- 1Catalan Institution for Research and Advanced Studies (ICREA), Barcelona, Spain
- 2Institut de Ciència i Tecnologia Ambientals (ICTA-UAB), Universitat Autònoma de Barcelona, Barcelona, Spain
- 3Earth and Planetary Sciences, McGill University, Montreal, QC, Canada
- 4Atmospheric and Oceanic Sciences, University of California, Los Angeles, Los Angeles, CA, United States
- 5Global Fishing Watch, Washington, DC, United States
It is well-established that phytoplankton growth can be limited by the vanishingly low concentrations of dissolved iron found in large areas of the open ocean. However, the availability of iron is not typically considered an important factor in the ecology of marine animals, including fish. Here, we compile observations to show that the iron contents of lower trophic level organisms in iron-limited regions can be an order of magnitude less than the iron contents of most fish. Although this shortfall could theoretically be overcome if iron assimilation rates were very high in fish, observations suggest this is not the case, consistent with the high recommended iron contents for mariculture feed. In addition, we highlight two occurrences among fish living in iron-poor regions that would conceivably be beneficial given iron scarcity: the absence of hemoglobin in Antarctic icefish, and the anadromous life history of salmon. Based on these multiple lines of evidence, we suggest that the iron content of lower trophic level organisms can be insufficient to support many fish species throughout their life cycles in iron-poor oceanic regions. We then use a global satellite-based estimate of fishing effort to show that relatively little fishing activity occurs in high nitrate low chlorophyll (HNLC) regions, the most readily identified iron-poor domains of the ocean, particularly when compared to satellite-based estimates of primary production and the observed mesozooplankton biomass in those waters. The low fishing effort is consistent with a low abundance of epipelagic fish in iron-limited regions, though other factors are likely to contribute as well. Our results imply that the importance of iron nutrition extends well beyond plankton and plays a role in the ecology of large marine animals.
Introduction
Iron is an essential element for virtually all organisms, playing a central role in many components of the photosynthetic machinery, respiratory reactions, DNA synthesis, and for the transport and storage of oxygen. Iron can be toxic at high concentrations, but in the oceans, the scarcity of dissolved iron tends to be more of a problem. Low dissolved iron concentrations are widely recognized to play a role in limiting the growth of marine phytoplankton (Marchetti and Maldonado, 2016), most importantly in the high nitrate low chlorophyll (HNLC) regions that constitute more than one quarter of the open ocean surface (Sarmiento and Gruber, 2006) (Figure 1). In these large regions, the dissolved nutrient nitrate persists at relatively high concentrations at the ocean surface throughout the year without causing strong phytoplankton blooms (Moore et al., 2013), a striking contrast with the adjacent coastal regions in which high nitrate concentrations would lead to rapid uptake by chlorophyll-rich phytoplankton. Experiments have shown that deliberate iron addition to HNLC waters accelerates the growth rates of phytoplankton and produces more biomass, confirming their iron-limited status (de Baar et al., 2005; Boyd et al., 2007). Iron scarcity has also been shown to limit the growth and abundance of marine heterotrophic bacteria that compete with phytoplankton for this resource (Tortell et al., 1999).
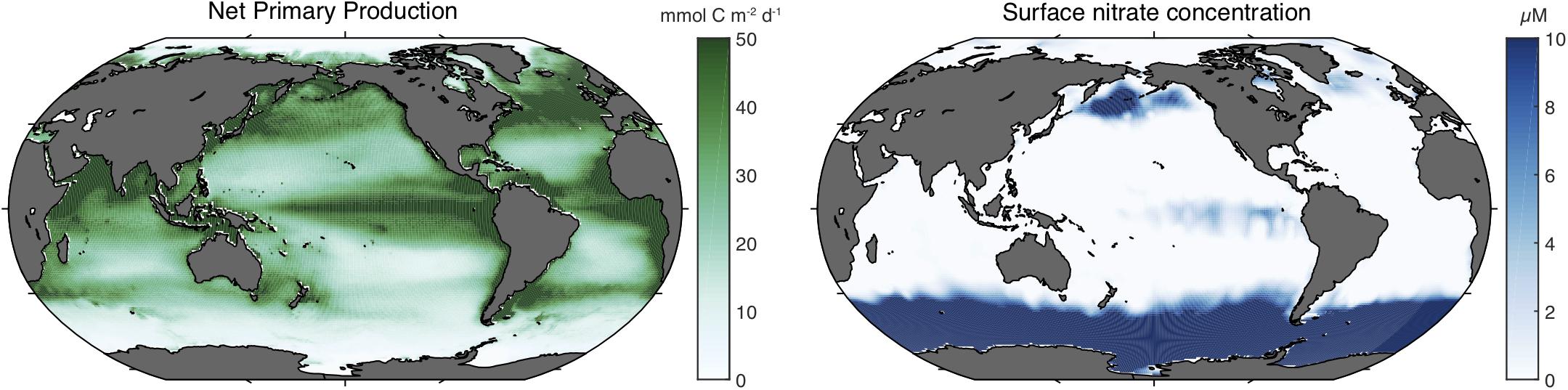
Figure 1. Net primary production and sea surface nitrate concentrations in the global ocean. The left panel shows the annual mean net primary production (in mmol C m– 2 day– 1) as estimated from satellite-observed sea surface color and temperature averaged from three algorithms (Dunne et al., 2007). The right panel shows the minimum monthly sea surface nitrate concentration (in μM) from the World Ocean Atlas (Boyer, 2013).
Despite its demonstrated importance for microbes, little attention has been paid to the possibility that iron availability is important for multicellular marine life. A relatively small number of studies have considered how higher trophic level organisms might impact the iron cycle, including estimates of iron extraction from the ocean by industrial fishing (Moreno and Haffa, 2014), the impact of whales on iron recycling (Lavery et al., 2010), and the roles of animals in ecosystem iron budgets (Maldonado et al., 2016; Ratnarajah et al., 2018). However, these studies all focus on the role of animals in modifying the iron limitation of phytoplankton, rather than the impact of iron availability on the animals themselves. There has been some suggestion that the low iron content of phytoplankton in HNLC regions may limit the growth rates of zooplankton (Chen et al., 2011; Baines et al., 2016), but this has not been widely investigated. We are not aware of prior work that has considered the possibility that the highly variable availability of iron in the ocean environment could be a factor in determining the growth, abundance and distribution of non-planktonic marine animals.
Here, we provide an overview of the state of knowledge regarding iron contents and requirements of marine organisms, first for plankton and then for fish, along with theoretical expectations for how iron contents will change between trophic levels. We then compare these observations with the conditions under which anemia has been shown to occur in fish grown in experimental aquaculture, and discuss two notable fish taxa with characteristics consistent with adaptation to low iron availability. Finally, we show that fishing effort appears to be anomalously low in iron-limited regions, which is consistent with relatively low fish biomass in these regions. Based on these lines of evidence, we propose the new hypothesis that iron availability plays an important role in the ecology of marine fish.
Iron Limitation of Phytoplankton
Iron is derived from rocks and supplied to the ocean surface primarily by rivers, continental dust and mobilization from the seafloor (Tagliabue et al., 2017). Dissolved iron concentrations tend to be relatively high in most coastal waters given their proximity to iron sources, and can exceed 10 nmol L–1 (Johnson et al., 1999). In contrast, dissolved iron concentrations are very low in much of the open ocean (often less than 0.1 nmol L–1 at the surface). This scarcity can be attributed to the fact that, in oxygenated seawater, iron is dominantly present as Fe3+ and has very low solubility (Liu and Millero, 2002). In fact, almost all of the dissolved iron pool is maintained in solution through complexation with organic molecules (Gledhill and Buck, 2012), without which it would be lost to sinking particles.
Iron has long been recognized as an essential nutrient that can potentially limit phytoplankton growth (Gran, 1931; Harvey, 1937), but it was only three decades ago that measurement techniques became sufficiently sensitive to quantify the concentrations in iron-poor surface ocean waters. Measurements showed extremely low concentrations in the HNLC waters of the subarctic North Pacific, and the addition of iron was shown to increase phytoplankton growth rates, supporting the idea that HNLC regions owe their existence to iron limitation (Martin and Fitzwater, 1988). Although the “iron hypothesis” was met with skepticism given competing ideas about light limitation and grazing (Banse, 1990), repeated experiments supported the growth-limiting role of iron in the North Pacific (Martin et al., 1990). Subsequent work confirmed that the addition of dissolved iron also accelerates phytoplankton growth in the other HNLC regions (primarily the Southern Ocean and eastern equatorial Pacific). These findings led to the broadly accepted understanding that the surface nitrate accumulation in HNLC regions reflects its slow uptake by phytoplankton due to iron limitation (Boyd et al., 2007; Moore et al., 2013). Iron limitation of phytoplankton has also been demonstrated outside of HNLC regions, such as the California Current (Hutchins et al., 1998), so the absence of nitrate at the surface cannot be taken as indicating an abundance of iron. Nonetheless, we make use of HNLC regions here as clearly identifiable ocean domains that are generally iron-poor.
Although iron limitation slows the growth rates of phytoplankton, they do continue to grow in HNLC regions, and some taxa are able to achieve large reductions in their Fe:C by modifying their cellular machinery (Strzepek and Harrison, 2004; Marchetti and Maldonado, 2016; Strzepek et al., 2019) (Supplementary Table 4). Eukaryotic phytoplankton appear to be particularly capable of lowering their cellular Fe:C, with some cultured diatoms containing less than 5 μmol Fe (mol C)–1 (Strzepek et al., 2011; Marchetti and Maldonado, 2016). In contrast, the cyanobacteria that dominate oligotrophic waters appear to maintain higher cellular Fe:C, more than 40 μmol Fe (mol C)–1 (Twining et al., 2010; Shire and Kustka, 2015; Marchetti and Maldonado, 2016). Consequently, lower iron contents are generally found for planktonic samples in iron-limited open ocean regions. The low iron contents of suspended particles found in these regions may be further amplified by an absence of iron-rich non-living detritus compared to coastal waters (Ho et al., 2007; King et al., 2012; Twining and Baines, 2013). Thus, the organic particles at the base of the food chain in HNLC regions can have very low Fe:C, compared to iron-rich coastal waters.
Elemental Transfer From Food to Consumer
Heterotrophs rely on their food to provide energy, in the form of reduced carbon, but also for the other elements required to construct their tissues (Sterner and Elser, 2002; Karimi et al., 2010). Thus, essentially all of the elements that compose the marine ecosystem are extracted from seawater by primary producers and are transferred through the web of heterotrophs via feeding. The dependence of heterotrophic organisms on the essential elements present in their food is often referred to as an aspect of “food quality” (Elser et al., 2000; Sterner and Elser, 2002).
In general, for some element “X,” the relationship between the X:C of a consumer (where C is carbon) and that of its food is given by the ratio of the two elemental trophic efficiencies,
The elemental trophic efficiency τ, as defined here, is the fraction of the element consumed that is incorporated in tissue, net of all loss through egestion of feces and excretion of dissolved compounds (Figure 2), and integrated over the full lifetime of the organism. For C, the trophic efficiency is also referred to as the “Gross Growth Efficiency,” and approximates the ratio of new biomass constructed to the biomass of prey consumed. Trophic assimilation efficiencies vary by organism and with food characteristics, and are lower than the uptake assimilation efficiency (A/I, Figure 2) for elements that are subsequently excreted. The theoretical limits of τC can exceed 0.7 for single-celled organisms and be as high as 0.5 for multi-cellular organisms, but they tend to be much lower in practice, often varying between 0.15 and 0.25 for ectotherms (Sterner and Elser, 2002). The trophic efficiency for C is much less than the assimilation efficiency across the gut, A/I, because most of the C is respired to support metabolism, and subsequently excreted (E). Thus, if we assume a τC of 0.20, a τX of 0.20 would result in X:Cconsumer being equal to X:Cfood, whereas a τX of 0.40 would cause X:Cconsumer to be twice that of X:Cfood. The ability of a consumer to homeostatically control their X:C relative to their food therefore depends on their capacity to modify τX and/or τC independently of each other.
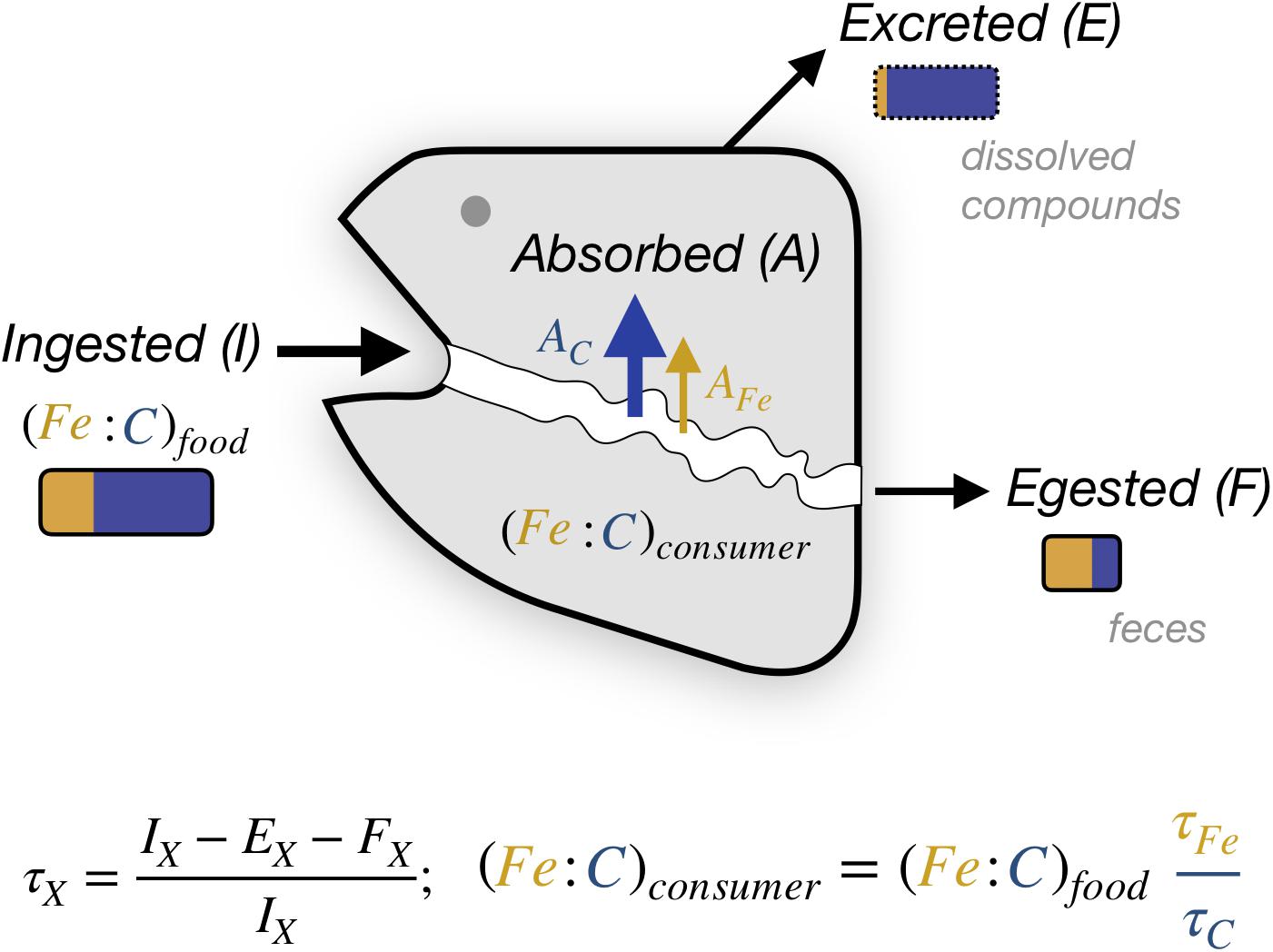
Figure 2. Elemental transfer from food to animal. The gray shape represents an animal that, for an element X, incorporates in biomass a lifetime-averaged fraction τX of the total ingested elemental flux IX. This differs from the absorbed flux AX (also termed gut assimilation) due to the excretion flux EX. Orange and blue rectangles illustrate the approximate relative changes expected for Fe and C, respectively, between food, feces and excretion for a growing animal. Note that feces may also include dissolved compounds.
For the essential element N, the fraction assimilated across the gut wall (A/I) is quite high, so that τN could potentially be much higher than τC, allowing a consumer to readily construct tissues with higher N:C than its prey. Since consumers tend to maintain N:C that is not very different than their food, they often excrete most of the N they consume. As we discuss next, the case appears to be very different for Fe, given that Fe uptake assimilation efficiency tends to be quite low among animals. We first consider the experimental evidence for Fe assimilation efficiencies in zooplankton.
Iron in Heterotophic Marine Plankton
Few experimental studies have attempted to estimate zooplankton τFe or Fe assimilation efficiencies. Those that do exist suggest that τFe is generally low (Hutchins and Bruland, 1994) and that, when confronted with iron-poor phytoplankton, zooplankton do not substantially increase their Fe assimilation efficiencies (Hutchins et al., 1995; Schmidt et al., 1999). This is not to say that the assimilation is constant: the fraction of total iron that is assimilated by copepods in culture has been shown to vary significantly. However, the small number of available studies appear to show that the Fe assimilation efficiency is most strongly related to the iron content within the prey cytoplasm (Chen et al., 2014), with little assimilation of non-cytoplasmic iron, rather than adjusting to compensate for the iron content of their diet. This finding mirrors the cytoplasm-dependent uptake of other metals by copepods (Reinfelder and Fisher, 1991), but contrasts with the strong homeostatic regulation of N:P among zooplankton. The dependence on food iron content implies that, rather than being globally constant, the Fe:C of zooplankton communities would be pulled toward the Fe:C of their food (Hutchins et al., 1995; Chase and Price, 1997; Schmidt et al., 1999; Chen et al., 2014). Consistent with this expectation, the Fe:C of zooplankton in the ocean has been found to vary by more than an order of magnitude, in concert with changes in phytoplankton Fe:C, with reported values exceeding 200 μmol Fe (mol C)–1 in iron-rich coastal regions and less than 14 μmol Fe (mol C)–1 in iron-poor regions of the Southern Ocean (Supplementary Table 5).
Despite the limited ability to compensate for low Fe:C food, zooplankton are not excluded from iron-limited waters. On the contrary, as shown in Figure 3, mesozooplankton abundances are quite high in HNLC regions of the ocean. It has been suggested that low iron availability retards the growth rates of zooplankton in these waters (Chase and Price, 1997; Chen et al., 2011), as it does phytoplankton, and likely contributes to determining which species are present according to their physiology and ecological strategies (Chen et al., 2014; Baines et al., 2016). For example, Antarctic krill (Euphausia superba), which are relatively abundant in the strongly iron-limited waters of the open Southern Ocean, can have very low Fe:C (Supplementary Table 5). Intriguingly, Antarctic krill have been observed migrating to the seabed, where they ingest detrital particles that appear to contribute to their iron nutrition (Schmidt et al., 2011, 2016), which may be an adaptation to the poor iron content of their food.
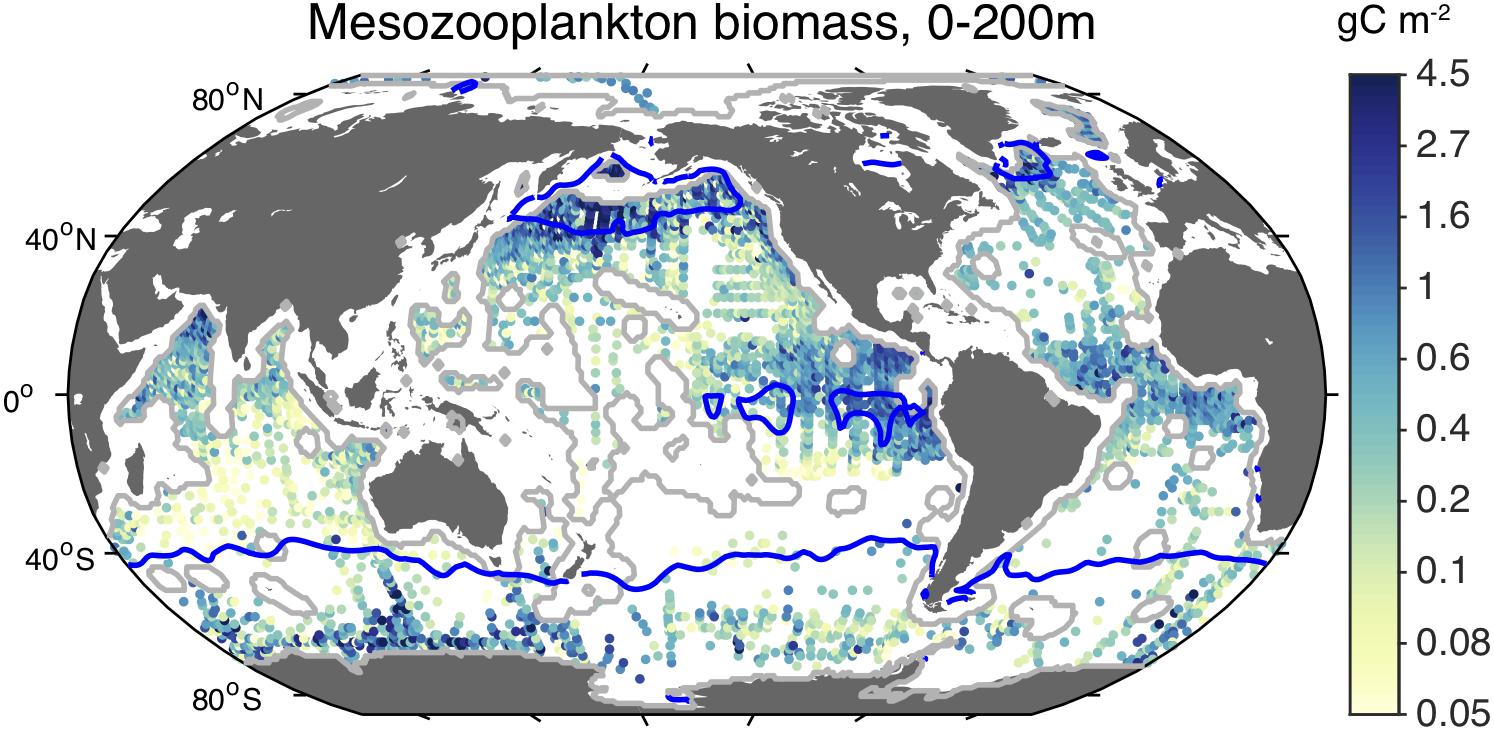
Figure 3. Observed concentration of epipelagic mesozooplankton. Shaded dots indicate the average carbon concentration of mesozooplankton biomass collected in net tows within the upper 200 m of the water column (Moriarty and O’Brien, 2013). Blue contour lines indicate HNLC regions, identified as surface waters with minimum monthly nitrate concentrations > 3 μM (Boyer, 2013).
Although the paucity of data leaves many open questions as to how iron limitation affects zooplankton, and our limited data compilation is strongly biased, it is clear that zooplankton Fe:C varies over at least an order of magnitude in the ocean, and can be very low in iron-limited waters. To first order, zooplankton Fe:C appears to follow the local phytoplankton Fe:C, consistent with an average τFe/τC close to 1.
Iron in Marine Fish
Fish use iron for many biochemical purposes, but one important use is the storage and transport of oxygen (Bury and Grosell, 2003). Iron is found at the center of hemoglobin and myoglobin, which bind oxygen for vascular transport and storage, respectively (Beard et al., 1996). In vertebrates, as much as 80% of the total iron content can be bound within these two metalloproteins (Kuhn et al., 2016). Although rainbow trout have been shown to take up iron across their gills in iron-rich freshwaters, this pathway requires very high dissolved iron concentrations (half-saturation constant of 21 nM) (Cooper and Bury, 2007) so that it should be negligible at the concentrations found in iron-poor seawater (less than 0.2 nM). Thus, the iron supply of marine fish in low-iron waters is likely to come entirely from their food, unless they have evolved a much higher affinity gill uptake system that has not yet been investigated. It is not known if fish have a regulatory mechanism for increasing iron excretion rates in order to prevent iron toxicity, suggesting that they may rely on down-regulating uptake when consuming iron-rich food (Bury et al., 2012).
Compared to plankton, there are few available measurements of Fe:C in whole marine fish. Figure 4 summarizes published, peer-reviewed measurements (see Supplementary Materials for details) made on experimentally reared Atlantic salmon, which ranged from 16 to 43 μmol Fe (mol C)–1 when consuming normal feed (Shearer, 1994; Andersen et al., 1996), and four groups of wild fishes in the Southern Ocean (three Nototheniidae and one myctophid), which ranged from 10 to 26 μmol Fe (mol C)–1 (Honda et al., 1987). The precise locations of the Southern Ocean samples are not given, but at least two were coastal (“near Showa Station”). The vertical bar within the whole fish range indicates the average value of 25 ± 7 μmol Fe (mol C)–1 for whole fish according to the meta-analysis of Prabhu et al. (2016). Larval fish are not included in the figure, but have been found to consistently contain between 9 and 17 μmol Fe (mol C)–1 (Wang and Wang, 2018).
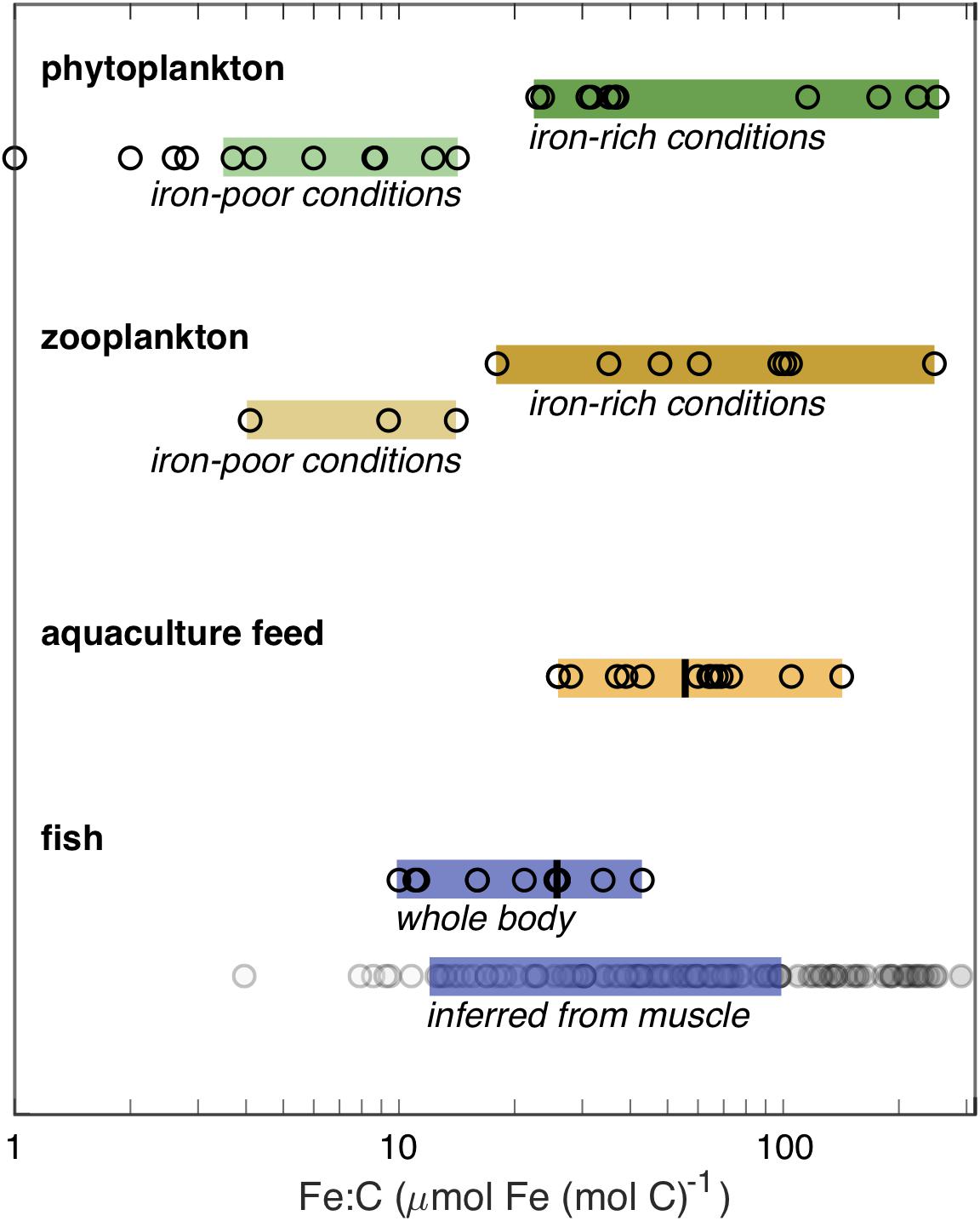
Figure 4. Measured Fe:C molar concentration ratios from published sources (see Supplementary Materials). The upper portion shows the Fe:C measured in plankton, differentiated by whether they were grown under iron-rich or iron-poor conditions. The very low phytoplankton values are not included within the colored-bar range as they were grown under an extreme iron limitation in culture. The lower portion shows measured adult fish Fe:C, using the mean of multiple measurements when available, and recommended Fe:C in feed for aquaculture of marine fish species, with vertical lines showing the mean values estimated by Prabhu et al. (2016). For the inferred whole-fish Fe:C, filled circles show upper (dark) and lower (pale) estimates assuming that muscle Fe:C is 14 and 45% of the whole-fish Fe:C, respectively; the blue bar indicates the most confident range, spanning from the upper estimate for the smallest measured value to the lower estimate for the largest measured value.
Measurements on the iron content of edible fish fractions (i.e., some portion of the fish muscle) are much more widely available than those on whole fish, due to their relevance for human nutrition. These measurements suggest that Fe:C may vary more significantly among fish than would be discerned from the handful of whole fish measurements, with reported values ranging from 2 to more than 40 μmol Fe (mol C)–1 (Supplementary Table 6). Interpreting these values is complicated by the fact that the iron content of fish muscle tissue can vary widely within a given fish. For example, red muscle contains more iron-rich myoglobin than white muscle, which can lead to more than ten-fold higher iron concentrations in red muscle (Carpene et al., 1998). Unfortunately, the published studies do not always identify whether the analyzed muscle tissues were white or red. In addition, even higher concentrations of iron can occur in organs including the liver and gill. Available comparisons suggest that muscle Fe:C is 14–45% of the whole fish Fe:C value (Honda et al., 1987), which would imply a total possible range from 4–12 to >90–300 μmol Fe (mol C)–1 on the basis of the muscle measurements (Figure 4). We would emphasize that this is a highly speculative conversion, and would greatly benefit from further whole fish measurements across a broader range of taxa. Nonetheless, the muscle data suggest that the whole body data, which are dominated by cultured salmon and Southern Ocean fish, lie at the lower end of the spectrum, and that the Fe:C of many fish can be higher.
When we compare the tentative range of fish Fe:C with plankton Fe:C (Figure 4), two important features are apparent. First, the Fe:C range of fish overlaps with the lower end of the Fe:C range of plankton in Fe-rich waters. Second, the Fe:C range of fish does not extend to the lowest Fe:C of plankton found in HNLC waters, even though some of the whole fish samples were collected from the broadly iron-limited Southern Ocean. Thus, this fragmentary view suggests that fish Fe:C is similar to – or lower than – the plankton of iron-rich waters, but is higher than the plankton of HNLC waters.
Following the reasoning illustrated in Figure 2, the low Fe:C of iron-limited plankton relative to fish would demand that a planktivorous fish in an HNLC region have a high τFe or a low τC. For example, maintaining an Fe:C of 20 μmol Fe (mol C)–1 by feeding on iron-poor zooplankton with an Fe:C of 10 μmol Fe (mol C)–1 would require a τFe/τC of about 2. So, given a standard τC of 0.2, the fish would need a τFe of 0.4. This would be much higher than τFe in humans, which ranges from 0.02 to 0.23 (Beard et al., 1996). The alternative possibility that τFe/τC could be raised by lowering τC through physiological or behavioral adaptations is theoretically interesting, but would equate to a sub-optimal use of the available food energy, which could be expected to reduce fitness. Following this reasoning, a high τFe would be the preferred means by which fish could raise their Fe:C.
Rather than having unusually high τFe, it appears that marine teleosts, the dominant fish group, are no better at assimilating iron than other vertebrates. They may even have a disadvantage to other groups, since their osmotic regulation strategy requires maintaining an alkaline intestinal environment, which results in the production of carbonate minerals (Wilson et al., 2002) and may interfere with iron uptake (Bury et al., 2012). This is consistent with relatively low measured iron somatic assimilation efficiencies among marine fish, which are estimated by feeding radio-isotope labeled food to cultured fish and measuring their retention of the radio-isotope over the following hours. Although not directly comparable to lifetime-integrated τFe, these reported assimilation efficiencies are <0.20 when fed artificial feed (Wang and Wang, 2016) and <0.08 for live feed (Wang et al., 2019). Fish do show an ability to regulate their assimilation, with lower assimilation efficiencies occurring in response to iron-rich feed (Wang and Wang, 2016), however, we were unable to find evidence of assimilation efficiencies above 0.20, consistent with a limit to the capacity for up-regulation, as found in mammals (Beard et al., 1996). The persistently low assimilation efficiencies may be attributable, in part, to the difficulty of accessing iron within tightly bound components of their food, as illustrated by the egestion of exoskeleton-bound trace metals by juvenile fish (Reinfelder and Fisher, 1994). A large fraction of inaccessible iron is also consistent with high measured Fe:C in fish feces, which exceed the Fe:C in fish stomachs by a factor of 10 or more (Geesey et al., 1984). Together, these observations imply a τFe/τC ≲ 1, suggesting that it is very difficult for fish to elevate their Fe:C above the Fe:C of their food.
Anemia Among Fish in Aquaculture
The apparent inability for fish to enrich their Fe:C significantly above that of their prey suggests that the survival of a given fish would require the average Fe:C of all prey items to be similar to, or greater than, the minimum whole fish Fe:C requirement for that species. This then raises the question of what the minimum Fe:C might be, and how fish welfare might deteriorate as their Fe:C approaches this minimum. Hypothetically, fish could experience a significant welfare decrease for any reduction below their optimal Fe:C or, alternatively, they may be able to cope very well over a broad range of Fe:C and then experience a sharp mortality threshold at the minimum Fe:C.
Studies on fish grown in mariculture, i.e., marine aquaculture, provide an experimental vantage on this question. Mariculturalists have long recognized the occurrence of anemia in fish, and have studied the problem for decades (Sakamoto and Yone, 1978; Watanabe et al., 1997). Some experiments in which fish were fed low-iron diets have shown changes in blood chemistry (Andersen et al., 1996) and growth retardation (Cooper et al., 2006) without causing mortality, suggesting that fish welfare does respond to Fe:C above the minimum threshold for immediate survival. As a result, aquaculture feed recommendations for marine fish give minimum Fe:C ratios that vary by species (Supplementary Table 9), with an average of 54 ± 15 μmol Fe (mol C)–1 according to Prabhu et al. (2016).
As shown in Figure 4, the recommended fish feed Fe:C values lie well within the range provided by coastal zooplankton prey, and tend to be equal to or higher than whole fish, as would be expected from the low τFe/τC for fish discussed above. The feed recommendations exceed the measured Fe:C of iron-limited oceanic zooplankton by a factor of 5–30. Some caution must be applied in comparing these Fe:C ratios directly, as the fish feeds are often supplemented with inorganic iron salts among which the iron can occur in varying proportions of Fe2+ and Fe3+, and which would be expected to be assimilated differently than the forms in which iron occurs in wild fish prey (Andersen et al., 1997). Nonetheless, a recent experimental study using live rotifer feed provided a minimum dietary recommendation of 30 μmol Fe (mol C)–1 (Wang and Wang, 2018), which falls within the range of recommended artificial feeds. Overall, these experiments provide direct evidence that iron-poor food can negatively impact the growth of marine fish, and suggest that the Fe:C levels for optimal fish growth can be well above those of the plankton in HNLC regions.
Possible Adaptations to Low Iron Availability
If iron availability can indeed place a constraint on fish growth, as it does for plankton, one would expect to find evolutionary adaptions to iron scarcity among fish, just as they are found among phytoplankton. One conceivable type of adaptation would be for fish to reduce their requirements for iron-rich proteins such as hemoglobin. Alternatively, fish could adapt their behavior to take advantage of iron-rich forage at places or times that it is available, as might be achieved through migrations between iron-rich and iron-poor environments. We identify two examples of fish groups that are consistent with each one of these feasible adaptation strategies.
First, fish of the suborder Notothenioidei, found only in the Southern Ocean, exhibit unique physiological adaptations that greatly reduce their iron requirements. The Notothenioidei diversified rapidly after the separation of Antarctica and South America by tectonic forces opened the Drake Passage in the late Eocene, and this single group now dominates the Antarctic shelves to an astonishing degree, comprising >90% of the fish biomass (Eastman, 2005). Notothenioids generally have reduced erythrocyte number, hemoglobin concentrations and hemoglobin diversity compared to temperate and tropical species (Verde et al., 2007), and the family Channichthyidae (“white-blooded icefish”) have gone to the extreme measure of eliminating the use of hemoglobin altogether, a unique occurrence among vertebrates (Ruud, 1954; Kock, 2005). Several species of icefish have even eliminated the use of myoglobin in their hearts (Sidell and O’Brien, 2006). These extremely unusual features have been most often associated with the low temperatures and high dissolved oxygen concentrations of Antarctic waters, which could reduce the requirement for oxygen transportation and storage (Kock, 2005). However, the loss of hemoglobin is accompanied by dramatic adaptations to maintain sufficient oxygen supply to their tissues, including up to a five-fold increase in ventricle size and four-fold increase in total blood volume compared to red-blooded fish of similar size (Kuhn et al., 2016). Even the increased blood volumes cannot overcome what appear to be many profound disadvantages, and explaining why the absence of hemoglobin persists has been recognized as an evolutionary conundrum (Sidell and O’Brien, 2006), even cited as an example of a deleterious adaptation or “disaptation” (Garofalo et al., 2009).
We propose that, rather than being an example of disaptation, the absence of hemoglobin in the Channichthyidae is a successful adaptation to the low iron availability in most parts of the Southern Ocean. The iron content of the white blood in icefish is on the order of one-twentieth that of standard red fish blood (Ruud, 1954), reflecting a vastly reduced requirement for iron. This adaptation may only be feasible given the high oxygen content of cold, well-ventilated Antarctic waters, but we propose that this is a secondary factor. In contrast to the cold-water explanation, the iron hypothesis can explain why no white-blooded fish occur in the arctic, where O2 is equally high but iron is more readily available. Indeed, although Arctic cod and Antarctic notothenioids both produce antifreeze glycoproteins, recognized as an example of convergent evolution to their similarly frigid environments (Chen et al., 1997), Arctic cod have multiple forms of hemoglobin that followed a very different evolutionary history (Verde et al., 2003). The unique characteristics of the icefish are therefore consistent with adaptation to low iron concentrations, facilitated by the cold, oxygen-rich environment.
Second, we suggest that the anadromous lifestyle of salmon provides an advantage for the exploitation of iron-poor forage, most importantly in the subarctic Pacific where they are an abundant epipelagic predator (Brodeur et al., 1999). As discussed above, larval fish have no iron reserves, so that they need to match their food content with the iron required to grow on a daily basis (Wang and Wang, 2016). However, as they age their relative growth rates decrease and they store increasing amounts of iron in their livers (Andersen et al., 1996), building up a reserve of iron. It therefore seems likely that rapidly growing larval and juvenile fish would be more immediately dependent on an iron-rich food supply, whereas more slowly growing mature individuals are able to store surplus iron in their livers that can then be drawn on to survive for longer periods on relatively iron-poor forage. If correct, this would suggest that iron availability could be a particularly important concern for determining the location of spawning, and the habitats of larval and juvenile fish.
The anadromous strategy of salmon, whereby spawning, larval and juvenile phases occur in iron-rich streams, estuaries and coastal waters, while adults gain a greater proportion of their diets from the relatively iron-limited offshore waters (Hansen and Quinn, 1998), would therefore appear to be a good strategy for exploiting the abundant iron-poor forage available in the open subarctic Pacific (Brodeur et al., 1999). The degree to which salmon follow this strategy is sure to vary among species, given that different varieties spend differing portions of their lives in coastal vs. offshore environments (Quinn, 2018). Despite the likelihood for variations among species, we suggest that the overwhelming success of salmon in the North Pacific reflects, at least in part, the ability of the anadromous life cycle to overcome key bottlenecks of iron nutrition at critical life stages.
The Case for Iron
We have identified three convergent lines of evidence that appear to support a role for iron in the ecology of marine fish. First, our compilation of published organismal Fe:C contents and τFe suggests that the dietary Fe:C requirements of fish can exceed what would be provided by zooplankton in iron-limited waters. Second, studies of anemia among fish raised in experimental mariculture confirm the importance of sufficient dietary iron supply for fish growth. Third, some evolutionary features of fish living in iron-limited waters – most dramatically, the Antarctic ice fish – are consistent with adaptation to low iron availability. Based on these three lines of evidence, we hypothesize that the highly variable availability of iron in the ocean plays a role in the ecology of marine fish that has, thus far, gone unrecognized.
On the one hand, this proposed role for iron could simply influence the relative abundances of species, and might be significant only under strong iron scarcity. For example, it may do no more than exclude fish with the highest iron requirements from the most iron-poor waters, where they would be outcompeted by fish with lower iron requirements. However, it is also conceivable that the total abundance of marine fish could be low in iron-poor regions relative to iron-rich regions. Testing this latter hypothesis requires data that includes a broad spectrum of fish species and can be directly compared between different regions of the global ocean. As a first attempt, we provide a test using global industrial fishing effort.
Fishing Effort as a Proxy for Fish Abundance
Despite their importance, both ecologically and as a food source for humans, the distribution of fish in the global ocean has been difficult to assess. Scientific surveys are frequently undertaken in national coastal waters, but fish are highly mobile and difficult to sample, so that the global distribution of fish biomass has an order-of-magnitude uncertainty (Irigoien et al., 2014). One approach to overcome the scarcity of direct observations is to use the exploitation of harvestable biomass by modern industrial fishing fleets as a proxy for fish abundance (Myers and Worm, 2003). Until recently, many fishing records were only available as aggregates provided by national agencies, often for specific taxa, and disaggregating these to the actual catch locations is fraught with uncertainty (Watson, 2017). However, a direct spatially resolved view on vessels ranging the global ocean is now provided by satellites that intercept radio transmissions from Automatic Identification System transponders, as part of the Global Fishing Watch (GFW) project (Kroodsma et al., 2018).
We use the GFW data for 2014–2016, inclusive, to provide a quantitative spatial estimate of fishing effort. Identification of fishing vessels and their activities are made using convolutional neural networks, as described by Kroodsma et al. (2018). Given that industrial fishing activity approximates a rational response to profit motives (Branch et al., 2006) and that most of the harvested fish are traded in a global market, the local fishing effort at any point on the high seas is expected to be roughly proportional to the catch. The catch, in turn, depends on the effort, the ability of fishermen to catch the available fish, and biomass density. We therefore interpret the distribution of industrial fishing effort on the high seas as a first-order proxy for the biomass density of catchable, commercially marketable fish.
The global distribution of fishing effort in the GFW database from 2014 through 2016 is shown in Figure 5A. The data only include vessels using transponders, and therefore underestimate the fishing effort that occurs within the Exclusive Economic Zones (EEZs) of countries that do not enforce transponder use, including those of the northern Indian Ocean, west Africa, and many Pacific islands. Nonetheless, a review of vessels that fish in the high seas outside of EEZs (beyond 200 nautical miles from shore) shows the GFW data includes 80% of the fishing effort in this area of the ocean (Sala et al., 2018), and we therefore limit our analysis to the domain beyond the EEZs.
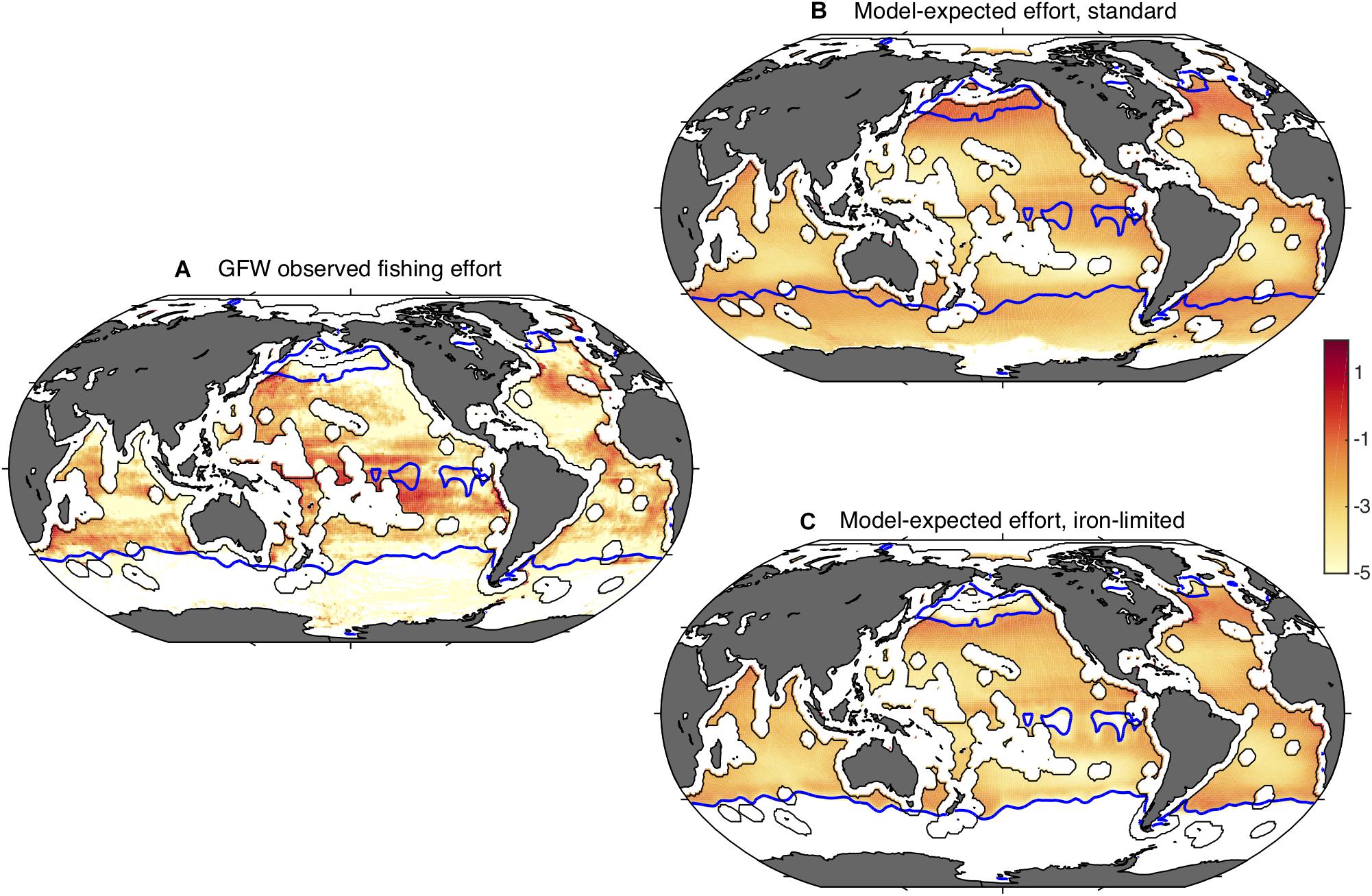
Figure 5. Global fishing effort, observed and modeled. Panel (A) shows the total satellite-observed fishing effort of 2014 through 2016 as estimated by Global Fishing Watch (h km– 2 year– 1). The blue contours indicate HNLC regions, identified as waters with minimum monthly nitrate concentrations >3 μmol kg– 1 (Boyer, 2013). In general, high surface nitrate concentrations occur where strong iron limitation occurs. Panel (B) shows a bio-economic model expectation of fishing effort (W m– 2), based on the satellite-observed primary production and the climatological water temperature, and panel (C) shows the same model prediction including the iron-limitation of carbon trophic transfer efficiency described in the text. Efforts are plotted as natural logarithms. Exclusive Economic Zones are excluded.
Figure 5A also shows blue contour lines corresponding to a minimum monthly surface nitrate concentration of 3 μM, which outline the HNLC regions and therefore indicate where low phytoplankton Fe:C would be expected. HNLC regions are not the only low-iron parts of the ocean, but they are the largest easily identified low-iron regions (Moore et al., 2013). Comparison of the GFW data with the blue contour lines shows that the fishing effort is relatively low in the HNLC waters, especially the subarctic Pacific and Southern Ocean. Indeed, when the average area-specific fishing effort is calculated at the global scale, the average effort in HNLC waters is found to be roughly one twentieth of that in all non-HNLC regions (Table 1). The average HNLC effort is less dramatically reduced in the tropics, where it is roughly half the average non-HNLC effort, while it is only one hundredth the non-HNLC effort in the northern oceans.
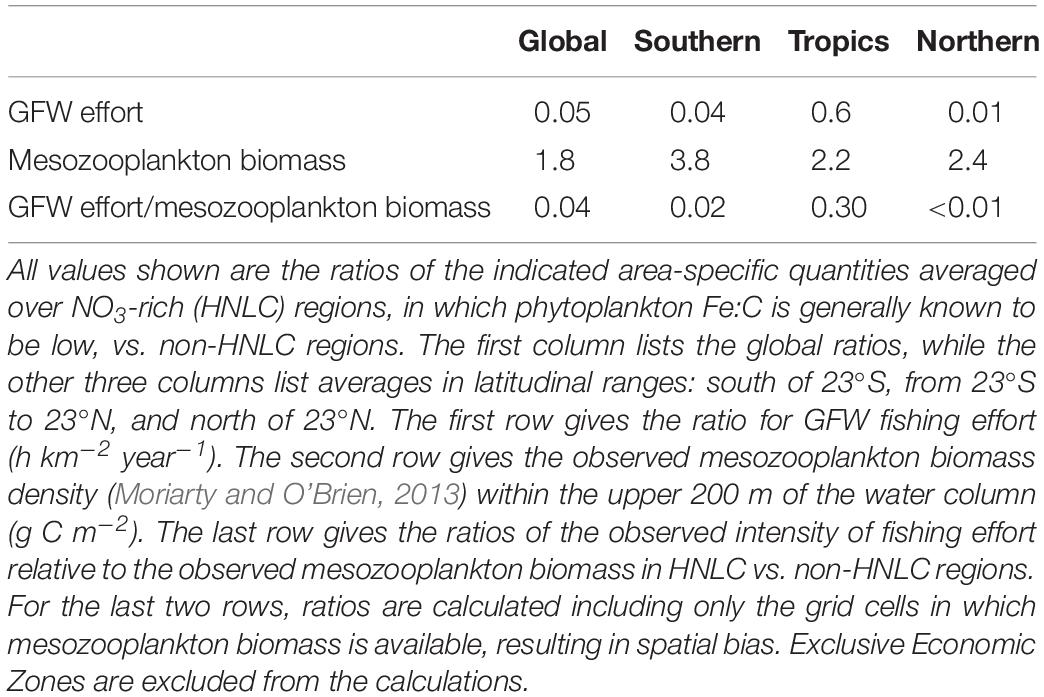
Table 1. Comparison of fishing effort and mesozooplankton biomass in HNLC vs. non-HNLC regions of the open ocean.
The observation of a relatively low fishing effort in HNLC regions, compared to non-HNLC regions, could have a number of possible explanations. We suggest the following possibilities, which may act independently or in concert, and which we then consider in turn: (1) physical access is prohibitive for fishing vessels; (2) fishery regulations prevent fishing; (3) other aspects of the environment (primary production and water temperature) are not conducive to abundant fish populations; and (4) epipelagic fish have a limited ability to exploit the existing planktonic food resource.
1. Physical access is prohibitive for fishing vessels
Fishing grounds that are remote from ports may attract less effort, given that fuel costs can constitute a significant amount of the total cost of fishing (Lam et al., 2011). As a result, fishing far from port tends to be more expensive than fishing close to port, all else being equal. This likely contributes to the low fishing effort in remote parts of the Southern Ocean and subarctic Pacific. However, as shown by Figure 5A, there is abundant fishing in remote parts of the tropical Pacific and southern Indian oceans. Conversely, there is negligible fishing in the subarctic Pacific HNLC waters closest to Japan, nor is there significant fishing in HNLC waters near South America, except in the coastal waters surrounding islands where iron concentrations would be expected to be high (Armand et al., 2008). Thus, distance can only be a contributing factor. Difficult sea conditions, including large waves and cold temperatures, could also be a factor in the subarctic Pacific and Southern Ocean. However, these conditions do not prohibit fishing activity on the Bering Shelf or in the Barents Sea; fishing even occurs during winter in these regions. And neither access nor weather can explain the relatively low fishing effort in the eastern equatorial Pacific.
2. Fishery regulations prevent fishing
On the high seas, only Regional Fisheries Management Organizations (RFMOs) provide any mechanism for regulation (Cullis-Suzuki and Pauly, 2010). The North Pacific Anadromous Fish Commission does prohibit the fishing of any salmonids outside of EEZs in the North Pacific (NPAFC, 2017), but there are no prohibitions on other types of fishing in this area. Thus, the apparent ability of the salmon fishing ban to eliminate most fishing would be consistent with salmon being the most abundant catchable, marketable fish in the subarctic Pacific. For comparison, the fishing of salmonids is also prohibited in the high seas of the North Atlantic by the North Atlantic Salmon Conservation Organization (NASCO, 1984), yet there remains significant fishing effort for other taxa in the open North Atlantic. In Antarctic waters, fishing can be managed through the Commission for the Conservation of Antarctic Marine Living Resources (Croxall and Nicol, 2004), but in practice this has focused only on reducing illegal, unreported and unregulated fishing in the productive coastal waters of Subantarctic islands (Österblom and Bodin, 2012) and to our knowledge there is no prohibition on fishing in the open Southern Ocean. Thus, although regulation contributes to low fishing effort in the subarctic Pacific through the specific ban on salmon, it does not seem to be able to explain the generally low effort in HNLC regions.
3. Primary production and water temperature do not support abundant fish populations
Primary production has been shown to limit fish catches (Chassot et al., 2010), and temperature has strong biological effects, including on trophic structure. To test how these factors might influence fish growth in HNLC regions we use the expectations provided by a global bioeconomic model of commercial fish and fisheries that accounts for spatial variations in primary production and water temperature. The model BOATS uses observed distributions of primary productivity and water temperature to estimate the growth, mortality, and reproduction rates of size-resolved fish populations based on empirical macroecological relationships (Carozza et al., 2016). An economic component, directly coupled to the fish biomass, estimates the fishing effort. The model sensitivities to NPP and water temperature are calibrated with historical catch records in coastal fisheries, among which it has good skill, explaining roughly 60% of the global variance in historical Large Marine Ecosystem catches (Carozza et al., 2017). We use an ensemble of five model configurations to span a broad range of the possible environmental sensitivities allowed by uncertain parameter values (Carozza et al., 2017).
When compared with the GFW data (Figure 5B), the model ensemble captures the >5 orders-of-magnitude range of effort between highly productive coastal waters and the subtropical gyres, and reproduces some features such as focused effort along the northern subtropical fronts. However, in contrast to the observations, the model expects that the Subantarctic Southern Ocean, eastern equatorial Pacific and subarctic Pacific would have a very high fishing effort. The magnitude of this discrepancy is shown by a comparison of normalized efforts, summarized in Figure 6. Essentially, the model expects fishing effort to be globally similar in HNLC and non-HNLC regions of the open ocean (i.e., close to 1) and higher in the eastern equatorial and subarctic Pacific given the relatively high NPP. We would caution that BOATS is a very simple model and does not discriminate benthic from pelagic ecosystems, which may lead to an overestimation of fish production in the pelagic HNLC regions (Stock et al., 2017). Perhaps even more importantly, it does not include fish movement, which is particularly important for the highly migratory species targeted in high seas fisheries (Lehodey et al., 1997; Guiet et al., 2019). Seasonal aggregation of tuna and billfish may explain many of the high concentrations of fishing effort which are not captured by the model. The model also ignores the cost of travel, which could decrease catches at inaccessible locations. Despite the many detailed shortcomings of the model, the first-order expectation based on the coastal fishery calibration to NPP and temperature is simply that there is no consistent difference in fish abundance between HNLC and non-HNLC regions of the high seas.
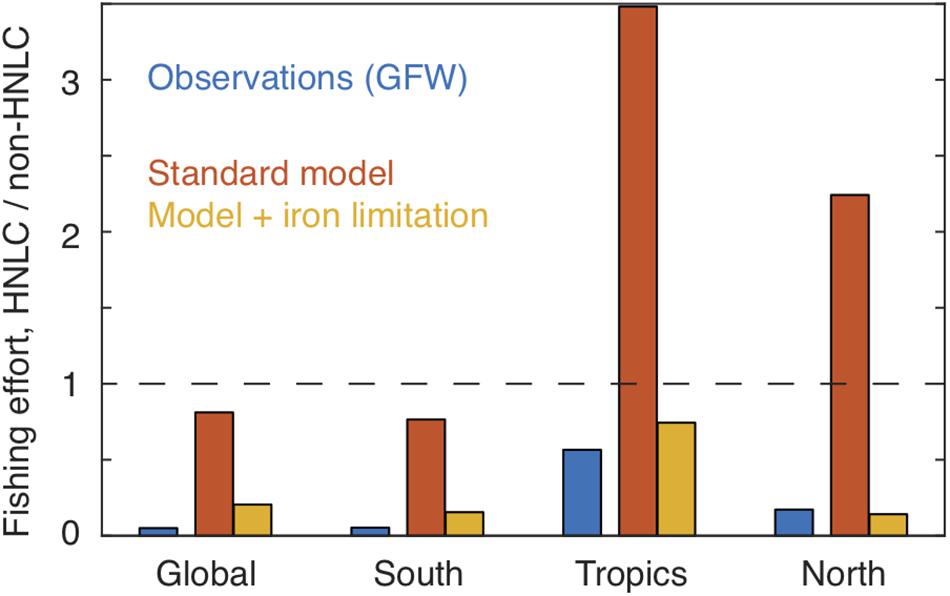
Figure 6. Comparison of observed and modeled fishing effort in HNLC vs. non-HNLC domains. A value of unity (dashed line) indicates the same average fishing intensity in the two domains. The standard model (red) does not show a significant difference in effort between HNLC and non-HNLC domains at the global scale, and actually shows relatively high effort in HNLC waters of the tropics and northern hemisphere. In contrast, both the Global Fishing Watch observations (blue) and the iron-limited model (orange) show lower effort in HNLC regions relative to non-HNLC regions. Latitude ranges are given in Table 1. EEZs are excluded from the calculation.
In addition, as mentioned above, the biomass of mesozooplankton is relatively large in HNLC regions (Figure 3). In fact, the average epipelagic mesozooplankton concentration is roughly twofold higher inside HNLC regions than outside of them (Table 1). Where mesozooplankton biomass data are available, the globally averaged ratio of GFW effort:mesozooplankton is 25-fold higher outside HNLC regions than within them, consistent with relatively little catchable, marketable fish biomass for the existing production of lower trophic level biomass.
4. Fish have a limited ability to exploit the existing planktonic food resource
The last possibility we address is that epipelagic fish are not able to fully exploit the existing planktonic food resource in HNLC waters, leading to lower overall abundance. Low water temperatures are likely to disadvantage ectothermic pelagic predators in the subarctic Pacific and Southern Ocean, and recent work has argued that endotherms including cetaceans, pinnipeds, and birds have a strong advantage in cold waters given their ability to maintain high levels of activity (Grady et al., 2019). This cannot be important in the eastern equatorial Pacific, but could be a major factor in the other two regions. However, given the case for iron limitation outlined above, it also appears feasible that the limitation of fish growth due to iron scarcity contributes to the anomalously low fishing effort identified in HNLC regions.
To illustrate this, we modified the BOATS model to provide a crude estimate of iron limitation, taking high surface nitrate concentration as a proxy for low iron concentrations. We modified the trophic efficiency for carbon as,
so that τC has the standard value τC0 when NO3 is low, and decreases smoothly as NO3 rises, with a value of 0.5 τC0 when NO3 = k. Figure 5C shows the global effort for this model version, using k = 5 μM. Although many biases remain, the overestimates of fishing effort in the three iron-limited regions are greatly reduced by this representation of iron limitation. This improvement is also evident in the global averages (Figure 6). To some degree, this ad hoc modification may compensate for other model biases, and should be seen only as an illustration. Nonetheless, it is consistent with a significant impact of iron limitation on the abundance of fish in iron-poor parts of the open ocean.
Outlook
This paper provides multiple lines of evidence that support a hypothetical role for iron in the ecology of marine fish (summarized in section “The Case for Iron,” above). In addition, industrial fishing effort is used as indirect evidence for low fish abundance in HNLC waters which may arise, at least in part, due to iron scarcity. Testing this hypothesis requires the collection of new observations, such as more extensive measurements of the iron contents of marine organisms, and dietary experiments that are relevant to wild animals rather than mariculture. The hypothesis also opens a host of new questions, a few of which we outline here.
Our discussion has mostly focused on the epipelagic (surface-dwelling) fish raised in aquaculture and targeted by industrial fisheries in the high seas. The abundance of mesopelagic fish, which are not commercially harvested, is very poorly known, but may exceed that of all other fish (Irigoien et al., 2014). Intriguingly, mesopelagic fish appear to be relatively abundant in HNLC waters. In the Southern Ocean, myctophids such as Electrona antarctica dominate the fish community in the iron-limited waters north of the shelf break (Moteki et al., 2011), while the subarctic Pacific shows a similarly high abundance of mesopelagic fish (Beamish et al., 1999). If mesopelagic fish are indeed less negatively impacted by iron limitation than commercially targeted epipelagic species, it would appear to be a topic worthy of further research.
Meanwhile, iron limitation is likely to affect other groups of marine animals to a greater or lesser degree. For example, the high metabolic demand of endothermy requires rapid respiration, resulting in τC < 0.03 (Humphreys, 1979), and consequently up to an order of magnitude more enrichment of Fe:C than expected for a fish given the same τFe. This could be expected to alleviate iron limitation among endothermic seabirds and mammals, giving them a relative advantage over ectotherms in iron-limited waters, abetting the thermal advantage endotherms have over ectotherms in the cold waters of the subarctic Pacific and Southern Ocean (Grady et al., 2019). As another example, cephalopods use the copper protein hemocyanin as an oxygen transporter, rather than hemoglobin (Schipp and Hevert, 1978). This use of copper could reduce the iron requirements of cephalopods, consistent with relatively low measured iron contents of edible squid tissue compared with fish (Kongkachuichai et al., 2002) and with the low measured iron content (4 μmol Fe mol C–1) of whole Loligo opalescens from the Californian coast (Falandysz, 1991). The low iron content of squid may therefore give them an advantage over hemoglobin-dependent fish when faced with severe iron limitation. The fact that squid are found as a direct predator on mesopelagic myctophid fish in the Antarctic Polar Frontal Zone, in the absence of any similar vertebrate fish predators (Rodhouse and White, 1995), would appear to be consistent with this possibility. In general, we suggest that the taxon-dependent susceptibility of animals to iron limitation will determine the degree to which they are constrained by iron availability, with consequences for the structure and diversity of marine ecosystems.
Finally, we have focused on iron because of its prominence as a limiting factor for phytoplankton. However, other micronutrients can also be limiting to phytoplankton (Moore et al., 2013) as well as larval fish (Wang and Wang, 2018). It is therefore conceivable that other micronutrients could also be limiting to fish in the marine environment. For example, Zn additions have been found to increase phytoplankton growth in HNLC regions (Crawford et al., 2003), suggesting the ambient concentrations are low, and Zn has also been found to be a critical element for the nutrition of larval fish (Wang and Wang, 2018).
Conclusion
The idea that dietary iron supply can constrain fish growth is not new: it has been recognized in mariculture for decades. The new hypothesis advanced here proposes that iron supply also plays a role in the wild. At the very least, the data summarized in Figure 4 imply that some fish have iron requirements that exclude them from iron-limited waters. The more consequential possibility is that the epipelagic fish biomass is significantly lower in iron-limited waters than it would be were iron more abundant. Although the latter possibility remains a hypothesis to be tested, it suggests that marine fish growth may be enhanced by iron fertilization, in addition to any impact the iron may have on primary production.
Author Contributions
PLM compiled and interpreted the literature data. DK provided and interpreted the fishing effort data. GH conducted the iron-limited model simulations. EG wrote the manuscript. All authors contributed to the revisions of the manuscript.
Funding
This study was supported by the European Research Council (ERC) under the European Union’s Horizon 2020 Research and Innovation Program (Grant Agreement No 682602) and the Spanish Ministry of Science, Innovation and Universities, through the “María de Maeztu” Program for Units of Excellence (MDM-2015-0552).
Conflict of Interest Statement
The authors declare that the research was conducted in the absence of any commercial or financial relationships that could be construed as a potential conflict of interest.
Acknowledgments
We thank Jérôme Guiet, Ian Hatton, Maite Maldonado, and Adrian Marchetti for helpful discussions.
Supplementary Material
The Supplementary Material for this article can be found online at: https://www.frontiersin.org/articles/10.3389/fmars.2019.00509/full#supplementary-material
References
Andersen, F., Lorentzen, M., Waagbø, R., and Maage, A. (1997). Bioavailability and interactions with other micronutrients of three dietary iron sources in Atlantic salmon, Salmo salar, smolts. Aquac. Nutr. 3, 239–246. doi: 10.1046/j.1365-2095.1997.00096.x
Andersen, F., Maage, A., and Julshamn, K. (1996). An estimation of dietary iron requirement of Atlantic salmon, Salmo salar L., parr. Aquac. Nutr. 2, 41–47. doi: 10.1111/j.1365-2095.1996.tb00006.x
Armand, L. K., Cornet-Barthaux, V., Mosseri, J., and Quéguiner, B. (2008). Late summer diatom biomass and community structure on and around the naturally iron-fertilised Kerguelen Plateau in the Southern Ocean. Deep Sea Res. Part 2 Top. Stud. Oceanogr. 55, 653–676. doi: 10.1016/j.dsr2.2007.12.031
Baines, S. B., Chen, X., Vogt, S., Fisher, N. S., Twining, B. S., and Landry, M. R. (2016). Microplankton trace element contents: implications for mineral limitation of mesozooplankton in an HNLC area. J. Plankton Res. 38, 256–270. doi: 10.1093/plankt/fbv109
Banse, K. (1990). Does iron really limit phytoplankton production in the offshore subarctic Pacific? Limnol. Oceanogr. 35, 772–775. doi: 10.4319/lo.1990.35.3.0772
Beamish, R., Leask, K., Ivanov, O., Balanov, A., Orlov, A., and Sinclair, B. (1999). The ecology, distribution, and abundance of midwater fishes of the Subarctic Pacific gyres. Prog. Oceanogr. 43, 399–442. doi: 10.1016/s0079-6611(99)00017-8
Beard, J. L., Dawson, H., and Piñero, D. J. (1996). Iron metabolism: a comprehensive review. Nutr. Rev. 54, 295–317. doi: 10.1111/j.1753-4887.1996.tb03794.x
Boyd, P. W., Jickells, T., Law, C. S., Blain, S., Boyle, E. A., Buesseler, K. O., et al. (2007). Mesoscale iron enrichment experiments 1993-2005: synthesis and future directions. Science 315, 612–617. doi: 10.1126/science.1131669
Boyer, T. (2013). “World Ocean Atlas 2013 product documentation,” in NOAA Atlas NESDIS 72. ed. A. V. Mishonov (Silver Spring, MD: NOAA publisher).
Branch, T. A., Hilborn, R., Haynie, A. C., Fay, G., Flynn, L., Griffiths, J., et al. (2006). Fleet dynamics and fishermen behavior: lessons for fisheries managers. Can. J. Fish. Aquat. Sci. 63, 1647–1668. doi: 10.1139/f06-072
Brodeur, R., McKinnell, S., Nagasawa, K., Pearcy, W., Radchenko, V., and Takagi, S. (1999). Epipelagic nekton of the North Pacific subarctic and transition zones. Prog. Oceanogr. 43, 365–397. doi: 10.1016/s0079-6611(99)00013-0
Bury, N., Boyle, D., and Cooper, C. A. (2012). Iron in Homeostasis and Toxicology of Essential Metals. Amsterdam: Elsevier.
Bury, N., and Grosell, M. (2003). Iron acquisition by teleost fish. Com. Biochem. Physiol. C Toxicol. Pharmacol. 135, 97–105. doi: 10.1016/s1532-0456(03)00021-8
Carozza, D. A., Bianchi, D., and Galbraith, E. D. (2016). The ecological module of BOATS-1.0: a bioenergetically constrained model of marine upper trophic levels suitable for studies of fisheries and ocean biogeochemistry. Geosci. Model Dev. 9, 1545–1565. doi: 10.5194/gmd-9-1545-2016
Carozza, D. A., Bianchi, D., and Galbraith, E. D. (2017). Formulation, general features and global calibration of a bioenergetically-constrained fishery model. PLoS One 12:e0169763. doi: 10.1371/journal.pone.0169763
Carpene, E., Martin, B., and Dalla Libera, L. (1998). Biochemical differences in lateral muscle of wild and farmed gilthead sea bream (series Sparus aurata L). Fish. Physiol. Biochem. 19, 229–238.
Chase, Z., and Price, N. (1997). Metabolic consequences of iron deficiency in heterotrophic marine protozoa. Limnol. Oceanog. 42, 1673–1684. doi: 10.4319/lo.1997.42.8.1673
Chassot, E., Bonhommeau, S., Dulvy, N. K., Mélin, F., Watson, R., Gascuel, D., et al. (2010). Global marine primary production constrains fisheries catches. Ecol. lett. 13, 495–505. doi: 10.1111/j.1461-0248.2010.01443.x
Chen, L., DeVries, A. L., and Cheng, C.-H. C. (1997). Convergent evolution of antifreeze glycoproteins in Antarctic notothenioid fish and Arctic cod. Proc. Natl. Acad. Sci. 94, 3817–3822. doi: 10.1073/pnas.94.8.3817
Chen, X., Baines, S. B., and Fisher, N. S. (2011). Can copepods be limited by the iron content of their food? Limnol. Oceanogr. 56, 451–460. doi: 10.4319/lo.2011.56.2.0451
Chen, X., Fisher, N. S., and Baines, S. B. (2014). Influence of algal iron content on the assimilation and fate of iron and carbon in a marine copepod. Limnol. Oceanogr. 59, 129–140. doi: 10.4319/lo.2014.59.1.0129
Cooper, C., and Bury, N. (2007). The gills as an important uptake route for the essential nutrient iron in freshwater rainbow trout Oncorhynchus mykiss. J. Fish. Biol. 71, 115–128. doi: 10.1111/j.1095-8649.2007.01474.x
Cooper, C., Handy, R., and Bury, N. (2006). The effects of dietary iron concentration on gastrointestinal and branchial assimilation of both iron and cadmium in zebrafish (Danio rerio). Aquat. Toxicol. 79, 167–175. doi: 10.1016/j.aquatox.2006.06.008
Crawford, D. W., Lipsen, M. S., Purdie, D. A., Lohan, M. C., Statham, P. J., Whitney, F. A., et al. (2003). Influence of zinc and iron enrichments on phytoplankton growth in the northeastern subarctic Pacific. Limnol. Oceanogr. 48, 1583–1600. doi: 10.4319/lo.2003.48.4.1583
Croxall, J. P., and Nicol, S. (2004). Management of Southern Ocean fisheries: global forces and future sustainability. Antarct. Sci. 16, 569–584. doi: 10.1017/s0954102004002330
Cullis-Suzuki, S., and Pauly, D. (2010). Failing the high seas: a global evaluation of regional fisheries management organizations. Mar. Policy 34, 1036–1042. doi: 10.1016/j.marpol.2010.03.002
de Baar, H. J. W., Boyd, P. W., Coale, K. H., Landry, M. R., Tsuda, A., Assmy, P., et al. (2005). Synthesis of iron fertilization experiments: from the iron age in the age of enlightenment. J. Geophys. Res. Oceans 110:C09S16. doi: 10.1029/2004JC002601
Dunne, J. P., Sarmiento, J. L., and Gnanadesikan, A. (2007). A synthesis of global particle export from the surface ocean and cycling through the ocean interior and on the seafloor. Global Biogeochem. Cycles 21:GB4006. doi: 10.1029/2006gb002907
Eastman, J. T. (2005). The nature of the diversity of Antarctic fishes. Polar Biol. 28, 93–107. doi: 10.1007/s00300-004-0667-664
Elser, J. J., Fagan, W. F., Denno, R. F., Dobberfuhl, D. R., Folarin, A., Huberty, A., et al. (2000). Nutritional constraints in terrestrial and freshwater food webs. Nature 408:578. doi: 10.1038/35046058
Falandysz, J. (1991). Concentrations of trace metals in various tissues of the squid Loligo opalescens and their redistribution after canning. J. Sci. Food Agric. 54, 79–87. doi: 10.1002/jsfa.2740540110
Garofalo, F., Pellegrino, D., Amelio, D., and Tota, B. (2009). The Antarctic hemoglobinless icefish, fifty five years later: a unique cardiocirculatory interplay of disaptation and phenotypic plasticity. Comp. Biochem. Physiol. A Mol. Integr. Physiol. 154, 10–28. doi: 10.1016/j.cbpa.2009.04.621
Geesey, G. G., Alexander, G. V., Bray, R. N., and Miller, A. C. (1984). Fish fecal pellets are a source of minerals for inshore reef communities. Mar. Ecol. Prog. Ser. 15, 19–25. doi: 10.3354/meps015019
Gledhill, M., and Buck, K. N. (2012). The organic complexation of iron in the marine environment: a review. Front. Microbiol. 3:69. doi: 10.3389/fmicb.2012.00069
Grady, J. M., Maitner, B. S., Winter, A. S., Kaschner, K., Tittensor, D. P., Record, S., et al. (2019). Metabolic asymmetry and the global diversity of marine predators. Science 363:eaat4220. doi: 10.1126/science.aat4220
Gran, H. H. (1931). On the conditions for the production of plankton in the sea. Rapp. Proc. Verb. Cons. Int. Explor. Mer. 75, 37–46.
Guiet, J., Galbraith, E., Kroodsma, D., and Worm, B. (2019). Seasonal variability in global industrial fishing effort. PLoS One 14:e0216819. doi: 10.1371/journal.pone.0216819
Hansen, L., and Quinn, T. (1998). The marine phase of the Atlantic salmon (Salmo salar) life cycle, with comparisons to Pacific salmon. Can. J. Fish. Aquat. Sci. 55, 104–118. doi: 10.1139/d98-010
Harvey, H. (1937). The supply of iron to diatoms. J. Mar. Biol. Assoc. United Kingdom 22, 205–219. doi: 10.1017/s0025315400011954
Ho, T.-Y., Wen, L.-S., You, C.-F., and Lee, D.-C. (2007). The trace metal composition of size-fractionated plankton in the South China sea: biotic versus abiotic sources. Limnol. Oceanogr. 52, 1776–1788. doi: 10.4319/lo.2007.52.5.1776
Honda, K., Yamamoto, Y., and Tatsukawa, R. (1987). Distribution of heavy metals in Antarctic marine ecosystem. Proc. NIPR Symp. Polar Biol. 1, 184–197.
Humphreys, W. F. (1979). Production and respiration in animal populations. J. Anim. Ecol. 48, 427–453.
Hutchins, D., and Bruland, K. (1994). Grazer-mediated regeneration and assimilation of Fe, Zn and Mn from planktonic prey. Mar. Ecol.Prog. Ser. 110, 259–259.
Hutchins, D., DiTullio, G., Zhang, Y., and Bruland, K. (1998). An iron limitation mosaic in the California upwelling regime. Limnol. Oceanogr. 43, 1037–1054. doi: 10.4319/lo.1998.43.6.1037
Hutchins, D. A., Wang, W.-X., and Fisher, N. S. (1995). Copepod grazing and the biogeochemical fate of diatom iron. Limnol. Oceanogr. 40, 989–994. doi: 10.4319/lo.1995.40.5.0989
Irigoien, X., Klevjer, T., Røstad, A., Martinez, U., Boyra, G., Acuña, J., et al. (2014). Large mesopelagic fishes biomass and trophic efficiency in the open ocean. Nat. Commun. 5:3271. doi: 10.1038/ncomms4271
Johnson, K. S., Chavez, F. P., and Friederich, G. E. (1999). Continental-shelf sediment as a primary source of iron for coastal phytoplankton. Nature 398:697. doi: 10.1038/19511
Karimi, R., Fisher, N. S., and Folt, C. L. (2010). Multielement stoichiometry in aquatic invertebrates: when growth dilution matters. Am. Nat. 176, 699–709. doi: 10.1086/657046
King, A., Sañudo-Wilhelmy, S., Boyd, P., Twining, B., Wilhelm, S., Breene, C., et al. (2012). A comparison of biogenic iron quotas during a diatom spring bloom using multiple approaches. Biogeosciences 9:667. doi: 10.5194/bg-9-667-2012
Kock, K.-H. (2005). Antarctic icefishes (Channichthyidae): a unique family of fishes. Rev. Polar Biol. 28, 862–895. doi: 10.1007/s00300-005-0019-z
Kongkachuichai, R., Napatthalung, P., and Charoensiri, R. (2002). Heme and nonheme iron content of animal products commonly consumed in Thailand. J. Food Compost. Anal. 15, 389–398. doi: 10.1006/jfca.2002.1080
Kroodsma, D. A., Mayorga, J., Hochberg, T., Miller, N. A., Boerder, K., Ferretti, F., et al. (2018). Tracking the global footprint of fisheries. Science 359, 904–908. doi: 10.1126/science.aao5646
Kuhn, D. E., O’Brien, K. M., and Crockett, E. L. (2016). Expansion of capacities for iron transport and sequestration reflects plasma volumes and heart mass among white-blooded notothenioid fishes. Am. J. Physiol. Regul. Integr. Comp. Physiol. 311, R649–R657. doi: 10.1152/ajpregu.00188.2016
Lam, V. W., Sumaila, U. R., Dyck, A., Pauly, D., and Watson, R. (2011). Construction and first applications of a global cost of fishing database. ICES J. Mar. Sci. 68, 1996–2004. doi: 10.1093/icesjms/fsr121
Lavery, T. J., Roudnew, B., Gill, P., Seymour, J., Seuront, L., Johnson, G., et al. (2010). Iron defecation by sperm whales stimulates carbon export in the Southern Ocean. Proc. Biol. Sci. 277, 3527–3531. doi: 10.1098/rspb.2010.0863
Lehodey, P., Bertignac, M., Hampton, J., Lewis, A., and Picaut, J. (1997). El Niño Southern Oscillation and tuna in the western Pacific. Nature 389:715. doi: 10.1038/39575
Liu, X., and Millero, F. J. (2002). The solubility of iron in seawater. Mar. Chem. 77, 43–54. doi: 10.1016/s0304-4203(01)00074-3
Maldonado, M. T., Surma, S., and Pakhomov, E. A. (2016). Southern Ocean biological iron cycling in the pre-whaling and present ecosystems. Phil. Trans. R. Soc. A 374:20150292. doi: 10.1098/rsta.2015.0292
Marchetti, A., and Maldonado, M. (2016). “Iron,” in The Physiology of Microalgae, eds M. A. Borowitzka, J. Beardall, and J. A. Raven (Switzerland: Springer).
Martin, J. H., Broenkow, W. W., Fitzwater, S. E., and Gordon, R. M. (1990). Yes, it does: a reply to the comment by Banse. Limnol. Oceanogr. 35, 775–777. doi: 10.4319/lo.1990.35.3.0775
Martin, J. H., and Fitzwater, S. E. (1988). Iron-deficiency limits phytoplankton growth in the Northeast Pacific subarctic. Nature 331, 341–343. doi: 10.1038/331341a0
Moore, C., Mills, M., Arrigo, K., Berman-Frank, I., Bopp, L., Boyd, P., et al. (2013). Processes and patterns of oceanic nutrient limitation. Nat. Geosci. 6, 701–710.
Moreno, A. R., and Haffa, A. L. (2014). The impact of fish and the commercial marine harvest on the ocean iron cycle. PloS One 9:e107690. doi: 10.1371/journal.pone.0107690
Moriarty, R., and O’Brien, T. (2013). Distribution of mesozooplankton biomass in the global ocean. Earth Syst. Sci. Data 5:45. doi: 10.5194/essd-5-45-2013
Moteki, M., Koubbi, P., Pruvost, P., Tavernier, E., and Hulley, P.-A. (2011). Spatial distribution of pelagic fish off adélie and george v Land, East Antarctica in the austral summer 2008. Polar Sci. 5, 211–224. doi: 10.1016/j.polar.2011.04.001
Myers, R. A., and Worm, B. (2003). Rapid worldwide depletion of predatory fish communities. Nature 423, 280–283. doi: 10.1038/nature01610
NASCO (1984). The Convention for the Conservation of Salmon in the North Atlantic Ocean. Available at: http://www.nasco.int/convention.html (accessed November 8, 2018).
NPAFC (2017). Convention for the Conservation of Anadromous Fish Stocks in the North Pacific Ocean. Available at: https://npafc.org/wp-content/uploads/2017/06/Handbook-3rd-E-Convention-Only-English.pdf (accessed November 8, 2018).
Österblom, H., and Bodin, Ö (2012). Global cooperation among diverse organizations to reduce illegal fishing in the Southern Ocean. Conserv. Biol. 26, 638–648. doi: 10.1111/j.1523-1739.2012.01850.x
Prabhu, P. A. J., Schrama, J. W., and Kaushik, S. J. (2016). Mineral requirements of fish: a systematic review. Rev. Aquac. 8, 172–219. doi: 10.1016/j.aninu.2017.05.005
Quinn, T. P. (2018). The Behavior and Ecology of Pacific Salmon and Trout. Seattle, WA: University of Washington Press.
Ratnarajah, L., Nicol, S., and Bowie, A. R. (2018). Pelagic iron recycling in the southern ocean: exploring the contribution of marine animals. Front. Mar. Sci. 5:109.
Reinfelder, J. R., and Fisher, N. S. (1991). The assimilation of elements ingested by marine copepods. Science 251, 794–796. doi: 10.1126/science.251.4995.794
Reinfelder, J. R., and Fisher, N. S. (1994). Retention of elements absorbed by juvenile fish (Menidia menidia, Menidia beryllina) from zooplankton prey. Limnol. Oceanogr. 39, 1783–1789. doi: 10.4319/lo.1994.39.8.1783
Rodhouse, P. G., and White, M. G. (1995). Cephalopods occupy the ecological niche of epipelagic fish in the Antarctic Polar frontal zone. Biol. Bull. 189, 77–80. doi: 10.2307/1542457
Ruud, J. T. (1954). Vertebrates without erythrocytes and blood pigment. Nature 173:848. doi: 10.1038/173848a0
Sakamoto, S., and Yone, Y. (1978). Requirement of red sea bream for dietary iron-II. Nippon Suisan Gakkaishi 44, 223–225. doi: 10.2331/suisan.44.223
Sala, E., Mayorga, J., Costello, C., Kroodsma, D., Palomares, M. L., Pauly, D., et al. (2018). The economics of fishing the high seas. Sci. Adv. 4:eaat2504. doi: 10.1126/sciadv.aat2504
Sarmiento, J., and Gruber, N. (2006). Ocean Biogeochemical Dynamics. Oxford: Princeton University Press.
Schipp, R., and Hevert, F. (1978). Distribution of copper and iron in some central organs of Sepia officinalis (Cephalopoda). A comparative study by flameless atomic absorption and electron microscopy. Mar. Biol. 47, 391–399. doi: 10.1007/bf00388931
Schmidt, K., Atkinson, A., Steigenberger, S., Fielding, S., Lindsay, M., Pond, D. W., et al. (2011). Seabed foraging by Antarctic krill: implications for stock assessment, bentho-pelagic coupling, and the vertical transfer of iron. Limnol. Oceanogr. 56, 1411–1428. doi: 10.4319/lo.2011.56.4.1411
Schmidt, K., Schlosser, C., Atkinson, A., Fielding, S., Venables, H. J., Waluda, C. M., et al. (2016). Zooplankton gut passage mobilizes lithogenic iron for ocean productivity. Curr. Biol. 26, 2667–2673. doi: 10.1016/j.cub.2016.07.058
Schmidt, M. A., Zhang, Y., and Hutchins, D. A. (1999). Assimilation of Fe and carbon by marine copepods from Fe-limited and Fe-replete diatom prey. J. Plankton Res. 21, 1753–1764. doi: 10.1093/plankt/21.9.1753
Shearer, K. D. (1994). Factors affecting the proximate composition of cultured fishes with emphasis on salmonids. Aquaculture 119, 63–88. doi: 10.1016/0044-8486(94)90444-8
Shire, D. M., and Kustka, A. B. (2015). Luxury uptake, iron storage and ferritin abundance in Prochlorococcus marinus (Synechococcales) strain MED4. Phycologia 54, 398–406. doi: 10.2216/14-109.1
Sidell, B. D., and O’Brien, K. M. (2006). When bad things happen to good fish: the loss of hemoglobin and myoglobin expression in Antarctic icefishes. J. Exp. Biol. 209, 1791–1802. doi: 10.1242/jeb.02091
Sterner, R. W., and Elser, J. J. (2002). Ecological Stoichiometry. Princeton: Princeton University Press.
Stock, C. A., John, J. G., Rykaczewski, R. R., Asch, R. G., Cheung, W. W., Dunne, J. P., et al. (2017). Reconciling fisheries catch and ocean productivity. Proc. Natl. Acad. Sci. 114, E1441–E1449. doi: 10.1073/pnas.1610238114
Strzepek, R. F., Boyd, P. W., and Sunda, W. G. (2019). Photosynthetic adaptation to low iron, light, and temperature in Southern Ocean phytoplankton. Proc. Natl. Acad. Sci. 116, 4388–4393. doi: 10.1073/pnas.1810886116
Strzepek, R. F., and Harrison, P. J. (2004). Photosynthetic architecture differs in coastal and oceanic diatoms. Nature 431, 689–692. doi: 10.1038/nature02954
Strzepek, R. F., Maldonado, M. T., Hunter, K. A., Frew, R. D., and Boyd, P. W. (2011). Adaptive strategies by Southern Ocean phytoplankton to lessen iron limitation: uptake of organically complexed iron and reduced cellular iron requirements. Limnol. Oceanogr. 56, 1983–2002. doi: 10.4319/lo.2011.56.6.1983
Tagliabue, A., Bowie, A. R., Boyd, P. W., Buck, K. N., Johnson, K. S., and Saito, M. A. (2017). The integral role of iron in ocean biogeochemistry. Nature 543:51. doi: 10.1038/nature21058
Tortell, P. D., Maldonado, M. T., Granger, J., and Price, N. M. (1999). Marine bacteria and biogeochemical cycling of iron in the oceans. FEMS Microbiol. Ecol. 29, 1–11. doi: 10.1016/s0168-6496(98)00113-5
Twining, B. S., and Baines, S. B. (2013). The trace metal composition of marine phytoplankton. Ann. Rev. Mar. Sci. 5, 191–215. doi: 10.1146/annurev-marine-121211-172322
Twining, B. S., Nunez-Milland, D., Vogt, S., Johnson, R. S., and Sedwick, P. N. (2010). Variations in Synechococcus cell quotas of phosphorus, sulfur, manganese, iron, nickel, and zinc within mesoscale eddies in the Sargasso Sea. Limnol. Oceanogr. 55, 492–506. doi: 10.4319/lo.2009.55.2.0492
Verde, C., Lecointre, G., and di Prisco, G. (2007). The phylogeny of polar fishes and the structure, function and molecular evolution of hemoglobin. Polar Biol. 30, 523–539. doi: 10.1007/s00300-006-0217-213
Verde, C., Parisi, E., and di Prisco, G. (2003). The Evolution of polar fish hemoglobin: a phylogenetic analysis of the ancestral amino acid residues linked to the root effect. J. Mol. Evol. 57, S258–S267. doi: 10.1007/s00239-003-0035-y
Wang, J., Shu, X., and Wang, W.-X. (2019). Micro-elemental retention in rotifers and their trophic transfer to marine fish larvae: influences of green algae enrichment. Aquaculture 499, 374–380. doi: 10.1016/j.aquaculture.2018.09.066
Wang, J., and Wang, W.-X. (2016). Novel insights into iron regulation and requirement in marine medaka oryzias melastigma. Sci. Rep. 6:26615. doi: 10.1038/srep26615
Wang, J., and Wang, W.-X. (2018). Understanding the micro-elemental nutrition in the larval stage of marine fish: a multi-elemental stoichiometry approach. Aquaculture 488, 189–198. doi: 10.1016/j.aquaculture.2017.12.036
Watanabe, T., Kiron, V., and Satoh, S. (1997). Trace minerals in fish nutrition. Aquaculture 151, 185–207. doi: 10.1016/s0044-8486(96)01503-7
Watson, R. A. (2017). A database of global marine commercial, small-scale, illegal and unreported fisheries catch 1950–2014. Sci. Data 4:170039.
Keywords: iron limitation, fish, fishing, Global Fishing Watch, marine ecology, HNLC region, ecosystem stoichiometry, trace metal
Citation: Galbraith ED, Le Mézo P, Solanes Hernandez G, Bianchi D and Kroodsma D (2019) Growth Limitation of Marine Fish by Low Iron Availability in the Open Ocean. Front. Mar. Sci. 6:509. doi: 10.3389/fmars.2019.00509
Received: 27 November 2018; Accepted: 05 August 2019;
Published: 28 August 2019.
Edited by:
Carol Robinson, University of East Anglia, United KingdomReviewed by:
Julian Blasco, Spanish National Research Council (CSIC), SpainPeter Croot, National University of Ireland Galway, Ireland
Rod W. Wilson, University of Exeter, United Kingdom
Copyright © 2019 Galbraith, Le Mézo, Solanes Hernandez, Bianchi and Kroodsma. This is an open-access article distributed under the terms of the Creative Commons Attribution License (CC BY). The use, distribution or reproduction in other forums is permitted, provided the original author(s) and the copyright owner(s) are credited and that the original publication in this journal is cited, in accordance with accepted academic practice. No use, distribution or reproduction is permitted which does not comply with these terms.
*Correspondence: Eric D. Galbraith, ZXJpYy5kLmdhbGJyYWl0aEBnbWFpbC5jb20=