total individual HMs analysis (body and tunic) as a marker.
“Ha’Sela” beach (Bat-Yam) is a popular recreational beach, situated in the center of Israel. It is highly visited throughout the year, and is subjected to many potential anthropogenic effects. Although the marine environment of Akko is considered as one of the most polluted in the country, the seawater collected at “Ha’Sela” beach revealed the highest concentrations of metals of all four sites sampled in spring. There are sporadic reports of sewage leakage and ground water pollution from this area, which can reach “Ha’Sela” beach with the currents, and demands future monitoring of the high levels reported here. M. exasperatus GI values were significantly lower in Bat-Yam compared to the other sites during this period, demonstrating an inverse relationship with the metal concentrations in the water samples. This may indicate seasonal changes in the reproductive cycles of different populations of M. exasperatus; or it may be a consequence of the relatively high metal pollution in the area. Studies have shown that some metals can disrupt various enzymatic mechanisms and DNA-related processes (Kouba et al., 2010), influence reproductive processes (Gallo et al., 2011; Gallo and Tosti, 2013), and cause other sub-lethal and lethal effects. For example, cadmium is known to increase atresia (the breakdown of oocytes) in the fish Oryzias javanicus, which can accumulate high Cd levels in its liver, resulting in a reduction in GI (Pereira et al., 1993).
Sindhe and Kulkarni (2004) conducted toxicity tests for several metals on Notopterus notopterus, and showed that upon exposure to HMs at sub-lethal concentrations, both GI and HSI were reduced. This could be the reason for the low GI and HSI values found for Bat-Yam, compared to the other sites.
Mean bio-accumulation factors (BAF) for the body and tunic of the samples collected for Ha’Sela Beach (Bat-Yam) were lower than the average values for the other study sites for the same season. DeForest et al. (2007) examined the relationship between bio-accumulation factors and exposure concentrations. They observed a statistically significant inverse relationship between these factors in seven out of the eight metals examined (the relevant metals for the current study: Cd, Cu, and Pb). Thus, a relatively high BAF value observed for a metal may also derive from the exposure concentration and from other metal and species-specific factors that influence the bioaccumulation of the metal. This could explain the lower BAF values in Bat-Yam, a site that was shown here to be the most metal-polluted of all four study sites. The Bat-Yam case study demonstrates the potential of P. nigra and M. exasperatus to function as bio-indicators on a wide geographic scale.
The present study is the first to examine concomitantly the potential use of two highly common invasive species as bio-indicators in two different bodies of water: the tropical Red Sea and the Mediterranean Sea. The findings have revealed the high potential of both species to serve as bio-indicators of metal accumulation in the marine environment. Both M. exasperatus and P. nigra accumulated high levels of HMs, up to 2.5 × 106-fold higher than the ambient seawater concentrations. The metal concentrations in the two species varied seasonally and spatially, and their distribution within the tissues of each species also differed. While in M. exasperatus the tunic served as the main site of bio-accumulation, in P. nigra the majority of metals accumulated in the body. Such physiological differences should be taken into consideration in future studies using ascidians as bio-indicators. A significant association between low HSI and GI indexes and heavy-metal levels was found in M. exasperatus, indicating a physiological response. The beach case study chosen for this research further illustrates the feasibility of using M. exasperatus and P. nigra as biological indicators of metal contamination on a large geographic scale, providing valuable information on the ecosystem’s health, and on the presence and bioavailability of pollutants.
All datasets generated for this study are included in the manuscript/Supplementary Files.
RT-M, AT, TB, and NS designed the study and analysis and wrote the manuscript. RT-M and NS research team have conducted the field work.
In this work, NS was supported by the Israel Science Foundation Grant Number 2182/15, additional support was provided by the Israel Science Foundation Grant Number 834/19 to AT.
The authors declare that the research was conducted in the absence of any commercial or financial relationships that could be construed as a potential conflict of interest.
The Supplementary Material for this article can be found online at: https://www.frontiersin.org/articles/10.3389/fmars.2019.00611/full#supplementary-material
Abdul Jaffar Ali, H., Tamilselvi, M., Akram, A. S., Kaleem Arshan, M. L., and Sivakumar, V. (2015). Comparative study on bioremediation of heavy metals by solitary ascidian, Phallusia nigra, between thoothukudi and vizhinjam ports of india. Ecotoxicol. Environ. Saf. 121, 93–99. doi: 10.1016/j.ecoenv.2015.04.052
Abreo, N. A. S., Macusi, E. D., Cuenca, G. C., Ranara, C. T. B., Cardona, L. T., and Arabejo, G. F. P. (2015). Nutrient enrichment, sedimentation, heavy metals and plastic pollution in the marine environment and its implications on Philippine marine biodiversity: a review. IAMURE Int. J. Ecol. Conserv. 15, 111–167.
Aydın-Önen, S. (2016). Styela plicata: a new promising bioindicator of heavy metal pollution for eastern Aegean Sea coastal waters. Environ. Sci. Pollut. Res. Int. 23, 21536–21553. doi: 10.1007/s11356-016-7298-5
Beliaeff, B., O’Connor, T. P., Daskalakis, D. K., and Smith, P. J. (1997). US mussel watch data from 1986 to 1994: temporal trend detection at large spatial scales. Environ. Sci. Technol. 31, 1411–1415. doi: 10.1021/es9606586
Bellas, J., Beiras, R., and Vázquez, E. (2004). Sublethal effects of trace metals (Cd, Cr, Cu, Hg) on embryogenesis and larval settlement of the ascidian Ciona intestinalis. Arch. Environ. Contam. Toxicol. 46, 61–66. doi: 10.1007/s00244-003-0238-7
Bellante, A., Piazzese, D., Cataldo, S., Parisi, M. G., and Cammarata, M. (2016). Evaluation and comparison of trace metal accumulation in different tissues of potential bioindicator organisms: macrobenthic filter feeders Styela plicata, Sabella spallanzanii, and Mytilus galloprovincialis. Environ. Toxicol. Chem. 35, 3062–3070. doi: 10.1002/etc.3494
Biller, D. V., and Bruland, K. W. (2012). Analysis of Mn, Fe, Co, Ni, Cu, Zn, Cd, and Pb in seawater using the Nobias-chelate PA1 resin and magnetic sector inductively coupled plasma mass spectrometry (ICP-MS). Mar. Chem. 130–131, 12–20. doi: 10.1016/j.marchem.2011.12.001
Canli, M., and Atl, I. G. (2003). The relationships between heavy metal (Cd, Cr, Cu, Fe, Pb, Zn) levels and the size of six mediterranean fish species. Environ. Poll. 121, 129–136. doi: 10.1016/s0269-7491(02)00194-x
Chiarelli, R., and Roccheri, M. (2015). Marine invertebrates as bioindicators of heavy metal pollution. OJ Metal. 4, 93–106. doi: 10.4236/ojmetal.2014.44011
Chiffoleau, J. F., Chauvaud, L., Amouroux, D., Barats, A., Dufour, A., Pécheyran, C., et al. (2004). Nickel and vanadium contamination of benthic invertebrates following the “Erika” wreck. Aquat. Living Resour. 17, 273–280. doi: 10.1051/alr:2004032
DeForest, D. K., Brix, K. V., and Adams, W. J. (2007). Assessing metal bioaccumulation in aquatic environments: the inverse relationship between bioaccumulation factors, trophic transfer factors and exposure concentration. Aquat. Toxicol. 84, 236–246. doi: 10.1016/j.aquatox.2007.02.022
Delsuc, F., Brinkmann, H., Chourrout, D., and Philippe, H. (2006). Tunicates and not cephalochordates are the closest living relatives of vertebrates. Nature 439, 965–968. doi: 10.1038/nature04336
Eisler, R. (1998). Nickel Hazards to Fish, Wildlife, and Invertebrates: a Synoptic Review. Biological Science Report 1998-0001. Washington, DC: United States Geological Survey.
El-Moselhy, K. M., Othman, A. I., Abd El-Azem, H., and El-Metwally, M. E. A. (2014). Bioaccumulation of heavy metals in some tissues of fish in the red sea. egypt. Egypt. J. Basic Appl. Sci. 1, 97–105. doi: 10.1016/j.ejbas.2014.06.001
Gallo, A., Silvestre, F., Cuomo, A., Papoff, F., and Tosti, E. (2011). The impact of metals on the reproductive mechanisms of the ascidian Ciona intestinalis. Mar. Ecol. 32, 222–231. doi: 10.3390/md11093554
Gallo, A., and Tosti, E. (2013). Adverse effect of antifouling compounds on the reproductive mechanisms of the ascidian Ciona intestinalis. Mar. Drugs 11, 3554–3568. doi: 10.3390/md11093554
Gallo, A., and Tosti, E. (2015). The ascidian Ciona intestinalis as model organism for ecotoxicological bioassays. J. Mar. Sci. Res. Dev. 5:e138
Gewing, M. T., Bronstein, O., Nagar, L. R., Granot, I., Frid, O., and Shenkar, N. (2016). First record of the non-indigenous ascidian Microcosmus exasperatus, Heller 1878, in cyprus. Mar. Biodivers 46, 937–941. doi: 10.1007/s12526-015-0442-5
Hedge, L. H., Knott, N. A., and Johnston, E. L. (2009). Dredging related metal bioaccumulation in oysters. Mar. Poll. Bull. 58, 832–840. doi: 10.1016/j.marpolbul.2009.01.020
Herut, B., Segal, Y., et al. (2017). The National Monitoring Program of Israel’s Mediterranean waters – Scientific Report for 2016. IOLR Report H48c/2017. Haifa: Israel Oceanographic and Limnological Research.
Holt, E. A., and Miller, S. W. (2010). Bioindicators: using organisms to measure environmental impacts. Nat. Educ. Knowl. 3:8.
Horwitz, R., Borell, E. M., Fine, M., and Shaked, Y. (2014). Trace element profiles of the sea anemone Anemonia viridis living nearby a natural CO2 vent. PeerJ 2:e538. doi: 10.7717/peerj.538
Jacobi, Y., Yahel, G., and Shenkar, N. (2018). Efficient filtration of micron and submicron particles by ascidians from oligotrophic waters. Limnol. Oceanogr. 63, S267–S279
Jiang, A., Yu, Z., and Wang, C. H. (2010). Bioaccumulation of cadmium in the ascidian Styela clava (Herdman 1881). Afr. J. Mar. Sci. 31, 289–295. doi: 10.2989/ajms.2009.31.3.2.990
Jitar, O., Teodosiu, C., Oros, A., Plavan, G., and Nicoara, M. (2014). Bioaccumulation of heavy metals in marine organisms from the romanian sector of the black Sea. N. Biotechnol. 32, 369–378. doi: 10.1016/j.nbt.2014.11.004
Kouba, A., Buřič, M., and Kozák, P. (2010). Bioaccumulation and effects of heavy metals in crayfish: a review. Water Air Soil Poll. 211, 5–16. doi: 10.1007/s11270-009-0273-8
Lambert, C. C., and Lambert, G. (1998). Non-indigenous ascidians in southern California harbors and marinas. Mar. Biol. 130, 675–688. doi: 10.1007/s002270050289
Muyssen, B. T., Brix, K. V., DeForest, D. K., and Janssen, C. R. (2004). Nickel essentiality and homeostasis in aquatic organisms. Environ. Rev. 12, 113–131. doi: 10.1139/a04-004
Odate, S., and Pawlik, J. R. (2007). The role of vanadium in the chemical defense of the solitary tunicate, Phallusia nigra. J. Chem. Ecol. 33, 643–654. doi: 10.1007/s10886-007-9251-z
Pereira, J. J., Mercaldo-Allen, R., Kuropat, C., Luedke, D., and Sennefelder, G. (1993). Effect of cadmium accumulation on serum vitellogenin levels and hepatosomatic and gonadosomatic indices of winter flounder (Pleuronectes americanus). Arch. Environ. Contam. Toxicol. 24, 427–431. doi: 10.1007/bf01146157
R Core Team (2017). R: A Language and Environment for Statistical Computing. Vienna: R Foundation for Statistical Computing. Available at: https://www.R-project.org
Radhalakshmi, R., Sivakumar, V., and Ali, H. A. J. (2014). Analysis of selected species of ascidians as bioindicators of metals in marine ecosystem. Int. J. Curr. Microbiol. Appl. Sci. 3, 755–764.
Rainbow, P. S. (1998). Phylogeny of trace metal accumulation in crustaceans. in Metal Metabolism in Aquatic Environments, eds W. J. Langston, and M. J. Bebianno (Boston, MA: Springer), 285–319. doi: 10.1007/978-1-4757-2761-6_9
Rainbow, P. (2002). Trace metal concentrations in aquatic invertebrates: why and so what? Environ. Poll. 120, 497–507. doi: 10.1016/s0269-7491(02)00238-5
Richir, J., and Gobert, S. (2014). A reassessment of the use of Posidonia oceanica and Mytilus galloprovincialis to biomonitor the coastal pollution of trace elements: new tools and tips. Mar. Poll. Bull. 89, 390–406. doi: 10.1016/j.marpolbul.2014.08.030
Rodríguez-Ortega, M. J., Grøsvik, B. E., Rodríguez-Ariza, A., Goksøyr, A., and López-Barea, J. (2003). Changes in protein expression profiles in bivalve molluscs (Chamaelea gallina) exposed to four model environmental pollutants. Proteomics 3, 1535–1543. doi: 10.1002/pmic.200300491
Schettino, T., Caricato, R., Calisi, A., Giordano, M. E., and Lionetto, M. G. (2012). Biomarker approach in marine monitoring and assessment: new insights and perspectives. Open Environ. Sci. 6, 20–27.
Shenkar, N., and Loya, Y. (2009). Non-indigenous ascidians along the mediterranean coast of Israel. Mar. Biodiver. Rec. 2:e116.
Sindhe, V., and Kulkarni, R. (2004). Gonadosomatic and hepatosomatic indices of the freshwater fish Notopterus notopterus (Pallas) in response to some heavy metal exposure. J. Environ. Biol. 25, 365–368.
Sohrin, Y., Urushihara, S., Nakatsuka, S., Kono, T., Higo, E., Minami, T., et al. (2008). Multielemental determination of GEOTRACES key trace metals in seawater by ICPMS after preconcentration using an ethylenediaminetriacetic acid chelating resin. Anal. Chem. 80, 6267–6273. doi: 10.1021/ac800500f
Stacey, J. E., and Driedzic, W. R. (2010). Temporal variability in, and impact of food availability on vanadium and iron concentrations in Ciona intestinalis tissues (Tunicata, Ascidiacea). J. Exp. Mar. Biol. Ecol. 386, 11–18. doi: 10.1016/j.jembe.2010.01.004
Tan, Q., Ke, C., and Wang, W. (2013). Rapid assessments of metal bioavailability in marine sediments using coelomic fluid of sipunculan worms? Environl. Sci. Technol. 47, 7499–7505. doi: 10.1021/es401112d
Tchounwou, P. B., Yedjou, C. G., Patlolla, A. K., and Sutton, D. J. (2012). Heavy metal toxicity and the enviornment. in Molecular, Clinical and Environmental Toxicology, ed Luch A. (Basel: Springer), 133–164.
Treberg, J. R., Stacey, J. E., and Driedzic, W. R. (2012). Vanadium accumulation in ascidian coelomic cells is associated with enhanced pentose phosphate pathway capacity but not overall aerobic or anaerobic metabolism. Comp. Biochem. Physiol. B Biochem. Mol. Biol. 161, 323–330. doi: 10.1016/j.cbpb.2011.12.007
Usero, J., Morillo, J., and Gracia, I. (2005). Heavy metal concentrations in molluscs from the Atlantic coast of southern Spain. Chemosphere 59, 1175–1181. doi: 10.1016/j.chemosphere.2004.11.089
Vioque-Fernández, A., Alves de Almeida, E., and López-Barea, J. (2009). Assessment of doñana national park contamination in Procambarus clarkii: integration of conventional biomarkers and proteomic approaches. Sci. Total Environ. 407, 1784–1797. doi: 10.1016/j.scitotenv.2008.11.051
Wang, W. X. (2016). Bioaccumulation and biomonitoring. in Marine Ecotoxicology Current Knowledge and Future Issues, eds J. Blasco, P. M. Chapman, O. Campana, and M. Hampel (Cambridge, MA: Academic Press), 99–119 doi: 10.1016/b978-0-12-803371-5.00004-7
A total of 125 ml of filtered (0.22 μm) seawater were bought up to pH 6.1 ± 0.2 with 3.7M Ammonium Acetate (NH4Ac), made up from trace metal grade Ammonium Hydroxide and Acetic Acid (Sigma Aldrich). Before loading the sample, resin columns (Biorad) were conditioned with a sequence of 1N HNO3, MQ water (18.2 MΩ⋅cm–1) and 0.05M NH4Ac (pH of 6.1 ± 0.2). After loading the sample, columns were rinsed with 0.05M NH4Ac to remove major salts and finally eluted with 1N HNO3. Concentrations were determined by a calibration curve of gravimetric standard addition to trace metal clean seawater (purified three times in NOBIAS PA-1 chelate resin). Concentration factors were calculated gravimetrically by weighing seawater and eluent tubes before and after pre-concentration. To evaluate procedural blanks, concentrated HNO3 and 3.7M NH4Ac (same amount as added to the samples) were added to 3 ml of trace metal clean seawater and were pre-concentrated as samples. Resin recovery was assessed by comparing the slopes of the seawater standards (extracted on the resin) to the slopes of standard addition to 1N HNO3, which represent a 100% recovery.
Keywords: benthic ecology, metal accumulation, biomonitoring Programs, tunicates, Red Sea, Mediterranean Sea
Citation: Tzafriri-Milo R, Benaltabet T, Torfstein A and Shenkar N (2019) The Potential Use of Invasive Ascidians for Biomonitoring Heavy Metal Pollution. Front. Mar. Sci. 6:611. doi: 10.3389/fmars.2019.00611
Received: 10 June 2019; Accepted: 17 September 2019;
Published: 11 October 2019.
Edited by:
Vincenzo Parrino, University of Messina, ItalyReviewed by:
Xuan-Vy Nguyen, Vietnam Academy of Science and Technology, VietnamCopyright © 2019 Tzafriri-Milo, Benaltabet, Torfstein and Shenkar. This is an open-access article distributed under the terms of the Creative Commons Attribution License (CC BY). The use, distribution or reproduction in other forums is permitted, provided the original author(s) and the copyright owner(s) are credited and that the original publication in this journal is cited, in accordance with accepted academic practice. No use, distribution or reproduction is permitted which does not comply with these terms.
*Correspondence: Noa Shenkar, c2hlbmthcm5AdGF1ZXgudGF1LmFjLmls
Disclaimer: All claims expressed in this article are solely those of the authors and do not necessarily represent those of their affiliated organizations, or those of the publisher, the editors and the reviewers. Any product that may be evaluated in this article or claim that may be made by its manufacturer is not guaranteed or endorsed by the publisher.
Research integrity at Frontiers
Learn more about the work of our research integrity team to safeguard the quality of each article we publish.