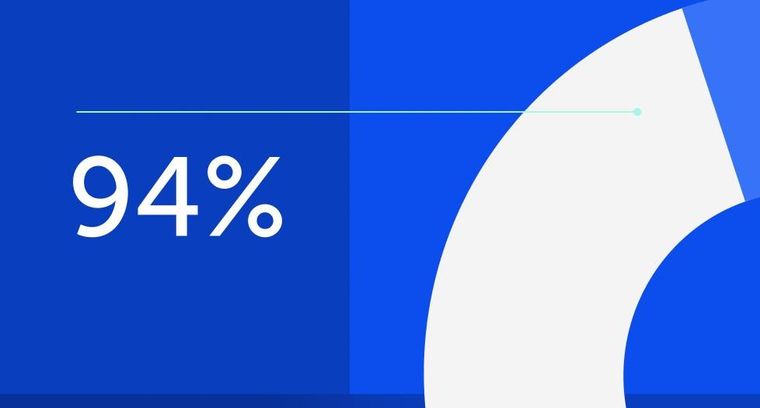
94% of researchers rate our articles as excellent or good
Learn more about the work of our research integrity team to safeguard the quality of each article we publish.
Find out more
POLICY AND PRACTICE REVIEWS article
Front. Mar. Sci., 26 July 2019
Sec. Coastal Ocean Processes
Volume 6 - 2019 | https://doi.org/10.3389/fmars.2019.00467
This article is part of the Research TopicCross-Disciplinary Marine Research: New Tools and Societal InterfacesView all 9 articles
The accumulation of aquatic organisms on the wetted surfaces of vessels (i.e., vessel biofouling) negatively impacts world-wide shipping through reductions in vessel performance and fuel efficiency, and increases in emissions. Vessel biofouling is also a potent mechanism for the introduction and spread of marine non-indigenous species. Guidance and regulations from the International Maritime Organization, New Zealand, and California have recently been adopted to address biosecurity risks, primarily through preventive management. However, appropriate reactive management measures may be necessary for some vessels. Vessel in-water cleaning or treatment (VICT) has been identified as an important tool to improve operating efficiency and to reduce biosecurity risks. VICT can be applied proactively [i.e., to prevent the occurrence of, or to remove, microfouling (i.e., slime) or prevent the occurrence of macrofouling organisms – large, distinct multicellular organisms visible to the human eye], or reactively (i.e., to remove macrofouling organisms). However, unmanaged VICT includes its own set of biosecurity and water quality risks. Regulatory policies and technical advice from California and New Zealand have been developed to manage these risks, but there are still knowledge gaps related to the efficacy of available technologies. Research efforts are underway to address these gaps in order to inform the regulatory and non-regulatory application of VICT.
Biofouling is the accumulation of aquatic organisms on immersed surfaces. Biofouling on maritime vessels is an ongoing burden for owners and operators (reviewed by Woods Hole Oceanographic Institute [WHOI], 1952), causing impacts on speed, maneuverability, operability, and durability. For example, biofouling on a vessel’s hull can result in reduced speed at a given level of power due to increased hydrodynamic frictional drag (Schultz, 2007; Buhaug et al., 2009; Schultz et al., 2011). That is, a higher rate of fuel use is required in order to produce the increased power necessary to achieve a given speed. Such an impact has far-reaching implications, as increased fuel consumption also influences shipping-induced greenhouse gas emissions (International Maritime Organization [IMO], 2011).
Vessel biofouling is also an important pathway for the human-mediated transport of marine non-indigenous species (NIS). For example, the biofouling pathway is a potential means of transfer for more than 80% of New Zealand’s and 60% of California’s marine and estuarine NIS (Kospartov et al., 2008; Ruiz et al., 2011). Further, a large proportion of marine NIS in Hawaii, North America, Port Phillip Bay (Australia), and Japan have likely been introduced via this pathway (Eldredge and Carlton, 2002; Fofonoff et al., 2003; Hewitt et al., 2004; Otani, 2006).
While not all NIS have associated impacts, a subset of NIS have a broad range of impacts on the marine environment and the people reliant upon it (see Ruiz et al., 1997; Molnar et al., 2008; Sorte et al., 2010). The specific impacts posed by marine NIS transfers associated with vessel biofouling are of growing global concern, given that the marine environment is a key part of much of the world’s economic, environmental, and socio-cultural values (International Maritime Organization [IMO], 2017; Food and Agriculture Organization of the United Nations [FAO], 2018; Carlton et al., 2019). For example, Hayward (1997) and Hayward et al. (1999) attributed major environmental changes in Waitemata Harbor, Auckland, New Zealand, to the non-indigenous bivalves Magallana gigas and Arcuatula senhousia. The bivalve Mytilus galloprovincialis has caused ecological impacts in South Africa, including species displacement and increased intertidal biomass (Robinson et al., 2005; Hanekom, 2008). Further, NIS biofouling on cultured shellfish has detrimental effects on growth and condition as well as appearance, marketability, and production costs (Fitridge et al., 2014; Forrest et al., 2014; Davidson et al., 2017).
Because of the difficulty in predicting the impacts that marine NIS may have, a preventive approach has been identified as the most effective way to manage the biosecurity risks associated with vessel biofouling (Bax et al., 2003; International Maritime Organization [IMO], 2011; Lewis, 2016). For vessels, the best practice approach includes, but is not limited to, the application of antifouling systems appropriate to the vessel’s operational profile, performance monitoring, ongoing maintenance of submerged surfaces, and contingency planning (Georgiades et al., 2018). By comparison, response measures applied to marine NIS incursions, such as containment and eradication of species, are labor-intensive, time-consuming, expensive, and are often of limited success (Anderson, 2005; Davidson et al., 2008; Branson, 2012). For example, the successful eradication of the black striped false mussel (Mytilopsis sallei) from three marinas in the Northern Territory, Australia, cost in excess of AU$ 2.2 million, directly involved more than 280 people, and required the use of large quantities of biocides to kill all marine life at the sites (Willan et al., 2000; Bax et al., 2002). In New Zealand, incursion responses and ongoing management of the Mediterranean fanworm (Sabella spallanzanii) have cost in excess of NZ$ 2 million (Bell et al., 2011). More recently, Marks et al. (2017) evaluated the removal of the introduced fucoid Sargassum horneri offshore of Santa Catalina Island (California, United States) and found that successful acute population size reductions were overwhelmed by subsequent recruitment.
To minimize the risk of marine NIS transfers associated with the vessel biofouling pathway, guidelines (International Maritime Organization [IMO], 2011, 2012) and regulations (Ministry for Primary Industries, New Zealand [MPI], 2014; California Code of Regulations, 2017) have been developed at international, national, and regional levels. Georgiades and Kluza (2017) used an evidence-based approach in their advice to underpin New Zealand’s regulations by recommending limits to species richness and maturity of biofouling present on the different submerged areas of a vessel while also taking the vessel’s itinerary into account. While differences in New Zealand and California regulations exists due to their different legislative frameworks, it is notable that both are aligned and consistent with voluntary IMO biofouling management guidelines that are based on following best practice to minimize the risk of marine NIS transfer (see International Maritime Organization [IMO], 2011). Importantly, all guidelines and regulations identify vessel in-water cleaning or treatment (VICT; Table 1) as an important tool to maintain a vessel as free of biofouling as practical thus minimizing biosecurity risk (International Maritime Organization [IMO], 2011; Department of the Environment [DOE] and New Zealand Ministry for Primary Industries [MPI], 2015; Scianni et al., 2017; Georgiades et al., 2018). VICT is of particular importance to larger vessels due to issues associated with dry-docking, such as cost, facility availability, and the potential of having to unload cargo prior to dry-docking (Inglis et al., 2012; Morrisey et al., 2013). Traditionally, development of VICT was driven by efforts to reduce drag on planar areas of the vessel hull, thus reducing fuel consumption (Naval Sea Systems Command [NAVSEA], 2006; Schultz et al., 2011). However, the advent of regulations to minimize biosecurity risk is expected to advance the improvement and uptake of these technologies and drive the development of those designed to clean or treat external and internal niche areas.
There are two approaches to VICT:
Proactive in-water cleaning (PIC) or treatment (PIT), which for the purposes of this review includes hull grooming (e.g., Tribou and Swain, 2015), is used to prevent or reduce the attachment and growth of microfouling (i.e., slime) on the vessel and to remove newly attached (i.e., microscopic) stages of macrofouling organisms. The economic benefit of removing the slime layer by PIC has been the subject of many studies (e.g., Schultz et al., 2011) and, while the magnitude of economic benefit requires further clarity, PIC aligns with efforts by the International Maritime Organization (IMO) to reduce greenhouse gas emissions by optimizing vessel fuel efficiency (International Maritime Organization [IMO], 2011). PIT has also been developed using heat as the mechanism of action (Inglis et al., 2012). A proactive in-water cleaning and capture (PICC) system includes capture and effluent treatment. PIC (and PICC), using cleaning tools such as soft brushes, water jets, or contactless systems, is a key component of the continual maintenance of the vessel’s submerged surfaces, as it prevents the accumulation of macrofouling and thus minimizes biosecurity risk (Department of the Environment [DOE] and New Zealand Ministry for Primary Industries [MPI], 2015; Georgiades et al., 2018).
Incidental amounts of macrofouling can establish on a vessel’s submerged surfaces even under best management practices (Georgiades and Kluza, 2017). Reactive in-water cleaning (RIC) or treatment (RIT) is used to remove or treat biofouling (i.e., macrofouling) from such vessels and those for which preventive management has been ineffective, those that have been inadequately maintained, or from areas where antifouling coatings have been poorly applied or have become damaged. Macrofouling is more difficult to remove than a slime layer and may contain a diverse range of organisms that are reproductively mature (Davidson et al., 2013; Morrisey et al., 2013; Department of the Environment [DOE] and New Zealand Ministry for Primary Industries [MPI], 2015). RIC or RIT are not suitable routine approaches to vessel biofouling management for several reasons discussed in this manuscript, including damage leading to premature antifouling coating depletion or failure. However, RIC and RIT remain important response tools to reduce the likelihood of species establishment from macrofouled vessels.
Regardless of the approach (i.e., proactive or reactive), two types of environmental risk are identified that may require management (Figure 1):
Figure 1. Identification of biosecurity [B] and chemical contamination [C] risks associated with operation of reactive in-water cleaning and capture (RICC) systems [Adapted from Morrisey and Woods (2015) and Alliance for Coastal Technologies Maritime Environmental Resource Center [ACT/MERC] (2019)].
(1) The release and environmental accumulation of chemical contaminants associated with antifouling coating systems; and
(2) The release of marine NIS (as adults, larvae, or viable propagules) into new environments (International Maritime Organization [IMO], 2011; Morrisey et al., 2013).
To provide regulators, vessel-related industries, and system operators with a scientific basis for the appropriate application of VICT, an understanding is needed of the risks associated with its application. This understanding requires a solid evidence-base from which to inform decision making (Morrisey et al., 2013, 2015).
This review provides a summary of the current knowledge regarding environmental risks and benefits of VICT technologies applied to external hull surfaces of commercial vessels (e.g., PIC, RIC). Also addressed are the technical obstacles related to the regulatory acceptance and responsible use of these tools to manage biosecurity and chemical contamination risk based on the experiences of New Zealand and California. In-water treatments (e.g., PIT, RIT) are not included in this manuscript, as efficacy of these methods has been recently reviewed by Growcott et al. (2017) and Cahill et al. (2019a).
Current cleaning and treatment approaches (Table 2) include systems that can be used to undertake a variety of in-water maintenance tasks. Proactive in-water cleaning or treatment (i.e., PIC, PIT) describe systems to prevent slime layer formation, to remove it from the hull, and to remove microscopic life-history stages of macrofouling organisms. RIC describes a system used to remove macrofouling from the hull without capture and effluent treatment. A reactive in-water cleaning and capture (RICC) system includes capture and effluent treatment. Reactive in-water treatment (RIT) describes the treatment of macrofouling. There may be some vessels that are predominately fouled with microfouling but have limited patches of macrofouling, particularly in and around niche areas (Georgiades and Kluza, 2017). In these instances, the use of a RICC system may be necessary to protect the receiving environment.
Table 2. Summary of approaches to vessel in-water cleaning or treatment (VICT) of commercial vessels (McClay et al., 2015; Morrisey and Woods, 2015).
The management of biofouling on hulls and other immersed vessel surfaces is typically achieved by the application of antifouling systems, including antifouling coatings, to prevent or minimize the accumulation of organisms (Lewis, 2016; Georgiades et al., 2018). Antifouling coating systems are broadly categorized as biocidal or non-biocidal.
Non-biocidal coating systems have physical properties to impair attachment (e.g., silicone-based fouling release coatings) or allow regular or abrasive cleaning with minimal effect on the surface (e.g., hard coating systems that are mechanically resistant to damage).
Biocidal coating systems prevent the attachment and growth of biofouling organisms through the release of biocides, such as copper and zinc compounds. Copper is the most commonly used biocide, however, co-biocides are often incorporated into coating systems to ensure efficacy over a range of species (Dafforn et al., 2011). The three main types of biocidal coating systems routinely used on commercial vessels are self-polishing copolymer, ablative, and insoluble matrix (Lewis, 2016). The application of VICT systems to vessels with biocidal antifouling coatings may result in an unacceptable release of chemical contaminants contained within or on the coatings (e.g., biocides from slime layer and coating, including dispersal of paint flakes) and their accumulation in the marine environment (i.e., water column, sediments, biota).
Conducted appropriately, PIC that is consistent with most antifouling system manufacturer’s recommendations may result in discharges that meet local standards or requirements (Morrisey et al., 2013; Department of the Environment [DOE] and New Zealand Ministry for Primary Industries [MPI], 2015). Intuitively, PIC will likely result in a lower environmental release of contaminants than RIC because less abrasive techniques are expected to minimize the release of biocides (Morrisey et al., 2013; Earley et al., 2014; Department of the Environment [DOE] and New Zealand Ministry for Primary Industries [MPI], 2015). However, cumulative release of biocides throughout a frequent PIC regime may also need to be taken into account. Therefore, water quality agencies are likely to require data on chemical discharges associated with both system types. PICC systems are designed to minimize the release of biocides further via capture and effluent treatment. However, independently generated data regarding chemical discharges associated with PIC and PICC systems are scarce.
Morrisey et al. (2013) assessed the potential chemical contamination risks of PIC by comparing predicted copper concentrations released against water quality guidelines under a range of scenarios. The water quality guideline values used were 4.8 μg Cu/L for acute risk (Code of Federal Regulations [CFR], 1983; Thursby and Hansen, 1995) and 3.1 μg Cu/L for chronic risk (Code of Federal Regulations [CFR], 1983; Australian and New Zealand Environment Conservation Council [ANZECC], 2000). Environmental copper concentrations were predicted using the Marine Antifoulant Model to Predict Environmental Concentrations (MAMPEC) model v 3.0 (Deltares, 2011). Although using the best available information based on extensive review, Morrisey et al. (2013) acknowledged that detailed information for many aspects related to the risks of PIC was limited, and that considerable uncertainty was associated with the information that was available.
Using the model, Morrisey et al. (2013) predicted that, in most scenarios, PIC of commercial vessels would not exceed water quality guidelines (i.e., the activity was a low risk) within the Port of Auckland, New Zealand. This prediction was based on the mixing zone and flushing within this port and application of the conservative upper copper release estimate (i.e., realistic worst case). A low-flushing port, Lyttleton, New Zealand, had a greater likelihood of guideline exceedance from this activity (Gadd et al., 2011; Morrisey et al., 2013).
Lewis (2013) describes the evaluation of a system applied proactively to a vessel with microfouling and a biocidal coating that was 13 months into its service life. System trials included the use of contactless bladed disks or soft nylon brushes attached to the cleaning head. Unsurprisingly, dissolved and particulate copper concentrations in effluent from the contactless blades were much lower than those generated by the brushes. While copper concentrations in water samples taken from the system effluent were far in excess of water quality standards, no elevated copper concentrations were recorded in the water column near the test vessel during or after the cleaning trial (Lewis, 2013).
The total quantity of copper released into the environment during the Lewis (2013) trial (estimated for a 45 m vessel to be 87.5 g) compared favorably against the calculated passive daily release of copper from antifouling coating systems from small (50 m; 40 g) and large vessels (200 m; 1,000 g). This calculation was based on an estimated copper release rate of 10 μg/cm2/day (Lewis, 2013; Morrisey et al., 2013). Normal vessel operations may also result in biocide entry into the environment through release from the paint to prevent attachment and growth of biofouling, sloughing of the slime layer containing accumulated copper (Morrisey et al., 2013), and mechanical damage of antifouling coating systems by anchor chains, tugs, and fenders (Anderson, 2004).
Reactive in-water cleaning methods, including abrasive brush systems and high-pressure water jets, may abrade biocidal antifouling coatings resulting in contaminant release into the surrounding marine environment (Valkirs et al., 2003; Inglis et al., 2012; Morrisey et al., 2013; Earley et al., 2014). RICC systems are being developed to mitigate the risks associated with contaminant release by capturing, filtering, and/or treating the removed debris and waste effluent (California Water Boards, 2013; Morrisey and Woods, 2015). However, the use of RIC and RICC systems may result in areas of spot fouling due to localized biocide depletion.
A range of factors influence the nature of discharges associated with RIC and RICC. These include the type(s) and age of the antifouling coating systems cleaned, the submerged areas cleaned, the amount and type of biofouling present, the method of in-water cleaning, and the hydrodynamic environment (Gadd et al., 2011; Inglis et al., 2012; Morrisey et al., 2013; Alliance for Coastal Technologies Maritime Environmental Resource Center [ACT/MERC], 2019).
Independently derived and publicly available data on the release of contaminants associated with RIC and RICC on actual vessels are scarce. Mean total copper concentrations in samples taken from the discharge plume from the Submerged Cleaning and Maintenance Platform (SCAMP) during the cleaning of three US Navy vessels ranged from 1.57 to 2.62 mg/L. The mean range of the dissolved copper fraction was 66 to 146 μg/L. The mass of copper released was estimated to be 4.8 g/m2 of surface cleaned (United States Environmental Protection Agency [EPA], 1999). More recently, Bohlander (2009) stated that the US Navy Advanced Hull Cleaning System (AHCS) was able to reduce the solids content of the effluent stream to <5 mg/L and copper to <1 mg/L. However, the data necessary to verify these findings are not publicly available.
Terraphase Engineering Inc. (2012) reported an evaluation of a diver-operated, hydraulic-driven, brush cart conducted for the U.S. Department of Transportation – Maritime Administration (MARAD). The objective of the evaluation was to demonstrate the effectiveness of capturing and containing particulate matter and soluble metals potentially removed during cleaning.
The effluent treatment system consisted of a particulate filter followed by a weir tank, which discharged through a filter cartridge array consisting of a 100 μm stainless steel mesh screen, two 10 μm filter cartridges in series, and a 5 μm filter cartridge. Effluent then entered a pressure vessel containing 2,000 pounds of organoclay (modified zeolite).
Treatment through the system, including two passes through the pressure vessel, reduced both the total and dissolved copper concentration in the effluent to less than 100 μg/L. The concentration of total and dissolved zinc was reduced to approximately 600 μg/L. The pressure vessel containing organoclay removed approximately 80% of the dissolved copper and approximately 25% of the dissolved zinc from the cleaning effluent (Terraphase Engineering Inc., 2012). The report states that a majority of the particulates greater than 5 μm were removed in the treatment process, however no data were shown.
The Terraphase Engineering Inc. (2012), trial was conducted on macrofouling (Chris Scianni, Personal Observation), however, further details of the type and coverage of fouling, type and age of antifouling paint, type of area and actual area cleaned are not provided in the report (see Morrisey et al., 2015 for advice on data requirements). Terraphase Engineering Inc. (2012), recommended cleaning of only soft fouling with this system to minimize impacts on antifouling coatings. Based on the above study, the interim best management practice required by the San Francisco Regional Water Quality Control Board for effluent discharges are ≤100 μg/L total copper and ≤700 μg/L total zinc (California Water Boards, 2013, 2015).
Using the MAMPEC model, Morrisey et al. (2013) reported that the likelihood of water quality guideline exceedance increased when using aggressive cleaning methods (i.e., reactive cleaning of hard macrofouling) and the conservative upper copper release estimate. A medium or high risk of water quality exceedance was shown for the majority of reactive cleaning scenarios within Lyttelton Port, New Zealand (low-flushing). The significant factors leading to unacceptable copper concentrations included the vessel area being cleaned, the number of vessels cleaned per day, the technique used (based on type of fouling), and rate of flushing (Morrisey et al., 2013). Similarly, Earley et al. (2014) observed that less aggressive cleaning of antifouling coatings on panels contributed one third of the amount of particulate loading compared to more aggressive, abrasive methods.
While PIC is likely to release significant amounts of microbial material and microscopic stages of macrofouling species into the marine environment, the biosecurity risk of proactive cleaning is widely viewed as acceptable, as it is currently not possible to manage vessel biofouling below the level of a slime layer (Dobretsov, 2010; Bell et al., 2011; Morrisey et al., 2013; Department of the Environment [DOE] and New Zealand Ministry for Primary Industries [MPI], 2015). The slime layer is typically sloughed from vessels as part of the mode of action of their antifouling coating systems (Morrisey et al., 2013) and during normal vessel operations, including interactions with tugs and fenders. Morrisey et al. (2013) noted that detached microscopic stages of macrofouling species may not be capable of reattachment and growth.
The effects of PIC and PICC on antifouling coatings is also important with respect to assessing the overall minimization of biosecurity risk (Morrisey and Woods, 2015). Depleted antifouling coating systems on hulls are likely to rapidly re-foul, subsequently increasing biosecurity risk for future recipient ports and reducing vessel operating efficiency (Department of the Environment [DOE] and New Zealand Ministry for Primary Industries [MPI], 2015). Using soft brushes, Tribou and Swain (2017) showed that ongoing hull grooming of plates coated with ablative biocidal paints had a mixed effect on coating thickness over 6 years (i.e., the duration of vessel’s in-service period), depending on the frequency of grooming. While coating depletion for weekly groomed panels was significantly different from ungroomed panels, the depletion recorded for monthly groomed panels was not significantly different from weekly or ungroomed panels. In contrast to weekly grooming, monthly grooming was unable to maintain a clean surface under high fouling pressure (Tribou and Swain, 2017).
From a biosecurity perspective, if PIC or PICC is permitted, it will be necessary to ensure with a high level of certainty that there are no specific biosecurity risks associated with the vessel (i.e., only a slime layer is being removed). Factors such as voyage history, cleaning history, and a pre-inspection of the areas to be cleaned may be considered prior to deployment (Morrisey et al., 2013). For management of ongoing risk, assurance would also be required regarding the efficacy of the removal (i.e., no areas are missed).
Reactive in-water cleaning systems can facilitate the release (e.g., stress-induced spawning, larval release, or non-capture of fragments) and establishment of marine NIS (Morrisey et al., 2013). Some uncaptured or dispersed macrofouling species are capable of remaining viable and establishing in the marine environment. Therefore, removal, capture, and treatment of effluent containing macrofouling and associated propagules are critical considerations for RIC systems (Woods et al., 2007; Hopkins et al., 2008; Department of the Environment [DOE] and New Zealand Ministry for Primary Industries [MPI], 2015; Morrisey and Woods, 2015). The organisms that are most likely to establish following such dispersal include clonal forms (e.g., colonial ascidians, bryozoans, and sponges), organisms capable of regeneration from fragments (e.g., some macroalgae, ascidians, and polychaetes), and mobile organisms (Morrisey et al., 2013).
While RICC systems are being developed to mitigate the biosecurity risks associated with this activity (Morrisey and Woods, 2015; Alliance for Coastal Technologies Maritime Environmental Resource Center [ACT/MERC], 2019), there are few published independent evaluations of their efficacy (Morrisey et al., 2013). Trials using a prototype, diver-operated brush system with waste capture resulted in the failure to contain, on average, 5% of the removed macrofouling (Hopkins et al., 2008, 2010). The waste capture shrouds were not able to seal against curved surfaces of the hull, leading to a large proportion of untreated material being released into the surrounding environment. This material included a wide range of intact organisms including mussels, barnacles, tubeworms, bryozoans, hydroids, and ascidians. Macrofouling was also dislodged from the hull by the divers’ fins, hoses, and other gear dragged across the vessel surfaces. RIC and RICC may also result in patches of mature fouling being missed, particularly as some hull and niche areas are inaccessible to the equipment (Floerl et al., 2008; Hopkins et al., 2008).
Cleaning efficacy is generally lower with harder types of fouling (e.g., calcareous or shell-forming species) and greater fouling extent (Davidson et al., 2008; Hopkins et al., 2008). For example, Davidson et al. (2008) observed a mean reduced fouling cover from 89 to 37% during SCAMP system trials on a heavily fouled vessel (e.g., sessile fouling assemblages dominated by encrusting spp., barnacles and filamentous spp.), with 81% of species still being present. At the time of their report, Morrisey et al. (2013) concluded that the efficacy of available RIC technologies decreases when macrofouling cover is greater than 15%.
While effluent treatment is a key component of a biosecure RICC system, it needs to be practically achievable. Morrisey et al. (2013) recommended that a filter of 2 μm pore size be applied to effluent from RICC of international vessels, as algal reproductive cells can range in size from 2 μm to more than 250 μm (Clayton, 1992; Maggs and Callow, 2003). While it was known at the time that systems could not achieve such a standard, the recommendation was given to stimulate system development (i.e., technology forcing). The authors also suggested filtration to 60 μm for reactive cleaning of domestic vessels to encourage development of technology for international vessels. Department of the Environment [DOE] and New Zealand Ministry for Primary Industries [MPI] (2015) recommend filtration to 50 μm, which was based on the original Australian and New Zealand in-water cleaning guidelines drafted in 2011 (Ministry of Agriculture, and Forestry [MAF], 2011). Based on the findings of Terraphase Engineering Inc. (2012), the San Francisco Regional Water Quality Control Board proposed filtration to 5 μm as best management practice for in-water cleaning (California Water Boards, 2013). This standard was unchanged in 2015 (California Water Boards, 2015). Morrisey and Woods (2015) and Morrisey et al. (2015) recommended a 12.5 μm filtration standard based on the results of a system evaluation reported by Lewis (2013). Independent data demonstrating the limits of current in-water effluent treatment technologies are scarce (but see Alliance for Coastal Technologies Maritime Environmental Resource Center [ACT/MERC], 2019).
Alternatives to filtration include effluent treatment via heat, biocides, or ultra-violet (UV) light to render propagules within the effluent non-viable, or direct disposal into municipal sewerage with secondary treatment (Morrisey and Woods, 2015). However, pre-filtration to reduce the particulate organic material present is known to improve the efficacy of effluent treatment with UV, ozone, or chlorine (Sippel, 1983). Filtration is also a critical step toward reducing total copper (or other biocides) concentration within the effluent.
Reactive in-water cleaning and RICC systems can physically damage antifouling coating systems or accelerate their biocide release rates, shortening the overall service life. Rapid re-fouling of such coatings subsequently increases the biosecurity risk for future recipient ports and reduces vessel operating efficiency (Department of the Environment [DOE] and New Zealand Ministry for Primary Industries [MPI], 2015). For example, baseplates and shells of non-viable organisms that remain after reactive cleaning can provide a substratum for chemo-induction of recruitment (Anil et al., 2010).
Approvals from the relevant authorities are often required to ensure that biosecurity and chemical contamination risks are being managed prior to undertaking VICT.
In New Zealand, discharges associated with VICT are governed by different legislative regimes including, but not limited to: the Resource Management Act 1991 (Ministry for the Environment; Department of Conservation), Biosecurity Act 1993 [Ministry for Primary Industries (MPIs)], Maritime Transport Act 1994 (Maritime New Zealand), and the Hazardous Substance and New Organisms Act 1996 (Environmental Protection Authority). Local government (i.e., Regional Councils and Unitary Authorities) also have responsibilities under the Resource Management Act and the Biosecurity Act. This makes for a complex regulatory management regime which may present unnecessary practical barriers for vessel and VICT operators to achieve compliance (Trecia Smith, Personal Communication, September 2018, Ministry for Primary Industries, New Zealand).
To ensure the biosecurity risks associated with VICT are managed, MPI has commissioned research to inform the development of performance criteria and evaluation methods for RICC systems (Morrisey and Woods, 2015; Morrisey et al., 2015). The use of VICT systems and their operation must be approved by MPI before they are deployed on international vessels non-compliant with New Zealand’s biofouling regulations (Ministry for Primary Industries, New Zealand [MPI], 2014). As of 2019, assessment of the utility of the technical advice for performance criteria and evaluation methods is subject to ongoing research and as such, there are no approved VICT providers in New Zealand for international vessels (Georgiades et al., 2018; McClary and Georgiades, 2018).
The regulation of VICT in California falls under multiple jurisdictions. Water quality and chemical contamination risks are currently regulated under the U.S. Clean Water Act’s (CWA) National Pollutant Discharge Elimination System (NPDES), the California Toxics Rule, and applicable water quality control plans including, but not limited to, the California Porter-Cologne Water Quality Control Act, the Clean Coast Act, the California Ocean Plan, the California Environmental Quality Act, and the Marine Managed Areas Improvement Act. Traditional PIC, RIC, and RIT systems (i.e., systems that do not include capture and effluent treatment) are all covered and permitted under the U.S. Environmental Protection Agency’s Vessel General Permit for Discharges Incidental to the Normal Operation of a Vessel (VGP), under authority of the CWA. California’s State Water Resources Control Board has added requirements to the VGP, essentially prohibiting VICT of copper-containing antifouling coating systems within waterbodies that have been listed by the U.S. Environmental Protection Agency through the CWA Section 303(d) (see California Water Boards, 2010) as impaired for copper (i.e., Ports of Los Angeles, Long Beach, and San Diego) unless using the Best Available Technologies Economically Feasible (BAT). Such technologies have yet to be identified.
Proactive and reactive in-water cleaning and capture systems (i.e., PICC, RICC), however, produce a new discharge (i.e., effluent from the RICC filtration or treatment system) that may not be covered by the VGP. In these cases, vendors of PICC and RICC systems may be required to obtain individual NPDES permits or comply with general NPDES permits to operate in specific waterbodies, with discharge limits that vary depending on the level of existing copper impairment in that waterbody. The San Francisco Bay Regional Water Quality Control Board has issued an in-water vessel hull cleaning best management practices document that describes system configuration and discharge limits for allowable PICC and RICC operations within the San Francisco Bay (see California Water Boards, 2015).
The current regulatory regime governed by the NPDES program and the VGP will change over the next 4 years, as the U.S. Environmental Protection Agency and the U.S. Coast Guard develop and implement new regulations required by the Vessel Incidental Discharge Act (VIDA) enacted in December 2018. The VIDA regulations will supersede and replace the requirements set through the VGP and will presumably cover PIC and RIC operations. However, uncertainty still exists about whether the VIDA regulations will also apply to PICC and RICC systems, as the VIDA narrowly applies to “discharges incidental to the normal operation of vessels,” essentially the same discharges that are currently covered by the VGP.
Biosecurity risks are managed in California by the State Lands Commission (the Commission) under the Marine Invasive Species Act. Commission staff are working cooperatively with regional counterparts (in nearby coastal states) through the Coastal Committee of the Western Regional Panel on Aquatic Nuisance Species to develop a consistent set of requirements to minimize biosecurity risks. These regionally consistent requirements would then be used by the local Regional Water Quality Control Boards agencies issuing NPDES permits, in concert with their own water quality requirements, to evaluate permit applications (see Scianni et al., 2017).
There is a paucity of robust and independently generated data to inform the assessment of biosecurity and chemical contamination risk arising from VICT using currently available technologies. This lack of reliable data has created significant uncertainty regarding approvals for VICT use (Morrisey et al., 2013; Drake et al., 2017). To facilitate the development and approval of VICT systems, feasible and defendable performance criteria and practical and repeatable evaluation procedures are needed to evaluate the biosecurity and chemical contamination risks posed. Such performance criteria, procedures, and associated guidance would enable the independent and transparent evaluation of the efficacy and reliability of VICT systems and their operators, to assess and minimize risks (e.g., Morrisey et al., 2015; Alliance for Coastal Technologies Maritime Environmental Resource Center [ACT/MERC], 2019).
Transparent and robust system evaluations conducted by appropriately qualified independent providers have the potential to facilitate the generation of VICT system data that has wide regulatory applicability, thus decreasing stakeholder costs and regulatory burden. As an appropriate analog, the accepted approach for the approval of ballast water management systems relies on aligned analytical and evaluation procedures and reporting of results (International Maritime Organization [IMO], 2016). The IMO recommend that testing facilities be independent of system manufacturers, and the acceptability of these facilities is determined by administrations that are a signatory to the International Convention for the Control and Management of Ships’ Ballast Water and Sediments (International Maritime Organization [IMO], 2004). By contrast, the U.S. Coast Guard specifies that testing of ballast water management systems must be conducted by accepted independent laboratories. This specification is a key difference between the IMO and U.S. Coast Guard testing regimes (United States Coast Guard [USGC], 2018). As the biosecurity risks arising from discharge of VICT systems are of a similar nature to ballast water discharges (Inglis et al., 2012; Morrisey et al., 2013), it would make sense that the approval of these systems is based on data generated through a consistent level of evaluation oversight, auditing, and validation. This would provide overall cost savings to the developer (e.g., the amount of system testing is minimized to meet agreed multilateral data requirements) and benefits the regulator (e.g., assurance that the system evaluation was conducted to a high standard with unbiased and consistent reporting of results).
Ministry for Primary Industries and the Commission have recently contributed funds and resources toward research programs aimed at providing protocol development for, and independent evaluations of, reactive in-water cleaning and capture (RICC) and proactive in-water cleaning (PIC) systems (McClary and Georgiades, 2018; Alliance for Coastal Technologies Maritime Environmental Resource Center [ACT/MERC], 2019). These research programs emphasize the following core principles:
• Vessel based evaluation using the full VICT system (e.g., cleaning head, effluent treatment system);
• VICT system evaluations should be a simulation of the intended use of the system (e.g., systems designed to remove hard fouling should be evaluated on hard fouling at the peak coverage of intended use; systems should be evaluated on different vessel surfaces as applicable to their intended use);
• VICT system evaluations should be conducted and supervised by appropriately qualified and approved, independent scientists (i.e., evaluation conduct and reporting should be objective and not be subject to bias);
• All evaluation failures should be reported (i.e., reporting should be transparent).
Ministry for Primary Industries has also recently commissioned research to help strengthen New Zealand’s approach to manage VICT across the various regulatory regimes (Ministry for Primary Industries, New Zealand [MPI], 2018). A key component of this research is to understand the perceptions, needs, concerns or barriers that stakeholders experience in undertaking VICT to meet all regulatory and non-regulatory requirements.
In addition to New Zealand and California, the Australian Government is endeavoring to create nationally consistent standards for VICT (Department of Agriculture, and Water Resources, 2019). Further, the Baltic and International Maritime Council (BIMCO), in conjunction with partners from the antifouling coating and VICT industries, are working toward the establishment of an internationally recognized VICT standard. Trials will be undertaken to verify the feasibility and practicality of the standard before endorsement by the appropriate international organizations (Kronholm Fraende, 2018). The communication between these groups has been ongoing and its importance cannot be overstated.
Vessel in-water cleaning or treatment regulatory authorities may include in their considerations the importance of marine stewardship at both domestic and international levels. For example, broad and inflexible prohibition of VICT is likely to reduce the incentive to develop environmentally acceptable technologies and encourages cleaning in locations where environmental considerations may be lower (Morrisey et al., 2013).
Vessel biofouling is not evenly distributed across the surface of a hull. Areas that are protected from a constant or uniform water flow, or susceptible to wear or damage of the antifouling coating tend to accumulate a higher biomass of organisms (Coutts et al., 2003, 2010; Davidson et al., 2016). These “niche” areas include sea chests and internal pipework. Biofouling of internal seawater systems can reduce their pumping rate and engine cooling efficiency (Pamitran et al., 2016). Further, obstruction of pipes can impact vessel safety (e.g., compromised firefighting systems; Palermo, 1992), lead to corrosion, and result in unscheduled maintenance and associated costs (Jones and Little, 1990; Grandison et al., 2011). Internal seawater systems have been identified as high biosecurity risk areas due to the diversity, abundance, and maturity of biofouling communities (Coutts et al., 2003; Coutts and Dodgshun, 2007; Lewis and Dimas, 2007; Frey et al., 2014; Lewis, 2016).
Given the difficulty of accessing niche areas relative to the general hull, there is little research, and thus much uncertainty, with respect to the efficacy of VICT for internal seawater systems (Growcott et al., 2017). To address the management gap, the Australian Government commissioned research to determine which internal seawater systems are likely to foul and to evaluate the compatibility and efficacy of potential treatment options (Peter Wilkinson, Personal Communication, September 2018, Department of Agriculture and Water Resources, Australia). Further, MPI has released technical advice for procedures to evaluate the cleaning or treatment of fouled internal seawater systems (Growcott et al., 2019).
Cahill et al. (2019b) developed and tested a thermal RIT system to treat the internal seawater systems of recreational vessels. This study provided some key insights which may assist the adaptation of RIT systems to commercial vessels, including the importance of data quality, laboratory testing, treatment circulation, and verification methods. The cleaning or treatment of internal seawater systems of commercial vessels is a complex problem and is identified as a priority for further research.
Vessel in-water cleaning or treatment has significant benefits as a tool to optimize vessel efficiency and curb emissions. However, considerable uncertainty exists with respect to the management of environmental risks associated with VICT technologies. Although the goals of some of the more recent technologies includes the minimization of biosecurity and chemical contamination risks, hastening the use of these tools before they have been properly evaluated may result in unacceptable risk to core values. Therefore, data generated to enable system approval should be subject to appropriate validation. Ongoing or recently completed research programs (see McClary and Georgiades, 2018; Alliance for Coastal Technologies Maritime Environmental Resource Center [ACT/MERC], 2019) to inform the development of robust and transparent procedures and criteria to evaluate VICT systems are likely to provide more clarity for regulators and stakeholders.
The following actions would further facilitate the development of VICT technologies and our understanding of their associated risks (if any):
• Identification and process mapping of relevant legislation and regulatory regimes responsible for approvals, application of consenting conditions, and ongoing monitoring within jurisdictions;
• Setting of practical and feasible performance criteria, particularly for reactive systems, that minimize biosecurity and chemical contamination risks, as applicable;
• Development of robust and transparent procedures for independent system evaluation and reporting;
• Development of an agreed approach for system approvals within and across jurisdictions, as possible.
Both authors contributed equally to the development of this manuscript.
The authors declare that the research was conducted in the absence of any commercial or financial relationships that could be construed as a potential conflict of interest.
Nicole Dobroski, Justin Wood, and Julisa Portugal (California State Lands Commission), Mario Tamburri (University of Maryland Center for Environmental Science), and Abraham Growcott, Christine Reed, Sudharma Leelawardana, Daniel Kluza, Trecia Smith, Tanayaz Patil, and Steve Hathaway (Ministry for Primary Industries) reviewed the draft versions of the manuscript. The input of the three reviewers greatly improved this manuscript.
Alliance for Coastal Technologies Maritime Environmental Resource Center [ACT/MERC] (2019). Evaluation of Subsea Global Solutions in-Water Cleaning and Capture Technology for Ships. ACT/MERC, Maryland, USA. ACT/MERC IWCC Evaluation Report ER01-19. Available at: http://www.maritime-enviro.org/Downloads/Reports/Other_Publications/ACT_MERC_SGS_IWCC_Evaluation_Report.pdf (accessed April 2019).
Anderson, C. D. (2004). The economic importance of hull condition. J. Prot. Coatings Linings. 21, 36–39.
Anderson, L. W. J. (2005). California’s reaction to Caulerpa taxifolia: a model for invasive species rapid response. Biol. Invasions 7, 1003–1016. doi: 10.1007/s10530-004-3123-z
Anil, A. C., Khandeparker, L., Desai, D. V., Baragi, L. V., and Gaonkar, C. A. (2010). Larval development, sensory mechanisms and physiological adaptations in acorn barnacles with special reference to Balanus amphitrite. J. Exp. Mar. Bio. Ecol. 392, 89–98. doi: 10.1016/j.jembe.2010.04.012
Australian and New Zealand Environment Conservation Council [ANZECC] (2000). Australian and New Zealand Guidelines for Fresh and Marine Water Quality. National Water Quality Management Strategy Paper No. 4. Canberra: Australian and New Zealand Environment Conservation Council and Agriculture and Resource Management Council of Australia and New Zealand.
Bax, N., Hayes, K., Marshall, A., Parry, D., and Thresher, R. (2002). “Man-made marinas as sheltered islands for alien marine organisms: establishment and eradication of an alien invasive marine species,” in Turning the Tide: The Eradication of Invasive Species, eds C. R. Veitch and M. N. Clout (Auckland: IUCN SSC Invasive Species Specialist Group), 26–39.
Bax, N., Williamson, A., Aguero, M., Gonzalez, E., and Geeves, W. (2003). Marine invasive alien species: a threat to global biodiversity. Mar. Policy. 27, 313–323. doi: 10.1016/s0308-597x(03)00041-1
Bell, A., Phillips, S., Denny, C., Georgiades, E., and Kluza, D. (2011). Risk Analysis: Vessel biofouling.Wellington: Ministry of Agriculture and Forestry Biosecurity, 145.
Bohlander, J. (2009). Review of Options for In-Water Cleaning of Ships. MAF Biosecurity New Zealand Technical Paper No:2009/42. Wellington: New Zealand Ministry of Agriculture And Forestry.
Branson, J. (2012). Cost-Benefit Analysis of Proposed Import Health Standard for Vessel Biofouling. Report Prepared for Ministry for Primary Industries. MPI technical paper 2018/68. Available at: https://www.mpi.govt.nz/dmsdocument/31686-cost-benefit-analysis-of-proposed-import-health-standard-for-vessel-biofouling (accessed December 2018).
Buhaug, Ø, Corbett, J. J., Endresen, Ø, Eyring, V., Faber, J., Hanayama, S., et al. (2009). Second IMO GHG Study 2009. London: International Maritime Organization, 220.
Cahill, P., Hickey, C., Lewis, P., Tait, L., and Floerl, O. (2019a). Treatment Agents for Biofouling in Internal Pipework of Recreational Vessels. Available at: https://www.mpi.govt.nz/dmsdocument/33606-treatment-agents-for-biofouling-in-internal-pipework-of-recreational-vessels-a-review-of-pipework-configurations-biofouling-risk-and-operational-considerations (accessed March 2019).
Cahill, P., Tait, L., Floerl, O., Bates, T., Growcott, A., and Georgiades, E. (2019b). Design and assessment of a thermal treatment system for fouled internal pipework of recreational vessels. Mar. Pollut. Bull. 139, 65–73. doi: 10.1016/j.marpolbul.2018.12.032
California Code of Regulations (2017). Biofouling Management to Minimize the Transfer of Nonindigenous Species from Vessels Arriving at California Ports. California, CA: California Code of Regulations.
California Water Boards (2010). 2010 Integrated Report: Clean Water Act Sections 303(d) and 305(b). Sacramento, CA: California State Water Resources Control Board.
California Water Boards (2013). In-water Vessel Hull Cleaning. Best Management Practice Fact Sheet – July 2013. Available at: http://www.waterboards.ca.gov/sanfranciscobay/publications_forms/documents/In-water_vessel_hull_cleaning_fact_sheet.pdf (accessed September 2018).
California Water Boards (2015). In-Water Vessel Hull Cleaning. Best Management Practice Fact Sheet – May 2015. Available at: https://www.waterboards.ca.gov/sanfranciscobay/publications_forms/documents/in_water_hull_cleaning_bmp_fact_sheet.pdf (accessed September 2018).
Carlton, J. T., Keith, I., and Ruiz, G. M. (2019). Assessing marine bioinvasions in the Galápagos Islands: implications for conservation biology and marine protected areas. Aquat. Invasions 14, 1–20. doi: 10.3391/ai.2019.14.1.01
Clayton, M. N. (1992). Propagules of marine macroalgae: structure and development. Br. Phycol. J. 27, 219–232. doi: 10.1371/journal.pone.0094647
Code of Federal Regulations [CFR] (1983). Water Quality Standards. U.S. Code of Federal Regulations, Title 40, Part 131. Ithaca, NY: Cornell Law School.
Coutts, A. D. M., and Dodgshun, T. J. (2007). The nature and extent of organisms in vessel sea-chests: a protected mechanism for marine bioinvasions. Mar. Pollut. Bull. 54, 875–886. doi: 10.1016/j.marpolbul.2007.03.011
Coutts, A. D. M., Moore, K. M., and Hewitt, C. L. (2003). Ships’ sea-chests: an overlooked transfer mechanism for non-indigenous marine species? Mar. Pollut. Bull. 46, 1510–1513. doi: 10.1016/s0025-326x(03)00292-3
Coutts, A. D. M., Piola, R. F., Hewitt, C. L., Connell, S. D., and Gardner, J. P. A. (2010). Effect of vessel voyage speed on survival of biofouling organisms: implications for translocation of non-indigenous marine species. Biofouling 26, 1–13. doi: 10.1080/08927010903174599
Dafforn, K. A., Lewis, J. A., and Johnston, E. L. (2011). Antifouling strategies: history and regulation, ecological impacts and mitigation. Mar. Pollut. Bull. 62, 453–465. doi: 10.1016/j.marpolbul.2011.01.012
Davidson, I., Ashton, G., Ruiz, G., Scianni, C., Brown, C., Pagenkopp Lohan, K., et al. (2013). Richness, Extent, Condition, Reproductive Status, and Parasitism of Fouling Communities on Commercial Vessels. Available at: https://www.researchgate.net/publication/265050162_Richness_extent_condition_reproductive_status_and_parasitism_of_fouling_communities_on_commercial_vessels (accessed June 2019).
Davidson, I. C., McCann, L. D., Sytsma, M. D., and Ruiz, G. M. (2008). Interrupting a multispecies bioinvasion vector: the efficacy of in-water cleaning for removing biofouling on obsolete vessels. Mar. Pollut. Bull. 56, 1538–1544. doi: 10.1016/j.marpolbul.2008.05.024
Davidson, I. C., Scianni, C., Hewitt, C., Everett, R., Holm, E., Tamburri, M., et al. (2016). Mini-review: assessing the drivers of ship biofouling management – aligning industry and biosecurity goals. Biofouling 32, 411–428. doi: 10.1080/08927014.2016.1149572
Davidson, J. D. P., Landry, T., Johnson, G. R., and Quijón, P. A. (2017). A cost-benefit analysis of four treatment regimes for the invasive tunicate Ciona intestinalis on mussel farms. Manag. Biol. Invasion 8, 163–170. doi: 10.3391/mbi.2017.8.2.04
Deltares (2011). MAMPEC model v 3.0. Available at: https://www.deltares.nl/en/software/mampec/ (accessed September 2018).
Department of Agriculture, and Water Resources (2019). Australian Biofouling Management Requirements for International Vessel Arrivals – Consultation Regulation Impact Statement. Canberra: Department of Agriculture.
Department of the Environment [DOE] and New Zealand Ministry for Primary Industries [MPI] (2015). Antifouling and in-Water Cleaning Guidelines. Canberra: Department of Agriculture, 31.
Dobretsov, S. (2010). “Marine biofilms,” in Biofouling, eds S. Dürr and J. C. Thomasson (Oxford: Wiley-Blackwell), 123–136. doi: 10.1002/9781444315462.ch9
Drake, L. A., Tamburri, M. N., Davidson, I. C., Ruiz, G. M., and First, M. R. (2017). Meeting Report: Approaches to Quantify Biofouling and Considerations of Hull Cleaning. Washington, DC: Naval Research Laboratory, 38.
Earley, P., Swope, B., Barbeau, K., Bundy, R., McDonald, J. A., and Rivera-Duarte, I. (2014). Life cycle contributions of copper from vessel painting and maintenance activities. Biofouling. 30, 51–68. doi: 10.1080/08927014.2013.841891
Eldredge, L. G., and Carlton, J. T. (2002). Hawaiian marine bioinvasions: a preliminary assessment. Pac. Sci. 56, 211–212. doi: 10.1353/psc.2002.0012
Fitridge, I., Sievers, M., Dempster, T., and Keough, M. J. (2014). Tackling a Critical Industry Bottleneck: Developing Methods to Avoid, Prevent and Treat Biofouling in Mussel Farms. Parkville VIC: University of Melbourne, 77.
Floerl, O., Smith, M., Inglis, G. J., Davey, N., Seaward, K., Johnston, O., et al. (2008). Vessel Biofouling as a Vector for the Introduction of Non-Indigenous Marine Species to New Zealand: Recreational Yachts. Report No:ZBS2004-03A. Wellington: National Institute of Water and Atmospheric Research.
Fofonoff, P. W., Ruiz, G. M., Steves, B., and Carlton, J. T. (2003). “In ships or on ships? Mechanisms of transfer and invasion for non-native species to the coasts of North America,” in Invasive Species: Vectors and Management Strategies, eds G. M. Ruiz and J. T. Carlton (Washington: Island Press), 152–182.
Food and Agriculture Organization of the United Nations [FAO] (2018). The State of World Fisheries and Aquaculture 2018 - Meeting the Sustainable Development Goals. Rome: FAO, 210.
Forrest, B., Cahill, P., Newcombe, E., and Taylor, D. (2014). Marine Pests and Management Concepts for Shellfish Aquaculture. Nelson: Cawthron Institute, 48.
Frey, M. A., Simard, N., Robichaud, D. D., Martin, J. L., and Therriault, T. W. (2014). Fouling around: vessel sea-chests as a vector for the introduction and spread of aquatic invasive species. Manag. Biol. Invasion 5, 21–30. doi: 10.3391/mbi.2014.5.1.02
Gadd, J., Depree, C., and Hickey, C. (2011). Relevance to New Zealand of the OECD Emission Scenario Document for Antifouling Paints: Phase 2 Report. Report for the Environmental Protection Authority (EPA). Hamilton: National Institute of Water and Atmospheric Research Ltd.
Georgiades, E., Growcott, A., and Kluza, D. (2018). Technical Guidance on Biofouling Management for Vessels Arriving to New Zealand. Wellington: Ministry for Primary Industries, 16.
Georgiades, E., and Kluza, D. (2017). Evidence-based decision making to underpin the thresholds in New Zealand’s CRMS: biofouling on vessels arriving to New Zealand. J. Mar. Sci. Technol. 51, 76–88. doi: 10.4031/mtsj.51.2.5
Grandison, C., Piola, R., and Fletcher, L. (2011). A Review of Marine Growth Protection System (MGPS) Options for the Royal Australian Navy. DSTO-TR-2631. Fairbairn: Defence Science and Technology Organisation, 37.
Growcott, A., Kluza, D., and Georgiades, E. (2017). Review: in-water systems to reactively manage biofouling in sea chests and internal pipework. J. Mar. Sci. Technol. 51, 89–104. doi: 10.4031/mtsj.51.2.3
Growcott, A., Kluza, D., and Georgiades, E. (2019). Technical Advice: Evaluation of in-Water Systems to Reactively Treat or Remove Biofouling within Vessel Internal Niche Areas. Wellington: Ministry for Primary Industries.
Hanekom, N. (2008). Invasion of an indigenous Perna perna mussel bed on the south coast of South Africa by an alien mussel Mytilus galloprovincialis and its effect on the associated fauna. Biol. Invasions 10, 233–244. doi: 10.1007/s10530-007-9125-x
Hayward, B. W. (1997). Introduced marine organisms in New Zealand and their impact in the Waitemata Harbour. Auckland Tane. 36, 197–223.
Hayward, B. W., Morley, M. S., Stephenson, A. B., Blom, W. M., Grenfell, H. R., Prasad, R., et al. (1999). Intertidal and Subtidal Biota and Habitats of the Central Waitemata Harbour. Technical Publication 127. Auckland: Auckland Regional Council, 44.
Hewitt, C. L., Campbell, M. L., Thresher, R. E., Martin, R. B., Boyd, S., Cohen, B. F., et al. (2004). Introduced and cryptogenic species in Port Phillip Bay, Victoria, Australia. Mar. Biol. 144, 183–202. doi: 10.1007/s00227-003-1173-x
Hopkins, G. A., Forrest, B. M., and Coutts, A. (2008). Determining the Efficiency of Incursion Response Tools: Rotating Brush Technology (coupled with suction capability). Available at: https://web.archive.org/web/20100522212027/http://www.biosecurity.govt.nz/files/pests/salt-freshwater/marine-response-tools-rotating-brushes.pdf (accessed September 2018).
Hopkins, G. A., Forrest, B. M., and Coutts, A. D. M. (2010). The effectiveness of rotating brush devices for management of vessel hull fouling. Biofouling 26, 555–566. doi: 10.1080/08927014.2010.494330
Inglis, G. J., Floerl, O., and Woods, C. (2012). Scenarios of Vessel Biofouling Risk and their Management: an Evaluation of Options. Technical Paper No: 2012/07. Wellington: New Zealand: Ministry of Agriculture and Forestry, 122.
International Maritime Organization [IMO] (2004). International Convention for the Control and Management of Ships’ Ballast Water and Sediments. London: International Maritime Organization, 38.
International Maritime Organization [IMO] (2011). Guidelines for the Control and Management of Ships’ Biofouling to Minimize the Transfer of Invasive Aquatic Species. London: International Maritime Organization.
International Maritime Organization [IMO] (2012). Guidance for Minimizing the Transfer of Invasive Aquatic Species as Biofouling (hull fouling) for Recreational Craft. London: International Maritime Organization.
International Maritime Organization [IMO] (2016). 2016 Guidelines for Approval of Ballast Water Management Systems (G8). London: International Maritime Organization.
International Maritime Organization [IMO] (2017). New Global Project to Address Bioinvasions Via Ship’s Hulls. London: International Maritime Organization.
Jones, J. M., and Little, B. (1990). USS Princeton (CG 59): Impact of Marine Macrofouling (mussels and hydroids) on Failure/Corrosion Problems in Seawater Piping Systems. Available at: https://apps.dtic.mil/dtic/tr/fulltext/u2/a476830.pdf (accessed September 2018).
Kospartov, M., Inglis, G., Seaward, K., van den Brink, A., D’ Archino, R., and Ahyong, S. (2008). Nonindigenous and Cryptogenic Marine Species in New Zealand – Current State of Knowledge: Interim Report. NIWA Research Report Prepared for MAF-BNZ Project BNZ10740. Wellington: NIWA.
Kronholm Fraende, M. (2018). BIMCO and Industry Partners to Launch Hull Underwater Cleaning Standard. Available at: https://www.bimco.org/news/priority-news/20181008—hull-cleaning-standard (accessed June 2019).
Lewis, J. A. (2013). In-Water Hull Cleaning and Filtration System: in-Water Cleaning Trials — 26-28 November 2012. Available at: http://www.fish.wa.gov.au/Documents/occasional_publications/fop114.pdf (accessed September 2018).
Lewis, J. A. (2016). Assessment of Preventative Biofouling Management Measures. Available at: http://mpi.govt.nz/document-vault/14530 (accessed September 2018).
Lewis, J. A., and Dimas, J. (2007). Treatment of Biofouling in Internal Seawater Systems – Phase 2. DSTO-TR-2081. Fairbairn: Defence Science and Technology Organisation, 24.
Maggs, C. A., and Callow, M. E. (2003). “Algal spores,” in Encyclopedia of Life Sciences, (London: MacMillan Publishers Ltd.), 6. doi: 10.1038/npg.els.0000311
Marks, L. M., Reed, D. C., and Obaza, A. K. (2017). Assessment of control methods for the invasive seaweed Sargassum horneri in California. USA. Manag. Biol. Invasion 8, 205–213. doi: 10.3391/mbi.2017.8.2.08
McClary, D., and Georgiades, E. (2018). “Evaluation of reactive in-water hull cleaning systems for biofouling management,” in Proceedings of the 19th International Congress for Marine Corrosion and Fouling, (Melbourne, FL).
McClay, T., Zabin, C., Davidson, I., Young, R., and Elam, D. (2015). Vessel biofouling prevention and management options report. Available at: https://apps.dtic.mil/dtic/tr/fulltext/u2/a626612.pdf (accessed September 2018).
Ministry for Primary Industries, New Zealand [MPI] (2014). Craft Risk Management Standard: Biofouling on Vessels Arriving to New Zealand. CRMS - BIOFOUL. Wellington: Ministry for Primary Industries.
Ministry for Primary Industries, New Zealand [MPI] (2018). Request for Proposal - 405760 Barriers to in-Water Biofouling Management. Wellington: Ministry for Primary Industries, 28.
Ministry of Agriculture, and Forestry [MAF] (2011). Draft Anti-Fouling and in-Water Cleaning Guidelines. MAF Discussion Paper no.: 2011/13. Wellington: Ministry for Agriculture and Forestry, 38.
Molnar, J. L., Gamboa, R. L., Revenga, C., and Spalding, M. D. (2008). Assessing the global threat of invasive species to marine biodiversity. \. Ecol. Environ. 6:485–492. doi: 10.1890/070064
Morrisey, D., Gadd, J., Page, M., Lewis, J., Bell, A., and Georgiades, E. (2013). In-Water Cleaning of Vessels: Biosecurity and Chemical Contamination Risks. Available at: https://mpi.govt.nz/document-vault/4092 (accessed September 2018).
Morrisey, D., Inglis, G., Tait, L., Woods, C., Lewis, J., and Georgiades, E. (2015). Procedures for Evaluating in-Water Systems to Remove or Treat Vessel Biofouling. Available at: http://www.mpi.govt.nz/document-vault/10811 (accessed September 2018).
Morrisey, D., and Woods, C. (2015). In-Water Cleaning Technologies: Review of Information. Available at: http://www.mpi.govt.nz/document-vault/10814 (accessed September 2018).
Naval Sea Systems Command [NAVSEA] (2006). Naval Ships’ Technical Manual Chapter 081. Waterborne Underwater Hull Cleaning of Navy Ships, Revision 5. Washington, DC: Naval Sea Systems Command.
Otani, M. (2006). “Important vectors for marine organisms unintentionally introduced to Japanese waters,” in Assessment and Control of Biological Invasion Risks, eds F. Koike, M. N. Clout, M. Kawamichi, M. De Poorter, and K. Iwatsuki (Gland: IUCN), 92–103.
Palermo, M. (1992). Components of Vessels and Dredges Susceptible to Zebra Mussel Infestations. Technical Note ZMR-3-07. Vicksburg, MS: US Army Engineer Waterways Experiment Station.
Pamitran, A. S., Adam, S. A., and Alhamid, M. I. (2016). Cost estimation study for the effect of biofouling on engine cooling system performance with an 8000 BHP vessel. Appl. Mech. Mater. 819, 427–431. doi: 10.4028/www.scientific.net/amm.819.427
Robinson, T. B., Griffiths, C. L., McQuaid, C. D., and Russ, M. (2005). Marine alien species of South Africa – status and impacts. Afr. J. Mar. Sci. 27, 297–306. doi: 10.1002/ece3.1303
Ruiz, G. M., Carlton, J. T., Grosholz, E. D., and Hines, A. H. (1997). Global invasions of marine and estuarine habitats by nonindigenous species: Mechanisms, extent, and consequences. Am. Zool. 37, 621–632. doi: 10.1093/icb/37.6.621
Ruiz, G. M., Fofonoff, P. W., Steves, B., Foss, S. F., and Shiba, S. N. (2011). Marine invasion history and vector analysis of California: a hotspot for western North America. Divers. Distrib. 17, 362–373. doi: 10.1111/j.1472-4642.2011.00742.x
Schultz, M. P. (2007). Effects of coating roughness and biofouling on ship resistance and powering. Biofouling 23, 331–341. doi: 10.1080/08927010701461974
Schultz, M. P., Bendick, J. A., Holm, E. R., and Hertel, W. M. (2011). Economic impact of biofouling on a naval surface ship. Biofouling 27, 87–98. doi: 10.1080/08927014.2010.542809
Scianni, C., Falkner, M., and DeBruyckere, L. (2017). Biofouling in the U.S. Pacific States and British Columbia. Coastal Commission of the Western Regional Panel on Aquatic Nuisance Species. Available at: https://www.fws.gov/answest/coastal%20group/Final%20CC%20Biofouling%20White%20Paper%2011Apr17.pdf (accessed September 2018).
Sippel, A. J. (1983). “Water supply sanitation,” in A Guide to Integrated Fish Health Management in the Great Lakes Basin, eds F. P. Meyer, J. W. Warren, and T. G. Carey (Ann Arbor MI: Great Lakes Fishery Commission), 49–58.
Sorte, C. J., Williams, S. L., and Carlton, J. T. (2010). Marine range shifts and species introductions: comparative spread rates and community impacts. Glob. Ecol. Biogeogr. 19, 303–316. doi: 10.1111/j.1466-8238.2009.00519.x
Terraphase Engineering Inc. (2012). In-Water Hull Cleaning Summary Report. Alameda, CA: US-DOT – Maritime Administration, 48.
Thursby, G., and Hansen, D. J. (1995). Ambient Water Quality Criteria-Saltwater Copper Addendum (Draft). Washington, DC: Office of Water, United States Environmental Protection Agency.
Tribou, M., and Swain, G. (2015). The effects of grooming on a copper ablative coating: a six year study. Biofouling 31, 309–319. doi: 10.1080/08927014.2017.1328596
Tribou, M., and Swain, G. (2017). Grooming using rotating brushes as a proactive method to control ship biofouling. Biofouling 33, 494–504. doi: 10.1080/08927014.2015.1041021
United States Coast Guard [USGC] (2018). Ballast Water Frequently Asked Questions. USGC Deputy Commandant for Operations. Available at: https://www.dco.uscg.mil/Portals/9/MSC/BWMS/Ballast_Water_FAQs.pdf?ver=2018-06-06-123015-850 (accessed September 2018).
United States Environmental Protection Agency [EPA] (1999). Underwater ship Husbandry: Nature of Discharge. Phase 1: Uniform national discharge standards for vessels of the armed forces, technical development document, appendix A. Available at: https://nepis.epa.gov/Exe/ZyPURL.cgi?Dockey = 20002KB0.TXT (accessed September 2018).
Valkirs, A. O., Seligman, P. F., Haslbeck, E., and Caso, J. S. (2003). Measurement of copper release rates from antifouling paint under laboratory and in situ conditions: implications for loading estimation to marine water bodies. Mar. Pollut. Bull. 46, 763–779. doi: 10.1016/s0025-326x(03)00044-4
Willan, R. C., Russell, B. C., Murfet, N. B., Moore, K. L., McEnnulty, F. R., Horner, S. K., et al. (2000). Outbreak of Mytilopsis sallei (Récluz, 1849) (Bivalvia: Dreissenidae) in Australia. Molluscan Res. 20, 25–30. doi: 10.1080/13235818.2000.10673730
Woods, C., Floerl, O., Fitridge, I., Johnston, O., Robinson, K., Rupp, D., et al. (2007). Efficacy of Hull Cleaning Operations in Containing Biological Material. II. Seasonal Variability. Available at: https://web.archive.org/web/20100522212446/http://www.biosecurity.govt.nz/files/pests/salt-freshwater/hull-cleaning-operations-efficacy-2.pdf (accessed September 2018).
Keywords: biofouling, in-water cleaning, copper, New Zealand, California
Citation: Scianni C and Georgiades E (2019) Vessel In-Water Cleaning or Treatment: Identification of Environmental Risks and Science Needs for Evidence-Based Decision Making. Front. Mar. Sci. 6:467. doi: 10.3389/fmars.2019.00467
Received: 14 December 2018; Accepted: 11 July 2019;
Published: 26 July 2019.
Edited by:
Daniel Rittschof, Duke University, United StatesReviewed by:
Eric Holm, Naval Surface Warfare Center Carderock Division, United StatesCopyright © 2019 Scianni and Georgiades. This is an open-access article distributed under the terms of the Creative Commons Attribution License (CC BY). The use, distribution or reproduction in other forums is permitted, provided the original author(s) and the copyright owner(s) are credited and that the original publication in this journal is cited, in accordance with accepted academic practice. No use, distribution or reproduction is permitted which does not comply with these terms.
*Correspondence: Chris Scianni, Q2hyaXMuU2NpYW5uaUBzbGMuY2EuZ292
Disclaimer: All claims expressed in this article are solely those of the authors and do not necessarily represent those of their affiliated organizations, or those of the publisher, the editors and the reviewers. Any product that may be evaluated in this article or claim that may be made by its manufacturer is not guaranteed or endorsed by the publisher.
Research integrity at Frontiers
Learn more about the work of our research integrity team to safeguard the quality of each article we publish.